- 1Hatherly Laboratories, College of Life and Environmental Sciences, University of Exeter, Exeter, United Kingdom
- 2Demersal Division, Marine and Freshwater Research Institute, Reykjavík, Iceland
- 3Teignmouth, United Kingdom
- 4School of Interdisciplinary Arts & Sciences, University of Washington Bothell, Bothell, WA, United States
- 5University Centre of the Westfjords, University of Akureyri, Ísafjörður, Iceland
- 6Met Office, Exeter, United Kingdom
- 7School of Social Sciences, University of Iceland, Reykjavík, Iceland
During the breeding season, seabirds are central place foragers and in order to successfully rear chicks they must adjust their foraging behaviours to compensate for extrinsic factors. When foraging, arctic terns Sterna paradisaea are restricted to the first 50 cm of the water column and can only carry a few prey items back to their nests at once. In Iceland, where 20–30% of the global population breed, poor fledging success has been linked to low food availability. Using GPS loggers, we investigated individual foraging behaviours of breeding adults during incubation from a large colony over four seasons. First, we tested whether foraging trip distance or duration was linked to morphology or sex. Second, we examined how trips vary with weather and overlap with commercial fisheries. Our findings reveal that arctic terns travel far greater distances during foraging trips than previously recorded (approximately 7.3 times further), and they forage around the clock. There was inter-annual variability in the foraging locations that birds used, but no relationship between size or sex differences and the distances travelled. We detected no relationship between arctic tern foraging flights and local prevailing winds, and tern heading and speed were unrelated to local wind patterns. We identified key arctic tern foraging areas and found little spatial or temporal overlap with fishing pelagic vessels, but larger home ranges corresponded with years with lower net primary productivity levels. This suggests that whilst changing polar weather conditions may not pose a threat to arctic terns at present, nor might local competition with commercial fisheries for prey, they may be failing to forage in productive areas, or may be affected by synergistic climatic effects on prey abundance and quality. Shifts in pelagic prey distributions as a result of increasing water temperatures and salinities will impact marine top predators in this region, so continued monitoring of sentinel species such as arctic terns is vital.
Introduction
Globally, populations of seabirds are declining, and pelagic species with expansive ranges are particularly vulnerable (Paleczny et al., 2015). There are numerous threats to seabirds including predation, disturbance and habitat loss, which particularly affect them during breeding, and bycatch, pollution and overfishing while they are at sea for much of the rest of the year (Croxall et al., 2012; Dias et al., 2019). As bioindicators, seabirds can offer insight into marine ecosystem changes (Piatt et al., 2007). They travel between profitable prey patches, and reduce movement when they reach profitable areas (Weimerskirch, 2007), so tracking seabirds can indicate areas of prey occurrence (Piatt et al., 2007). Seabird foraging ranges can be used to identify candidate locations for protection (Thaxter et al., 2012), and protecting these areas could benefit whole ecosystems (Hooker and Gerber, 2004).
While it is particularly challenging to understand and mitigate threats across seabirds’ annual migratory ranges, which can be hundreds of thousands of square kilometres (Lascelles et al., 2014), there are opportunities to focus on threats and management at their breeding areas, which tend to be much more spatially restricted. In this study, we investigate the overlap of tern foraging areas with local fisheries and the impact of weather on the intra-breeding season foraging behaviour of arctic terns Sterna paradisaea, which are known to have poor breeding success in years with low prey abundance (Monaghan et al., 1992; Vigfúsdóttir et al., 2013).
Arctic terns annually undertake the longest recorded migration of any organism (Egevang et al., 2010), travelling over 100,000 km (Volkov et al., 2017) from circumpolar breeding locations in the Temperate (southernmost at 41°N) and Polar zones to overwintering sites in the Southern Ocean (Hatch, 2002). Populations appear to be declining at many locations across the breeding range [e.g., in Canadian High Arctic (Mallory et al., 2018), Western Iceland (Petersen et al., 2020), Greenland (Burnham et al., 2017), and Norway (Barrett et al., 2006)]. Generally there is high adult survival (Devlin et al., 2008; Mallory et al., 2018), with very high regional (Egevang and Frederiksen, 2011) and colonial breeding site fidelity (Devlin et al., 2008). Declines are therefore thought to be likely due to low recruitment or survival of juveniles, although some dispersal of adults to new breeding sites is possible (Vigfúsdóttir et al., 2013; Mallory et al., 2018). Food availability during the early stages of breeding will influence recruitment by altering laying date, clutch size or leading to egg abandonment (Suddaby and Ratcliffe, 1997). In Iceland, the collapse of sandeels (Ammodytes sp.), a key prey species, has coincided with lower adult survival since 2000 (Petersen et al., 2020), and low fledgling rates caused by starvation (Vigfúsdóttir et al., 2013). It is therefore key to understand how and where arctic terns forage during the breeding season, and whether their behaviour alters in relation to weather and fish stocks.
Wind is a key abiotic factor that impacts seabird demography; it can affect adult survival (Frederiksen et al., 2008), or breeding success (e.g., Robinson et al., 2002; Mallory et al., 2009; Johnson and Colombelli-Négrel, 2021). Changes to wind patterns can alter foraging seabird behaviour, with strong winds impacting trip duration, flight speeds, trip success (e.g., foraging mass gain, capturing smaller prey, difficulty locating prey) and target prey species (Finney et al., 1999; Weimerskirch et al., 2012; Dehnhard et al., 2013; Elliott et al., 2014; Saraux et al., 2016). Breeding seabirds have been shown to mitigate the impact of strong winds during foraging trips by altering foraging search strategies (e.g., Scopoli’s shearwaters, Calonectris diomedea; De Pascalis et al., 2020), flight altitudes (e.g., Antarctic petrels, Thalassoica antarctica; Tarroux et al., 2016), and altering flight air speeds (Spear and Ainley, 1997). In years with higher wind speeds, flight may be more costly for arctic terns, particularly if it is greater than their minimum power speed (i.e., the most energetically efficient speed to fly, 6.8 m.s–1; Hedenström and Åkesson, 2016). During migratory flights, arctic terns adjusted air speed in relation to side-winds, but did not fully compensate for wind drift (Hedenström and Åkesson, 2016). It is therefore possible that during foraging trips arctic terns could also be impacted by wind speeds and may compensate by adjusting heading or air speed in order to maintain a course. In years with stronger winds, foraging trips may therefore be more energetically demanding. However, moderate increases in wind speed could have a positive effect on prey capture rate. For example, Sandwich terns Sterna sandvicensis exerted less effort hovering prior to a dive and the prey were less likely to see them in moderate winds due to the choppy waters (Dunn, 1973). Additionally, as a driver of oceanographic processes such as upwelling and vertical mixing, wind conditions can also impact prey availability and distribution (e.g., Cox et al., 2018). Therefore wind is likely to have indirect effects on arctic tern foraging behaviour as well.
Arctic terns have the capability to fly at high altitudes. During migration, radar observations recorded arctic terns flying at altitudes greater than 1,000 m (Gudmundsson et al., 1992) and they have been recorded traversing the Andes (Duffy et al., 2013). Arctic terns generally fly low whilst searching for food (Hedenström and Åkesson, 2016), but likely higher if transiting to a prey patch. We aim to describe arctic tern foraging flight altitudes using GPS devices for the first time.
Arctic terns feed on small schooling fish such as herring Clupeidae, sandeels, capelin Mallotus villosus, or crustaceans such as krill Euphausiacea (Hatch, 2002). They apparently have short foraging ranges, generally remaining within 10 km of the colony, only occasionally travelling further (Rock et al., 2007; Cabot and Nisbet, 2013). Foraging trip duration and distance are further constrained during chick rearing by arctic terns being limited to carrying only one to a few prey items at a time, and by shallow plunge dives to a maximum depth of 50 cm (Hatch, 2002). Arctic terns are therefore particularly vulnerable to local food shortages (Robertson et al., 2014b), such that smaller clutches are laid in response to lower quality food during courtship feeding (Suddaby and Ratcliffe, 1997), brood reduction is more likely in nests where adults have lower body condition (Monaghan et al., 1989), and breeding attempts are usually abandoned in years with low food availability (Monaghan et al., 1992). In addition, prey availability during the early stages of breeding can also impact the body condition of Sterna species, with significant declines in incubating female common tern S. hirundo body mass reported in poor foraging conditions (Wendeln and Becker, 1996). Understanding arctic tern intra-seasonal foraging ecology is vital to investigate a key cause of low breeding success (Vigfúsdóttir et al., 2013), and could also identify early signs of changing conditions that will affect other seabird species foraging further from the coast (Pratte et al., 2018).
Iceland supports c. 20–30% of the world’s population of breeding arctic terns (Asbirk et al., 1997), a population now listed on the Icelandic Red List as vulnerable due to recent population decline (Icelandic Institute of Natural History, 2018). In the warmer waters in the south and west of Iceland, seabirds rely mainly on sandeels, and in the cooler waters in the north and east on capelin and krill (Lilliendahl, 2009). Following the collapse of the sandeel population in 2000, annual arctic tern adult survival reduced by 10% in west Iceland (Petersen et al., 2020). The present study focuses on the foraging behaviours of arctic terns in the west and southwest regions (west of the Reykjanes peninsula and Faxaflói bay), and highlights potentially important foraging areas that are used by commercial fisheries.
Today, 38% of the Icelandic coastal fleet operate in the west and southwest regions (ϸórðarson and Viðarsson, 2014), and in 2014 the majority of landings occurred at the harbour in Reykjavík (Edvardsson et al., 2018). The coastal fleet primarily lands cod Gadus morhua, which feeds on similar pelagic species to arctic terns, but the fleet also lands pelagic species such as lumpfish Cyclopterus lumpus, another arctic tern prey species (ϸórðarson and Viðarsson, 2014). Demersal fisheries are the most valuable sector but the landed tonnage of pelagic fisheries, although very variable, is generally greater than that of the demersal (Edvardsson et al., 2018) and consists of c. 60–70% of the average annual catch by weight (Saevaldsson and Gunnlaugsson, 2015). Historically the pelagic fisheries in Iceland landed mostly capelin (a known prey species of arctic terns and other seabirds off the north coast of Iceland, but have not been documented in their diet in the south) for the fishmeal industry (generally greater than 80% of pelagic landings), but due to declines in capelin stocks and new, more valuable species (e.g., herring Clupea harengus and mackerel Scomber scombrus) entering Iceland’s waters, capelin landings have declined recently (Saevaldsson and Gunnlaugsson, 2015). Given the importance of this area for breeding arctic terns and other seabird species (Garðarsson, 1999), the link between poor fledging success and food availability (Vigfúsdóttir et al., 2013; Petersen et al., 2020), and the increase in pelagic landings in the region (Edvardsson et al., 2018), it is important to investigate the potential impact of the fisheries industry. Addressing factors that influence arctic tern foraging success will shed light on the potential causes of the declines, which is key to preserve this population, and will also benefit the wide range of other species that breed in the rich waters around Iceland.
We aim to: (1) describe for the first time where and how far away from the colony Icelandic arctic terns forage, (2) to understand whether these movements can be explained by local wind conditions, and (3) to understand to what extent the movements and key foraging areas may overlap with local fisheries.
Materials and Methods
Field Site
On the Southern Reykjanes Peninsula in Iceland is a breeding site with >10,000 pairs of arctic terns (near Sandgerði, Iceland, 64.015°N, 22.707°W). The colony is located in a privately owned site, Norðurkot farm, used as a nesting area for eider ducks (Somateria mollissima), and operated as a wild down farm. The study site was accessed annually, with landowner permission, for 3–4 weeks during June 2018, 2019, 2020, and 2021 for tracking device deployment and retrieval. Predation of arctic tern eggs and chicks was minimal due to local landowners monitoring and removing predators with 24/7 predator watches.
