- 1Zoology Department, Faculty of Science, Assiut University, Assiut, Egypt
- 2Department of Integrated Biosciences, Graduate School of Frontier Sciences, The University of Tokyo, Kashiwa, Japan
- 3Department of Radiation Effects Research, National Institute of Radiological Sciences, Quantum Life and Medical Science Directorate, National Institutes for Quantum Science and Technology, Chiba, Japan
- 4Department of Genome Repair Dynamics, Radiation Biology Center, Graduate School of Biostudies, Kyoto University, Kyoto, Japan
- 5Department of Radiological Sciences, School of Health Sciences at Narita, IUHW, Narita, Japan
Morphological alterations and nuclear abnormalities in fish erythrocytes have been used in many studies as bioindicators of environmental mutagens including ionizing radiation. In this study, adult Japanese medaka (Oryzias latipes) were irradiated with gamma rays at a low dose rate (9.92 μGy/min) for 7 days, giving a total dose of 100 mGy; and morphological alterations, nuclear abnormalities, and apoptotic cell death induced in peripheral erythrocytes were investigated 8 h and 7 days after the end of the irradiation. A variety of abnormalities, such as tear-drop cell, crenated cell, acanthocyte, sickled cell, micronucleated cell, eccentric nucleus, notched nucleus, and schistocyte, were induced in the peripheral erythrocytes of the wild-type fish, and a less number of abnormalities and apoptotic cell death were induced in the p53-deficient fish. These results indicate that low dose-rate chronic irradiation of gamma rays can induce cytotoxic and genotoxic effects in the peripheral erythrocytes of medaka, and p53-deficient medaka are tolerant to the gamma-ray irradiation than the wild type on the surface.
Introduction
Gamma irradiation induces ionization and cellular damage, including morphological alterations, nuclear abnormalities, DNA damage, and apoptosis (Muslimovic et al., 2012; Sayed et al., 2014, 2016b; Mohamed et al., 2016). In medaka fish blood cells, Sayed et al. (2014) reported that gamma radiation induces DNA aberrations such as nuclear abnormalities. The tumor protein p53 plays a critical role in determining whether DNA will be repaired or the damaged cell will undergo apoptosis. A mutant medaka strain has a p53 gene after x-rays (Takai T. et al., 2004). P53-deficient medaka fish showed less degree of damage after exposure to γ-irradiation, UVA, and 4-non-ylphenol (Sayed et al., 2014, 2016a, 2017, 2018a,b, 2020) confirming the resistance of p53 mutant to apoptosis and morphological alterations after gamma-ray irradiation.
Ionizing radiation (IR) directly and/or indirectly damages DNA double strands, and the accumulated DNA lesions would lead to the transformation of the cell. In the case where the dose of radiation is low and the induced DNA lesions are repaired by the cells, it has been considered that the risk of carcinogenesis can be negligible (International Commission on Radiological Protection [ICRP], 2007). When cells are exposed to the same dose of IR, the lower the dose rate is, the less the risk of carcinogenesis per dose is, since induced DNA lesions would be repaired by the cell during the irradiation period and genomic damage can be reduced (“dose-rate effect”; Steel et al., 1987; Nakamura et al., 2005, 2006). Since 100 mGy is the dose for the lower limit of epidemiologically evaluated carcinogenic risk (International Commission on Radiological Protection [ICRP], 2007), IR exposure at doses lower than 100 mGy is considered not to increase carcinogenic risk, and its biological effects have not been emphasized.
After the accident of the Chernobyl nuclear power plant in 1986, researchers recognized the need to closely examine the impacts of low dose and low dose-rate irradiation on living organisms and ecosystems, and numerous studies have been started and are still ongoing (International Atomic Energy Agency [IAEA], 2006; Braga-Tanaka et al., 2018; Kamstra et al., 2018). In 2011, the Fukushima Daiichi nuclear power plant caused the accident to contaminate the wide area of east Japan (International Atomic Energy Agency [IAEA], 2015; Johansen et al., 2015; Maruyama et al., 2021). It has made the researchers to recognize again the need to elucidate the impacts of low dose and low dose-rate irradiation and has accelerated the research, especially in Japan (Hiyama et al., 2012; Braga-Tanaka et al., 2018; Shimura et al., 2018). As a result of these studies, many biological effects of low dose and low dose-rate chronic irradiation other than potential carcinogenic promotion have been revealed or confirmed, and the effects on human health and ecosystems are attracting researchers and social attention.
Even though DNA lesions by low dose and low dose-rate irradiation would be less than to induce carcinogenic risk epidemiologically, it is obvious that low dose and low dose-rate irradiation, no matter how small it is, induces DNA lesions at some degree and instabilizes genomes (Rothkamm and Löbrich, 2003; Nakamura et al., 2005, 2006). Based on this fact, International Commission on Radiological Protection (ICRP) recommends to use the linear no-threshold model (LNT model) in radiation protection (International Commission on Radiological Protection [ICRP], 2007), which assumes the absence of a lower threshold for stochastic effects by radiation and assumes a linear relationship between dose and the health risk by irradiation.
The level of DNA damage and repair capacity can be measured directly after irradiation by rapid formation of γ-H2AX foci (Gwozdzinski, 1991; Rogakou et al., 1998). Since teleosts erythrocytes are nucleated and have limited ability to repair DNA damages, genome instability induced by environmental mutagens can be evaluated with high sensitivity, monitoring the nuclear abnormalities like micronucleus (MN) in teleosts erythrocytes (Al-Sabti and Metcalfe, 1995; Mekkawy et al., 2011; Anbumani and Mohankumar, 2012; Praveen Kumar et al., 2015; Sayed et al., 2016a). Japanese medaka (Oryzias latipes) has been a useful animal model in radiation biology, and a large body of knowledge of the biological impacts of irradiation have been accumulated (Shima and Shimada, 1991; Shimada and Shima, 2001; Kubota et al., 1992; Kuwahara et al., 2003; Tsyusko et al., 2011; Yasuda et al., 2012, 2016; Sayed et al., 2014, 2016b,2017; Perez-Gelvez et al., 2021a,b). To estimate the genome instability induced by low dose and low dose-rate irradiation, we irradiated adult medaka with low dose and low dose-rate gamma rays and examined the nuclear abnormalities induced in erythrocytes in this study.
Materials and Methods
Medaka
Adult (about 6 months old) wild-type (Hd-rR strain) female medaka fish (O. latipes) and homozygous p53-deficient female (Yasuda et al., 2012, 2016) fish were used. They were kept at 26–28°C with a 14 h light: 10 h dark cycle and fed brine shrimp (Artemia franciscana) once a day and powder diet (Tetra-fin, Spectrum Brands Japan Inc., Tokyo, Japan) twice a day, in a fish facility in The University of Tokyo.
