- 1Leibniz Institute for Baltic Sea Research, Leibniz Science Campus Phosphorus Research, Rostock, Germany
- 2Department of Marine Biology, Institute for Biological Sciences, University of Rostock, Rostock, Germany
- 3Leibniz Institute for Farm Animal Biology (FBN), Institute of Genome Biology, Dummerstorf, Germany
- 4Department of Pharmaceutical Biotechnology, Institute of Pharmacy, University of Greifswald, Greifswald, Germany
- 5Institute of Marine Biotechnology, Greifswald, Germany
- 6Department of Biology, Aarhus University, Aarhus, Denmark
- 7Institute for Microbiology, University of Greifswald, Greifswald, Germany
- 8Department of Maritime Systems, Interdisciplinary Faculty, University of Rostock, Rostock, Germany
Hypoxia is common in marine environments and a major stressor for marine organisms inhabiting benthic and intertidal zones. Several studies have explored the responses of these organisms to hypoxic stress at the whole organism level with a focus on energy metabolism and mitochondrial response, but the instrinsic mitochondrial responses that support the organelle’s function under hypoxia and reoxygenation (H/R) stress are not well understood. We studied the effects of acute H/R stress (10 min anoxia followed by 15 min reoxygenation) on mitochondrial respiration, production of reactive oxygen species (ROS) and posttranslational modifications (PTM) of the proteome in a marine facultative anaerobe, the blue mussel Mytilus edulis. The mussels’ mitochondria showed increased OXPHOS respiration and suppressed proton leak resulting in a higher coupling efficiency after H/R stress. ROS production decreased in both the resting (LEAK) and phosphorylating (OXPHOS) state indicating that M. edulis was able to prevent oxidative stress and mitochondrial damage during reoxygenation. Hypoxia did not lead to rearrangement of the mitochondrial supercomplexes but impacted the mitochondrial phosphoproteome including the proteins involved in OXPHOS, amino acid- and fatty acid catabolism, and protein quality control. This study indicates that mussels’ mitochondria possess intrinsic mechanisms (including regulation via reversible protein phosphorylation) that ensure high respiratory flux and mitigate oxidative damage during H/R stress and contribute to the hypoxia-tolerant mitochondrial phenotype of this metabolically plastic species.
Introduction
Oxygen deficiency (hypoxia) is a major stressor for aerobic eukaryotes that depend on oxygen to drive mitochondrial ATP synthesis. For these organisms, oxygen fluctuations are stressful due to the limitations of energy supply during hypoxia, as well as the oxidative stress and high costs of recovery during reoxygenation (Garbarino et al., 2015; Lesnefsky et al., 2017; Sokolova et al., 2019). Oxygen is rarely limiting in terrestrial habitats (except underground burrows and high altitudes) but in aquatic environments oxygen fluctuations are common. Many benthic marine organisms – particularly sessile species from the habitats where oxygen availability varies regularly and predictably such as the intertidal zone – have adapted to survive under the fluctuating oxygen conditions. The ecological success of these species is often linked with their ability to transition to anaerobiosis and enter the metabolically depressed state during hypoxia (Hochachka and Mustafa, 1972; Guppy et al., 1994; Hochachka and Somero, 2002). The metabolic rate depression in facultative anaerobes involves complex regulatory mechanisms that ensure a concomitant decrease in ATP consumption and production, onset of anaerobic glycolysis, and changes in membrane properties to maintain ion homeostasis with minimum energy input (Hochachka and Mustafa, 1972; Hochachka and Mommsen, 1983; Hochachka and Guppy, 1987; Buck and Hochachka, 1993; Guppy et al., 1994; Storey and Storey, 2004; Storey, 2015). However, the metabolically arrested state allows only time-limited survival, and to continue the life cycle, the organisms depend on the return of oxygen. Therefore, tolerance to frequent oxygen fluctuations requires maintenance of the mitochondrial integrity and rapid restoration of the energetic and redox homeostasis during reoxygenation (Honda et al., 2005; Pamenter, 2014; Pell et al., 2016; Sokolova et al., 2019).
Recent studies indicate that mitochondria of hypoxia-tolerant organisms are capable of preserving the mitochondrial integrity and function during hypoxia-reoxygenation (H/R) stress via rapid suppression and restoration of oxidative phosphorylation (OXPHOS) subject to oxygen availability, mitochondrial proteome reorganization to limit production of reactive oxygen species (ROS), and upregulation of mitochondrial quality control pathways (Buck and Pamenter, 2006; Pamenter, 2014; Pamenter et al., 2016; Bundgaard et al., 2019; Sokolov et al., 2019; Steffen et al., 2020). These changes are observed during long-term (hours to days) exposures of the organisms to hypoxia and subsequent reoxygenation in vivo (Kurochkin et al., 2008; Ivanina et al., 2010, 2011, 2012, 2016; Pamenter et al., 2016; Steffen et al., 2020; Ouillon et al., 2021) and likely reflect a complex integrated response encompassing the direct impact of the oxygen fluctuations on the mitochondria, indirect effects of the changes in intracellular milieu, responses driven by retrograde signaling to the nucleus, as well as endocrine and/or neural regulation (Lee et al., 2020; Du et al., 2021). This complexity, while physiologically relevant and important, makes it difficult to disentangle the contributions of different mechanisms to the functional changes of the mitochondria during H/R stress. Therefore, a reductionist approach using a simplified and thus more tractable system (such as the isolated mitochondria) could provide useful insights. Mitochondria are semi-autonomous organelles that possess their own DNA and protein translation machinery, as well as a dedicated system of quality control and post-translational regulatory mechanisms (McBride et al., 2006) making them capable of direct responses to H/R transitions independently of the rest of the cell. The functional changes during acute H/R exposures of isolated mitochondria provide evidence for such intrinsic mitochondrial regulation (Onukwufor et al., 2014, 2016, 2017; Sappal et al., 2015), but the molecular mechanisms of this regulation have not yet been investigated.
The intrinsic mitochondrial responses to H/R transitions must rely on post-translational regulatory mechanisms such as the changing levels of the metabolic intermediates, shifts in the state of the electron carriers of the ETS, and post-translational modifications (PTM) of mitochondrial proteins. Among the PTM mechanisms, assembly of the respiratory supercomplexes and reversible protein phosphorylation (catalyzed by mitochondrial phosphatases and kinases) are proposed to play a key role in the modulation of mitochondrial metabolism (Pagliarini and Dixon, 2006; Lapuente-Brun et al., 2013; Chaban et al., 2014; Mathers and Staples, 2019). Mitochondrial supercomplexes (SC) involve a close association between complexes CI, CIII, and CIV as a potential mechanism to channel electron flux, increase the efficiency of OXPHOS and mitigate ROS generation (Guerra-Castellano et al., 2018). The functional consequences of SC rearrangement are debated, but recent studies showed links between SC stability and plasticity and metabolic responses to stress in animals (Bundgaard et al., 2018, 2020a; Falfushynska et al., 2020). Furthermore, reversible phosphorylation of the proteins of the mitochondrial complexes CI and CIV can contribute to the regulation of the mitochondrial function during H/R stress by modulating ETS activity, protein-protein and protein-lipid interactions, and ROS production (Helling et al., 2012; Gowthami et al., 2019; Kalpage et al., 2019; Mathers and Staples, 2019). Stress-induced shifts in phosphoproteome and modulation of the activity of protein phosphatases and kinases have been shown in marine mollusks exposed to H/R stress (Sokolov et al., 2019; Falfushynska et al., 2020); however, these studies have been conducted during the whole-organism exposures to H/R stress, which does not allow discerning the intrinsic mitochondrial mechanisms of the observed changes. Overall, the potential contribution of the supercomplex rearrangement and reversible protein phosphorylation to hypoxia-tolerant mitochondrial phenotype has not been well studied in facultative anaerobes and warrants further investigation.
The mussels of the genus Mytilus are widespread facultative anaerobes with ecological and economical importance (Astorga, 2014). Their habitats (including the intertidal zone, eutrophicated estuaries, and coastal habitats) are characterized by recurrent oxygen fluctuations, hence they are highly adapted to these conditions (Gosling, 1992). The energy metabolism pathways of Mytilus spp. are well characterized (Gosling, 1992; Babarro et al., 2007; Connor and Gracey, 2011), and the genomes and transcriptomes of several Mytilus species have been recently published (Martino et al., 2019; Paterno et al., 2019; Li et al., 2020) making these organisms an excellent model to study the molecular and physiological mechanisms of mitochondrial responses to H/R stress. Our present study aimed to elucidate the potential autonomous regulatory mechanisms (i.e., those independent of the cellular metabolic context and regulatory mechanisms) of mitochondrial responses to H/R stress in the blue mussels Mytilus edulis. We hypothesized that isolated mitochondria of a hypoxia-tolerant facultative anaerobe, M. edulis, possess intrinsic mechanisms that permit maintaining the respiratory flux and ATP synthesis and minimize ROS production during acute H/R stress. We also hypothesized that posttranslational mechanisms such as the rearrangement of supercomplexes and reversible protein phosphorylation would be involved in the intrinsic mitochondrial response to H/R transitions. To test these hypotheses, we investigated the direct effects of hypoxia and reoxygenation on the respiration rate under phosphorylating conditions (measured as the OXPHOS activity), proton leak and ROS generation in the isolated mitochondria from the digestive gland of M. edulis. Furthermore, we determined the abundance of the supercomplexes (formed by associations between CI, CIII, and CIV) and shifts in the mitochondrial phosphoproteome in the isolated mitochondria of M. edulis exposed to H/R stress in vitro. The results of this study shed light on the metabolic plasticity of mitochondrial function and demonstrate autonomous mitochondrial mechanisms involved in adaptive metabolic responses to oxygen fluctuations in the hypoxia-tolerant facultative anaerobe M. edulis.
Materials and Methods
Animal Collection and Maintenance
Experimental blue mussels Mytilus edulis were collected in Warnemünde harbor in the Baltic Sea near Rostock, Germany. The animals were acclimated at salinity 15 and 15°C for a week before the experiment. The acclimation temperature and salinity were close to the habitat conditions at the time of collection. During the acclimation, the mussels were continuously fed ad libitum with Premium Reef Blend (CoralSands, Wiesbaden, Germany).
Mitochondrial ṀO2 and ROS Production
Mitochondria were isolated from the mussels’ digestive gland as described elsewhere (Haider et al., 2018; Sokolov et al., 2019). The digestive gland was chosen as one of the most metabolically active organs and a main site of energy storage in mussels (Gosling, 1992). The osmolarity of isolation and assay buffers were optimized in pilot experiments to achieve high quality of mitochondrial isolations (assessed by the respiratory control ratio and cytochrome c test of mitochondrial integrity) for the studied M. edulis population (data not shown). Briefly, 0.5–1.2 g tissue was homogenized on ice in a glass-Teflon homogenizer containing 10 ml of isolation buffer (∼630 mOsm) containing 100 mM sucrose, 200 mM KCl, 50 mM NaCl, 30 mM HEPES pH 7.5, and 8 mM EGTA supplemented with protease inhibitors (1 mM phenylmethylsulfonyl fluoride (PMSF) and 2 μg/ml aprotinin). Homogenate was centrifuged at 4°C and 2000 × g for 5 min, the supernatant was collected and centrifuged at 8000 × g for 8 min. The resulting mitochondrial pellet was resuspended in isolation buffer and kept on ice. All assays commenced within 5 min of mitochondrial isolation.
Mitochondrial respiration rate (ṀO2) and ROS production were measured simultaneously in the high-resolution respirometer Oxygraph 2 k (Oroboros, Innsbruck, Austria) at 15 and 25°C using the DatLab6 software. The assay temperatures were chosen to reflect the acclimation temperature (15°C) typical for early summer at the study site (June average monthly surface water temperature ∼14.8°C), and the elevated temperature close to the summer maximum (25°C). Mitochondrial suspension was added to the instrument’s chamber filled with 2 ml of assay buffer (∼525 mOsm) containing 30 mM HEPES pH 7.2, 185 mM sucrose, 130 mM KCl, 10 mM NaCl, buffer, 10 mM glucose, 1 mM MgCl2, 10 mM KH2PO4, and 1% fatty acid-free bovine serum albumin (BSA). The final mitochondrial protein concentrations in the assay chamber were ∼1–1.5 mg ml–1. Because earlier studies in bivalves (Sokolov and Sokolova, 2019; Haider et al., 2020) and mammals (Hansen et al., 2010; Wang et al., 2016) showed that a common intracellular osmolyte, taurine, might improve mitochondrial function, we tested the potential mitoprotective effect of taurine in the mussel mitochondria. For this, assay buffer was supplemented either with 50 mM taurine or 50 mM glycine. Glycine is the most abundant free amino acid in oyster tissues (Haider et al., 2020) and was used to distinguish taurine-specific effects on mitochondria (if any) from non-specific effects of amino acid addition. After addition of mitochondrial suspension and stabilization of the polarographic oxygen sensor, the following substrate–uncoupler–inhibitor titration (SUIT) protocol was used: 1) 5 mM pyruvate, 0.5 mM malate to spark Complex I-driven respiration (LEAK I); 2) 10 mM succinate to stimulate Complex II (LEAK I + II); 3) 2.5 mM ADP to stimulate OXPHOS; 4) 2.5 μM oligomycin to inhibit mitochondrial FO,F1-ATPase followed by titration (in 0.5 μM steps) with the uncoupler carbonyl cyanide-chlorophenyl hydrazine (CCCP) to measure maximum ETS activity. ROS production was measured in parallel with ṀO2 using the Oxygraph-2K LED2 module with Fluorescent Sensor Green (525 nm) and Amplex Red (Thermo Fisher Scientific, Waltham, MA, United States) as described elsewhere (Ouillon et al., 2021). Addition of cytochrome C (0.8 μM) in the OXPHOS state (Gnaiger, 2012) increased mitochondrial respiration by <10% indicating integrity of isolated mitochondria (data not shown). The LEAK state ṀO2 indicates the proton leak rate of resting (non-phosphorylating) mitochondria, ṀO2 of ADP-stimulated mitochondria corresponds to the OXPHOS capacity, and CCCP-uncoupled ṀO2 reflects the maximum ETS capacity. Respiratory control ratio was calculated by dividing OXPHOS by LEAKI + II respiration (Estabrook, 1967).