Animal Capture
Since June 2018, 331 breeding arctic terns have been caught and ringed at the study site using a tent spring trap (Moudry traps: TW45). The primed trap is placed on a nest with a trigger line over the eggs, which is triggered by the incubating bird landing back on the nest. This method does not cause damage to the adult or the eggs. The eggs in each nest were measured and the stage of development was determined with egg flotation (Liebezeit et al., 2007) adapted for arctic terns (Supplementary Figure 1). Any arctic tern captured with eggs less than a third of the way through development was not considered for tracking device deployment as birds captured on early stage eggs were more likely to abandon their nests (Vigfúsdóttir, Pers. Comm.). All birds were ringed on their right tarsus with a unique metal ring from the Icelandic Institute of Natural History, and 260 were fitted with leg flags (blue with a white two character alpha code, sealed with waterproof plastic glue) on the left tarsus. All captured terns were measured (head and bill length: from the centre of the back of skull to the tip of the bill, bill length: from the start of feathering to the tip, and bill depth: on a closed bill at the nostrils) with Vernier callipers (to 0.1 mm). Wing length was measured (1.0 mm accuracy) from the carpal joint to the tip of the longest primary and mass was measured using a cotton bag with a Pesola spring balance (to 1.0 g accuracy). Six breast feathers were plucked with the calamus attached and stored dry at room temperature in an envelope to determine sex using molecular techniques adapted from Fridolfsson and Ellegren (1999; Supplementary Material).
Tag Deployment
Tracking devices (Pathtrack GPS nanoFix Geo + RF) were deployed for one breeding season on 29 arctic terns, in June 2019 (n = 11), June 2020 (n = 11) and June 2021 (n = 7). They were scheduled to record GPS locations every 15 min and would transmit these data to a base station that was placed in the centre of the colony at a height of c. 2–5 m. Additionally, two archival devices were deployed in 2018 (Pathtrack GPS nanoFix Geo) and were recaptured within the same season; these were scheduled to record locations every 30 min. Tags were attached using a leg loop harness (using 2.54 mm Spectra tape, Bally Ribbons, PA, United States), and weighed 2.9 g (± 0.3 g), which is approximately 2.65% of the mean mass of all arctic terns caught and ringed to date. The device was positioned on the lower back of the tern, with the antennae pointing toward the tail. Total handling time was always less than 12 min. Only arctic terns with a mass >100 g, and those that returned to incubate their eggs within 5 min after the trap was set, had tracking devices attached. Any individuals that were captured after more than 5 min were measured, ringed, and released without tracking devices, so that they could be considered as a control treatment to understand the effect of tracking tags on post release survival and nest return rate. If no incubating adult returned to the nest after 10 min the trap was removed. The nests of the tracked birds were monitored regularly during the deployment period between 05:00 and 00:00. Recapture of arctic terns carrying tracking devices was attempted prior to egg hatching within the same season.
Data Retrieval and Recapture
Of the 11 deployed tracking devices in 2019, nine were recaptured and the data successfully downloaded. No data were received remotely from the other two devices, and these birds abandoned their nests so device recapture was not possible. In 2020 data were received remotely from all 11 devices, and seven individuals were recaptured and the devices removed. The other four abandoned their breeding attempt, but continued to visit the colony, and data were therefore successfully downloaded remotely. In 2021 data were received from all seven devices, and four individuals were recaptured and devices removed. The other three did not return to their nests. Recapture and device removal was not possible without the birds returning to a consistent location. Additionally, two archival GPS devices were deployed and recaptured during the 2018 field season.
Wind Modelling
In order to investigate the effect of environmental variables, wind data were obtained from operational forecasts of the UK Met Office global deterministic numerical weather prediction model (Walters et al., 2019), with hourly wind forecasts for the duration of each field season on a horizontal grid of approximately 10-km spacing. Arctic terns consistently flew at a median height of 62.75 m above sea level (MASL; Supplementary Figure 2), so the modelled wind speeds used in all analyses were at 50 MASL, the closest height available. The model outputs included the u (zonal i.e., west: east) and v (meridional i.e., north: south) components of wind in netcdf format and these were accessed using the ncdf4 R package (Pierce, 2019). Wind direction and speed were calculated using the rWind R package (Fernández-López and Schliep, 2018). The foraging season temporal mean wind speed and direction at each grid location was determined by calculating the mean of the u and v wind components separately at each location across the duration of the available tracking data, and the mean speed and direction at each location could be derived from these values [square root (mean u2 + mean v2)].
Net Primary Productivity
Vertically generalised production model (VGPM) “chlorophyll-based” net primary productivity (NPP) monthly averaged data (mg C m–2 day–1) were downloaded from the standard products section of the Ocean Productivity website in hdf format on a global grid of 1,080 × 2,160 (Behrenfield and Falkowski, 1997).1 Data were only available during the first 3 years of the study (2018–2020), and only productivity levels during the tracking period in June were used in analyses. Hdf files were opened in R using the raster package, and the raster layers were cropped to the area around the southwest peninsula of Iceland.
Overlap Between Arctic Terns and Fishing Vessels
Vessels in Icelandic waters are recorded using vessel monitoring systems implemented by the ICG Operations Centre, with the Icelandic Directorate of Fisheries (Fiskistofa) responsible for fishing related activities. The integrated system includes monitoring activities through radio-based systems such as the global Automatic Identification System (AIS), which records the locations of any vessels over 15 m in length and extends 30–60 nautical miles from shore, and satellite based systems that record the movements of all vessels through transmitters fitted to ships that send data to a land-based station via a satellite system (Geirsson, 2011).
Fishing vessel data were available from 1st June to 30th June (the period at this site where breeding arctic terns are incubating and chick rearing) for each of the four field seasons with tracking data (2018–2021), and in the range that the arctic terns travelled (63°N–65°N and 25°W–22.5°W). The data provided by Fiskistofa were downsampled to a position every 10 min and only included vessel locations whilst fishing, which was determined through a combination of logbook records and a speed filter. Demersal gear types were not used in analysis, and pelagic fishing gear types (longline, gillnet and handline) were retained (a total of 26,800 pelagic fishing gear type locations of 184,309 total locations during the 4 years). The frequency that they occurred in 0.01 degree grids were calculated with the raster package (Hijmans, 2020). The frequency in which arctic terns and vessels overlapped temporally as well as spatially was determined by matching tern locations within 1 km of vessels and 1 h and within 2 km of vessels and 6 h. The temporal scales were selected as they would include the average duration of a foraging trip recorded in this study, and the spatial scales were arbitrarily selected to be cautious and should be generous enough to include overlap.
Data and Analysis
Unless otherwise stated all analyses were conducted in R (R Core Team, 2018) and spatial calculations used the geosphere R package (Hijmans et al., 2017) using projected data (EPSG: 3057). The R scripts for the following analyses can be found at https://github.com/JoMort/ArcticTern_Foraging. Foraging trips by arctic terns were considered to begin as soon as any locations were received from more than 500 m from the colony area (to remove any locations where terns were visiting a freshwater bathing location c. 0.25 km from the colony). Flight bearing was calculated from the geodesic distance (the shortest path over an ellipsoid) between two subsequent locations using the bearing function. Arctic tern ground speed was calculated from the time and distance between two subsequent locations and the air speed was derived from the modelled wind velocities and the tracking location vectors using the triangle of velocities (see Hedenström and Åkesson, 2016). The outbound and return sections of foraging trips were compared separately using the maximum displacement from the colony (including the maximum displacement location) to mark the outbound section of a foraging trip, and locations recorded afterward were considered the return section of the foraging trip.
To test whether arctic tern size (mean, wing length, head and bill length, and culmen) was related to the mean distance travelled by each individual during foraging trips (for example whether larger birds flew further), we tested for normality and then calculated Pearson’s correlations for each of the biometric measures (head-bill length was square transformed which yielded a normal distribution, all other variables were normally distributed). To describe the general body condition of the birds in the colony, the masses of all arctic terns captured in each of the study years were tested for normality and compared with a one-way ANOVA. If individuals were captured and weighed more than once either within or between years, only the first recorded mass was included to avoid pseudoreplication. Likewise, we also performed Welch’s t-tests to determine whether there was a significant difference in the mean and maximum displacement (variables were tested for normality using Shapiro-Wilk test and then square and log transformed, respectively, for normal distributions) from the colony between males and females. The sex of one bird could not be determined so this individual was excluded from these analyses.
Due to the low sample size in 2018, the following analyses were only conducted on the 2019–2021 datasets. The mean wind direction in each year encountered by arctic terns during foraging trips was calculated with the R package circular (Agostinelli and Lund, 2017). To test for a significant relationship between the wind speed encountered at sea during foraging trips and arctic tern flight speeds, a repeated measures correlation was performed using the r package rmcorr (Bakdash and Marusich, 2021). The mean ground speed per foraging trip was used, with arctic tern identity as the repeated measure. To test whether wind speed affected the outbound and return sections of foraging trips repeated measures correlations were also performed on the mean ground speed of outbound and return sections of foraging trip separately.
The autocorrelated kernel density estimates (AKDE) of each individual with five or more foraging trips were calculated using continuous-time movement modelling (ctmm) and implemented using the ctmm package (Fleming and Calabrese, 2021) following the workflow described in Calabrese et al. (2016). The ctmm package calculates the kernel density home range estimates from autocorrelated GPS data and is not biased by small sample sizes. The best performing model [Ornstein-Uhlenbeck-F (OUF)] was selected based on the lowest Akaike Information Criterion (AIC) for each individual. The smoothing bandwidth is calculated by the akde function. The estimated isopleths at 25, 50, 75, 90, and 95% for arctic terns tracked in 2019, 2020, and 2021 were then merged within years and their areas calculated using the sf package (Pebesma, 2018).
Spatial overlap of arctic tern locations with pelagic fishing vessels were determined using the R package fuzzyjoin (Robinson, 2020). All arctic tern locations with a great circle distance within a 1 km radius and ± 1 h of a fishing vessel location, and the number of arctic tern locations recorded within a 2 km radius and ± 6 h of a fishing vessel location, were extracted and then calculated as a percentage of the total locations.
Results
Foraging Behaviour
During egg incubation, a total of 374 foraging trips were recorded for 29 individuals (2 in 2018, 9 in 2019, 11 in 2020, 7 in 2021). Devices were deployed in 2018 for 1 and 6 days respectively, in 2019 for 8–10 days, in 2020 for 3–8 days, and in 2021 for 5–9 days, but devices did not all function for the whole period (Supplementary Table 1). One trip in 2021 along the south coast by an individual that abandoned incubation lasted 3.3 days and was not considered a foraging trip during analyses. Trip duration varied from 14.9–2355 min (mean = 346.1 ± 384.7 min, median = 225 min) and was more variable within individuals in the 2020 [tracked individual foraging trip duration interquartile ranges (IQRs) ranged from 89.9–615.0] and 2021 (IQRs 285.0–663.8) breeding seasons compared to 2019 (IQRs 21.2–219.0; Supplementary Figure 3). The mean distance travelled per trip varied between individuals, and the mean displacement from the colony was 36.1 ± 37.1 km, with a recorded maximum trip distance of 218.9 km.
Arctic tern foraging locations varied between years. In 2018 they generally foraged to the west and south west of the colony, in 2019 to the west and north west, in 2020 they generally foraged to the south west with the exception of one individual that foraged within Faxaflói bay to the north of the colony, and in 2021 foraging locations were generally to the west and north west with occasional very long trips to the south and south east of the colony (Figure 1). In 2019 most tracked arctic terns departed toward the north west, whereas in 2020 the departure direction ranged from 180 to 360° and in 2021 trip departures were toward the west or south west (Figures 2A–C). Arctic terns departed for foraging trips throughout the day, including during the short “night” (the period between sunset and sunrise was less than 2 h throughout the study period). Of all the foraging trips recorded from 2019 to 2021, 25% fewer trips began in the 2 h preceding sunset compared with other times of day (Figures 2D–F). The sample size in 2018 (7 foraging trips) was too small to be included in these analyses.
