- 1Department of Biological, Geological, and Environmental Sciences, University of Bologna, Ravenna, Italy
- 2Chioggia Hydrobiological Station “Umberto D’Ancona”, Department of Biology, University of Padova, Uo CoNISMa, Chioggia, Italy
- 3Department for the Cultural Heritage and Centro Interdipartimentale di Ricerca per le Scienze Ambientali (CIRSA), University of Bologna, Ravenna, Italy
Marine infrastructures are increasing, generating a variety of impacts and introducing artificial habitats which have low ecological value and support assemblages that differ significantly from those on natural rocky coasts. While in the past there was little ecological consideration as to how artificial structures were built, now the trend is to look for “greener” designs inspired by or mimicking nature. These greening efforts have had a strong focus on enhancing physical habitat structure to support more diverse assemblages, driven by the untested assumption that artificial habitats lack the physical structure proper to natural habitats. We tested this assumption by comparing five descriptors of physical structure (inclination; exposure; roughness; abundance, and diversity of surface morphological microelements) across a combination of natural and artificial habitats of regular and irregular morphologies (seawalls = artificial regular; cliffs = natural regular; breakwaters = artificial, irregular; and boulder fields = natural irregular) in the North Adriatic Sea. Most structural descriptors were similar between artificial and natural habitats. Only inclination was consistently steeper in the artificial than in the natural habitats. Other minor differences in roughness or in the abundance of some surface microelements were related to the general morphology (regular or irregular) of the habitat rather than to its artificial or natural identity. The outcomes challenge the widespread assumption that artificial habitats lack the physical structure proper to natural habitats and stimulate renewed consideration about other structural and non-structural elements that could enhance the performance and sustainability of artificial marine structures, such as construction material, environmental setting or maintenance. They also encourage a wider reflection about what makes an artificial building surface “greener”: structural complexity is an important ecological parameter, and its deliberate increase will lead to responses in the biota, however, this may not necessarily match “more natural” conditions.
Introduction
Urban waterfronts are replete with hard built infrastructure for coastal protection, trade, transportation, and recreation (e.g., seawalls, jetties, breakwaters, groins, docks, pontoons; Airoldi and Beck, 2007). This infrastructure comes in a variety of designs, materials and spatial configurations, depending on the primary function (Firth et al., 2016). It has a clear construction footprint on native habitats, causing permanent modification in the abiotic and biotic state around and on the structure (Heery et al., 2017), and it provides habitat that consistently performs poorly compared to natural reefs (Moschella et al., 2005; Perkol-Finkel et al., 2006; Carvalho et al., 2013), supporting distinct assemblages with low species and genetic diversity (Fauvelot et al., 2009) and dominance by opportunistic and invasive species (Bulleri and Airoldi, 2005; Airoldi et al., 2015). Recent estimates suggest that marine infrastructure globally modifies 2,072,603 km2 of the seafloor, which makes up 1.5% of the global coverage of the ocean exclusive economic zones (Bugnot et al., 2021).
While in the past there was little ecological consideration as to how marine artificial infrastructure was built (Airoldi et al., 2005), now the trend is to look for “greener” designs inspired by or mimicking nature, that introduce less alterations to the natural environment, support wildlife, or recover critical ecosystem functions (Firth et al., 2014b; Dafforn et al., 2015; Airoldi et al., 2021). These greening efforts have had a strong focus on physical habitat structure as a key factor for enhancing natural communities (Loke et al., 2015; Morris et al., 2017). Ongoing pilot projects have increased surface area, microhabitats available and/or topographic complexity by including for example pools, pits, and ledges in concrete walls, or retrofitting planters and panels with microhabitats on existing infrastructure (reviewed in Schoonees et al., 2019; O’Shaughnessy et al., 2020). A recent meta-analysis has shown that these enhancements often increased the abundance and number of species, yet the outcomes were inconsistent across species and studies, with overall uncertain benefits (Strain et al., 2018b).
The explicit and/or implicit assumption of greening projects that focus on structural enhancements is that artificial infrastructure lacks the physical structure proper to natural reefs, which would impair a variety of species (Bulleri and Chapman, 2004; Burt et al., 2009; Firth et al., 2014b; Dafforn et al., 2015). However, an extensive search in the scientific literature revealed a lack of formal quantitative comparisons of structural physical characteristics between natural and artificial habitats, with the only exception of Aguilera et al. (2014). We also noticed that topographic heterogeneity was especially emphasized as a limiting factor for the biota by papers dealing with morphologically regular seawalls (Bulleri and Chapman, 2004; Bulleri, 2005; Morris et al., 2017). Conversely, papers dealing with irregular infrastructures (i.e., built with quarried rocks or molded concrete elements) highlighted other potentially limiting factors for the biota, including greater pressures from fish (Ferrario et al., 2016; Gianni et al., 2018), greater disturbances (Airoldi and Bulleri, 2011), or a greater influence of the surrounding sedimentary environment (Franzitta and Airoldi, 2019; Komyakova et al., 2019). Finally, although physical habitat structure affects organisms differently at multiple spatial scales (Kovalenko et al., 2012; Loke et al., 2015), multi-scale measurements are still rare in artificial habitats, and most studies have focused on very small spatial scales from centimeters to meters (Strain et al., 2018b).
Here we present a multi-scale, quantitative comparison of physical structure among a variety of artificial and natural hard-bottom intertidal habitats along the Monte Conero promontory (North Adriatic Sea, Italy) aiming to test the general hypothesis that physical structure differs between artificial and natural habitats. Physical structure is an emergent property that can be associated to numerous key physical components of the habitat. Different terms have been used interchangeably to describe it (e.g., “heterogeneity” or “complexity”), and there are not univocal metrics to quantify it (Stein et al., 2014; Loke et al., 2015). We focused on a group of descriptors of physical surface properties that are most often claimed to be limiting for the growth of biota in artificial habitats and that could be more easily translated into design enhancement in eco-engineering applications (sensu Morris et al., 2019), specifically: (i) inclination; (ii) exposure; (iii) roughness; and (iv) abundance, and (v) diversity of surface morphological microelements like crevices, holes, grooves and pits. We tested the hypothesis that these descriptors differed between natural and artificial habitats not only in their average values but also in their variance distributions (Benedetti-Cecchi, 2003). Specifically: the inclination of artificial structures could be more vertical and more uniform than that of rocky shores to minimize construction materials and the footprint on the seafloor; the exposure of artificial habitats could face prevailing winds and be more uniform than that of natural habitats to provide shelter from waves to coastal assets; and the roughness and abundance and diversity of surface morphological microelements of artificial habitats could be lower and more uniform than those of natural habitats due to design constraints.
Because in our search of the scientific literature we had observed that papers dealing with morphologically regular seawalls placed greater emphasis on the limiting role of physical structure than papers dealing with irregular infrastructures, we formally tested if the five structural descriptors differed across two broad morphologies of artificial and natural habitats: regular (artificial seawalls and rocky cliffs) and irregular (block armored infrastructures and rocky boulder fields). We hypothesized that the differences between artificial and natural habitats per se could be smaller than those observed between regular and irregular habitat morphologies.
We finally tested the hypothesis that structural differences between habitats and morphologies were consistent across different spatial scales. In landscape analysis, the spatial scale consists of two components, grain and extent, which both affect estimates of physical structure (Wiens, 1989). Here we focused on a relatively small and fixed grain size (a small sample quadrat that fitted the size of the blocks that are used for the construction of marine infrastructures), while tested the variations in structural descriptors across different spatial extents: small (centimeters to meters); meso (meters to 10 s of meters); and large (100 s meters to kilometers).
Materials and Methods
Study Area and Field Sampling
This study took place over ≈ 8 km of coastline at Monte Conero promontory (Adriatic Sea, Italy, Figure 1). Here artificial rock-mold groins and breakwaters (hereafter both referred to only as “breakwaters”) and concrete-mold seawalls, built to protect small marinas and tourist beaches, are interspersed with marl and limestone subvertical cliffs and boulder fields. A more detailed description of the characteristics of both artificial and natural habitats can be found in Perkol-Finkel et al. (2012). The study focused on the low-intertidal (≈ 0 to + 20 cm relative to mean low-water level, average tidal amplitude ≈ 30 cm), as this is where most eco-engineering tests are in progress (O’Shaughnessy et al., 2020; Strain et al., 2021).
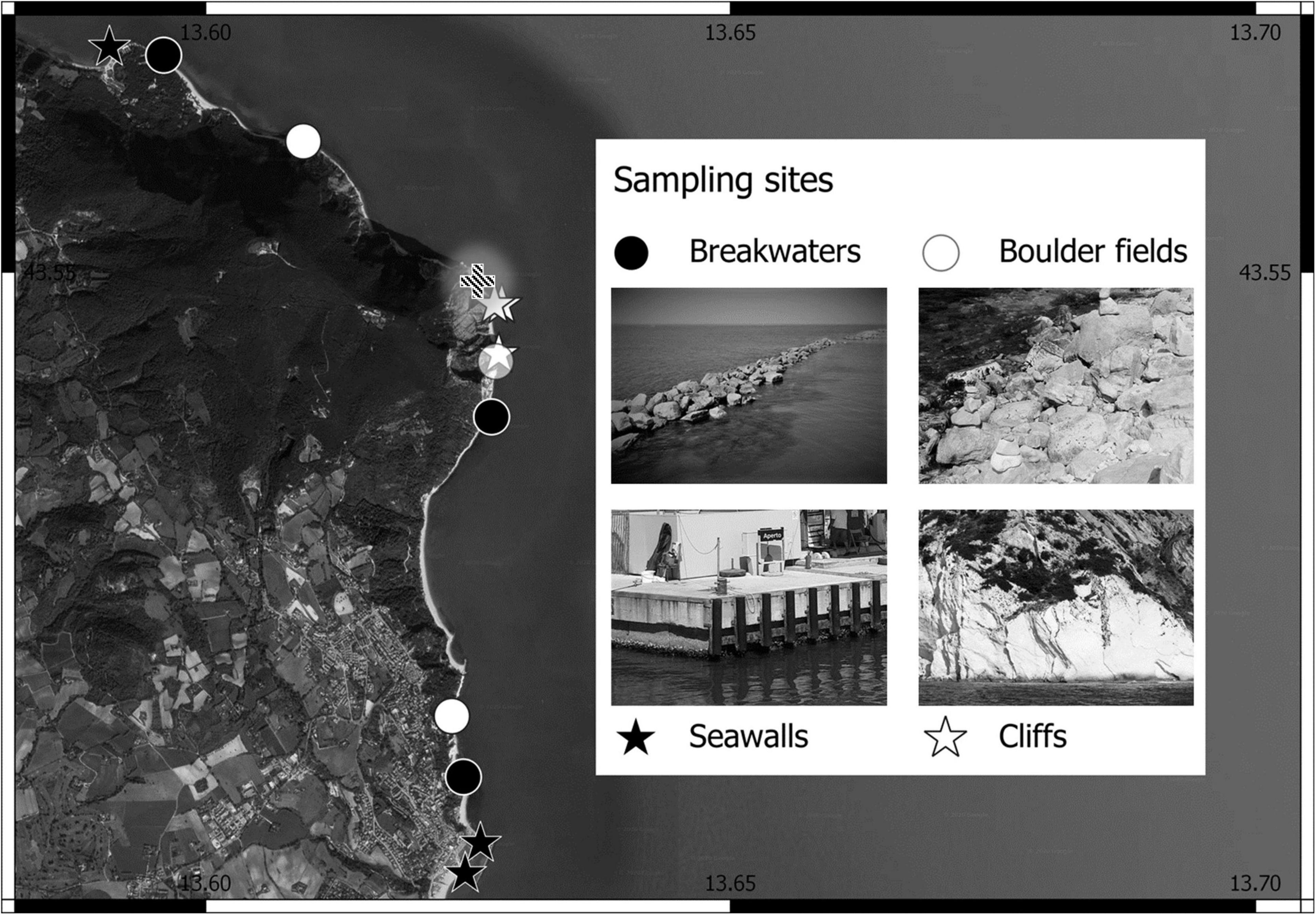
Figure 1. Map with site locations for each combination of habitat Type (Artificial = black symbols, Natural = white symbols) and Morphology (Irregular = circle symbols, Regular = star symbols). The dashed cross represents the Due Sorelle site.