To test for the effects of H/R stress on mitochondrial respiration and ROS production, steps 1–3 (up to and including ADP addition) of the above-described SUIT protocol were carried out to measure the pre-hypoxic (baseline) LEAK I + II and OXPHOS oxygen consumption and ROS production rates. The OXPHOS respiration continued until all oxygen in the chamber was consumed, and mitochondria were held in anoxia for 10 min. The chamber was then opened for reoxygenation (2.5–5 min to achieve >80% of air saturation), closed and the post-reoxygenation OXPHOS oxygen consumption rate was measured. Next, 2.5 μM of oligomycin was added to record oxygen consumption in the post-reoxygenation LEAK state (LEAK I + II). At the end of the assay, 0.5 μM of rotenone and 2.5 μM of antimycin A were added to assess non-mitochondrial respiration (<10% of the total oxygen consumption rate, data not shown). The effects of H/R stress on the oxygen consumption rates were tested at 15 and 25°C, and ROS production at 25°C due to low ROS generation rates at 15°C.
The impact of H/R stress on mitochondrial ETS activity was measured in CCCP-uncoupled mitochondria at 15°C using pyruvate (5 mM), succinate (10 mM), or the mixture of pyruvate and succinate as substrates. Malate (0.5 mM) was added to spark the respiration. Mitochondria were allowed to respire until all oxygen was consumed in the chamber, kept in anoxia for 10 min, and re-oxygenated to measure the post-hypoxic ETS activity.
After completion of the assays, an aliquot of each mitochondrial suspension was lysed in hypoosmotic solution using three freezing-thawing cycles followed by sonication, and mitochondrial protein content was measured according to Bradford using a commercial kit (Merck KGaA, Darmstadt, Germany) with BSA as a standard. Respiration rates were expressed in μmol O2 min–1 g–1 protein and ROS production in μmol H2O2 min–1 g–1 protein.
Isolation of Phosphoproteins for LC/MS Analysis
Isolation of mitochondrial phosphoproteins was done using a chromatography approach with the Pro-Q® Diamond Phosphoprotein Enrichment Kit (Invitrogen, Carlsbad, CA, United States) according to the manufacturer’s recommendations. Briefly, 40–60 mg of mitochondrial suspension was lysed with a proprietary Invitrogen’s buffer, diluted to 0.1–0.2 mg ml–1 protein and applied to a prewashed chromatographic column. After the lysate passed through the column, the column was intensively washed to remove unbound proteins, followed by elution of an enriched fraction of phosphoproteins. The phosphoproteins were concentrated using several rounds of centrifugation with Vivaspin concentrators (Sartorius, Göttingen, Germany). Samples were stored at −80°C in 25 mM Tris, pH 7.5, 0.25% CHAPS until further analyses.
LC/MS Analysis
Sample preparation for mass spectrometric analysis of enriched M. edulis mitochondrial phosphoproteins was performed as described in detail previously (Sokolov et al., 2019). Five biological replicates per condition including normoxic controls (i.e., isolated mitochondria prior to H/R stress) and mitochondria exposed to reoxygenation following 10 min of experimental anoxia in vitro were prepared for MS analysis. In short, protein extracts were concentrated to 15 μl, mixed with sample buffer [200 mM Tris–HCl pH 6.8, 50 mM EDTA pH 8.0, 40% glycerol, 8% SDS, 0.08% bromophenol blue, 1 mM dithiothreitol (DTT)], denatured at 90°C for 10 min, and briefly separated in 10% SDS mini gels. After Coomassie staining, the protein-containing gel areas (upper 10 mm of each gel band) were excised, dried in a vacuum centrifuge, reduced (20 mM DTT, 30 min, 55°C, 800 rpm), alkylated (40 mM iodoacetamide, 30 min, room temperature in the dark), and dehydrated (100% acetonitrile HPLC grade, 2 min, room temperature). Proteins were then digested (4 μg/μl trypsin solution, 37°C, 16 h), and peptides were purified by Stage Tipping, adjusted to 2 μg/μl and frozen at −80°C until analysis. Purified peptides were analyzed by reversed phase liquid chromatography (LC) electrospray ionization (ESI) MS/MS. Thus, an EASY nLC 1000 (Thermo Fischer Scientific, Bremen, Germany) was coupled to a QExactive mass spectrometer (Thermo Fischer Scientific, Bremen, Germany). Peptides were loaded onto in-house self-packed fused silica columns (100 μm × 20 cm) containing reverse-phase C18 material (ReproSil-Pur 120-AQ 3.0 μm, Dr. Maisch GmbH, Ammerbuch, Germany). Peptides were eluted using a non-linear binary gradient of 166 min from 2 to 99% solvent B [0.1% (v/v) acetic acid in acetonitrile] in solvent A [0.1% (v/v) acetic acid] at a constant flow rate of 300 nl/min. The full scan was recorded with a mass range from 300 - 1650 m/z and a resolution of 70,000 at 200 m/z. The 15 most abundant ions were isolated with an isolation width of 2 Th and fragmented by higher-energy collisional dissociation (HCD) at a normalized collision energy (NCE) of 27.5. Fragment ion spectra were recorded with a resolution of 17,500 at 200 m/z. Ions with unassigned charge states as well as charge 1 and higher than 6 were excluded from fragmentation. Fragmented ions were excluded from fragmentation for 30 s. Lock mass correction was enabled.
MS Data Analysis
MS/MS spectra were extracted and analyzed using the Sorcerer Sequest software (v5.1.1, Sage-N Research) and a combined target-decoy database. As a dedicated complete M. edulis genome sequence was not yet available at the time, we constructed the database from all available related Mytilus species. To this end, all NCBI protein entries listed for the taxon “Mytilus” (77,824 proteins on 09/08/2020; Supplementary Table 1) were retrieved and clustered at 97% identity with Cd-Hit (Li and Godzik, 2006) to remove redundant proteins. A set of common laboratory contaminants was added and all sequence entries were reversed and appended to the database as decoy sequences (to allow for false discovery rate (FDR) calculation), resulting in a total of 127,890 sequences in the database. MS/MS-based peptide and protein identifications of all 10 samples were merged and evaluated with Scaffold version 4.8.41, with the clustering feature enabled. Protein level FDR and peptide level FDR were set to 1%. A minimum of 2 peptides was required for protein identification. For semi-quantitative analysis and comparison of relative protein abundances between samples, normalized spectral abundance factors (NSAF) were calculated using total spectrum counts (Zybailov et al., 2006). The mass spectrometry proteomics data were deposited to the ProteomeXchange Consortium via the PRIDE partner repository (Vizcaíno et al., 2016) with the dataset identifier PXD027955 and 10.6019/PXD027955.
Blue Native PAGE
Mitochondrial membrane proteins from isolated M. edulis mitochondria were separated by Blue Native PAGE (BN-PAGE) as previously described (Bundgaard et al., 2018, 2020a). Membrane proteins were extracted with 1% dodecyl maltoside (DDM) or 3 g g–1 protein digitonin on ice for 15 or 5 min, respectively. Mouse mitochondrial membrane proteins extracted with 1% DDM were used as a size marker to identify the M. edulis bands. In-gel complex I activity was assessed as a purple stain after incubation for 30 min at room temperature with 1 mg/mL nitroblue tetrazolium with 0.1 μM NADH (Greggio et al., 2017; Bundgaard et al., 2020a). The gels were scanned using a Bio-Rad ChemiDoc MP imager and analyzed using the Bio-Rad Image Lab band detection software (Bio-Rad, Hercules, CA, United States). The intensity of the supercomplex band was expressed relative to the intensity of the strong complex V (ATP synthase) band to control for loading differences.
Statistics
The significance of the effects of amino acid addition or H/R stress on the studied mitochondrial traits was tested using paired Student’s t-test. The effects of H/R stress on the abundance of mitochondrial supercomplexes were assessed using unpaired t-test. Statistical analyses were conducted using GraphPad Prism v. 7.02 (GraphPad Software Inc., La Jolla, CA, United States) software. All differences were considered significant if the probability of Type II error was <0.05. Testing for statistically significant differences in protein abundance between hypoxia and control samples in the phosphoproteome NSAF data set was performed with Perseus (Tyanova and Cox, 2018) using a p-value-based Welch’s t-test (p-value threshold 0.05) after imputation of missing values.
Results
Effects of Amino Acids and Temperature on Mitochondrial Functions
Addition of 50 mM taurine or glycine had no significant effect on mitochondrial oxygen consumption or ROS production regardless of the temperature (Table 1). Therefore, amino acid addition has not been considered in the further analyses of the effects of temperature and H/R stress on the studied mitochondrial traits.
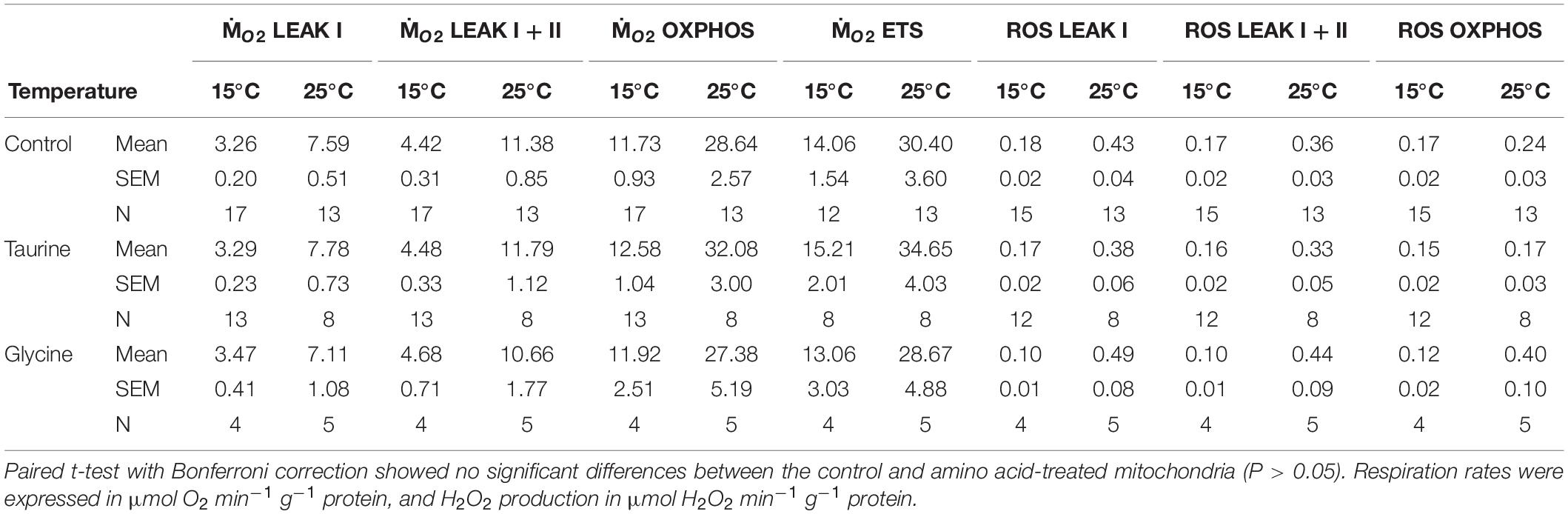
Table 1. Effect of amino acid addition (50 mM taurine or glycine) on oxygen consumption (ṀO2) and ROS efflux of isolated mitochondria of M. edulis.
Elevated temperature (25°C) led to an increase in mitochondrial respiration and ROS emission in the LEAK and OXPHOS states (Figure 1). Likewise, ETS activity was higher at 25°C than at 15°C (Figure 1). Q10 for the mitochondrial oxygen consumption was 2.2–2.5 regardless of the mitochondrial activity state. Q10 for ROS emission was 2.4–2.5 in the LEAK state and 1.6 in the OXPHOS state.