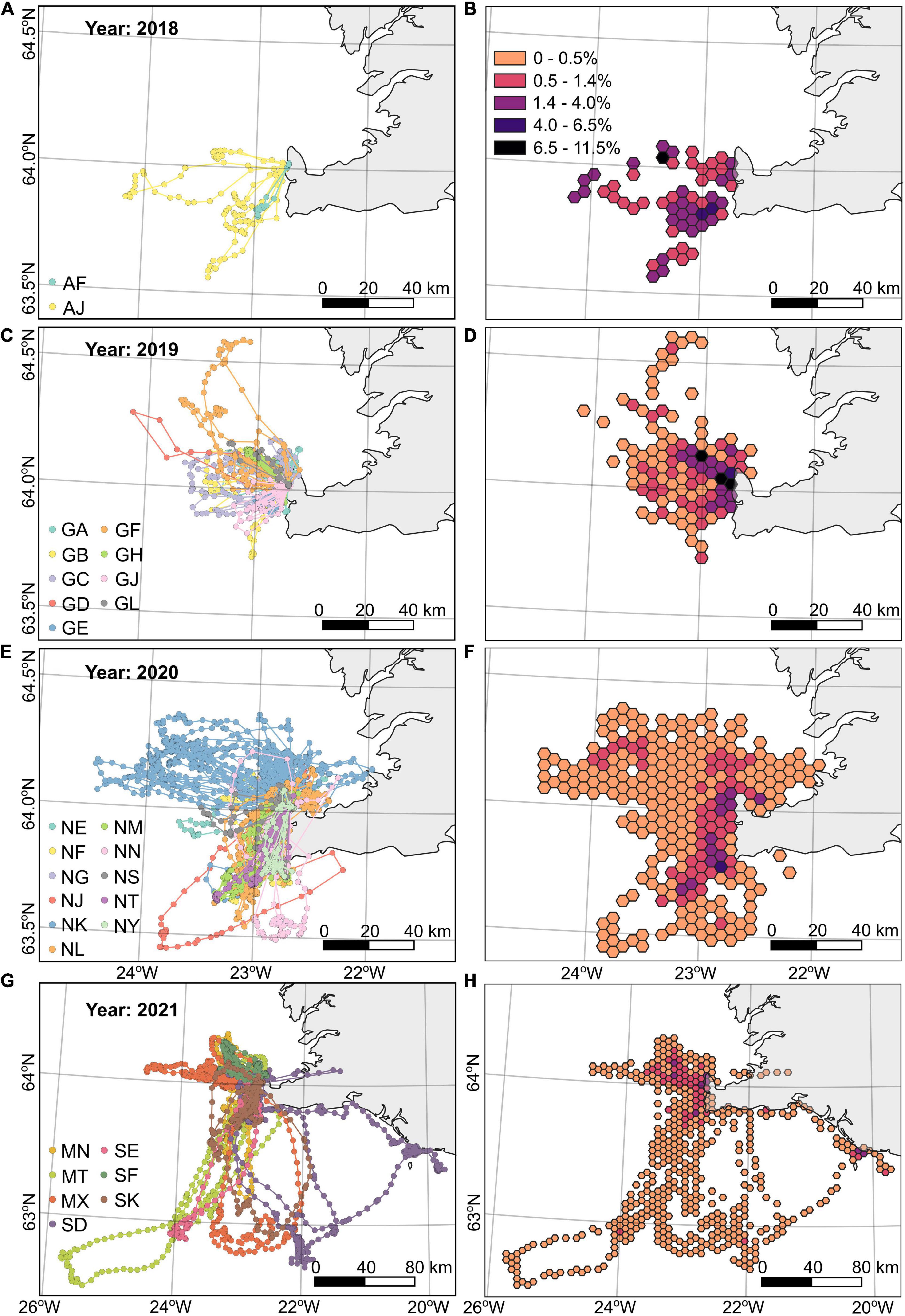
Figure 1. Arctic tern foraging trips and location densities shown as hexbins in 5 km grids (cell area = 21.65 km2, short diagonal = 5 km; B,D,F,H) during four breeding seasons: (A,B) 2018, (C,D) 2019, (E,F) 2020, and (G,H) 2021 by 29 individuals (n = 2, n = 9, n = 11, and n = 7, respectively). The map extent in 2021 is larger due to the greater distances travelled in that breeding season. The projected coordinate reference system EPSG: 3057 is used.
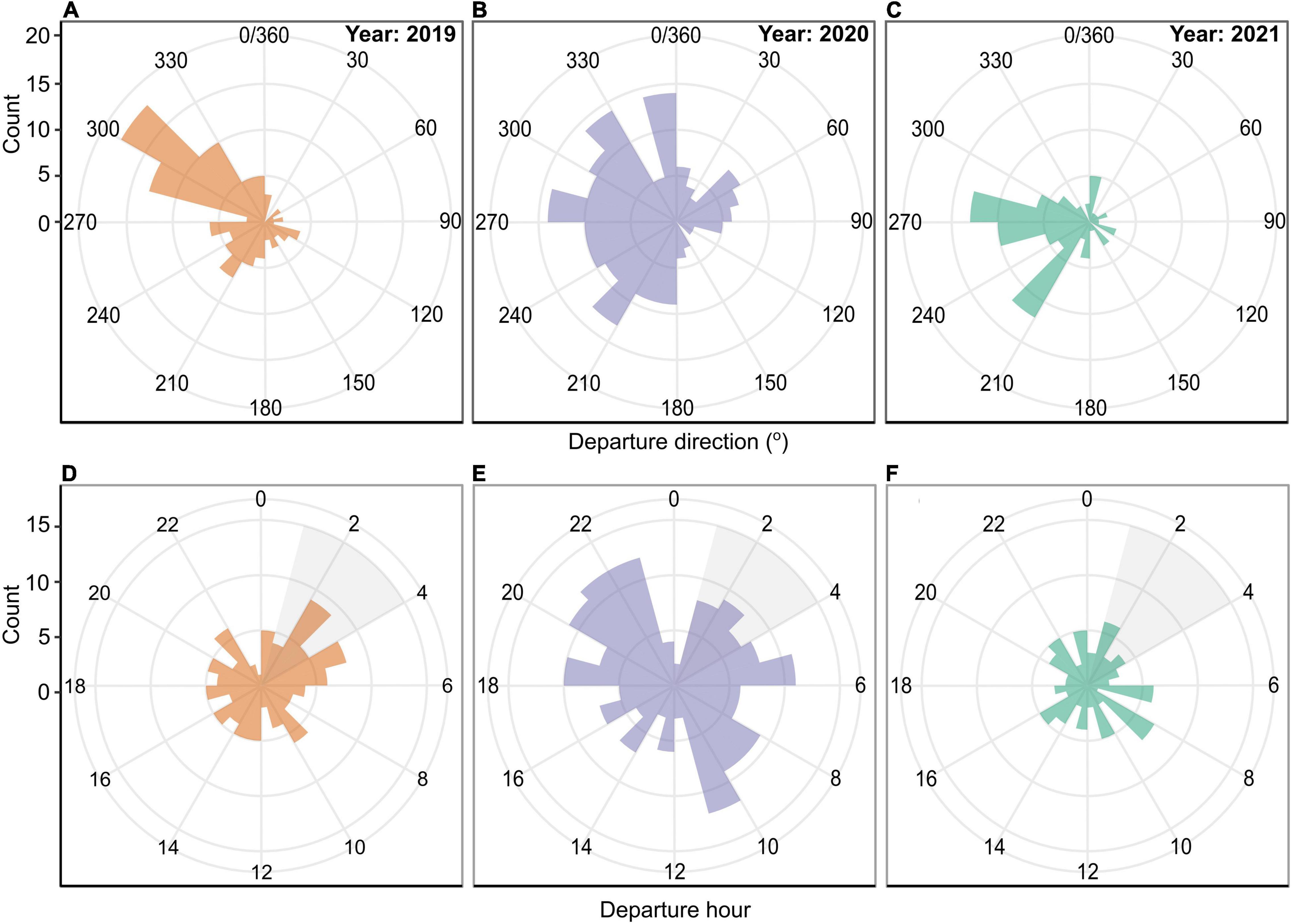
Figure 2. The direction (A–C) and hour of the day (D–F) that tracked arctic terns departed for foraging trips in 2019 (n = 9), 2020 (n = 11), and 2021 (n = 7). The grey area in (D–F) represents “night” with sunset ranging from 00:48 to 01:18 and sunrise from 03:35 to 04:24 during the tracking periods. The sample size in 2018 was too small to be included in these analyses.
There was no relationship evident from a visual inspection of arctic tern size and the mean distance travelled per foraging trip, suggesting that if any relationship were present (regardless of its shape) that the effect size would be very small. Nevertheless we tested for a linear relationship, in the absence of a rationale for using a quadratic model, and there was no statistical relationship either [mass r(27) = −0.16, p = 0.41; wing length r(27) = 0.02, p = 0.93; head and bill length (squared) r(27) = 0.06, p = 0.77; culmen r(27) = −0.09, p = 0.66; Supplementary Figure 4]. There was a significant difference in the mass of all arctic terns (both controls and tagged with biologging devices) caught during each of the study years [F(3) = 20.86, p < 0.001]. The mean mass of arctic terns captured in 2018 (n = 73, 105.4 ± 6.0 g) was significantly lower than terns captured in 2019 (n = 144, 110.5 ± 6.2 g) and 2020 (n = 61, 114.6 ± 8.7 g; Tukey’s HSD p < 0.001, 95% CI = 2.5, 7.7 and p < 0.001, 95% CI = 6.0, 12.3, respectively). While there was no significant difference in mass, arctic terns captured in 2021 (n = 36, 107.4 ± 8.5 g) were significantly smaller than those captured in 2020 (Tukey’s HSD p < 0.001, 95% CI = −11.0, −3.4). There was also no significant difference between the square root transformed mean or log transformed maximum displacement distance that males and females travelled from the colony during foraging trips (t-test: t = −1.44, p = 0.16, and t = −0.93, p = 0.365, respectively).
The sizes of home ranges varied between individuals within years, with some foraging within much smaller areas (e.g., GA, GJ, NG, NY, SF) and others covering much larger areas (e.g., GB, GF, NF, NK, MT, SD; Supplementary Table 2 and Supplementary Figures 5–7). The combined home range of tracked arctic terns with more than 5 foraging trips recorded in 2019 (n = 6; 50% AKDE 1136 km2, 75% 2157 km2, 95% 4309 km2) were 1.65 times smaller than those tracked in 2020 (n = 9; 50% 1879 km2, 75% 3562 km2, 95% 7098 km2) and 15.9–18.2 times smaller than those tracked in 2021 (n = 7; 50% 20,677 km2, 75% 37,361 km2 and 95% 68,477 km2; Supplementary Table 2 and Figures 3A,D,G,J).
