- 1Museo Argentino de Ciencias Naturales “Bernardino Rivadavia” (MACN-CONICET), Buenos Aires, Argentina
- 2School of Geography and the Environment, University of Oxford, Oxford, United Kingdom
- 3Grupo de Investigación y Educación en Temas Ambientales, Estación Biológica las Brusquitas, San Eduardo del Mar, Argentina
- 4Facultad de Ciencias Exactas y Naturales, Instituto de Geología de Costas y del Cuaternario (IGCyC, UNMdP/CIC), Instituto de Investigaciones Marinas y Costeras (IIMyC, UNMdP/CONICET), Universidad Nacional de Mar del Plata, Mar del Plata, Argentina
The organisms inhabiting intertidal platforms can affect their weathering and erosion rates. Research on biotic influences on platform integrity has traditionally emphasized the role of bioeroders (i.e., organisms that scrap or bore into platforms via mechanical and chemical means). Yet, recent studies illustrate that covers of sessile organisms on the surfaces of intertidal platforms can have bioprotective effects by reducing the efficacy of physical weathering and erosion agents. Eroding cliffs fronted by cohesive shore platforms are a pervasive feature along the continental Argentinean coastline (37–52°S). In this study, we investigated how mussel (Brachidontes rodriguezii) cover mediates weathering and erosion of a cohesive, consolidated silt platform at Playa Copacabana (5 km north of Miramar, Buenos Aires Province; 38° 14′ S, 57° 46′ W). By means of mussel removal experiments, we found that mussel cover attenuates variations in platform surface temperatures, enhances moisture retention during low tide, reduces rates of salt crystallization within the pores of the platform material, and attenuates hydrodynamic forcing on the platform surface. Mussel removal also led to a 10% decrease in surface hardness and a 2-mm reduction in platform height after 5 months. Collectively, our findings indicate that mussel beds limit substrate breakdown via heating-cooling, wetting-drying, and salt crystallization and provide some of the first experimental field evidence for the direct impacts of biotic cover on platform erosion. As intertidal platforms protect the cliffs behind from the hydraulic impact of waves, which may be enhanced with future sea-level rise, we posit that the protection of platforms by mussels indirectly moderates coastline retreat, especially on soft cohesive shores.
Introduction
Shore platforms are horizontal or gently sloping rock surfaces usually backed by cliffs that are widespread throughout the world’s intertidal zones (Trenhaile, 1987; Sunamura, 1992). They have traditionally been viewed as being shaped by waves (e.g., hydraulic action as well as abrasion by mobile clasts), subaerial weathering (i.e., in situ breakdown of rock driven by climatic variations and cycles of tidal exposure), or some combination of the two (Trenhaile, 2002; Mottershead, 2013; Coombes, 2014; Moses, 2014). In comparison, biotic influences on shore platform dynamics remain relatively understudied, especially given the diversity of taxa and biogeomorphic mechanisms that affect them (see reviews in Naylor et al., 2012; Coombes, 2014). Indeed, research on shore platform biogeomorphology has placed focus on the role of bioeroders (i.e., organisms that scrape consolidated substrates or bore into them via mechanical and chemical means; see Healy, 1968; Trudgill, 1987; Spencer, 1988; Pinn et al., 2005; Bagur et al., 2014; Coombes, 2014; Dodge-Wan and Nagarajan, 2020), whereas the potential “bioprotective” roles (sensu Carter and Viles, 2005) of sessile organisms that cover the surfaces of shore platforms have been considered only relatively recently (e.g., Coombes et al., 2013, 2017; La Marca et al., 2014; Gowell et al., 2015; Naylor and Coombes, 2015).
The evidence available to date indicates that covers of sessile organisms, such as seaweeds or barnacles, can limit the impacts of physical weathering agents on shore platforms via a range of mechanisms. This includes moisture retention and buffering effects on temperature variations, which are thought to reduce the efficacy of substrate weathering via heating-cooling, wetting-drying, and salt crystallization (Stephenson and Kirk, 2000; Moura et al., 2012; Gowell et al., 2015). Through these mechanisms and their potential scale linkages that are thought to mediate wave-driven erosion (e.g., Naylor et al., 2012), bioprotection may reduce overall rates of platform lowering and retreat, particularly on shores and at tidal heights where biological covers are well developed. There is, however, no manipulative experimental field evidence for the direct impacts of biotic cover on platform weathering and erosion (but see Moura et al., 2012 for a comparative study). Evaluating such effects is especially relevant on relatively soft cohesive shore platforms (i.e., those formed by poorly consolidated sediments with a high silt and clay content), where erosion can proceed quickly relative to the time taken for the recovery of some sessile organisms (e.g., 5 years for mussels and erect macroalgae; Micheli et al., 2016) following mortality and/or dislodgement (Charman et al., 2007; Moses, 2014). Improving our understanding of biogeomorphological processes is also crucial to inform predictions of coastline responses to climate change, including shifts in the spatial arrangement of organisms and physical processes as a result of sea-level rise.
Eroding cliffs fronted by cohesive shore platforms are a pervasive feature along the Argentinean coastline (37–52°S). At mid intertidal elevations, the cohesive platforms are dominated by bed-forming mussels (either Brachidontes rodriguezii, Perumytilus purpuratus, or both; see Arribas et al., 2013; Trovant et al., 2015). Mussels are known to physically modify environments (via physical ecosystem engineering; sensu Jones et al., 1994) in ways that affect the abiotic conditions and resources available to other organisms (Gutiérrez et al., 2003, 2011). For instance, flow speeds, sediment transport, and temperature and moisture fluctuations are reduced in the interstices of mussel beds relative to adjacent bare rock (e.g., Carrington et al., 2008; Silliman et al., 2011; Bagur et al., 2016; Gutiérrez et al., 2019). While the ecological effects of physical ecosystem engineering by mussels are well understood (e.g., Borthagaray and Carranza, 2007; Silliman et al., 2011; Bagur et al., 2016; Gutiérrez et al., 2019), their potential to reduce weathering and erosion of the underlying rock remains largely unknown (see Baxter, 2015). Yet, mussel cover may protect the underlying rock from the mechanical impacts of waves and sediments as well as buffer variations in temperature and moisture, thus reducing the efficacy of weathering processes linked to rock expansion-contraction cycles and the crystallization of salts within rock pores.
Examining the effects of mussel cover on the weathering and erosion of cohesive shore platforms is particularly important in view of multiple, ongoing sources of mussel cover loss, including human harvesting and trampling as well as increased dislodgement/mortality due to the increased frequency, magnitude, and duration of storms and heatwaves (e.g., Tsuchiya, 1983; Erlandsson et al., 2006, 2011; Micheli et al., 2016; Mendez et al., 2017; Seuront et al., 2019). Here, we investigated mussel cover impacts on the weathering and erosion of intertidal cohesive platforms using removal experiments on the Argentinean coastline. Specifically, we evaluated the effects of mussel cover on water flow and abrasion, oscillations in rock temperature and moisture, and the accumulation of salts within the platform material. We predicted that (a) the hydraulic and abrasive impacts of sand-laden waves and currents, as well as the potential for thermal, wetting-drying, and salt-related weathering, would be higher on bare relative to the mussel-covered substrate, and (b) enhanced weathering and erosion following the removal of mussels would lead to substrate softening and platform lowering.