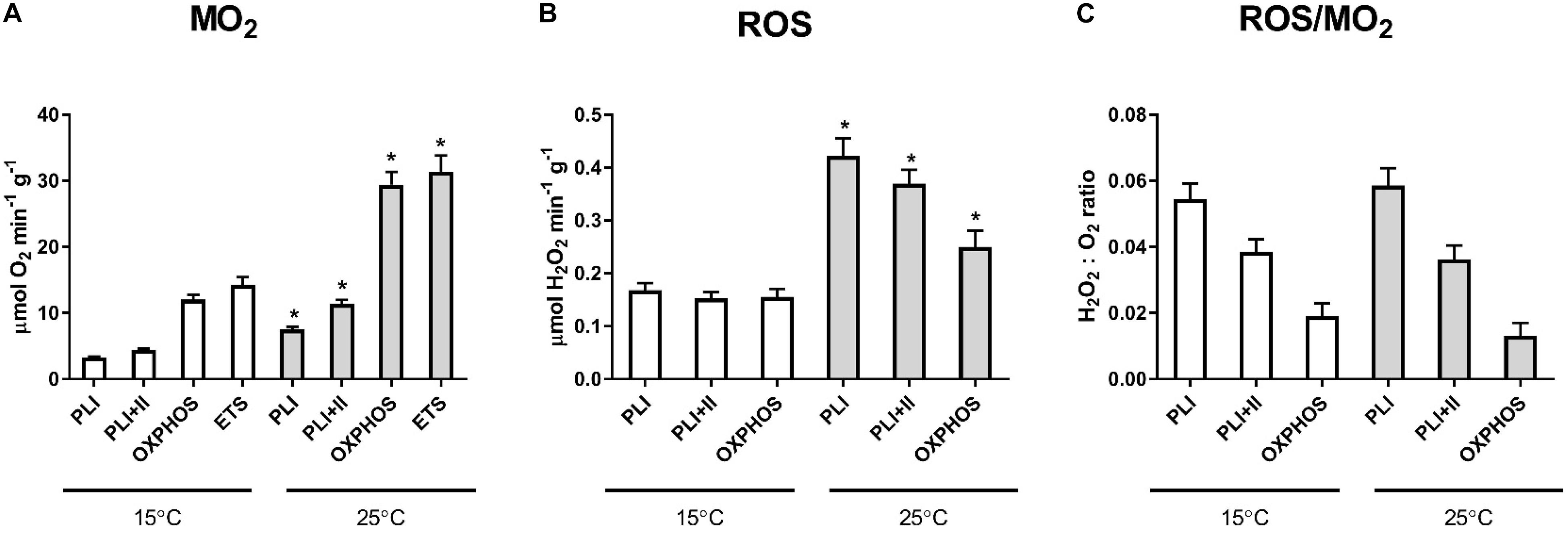
Figure 1. Oxygen consumption rates (ṀO2) and ROS efflux of isolated mitochondria respiring at low (15°C) and high temperatures (25°C) under control conditions. Significant differences between mitochondrial traits measured at different temperatures are denoted by asterisks (*P < 0.05). PL I indicates proton LEAK I, PLI+II - LEAK I+II, ETS - activity of ETS. ROS/MO2 ratio indicates the ratio of H2O2 produced to O2 consumed in the respective states.
The rate of the ROS emission was higher in the LEAK state compared with the OXPHOS state regardless of the temperature (Table 1 and Figure 1). At both 15 and 25°C, mitochondria in the LEAK state converted ∼5.4–5.9% of consumed oxygen into H2O2 when respiring on pyruvate. When a mixture of pyruvate and succinate was used, the fraction of consumed O2 converted to H2O2 in the LEAK state decreased to ∼3.6–3.8%. Mitochondria in the OXPHOS state respiring on pyruvate and succinate converted ∼1.3–1.9% of consumed O2 into H2O2.
Effects of H/R Stress on Mitochondria Functional Traits and Supercomplex Formation
Effects of H/R stress on mitochondrial oxygen consumption were similar at 15 and 25°C showing suppression of LEAK respiration, elevated OXPHOS activity and increased RCR after H/R stress (Figure 2). ETS activity was elevated after H/R stress in mitochondria respiring on succinate but not in those using pyruvate or pyruvate-succinate mixture (Figure 3).
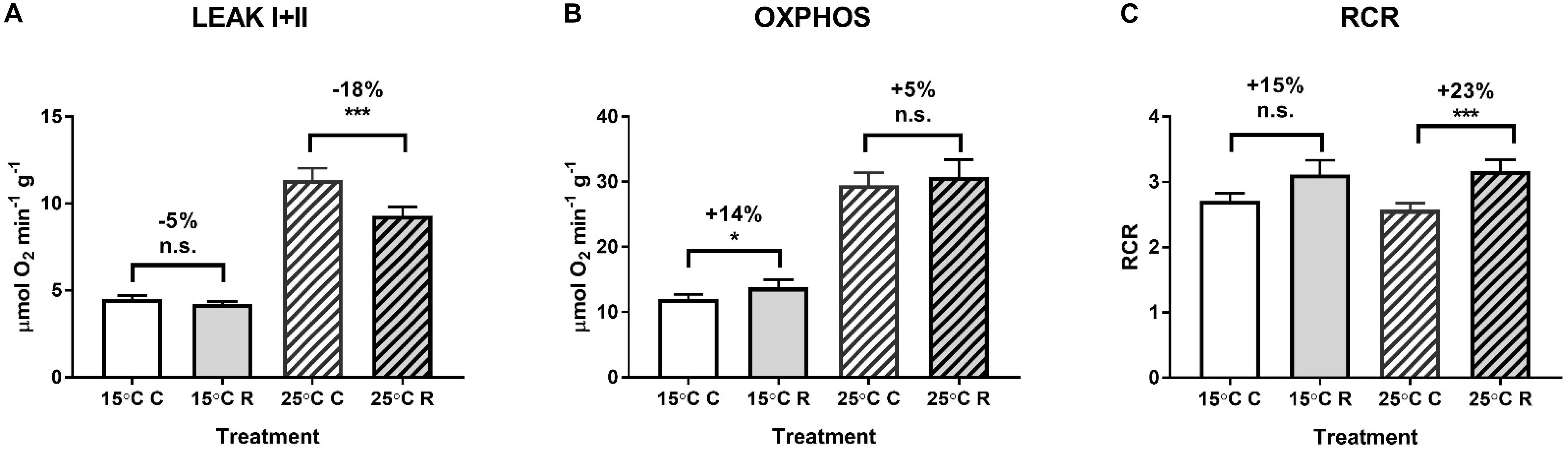
Figure 2. Effects of hypoxia and reoxygenation (H/R) on respiration of isolated digestive gland mitochondria of M. edulis at different temperatures (15 or 25°C). (A) LEAK respiration, (B) OXPHOS respiration, and (C) respiratory control ratios. The percentage change following H/R stress was calculated by standardizing the control values at the respective temperatures to 100%. Significant differences in each mitochondrial trait between normoxia (C) and reoxygenation (R) are denoted by asterisks (*P < 0.05, ***P < 0.001). n.s. indicates differences that were not statistically significant (P > 0.05).
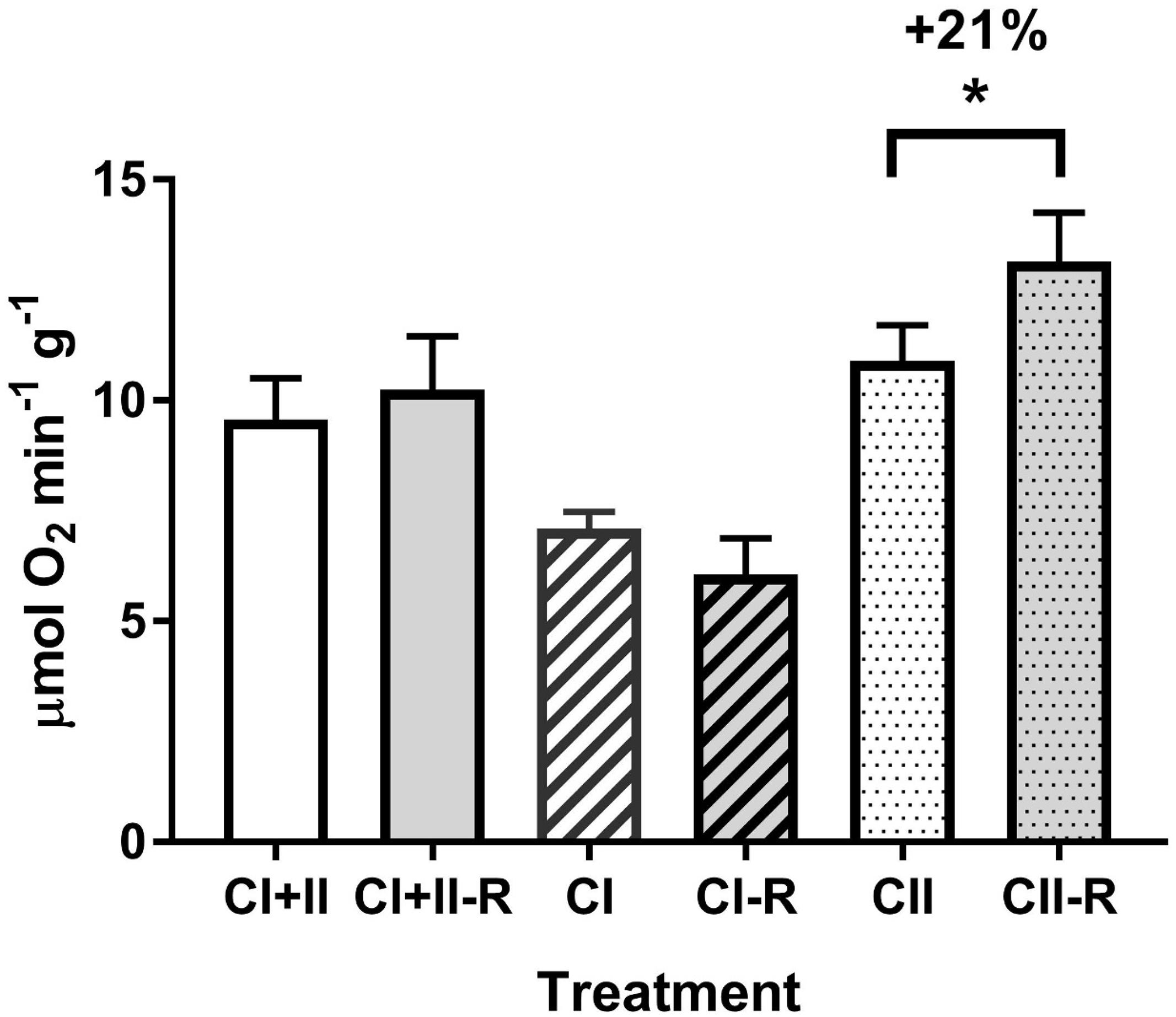
Figure 3. Effect of hypoxia and reoxygenation (H/R) on the ETS activity of isolated digestive gland mitochondria of M. edulis respiring on different substrates. The percentage change following H/R stress was calculated by standardizing the control values at the respective temperatures to 100%. Significant differences in each mitochondrial trait between normoxia and reoxygenation are denoted by asterisks (*P < 0.05). CI - ETS activity with Complex I substrates (pyruvate and malate), CII - ETS activity with Complex II substrate (succinate), CI+II - ETS activity with pyruvate, malate and succinate. CI, CII, CI+II indicate control values before H/R stress, CI-R, CII-R and CI+II-R - the corresponding values after H/R stress.
To improve the assay sensitivity and decrease the time for aerobic-anaerobic transition, all subsequent analyses (including ROS emission, supercomplex formation and phosphoproteomics) were conducted in mitochondria respiring at 25°C where the mitochondrial activity was higher. At 25°C, ROS emission in the LEAK state was strongly (by 56%) suppressed after the H/R exposure, whereas ROS emission in the OXPHOS state was not significantly affected by the H/R stress (Figure 4). The rate of the electron leak (measured as a ratio of H2O2 production to O2 consumption) decreased by 49 and 28% in the LEAK and OXPHOS state mitochondria, respectively, after the H/R stress (Figure 4C). The LEAK state mitochondria converted ∼3.6% of consumed O2 to H2O2 under the control conditions, but only ∼1.9% after the H/R stress (Figure 2C). In the OXPHOS state, the mussels’ mitochondria converted ∼1.3% and <1% of consumed O2 to H2O2 under before and after the H/R stress, respectively (Figure 4C).
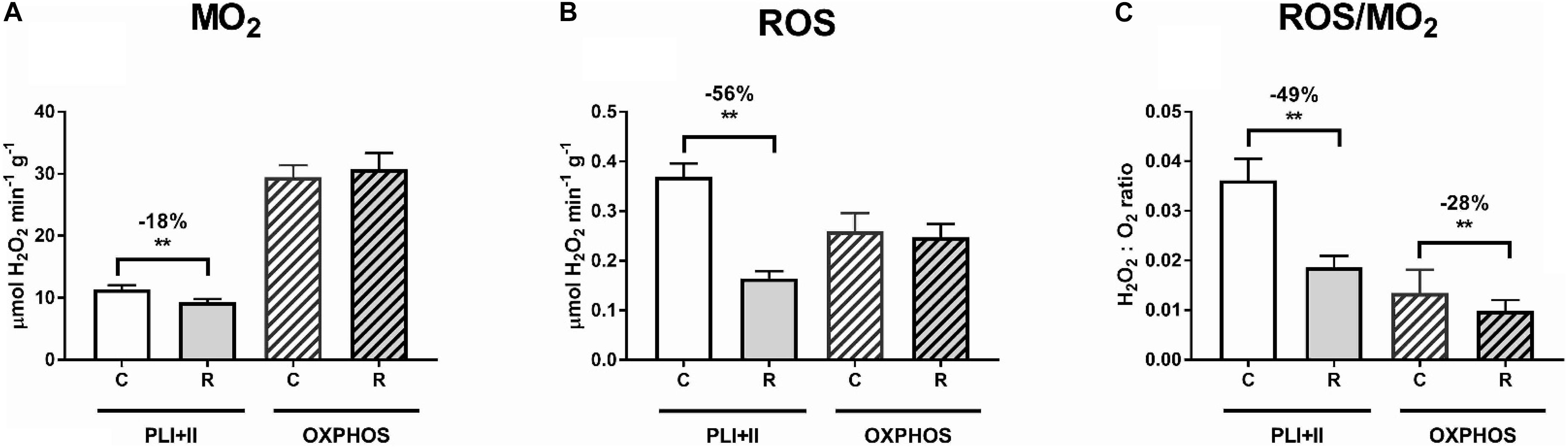
Figure 4. Effects of hypoxia and reoxygenation (H/R) on oxygen consumption rates (ṀO2) and ROS efflux of isolated digestive gland mitochondria of M. edulis. (A) oxygen consumption rates during LEAK I+II (PLI+II) and OXPHOS respiration, (B) ROS efflux during LEAK I+II (PLI+II) and OXPHOS respiration, (C) Electron leak rate during LEAK I+II (PLI+II) and OXPHOS respiration. All activities were assessed at 25°C. The percentage change following H/R stress was calculated by standardizing the control values at the respective temperatures to 100%. Significant differences in each mitochondrial trait between normoxia (C) and reoxygenation (R) are denoted by asterisks (**P < 0.01).