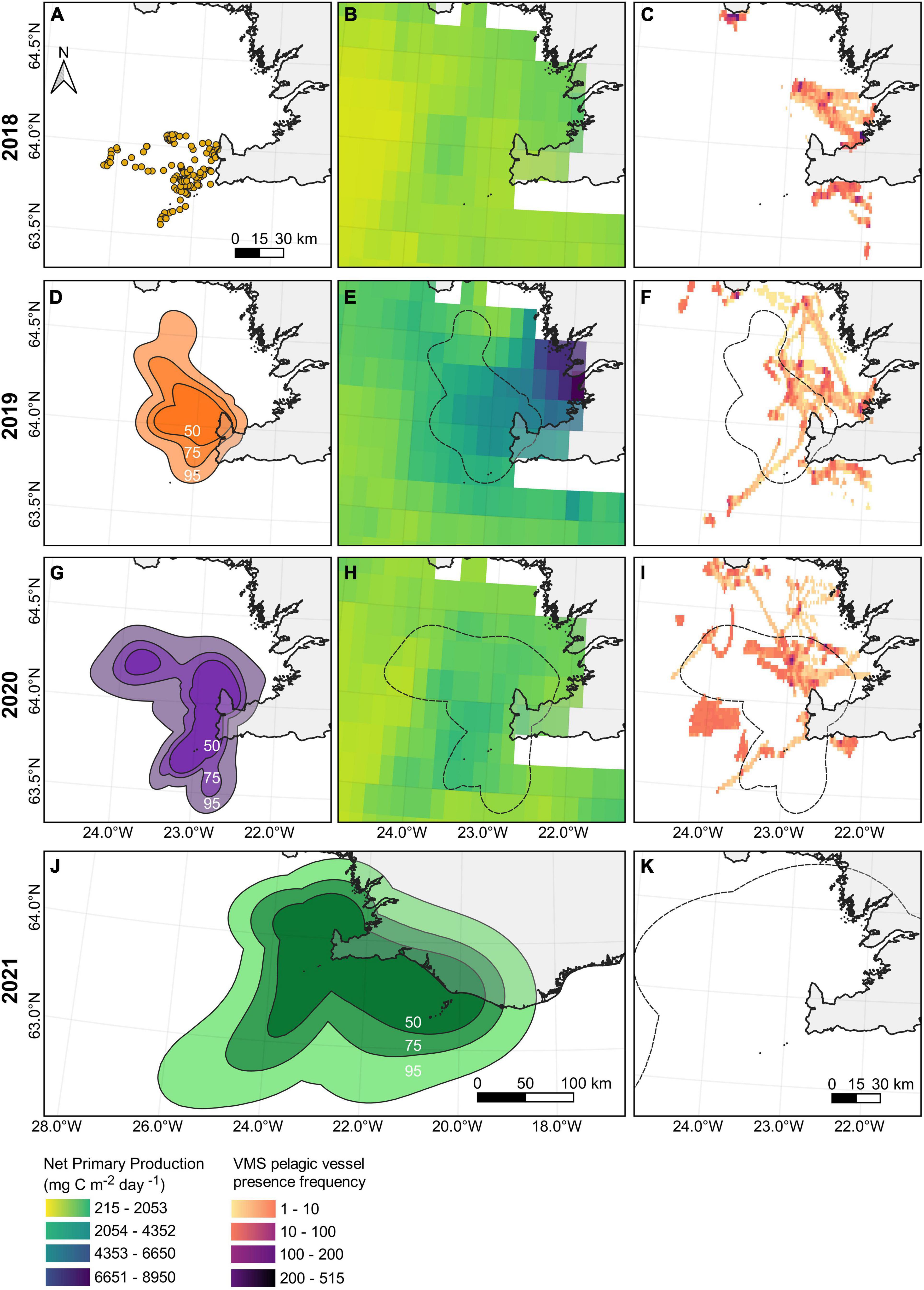
Figure 3. Arctic tern locations, average monthly net primary productivity and fishing vessel locations in 2018 (A–C), 2019 (D–F), 2020 (G–I), and 2021 (J,H). The locations of arctic terns are shown in 2018 (A). In 2019 (D), 2020 (G), and 2021 (J), when there were larger sample sizes, automated kernel density estimations were calculated with the estimated isopleth sizes shown. The monthly average net primary productivity levels in June 2018 (B), 2019 (E), and 2020 (H) (estimated by Ocean Productivity Oregon State, 2021) are plotted on a grid with 100 degree resolution. Net primary productivity data were unavailable for 2021. Pelagic fishing intensity during June 2018 (C), 2019 (F), 2020 (I), 2021 (K). Arctic tern 95% isopleths are overlaid on the fishing data as dashed lines. The projected coordinate reference system EPSG: 3057 is used.
Impact of Wind Conditions on Foraging Behaviour
The wind speeds surrounding the Reykjanes peninsula (19.5–26°W and 62.5–64.6°N) during tracking device deployments were highest in 2018 and 2021 (median 8.1 and 8.3 m.s–1, respectively), and lowest in 2020 [median 6.2 m.s–1, compared with 6.8 m.s–1 minimum power speed for arctic terns (Hedenström and Åkesson, 2016; Figure 4)]. In 2018, 2019, and 2021, the average wind conditions experienced at the colony were westerly and faster compared to the average southerly and lower speed winds in 2020 (Figures 4E–H). Whilst foraging, the arctic terns encountered south westerly winds most often in 2019 (mean wind direction = 87.1°), with more occasional north easterlies (Figure 5A), compared to predominantly south easterly winds (mean wind direction = 330.5°) during foraging trips in 2020 (Figure 5B) and predominantly north westerlies with occasional south easterlies in 2021 (mean wind direction = 88.7°; Figure 5C). Despite the wind being close to the minimum power speeds (most efficient forward flight speed) for arctic terns, there was no evidence that they adjusted their direction of travel in relation to wind (Figure 5) and the ground speed travelled during foraging trips was not strongly correlated with the wind speed [repeated measures correlation 2019: r(104) = −0.13, p = 0.19, 2020: r(171) = −0.08, p = 0.27, 2021: r(79) = −0.29, p = 0.01; Supplementary Figure 8]. When the outbound and return sections of foraging trips were considered separately the relationship between arctic tern ground speed and wind speeds were either not significant, or had very weak negative correlations [repeated measures correlation 2019: r(97) = −0.04, p = 0.67 (outbound), r(70) = −0.21, p = 0.08 (return), 2020: r(163) = −0.22, p = 0.01 (outbound), r(132) = −0.05, p = 0.6 (return), 2021: r(78) = −0.29, p = 0.01 (outbound), r(63) = −0.20, p = 0.1 (return); Supplementary Figure 9]. Likely air speeds for the arctic terns ranged from 0.14 to 24.63 m.s–1, with a mean (± standard deviation) of 9.04 (± 3.52) m.s–1. However, these should be interpreted with caution because the temporal (hourly) and spatial (∼10 km) resolution of the modelled wind data was much coarser than the bird data (every 15 min).
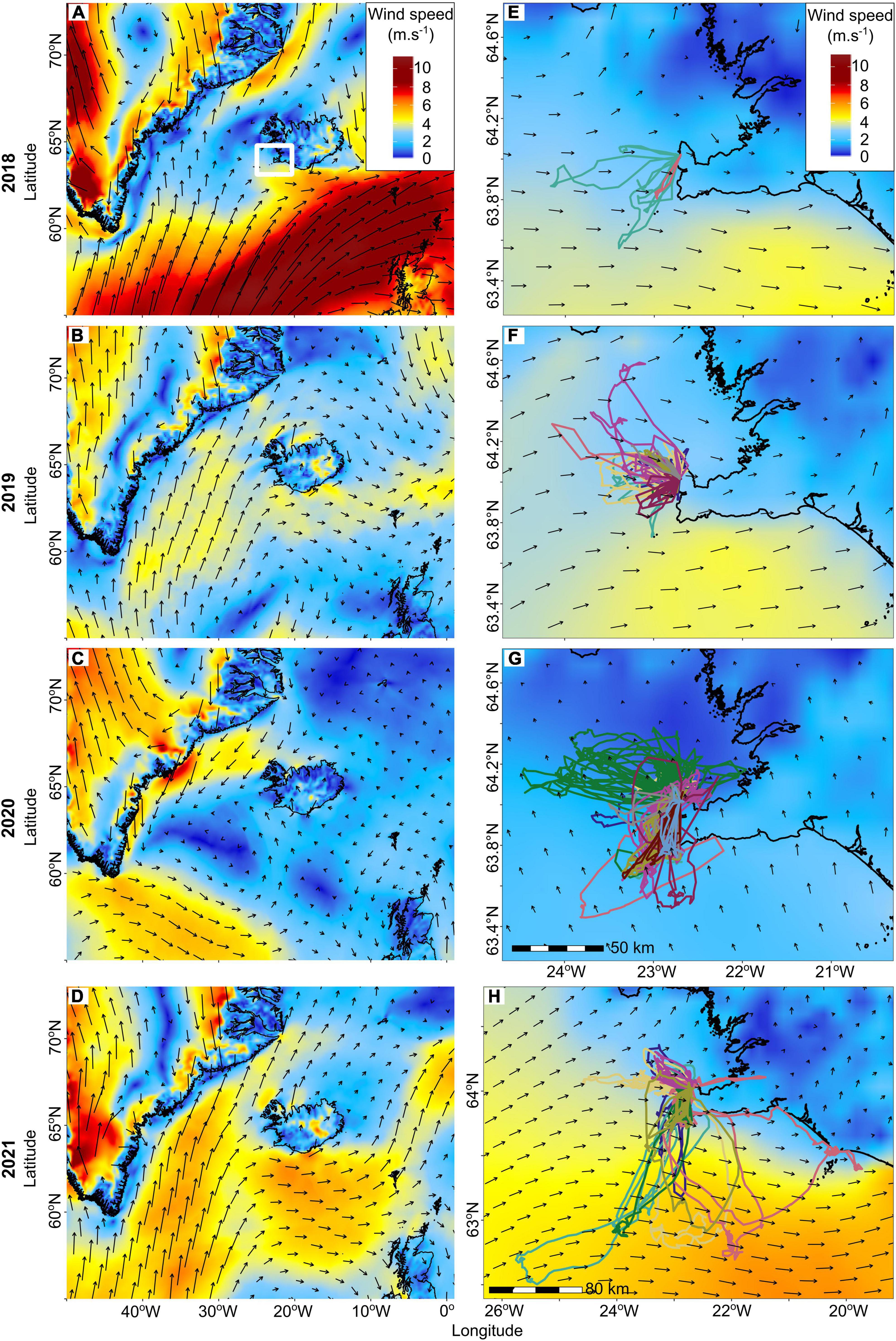
Figure 4. Modelled mean wind speed and direction at 50 m altitude in the wider area around Iceland (A–D) and surrounding an arctic tern breeding colony (E–H). Arctic terns were tracked in 2018 (A,E), 2019 (B,F), 2020 (C,G), and 2021 (D,H) and the wind data were averaged for the duration of the tracking periods [17/06–23/06/2018 (6 days), 20/06–30/06/2019 (10 days), 10/06–09/07/2020 (29 days), 17/06–28/06/2021 (11 days)]. All recorded arctic tern foraging trips are shown (D–F), with colours corresponding to different individuals. The map extent in (H) is larger due to the greater distances travelled in that breeding season.
