- 1Section for Microbiology, Department of Biology, Center for Electromicrobiology, Aarhus University, Aarhus, Denmark
- 2Marine Biological Section, Department of Biology, University of Copenhagen, Helsingør, Denmark
- 3Section for Microbiology, Department of Biology, Aarhus University Centre for Water Technology (WATEC), Aarhus University, Aarhus, Denmark
Steep geochemical gradients surround roots and rhizomes of seagrass and protect the plants against the harsh conditions in anoxic sediment, while enabling nutrient uptake. Imbalance of these gradients, due to e.g., low plant performance and/or changing sediment biogeochemical conditions, can lead to plant stress and large-scale seagrass meadow die-off. Therefore, measuring and mapping the dynamic gradients around seagrass roots and rhizomes is needed to better understand plant responses to human impact and environmental changes. Historically, electrochemical microsensors enabled the first measurements of important chemical species like O2, pH or H2S with high sensitivity and spatial resolution giving important insights to the seagrass rhizosphere microenvironment; however, such measurements only provide information in one dimension at a time. In recent years, the use of reversible optical sensors (in the form of planar optodes or nanoparticles) and accumulative gel sampling methods like Diffusive Gradients in Thin films (DGT) have extended the array of analytes and allowed 2-D mapping of chemical gradients in the seagrass rhizosphere. Here, we review and discuss such microscale methods from a practical angle, discuss their application in seagrass research, and point toward novel experimental approaches to study the (bio)geochemistry around seagrass roots and rhizomes using a combination of available techniques, both in the lab and in situ.
Introduction
Seagrass meadows are important ecosystems that play an important role for marine biodiversity (Bertelli and Unsworth, 2014), coastal protection (Fonseca and Cahalan, 1992) and not the least sediment biogeochemistry and carbon sequestration (Mcleod et al., 2011; Fourqurean et al., 2012). Photo-assimilated carbon moves from the seagrass leaves to the roots, and into the anoxic sediment, where the anoxic conditions prevent rapid microbial mineralization (Duarte et al., 2005). Besides plant debris, organic carbon from plant excretions involving sugars and organic acids can have a major impact on the carbon sequestration capability (Wetzel and Penhale, 1979; Long et al., 2008). Up to 40% of the dissolved organic carbon under seagrass meadows was recently estimated to be in the form of plant-derived sucrose that accumulates in the seagrass rhizosphere and seems to largely escape from microbial degradation due to the concomitant excretion of phenolic compounds (Sogin et al., 2021). However, the organic carbon can also find its way back to the atmosphere through re-mobilization, e.g., via the continuous emission of the greenhouse gas methane from seagrass meadows (Garcias-Bonet and Duarte, 2017) or via physical disturbance by humans that exposes the reduced sediment to O2 and thereby stimulates aerobic mineralization processes with faster carbon turnover rates and complete oxidation of organic carbon to CO2 (Brodersen et al., 2019). Nevertheless, plant-microbe and other microbial interactions in the seagrass rhizosphere are not well understood and it is important to resolve biogeochemical element cycling underneath seagrass meadows and its role for seagrass plant fitness.
Growth of seagrass roots into reduced, anoxic sediment relies strongly on gas-filled plant tissue layers, aerenchyma, which allows O2 in the seagrass leaves, originating either via photosynthesis or via diffusion from the water column, to reach and ventilate the belowground tissue (Brodersen et al., 2018a). While the mature parts of the seagrass roots develop a gas-impermeable tissue layer—a Casparian band-like structure mainly composed of suberin—that enables efficient internal transport of O2 and prevents radial O2 loss (ROL) and influx of phytotoxins such as sulfide (H2S) into the plant tissue (Barnabas, 1996; Colmer, 2003), O2 eventually leaks from the growing root tips and the root/shoot junctions and oxidizes the rhizosphere (Jensen et al., 2005; Brodersen et al., 2015b; Koren et al., 2015). For instance, Jensen et al. (2005) reported that O2 diffused from the roots 2 mm into the sediment. The extent of the radial O2 loss varies with changing light conditions and root age resulting in hetereogenous and dynamic distributions of oxic zones underneath a seagrass meadow (Frederiksen and Glud, 2006). However, the extent of radial O2 loss is not only determined by the capacity of the root to leak O2 but also by the capability of the sediment to consume the released O2 (i.e., the sediment O2 demand). For example, if seagrass is transplanted into oligotrophic sediment with less bacterial density, the O2 released from roots will diffuse further into the sediment before it is consumed by abiotic and biotic oxidation reactions than in highly reduced, sulfidic sediment with a high content of organic material and resulting high bacterial density. Taken together, the presence of organic carbon from plant debris and root exudates together with the spatially restricted oxic zones create hotspots of microbial activity below a seagrass meadow (Blaabjerg et al., 1998; Nielsen et al., 2001; Jensen et al., 2007; Brodersen et al., 2018b), which renders the seagrass rhizosphere into a diurnally changing, mosaic of steep geochemical gradients.
Despite their ability to sustain the harsh geochemical conditions of anoxic sediment, seagrasses are currently challenged by human impact such as coastal development and eutrophication, which lead to increased water turbidity and hypoxia, as well as, increased epiphyte growth on seagrass leaves (Erftemeijer and Robin Lewis, 2006; Orth et al., 2006; Waycott et al., 2009). This results in prolonged, low-light availability for photosynthesis altering the balance of the chemical gradients that form around the roots protecting seagrasses and enabling nutrient uptake (Brodersen et al., 2015a, 2017a). The source of the stress originates from above the meadow through human impact and environmental changes, but the resulting danger often comes from underneath and that is H2S (Holmer and Bondgaard, 2001). Under healthy conditions, the aeration of the below-ground tissues and oxygenation of the rhizosphere around the root tips, root/shoot junctions and the base of the shoot (i.e., the basal leaf meristem) facilitates the abiotic and microbial oxidation of the toxic H2S (Brodersen et al., 2018b). However, during periods of low water-column O2 content, e.g., during nighttime when photosynthesis-driven O2 production is halted, the supply of O2 to the below-ground tissues cannot counterbalance the H2S intrusion. Thus, prolonged O2 limitations initiate large-scale seagrass meadow die-off (Borum et al., 2005). Therefore, it is important to determine how human impact can change geochemical gradients in the seagrass meadow sediment and rhizosphere.
Vertical concentration gradients in sediments of a broad variety of analytes can be determined by analyzing extracted porewater (e.g., McGlathery et al., 2001). Typically, cylindrical push cores have been used to section the sediment horizons on the cm-scale (Toshihiro et al., 2001; Sogin et al., 2021). However, this technique provides average concentrations from large sample volumes; hence, steep gradients in the mm-range, as well as, small-scale heterogeneities are overlooked (Huang et al., 2019). To fully account for the pronounced structural and chemical heterogeneity of the seagrass rhizosphere it is thus necessary to employ tools for microenvironmental sensing and analysis at high spatio-temporal resolution. In the following, we review experimental tools for exploring the seagrass rhizosphere microenvironment ranging from one-dimensional measurements of chemical concentration gradients with different types of microsensors, to mapping of the two-dimensional distribution of chemical species in the rhizosphere using optode-based chemical imaging and gel-sampling techniques. We exemplify how these techniques have been used to study the seagrass rhizosphere and discuss future needs and possibilities for multidimensional sensing approaches to further resolve how the seagrass rhizosphere microenvironment modulates plant fitness and sediment biogeochemistry.
One-Dimensional Probing of the Seagrass Rhizosphere Microenvironment
Microsensors
Electrochemical and fiber-optic microsensors enable measurements of concentration profiles in 1-D with high sensitivity and spatial resolution owing to the small tip sizes in the μm-range. The available gas, ion, bio and micro-optode sensors cover a wide range of analytes (Kühl and Revsbech, 2001; Revsbech, 2021), but electrochemical O2 and H2S microsensors (Revsbech and Jørgensen, 1986; Revsbech, 1989; Jeroschewski et al., 1996) are still the most used ones in the context of seagrass ecology (Jensen et al., 2005; Trevathan-Tackett et al., 2017; Schrameyer et al., 2018; Brodersen et al., 2019). Further information on the working principles of microsensors and their application in plant biology can be found in recent reviews by Pedersen et al. (2020) and Revsbech (2021).