In April 2019, we selected 12 interspersed sites, 100 s meters to kilometers apart (Figure 1), three for each of four habitats: seawalls (type artificial, morphology regular), cliffs (type natural, morphology regular), breakwaters (type artificial, morphology irregular), and boulder fields (type natural, morphology irregular). At each site, with the aid of a tape measure, we located four replicated areas 200 cm × 20 cm and 10 m apart. Within each area, we further located four replicated 10 cm × 10 cm sampling quadrats (hereafter plots), 50 cm apart. In total, we sampled 192 plots (4 plots per area, and 4 areas per each of 12 sites) to provide comparisons of physical structure across orthogonal combinations of two habitat types (artificial and natural) and two morphologies (irregular and regular), and across three spatial scales (small: differences among plots; meso: differences among areas; and large, differences among sites).
Before sampling, we removed any species from the plots, as we focused on the physical structure of the primary substrata. Then, in each plot we quantified (i) Inclination; (ii) Exposure; (iii) Roughness; and (iv) Abundance, and (v) Diversity of morphological elements. Inclination was estimated using a hand-held clinometer positioned in the center of the plot. Exposure (i.e., aspect) was estimated using a compass positioned in the center of the plot and facing the sea. Exposure was not measured when Inclination was <10, as exposure of flat substrates is meaningless, which occurred at 9 plots in boulder fields.
To estimate Roughness as well as Abundance and Diversity of morphological elements, we filmed each plot with a digital camera (Canon PowerShot G12 or S95 equipped with underwater housing, resolution 1280 × 720 pixels) as from Figueira et al. (2015), doing two 360° passes around the plot at distances of 30 and 15 cm, and then two perpendicular arc passes over the top of each plot. This allows to record the plot from every angle, and obtain high-quality overlapping stereo-photos for the subsequent reconstruction of high-resolution 3D structure model (Westoby et al., 2012).
Photogrammetric Analysis
The videos were analyzed using the Structure-from-Motion (SfM) photogrammetric technique (Westoby et al., 2012). The SfM workflow requires the selection from the video of an adequate number of partially overlapping and not-fuzzy photos to represent the surface from each angle. Preliminary tests identified in 25–30 the adequate number of stereo-photos to obtain a high-resolution 3D-model without slowing down excessively the whole SfM procedure. For 12 plots (6 from breakwaters, 4 from boulder fields and 2 from cliffs) we did not obtain sufficient high-quality photos for further SfM analysis due to wave splashing.
A textured digital surface model of the plot was created from the stereo-photos using Agisoft Metashape Standard v. 1.5 software, setting the workflow as in Supplementary Table 1. Briefly, photos were aligned based on the shared invariant features at the highest accuracy possible. The position of each feature was triangulated to recreate a high-quality 3D dense point cloud, which was used to build a mesh of triangular polygons and obtain a continuous surface. The “height field (2.5D)” surface type was preferred to the “arbitrary (3D)” as recommended for planar surfaces. On this continuous surface, a fitted texture was applied from the original stereo-photos. When occasional misaligned points occurred, i.e., when waves splashed the plots, we performed a post-processing cleaning to correct the error and avoid a complexity overestimation (Bayley et al., 2019). Finally, the x-y-z orientation of each model was done manually, leveling the base of the plot on the x-y plane. Each digital 3D plot model was exported from Agisoft as a 3-columns XYZ ASCII file and imported in R v.3.5.3 to quantify surface Roughness, and Abundance and Diversity of morphological elements. Each ASCII file, representing a 3D plot model, was rasterized at a spatial resolution (cell size) of 1 mm2.
Surface Roughness was computed as the ratio between the real plot surface (3D area), calculated with the “computeAreaRaster” function (“mkde” package), and its orthogonal planar projection (2D area). In this way, Roughness values ranged between 1 to a theoretical infinite maximal. We preferred surface roughness to other correlated measures (i.e., linear roughness, random roughness or fractal; Shepard et al., 2001; Kovalenko et al., 2012) because it is not biased by any specific placement of the linear paths (Figueira et al., 2015).
The Abundance and Diversity of discrete surface morphological elements were estimated using a landform classification based on the Topographic Position Index (TPI). TPI is an algorithm that measures the relative topographic position of each cell of the rasterized 3D plot models as the difference between the elevation at this cell and the average elevations of the adjacent cells within a predetermined neighborhood (Weiss, 2001). TPI was calculated with the “tpi” function in the “spatialEco” package (scale 0.5 cm, window type “circle,” and normalization “TRUE”). A range of 0.5 cm was chosen as the best compromise between the average dimension of the colonizing organisms (and consequently of the structural elements perceived by them; Warfe et al., 2008), and the loss of assessed area at the edge of the model after the TPI is computed. Normalization was set to “TRUE” as suggested by De Reu et al. (2013) because TPI classification in complex surfaces could be biased by roughness. Thereafter, each cell was classified into one of six morphological elements (ridges, upper slopes, middle slopes, flat slopes, lower slopes and valleys, see Supplementary Figure 1) by thresholding their continuous TPI values using standard deviations units and checking the slope of the cell for TPI values near 0 (see Weiss, 2001 for details). The abundances of the morphological elements were expressed as percentage over the total number of cells, and a variation of the Shannon Diversity Index, the Shannon’s Entropy 3D (H-3D), reported in the CASU software (Loke et al., 2014), was applied to provide an estimate of the surface morphological diversity. H-3D measures the relative abundance (pi) of the morphological elements as proportion of the surface area rather than of the total number of elements. This provides more meaningful H’ values when the dimensions of the elements are heterogeneous, as in our case. Because we identified six Topographic “forms” using the TPI, the maximal value that H-3D could assume was 2.58 (Eq. 1), with maximal relative abundance (pi) of each element equal to 1/6.
Statistical Analyses
Differences in the average descriptors of physical structure were analyzed using a four-way univariate permutational analyses of variance (PERMANOVA), including factors habitat Type (artificial vs. natural, fixed), Morphology (regular vs. irregular, fixed) crossed to Type, Site (three levels, random) nested in Type × Morphology, and Area (four levels, random) nested in Site. For Inclination, there were 4 replicates for each combination of factors. For Exposure replicates varied between 2 and 4, due to 9 missing values for horizontal substrata. Similarly, for Roughness and Abundance and Diversity of morphological elements replicates varied between 2 and 4, due to missing values from 12 photos that could not be analyzed via SfM. We used PERMANOVA instead of classical ANOVA because of ease of use with unbalanced replication and because of lack of normality assumptions (Anderson et al., 2008).
The use of ANOVA is discouraged in circular statistics (Mardia and Jupp, 2000; Jammalamadaka and SenGupta, 2001), therefore Exposure could not be directly analyzed due to the vectorial essence of these complex numbers (a + bi, where a is the real part and bi the imaginary part). As a proxy, we quantified the angle formed between the Exposure of each plot and the prevailing exposure of the Monte Conero coastline. This was estimated from the map as ≈ 30° and 90° for the sites north to and south to the “Due Sorelle,” respectively (Figure 1). We obtained non-vector values ranging from 0° (maximal concordance with coastline direction, seaward exposure) to 180° (maximal discordance with coastline direction, landward exposure), which expressed the plot deviations from the prevailing coastline Exposure.
We used PERMANOVA + for PRIMER (Anderson et al., 2008) to partition the variability and obtain F-statistics on a matrix of Euclidean distances. P-values were calculated using 9,999 random permutations of the appropriate exchangeable units and Type III sums of squares. In case of few unique permutations (<100), Monte Carlo (MC) p-values replaced the permuted p-values in the analysis.
Differences in the variance distributions of the descriptors of physical structure at each spatial scale (small, meso and large) were analyzed following Dal Bello et al. (2017). For this analysis, we considered seawalls, breakwaters, cliffs and boulder fields as four levels of a single factor “Habitat”. While this procedure did not test for the interaction Type × Morphology, it considerably simplified the analysis allowing to run a fully nested model. Briefly, for each physical descriptor, the “VarCor” function of “lmerTest” package (in R v.3.5.3) extracted the bias-corrected variance components associated to the three spatial scales for each habitat. We then calculated the maximal differences among these variance components (VarDIF-small, VarDIF-meso, VarDIF-large), which were each compared with a null distribution of values generated through bootstrapping (randomly reallocating samples 10,000 times) given that the null hypothesis is true (variance at each spatial scale is the same for all habitats). The observed variance components were deemed different across habitats if VarDIF at each scale exceeded the critical threshold (α = 0.05) obtained from the null distribution (for details see Dal Bello et al., 2017). In case of significant differences, post hoc pairwise comparisons were performed, using a threshold of 0.01.