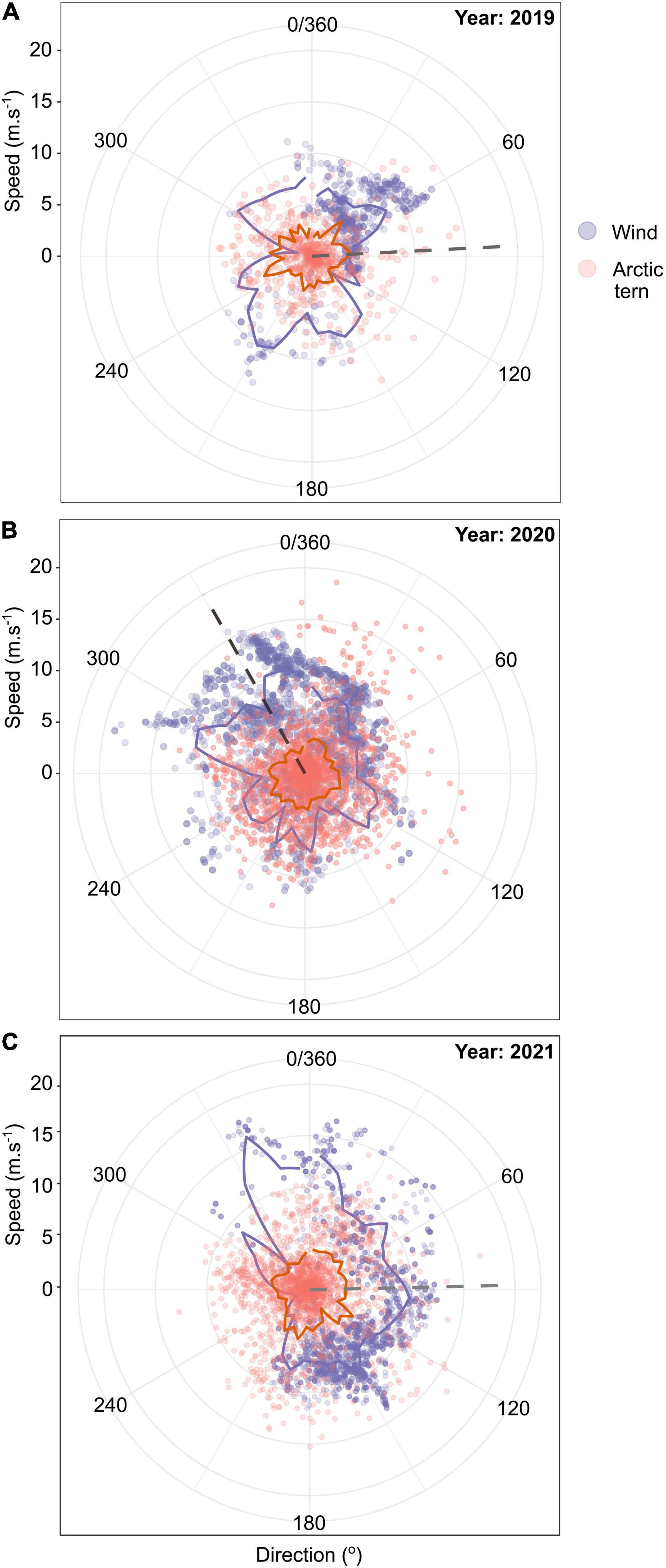
Figure 5. The wind speed (m.s–1) and direction (purple) encountered by arctic terns (n = 29) during foraging trips in (A) 2018, (B) 2019, and (C) 2020, with the bearing and ground speed (m.s–1) of the arctic terns (orange). Solid lines show the mean speeds binned over 9° and the dashed lines show the mean wind direction.
Use of Productive Areas: Net Primary Productivity and Overlap Between Arctic Terns and Fishing Vessels
Of the 3 years with available NPP data (2018–2020), the monthly average of NPP in June was greatest in 2019 (Figures 3B,E,H). In 2019 the most productive areas were toward the north and north east of the colony, whereas in 2020 the more productive areas were west and south west of the colony. Vessels fishing in pelagic waters surrounding the colony area (to the south west of the Reykjanes peninsula) generally remained within the Faxaflói bay area, with some fishing activity occurring to the south west of the Reykjanes peninsula (Figures 3C,F,I,K). Although the tracked arctic terns were recorded visiting different areas during the four study years, the vessels consistently targeted similar locations, and for the most part these did not overlap with the home ranges of the tracked arctic terns (Figure 3). There were fewer pelagic vessel locations recorded over the same time period and area in 2021 compared to the other threes study years (2018: 8895 locations, 2019: 6675 locations, 2020: 9130 locations and 2021: 2100 locations). During the four study years, the percentage of arctic tern locations within close proximity to actively fishing pelagic vessels (within 1 km and 1 h) was 0, 0, 1, and 0%, respectively. This increased marginally if the spatio-temporal proximity was extended to 2 km and 6 h (0, 0.94, 10.4, and 0%). Based on the pelagic fishing activities identified through the AIS and VMS data, arctic terns appear to be for the most part, searching and foraging in different areas to fishing vessels.
Discussion
This study provides the most comprehensive analysis of intra-breeding season foraging behaviours of arctic terns to date. Using biologging tags, we have been able to record arctic tern trips over multiple seasons and investigate the impact of wind conditions and fishing activity on their behaviour.
Foraging Behaviour
It is likely that the distances and home ranges that we report here reflect the space use of arctic terns breeding elsewhere in Iceland as Seward et al. (2020) reported that the home ranges of GPS tagged and non-GPS tagged arctic terns were comparable. Indeed, if there is an effect of carrying the device on the birds (Geen et al., 2019; Tomotani et al., 2019), we might expect other arctic terns to travel even further without the burden of a tracking tag. Additionally, with a resolution of a location every 900 s, the foraging distances that we report could be an underestimate of the actual distances travelled.
Mean foraging distances from the colony were 27–34 km, longer than the average distances reported in previous studies. The maximum displacement distances recorded in this study were also much greater; and the furthest distance any tern that did not abandon its breeding attempt travelled was 141 km, with nineteen terns travelling more than 40 km from the colony to forage. The shorter foraging distances reported elsewhere could be related to the ability of prior observers to physically follow terns so far offshore. Black and Diamond (2005) and Rock et al. (2007) both recorded trips with radio telemetry and limited searches to within 20–30 km from the colony, whilst Perrow et al. (2011) visually tracked foraging arctic terns and reported a maximum of 29 km from the colony. Although we only report the distances travelled during incubation in this study, this is unlikely to account for the differences in trip distances reported in previous studies as terns were tracked at a variety of incubation stages, from unknown breeding stages to during both incubation and chick rearing. During egg incubation most seabirds generally have larger foraging ranges compared to during chick rearing (Oppel et al., 2018). For example, red-billed tropicbird Phaethon aethereus foraging trips were longer and further during incubation compared to chick rearing, when adults likely fed in less profitable areas closer to the nest (Diop et al., 2018). Although the distance and duration of trips by brown skuas Stercorarius lonnbergi did not vary during brooding stage, they made fewer foraging trips whilst incubating, and whilst chick rearing skuas fed in areas with more varied prey resources (Carneiro et al., 2014). Thus, it is possible that Icelandic arctic terns may forage over a smaller range during chick rearing, but it was not possible to investigate this during the present study, as recapture of tags once the chicks hatched and moved away from the nests was not possible at this colony.
The total area across which arctic terns foraged (average 95% isopleth area) was 16–218 times larger than reported by Robertson et al. (2014b) who tracked terns visually. Visually tracking arctic terns in boats appears to lead to smaller utilisation distributions than studies using tracking devices (Seward et al., 2020). However, arctic terns carrying GPS tracking devices in the Skerries, Wales also had smaller mean estimated home ranges (half to one fortieth of those recorded in this study) (Seward et al., 2020). The smaller home ranges reported in Wales compared to those we recorded in Iceland could be a result of tracking terns during chick rearing, restrictions by land masses, or interspecific competition with other tern species reducing foraging areas due to resource or niche partitioning (Robertson et al., 2014b).
Many species of foraging seabirds associate with shelf breaks due to a concentration of prey at these upwelling or front regions (e.g., Freeman et al., 2010; Amelineau et al., 2016). However, there are no obvious differences in the shelf depth or extent between arctic tern colonies from which birds have been tracked so far, suggesting in this case bathymetry is not likely to be the major explanatory variable for the far greater foraging trip distances reported here. Alternatively, prey abundance and distribution may differ between these colonies. For example, the duration of foraging trips recorded for breeding kittiwakes Rissa tridactyla using GPS tags were double the length compared to those recorded 11–49 years earlier using activity loggers or field observations, possibly linked to changes in food availability (Redfern and Bevan, 2014). Electronic tracking of arctic terns from the aforementioned colonies may reveal if foraging ranges are truly smaller than arctic terns in Iceland, or if differences in methodology could explain the difference.
Regardless of the underlying causes of these greater reported foraging ranges, there are notable conservation and management implications across arctic tern breeding ranges. Incorporating available movement ecology data into policy and management strategies is an important conservation measure (e.g., Allen and Singh, 2016; McGowan et al., 2017; Oppel et al., 2018). Any protection measures around arctic tern colonies must consider the larger spatial scale that they use during the breeding season. For example, although some European offshore wind farms are within previously reported arctic tern foraging ranges, the average distance to shore of wind farms under construction in 2020 was 44 km (Wind Europe, 2021), a distance previously thought to be outside arctic tern foraging ranges. The data in the present study suggests this may not always be the case.
Contrary to previous suggestions that arctic terns are exclusively diurnal foragers (McKnight et al., 2013), we recorded arctic terns making foraging trips at the breeding colony throughout the 24 h cycle, but with fewer trips in the 2 h preceding sunset at 01:00. However, we note that during the breeding period, there is just 154–161 min between sunrise and sunset, meaning that the terns nesting in southwest Iceland never experience complete darkness. Indeed, arctic terns experience some of the greatest periods of light exposure, as they annually migrate between the boreal and austral summers (Sockman and Hurlbert, 2020). Flexibility in foraging behaviour has been recorded in other visual predators. For example, common guillemots Uria aalge will forage at night using moon or star light to locate prey, with shallower dives in the latter when the light levels are lower (Regular et al., 2011). Across their range Cory’s shearwaters Calonectris diomedea display either diurnal or nocturnal foraging behaviours depending on the water temperature and depths, with diurnal foraging in warmer and shallower waters (Dias et al., 2012). Phenotypic plasticity in various aspects of foraging behaviour, such as prey switching or temporal changes, improve population resilience to global changes (e.g., Grémillet et al., 2012).
We found no relationship between individual size or sex and the distances travelled during foraging trips, suggesting that extrinsic rather than intrinsic factors are more likely to impact arctic tern foraging behaviour. This is further supported by the generally lower mass of individuals in 2021 compared to 2019 and 2020. If, for example, prey were less abundant, had lower profitability, or the distribution had shifted in 2021, then foraging arctic terns may have had to travel further to reach their daily energy intake requirements. However, to investigate this we would require detailed information on the prey captured or record the mass change at the start and end of a foraging trip, but it was not possible to collect these data during this study. It is also possible that a combination of extrinsic and intrinsic factors affect arctic tern foraging trip metrics, but these were not detected due to the small sample size of tracked individuals (which was biased toward heavier individuals), and that foraging behaviours were only monitored during the egg incubation stage. Foraging conditions prior to egg laying or during incubation affect breeding success (such as reduced clutch size, or even increased adult mortality; Suddaby and Ratcliffe, 1997) and it is therefore likely that in years with low prey abundance only individuals with greater body condition were tracked, as tags were deployed on birds with eggs at least one third through incubation. Additionally, differences in the foraging behaviours of sexually monomorphic seabird species have been reported in multiple seabird families (e.g., Lewis et al., 2002; Thaxter et al., 2009; Welcker et al., 2009; Hedd et al., 2014; Clark et al., 2021) but can vary with the breeding stage. Foraging niche segregation by sex may therefore exist in arctic terns during other breeding stages, but currently we were only able to collect data during incubation as terns can only be reliably recaptured (to remove biologging tags at the conclusion of the study) on their nest. It is also possible that other intrinsic and extrinsic variables that did not affect foraging behaviours during incubation may alter behaviour during later breeding stages when the adults may travel shorter distances from the colony (e.g., Weimerskirch et al., 1993; Barlow and Croxall, 2002; Robertson et al., 2014a), and are limited to carrying one prey item at once (Hatch, 2002).
