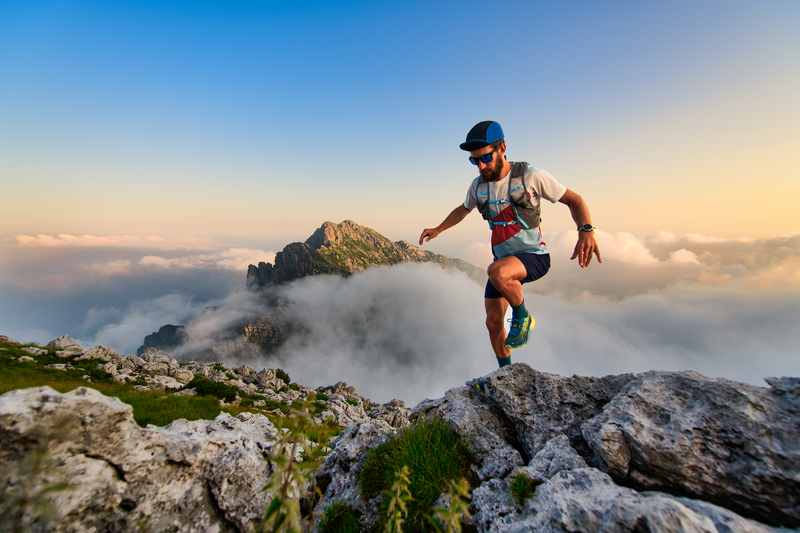
94% of researchers rate our articles as excellent or good
Learn more about the work of our research integrity team to safeguard the quality of each article we publish.
Find out more
ORIGINAL RESEARCH article
Front. Mar. Sci. , 07 February 2022
Sec. Marine Fisheries, Aquaculture and Living Resources
Volume 8 - 2021 | https://doi.org/10.3389/fmars.2021.770805
This article is part of the Research Topic How Overfishing Handicaps Resilience of Marine Resources Under Climate Change View all 10 articles
Previous studies have shown that multiple-environmental stressors are expected to have significant and geographically differential impacts on the health and abundance of marine species. In this paper, we analyze the combined impacts of ocean warming, overfishing and mercury pollution in European waters by projecting the impacts of climatic and non-climate drivers on marine species in European waters. Our findings suggest that the impacts vary widely depending on different species and their mean temperature tolerance (MTT). We find for instance, that more than 5 temperate benthopelagic species including, bobtail squids (Sepiida) frogfishes (Lophius) great Atlantic scallop (Pecten maximus) red mullet (Mullus barbatus barbatus) and common octopus (Octopus vulgaris) are affected (i.e., weakens their resilience to climate change) by the increase in sea surface temperature (SST) under RCP 8.5 in 2050 and 2100. Mercury contamination was estimated to increase in some species (e.g., ∼50% in swordfish), exceeding mercury consumption guideline thresholds (>1 mg/kg). This negative impact may limit the capacity of fisheries and marine ecosystem to respond to the current climate induced pollution sensitivity. An implication of our study is that the international community should strengthen a global ban on mercury emissions under the mandate of the Minamata Convention, comparable to the United Nations framework for persistent organic pollutant emission sources. Ongoing global efforts aimed at minimizing carbon footprint and mercury emissions need to be enhanced in concert with a reduction in fishing intensity to maintain effective conservation measures that promote increased resilience of fisheries to climate change and other stressors.
Marine ecosystems and biodiversity provide important and valuable goods and services such as food, amenity benefits, tourism, and carbon sink, but a myriad of anthropogenic activities have also altered and are changing the biogeochemistry and biophysics of the oceans, affecting marine species through direct and indirect impacts (Lotze et al., 2006; McCauley et al., 2015; Halpern et al., 2019). In most parts of the world, overfishing, anthropogenic climate change and pollution are already having quantifiable effects on the marine ecosystem, and their implications for the future are of great concern. As stated by the Intergovernmental Panel on Climate Change (IPCC), human influence on the climate system is clear, and anthropogenic carbon dioxide (CO2) emissions have impacted on the marine environment at unprecedented levels (IPCC, 2014, 2018, 2021).
Particularly, the ocean, the marine species biodiversity it holds and the fisheries they sustain are facing many threats, e.g., ocean pollution by chemical assaults [e.g., plastics, persistent organic pollutants (POPs), and mercury (methyl-mercury, MeHg)]; (e.g., Alava et al., 2017a, 2018; Schartup et al., 2019; Issifu and Sumaila, 2020; National Academies of Sciences, Engineering, and Medicine, 2021), global climate change (Noone et al., 2013; IPCC, 2018, 2021), and overfishing (Pauly et al., 1998, 2005; Rogers and Laffoley, 2011; Sumaila et al., 2011; McCauley et al., 2015). Researchers have observed that the interaction of most of these stressors in the ocean is damaging the health of marine wildlife, and reducing fisheries quality and quantity (McCauley et al., 2015; Alava et al., 2017a; Halpern et al., 2019; Schartup et al., 2019).
There have been some decades of individual studies on climate change events, pollution, and overfishing. However, it is only recently that linkages and combined impacts of these previously dispersed anthropogenic stressors are being established to holistically adapt to risk management in fisheries (Booth and Zeller, 2005; Noone et al., 2013; Schartup et al., 2019). For example, the ongoing Nippon Foundation-Nexus Program,1 and Stockholm Resilience Center2 aspire to address some of the challenges of ocean health (Leape et al., 2021).
This study offers conceptual framework and assess the weight of evidence of overfishing, marine pollution and climate change interactions. We argue that reducing pollution and overfishing is also climate actions. Therefore, the overarching goal of this paper includes: (1) review the combined impacts of multiple stressors on European fisheries; (2) examine the interactive impacts of multiple anthropogenic stressors (i.e., overfishing, climate change and ocean pollution) on fisheries; (3) investigate top fish species in the European waters that are vulnerable to the onslaught of climatic and non-climate stressors; (4) explore management policy options to address the impacts of climate change, overfishing and pollution on marine ecosystems.
The expansion of fisheries and overfishing inflict changes in the community structure and fish size because of selective harvesting of target species and bycatch of non-target species, as well as via habitat modification, triggering changes in the biomass, species composition and size structure (Pauly et al., 1998; Bianchi et al., 2000; Jennings and Blanchard, 2004). According to FAO (2012), 87% of global fish stocks are either overexploited or fully exploited. The status of other species, such as brown shrimp is uncertain, while others are classified as underexploited (e.g., yellowfin tuna, Tunnus albacares). Recent estimates indicate that between 40 and 70% of fish stocks in European waters are currently at an unsustainable level—either overfished or at their lower biomass limits (Dulvy et al., 2003; Sumaila and Tai, 2020). European stock assessments report that the current size and capacity of the European Union (EU) fleet is estimated to be 2–3 times above the sustainable level in a number of fisheries (European Commission, 2008). Several offshore fisheries capture species in European waters are classified as fully exploited; e.g., herring, Norway lobster, mackerel, and horse mackerel (STECF, 2017).
Illegal fishing targeting tuna and other tuna fish species in West Africa (Sumaila, 2018; EJF, 2020) and the eastern tropical Pacific (Alava et al., 2015, 2017b; Martínez-Ortiz et al., 2015; Alava and Paladines, 2017), are exported to EU markets (Ministerio de Comercio Exterior, 2017; EJF, 2020; Monnier et al., 2020). On average, for example, the value of exports canned tuna and tuna loins from Ecuador to the EU over the 2007–2016 period accounted for 343 million USD and 124 million USD, respectively (Ministerio de Comercio Exterior, 2017). While the EU strictly regulates and supervises certified fish products and exports from fisheries overseas to mitigate and prevent illegal, unreported and unregulated (IUU) fishing, questions linger as to whether illegally harvested tuna exports are reaching the EU fish market chains.
