- 1National Center for Genetic Engineering and Biotechnology, National Science and Technology Development Agency, Pathum Thani, Thailand
- 2National Biobank of Thailand, National Science and Technology Development Agency, Pathum Thani, Thailand
Long-chain polyunsaturated fatty acids (LC-PUFAs) are essential for growth and health of larval marine animals. Marine animals have a limited capability for LC-PUFA synthesis, and the larvae must obtain LC-PUFAs from diet. The protist Aurantiochytrium limacinum (AL) is abundant in 22:6 n-3 (docosahexaenoic acid, DHA), 22:5 n-3 (docosapentaenoic acid, DPA) and 16:0 fatty acids, which qualifies it as an LC-PUFA source for feed application. Therefore, in this study, a common feed containing lower amounts of total LC-PUFAs, Thalasiosira weissflogii, was replaced with AL at graded proportions and supplied to Penaeus monodon larvae from mysis (M) 1 to post-larval (PL) 2 stages to supplement LC-PUFAs in the diet. After that, all shrimp from PL2 to PL12 were continuously reared and subjected to the same diet regime, which was a combination of Artemia and commercial dried feed. The AL-supplemented PL2 shrimp demonstrated marked accumulation of the key fatty acids present in AL—16:0, DPA and DHA. The supplemented larvae showed no difference in growth during the supplementation period from M1 to PL2; however, average body weight and biomass were increased in PL12 shrimp that were fed earlier with AL. Lipidomic analysis revealed that profiles of fatty acids but not lipid classes/subclasses in PL shrimp reflected the supplied diet. The main saturated fatty acid (SFA, 16:0) predominantly accumulated in acylglycerols, which are energy-reserve lipids, in PL2 shrimp. Both LC-PUFAs (DHA and DPA) were preferentially deposited in phospholipids or structural lipids. Furthermore, while the amounts of both LC-PUFAs increased along with the amount of supplied AL, that of the SFA did not. This suggests that LC-PUFAs were prioritized to be stored over SFA when both types of fatty acids were present in high amounts. This analysis substantiates the importance of LC-PUFAs and provides an insight into how different types of the dietary fatty acids were differentially accumulated in lipid classes and subclasses for their biological functions.
Introduction
Lipids, represented by various classes and subclasses, perform various biological functions in living organisms. In aquatic animals, acylglycerols, particularly triglycerides, are the major source of energy for physical activities and cellular functions (Sargent et al., 1993; Tocher et al., 2008; Rey et al., 2018; Xie et al., 2020). Most energy in the form of ATP is produced from fatty acids associated with triglycerides through the beta-oxidation process (Berg et al., 2002a). In addition to energy provision, lipids play essential roles in cellular structure (Alberts et al., 2002). Phospholipids and sterols are key components of cell membrane structure, which is essential for the osmoregulation mechanism of marine animals (Chapelle et al., 1982; Sargent et al., 1993; Huang et al., 2019); moreover, phospholipids and sterols are also precursors of cellular signaling molecules and hormones that regulate growth, molting, the immune system and reproduction (Tocher et al., 2008).
The core of lipid molecules is esterified with diverse fatty acid constituents, which vary in chain length and saturation. Among the varieties of fatty acid types, n-3 and n-6 long-chain polyunsaturated fatty acids (LC-PUFAs) are essential for growth and health of marine animals, in particular at the larval stage (Glencross, 2009). They are an energy source and precursors of eicosanoids, which are local hormones that function in signaling and regulation of several biological mechanisms including immune response, growth and development (Bhathena, 2006; Wimuttisuk et al., 2013; Engelking, 2015; Stillwell, 2016). However, these fatty acids must be obtained from the diet because of the low activities of fatty acid elongation and desaturation in marine animals (Kanazawa et al., 1979; Glencross, 2009). Deficiency of LC-PUFAs causes abnormal development and growth of several marine animals (Watanabe, 1993; Harel et al., 2002; Samocha et al., 2010). Several studies have been carried out to supplement LC-PUFAs in the diet for marine animals (Ishizaki et al., 1998; Boglino et al., 2012; Lund et al., 2012; Watanabe et al., 2016; Visudtiphole et al., 2018).
Thraustochytrids are single-cell protists, which are abundant producers of LC-PUFAs including docosahexaenoic acid (DHA, 22:6 n-3), eicosapentaenoic acid (EPA, 20:5 n-3), docosapentaenoic acid (DPA, 22:5 n-3), and arachidonic acid (ARA, 20:4 n-6). They have been applied to aquafeed as an alternative LC-PUFA source to fish oil (Lewis et al., 1999; Unagul et al., 2017). In larviculture, they are used to enrich LC-PUFAs in live Artemia or rotifer, which are fed to marine animal larvae to promote growth and health (Leger et al., 1986; Barclay and Zeller, 1996; Harel et al., 2002). The effect of thraustochytrid enrichment on shrimp larviculture has been studied at the early post-larval stage (PL1), the earliest that can ingest enriched Artemia (Visudtiphole et al., 2018). However, supplementation of dietary LC-PUFAs for shrimp larvae at earlier developmental stages was not studied. Therefore, in this study, a thraustochytrid, Aurantiochytrium limacinum BCC52274 (AL), was applied to Penaeus monodon larvae from mysis 1 (M1) to PL2 to supplement DHA in the diet. To do so, a common feed for the larvae, Thalasiosira weissflogii (TW), which is deficient in LC-PUFAs, was replaced with AL at graded ratios and the effects of the supplementation on larval growth and lipidomics were then evaluated.
Lipidomics is the holistic study of lipid molecules found in biological organisms. With the complexity of lipid molecules, analytical tools in combination with lipid structure database are required to elucidate lipid molecules from unknown samples in both qualitative and quantitative analyses (Cajka and Fiehn, 2014). Lipidomic analysis using liquid chromatography-high resolution mass spectrometry (LC-HRMS) has been employed to understand the physiological processes of marine species including blue mussels and Pacific white shrimp (Huang et al., 2019; Laudicella et al., 2020). The technique has also been applied to study the diet effect on aquatic animals—for example, different lipid sources and fish meal replacement in swimming crab and Pacific white shrimp, respectively (Xie et al., 2020; Yuan et al., 2021). Therefore, as well as growth evaluation, lipidomic analysis using LC-HRMS was carried out to examine the effects of the AL supplement on lipid profiles in shrimp. Moreover, since AL was found to contain distinctly high amounts of not only LC-PUFAs (DHA and DPA) but also a saturated fatty acid (16:0), the acquired lipidomic data were further analyzed to understand how the two different types of fatty acids were differentially accumulated in lipid classes and subclasses of the shrimp. The obtained results substantiate different biological roles of the two types of fatty acids in shrimp larvae.
Materials and Methods
Aurantiochytrium limacinum BCC52274 Preparation
AL strain BCC52274 (obtained from National Biobank of Thailand) was cultured at 28°C in a 10-L fermenter with aeration for 3 days (Unagul et al., 2017). The medium was composed of 1.5, 10, and 2% w/v sea salt (Sigma), Glucose (Difco), yeast extract (Difco), respectively. Cells were then centrifuged at 3,000 × g and washed twice with 0.9% normal saline. Cells were stored at 4°C in sealed vacuum plastic bags protected from light and used within 1 month. For feeding, AL cells were suspended in 30-ppt sea water through 100T polyester mesh before the suspension was poured into the larviculture.
Experimental Animals
Specific pathogen-free P. monodon larvae were obtained from Shrimp Genetic Improvement Center, Surat Thani, Thailand. Experiments were carried out in a hatchery at the same location. Larvae were maintained from nauplius to zoea 3, during which zoea were fed solely with Thalasiosira weissflogii (TW). The experiment was started when the larvae entered the mysis 1 (M1) stage. All animal experiment protocols were approved by National Center for Genetic Engineering and Biotechnology Institutional Animal Care and Use Committee (Approval code: BT-Animal 25/2560) and carried out in accordance with the relevant guidelines and regulations.
Feeding Experiments
Five hundred-liter dark plastic tanks were each stocked with 40,000 M1 larvae and 300 L of 30-ppt water with aeration. The stocking tanks were randomly assigned to the dietary treatments (N = 3 tanks for each treatment group). The experimental design of treatment groups is shown in Table 1. Mysis were fed 8 times/day. Anesthetized frozen instar-I Artemia was supplied every other meal, alternating with either AL or TW. The numbers of meals with either TW or AL varied among treatment groups while the number of Artemia meals was the same for all groups. Each experimental group was fed to saturation. The feeding amount of each group was separately adjusted according to the unconsumed amount, which was assessed by a hematocrit after the feed time for 3 h. Supplementation experiments were carried out from M1 to PL2 (4.5 days).
To examine the post-supplementation effect of AL, PL2 shrimp of all experimental groups were raised until PL12. To do so, 5,000 PL2 shrimp individuals from each tank were randomly taken and transferred to a dark plastic tank containing 150 L of 30-ppt sea water. During this period, all experimental groups were subjected to the same dietary regime and treatment. PL3-5 shrimp were fed with live Artemia for every other meal, alternating with a commercial flake feed (#2TNT, Advance Pharma, Thailand) while PL6-12 shrimp were fed only with PL300 flake (Inve). All PL shrimp were fed 8 meals/day. To mimic the actual common commercial practice, salinity of the culture water was gradually reduced. From PL3-6 and PL7-11, the culture water was exchanged by 20 and 30%, respectively, with 17-ppt water every day until the final salinity reached 17 ppt at PL12.
During the entire rearing experiment, the water quality of all tanks was controlled within the following ranges: pH at 7.5–8.5, temperature at 29–32°C, ammonia-N below 1.0 mg/L, nitrite-N below 2.0 mg/L, nitrate-N less than 3.0 mg/L and alkalinity between 140 and 220 mg CaCO3/L.
Assessment of Growth Performance
At PL2 and PL12, growth performance assessment and sample collection were carried out, as previously described in Visudtiphole et al. (2018). Briefly, the animals were fasted by withholding a meal ahead of the process. Average body weight (ABW) was determined from the PL2 and PL12 individuals with a combined weight of 120 and 500 mg, respectively (2 count replicates/tank). Survival rate was calculated from the final biomass weight and average body weight data. Average body length was determined from 50 individuals for each tank, measuring from both ends of rostrum to telson. Percent coefficient of body length variation was calculated as standard deviation/mean × 100. Shrimp samples for the biochemical analyses were collected, immediately snap-frozen in liquid nitrogen and stored at -80°C until used.
Proximate Analysis
Crude protein, lipids, fiber and ash contents in the feed (2-g dried sample) were analyzed through the service provided by Animal Husbandry Department, Faculty of Veterinary Science, Chulalongkorn University (Thailand), using the standard methods of AOAC (Horwitz and Latimer, 2005). Total calories were determined through the service provided by Scientific and Technological Equipment Research Center, Chulalongkorn University (Thailand), using a Bomb Calorimeter AC-500 (Leco, United States).
