- 1Faculty of Fisheries and Protection of Waters, South Bohemian Research Centre of Aquaculture and Biodiversity of Hydrocenoses, University of South Bohemia in České Budějovice, České Budějovice, Czechia
- 2School of Environment and Natural Resources, The Ohio State University, Columbus, OH, United States
- 3Aquaculture Research Group (GIA), Institute of Sustainable Aquaculture and Marine Ecosystems (ECOAQUA), Universidad de Las Palmas de Gran Canaria, Las Palmas, Spain
This 10-week feeding experiment examined the effects of supplementing vitamin C (VC) and E (VE) in oxidized oil diets on growth, fatty acid composition, blood physiological indicators, innate immunity, antioxidant capacity, and liver and intestine histology of rainbow trout (Oncorhynchus mykiss). Four diets were produced including a fresh fish oil containing diet (FFO diet), an oxidized fish oil containing diet (OFO diet), and OFO diet supplemented with 500 mg kg–1 VC and 400 mg kg–1 VE (OFO+C500+E400 diet) or 1,000 mg kg–1 VC and 800 mg kg–1 VE (OFO+C1000+E800 diet). Four hundred and twenty fish averaging 46.9 ± 0.32 g were stocked into 12 tanks and fed the diets twice a day to visual satiety. The results showed no significant effect of OFO or vitamins supplementation on growth, feed intake and feed utilization (P > 0.05). The groups that received OFO and OFO+C1000+E800 diets had significantly (P < 0.05) lower hepatosomatic indices than the other groups. Muscle fatty acid composition showed decreased proportion of 15:0, 16:0, 16:1n-7, 16:2n-4, 18:1n-5, 18:2n-6 (linoleic acid) and total saturated fatty acids in OFO group. Whereas higher percentages of 20:3n-6 and 20:3n-3 were found in OFO group. In addition, the highest ratio of docosahexaenoic acid (DHA, 22:6n-3) to eicosapentaenoic acid (EPA, 20:5n-3) was detected in OFO+C500+E400 group. The highest serum triglyceride concentration was recorded in the OFO group. Serum aspartate aminotransferase activity increased in OFO and OFO+C1000+E800 groups compared to OFO+C500+E400 group. Furthermore, significantly higher alkaline phosphatase activity in blood was found in OFO and OFO+C500+E400 groups. Significantly lower serum lysozyme, antiprotease, superoxide dismutase, and catalase activities were recorded in OFO group compared to FFO and OFO+C500+E400 groups, and an opposite trend was observed for malondialdehyde concentration. Muscle VC and VE concentrations, and liver and intestine histology remained unaffected. To conclude, feeding diet containing oxidized oil with peroxide value of 182 meq kg–1 with/without VC and VE supplementation did not influence growth and tissue VC and VE concentrations of rainbow trout. However, supplementing 500 mg kg–1 VC and 400 mg kg–1 VE reversed the adverse effects of OFO on non-specific immune response and antioxidant capacity.
Introduction
Incorporating sufficient supply of polyunsaturated n-3 fatty acids (PUFAs) particularly eicosapentaenoic acid (EPA) and docosahexaenoic acid (DHA) into aquafeed is crucial for maintaining optimal growth, skeletal development and physiological functions of fish due to the lack of desaturation and elongation pathways for their biosynthesis in fish (Sargent et al., 1999; Mourente et al., 2000). However, these PUFAs are highly susceptible to oxidation and are readily oxidized during feed manufacturing process and storage leading to formation of primary oxidation products such as hydroperoxides and secondary products including aldehydes, ketones, alcohols, hydrocarbons, volatile organic acids, and epoxy compounds with damaging effects on cellular molecules and subsequently cellular integrity (Janssens et al., 2000; Chen et al., 2012; Wang et al., 2015). Oxidized lipid diets are not only off-flavored but also are deficient in vitamins, and essential fatty acids and amino acids (Sutton et al., 2006; NRC, 2011). Moreover, oxidation of dietary lipid results in loss of appetite, and reduced digestibility and availability of nutrients (Marmesat et al., 2009). The reported impacts of feeding oxidized oils diet in fish include deterioration of growth and feed utilization, bone malformation, liver degradation, interruption of lipid metabolism and synthesis of essential fatty acids, depression of tissue vitamin C and E contents, suppression of immune function, dwindled disease tolerance, and ultimately increased mortality (Fontagné et al., 2006; Alves Martins et al., 2007; Boglino et al., 2014; Gao et al., 2014). Furthermore, oxidized oil may trigger overproduction of reactive oxygen species causing oxidative stress with adverse effects on physiological functions in fish (Boglino et al., 2014; Song et al., 2019). Hence, inhibition/reduction of lipid oxidation to prevent its harmful impacts on fish health is crucial for both aquafeed manufacturers and fish farmers.
Antioxidant system components including superoxide dismutase and catalase provide protection against oxidative damage caused by free radicals. Moreover, application of exogenous compounds with antioxidant properties such as vitamins C and E which exert free radical scavenging activity can contribute to protection of cells and tissues against oxidative stress (Winston and Digiulio, 1991). Vitamin C (VC) plays key roles as an essential nutrient and a water-soluble antioxidant in various physiological functions in fish (Lim and Lovell, 1978). Vitamin E (VE) is also a well-known antioxidant that acts as a shield against oxidizing agents (Tocher et al., 2002). Generation of free radicals during lipid peroxidation results in tissue VE depletion in animals (McDowell, 1989). Literature review shows that VE requirement of fish changes with peroxidation of dietary lipid (Zhong et al., 2008), and that supplementing higher doses of VE can inhibit lipid peroxidation and strengthen fish antioxidant capacity (Tocher et al., 2003). There are several reports indicating that there is an interactive effect between VC and VE in precluding lipid peroxidation (Niki et al., 1982; Gao et al., 2014), and that VC is capable of sparing VE in various fish species (Lee and Dabrowski, 2003; Gao et al., 2012).
The objectives of this research were to examine (a) effects of oxidized fish oil on growth, muscle fatty acid profile, blood biochemistry, innate immunity, antioxidant activity and histology of rainbow trout (Oncorhynchus mykiss), and (b) whether combined supplementation of VC and VE could ameliorate the deleterious effects of oxidized fish oil.
Materials and Methods
Experimental Diets
Formulation and proximate composition of the experimental diets are provided in Table 1. Four experimental diets were formulated to be isonitrogenous (42% crude protein) and isolipidic (20% crude lipid). Fresh salmon oil was used as the lipid source in the basal diet (FFO diet), and another diet was prepared by replacing FFO with oxidized fish oil (OFO diet). A basal level of vitamin C and E were included in the FFO and OFO diets to meet the requirement of rainbow trout (Table 1). Two additional diets were prepared using OFO as the lipid source and formulated to contain 500 or 1,000 mg kg–1 VC (ascorbic acid) and 400 or 800 mg kg–1 VE (α-tocopherol) (OFO+C500+E400 and OFO+C1000+E800 diets) (Huang and Huang, 2004; Gao and Koshio, 2015; Fatima et al., 2019). OFO was prepared by heating fresh salmon oil at 70°C for 16 h with vigorous aeration in an incubator. Peroxide value of OFO was measured to be 182 meq kg–1. All dry ingredients were thoroughly mixed and after addition of FFO/OFO and double distilled water extruded through a laboratory pelleting machine in 3 mm diameter. Then, the diets were dried at 35°C overnight, sealed in bags and stored at 4°C until used.
Fish and Feeding Trial
Rainbow trout juveniles were transported from a commercial farm (BioFish Ltd.) to experimental facility of University of South Bohemia. All the fish were fed a commercial diet (Inicio Plus, BioMar) for 2 weeks to acclimatize them to the experimental conditions and facilities. At the end of the acclimation period, 35 randomly captured fish (46.9 ± 0.32 g) were distributed into each polyvinyl circular tank of 150 L capacity and supplied with freshwater at a flow rate of 2 L min–1 and aeration. Triplicate groups of fish were hand-fed the test diets to satiation twice daily (07:00 and 15:00 h) for 10 weeks. Feeding was stopped 24 h prior to weighing or blood sampling to minimize handling stress. Rearing water temperature, pH, and concentrations of dissolve oxygen (DO), nitrite (NO2–) and ammonium nitrogen (NH4+-N) were measured daily, and their values were estimated at 14.6 ± 0.08°C, 6.82 ± 0.03, 11.3 ± 0.19 mg l–1, 0.47 ± 0.02 mg l–1, and 0.35 ± 0.02 mg l–1, respectively. Photoperiod was maintained on a 12:12 light:dark schedule.
Sample Collection
At the end of the feeding trial, all the fish in each tank were bulk-weighed and counted for calculation of growth parameters and survival. Twelve fish per tank were randomly captured, anesthetized with 2-phenoxyethanol (200 mg l–1), and blood samples were collected from the caudal vein with non-heparinized syringes, allowed to clot at 4°C for 24 h, then serum was separated by centrifugation for 10 min at 5,000 × g and stored at −70°C for the analysis of blood biochemical, innate immunity and antioxidant parameters. Three different sets of three fish per tank were collected for analysis of muscle proximate composition, VC and VE concentrations, and fatty acid composition. Five fish per tank were used for calculation of viscerosomatic index (VSI) and hepatosomatic index (HSI). Liver and intestine samples were collected from three fish per tank (nine fish per treatment) for histological examinations.
Analytical Methods
Muscle Chemical and Fatty Acid Composition
Analyses of moisture, crude protein, crude lipid and ash contents of the experimental diets and muscle samples were performed based on the standard procedures (Asociation of Official Analytical Chemists (AOAC), 2002). Moisture was determined by drying the samples in an oven at 105°C to constant weight; crude protein was analyzed by the Kjeldahl method (N × 6.25) with a FOSS Kjeltec 8400 analyser after acid digestion in an autodigester (FOSS; Tecator); crude lipid was determined by Soxhlet extraction in ether; ash content was measured by the combustion method in a muffle furnace at 550°C for 8 h. Peroxide value was determined according to AOCS (1990). VC concentrations of diet and muscle samples were analyzed using high-performance liquid chromatography (HPLC) according to Sakakura et al. (1998). VE concentration was quantified using HPLC with a fluorescence detector as described by Gao et al. (2012). Fatty acid methyl esters of the lipids were obtained by transmethylation (Christie, 1989), which were subsequently separated by gas liquid chromatography as explained earlier (Izquierdo et al., 1990).
Serum Biochemical, Immune, and Antioxidant Parameters
Serum biochemical parameters including total protein [TP], total cholesterol [T-CHO], triglyceride [TG], glucose [GLU], alanine aminotransferase [ALT], aspartate aminotransferase [AST], and alkaline phosphatase [ALP] were measured using kits with a VET-TEST 8008 analyzer (IDEXX Laboratories Inc., Maine, United States).
Antioxidant capacity parameters such as superoxide dismutase [SOD] and catalase [CAT] activities, and malondialdehyde [MDA] concentration were analyzed spectrophotometrically using commercial diagnostic kits (Sigma).