It is important to note that this sobering statistic only considers individual stocks that are deemed commercially valuable and does not consider the amount of environmental degradation and ecosystem destruction that accompanies overfishing. The FAO also estimates that “oceans are cleared at twice the rate of forests” (FAO, 2009). Overfishing can be defined as fishing down marine food web (Pauly et al., 1998, 2005) or depleting populations due to excessive fishing mortality and defaunation (McCauley et al., 2015; Baum and Fuller, 2016). To put the deleterious impact of excessive fishing into perspective, the European hake (Merluccius merluccius) stocks are among the fish species under more intense overfishing, with fishing mortality rates up to 10 times higher than the optimal target (STECF, 2017). Overfishing pose one of the greatest threats to ocean health (Pauly et al., 1998, 2005; McCauley et al., 2015). Apart from depleting populations (STECF, 2017), overfishing can erode the age and size structure and spatial distribution of stocks making populations more susceptible to environmental fluctuations. This is particularly relevant for highly impacted areas and vulnerable species (e.g., elasmobranchs). Overfishing of top predators and pelagic resources has also been associated with trophic cascades and ecosystem regime shifts in the Black Sea (Daskalov et al., 2017). A combination of climate-related stresses and widespread over-exploitation of fisheries reduces the scope for adaptation and increases risks of stock collapse (Allison et al., 2009). Overfishing makes marine fisheries production more vulnerable to ocean warming by compromising the resilience of many marine species to climate change, and continued warming will hinder efforts to rebuild overfished populations (Free et al., 2019). It can also exacerbate the mercury levels in some fish species. For instance, recent studies show that Pacific salmon, squid and forage fish, as well as Atlantic bluefin tuna and Atlantic cod and other fish species are susceptible to increases in methylmercury (MeHg) due to overfishing (Schartup et al., 2019) and rising ocean temperatures (Alava et al., 2018). Overfishing weakens the resilience of fish stocks and marine ecosystems to climate change (Sumaila and Tai, 2020), and has even been identified as one of the greatest threats to ocean health (Pauly et al., 2005; Halpern et al., 2015; Gattuso et al., 2018). Indeed, after the collapse of northern cod stocks in Canada due to overfishing, Newfoundland and other Canadian coastal areas changed to shellfish, shrimp and crab which dominates the industry today. This transition is known as fishing down the food web and is usually the result of unsustainable fishing practices (Pauly et al., 1998).
Overfishing is linked directly to multiple destructive fishing practices such as trawling, IUU fishing, bycatch, and harmful subsidies (Sumaila et al., 2006, 2021; Agnew et al., 2009; Moomaw and Blankenship, 2014). Continued use of destructive fishing practices such as bottom trawling, which has an impact on both targeted and non-targeted species and damages ocean sea floors, may lead to overfishing. In addition to this, overfishing often correlates with large amounts of bycatch as increased effort is translated directly into unintentionally catching non-targeted species which harms marine ecosystem. Also, harmful subsidies encourage overfishing by supporting fleets that are over capacity in terms of number of ships, effort and technology (Schuhbauer et al., 2017; Sumaila et al., 2021).
In the near future, climate-driven phenomenon including deoxygenation and ocean acidification, are likely to have a growing effect on the productivity of global fisheries. Recent studies have observed fundamental changes to ocean biogeochemistry, including rising sea surface and bottom temperatures, changes in primary production, reduced pH, decreased subsurface oxygen levels (i.e., hypoxia) in coastal waters (Bindoff et al., 2019). Most of these anthropogenic disturbances are linked to fossil fuel emissions and fertilizer use, which is expected to increase in the years to come, placing further pressures on marine ecosystem (Doney, 2010).
Globally, rising sea temperature will likely shift the location, distribution and abundance of marine fisheries. In fact, Cheung et al. (2010) demonstrated that fisheries in some regions stand to gain from climate change (“winners”), while others stand to lose (“losers”). Their study estimates that the average catch potential in high-latitude regions will increase by 30–70% by 2055, benefiting countries such as Norway, Greenland, and Russia. On the other hand, average catch potential in the tropics is projected to drop by 40% by 2055, resulting in substantial losses for countries such as Indonesia, Chile, and China (Cheung et al., 2010). In effect, shifts in species distributions can create incentives for overharvest. For example, a country that is losing a fishery due to climate change may overfish the target species to compensate for the anticipated loss.
In addition to rising temperatures, rising atmospheric CO2 levels pose a major threat to the ocean and fisheries resources. In general, alterations to ocean chemistry hinders the ability of a wide range of marine organisms such as corals, mollusks, and some plankton to grow and maintain external calcium carbonate skeletons (Orr et al., 2005). As a result, declining fisheries harvests are expected once ocean chemistry moves outside the present range of natural variability, which is expected to occur as early as 2025 in some regions of the Southern Pacific (Cooley et al., 2012). Already, high-trophic level large pelagic species such as salmon, tunas, billfish, and sharks, as well as the mid-trophic level small pelagic species such as sardines, anchovies, and squids are particularly sensitive to climate impacts (Chavez et al., 2003; Cheung et al., 2013).
In the European shelf seas, the impacts of climate change on fisheries have been noted for several important commercial species, notably nephrops, mussels, oysters, and lobster (e.g., Styf et al., 2013; Ostle et al., 2016). Fernandes et al. (2017) quantified the potential effects of ocean warming and acidification on fisheries catches, resulting revenues and employment in the United Kingdom of Great Britain and Northern Ireland under different greenhouse gas emission scenarios. Standing stock biomasses were projected to decrease significantly by 2050 and the main driver of this decrease was rising sea surface temperature (SST). The European waters account for about 14 and 15 percent of global carbon sink and fishing intensity, respectively (Cavan and Hill, 2020). In effect, losses in revenue were estimated to range between 1 and 21 percent in the short-term (2020–2050). For Europe as a whole, the annual impact was estimated to be over 1 billion USD by 2100 although subject to considerable uncertainty. Figure 1 shows that European seas are the most fished with the highest carbon sink.
Figure 1. European shelfregion (black rectangle) has one of the highest global fishing and catch areas and carbon sink intensity, especially the Northeast Atlantic (NEA). The NEA area is one of the highest-ranking areas, for both carbon sink and fishing intensity and responsible for about 14 and 15% of global carbon sink and global fishing effort, respectively (see Cavan and Hill, 2020). Map retrieved and modified from the Sea Around Us database (https://www.seaaroundus.org/data/#/spatial-catch; Pauly et al., 2020).
The proliferation of chemical pollutants (e.g., POPs, mercury) has become a prominent environmental issue in recent years, as growing evidence draws attention to its negative impacts on marine fisheries and food webs under the impact of climate change (Booth and Zeller, 2005; Alava et al., 2017a, 2018; Schartup et al., 2019). While evidence suggests that large amounts of emerging anthropogenic pollutants such as ocean macroplastics and microplastics (Eriksen et al., 2014; Jambeck et al., 2015; Lebreton et al., 2018; Alava, 2019; Issifu and Sumaila, 2020) are accumulating in the deep sea (Rochman et al., 2014; Choy et al., 2019; Kane et al., 2020), mercury concentrations in the North Pacific Ocean are predicted to double by 2050 (Sunderland et al., 2009). Small-scale gold mining, coal and fossil fuel burning and industrial emissions are the major contributors of mercury into oceans (Mason and Sheu, 2002; European Environment Agency, 2018). The global spread of mercury and other industrial pollutants is of immediate concern, as these pollutants bioaccumulate in the tissues of marine organisms and are passed up the food chain, posing a serious threat to human health. Additionally, methylation of mercury to form MeHg has been found to increase when temperatures rise (Johnson et al., 2016). The effect of these perturbations on global fisheries is only projected to grow as industrial activity and fertilizer use increases over the next two decades (Doney, 2010).