Materials and Methods
Study Site
This study was carried out on intertidal cohesive platforms at Copacabana (38° 14′ S, 57° 46′ W), 5 km northeast of Miramar, Buenos Aires Province, Argentina (Figure 1A). These platforms are located at the base of an 8–12 m high cohesive cliff (known as Barranca Parodi in the stratigraphic literature; Figure 1B) developed in the Chapadmalal Formation (3.2–4.5 million years BP), which is primarily composed of reddish-brown, sandy siltstones originating from reworked loess deposits and sparse indurated caliche levels and nodules (Zárate and Fasano, 1989; Isla et al., 2015; Rico et al., 2020). The shore in this area faces southeast, which is the direction of the strongest swells (Fiore et al., 2009), and can be classified as ‘‘exposed’’ according to the MarLin wave exposure categories (i.e., an open coast facing away from prevailing winds but with a long fetch, and where strong winds are frequent)1. Current velocities on the surface of the study platforms can be as high as 13 m s–1 (Gutiérrez et al., 2018). The tidal regime is semidiurnal and microtidal (0.80 m mean amplitude; Servicio de Hidrografía Naval, Argentina)2. Current coastal retreat rates in this area average 0.8 m yr–1 (Isla et al., 2018).
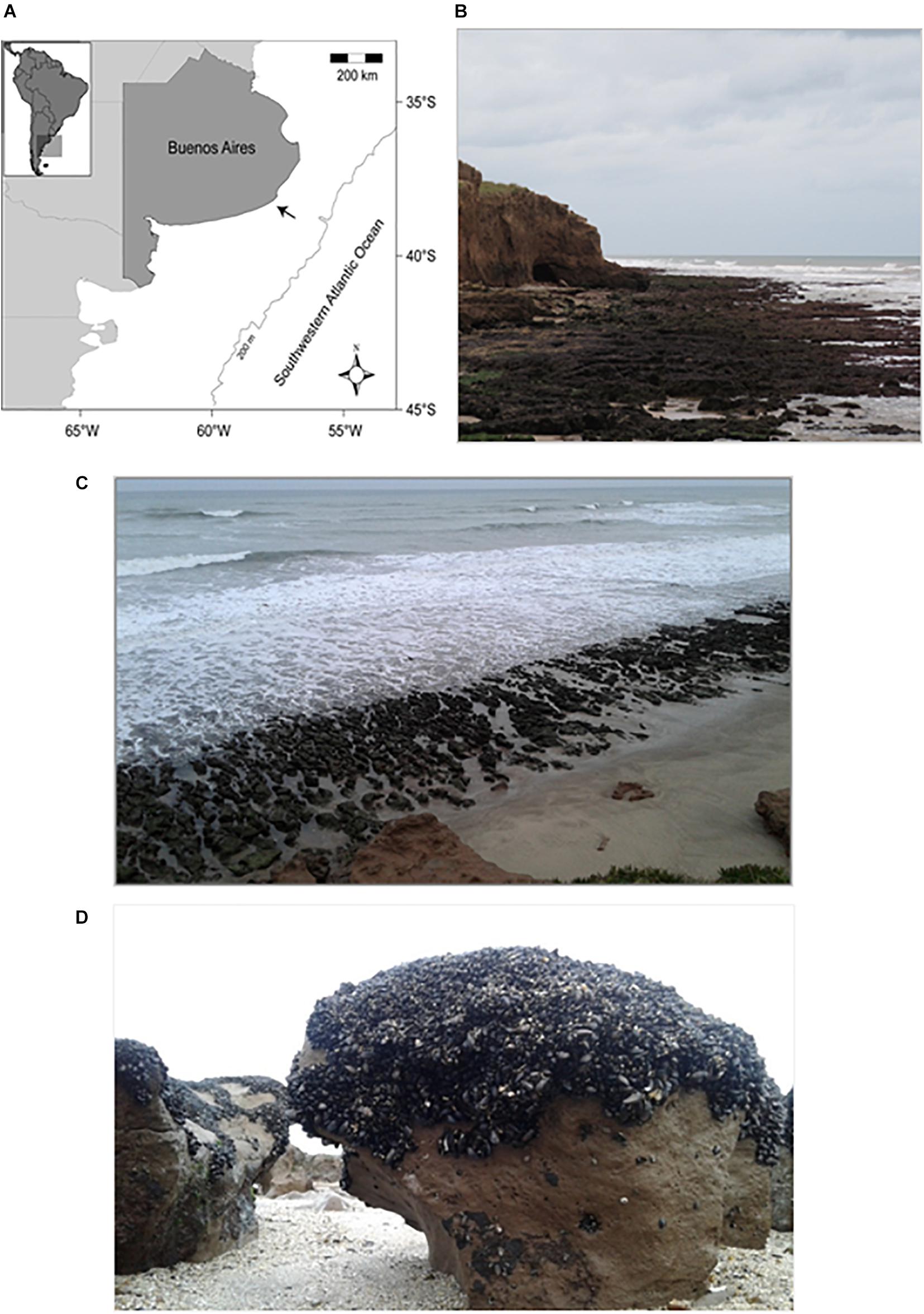
Figure 1. (A) Location of the study site on the coast of Buenos Aires province, Argentina (indicated by an arrow). (B) Panoramic view of a studied platform and its backing cohesive cliff (8–12 m high) during a very low tide. (C) Clifftop view of the study site at low tide. The platform is incised by rills and fragmented into benches of varying sizes (typically <4 m2). (D) Close-up view of a bench showing mussel cover on the top surface and the upper portion of the sides. Note the sand and gravel accumulation at the bottom of the rill. The approximate height of this bench above the sand/gravel level is 50 cm.
As is common for cohesive and soft rock platforms (e.g., Ledesma-Vázquez and Johnson, 1994; Gómez-Pujol et al., 2014; Moses, 2014), the studied platforms are cut by interconnected rills that run mostly perpendicular to the shoreline and are, thus, fragmented into elongated benches of varying size from a few square decimeters to a few square meters (see Figure 1C). The relatively small mussel, Brachidontes rodriguezii (up to 55 mm in length, most individuals <30 mm length), forms dense and primarily single-layered beds (200–800 ind. dm–2; Arribas et al., 2015; Gutiérrez et al., 2015) along the top and along the upper sides of the benches located within the mid intertidal zone (Figure 1D). The height of these mid intertidal benches varies between 50 and 80 cm, though their top surfaces are relatively level with each other (i.e., height differences are mostly associated with variations in rill depth). Their lower sides and the bottoms of rills are periodically buried and abraded by sand and gravel (both sourced from nearby beaches; see Lamarchina et al., 2021) and, thus, lack biotic cover (Figure 1D). Abrasion and undercutting of the benches is likely a significant mechanism of overall platform erosion independently of the biotic platform-lowering effects investigated here.