Lysozyme activity was measured according to the turbidimetric method described by Swain et al. (2007) with some modifications. Myeloperoxidase (MPO) activity was measured according to Quade and Roth (1997). Shortly, 20 μl of serum was diluted with HBSS (Hanks Balanced Salt Solution) without Ca2+ or Mg2+ (Sigma-Aldrich, United States) in 96-well plates. Then, 35 μl of 3,3′,5,5′-tetramethylbenzidine hydrochloride (TMB, 20 mM) (Sigma-Aldrich, United States) and H2O2 (5 mM) were added. The color change reaction was stopped after 2 min by adding 35 μl of 4 M sulfuric acid. Finally, the optical density was read at 450 nm in a microplate reader.
Antiprotease activity was measured according to the method described by Ellis et al. (1990) with slight modifications (Magnadóttir et al., 1999). Twenty microliters of serum was incubated with 20 μl of standard trypsin solution (Type II-S, from porcine pancreas, 5 mg ml–1, Sigma-Aldrich) for 10 min at 22°C. Then, 200 μl of phosphate buffer (0.1 M, pH 7.0) and 250 μl azocasein (2%) (Sigma) were added and incubated for 1 h at 22°C. Thereafter, 500 μl of trichloroacetic acid (10%) was added and incubated for 30 min at 22°C. Subsequently, the mixture was centrifuged at 6,000 × g for 5 min and 100 μl of the supernatant was placed in the wells of 96-well flat-bottomed microplate containing 100 μl of 1 N NaOH, and the OD was read at 430 nm. For positive control, buffer replaced the serum, and for the negative control buffer replaced both serum and trypsin. The inhibition percentage of trypsin was estimated as follows:
where A1 = control trypsin activity (without serum); A2 = trypsin activity remained after adding serum.
Histology
The liver samples were fixed in neutral buffered formalin 10%. Intestine samples were transferred into Davidson’s fixative (preserved overnight) and subsequently transferred into ethanol (70%). The samples were dehydrated in ascending ethanol concentrations (70, 95, and 100%), cleared in xylene, embedded in paraffin, and cut into a series of 5 μm longitudinal and crossed sections using a rotary microtome (Galileo, Italy). Sections were subsequently stained with hematoxylin and eosin (H&E) using a staining robot (Tissue-Tek DRS 2000, Sakura). The slides were assessed for general histopathological alterations and tissue structure, and later assessment of selected cells in each tissue was conducted. Pathological alterations were identified as described by Takashima and Hibiya (1995); Bernet et al. (1999), and Saraiva et al. (2016). The evaluation of the slides was conducted blinded by three independent observers. Histological slides were analyzed and photographed using an Olympus EX51 light microscope fitted with Canon E600 digital camera and the program QuickPhoto 3.2.
Statistical Analysis
All dietary treatments were assigned by a completely randomized design. Data were analyzed by one-way analysis of variance (ANOVA) in SPSS version 20 (SPSS Inc., Chicago, IL, United States). When ANOVA identified differences among groups, the difference in means was made with Duncan multiple range test. Statistical significance was determined at P < 0.05. Data are presented as mean ± SE. Percentage data were arcsine transformed before statistical analysis.
Results
Growth Performance
The results showed no significant effects of OFO or VC and VE supplementation on growth, feed intake, feed utilization and survival rate of rainbow trout (P > 0.05) (Table 2). Significantly (P < 0.05) lower HSI values were found in the groups received OFO and OFO+C1000+E800 diets compared to FFO and OFO+C500+E400 groups.
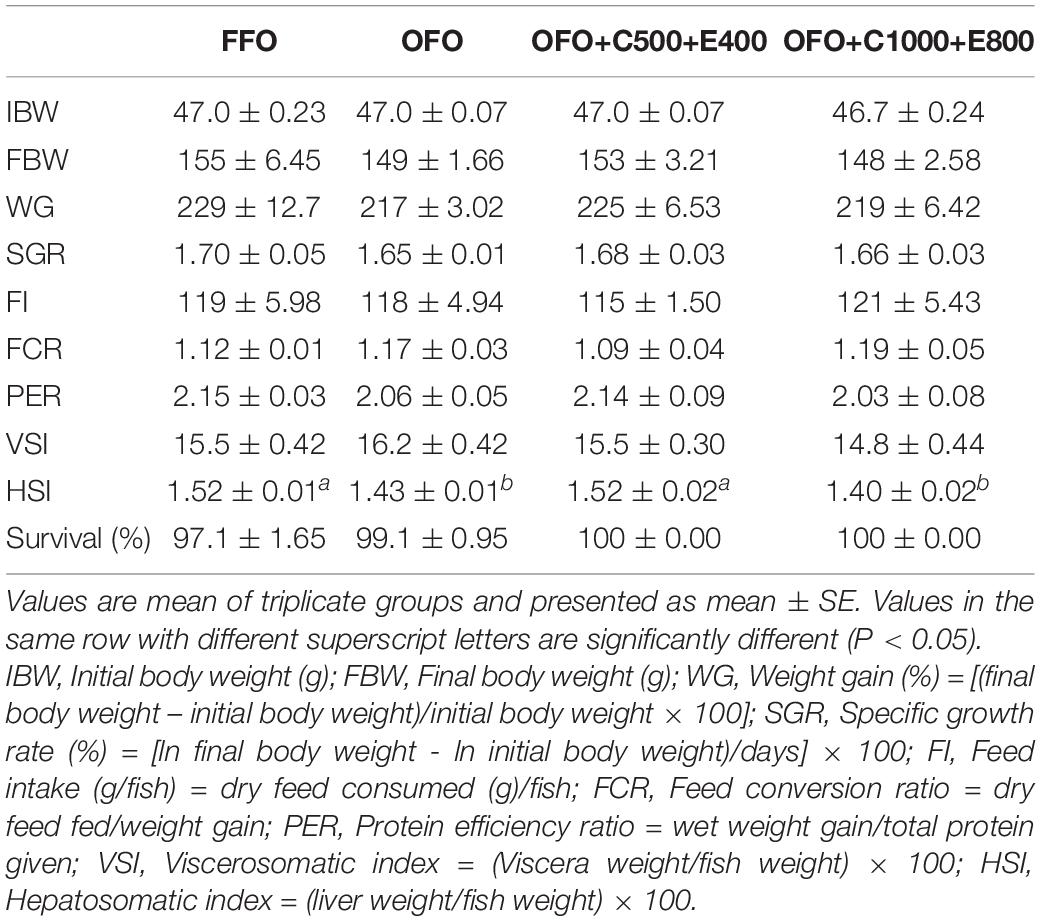
Table 2. Growth, feed utilization, organosomatic indices, and survival rate of rainbow trout (Oncorhynchus mykiss) fed the experimental diets for 10 weeks.
Muscle Proximate and Fatty Acid Composition
Muscle protein, lipid and ash contents were not influenced by dietary treatments (Table 3). Lower muscle moisture content was found in OFO+C1000+E800 group than the other groups. Muscle fatty acid composition analysis revealed reduction of 15:0, 16:0, 18:2n-6 (linoleic acid) and total saturated fatty acids (SFA) in OFO group compared to the FFO group (Table 4). Moreover, significantly lower 16:1n-7, 16:2n-4, 18:1n-5, and 18:2n-4 proportions were found in OFO and OFO+C500+E400 groups than the FFO group. In contrast, higher concentration of 18:3n-6 and 20:3n-6 were detected in OFO and OFO+C500+E400 groups. Moreover, OFO+C500+E400 group exhibited the highest docosahexaenoic acid (DHA, 22:6n-3) to eicosapentaenoic acid (EPA, 20:5n-3) ratio.
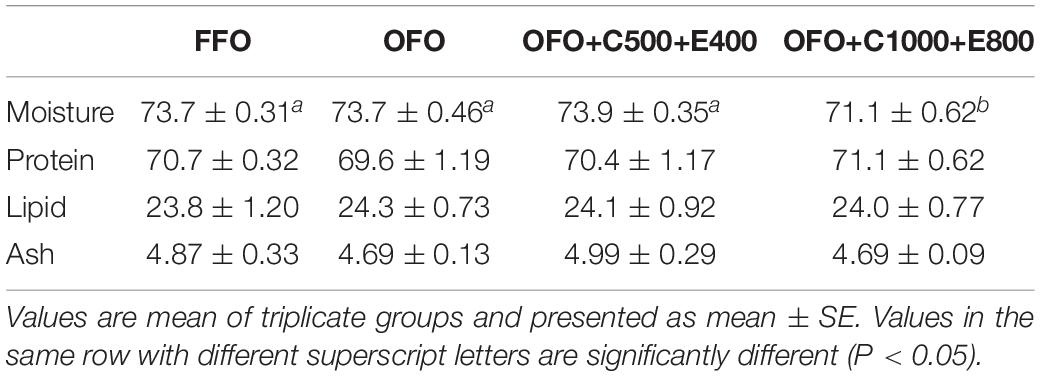
Table 3. Muscle proximate composition of rainbow trout fed the experimental diets for 10 weeks (% dry weight basis).
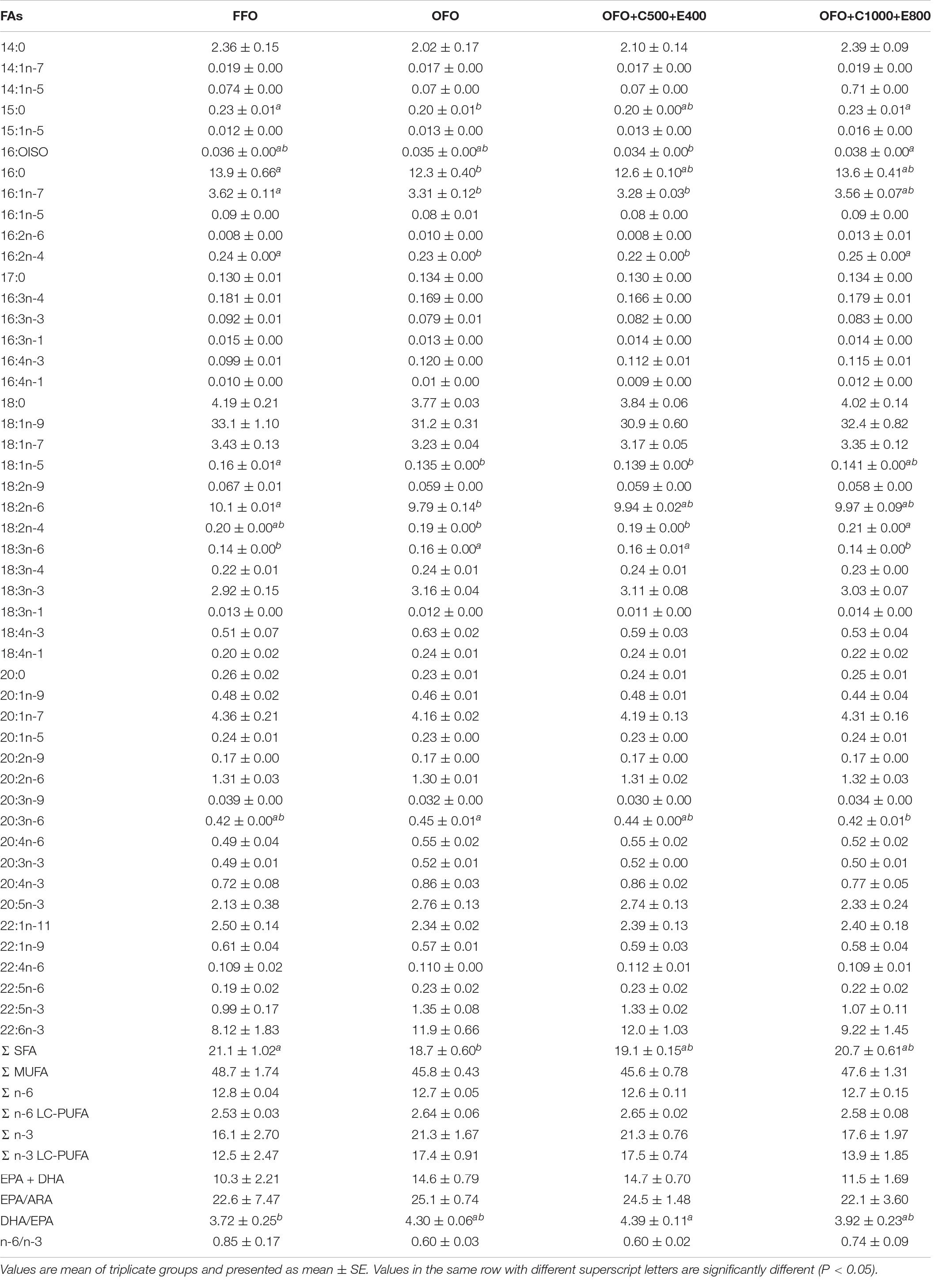
Table 4. Muscle fatty acid (FA) composition of rainbow trout fed the experimental diets for 10 weeks (% total identified FA).