Microsensors are usually guided vertically into the sediment using a micromanipulator to measure depth profiles, from which the analyte fluxes between sediment and water and inside the sediment can be calculated or modeled (e.g., Berg et al., 1998). The μm tip diameter and the slender shaft of microsensors enable minimally invasive measurements of the steep chemical gradients in the sediment. For example, Schrameyer et al. (2018) carried out an in situ shading experiment and used microsensors to measure H2S and O2 in retrieved sediment cores in the laboratory, among others, to calculate the diffusive O2 uptake. It is more convenient to carry out microsensor probing on retrieved cores in aquaria in the laboratory (see an example setup in Figure 1A). For instance, in the laboratory the sensor tip can be positioned on the sediment surface by using a dissecting microscope, which serves as a reference point during profiling. Anyhow, in situ microsensor measurements are cumbersome but feasible (e.g., Brodersen et al., 2017a; Figure 1C). The analyst should then be experienced enough to handle the sensors and judge the sensor position in the sediment by following the signal response. In this way, microsensor tips can be positioned within the root tissue or in the base of the leaf under field conditions (Borum et al., 2005). The microsensor tips are typically robust enough to penetrate root and rhizome/leaf tissue but break if they hit a solid surface like a bivalve shell or larger, motile infauna. Using microsensors in un-sieved, natural sediment is thus risky, albeit microsensors often break due to incautious handling during (dis)mounting.
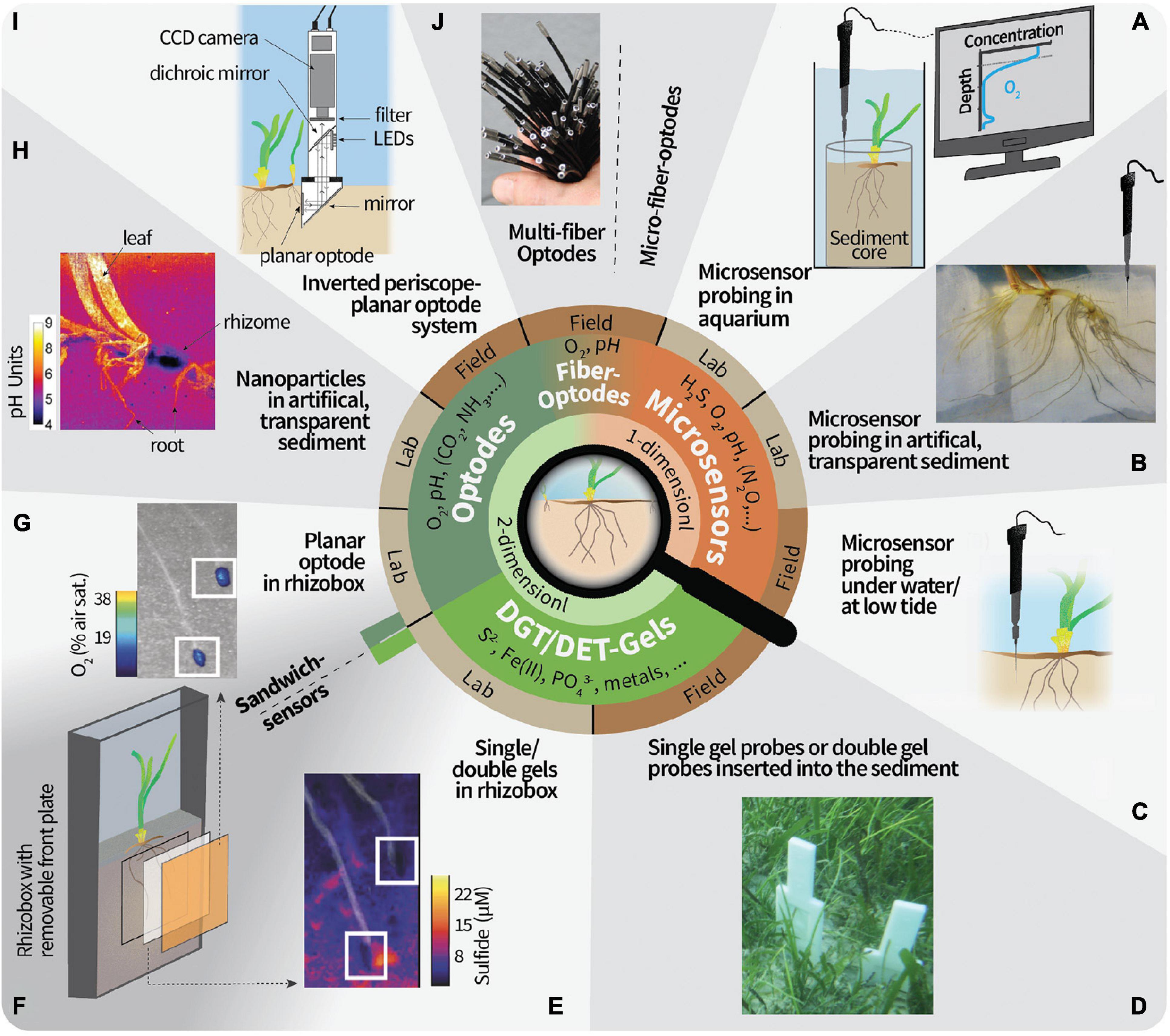
Figure 1. Overview of 1- and 2-D techniques to study the geochemistry in the seagrass rhizosphere microenvironment. (A) Microsensor probing on sediment cores retrieved from the field or seagrass transplanted into sediment in the laboratory. The conceptual microsensor profile of O2 is taken vertically through the upper layers of the sediment core and allows the calculation of, for example, the sediment diffusive O2 uptake and the O2 penetration depth. O2 excursions below the O2 penetration depth could be from radial O2 loss from roots/rhizomes or ventilated fauna burrows. (B) Artificial, transparent sediment in a custom-made split flow chamber (Brodersen et al., 2015b) allows to approach the roots with microsensors from different directions. (C) Microsensor probing under submerged conditions or when the seagrass plants are emerged during low tide have been proven challenging but feasible. (D) Gel probes or gel-sandwich (double) probes have been proven to be a robust field technique. (E) Example result of a sulfide-sensitive gel from a laboratory deployment. The image is modified from Martin et al. (2018) and white squares mark the root tips. (F) Gels can be mounted in between the sediment and planar optodes. (G) Example result of an O2-sensitive planar optode. An imaging system needs to be brought in position for the excitation in the dark and read-out of the planar optode. The image is modified from Martin et al. (2018) and white squares mark the root tips. (H) Optode nanoparticles in artificial, transparent sediment overcomes limitations of planar optodes, but cannot be done in natural sediment. Image is taken from Brodersen et al. (2016). (I) In situ instrument for planar optode measurements. Note that this has not yet been deployed in seagrass meadows. Image was modified from Glud et al. (2001). (J) O2 multi-fiber optode system for the simultaneous recording of 100 datapoints in the field. Note that this has not yet been deployed in seagrass meadows. Image was modified from Fischer and Koop-Jakobsen (2012). Micro-fiber optodes are more robust but with a lower resolution due to the bigger tip size than microsensors made out of glass.