In the European waters, pollution is particularly important in the Black Sea, where the ecosystem has undergone different phases of eutrophication caused by increased input of nutrients, intensive farming and the use of agrochemicals and phosphate detergents. It is also noteworthy that the Black Sea and particularly the Mediterranean are hotspots for plastic and mercury pollution. Fishes absorb contaminants directly from the water and sediment and indirectly through food web transport. Higher concentration of mercury on several important commercial fish species such as anglerfish, common sole, striped mullet, swordfish, mackerel, and cod have been documented (Storelli and Marcotrigiano, 2001). Though mercury concentrations vary widely by species and ocean. There are varying health impacts associated with mercury pollution in different fish species, but the primary consequence is lower reproductive success such as decreased spawning and increased embryo mortality leading to reduced reproductive success (Sandheinrich and Wiener, 2011), increased vulnerability due to reproductive and neurological problems, which can lead to behavioral abnormalities (Dawson, 1982). In addition, elevated mercury levels have altered hormone profiles, indicating that the mercury is also affecting the health of the fish themselves (National Wildlife Federation, 2006), as well as the hatching times and the survival rates of offspring (Bridges et al., 2016) and ultimately death since fishes’ inability to survive extremely high levels of mercury (Matta et al., 2001). In effect, mercury pollution should be considered a stressor that reduces the resilience of fish assemblages to climate changes.
Fish consumption is known to have beneficial effects on human health due to its nutrients—the presence of long-chain, poly-unsaturated fatty acids (LC-PUFAs). For instance, provide protection against diseases such as coronary heart diseases, high blood pressure (Oomen et al., 2000; Miles and Calder, 2012; Rangel-Huerta et al., 2012). On the other hand, fish is the main dietary source of methylmercury for all age groups in Europe, given that many of the most popular species such as the hake, swordfish, whiting and cod are among those with the highest levels of mercury (EFSA, 2015). Substantial amount of methylmercury from the consumption of fish can have an effect on the nervous system, cardiovascular, immune and reproductive systems (Carta et al., 2003; Yokoo et al., 2003; Stern, 2005; Oken et al., 2008; Houston, 2011). Sandborgh-Englund et al. (1998) found that children exposed to mercury in the prenatal period had defects in attention, language, memory, and motor function. Children born in countries with high fish consumption, such as Portugal and Spain, received most exposure to methylmercury (Science for Environment Policy, 2017). Višnjevec et al. (2013) carried out a comprehensive Europe-based review of exposure to mercury, looking at studies published since 2000 and found out that the highest exposure to mercury was in coastal populations, due to their higher consumption of fish compared to inland residents.
Fish like all living organisms exhibits a temperature range within which they thrive. There are a number of approaches available for measuring the distribution of temperature for fish species. Here we calculated the temperature tolerance index (TTI), by using the average temperature preference range of our selected fish species. We estimated the percentage change in SST, as well as the bottom temperature in the 2050s/2100s under different climate change scenarios, using the Representative Concentrations Pathways (RCPs): RCP 2.6 (i.e., low CO2 emission/high mitigation scenario) and RCP8.5 (high CO2 emissions or business-as-usual). Data for SST and bottom temperature for RCP 2.6 and RCP 8.5 covers all European Exclusive Economic Zones (EEZs) and were retrieved from the NOAA’s Geophysical Fluid Dynamics Laboratory Earth System Model 2M (GFDL ESM2M; Dunne et al., 2012). We included 38 EEZs of 27 European countries in the European FAO region as presented in Supplementary Material.
To estimate TTI, the following three equations were derived. We expressed the mean temperature preference (MTP) for each species as follow
where TMAX and TMIN are maximum and minimum temperatures within the temperature preference range of each species i, respectively.
We estimated the change in temperature within the distribution range of each species using the following equation:
Let △T denotes the change in temperature within the distribution range for each fish species, SST2050 represents the SST in the year 2050 under RCP 2.6 or RCP 8.5, while SSTcurrents stands for the prevailing SST. We assume each species is living within its SST preference under the current status. The corresponding calculations were also done for 2100.
Finally, we calculated the TTI using mean temperature preference range (MTP) for species under each climate change scenario (i.e., RCP 2.6 and RCP 8.5) along with TMAX the upper limit temperature for a given fish species.
Based on Equation (3), we can check whether the projected change in temperature will exceed the highest temperature range of fish species or otherwise. The corresponding calculations were also done for 2100. We can infer whether the projected change in temperature will exceed the highest temperature range of fish species or otherwise. We assume that if the estimated (TTI) > 1, then the species cannot tolerate exposure and is affected by ocean warming due to climate change; if the estimated (TTI) = 1, no exposure to thermal stress or eco-physiological health effect. On the other hand, if (TTI) < 1, the projected SST is still within the temperature preference range of the species, which implies the species can still tolerate extreme SST anomalies and survive under climate change.
In an effort to capture the exposure risk to SST under climate change forcing (RCP 2.6 or RCP 8.5), a pragmatic ecotoxicological risk index to calculate the TTIER was also applied, using the mean temperature tolerance (MTT), as follows:
Where, TTIER denotes ecotoxicological risk index to estimate the TTI, SSTCC is a term to express the overall average of SST to reflect the climate change forcing based on SST predictions for RCP 2.6 (i.e., SSTRCP2.6) and RCP 8.5 (SSTRCP8.5) by 2050 and 2100, respectively. A major advantage of using MTT is that it captures the distribution of the temperatures (means and variability/SD) of a given species of fish in terms of the metabolic scope (e.g., oxygen consumption influenced by ambient sea surface or bottom temperature) and affected by changes in temperatures, i.e., ocean warming (Cheung et al., 2013).
The outcomes resulting from Equation (3) were also correlated against the MTT to explore the relationship between TTIER and MTT.
The EU represents the largest single market for fish and fish products in the world. Table 1 provides the breakdown of the top 20 taxa with the highest annual catch (tons) and landed values (USD) taken from the European waters by EU countries from 2007 to 2016. In 2015, the EU fishing fleet comprised of 63,976 active vessels, of which 74% were classified as small-scale coastal vessels, 25% as large-scale and remaining 1%, distant-water vessels. These EU fleets spent 4.8 million days at sea and consumed 2.3 billion liters of fuel to land over 5 million tons of seafood with a reported value of €7 billion (STECF, 2017). The EU fishing fleet operate in major sea basin including: North Sea and Eastern Arctic, Baltic Sea, North East Atlantic, Mediterranean and Black Sea, as well as fleets operating in other fishing regions, such as the Northwest Atlantic (STECF, 2017).
Table 1. List of top 20 fish species by average annual catch (tons) and landed values (USD) taken from European waters by EU countries between 2007 and 2016.
In terms of volume of catches, Atlantic herring was the most important species by annual catch (233 thousand tons), followed by European pilchard (188 thousand tons), Blue whiting (134 thousand tons) and European anchovy (117 thousand tons) as shown in Table 1. In terms of annual landed values, landings of European hake generated the most value (358USD million), followed by Norway lobster (281USD million), European anchovy (276 USD million), and Common sole (263 USD million).
Within the group of the top 20 marine taxa (Table 1 above) with the highest annual average landed values taken from the European waters by EU countries from 2007 to 2016, 99% of all fishes had mercury concentrations below the U.S. EPA human health criteria of 0.30 mg/kg wet weight (ww) (Table 2). This group of low mercury commercial fishes includes several commercially important marine species of European hake (Merluccius merluccius) and Great Atlantic scallop (Pecten maximus). Other notable low-mercury fish within this group of commercial fishes includes the Common shrimp (Crangon crangon), a widely distributed and commonly consumed fish across the North Sea and Eastern Arctic region (STECF, 2017). Swordfish (Xiphias gladius) from the Mediterranean also had elevated mercury concentrations (0.995 ± 0.539 mg/kg). In general, mercury levels are the lowest in smaller, mid-trophic or intermediate level pelagic species such as anchovies and always below (European Commission, 2002; World Health Organization and Food and Agriculture Organization of the United Nations, 2010) general guideline level of 0.5 and 1.0 mg/kg ww, respectively. Conversely, highest mercury concentrations were found in large high-trophic level pelagic species such as swordfish.
Table 2. Results from the preliminary analysis of mercury levels in commercial fish and shellfish species in European waters.
Based on Schartup et al. (2019), we estimated the increase in mercury concentration (ppm) by assuming the percent increase in concentrations predicted (i.e., mercury concentration increasing from > 30 to 50% at a temperature increase of 1°C under warming conditions) in fish species from the North Atlantic (e.g., Atlantic cod, Bluefin tuna) based on the data reported by Schartup et al. (2019), as follows:
Understanding average concentrations of mercury in fish and fish products by EU and WHO can help reduce mercury intake by consumers, including vulnerable populations like infants and young children as well as pregnant and breastfeeding mothers. Following fish-consumption advisories attributed to mercury (European Commission, 2002; U.S. EPA, 2002; World Health Organization and Food and Agriculture Organization of the United Nations, 2010) can help consumers make informed choices when choosing fish and fish products that are nutritious and safe to eat.