Results
Inclination
Artificial habitats were on average more vertical than natural habitats, and regular habitats were on average more vertical than irregular habitats (Figure 2A and Table 1). This made the seawalls the most vertical and the boulder fields the least vertical of the four habitat combinations explored, with an average plot inclination of 92.6 and 53.9°, respectively. At the small and meso scales, regular habitats had more homogeneous inclinations than irregular habitats, and artificial habitats had more homogeneous inclinations than natural habitats (Figure 2B). Overall, seawalls were the most homogeneous at both the small and meso scales, while boulder fields had the greatest variability in inclination at the meso-scale (Supplementary Table 2). These differences, however, became negligible at the large scale, where all habitats showed consistent (i.e., low variance) inclinations at all sites (Figure 2B).
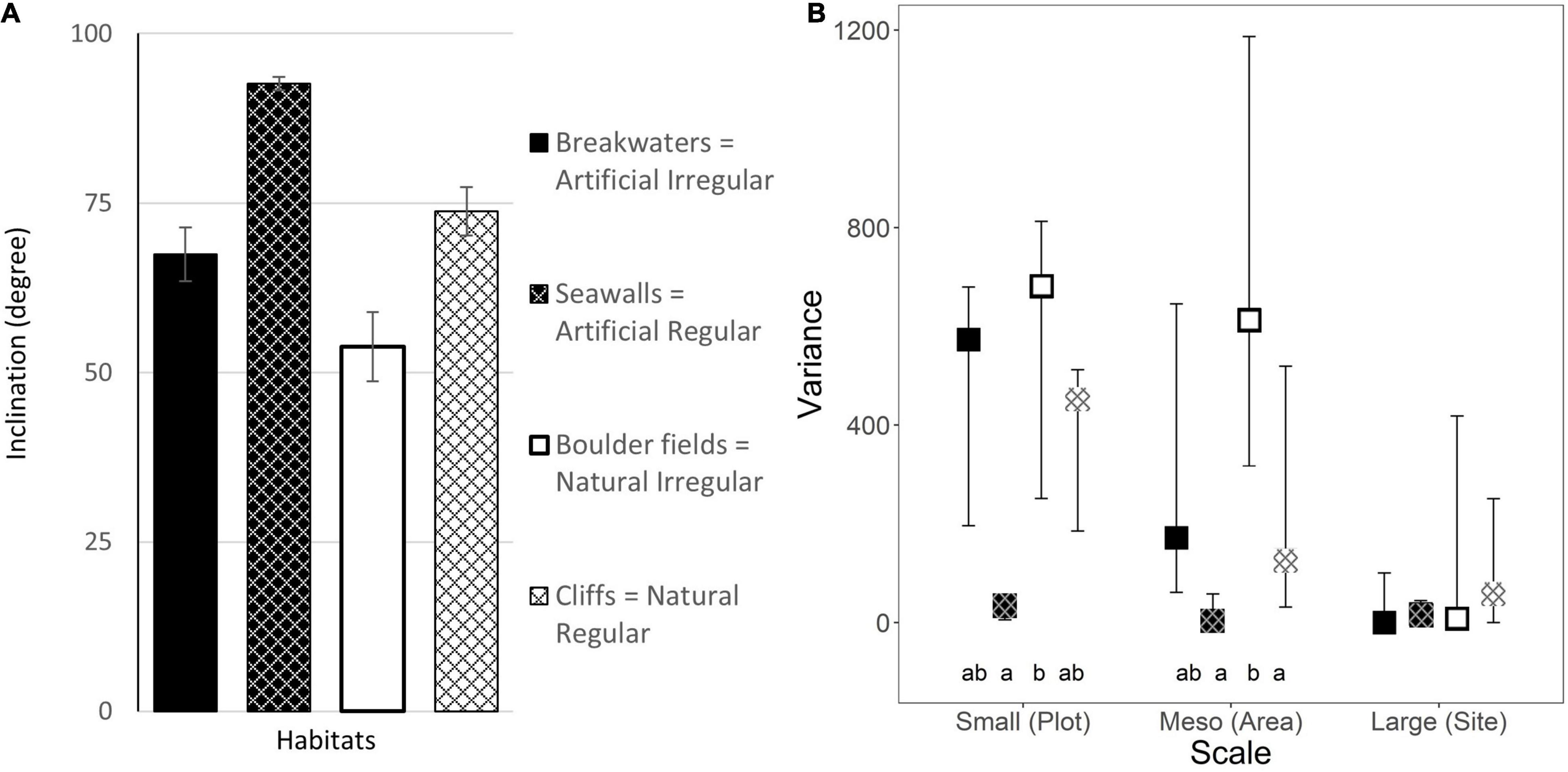
Figure 2. (A) Mean inclination (± 1 SE; data are averages of 4 plots at each of 4 areas at each of 3 sites, n = 48) and (B) estimated variance in inclination (±95% CI from bootstrapping) at each scale for the four combinations of habitat Types and Morphologies. Letters indicate significant differences from pairwise-tests (Supplementary Table 2).
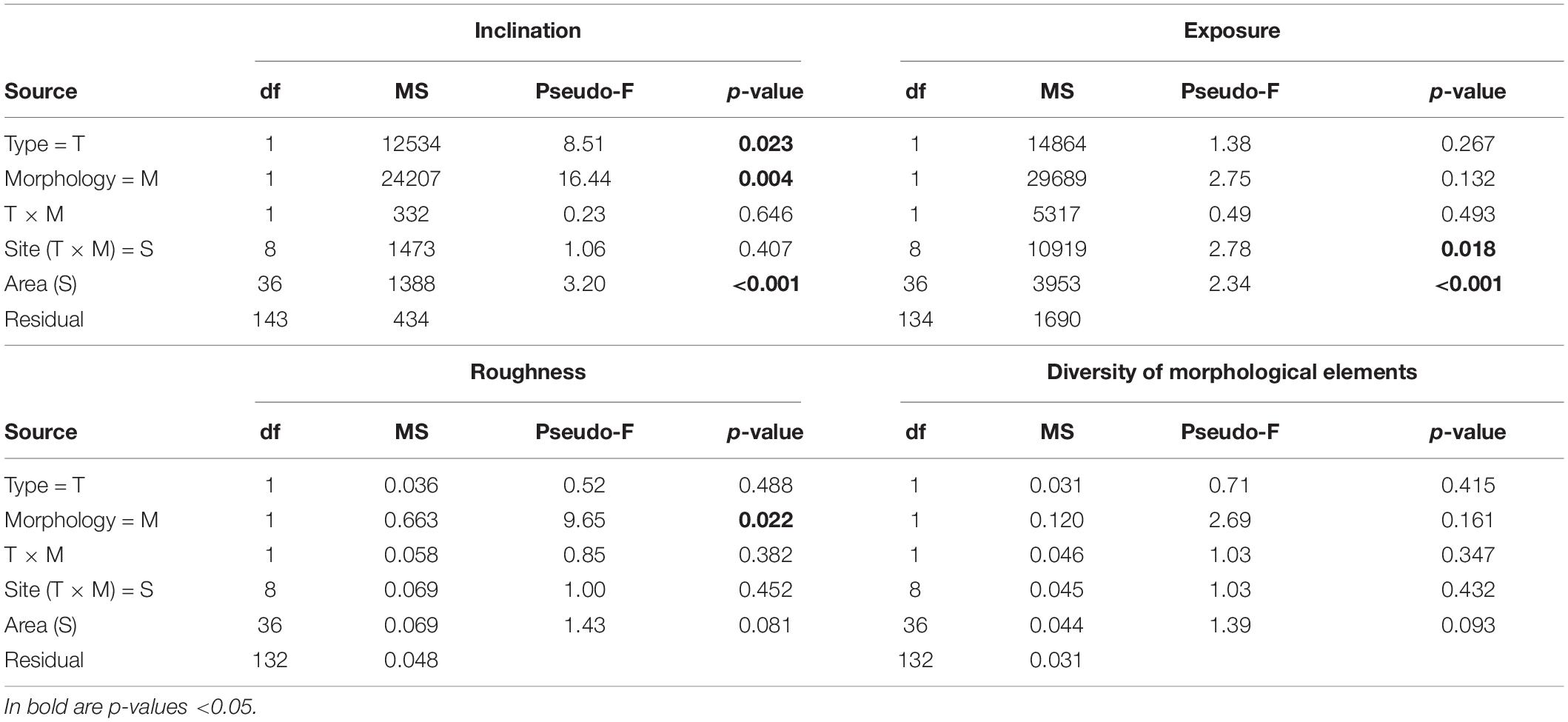
Table 1. Four-way PERMANOVA results testing the effects on inclination, exposure, roughness, and diversity of morphological microelements.
Exposure
When the compass point directions faced by each plot surface were grouped into the 16 major cardinal and intercardinal directions, we found that plots faced a broad range of directions, the only exception being represented by seawalls which mostly faced North-West or South (Figure 3). When the compass degrees were transformed into deviations from the prevailing coastline exposure, no differences were observed between habitat Types or Morphologies (Table 1). Although seawalls had almost 50% more landward microhabitats (represented by the greatest average deviation from prevailing seaward exposure, Figure 4A) than any other habitat, this difference was not significant, probably due to the large variability among both areas and sites (Table 1) and to loss of power from the lack of the horizontal replicates. At the small scale, seawalls had virtually no variation in exposure among plots (Figure 4B). This differed from all other habitats which showed high variability, with the greatest small-scale variation in exposure for boulder fields (Supplementary Table 2). At the meso and large scales, the trend was opposite, with seawalls showing the greatest variation in exposure among areas and sites. At the meso scale, this difference was not detected as significant. At the large scale, exposure was more variable among sites for regular than irregular habitats, and for artificial than natural habitats, which resulted in the greatest and lowest variability for seawalls and boulder fields, respectively (Figure 4B and Supplementary Table 2).