Supercomplex (CI-CIII) formation was detected in mussels but it was not affected by exposure of isolated mussel mitochondria to H/R stress (Figure 5). The SC abundance relative to that of complex V was instead dependent on the use of detergent, as found previously in several species (Bundgaard et al., 2020a,b).
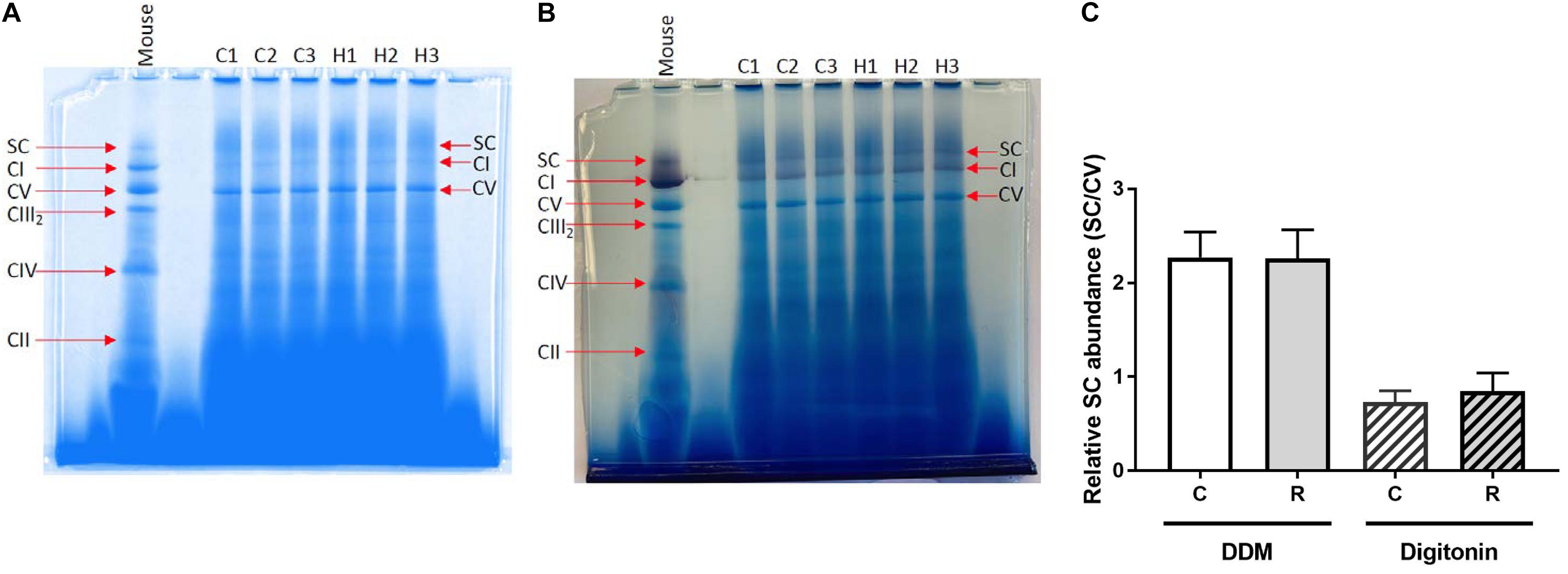
Figure 5. Resolution of mitochondrial ETS complexes of M. edulis. BN-PAGE of ETS complexes (CI-CV) along with supercomplexes (SC) from M. edulis mitochondria solubilized with DDM or digitonin, with mouse mitochondria (left) used for comparison to identify bands. (A) image of the gel with DDM-treated samples, (B) image of the gel with digitonin-treated samples, and (C) – quantification of the SC band intensity relative to CV band C - control, R - reoxygenation. The gels were stained for protein (blue) and Complex I activity (purple) of three biological replicates (C1-3, controls and H1-3, after H/R stress).
Mitochondrial Phosphoproteome Shifts During the H/R Stress
Proteomic analysis identified 169 mitochondrial phosphorylated proteins in the isolated mitochondria of M. edulis (Supplementary Table 2) out of which 25 (15%) significantly changed in abundance in response to the H/R stress (Welch’s t-test, P < 0.05; Table 2; Supplementary Table 2). Of these, 16 phosphorylated proteins showed an increase in abundance, whereas abundance of 9 phosphorylated proteins decreased.
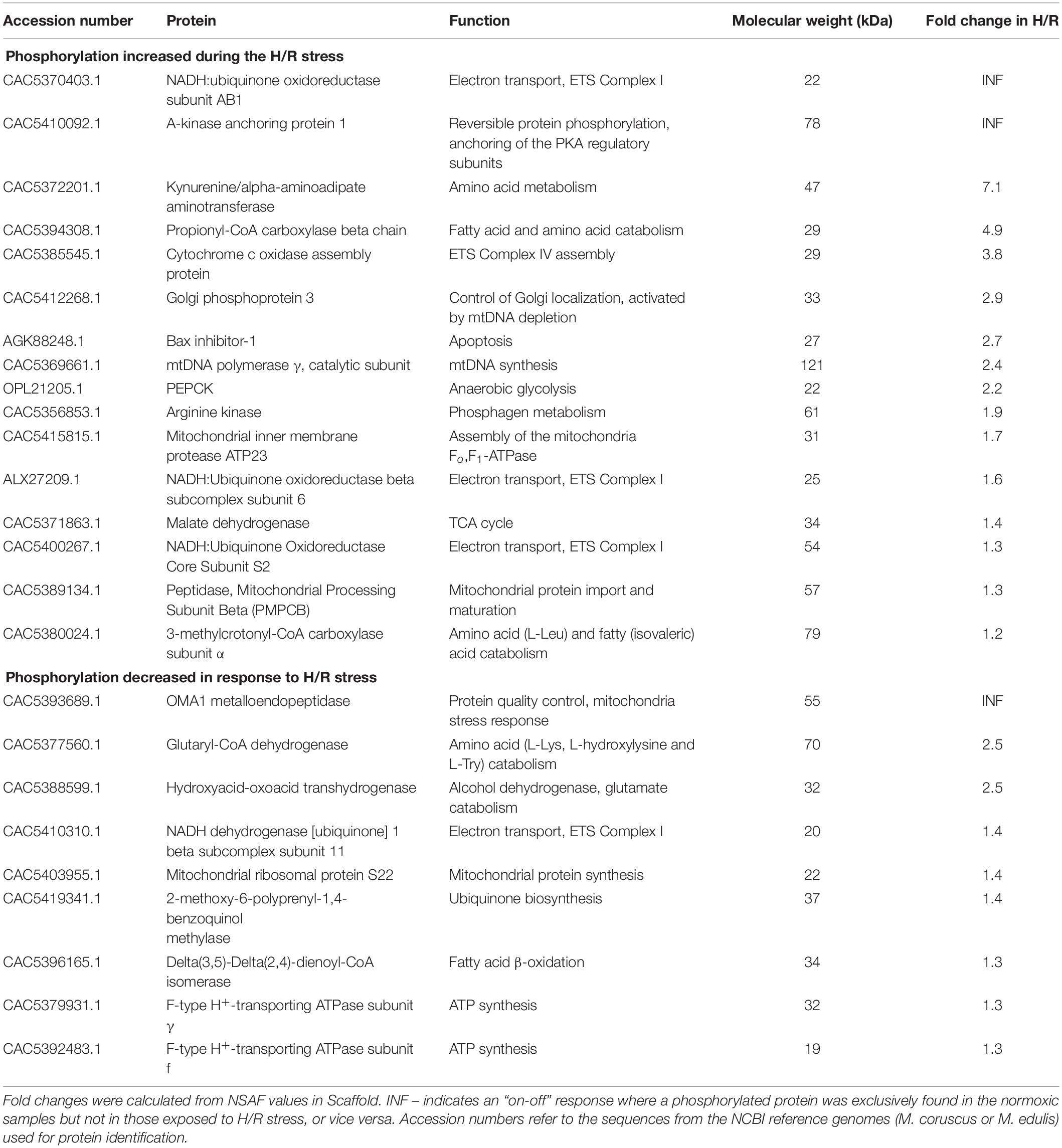
Table 2. List of the mitochondrial phosphoproteins that significantly changed in abundance in response to the exposure of the isolated mitochondria of M. edulis to H/R stress in vitro according to a p-value-based Welch’s t-test (p-value threshold 0.05) performed in Perseus.
Among the phosphoproteins upregulated during the H/R stress, two (the NDUFAB1 subunit of the mitochondrial Complex I and A-kinase anchoring protein 1) showed an “off-on” response and were found exclusively in the samples exposed to the H/R stress but not in normoxia (Table 2). Furthermore, eight more phosphorylated proteins showed a large increase in abundance after the H/R stress including kynurenine/α-aminoadipate aminotransferase (7.1-fold increase), propionyl-CoA carboxylase β chain (4.9-fold), cytochrome c oxidase assembly protein (3.8-fold), Golgi phosphoprotein 3 (2.9-fold), Bax inhibitor 1 (2.7-fold), the catalytic subunit of mtDNA polymerase γ (2.4-fold), phosphoenolpyruvate carboxykinase (PEPCK) (2.2-fold) and arginine kinase (1.9-fold) (Table 2). Other upregulated mitochondrial phosphoproteins (involved in the ETS, TCA cycle, amino acid metabolism, and protein processing) showed a 10–70% increase in abundance in response to H/R exposure (Table 2).
Among the phosphorylated mitochondrial proteins that were significantly less abundant after the H/R stress, two proteins (mitochondrial metalloendopeptidase OMA1 and NADH:ubiquinone oxidoreductase subunit AB1) showed an “on-off” response (i.e. were not detected in the phosphorylated fraction after the H/R stress), and two (glutaryl-CoA-dehydrogenase and hydroxyacid-oxoacid transhydrogenase) showed a 2.5-fold decrease in the abundance after the H/R stress (Table 2). Six phosphorylated proteins including the NDUFB11 subunit of the mitochondrial Complex I, F-type H+-transporting ATPase subunits γ and f, mitochondrial ribosomal protein S22, and two enzymes involved in the intermediary metabolism [Δ(3,5)-Δ(2,4)-dienoyl-CoA isomerase, and 2-methoxy-6-polyprenyl-1,4-benzoquinol methylase] showed a modest but statistically significant decrease (by 30–40%) in abundance after the H/R stress (Table 2). Two more proteins showed large but statistically non-significant changes in the abundance of the phosphorylated form after H/R stress, including translocase of outer membrane 20 (TOM20) that was not found in the mitochondrial phosphoproteome after H/R stress, and reticulon-4-interacting protein that showed a >5-fold decrease in phosphorylated form abundance (Supplementary Table 2).
Discussion
Mussels’ mitochondria show robust coupling and 2.2–2.5-fold increase in both LEAK and OXPHOS respiration during a temperature increase from 15 to 25°C. Elevated temperature led to higher ROS efflux in the mussels mitochondria proportional to the increase in ṀO2 so that electron leak rate (i.e., the fraction of consumed oxygen converted to ROS) did not change during warming. Interestingly, the ROS emission in the OXPHOS state was less temperature sensitive (Q10 = 1.6) than ROS emission of the resting (LEAK state) mitochondria (Q10 = 2.4–2.5). Such thermally robust mitochondrial function might reflect adaptations to fluctuating temperature regimes in this typical intertidal species that colonized the subtidal zone of the Baltic Sea about 10,000 years ago (Śmietanka et al., 2014).