Field Experiment
Sixteen benches (0.4–0.8 m–2 top surface area) were selected on June 29, 2018, and mussels were removed from half while the remaining benches were left as mussel-covered controls. The mussel layer was pulled by hand or with the aid of a putty knife when strongly attached, but taking care not to scrape the bench surface. Treatments were assigned systematically to ensure adequate interspersion. Platform height, substrate hardness, flow/abrasion rates, near-surface temperatures, substrate moisture, and subsurface salt concentrations were subsequently measured over the course of the experiment (see section “Measurements of Response Variables”). The experiment was ended on November 21, 2018, following the incipient recruitment of barnacles, Balanus glandula, to the mussel-removal benches. While the size (<1 mm) and surface area covered by these barnacles (<5%) were small so as not to interfere with our measurements and sampling around this date, we predicted that barnacle coverage would become significant in the following weeks due to continuing recruitment and individual growth. This was confirmed in late December when the barnacle cover reached 20–30%.
Measurements of Response Variables
Platform Height
Changes in the height of the mussel-removal and control benches were evaluated monthly for approximately 5 months until November 20, 2018. Variations in bench height were assessed as changes in surface level relative to benchmarks established within the platform. For this, we used a cost-effective, modified version of the micro-erosion meter (Stephenson and Finlayson, 2009), which we tailored to the specific needs of this research. Four nails (1.5 inches long) were driven into the substrate at each corner of a 15-cm2 quadrat normal to the top of each bench, leaving ca. 1.5-cm section protruding from the surface (see Supplementary Figure 1A). At the time of each measurement, a square, removable PVC plate (sides = 15.5 cm) was mounted on top of the four nails (Supplementary Figure 1B) and the height of the substrate relative to the PVC plate (i.e., the benchmark level) was measured by inserting the depth measuring probe of a Vernier caliper through a tightly fitting hole made in the center of the plate (Supplementary Figure 1C). The PVC plate was removed after measurement so that only the nails remained in the field throughout the study period. To ensure that the PVC plate was mounted in the same position each time and relative height data were obtained at the same points on the bench surfaces, circular notches were made in each corner of the plate into which the nail heads could be fitted. The nail heads themselves could not be used as benchmarks (i.e., as erosion pins; e.g., Turowski and Cook, 2017) as surface cracking and pitting occurred within a ca. 0.5-cm radius around the nails. It was also not possible to use screw bolts instead of nails as the substrate easily disaggregated when attempting to secure them.
Substrate Hardness
The surface hardness of the benches was measured immediately after the final height measurements (on November 21, 2018) as this involved destructive sampling. Specifically, a substrate chip (∼300–400 cm3) was extracted from each experimental bench with a hammer and chisel. To minimize any initial differences in moisture content, which may have affected the hardness measurements, the samples were sun-dried outdoors for a total of 10 h over the two subsequent days (in dry, sunny conditions) and stored indoors overnight to avoid moisture uptake from dew and mist. The samples were not oven-dried to constant weight as they readily disaggregate, which would prevent the subsequent hardness measurements.
A Shore A durometer was subsequently used to measure the hardness of the upper (i.e., exposed) face of the dry samples. The Shore A durometer is designed to measure resistance to indentation in flexible-to-hard rubber and semi-rigid plastics but has also been used to measure the surface hardness and, indirectly, cohesion of other low-strength consolidated materials including cement-stabilized clay, plasters, and lime-based mortars (e.g., Wang et al., 2015; Santos et al., 2018, 2020). The A-type durometer has a small pin at the end (a 35° truncated cone with a 0.79-mm diameter tip and a 1.40-mm base) that indents the test material under a fixed-force spring. Hardness values are measured in “Shore A Units” (ranging from 0 to 1 indicating low and high indentation strength, respectively), read from a digital LCD. Although several alternative methods for measuring surface hardness in situ and non-destructively are increasingly applied in geomorphological research, including on rock platforms (e.g., Knight and Burningham, 2020), we used destructive sampling and the durometer both for simplicity and to control for the potential impact of moisture content on substrate hardness and surface roughness, which are known to influence other measurement devices (e.g., Viles et al., 2011; Wilhelm et al., 2016; Desarnaud et al., 2019).
Flow/Abrasion Rates
The effects of mussel cover on water flow and abrasion at the surface of the benches were evaluated on August 27–29, 2018. For this, oven-dried (48 h at 60°C) and pre-weighed plaster discs (5 cm in diameter and 1.5 cm in height, approximating the thickness of the mussel bed) were nailed to the mussel-removal and control benches (one disc per experimental bench, n = 8; Supplementary Figure 2). These discs were prepared using three parts Plaster of Paris and four parts water and were molded using discoidal casts. The discs were installed at least 40 cm from the points where the height measurements were made to minimize any possible interference. On the mussel-covered benches, the discs were attached after a small number of mussels were removed, so that they could be secured directly onto the substrate with their sides shielded by the surrounding mussels (see Supplementary Figure 2). After 48 h of exposure (i.e., four semidiurnal tides), the discs were removed, taken to the laboratory, oven-dried, and re-weighed. Plaster weight loss was used as a surrogate measure of relative flow rates/turbulence and abrasion around and within the mussel beds and cleared areas (e.g., Guichard and Bourget, 1998; Baxter, 2015).
Substrate Surface Temperature
Waterproof temperature loggers (Hobo Pendant MX2201) were installed on the mussel-removal and control benches (two benches per treatment, with a single logger per bench). As these loggers are small and flat (3 cm in diameter and 2 cm in thickness), they allowed us to measure air temperature at the surface of the substrate, and between the rock and the overlying mussel bed. Holes were drilled in the substrate into which the loggers fitted, so that their tops were flush with the surface (i.e., the holes were the same width and depth as the loggers). The loggers were then nailed in place, within their mounting hole. For the mussel-covered control surfaces, a square piece of the mussel layer (sides = 15 cm) was first carefully removed with the aid of a putty knife (Supplementary Figure 3A). A hole was then made into which a logger was fitted and fastened (Supplementary Figures 3B,C), and the square piece of mussel bed was then replaced in its original position (Supplementary Figure 3D). To our knowledge, this is the first time that temperature data have been obtained on a shore platform beneath an existing cover of sessile invertebrates, similar to previous studies on seaweed canopies (Coombes et al., 2013).
Data were recorded on an exceptionally hot late-spring day on November 21, 2018, when the sky was clear, the platform was air-exposed at noon (low tide slack occurred at 11:50 PM), and temperatures reached 31°C as recorded by a nearby weather station (Mar del Plata Airport, 37° 55′ S, 57° 34′ W, ca. 40 km from the study site). On this day, temperature data were obtained during the low-tide air exposure period between 11:10 AM and 2:05 PM, when the platform began to be flooded again by the rising tide. Therefore, the data obtained reflect the influence of mussel cover on rock-surface temperatures on a typical hot summer’s day in this area (mean maximum daily temperature in January = 25°C; Giampietri and Piccolo, 2000).
Substrate Moisture Content
Two rock samples per experimental bench were obtained on November 21 in addition to those used for the hardness analysis (see section “Substrate Hardness”) using a 2-cm diameter steel core driven to a depth of 1.5 cm. The day was clear and hot, and the platform was air-exposed at noon; the samples were taken between 1:00 PM and 2:00 PM, more than two and a half hours after the platform was first exposed to the air. All of the samples were sliced into three 0.5-cm depth sections with the aid of a putty knife. One sample per bench (i.e., one set of three 0.5-cm sections) was analyzed for salt content (see section “Subsurface Salt Content”), and moisture content was determined using the remaining samples, calculated as the difference between their wet and dry weights. All wet-weight measurements were made in the field immediately after the samples were collected using a portable scale (precision = 0.001 g). Their dry weights were subsequently determined in the laboratory after oven-drying (60°C) to constant weight.