One of the main challenges is to relate the position of the sensor tip in the sediment to the below-ground root structures if the roots cannot be visually seen. In order to know the sensor tip proximity to the roots during measurements, Jensen et al. (2005) placed a seagrass root horizontally on the sediment, positioned the microsensor tip at the root surface as reference point, and covered the roots again with sediment. However, this approach is very time-consuming as profiles can only commence when the geochemical gradients have re-established (often after more than 3 h depending on the analyte) and the connection between sensor and root is often lost during the sediment re-covering process. To accommodate this, artificial, transparent sediment can be used, for example, in a split flow chamber which enables approaching the roots with the microsensors from various angles (Brodersen et al., 2014, 2015b; Figure 1B). However, the use of non-natural sediment, with e.g., different redox conditions, microbial communities, and O2 demand, for laboratory incubations should be carefully considered as this can deviate the measured gradients from the ones which would be found in natural sediment. One way to alleviate this experimental limitation is, e.g., to utilize a reducing agent such as H2S, which can be applied to the artificial sediment to function as an O2 scavenger (Brodersen et al., 2014). Moreover, it is possible to perform spatial measurements of 2-D transects and 3-D grids of concentration profiles with microsensors using dedicated motorized microprofiling setups (e.g., Lichtenberg et al., 2017; Herschend et al., 2018), but such approach has limited applicability for rhizosphere studies, where the exact position of the plant biomass in the sediment is uncertain. Although this to some extent can be alleviated by employing artificial, transparent sediment it is a very time-consuming process, especially if the whole rhizosphere and/or several analytes are analyzed. Despite the fast response time (time that is needed for a stable read-out) of microsensors, typically in the range of seconds, recording one profile can take more than a few minutes. The time for acquiring one concentration profile will depend on the sensor response time, the amount of datapoints recorded, i.e., the given step size between data points, and the motor velocity used for positioning. The lowest possible step size is roughly the diameter of the sensor tip. During prolonged measuring periods roots can grow into new sediment regions (e.g., root growth of 5 mm day–1 for Z. marina; cf. Jensen et al., 2005) and thus can lead to difficulties to overlay the results from subsequently recorded profiles. Therefore, it is recommended to aim for chemical hotspots like the root tip region and root/shoot junction when analyzing the seagrass rhizosphere with detailed microsensor measurements.
In summary, microsensors cannot fully resolve the complex spatial heterogeneity of chemical gradients around seagrass roots but allow detailed, local measurements within the plant tissue, as well as local measurements of concentration profiles between the plant and surrounding sediment at very high spatio-temporal resolution. The commercial availability of microsensors for a wide array of analytes are additional benefits. In fact, for several analytes of interest microsensors represent the only option for microscale measurements (e.g., H2S, N2O, H2, CH4 and most ionic species).
Multi-Dimensional Mapping of the Seagrass Rhizosphere Microenvironment
Optode-Based Techniques for Reversible, Chemical Imaging
In contrast to microsensors, the use of planar optical sensors (planar optodes) in combination with imaging enables the measurement of analytes in 2-D and can therefore visualize dynamic changes in the chemical microenvironment and link them to small-scale heterogeneities around seagrass roots. For seagrass, planar optodes sensitive to O2 (Jensen et al., 2005; Frederiksen and Glud, 2006; Jovanovic et al., 2015) and pH (Brodersen et al., 2017b) have been used, whereas CO2-sensitive optodes have only been used for other aquatic and saltmarsh plants (Koop-Jakobsen et al., 2018; Lenzewski et al., 2018). The rectangular planar optode foils need to be brought in close contact with the below-ground plant tissue in the sediment. In order to ensure such close contact, planar optodes can be integrated in custom-made chambers with a flat, transparent wall. For instance, Martin et al. (2018) used narrow (2 cm wide) chambers that were placed in an aquarium and incubated in a tilted position to encourage root growth against the detachable glass wall mounted with a planar optode (Figure 1F). A practical video tutorial for the assembly of a similar incubation chamber has been published by Moßhammer et al. (2019b).
Planar optodes consist of an analyte-sensitive indicator dye dispersed in a polymer matrix and coated on solid supports such as plastic foils (Larsen et al., 2011). They are excited externally with a light source, and an image is taken quantifying either the emitted luminescence intensity or the decay time (Schäferling, 2012; Koren and Kühl, 2018), which change as function of the analyte concentration. Data acquisition can rely on simple ratiometric imaging (e.g., Larsen et al., 2011), or more advanced measurements, such as the luminescence decay time (e.g., Koren et al., 2019) and hyperspectral imaging (i.e., the recording of the continuous spectrum of light in each pixel of the image, which allows the simultaneous analysis of emissions from different analyte-sensitive indicators, see Zieger et al., 2021, for more details). The acquired images can be transformed into a calibrated, color-coded image of analyte concentration distributions, which can be aligned with the underlying root/rhizome structure. Hence, hotspots of enhanced biogeochemical reactions can be identified based on where distinct concentration variations are seen (e.g., Jensen et al., 2005; Frederiksen and Glud, 2006) (example result can be seen in Figure 1G). In order to link the measured gradients to the actual below-ground tissue, a photograph should be taken of the sediment cross-section that faced the planar optode after disassembly. If the optode foil is transparent enough, the root structures and positions can also be followed directly during measurement (Frederiksen and Glud, 2006; Martin et al., 2018). Generally, planar optodes have high spatial resolution (μm2-range), can be made in large sizes (>400 cm2) to cover the entire area of interest, and have a response time in the range of seconds to a few minutes depending on the type of planar optode and the thickness of the sensor layer and/or the optical isolation layer on top of the sensor layer (Santner et al., 2015). The response time, however, is often not a critical factor in seagrass studies, as the planar optode stays in the same position during the experiment and high measuring frequencies (more than one image per minute) is usually not needed. Besides the preparation of the imaging and incubation setup, calibration is one of the most laborious steps of planar optode measurements and is usually carried out in sediment-free aquaria prior to measurements. The imaging step itself only takes a few seconds. Therefore, once the setup is ready, dynamic changes over day and night cycles can be easily monitored due to the fully reversible response of the planar optodes to e.g., O2 or pH. For example, Frederiksen and Glud (2006) measured O2 distributions at regular time intervals around roots of Zostera marina over 44 h and showed that O2 release was reduced by 60% during darkness. We note that the excellent long-term stability of O2 optodes and their temperature sensitivity, enabling temperature compensated measurements during deployment, allows for measuring over weeks and even months (e.g., Rickelt et al., 2014). Simple, yet powerful planar optode systems can be assembled from commercially available components (e.g., Larsen et al., 2011; Staal et al., 2011) and are also commercially available as complete systems. Instrumentation for underwater in situ imaging with planar optodes has also been developed (Glud et al., 2001, 2005; Figure 1I), but awaits first applications in seagrass meadows.
When assembling the planar optode-seagrass chambers close contact of the optode with the root biomass is important, because otherwise the true distribution of gradients cannot be resolved. Another challenge during the assembly is to avoid air bubbles trapped on either side of the optode foil. Furthermore, the plant tissue and the microbial community around the roots should be given time to recover after assembly of the incubation chamber. While gradients from purely abiotic sources to sinks establish relatively fast, the biotic components of the rhizosphere can sometimes need several weeks to recover from manipulation/disturbance (Wang et al., 2021). This could e.g., be due to the recovery of root hairs that were broken off during transplantation or the slow re-establishment of a microbial network including filamentous bacteria (Scholz et al., 2021) and fungal associations (Borovec and Vohník, 2018). The plant itself can also experience stress during sampling and separation into single shoots followed by transplantation into the experimental chamber.
One constraint of planar optode-based imaging is that the diffusive fluxes are cut off by the solid support on one side which can distort the true analyte gradients around a 3-D structure positioned against the planar optode (Li et al., 2019). An alternative approach to coating the optode matrix on a solid support is to use optode-nanoparticles (Moßhammer et al., 2019a), e.g., in combination with magnetite to force the optode particles against the seagrass leaves in a magnetic field (Brodersen et al., 2020) or dispersed in a transparent, artificial sediment embedding the below-ground tissues of a seagrass plant (Koren et al., 2015; Brodersen et al., 2016; Figure 1H). Such dispersed optical sensor nanoparticle approaches are read out by the same imaging systems as planar optodes, but alleviate the limitations of planar optodes by avoiding the sudden cut off of gradients at the plastic foil on which the optode is coated and the need to ensure close contact between the optode foil and the sample. Sensor nanoparticles have, for example, been used to resolve radial O2 loss from below-ground seagrass tissues on an entire rhizosphere level and how this is affected by changing light and water-column O2 conditions (Koren et al., 2015).
In summary, optode-based imaging of the below-ground chemical microenvironment with planar optodes or dispersed sensor nanoparticles is fast and reversible, enabling time-series measurements. The incubation chambers can be custom-made and flexibly adjusted to fit the experimental needs, but challenges are to ensure close contact of root/rhizome tissue with the optode and to avoid air bubbles being trapped behind the foil during assembly. Once the setup is ready, small-scale heterogeneities and spatio-temporal dynamics can be visualized. Optical nanoparticles to study O2 dynamics around the below-ground structure of seagrass circumvents drawbacks of the planar optode technique, but requires the incubation in artificial, transparent sediment. Currently, such reversible optode-based imaging is limited to a handful of analytes (mainly O2, pH, CO2). Novel optodes for important analytes like NH3 are being developed (Merl and Koren, 2020), but so far have not been used in seagrass research. Also, multi-analyte optodes exist (Moßhammer et al., 2016) and novel imaging approaches are promising to push multi-parameter chemical mapping in the rhizosphere even further (Koren and Zieger, 2021).