H/R Stress Improves Mitochondrial Efficiency and Suppresses ROS Formation
Mitochondrial oxygen consumption is a driving force of the electron flux in the ETS and ATP production by OXPHOS, as well as a protective mechanism against oxygen toxicity (Sung et al., 2010). As the major oxygen consumers and ROS producers, mitochondria are key targets of H/R stress. Mitochondrial tolerance to intermittent hypoxia requires the ability to maintain high respiration flux while avoiding excessive ROS production during oxygen fluctuations (Sokolova et al., 2019). Our present study indicates that the mitochondria of the blue mussels M. edulis show features consistent with the hypoxia-tolerant phenotype. Thus, isolated mitochondria of M. edulis exposed to H/R stress in vitro showed a 5–18% decrease in proton leak rate and a 5–14% increase in OXPHOS activity resulting in higher coupling efficiency of mitochondria. Mitochondrial proton leak causes uncoupling of oxygen consumption from ATP synthesis and must be compensated by consumption of additional oxygen and substrates to maintain the activity of ETS proton pumps and prevent depolarization of the mitochondrial membrane. Therefore, lower proton leak during reoxygenation in the mussel mitochondria can contribute to improved ATP synthesis efficiency and decrease energy costs of mitochondrial maintenance. Furthermore, an increase in the OXPHOS rate after reoxygenation indicates improved ATP synthesis capacity in the mussel mitochondria. This increase appears largely driven by improved capacity of the mitochondrial ETS to utilize succinate whereas the ability to oxidize NADH-linked substrates such as pyruvate is not affected. Succinate is a common anaerobic end product in marine bivalves that accumulates in high concentrations during hypoxia (Hochachka and Mustafa, 1972; Bayne, 2017). High succinate oxidation capacity might be metabolically beneficial during post-hypoxic recovery in mussels helping to generate ATP with a highly abundant substrate (succinate) and restore normal levels of metabolic intermediates. Elevated OXPHOS and ETS capacity after H/R stress might thus assist in restoring of energy homeostasis after hypoxia and contribute to the increase in oxygen consumption during post-hypoxic recovery (so called “oxygen debt”) commonly found in hypoxia-tolerant invertebrates and fish (Ellington, 1983; Lewis et al., 2007; Vismann and Hagerman, 2008).
Exposure of isolated mussels’ mitochondria to H/R stress strongly modulated mitochondrial ROS emission, which is considered a main driver of H/R-induced mitochondrial stress and injury (Honda et al., 2005; Cadenas, 2018). In hypoxia-sensitive organisms such as terrestrial mammals, post-hypoxic reoxygenation commonly leads to a burst of ROS production causing damage to mitochondrial membrane and proteins and suppression the ETS activity and ATP production (Honda et al., 2005; Venditti et al., 2013; Cadenas, 2018). The damage to ETS further enhances electron leak and ROS production resulting in a vicious cycle of ROS-induced ROS release (Zorov et al., 2006). Unlike this detrimental pattern commonly observed in mammals, mitochondria of M. edulis suppressed electron leak in response to H/R stress. The overall rate of ROS emission and the electron leak rate were strongly (by ∼56 and ∼49%, respectively) suppressed in the LEAK state mitochondria of mussels after H/R stress. The ROS emission rate in the OXPHOS state did not significantly decrease after H/R stress, but the opposing effects of H/R stress on the oxygen consumption (i.e., a slight increase) and ROS emission (a slight decrease) combined to produce a significant (by ∼28%) suppression of the electron leak rate in the OXPHOS state mitochondria. These changes in the ROS emission and electron leak rates induced by the H/R stress can prevent oxidative stress and mitochondrial damage during reoxygenation and might reflect adaptation of the mussels to frequent fluctuation in the oxygen conditions experienced in intertidal and estuarine habitats. From the perspective of oxidative stress mitigation, suppression of the ROS emission in the LEAK state, where electron leak rates and ROS generation rates are high due to the high mitochondrial membrane potential (Miwa and Brand, 2003; Lambert and Brand, 2004), might play an especially important protective role during H/R stress in mussel mitochondria.
Earlier studies using exposures to H/R in vivo (e.g., in whole organisms) or in situ (e.g., during ischemia-reperfusion of organs, tissues, or intact cells) also show robust mitochondrial ETS and OXPHOS function in hypoxia-tolerant species contrasting the loss of OXPHOS capacity and suppressed ETS activity commonly observed in hypoxia-tolerant species (Pamenter, 2014; Sokolova, 2018; Cheng et al., 2021). Thus, OXPHOS respiration and ETS activity were greatly impaired after H/R exposure in hypoxia-sensitive organisms like rainbow trout (Oncorhynchus mykiss) (Onukwufor et al., 2014, 2016, 2017), bay scallops (Argopecten irradians) (Ivanina et al., 2016) and mammals (Paradis et al., 2016; Lesnefsky et al., 2017). A decrease in the mitochondrial OXPHOS rates was also found in isolated rainbow trout mitochondria (Onukwufor et al., 2014) and in permeabilized heart fibers of a hypoxia-sensitive shovelnose ray (Aptychotrema rostrata) (Hickey et al., 2012) after exposure to H/R stress in vitro. Mitochondrial ETS and OXPHOS rates of hypoxia-tolerant organisms such as freshwater turtles, fish, and benthic and intertidal marine bivalves (clams, oysters, mussels) remained constant or increased after H/R exposure in vivo (Hickey et al., 2012; Cook et al., 2013; Galli and Richards, 2014; Du et al., 2016; Ivanina et al., 2016; Gerber et al., 2019; Napolitano et al., 2019; Ouillon et al., 2021) but not after anoxic exposure in freshwater turtles, where mitochondrial respiration was suppressed (Galli et al., 2013; Bundgaard et al., 2018, 2020a). Similarly, an increase in OXPHOS activity was found in isolated mitochondria of hypoxia-tolerant zebrafish (Danio rerio) after H/R exposure in vitro (Napolitano et al., 2019) but a decrease in OXPHOS was found after in vivo prolonged hypoxia of zebrafish in permeabilized skeletal muscle fibers (Cadiz et al., 2019). Taken together, our present findings and earlier published research indicate that, although different responses to hypoxia may take place in some vertebrate species (Pamenter, 2014; Pamenter et al., 2016, 2018; Bundgaard et al., 2020b), in bivalves the mitochondrial phenotype associated with hypoxia tolerance involves the ability to maintain or enhance OXPHOS capacity, mitigate ROS emission and prevent collapse of the ETS during reoxygenation, and that these mitochondrial traits are partially independent of cellular or organismal regulatory mechanisms.
Mitochondrial Proteome Rearrangements During H/R Stress: Dynamics of Supercomplexes
Supercomplexes are dynamic structures consisting of different combinations of complexes involved in the ETS system that can undergo rearrangement in response to varying physiological or environmental conditions including hypoxia (Ramírez-Aguilar et al., 2011; Timón-Gómez and Barrientos, 2020). In hypoxia-exposed mammalian mitochondria, CI was reversibly deactivated (Galkin et al., 2009) likely due to dissociation of the large supercomplexes containing CI, CIII, and CIV, although the capacity of the individual respiratory complexes was intact (Rosca et al., 2008). In plants, SCs were observed to dissociate and reassemble during H/R stress as a result of cellular acidification (Ramírez-Aguilar et al., 2011). However, our present study in mussels as well as recent research in hypoxia-tolerant freshwater turtles (Bundgaard et al., 2018) shows that the dynamic rearrangement of SCs is not involved in response to hypoxia in these species. In M. edulis, H/R stress did not influence the SCs’ abundance (Figure 5), and stability of CICIII2 SC during H/R stress was reported in freshwater turtles (Bundgaard et al., 2018). The functional significance of SC rearrangement remains debatable (Signes and Fernandez-Vizarra, 2018; Timón-Gómez and Barrientos, 2020), and the role of SC stability in maintaining robust ETS activity or in limiting ROS during oxygen fluctuations in hypoxia-tolerant organisms requires further investigation. It is worth noting that SC of ectotherms appear generally more stable than those of mammals, regardless of the species-specific hypoxia tolerance (Bundgaard et al., 2020a,b). Thus, it is possible that the stability of SCs in the mitochondria of mussels observed in our present study is a shared ectotherm trait rather than adaptation to hypoxic stress.
Phosphoproteome Shifts
Reversible phosphorylation is emerging as a pivotal posttranslational modification (PTM) mechanism involved in the regulation of mitochondrial functions (Pagliarini and Dixon, 2006; Helling et al., 2012). The steadily increasing numbers of reported mitochondrial kinases, phosphatases, and phosphoproteins imply an important role of protein phosphorylation in different mitochondrial processes, including responses to H/R stress (Goldenthal and Marín-García, 2004; Horbinski and Chu, 2005). Mitochondrial proteome phosphorylation patterns and their role in the responses to H/R stress are less well studied in hypoxia-tolerant organisms including mollusks. Differential phosphorylation of proteins associated with OXPHOS, Krebs cycle, and intermediary metabolism has been reported during H/R exposure in vivo in the hypoxia-tolerant Pacific oyster, C. gigas (Sokolov et al., 2019). Furthermore, H/R exposure in vivo modulated activities of protein kinases A and C in the mitochondria of M. edulis, C. gigas, and Arctica islandica (Falfushynska et al., 2020). Our present study demonstrates that reversible protein phosphorylation is also involved in the intrinsic mitochondrial responses to H/R stress that act independently of the cellular context during in vitro exposures to fluctuating oxygen conditions. The pathways regulated by reversible phosphorylation during H/R stress in isolated mussel mitochondria include ETS and OXPHOS (10 out of 25 differentially phosphorylated proteins), amino acid and fatty acid metabolism (6 proteins), mitochondrial protein and mtDNA homeostasis (5 proteins), and substrate-level phosphorylation (2 proteins). It is worth noting that in our present study, the change in the protein abundance in the phosphoprotein fraction after H/R stress was interpreted as a change in the phosphorylation state of the respective protein due to PTM. In principle, changes in the abundance of a certain phosphoprotein might also reflect changes in the total protein abundance in the mitochondria due to de novo synthesis and/or protein degradation. However, these alternative explanations appear less likely given the cell-free system and the short exposure times (10 min in anoxia followed by immediate sample collection after reoxygenation) used in the present study.
Of note, H/R exposure of isolated mussels’ mitochondria led to a major increase in abundance of phosphorylated A-kinase anchoring protein 1 (AKAP1). AKAP1 is a scaffold protein that recruits protein kinase A (PKA) and other signaling proteins, as well as RNA, to the outer mitochondrial membrane (Liu et al., 2020). AKAP1 acts as a signaling hub regulating metabolic homeostasis and mitochondrial quality control and is involved in response to H/R stress in mitochondria (Merrill and Strack, 2014; Liu et al., 2020). In mammalian models, AKAP1 becomes phosphorylated in response to energy stress via AMP-activated protein kinase (AMPK)-dependent mechanism, and this phosphorylation is essential for activation of mitochondrial respiration (Liu et al., 2020). Although the mechanisms of AKAP1-dependent mitochondrial regulation are not yet fully unraveled, multiple studies indicate that functional AKAP1 is essential for cell survival during H/R stress and is involved in regulation of PKA activity in mitochondria [review in Merrill and Strack (2014); Liu et al. (2020)]. If similar mechanisms exist in mussel mitochondria, phosphorylation of AKAP1 might indicate an adaptive response to a decrease in ATP levels during H/R stress and positively modulate mitochondrial respiration and PKA recruitment. The latter hypothesis is also consistent with an important role of PKA in regulating the mitochondrial ETS activity in mussels (Falfushynska et al., 2020).
Mitochondrial Complex I is an important target for PTM-dependent regulation during H/R stress in animals including mammals (Gowthami et al., 2019) and mollusks (Falfushynska et al., 2020). Complex I activity is often down-regulated in response to H/R stress as a mechanism to suppress mitochondrial ROS generation by reversible electron transport (RET) (Pell et al., 2016); however, the role of reversible protein phosphorylation in this regulation is not known. In marine bivalves including mussels, Complex I activity is responsive to experimental manipulation of the phosphorylation status (Falfushynska et al., 2020). In M. edulis, PKA-dependent phosphorylation led to an increase in Complex I activity whereas PKC-dependent phosphorylation had no effect (Falfushynska et al., 2020). Unspecific dephosphorylation (by cerium oxide) suppressed Complex I activity (Falfushynska et al., 2020). Our present study strongly indicates that H/R stress affects phosphorylation status of several Complex I subunits in M. edulis. Thus, the subunit NDUFAB1 of Complex I was exclusively phosphorylated in the mitochondrial samples exposed to H/R, and the abundance of the phosphorylated forms of NDUFS2 and NDUFB6 increased by 30–60% after the H/R stress. In contrast, the abundance of the phosphorylated NDUFB11 decreased by 40% after the H/R stress. Functional implications of PTM of these subunits are not known in M. edulis. Interestingly, the subunit NDUFAB1 that is exclusively phosphorylated in the mussels after H/R stress is known to play a protective role during H/R stress in mammalian mitochondria supporting high respiratory flux, stabilizing supercomplexes and mitigating ROS generation (Hou et al., 2019). It is tempting to speculate that a similar protective mechanism mediated by the PTM of NDUFAB1 subunit might be in play in the mussels’ mitochondria during H/R stress, but this hypothesis requires further investigation.