Slight reductions in filet VC and VE concentrations were found in OFO group compared to FFO group, and their concentrations increased by increasing dietary VC and VE levels in a dose dependent manner, however, the observed differences were not statistically significant (Figure 1).
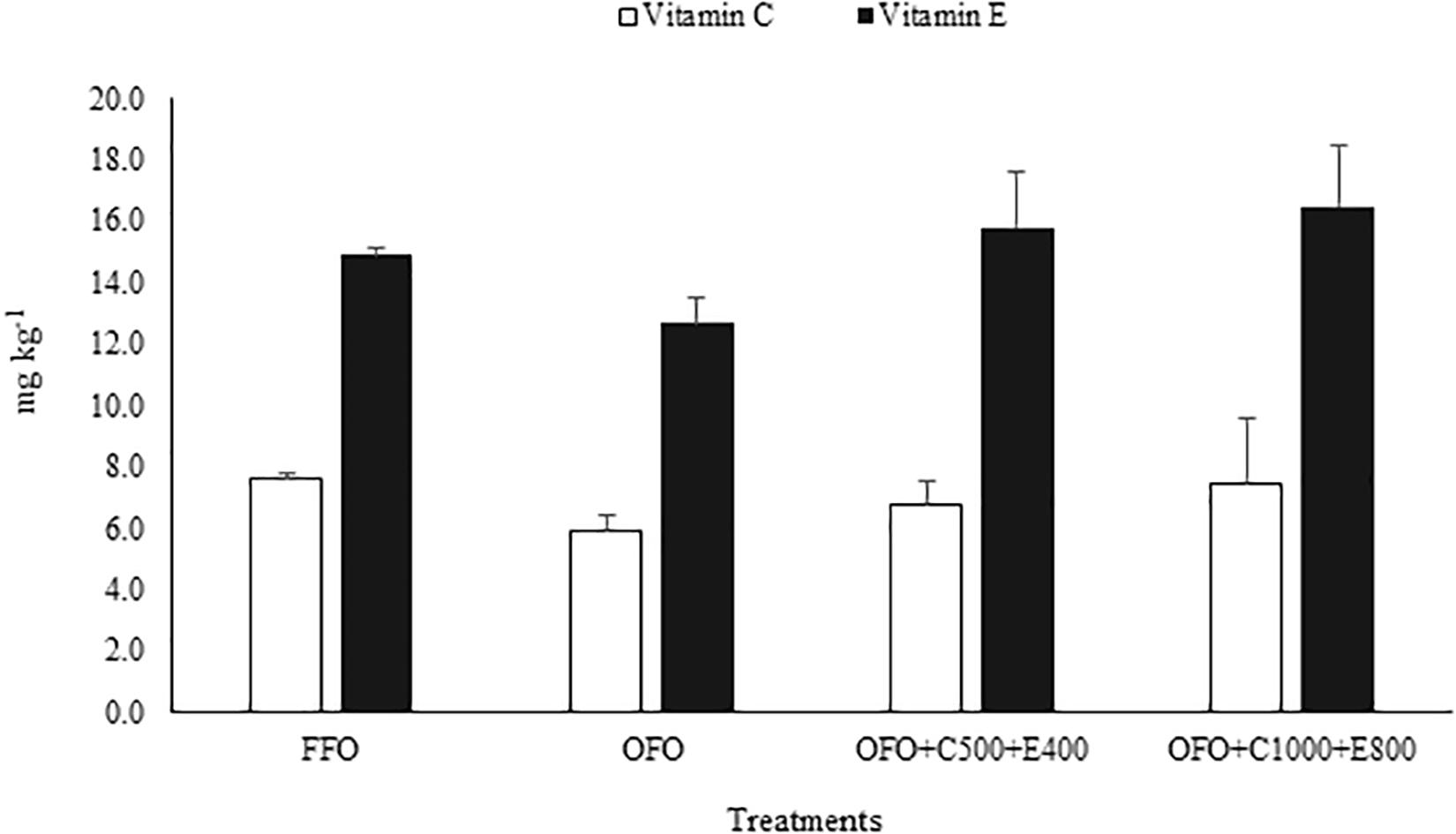
Figure 1. Vitamin C and E concentrations in dorsal muscle of rainbow trout fed fresh fish oil diet (FFO diet), oxidized fish oil containing diet (OFO diet), OFO diet supplemented with 500 mg kg–1 VC and 400 mg kg–1 VE (OFO+C500+E400) or 1,000 mg kg–1 VC and 800 mg kg–1 VE (OFO+C1000+E800) for 10 weeks. No significant difference was found among treatments.
Serum Biochemical, Antioxidant, and Immune Parameters
OFO group exhibited drastically higher serum TG concentration than the other groups. Also, significantly higher serum AST activity was detected in OFO and OFO+C1000+E800 groups compared to the other treatments. Moreover, OFO and OFO+C500+E400 groups showed significantly higher serum ALP activity. However, serum TP, T-CHO, and GLU concentrations and ALT activity were not affected by dietary treatments (Table 5).
Serum lysozyme and antiprotease activities significantly decreased in OFO group compared to the FFO and OFO+C500+E400 groups. No significant difference was found for serum myeloperoxidase activity among the experimental treatments (Table 6). The groups fed FFO and OFO+C500+E400 diets revealed remarkably higher SOD and CAT activities than OFO and OFO+C1000+E800 groups, and an opposite trend was observed for serum MDA concentration (Table 6).
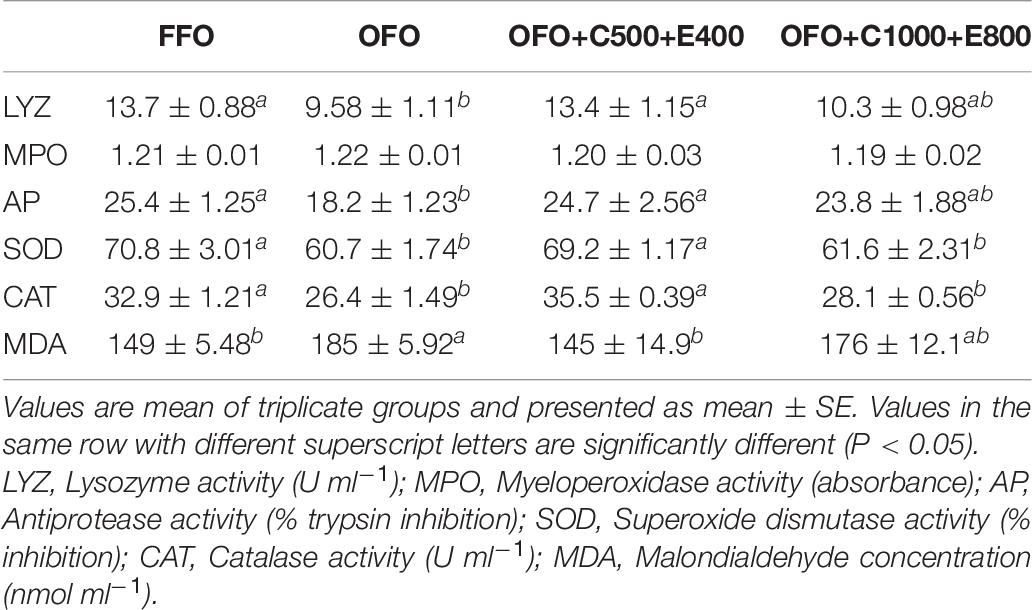
Table 6. Serum innate immunity and antioxidant parameters of rainbow trout fed the experimental diets for 10 weeks.
Liver and Intestine Histology
The results of histological examination of liver are presented in Figure 2. Hepatocytes of the fish were characterized by varying amounts of vacuoles and the cytoplasm showed mild to moderate granulation, however, no statistically significant differences were found among the treatment groups (Figure 3). No histopathological changes were observed in the intestines of examined fish (Figure 4). The mucosal epithelium was well developed as a single layer, the epithelial cells were uniform in size and arrangement, without indication of hypo/hyperplasia or hypo/hypertrophy. No indication for atrophic or necrotic alterations of the epithelial cells and indication for inflammation by infiltration of leukocytes or lymphocytes were observed.