Fatty Acid Profile Analysis by Gas Chromatography-Flame Ionization Detector
Fatty acids from freeze-dried samples (from 0.20 to 0.27 g fresh samples) were directly extracted and transesterified in a single step by heating at 90°C with 4% sulfuric acid in methanol for 1 h (Lepage and Roy, 1986). Heptadecanoic or nonadecanoic acid (17:0 and 19:0, respectively) was used as the internal standard (Sigma-Aldrich). The esterified samples were applied to GC (GC17A, Shimadzu), equipped with a 30 m × 0.25 mm OmegawaxTM 250 fused silica capillary column (Supelco), an automatic sampler and flame ionization detector (FID). The injector and detector temperatures were maintained at 250 and 260°C, respectively. Helium was used as a carrier gas at a linear velocity of 30 cm/s. The initial column temperature of 200°C was held for 10 min and then increased at the rate of 20°C/min to 230°C, which was then maintained for 17 min. Peak analysis (identification and quantification) was carried out based on the retention times relative to fatty acid methyl ester standards (Supelco 18919-1 AMP) (all from Sigma-Aldrich).
Lipidomic Analysis
Lipid Sample Preparation
Crude lipid was extracted from freeze-dried samples (from 13 ± 2 g wet weight), using chloroform/methanol (Folch et al., 1957). In brief, samples were homogenized in chloroform: methanol (2:1). After filtration to remove the debris, samples were washed with 0.15% NaCl and centrifuged to collect the lower lipid phase. The NaCl-wash step was repeated twice before the organic solvent was finally removed by evaporation with N2. The dried lipid was then dissolved in chloroform, diluted with isopropanol: methanol (1:1) and filtered through PVDF membrane. After that, an internal standard (tributyrin) was added into the samples.
Lipidomics Acquisition Using Liquid Chromatography-High Resolution Mass Spectrometry
Liquid chromatography was performed by using an Acquity UHPLC HSST3 C18 column (Water, United States) with 100 × 2.1 mm inner diameter and 1.8 μm particle size. The column temperature was set at 45°C. Mobile phases were composed of solvent A (acetonitrile: water (60:40) in 0.1% formic acid with 10 mM ammonium formate) and solvent B (isopropanol: acetonitrile: water (88:10:2) in 0.1% formic acid with 10 mM ammonium formate). Elution was performed in 6 isocratic steps with graded concentration of solvent B: 40–55% at 0–7 min, 55–65% at 7–10 min, 65–70% at 10–19 min, 70–88% at 19–21 min, 88–95% at 21–23 min and 95–100% at 23–25 min and held for 5 min. The flow rate was 0.3 μL/min. Between each sample run, the system was re-equilibrated to the initial condition for 0.1 min (40% solvent B) and held for 4.9 min before starting the next run. LC-HRMS runs of pure lipid standards, triacylglycerol (TG 18:1/18:1/18:1), diacylglycerol (DG 18:1/18:1), lysophosphatidylcholine (LPC 18:0), phosphatidylcholine (PC 16:0,18:0,18:1,18:2), phosphatidylethnolamine (PE), phosphatidylglycerol (PG 18:0,18:1), phosphatidylinositol (PI), phosphatidylserine (PS) (Avanti Polar Lipids, United States), cholesteryl ester (with 18:1), and cholesterol (Sigma Aldrich, United States) were performed for validation of the lipid-class identification.
Electrospray ionization (ESI) was performed for mass spectrometry (MS), using both positive and negative ionization modes. The ion source settings were 3,000 V, 320°C for ion transferring and 300°C for vaporization. High-resolution MS data were acquired in two separate methods, namely, (i) full scan data dependent-tandem MS (full scan dd-MS2) method for untargeted lipids analysis through LipidSearchTM 4.0 processing software (Thermo Fisher Scientific, United States) and (ii) parallel reaction monitoring (PRM) method for targeted lipids unavailable in LipidSearchTM. Full scan dd-MS2 was used to collect MS and MS2 spectra over the mass range of 100–1,200 Da using an Orbitrap detector with a resolution of 120,000. The 10 most intense precursor ions of the spectra throughout the chromatographic run were fragmented using higher-energy collisional dissociation (HCD) at 25 ± 5% of collision energy with a resolution of 30,000. The PRM method was used to monitor cholesterol (369.3516 m/z) and tributyrin (320.2067 m/z) with 30 and 15% HCD, respectively, using an Orbitrap detector with a resolution of 60,000.
Data Processing and Analysis
The acquired MS data were imported to the LipidSearchTM software and subjected to the following steps: (i) peak detection from high resolution mass spectral intensity of each sample, (ii) identification of lipid class by matching precursor and product ions to the database to define their structures, and (iii) alignment of the identified peaks across the samples for quantification. Analysis of the processed data generated lipid features (identified lipid compound with a specific retention time) and their abundances (areas under the corresponding peaks). After subtraction of the blank sample value, test sample intensity values were normalized to that of the internal standard, tributyrin. The normalized abundance data were then used for comparative analysis across the sample groups.
For analysis of 16:0, 22:5 n-3, and 22:6 n-3 fatty acids incorporated in lipid classes and subclasses, percent of sites esterified with the fatty acid of interest on the lipid molecule were determined, using the following equation:
in which A = normalized abundance.
n = the number of sites esterified with the fatty acid of interest.
N = the number of sites esterified with all fatty acids on the lipid molecule backbone (3 for TG; 2 for DG and phospholipids; 1 for lysophospholipids).
Statistical Analyses
Statistical analyses of the data were performed separately for each PL stage, using one-way analysis of variance (ANOVA) by Duncan’s multiple range test (SPSS 11.5.0). Differences were considered to be significant when P < 0.05.
Results
Analysis of the Experimental Feed
The nutritional contents of the TW and AL feeds were determined before comparing the effects of different feeds on the larvae. In line with the crude lipid content, the total fatty acid (TFA) content of AL was also much higher than that of TW (Supplementary Table 1 and Table 2). The predominant fatty acids in AL were palmitic acid (16:0) and DHA, which were present in much lower amounts in TW. In addition to DHA, DPA was also present in AL but absent in TW. In contrast, the amount of EPA in TW was slightly higher than that in AL. When comparing fatty acid types, AL contained more saturated fatty acids (SFAs), n-3 polyunsaturated fatty acids (PUFAs) and LC-PUFAs but had less monounsaturated fatty acids (MUFAs), and n-6 PUFAs than TW.
The contents of total acylglycerols, diacylglycerols (DG), and triacylglycerols (TG) in AL were higher than those of TW (Figure 1). However, the phospholipid and lysophospholipid contents in AL were extremely low, compared with those in TW (Figures 2, 3). This indicates that most fatty acids in AL were present as acylglycerols. Only two types of phospholipids, i.e., phosphatidylcholine (PC) and phosphatidylethanolamine (PE), were detected in AL while PC, PE, phosphatidylglycerol (PG), and phosphatidylserine (PS) were all found in TW (Figure 2). Cholesterol was absent in both AL and TW whereas only a small amount of cholesteryl esters was present in AL (Figure 4).
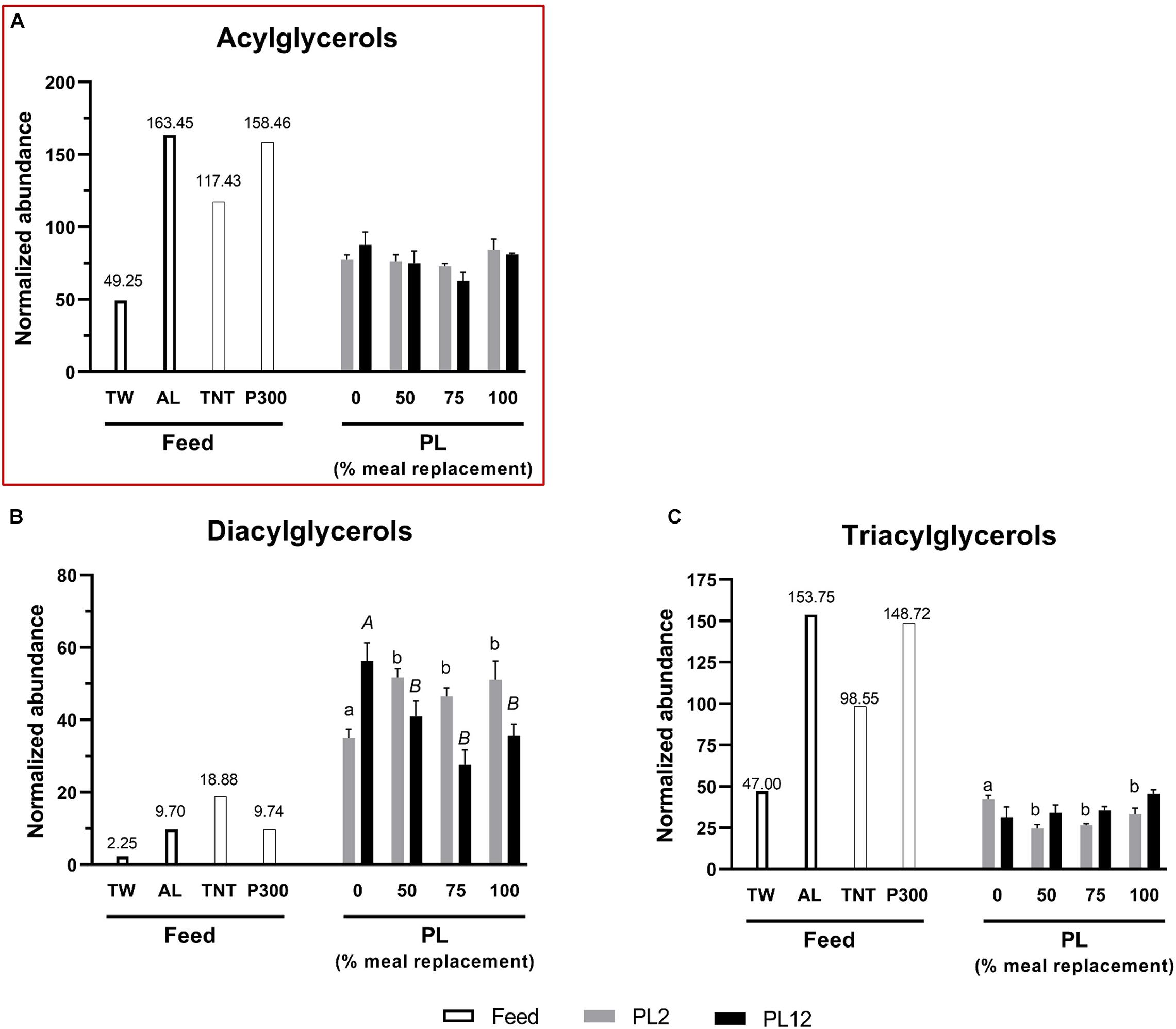
Figure 1. Analysis of acylglycerols in feed and PL shrimp by LC-HRMS. Percent of meal replacement was percent of the number of meals for which TW was replaced by AL. Normalized abundance was derived from the peak intensity value normalized to that of tributyrin, the internal standard. Error bars represent standard errors (N = 3). Statistical analyses of the PL2 and PL12 data sets were performed separately, using ANOVA. Significant differences among subsets of treatment groups were determined by Duncan’s multiple range test (P < 0.05); subsets with means not significantly different from one another are identified with the same letter above the bar. Small- and big-capital letters are individually for the PL2 and PL12 data sets, respectively. Bars with no specified letters mean no significant differences were identified among the groups (P > 0.05).