Irreversible Chemical Mapping With Gel Probes
Gel-sampling methodologies using either Diffusive Gradients in Thin-films (DGT) or Diffusive Equilibration in Thin-Films (DET) enable irreversible, spatial 2-D mapping of chemical species after sample exposure to the gel for a defined time interval (see Davison et al., 2000; Santner et al., 2015 for a more detailed account of these experimental methods). Both techniques rely on passive diffusion of the sediment solutes from the target area into a gel, where they are either continuously accumulated (DGT) or in equilibrium with the porewater concentrations (DET). Hence, similar experimental incubation chambers as explained for the planar optode systems can be used (Figure 1F). But in contrast to the planar optodes, the gels can relatively easily be deployed in situ and subsequently analyzed after retrieval (Figure 1D). One of the most targeted analytes for single-analyte-binding DGT gel analysis in the seagrass rhizosphere is H2S (Brodersen et al., 2017b; Martin et al., 2018; Figure 1E), which can e.g., be mapped with a silver iodide gel which continuously binds ΣH2S (i.e., H2S, HS–, and S2–) from the porewater through a precipitation reaction to silver sulfide (Teasdale et al., 1999). The precipitation reaction induces a dark coloration, which can then be analyzed after incubation by computer imaging densitometry (CID), e.g., using a common flatbed scanner. In contrast to DGT gels, the DET gels do not bind the analyte and hence concentrations within the gel represent porewater concentrations at the time of retrieval. One of the most common analytes for DET gels in seagrass beds is Fe(II) (Pagès et al., 2012; Kankanamge et al., 2017, 2020), where the analyte concentrations can be quantified by covering the gel with a ferrozine reagent staining gel after sampling to induce a colorimetric reaction (Jézéquel et al., 2007; Robertson et al., 2008; Bennett et al., 2012). This staining reaction is not necessary if the gels are directly analyzed after drying (in the case for DET gels, the analytes must be immobilized after sampling) using a beam technology such as laser ablation inductively coupled mass spectrometer (LA-ICP-MS), which allows to simultaneously analyze and map the distribution of several ionic species in the seagrass rhizosphere with a spatial resolution of around 100 μm (Brodersen et al., 2017b).
When the DGT and DET gels are deployed in incubation chambers (as described above for planar optodes) the following practical aspects should be considered: (1) close contact of the gel with the root/rhizome tissue, (2) avoiding entrapment of air pockets between gel and sediment, and (3) sufficient pre-incubation for the recovery of plant and microbial community, as well as, chemical gradients after transplanting. A defined onset of gel incubation can be accommodated by placing a retrievable, sterilized plastic foil in between the sample/sediment and the gel (Brodersen et al., 2017b), which can be removed to initiate sample contact to the gel when steady state biogeochemical conditions have been reached. While planar optodes allow continuous and dynamic recording of data over time using the same optode foil, measurements with DGT gels represent accumulated signals during a defined incubation time, i.e., a one-time irreversible readout. Another drawback of DGT gels is the continuous removal of the analyte that can affect processes within the sediment, which is especially critical e.g., at low solute concentrations. Hence, careful planning of the cultivation/measuring period is required. This is not the case for DET gels, because the solutes are not bound and accumulated within gel. But therefore, it is also crucial to process the DET gels immediately after sampling, as otherwise the analyte diffuses within the gel in all directions and blurs the actual gradients and distributions.
These passive gel-sampling techniques can also be used in situ, as probes of single gels and even combined DET-DGT and DET-DET gels can be pushed into the seagrass sediment when the meadow is exposed at low-tide (Simpson et al., 2018) or under water (Brodersen et al., 2017b; Figure 1D). The combination of multiple gels in one probe with in situ deployment enables the simultaneous measurement of multiple analytes over similar temporal and spatial scales, which can visualize the chemical heterogeneity below a seagrass meadow, including the contribution from multiple seagrass shoots and bioturbation. The in situ gel deployments and sandwich-gel approaches of combined DGT-DET or DET-DET gels also have several drawbacks, which should be considered when interpreting the imaging results. First, pushing the gel probes into the sediment can drag upper sediment layers deeper down causing artifacts (Santner et al., 2015). Second, no structural photographs can be taken from the measured cross-section of the sediment and hence correlations of solute distributions with below-ground structures such as roots or burrows are speculative. Third, DGT gels provide integrated and averaged concentrations over the entire deployment time, while DET gels represent the porewater concentrations over the last period of the deployment time. Therefore, the combination and deployment of DGT and DET gels at the same time may lead to artifacts depending on the change of gradients during the deployment time (Robertson et al., 2008). An alternative to circumvent this issue for H2S is a novel DET gel (Kankanamge et al., 2020), which allows to deploy a DET(H2S)-DET probe rather than a DGT(H2S)-DET-probe.
One simple but yet elegant approach was reported by Marzocchi et al. (2019), who investigated the PO43– and NH4+ porewater concentrations around roots of the freshwater plant Vallisneria spiralis by assembling a sediment sandwich with 96-well microplates covered with a membrane on each side. The microtiter plates can be considered a variant of DET gels, where each well goes in equilibrium with the porewater concentrations. After deployment, the analyte concentrations were quantified by standard colorimetric reactions. With this approach, the spatial resolution is limited by the diameter of the wells (approximately 7 mm) and the spacing between the wells (approximately 1 mm).
In summary, gels are passive sampling techniques that extend the array of analytes for 2-D mapping. In contrast to reversible optodes, the gel-based approaches allow only the read-out of analyte distributions at a given time period. However, for several key analytes such as sulfide, metals and other ionic species these gel-sampling techniques are presently the only option for 2-D mapping in the seagrass rhizosphere. Advantages of these techniques include the versatility of the gels as they can be deployed in various configurations including as sandwich sensors and for in situ measurements.
Future Developments
A better understanding of the heterogeneous distribution of geochemical hotspots around seagrass roots/rhizome, and their dynamic changes over day/night and seasonal cycles is instrumental for enhancing our knowledge about how the seagrass plant is adapted to life in sediments and how it is affected by environmental stress. As mentioned above, the seagrass rhizosphere is a dynamic chemical environment where several chemical parameters exhibit changes at different time scales and with different interdependences. Hence, the analysis of multiple analytes in the same spatial area and time is needed. This can be achieved through various approaches.
For instance, Brodersen et al. (2017b) used a narrow sandwich chamber (width: 1 cm) design for the deployment of O2 and/or pH planar optodes on one side of the below-ground tissue structures and single- or multi-binding DGT gels on the other side. With this approach the authors could show that the release of O2 and organic compounds from the roots and rhizome stimulate nutrient mobilization in the rhizosphere, where (i) protolytic dissolution (protons generated from protective sulfide oxidation stimulated through radial O2 loss from the roots and rhizome) mobilized phosphate mainly in the light and (ii) reductive dissolution [reduction of insoluble Fe(III)oxyhydroxides to dissolved Fe(II) driven by sulfide generated by sulfate reducing bacteria that are stimulated by organic carbon exudations from the roots] mobilized phosphate and increases Fe(II) availability mainly in the dark. These are important seagrass-driven nutrient mobilization mechanisms that are further supported by the release of organic acids (Long et al., 2008) and microbial Fe(III) reduction linked to organic carbon oxidation. However, optodes can also be directly coated with a sampling gel (Figure 1F). By means of such a combined optode-gel-sensor, Martin et al. (2018) monitored the O2 concentrations and ensured sediments remained anoxic during deployment of the H2S-sensitive DGT gel. Other sensor combinations are the above-mentioned DGT-DET and DET-DET gel-sandwiches, which can also be applied in the field (Robertson et al., 2008; Pagès et al., 2012). Moreover, dual optode sensors for pH and O2 measurements (Moßhammer et al., 2016) and the advances in hyperspectral imaging (Zieger et al., 2021) will facilitate further development and applications of multi-analyte optodes in future studies. A comprehensive model example of combining multidimensional techniques has been provided by Williams et al. (2014), who measured the O2 or pH and metal distributions around rice roots by using combined optode-DGT gel sensors.