Impact of Wind Conditions on Foraging Behaviour
During the breeding season arctic terns are central place foragers and need to return to their nest after every trip, meaning that any deviations caused by winds need to be countered with adjustments to flight speeds and heading, which is likely to occur through visual cues as the terns are close to the colony (Hedenström and Åkesson, 2016). However, in this study we found limited effects of wind conditions impacting arctic tern flights during foraging trips. This might have been because terns were compensating in other ways that we were unable to detect with the temporal resolution of the tracking tags. For example, by altering pre-dive flight behaviours in strong winds (e.g., Forster’s terns Sterna forsteri change from hovering to undulating flights; Salt and Willard, 1971), by increasing wing beat strength and air speed when flying into headwinds (e.g., European shags Phalacrocorax aristotelis; Kogure et al., 2016), or by spending longer foraging and diving more frequently in stronger winds due to altered prey visibility (e.g., Northern gannets Morus bassanus; Lane et al., 2019). Higher resolution tracking in the future, or use of alternative devices such as accelerometers to determine wing beat frequency could determine how arctic terns may adjust flight behaviours in response to wind conditions. Alternatively, wind conditions that the tracked arctic terns encountered during the breeding season are much weaker compared to the winds they experience during the non-breeding season in the Southern Ocean (Supplementary Figure 10) and may therefore pose little relative challenge.
Our results indicate that arctic terns foraged in different areas in 2019, 2020, and 2021. They foraged more to the north-west and west in 2019 when the winds were stronger and in a westerly direction, they foraged toward the south-east in 2020 when the winds were weaker and more south to south westerly, and foraged far greater distances in 2021 to the west, south west, south and south east when the winds were strong and north westerly to westerly. Although the wind conditions did not appear to affect the arctic tern flights, the difference in foraging areas between the three years suggests that wind may still act indirectly on terns, perhaps as a driver of coastal circulation patterns affecting upwelling. Thus, the speed, direction and duration of wind could impact prey species distribution and abundance, particularly in the shallowest surface waters where arctic terns feed (e.g., Sætre et al., 2002; Mills et al., 2008; Bakun et al., 2015; Wilson and Laman, 2021). Additionally, there could be other extrinsic factors that we did not record that could influence foraging locations. Collins et al. (2020) reported that prey availability was more likely to affect black-legged kittiwake Rissa tridactyla foraging trip timing and direction than the wind conditions, and during short foraging trips any benefit gained from tailwind support will be countered by travelling into headwinds during the reverse journey.
Use of Productive Areas: Net Primary Productivity and Overlap Between Arctic Terns, and Fishing Vessels
Arctic terns are not thought to be susceptible to fisheries bycatch (Žydelis et al., 2013) and are generally not observed following fishing vessels (e.g., Garthe and Hüppop, 1994; Weimerskirch et al., 2000), although they have been recorded scavenging shrimper discards (Walter and Becker, 1998). In Iceland however, the practice of discarding by-catch is banned (Condie et al., 2014) so any overlap between pelagic fishing vessels and foraging arctic terns should be due to targeting the same productive areas, rather than the terns following vessels for discard. We found very little overlap between the areas that pelagic vessels were fishing and the areas that the breeding arctic terns were foraging, and further while the vessels consistently foraged in the same locations between the four study years, the terns changed their foraging locations, perhaps indicating that they are able to adapt to moving prey resources and locate areas with higher NPP levels. In 2019, the study year with the greatest monthly average NPP levels, tracked arctic terns had the smallest home ranges. This suggests that their foraging ranges may be likely to increase in years with lower productivity, although this is based on a small number of years with tracking data so may warrant further investigation.
Whilst in southwest Iceland there doesn’t appear to be an overlap between fishing vessels and the breeding arctic terns, future monitoring of seabirds during the breeding season can be used to inform fisheries management (Einoder, 2009) and identify areas that are important for marine predators like seabirds. Additionally, further investigations of prey patch dynamics and any between year variations are warranted to better understand the energy landscape for arctic terns and other species foraging in this region.
Conclusion
In this study we have demonstrated that during the egg incubation stage, arctic terns travel much further out to sea to forage than previously recorded. They are still bound within 220 km of their breeding site, with average foraging trip distances of 36 km. During later breeding stages they are likely to be even further restricted in the distances that they travel, so local prey availability will have a profound impact on their success or the choice to abandon the breeding attempt. Vigfúsdóttir et al. (2013) concluded that a key issue causing low fledging success in arctic terns breeding in Iceland was food shortages and if this is the case, we have demonstrated that poor fledging rates are likely not as a result of directly competing for prey with commercial fisheries, but could, however, be due to changes in climatic forcing factors altering ocean structuring and overturning, with knock-on effects for ocean productivity and subsequent prey distribution (Hoegh-Guldberg and Bruno, 2010; Doney et al., 2012; Sydeman et al., 2015; Edwards et al., 2021). We also show that changes in wind strength and direction are unlikely to directly affect arctic terns, but further investigations are warranted both in Iceland and other areas where the breeding successes of arctic terns are poor in order to establish the underlying causes of population declines. With the continual developments of tracking technologies, it is vital to continue unravelling the foraging behaviours of arctic terns at colonies, prioritising the chick-rearing and post-fledging behaviours that are still little understood.
Data Availability Statement
The raw data supporting the conclusions of this article will be made available by the authors, without undue reservation.
Ethics Statement
The animal study was reviewed and approved by the University of Exeter Ethics Committee (ethics ID eCLESBio000180).
Author Contributions
LH, SM, and FV conceived the study. JM, LC, SM, E-JM, NP, FV, MW, and LH contributed to data collection. JB provided fisheries data. WT, MW, and LH supervised the research. JM analysed the data and prepared the first draft. All authors revised the first draft and worked on the final version of the article.
Funding
This work was funded by a National Geographic grant (GR-000044390) awarded to LH funded field work and equipment. JM was supported by a NERC GW4+ Doctoral Training Partnership studentship from the Natural Environment Research Council (NE/L002434/1).
Conflict of Interest
The authors declare that the research was conducted in the absence of any commercial or financial relationships that could be construed as a potential conflict of interest.
Publisher’s Note
All claims expressed in this article are solely those of the authors and do not necessarily represent those of their affiliated organizations, or those of the publisher, the editors and the reviewers. Any product that may be evaluated in this article, or claim that may be made by its manufacturer, is not guaranteed or endorsed by the publisher.
Acknowledgments
We would like to thank the two reviewers for providing insightful and constructive comments on this manuscript, which have greatly improved its quality. We are grateful to Sigríður Hanna Sigurðardóttir and Páll ϸórðarson for allowing us access to their land and being wonderful hosts throughout our fieldwork. We thank Solveig Davidsdóttir, Giulia Bellon, and Jessica Rudd for their assistance during the field seasons. We thank Andrew King for teaching JM the molecular sexing methods and providing reagents. Gary Brodin and Andrew Howarth provided valuable advice on the scheduling of the GPS tags and technical assistance.
Supplementary Material
The Supplementary Material for this article can be found online at: https://www.frontiersin.org/articles/10.3389/fmars.2021.760670/full#supplementary-material
Footnotes
References
Allen, A. M., and Singh, N. J. (2016). Linking movement ecology with wildlife management and conservation. Front. Ecol. Evol. 3:155. doi: 10.3389/fevo.2015.00155
Amelineau, F., Gremillet, D., Bonnet, D., Le Bot, T., and Fort, J. (2016). Where to forage in the absence of sea ice? Bathymetry as a key factor for an Arctic seabird. PLoS One 11:e0157764. doi: 10.1371/journal.pone.0157764
Asbirk, S., Berg, L., Hardeng, G., Koskimies, P., and Petersen, A. (1997). Population sizes and trends of birds in the Nordic countries: 1978–1994. TemaNord 614, 1–88.
Bakdash, J. Z., and Marusich, L. R. (2021). rmcorr: Repeated Measures Correlation. R Package Version 0.4.3.
Bakun, A., Black, B. A., Bograd, S. J., García-Reyes, M., Miller, A. J., Rykaczewski, R. R., et al. (2015). Anticipated effects of climate change on coastal upwelling ecosystems. Curr. Clim. Chang. Rep. 1, 85–93. doi: 10.1007/s40641-015-0008-4
Barlow, K. E., and Croxall, J. P. (2002). Seasonal and interannual variation in foraging range and habitat of macaroni penguins Eudyptes chrysolophus at South Georgia. Mar. Ecol. Prog. Ser. 232, 291–304. doi: 10.3354/meps232291
Barrett, R. T., Lorentsen, S.-H., and Anker-Nilssen, T. (2006). The status of breeding seabirds in mainland Norway. Atl. Seabirds 8, 97–126.
Behrenfield, M. J., and Falkowski, P. G. (1997). Photosynthetic rates derived from satellite-based chlorophyll concentration. Limnol. Oceanogr. 42, 1–20. doi: 10.4319/lo.1997.42.1.0001
Black, A. L., and Diamond, A. W. (2005). “Feeding areas of arctic terns (Sterna paradisaea) and common terns (Sterna hirundo) breeding on Machias Seal Island, New Brunswick,” in Proceedings of the 6th Bay of Fundy Workshop, Cornwallis, Nova Scotia, September 29th – October 2nd, 2004, The Changing Bay of Fundy: Beyond 400 years, (Cornwallis, NS: Environment Canada), 101–104.
Burnham, K. K., Burnham, J. L., Konkel, B. W., and Johnson, J. A. (2017). Significant decline observed in Arctic Tern Sterna paradisaea population in northwest Greenland. Seabird 30, 39–50.