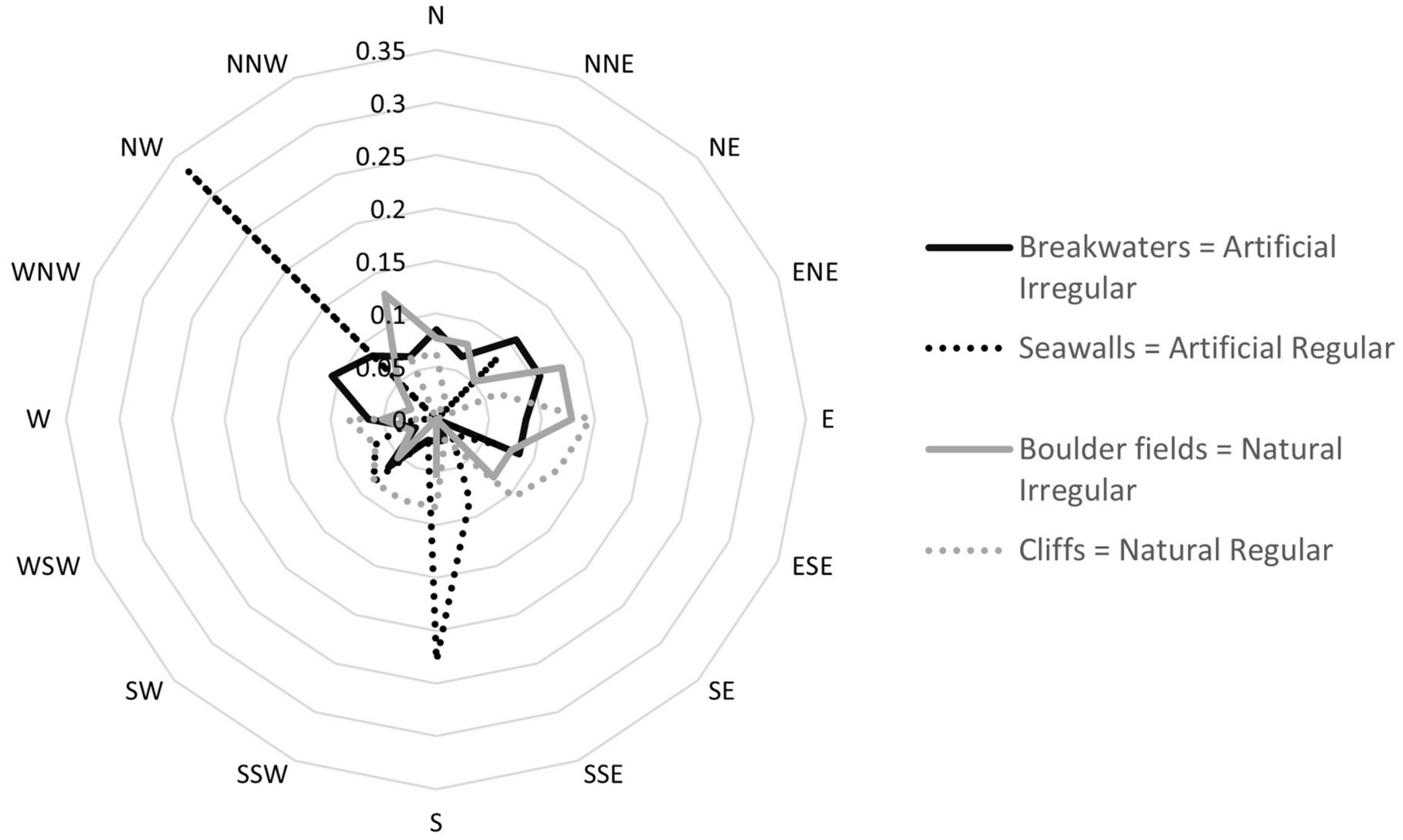
Figure 3. Radar graph representing the frequency (observed/total plots per habitat) of the exposures for the four combinations of habitat Types and Morphologies. The compass values were grouped into 16 major cardinal and intercardinal directions by approximating each point value to the nearest cardinal direction.
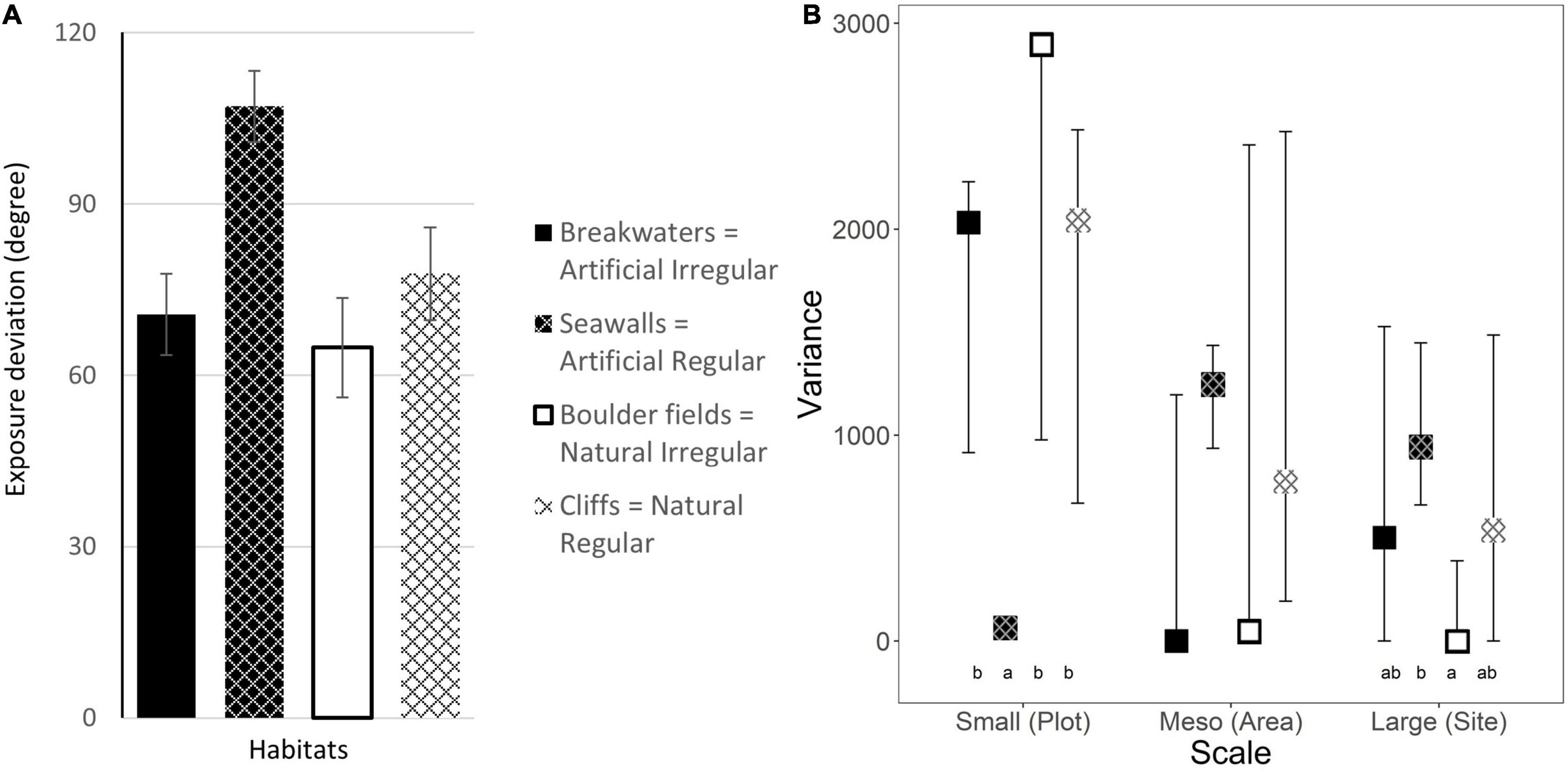
Figure 4. (A) Mean exposure deviation (±1 SE; data are averages of 2–4 plots at each of 4 areas at each of 3 sites, n = 39–48) and (B) estimated variance in Exposure (±95% CI from bootstrapping) at each scale for the four combinations of habitat Types and Morphologies. Letters indicate significant differences from pairwise-tests (Supplementary Table 2).
Roughness
Irregular habitats were significantly rougher than regular habitats, with an average roughness of 1.25 and 1.14, respectively. Conversely, there were no differences between artificial and natural habitats, which showed comparable rugosity (Figure 5A and Table 1). Roughness was homogeneous for all habitats at all spatial scales considered (Figure 5B). The only exception was represented by breakwaters, which showed some variation in roughness at the small scale among plots, but the difference was not significant (Figure 5B and Supplementary Table 2).
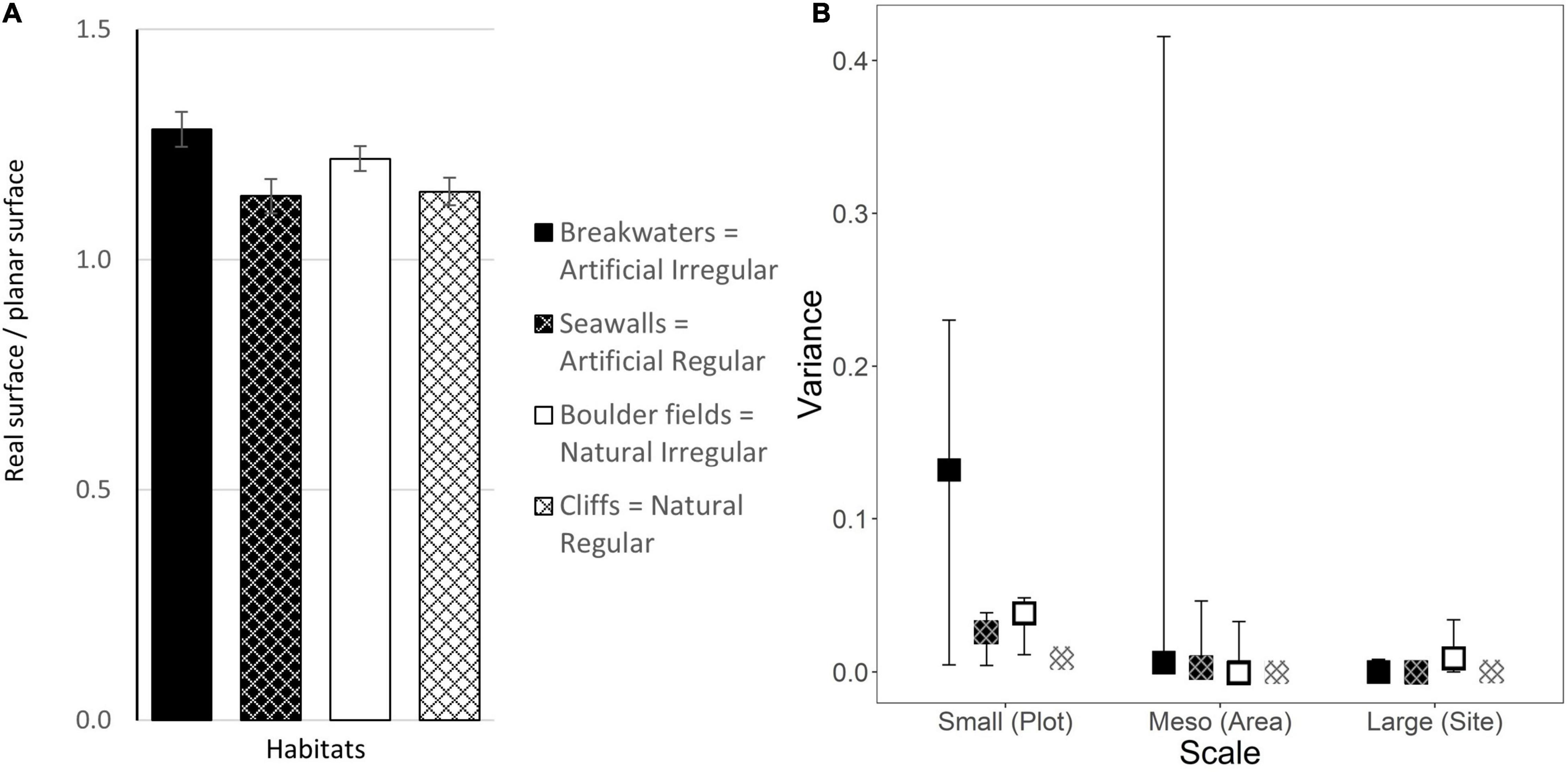
Figure 5. (A) Mean surface roughness (±1 SE; data are averages of 2–4 plots at each of 4 areas at each of 3 sites, n = 42–48) and (B) estimated variance in roughness (±95% CI from bootstrapping) at each scale for the four combinations of habitat Types and Morphologies. Letters indicate significant differences from pairwise-tests (Supplementary Table 2).