It is worth noting that mitochondrial Complex IV was not affected by PTM during H/R stress in the mussels’ mitochondria. In mammals, Complex IV is considered a key regulatory target during hypoxia whose activity and oxygen sensitivity is modulated by PTM (including reversible phosphorylation) (Prabu et al., 2006; Kadenbach, 2021) and differential expression of hypoxia-specific subunits (Kocha et al., 2015). In M. edulis, Complex IV can also be modulated by experimental phosphorylation/dephosphorylation, albeit to a lesser extent than Complex I (Falfushynska et al., 2020). In marine mollusks including M. edulis, cytochrome c oxidase is not considered rate-limiting due to the high apparent excess capacity of this enzyme relative to the maximum ETS activity (Sokolov et al., 2019; Sokolov and Sokolova, 2019; Ouillon et al., 2021). Furthermore, oxygen affinity of cytochrome c oxidase does not appear modulated by H/R stress in marine bivalves (Ouillon et al., 2021). This indicates that cytochrome c oxidase might be less important as a regulatory target of ETS during H/R stress in mollusks. Consistent with this notion, none of the Complex IV subunits were identified as differentially phosphorylated during H/R stress in our present analysis. Interestingly, H/R stress led to a major increase (by ∼3.8-fold) of the phosphorylated form of cytochrome c oxidase assembly protein COX11. This protein acts as copper chaperone delivering Cu2+ to the active center of cytochrome c oxidase and might be involved in mitigation of ROS generation in mitochondria (Radin et al., 2015). It is possible that elevated phosphorylation levels of this protein might affect redox properties or Cu2+ binding affinity of this protein; however, at present the functional implications of COX11 PTM remain speculative.
H/R stress resulted in a modest (by ∼30%) but statistically significant decrease in the abundance of phosphorylated subunits γ and f of the mitochondrial Fo, F1-ATPase in the mussels and a ∼70% increase in abundance of phosphorylated mitochondrial protease ATP23 responsible for F0, F1-ATPase assembly. These findings indicate that Fo, F1-ATPase is another target of regulatory PTM during H/R stress in bivalve mitochondria. Phosphorylation of ATPase subunits (particularly subunit γ) plays an important role in Fo, F1-ATPase assembly (Reinders et al., 2007). In yeast, dephosphorylation of subunit γ facilitates oligomerization of Fo, F1-ATPase enhancing its activity (Reinders et al., 2007). If a similar mechanism exists in mussels, dephosphorylation of subunit γ of Fo, F1-ATPase would activate Fo, F1-ATPase through enhanced oligomerization in the inner mitochondrial membrane and might contribute to the observed increase in the OXPHOS activity after the H/R stress in mussels.
Amino acid and fatty acid catabolism in mitochondria represents another key target for regulation through PTM in bivalve mitochondria as shown by differential phosphorylation levels induced by the H/R stress in multiple enzymes involved in the respective pathways. Overall, six enzymes involved in amino acid and fatty acid catabolism appeared differentially phosphorylated in the control and H/R exposed mitochondria of mussels (Table 2). Most of these phosphorylated enzymes showed a modest change in abundance (typically, ∼20–40% change compared with the controls), except glutaryl-CoA dehydrogenase (showed a 2.5 decrease in the phosphorylation level) and phosphorylated kynurenine/alpha-aminoadipate aminotransferase and propionyl-CoA carboxylase beta chain that showed a ∼−5–7-fold higher abundance after H/R stress. In oysters (C. gigas), phosphorylated forms of enoyl-CoA isomerase and acyl-CoA dehydrogenase family enzymes (involved in amino acid and fatty acid catabolism) decreased in abundance after H/R stress in vivo (Sokolov et al., 2019). Presently, the functional consequences of reversible phosphorylation of these enzymes are not known but their high representation in differentially expressed mitochondrial phosphoproteome of bivalves after H/R stress indicates the importance of modulation of amino acid and fatty acid metabolism, possibly to facilitate the use of diverse mitochondrial substrates for ATP production during post-hypoxic recovery.
Mitochondrial protein quality control mechanisms play a key role in maintaining the integrity of the mitochondrial proteome and are commonly regulated during H/R stress at the transcriptional, translational and post-translational levels (Sokolov et al., 2019; Falfushynska et al., 2020; Steffen et al., 2020). Among these proteins, mitochondrial metalloendopeptidase OMA1 appears an important target for PTM. In M. edulis, the abundance of phosphorylated OMA1 form was strongly down-regulated in H/R stress, so that no phosphorylated form of OMA1 was found in the mitochondrial proteome after H/R stress. Similarly, in C. gigas exposed to hypoxia in vivo the abundance of phosphorylated OMA1 decreased ∼6-fold in hypoxia, and no phosphorylated OMA1 was found after 1 h of reoxygenation (Sokolov et al., 2019). These findings indicate that dephosphorylation of OMA1 plays an important role in response to H/R stress, particularly the post-hypoxic recovery, in facultative anaerobes such as marine bivalves, and can occur via intrinsic mitochondrial mechanisms. Dephosphorylation of OMA1 in response to low mitochondrial ATP levels might activate this enzyme, thereby fostering mitochondrial fragmentation and suppressing mitochondrial fusion in stress (Baker et al., 2014; Bohovych et al., 2015; Sokolov et al., 2019). Other important proteins involved in the maintenance of mitochondrial proteome showed an apparent change in phosphorylation status after the H/R stress (including the mitochondrial ribosomal protein S22, TOM20 and mitochondrial processing peptidase subunit beta), consistent with the key role of protein homeostasis in mitochondrial stress resistance (Sokolova, 2018). However, the functional implications of PTM of these proteins are not yet known even in model organisms and therefore, no conclusions can presently be drawn with regard to their possible effects on mussel mitochondria.
In conclusion, our study shows that isolated digestive gland mitochondria of the blue mussel M. edulis possess intrinsic regulatory mechanisms that improve mitochondrial efficiency and limit ROS generation during hypoxia-reoxygenation transition. These adaptive functional changes are triggered by oxygen fluctuations in isolated mitochondria within minutes and thus reflect autonomous mitochondrial mechanisms that do not require an intact cellular environment or retrograde nuclear signaling. The intrinsic mitochondrial responses to H/R stress are at least in part dependent on the shifts in the mitochondria phosphoproteome including such key pathways as OXPHOS, amino acid and fatty acid catabolism, and protein quality control, but do not appear to involve a change in SC formation. The findings of our present study complement earlier research that showed an important role of PTM in regulating glycolytic metabolic fluxes and substrate-level phosphorylation (Brooks and Storey, 1995, 1997; Russell and Storey, 1995; Dawson et al., 2013; Lama et al., 2013; Storey, 2015) and highlight the importance of reversible protein phosphorylation as a global metabolic regulatory mechanism during oxygen fluctuations in facultative anaerobes.
Data Availability Statement
The mass spectrometry proteomics data were deposited to the ProteomeXchange Consortium via the PRIDE partner repository (Vizcaíno et al., 2016) with the dataset identifier PXD027955 and 10.6019/PXD027955.
Author Contributions
ES and AB: methodology, investigation, data curation, validation, formal analysis, and writing - review and editing. LA: data curation, visualization, writing - original draft, and writing - review and editing. SM: data curation, validation, formal analysis, resources, and writing - review and editing. AF: methodology, resources, supervision, and writing - review and editing. DB: methodology, resources, supervision, validation, and writing - review and editing. CH: methodology, investigation, data curation, validation, and writing - review and editing. IS: conceptualization, methodology, validation, formal analysis, resources, supervision, project administration, funding acquisition, writing - original draft, and writing - review and editing. All authors contributed to the article and approved the submitted version.
Funding
This work was in part supported by the German Research Foundation (DFG) project “MitoBOX: the basis of hypoxia tolerance in marine mollusks” (DFG Award Nos. 415984732 and GZ: SO 1333/5-1) to IS, by the funding line Strategic Networks of the Leibniz Association within the scope of the Leibniz ScienceCampus Phosphorus Research Rostock (www.sciencecampus-rostock.de) to ES, IS, and LA, and by a Villum International Postdoc from Villum Fonden to AB.
Conflict of Interest
The authors declare that the research was conducted in the absence of any commercial or financial relationships that could be construed as a potential conflict of interest.
Publisher’s Note
All claims expressed in this article are solely those of the authors and do not necessarily represent those of their affiliated organizations, or those of the publisher, the editors and the reviewers. Any product that may be evaluated in this article, or claim that may be made by its manufacturer, is not guaranteed or endorsed by the publisher.
Supplementary Material
The Supplementary Material for this article can be found online at: https://www.frontiersin.org/articles/10.3389/fmars.2021.773734/full#supplementary-material
Footnotes
References
Astorga, M. P. (2014). Genetic considerations for mollusk production in aquaculture: current state of knowledge. Front. Genet. 5:435.
Babarro, J. M. F., Labarta, U., and Reiriz, M. J. F. (2007). Energy metabolism and performance of Mytilus galloprovincialis under anaerobiosis. J. Mar. Biol. Assoc. U. K. 87, 941–946. doi: 10.1017/S0025315407053726
Baker, M. J., Lampe, P. A., Stojanovski, D., Korwitz, A., Anand, R., Tatsuta, T., et al. (2014). Stress-induced OMA1 activation and autocatalytic turnover regulate OPA1-dependent mitochondrial dynamics. EMBO J. 33, 578–593.
Bayne, B. L. (2017). Chapter 6 - Metabolic Expenditure. Dev. Aquac. Fish. Sci. 41, 331–415. doi: 10.1016/B978-0-12-803472-9.00006-6
Bohovych, I., Fernandez, M. R., Rahn, J. J., Stackley, K. D., Bestman, J. E., Anandhan, A., et al. (2015). Metalloprotease OMA1 Fine-tunes Mitochondrial Bioenergetic Function and Respiratory Supercomplex Stability. Sci. Rep. 5:13989. doi: 10.1038/srep13989
Brooks, S. P. J., and Storey, K. B. (1995). Protein phosphorylation patterns during aestivation in the land snail Otala lactea. Mol. Cell. Biochem. 143, 7–13. doi: 10.1007/BF00925921
Brooks, S. P. J., and Storey, K. B. (1997). Glycolytic controls in estivation and anoxia: a comparison of metabolic arrest in land and marine molluscs. Comp. Biochem. Physiol. 118A, 1103–1114. doi: 10.1016/S0300-9629(97)00237-5
Buck, L. T., and Hochachka, P. W. (1993). Anoxic suppression of Na(+)-K(+)-ATPase and constant membrane potential in hepatocytes: support for channel arrest. Am. J. Phys. Regul. Integr. Compar. Physiol. 265, R1020–R1025. doi: 10.1152/ajpregu.1993.265.5.R1020
Buck, L. T., and Pamenter, M. E. (2006). Adaptive responses of vertebrate neurons to anoxia—Matching supply to demand. Respir. Physiol. Neurobiol. 154, 226–240. doi: 10.1016/j.resp.2006.03.004
Bundgaard, A., James, A. M., Harbour, M. E., Murphy, M. P., and Fago, A. (2020a). Stable mitochondrial CICIII2 supercomplex interactions in reptiles versus homeothermic vertebrates. J. of Exp. Biol. 223:jeb223776. doi: 10.1242/jeb.223776
Bundgaard, A., James, A. M., Joyce, W., Murphy, M. P., and Fago, A. (2018). Suppression of reactive oxygen species generation in heart mitochondria from anoxic turtles: the role of complex I S-nitrosation. J. Exp. Biol. 221:jeb174391. doi: 10.1242/jeb.174391
Bundgaard, A., Qvortrup, K., Rasmussen, L. J., and Fago, A. (2019). Turtles maintain mitochondrial integrity but reduce mitochondrial respiratory capacity in the heart after cold acclimation and anoxia. J. Exp. Biol. 222:jeb200410. doi: 10.1242/jeb.200410
Bundgaard, A., Ruhr, I. M., Fago, A., and Galli, G. L. J. (2020b). Metabolic adaptations to anoxia and reoxygenation: new lessons from freshwater turtles and crucian carp. Curr. Opin. Endocr. Metab. Res. 11, 55–64. doi: 10.1016/j.coemr.2020.01.002
Cadenas, S. (2018). Mitochondrial uncoupling, ROS generation and cardioprotection. Biochim. Biophys. Acta 1859, 940–950. doi: 10.1016/j.bbabio.2018.05.019
Cadiz, L., Bundgaard, A., Malte, H., and Fago, A. (2019). Hypoxia enhances blood O(2) affinity and depresses skeletal muscle O(2) consumption in zebrafish (Danio rerio). Comp. Biochem. Physiol. B Biochem. Mol. Biol. 234, 18–25. doi: 10.1016/j.cbpb.2019.05.003
Chaban, Y., Boekema, E. J., and Dudkina, N. V. (2014). Structures of mitochondrial oxidative phosphorylation supercomplexes and mechanisms for their stabilisation. Biochim. Biophys. Acta 1837, 418–426. doi: 10.1016/j.bbabio.2013.10.004
Cheng, H., Munro, D., Huynh, K., and Pamenter, M. E. (2021). Naked mole-rat skeletal muscle mitochondria exhibit minimal functional plasticity in acute or chronic hypoxia. Compar. Biochem. Physiol. Part B Biochem. Mol. Biol. 255:110596. doi: 10.1016/j.cbpb.2021.110596
Connor, K. M., and Gracey, A. Y. (2011). High-resolution analysis of metabolic cycles in the intertidal mussel Mytilus californianus. Am. J. Physiol. Regul. Integr. Compar. Physiol. 302, R103–R111. doi: 10.1152/ajpregu.00453.2011
Cook, D. G., Iftikar, F. I., Baker, D. W., Hickey, A. J. R., and Herbert, N. A. (2013). Low-O2 acclimation shifts the hypoxia avoidance behaviour of snapper (Pagrus auratus) with only subtle changes in aerobic and anaerobic function. J. Exp. Biol. 216, 369–378. doi: 10.1242/jeb.073023
Dawson, N. J., Biggar, K. K., and Storey, K. B. (2013). Characterization of Fructose-1,6-Bisphosphate Aldolase during Anoxia in the Tolerant Turtle, Trachemys scripta elegans: an Assessment of Enzyme Activity, Expression and Structure. PLoS One 8:e68830. doi: 10.1371/journal.pone.0068830
Du, D., Zhang, Y., Zhu, C., Chen, H., and Sun, J. (2021). Metabolic Regulation of Hypoxia-Inducible Factors in Hypothalamus. Front. Endocrinol. 12:650284. doi: 10.3389/fendo.2021.650284
Du, S. N. N., Mahalingam, S., Borowiec, B. G., and Scott, G. R. (2016). Mitochondrial physiology and reactive oxygen species production are altered by hypoxia acclimation in killifish (Fundulus heteroclitus). J. Exp. Biol. 219, 1130–1138. doi: 10.1242/jeb.132860
Ellington, W. R. (1983). The recovery from anaerobic metabolism in invertebrates. J. Exp. Zool. 228, 431–444. doi: 10.1002/jez.1402280305
Estabrook, R. W. (1967). [7] Mitochondrial respiratory control and the polarographic measurement of ADP: O ratios ✩. Methods Enzymol. 10, 41–47. doi: 10.1016/0076-6879(67)10010-4
Falfushynska, H. I., Sokolov, E., Piontkivska, H., and Sokolova, I. M. (2020). The Role of Reversible Protein Phosphorylation in Regulation of the Mitochondrial Electron Transport System During Hypoxia and Reoxygenation Stress in Marine Bivalves. Front. Mar. Sci. 7:467. doi: 10.3389/fmars.2020.00467
Galkin, A., Abramov, A. Y., Frakich, N., Duchen, M. R., and Moncada, S. (2009). Lack of Oxygen Deactivates Mitochondrial Complex I. J. Biol. Chem. 284, 36055–36061. doi: 10.1074/jbc.M109.054346
Galli, G. L. J., and Richards, J. G. (2014). Mitochondria from anoxia-tolerant animals reveal common strategies to survive without oxygen. J. Compar. Physiol. B 184, 285–302. doi: 10.1007/s00360-014-0806-3
Galli, G. L. J., Lau, G. Y., and Richards, J. G. (2013). Beating oxygen: chronic anoxia exposure reduces mitochondrial F1FO-ATPase activity in turtle (Trachemys scripta) heart. J. Exp. Biol. 216, 3283–3293. doi: 10.1242/jeb.087155
Garbarino, V. R., Orr, M. E., Rodriguez, K. A., and Buffenstein, R. (2015). Mechanisms of oxidative stress resistance in the brain: lessons learned from hypoxia tolerant extremophilic vertebrates. Arch. Biochem. Biophys. 576, 8–16. doi: 10.1016/j.abb.2015.01.029
Gerber, L., Clow, K. A., Katan, T., Emam, M., Leeuwis, R. H. J., Parrish, C. C., et al. (2019). Cardiac mitochondrial function, nitric oxide sensitivity and lipid composition following hypoxia acclimation in sablefish. J. Exp. Biol. 222:jeb208074. doi: 10.1242/jeb.208074
Gnaiger, E. (2012). “Mitochondrial pathways and respiratory control: an introduction to OXPHOS analysis” in Mitochondrial Physiological Network (MiPNet) 17.18, OROBOROS MiPNet Publications 2012. Available online at: Innsbruck., 1-64. Open access: http://www.bioblast.at/index.php/Gnaiger_2012_MitoPathways (accessed August 19, 2021).