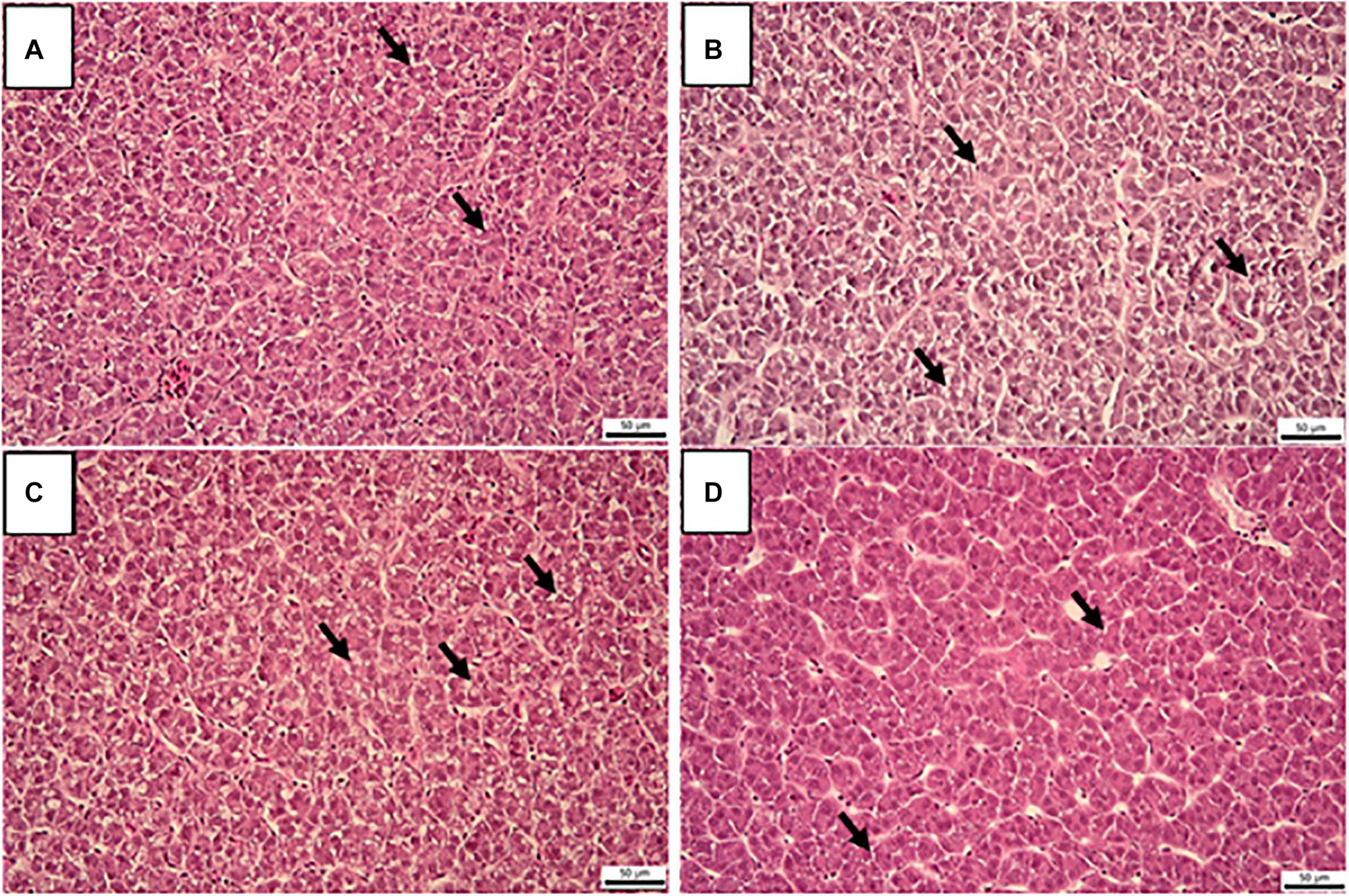
Figure 2. Cross sections of liver in rainbow trout fed fresh fish oil diet (FFO diet, (A)), oxidized fish oil containing diet (OFO diet, (B)), OFO diet supplemented with 500 mg kg–1 VC and 400 mg kg–1 VE (OFO+C500+E400, (C)) or 1,000 mg kg–1 VC and 800 mg kg–1 VE (OFO+C1000+E800, (D)) for 10 weeks. Arrows indicate vacuoles in the cytoplasm (H&E, scale bar 50 μm).
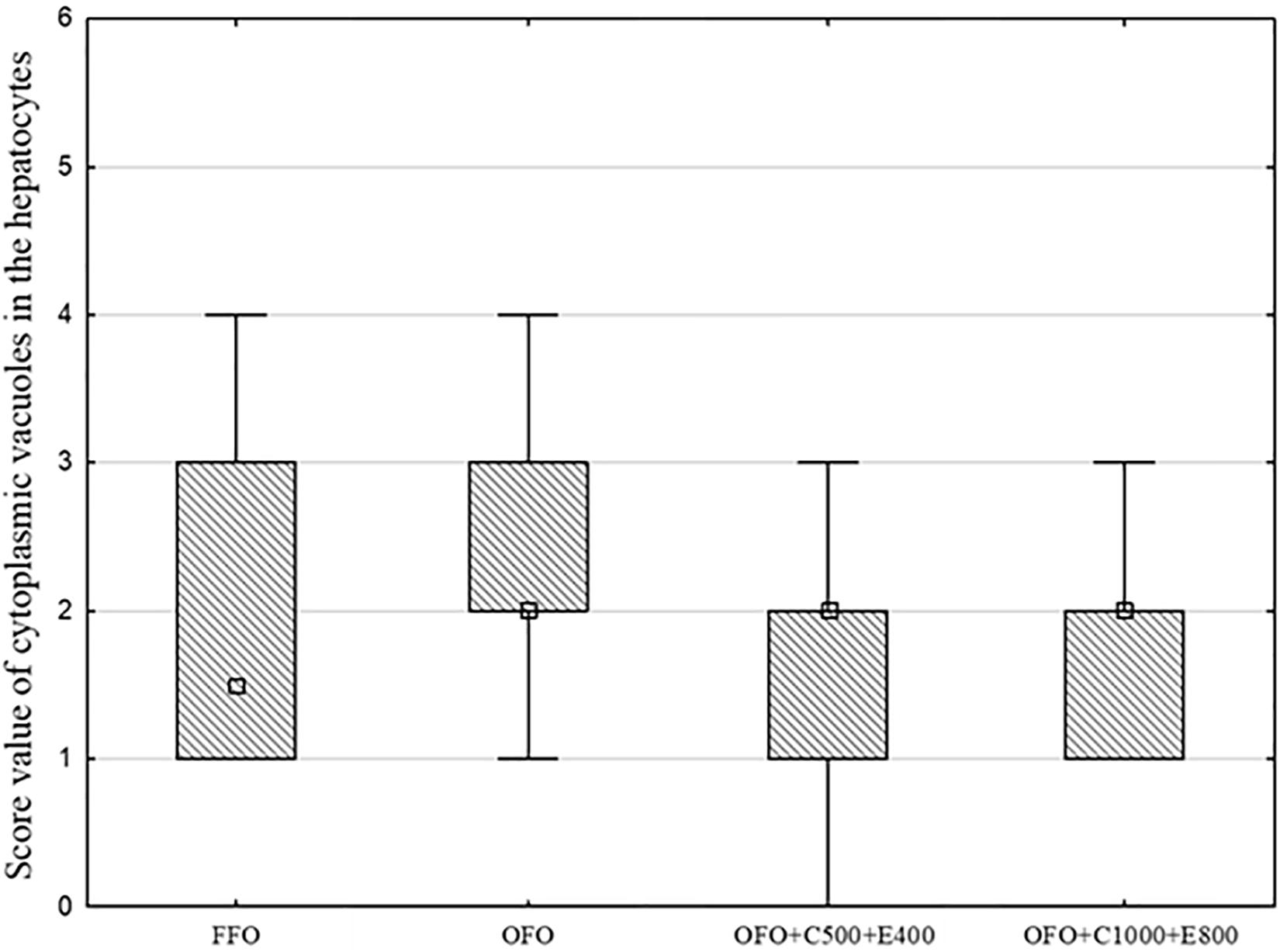
Figure 3. Box Plot of vacuoles in the cytoplasm of liver of rainbow trout fed fresh fish oil diet (FFO diet), oxidized fish oil containing diet (OFO diet), OFO diet supplemented with 500 mg kg–1 VC and 400 mg kg–1 VE (OFO+C500+E400) or 1,000 mg kg–1 VC and 800 mg kg–1 VE (OFO+C1000+E800). The absence of superscript letters indicates no significant differences among treatments (P > 0.05), n = 5–7.
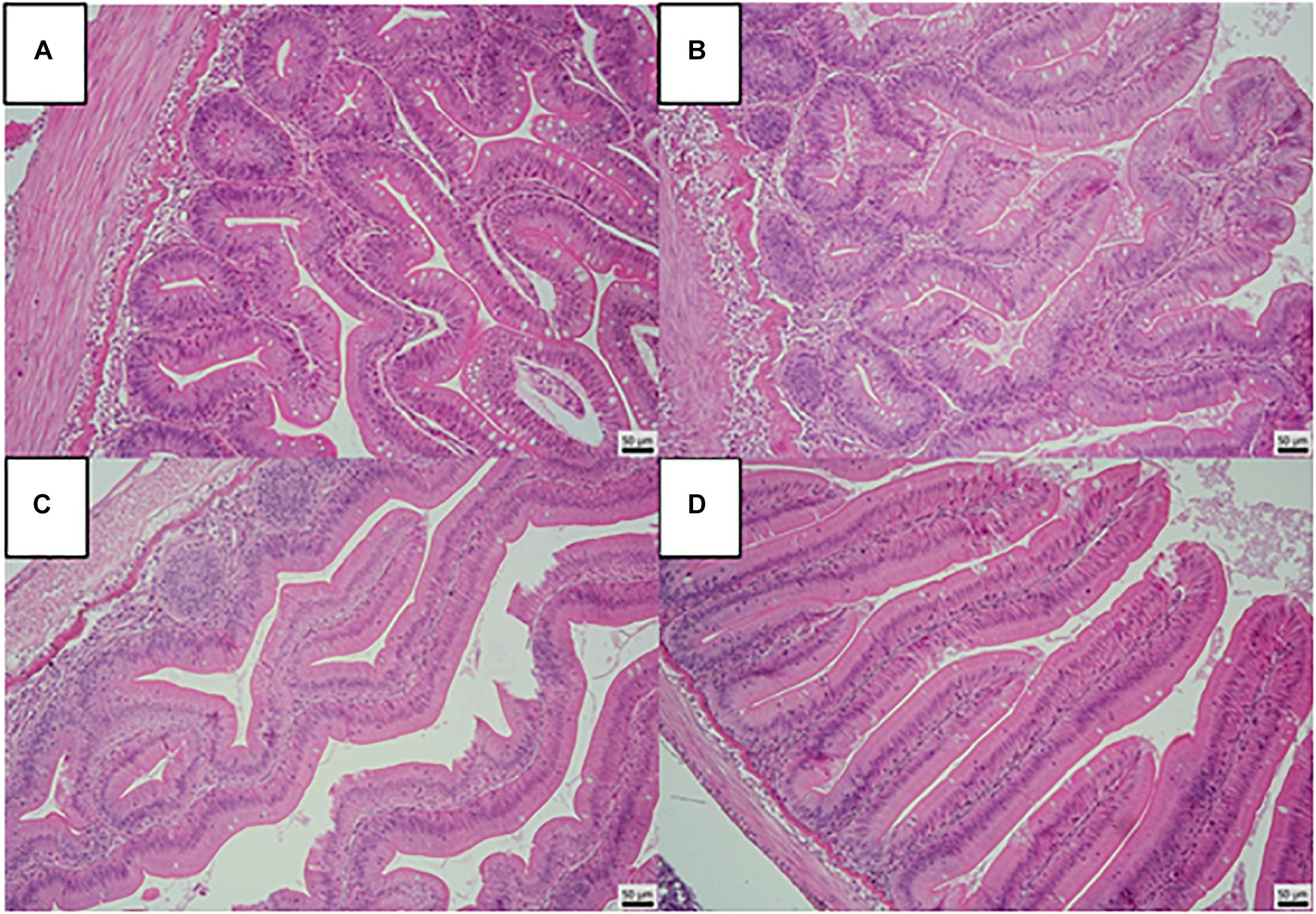
Figure 4. Foregut structure of rainbow trout fed fresh fish oil diet (FFO diet, (A)), oxidized fish oil containing diet (OFO diet, (B)), OFO diet supplemented with 500 mg kg–1 VC and 400 mg kg–1 VE (OFO+C500+E400, (C)) or 1,000 mg kg–1 VC and 800 mg kg–1 VE (OFO+C1000+E800, (D)). (H&E, scale bar 50 μm).