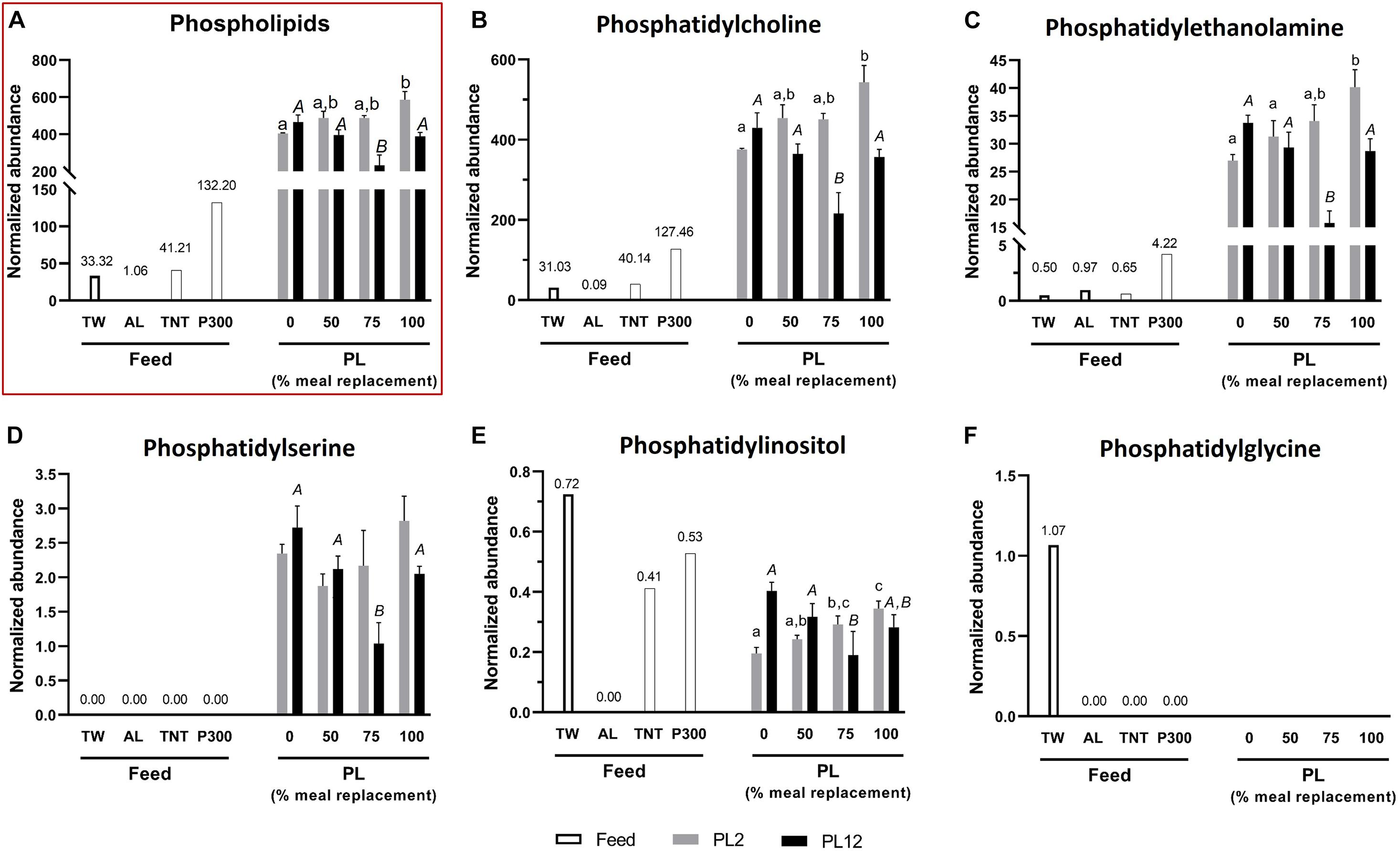
Figure 2. Analysis of phospholipids in feed and PL shrimp by LC-HRMS. Percent of meal replacement was percent of the number of meals for which TW was replaced by AL. Normalized abundance was derived from the peak intensity value normalized to that of tributyrin, the internal standard. Error bars represent standard errors (N = 3). Statistical analyses of the PL2 and PL12 data sets were performed separately, using ANOVA. Significant differences among subsets of treatment groups were determined by Duncan’s multiple range test (P < 0.05); subsets with means not significantly different from one another are identified with the same letter above the bar. Small- and big-capital letters are individually for the PL2 and PL12 data sets, respectively. Bars with no specified letters mean no significant differences were identified among the groups (P > 0.05).
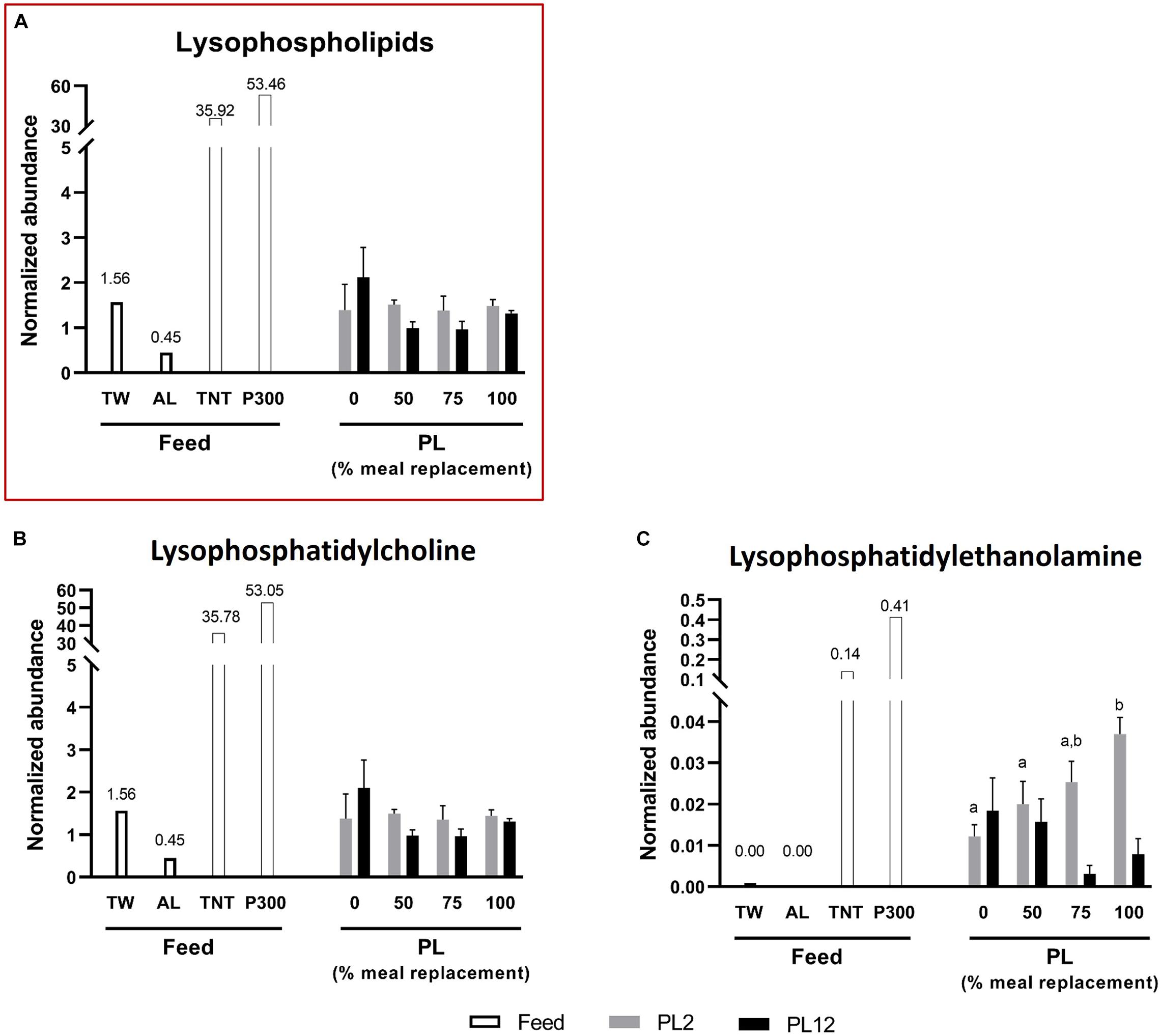
Figure 3. Analysis of lysophospholipids in feed and PL shrimp by LC-HRMS. Percent of meal replacement was percent of the number of meals for which TW was replaced by AL. Normalized abundance was derived from the peak intensity value normalized to that of tributyrin, the internal standard. Error bars represent standard errors (N = 3). Statistical analyses of the PL2 and PL12 data sets were performed separately, using ANOVA. Significant differences among subsets of treatment groups were determined by Duncan’s multiple range test (P < 0.05); subsets with means not significantly different from one another are identified with the same letter above the bar. Small- and big-capital letters are individually for the PL2 and PL12 data sets, respectively. Bars with no specified letters mean no significant differences were identified among the groups (P > 0.05).