Goldenthal, M. J., and Marín-García, J. (2004). Mitochondrial signaling pathways: a receiver/integrator organelle. Mol. Cell. Biochem. 262, 1–16. doi: 10.1023/B:MCBI.0000038228.85494.3b
Gosling, E. G. (1992). The Mussel Mytilus: ecology, Physiology, Genetics and Culture. Dev. Aquac. Fish. Sci. 25:589.
Gowthami, N., Sunitha, B., Kumar, M., Keshava Prasad, T. S., Gayathri, N., Padmanabhan, B., et al. (2019). Mapping the protein phosphorylation sites in human mitochondrial complex I (NADH: ubiquinone oxidoreductase): a bioinformatics study with implications for brain aging and neurodegeneration. J. Chem. Neuroanat. 95, 13–28. doi: 10.1016/j.jchemneu.2018.02.004
Greggio, C., Jha, P., Kulkarni, S. S., Lagarrigue, S., Broskey, N. T., Boutant, M., et al. (2017). Enhanced Respiratory Chain Supercomplex Formation in Response to Exercise in Human Skeletal Muscle. Cell. Metab. 25, 301–311. doi: 10.1016/j.cmet.2016.11.004
Guerra-Castellano, A., Díaz-Quintana, A., Pérez-Mejías, G., Elena-Real, C. A., González-Arzola, K., García-Mauriño, S. M., et al. (2018). Oxidative stress is tightly regulated by cytochrome phosphorylation and respirasome factors in mitochondria. Proc. Natl. Acad. Sci. 115, 7955–7960. doi: 10.1073/pnas.1806833115
Guppy, M., Fuery, C. J., and Flanigan, J. E. (1994). Biochemical principles of metabolic depression. Compar. Biochem. Physiol. Part B Biochem. Mol. Biol. 109, 175–189. doi: 10.1016/0305-0491(94)90001-9
Haider, F., Falfushynska, H. I., Timm, S., and Sokolova, I. M. (2020). Effects of hypoxia and reoxygenation on intermediary metabolite homeostasis of marine bivalves Mytilus edulis and Crassostrea gigas. Comp. Biochem. Physiol. A Mol. Integr. Physiol. 242:110657. doi: 10.1016/j.cbpa.2020.110657
Haider, F., Sokolov, E. P., and Sokolova, I. M. (2018). Effects of mechanical disturbance and salinity stress on bioenergetics and burrowing behavior of the soft-shell clam Mya arenaria. J. Exp. Biol. 221:jeb172643. doi: 10.1242/jeb.172643
Hansen, S. H., Andersen, M. L., Cornett, C., Gradinaru, R., and Grunnet, N. (2010). A role for taurine in mitochondrial function. J. Biomed. Sci. 17, S23–S23. doi: 10.1186/1423-0127-17-S1-S23
Helling, S., Huttemann, M., Ramzan, R., Kim, S. H., Lee, I., Muller, T., et al. (2012). Multiple phosphorylations of cytochrome c oxidase and their functions. Proteomics 12, 950–959. doi: 10.1002/pmic.201100618
Hickey, A. J. R., Renshaw, G. M. C., Speers-Roesch, B., Richards, J. G., Wang, Y., Farrell, A. P., et al. (2012). A radical approach to beating hypoxia: depressed free radical release from heart fibres of the hypoxia-tolerant epaulette shark (Hemiscyllum ocellatum). J. Compar. Physiol. B 182, 91–100. doi: 10.1007/s00360-011-0599-6
Hochachka, P. W., and Guppy, M. (1987). Metabolic Arrest and the Control of Biological Time. London: Harvard University Press. 227. doi: 10.4159/harvard.9780674184589
Hochachka, P. W., and Mommsen, T. P. (1983). Protons and anaerobiosis. Science 219, 1391–1397. doi: 10.1126/science.6298937
Hochachka, P. W., and Mustafa, T. (1972). Invertebrate facultative anaerobiosis. Science 178, 1056–1178. doi: 10.1126/science.178.4065.1056
Hochachka, P. W., and Somero, G. N. (2002). Biochemical Adaptation: mechanism and Process in Physiological Evolution. United Kingdom: Oxford University Press.
Honda, H. M., Korge, P., and Weiss, J. N. (2005). Mitochondria and ischemia/reperfusion injury. Ann. N. Y. Acad. Sci. 1047, 248–258. doi: 10.1196/annals.1341.022
Horbinski, C., and Chu, C. T. (2005). Kinase signaling cascades in the mitochondrion: a matter of life or death. Free Radic. Biol. Med. 38, 2–11. doi: 10.1016/j.freeradbiomed.2004.09.030
Hou, T., Zhang, R., Jian, C., Ding, W., Wang, Y., Ling, S., et al. (2019). NDUFAB1 confers cardio-protection by enhancing mitochondrial bioenergetics through coordination of respiratory complex and supercomplex assembly. Cell Res. 29, 754–766. doi: 10.1038/s41422-019-0208-x
Ivanina, A. V., Eilers, S., Kurochkin, I. O., Chung, J. S., Techa, S., Piontkivska, H., et al. (2010). Effects of cadmium exposure and intermittent anoxia on nitric oxide metabolism in eastern oysters, Crassostrea virginica. J. Exp. Biol. 213, 433–444. doi: 10.1242/jeb.038059
Ivanina, A. V., Froelich, B., Williams, T., Sokolov, E. P., Oliver, J. D., and Sokolova, I. M. (2011). Interactive effects of cadmium and hypoxia on metabolic responses and bacterial loads of eastern oysters Crassostrea virginica Gmelin. Chemosphere 82, 377–389. doi: 10.1016/j.chemosphere.2010.09.075
Ivanina, A. V., Kurochkin, I. O., Leamy, L., and Sokolova, I. M. (2012). Effects of temperature and cadmium exposure on the mitochondria of oysters (Crassostrea virginica) exposed to hypoxia and subsequent reoxygenation. J. Exp. Biol. 215, 3142–3154. doi: 10.1242/jeb.071357
Ivanina, A. V., Nesmelova, I., Leamy, L., Sokolov, E. P., and Sokolova, I. M. (2016). Intermittent hypoxia leads to functional reorganization of mitochondria and affects cellular bioenergetics in marine molluscs. J. Exp. Biol. 219, 1659–1674. doi: 10.1242/jeb.134700
Kadenbach, B. (2021). Complex IV - The regulatory center of mitochondrial oxidative phosphorylation. Mitochondrion 58, 296–302. doi: 10.1016/j.mito.2020.10.004
Kalpage, H. A., Bazylianska, V., Recanati, M. A., Fite, A., Liu, J., Wan, J., et al. (2019). Tissue-specific regulation of cytochrome c by post-translational modifications: respiration, the mitochondrial membrane potential, ROS, and apoptosis. FASEB J. 33, 1540–1553. doi: 10.1096/fj.201801417R
Kocha, K. M., Reilly, K., Porplycia, D. S. M., McDonald, J., Snider, T., and Moyes, C. D. (2015). Evolution of the oxygen sensitivity of cytochrome c oxidase subunit 4. Am. J. Physiol. Regul. Integr. Comp. Physiol. 308, R305–R320. doi: 10.1152/ajpregu.00281.2014
Kurochkin, I., Ivanina, A., Eilers, S., and Sokolova, I. (2008). Effects of environmental anoxia and re-oxygenation on mitochondrial function and metabolism of eastern oysters(Crassostrea virginica). Comp. Biochem. Physiol. A Mol. Integr. Physiol. 150:S161. doi: 10.1016/j.cbpa.2008.04.420
Lama, J. L., Bell, R. A., and Storey, K. B. (2013). Hexokinase regulation in the hepatopancreas and foot muscle of the anoxia-tolerant marine mollusc, Littorina littorea. Comp. Biochem. Physiol. B Biochem. Mol. Biol. 166, 109–116. doi: 10.1016/j.cbpb.2013.07.001
Lambert, A. J., and Brand, M. D. (2004). Superoxide production by NADH:ubiquinone oxidoreductase (complex I) depends on the pH gradient across the mitochondrial inner membrane. Biochem. J. 382, 511–517. doi: 10.1042/BJ20040485
Lapuente-Brun, E., Moreno-Loshuertos, R., Acín-Pérez, R., Latorre-Pellicer, A., Colás, C., Balsa, E., et al. (2013). Supercomplex Assembly Determines Electron Flux in the Mitochondrial Electron Transport Chain. Science 340, 1567L–1570. doi: 10.1126/science.1230381
Lee, P., Chandel, N. S., and Simon, M. C. (2020). Cellular adaptation to hypoxia through hypoxia inducible factors and beyond. Nat. Rev. Mol. Cell Biol. 21, 268–283. doi: 10.1038/s41580-020-0227-y
Lesnefsky, E. J., Chen, Q., Tandler, B., and Hoppel, C. L. (2017). Mitochondrial Dysfunction and Myocardial Ischemia-Reperfusion: implications for Novel Therapies. Annu. Rev. Pharmacol. Toxicol. 57, 535–565. doi: 10.1146/annurev-pharmtox-010715-103335
Lewis, J. M., Costa, I., Val, A. L., Almeida-Val, V. M., Gamperl, A. K., and Driedzic, W. R. (2007). Responses to hypoxia and recovery: repayment of oxygen debt is not associated with compensatory protein synthesis in the Amazonian cichlid. Astronotus ocellatus. J. Exp. Biol. 210, 1935–1943. doi: 10.1242/jeb.005371
Li, R., Zhang, W., Lu, J., Zhang, Z., Mu, C., Song, W., et al. (2020). The Whole-Genome Sequencing and Hybrid Assembly of Mytilus coruscus. Front. Genet. 11:440. doi: 10.3389/fgene.2020.00440
Li, W., and Godzik, A. (2006). Cd-hit: a fast program for clustering and comparing large sets of protein or nucleotide sequences. Bioinformatics 22, 1658–1659. doi: 10.1093/bioinformatics/btl158
Liu, Y., Merrill, R. A., and Strack, S. (2020). A-Kinase Anchoring Protein 1: emerging Roles in Regulating Mitochondrial Form and Function in Health and Disease. Cells 9:298. doi: 10.3390/cells9020298
Martino, P. A., Carlon, D. B., and Kingston, S. E. (2019). Blue Mussel (Genus Mytilus) Transcriptome Response to Simulated Climate Change in the Gulf of Maine. J. Shellfish Res. 38, 587–602. doi: 10.2983/035.038.0310
Mathers, K. E., and Staples, J. F. (2019). Differential posttranslational modification of mitochondrial enzymes corresponds with metabolic suppression during hibernation. Am. J. Physiol. Regul. Integr. Comp. Physiol. 317, R262–R269. doi: 10.1152/ajpregu.00052.2019
McBride, H. M., Neuspiel, M., and Wasiak, S. (2006). Mitochondria: more than just a powerhouse. Curr. Biol. 16, R551–R560. doi: 10.1016/j.cub.2006.06.054
Merrill, R. A., and Strack, S. (2014). Mitochondria: a kinase anchoring protein 1, a signaling platform for mitochondrial form and function. Int. J. Biochem. Cell Biol. 48, 92–6. doi: 10.1016/j.biocel.2013.12.012
Miwa, S., and Brand, M. D. (2003). Mitochondrial matrix reactive oxygen species production is very sensitive to mild uncoupling. Biochem. Soc. Trans. 31, 1300–1301. doi: 10.1042/bst0311300
Napolitano, G., Venditti, P., Fasciolo, G., Esposito, D., Uliano, E., and Agnisola, C. (2019). Acute hypoxia/reoxygenation affects muscle mitochondrial respiration and redox state as well as swimming endurance in zebrafish. J. Comp. Physiol. B 189, 97–108. doi: 10.1007/s00360-018-1198-6