Calabrese, J. M., Fleming, C. H., Gurarie, E., and Freckleton, R. (2016). ctmm: an r package for analyzing animal relocation data as a continuous-time stochastic process. Methods Ecol. Evol. 7, 1124–1132. doi: 10.1111/2041-210X.12559
Carneiro, A. P. B., Manica, A., and Phillips, R. A. (2014). Foraging behaviour and habitat use by brown skuas Stercorarius lonnbergi breeding at South Georgia. Mar. Biol. 161, 1755–1764. doi: 10.1007/s00227-014-2457-z
Clark, B. L., Cox, S. L., Atkins, K., Bearhop, S., Bicknell, A. W. J., Bodey, T., et al. (2021). Sexual segregation of gannet foraging over 11 years: movements vary but isotopic differences remain stable. Mar. Ecol. Prog. Ser. 661, 1–16. doi: 10.3354/meps13636
Collins, P. M., Green, J. A., Elliott, K. H., Shaw, P. J. A., Chivers, L., Hatch, S. A., et al. (2020). Coping with the commute: behavioural responses to wind conditions in a foraging seabird. J. Avian Biol. 51. doi: 10.1111/jav.02057
Condie, H. M., Grant, A., and Catchpole, T. L. (2014). Incentivising selective fishing under a policy to ban discards; lessons from European and global fisheries. Mar. Policy 45, 287–292. doi: 10.1016/j.marpol.2013.09.001
Cox, S. L., Embling, C. B., Hosegood, P. J., Votier, S. C., and Ingram, S. N. (2018). Oceanographic drivers of marine mammal and seabird habitat-use across shelf-seas: a guide to key features and recommendations for future research and conservation management. Estuar. Coast. Shelf Sci. 212, 294–310. doi: 10.1016/j.ecss.2018.06.022
Croxall, J. P., Butchart, S. H. M., Lascelles, B. E. N., Stattersfield, A. J., Sullivan, B. E. N., Symes, A., et al. (2012). Seabird conservation status, threats and priority actions: a global assessment. Bird Conserv. Int. 22, 1–34. doi: 10.1017/S0959270912000020
De Pascalis, F., Imperio, S., Benvenuti, A., Catoni, C., Rubolini, D., and Cecere, J. G. (2020). Sex-specific foraging behaviour is affected by wind conditions in a sexually size dimorphic seabird. Anim. Behav. 166, 207–218. doi: 10.1016/j.anbehav.2020.05.014
Dehnhard, N., Ludynia, K., Poisbleau, M., Demongin, L., and Quillfeldt, P. (2013). Good days, bad days: wind as a driver of foraging success in a flightless seabird, the southern rockhopper penguin. PLoS One 8:e79487. doi: 10.1371/journal.pone.0079487
Devlin, C. M., Diamond, A. W., Kress, S. W., Hall, C. S., and Welch, L. (2008). Breeding dispersal and survival of arctic terns (Sterna Paradisaea) nesting in the Gulf of Maine. Auk 125, 850–858. doi: 10.1525/auk.2008.07060
Dias, M. P., Granadeiro, J. P., and Catry, P. (2012). Working the day or the night shift? Foraging schedules of Cory’s shearwaters vary according to marine habitat. Mar. Ecol. Prog. Ser. 467, 245–252. doi: 10.3354/meps09966
Dias, M. P., Martin, R., Pearmain, E. J., Burfield, I. J., Small, C., Phillips, R. A., et al. (2019). Threats to seabirds: a global assessment. Biol. Conserv. 237, 525–537. doi: 10.1016/j.biocon.2019.06.033
Diop, N., Zango, L., Beard, A., Bâ, C. T., Ndiaye, P. I., Henry, L., et al. (2018). Foraging ecology of tropicbirds breeding in two contrasting marine environments in the tropical Atlantic. Mar. Ecol. Prog. Ser. 607, 221–236. doi: 10.3354/meps12774
Doney, S. C., Ruckelshaus, M., Duffy, J. E., Barry, J. P., Chan, F., English, C. A., et al. (2012). Climate change impacts on marine ecosystems. Annu. Rev. Mar. Sci. 4, 11–37. doi: 10.1146/annurev-marine-041911-111611
Duffy, D. C., Mcknight, A., and Irons, D. B. (2013). Trans-Andean passage of migrating arctic terns over Patagonia. Mar. Ornithol. 41, 155–159.
Dunn, E. K. (1973). Changes in fishing ability of terns associated with windspeed and sea surface conditions. Nature 244, 520–521. doi: 10.1038/244520a0
Edvardsson, K. N., Păstrăv, C., and Benediktsson, K. (2018). Mapping the geographical consolidation of fishing activities in Iceland during the maturation of the ITQ fisheries management system. Appl. Geogr. 97, 85–97. doi: 10.1016/j.apgeog.2018.05.013
Edwards, M., Hélaouët, P., Goberville, E., Lindley, A., Tarling, G. A., Burrows, M. T., et al. (2021). North Atlantic warming over six decades drives decreases in krill abundance with no associated range shift. Commun. Biol. 4:644. doi: 10.1038/s42003-021-02159-1
Egevang, C., and Frederiksen, M. (2011). Fluctuating breeding of arctic terns (Sterna paradisaea) in arctic and high-arctic colonies in Greenland. Waterbirds 34, 107–111. doi: 10.1675/063.034.0114
Egevang, C., Stenhouse, I. J., Phillips, R. A., Petersen, A., Fox, J. W., and Silk, J. R. (2010). Tracking of Arctic terns Sterna paradisaea reveals longest animal migration. Proc. Natl. Acad. Sci. U.S.A. 107, 2078–2081. doi: 10.1073/pnas.0909493107
Einoder, L. D. (2009). A review of the use of seabirds as indicators in fisheries and ecosystem management. Fish. Res. 95, 6–13. doi: 10.1016/j.fishres.2008.09.024
Elliott, K. H., Chivers, L. S., Bessey, L., Gaston, A. J., Hatch, S. A., Kato, A., et al. (2014). Windscapes shape seabird instantaneous energy costs but adult behavior buffers impact on offspring. Mov. Ecol. 2:17. doi: 10.1186/s40462-014-0017-2
Fernández-López, J., and Schliep, K. (2018). rWind: download, edit and include wind data in ecological and evolutionary analysis. Ecography 42, 804–810. doi: 10.1111/ecog.03730
Finney, S. K., Wanless, S., and Harris, M. P. (1999). The effect of weather conditions on the feeding behaviour of a diving bird, the common guillemot Uria aalge. J. Avian Biol. 30, 23–30. doi: 10.2307/3677239
Fleming, C. H., and Calabrese, J. M. (2021). ctmm: Continuous-Time Movement Modeling. R Package Version 0.6.0.
Frederiksen, M., Daunt, F., Harris, M. P., and Wanless, S. (2008). The demographic impact of extreme events: stochastic weather drives survival and population dynamics in a long-lived seabird. J. Anim. Ecol. 77, 1020–1029. doi: 10.1111/j.1365-2656.2008.01422.x
Freeman, R., Dennis, T., Landers, T., Thompson, D., Bell, E., Walker, M., et al. (2010). Black Petrels (Procellaria parkinsoni) patrol the ocean shelf-break: GPS tracking of a vulnerable procellariiform seabird. PLoS One 5:e9236. doi: 10.1371/journal.pone.0009236
Fridolfsson, A.-K., and Ellegren, H. (1999). A simple and universal method for molecular sexing of non-ratite birds. J. Avian Biol. 30, 116–121. doi: 10.2307/3677252
Garthe, S., and Hüppop, O. (1994). Distribution of ship-following seabirds and their utilization of discards in the North Sea in summer. Mar. Ecol. Prog. Ser. 106, 1–9. doi: 10.3354/meps106001
Geen, G. R., Robinson, R. A., and Baillie, S. R. (2019). Effects of tracking devices on individual birds – a review of the evidence. J. Avian Biol. 50. doi: 10.1111/jav.01823
Geirsson, G. (2011). Case Study of the Icelandic Integrated System for Monitoring, Control and Surveillance. FAO Fisheries and Aquaculture Circular No. 1053. Rome: FAO.
Grémillet, D., Welcker, J., Karnovsky, N., Walkusz, W., Me, H., Fort, J., et al. (2012). Little auks buffer the impact of current Arctic climate change. Mar. Ecol. Prog. Ser. 454, 197–206. doi: 10.3354/meps09590
Gudmundsson, G. A., Alerstam, T., and Larsson, B. (1992). Radar observations of northbound migration of the Arctic tern, Sterna-paradisaea, at the Antarctic Peninsula. Antarct. Sci. 4, 163–170.
Hatch, J. J. (2002). “Arctic tern (Sterna paradisaea),” in The Birds of North America Online, eds A. Poole and F. Gill (Ithaca, NY: Cornell Lab of Ornithology). doi: 10.2173/bna.707
Hedd, A., Montevecchi, W. A., Phillips, R. A., and Fifield, D. A. (2014). Seasonal sexual segregation by monomorphic sooty shearwaters Puffinus griseus reflects different reproductive roles during the pre-laying period. PLoS One 9:e85572. doi: 10.1371/journal.pone.0085572
Hedenström, A., and Åkesson, S. (2016). Ecology of tern flight in relation to wind, topography and aerodynamic theory. Philos. Trans. R. Soc. Lond. B Biol. Sci. 371:20150396. doi: 10.1098/rstb.2015.0396
Hoegh-Guldberg, O., and Bruno, J. F. (2010). The impact of climate change on the world’s marine ecosystems. Science 328, 1523–1528. doi: 10.1126/science.1189930
Hooker, S. K., and Gerber, L. R. (2004). Marine reserves as a tool for ecosystem-based management: the potential importance of megafauna. BioScience 54, 27–39. doi: 10.1641/0006-3568(2004)054[0027:mraatf]2.0.co;2
Icelandic Institute of Natural History (2018). Red List for Birds. Available online at: https://en.ni.is/node/27843 (accessed August 16, 2021).
Johnson, B., and Colombelli-Négrel, D. (2021). Breeding success in Southern Australian Little Penguins is negatively correlated with high wind speeds and sea surface temperatures. Ornithol. Appl. 123:duaa062. doi: 10.1093/ornithapp/duaa062
Kogure, Y., Sato, K., Watanuki, Y., Wanless, S., and Daunt, F. (2016). European shags optimize their flight behavior according to wind conditions. J. Exp. Biol. 219, 311–318. doi: 10.1242/jeb.131441
Lane, J. V., Spracklen, D. V., and Hamer, K. C. (2019). Effects of windscape on three-dimensional foraging behaviour in a wide-ranging marine predator, the northern gannet. Mar. Ecol. Prog. Ser. 628, 183–193. doi: 10.3354/meps13089
Lascelles, B., Di Sciara, G. N., Agardy, T., Cuttelod, A., Eckert, S., Glowka, L., et al. (2014). Migratory marine species: their status, threats and conservation management needs. Aquat. Conserv. Mar. Freshw. Ecosyst. 24, 111–127. doi: 10.1002/aqc.2512
Lewis, S., Benvenuti, S., Dall–Antonia, L., Griffiths, R., Money, L., Sherratt, T. N., et al. (2002). Sex-specific foraging behaviour in a monomorphic seabird. Proc. R. Soc. Lond. B Biol. Sci. 269, 1687–1693. doi: 10.1098/rspb.2002.2083
Liebezeit, J. R., Smith, P. A., Lanctot, R. B., Schekkerman, H., Tulp, I., Kendall, S. J., et al. (2007). Assessing the development of shorebird eggs using the flotation method: species-specific and generalized regression models. Condor 109, 32–47. doi: 10.1093/condor/109.1.32
Lilliendahl, K. (2009). Winter diets of auks in Icelandic coastal waters. Mar. Biol. Res. 5, 143–154. doi: 10.1080/17451000802279636
Mallory, M. L., Davis, S. E., Maftei, M., Fife, D. T., and Robertson, G. J. (2018). Adult survival of Arctic terns in the Canadian High Arctic. Polar Res. 37:1537710. doi: 10.1080/17518369.2018.1537710
Mallory, M. L., Gaston, A. J., Forbes, M. R., and Gilchrist, H. G. (2009). Influence of weather on reproductive success of northern fulmars in the Canadian high Arctic. Polar Biol. 32, 529–538. doi: 10.1007/s00300-008-0547-4
McGowan, J., Beger, M., Lewison, R. L., Harcourt, R., Campbell, H., Priest, M., et al. (2017). Integrating research using animal-borne telemetry with the needs of conservation management. J. Appl. Ecol. 54, 423–429. doi: 10.1111/1365-2664.12755
McKnight, A., Allyn, A. J., Duffy, D. C., and Irons, D. B. (2013). ‘Stepping stone’ pattern in Pacific Arctic tern migration reveals the importance of upwelling areas. Mar. Ecol. Prog. Ser. 491, 253–264. doi: 10.3354/meps10469
Mills, J. A., Yarrall, J. W., Bradford-Grieve, J. M., Uddstrom, M. J., Renwick, J. A., and Merilä, J. (2008). The impact of climate fluctuation on food availability and reproductive performance of the planktivorous red-billed gull Larus novaehollandiae scopulinus. J. Anim. Ecol. 77, 1129–1142. doi: 10.1111/j.1365-2656.2008.01383.x
Monaghan, P., Uttley, J. D., and Burns, M. D. (1992). Effect of changes in food availability on reproductive effort in Arctic terns Sterna-paradisaea. Ardea 80, 70–81.