Microbial communities drive geochemical changes and conditions in the rhizosphere, but their specific associations with hotspots along the roots/rhizome and in different distances away from seagrass root/rhizome surfaces have only been poorly resolved. One approach for the sampling of microbial communities and correlation with geochemical gradients measured with microsensors has been reported by Brodersen et al. (2018b). The authors made use of artificial, transparent sediment with added porewater microbes in the previously reported, custom made split flow chamber (Brodersen et al., 2014). Moreover, Martin et al. (2018) used O2-sensitive planar optodes to distinguish microbial communities on O2-releasing and non-leaking roots with particular focus on cable bacteria, which are filamentous, cm-long bacteria that change their surrounding sediment geochemistry with major impact on the pH and sulfur-cycle (Pfeffer et al., 2012). The colonization pattern of cable bacteria and their geochemical impact in surface sediments and around worm tubes have been visualized using combinations of planar optodes and gels (Aller et al., 2019; Yin et al., 2021a, b). Interestingly, cable bacteria appear to be a common member of the root microbiome of various seagrass species (Scholz et al., 2021) but their potential geochemical impact in the seagrass rhizosphere has not yet been shown. The identification of hotspots by multidimensional imaging could also be used for targeted sampling for metabolomics or chemical analyses.
Last, not least, there is a strong need for better in situ mapping of the seagrass rhizosphere microenvironment. Microenvironmental analyses of the seagrass rhizosphere have mostly been done in the laboratory, with the exception of (i) diver-operated in situ microsensor measurements, typically positioned in seagrass leaf meristems to follow the O2 and H2S status of the plant over diel cycles (e.g., Brodersen et al., 2017a; Olsen et al., 2018), and (ii) the in situ deployment of gel samplers (e.g., Pagès et al., 2012; Brodersen et al., 2017b, see Supplementary Table 1 for an overview of selected key references). However, laboratory measurements cannot fully replicate the dynamic environmental conditions found in situ and often involve manipulation of the rhizosphere and surrounding sediment during assembly of the setup and sampling. This can potentially change redox conditions and other aspects of the in situ microenvironment including the spatial arrangement of microbes relative to the plant roots. Thus, it should be kept in mind that measurements in laboratory setups with sieved, homogenized sediment and mainly on a single shoot do not represent the natural environment below a seagrass meadow, where bioturbation, multiple shoots, and plant debris cause even more heterogenous distributions of gradients (Edward and Mark, 1998; Simpson et al., 2018). While in situ mapping of chemical species over hours/days with planar optodes mounted on dedicated underwater imaging platforms and in mesocosms is well established in soil and sediment biogeochemistry (Glud et al., 2001; Askaer et al., 2010; Santner et al., 2015) such technologies have, surprisingly, not yet been applied in seagrass meadows. For instance, potential issues to be solved are how to avoid scratching and other damage of the planar optode surface when inserting it into the tough matrix of seagrass covered sediments. The insertion would also break the root/rhizome network and disrupt the gradients in a seagrass meadow. But this could be overcome if the instrument would be deployed at the fringe of a seagrass bed or in newly restored seagrass beds where seagrass seedlings were just transplanted. It should be noted that no perfect alignment of roots and rhizome to the measuring devices (planar optodes or gel probes) can be assured under in situ conditions, but rather the natural distribution of gradients will be determined. Also more long-term in situ measurements, e.g., of seasonal changes in the seagrass rhizosphere or long-term changes due to environmental impacts such as dredging, heat waves or hypoxia events, remain to be realized. A suitable starting point for such measurements could, e.g., be based on systems for distributed sensing with fiber-optic optode arrays (e.g., Fischer and Koop-Jakobsen, 2012; Figure 1J) that can be inserted into the sediment at various positions/depths to monitor rhizosphere oxygenation over hours/days (Koop-Jakobsen et al., 2017) or robust spears with defined measuring spots that are able to monitor, e.g., O2 and temperature profiles in soils and sediment over several months (Rickelt et al., 2014). Adaptation and expansion of such techniques for optode-based, long-term in situ measurements of key parameters like O2, pH and temperature in seagrass rhizospheres is a promising research direction, which will enhance insight to in situ characteristics of the seagrass rhizosphere and could become a valuable tool for environmental monitoring and management (e.g., as an early warning system for environmental stress in seagrass meadows).
Author Contributions
VVS, KEB, MK, and KK developed the concept for this review. VVS wrote the manuscript with input from all co-authors. All authors approved the submitted version.
Funding
We acknowledge financial support by the Danish National Research Foundation (DNRF136 to VVS), the Grundfos Foundation (KK), the Independent Research Fund Denmark (DFF-8048-00057B to KK), (DFF-8022-00301B and DFF-8021-00308B to MK), and the Villum Foundation (00028156 to KEB).
Conflict of Interest
The authors declare that the research was conducted in the absence of any commercial or financial relationships that could be construed as a potential conflict of interest.
Publisher’s Note
All claims expressed in this article are solely those of the authors and do not necessarily represent those of their affiliated organizations, or those of the publisher, the editors and the reviewers. Any product that may be evaluated in this article, or claim that may be made by its manufacturer, is not guaranteed or endorsed by the publisher.
Acknowledgments
Lars Peter Nielsen and Nils Risgaard-Petersen are thank for discussions about potential novel directions in seagrass research, as well as Silvia Zieger and Fabian Steininger for discussions about the figure design. We also acknowledge the input from Belinda C. Martin in regard to the potential root hair breakage during seagrass transplantation.
Supplementary Material
The Supplementary Material for this article can be found online at: https://www.frontiersin.org/articles/10.3389/fmars.2021.771382/full#supplementary-material
References
Aller, R. C., Aller, J. Y., Zhu, Q., Heilbrun, C., Klingensmith, I., and Kaushik, A. (2019). Worm tubes as conduits for the electrogenic microbial grid in marine sediments. Sci. Adv. 5:eaaw3651. doi: 10.1126/sciadv.aaw3651
Askaer, L., Elberling, B., Glud, R. N., Kühl, M., Lauritsen, F. R., and Joensen, H. P. (2010). Soil heterogeneity effects on O2 distribution and CH4 emissions from wetlands: in situ and mesocosm studies with planar O2 optodes and membrane inlet mass spectrometry. Soil Biol. Biochem. 42, 2254–2265. doi: 10.1016/j.soilbio.2010.08.026
Barnabas, A. D. (1996). Casparian band-like structures in the root hypodermis of some aquatic angiosperms. Aquat. Bot. 55, 217–225. doi: 10.1016/S0304-3770(96)01072-8
Bennett, W. W., Teasdale, P. R., Welsh, D. T., Panther, J. G., and Jolley, D. F. (2012). Optimization of colorimetric DET technique for the in situ, two-dimensional measurement of iron(II) distributions in sediment porewaters. Talanta 88, 490–495. doi: 10.1016/j.talanta.2011.11.020
Berg, P., Risgaard-Petersen, N., and Rysgaard, S. (1998). Interpretation of measured concentration profiles in sediment pore water. Limnol. Oceanogr. 43, 1500–1510. doi: 10.4319/lo.1998.43.7.1500
Bertelli, C. M., and Unsworth, R. K. F. (2014). Protecting the hand that feeds us: seagrass (Zostera marina) serves as commercial juvenile fish habitat. Mar. Pollut. Bull. 83, 425–429. doi: 10.1016/j.marpolbul.2013.08.011
Blaabjerg, V., Mouritsen, K. N., and Finster, K. (1998). Diel cycles of sulphate reduction rates in sediments of a Zostera marina bed (Denmark). Aquat. Microb. Ecol. 15, 97–102. doi: 10.3354/ame015097
Borovec, O., and Vohník, M. (2018). Ontogenetic transition from specialized root hairs to specific root-fungus symbiosis in the dominant Mediterranean seagrass Posidonia oceanica. Sci. Rep. 8:10773. doi: 10.1038/s41598-018-28989-4
Borum, J., Pedersen, O., Greve, T., Frankovich, T., Zieman, J., Fourqurean, J. W., et al. (2005). The potential role of plant oxygen and sulphide dynamics in die-off events of the tropical seagrass, Thalassia testudinum. J. Ecol. 93, 148–158. doi: 10.1111/j.1365-2745.2004.00943.x
Brodersen, K. E., Hammer, K. J., Schrameyer, V., Floytrup, A., Rasheed, M. A., Ralph, P. J., et al. (2017a). Sediment resuspension and deposition on seagrass leaves impedes internal plant aeration and promotes phytotoxic H2S intrusion. Front. Plant Sci. 8:657. doi: 10.3389/fpls.2017.00657
Brodersen, K. E., Koren, K., Moßhammer, M., Ralph, P. J., Kühl, M., and Santner, J. (2017b). Seagrass-mediated phosphorus and iron solubilization in tropical sediments. Environ. Sci. Technol. 51, 14155–14163. doi: 10.1021/acs.est.7b03878
Brodersen, K. E., Koren, K., Lichtenberg, M., and Kühl, M. (2016). Nanoparticle-based measurements of pH and O2 dynamics in the rhizosphere of Zostera marina L.: effects of temperature elevation and light-dark transitions. Plant Cell Environ. 39, 1619–1630. doi: 10.1111/pce.12740
Brodersen, K. E., Kühl, M., Nielsen, D. A., Pedersen, O., and Larkum, A. W. D. (2018a). “Rhizome, root/sediment interactions, aerenchyma and internal pressure changes in seagrasses,” in Seagrasses of Australia: Structure, Ecology and Conservation, eds A. W. D. Larkum, G. A. Kendrick, and P. J. Ralph (Cham: Springer International Publishing), 393–418.