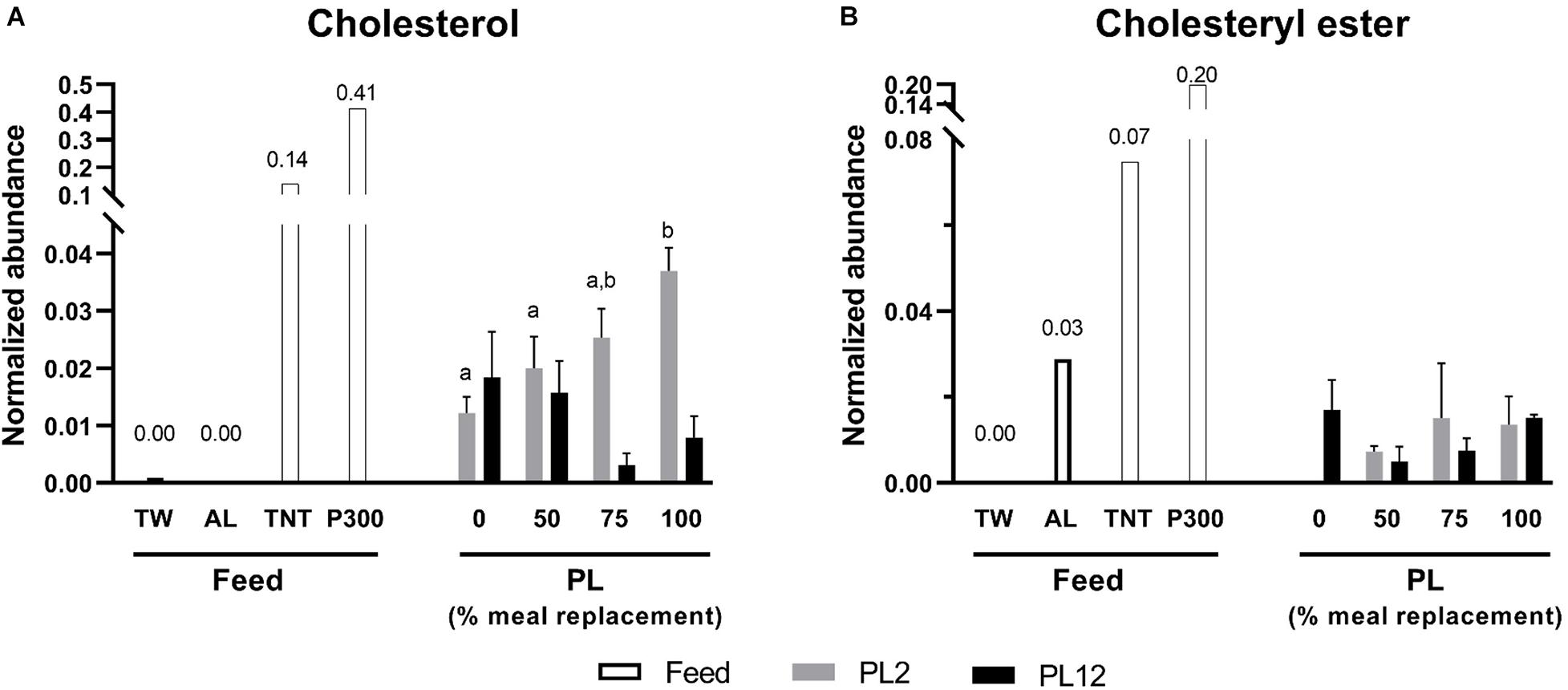
Figure 4. Analysis of cholesterol and cholesteryl esters in feed and PL shrimp by LC-HRMS. Percent of meal replacement was percent of the number of meals for which TW was replaced by AL. Normalized abundance was derived from the peak intensity value normalized to that of tributyrin, the internal standard. Error bars represent standard errors (N = 3). Statistical analyses of the PL2 and PL12 data sets were performed separately, using ANOVA. Significant differences among subsets of treatment groups were determined by Duncan’s multiple range test (P < 0.05); subsets with means not significantly different from one another are identified with the same letter above the bar. Small- and big-capital letters are individually for the PL2 and PL12 data sets, respectively. Bars with no specified letters mean no significant differences were identified among the groups (P > 0.05).
Larval Growth Performance
Replacement of TW with AL did not affect growth of the larvae from mysis to PL2. All growth parameters of all treatment groups among PL2 shrimp were not significantly different (Table 3). However, a significant effect of AL on growth was observed during the post-supplementation period from PL2 to PL12. Average body weight and biomass of PL12 shrimp significantly increased with increasing amounts of AL supplied earlier to the larvae.
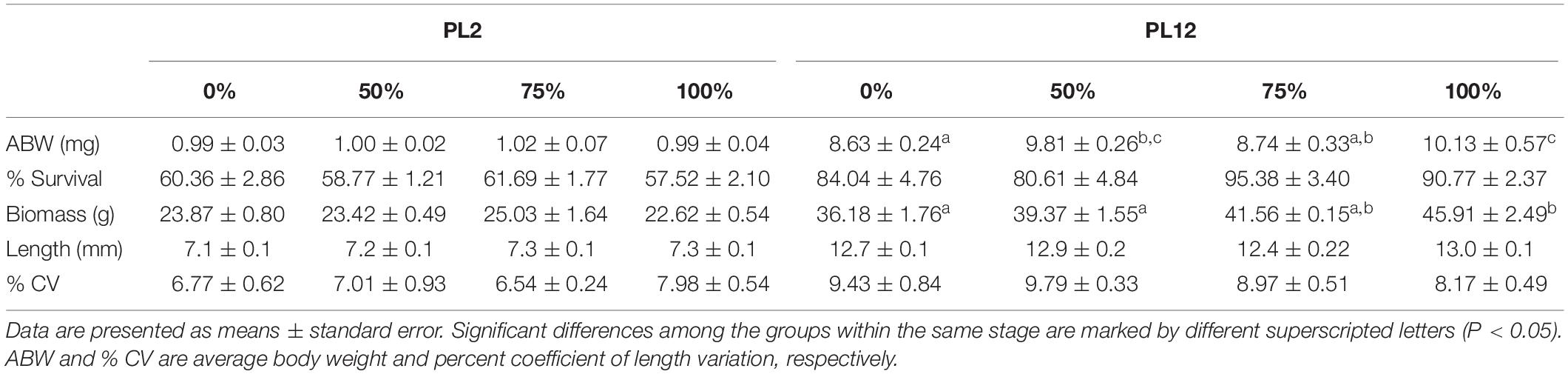
Table 3. Growth parameters of PL2 and PL12 shrimp fed with graded percent of meals supplied with AL in replacement of TW.
Analysis of Post-larval Shrimp’s Fatty Acid Compositions
Fatty acid profiles of PL2 shrimp differed according to the diet supplied to the larvae during M1 to PL2 (Table 4). Replacement of TW with AL led to significantly increased total amounts of SFAs, PUFAs, n-3, and LC-PUFAs in PL2 shrimp, predominantly resulting from the increased levels of 16:0, DPA, and DHA, respectively. Nevertheless, levels of the three less complex n-3 PUFAs i.e., linolenic acid (18:3), eicosastrienoic acid (20:3), and EPA were significantly lower when AL was supplied. The total n-6 PUFA amount in PL2 shrimp was significantly decreased in treatments with AL, which can be attributed to the low content of linoleic acid (18:2 n-6) in AL. Ratios of n-3/n-6 and DHA/EPA in PL2 shrimp, as a result, were higher, reflecting the higher proportion of AL:TW in the diet. Finally, the TFA content in PL2 shrimp significantly increased with the increasing AL amounts, most likely due to the high TFA content in AL.
When AL was no longer supplemented and all the experimental groups were fed with the same diet during later stages of development from PL2 to PL12, levels of most fatty acids, including 16:0 and DHA, in PL12 shrimp among all treatment groups were not significantly different (Table 4). Both 16:0 and DHA contents of all the groups fed at earlier stages with AL decreased to the same level in PL12 shrimp after AL was no longer supplied. The contents of some fatty acids such as 20:3 n-3 and DPA and the n-3/n-6 ratio were still significantly different among the treatment groups of PL12 shrimp; however, the degree of difference was much less than that in PL2 shrimp.
Analysis of Lipid Classes and Subclasses in Post-larval Shrimp
While TG constitutes the main subclass of acylglycerols in both TW and AL feeds, DG and TG in the shrimp were present at the comparable levels (Figures 1B,C). The DG content in PL2 shrimp was significantly increased by the AL diet replacement (Figure 1B). This was in line with the higher DG content in AL than that in TW. Nevertheless, the TG content in PL2 shrimp was significantly decreased by the replacement although the level of TG in AL feed was higher than that in TW (Figure 1C). The opposite trends of changes in DG and TG contents resulted in insignificant differences of the total acylglycerols levels in PL2 shrimp among all groups (Figure 1A).
The content of total phospholipids in PL2 shrimp of the 100% replacement group was significantly higher than those of all the other groups (Figure 2A). The most and second most abundant phospholipids in the PL shrimp were PC and PE (Figures 2B,C), respectively, both of which are the major components of cell membranes in juvenile shrimp and also other species (Ronnestad et al., 1995; Coutteau et al., 1997; Caers et al., 2000a; Ju et al., 2011; Rey et al., 2015; Huang et al., 2019). PS and PI phospholipids were also detected in the PL shrimp (Figures 2D,E); however, they were present in much lower amounts than PC and PE. The PC, PE, and PI contents in PL2 shrimp were significantly increased with the increasing amounts of AL (Figures 2B–E). In contrast, the PS content in PL2 shrimp was not significantly different across all treatment groups (Figure 2D).
Lysophosphatidylcholine (LPC) constitutes the major subclass of lysophospholipids in PL2 and PL12 shrimp. The contents of LPC and total lysophospholipids were not significantly different among the treatment groups (Figures 3A,B). In contrast, the content of lysophosphatidylethanolamine (LPE) in PL2 shrimp increased as more AL was supplied to the larvae (Figure 3C).
The cholesterol content in PL2 shrimp was significantly higher as the larvae were fed with more AL (Figure 4A). In contrast, levels of cholesteryl esters in PL2 shrimp were not significantly different; however, no cholesteryl esters were found in the PL2 shrimp fed with no AL (Figure 4B).
After the post AL-supplementation period, the contents of most lipid classes and subclasses in PL12 shrimp were not significantly different across the treatment groups. Nevertheless, PL12 shrimp fed earlier with AL during their mysis development contained lower DG than those fed with only TW (Figure 1B).
Analysis of Palmitic Acid (16:0), Docosahexaenoic Acid (22:6 n-3), and Docosapentaenoic Acid (22:5 n-3) Incorporated in Lipid Classes and Subclasses
The fatty acid profile result analyzed by GC in Table 4 showed that increased ingestion of AL resulted in significant accumulation of three dietary fatty acids, 16:0, 22:5 n-3, and 22:6 n-3, in PL2 shrimp. Therefore, the lipidomic data were further analyzed to reveal the accumulation of these fatty acids distributed among lipid classes and subclasses in PL shrimp.
16:0 Fatty Acid
The percent of 16:0-esterified sites on acylglycerol backbones in PL2 shrimp of the AL-supplemented groups were significantly greater than that of the 0% meal replacement control group (Figure 5A). However, among the AL-supplemented groups, the percent of 16:0-esterified sites were not different or did not demonstrate further increases as the percent of the diet replacement was increased above 50%. A similar trend was also observed for the TG subclass whereas the percent of 16:0-esterified sites on DG were not significantly different among any of the treatment groups.