We assumed a conservative increase of 50% used for all pelagic fish (i.e., small and large pelagic fish); and for demersal or bottom fish an increase of 33% was used, based on the mercury concentration increase for Atlantic Cod reported in Schartup et al. (2019). We observed that mercury concentrations in our 20 fish species (except the predatory sword fish) were all below the established general fish consumption threshold by the EU (i.e., 0.5 mg/kg, 1.0 mg/kg predatory fish), U.S. EPA (i.e., 0.30 mg/kg, ww), and World Health Organization (WHO) (i.e., 0.50 mg/kg, ww).
In this study, we encountered a rich knowledge base about climate change via ocean warming, overfishing and pollution and its effects on European fisheries. We constructed the conceptual framework following the approaches of Alava et al. (2017a, 2018), Bindoff et al. (2019), and Schartup et al. (2019), in order to display the coherences of the interactions of climate change and environmental stressors to assess their impacts on fisheries. Figure 2 illustrates the interactions of climate change, overfishing and pollutants on marine fisheries and food webs.
Figure 2. Climate change-overfishing-pollution assessment framework. The pathways through which scenario of climatic, overfishing and pollutants hazards (orange, blue, and green boxes, respectively) and their interactions can lead to increases in exposure to hazards by the biota, ecosystems and people sensitivity (dashed gray box) and the risk of impacts to ecosystem and human health and societies (red box). Climate change is a threat multiplier that compounds overfishing and pollution. For instances, climate change induces fisheries susceptibility and vulnerability to overfishing; while overfishing and climate change influence pollution sensitivity; thus, the resilience of fish stocks and marine ecosystems can be weakened. The synthesis is based on literature review and framework presented in Alava et al. (2017a). Adapted from Alava et al. (2017a) and Bindoff et al. (2019).
Trends reported in Table 2 indicate that organisms with the highest mercury concentration are swordfish (1.495 mg/kg), followed by European seabass (0.25 mg/kg) and Atlantic herring (0.135 mg/kg). Comparing these trends with World Health Organization (WHO) and European Commission (EC) limits, the mercury levels are the lowest in smaller, short-lived fish and always below (European Commission, 2002; World Health Organization and Food and Agriculture Organization of the United Nations, 2010) general guideline level of 0.5 and 1.0 mg/kg ww, respectively (European Commission, 2002; World Health Organization and Food and Agriculture Organization of the United Nations, 2010). Conversely, highest mercury concentrations were found in large, long-lived species such as swordfish.
The average temperature of surface waters of European continental shelf areas such as the southern North Sea has experienced one of the greatest warming rates (Levitus et al., 2009; González-Taboada and Anadón, 2012). We explored the impacts of climate change including SST and bottom water temperature on future fisheries resilience. Figure 3 illustrates the relationship of the estimated TTI for all fish species assessed vs. the species-specific MTT under the strong mitigation scenario (RCP 2.6), in which there is a drastic reduction in global fossil fuel emissions, and under the business-as-usual scenario (RCP 8.5).
Figure 3. The relationship between the estimated TTI () for all fish species assessed vs. the species-specific MTT under RCP 2.6 (i.e., low CO2 emission/high mitigation scenario) for (A) 2050 and (B) 2100; and RCP 8.5 (high CO2 emissions or business-as-usual) for (C) 2050 and (D) 2100. The relationship between TTI and MTT under RCP 8.5 shows that most temperate pelagic and benthopelagic species will be affected either by high SST under RCP 8.5 by 2100 (D), as an indication of high sensitivity of exposure to ocean warming.
The relationships observed in Figure 3 shows positive correlations and significant linear regression between TTI and MTT under RCP 2.6 and RCP 8.5 by either 2050 or 2100 (r2 = 0.39, r = 0.62, p = 0.003; Figures 3A–D), projecting that both TTI and MTT increase, as well. Some benthopelagic species (i.e., Great Atlantic scallop, red mullet, cuttlefishes, bobtail squids, frogfishes; Figure 3D) exhibit TTI > 1 under RCP 8.5 by 2100, as an indication of high sensitivity and exposure to increasing SST.
Conversely, when applying the ecotoxicological risk index (i.e., TTI = SSTCC/MTT), the relationships observed in Figure 4 illustrate negative correlations, in which the TTI significantly decreases as the MTT increases in fish for both RCP 2.6 and RCP 8.5 scenarios by 2020 and 2100 (r = −0.97; p < 0.00001). These trends indicate that fish with higher MTT values (e.g., sword fish, gilthead seabream) are less impacted by and more tolerant to increasing changes of SST, while fish species (i.e., Atlantic cod; Atlantic herring) with lower MTT and higher TTI values are the most affected due to the exposure to ocean warming (i.e., RCP 8.5), appearing to be susceptible even under the mitigation or low emissions scenario (RCP 2.6), as shown in Figures 3A–D. The impact of SST under RCP 2.6 is relatively lower than under RCP 8.5 (Figure 3), with TTI increasing by an average of 5.0 and 35%, respectively, by 2050 and 2100 relative to current temperature 2001–2020. We found that bobtail squids, frogfishes, great Atlantic scallop, red mullet and common octopus will be affected by high SST under RCP 8.5 in 2050 and 2100 (i.e., potential impacts on abundance and distribution due to less resistant to changes in SST). Likewise, under the strong mitigation scenario (RCP 2.6), the impact of bottom sea temperature is lower than that under RCP 8.5, with TTI increasing by an average of 5.0 and 30%, respectively, by 2050 and 2100 relative to current temperature 2001–2020. In particular, based on the ecotoxicological risk index, these species exhibited a TTI > 1, ranging from 1.3 to 1.5 under RCP 2.6 and RCP 8.5, corroborating its lack of tolerance to ocean warming (Figure 4).
Figure 4. The relationship between the estimated TTI (TTI = SSTCC/MTT) for all fish species assessed vs. the species-specific MTT under RCP 2.6 (i.e., low CO2 emission/high mitigation scenario) for (A) 2050 and (B) 2100; and RCP 8.5 (high CO2 emissions or business-as-usual) for (C) 2050 and (D) 2100. The relationship between TTI and MTT under RCP 8.5 shows that most temperate pelagic and benthopelagic species will be affected either by high SST under RCP 8.5 and even under the mitigation scenario (RCP 2.6), as an indication of high sensitivity of exposure to ocean warming.
The combined interaction of fishing pressure impacts in tandem with climate change exacerbating mercury biomagnification has been modeled in a foodwebs of the Faroe Islands (North Atlantic), involving a depleted fish stock and cetacean species, including the Atlantic cod (G. morhua) and long-finned pilot whale (Globicephala melas), resulting in high concerns for food safety and neurotoxic effects to human health because of the high consumption of mercury-contaminated fish and marine mammal meat (Booth and Zeller, 2005). More recently, this cumulative multiple-stressor interaction was corroborated by Schartup et al. (2019), uncovering that the combination of climate change and overfishing in depleted fish populations such as Atlantic cod (Gadus morhua) and bluefin tuna (Thunnus thynnus) further contaminate fish and exacerbate bioaccumulation of the neurotoxic MeHg in foodwebs. This has obvious implications for healthy marine ecosystems, but also for the public health of coastal communities strongly relying on seafoods.
The combined onslaught of ocean warming, overfishing and pollution on fisheries in European waters may have significant implications for fish distribution, food security, and livelihoods. Pollution, overfishing, and rising SST, among other anthropogenic pressures, put at risk future prospects for food security and nutrition, and resilient livelihoods in the longer term. For instance, overfishing results in overexploitation of fish stocks, threatening the health of the ecosystem and fish stocks while generating losses in fishers’ revenues, as well as a loss in socio-economic benefits such as food and nutritional security of people (Bondaroff et al., 2015; Sumaila et al., 2020). One major impact of climatic and non-climate stressors on fisheries is the changes in stock distributions, which affect where fish can be caught and who might catch them. These stressors might alter the conditions of marine ecosystems and the distributions of fish species across the oceans shift in response to climate change. This implies some traditional fisheries will move into new jurisdictions and those that cannot move fast enough perish. That means our results have significant implications to the decision makers for management risks and designing policies for sustainable fisheries. It is therefore crucial for us to incorporate the outputs from this study into to risk management and policy solution framework.