Irradiation
Low dose-rate and long-term irradiation of gamma rays was conducted with the Low Dose and Low Dose-rate Irradiation System (137Cs, Radiation Biology Center, Kyoto University) with a dose rate of 9.92 μGy/min for 7 days to give a total dose of 100 mGy. During the irradiation, the fish were put in a water tank (width 30 cm × depth 10 cm × height 20 cm) with free swimming and kept at 26–28°C with a 14 h light: 10 h dark cycle and fed powder diet (Tetra-fin) once a day. Irradiation was stopped temporarily (for 15–30 min) once a day to feed the fish. The concentrations of NH4+, NO2–, and NO3– of the water in the tanks were measured daily with Packtest™ ammonium, nitrite, and nitrate (Kyoritsu Chemical-check Lab., Corp., Yokohama, Japan), respectively, according to the instructions of the manufacturer, and the water was replaced if necessary.
Blood Sample Collection From Fish and Cell Viability Assay
Blood samples were collected from the caudal veins of the control and the irradiated fish after they were deeply anesthetized with 0.02% (w/v) MS-222 solution. Each sample was immediately placed on ice to prevent endogenous DNA destruction and to inhibit DNA repair before the chemical fixation of the cells during preparation. Blood smears were prepared on clean glass slides (triplicate slides from each fish). The numbers of blood cells in the blood samples were counted, and their viability was checked with a hemocytometer and trypan blue dye exclusion test before fixation. Only blood samples showing >90% viability and with more than 106 cells/100 μl cells were used.
Observation of Morphological Alterations and Nuclear Abnormalities in Blood Cells
Blood smears were fixed by dipping the slides in absolute methanol, air-dried, and stained with May-Grünwald solution for 15 min following 6% Giemsa staining for 30 min as reported previously (Tavares-Dias and Moraes, 2003). Slides with high staining quality were selected and coded, randomized, and scored in a blinded manner. In each group, 10,000 cells (minimum of 1,000 cells per slide) were examined as previously reported (Al-Sabti and Metcalfe, 1995) under a 40x objective with a 10x eyepiece to identify morphologically altered erythrocytes. Established criteria for identifying micronuclei (Schmidt, 1975) were strictly followed to ensure authentic scoring.
Apoptosis Detection
Apoptotic erythrocytes were stained by acridine orange (AO; A1031, Life Technologies, Carlsbad, CA, United States) in a modified protocol by Sayed et al. (2016b). In brief, blood samples were smeared on clean glass slides and rinsed in phosphate-buffered saline (PBS; pH = 7.2), and AO solution (17 μg/L AO in PBS buffer) was added to the slides. The slides were incubated for 30 min in the dark and then washed with PBS four times every 30 min. Decolorized blood cells were fixed in 4% paraformaldehyde in PBS for 5 min and observed under a fluorescence microscope (BX50; Olympus, Tokyo, Japan) equipped with a digital color still camera (DP-70; Olympus, Tokyo, Japan) (Abrams et al., 1993; Furutani-Seiki et al., 1996).
Immunostaining of Cleaved Caspase 3
Immunostaining was performed as previously described with minor modifications (Sayed et al., 2016b,2018b). Blood samples (2 μl) were collected from the fish and mixed with 5 μl of PBS, further diluted to 106 cells/ml in PBS, and then 100 μl of cell suspension (106 cells/ml) was mixed with 100 μl of 2x fixation buffer [50 mM Tris (pH 7.5), 2% Triton-X100, 2 mM EDTA, 0.02% BSA, and 0.4% paraformaldehyde]. The mixture was immediately vortexed and incubated for 10 min at room temperature in the dark. Then, the fixed erythrocytes were centrifuged at 2,500 rpm for 5 min at 4°C, washed twice with 200 μl PBS, and further incubated for 30 min with blocking buffer (0.5% Triton X-100, 0.2% normal goat serum). The cells were again centrifuged at 2,500 rpm for 5 min at 4°C, resuspended in 0.1% BSA/PBS (−), and incubated with the primary antibody [1:250, #9661, Cleaved Caspase-3 (Asp175), Cell Signaling Technology, Danvers, MA, United States] for 3 h at room temperature. The cells were collected by centrifugation and kept in 0.1% BSA/PBS (−) overnight at 4°C. After that, the cells were further incubated with Alexa Fluor 488-conjugated secondary antibody [A-11008, Goat anti-Rabbit IgG (H + L), Thermo Fisher Scientific, Waltham, MA, United States] in 3% BSA/PBS (−) for 3 h at room temperature. Then, the cells were washed three times with 0.1% BSA/PBS (−), counterstained with 4-,6- diamino-2-phenylindole (DAPI, Fujifilm Wako Pure Chemical, Osaka, Japan) for 5 min at room temperature, washed with PBS, re-suspended in 4 μl of PBS, and mounted on a new glass slide. Fluorescence images were captured using an inverted fluorescence microscope (IX-81, Olympus, Tokyo, Japan) equipped with a digital still camera (DS Ri1, Nikon, Tokyo, Japan).
Statistical Analysis
The data are presented as mean ± standard deviation (SD). Multiple comparisons were performed using one-way ANOVA using the SPSS package and the Tukey’s test at 0.05 significance level.
Ethics Statement
All experiments were performed in accordance with the Japanese laws and guidelines for the care of experimental animals according to the University of Tokyo Animal Experiment Enforcement Rule. The protocol was approved by the Committee on the Ethics of Animal Experiments of the University of Tokyo (Permit Number: C-19-05). All surgery was performed under MS-222, and all efforts were made to minimize suffering.
Results
Morphological Alterations and Nuclear Abnormalities in Erythrocytes of Irradiated Wild-Type and p53-Deficient Medaka
Morphologically altered erythrocytes or those with nuclear abnormalities were seldom observed in the non-irradiated wild-type fish. Normal erythrocytes in the non-irradiated wild-type and p53-deficient fish were shown in Figures 1A,D,G,J, respectively. After the low dose-rate irradiation (100 mGy in total at 9.92 μGy/min) of gamma rays, morphological alterations and nuclear abnormalities were induced in the erythrocytes of the wild-type and p53-deficient medaka, such as micronucleated cell (Mn; Figure 1B), acanthocyte (Ac; Figures 1C,H,I), crenated cell (Cr; Figures 1C,H,I), sickled cell (Sk; Figures 1C,E,I,K), cell with notched nucleus (Nn; Figures 1E,K), tear-drop cell (Tr; Figures 1F,I), cell with eccentric nucleus (Ecn; Figure 1H), and schistocyte (Sch; Figure 1L). These altered cells were observed 8 h and 7 days after the end of the irradiation, whereas the micronuclei cells were observed only in the irradiated wild-type fish 8 h after the end of the irradiation (Figure 1B). The erythrocytes of the irradiated wild-type fish showed more serious morphological alterations than the erythrocytes of the irradiated p53-deficient fish. These results clearly demonstrated that the gamma-ray irradiation of low dose (100 mGy in total) at low dose-rate (9.92 μGy/min) for 7 days can induce various types of morphological abnormalities in peripheral erythrocytes of medaka fish.