Discussion
There are several reports indicating that lipid oxidation adversely impacts feed intake and growth performance in various fish species (Kubiriza et al., 2017; Fatima et al., 2019), however, the results have been controversial. Chen X. et al. (2019) examined the effects of diets containing OFO with various degrees of oxidation and peroxide values (POV) of 6.43–402.7 meq kg–1 on growth of Rhynchocypris lagowski Dybowski, and their results showed the progressive reduction of growth, feed efficiency and protein efficiency as oxidation degree of fish oil increased. The results of a red sea bream (Pagrus major) study showed that offering diets with low (POV = 83.8 meq kg–1) and high degree of oxidation (POV = 159 meq kg–1) results in profound reduction of growth performance (Gao et al., 2012). Also, reduction of growth performance after administration of OFO diets has been reported in Atlantic salmon (Salmo salar L.) (Sutton et al., 2006) and Labeo rohita (Fatima et al., 2019). In contrary, studies on gilthead sea bream (Sparus aurata L.) (Tocher et al., 2003) and largemouth bass (Micropterus salmoides) (Chen et al., 2012; Yin et al., 2019) showed the improvement of growth performance by feeding OFO diets. However, there are several reports indicating no significant effect of oxidized oil diet on growth of Atlantic halibut (Hippoglossus hippoglossus) (Lewis-McCrea and Lall, 2007), Atlantic cod (Gadus morhua) (Zhong et al., 2008), Chinese longsnout catfish (Leiocassis longirostris) (Dong et al., 2011), red sea bream (Gao et al., 2013), and Senegalese sole (Solea senegalensis) (Boglino et al., 2014). Likewise, in the current study inclusion of OFO (POV = 182 meq kg–1) in rainbow trout feed did not influence growth performance which agrees with a previous rainbow trout study (Fontagné-Dicharry et al., 2018). These inconsistencies may stem from the variation in oxidation degree of oil, feeding period, different tolerance of fish species to oxidized oil, or differences in degree of protection against oxidative damage by antioxidant defense system (Tocher et al., 2003; Dong et al., 2011; Gao et al., 2013; Yin et al., 2019). Also, different species may disparately react to the changes in feed odor due to OFO which will impact feed intake, some fish might be sensitive to particular odors while the smell of OFO may stimulate feed intake in some other fish species (Dong et al., 2014; Chen X. et al., 2019). Moreover, feed composition may influence the effects of OFO on fish growth as some feed ingredients could counteract the adverse impacts of OFO through disguising the odor of OFO, dilution of oxidation products or reducing OFO toxicity (Chen et al., 2012; Chen X. et al., 2019).
On the other hand, in this study supplementing VC and VE in OFO diet did not influence fish growth, although OFO+C500+E400 group showed slightly higher weight gain (WG) and specific growth rate (SGR) than the OFO group. Similarly, no improvements were found in growth performance of turbot (Scophthalmus maximus) (Tocher et al., 2003), halibut (Hippoglossus hippoglossus) (Tocher et al., 2003), and Atlantic cod (Zhong et al., 2008) by VE addition to oxidized oil diets. Moreover, combined supplementation of different doses of VC and VE in oxidized oil diets for red sea bream did not result in any changes in growth performance compared to fish received a FFO diet (Gao and Koshio, 2015). However, Gao et al. (2012) showed that supplementing 100 or 200 mg kg–1 VE in OFO diet improves growth performance of red sea bream. Also, another red sea bream study showed the improvement of growth by supplementing 400 or 800 mg kg–1 VC in oxidized oil diets (Gao et al., 2013). In contrast, Zhang et al. (2016) reported the deleterious effect of supplementing 200 mg kg–1 VE in oxidized diets on growth performance of Japanese seabass (Lateolabrax japonicus). Also, Gao et al. (2014) examined the interactive effects of adding varying doses of VC and VE to oxidized oil diets, and their results showed that co-supplementation of 200 mg kg–1 VE and 1,000 mg kg–1 VC leads to suppressed growth of Japanese flounder (Paralichthys olivaceus).
In this study, OFO and OFO+C1000+E800 groups exhibited significantly lower HSI values than the other treatments. Likewise, a largemouth bass study showed the reduction of HSI in fish fed oxidized diet, but no changes were observed by VE application (Chen et al., 2013). In contrast, black sea bream (Acanthopagrus schlegeli) fed oxidized diet showed higher HSI than those offered FFO diet, and intermediary values were found for groups received oxidized diet supplemented with varying levels of VE (Peng et al., 2009). Also, a red sea bream study showed the reduction of HSI by increasing VE supplementation level in low and high oxidized diets (Gao et al., 2012). No significant effect of dietary treatments was found on muscle protein and lipid contents which is in parallel to studies on hybrid tilapia (Oreochromis niloticus × O. aureus) (Huang and Huang, 2004) and Japanese flounder (Gao et al., 2014).
Fatty acid composition analysis showed decreased muscle 15:0, 16:0, 16:1n-7, 16:2n-4, 18:1n-5, 18:2n-6, 18:2n-4, and total SFA contents in fish fed OFO diet, and addition of 1,000 mg kg–1 VC and 800 mg kg–1 VE in OFO diet enhanced their proportion. Similarly, feeding oxidized oil diet led to remarkable reduction of 16:1, 18:2, 18:3n-3, 20:3n-3, and 20:5-n3 in muscle of Atlantic cod, and VE supplementation retrieved their values with the exception of 20:3n-3 (Zhong et al., 2008). Also, an Atlantic halibut study showed decreased muscle 18:2n-6, 20:1n-9, 22:5n-3, and total monounsaturated fatty acids (MUFA) contents in groups received moderately or highly oxidized fish oil but their values were not affected by VE supplementation (Lewis-McCrea and Lall, 2007). Moreover, feeding low/high oxidized oil diets led to reduced 18:2n-6, 20:5n-3, 22:5n-3, and 22:6n-3, and increased 16:1n-7, 18:1n9, and 22:1n-11 contents in muscle of red sea bream, and VC supplementation in low oxidized oil diet recovered 22:5n-3 and 22:6n-3 contents (Gao et al., 2013). On the other hand, in the current study higher proportion of eicosapentaenoic acid (EPA, 20:5n-3), docosahexaenoic acid (DHA, 22:6n-3), total n-3, and total n-3 long-chain polyunsaturated fatty acids (LC-PUFA) were found in OFO group and their percentages decreased in OFO+C1000+E800 group, although the differences were not statistically significant. A similar observation has also been found in African catfish (Clarias gariepinus) (Baker and Davies, 1996), black sea bream (Peng et al., 2009), and Labeo rohita (Fatima et al., 2019). The higher proportion of LC-PUFA in fish fed oxidized oil diet could be due to higher energy demand of fish in response to oxidized oil which is met through utilization of other fatty acids such as SFA and MUFA (confirmed by their decreased proportion in this study) leading to increased relative percentage of LC-PUFA (Peng et al., 2009; Fatima et al., 2019). It has also been suggested that peroxidation of LC-PUFA by free radicals may induce compensatory overproduction of these fatty acids (Buttriss and Diplock, 1988).
Feeding oxidized diets is associated with depletion of antioxidants such as VE in tissues (Baker and Davies, 1997). Our results showed the non-significant reduction of dorsal muscle VC and VE contents in OFO group and their concentrations numerically increased by VC and VE supplementation. This may indicate that the basal levels of VC and VE were adequate to restrain lipid peroxidation in the body. Likewise, Gao et al. (2013) found no significant changes in muscle VC and VE contents of red sea bream fed diets with different degree of oxidation and VC levels. Also, feeding diets containing moderately and highly oxidized oil did not impact muscle and liver VE concentration in Atlantic halibut (Lewis-McCrea and Lall, 2007). However, a largemouth bass study showed the progressive reduction of muscle and liver VE concentrations following administration of diets with different degrees of oxidation (Chen et al., 2012). Also, Fatima et al. (2019) showed that fish oil oxidation results in decreased muscle and liver VE concentrations in Labeo rohita fingerlings, but no changes could be found due to VE addition. These discrepancies could be due to different absorption rate of lipid oxidation products among species, experimental conditions (Fatima et al., 2019), and differences in degree of oil oxidation, and supplemental doses of VC and VE in the basal diet. Furthermore, Dabrowski et al. (1990) indicated that 8–10-fold body weight increase is required to demonstrate ascorbate deficiency in rainbow trout whereas in the current study fish weight gain was much smaller.
Blood biochemical parameters are used as reliable means for monitoring fish health (Rahimnejad et al., 2019). We found a drastic enhancement in serum TG concentration in fish fed oxidized oil diet and co-supplementation of VC and VE significantly decreased its concentration. Likewise, Gao et al. (2012) reported the increment of plasma TG concentration in red sea bream fed OFO and increasing dietary VE level reduced its concentration. Also, feeding diets containing highly oxidized fish oil led to elevated serum TG level in orange spotted grouper (Epinephelus coioides) (Liu et al., 2019). Impacts of oil oxidation on lipid metabolism has been barely investigated in fish, however, studies on rats revealed suppressed activity of hepatic enzymes which are involved in fatty acids synthesis (Eder and Kirchgessner, 1998; Lamboni et al., 1998). Yuan et al. (2014) showed that oil oxidation results in decreased liver fat content, and increased plasma TG and CHO concentrations in largemouth bass and suggested that oil oxidation may lead to deteriorated absorption of these nutrients in the liver. Also, Gao et al. (2012) noted that the reduction of plasma TG concentration by VE supplementation could be associated with reduction of TG in chylomicrons. Moreover, TG lowering property of VC has been documented in several fish species such as red sea bream (Tongjun et al., 2008), yellow catfish (Pelteobagrus fulvidraco) (Liang et al., 2017), and Nile tilapia (Oreochromis niloticus) (El Basuini et al., 2021). Serum/plasma ALT and AST activities are used as diagnostic tools for liver damage. Xie et al. (2020) reported the progressive increment of plasma ALT and AST activities in largemouth bass by increasing oxidation degree of fish oil. Similarly, in this study the highest serum AST activity was detected in OFO group which significantly differed from that of the OFO+C500+E800 group. Gao et al. (2014) showed that combined supplementation of 1,000 mg kg–1 VC and 100 mg kg–1 VE in OFO diet results in reduced plasma AST activity in Japanese flounder whereas increasing VE dose to 200 mg kg–1 led to a doubled AST activity which is consistent with the present study. These authors pointed out that overdose supplementation of VC and VE can result in lipid peroxidation leading to liver damage. Elevated serum ALP activity is also inferred as a sign of liver dysfunction (Owu et al., 1998). In this study, serum ALP activity increased in OFO and OFO+C500+E400 groups. Similarly, Lewis-McCrea and Lall (2007) found numerical increase of serum ALP activity in Atlantic halibut fed oxidized diets. Also, offering oxidized oil containing diet led to drastic enhancement of serum ALP activity in Wistar rats (Owu et al., 1998).