This projected changes in distribution are likely to exacerbate existing conflicts between stakeholders, both within nations and when the distribution of important species changes across boundaries between neighboring economies or between country EEZs and the high seas. For instance, the rapid northwards shift of Atlantic mackerel (Scomber scombrus) distribution from Norwegian waters to the waters of the Faroe Islands and Iceland led to conflict over allocations between the affected countries (Jensen et al., 2015).
Another devastating impact of environmental and anthropogenic stressors on fisheries is changes in stock productivity, which affect potential yields and profits. As an example, Lam et al. (2016) modeled the impacts of climate change on fish revenues through changes in the amount and composition of catches and found that global fishers’ revenues could drop by 35% due to decrease in catches by developing countries vessels operating in more severely impacted distant waters.
Our study has limitations. First, the equation TTI = (MTP+MTP*ΔT)/TMAX uses maximum temperature (TMAX) or the upper limit temperature tolerated by a given fish species as the denominator, in which the numerator is basically adjusted to the maximum temperature creating a maximum temperature-normalization of the fish data to produce a TTI with Equation (3). Second, while this application is fairly sound, an aspect to consider is that by using TMAX in this equation, we may well overestimate or generate over-projection in terms of the thermal capacity of a fish species to tolerate larger changes in SST (i.e., not all individuals of a population are metabolically and physiologically able to exhibit a TMAX and only a few or some, depending on the most temperature-tolerant individuals of the same species, which may well include outliers). Conversely, using the MTT will help to recognize and represent the species’ overall mean thermal tolerance. Doing so, the basic equation TTI = SSTCC/MTT, where SSTCC is a term to express the overall average of SST to reflect the climate change forcing based on the SST predictions for RCP 2.6 and RCP 8.5 by 2050 and 2100, may well be applied to compare both methods. Again, while the use of TMAX is useful, how sensitive on average a given fish species as a whole is to SST changes or scenarios under climate changes, using the MTT? Thus, future work should be conducted to test if this is the case by comparing both estimation methods.
Temperature Tolerance Index and mercury concentration patterns analysis of the European waters data series show that there is some evidence of weakening the resilience of fisheries to climatic and non-climate stressors. Our results highlight that SST could rise between 0.5 and 0.7°C by 2100 for the lowest carbon emissions scenario (RCP 2.6) and in excess of 2°C under RCP 8.5. This will ultimately weaken the resilience of fish stocks and marine ecosystem in European waters. The study has found that over 5 temperate benthopelagic species such as Norway lobster, common sole, great Atlantic scallop, red mullet, European hake and European seabass will be negatively affected (in terms of abundance and distribution) by high SST under RCP 8.5 in 2050 and 2100 because their estimated TTI > 1. Therefore, global effort that is already ongoing to minimize carbon footprint need to be intensified. It is essential for stakeholders, including governments, fishers and resource managers and citizens, should focus more attention on the monitoring of environmental parameters, such as SST, mercury pollution, to determine the resilience of fishery such as bobtail squids, frogfishes, great Atlantic scallop, red mullet, and common octopus that are more vulnerable to climate change and non-climate stressors. In addition, a prevention risk management plan based on the weight of evidence and conceptual framework proposed here (Figure 2) for European fisheries in tandem with national and international instruments is of paramount to proactively address and combat the multiple anthropogenic stressors, resulting from the combined interaction of warming oceans, mercury pollution, overfishing in the face of global changes. Precautionary decision-making processes and development of concerted management actions and mitigation policies for climate change, chemical pollution, and fishing activities may well follow a proactive bottom-up policy, supporting the prevention pathway and precautionary approach to mitigate and eliminate mercury pollution and neutralize carbon emissions (e.g., net-zero emissions and decarbonized economy) from anthropogenic sources (Alava et al., 2017a; Alava, 2019), as well as championing sustainable fishing activities by eradicating harmful fisheries subsidies (Sumaila et al., 2021), instead of the classic, imposed top-down policy perpetuating “business as usual” and status quo.
Also related are awareness raising, improving education, and human and institutional capacity on climate change mitigation. Anthropogenic-induced pressures such as mercury pollution from human-made sources may reduce the ability of fisheries and marine ecosystem to respond to present day climatic pressures. Enhanced resilience of fisheries and marine ecosystem by reducing stressors, including pollution and the use of habitat destructive fishing gears (e.g., dredge, bottom trawl). Also, the international community should strengthen a global ban on mercury and worldwide control of persistent organic pollutants’ emission sources within the United Nations framework as well as increase fish consumption advisories for methylmercury.
The next pathway, in terms of reducing fisheries and ecosystem resilience is industrial fishing. Overfishing is the most serious threat to fisheries in the European waters, and therefore effective fisheries management measures are required in order to decrease the ecological effects of overfishing and increase the food security especially for the coastal communities in the Europe. Hence the ongoing effort in the fisheries sector as a whole on reversing overfishing on target stocks and fisheries impacts on non-commercially fished species (Garcia et al., 2018) as well as increase efforts to rebuild fisheries and promote the restoration of the fisheries (Worm et al., 2009) in European waters need to be intensified to enhance fisheries’ resilience to climatic and non-climate stressors. Generally, reduction in fishing intensity including measures that promote social resilience within the fishing sector while maintaining effective conservation measures will increase resilience of the fisheries. Such strategies include enhancing transferable fishing quotas, alternative fisheries and livelihood diversification. Future research can incorporate ecosystem and foodwebs modeling experiments that explore the impact of combined environmental stressors (e.g., addressing mercury pollution, overfishing, and climate change forcing simultaneously).
The raw data supporting the conclusions of this article will be made available by the authors, without undue reservation.
Ethical review and approval were not required for the study because we used public information.
II, JA, VL, and US: conceptualization, methodology, writing—original draft, and writing—reviewing and editing. All authors contributed to the article and approved the submitted version.
The authors declare that the research was conducted in the absence of any commercial or financial relationships that could be construed as a potential conflict of interest.
All claims expressed in this article are solely those of the authors and do not necessarily represent those of their affiliated organizations, or those of the publisher, the editors and the reviewers. Any product that may be evaluated in this article, or claim that may be made by its manufacturer, is not guaranteed or endorsed by the publisher.
We thank Cheung W.W. for helpful discussion and data. We thank to Fisheries Economics Research Unit and Ocean Pollution Research Unit for invaluable advice on the original draft, and SeaAroundUs for giving us access to their useful data. We acknowledge support from OurFish. We are grateful to Rebecca Hubbard and Mike Walker for offering invaluable advice during Symposium presentation entitled: Delivering on Climate and Biodiversity Targets Through Better Fisheries Management on 23 March, 2021.
The Supplementary Material for this article can be found online at: https://www.frontiersin.org/articles/10.3389/fmars.2021.770805/full#supplementary-material
Agnew, D. J., Pearce, J., Pramod, G., Peatman, T., Watson, R., Beddington, J. R., et al. (2009). Estimating the worldwide extent of illegal fishing. PLoS One 4:e4570. doi: 10.1371/journal.pone.0004570
Alava, J. J. (2019). “Ocean pollution and warming oceans: towards ocean solutions and natural marine bioremediation,” in Predicting Future Oceans: Sustainability of Ocean and Human Systems amidst Global Environmental Change, eds A. M. Cisneros-Montemayor, W. W. L. Cheung, and Y. Ota (Amsterdam: Elsevier), 495–518.
Alava, J. J., Cheung, W. W. L., Ross, P. S., and Sumaila, R. U. (2017a). Climate change-contaminant interactions in marine food webs: towards a conceptual framework. Glob. Chang. Biol. 23, 3984–4001. doi: 10.1111/gcb.13667
Alava, J. J., Barragán-Paladines, M. J., Denkinger, J., Muñoz-Abril, L., Jiménez, P. J., Paladines, F., et al. (2017b). Massive Chinese fleet jeopardizes threatened shark species around the Galápagos Marine Reserve and waters off ecuador: implications for National and International Fisheries Policy. Int. J. Fish. Sci. Res. 1:1001.