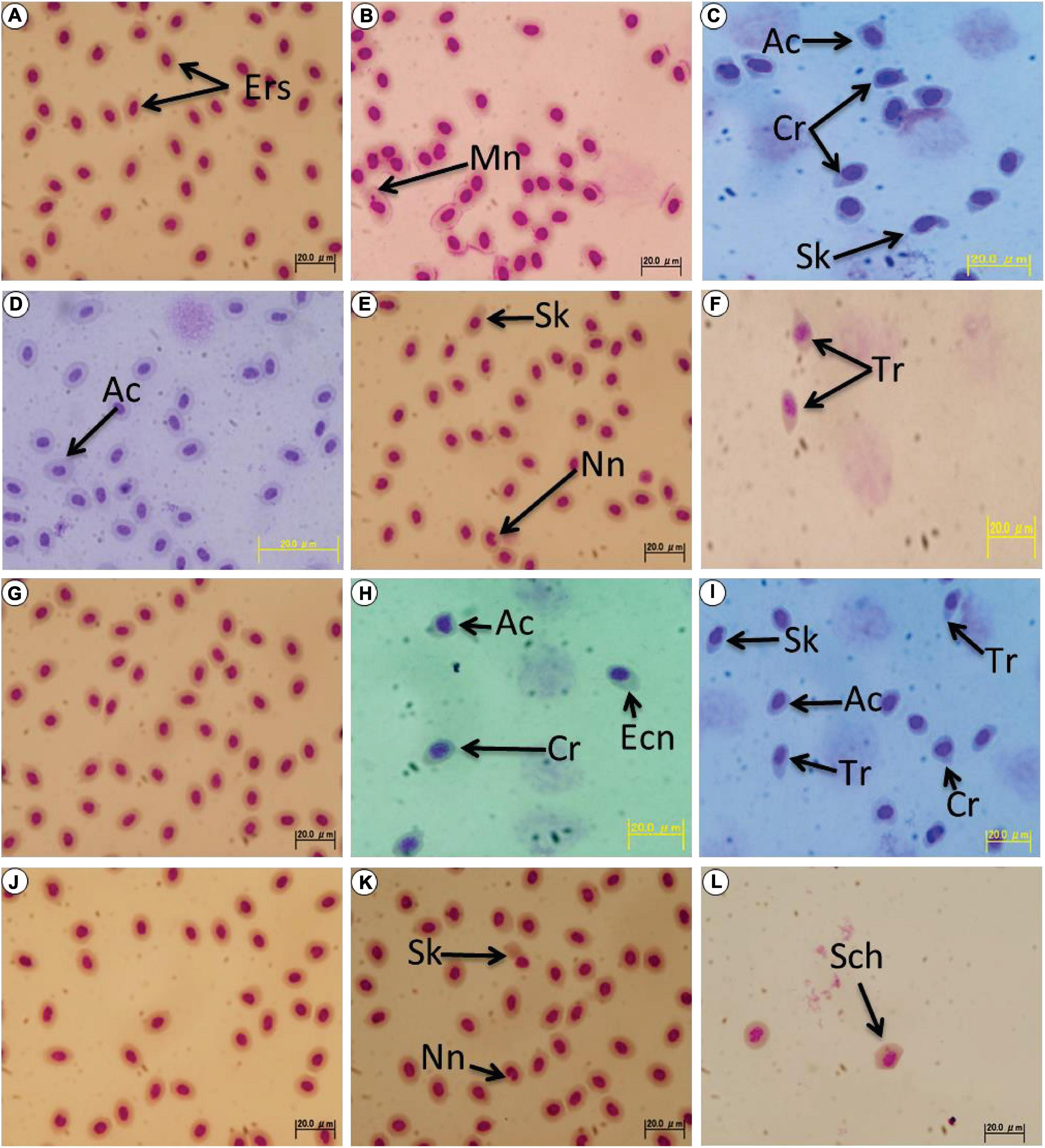
Figure 1. Wright-Giemsa stained blood smears showing morphological and nuclear abnormal erythrocytes found in the irradiated medaka. (A,D) Normal erythrocytes collected from the non-irradiated wild-type female (Hd-rR strain) are presented. Morphologically altered erythrocytes or those with nuclear abnormalities were seldom observed in the non-irradiated wild-type fish, and an acanthocyte (Ac) is shown in d. (B,C) The erythrocytes of the wild-type fish 8 h after the end of the irradiation. (E,F) The erythrocytes of the wild-type fish 7 days after the end of the irradiation. (G,J) The erythrocytes of the non-irradiated p53-deficient fish. Abnormal erythrocytes were seldom observed. (H,I) The erythrocytes of the p53-deficient fish 8 h after the end of the irradiation. (K,L) The erythrocytes of the p53-deficient fish 7 days after the end of the irradiation. Ers, erythrocytes (normal erythrocytes); Tr, tear-drop cell; Cr, crenated cell; Ac, acanthocyte; Sk, sickled cell; Mn, micronucleated cell; Ecn, cell with eccentric nucleus; Nn, notched nucleus; and Sch, schistocyte.
We found that 4.28 ± 1.33% of the erythrocytes of the irradiated wild-type fish altered morphologically 8 h after the end of the irradiation, and the morphologically altered cells decreased to 3.10 ± 1.00% 7 days after the end of the irradiation. The background level of the morphologically altered erythrocytes in the non-irradiated wild-type fish was less than 2% (Table 1). These results strongly suggest that the damages in the erythrocytes and erythropoietic cells induced by the irradiation were removed via apoptosis or repaired over 7 days after the irradiation in the wild-type fish. In contrast, in the p53-deficient fish, 2.60 ± 1.05% erythrocytes had altered morphology 8 h after the end of the irradiation, and the percentage of the altered erythrocytes decreased to the control level (1.68 ± 0.98%) within 7 days after the irradiation. These results demonstrated that p53-deficient fish were more tolerant to the low dose and low dose-rate irradiation of gamma rays on the surface.
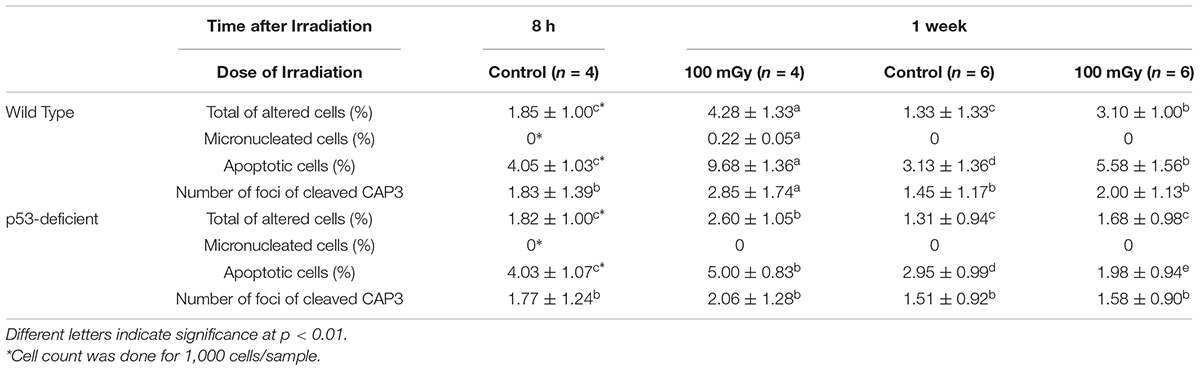
Table 1. Percentage of altered, micronucleated, apoptotic erythrocytes (mean ± SD) per 100 erythrocytes in the irradiated medaka (O. latipes) after the low dose (100 mGy in total) and low dose-rate irradiation (at 9.92 μGy/min for 7 days) of gamma-rays.