Subsurface Salt Content
The dried sample sections from each bench (at three depths of 0–0.5, 0.5–1.0, and 1.0–1.5 cm) were ground to dust with a glass mortar and pestle and subsequently analyzed for chloride and sulfate ions using ion chromatography (Dionex IC DX500; see Coombes et al., 2017).
Data Analyses
Repeated measures ANOVA (Neter et al., 1990) was used to evaluate the effect of Treatment (i.e., mussel removal; fixed factor) and Time (i.e., sampling date; random repeated measures variable) on platform height loss. Beta regression (i.e., a technique to model continuous random variables that assume values in the 0–1 interval; see Cribari-Neto and Zeileis, 2010) was used to evaluate the effect of Treatment on flow/abrasion rates (proportional weight losses from the plaster discs) and substrate hardness (Shore A units ranging between 0 and 1), as well as the effect of Treatment and Depth (fixed variable) on substrate moisture (proportional weight loss after oven drying) and salt content (salt weight/sample weight). All analyses were run in R (R Core Team, 2020) using the “lme4” (Bates et al., 2015) and “betareg” (Cribari-Neto and Zeileis, 2010) packages for the repeated measures ANOVA and beta regression, respectively.
Results and Discussion
Our findings illustrate that both weathering regimes and wave-driven processes can be attenuated by mussel cover on intertidal cohesive shore platforms. Specifically, we found that (a) mussel cover buffers multiple subaerial weathering agents, i.e., temperature and moisture variations, and salt crystallization, and (b) mussel cover modulates the hydrodynamic/abrasive forces acting on the platform surface that contribute to platform erosion via their own mechanical impacts and/or by removing previously weathered material. We have also observed that (c) mussel removal from these platforms is followed by decreases in surface hardness and platform height. In the following sections, we discuss these mechanisms in detail and evaluate their roles in the context of local and temporal factors that may mediate their occurrence and efficacy both on our study platform and elsewhere (e.g., substrate type, wave climate, and seasonal influences on temperature and desiccation).
Effects of Mussels on Weathering Regimes
Mussel cover efficiently buffered high temperatures during air exposure; near-surface temperatures on the mussel-covered benches during a period of exposure on a hot spring day (11:10 AM to 2:05 PM) were lower than on the cleared bench surfaces (Figure 2). This is in line with previous studies showing the dampening effects of algae or sessile invertebrate covers on the temperature of intertidal consolidated substrates during daytime air exposure (Coombes et al., 2013, 2017; Baxter, 2015; Gowell et al., 2015; Scrosati and Ellrich, 2018). The heat-attenuating effects of mussels were striking, with reductions ranging between 7 and 12°C (Figure 2). This is attributed to substrate shading and, possibly, to increased evaporative cooling due to the water retained in the interstitial spaces of the mussel beds as well as the mussel bodies themselves (see Helmuth, 1998). Evaporative cooling mechanisms have also been proposed to explain lower substrate temperatures in the presence of seaweeds and barnacles (Gowell et al., 2015; Coombes et al., 2017).
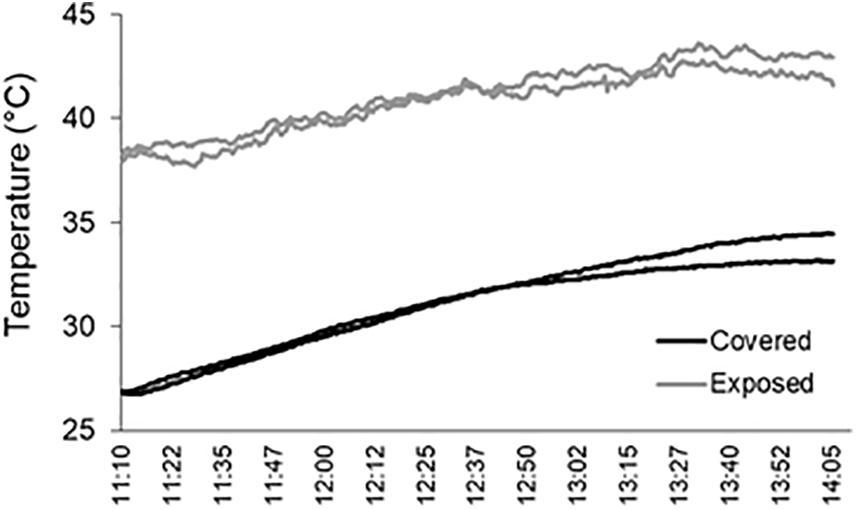
Figure 2. Air temperatures at the surface of mussel-covered and mussel-removal (exposed) benches during a 3-h air-exposure period at noon on an exceptionally hot late-spring day (November 21, 2018). Each curve plots the data registered every 15 s by temperature loggers installed in two mussel-covered and two exposed benches (one logger per bench). The loggers attached to the mussel-covered benches were placed underneath the mussel layer.
As well as general reductions in temperature, mussel cover was found to reduce short-term, seconds-to-minute fluctuations in temperatures during air exposure, indicated by the more jagged temperature curves for the mussel-removed surfaces in Figure 2. By modulating these rapid temperature fluctuations (attributable to passing cloud and wind gusts) as well as the amplitude of daily thermal cycles (associated with tidal regimes and day–night cycles, e.g., Coombes, 2011; Pappalardo and D’Olivo, 2019), mussels are expected to reduce the frequency, magnitude, and rate of substrate expansion/contraction. While not likely sufficient to initiate breakdown via thermal “shock” effects (see Hall and Thorn, 2014), the buffering of such repeated, low-magnitude thermal changes is thought to limit the potential for thermal “fatigue” in a range of environments including on rocky coasts (Coombes and Naylor, 2012; Coombes et al., 2013; Hall and Thorn, 2014; Molaro et al., 2020). From the data in Figure 2, it can also be inferred that sudden temperature decreases occur when air-exposed substrates heated by the sun are rapidly cooled by the incoming tidal waters (the typical water temperature in this area and time of the year is 16°C) and that mussel layers can also modulate these changes. It remains to be evaluated whether buffering of such higher-magnitude and rapid thermal changes by mussels and other forms of biota alters the potential for thermal shock-related breakdown on coastal platforms (Coombes, 2011, 2014).
The dampening effects of mussel cover on high near-substrate temperatures were associated with concurrent decreases in substrate desiccation and salt crystallization relative to cleared areas. The moisture content of the mussel-covered substrates was significantly higher than that of the cleared substrates after a few hours of exposure to hot air temperatures at low tide, and this difference was consistent across the three 5-mm depth ranges of the substrate samples (Table 1 and Figure 3). This indicates that mussel covers slow substrate desiccation rates and reduce net substrate desiccation, at least during hot periods of tidal exposure. This has implications for the efficacy of wetting and drying as a weathering mechanism across the intertidal and splash zones in association with the repeated expansion and contraction and microtopographic fluctuation of the substrate (e.g., Kanyaya and Trenhaile, 2005; Trenhaile, 2006; Porter and Trenhaile, 2007). Indeed, the modulation of wetting and drying cycles (and associated expansion and contraction) by biota, including microorganisms (e.g., Mayaud et al., 2014), may have significant weathering implications for a range of substrates, especially clay-bearing types including mudstones (e.g., Stephenson and Kirk, 2000; Baxter, 2015) and the sandy siltstones of our study platform composed of 10–20% coarse-to-medium silts and 60–70% fine silt and clay (Rico et al., 2020).