Brodersen, K. E., Siboni, N., Nielsen, D. A., Pernice, M., Ralph, P. J., Seymour, J., et al. (2018b). Seagrass rhizosphere microenvironment alters plant-associated microbial community composition. Environ. Microbiol. 20, 2854–2864. doi: 10.1111/1462-2920.14245
Brodersen, K. E., Kühl, M., Trampe, E., and Koren, K. (2020). Imaging O2 dynamics and microenvironments in the seagrass leaf phyllosphere with magnetic optical sensor nanoparticles. Plant J. 104, 1504–1519. doi: 10.1111/tpj.15017
Brodersen, K. E., Nielsen, D. A., Ralph, P. J., and Kühl, M. (2014). A split flow chamber with artificial sediment to examine the below-ground microenvironment of aquatic macrophytes. Mar. Biol. 161, 2921–2930. doi: 10.1007/s00227-014-2542-3
Brodersen, K., Lichtenberg, M., Paz, L.-C., and Kühl, M. (2015a). Epiphyte-cover on seagrass (Zostera marina L.) leaves impedes plant performance and radial O2 loss from the below-ground tissue. Front. Mar. Sci. 2:58. doi: 10.3389/fmars.2015.00058
Brodersen, K. E., Nielsen, D. A., Ralph, P. J., and Kühl, M. (2015b). Oxic microshield and local pH enhancement protects Zostera muelleri from sediment derived hydrogen sulphide. New Phytol. 205, 1264–1276. doi: 10.1111/nph.13124
Brodersen, K. E., Trevathan-Tackett, S. M., Nielsen, D. A., Connolly, R. M., Lovelock, C. E., Atwood, T. B., et al. (2019). Oxygen consumption and sulfate reduction in vegetated coastal habitats: effects of physical disturbance. Front. Mar. Sci. 6:14. doi: 10.3389/fmars.2019.00014
Colmer, T. D. (2003). Long-distance transport of gases in plants: a perspective on internal aeration and radial oxygen loss from roots. Plant Cell Environ. 26, 17–36. doi: 10.1046/j.1365-3040.2003.00846.x
Davison, W., Fones, G., Harper, M., Teasdale, P., and Zhang, H. (2000). “Dialysis, DET and DGT: in situ diffusional techniques for studying water, sediments and soils,” in In Situ Monitoring of Aquatic Systems: Chemical Analysis and Speciation, eds J. Buffle and G. Horvai (New York, NY: John Wiley & Sons), 495–569.
Duarte, C. M., Middelburg, J. J., and Caraco, N. (2005). Major role of marine vegetation on the oceanic carbon cycle. Biogeosciences 2, 1–8. doi: 10.5194/bg-2-1-2005
Edward, C. T., and Mark, S. F. (1998). Bioturbation as a potential mechanism influencing spatial heterogeneity of North Carolina seagrass beds. Mar. Ecol. Prog. Ser. 169, 123–132. doi: 10.3354/meps169123
Erftemeijer, P. L. A., and Robin Lewis, R. R. (2006). Environmental impacts of dredging on seagrasses: a review. Mar. Pollut. Bull. 52, 1553–1572. doi: 10.1016/j.marpolbul.2006.09.006
Fischer, J. P., and Koop-Jakobsen, K. (2012). The multi fiber optode (MuFO): a novel system for simultaneous analysis of multiple fiber optic oxygen sensors. Sens. Actuators B Chem. 168, 354–359. doi: 10.1016/j.snb.2012.04.034
Fonseca, M. S., and Cahalan, J. A. (1992). A preliminary evaluation of wave attenuation by four species of seagrass. Estuar. Coast. Shelf Sci. 35, 565–576. doi: 10.1016/S0272-7714(05)80039-3
Fourqurean, J. W., Duarte, C. M., Kennedy, H., Marbà, N., Holmer, M., Mateo, M. A., et al. (2012). Seagrass ecosystems as a globally significant carbon stock. Nat. Geosci. 5, 505–509. doi: 10.1038/ngeo1477
Frederiksen, M. S., and Glud, R. N. (2006). Oxygen dynamics in the rhizosphere of Zostera marina: a two-dimensional planar optode study. Limnol. Oceanogr. 51, 1072–1083. doi: 10.4319/lo.2006.51.2.1072
Garcias-Bonet, N., and Duarte, C. M. (2017). Methane production by seagrass ecosystems in the red sea. Front. Mar. Sci. 4:340. doi: 10.3389/fmars.2017.00340
Glud, R. N., Tengberg, A., Kühl, M., Hall, P. O. J., and Klimant, I. (2001). An in situ instrument for planar O2 optode measurements at benthic interfaces. Limnol. Oceanogr. 46, 2073–2080. doi: 10.4319/lo.2001.46.8.2073
Glud, R. N., Wenzhöfer, F., Tengberg, A., Middelboe, M., Oguri, K., and Kitazato, H. (2005). Distribution of oxygen in surface sediments from central Sagami Bay, Japan: in situ measurements by microelectrodes and planar optodes. Deep Sea Res. I Oceanogr. Res. Pap. 52, 1974–1987. doi: 10.1016/j.dsr.2005.05.004
Herschend, J., Koren, K., Røder, H. L., Brejnrod, A., Kühl, M., Burmølle, M., et al. (2018). In vitro community synergy between bacterial soil isolates can be facilitated by pH stabilization of the environment. Appl. Environ. Microbiol. 84, e01450–18. doi: 10.1128/AEM.01450-18
Holmer, M., and Bondgaard, E. J. (2001). Photosynthetic and growth response of eelgrass to low oxygen and high sulfide concentrations during hypoxic events. Aquat. Bot. 70, 29–38. doi: 10.1016/S0304-3770(00)00142-X
Huang, J., Franklin, H., Teasdale, P. R., Burford, M. A., Kankanamge, N. R., Bennett, W. W., et al. (2019). Comparison of DET, DGT and conventional porewater extractions for determining nutrient profiles and cycling in stream sediments. Environ. Sci. Process. Impacts 21, 2128–2140. doi: 10.1039/C9EM00312F
Jensen, S. I., Kühl, M., and Priemé, A. (2007). Different bacterial communities associated with the roots and bulk sediment of the seagrass Zostera marina. FEMS Microbiol. Ecol. 62, 108–117. doi: 10.1111/j.1574-6941.2007.00373.x
Jensen, S. I., Kühl, M., Glud, R. N., Jørgensen, L. B., and Priemé, A. (2005). Oxic microzones and radial oxygen loss from roots of Zostera marina. Mar. Ecol. Prog. Ser. 293, 49–58. doi: 10.3354/meps293049
Jeroschewski, P., Steuckart, C., and Kühl, M. (1996). An amperometric microsensor for the determination of H2S in aquatic environments. Anal. Chem. 68, 4351–4357. doi: 10.1021/ac960091b
Jézéquel, D., Brayner, R., Metzger, E., Viollier, E., Prévot, F., and Fiévet, F. (2007). Two-dimensional determination of dissolved iron and sulfur species in marine sediment pore-waters by thin-film based imaging. Thau lagoon (France). Estuar. Coast. Shelf Sci. 72, 420–431. doi: 10.1016/j.ecss.2006.11.031
Jovanovic, Z., Pedersen, M., Larsen, M., Kristensen, E., and Glud, R. N. (2015). Rhizosphere O2 dynamics in young Zostera marina and Ruppia maritima. Mar. Ecol. Prog. Ser. 518, 95–105. doi: 10.3354/meps11041
Kankanamge, N. R, Bennett, W. W., Teasdale, P. R., Huang, J., and Welsh, D. T. (2017). Comparing in situ colorimetric DET and DGT techniques with ex situ core slicing and centrifugation for measuring ferrous iron and dissolved sulfide in coastal sediment pore waters. Chemosphere 188, 119–129. doi: 10.1016/j.chemosphere.2017.08.144
Kankanamge, N. R., Bennett, W. W., Teasdale, P. R., Huang, J., and Welsh, D. T. (2020). A new colorimetric DET technique for determining mm-resolution sulfide porewater distributions and allowing improved interpretation of iron(II) co-distributions. Chemosphere 244:125388. doi: 10.1016/j.chemosphere.2019.125388
Koop-Jakobsen, K., Fischer, J., and Wenzhöfer, F. (2017). Survey of sediment oxygenation in rhizospheres of the saltmarsh grass - Spartina anglica. Sci. Total Environ. 589, 191–199. doi: 10.1016/j.scitotenv.2017.02.147
Koop-Jakobsen, K., Mueller, P., Meier, R. J., Liebsch, G., and Jensen, K. (2018). Plant-sediment interactions in salt marshes–an optode imaging study of O2, pH, and CO2 gradients in the rhizosphere. Front. Plant Sci. 9:541. doi: 10.3389/fpls.2018.00541
Koren, K., and Kühl, M. (2018). “Optical O2 sensing in aquatic systems and organisms,” in Quenched-Phosphorescence Detection of Molecular Oxygen: Applications in Life Sciences, eds D. B. Papkovsky and R. I. Dmitriev (London: The Royal Society of Chemistry), 145–174.