Apoptosis in Erythrocytes of Irradiated Wild-Type and p53-Deficient Medaka
In the blood smear prepared from the irradiated wild-type fish, 9.68 ± 1.36% and 5.58 ± 1.56% of the peripheral erythrocytes were apoptotic 8 h and 7 days after the end of the irradiation, respectively. Since 4.05 ± 1.03% and 3.13 ± 1.36% of the erythrocytes were apoptotic 8 h and 7 days after the end of the irradiation in the non-irradiated fish, respectively, the low dose (100 mGy in total) and low dose-rate (9.92 μGy/min) irradiation of gamma rays significantly induced apoptosis in peripheral erythrocytes of medaka (p < 0.01; Table 1). On the other hand, 5.00 ± 0.83% and 1.98 ± 0.94% of the erythrocytes were apoptotic in the irradiated p53-deficient medaka 8 h and 7 days after the end of the irradiation, respectively, demonstrating that significantly less apoptotic cell death was induced in the peripheral erythrocytes of the p53-deficient fish (p < 0.01) after the irradiation (Table 1). In the non-irradiated p53-deficient fish, 4.03 ± 1.07 and 2.95 ± 0.99% of the erythrocytes were apoptotic 8 h and 7 days after the end of the irradiation, respectively, demonstrating that the background level of the apoptosis in erythrocytes is the same between the wild-type and p53-deficient fish without irradiation.
Formation of Cleaved CAP3 Foci in Erythrocytes of Irradiated Wild-Type and p53-Deficient Medaka
Erythrocytes of medaka have a nucleus and can conduct apoptotic cell death (Sayed et al., 2016b,2017). We found that foci of cleaved CAP3 obviously increased in the erythrocytes of irradiated wild-type fish 8 h after the end of the irradiation (2.85 ± 1.74 foci) and returned to the background level 7 days after the end of the irradiation (Figure 2 and Table 1). In contrast, foci of cleaved CAP3 did not change in the p53-deficient erythrocytes after the irradiation. These results demonstrate that the erythrocytes of wild-type medaka conduct both CAP3-dependent and CAP3 independent apoptosis after the low dose and low dose-rate irradiation of gamma rays, while the erythrocytes of p53-deficient fish only conduct CAP3 independent apoptosis after the irradiation.
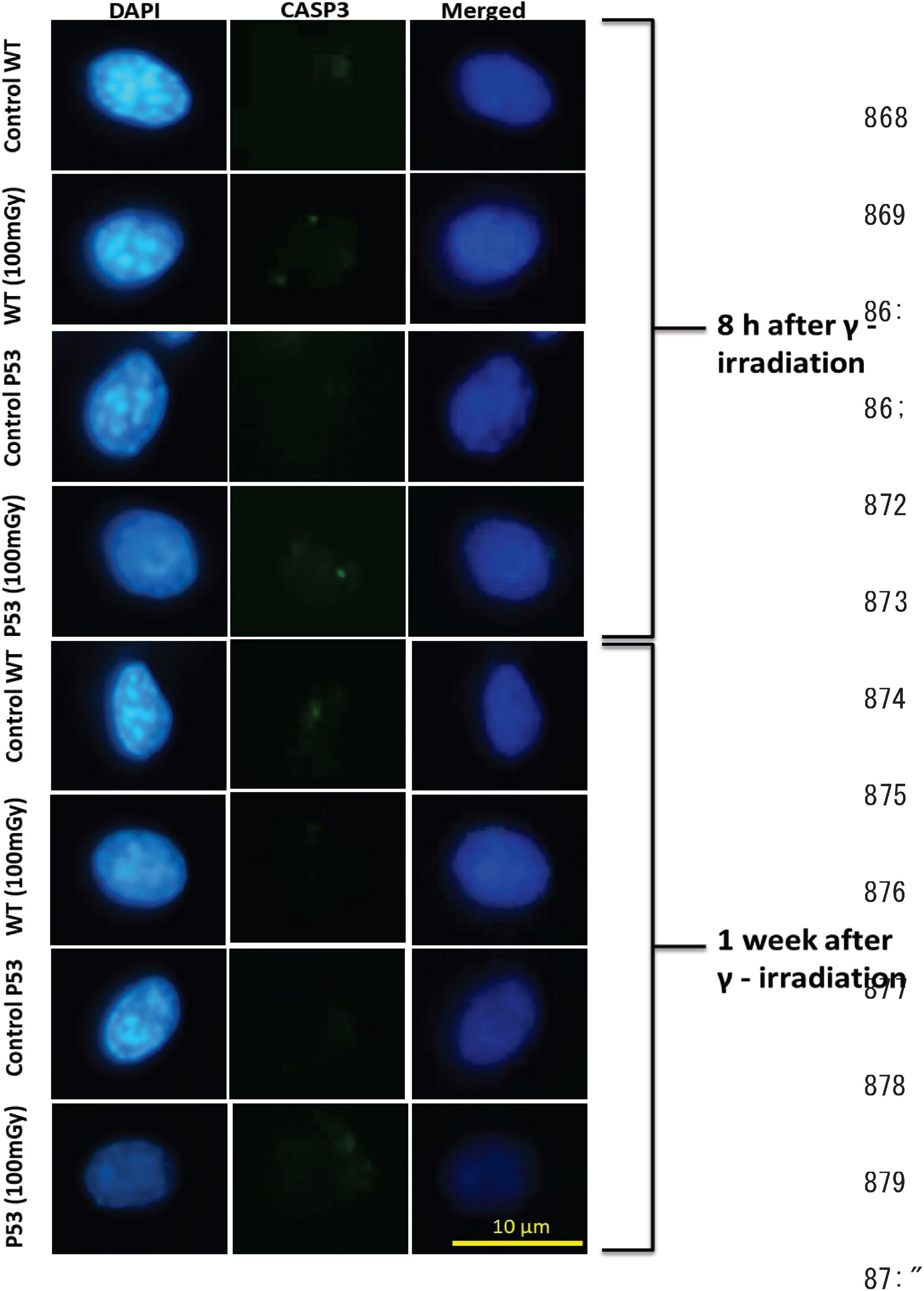
Figure 2. Foci of cleaved caspase 3 (CASP3) in the erythrocytes of the irradiated medaka (O. latipes). Left/center/right column shows DAPI-stained (blue), anti-cleaved CASP3 immunostained (green), and merged images of nuclei of the erythrocytes 8 h and 7 days after the end of the irradiation, respectively. Scale bar represents 10 μm.