Monaghan, P., Uttley, J. D., Burns, M. D., Thaine, C., and Blackwood, J. (1989). The relationship between food supply, reproductive effort and breeding success in Arctic terns Sterna paradisaea. J. Anim. Ecol. 58, 261–274. doi: 10.2307/4999
Ocean Productivity Oregon State (2021). Standard Products. Available online at: http://sites.science.oregonstate.edu/ocean.productivity/standard.product.php (accessed October 20, 2021)
Oppel, S., Campos, S., Carneiro, A. P. B., Dias, M. P., Green, J. A., Masello, J. F., et al. (2018). Spatial scales of marine conservation management for breeding seabirds. Mar. Policy 98, 37–46. doi: 10.1016/j.marpol.2018.08.024
Paleczny, M., Hammill, E., Karpouzi, V., and Pauly, D. (2015). Population trend of the world’s monitored seabirds, 1950-2010. PLoS One 10:e0129342. doi: 10.1371/journal.pone.0129342
Pebesma, E. (2018). Simple features for R: standardized support for spatial vector data. R J. 10, 439–446. doi: 10.32614/rj-2018-009
Perrow, M. R., Skeate, E. R., and Gilroy, J. J. (2011). Visual tracking from a rigid-hulled inflatable boat to determine foraging movements of breeding terns. J. Field Ornithol. 82, 68–79. doi: 10.1111/j.1557-9263.2010.00309.x
Petersen, A., Robertson, G. J., Thorstensen, S., and Mallory, M. L. (2020). Annual survival of Arctic terns in western Iceland. Polar Biol. 43, 1843–1849. doi: 10.1007/s00300-020-02749-5
Piatt, J. F., Sydeman, W. J., and Wiese, F. (2007). Introduction: a modern role for seabirds as indicators. Mar. Ecol. Prog. Ser. 352, 199–204.
Pierce, D. (2019). ncdf4: Interface to Unidata netCDF (Version 4 or Earlier) Format Data Files. R Package Version 1.17.
Pratte, I., Boadway, K. A., Diamond, A. W., Mallory, M. L., and Giguère, N. (2018). Changes in isotopic niches across stages of the annual cycle in the Arctic tern (Sterna paradisaea). Arctic 71, 259–268. doi: 10.2307/26503284
R Core Team (2018). R: A Language and Environment for Statistical Computing 3.5.1. Vienna: R Foundation for Statistical Computing.
Redfern, C. P. F., and Bevan, R. M. (2014). A comparison of foraging behaviour in the North Sea by Black-legged Kittiwakes Rissa tridactyla from an inland and a maritime colony. Bird Study 61, 17–28. doi: 10.1080/00063657.2013.874977
Regular, P. M., Hedd, A., and Montevecchi, W. A. (2011). Fishing in the dark: a pursuit-diving seabird modifies foraging behaviour in response to nocturnal light levels. PLoS One 6:e26763. doi: 10.1371/journal.pone.0026763
Robertson, G. S., Bolton, M., Grecian, W. J., Wilson, L. J., Davies, W., and Monaghan, P. (2014b). Resource partitioning in three congeneric sympatrically breeding seabirds: foraging areas and prey utilization. Auk 131, 434–446. doi: 10.1642/auk-13-243.1
Robertson, G. S., Bolton, M., Grecian, W. J., and Monaghan, P. (2014a). Inter- and intra-year variation in foraging areas of breeding kittiwakes (Rissa tridactyla). Mar. Biol. 161, 1973–1986. doi: 10.1007/s00227-014-2477-8
Robinson, J. A., Hamer, K. C., and Chivers, L. S. (2002). Developmental plasticity in Arctic terns Sterna paradisaea and common terns S. hirundo in response to a period of extremely bad weather. Ibis 144, 344–346. doi: 10.1046/j.1474-919X.2002.00061.x
Rock, J. C., Leonard, M. L., and Boyne, A. W. (2007). Do co-nesting Arctic and common terns partition foraging habitat and chick diets? Waterbirds 30, 579–587. doi: 10.1675/1524-4695(2007)030[0579:dcaact]2.0.co;2
Sætre, R., Toresen, R., and Anker-Nilssen, T. (2002). Factors affecting the recruitment variability of the Norwegian spring-spawning herring (Clupea harengus L.). ICES J. Mar. Sci. 59, 725–736. doi: 10.1006/jmsc.2002.1180
Saevaldsson, H., and Gunnlaugsson, S. B. (2015). The Icelandic pelagic sector and its development under an ITQ management system. Mar. Policy 61, 207–215. doi: 10.1016/j.marpol.2015.08.016
Salt, G. W., and Willard, D. E. (1971). The hunting behavior and success of Forster’s tern. Ecology 52, 989–998. doi: 10.2307/1933804
Saraux, C., Chiaradia, A., Salton, M., Dann, P., and Viblanc, V. A. (2016). Negative effects of wind speed on individual foraging performance and breeding success in little penguins. Ecol. Monogr. 86, 61–77. doi: 10.1890/14-2124.1
Seward, A., Taylor, R. C., Perrow, M. R., Berridge, R., Bowgen, K. M., Dodd, S., et al. (2020). Effect of GPS tagging on behaviour and marine distribution of breeding Arctic terns Sterna paradisaea. Ibis 163, 197–212. doi: 10.1111/ibi.12849
Sockman, K. W., and Hurlbert, A. H. (2020). How the effects of latitude on daylight availability may have influenced the evolution of migration and photoperiodism. Funct. Ecol. 34, 1752–1766. doi: 10.1111/1365-2435.13578
Spear, L. B., and Ainley, D. G. (1997). Flight speed of seabirds in relation to wind speed and direction. Ibis 139, 234–251. doi: 10.1111/j.1474-919X.1997.tb04621.x
Suddaby, D., and Ratcliffe, N. (1997). The effects of fluctuating food availability on breeding Arctic terns (Sterna paradisaea). Auk 114, 524–530. doi: 10.2307/4089260
Sydeman, W. J., Poloczanska, E., Reed, T. E., and Thompson, S. A. (2015). Climate change and marine vertebrates. Science 350, 772–777. doi: 10.1126/science.aac9874
Tarroux, A., Weimerskirch, H., Wang, S. H., Bromwich, D. H., Cherel, Y., Kato, A., et al. (2016). Flexible flight response to challenging wind conditions in a commuting Antarctic seabird: do you catch the drift? Anim. Behav. 113, 99–112. doi: 10.1016/j.anbehav.2015.12.021
Thaxter, C. B., Daunt, F., Hamer, K. C., Watanuki, Y., Harris, M. P., Grémillet, D., et al. (2009). Sex-specific food provisioning in a monomorphic seabird, the common guillemot Uria aalge: nest defence, foraging efficiency or parental effort? J. Avian Biol. 40, 75–84. doi: 10.1111/j.1600-048X.2008.04507.x
Thaxter, C. B., Lascelles, B., Sugar, K., Cook, A. S., Roos, S., Bolton, M., et al. (2012). Seabird foraging ranges as a preliminary tool for identifying candidate Marine protected areas. Biol. Conserv. 156, 53–61. doi: 10.1016/j.biocon.2011.12.009
Tomotani, B. M., Bil, W., van der Jeugd, H. P., Pieters, R. P. M., and Muijres, F. T. (2019). Carrying a logger reduces escape flight speed in a passerine bird, but relative logger mass may be a misleading measure of this flight performance detriment methods. Ecol. Evol. 10, 70–79. doi: 10.1111/2041-210X.13112
Vigfúsdóttir, F., Gunnarsson, T. G., and Gill, J. A. (2013). Annual and between-colony variation in productivity of Arctic terns in West Iceland. Bird Study 60, 289–297. doi: 10.1080/00063657.2013.811214
Volkov, A. E., Loonen, M. J. J. E., Volkova, E. V., and Denisov, D. A. (2017). New data for Arctic terns (Sterna paradisaea) migration from White Sea (Onega Peninsula). Ornithologia 41, 58–68.
Walter, U., and Becker, P. H. (1998). Influence of physical factors and fishing activity on the occurrence of seabirds scavenging around shrimpers in the Wadden Sea. Senckenb. Maritima 29, 155–162. doi: 10.1007/BF03043953
Walters, D., Baran, A. J., Boutle, I., Brooks, M., Earnshaw, P., Edwards, J., et al. (2019). The Met Office Unified Model global atmosphere 7.0/7.1 and JULES global land 7.0 configurations. Geosci. Model Dev. 12, 1909–1963. doi: 10.5194/gmd-12-1909-2019
Weimerskirch, H. (2007). Are seabirds foraging for unpredictable resources? Deep Sea Res. II Top. Stud. Oceanogr. 54, 211–223. doi: 10.1016/j.dsr2.2006.11.013
Weimerskirch, H., Capdeville, D., and Duhamel, G. (2000). Factors affecting the number and mortality of seabirds attending trawlers and long-liners in the Kerguelen area. Polar Biol. 23, 236–249. doi: 10.1007/s003000050440
Weimerskirch, H., Louzao, M., de Grissac, S., and Delord, K. (2012). Changes in wind pattern alter albatross distribution and life-history traits. Science 335, 211–214. doi: 10.1126/science.1210270
Weimerskirch, H., Salamolard, M., Sarrazin, F., and Jouventin, P. (1993). Foraging strategy of wandering albatrosses through the breeding season: a study using satellite telemetry. Auk 110, 325–342. doi: 10.1093/auk/110.2.325
Welcker, J., Steen, H., Harding, A., and Gabrielsen, G. W. (2009). Sex-specific provisioning behaviour in a monomorphic seabird with a bimodal foraging strategy. Ibis 151, 502–513. doi: 10.1111/j.1474-919X.2009.00931.x
Wendeln, H., and Becker, P. H. (1996). Body mass change in breeding common terns Sterna hirundo. Bird Study 43, 85–95. doi: 10.1080/00063659609460998
Wilson, M. T., and Laman, N. (2021). Interannual variation in the coastal distribution of a juvenile gadid in the northeast Pacific Ocean: the relevance of wind and effect on recruitment. Fish. Oceanogr. 30, 3–22. doi: 10.1111/fog.12499
Keywords: arctic tern, biologging, foraging behaviour, wind, fisheries
Citation: Morten JM, Burgos JM, Collins L, Maxwell SM, Morin E-J, Parr N, Thurston W, Vigfúsdóttir F, Witt MJ and Hawkes LA (2022) Foraging Behaviours of Breeding Arctic Terns Sterna paradisaea and the Impact of Local Weather and Fisheries. Front. Mar. Sci. 8:760670. doi: 10.3389/fmars.2021.760670
Received: 18 August 2021; Accepted: 07 December 2021;
Published: 21 January 2022.
Edited by:
Yan Ropert-Coudert, UMR 7372 Centre d’Études Biologiques de Chizé (CEBC), FranceReviewed by:
Amanda J. Gladics, Oregon Sea Grant (NOAA), United StatesArnaud Tarroux, Norwegian Institute for Nature Research (NINA), Norway
Copyright © 2022 Morten, Burgos, Collins, Maxwell, Morin, Parr, Thurston, Vigfúsdóttir, Witt and Hawkes. This is an open-access article distributed under the terms of the Creative Commons Attribution License (CC BY). The use, distribution or reproduction in other forums is permitted, provided the original author(s) and the copyright owner(s) are credited and that the original publication in this journal is cited, in accordance with accepted academic practice. No use, distribution or reproduction is permitted which does not comply with these terms.
*Correspondence: Lucy A. Hawkes, bC5oYXdrZXNAZXhldGVyLmFjLnVr
†ORCID: Joanne M. Morten, orcid.org/0000-0002-5783-9777; Sara M. Maxwell, orcid.org/0000-0002-4425-9378; Nicole Parr, orcid.org/0000-0001-6115-6816; William Thurston, orcid.org/0000-0003-4157-517X; Freydís Vigfúsdóttir, orcid.org/0000-0002-2162-837X; Matthew J. Witt, orcid.org/0000-0002-9498-5378; Lucy A. Hawkes, orcid.org/0000-0002-6696-1862