Abundance and Diversity of Morphological Microelements
Except for sparse “Flat slopes” (<4% cover), the other morphological microelements were evenly represented, with abundances between 16% for “Lower slopes” in breakwaters to 25.9% for “Valleys” in cliffs (Figure 6). The abundances of the microelements were comparable among habitat Types (Figure 6). Significant differences were detected only between habitat Morphologies, with “Lower slopes” more abundant in the regular than in the irregular habitats and, vice versa, with “Ridges” more abundant in the irregular than in the regular habitats (Table 2). In addition, “Valleys,” equally represented in regular and irregular artificial habitats (Breakwaters: 20.9%, Seawalls: 20.7%), were more abundant in irregular (19.7%) than regular (25.9%) natural habitats (significant interaction Type × Morphology, Table 2).
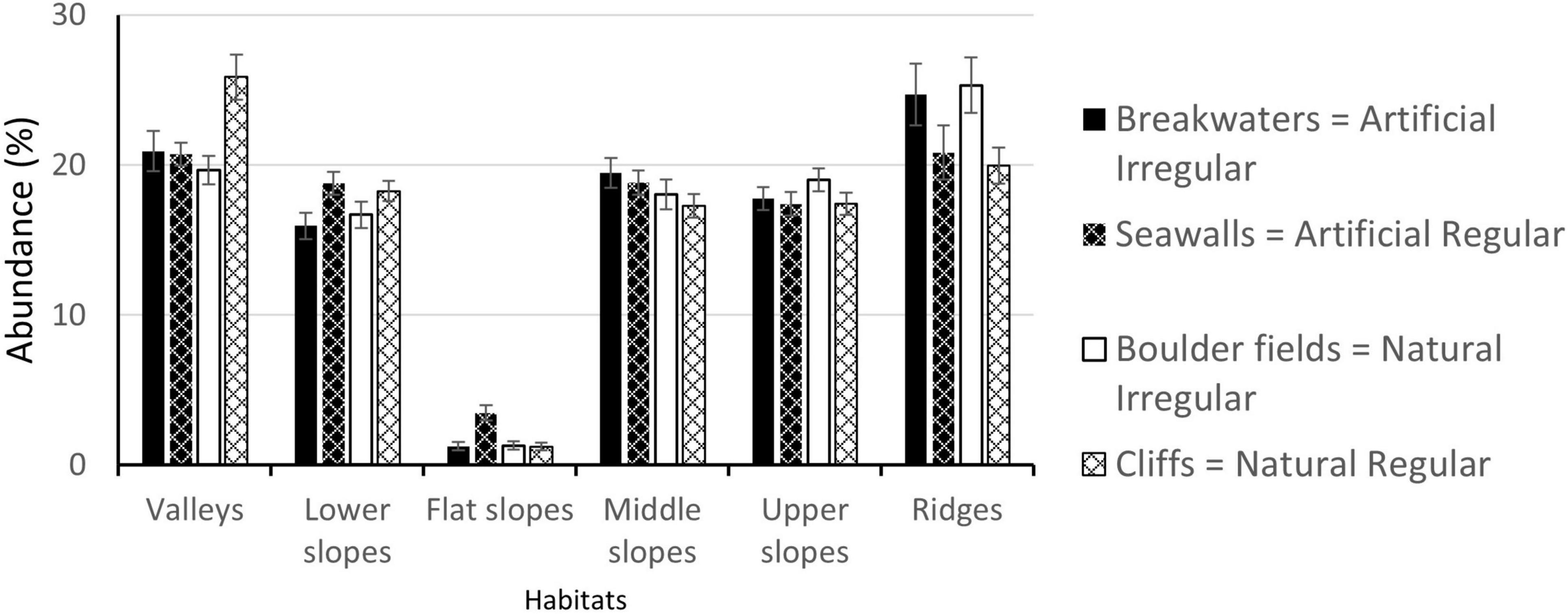
Figure 6. Mean abundances (±1 SE; data are averages of 2–4 plots at each of 4 areas at each of 3 sites, n = 42–48) of each of the six morphological microelements in the four habitats.
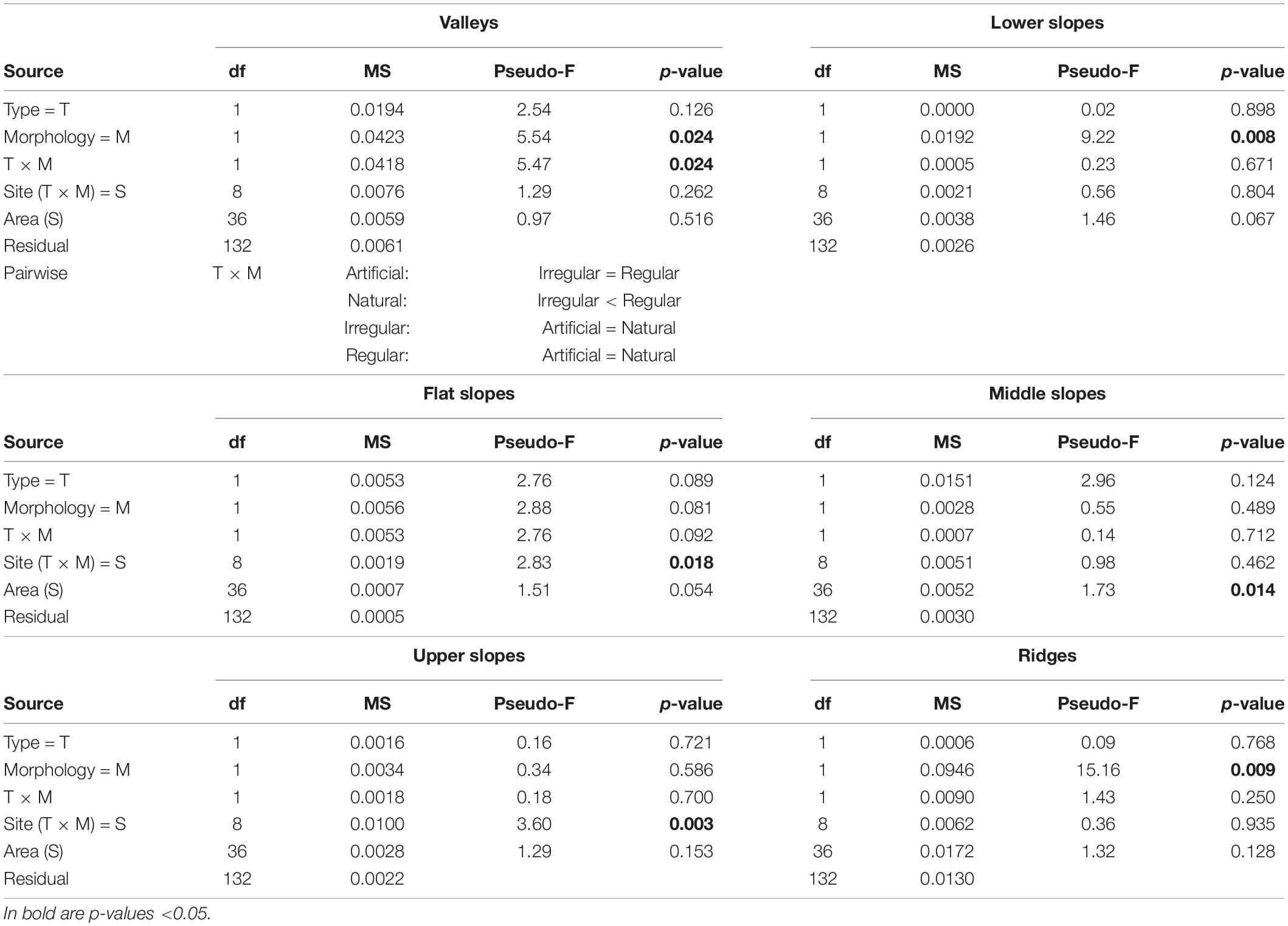
Table 2. Four-way PERMANOVA results testing the effects on the abundances of six morphological microelement (valleys, lower slopes, flat slopes, middle slopes, upper slopes, ridges).
No differences were detected in the Diversity of morphological elements which was comparable among habitats (Figure 7A and Table 1). Furthermore, Diversity of morphological elements was homogeneous for all habitats at all scales considered (Figure 7B and Supplementary Table 2).