Koren, K., and Zieger, S. E. (2021). Optode based chemical imaging—possibilities, challenges, and new avenues in multidimensional optical sensing. ACS Sensors 6, 1671–1680. doi: 10.1021/acssensors.1c00480
Koren, K., Brodersen, K. E., Jakobsen, S. L., and Kühl, M. (2015). Optical sensor nanoparticles in artificial sediments–a new tool to visualize O2 dynamics around the rhizome and roots of seagrasses. Environ. Sci. Technol. 49, 2286–2292. doi: 10.1021/es505734b
Koren, K., Moßhammer, M., Scholz, V. V., Borisov, S. M., Holst, G., and Kühl, M. (2019). Luminescence lifetime imaging of chemical sensors—a comparison between time-domain and frequency-domain based camera systems. Anal. Chem. 91, 3233–3238. doi: 10.1021/acs.analchem.8b05869
Kühl, M., and Revsbech, N. P. (2001). “Biogeochemical microsensors for boundary layer studies,” in The Benthic Boundary Layer: Transport Processes and Biogeochemistry, eds B. P. Boudreau and B. B. Jørgensen (Oxford: Oxford University Press), 180–210.
Larsen, M., Borisov, S. M., Grunwald, B., Klimant, I., and Glud, R. N. (2011). A simple and inexpensive high resolution color ratiometric planar optode imaging approach: application to oxygen and pH sensing. Limnol. Oceanogr. 9, 348–360. doi: 10.4319/lom.2011.9.348
Lenzewski, N., Mueller, P., Meier, R. J., Liebsch, G., Jensen, K., and Koop-Jakobsen, K. (2018). Dynamics of oxygen and carbon dioxide in rhizospheres of Lobelia dortmanna – a planar optode study of belowground gas exchange between plants and sediment. New Phytol. 218, 131–141. doi: 10.1111/nph.14973
Li, C., Ding, S., Yang, L., Zhu, Q., Chen, M., Tsang, D. C. W., et al. (2019). Planar optode: a two-dimensional imaging technique for studying spatial-temporal dynamics of solutes in sediment and soil. Earth Sci. Rev. 197:102916. doi: 10.1016/j.earscirev.2019.102916
Lichtenberg, M., Nørregaard, R. D., and Kühl, M. (2017). Diffusion or advection? Mass transfer and complex boundary layer landscapes of the brown alga Fucus vesiculosus. J. R Soc. Interface 14:20161015. doi: 10.1098/rsif.2016.1015
Long, M. H., McGlathery, K. J., Zieman, J. C., and Berg, P. (2008). The role of organic acid exudates in liberating phosphorus from seagrass-vegetated carbonate sediments. Limnol. Oceanogr. 53, 2616–2626. doi: 10.4319/lo.2008.53.6.2616
Martin, B. C., Bougoure, J., Ryan, M. H., Bennett, W. W., Colmer, T. D., Joyce, N. K., et al. (2018). Oxygen loss from seagrass roots coincides with colonisation of sulphide-oxidising cable bacteria and reduces sulphide stress. ISME J. 13, 707–719. doi: 10.1038/s41396-018-0308-5
Marzocchi, U., Benelli, S., Larsen, M., Bartoli, M., and Glud, R. N. (2019). Spatial heterogeneity and short-term oxygen dynamics in the rhizosphere of Vallisneria spiralis: implications for nutrient cycling. Freshw. Biol. 64, 532–543. doi: 10.1111/fwb.13240
McGlathery, K. J., Berg, P., and Marino, R. (2001). Using porewater profiles to assess nutrient availability in seagrass-vegetated carbonate sediments. Biogeochemistry 56, 239–263. doi: 10.1023/A:1013129811827
Mcleod, E., Chmura, G. L., Bouillon, S., Salm, R., Björk, M., Duarte, C. M., et al. (2011). A blueprint for blue carbon: toward an improved understanding of the role of vegetated coastal habitats in sequestering CO2. Front. Ecol. Environ. 9:552–560. doi: 10.1890/110004
Merl, T., and Koren, K. (2020). Visualizing NH3 emission and the local O2 and pH microenvironment of soil upon manure application using optical sensors. Environ. Int. 144:106080. doi: 10.1016/j.envint.2020.106080
Moßhammer, M., Scholz, V. V., Holst, G., Kühl, M., and Koren, K. (2019b). Luminescence lifetime imaging of O2 with a frequency-domain-based camera system. J. Vis. Exp. 154:e60191. doi: 10.3791/60191
Moßhammer, M., Brodersen, K. E., Kühl, M., and Koren, K. (2019a). Nanoparticle- and microparticle-based luminescence imaging of chemical species and temperature in aquatic systems: a review. Microchim. Acta 186:126. doi: 10.1007/s00604-018-3202-y
Moßhammer, M., Strobl, M., Kühl, M., Klimant, I., Borisov, S. M., and Koren, K. (2016). Design and application of an optical sensor for simultaneous imaging of pH and dissolved O2 with low cross-talk. ACS Sensors 1, 681–687. doi: 10.1021/acssensors.6b00071
Nielsen, L. B., Finster, K., Welsh, D. T., Donelly, A., Herbert, R. A., De Wit, R., et al. (2001). Sulphate reduction and nitrogen fixation rates associated with roots, rhizomes and sediments from Zostera noltii and Spartina maritima meadows. Environ. Microbiol. 3, 63–71. doi: 10.1046/j.1462-2920.2001.00160.x
Olsen, Y. S., Fraser, M. W., Martin, B. C., Pomeroy, A., Lowe, R., Pedersen, O., et al. (2018). In situ oxygen dynamics in rhizomes of the seagrass Posidonia sinuosa: impact of light, water column oxygen, current speed and wave velocity. Mar. Ecol. Prog. Ser. 590, 67–77. doi: 10.3354/meps12477
Orth, R. J., Carruthers, T. J. B., Dennison, W. C., Duarte, C. M., Fourqurean, J. W., Heck, K. L., et al. (2006). A global crisis for seagrass ecosystems. BioScience 56, 987–996.