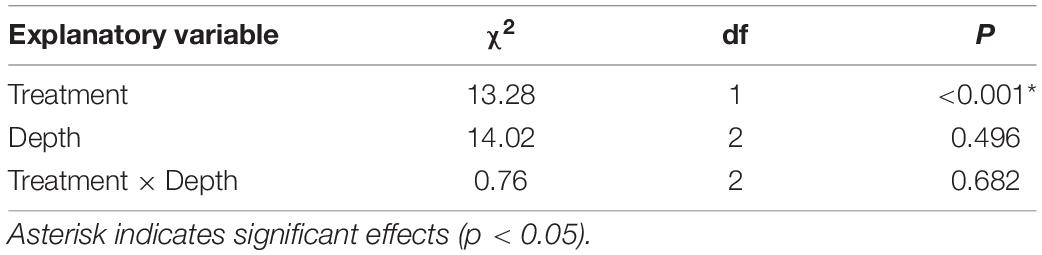
Table 1. Analysis of deviance for beta regression model relating substrate moisture to treatment (mussel-removal vs. control benches) and depth layer (0–5, 5–10, and 10–15 mm).
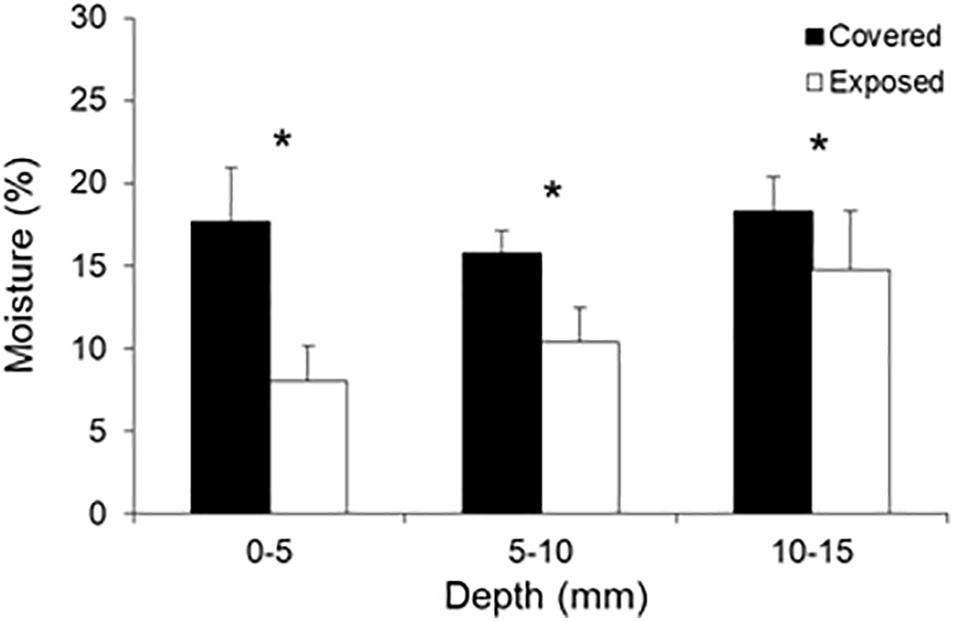
Figure 3. Substrate moisture content (water mass as a percentage of sample mass) of mussel-covered and mussel-removal (exposed) bench materials at three depth ranges. Substrate samples were collected on a clear and exceptionally hot late-spring day (November 21, 2018) 2.5–3 h after the tide had receded and the platform was air-exposed. The vertical lines above the bars indicate 1 SD. Asterisks indicate significant treatment effects at the corresponding depth level (p < 0.05).
The subsurface salt content of the platform material further indicates the moisture-modulating role of mussels. Specifically, we found higher concentrations of both chloride and sulfate ions in the upper 5-mm layer of the substrate from the mussel-removal benches relative to mussel-covered controls, while there was no difference in the deeper layers (5–15 mm) (Table 2 and Figure 4). This indicates that salt crystallization is reduced in the uppermost layer of the platform when covered by mussels—probably in association with their temperature-buffering and moisture-retaining functions. While such effects have been proposed based on laboratory simulations (see Gowell et al., 2015), this provides some of the first direct field evidence that biotic cover can reduce the efficacy of salt crystallization in the surface layers of intertidal platforms (also see Baxter, 2015 and Coombes et al., 2017). Salt weathering is thought to be important on rocky shores during hot weather, when desiccation during daytime tidal exposure causes the crystallization of dissolved salts in confined rock pores and associated destructive forces (Mottershead, 1989, 2013; Stephenson and Kirk, 2000; Porter and Trenhaile, 2007; Coombes, 2014). As well as reduced amounts of salt overall, lower crystallization frequencies and the less frequent hydration and thermal expansion/contraction of salt crystals within the upper layers of mussel-covered substrates due to the observed moisture/temperature-buffering effects may limit weathering relative to exposed substrates (e.g., Gowell et al., 2015; Coombes et al., 2017).
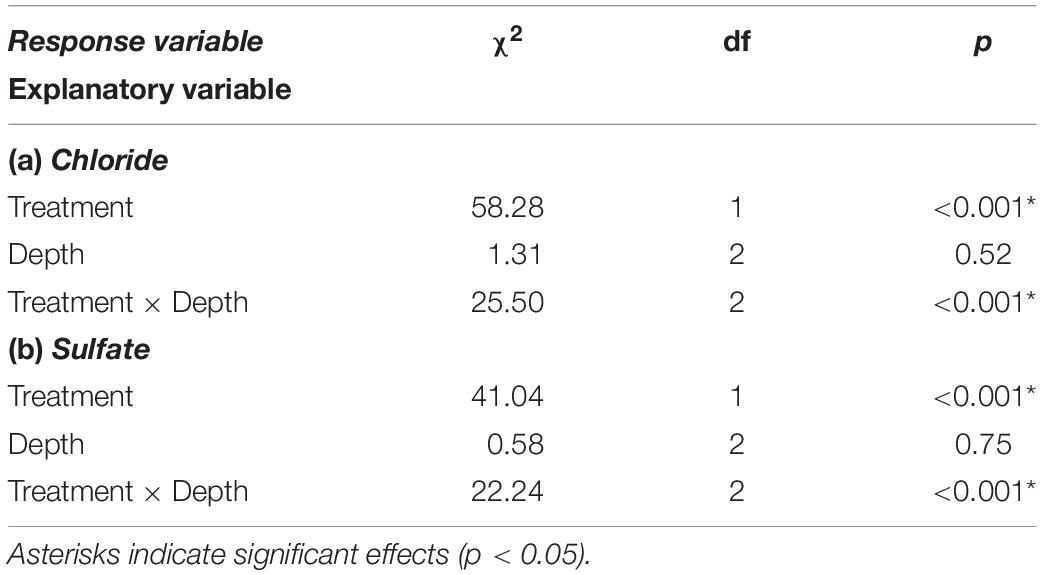
Table 2. Analysis of deviance for beta regression models relating subsurface salt content of the platform substrate to treatment (mussel-removal vs. control benches) and depth layer (0–5, 5–10, and 10–15 mm).