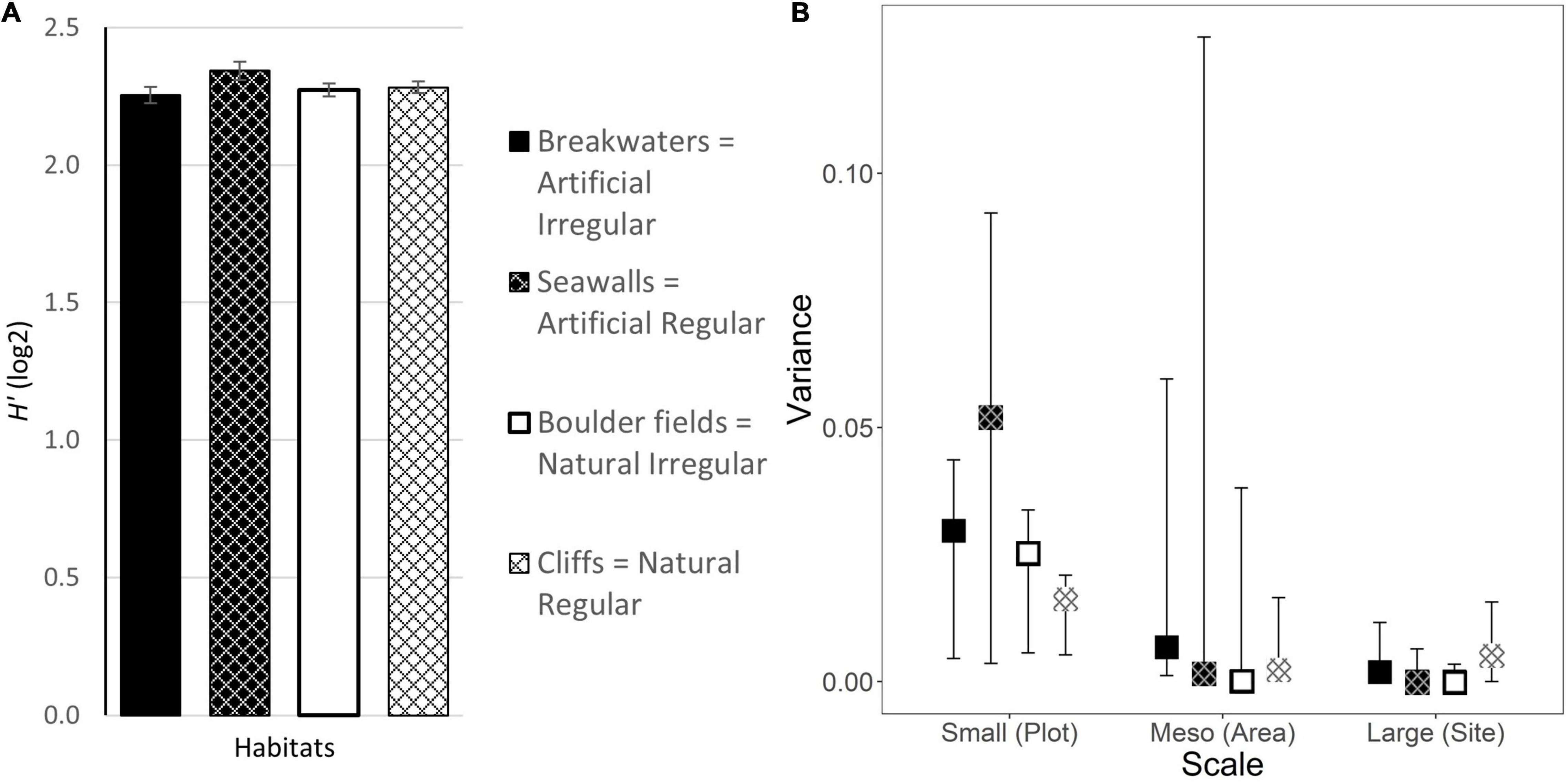
Figure 7. (A) Mean diversity of morphological microelements (±1 SE; data are averages of 2–4 plots at each of 4 areas at each of 3 sites, n = 42–48) and (B) estimated variance in diversity of morphological microelements (±95% CI from bootstrapping) at each scale for the four combinations of habitat Types and Morphologies. Letters indicate significant differences from pairwise-tests (Supplementary Table 2).
Discussion
We provide the first formal quantitative comparison of several descriptors of physical structure among a variety of artificial and natural marine habitats. Contrary to what generally assumed, at the scales covered by this study most descriptors were relatively similar between artificial and natural habitats. The only notable structural difference was inclination, which was consistently steeper in the artificial than in the natural habitats. Other minor differences observed, for example in roughness or in the abundance of some textural elements–frequently controlled in eco-engineer enhancements–were related to the general morphology (regular or irregular) of the habitat rather than to its artificial or natural identity.
Our analysis formally confirmed previous observations that artificial habitats are consistently steeper than natural shorelines (Rilov and Benayahu, 2000; Knott et al., 2004; Perkol-Finkel et al., 2006). Slope is an important factor in the design of artificial structures (Burcharth et al., 2007), affecting the stability and reflection properties of the artificial structures, as well as the costs, as milder slopes require larger volumes of construction material. Inclination is also an important determinant of the structure of assemblages of hard bottom substrata (Glasby, 2000; Glasby and Connell, 2001; Knott et al., 2004; Virgilio et al., 2006; Hanlon et al., 2018), affecting the abundance and distribution of species via changes in light, temperature, water flow, sedimentation, larval behavior and access of predators. In general seaweeds are more abundant on subvertical or horizontal surfaces, while invertebrates tend to be more abundant on steeper surfaces. Steeper surfaces also quantitatively decrease the area of intertidal habitat compared to subvertical or horizontal surfaces (Vaselli et al., 2008). As such, inclination should be a crucial factor that is considered also for an ecologically informed, “greener” design of artificial structures. In principle, efforts should be made to match more closely the milder slope of the natural habitats (Chapman and Underwood, 2011). At the same time, steeper artificial structures not only minimize material, space use and costs, but also reduce the physical footprint of the construction (i.e., Bugnot et al., 2021) on the native, often sedimentary, habitats (Schoonees et al., 2019). Any eco-engineering approach would probably require a balanced consideration of the costs and benefits of modifications in the inclination of the artificial habitats. An alternative approach could be to focus on the small- and meso-scale heterogeneity in the inclination of artificial habitats to match more closely that of natural habitats. At the small- and meso-scales artificial habitats were more homogeneous in inclination than natural habitats of comparable morphologies, and this was particularly notable for seawalls. Potential greening options to reproduce the milder slopes of natural habitats could include stepped seawalls, such as those used at Barangaroo in Sydney Harbor (Airoldi et al., 2021), or construction blocks of less uniform shapes.
Despite artificial structures are often built to provide landward shelter along wave-exposed shorelines for mooring and coastal protection, we could not detect significant differences in exposure between artificial and natural habitats, possibly due to the missing horizontal replicates. Seawalls, however, had the greatest number of plots deviating from the prevailing coastline exposure compared to all other habitats, and virtually no variations in exposure at the smallest scale. This suggests that the extensive and accelerating construction of artificial habitats such as seawalls along urbanized shorelines (Bugnot et al., 2021) might introduce considerable amounts of sheltered hard-bottom habitats at the regional scales (Airoldi et al., 2015). Exposure is a crucial determinant of rocky shore assemblages (Denny, 1988; Burrows, 2012), and shelter could facilitate the spread of non-indigenous species in artificial habitats (Floerl and Inglis, 2003; Bulleri and Airoldi, 2005). Research on the effects of the sheltered habitats introduced by artificial structures relative to natural reefs at the regional scales would be required to establish the importance of exposure as a moderator factor in eco-engineering efforts.
Increasing surface topographic complexity generally has positive effects on biodiversity in both natural and artificial habitats (Stein et al., 2014; Loke and Todd, 2016; Strain et al., 2021). These effects are related to both increased surface area and supply of microhabitats which can differ in light, temperature, humidity and predation access (Strain et al., 2018a). A recent meta-analysis of eco-engineered surfaces (Strain et al., 2018b) and a global experiment (Strain et al., 2021) have both highlighted, however, that the outcomes of topographic enhancements can be extremely variable among locations, tidal levels, latitudes and studies, warning about generalizations. Our results raise additional questions about the generalized benefits provided by topographic enhancements of artificial surfaces, as this may not always reproduce relevant natural features. In fact, in our region, we found little differences in surface structure complexity between artificial and natural habitat both when using rugosity as a proxy for topographic complexity (Shumway et al., 2007; MacArthur et al., 2019) and when applying TPI to quantify the abundance and diversity of morphological microelements. The only minor, yet significant, differences in the abundance of ridges and lower slopes were related to habitat morphology (regular and irregular) and not to habitat type, which suggests that some differences attributed so far to the artificial nature of the habitat might be in fact confounded by differences related to the morphology.
At the scales covered by this study, most descriptors of physical structure were relatively similar between artificial and natural habitats. Yet studies showed that assemblages associated to artificial and natural habitats consistently differ (Bulleri, 2005; Perkol-Finkel et al., 2006; Carvalho et al., 2013), including works in the study region (Moschella et al., 2005; Airoldi et al., 2015). This suggests that eco-engineering tests aiming to enhance the ecological performance of artificial habitats should also pay attention to other structural and non-structural elements that have been reported to differ between artificial and natural habitats, but which so far have been largely neglected (Airoldi et al., 2021). These elements include among others: construction material (Glasby, 2000; Sella and Perkol Finkel, 2015; McManus et al., 2018); careful planning of maintenance (Airoldi and Bulleri, 2011); distance from pollution sources (Strain et al., 2021); position (Glasby and Connell, 2001; Moschella et al., 2005); and the environmental (Franzitta and Airoldi, 2019), biotic (e.g., predation pressure Ferrario et al., 2016; Gianni et al., 2018), and social (Strain et al., 2019) settings. It is also important to consider that our study covered only about 8 km of coastlines in a region characterized by small tides and moderate wave action, which may affect the design of artificial structures. It is possible that in other geographical regions structural differences among artificial and natural habitats might be greater than those observed in our study region. For example, in other macrotidal regions rock pools (which were virtually absent at our natural sites) might be a relevant element causing structural differences between artificial and natural structures at small/medium scales (Aguilera et al., 2014), which would justify their engineered enhancement (Firth et al., 2014a). Also the scales covered by the study should be expanded in future studies, in order to identify at which scale structural differences between the two habitats might become more relevant.