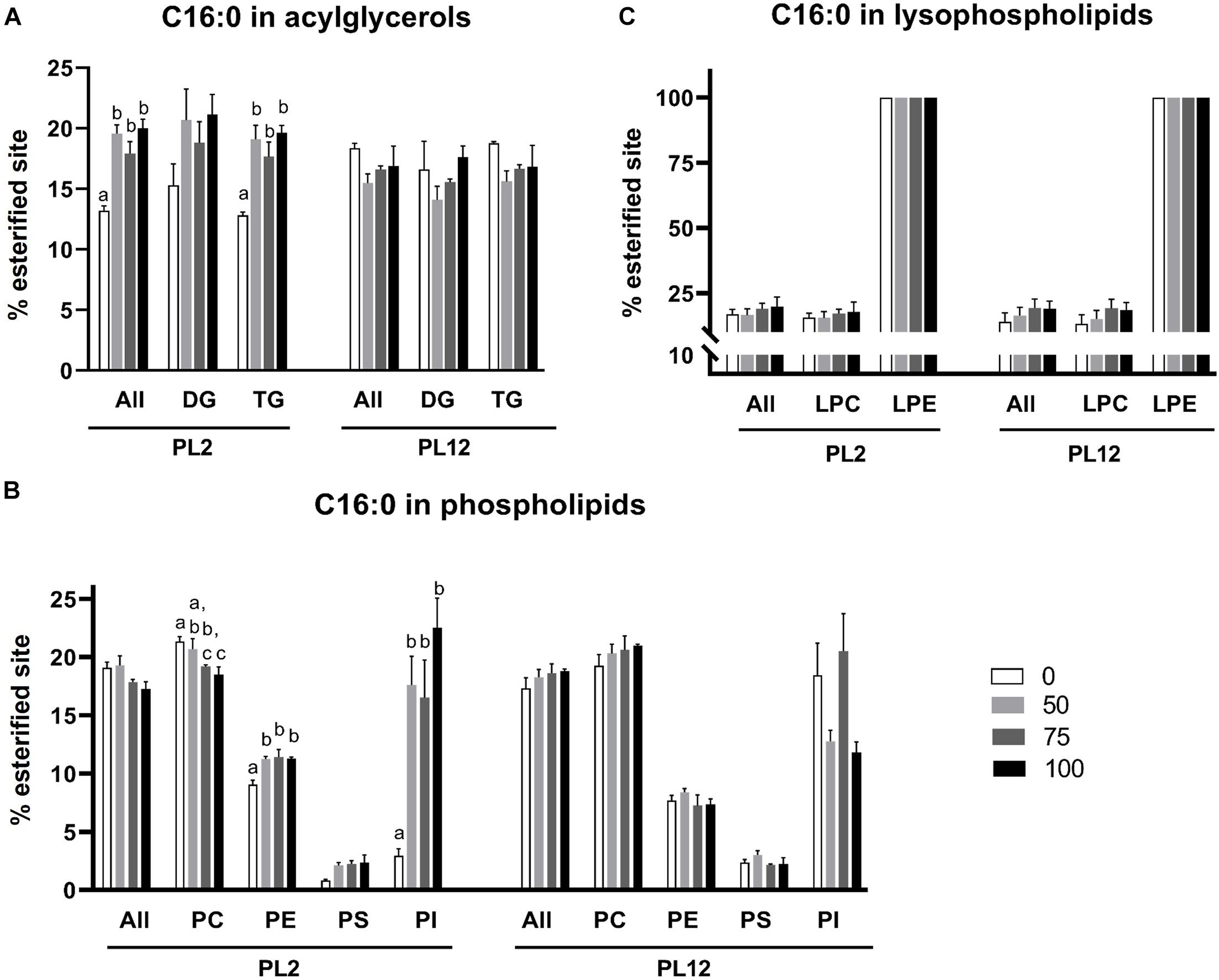
Figure 5. Analysis of the 16:0 fatty acid distribution in lipid classes and subclasses by LC-HRMS. Percent of meal replacement was the percent of meals for which TW was replaced by AL. Percent esterified site is the percent of sites esterified with 16:0 compared with all the sites esterified with any types of fatty acids on the backbone. Error bars represent standard errors (N = 3). Statistical analyses of the PL2 and PL12 data sets were performed separately, using ANOVA. Significant differences among subsets of treatment groups were determined by Duncan’s multiple range test (P < 0.05); subsets with means not significantly different from one another are identified with the same letter above the bar. Bars with no specified letters mean no significant differences were identified among the groups (P > 0.05).
Unlike that of acylglycerols, the percent of 16:0-esterified sites on phospholipids in PL2 shrimp were not significantly different among all treatment groups (Figure 5B). At the subclass level, the percent of 16:0-esterified sites on the major phospholipid, PC, were reduced in groups with higher 16:0 content in the diet. On the other hand, the percent of 16:0-esterified sites on PE and PI increased while the percent of those on PS were unchanged. However, because the PE and PI contents in PL shrimp were far lower than that of PC (Figure 2), the increase of 16:0 on PE and PI had no effect on the 16:0 percent of total phospholipids.
For lysophospholipids, 16:0 fatty acid was detected to esterify with LPC and LPE (Figure 5C). The percent of LPC and LPE esterified with 16:0 fatty acid were not significantly different across the treatment groups, and all LPE molecules were esterified with 16:0.
22:6 n-3 and 22:5 n-3 Fatty Acids
22:6 n-3 and 22:5 n-3 LC-PUFAs accumulated in lipid classes and subclasses in patterns that were different from those of the 16:0 SFA. The percent of DHA- or DPA esterified sites on phospholipids and their subclasses in PL2 shrimp of the AL-supplemented groups were significantly greater than that of the control group but did not further increase as the percent of the replacement was increased above 50% (Figures 6B, 7B). However, with an exception, more DHA incorporated in PC when the replacement was increased to 100%. DHA but not DPA was detected accumulating on PS and LPC (Figure 6C). Unlike that of 16:0, the percent of DHA-esterified sites on LPC were significantly increased by AL feeding. LPE, which was shown above to be specific to 16:0, was not esterified by DHA and DPA.
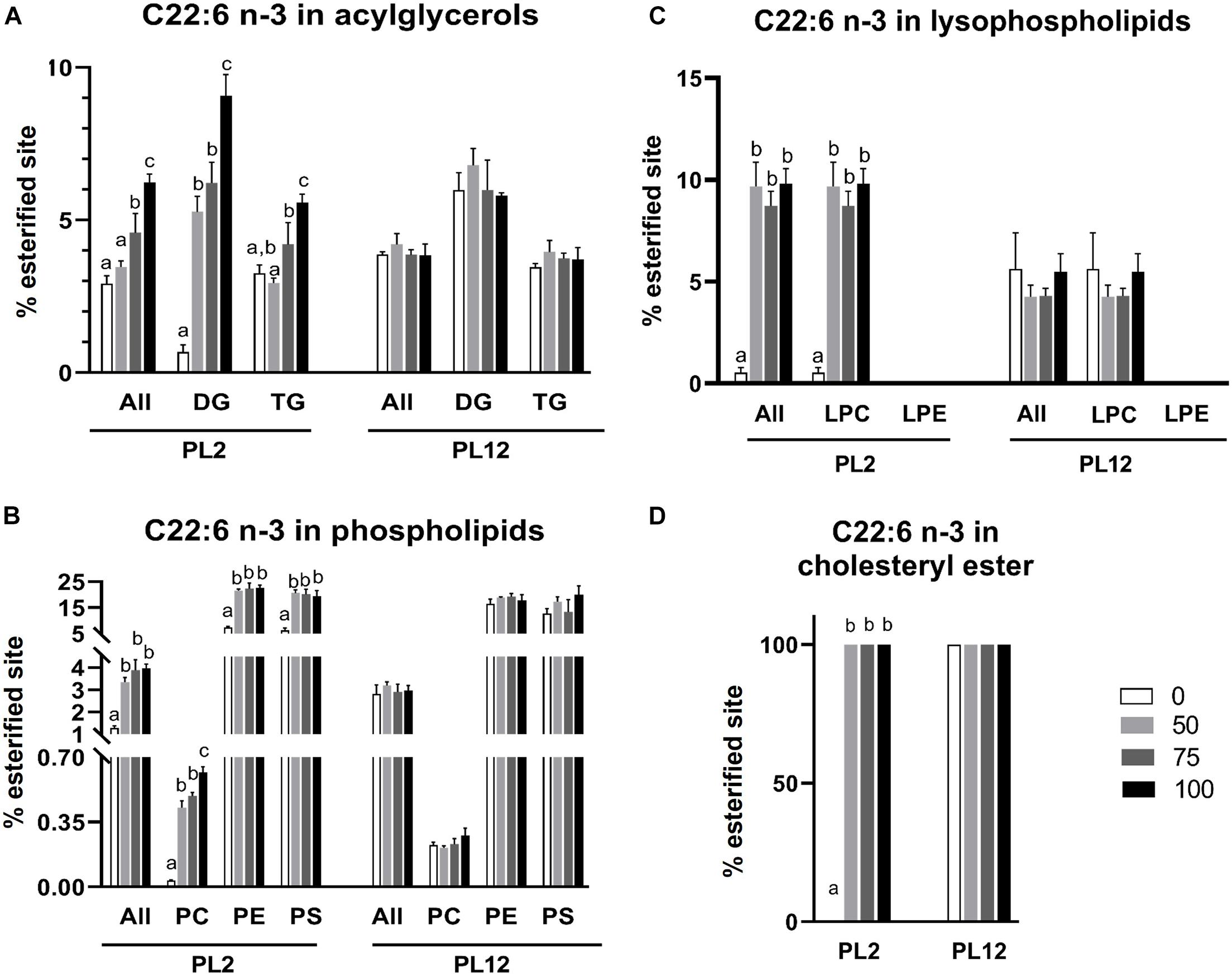
Figure 6. Analysis of the 22:6 n-3 fatty acid distribution in lipid classes and subclasses by LC-HRMS. Percent of meal replacement was the percent of meals for which TW was replaced by AL. Percent esterified site is the percent of sites esterified with 16:0 compared with all the sites esterified with any types of fatty acids on the backbone. Error bars represent standard errors (N = 3). Statistical analyses of the PL2 and PL12 data sets were performed separately, using ANOVA. Significant differences among subsets of treatment groups were determined by Duncan’s multiple range test (P < 0.05); subsets with means not significantly different from one another are identified with the same letter above the bar. Bars with no specified letters mean no significant differences were identified among the groups (P > 0.05).