Alava, J. J., Cisneros-Montemayor, A. M., Sumaila, U. R., and Cheung, W. W. (2018). Projected amplification of food web bioaccumulation of MeHg and PCBs under climate change in the Northeastern Pacific. Sci. Rep. 8:13460. doi: 10.1038/s41598-018-31824-5
Alava, J. J., Lindop, A., and Jacquet, J. (2015). Reconstruction of Marine Fisheries Catches for Continental ECUADOR, 1950-2010. UBC Fisheries Centre Working Paper # 2015–34. Vancouver, BC: University of British Columbia.
Alava, J. J., and Paladines, F. (2017). Illegal fishing on the Galápagos high seas. Science 357, 1362–1363.
Allison, E. H., Perry, A. L., Badjeck, M. C., Adger, W. N., Brown, K., Conway, D., et al. (2009). Vulnerability of national economies to the impacts of climate change on fisheries. Fish Fish. 10, 173–196. doi: 10.1111/j.1467-2979.2008.00310.x
Baum, J. K., and Fuller, S. D. (2016). Canada’s Marine Fisheries: Status, Recovery Potential and Pathways to Success. Toronto, ON: Ocean Canada.
Bianchi, G., Gislason, H., Graham, K., Hill, L., Jin, X., Koranteng, K., et al. (2000). Impact of fishing on size composition and diversity of demersal fish communities. ICES J. Mar. Sci. 57, 558–571.
Bindoff, N. L., Cheung, W. W. L., Kairo, J. G., Arístegui, J., Guinder, V. A., Hallberg, R., et al. (2019). “Changing ocean, marine ecosystems, and dependent communities,” in IPCC Special Report on the Ocean and Cryosphere in a Changing Climate, eds H.-O. Pörtner, D. C. Roberts, V. Masson-Delmotte, P. Zhai, M. Tignor, E. Poloczanska, et al. (Geneva: Intergovernmental Panel on Climate Change (IPCC)).
Bondaroff, T. N. P., Werf, V. D., and Reitano, T. (2015). The Illegal Fishing and Organized Crimenexus: Illegal Fishing as Transnational Organized Crime. Geneva: The Global Initiative Against Transnational Organized Crime and The Black Fish.
Booth, S., and Zeller, D. (2005). Mercury, food webs, and marine mammals: implications of diet and climate change for human health. Environ. Health Perspect. 113, 521–526. doi: 10.1289/ehp.7603
Bridges, K. N., Soulen, B. K., Overturf, C. L., Drevnick, P. E., and Roberts, A. P. (2016). Embryotoxicity of maternally transferred methylmercury to fathead minnows (Pimephales promelas). Environ. Toxicol. Chem. 35, 1436–1441. doi: 10.1002/etc.3282
Carta, P., Flore, C., Alinovi, R., Ibba, A., Tocco, M. G., Aru, G., et al. (2003). Sub-clinical neurobehavioral abnormalities associated with low level of mercury exposure through fish consumption. Neurotoxicology 24, 617–623. doi: 10.1016/S0161-813X(03)00080-9
Cavan, E. L., and Hill, S. L. (2020). Commercial fishery disturbance of the global open-ocean carbon sink. bioRxiv [Preprint] doi: 10.1101/2020.09.21.307462
Chavez, F. P., Ryan, J., Lluch-Cota, S. E., and Niquen, M. (2003). From anchovies to sardines and back: multidecadal change in the Pacific Ocean. Science 299, 217–221. doi: 10.1126/science.1075880
Cheung, W. W., Lam, V. W., Sarmiento, J. L., Kearney, K., Watson, R. E. G., Zeller, D., et al. (2010). Large scale redistribution of maximum fisheries catch potential in the global ocean under climate change. Glob. Chang. Biol. 16, 24–35.
Cheung, W. W., Sarmiento, J. L., Dunne, J., Frölicher, T. L., Lam, V. W., Palomares, M. D., et al. (2013). Shrinking of fishes exacerbates impacts of global ocean changes on marine ecosystems. Nat. Clim. Chang. 3, 254–258.
Choy, C. A., Robison, B. H., Gagne, T. O., Erwin, B., Firl, E., Halden, R. U., et al. (2019). The vertical distribution and biological transport of marine microplastics across the epipelagic and mesopelagic water column. Sci. Rep. 9:7843.
Cooley, S. R., Lucey, N., KitePowell, H., and Doney, S. C. (2012). Nutrition and income from molluscs today imply vulnerability to ocean acidification tomorrow. Fish Fish. 13, 182–215.
Daskalov, G. M., Boicenko, L., Grishin, A. N., Lazar, L., Mihneva, V., Shlyahov, V. A., et al. (2017). Architecture of collapse: regime shift and recovery in a hierarchically structured marine ecosystem. Glob. Chang. Biol. 23, 1486–1498. doi: 10.1111/gcb.13508
Dawson, M. A. (1982). Effects of long-term mercury exposure on hematology of striped bass. Morone saxatilis. Fish. Bull. 80, 389–392.
Doney, S. C. (2010). The growing human footprint on coastal and open-ocean biogeochemistry. Science 328, 1512–1516. doi: 10.1126/science.1185198
Dulvy, N. K., Sadovy, Y., and Reynolds, J. D. (2003). Extinction vulnerability in marine populations. Fish Fish. 4, 25–64.
Dunne, J. P., John, J., Adcroft, A., Griffies, S., Griffies, R. W., Shevliakova, E., et al. (2012). GFDL’s ESM2 global coupled climate–carbon earth system models. Part I: physical formulation and baseline simulation characteristics. J. Clim. 25, 6646–6665.
EFSA (2015). Statement on the benefits of fish/seafood consumption compared to the risks of methylmercury in fish/seafood. EFSA J. 13:3982. doi: 10.2903/j.efsa.2015.3982
EJF (2020). Europe – A Market for Illegal Seafood from West Africa: The Case of Ghana’s Industrial Trawl Sector. Availabl online at: https://ejfoundation.org/resources/downloads/EJF_Europe-A-Market-for-Illegal-Seafood-from-West-Africa_2020_final.pdf (accessed December 13, 2021).
Eriksen, M., Lebreton, L. C. M., Carson, H. S., Thiel, M., Moore, C. J., Borerro, J. C., et al. (2014). Plastic pollution in the world’s oceans: more than 5 trillion plastic pieces weighing over 250,000 tons afloat at sea. PLoS One 9:e111913. doi: 10.1371/journal.pone.0111913
European Commission (2002). Amending Regulation (EC) No. 466/2001 Setting Maximum Levels for Certain Contaminants in Foodstufs. Brussels: European Commission.
European Commission (2008). Reflections on Further Reform of the Common Fisheries Policy. Brussels: European Commission.
European Environment Agency (2018). Mercury in Europe’s Environment: A priority for Europeans and global action. København: European Environment Agency.
Fernandes, J. A., Papathanasopoulou, E., Hattam, C., Queirós, A. M., Cheung, W. W. L., Yool, A., et al. (2017). Estimating the ecological, economic and social impacts of ocean acidification and warming on UK fisheries. Fish Fish. 18, 389–444. doi: 10.1111/faf.12183
Free, C. M., Thorson, J. T., Pinsky, M. L., Oken, K. L., Wiedenmann, J., and Jensen, O. P. (2019). Impacts of historical warming on marine fisheries production. Science 363, 979–983.
Garcia, S. M., Ye, Y., Rice, J., and Charles, A. (Eds). (2018). Rebuilding of Marine Fisheries. Part 1: Global Review. FAO Fisheries and Aquaculture Technical Paper No. 630/1. Rome: FAO.
Gattuso, J., Magnan, A. K., Bopp, L., Cheung, W. W. L., Duarte, C. M., Hinkel, J., et al. (2018). Ocean solutions to address climate change and its effects on marine ecosystems. Front. Mar. Sci. 5:337.