In conclusion, our results challenge the widespread simplified assumption that artificial habitats lack the physical structure proper to natural reefs. The structural difference between a seawall and a rocky reef could be less than that between a seawall and a rubble-mound breakwater, and at small and meso-scales a rubble-mound breakwater could be more heterogeneous (i.e., in inclination) than a subvertical rocky cliff. This encourages a wider reflection about what makes a construction surface “greener”: surface topographic complexity is an important ecological parameter, and its deliberate increase will likely lead to responses in the biota. However, if the intervention is not designed to mimic structural natural patters at the adequate scale, increasing habitat complexity may not lead to assemblages that converge toward a “more natural” state.
Data Availability Statement
The original data presented in the study are publicly available on figshare: doi: 10.6084/m9.figshare.16918465.
Author Contributions
LA and FG conceived the idea and designed the methodology. FG collected and analyzed the data and contributed to the writing. LA lead the writing. Both authors contributed to the article and approved the submitted version.
Funding
Funding was from project POR FSE 2014/2020, from Regione Emilia Romagna.
Conflict of Interest
The authors declare that the research was conducted in the absence of any commercial or financial relationships that could be construed as a potential conflict of interest.
Publisher’s Note
All claims expressed in this article are solely those of the authors and do not necessarily represent those of their affiliated organizations, or those of the publisher, the editors and the reviewers. Any product that may be evaluated in this article, or claim that may be made by its manufacturer, is not guaranteed or endorsed by the publisher.
Acknowledgments
We thank Paolo Comandini, Francesco Mugnai, Sara Scapinello, and Eva Turicchia for help in the field. We also thank Lisandro Benedetti-Cecchi and Martina Dal Bello for assistance with spatial variability analysis.
Supplementary Material
The Supplementary Material for this article can be found online at: https://www.frontiersin.org/articles/10.3389/fmars.2021.766903/full#supplementary-material
References
Aguilera, M. A., Broitman, B. R., and Thiel, M. (2014). Spatial variability in community composition on a granite breakwater versus natural rocky shores: lack of microhabitats suppresses intertidal biodiversity. Mar. Pollut. Bull. 87, 257–268. doi: 10.1016/j.marpolbul.2014.07.046
Airoldi, L., Abbiati, M., Beck, M. W., Hawkins, S. J., Jonsson, P. R., Martin, D., et al. (2005). An ecological perspective on the deployment and design of low-crested and other hard coastal defence structures. Coast. Eng. 52, 1073–1087. doi: 10.1016/j.coastaleng.2005.09.007
Airoldi, L., and Beck, M. (2007). Loss, status and trends for coastal marine habitats of Europe. Oceanogr. Mar. Biol. 45, 345–405. doi: 10.1201/9781420050943.ch7
Airoldi, L., Beck, M. W., Firth, L. B., Bugnot, A. B., Steinberg, P. D., and Dafforn, K. A. (2021). Emerging solutions to return nature to the urban ocean. Annu. Rev. Mar. Sci. 13, 445–477. doi: 10.1146/annurev-marine-032020-020015
Airoldi, L., and Bulleri, F. (2011). Anthropogenic disturbance can determine the magnitude of opportunistic species responses on marine urban infrastructures. PLoS One 6:e0022985. doi: 10.1371/journal.pone.0022985
Airoldi, L., Turon, X., Perkol-Finkel, S., and Rius, M. (2015). Corridors for aliens but not for natives: effects of marine urban sprawl at a regional scale. Divers. Distribut. 21, 755–768. doi: 10.1111/ddi.12301
Anderson, M., Gorley, R. N., and Clarke, K. R. (2008). PERMANOVA+ for PRIMER: Guide to Software and Statistical Methods. Plymouth: PRIMER-E Ltd.
Bayley, D. T. I., Mogg, A. O. M., Koldewey, H., and Purvis, A. (2019). Capturing complexity: field-testing the use of “structure from motion” derived virtual models to replicate standard measures of reef physical structure. PeerJ 2019, 1–17. doi: 10.7717/peerj.6540
Benedetti-Cecchi, L. (2003). The importance of the variance around the mean effect size of ecological processes. Ecology 84, 2335–2346. doi: 10.1890/02-8011
Bugnot, A. B., Mayer-Pinto, M., Airoldi, L., Heery, E. C., Johnston, E. L., Critchley, L. P., et al. (2021). Current and projected global extent of marine built structures. Nat. Sustain. 4, 33–41. doi: 10.1038/s41893-020-00595-1
Bulleri, F. (2005). Experimental evaluation of early patterns of colonisation of space on rocky shores and seawalls. Mar. Environ. Res. 60, 355–374. doi: 10.1016/j.marenvres.2004.12.002
Bulleri, F., and Airoldi, L. (2005). Artificial marine structures facilitate the spread of a non-indigenous green alga, Codium fragile ssp. tomentosoides, in the north Adriatic Sea. J. Appl. Ecol. 42, 1063–1072. doi: 10.1111/j.1365-2664.2005.01096.x
Bulleri, F., and Chapman, M. G. (2004). Intertidal assemblages on artificial and natural habitats in marinas on the north-west coast of Italy. Mar. Biol. 145, 381–391. doi: 10.1007/s00227-004-1316-8
Burcharth, H. F., Hawkins, S. J., Zanuttigh, B., and Lamberti, A. (2007). Environmental Design Guidelines for Low Crested Coastal Structures. Amsterdam: Elsevier, doi: 10.1016/B978-0-08-044951-7.X5019-2
Burrows, M. T. (2012). Influences of wave fetch, tidal flow and ocean colour on subtidal rocky communities. Mar. Ecol. Prog. Ser. 445, 193–207. doi: 10.3354/meps09422
Burt, J., Bartholomew, A., Usseglio, P., Bauman, A., and Sale, P. F. (2009). Are artificial reefs surrogates of natural habitats for corals and fish in Dubai, United Arab Emirates? Coral Reefs 28, 663–675. doi: 10.1007/s00338-009-0500-1
Carvalho, S., Moura, A., Cúrdia, J., Cancela da Fonseca, L., and Santos, M. N. (2013). How complementary are epibenthic assemblages in artificial and nearby natural rocky reefs? Mar. Environ. Res. 92, 170–177. doi: 10.1016/j.marenvres.2013.09.013
Chapman, M. G., and Underwood, A. J. (2011). Evaluation of ecological engineering of “armoured” shorelines to improve their value as habitat. J. Exp. Mar. Biol. Ecol. 400, 302–313. doi: 10.1016/j.jembe.2011.02.025
Dafforn, K. A., Glasby, T. M., Airoldi, L., Rivero, N. K., Mayer-Pinto, M., and Johnston, E. L. (2015). Marine urbanization: an ecological framework for designing multifunctional artificial structures. Front. Ecol. Environ. 13:82–90. doi: 10.1890/140050
Dal Bello, M., Leclerc, J. C., Benedetti-Cecchi, L., De Lucia, G. A., Arvanitidis, C., Van Avesaath, P., et al. (2017). Consistent patterns of spatial variability between NE Atlantic and mediterranean rocky shores. J. Mar. Biol. Assoc. U. K. 97, 539–547. doi: 10.1017/S0025315416001491
De Reu, J., Bourgeois, J., Bats, M., Zwertvaegher, A., Gelorini, V., De Smedt, P., et al. (2013). Application of the topographic position index to heterogeneous landscapes. Geomorphology 186, 39–49. doi: 10.1016/j.geomorph.2012.12.015
Denny, M. (1988). Biology and the Mechanics of the Wave-Swept Environment. Princeton, NJ: Princenton University Press, doi: 10.1515/9781400852888
Fauvelot, C., Bertozzi, F., Costantini, F., Airoldi, L., and Abbiati, M. (2009). Lower genetic diversity in the limpet Patella caerulea on urban coastal structures compared to natural rocky habitats. Mar. Biol. 156, 2313–2323. doi: 10.1007/s00227-009-1259-1
Ferrario, F., Iveša, L., Jaklin, A., Perkol-Finkel, S., and Airoldi, L. (2016). The overlooked role of biotic factors in controlling the ecological performance of artificial marine habitats. J. Appl. Ecol. 53, 16–24. doi: 10.1111/1365-2664.12533
Figueira, W., Ferrari, R., Weatherby, E., Porter, A., Hawes, S., and Byrne, M. (2015). Accuracy and precision of habitat structural complexity metrics derived from underwater photogrammetry. Remote Sens. 7, 16883–16900. doi: 10.3390/rs71215859
Firth, L. B., Knights, A. M., Bridger, D., Evans, A. J., Mieszkowska, N., Moore, P. J., et al. (2016). Ocean sprawl: challenges and opportunities for biodiversity management in a changing world. Oceanogr. Mar. Biol. 54, 189–262.