Pagès, A., Welsh, D. T., Robertson, D., Panther, J. G., Schäfer, J., Tomlinson, R. B., et al. (2012). Diurnal shifts in co-distributions of sulfide and iron(II) and profiles of phosphate and ammonium in the rhizosphere of Zostera capricorni. Estuar. Coast. Shelf Sci. 115, 282–290. doi: 10.1016/j.ecss.2012.09.011
Pedersen, O., Revsbech, N. P., and Shabala, S. (2020). Microsensors in plant biology – in vivo visualization of inorganic analytes with high spatial and/or temporal resolution. J. Exp. Bot. 71, 3941–3954. doi: 10.1093/jxb/eraa175
Pfeffer, C., Larsen, S., Song, J., Dong, M., Besenbacher, F., Meyer, R. L., et al. (2012). Filamentous bacteria transport electrons over centimetre distances. Nature 491:218. doi: 10.1038/nature11586
Revsbech, N. P. (1989). An oxygen microsensor with a guard cathode. Limnol. Oceanogr. 34, 474–478. doi: 10.4319/lo.1989.34.2.0474
Revsbech, N. P. (2021). Simple sensors that work in diverse natural environments: the micro-Clark sensor and biosensor family. Sens. Actuators B Chem. 329:129168. doi: 10.1016/j.snb.2020.129168
Revsbech, N. P., and Jørgensen, B. B. (1986). “Microelectrodes: their use in microbial ecology,” in Advances in Microbial Ecology, ed. K. C. Marshall (Boston, MA: Springer), 293–352.
Rickelt, L. F., Askaer, L., Walpersdorf, E., Elberling, B., Glud, R. N., and Kühl, M. (2014). An optode sensor array for long-term in situ oxygen measurements in soil and sediment. J. Environ. Qual. 43:1821. doi: 10.2134/jeq2012.0334er
Robertson, D., Teasdale, P. R., and Welsh, D. T. (2008). A novel gel-based technique for the high resolution, two-dimensional determination of iron (II) and sulfide in sediment. Limnol. Oceanogr. Methods 6, 502–512. doi: 10.4319/lom.2008.6.502
Santner, J., Larsen, M., Kreuzeder, A., and Glud, R. N. (2015). Two decades of chemical imaging of solutes in sediments and soils – a review. Anal. Chim. Acta 878, 9–42. doi: 10.1016/j.aca.2015.02.006
Schäferling, M. (2012). The art of fluorescence imaging with chemical sensors. Angew. Chem. Int. Edn. 51, 3532–3554. doi: 10.1002/anie.201105459
Scholz, V. V., Martin, B. C., Meyer, R., Schramm, A., Fraser, M. W., Nielsen, L. P., et al. (2021). Cable bacteria at oxygen-releasing roots of aquatic plants: a widespread and diverse plant–microbe association. New Phytol. (in press). doi: 10.1111/nph.17415
Schrameyer, V., York, P. H., Chartrand, K., Ralph, P. J., Kühl, M., Brodersen, K. E., et al. (2018). Contrasting impacts of light reduction on sediment biogeochemistry in deep- and shallow-water tropical seagrass assemblages (Green Island, Great Barrier Reef). Mar. Environ. Res. 136, 38–47. doi: 10.1016/j.marenvres.2018.02.008
Simpson, A. G., Tripp, L., Shull, D. H., and Yang, S. (2018). Effects of Zostera marina rhizosphere and leaf detritus on the concentration and distribution of pore-water sulfide in marine sediments. Estuar. Coast. Shelf Sci. 209, 160–168. doi: 10.1016/j.ecss.2018.05.024
Sogin, E. M., Michellod, D., Gruber-Vodicka, H., Bourceau, P., Geier, B., Meier, D. V., et al. (2021). Sugars dominate the seagrass rhizosphere. bioRxiv [Preprint]. doi: 10.1101/797522
Staal, M., Prest, E. I., Vrouwenvelder, J. S., Rickelt, L. F., and Kühl, M. (2011). A simple optode based method for imaging O2 distribution and dynamics in tap water biofilms. Water Res. 45, 5027–5037. doi: 10.1016/j.watres.2011.07.007
Teasdale, P. R., Hayward, S., and Davison, W. (1999). In situ, high-resolution measurement of dissolved sulfide using diffusive gradients in thin films with computer-imaging densitometry. Anal. Chem. 71, 2186–2191. doi: 10.1021/ac981329u
Toshihiro, M., Masahiro, S., Yu, U., and Isao, K. (2001). Microbiological nitrogen transformation in carbonate sediments of a coral-reef lagoon and associated seagrass beds. Mar. Ecol. Prog. Ser. 217, 273–286. doi: 10.3354/meps217273
Trevathan-Tackett, S. M., Seymour, J. R., Nielsen, D. A., Macreadie, P. I., Jeffries, T. C., Sanderman, J., et al. (2017). Sediment anoxia limits microbial-driven seagrass carbon remineralization under warming conditions. FEMS Microbiol. Ecol. 93:fix033. doi: 10.1093/femsec/fix033
Wang, L., English, M. K., Tomas, F., Mueller, R. S., and Cann, I. (2021). Recovery and community succession of the Zostera marina rhizobiome after transplantation. Appl. Environ. Microbiol. 87, e02326–20. doi: 10.1128/AEM.02326-20
Waycott, M., Duarte, C. M., Carruthers, T. J. B., Orth, R. J., Dennison, W. C., Olyarnik, S., et al. (2009). Accelerating loss of seagrasses across the globe threatens coastal ecosystems. Proc. Natl. Acad. Sci. U.S.A. 106, 12377–12381. doi: 10.1073/pnas.0905620106
Wetzel, R. G., and Penhale, P. A. (1979). Transport of carbon and excretion of dissolved organic carbon by leaves and roots/rhizomes in seagrasses and their epiphytes. Aquat. Bot. 6, 149–158. doi: 10.1016/0304-3770(79)90058-5
Williams, P. N., Santner, J., Larsen, M., Lehto, N. J., Oburger, E., Wenzel, W., et al. (2014). Localized flux maxima of arsenic, lead, and iron around root apices in flooded lowland rice. Environ. Sci. Technol. 48, 8498–8506. doi: 10.1021/es501127k
Yin, H., Aller, R. C., Zhu, Q., and Aller, J. Y. (2021b). The dynamics of cable bacteria colonization in surface sediments: a 2D view. Sci. Rep. 11:7167. doi: 10.1038/s41598-021-86365-1
Yin, H., Aller, R. C., Zhu, Q., and Aller, J. Y. (2021a). Biogenic structures and cable bacteria interactions: redox domain residence times and the generation of complex pH distributions. Mar. Ecol. Prog. Ser. 669, 51–63. doi: 10.3354/meps13722
Keywords: planar-optode, microsensor, rhizosphere, geochemistry, imaging, multidimensional, DGT, DET
Citation: Scholz VV, Brodersen KE, Kühl M and Koren K (2021) Resolving Chemical Gradients Around Seagrass Roots—A Review of Available Methods. Front. Mar. Sci. 8:771382. doi: 10.3389/fmars.2021.771382
Received: 06 September 2021; Accepted: 08 October 2021;
Published: 29 October 2021.
Edited by:
Lars Wörmer, University of Bremen, GermanyReviewed by:
Marshall Wayne Bowles, Louisiana Universities Marine Consortium, United StatesKetil Koop-Jakobsen, Alfred Wegener Institute Helmholtz Centre for Polar and Marine Research (AWI), Germany
Copyright © 2021 Scholz, Brodersen, Kühl and Koren. This is an open-access article distributed under the terms of the Creative Commons Attribution License (CC BY). The use, distribution or reproduction in other forums is permitted, provided the original author(s) and the copyright owner(s) are credited and that the original publication in this journal is cited, in accordance with accepted academic practice. No use, distribution or reproduction is permitted which does not comply with these terms.
*Correspondence: Vincent V. Scholz, dmluY2VudC5zY2hvbHpAYmlvLmF1LmRr; Klaus Koren, a2xhdXMua29yZW5AYmlvLmF1LmRr