Discussion
To date, the effects of gamma-ray irradiation on fish erythrocytes have been studied after acute exposure of high-dose (>1 Gy) gamma rays, and induction of DNA damage has been reported (Anbumani and Mohankumar, 2012; Sayed et al., 2014, 2016b,2017; Praveen Kumar et al., 2015; Lemos et al., 2017). On the other hand, it was reported that the dose was 130–140 μGy/h in the aquatic environment after the Fukushima accident, which was extensively lower than those used in the previous studies (Johansen et al., 2015). In this study, we conducted low dose and low dose-rate irradiation of gamma rays (100 mGy in total at 9.92 μGy/min for 7 days) on adult medaka fish and found that significant increases of nuclear abnormalities and apoptotic cell death could be induced in the erythrocytes of the irradiated fish after the irradiation. We also found that p53 deficiency suppressed the induction of nuclear abnormalities and apoptotic cell death in peripheral erythrocytes after the low dose and low dose-rate irradiation.
Since erythrocytes are the major site of production of reactive oxygen species (Khoory et al., 2016), as the site of oxygen transport and fish erythrocytes are nucleated, they are highly sensitive to oxidative mutagens and have been studied to evaluate DNA damage induced by mutagens in the environments, including IR irradiation (Al-Sabti and Metcalfe, 1995; Sawhney and Johal, 2000; Sayed et al., 2007, 2014, 2016b, 2017, 2018a,b; Mekkawy et al., 2011; Anbumani and Mohankumar, 2012). There are no studies in the literature on the cytotoxic and genotoxic effects on fish erythrocytes of chronic or long-period irradiation of low dose (<1 Gy) IR, although DNA damage after acute irradiation of x-rays (0.1–1 Gy) or gamma rays (2–15 Gy) is reported in erythrocytes of adult zebrafish (Lemos et al., 2017), carp (Praveen Kumar et al., 2015), and medaka (Sayed et al., 2014, 2017). Anbumani and Mohankumar (2012) reported a significant increase of micronuclei and cellular abnormalities in the erythrocytes of Cyprinidae fish, Catla catla, after the irradiation of gamma rays (5 Gy) at two dose rates of 3.2 and 0.002 Gy/min, and they suggested that prolonged exposure induced less apoptotic cell death in the erythrocytes than acute exposure. It is widely accepted that IR irradiation at a low dose-rate induces weaker cytotoxicity and genotoxicity presumably because induced DNA lesions are repaired by the cell during irradiation (“dose-rate effect”; Steel et al., 1987; Nakamura et al., 2005, 2006; Tanaka et al., 2013; Rühm et al., 2015). Because of the “dose-rate effect,” it was expected that DNA damage induced by low dose and low dose-rate irradiation of IR will be repaired by the cell, and the cytotoxicity and genotoxicity induced would be negligible and hard to be detected. However, the results presented here indicate that the potential cytotoxicity and genotoxicity of low dose and low dose-rate irradiation in the environments can be evaluated on medaka peripheral erythrocytes.
Vertebrate embryos, especially early-stage embryos, are highly proliferative and susceptible to IR irradiation (Jacquet, 2004; Streffer, 2004; De Santis et al., 2007; Hurem et al., 2017; Yasuda et al., 2020). Since fish are oviparous and their embryos develop outside the body of the female, the fish embryo has been the major model to investigate the impacts of IR irradiation on embryonic development, and the researchers reported that the low dose irradiation less than 100 mGy of gamma rays or x-rays could disturb the embryonic development of zebrafish (Miyachi et al., 2003; Bladen et al., 2007; Jaafar et al., 2013; Freeman et al., 2014; Gagnaire et al., 2015). More recently, the chronic or long-term exposure of low dose gamma rays at low dose-rate was conducted, and the adverse effects on zebrafish embryonic development have been revealed (36.8 mGy at 0.4 mGy/h, Hurem et al., 2017; 0.01–0.10 Gy at 55 mGy/min, Zhao et al., 2019). Transcriptome analysis revealed that low dose IR irradiation as low as 1.62 mGy can alter the gene expression profile of zebrafish embryo, inducing abnormalities in embryonic development (Hurem et al., 2017). Kamstra et al. (2018) found that the IR irradiation at low dose-rate (8.7 mGy/day for 27 days) alters DNA methylation in zebrafish. In this study, we conducted low dose-rate irradiation of gamma rays at 9.92 μGy/min ( = 14.3 mGy/day) for 7 days, and it can be reasonable that the adverse effects on the adult fish erythropoiesis were detected.
Micronucleus test is frequently used in fish erythrocytes to evaluate environmental pollution with mutagens, since MN has a relationship with DNA damage and mutation rate (Takai A. et al., 2004; Praveen Kumar et al., 2015; Sayed et al., 2017, 2018b). It would be worth noting that the low dose and low dose-rate irradiation of gamma rays in this study induced MN in the peripheral erythrocytes of wild-type medaka; in contrast, high dose (≤15 Gy) of gamma rays was necessary to induce MN in wild-type erythrocytes after the acute irradiation (7.8 Gy/mi) in our previous studies (Sayed et al., 2014, 2016b,2017). Considering the result of this study that the low dose and low dose-rate irradiation of gamma rays did not induce MN in the erythrocytes of the p53-deficient medaka, the p53 functions to regulate cell cycle checkpoints and/or DNA repair might be crucial to induce MN after irradiation.
We also found that apoptotic cell death in the irradiated erythrocytes was suppressed in the absence of p53 functions, strongly suggesting that p53 has a central role in the cellular responses against the low dose and low dose-rate irradiation as well as against acute high-dose irradiation as previously reported (Yasuda et al., 2012; Sayed et al., 2017). Attenuation of p53 function extends the survival after irradiation with high-dose (10–40 Gy) gamma rays in zebrafish embryos (Duffy and Wickstrom, 2007); of course, p53 plays a crucial role as a tumor suppressor in zebrafish (Guo et al., 2013). Reduction of apoptotic cell death in irradiated medaka erythrocytes may look like an advantage, but it may also have disadvantages.
The current radiation protection is based on the LNT model (Biological Effects of Ionizing Radiation [BEIR], 2006; International Commission on Radiological Protection [ICRP], 2007); however, the argument is still ongoing (Rühm et al., 2015; Cardarelli and Ulsh, 2018; Leblanc and Burtt, 2019). This study provided the point of view that low dose and low dose-rate irradiation induces negligible biological effects on the cell surface, because the induced cytotoxicity and genotoxicity are counteracted and canceled by the protecting mechanisms of the cell. Actually, ataxia telangiectasia (AT)-deficient cultured cells show hypersensitivity to low dose-rate irradiation of x-rays (Nakamura et al., 2006), and knockdown of the 80-kDa subunit of Ku protein, an essential non-homologous end-joining pathway of DNA repair, made the zebrafish embryos hyper-sensitive to gamma-ray irradiation (Bladen et al., 2007). Any low dose and low dose-rate IR irradiation can have an adverse effect on living cells, organisms, and ecosystems, and they might be accepting the risks and paying costs to protect themselves. It is necessary to keep this point of view in mind to deal with radiation protection.
Conclusion
This study presented the evidence that low dose (100 mGy) and low dose-rate (9.92 μGy/min) gamma-ray irradiation induces nuclear and cytosolic abnormalities such as MN and apoptotic cell death in peripheral erythrocytes in adult medaka fish and that the potential cytotoxicity and genotoxicity by low dose and low dose-rate IR irradiation in the environments can be evaluated with peripheral erythrocytes of medaka.