Lysozyme is a bactericidal enzyme which is considered as a key component of humoral innate immunity providing protection against pathogens in fish (Ren et al., 2007; Rahimnejad and Lee, 2014). Our results showed the suppression of lysozyme activity in OFO group and a significant improvement was found by supplementing 500 mg kg–1 VC and 400 mg kg–1 VE. Similar to our results, replacing FFO with OFO led to decreased serum/plasma lysozyme activity in sea bass (Dicentrarchus labrax) (Obach et al., 1993) and Japanese sea bass (Han et al., 2012). Antiprotease activity is an inhibitory component of fish innate immunity which acts through inhibiting the intrusion of pathogens via chelating their produced protease enzyme for digestion of the host protein (Ellis, 2001). Our results showed the significant enhancement of antiprotease activity in OFO+C500+E400 group compared to OFO group indicating enhanced protection of fish against invading pathogens (Shin et al., 2014). Likewise, Priyadarsani et al. (2021) reported elevated serum antiprotease activity by VE supplementation in diets for L. rohita. However, no effect of VC was found on plasma antiprotease activity in hybrid striped bass (Morone chrysops × M. saxatilis) (Yamamoto et al., 2020).
Deleterious impacts of oxidized oil on antioxidant system have been demonstrated in several fish and shellfish species (Fontagné-Dicharry et al., 2014; Chen S. et al., 2019; Song et al., 2019). VE and VC application has been used as an efficient strategy for attenuating oxidative stress in various fish species (Tocher et al., 2003; Chen et al., 2013; Gao et al., 2013; Fatima et al., 2019), however, both vitamins may act as a pro-oxidant at high doses (Kontush et al., 1996; Pearson et al., 2006; Poljsak and Ionescu, 2010). SOD, CAT, and glutathione peroxidase are the primary antioxidant enzymes that intercept the overproduction of reactive oxygen species in fish. MDA as the end product of lipid peroxidation is used as a key indicator for assessment of oxidative damage. Our results showed the significant reduction of SOD and CAT activates in OFO group and an opposite trend was observed for MDA concentration. Additionally, SOD and CAT activities increased, and MDA concentration decreased in OFO+C500+E400 group indicating that the moderate doses of VC and VE are adequate to prevent the oxidative stress caused by OFO in rainbow trout. Chen X. et al. (2019) reported the significant reduction of liver CAT and SOD activities and mRNA levels, and enhancement of liver MDA concentration by increasing oxidation degree of fish oil in R. lagowski Dybowski juveniles. Also, Gao et al. (2014) reported the increment of reactive oxygen metabolites (ROMs) and depressed biological antioxidant potential (BAP) in Japanese flounder fed OFO diet compared to those fed FFO diet. Moreover, their results showed the reduction of ROMs and enhancement of BAP in groups of fish received OFO+C500+E200 or OFO+C1000+E100 while detrimental impacts were found in fish fed OFO+C1000+E200 diet. In addition, Zhang et al. (2016) showed that supplementing 60 mg kg–1 VE in oxidized diet results in enhanced liver SOD and CAT activities and decreased MDA concentration while negative impacts were observed when VE dose was increased to 200 mg kg–1 diet. Our results provides further evidence that high doses of VC and VE may exert pro-oxidant effects. Kaewsrithong et al. (2001) noted that excessive supplementation level of VE can accelerate lipid peroxidation. Moreover, Eder et al. (2002) reported that excessive intake of VE when combined with salmon oil in the diet results in decreased antioxidant enzymes activity in erythrocytes of rats. VE protects cell membrane through donating hydrogen to lipid peroxyl radicals leading to formation of α-tocopher-oxyl radical which is an oxidant and is reduced by the act of VC or reduced glutathione (GSH). High dose of VE may trigger more creation of α-tocopher-oxyl radical which requires more VC and GSH to be reduced. Since VC and GSH are not capable of reducing all the produced α-tocopher-oxyl radical, excess α-tocopher-oxyl radical may act as a pro-oxidant (Jiang et al., 2009).
Increasing oxidation degree of fish oil resulted in degeneration of hepatocytes, characterized by lipid vacuoles and nuclear migration, in largemouth bass (Chen et al., 2012). The lack of significant difference in vacuolation of hepatocytes between FFO and OFO groups in this study may indicate that rainbow trout can tolerate diet containing oxidized fish oil with POV value of 182 meq kg–1. In addition, VC and VE supplementation partially reduced vacuolation of hepatocytes in this study indicating their protective effects (Chen et al., 2012). Absence of pathological effects of OFO on foregut in the current study agrees with an Atlantic cod research (Kjær et al., 2014).
Conclusion
To sum up, data generated in this study showed that fish oil with the oxidation degree used in this study or combined supplementation of VC and VE did not influence growth, feed intake, feed utilization, and muscle VC and VE concentrations of rainbow trout. Addition of 1,000 mg kg–1 VC and 800 mg kg–1 VE in OFO diet recovered muscle 16:1n-7, 16:2n-4, 18:1n-5, 18:2n-6, and 18:2n-4 contents. Moreover, non-specific immune response and antioxidant capacity were improved by application of 500 mg kg–1 VC and 400 mg kg–1 VE in OFO diet.
Data Availability Statement
The raw data supporting the conclusions of this article will be made available by the authors, without undue reservation.
Ethics Statement
All the experimental procedures were performed in compliance with valid legislative regulations in Czech Republic (law nos. 166/1996 and 246/1992); the permit was issued to Nos. 2293/2015-MZE-17214 and 55187/2016-MZE-17214. All samplings were carried out with the relevant permission from the Departmental Expert Committee for Authorization of Experimental Projects of the Ministry of Education, Youth and Sports of the Czech Republic (permit no. MSMT 4394/2017-2).
Author Contributions
SR and KD: conceptualization, validation, visualization, methodology, writing, review, and editing. SR, MI, NH, AI, and CS: formal analysis. SR: investigation, collection and interpretation of data, and manuscript drafting. TP: funding acquisition, project administration, and resources. All authors contributed to the article and approved the submitted version.
Funding
The study was supported by the Ministry of Agriculture of the Czech Republic, project NAZV QK1710310, and by the Ministry of Education, Youth and Sports of the Czech Republic, project Biodiversity (CZ.02.1.01./0.0/0.0/16_025/0007370).
Conflict of Interest
The authors declare that the research was conducted in the absence of any commercial or financial relationships that could be construed as a potential conflict of interest.
Publisher’s Note
All claims expressed in this article are solely those of the authors and do not necessarily represent those of their affiliated organizations, or those of the publisher, the editors and the reviewers. Any product that may be evaluated in this article, or claim that may be made by its manufacturer, is not guaranteed or endorsed by the publisher.
References
Alves Martins, D., Afonso, L. O. B., Hosoya, S., Lewis-McCrea, L. M., Valente, L. M. P., and Lall, S. P. (2007). Effects of moderately oxidized dietary lipid and the role of vitamin E on the stress response in Atlantic halibut (Hippoglossus hippoglossus L.). Aquaculture 272, 573–580. doi: 10.1016/j.aquaculture.2007.08.044
AOCS (1990). Official Methods and Recommendation Practices of the American Oil Chemists’ Society. Champaign, IL: American Oil Chemists’ Society.
Asociation of Official Analytical Chemists (AOAC) (2002). Official Methods of Analysis of Official Analytical Chemists International, 17th Edn. Arlington, VA: Association of Official Analytical Chemists.
Baker, R. T. M., and Davies, S. J. (1996). Increased production of docosahexaenoic acid (22:6 n-3, DHA) in catfish nutritionally stressed by the feeding of oxidized oils and the modulatory effect of dietary α-tocopheryl acetate. J. Fish Biol. 49, 748–752. doi: 10.1006/jfbi.1996.0205
Baker, R. T. M., and Davies, S. J. (1997). Muscle and hepatic fatty acid profiles and α-tocopherol status in African catfish (Clarias gariepinus) given diets varying in oxidative state and vitamin E inclusion level. Anim. Sci. 64, 187–195. doi: 10.1017/s1357729800015708
Bernet, D., Schmidt, H., Meier, W., Burkhardt-Holm, P., and Wahli, T. (1999). Histopathology in fish: proposal for a protocol to assess aquatic pollution. J. Fish Dis. 22, 25–34. doi: 10.1046/j.1365-2761.1999.00134.x
Boglino, A., Darias, M. J., Estevez, A., Andree, K. B., Sarasquete, C., Ortiz-Delgado, J. B., et al. (2014). The effect of dietary oxidized lipid levels on growth performance, antioxidant enzyme activities, intestinal lipid deposition and skeletogenesis in Senegalese sole (Solea senegalensis) larvae. Aquac. Nutr. 20, 692–711. doi: 10.1111/anu.12123
Buttriss, J. L., and Diplock, A. T. (1988). The ga-tocopherol and phospholipid fatty acid content of rat liver subcellular membranes in vitamin E and selenium deficiency. Biochim. Biophys. Acta 963, 61–69. doi: 10.1016/0005-2760(88)90338-4
Chen, S., Zhuang, Z., Yin, P., Chen, X., Zhang, Y., Tian, L., et al. (2019). Changes in growth performance, haematological parameters, hepatopancreas histopathology and antioxidant status of pacific white shrimp (Litopenaeus vannamei) fed oxidized fish oil: regulation by dietary myo-inositol. Fish Shellfish Immunol. 88, 53–64. doi: 10.1016/j.fsi.2019.02.023
Chen, X., Wang, Q., Guo, Z., Zhao, Y., Gao, Y., Yu, T., et al. (2019). Effects of dietary oxidized fish oil on growth performance and antioxidant defense mechanism of juvenile Rhynchocypris lagowski Dybowski. Aquaculture 512:734368. doi: 10.1016/j.aquaculture.2019.734368
Chen, Y. J., Liu, Y. J., Tian, L. X., Niu, J., Liang, G. Y., Yang, H. J., et al. (2013). Effect of dietary vitamin E and selenium supplementation on growth, body composition, and antioxidant defense mechanism in juvenile largemouth bass (Micropterus salmoides) fed oxidized fish oil. Fish Physiol. Biochem. 39, 593–604. doi: 10.1007/s10695-012-9722-1
Chen, Y.-J., Liu, Y.-J., Yang, H.-J., Yuan, Y., Liu, F.-J., Tian, L.-X., et al. (2012). Effect of dietary oxidized fish oil on growth performance, body composition, antioxidant defence mechanism and liver histology of juvenile largemouth bass Micropterus salmoides. Aquac. Nutr. 18, 321–331. doi: 10.1111/j.1365-2095.2011.00900.x
Dabrowski, K., El-Fiky, N., Köck, G., Frigg, M., and Wieser, W. (1990). Requirement and utilization of ascorbic acid and ascorbic sulfate in juvenile rainbow trout. Aquaculture 91, 317–337.