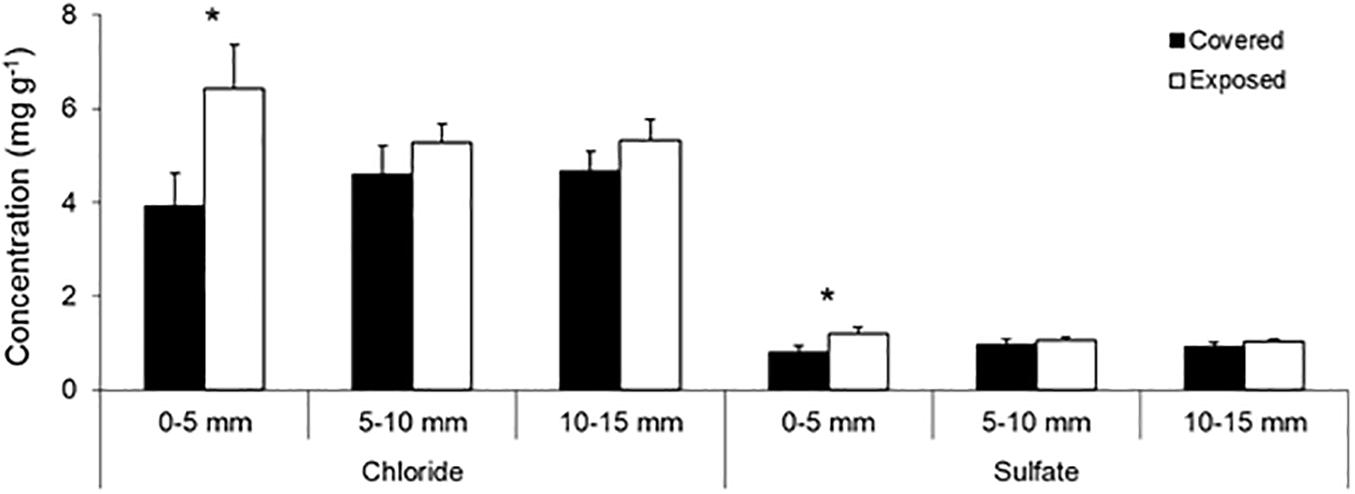
Figure 4. Subsurface salt content of the mussel-covered and mussel-removal (exposed) bench substrates at three depth ranges. Substrate samples were collected on a clear and exceptionally hot late-spring day (November 21, 2018) 2.5–3 h after the tide had receded and the platform was air-exposed. The vertical lines above the bars indicate 1 SD. Asterisks indicate significant treatment effects at the corresponding depth level (p < 0.05).
Effects of Mussels on Hydrodynamic and Abrasive Forces
Based on the significantly greater weight losses of the plaster discs placed on mussel-covered benches relative to those without mussels (χ2 = 12.81, df = 1, p < 0.001; Figure 5), our results indicate that hydrodynamic and abrasive forces are lower within mussel beds. This conforms to the findings of Carrington et al. (2008), who observed that water flow in the interstitial spaces of mussel beds is low relative to above mussel beds and in mussel-free estuarine bottoms. This provides some initial support for mussel beds reducing the mechanical impacts of sediment-laden waves and tidal flows on platform rock—especially very soft rock as studied here—both by physically armoring the surface and moderating near-surface water flows. Similar findings were reported by Baxter (2015) for mussel-colonized and artificially cleared patches on an intertidal mudstone platform in Wales, United Kingdom. Further work is now needed to determine whether such effects translate into altered rates of erosion and direct loss of material from the surfaces of colonized intertidal rock.
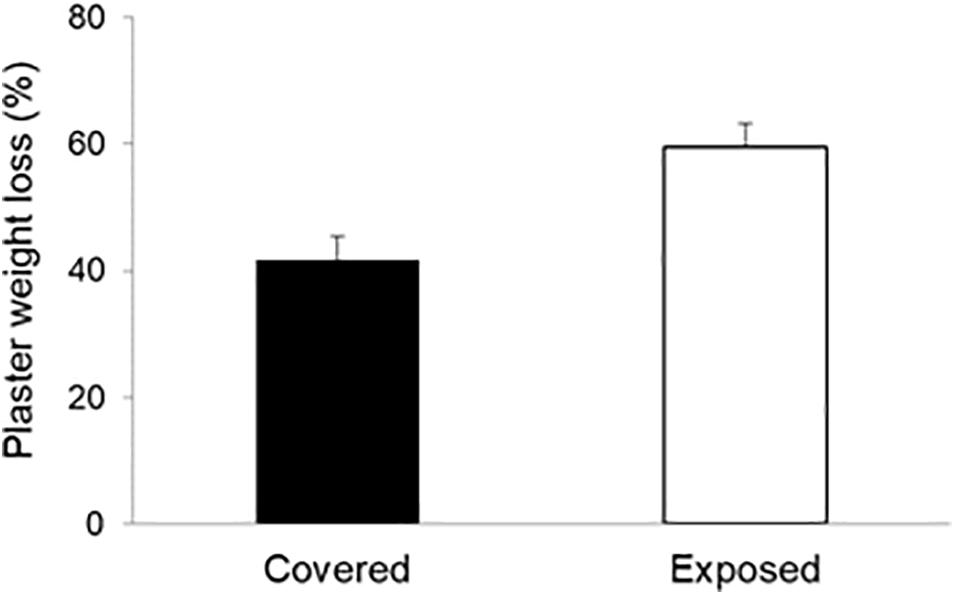
Figure 5. Flow/abrasion rates at the surfaces of mussel-covered and mussel-removal (exposed) benches based on the weight losses of plaster discs (5 cm diameter, 1.5 cm height). Discs attached to the mussel-covered benched had their sides shielded by the surrounding mussels. The vertical lines above the bars indicate 1 SD. Differences among treatments were significant (p < 0.001).