González-Taboada, F., and Anadón, R. (2012). Patterns of change in sea surface temperature in the North Atlantic during the last three decades: beyond mean trends. Clim. Chang. 115, 419–431. doi: 10.1007/s10584-012-0485-6
Halpern, B. S., Frazier, M., Afflerbach, J., Lowndes, J. S., Micheli, F., O’Hara, C., et al. (2019). Recent pace of change in human impact on the world’s ocean. Sci. Rep. 9:11609. doi: 10.1038/s41598-019-47201-9
Halpern, B. S., Frazier, M., Potapenko, J., Casey, K. S., Koenig, K., Longo, C., et al. (2015). Spatial and temporal changes in cumulative human impacts on the world’s ocean. Nat. Commun. 6:7615. doi: 10.1038/ncomms8615
Houston, M. (2011). Role of mercury toxicity in hypertension, cardiovascular disease, and stroke. Journal of Clinical Hypertension 13, 621–627. doi: 10.1111/j.1751-7176.2011.00489.x
IPCC (2014). Summary for Policy Makers. Climate Change 2014: Impacts, Adaptation and Vulnerability - Contributions of the Working Group II to the Fifth Assessment Report. Geneva: IPCC, 1–32.
IPCC (2018). in Summary for Policymakers, eds V. Masson-Delmotte, P. Zhai, H.-O. Pörtner, D. Roberts, J. Skea, P. R. Shukla, et al. (Geneva: World Meteorological Organization).
IPCC (2021). “Summary for Policymakers,” in Climate Change 2021: The Physical Science Basis. Contribution of Working Group I to the Sixth Assessment Report of the Intergovernmental Panel on Climate Change, eds V. Masson-Delmotte, P. Zhai, A. Pirani, S. L. Connors, C. Péan, S. Berger, et al. (Cambridge: Cambridge University Press).
Issifu, I., and Sumaila, U. R. (2020). A review of the production, recycling and management of marine plastic pollution. J. Mar. Sci. Eng. 8, 1–16.
Jambeck, J. R., Geyer, R., Wilcox, C., Siegler, T. R., Perryman, M., Andrady, A., et al. (2015). Plastic waste inputs from land into the ocean. Science 347, 768–771. doi: 10.1126/science.1260352
Jennings, S., and Blanchard, J. L. (2004). Fish abundance with no fishing: predictions based on macroecological theory. J. Anim. Ecol. 73, 632–642. doi: 10.1111/j.1365-2656.2008.01466.x
Jensen, T., Frost, H., Gersen, T., and Andersen, J. L. (2015). Game theory and fish wars: the case of the Northeast Atlantic mackerel fishery. Fish. Res. 172, 7–16.
Johnson, N. W., Mitchell, C. P. J., Engstrom, D. R., Bailey, L. T., Wasik, C. J. K., and Berndt, M. E. (2016). Methylmercury production in a chronically sulfate-impacted sub-boreal wetland. Environ. Sci. Process. Impacts 18, 725–734. doi: 10.1039/c6em00138f
Kane, I. A., Clare, M. A., Miramontes, E., Wogelius, R., Rothwell, J. J., Garreau, P., et al. (2020). Seafloor microplastic hotspots controlled by deep-sea circulation. Science 368, 1140–1145. doi: 10.1126/science.aba5899
Lam, V. W. Y., Cheung, W. W. L., Reygondeau, G., and Sumaila, U. R. (2016). Projected change in global fisheries revenues under climate change. Sci. Rep. 6:32607. doi: 10.1038/srep32607
Leape, J., Micheli, F., Tigchelaar, M., Allison, E. H., Basurto, X., Bennett, A., et al. (2021). The Vital Roles of Blue Foods in the Global Food System. Available online at https://sc-fss2021.org/wp-content/uploads/2021/04/FSS_Brief_Blue_Economy_MT.pdf (accessed July 18, 2021).
Lebreton, L., Slat, B., Ferrari, F., Sainte-Rose, B., Aitken, J., Marthouse, R., et al. (2018). Evidence that the Great Pacific Garbage Patch is rapidly accumulating plastic. Sci. Rep. 8:4666. doi: 10.1038/s41598-018-22939-w
Levitus, S., Antonov, J. I., Boyer, T. P., Locarnini, R. A., Garcia, H. E., and Mishonov, A. V. (2009). Global ocean heat content 1955–2008 in light of recently revealed instrumentation problems. Geophys. Res. Lett. 36:L07608.
Lotze, H. K., Lenihan, H. S., Bourque, B. J., Bradbury, R. H., Cooke, R. G., Kay, M. C., et al. (2006). Depletion, degradation, and recovery potential of estuaries and coastal seas. Science 312, 1806–1809. doi: 10.1126/science.1128035
Martínez-Ortiz, J., Aires-da-Silva, A. M., Lennert-Cody, C. E., and Maunder, M. N. (2015). The Ecuadorian artisanal fishery for large pelagics: species composition and spatio-temporal dynamics. PLoS One 10:e0135136. doi: 10.1371/journal.pone.0135136
Mason, R. P., and Sheu, G. R. (2002). Role of the ocean in the global mercury cycle. Glob. Biogeochem. Cycles 16, 40–41.
Matta, M. B., Linse, J., Cairncross, C., Francendese, L., and Kocan, R. M. (2001). Reproductive and transgenerational effects of methylmercury or aroclor 1268 on Fundulus heteroclitus. Environ. Toxicol. Chem. 20, 327–335.
McCauley, D. J., Pinsky, M. L., Palumbi, S. R., Estes, J. A., Joyce, F. H., and Warner, R. R. (2015). Marine defaunation: animal loss in the global ocean. Science 347:1255641. doi: 10.1126/science.1255641
Miles, E. A., and Calder, P. C. (2012). Influence of marine n-3 polyunsaturated fatty acids on immune function and a systematic review of their effects on clinical outcomes in rheumatoid arthritis. Br. J. Nutr. 107, S171–S184. doi: 10.1017/S0007114512001560
Ministerio de Comercio Exterior (2017). Report of the Ecuadorian Tuna Sector. August 2017, Ecuador. 30. Available online at: https://www.produccion.gob.ec/wp-content/uploads/2019/06/Reporte-del-sector-atunero-ingles.pdf (accessed October 11, 2021)
Monnier, L., Gascuel, D., Alava, J. J., Barragán, M. J., Gaibor, N., Hollander, F. A., et al. (2020). Small-Scale Fisheries in a Warming Ocean: Exploring Adaptation to Climate Change. Scientific Report. Hamburg: International WWF Centre for Marine Conservation.
Moomaw, W., and Blankenship, S. (2014). Charting a New Course for the Ceans: A Report on the State of the World’s Oceans, Global Fisheries and Fisheries Treaties, and Potential Strategies for Reversing the Decline in Ocean Health and Productivity. The Center for International Environment & Resource Policy, Number 011. Medford, MA: Center for International Environment and Resource Policy.
National Academies of Sciences, Engineering, and Medicine (2021). Reckoning with the U.S. Role in Global Ocean Plastic Waste. Washington, DC: The National Academies Press.
National Wildlife Federation (2006). Poisoning Wildlife: the Reality of Mercury Pollution. Reston, VA: National Wildlife Federation.
Noone, K. J., Sumaila, U. R., and Diaz, R. J. (2013). Managing Ocean Environments in a Changing Climate: Sustainability and Economic Perspectives. Amsterdam: Elsevier publication.
Oken, E., Radesky, J. S., Wright, R. O., Bellinger, D. C., Amarasiriwardena, C. J., Kleinman, K. P., et al. (2008). Maternal fish intake during pregnancy, blood mercury levels, and child cognition at age 3 years in a US cohort. Am. J. Epidemiol. 167, 1171–1181.