Data Availability Statement
The raw data supporting the conclusions of this article will be made available by the authors, without undue reservation.
Ethics Statement
This animal study was reviewed and approved by the Committee on the Ethics of Animal Experiments of The University of Tokyo (Permit Number: C-19-05).
Author Contributions
AS, KN, HM, JK, and SO designed the experiments. AS, KN, TN, JK, and SO conducted the experiments. AS and SO analyzed the data and drafted the manuscript. AS, KN, HM, JK, and SO interpreted the result of the experiment. KN, HM, and JK edited the manuscript. All authors read and approved the final manuscript.
Funding
This research was supported in part by the Grants-in-Aid for Scientific Research (18H04135 to HM, 17J03688 to KN, and 21K12236 to SO) from the Ministry of Education, Culture, Sports, Science and Technology (MEXT) of Japan. This study was conducted through the Joint Usage/Research Center Program of the Radiation Biology Center, Kyoto University.
Conflict of Interest
The authors declare that the research was conducted in the absence of any commercial or financial relationships that could be construed as a potential conflict of interest.
Publisher’s Note
All claims expressed in this article are solely those of the authors and do not necessarily represent those of their affiliated organizations, or those of the publisher, the editors and the reviewers. Any product that may be evaluated in this article, or claim that may be made by its manufacturer, is not guaranteed or endorsed by the publisher.
References
Abrams, J. M., White, K., Fessler, L. I., and Steller, H. (1993). Programmed cell death during Drosophila embryogenesis. Development 117, 29–43.
Al-Sabti, K., and Metcalfe, C. D. (1995). Fish micronuclei for assessing genotoxicity in water. Mutat. Res. 343, 121–135. doi: 10.1016/0165-1218(95)90078-0
Anbumani, S., and Mohankumar, M. N. (2012). Gamma radiation induced micronuclei and erythrocyte cellular abnormalities in the fish Catla catla. Aqua. Toxicol. 122-123, 125–132. doi: 10.1016/j.aquatox.2012.06.001
Biological Effects of Ionizing Radiation [BEIR] (2006). Health Risks From Exposure to Low Levels of Ionizing Radiation. BEIR VII Phase 2. Washington, DC: National Academies Press.
Bladen, C. L., Flowers, M. A., Miyake, K., Podolsky, R. H., Barrett, J. T., Kozlowski, D. J., et al. (2007). Quantification of ionizing radiation-induced cell death in situ in a vertebrate embryo. Radiat. Res. 168, 149–157. doi: 10.1667/RR0803.1
Braga-Tanaka, I., Tanaka, S., Kohda, A., Takai, D., Nakamura, S., Ono, T., et al. (2018). Experimental studies on the biological effects of chronic low dose-rate radiation exposure in mice: overview of the studies at the institute for environmental sciences. Int. J. Rad. Biol. 94, 423–433. doi: 10.1080/09553002.2018.1451048
Cardarelli, J. J., and Ulsh, B. A. (2018). It is time to move beyond the linear no-threshold theory for low-dose radiation protection. Dose-Response 16:1559325818779651. doi: 10.1177/1559325818779651
De Santis, M., Cesari, E., Nobili, E., Straface, G., Cavaliere, A. F., and Caruso, A. (2007). Radiation effects on development. Birth Defects Res. Part C Embryo Today 81, 177–182. doi: 10.1002/bdrc.20099
Duffy, K. T., and Wickstrom, E. (2007). Zebrafish tp53 knockdown extends the survival of irradiated zebrafish embryos more effectively than the p53 inhibitor pifithrin. Cancer Biol. Ther. 6, 675–678. doi: 10.4161/cbt.6.5.3956
Freeman, J. L., Weber, G. J., Peterson, S. M., and Nie, L. H. (2014). Embryonic ionizing radiation exposure results in expression alterations of genes associated with cardiovascular and neurological development, function, and disease and modified cardiovascular function in zebrafish. Front. Genet. 5:268. doi: 10.3389/fgene.2014.00268
Furutani-Seiki, M., Jiang, Y. J., Brand, M., Heisenberg, C. P., Houart, C., Beuchle, D., et al. (1996). Neural degeneration mutants in the zebrafish, Danio rerio. Development 123, 229–239.
Gagnaire, B., Cavalié, I., Pereira, S., Floriani, M., Dubourg, N., Camilleri, V., et al. (2015). External gamma irradiation-induced effects in early-life stages of zebrafish. Danio rerio. Aquat. Toxicol. 169, 69–78. doi: 10.1016/j.aquatox.2015.10.005
Guo, L., Liew, H., Camus, S., Goh, A. M., Chee, L. L., Lunny, D. P., et al. (2013). Ionizing radiation induces a dramatic persistence of p53 protein accumulation and DNA damage signaling in mutant p53 zebrafish. Oncogene 32, 4009–4016. doi: 10.1038/onc.2012.409
Gwozdzinski, K. (1991). Ionizing radiation-induced structural modification of human red blood cells. Radiat. Environ. Biophys. 30, 45–52. doi: 10.1007/BF01595573
Hiyama, A., Nohara, C., Kinjo, S., Taira, W., Gima, S., Tanahara, A., et al. (2012). The biological impacts of the Fukushima nuclear accident on the pale grass blue butterfly. Sci. Rep. 2:570. doi: 10.1038/srep00570
Hurem, S., Martín, L. M., Brede, D. A., Skjerve, E., Nourizadeh-Lillabadi, R., Lind, O. C., et al. (2017). Dose-dependent effects of gamma radiation on the early zebrafish development and gene expression. PLoS One 12:e0179259. doi: 10.1371/journal.pone.0179259
International Atomic Energy Agency [IAEA] (2006). Environmental Consequences of the Chernobyl Accident and their Remediation: Twenty Years of Experience. Vienna: International Atomic Energy Agency.
International Atomic Energy Agency [IAEA] (2015). The Fukushima Daiichi Accident – The Report by the Director General. Vienna: International Atomic Energy Agency.
International Commission on Radiological Protection [ICRP] (2007). Publication 103. The 2007 Recommendations of the International Commission on Radiological Protection. Oxford: Pergamon Press. Ann ICRP 37 (2–4).
Jaafar, L., Podolsky, R. H., and Dynan, W. S. (2013). Long-term effects of ionizing radiation on gene expression in a zebrafish model. PLoS One 8:e69445. doi: 10.1371/journal.pone.0069445
Jacquet, P. (2004). Sensitivity of germ cells and embryos to ionizing radiation. J. Biol. Regul. Homeost. 18, 106–114.