Effects of Mussels on Platform Height and Substrate Hardness
Mussel removal led to significant decreases in platform height (Table 3). Height losses in the mussel-removal treatments averaged 2 mm after 6 months, while the height of mussel-covered benches remained relatively constant over the same period, with minor variations attributable to measurement error as well as possible swelling and shrinking in response to wetting-drying effects (Figure 6). The observed decreases in platform height following mussel removal cannot directly be ascribed to the weathering processes discussed in section “Effects of Mussels on Weathering Regimes” as the height losses mostly occurred during the first 2 months of the experiment and under winter conditions (see Figure 6). Compared to other times of the year, wetting-drying and salt weathering are expected to be less efficient during winter, when desiccation is limited due to relatively low temperatures and irradiation. On the other hand, thermal fatigue due to short-term temperature variations (i.e., second-to-minute fluctuations attributable to winds and clouds; see section “Effects of Mussels on Weathering Regimes”) might continue to operate in winter and may, therefore, have contributed to the observed lowering of the mussel-removal benches. The likelihood that frost weathering occurred during these months is also low as minimum air temperatures fell below the freezing point of seawater (−2.5°C) on only two occasions, when the platform was exposed to such temperatures for less than 2 h (see Table 4). This suggests that the duration of freezing periods and the number of freeze-thaw cycles were well below those required for the weathering of consolidated intertidal substrates (see Robinson and Jerwood, 1987; Dewez et al., 2015). Nevertheless, the potential impacts of biotic cover on the ice-driven breakdown of coastal rocks warrants further investigation, as freezing processes can be important in some situations (e.g., Trenhaile and Rudakas, 1981; Trenhaile and Mercan, 1984; Robinson and Jerwood, 1987; Dewez et al., 2015). Mussels may be especially suited to protect intertidal rocks from frost weathering as they have evolved local adaptations to survive freezing temperatures (e.g., down to −13°C in the case of Arctic populations of Mytilus edulis; Thyrring et al., 2020).
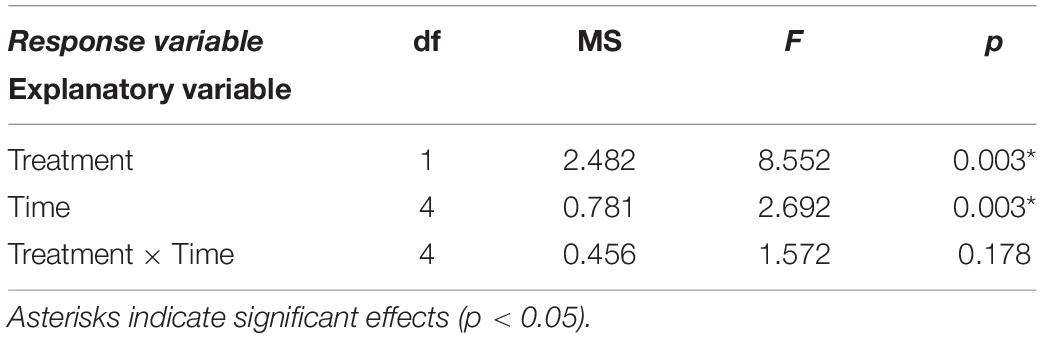
Table 3. Results of repeated-measures ANOVA testing the effects of treatment (mussel-removal vs. control) and sampling date (Time) on platform height loss.
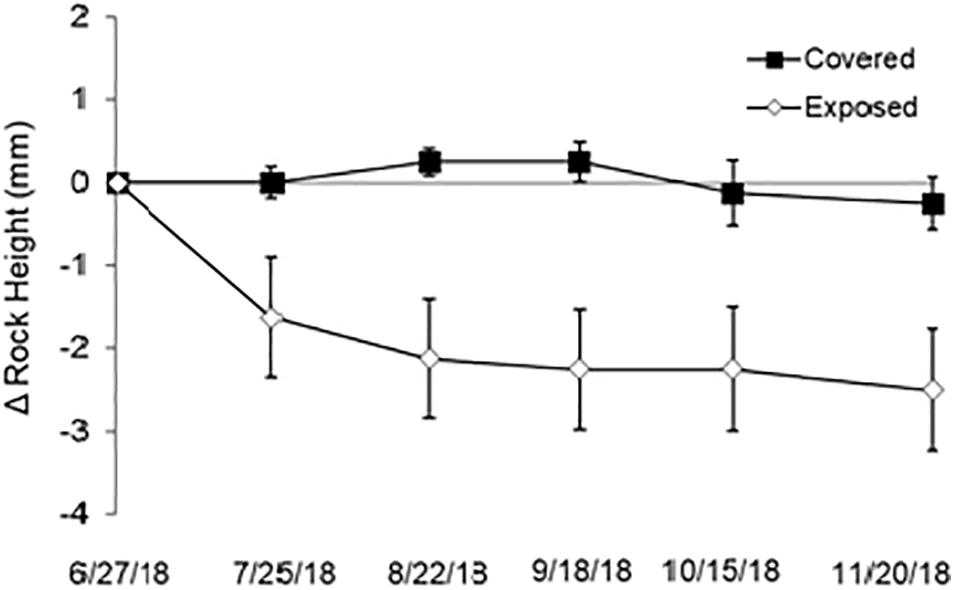
Figure 6. Variations in the height of mussel-covered and mussel-removal (exposed) benches during the study period. The experiment began on June 27, 2018, and the differences among treatments were significant on all subsequent sampling dates (p < 0.05). The vertical lines above and below the data points indicate 1 SD.

Table 4. Dates with air temperatures below the freezing point of seawater (i.e., −2.5°C) and associated platform exposure times quantified as the number of hourly air-exposed temperature records <−2.5°C.
The hydraulic and abrasive impacts of sediment-laden waves are also unlikely to be the cause of the measured lowering of the mussel-removal benches during the first 2 months of the experiment. Although storm conditions conducive to erosion in the study area tend to be more frequent during the winter (i.e., the early phases of our experiment), they occur during all seasons (see Fiore et al., 2009). Within the specific timeframe of this experiment, we found no evidence of increased storm frequency or intensity during the earlier, winter months. Indeed, based on an analysis of positive storm surges (i.e., positive deviations of the observed tide relative to the astronomic tide that exceeded 30 cm and persisted for 6 h or more; see Fiore et al., 2009), storms and wave energy were evenly distributed across the experimental period (see Figure 7) and cannot, therefore, directly explain the temporal pattern of height loss on the mussel-removal benches.
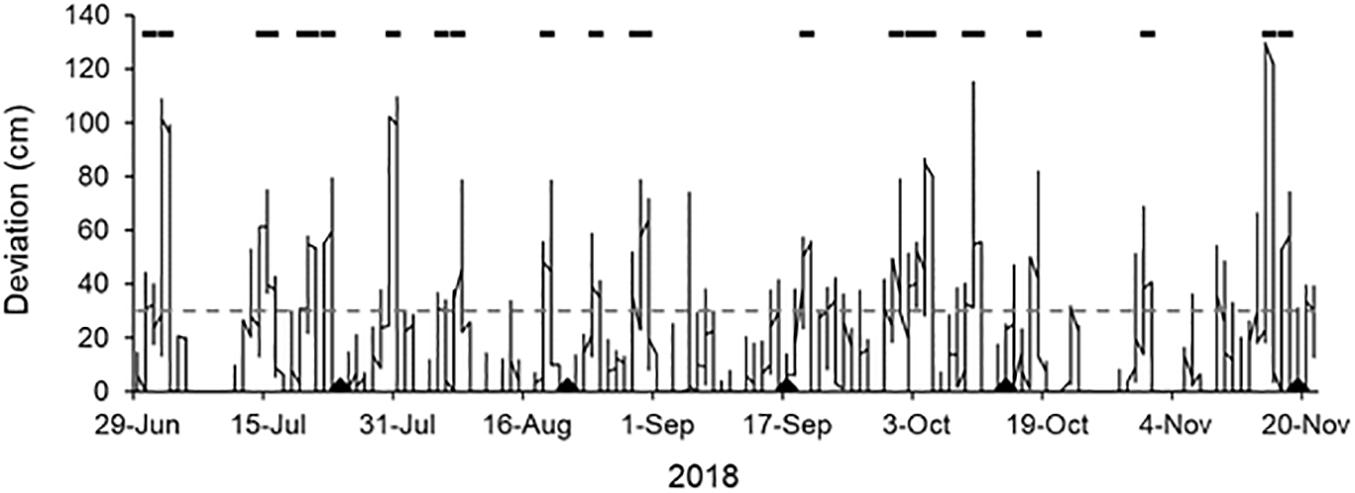
Figure 7. Positive deviations in the tide relative to tidal predictions during the experimental period. The black bars above the curve indicate storms, defined as periods with deviations >30 cm (gray dashed line) lasting for more than 6 h (see Fiore et al., 2009). Sampling dates are indicated as triangles on the x-axis. Tidal records were obtained from the tide gauge at Puerto Mar del Plata (38°02′08′′ S, 57°31′52′′ W, ∼ 35 km north of the study site). Tidal records and predictions were provided by the Servicio de Hidrografía Naval, Ministerio de Defensa, Argentina.