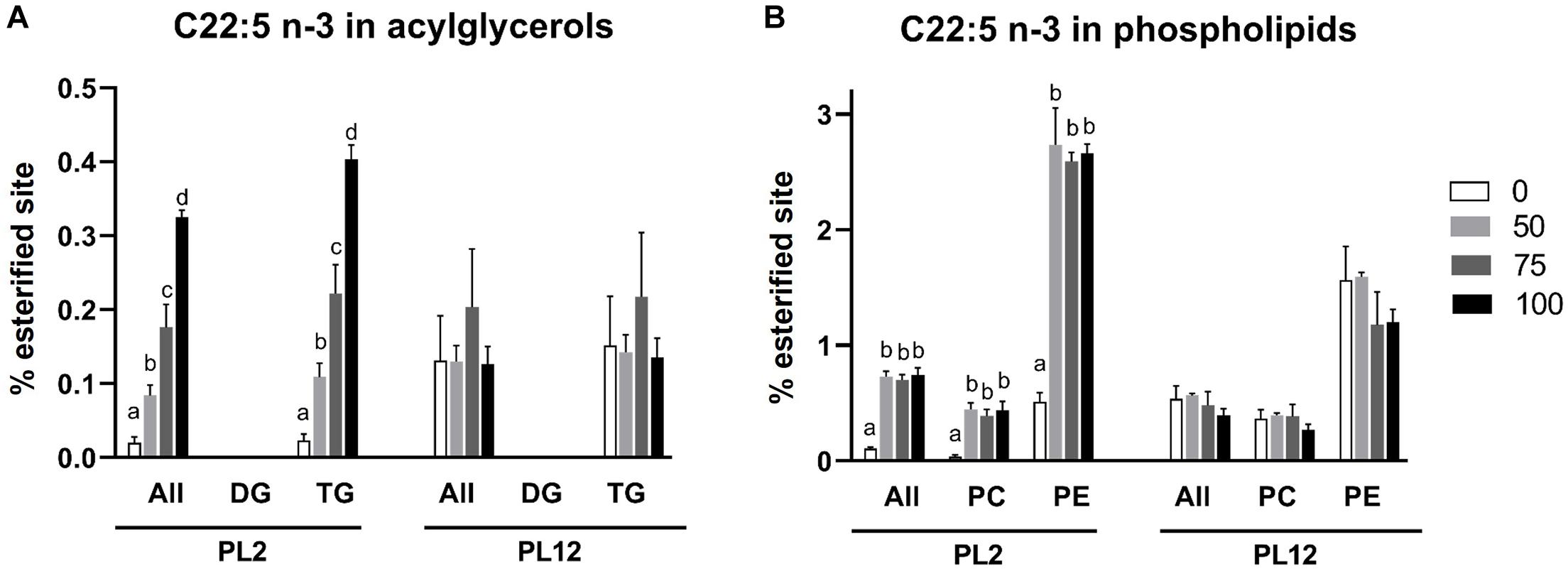
Figure 7. Analysis of the 22:5 n-3 fatty acid distribution in lipid classes and subclasses by LC-HRMS. Percent of meal replacement was the percent of meals for which TW was replaced by AL. Percent esterified site is the percent of sites esterified with 16:0 compared with all the sites esterified with any types of fatty acids on the backbone. Error bars represent standard errors (N = 3). Statistical analyses of the PL2 and PL12 data sets were performed separately, using ANOVA. Significant differences among subsets of treatment groups were determined by Duncan’s multiple range test (P < 0.05); subsets with means not significantly different from one another are identified with the same letter above the bar. Bars with no specified letters mean no significant differences were identified among the groups (P > 0.05).
In contrast to those on phospholipids, the patterns of the DPA and DHA accumulation on acylglycerols were different. The percent of DPA- or DHA-esterified sites on total acylglycerols and TG in PL2 shrimp were significantly different in treatments with higher amounts of AL supplied to the larvae (Figures 6A, 7A). DHA was also found to accumulate on DG with a similar pattern while DPA was not detected on the DG molecule. Furthermore, all cholesteryl ester molecules in both PL2 and PL12 shrimp were esterified specifically only with DHA (Figure 6D).
Similar to the aforementioned lipid class and subclass results, after the feed was changed to the same diet for all test groups during PL2–PL12, the percent of sites esterified with 16:0, 22:5 n-3, or 22:6 n-3 fatty acid in all lipid classes and subclasses were not significantly different among any of the sample groups (Figures 5–7).
Discussion
Previously, dietary supplementation of AL through Artemia enrichment had been studied to promote growth performance of P. vannamei post-larvae from PL1 to PL19 (Visudtiphole et al., 2018). In the present study, AL supplementation was conducted in P. monodon larvae from M1 to PL2 by direct suspension of the AL cells into the larviculture. This approach is more efficient than the enrichment technique because all fatty acids in AL are taken in directly by the shrimp and not retroconverted by the enriched Artemia (Navarro et al., 1999; Han et al., 2001; Visudtiphole et al., 2018). The supplementation resulted in accumulation of the key fatty acids present in AL i.e., 16:0, DPA, and DHA, in PL2 shrimp. No effect on the growth of larvae was observed during the supplementation period; however, the effect on growth was exhibited later in the post-supplementation period from PL2 to PL12. Average body weight and biomass were increased in PL12 shrimp that had been fed earlier with higher amounts of AL. Dietary supplementation using other high-n-3 LC-PUFA substances, such as fish oil, was also shown to enhance growth performance of shrimp at the PL stage (Leger et al., 1985; Immanuel et al., 2001). Taken together with the previous results in P. vannamei PL and from the other studies, dietary n-3 LC-PUFAs may be more important for growth of shrimp during PL than the larval stage. This result could be ascribed for two reasons. First, the supplementation period during the mysis stage might not be long enough for difference in growth to be exhibited. Second, development of penaeid mysis is secondary lecithotrophy, in which the larvae partially rely on the nutrients from maternal reserves in eggs and also from the food (Mourente et al., 1995; Palacios et al., 2001). The supplemented LC-PUFAs were thus accumulated during the mysis stage and subsequently used for growth during the later PL stage, when AL was no longer supplied. This is also supported by the indifferent levels of most LC-PUFAs in PL12 shrimp.
The fatty acid profile of the aquatic animal normally reflects that of the ingested diet (Glencross, 2009; Kumar et al., 2018a). Likewise, fatty acid profiles of the PL shrimp in this study reflected those of the supplied diet, both at PL2 in which marked differences in fatty acid profiles were observed among groups with different feeding regimens, and later at PL12 when fatty acid profiles became more similar after all groups were later fed with the same diet. In contrast to the fatty acid profile, lipid class and subclass profiles of the PL shrimp and feed showed weaker correspondence. This could be accounted for digestion of macro-lipid molecules in the digestive tract and subsequent modification of lipid molecules in the animal. Macro-lipid molecules, especially triacylglycerols, have to be digested to smaller-sized molecules before being absorbed through the intestine and subsequently repacked and modified to various lipid class products (Senior, 1964; Iqbal and Hussain, 2009).
Interestingly, increased ingestion of TG present in AL was associated with a lower TG level in PL2 shrimp. This result is consistent with a study on dietary LC-PUFA supplements for juvenile grass carp, in which reduced TG in the supplemented animals was explained by down-regulated expression of diacylglycerol O-acyltransferase, encoding the key enzyme that synthesizes TG from DG (Tian et al., 2017). Therefore, the downregulation of conversion from DG to TG might be the reason for the decrease of TG and the accumulation of DG in PL2 shrimp supplemented with the high DHA-containing AL in this study. It is known that DG acts as a second messenger for cell signaling by increasing the affinity of protein kinase C to bind with substrates; DG is thus involved in the signal transduction of several pathways related to immunity, cell activation, regulation, and function (Berg et al., 2002b; Singh and Kambayashi, 2016).
Analysis of the fatty acid distribution in lipid classes and subclasses showed differential accumulation of two distinct types of fatty acids present in AL i.e., SFA (16:0) and n-3 LC-PUFAs (DPA and DHA), among lipid classes of the shrimp. Increased 16:0 levels in the diet were associated with a greater percent of sites esterified with the fatty acid on acylglycerols but not phospholipids and lysophospholipids, consistent with the role of 16:0 fatty acid as a reserve for energy and substrate for the synthesis of other fatty acids (Caers et al., 2000b; Rey et al., 2018). In contrast, both of the LC-PUFAs accumulated on phospholipids and acylglycerols. The percent of DHA- and DPA-esterified sites on phospholipids in PL2 shrimp of the AL supplemented groups were higher than the percent of those of the control group. However, they did not increase further with more AL in the diet (Figures 6B, 7B) whilst the percent of those on acylglycerols increased along with the amount of AL meal replacement (Figures 6A, 7A). This suggests that DHA and DPA were prioritized to be incorporated in phospholipids, which are the main component of cell membrane (Tian et al., 2017; Rey et al., 2018). Nonetheless, if phospholipids with excess LC-PUFA esterified sites are present, they cannot be packed easily into the membrane bilayer structure and may disrupt the membrane’s integrity owing to too much fluidity (Chapelle et al., 1982; Rajamoorthi et al., 2005; Bradbury, 2011; Parisi et al., 2019). The integration of simpler fatty acids into the membrane structure is also required to maintain the membrane’s stability (Huang et al., 2019). From our data, it can be inferred that dietary DHA and DPA were first preferentially deposited in the membrane and the excess was then stored with acylglycerols since the percent of sites esterified with these fatty acids continuously increased with the higher amounts of supplied AL. This result was in agreement with those in Crassostea gigas spat and juvenile Mytilus edulis fed with high LC-PUFA diets (Caers et al., 2000b; Laudicella et al., 2020). In contrast to that of the LC-PUFAs, deposition of 16:0 fatty acid in acylglycerols did not increase further with the higher amounts of supplied AL (Figure 5A). This result additionally suggests that LC-PUFAs were prioritized to be reserved over SFA when both types of fatty acids were present in high amounts in the diet. The preferential accumulation of LC-PUFAs over other types of fatty acids was also observed in other marine larvae (Caers et al., 1999, 2000b). The importance of LC-PUFAs is also corroborated by their selective retention in animals that were starved or fed with DHA-deficient diet (Coutteau et al., 1996; Knauer and Southgate, 1997).
The 16:0 fatty acid was markedly more abundant on PC than DHA or DPA (Figures 5B, 6B, 7B). In contrast, DHA was the most common modification of PE and PS. Moreover, unlike the 16:0 fatty acid, both n-3 LC-PUFAs were not detected to associate with PI, which is a minor component on the cytosolic side of cell membrane. Instead, the percent of 16:0-esterified sites on PI were significantly greater in PL2 shrimp fed with AL (Figure 5B). These results are in line with those in other species. Namely, DHA is more abundant on PE and PS than on PC and PI, and DHA supplementation resulted in the highest DHA accumulation on PE (Gillis and Ballantyne, 1999; Axelsen and Murphy, 2010; Rey et al., 2015; Bascoul-Colombo et al., 2016; Huang et al., 2019). In fact, PE is regarded as the most highly unsaturated phospholipid in marine animals (Ronnestad et al., 1995; Huang et al., 2019). Furthermore, PI is more specific to LC-PUFAs with a Δ5 double bond, like ARA and EPA, than those with a Δ3 double bond, which are DHA and DPA (Bascoul-Colombo et al., 2016). This also agrees with our result, in which the only LC-PUFAs detected to associate with PI were ARA and EPA (see Supplementary Table 2). Additionally, the high content of 16:0 fatty acid in PC coincides with a role of PC as a secondary energy source when TG is nearly depleted (Sasaki et al., 1986).
Cholesteryl ester was detected only in PL2 shrimp supplemented with AL and associated only with DHA (Figure 6D). Esterification of cholesterol with long-chain fatty acids makes cholesterol become more hydrophobic, which can be packed more easily inside the core of lipoproteins for the transportation in plasma (Maranhão and Freitas, 2014). AL supplementation in PL2 shrimp resulted in higher levels of cholesterol and the presence of cholesteryl ester (Figure 4). The presence of the latter indicates cholesterol transport between organs in shrimp supplemented with AL since cholesterol is transported in plasma as cholesteryl esters. Cholesterol is the precursor of steroid hormones that activate molting and growth such as ecdysteroids, which are synthesized specifically in the Y-organ of shrimp (Huberman, 2000; Kumar et al., 2018b). This suggests an additional role of DHA as a chaperone for cholesterol transportation in plasma to the target organ for the steroid hormone synthesis.