Onukwufor, J. O., Kibenge, F., Stevens, D., and Kamunde, C. (2016). Hypoxia-reoxygenation differentially alters the thermal sensitivity of complex I basal and maximal mitochondrial oxidative capacity. Comp. Biochem. Physiol. A Mol. Integr. Physiol. 201, 87–94. doi: 10.1016/j.cbpa.2016.06.033
Onukwufor, J. O., MacDonald, N., Kibenge, F., Stevens, D., and Kamunde, C. (2014). Effects of hypoxia-cadmium interactions on rainbow trout (Oncorhynchus mykiss) mitochondrial bioenergetics: attenuation of hypoxia-induced proton leak by low doses of cadmium. J. Exp. Biol. 217, 831–840. doi: 10.1242/jeb.093344
Onukwufor, J. O., Stevens, D., and Kamunde, C. (2017). Combined effects of cadmium, temperature and hypoxia-reoxygenation on mitochondrial function in rainbow trout (Oncorhynchus mykiss). Aquat. Toxicol. 182, 129–141. doi: 10.1016/j.aquatox.2016.11.015
Ouillon, N., Sokolov, E. P., Otto, S., Rehder, G., and Sokolova, I. M. (2021). Effects of variable oxygen regimes on mitochondrial bioenergetics and reactive oxygen species production in a marine bivalve Mya arenaria. J. Exp. Biol. 22:jeb237156. doi: 10.1242/jeb.237156
Pagliarini, D. J., and Dixon, J. E. (2006). Mitochondrial modulation: reversible phosphorylation takes center stage? Trends Biochem. Sci. 31, 26–34. doi: 10.1016/j.tibs.2005.11.005
Pamenter, M. E. (2014). Mitochondria: a multimodal hub of hypoxia tolerance. Can. J. Zool. 92, 569–589. doi: 10.1139/cjz-2013-0247
Pamenter, M. E., Gomez, C. R., Richards, J. G., and Milsom, W. K. (2016). Mitochondrial responses to prolonged anoxia in brain of red-eared slider turtles. Biol. Lett. 12:20150797. doi: 10.1098/rsbl.2015.0797
Pamenter, M. E., Lau, G. Y., Richards, J. G., and Milsom, W. K. (2018). Naked mole rat brain mitochondria electron transport system flux and H(+) leak are reduced during acute hypoxia. J. Exp. Biol. 221:jeb171397. doi: 10.1242/jeb.171397
Paradis, S., Charles, A.-L., Meyer, A., Lejay, A., Scholey, J. W., Chakfé, N., et al. (2016). Chronology of mitochondrial and cellular events during skeletal muscle ischemia-reperfusion. Am. J. Physiol. Cell Physiol. 310, C968–C982. doi: 10.1152/ajpcell.00356.2015
Paterno, M., Bat, L., Souissi, J. B., Boscari, E., Chassanite, A., Congiu, L., et al. (2019). A Genome-Wide Approach to the Phylogeography of the Mussel Mytilus galloprovincialis in the Adriatic and the Black Seas. Front. Mar. Sci. 6:566. doi: 10.3389/fmars.2019.00566
Pell, V. R., Chouchani, E. T., Murphy, M. P., Brookes, P. S., and Krieg, T. (2016). Moving Forwards by Blocking Back-Flow: the Yin and Yang of MI Therapy. Circul. Res. 118, 898–906. doi: 10.1161/CIRCRESAHA.115.306569
Prabu, S. K., Anandatheerthavarada, H. K., Raza, H., Srinivasan, S., Spear, J. F., and Avadhani, N. G. (2006). Protein Kinase A-mediated Phosphorylation Modulates Cytochrome Oxidase Function and Augments Hypoxia and Myocardial Ischemia-related Injury. J. Biol. Chem. 281, 2061–2070. doi: 10.1074/jbc.M507741200
Radin, I., Mansilla, N., Rödel, G., and Steinebrunner, I. (2015). The Arabidopsis COX11 Homolog is Essential for Cytochrome c Oxidase Activity. Front. Plant Sci. 6:1091. doi: 10.3389/fpls.2015.01091
Ramírez-Aguilar, S. J., Keuthe, M., Rocha, M., Fedyaev, V. V., Kramp, K., Gupta, K. J., et al. (2011). The composition of plant mitochondrial supercomplexes changes with oxygen availability. J. Biol. Chem. 286, 43045–43053. doi: 10.1074/jbc.M111.252544
Reinders, J., Wagner, K., Zahedi, R. P., Stojanovski, D., Eyrich, B., van der Laan, M., et al. (2007). Profiling Phosphoproteins of Yeast Mitochondria Reveals a Role of Phosphorylation in Assembly of the ATP Synthase. Mol. Cell. Proteomics 6, 1896–1906. doi: 10.1074/mcp.M700098-MCP200
Rosca, M. G., Vazquez, E. J., Kerner, J., Parland, W., Chandler, M. P., Stanley, W., et al. (2008). Cardiac mitochondria in heart failure: decrease in respirasomes and oxidative phosphorylation. Cardiovasc. Res. 80, 30–39. doi: 10.1093/cvr/cvn184
Russell, E. L., and Storey, K. B. (1995). Regulation of enzymes of carbohydrate metabolism during anoxia in the salt marsh bivalve Geukensia demissus. Physiol. Zool. 68, 567–582. doi: 10.1086/physzool.68.4.30166345
Sappal, R., MacDougald, M., Fast, M., Stevens, D., Kibenge, F., Siah, A., et al. (2015). Alterations in mitochondrial electron transport system activity in response to warm acclimation, hypoxia-reoxygenation and copper in rainbow trout. Oncorhynchus mykiss. Aquat. Toxicol. 165, 51–63. doi: 10.1016/j.aquatox.2015.05.014
Signes, A., and Fernandez-Vizarra, E. (2018). Assembly of mammalian oxidative phosphorylation complexes I–V and supercomplexes. Essays Biochem. 62, 255–270. doi: 10.1042/EBC20170098
Śmietanka, B., Burzyński, A., Hummel, H., and Wenne, R. (2014). Glacial history of the European marine mussels Mytilus, inferred from distribution of mitochondrial DNA lineages. Heredity 113, 250–258. doi: 10.1038/hdy.2014.23
Sokolov, E. P., and Sokolova, I. M. (2019). Compatible osmolytes modulate mitochondrial function in a marine osmoconformer Crassostrea gigas (Thunberg, 1793). Mitochondrion 45, 29–37. doi: 10.1016/j.mito.2018.02.002
Sokolov, E. P., Markert, S., Hinzke, T., Hirschfeld, C., Becher, D., Ponsuksili, S., et al. (2019). Effects of hypoxia-reoxygenation stress on mitochondrial proteome and bioenergetics of the hypoxia-tolerant marine bivalve Crassostrea gigas. J. Proteomics 194, 99–111. doi: 10.1016/j.jprot.2018.12.009
Sokolova, I. (2018). Mitochondrial Adaptations to Variable Environments and Their Role in Animals’. Stress Tolerance. Integr. Comp. Biol. 58, 519–531. doi: 10.1093/icb/icy017
Sokolova, I. M., Sokolov, E. P., and Haider, F. (2019). Mitochondrial Mechanisms Underlying Tolerance to Fluctuating Oxygen Conditions: lessons from Hypoxia-Tolerant Organisms. Integr. Comp. Biol. 59, 938–952. doi: 10.1093/icb/icz047
Steffen, J. B. M., Falfushynska, H. I., Piontkivska, H., and Sokolova, I. M. (2020). Molecular Biomarkers of the Mitochondrial Quality Control Are Differently Affected by Hypoxia-Reoxygenation Stress in Marine Bivalves Crassostrea gigas and Mytilus edulis. Front. Mar. Sci. 7:604411. doi: 10.3389/fmars.2020.604411
Storey, K. B. (2015). Regulation of hypometabolism: insights into epigenetic controls. J. Exp. Biol. 218, 150–159. doi: 10.1242/jeb.106369
Storey, K. B., and Storey, J. M. (2004). Metabolic rate depression in animals: transcriptional and translational controls. Biol. Rev. 79, 207–233. doi: 10.1017/S1464793103006195
Sung, H. J., Ma, W., Wang, P.-Y., Hynes, J., O’Riordan, T. C., Combs, C. A., et al. (2010). Mitochondrial respiration protects against oxygen-associated DNA damage. Nat. Commun. 1:5. doi: 10.1038/ncomms1003
Timón-Gómez, A., and Barrientos, A. (2020). Mitochondrial respiratory chain composition and organization in response to changing oxygen levels. J. Life Sci. 2:10.36069/JoLS/20200601.
Tyanova, S., and Cox, J. (2018). Perseus: a Bioinformatics Platform for Integrative Analysis of Proteomics Data in Cancer Research. Methods Mol. Biol. 1711, 133–148. doi: 10.1007/978-1-4939-7493-1_7
Venditti, P., Di Stefano, L., and Di Meo, S. (2013). Mitochondrial metabolism of reactive oxygen species. Mitochondrion 13, 71–82. doi: 10.1016/j.mito.2013.01.008
Vismann, B., and Hagerman, L. (2008). Recovery from hypoxia with and without sulfide in Saduria entomon: potassium. ATP and behavior. Mar. Biol. Res. 4, 215–223. doi: 10.1080/17451000701877282
Vizcaíno, J. A., Csordas, A., del-Toro, N., Dianes, J. A., Griss, J., Lavidas, I., et al. (2016). 2016 update of the PRIDE database and its related tools. Nucleic Acids Res. 44, D447–D456. doi: 10.1093/nar/gkw880
Wang, Q., Fan, W., Cai, Y., Wu, Q., Mo, L., Huang, Z., et al. (2016). Protective effects of taurine in traumatic brain injury via mitochondria and cerebral blood flow. Amino. Acids 48, 2169–2177. doi: 10.1007/s00726-016-2244-x
Zorov, D. B., Juhaszova, M., and Sollott, S. J. (2006). Mitochondrial ROS-induced ROS release: an update and review. Biochim. Biophys. Acta 1757, 509–517. doi: 10.1016/j.bbabio.2006.04.029
Keywords: bioenergetics, mitochondria, respiration, oxidative stress, proteomics, posttranslational modification (PTM), bivalve, supercomplexes
Citation: Sokolov EP, Adzigbli L, Markert S, Bundgaard A, Fago A, Becher D, Hirschfeld C and Sokolova IM (2021) Intrinsic Mechanisms Underlying Hypoxia-Tolerant Mitochondrial Phenotype During Hypoxia-Reoxygenation Stress in a Marine Facultative Anaerobe, the Blue Mussel Mytilus edulis. Front. Mar. Sci. 8:773734. doi: 10.3389/fmars.2021.773734
Received: 10 September 2021; Accepted: 18 October 2021;
Published: 05 November 2021.
Edited by:
Vengatesen Thiyagarajan (Rajan), The University of Hong Kong, Hong Kong SAR, ChinaCopyright © 2021 Sokolov, Adzigbli, Markert, Bundgaard, Fago, Becher, Hirschfeld and Sokolova. This is an open-access article distributed under the terms of the Creative Commons Attribution License (CC BY). The use, distribution or reproduction in other forums is permitted, provided the original author(s) and the copyright owner(s) are credited and that the original publication in this journal is cited, in accordance with accepted academic practice. No use, distribution or reproduction is permitted which does not comply with these terms.
*Correspondence: Inna M. Sokolova, aW5uYS5zb2tvbG92YUB1bmktcm9zdG9jay5kZQ==