Johansen, M. P., Ruedig, E., Tagami, K., Uchida, S., Higley, K., and Beresford, N. A. (2015). Radiological dose rates to marine fish from the fukushima daiichi accident: the first three years across the North Pacific. Environ. Sci. Technol. 49, 1277–1285. doi: 10.1021/es505064d
Kamstra, J. H., Hurem, S., Martin, L. M., Lindeman, L. C., Legler, J., Oughton, D., et al. (2018). Ionizing radiation induces transgenerational effects of DNA methylation in zebrafish. Sci. Rep. 8:15373. doi: 10.1038/s41598-018-33817-w
Khoory, J., Estanislau, J., Elkhal, A., Lazaar, A., Melhorn, M. I., Brodsky, A., et al. (2016). Ligation of glycophorin a generates reactive oxygen species leading to decreased red blood cell function. PLoS One 11:e0141206. doi: 10.1371/journal.pone.0141206
Kubota, Y., Shimada, A., and Shima, A. (1992). Detection of y-ray-induced DNA damages in malformed dominant lethal embryos of the Japanese medaka (Oryzias latipes) using AP-PCR fingerprinting. Mutat Res. 283, 263–270.
Kuwahara, Y., Shimada, A., Mitani, H., and Shima, A. (2003). Gamma-Ray exposure accelerates spermatogenesis of medaka fish, Oryzias latipes. Mol. Reprod. Dev. 65, 204–211. doi: 10.1002/mrd.10261
Leblanc, J. E., and Burtt, J. J. (2019). Radiation biology and its role in the canadian radiation protection framework. Health Phys. 117, 319–329. doi: 10.1097/HP.0000000000001060
Lemos, J., Neuparth, T., Trigo, M., Costa, P., Vieira, D., Cunha, L., et al. (2017). Single low-dose ionizing radiation induces genotoxicity in adult zebrafish and its non-irradiated progeny. Bull. Environ. Contam. Toxicol. 98, 190–195. doi: 10.1007/s00128-016-2006-1
Lenarczyk, M., and Slowikowska, M. G. (1995). The micronucleus assay using peripheral blood reticulocytes from X-ray-exposed mice. Mutat. Res. Environ. Mutagen. Relat. Subj. 335, 229–234. doi: 10.1016/0165-1161(95)00025-9
Maruyama, K., Wang, B., Doi, K., Ishibashi, K., Ichikawa, S., Furuhata, Y., et al. (2021). Radiation effects on wild medaka around Fukushima Dai-ichi nuclear power plant assessed by micronucleus assay. J. Radiat. Res. 62, 79–85. doi: 10.1093/jrr/rraa116
Mekkawy, I. A. A., Mahmoud, U. M., and Sayed, A. H. (2011). Effects of 4-nonylphenol on blood cells of the African catfish Clarias gariepinus (Burchell, 1822). Tissue Cell 43, 223–229. doi: 10.1016/j.tice.2011.03.006
Miyachi, Y., Kanao, T., and Okamoto, T. (2003). Marked depression of time interval between fertilization period and hatching period following exposure to low-dose X-rays in zebrafish. Environ. Res. 93, 216–219. doi: 10.1016/S0013-9351(03)00042-2
Mohamed, M. A., El Saeid, A. A., and Ahmed, M. A. (2016). Cellular response of blood and hepatic tissue to gamma irradiation. J. Radiat. Res. Appl. Sci. 9, 242–248.
Muslimovic, A., Johansson, P., and Hammarsten, O. (2012). “Measurement of H2AX phosphorylation as a marker of ionizing radiation induced cell damage,” in Current Topics in Ionizing Radiation Research, ed. M. Nenoi (London: IntechOpen).
Nakamura, H., Fukami, H., Hayashi, Y., Tachibana, A., Nakatsugawa, S., Hamaguchi, M., et al. (2005). Cytotoxic and mutagenic eEffects of chronic low-dose-rate irradiation on TERT-immortalized human cells. Radiat. Res. 163, 283–288. doi: 10.1667/RR3310
Nakamura, H., Yasui, Y., Saito, N., Tachibana, A., Komatsu, K., and Ishizaki, K. (2006). DNA repair defect in AT cells and their hypersensitivity to low-dose-rate radiation. Radiat. Res. 165, 277–282. doi: 10.1667/RR3519.1
Perez-Gelvez, Y. N. C., Perez-Gelvez, Y. N. C., Unger, S., Kurz, S., Rosenbalm, K., Wright, W. M., et al. (2021a). Chronic exposure to low doses of ionizing radiation impacts the processing of glycoprotein N-linked glycans in Medaka (Oryzias latipes). Int. J. Radiat. Biol. 97, 401–420. doi: 10.1080/09553002.2021.1864500
Perez-Gelvez, Y. N. C., Camus, A. C., Bridger, R., Wells, L., Rhodes, O. E. Jr., and Bergmann, C. W. (2021b). Effects of chronic exposure to low levels of IR on Medaka (Oryzias latipes): a proteomic and bioinformatic approach. Int. J. Radiat. Biol. 97, 1485–1501. doi: 10.1080/09553002.2021.1962570
Praveen Kumar, M. K., Shyama Soorambail, K., Soorambail, K. S., Bhagatsingh Harisingh, S., D’costa, A., and Ramesh Chandra, C. (2015). The effect of gamma radiation on the Common carp (Cyprinus carpio): In vivo genotoxicity assessment with the micronucleus and comet assays. Mutat. Res. Genet. Toxicol. Environ. Mutagen. 792, 19–25. doi: 10.1016/j.mrgentox.2015.08.005
Rogakou, E. P., Pilch, D. R., Orr, A. H., Ivanova, V. S., and Bonner, W. M. D. N. A. (1998). Double-stranded breaks induce histone H2AX phosphorylation on serine 139. J. Biol. Chem. 273, 5858–5868. doi: 10.1074/jbc.273.10.5858
Rothkamm, K., and Löbrich, M. (2003). Evidence for a lack of DNA double-strand break repair in human cells exposed to very low x-ray doses. Proc. Natl. Acad. Sci. U.S.A. 100, 5057–5062. doi: 10.1073/pnas.0830918100
Rühm, W., Woloschak, G. E., Shore, R. E., Azizova, T. V., Grosche, B., Niwa, O., et al. (2015). Dose and dose-rate effects of ionizing radiation: a discussion in the light of radiological protection. Radiat. Environ. Biophys. 54, 379–401. doi: 10.1007/s00411-015-0613-6
Sawhney, A. K., and Johal, M. S. (2000). Erythrocyte alterations induced by malathion in Channa punctatus (Bloch). Bull. Environ. Contam. Toxicol. 64, 398–405. doi: 10.1007/s001280000014
Sayed, A.-D., Ibrahim, A. T., Mekkawy, I. A., and Mahmoud, U. M. (2007). Acute effects of ultraviolet-A radiation on African catfish Clarias gariepinus (Burchell, 1822). J. Photochem. Photobiol. B Biol. 89, 170–174. doi: 10.1016/j.jphotobiol.2007.09.010
Sayed, A. E.-D. H., Saleh, S. M. M., and Mitani, H. (2020). Comparative histology of wild-type and p53-deficient medaka (Oryzias latipes): nephrotoxic effect of ultraviolet A radiation. Photochem. Photobiol. Sci. 19, 261–273.