Oomen, C. M., Feskens, E. J., Räsänen, L., Fidanza, F., Nissinen, A. M., Menotti, A., et al. (2000). Fish consumption and coronary heart disease mortality in Finland, Italy, and The Netherlands. Am. J. Epidemiol. 151, 999–1006. doi: 10.1093/oxfordjournals.aje.a010144
Orr, J. C., Fabry, V. J., Aumont, O., Bopp, L., Doney, S. C., Feely, R. A., et al. (2005). Anthropogenic ocean acidification over the twenty-first century and its impact on calcifying organisms. Nature 437, 681–686. doi: 10.1038/nature04095
Ostle, C., Williamson, P., Artioli, Y., Bakker, D. C. E., Birchenough, S., Davis, C. E., et al. (2016). Carbon Dioxide and Ocean Acidification Observations in UK Waters: Synthesis Report with a Focus From 2010 to 2015. Norwich: University of East Anglia.
Pauly, D., Christensen, V., Dalsgaard, J., Froese, R., and Torres, F. (1998). Fishing down marine food webs. Science 279, 860–863.
Pauly, D., Watson, R., and Alder, J. (2005). Global trends in world fisheries: impacts on marine ecosystems and food security. Philos. Trans. R. Soc. B Biol. Sci. 360, 5–12. doi: 10.1098/rstb.2004.1574
Pauly, D., Zeller, D., and Palomares, M. L. D. (eds) (2020). Sea Around Us Concepts, Design and Data. Available online at: seaaroundus.org (accessed January 7, 2022).
Rangel-Huerta, O. D. R., Aguilera, C. M., Mesa, M. D., and Gil, A. (2012). Omega-3 long-chain polyunsaturated fatty acids supplementation on inflammatory biomakers: a systematic review of randomised clinical trials. Br. J. Nutr. 107, S159–S170. doi: 10.1017/S0007114512001559
Rochman, C. M., Lewison, R. L., Eriksen, M., Allen, H., Cook, A. M., and Teh, S. J. (2014). Polybrominated diphenyl ethers (PDBEs) in fish tissue may be an indicator of plastic contamination in marine habitats. Sci. Total Environ. 476–477, 622–633. doi: 10.1016/j.scitotenv.2014.01.058
Rogers, A. D., and Laffoley, D. A. (2011). International Earth System Expert Workshop on Ocean Stresses and Impacts. Summary report. Oxford: International Programme on the State of the Ocean.
Sandborgh-Englund, G., Elinder, C. G., Langworth, S., Schütz, A., and Ekstrand, J. (1998). Mercury in biological fluids after amalgam removal. J. Dental Res. 77, 615–624. doi: 10.1177/00220345980770041501
Sandheinrich, M. B., and Wiener, J. G. (2011). “Methylmercury in freshwater fish: recent advances in assessing toxicity of environmentally relevant exposures,” in Environmental Contaminants in Biota: Interpreting Tissue Concentrations, eds W. N. Beyer and J. P. Meador (Boca Raton, FL: CRC Press).
Schartup, A., Thackray, C., Qureshi, A., Dassuncao, C., Gillespie, K., Hanke, A., et al. (2019). Climate change and overfishing increase neurotoxicant in marine predators. Nature 572, 648–650. doi: 10.1038/s41586-019-1468-9
Schuhbauer, A., Chuenpagdee, R., Cheung, W. W., Greer, K., and Sumaila, U. R. (2017). How subsidies affect the economic viability of small-scale fisheries. Mar. Policy 82, 114–121. doi: 10.1016/j.marpol.2017.05.013
Science for Environment Policy (2017). Tackling Mercury Pollution in the EU and Worldwide. Bristol: European Commission.
STECF (2017). The 2017 Annual Economic Report on the EU Fishing Fleet (STECF-17-12). Luxembourg: Scientific, Technical and Economic Committee for Fisheries (STECF).
Stern, A. H. (2005). A review of the studies of the cardiovascular health effects of methylmercury with consideration of their suitability for risk assessment. Environ. Res. 98, 133–142. doi: 10.1016/j.envres.2004.07.016
Storelli, M. M., and Marcotrigiano, G. O. (2001). Total mercury levels in muscle tissue of Swordfish (Xiphias gladius) and Bluefin Tuna (Thunnus thynnus) from the Mediterranean Sea (Italy). J. Food Protect. 64, 1058–1061. doi: 10.4315/0362-028x-64.7.1058
Styf, H. K., Sköld, H. N., and Eriksson, S. P. (2013). Embryonic response to long-term exposure of the marine crustacean Nephrops norvegicus to ocean acidification and elevated temperature. Ecol. Evol. 3, 5055–5065. doi: 10.1002/ece3.860
Sumaila, U. R. (2018). Illicit trade in the marine resources of West Africa. Ghana. J. Econ. 6, 108–116.
Sumaila, U. R., Alder, J., and Keith, H. (2006). Global scope and economics of illegal fishing. Mar. Policy 30, 696–703.
Sumaila, U. R., Cheung, W. W. L., Lam, V. W. Y., Pauly, D., and Herrick, S. (2011). Climate change impacts on the biophysics and economics of world fisheries. Nat. Clim. Chang. 1, 449–456.
Sumaila, U. R., Skerritt, D. J., Schuhbauer, A., Villasante, S., Cisneros-Montemayor, A. M., Sinan, H., et al. (2021). WTO must ban harmful fisheries subsidies. Science 374, 544–544. doi: 10.1126/science.abm1680
Sumaila, U. R., and Tai, T. C. (2020). End overfishing and increase the resilience of the ocean to climate change. Front. Mar. Sci. 7:523. doi: 10.1073/pnas.2008256117
Sumaila, U. R., Zeller, D., Hood, L., Palomares, M. L. D., Li, Y., and Pauly, D. (2020). Illicit trade in marine fish catch and its effects on ecosystems and people worldwide. Sci. Adv. 6:eaaz3801. doi: 10.1126/sciadv.aaz3801
Sunderland, E. M., Krabbenhoft, D. P., Moreau, J. W., Strode, S. A., and Landing, W. M. (2009). Mercury sources, distribution, and bioavailability in the North Pacific Ocean: insights from data and models. Glob. Biogeochem. Cycles 23:GB2010.
U.S. EPA (2002). Toxic Release Inventory – 2002. Data Release Washington D. C: US. Washington DC: EPA.
Višnjevec, A. M., Kocman, D., and Horvat, M. (2013). Human mercury exposure and effects in Europe. Environ. Toxicol. Chem. 33, 1259–1270. doi: 10.1002/etc.2482
World Health Organization and Food and Agriculture Organization of the United Nations (2010). Report of the Joint FAO/WHO Expert Consultation on the Risks and Benefits of Fish Consumption. Rome: World Health Organization.
Worm, B., Hilborn, R., Baum, J. K., Branch, T. A., Collie, J. S., Costello, C., et al. (2009). Rebuilding global fisheries. Science 325, 578–585.
Keywords: Europeans waters, climate change, overfishing, pollution, mercury
Citation: Issifu I, Alava JJ, Lam VWY and Sumaila UR (2022) Impact of Ocean Warming, Overfishing and Mercury on European Fisheries: A Risk Assessment and Policy Solution Framework. Front. Mar. Sci. 8:770805. doi: 10.3389/fmars.2021.770805
Received: 05 September 2021; Accepted: 27 December 2021;
Published: 07 February 2022.
Edited by:
Athanassios C. Tsikliras, Aristotle University of Thessaloniki, GreeceReviewed by:
Dimitrios K. Moutopoulos, University of Patras, GreeceCopyright © 2022 Issifu, Alava, Lam and Sumaila. This is an open-access article distributed under the terms of the Creative Commons Attribution License (CC BY). The use, distribution or reproduction in other forums is permitted, provided the original author(s) and the copyright owner(s) are credited and that the original publication in this journal is cited, in accordance with accepted academic practice. No use, distribution or reproduction is permitted which does not comply with these terms.
*Correspondence: Ibrahim Issifu, aS5pc3NpZnVAb2NlYW5zLnViYy5jYQ==; Juan José Alava, ai5hbGF2YUBvY2VhbnMudWJjLmNh
Disclaimer: All claims expressed in this article are solely those of the authors and do not necessarily represent those of their affiliated organizations, or those of the publisher, the editors and the reviewers. Any product that may be evaluated in this article or claim that may be made by its manufacturer is not guaranteed or endorsed by the publisher.
Research integrity at Frontiers
Learn more about the work of our research integrity team to safeguard the quality of each article we publish.