Dong, G., Zhu, X., Ren, H., Nie, B., Chen, L., Li, H., et al. (2014). Effects of oxidized fish oil intake on tissue lipid metabolism and fatty acid composition of channel catfish (Ictalurus punctatus). Aquac. Res. 45, 1867–1880. doi: 10.1111/are.12138
Dong, X. L., Lei, W., Zhu, X. M., Han, D., Yang, Y. X., and Xie, S. Q. (2011). Effects of dietary oxidized fish oil on growth performance and skin colour of Chinese longsnout catfish (Leiocassis longirostris Günther). Aquac. Nutr. 17, e861–e868. doi: 10.1111/j.1365-2095.2011.00854.x
Eder, K., and Kirchgessner, M. (1998). The effect of dietary vitamin E supply and a moderately oxidized oil on activities of hepatic lipogenic enzymes in rats. Lipids 33, 277–283. doi: 10.1007/s11745-998-0206-x
Eder, K., Flader, D., Hirche, F., and Brandsch, C. (2002). Excess dietary vitamin E lowers the activities of antixidative enzymes in erythrocytes of rats fed salmon oil. J. Nutr. 132, 3400–3404. doi: 10.1093/jn/132.11.3400
El Basuini, M. F., Shahin, S. A., Teiba, I. I., Zaki, M. A. A., El-Hais, A. M., Sewilam, H., et al. (2021). The influence of dietary coenzyme Q10 and vitamin C on the growth rate, immunity, oxidative-related genes, and the resistance against Streptococcus agalactiae of Nile tilapia (Oreochromis niloticus). Aquaculture 531:735862. doi: 10.1016/j.aquaculture.2020.735862
Ellis, A. E. (2001). Innate host defense mechanisms of fish against viruses and bacteria. Dev. Comp. Immunol. 25, 827–839. doi: 10.1016/S0145-305X(01)00038-6
Ellis, A. E., Stolen, J. S., Fletcher, T. C., Anderson, D. P., Roberson, W. B., and Van muiswinker, W. B. (1990). “Serum Antiproteases In Fish,”in Techniques in Fish Immunology, eds J.S. Stolen, T.C. Fletcher, D.P. Anderson, B.S. Roberson, and W.B. van Muiswinkel (Fair Haven, NJ: SOS Publications).
Fatima, M., Afzal, M., and Shah, S. Z. H. (2019). Effect of dietary oxidized oil and vitamin E on growth performance, lipid peroxidation and fatty acid profile of Labeo rohita fingerlings. Aquac. Nutr. 25, 281–291. doi: 10.1111/anu.12851
Fontagné, S., Bazin, D., Brèque, J., Vachot, C., Bernarde, C., Rouault, T., et al. (2006). Effects of dietary oxidized lipid and vitamin A on the early development and antioxidant status of Siberian sturgeon (Acipenser baeri) larvae. Aquaculture 257, 400–411. doi: 10.1016/j.aquaculture.2006.01.025
Fontagné-Dicharry, S., Larroquet, L., Dias, K., Cluzeaud, M., Heraud, C., and Corlay, D. (2018). Effects of dietary oxidized fish oil supplementation on oxidative stress and antioxidant defense system in juvenile rainbow trout (Oncorhynchus mykiss). Fish Shellfish Immunol. 74, 43–51. doi: 10.1016/j.fsi.2017.12.039
Fontagné-Dicharry, S., Lataillade, E., Surget, A., Larroquet, L., Cluzeaud, M., and Kaushik, S. (2014). Antioxidant defense system is altered by dietary oxidized lipid in first-feeding rainbow trout (Oncorhynchus mykiss). Aquaculture 42, 220–227. doi: 10.1016/j.aquaculture.2014.01.009
Gao, J., and Koshio, S. (2015). Effect of dietary lipid oxidation with vitamin C and E supplementation on fillet quality of red sea bream, Pagrus major (Temminck & Schlegel) during storage. Aquac. Res. 46, 2382–2391. doi: 10.1111/are.12395
Gao, J., Koshio, S., Ishikawa, M., Yokoyama, S., and Mamauag, R. E. P. (2014). Interactive effects of vitamin C and E supplementation on growth performance, fatty acid composition and reduction of oxidative stress in juvenile Japanese flounder Paralichthys olivaceus fed dietary oxidized fish oil. Aquaculture 42, 84–90. doi: 10.1016/j.aquaculture.2013.11.031
Gao, J., Koshio, S., Ishikawa, M., Yokoyama, S., Mamauag, R. E. P., and Han, Y. (2012). Effects of dietary oxidized fish oil with vitamin E supplementation on growth performance and reduction of lipid peroxidation in tissues and blood of red sea bream Pagrus major. Aquaculture 35, 73–79. doi: 10.1016/j.aquaculture.2012.05.034
Gao, J., Koshio, S., Ishikawa, M., Yokoyama, S., Nguyen, B. T., and Mamauag, R. E. (2013). Effect of dietary oxidized fish oil and vitamin C supplementation on growth performance and reduction of oxidative stress in Red Sea Bream Pagrus major. Aquac. Nutr. 19, 35–44. doi: 10.1111/j.1365-2095.2011.00921.x
Han, Y. Z., Ren, T. J., Jiang, Z. Q., Jiang, B. Q., Gao, J., Koshio, S., et al. (2012). Effects of palm oil blended with oxidized fish oil on growth performances, hematology, and several immune parameters in juvenile Japanese sea bass. Lateolabrax japonicas. Fish Physiol. Biochem. 38, 1785–1794. doi: 10.1007/s10695-012-9675-4
Huang, C. H., and Huang, S. L. (2004). Effect of dietary vitamin E on growth, tissue lipid peroxidation, and liver glutathione level of juvenile hybrid tilapia, Oreochromis niloticus × O. aureus, fed oxidized oil. Aquaculture 237, 381–389. doi: 10.1016/j.aquaculture.2004.04.002
Izquierdo, M., Watanabe, T., Takeuchi, T., Arakawa, T., and Kitajima, C. (1990). “Optimum EFA levels in Artemia to meet the EFA requirements of red seabream (Pagrus major),” in The Current Status of Fish Nutrition in Aquaculture, eds T. Takeda and M. Watanabe (Tokyo: Tokyo Univ. Fisheries), 221–223.
Janssens, B. J., Childress, J. J., Baguet, F., and Rees, J. F. (2000). Reduced enzymatic antioxidative defense in deep-sea fish. J. Exp. Biol. 203, 3717–3725. doi: 10.1242/jeb.203.24.3717
Jiang, J., Zheng, T., Zhou, X. Q., Liu, Y., and Feng, L. (2009). Influence of glutamine and vitamin E on growth and antioxidant capacity of fish enterocytes. Aquac. Nutr. 15, 409–414.
Kaewsrithong, J., Ohshima, T., Ushio, H., Nagasaka, R., Maita, M., and Sawada, M. (2001). Effects of an excess dose of dietary α-tocopherol on hydroperoxide accumulation and erythrocyte osmotic fragility of sweet smelt Plecoglossus altivelis (Temminck et Schlegel). Aquac. Res. 32, 191–198.
Kjær, M. A., Aursnes, I. A., Berge, G. M., Sørensen, M., Marchenko, Y., Gjøen, T., et al. (2014). The influence of different dietary oil qualities on growth rate, feed utilization and oxidative stress in Atlantic cod. Aquac. Nutr. 20, 192–204. doi: 10.1111/anu.12065
Kontush, A., Finckh, B., Karten, B., Kohlschütter, A., and Beisiegel, U. (1996). Antioxidant and prooxidant activity of α-tocopherol in human plasma and low density lipoprotein. J. Lipid Res. 37, 1436–1448. doi: 10.1016/s0022-2275(20)39128-8
Kubiriza, G. K., Árnason, J., Sigurgeirsson, Ó, Hamaguchi, P., Snorrason, S., Tómasson, T., et al. (2017). Dietary lipid oxidation tolerance of juvenile Arctic charr (Salvelinus alpinus) and Nile tilapia (Oreochromis niloticus). Aquaculture 467, 102–108. doi: 10.1016/j.aquaculture.2016.04.006
Lamboni, C., Sebedio, J. L., and Perkins, E. G. (1998). Cyclic fatty acid monomers from dietary heated fats affect rat liver enzyme activity. Lipids 33, 675–681. doi: 10.1007/s11745-998-0256-0
Lee, K. J., and Dabrowski, K. (2003). Interaction between vitamins C and E affects their tissue concentrations, growth, lipid oxidation, and deficiency symptoms in yellow perch (Perca flavescens). Br. J. Nutr. 89, 589–596. doi: 10.1079/BJN2003819
Lewis-McCrea, L. M., and Lall, S. P. (2007). Effects of moderately oxidized dietary lipid and the role of vitamin E on the development of skeletal abnormalities in juvenile Atlantic halibut (Hippoglossus hippoglossus). Aquaculture 262, 142–155. doi: 10.1016/j.aquaculture.2006.09.024
Liang, X. P., Li, Y., Hou, Y. M., Qiu, H., and Zhou, Q. C. (2017). Effect of dietary vitamin C on the growth performance, antioxidant ability and innate immunity of juvenile yellow catfish (Pelteobagrus fulvidraco Richardson). Aquac. Res. 48, 149–160. doi: 10.1111/are.12869
Lim, C., and Lovell, R. T. (1978). Pathology of the vitamin C deficiency syndrome in channel catfish (Ictalurus punctatus). J. Nutr. 108, 1137–1146. doi: 10.1093/jn/108.7.1137
Liu, D., Chi, S., Tan, B., Dong, X., Yang, Q., Liu, H., et al. (2019). Effects of fish oil with difference oxidation degree on growth performance and expression abundance of antioxidant and fat metabolism genes in orange spotted grouper, Epinephelus coioides. Aquac. Res. 50, 188–197. doi: 10.1111/are.13883
Magnadóttir, B., Jonsdottir, H., Helgason, S., Bjornsson, B., Jørgensen, T., and Pilströ, L. (1999). Humoral immune parameters in Atlantic cod Gadus morhua L. I: the effects of environmental temperature. Comp. Biochem. Physiol. 122, 173–180.
Marmesat, S., Morales, A., Velasco, J., Ruiz-Mendez, M. V., and Dobarganes, M. C. (2009). Relationship between changes in peroxide value and conjugated dienes during oxidation of sunflower oils with different degree of unsaturation. Grasas Y Aceites 60, 155–160. doi: 10.3989/gya.096908
McDowell, L. R. (1989). “Vitamin E,” in Vitamins in Animal Nutrition: Comparative Aspects to Human Nutrition, ed. L. McDowell (London: Academic Press), 93–131.