Firth, L. B., Schofield, M., White, F. J., Skov, M. W., and Hawkins, S. J. (2014a). Biodiversity in intertidal rock pools: informing engineering criteria for artificial habitat enhancement in the built environment. Mar. Environ. Res. 102, 122–130. doi: 10.1016/j.marenvres.2014.03.016
Firth, L. B., Thompson, R. C., Bohn, K., Abbiati, M., Airoldi, L., Bouma, T. J., et al. (2014b). Between a rock and a hard place: environmental and engineering considerations when designing coastal defence structures. Coast. Eng. 87, 122–135. doi: 10.1016/j.coastaleng.2013.10.015
Floerl, O., and Inglis, G. J. (2003). Boat harbour design can exacerbate hull fouling. Austr. Ecol. 28, 116–127. doi: 10.1046/j.1442-9993.2003.01254.x
Franzitta, G., and Airoldi, L. (2019). Fish assemblages associated with coastal defence structures: does the surrounding habitat matter? Reg. Stud. Mar. Sci. 31:100743. doi: 10.1016/j.rsma.2019.100743
Gianni, F., Bartolini, F., Airoldi, L., and Mangialajo, L. (2018). Reduction of herbivorous fish pressure can facilitate focal algal species forestation on artificial structures. Mar. Environ. Res. 138, 102–109. doi: 10.1016/j.marenvres.2018.04.007
Glasby, T. M. (2000). Surface composition and orientation interact to affect subtidal epibiota. J. Exp. Mar. Biol. Ecol. 248, 177–190. doi: 10.1016/S0022-0981(00)00169-6
Glasby, T. M., and Connell, S. D. (2001). Orientation and position of substrata have large effects on epibiotic assemblages. Mar. Ecol. Prog. Ser. 214, 127–135. doi: 10.3354/meps214127
Hanlon, N., Firth, L. B., and Knights, A. M. (2018). Time-dependent effects of orientation, heterogeneity and composition determines benthic biological community recruitment patterns on subtidal artificial structures. Ecol. Eng. 122, 219–228. doi: 10.1016/j.ecoleng.2018.08.013
Heery, E. C., Bishop, M. J., Critchley, L. P., Bugnot, A. B., Airoldi, L., Mayer-Pinto, M., et al. (2017). Identifying the consequences of ocean sprawl for sedimentary habitats. J. Exp. Mar. Biol. Ecol. 492, 31–48. doi: 10.1016/j.jembe.2017.01.020
Jammalamadaka, S. R., and SenGupta, A. (2001). Topics in Circular Statistics. Singapore: World Scientific, doi: 10.1142/4031
Knott, N. A., Underwood, A. J., Chapman, M. G., and Glasby, T. M. (2004). Epibiota on vertical and on horizontal surfaces on natural reefs and on artificial structures. J. Mar. Biol. Assoc. U. K. 84, 1117–1130. doi: 10.1017/S0025315404010550h
Komyakova, V., Chamberlain, D., Jones, G. P., and Swearer, S. E. (2019). Assessing the performance of artificial reefs as substitute habitat for temperate reef fishes: implications for reef design and placement. Sci. Total Environ. 668, 139–152. doi: 10.1016/j.scitotenv.2019.02.357
Kovalenko, K. E., Thomaz, S. M., and Warfe, D. M. (2012). Habitat complexity: approaches and future directions. Hydrobiologia 685, 1–17. doi: 10.1007/s10750-011-0974-z
Loke, L. H. L., Jachowski, N. R., Bouma, T. J., Ladle, R. J., and Todd, P. A. (2014). Complexity for artificial substrates (CASU): software for creating and visualising habitat complexity. PLoS One 9:e0087990. doi: 10.1371/journal.pone.0087990
Loke, L. H. L., Ladle, R. J., Bouma, T. J., and Todd, P. A. (2015). Creating complex habitats for restoration and reconciliation. Ecol. Eng. 77, 307–313. doi: 10.1016/j.ecoleng.2015.01.037
Loke, L. H. L., and Todd, P. A. (2016). Structural complexity and component type increase intertidal biodiversity independently of area. Ecology 97, 383–393. doi: 10.1890/15-0257.1
MacArthur, M., Naylor, L. A., Hansom, J. D., Burrows, M. T., Loke, L. H. L., and Boyd, I. (2019). Maximising the ecological value of hard coastal structures using textured formliners. Ecol. Eng. 1:100002. doi: 10.1016/j.ecoena.2019.100002
Mardia, K. V., and Jupp, P. E. (2000). Directional Statistic. Chichester: Academic Press Inc. (London) Ltd.
McManus, R. S., Archibald, N., Comber, S., Knights, A. M., Thompson, R. C., and Firth, L. B. (2018). Partial replacement of cement for waste aggregates in concrete coastal and marine infrastructure: a foundation for ecological enhancement? Ecol. Eng. 120, 655–667. doi: 10.1016/j.ecoleng.2017.06.062
Morris, R. L., Chapman, M. G., Firth, L. B., and Coleman, R. A. (2017). Increasing habitat complexity on seawalls: investigating large- and small-scale effects on fish assemblages. Ecol. Evol. 7, 9567–9579. doi: 10.1002/ece3.3475
Morris, R. L., Heery, E. C., Loke, L. H. L., Lau, E., Strain, E. M. A., Airoldi, L., et al. (2019). Design options, implementation issues and evaluating success of ecologically engineered shorelines. Oceanogr. Mar. Biol. 57, 169–228. doi: 10.1201/9780429026379-4
Moschella, P. S., Abbiati, M., Åberg, P., Airoldi, L., Anderson, J. M., Bacchiocchi, F., et al. (2005). Low-crested coastal defence structures as artificial habitats for marine life: using ecological criteria in design. Coast. Eng. 52, 1053–1071. doi: 10.1016/j.coastaleng.2005.09.014
O’Shaughnessy, K. A., Hawkins, S. J., Evans, A. J., Hanley, M. E., Lunt, P., Thompson, R. C., et al. (2020). Design catalogue for eco-engineering of coastal artificial structures: a multifunctional approach for stakeholders and end-users. Urban Ecosyst. 23, 431–443. doi: 10.1007/s11252-019-00924-z
Perkol-Finkel, S., Ferrario, F., Nicotera, V., and Airoldi, L. (2012). Conservation challenges in urban seascapes: promoting the growth of threatened species on coastal infrastructures. J. Appl. Ecolo. 49, 1457–1466. doi: 10.1111/j.1365-2664.2012.02204.x
Perkol-Finkel, S., Shashar, N., and Benayahu, Y. (2006). Can artificial reefs mimic natural reef communities? The roles of structural features and age. Mar. Environ. Res. 61, 121–135. doi: 10.1016/j.marenvres.2005.08.001
Rilov, G., and Benayahu, Y. (2000). Fish assemblage on natural versus vertical artificial reefs: the rehabilitation perspective. Mar. Biol. 136, 931–942. doi: 10.1007/s002279900250
Schoonees, T., Gijón Mancheño, A., Scheres, B., Bouma, T. J., Silva, R., Schlurmann, T., et al. (2019). Hard structures for coastal protection, towards greener designs. Estuar. Coast. 42, 1709–1729. doi: 10.1007/s12237-019-00551-z
Sella, I., and Perkol Finkel, S. (2015). Blue is the new green - Ecological enhancement of concrete based coastal and marine infrastructure. Ecol. Eng. 84, 260–272. doi: 10.1016/j.ecoleng.2015.09.016
Shepard, M. K., Campbell, B. A., Bulmer, M. H., Farr, T. G., Gaddis, L. R., and Plaut, J. J. (2001). The roughness of natural terrain: a planetary and remote sensing perspective. J. Geophys. Res. E Planets 106, 32777–32795. doi: 10.1029/2000JE001429
Shumway, C. A., Hofmann, H. A., and Dobberfuhl, A. P. (2007). Quantifying habitat complexity in aquatic ecosystems. Freshw. Biol. 52, 1065–1076. doi: 10.1111/j.1365-2427.2007.01754.x
Stein, A., Gerstner, K., and Kreft, H. (2014). Environmental heterogeneity as a universal driver of species richness across taxa, biomes and spatial scales. Ecol. Lett. 17, 866–880. doi: 10.1111/ele.12277
Strain, E. M. A., Alexander, K. A., Kienker, S., Morris, R., Jarvis, R., Coleman, R., et al. (2019). Urban blue: a global analysis of the factors shaping people’s perceptions of the marine environment and ecological engineering in harbours. Sci. Total Environ. 658, 1293–1305. doi: 10.1016/j.scitotenv.2018.12.285
Strain, E. M. A., Steinberg, P. D., Vozzo, M., Johnston, E. L., Abbiati, M., Aguilera, M. A., et al. (2021). A global analysis of complexity–biodiversity relationships on marine artificial structures. Glob. Ecol. Biogeogr. 30, 140–153. doi: 10.1111/geb.13202
Strain, E. M. A., Morris, R. L. L., Coleman, R. A. A., Figueira, W. F. F., Steinberg, P. D. D., Johnston, E. L. L., et al. (2018a). Increasing microhabitat complexity on seawalls can reduce fish predation on native oysters. Ecol. Eng. 120, 637–644. doi: 10.1016/j.ecoleng.2017.05.030
Strain, E. M. A., Olabarria, C., Mayer-Pinto, M., Cumbo, V., Morris, R. L., Bugnot, A. B., et al. (2018b). Eco-engineering urban infrastructure for marine and coastal biodiversity: which interventions have the greatest ecological benefit? J. Appl. Ecol. 55, 426–441. doi: 10.1111/1365-2664.12961
Vaselli, S., Bertocci, I., Maggi, E., and Benedetti-Cecchi, L. (2008). Assessing the consequences of sea level rise: effects of changes in the slope of the substratum on sessile assemblages of rocky seashores. Mar. Ecol. Prog. Ser. 368, 9–22. doi: 10.3354/meps07625
Virgilio, M., Airoldi, L., and Abbiati, M. (2006). Spatial and temporal variations of assemblages in a Mediterranean coralligenous reef and relationships with surface orientation. Coral Reefs 25, 265–272. doi: 10.1007/s00338-006-0100-2
Warfe, D. M., Barmuta, L. A., and Wotherspoon, S. (2008). Quantifying habitat structure: surface convolution and living space for species in complex environments. Oikos 117, 1764–1773. doi: 10.1111/j.1600-0706.2008.16836.x
Weiss, A. (2001). “Topographic position and landforms analysis,” in Poster Presentation, ESRI User Conference, Vol. 64, San Diego, CA, 227–245.
Westoby, M. J., Brasington, J., Glasser, N. F., Hambrey, M. J., and Reynolds, J. M. (2012). “Structure-from-Motion” photogrammetry: a low-cost, effective tool for geoscience applications. Geomorphology 179, 300–314. doi: 10.1016/j.geomorph.2012.08.021
Keywords: artificial marine structure, greening of gray infrastructure, habitat complexity, intertidal rocky shore, spatial variability, structure from motion, surface design, topography
Citation: Grasselli F and Airoldi L (2021) How and to What Degree Does Physical Structure Differ Between Natural and Artificial Habitats? A Multi-Scale Assessment in Marine Intertidal Systems. Front. Mar. Sci. 8:766903. doi: 10.3389/fmars.2021.766903
Received: 30 August 2021; Accepted: 25 October 2021;
Published: 17 November 2021.
Edited by:
Susana Carvalho, King Abdullah University of Science and Technology, Saudi ArabiaReviewed by:
Rodrigo Riera, University of Las Palmas de Gran Canaria, SpainAnna Maria Addamo, European Commission, Joint Research Centre (JRC), Italy
Copyright © 2021 Grasselli and Airoldi. This is an open-access article distributed under the terms of the Creative Commons Attribution License (CC BY). The use, distribution or reproduction in other forums is permitted, provided the original author(s) and the copyright owner(s) are credited and that the original publication in this journal is cited, in accordance with accepted academic practice. No use, distribution or reproduction is permitted which does not comply with these terms.
*Correspondence: Laura Airoldi, bGF1cmEuYWlyb2xkaUB1bmlwZC5pdA==