Sayed, A. E. H., Igarashi, K., Watanabe-Asaka, T., and Mitani, H. (2017). Double strand break repair and gamma-H2AX formation in erythrocytes of medaka (Oryzias latipes) after gamma-irradiation. Environ. Pollut. 224, 35–43.
Sayed, A. H., Kataoka, C., Oda, S., Kashiwada, S., and Mitani, H. (2018a). Sensitivity of medaka (Oryzias latipes) to 4-nonylphenol subacute exposure; erythrocyte alterations and apoptosis. Environ. Toxicol. Pharmacol. 58, 98–104.
Sayed, A. H., Watanabe-Asaka, T., Oda, S., Kashiwada, S., and Mitani, H. (2018b). gamma-H2AX foci as indication for the DNA damage in erythrocytes of medaka (Oryzias latipes) intoxicated with 4-nonylphenol. Environ. Sci. Pollut. Res. Int. 27, 18966–18971.
Sayed, A. H., Oda, S., and Mitani, H. (2014). Nuclear and cytoplasmic changes in erythrocytes of p53-deficient medaka fish (Oryzias latipes) after exposure to gamma-radiation. Mutat. Res. Genet. Toxicol. Environ. Mutagen. 771, 64–70. doi: 10.1016/j.mrgentox.2014.01.013
Sayed, A. H., Watanabe-Asaka, T., Oda, S., and Mitani, H. (2016b). Apoptotic cell death in erythrocytes of p53-deficient medaka (Oryzias latipes) after gamma-irradiation. Int. J. Radiat. Biol. 92, 572–576. doi: 10.1080/09553002.2016.1222091
Sayed, A. H., Watanabe-Asaka, T., Oda, S., and Mitani, H. (2016a). Apoptosis and morphological alterations after UVA irradiation in red blood cells of p53 deficient Japanese medaka (Oryzias latipes). J. Photochem. Photobiol. B Biol. 161, 1–8.
Shima, A., and Shimada, A. (1991). Development of a possible nonmammalian test system for radiation-induced germ-cell mutagenesis using a fish, the Japanese medaka (Oryzias latipes). PNAS 88, 2545–2549. doi: 10.1073/pnas.88.6.2545
Shimada, A., and Shima, A. (2001). High incidence of mosaic mutations induced by irradiating paternal germ cells of the medaka fish, Oryzias latipes. Mutat. Res. Genet. Toxicol. Environ. Mutagen. 495, 33–42. doi: 10.1016/S1383-5718(01)00193-0
Shimura, T., Yamaguchi, I., Terada, H., and Kunugita, N. (2018). Lessons learned from radiation biology: health effects of low levels of exposure to ionizing radiation on humans regarding the Fukushima accident. J. Natl. Inst. Public Health 67, 115–122. doi: 10.20683/jniph.67.1_115
Steel, G. G., Deacon, J. M., Duchesne, G. M., Horwich, D. A., Kelland, L. R., and Peacock, J. H. (1987). The dose-rate effect in human tumour cells. Radiother. Oncol. 9, 299–310. doi: 10.1016/S0167-8140(87)80151-2
Streffer, C. (2004). (2004) Bystander effects, adaptive response and genomic instability induced by prenatal irradiation. Mutat. Res. 568, 79–87. doi: 10.1016/j.mrfmmm.2004.07.014
Takai, A., Kagawa, N., and Fujikawa, K. (2004). Susceptibility of male and female medaka (Oryzias latipes) fish to spontaneous and X-ray induced micronucleus formation in gill cells. Mutat. Res. Genet. Toxicol. Environ. Mutagen. 558, 131–136. doi: 10.1016/j.mrgentox.2003.11.009
Takai, T., Kagawa, N., and Fujikawa, K. (2004). Dose and Time-Dependent Responses for micronucleus induction by x-rays and fast neutrons in gill cells of Medaka (Oryzias latipes). Env. Mol. Mut. 44, 108–112.
Tanaka, K., Kohda, A., and Satoh, K. (2013). Dose-rate effects and dose and dose-rate effectiveness factor on frequencies of chromosome aberrations in splenic lymphocytes from mice continuously exposed to low-dose-rate gamma-radiation. J. Radiol. Protect. 33, 61–70. doi: 10.1667/RR1238.1
Tavares-Dias, M., and Moraes, F. R. (2003). Haematological evaluation of Tilapia rendalli Boulenger, 1896 (Osteichthyes: Cichilidae) captured in a fee fishing farm in Franca. Sao Paulo state, Brazil. (in Portuguese). Biosci. J. 19, 103–110.
Tsyusko, O., Glenn, T., Joice, Y., Yi, G., Jones, K., Aizawa, K., et al. (2011). Differential genetic responses to ionizing irradiation in individual families of Japanese medaka, Oryzias latipes. Mut. Res. Genet. Toxicol. Environ. Mutag. 718, 18–23. doi: 10.1016/j.mrgentox.2010.11.001
Yasuda, T., Funayama, T., Nagata, K., Li, D., Endo, T., Jia, Q., et al. (2020). Collimated microbeam reveals that the proportion of non-damaged cells in irradiated blastoderm determines the success of development in medaka (Oryzias latipes) Embryos. Biology 9:447. doi: 10.3390/biology9120447
Yasuda, T., Kimori, Y., Nagata, K., Igarashi, K., Watanabe-Asaka, T., Oda, S., et al. (2016). Irradiation-injured brain tissues can self-renew in the absence of the pivotal tumor suppressor p53 in the medaka (Oryzias latipes) embryo. J. Radiat. Res. 57, 9–15. doi: 10.1093/jrr/rrv054
Yasuda, T., Oda, S., Li, Z., Kimori, Y., Kamei, Y., Ishikawa, T., et al. (2012). Gamma-ray irradiation promotes premature meiosis of spontaneously differentiating testis-ova in the testis of p53-deficient medaka (Oryzias latipes). Cell Death Dis. 3:e395. doi: 10.1038/cddis.2012.133
Keywords: gamma-rays, low dose-rate irradiation, erythrocyte, apoptosis, medaka
Citation: Sayed AE-DH, Nagata K, Nakazawa T, Mitani H, Kobayashi J and Oda S (2021) Low Dose-Rate Irradiation of Gamma-Rays-Induced Cytotoxic and Genotoxic Alterations in Peripheral Erythrocytes of p53-Deficient Medaka (Oryzias latipes). Front. Mar. Sci. 8:773481. doi: 10.3389/fmars.2021.773481
Received: 10 September 2021; Accepted: 11 October 2021;
Published: 01 December 2021.
Edited by:
Daniel Carneiro Moreira, University of Brasilia, BrazilCopyright © 2021 Sayed, Nagata, Nakazawa, Mitani, Kobayashi and Oda. This is an open-access article distributed under the terms of the Creative Commons Attribution License (CC BY). The use, distribution or reproduction in other forums is permitted, provided the original author(s) and the copyright owner(s) are credited and that the original publication in this journal is cited, in accordance with accepted academic practice. No use, distribution or reproduction is permitted which does not comply with these terms.
*Correspondence: Alaa El-Din H. Sayed, YWxhYXNheWVkQGF1bi5lZHUuZWc=
†These authors have contributed equally to this work