Mourente, G., Diaz-Salvago, E., Tocher, D. R., and Bell, J. G. (2000). Effects of dietary polyunsaturated fatty acid/vitamin E (PUFA/tocopherol ratio on antioxidant defence mechanisms of juvenile gilthead sea bream (Sparus aurata L. Osteichthyes, Sparidae). FISH Physiol. Biochem. 23, 337–351. doi: 10.1023/A:1011128510062
Niki, E. J., Tsuchiya, R., and Tanimura, Y. K. (1982). Regeneration of vitamin E from alpha-chromanoxyl radical by glutathione and vitamin C. Chem. Lett 11, 789–792.
Obach, A., Quentel, C., and Laurencin, F. (1993). Effects of alphatocopherol and dietary oxidized fish oil on the immune response of sea bass Dicentrarchus labrax. Dis. Aquat. Organ 15, 175–185.
Owu, D. U., Osim, E. E., and Ebong, P. E. (1998). Serum liver enzymes profile of Wistar rats following chronic consumption of fresh or oxidized palm oil diets. Acta Trop. 69, 65–73. doi: 10.1016/S0001-706X(97)00115-0
Pearson, P., Lewis, S. A., Britton, J., Young, I. S., and Fogarty, A. (2006). The pro-oxidant activity of high-dose vitamin E supplements in vivo. Biodrugs 20, 271–273. doi: 10.2165/00063030-200620050-00002
Peng, S., Chen, L., Qin, J. G., Hou, J., Yu, N., Long, Z., et al. (2009). Effects of dietary vitamin E supplementation on growth performance, lipid peroxidation and tissue fatty acid composition of black sea bream (Acanthopagrus schlegeli) fed oxidized fish oil. Aquac. Nutr. 15, 329–337. doi: 10.1111/j.1365-2095.2009.00657.x
Poljsak, B., and Ionescu, J. G. (2010). “Pro-oxidant vs. antioxidant effects of vitamin C,” in Handbook of Vitamin C Research: Daily Requirements, Dietary Sources and Adverse Effects, eds H. Kucharski and J. Zajac (New York, NY: Nova Science Publishers, Inc).
Priyadarsani, L., Abraham, T. J., Adikesavalu, H., Dash, G., and Nagesh, T. S. (2021). Effects of dietary supplementation of vitamin-E and commercial probiotics on the innate immunity of Labeo rohita against Aeromonas hydrophila infection. Fish Shellfish Immunol. Rep. 2:100013. doi: 10.1016/j.fsirep.2021.100013
Quade, M. J., and Roth, J. (1997). A rapid, direct assay to measure degranulation of bovine neutrophil primary granules. Vet. Immunol. Immunopathol. 58, 239–248.
Rahimnejad, S., and Lee, K. J. (2014). Dietary arginine requirement of juvenile red sea bream Pagrus major. Aquaculture 434, 418–424. doi: 10.1016/j.aquaculture.2014.09.003
Rahimnejad, S., Lu, K., Wang, L., Song, K., Mai, K., Davis, D. A., et al. (2019). Replacement of fish meal with Bacillus pumillus SE5 and Pseudozyma aphidis ZR1 fermented soybean meal in diets for Japanese seabass (Lateolabrax japonicus). Fish Shellfish Immunol. 84, 987–997. doi: 10.1016/j.fsi.2018.11.009
Ren, T., Koshio, S., Ishikawa, M., Yokoyama, S., Micheal, F. R., Uyan, O., et al. (2007). Influence of dietary vitamin C and bovine lactoferrin on blood chemistry and non-specific immune responses of Japanese eel, Anguilla japonica. Aquaculture 267, 31–37.
Sakakura, Y., Koshio, S., Iida, Y., Tsukamoto, K., Kida, T., and Blom, J. H. (1998). Dietary vitamin C improves the quality of yellowtail (Seriola quinqueradiata) seedlings. Aquaculture 161, 427–436.
Saraiva, A., Costa, J., Eiras, J. C., and Cruz, C. (2016). Histological study as indicator of juveniles farmed turbot. Scophthalmus maximus L. health status. Aquaculture 459, 210–215. doi: 10.1016/j.aquaculture.2016.03.048
Sargent, J., Bell, G., McEvoy, L., Tocher, D., and Estevez, A. (1999). Recent developments in the essential fatty acid nutrition of fish. Aquaculture 177, 191–199. doi: 10.1016/S0044-8486(99)00083-6
Shin, C. H., Bui, H. T. D., Rahimnejad, S., Cha, J. H., Yoo, B. W., Lee, B. K., et al. (2014). Effects of dietary supplementation of barodon on growth performance, innate immunity and disease resistance of juvenile olive flounder, Paralichthys olivaceus, against Streptococcus iniae. J. World Aquac. Soc. 45, 258–268. doi: 10.1111/jwas.12120
Song, X., Yang, C., Tang, J., Yang, H., Xu, X., Wu, K., et al. (2019). A combination of vitamins C and E alleviates oxidized fish oil-induced hepatopancreatic injury in juvenile Chinese mitten crab Eriocheir sinensis. Aquac. Res. 50, 1585–1598. doi: 10.1111/are.14036
Sutton, J., Balfry, S., Higgs, D., Huang, C.-H., and Skura, B. (2006). Impact of iron-catalyzed dietary lipid peroxidation on growth performance, general health and flesh proximate and fatty acid composition of Atlantic salmon (Salmo salar L.) reared in seawater. Aquaculture 257, 534–557. doi: 10.1016/j.aquaculture.2006.03.013
Swain, P., Dash, S., Sahoo, P. K., Routray, P., Sahoo, S. K., Gupta, S. D., et al. (2007). Non-specific immune parameters of brood Indian major carp Labeo rohita and their seasonal variations. Fish Shellfish Immunol. 22, 38–43. doi: 10.1016/j.fsi.2006.03.010
Takashima, F., and Hibiya, T. (1995). An Atlas Of Fish Histology: Normal And Pathological Features. Kodansha, Tokyo. (Stuttgart: Gustav Fischer), 74–90.
Tocher, D. R., Mourente, G., der Eecken, A., Evjemo, J. O., Diaz, E., Bell, J. G., et al. (2002). Effects of dietary vitamin E on antioxidant defence mechanisms of juvenile turbot (Scophthalmus maximus L.), halibut (Hippoglossus hippoglossus L.) and sea bream (Sparus aurata L.). Aquac. Nutr. 8, 195–207. doi: 10.1046/j.1365-2095.2002.00205.x
Tocher, D. R., Mourente, G., Van Der Eecken, A., Evjemo, J. O., Diaz, E., Wille, M., et al. (2003). Comparative study of antioxidant defence mechanisms in marine fish fed variable levels of oxidised oil and vitamin E. Aquac. Int. 11, 195–216. doi: 10.1023/A:1024127003997
Tongjun, R., Koshio, S., Uyan, O., Komilus, C. F., Yokoyama, S., Ishikawa, M., et al. (2008). Effects of dietary vitamin C on blood chemistry and non-specific immune response of juvenile red sea bream, Pagrus major. J. World Aquac. Soc. 39, 797–803. doi: 10.1111/j.1749-7345.2008.00216.x
Wang, L. G., Li, E. C., Qin, J. G., Du, Z. Y., Yu, N., Kong, Y. Q., et al. (2015). Effect of oxidized fish oil and α-tocopherol on growth, antioxidation status, serum immune enzyme activity and resistance to Aeromonas hydrophila challenge of Chinese mitten crab Eriocheir sinensis. Aquac. Nutr. 21, 414–424. doi: 10.1111/anu.12171
Winston, G. W., and Digiulio, R. T. (1991). Prooxidant and antioxidant mechanisms in aquatic organisms. Aquat. Toxicol. 19, 137–161. doi: 10.1016/0166-445X(91)90033-6
Xie, S., Yin, P., Tian, L., Liu, Y., and Niu, J. (2020). Lipid metabolism and plasma metabolomics of juvenile largemouth bass Micropterus salmoides were affected by dietary oxidized fish oil. Aquaculture 522:735158. doi: 10.1016/j.aquaculture.2020.735158
Yamamoto, F. Y., Castillo, S., de Cruz, C. R., Chen, K., Hume, M. E., and Gatlin, D. M. (2020). Synergistic effects of the β-1,3 glucan paramylon and vitamin C on immunological responses of hybrid striped bass (Morone chrysops × M. saxatilis) were pronounced in vitro but more moderate in vivo. Aquaculture 526:735394. doi: 10.1016/j.aquaculture.2020.735394
Yin, P., Xie, S., Huo, Y., Guo, T., Fang, H., Zhang, Y., et al. (2019). Effects of dietary oxidized fish oil on growth performance, antioxidant defense system, apoptosis and mitochondrial function of juvenile largemouth bass (Micropterus salmoides). Aquaculture 500, 347–358. doi: 10.1016/j.aquaculture.2018.09.009
Yuan, Y., Chen, Y. J., Liu, Y. J., Yang, H. J., Liang, G. Y., and Tian, L. X. (2014). Dietary high level of vitamin premix can eliminate oxidized fish oil-induced oxidative damage and loss of reducing capacity in juvenile largemouth bass (Micropterus salmoides). Aquac. Nutr. 20, 109–117. doi: 10.1111/anu.12057
Zhang, C. X., Huang, F., Li, J., Wang, L., Song, K., and Mai, K. S. (2016). Interactive effects of dietary magnesium and vitamin E on growth performance, body composition, blood parameters and antioxidant status in Japanese seabass (Lateolabrax japonicus) fed oxidized oil. Aquac. Nutr. 22, 708–722. doi: 10.1111/anu.12393
Keywords: rainbow trout, oxidized fish oil, vitamins supplementation, innate immunity, antioxidant capacity, blood biochemistry, fatty acid composition
Citation: Rahimnejad S, Dabrowski K, Izquierdo M, Hematyar N, Imentai A, Steinbach C and Policar T (2021) Effects of Vitamin C and E Supplementation on Growth, Fatty Acid Composition, Innate Immunity, and Antioxidant Capacity of Rainbow Trout (Oncorhynchus mykiss) Fed Oxidized Fish Oil. Front. Mar. Sci. 8:760587. doi: 10.3389/fmars.2021.760587
Received: 18 August 2021; Accepted: 20 September 2021;
Published: 12 October 2021.
Edited by:
Liping Liu, Shanghai Ocean University, ChinaReviewed by:
Songlin Li, Shanghai Ocean University, ChinaFrancisco Javier Moyano, University of Almería, Spain
Copyright © 2021 Rahimnejad, Dabrowski, Izquierdo, Hematyar, Imentai, Steinbach and Policar. This is an open-access article distributed under the terms of the Creative Commons Attribution License (CC BY). The use, distribution or reproduction in other forums is permitted, provided the original author(s) and the copyright owner(s) are credited and that the original publication in this journal is cited, in accordance with accepted academic practice. No use, distribution or reproduction is permitted which does not comply with these terms.
*Correspondence: Samad Rahimnejad, c3JhaGltbmVqYWRAZnJvdi5qY3UuY3o=