Conclusion
In conclusion, supplementation of AL as a source of dietary n-3 LC-PUFAs was demonstrated for the first time in shrimp larvae during the mysis developmental stage. The growth results from this and other previous studies suggests that dietary n-3 LC-PUFAs are more important and beneficial to PL shrimp than to the larvae at earlier stages. Analysis of differential accumulation of different fatty acid types provides an additional evidence on the importance and biological roles of LC-PUFAs in the larval diet.
Data Availability Statement
The original contributions presented in the study are included in the article/Supplementary Material, further inquiries can be directed to the corresponding author/s.
Author Contributions
VV: experimental design and investigation, data analysis, and manuscript preparation. JK, PC, JS, and SL: shrimp rearing and feeding experiments. SP, AK, TR, KS, LK, and NR: laboratorial experiments. PU: experimental design and investigation. UU: experimental design and investigation, manuscript preparation. All authors contributed to the article and approved the submitted version.
Funding
This research was supported by the National Science and Technology Development Agency (NSTDA), Thailand (grant no. P-17-51535). The publication fee was supported by the Functional ingredients and food innovation program, NSTDA (grant no. P-19-51807).
Conflict of Interest
The authors declare that the research was conducted in the absence of any commercial or financial relationships that could be construed as a potential conflict of interest.
Publisher’s Note
All claims expressed in this article are solely those of the authors and do not necessarily represent those of their affiliated organizations, or those of the publisher, the editors and the reviewers. Any product that may be evaluated in this article, or claim that may be made by its manufacturer, is not guaranteed or endorsed by the publisher.
Acknowledgments
We would like to express gratitude to Somjai Wongtriphop, the manager of Shrimp Genetic Improvement Center (SGIC, Surat Thani) for her help and advice on the rearing experiment and all the SGIC staff for their facilitation during the rearing experimental work. Last, we thank the Writing Clinic team at National Center for Genetic Engineering and Biotechnology for the language editing of this manuscript.
Supplementary Material
The Supplementary Material for this article can be found online at: https://www.frontiersin.org/articles/10.3389/fmars.2021.771929/full#supplementary-material
Abbreviations
ABW, average body weight; AL, Aurantiochytrium limacinum; ARA, arachidonic acid; % CV, percent coefficient of length variation; DG, diacylglycerols; DHA, docosahexaenoic acid; DPA, docosapentaenoic acid; EPA, eicosapentaenoic acid; HCD, higher-energy collisional dissociation; LC-HRMS, liquid chromatography-high resolution mass spectrometry; LC-PUFA, long-chain polyunsaturated fatty acid; LPC, lysophosphatidylcholine; LPE, lysophosphatidylethanolamine; M, mysis; MS, mass spectrometry; MUFA, monounsaturated fatty acid; PC, phosphatidylcholine; PE, phosphatidylethnolamine; PG, phosphatidylglycerol; PI, phosphatidylinositol; PL, post-larval; PRM, parallel reaction monitoring; PS, phosphatidylserine; PUFA, polyunsaturated fatty acid; SFA, saturated fatty acid; TFA, total fatty acid; TG, triacylglycerols; TW, Thalasiosira weissflogii.
References
Alberts, B., Johnson, A., Lewis, J., Raff, M., Roberts, K., and Walter, P. (2002). “The Lipid Bilayer,” in Molecular Biology of the Cell, eds B. Alberts, A. Johnson, J. Lewis, M. Raff, K. Roberts, and P. Walter (New York, NY: Garland Science).
Axelsen, P. H., and Murphy, R. C. (2010). Quantitative analysis of phospholipids containing arachidonate and docosahexaenoate chains in microdissected regions of mouse brain. J. Lipid Res. 51, 660–671. doi: 10.1194/jlr.D001750
Barclay, W., and Zeller, S. (1996). Nutritional enhancement of n-3 and n-6 fatty acids in rotifers and Artemia nauplii by feeding spray-dried Schizochytrium sp. J. World Aquac. Soc. 27, 314–322. doi: 10.1111/j.1749-7345.1996.tb00614.x
Bascoul-Colombo, C., Guschina, I. A., Maskrey, B. H., Good, M., O’Donnell, V. B., and Harwood, J. L. (2016). Dietary DHA supplementation causes selective changes in phospholipids from different brain regions in both wild type mice and the Tg2576 mouse model of Alzheimer’s disease. Biochim. Biophys. Acta Mol. Cell Biol. Lipids 1861, 524–537. doi: 10.1016/j.bbalip.2016.03.005
Berg, J. M., Tymoczko, J. L., and Stryer, L. (2002a). “Fatty acid metabolism,” in Biochemistry, eds J. M. Berg, J. L. Tymoczko, and L. Stryer (New York, NY: W H Freeman).
Berg, J. M., Tymoczko, J. L., and Stryer, L. (2002b). “Signal-transduction pathways: an introduction to information metabolism,” in Biochemistry (New York: WH Freeman). Available online at: https://www.ncbi.nlm.nih.gov/books/NBK21205/ (accessed October 4, 2021).
Bhathena, S. J. (2006). Relationship between fatty acids and the endocrine and neuroendocrine system. Nutr. Neurosci. 9, 1–10. doi: 10.1080/10284150600627128
Boglino, A., Darias, M. J., Estévez, A., Andree, K. B., and Gisbert, E. (2012). The effect of dietary arachidonic acid during the Artemia feeding period on larval growth and skeletogenesis in Senegalese sole, Solea senegalensis. J. Appl. Ichthyol. 28, 411–418. doi: 10.1111/j.1439-0426.2012.01977.x
Bradbury, J. (2011). Docosahexaenoic acid (DHA): an ancient nutrient for the modern human brain. Nutrients 3, 529–554. doi: 10.3390/nu3050529
Caers, M., Coutteau, P., and Sorgeloos, P. (1999). Dietary impact of algal and artificial diets, fed at different feeding rations, on the growth and fatty acid composition of Tapes philippinarum (L.) spat. Aquaculture 170, 307–322. doi: 10.1016/S0044-8486(98)00410-4
Caers, M., Coutteau, P., and Sorgeloos, P. (2000a). Impact of starvation and of feeding algal and artificial diets on the lipid content and composition of juvenile oysters (Crassostrea gigas) and clams (Tapes philippinarum). Mar. Biol. 136, 891–899. doi: 10.1007/s002270000295
Caers, M., Coutteau, P., and Sorgeloos, P. (2000b). Incorporation of different fatty acids, supplied as emulsions or liposomes, in the polar and neutral lipids of Crassostrea gigas spat. Aquaculture 186, 157–171. doi: 10.1016/S0044-8486(99)00364-6
Cajka, T., and Fiehn, O. (2014). Comprehensive analysis of lipids in biological systems by liquid chromatography-mass spectrometry. Trends Anal. Chem. 61, 192–206. doi: 10.1016/j.trac.2014.04.017
Chapelle, S., Chantraine, J. M., and Pequeux, A. (1982). Gill Phospholipids of mitochondria in euryhaline crustaceans as related to changes in environmental salinity. Biochem. Syst. Ecol. 10, 65–70. doi: 10.1016/0305-1978(82)90053-9
Coutteau, P., Castell, J. D., Ackman, R. G., and Sorgeloos, P. (1996). The use of lipid emulsions as carriers for essential fatty acids in bivalves: a test case with juvenile Placopecten magellanicus. J. shellfish Res. 15, 259–264.
Coutteau, P., Geurden, I., Camara, M. R., Bergot, P., and Sorgeloos, P. (1997). Review on the dietary effects of phospholipids in fish and crustacean larviculture. Aquaculture 155, 149–164. doi: 10.1016/S0044-8486(97)00125-7
Engelking, L. R. (2015). “Eicosanoids I,” in Textbook of Veterinary Physiological Chemistry, ed. L. R. Engelking (Amsterdam: Elsevier), 434–438. doi: 10.1016/B978-0-12-391909-0.50068-2
Folch, J., Lees, M., and Sloane Stanley, G. H. (1957). A simple method for the isolation and purification of total lipides from animal tissues. J. Biol. Chem. 226, 497–509.
Gillis, T. E., and Ballantyne, J. S. (1999). Mitochondrial membrane composition of two arctic marine bivalve mollusks, Serripes groenlandicus and Mya truncata. Lipids 34, 53–57. doi: 10.1007/s11745-999-337-0
Glencross, B. D. (2009). Exploring the nutritional demand for essential fatty acids by aquaculture species. Rev. Aquac. 1, 71–124. doi: 10.1111/j.1753-5131.2009.01006.x
Han, K., Geurden, I., and Sorgeloos, P. (2001). Fatty acid changes in enriched and subsequently starved Artemia franciscana nauplii enriched with different essential fatty acids. Aquaculture 199, 93–105. doi: 10.1016/S0044-8486(00)00596-2
Harel, M., Koven, W., Lein, I., Bar, Y., Behrens, P., Stubblefield, J., et al. (2002). Advanced DHA, EPA and ARA enrichment materials for marine aquaculture using single cell heterotrophs. Aquaculture 213, 347–362. doi: 10.1016/S0044-8486(02)00047-9
Horwitz, W., and Latimer, G. W. (2005). Official Methods of Analysis of AOAC International. Gaithersburg, MD: AOAC International.
Huang, M., Dong, Y., Zhang, Y., Chen, Q., Xie, J., Xu, C., et al. (2019). Growth and lipidomic responses of juvenile Pacific white shrimp Litopenaeus vannamei to low salinity. Front. Physiol. 10:1087. doi: 10.3389/fphys.2019.01087
Huberman, A. (2000). Shrimp endocrinology. A review. Aquaculture 191, 191–208. doi: 10.1016/S0044-8486(00)00428-2
Immanuel, G., Palavesam, A., and Petermarian, M. (2001). Effects of feeding lipid enriched Artemia nauplii on survival, growth, fatty acids and stress resistance of postlarvae Penaeus indicus. Asian Fish. Sci. 14, 377–388.
Iqbal, J., and Hussain, M. M. (2009). Intestinal lipid absorption. Am. J. Physiol. Endocrinol. Metab. 296, 1183–1194. doi: 10.1152/ajpendo.90899.2008
Ishizaki, Y., Takeuchi, T., Watanabe, T., Arimoto, M., and Shimizu, K. (1998). A preliminary experiment on the effect of Artemia enriched with arachidonic acid on survival and growth of Yellowtail. Fish. Sci. 64, 295–299. doi: 10.2331/fishsci.64.295
Ju, Z. Y., Forster, I., Dominy, W., and Lawrence, A. (2011). Classification and quantification of phospholipids and dietary effects on lipid composition in Pacific white shrimp Litopenaeus vannamei. N. Am. J. Aquac. 73, 221–229. doi: 10.1080/15222055.2011.579035
Kanazawa, A., Teshima, S., and Ono, K. (1979). Relationship between essential fatty acid requirements of aquatic animals and the capacity for bioconversion of linolenic acid to highly unsaturated fatty acids. Comp. Biochem. Physiol. B Comp. Biochem. 63, 295–298.
Knauer, J., and Southgate, P. C. (1997). Growth and fatty acid composition of Pacific oyster (Crassostrea gigas) spat fed a spray-dried freshwater microalga (Spongiococcum excentricum) and microencapsulated lipids. Aquaculture 154, 293–303. doi: 10.1016/S0044-8486(97)00056-2
Kumar, V., Habte-Tsion, H. M., Allen, K. M., Bowman, B. A., Thompson, K. R., El-Haroun, E., et al. (2018a). Replacement of fish oil with Schizochytrium meal and its impacts on the growth and lipid metabolism of Pacific white shrimp (Litopenaeus vannamei). Aquac. Nutr. 24, 1769–1781. doi: 10.1111/anu.12816
Kumar, V., Sinha, A. K., Romano, N., Allen, K. M., Bowman, B. A., Thompson, K. R., et al. (2018b). Metabolism and nutritive role of cholesterol in the growth, gonadal development, and reproduction of crustaceans. Rev. Fish. Sci. Aquac. 26, 254–273. doi: 10.1080/23308249.2018.1429384
Laudicella, V. A., Beveridge, C., Carboni, S., Franco, S. C., Doherty, M. K., Long, N., et al. (2020). Lipidomics analysis of juveniles’ blue mussels (Mytilus edulis L. 1758), a key economic and ecological species. PLoS One 15:e0223031. doi: 10.1371/journal.pone.0223031
Leger, P., Bengtson, D. A., Simpson, K. L., and Sorgeloos, P. (1986). The use and nutritional value of Artemia as a food source. Oceanogr. Mar. Biol. Ann. Rev. 24, 521–623.
Leger, P., Bieber, G. F., and Sorgeloss, P. (1985). International study on Artemia XXXIII. Promising results in larval rearing of Penaeus stylirostris using a prepared diet as algal substitute and for Artemia enrichment. J. World Maricul. Soc. 16, 354–367.
Lepage, G., and Roy, C. C. (1986). Direct transesterification of all classes of lipids in a one-step reaction. J. Lipid Res. 27, 114–120.
Lewis, T. E., Nichols, P. D., and McMeekin, T. A. (1999). The biotechnological potential of thraustochytrids. Mar. Biotechnol. 1, 580–587. doi: 10.1007/PL00011813
Lund, I., Skov, P. V., and Hansen, B. W. (2012). Dietary supplementation of essential fatty acids in larval pikeperch (Sander lucioperca); short and long term effects on stress tolerance and metabolic physiology. Comp. Biochem. Physiol. A Mol. Integr. Physiol. 162, 340–348. doi: 10.1016/j.cbpa.2012.04.004
Maranhão, R. C., and Freitas, F. R. (2014). “HDL metabolism and atheroprotection. Predictive value of lipid transfers,” in Advances in Clinical Chemistry, ed. G. S. Makowski (Cambridge, MA: Academic Press Inc.), 1–41. doi: 10.1016/B978-0-12-800141-7.00001-2
Mourente, G., Medina, A., González, S., and Rodríguez, A. (1995). Variations in lipid content and nutritional status during larval development of the marine shrimp Penaeus kerathurus. Aquaculture 130, 187–199. doi: 10.1016/0044-8486(94)00218-D
Navarro, J. C., Henderson, R. J., McEvoy, L. A., Bell, M. V., and Amat, F. (1999). Lipid conversions during enrichment of Artemia. Aquaculture 174, 155–166. doi: 10.1016/S0044-8486(99)00004-6
Palacios, E., Racotta, I. S., Heras, H., Marty, Y., Moal, J., and Samain, J.-F. (2001). Relation between lipid and fatty acid composition of eggs and larval survival in white pacific shrimp (Penaeus vannamei, Boone, 1931). Aquac. Int. 2001, 531–543. doi: 10.1023/A:1020589924810
Parisi, L. R., Sowlati-Hashjin, S., Berhane, I. A., Galster, S. L., Carter, K. A., Lovell, J. F., et al. (2019). Membrane disruption by very long chain fatty acids during necroptosis. ACS Chem. Biol. 14, 2286–2294. doi: 10.1021/acschembio.9b00616
Rajamoorthi, K., Petrache, H. I., McIntosh, T. J., and Brown, M. F. (2005). Packing and viscoelasticity of polyunsaturated ω-3 and ω-6 lipid bilayers as seen by 2H NMR and X-ray diffraction. J. Am. Chem. Soc. 127, 1576–1588. doi: 10.1021/ja046453b
Rey, F., Alves, E., Domingues, P., Domingues, M. R. M., and Calado, R. (2018). A lipidomic perspective on the embryogenesis of two commercially important crabs, Carcinus maenas and Necora puber. Bull. Mar. Sci. 94, 1395–1411. doi: 10.5343/bms.2017.1140
Rey, F., Alves, E., Melo, T., Domingues, P., Queiroga, H., Rosa, R., et al. (2015). Unravelling polar lipids dynamics during embryonic development of two sympatric brachyuran crabs (Carcinus maenas and Necora puber) using lipidomics. Sci. Rep. 5:14549. doi: 10.1038/srep14549
Ronnestad, I., Finn, R. N., Lie, Ø, and Lein, I. (1995). Compartmental changes in the contents of total lipid, lipid classes and their associated fatty acids in developing yolk-sac larvae of Atlantic halibut, Hippoglossus hippoglossus (L.). Aquac. Nutr. 1, 119–130. doi: 10.1111/j.1365-2095.1995.tb00027.x
Samocha, T. M., Patnaik, S., Davis, D. A., Bullis, R. A., and Browdy, C. L. (2010). Use of commercial fermentation products as a highly unsaturated fatty acid source in practical diets for the Pacific white shrimp Litopenaeus vannamei. Aquac. Res. 41, 961–967. doi: 10.1111/j.1365-2109.2009.02378.x
Sargent, J. R., Bell, J. G., Bell, M. V., Henderson, R. J., and Tocher, D. R. (1993). “The metabolism of phospholipids and polyunsaturated fatty acids in fish,” in Aquaculture: Fundamental and Applied Research, eds B. Lahlou and P. Vitiello (Washington, DC: American Geophysical Union (AGU)), 103–124. doi: 10.1029/CE043p0103
Sasaki, G. C., Capuzzo, J. M., and Biesiot, P. (1986). Nutritional and bioenergetic considerations in the development of the American lobster Homarus americanus. Can. J. Fish. Aquat. Sci. 43, 2311–2319. doi: 10.1139/f86-283
Senior, J. R. (1964). Intestinal absorption of fats. J. Lipid Res. 5, 495–521. doi: 10.1016/s0022-2275(20)40178-6
Singh, B. K., and Kambayashi, T. (2016). The immunomodulatory functions of diacylglycerol kinase ζ. Front. Cell Dev. Biol. 4:96. doi: 10.3389/fcell.2016.00096
Stillwell, W. (2016). “Bioactive lipids,” in An Introduction to Biological Membranes, ed. W. Stillwell (Amsterdam: Elsevier), 453–478. doi: 10.1016/B978-0-444-63772-7.00020-8
Tian, J., Lei, C., Ji, H., Kaneko, G., Zhou, J., Yu, H., et al. (2017). Comparative analysis of effects of dietary arachidonic acid and EPA on growth, tissue fatty acid composition, antioxidant response and lipid metabolism in juvenile grass carp, Ctenopharyngodon idellus. Br. J. Nutr. 118, 411–422. doi: 10.1017/S000711451700215X
Tocher, D. R., Bendiksen, E. Å, Campbell, P. J., and Bell, J. G. (2008). The role of phospholipids in nutrition and metabolism of teleost fish. Aquaculture 280, 21–34. doi: 10.1016/j.aquaculture.2008.04.034
Unagul, P., Suetrong, S., Preedanon, S., Klaysuban, A., Gundool, W., Suriyachadkun, C., et al. (2017). Isolation, fatty acid profiles and cryopreservation of marine thraustochytrids from mangrove habitats in Thailand. Botanica Marina 60, 363–379. doi: 10.1515/bot-2016-0111
Visudtiphole, V., Phromson, M., Tala, S., Bunphimpapha, P., Raweeratanapong, T., Sittikankaew, K., et al. (2018). Aurantiochytrium limacinum BCC52274 improves growth, hypo-salinity tolerance and swimming strength of Penaeus vannamei post larvae. Aquaculture 495, 849–857. doi: 10.1016/J.AQUACULTURE.2018.06.066
Watanabe, T. (1993). Importance of docosahexaenoic acid in marine larval fish. J. World Aquac. Soc. 24, 152–161. doi: 10.1111/j.1749-7345.1993.tb00004.x
Watanabe, W. O., Alam, M. S., Ostrowski, A. D., Montgomery, F. A., Gabel, J. E., Morris, J. A., et al. (2016). Live prey enrichment and artificial microdiets for larviculture of Atlantic red porgy Pagrus pagrus. Aquac. Rep. 3, 93–107. doi: 10.1016/j.aqrep.2016.01.003
Wimuttisuk, W., Tobwor, P., Deenarn, P., Danwisetkanjana, K., Pinkaew, D., Kirtikara, K., et al. (2013). Insights into the prostanoid pathway in the ovary development of the penaeid shrimp Penaeus monodon. PLoS One 8:e76934. doi: 10.1371/journal.pone.0076934
Xie, S., Liu, Y., Tian, L., Niu, J., and Tan, B. (2020). Low dietary fish meal induced endoplasmic reticulum stress and impaired phospholipids metabolism in juvenile Pacific white shrimp, Litopenaeus vannamei. Front. Physiol. 11:1024. doi: 10.3389/fphys.2020.01024
Keywords: long-chain polyunsaturated fatty acids, lipids, lipidomics, shrimp larvae, Aurantiochytrium limacinum, diet supplement
Citation: Visudtiphole V, Khudet J, Chaitongsakul P, Plaisen S, Siriwattano J, Laiphrom S, Klaysuban A, Raweeratanapong T, Sittikankaew K, Rattanaphan N, Koichai L, Unagul P and Uawisetwathana U (2021) Growth and Lipidomic Analyses of Penaeus monodon Larvae Supplemented With Aurantiochytrium limacinum BCC52274. Front. Mar. Sci. 8:771929. doi: 10.3389/fmars.2021.771929
Received: 07 September 2021; Accepted: 27 September 2021;
Published: 18 October 2021.
Edited by:
Chuangye Yang, Guangdong Ocean University, ChinaReviewed by:
Jianchun Shao, Fujian Agriculture and Forestry University, ChinaBruno Cavalheiro Araújo, University of Mogi das Cruzes, Brazil
Copyright © 2021 Visudtiphole, Khudet, Chaitongsakul, Plaisen, Siriwattano, Laiphrom, Klaysuban, Raweeratanapong, Sittikankaew, Rattanaphan, Koichai, Unagul and Uawisetwathana. This is an open-access article distributed under the terms of the Creative Commons Attribution License (CC BY). The use, distribution or reproduction in other forums is permitted, provided the original author(s) and the copyright owner(s) are credited and that the original publication in this journal is cited, in accordance with accepted academic practice. No use, distribution or reproduction is permitted which does not comply with these terms.
*Correspondence: Virak Visudtiphole, dmlyYWsudmlzQGJpb3RlYy5vci50aA==