One possible explanation is that mussels weaken the rock they are attached to, for example by enhancing chemical weathering via the retention of water and organic matter (see Moura et al., 2012), but continue to keep these surfaces consolidated as long as they remain in place. Once detached or removed, lowering rates would be relatively rapid at first and then stabilize. This process is analogous to the rapid, catastrophic loss of rock and stone in the terrestrial environment following the removal of protective weathering crusts, which exposes the softened, highly erodible material underneath (e.g., Smith et al., 2010). While it is difficult to confirm such effects, it is possible that the impacts of mussels and other forms of biotic cover on intertidal rock weathering may involve a complex balance of bioprotective (when in place) and bioerosive (once detached/removed) effects.
Another possible explanation is that the experimental removal of the mussels weakened the surfaces of the benches. Mussel detachment involves the failure of the collagenous byssal threads they produce to adhere to the substrate. Typically, byssus failure occurs either because the thread itself or its basal portion breaks (thread and root failure, respectively), the adhesive plaque at the base of the thread detaches from the substrate (plaque failure), or the substrate to which the adhesive plaque attaches is dislodged (substrate failure) (see Bell and Gosline, 1997). Individually, a substrate failure implies the removal of a small substrate chip from the platform surface (i.e., at scales of tens to hundreds of micrometers, based on adhesive plaque size). Yet, a high number of substrate failures per unit area can occur during the mass detachment of mussels. For instance, mussels 15–20 mm in length at our study area have approximately 80 byssal threads on average, and 30% of the failures observed during their detachment occur at the substrate level (Gutiérrez et al., 2018). Considering that mussels within this size range can occur at densities of 200 ind. dm–2 at this site (Soria, 2020), their mass detachment (either due to experimental removal or storm wave action) could cause approximately 4,800 substrate failures per dm–2. Although an underestimate is based on a subset of mussel size classes occurring in the field, this calculation illustrates that these highly friable bench surfaces could be densely chipped and weakened during mussel detachment.
While most of the observed decrease in platform height occurred within the first 2 months after mussel removal, with a slower rate of lowering thereafter, it is not possible to rule out the potential for a subsequent period of rapid lowering. Unfortunately, barnacle recruitment prevented us from continuing the experiment over the summer (see section “Field Experiment”) under higher temperature, irradiation, and desiccation conditions. However, our substrate hardness measurements, which were carried out at the end of the experiment in late spring, indicated significantly lower surface hardnesses in the mussel-removal patches compared to the mussel-covered controls (χ2 = 12.36, df = 1, p < 0.001; Figure 8). This suggests that progressive surface weathering during the 6 months following mussel removal was linked to the development of weaker bench surfaces relative to those with mussels still attached.
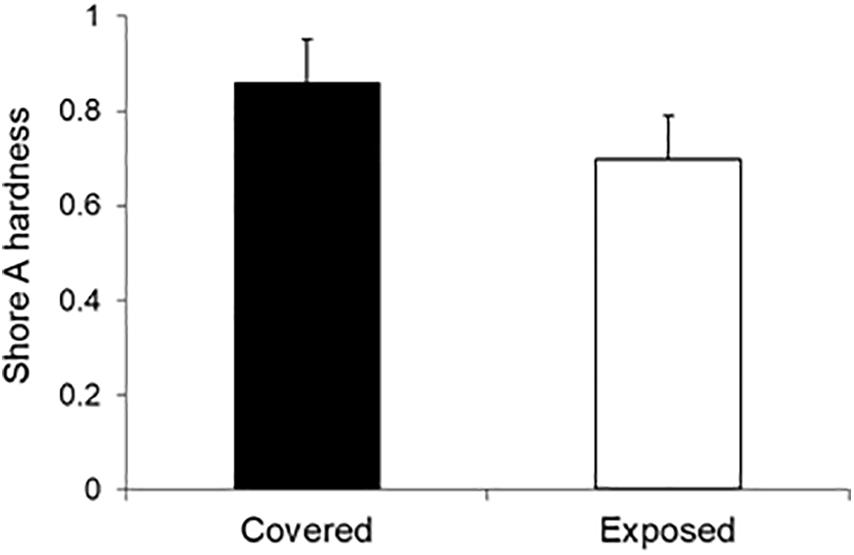
Figure 8. Substrate hardness of the mussel-covered and mussel-removal (exposed) benches. Hardness was measured in Shore A units, which range from 0 (low) to (1) high. The vertical lines above the bars indicate 1 SD. Differences among treatments were significant (p < 0.001).
Conclusion and Prospects
This study shows that mussel cover has clear bioprotective potential by (1) modulating temperature, desiccation, and salt crystallization regimes; and (2) reducing the impacts of water flow and abrasion, which is in line with previous findings for seaweeds and barnacles (Coombes et al., 2013, 2017; Gowell et al., 2015). Additionally, we illustrate for the first time that biotic cover can limit the erosion of shore platforms based on observed rates of surface lowering. Aside from the possible mechanisms of platform lowering following mussel removal (i.e., loss of protective mussel cover and/or exposure of relatively weaker/softer surfaces, weakening of the platform surface during mussel detachment, or a combination of these; see section “Effects of Mussels on Platform Height and Substrate Hardness”), our observations highlight the importance of persistent biotic covers for the stability of friable and soft platform materials. Indeed, in the case of semi-consolidated shore platforms, which can undergo weathering and erosion over relatively short timescales, this implies that the loss and recovery dynamics of ecological communities can have particularly important geomorphological implications. Studies involving other forms of biotic cover and different physical settings are now needed to explore these linkages.
In addition to their stabilizing effects on cohesive shore platforms, mussels may also have indirect stabilizing impacts on the cliffs they front. Shore platforms are a by-product of cliff retreat but also act as a natural defense against wave attack (Balson et al., 2006; Brew, 2007; Moses, 2014; Payo et al., 2015). Consequently, the protective role of platforms gradually decreases as they are progressively lowered by weathering and erosion (Trenhaile, 2002). It follows that, by stabilizing the studied platforms, mussels, which typically form beds at mid-intertidal elevations and persist all year round, help maintain the protective function of these platforms with respect to wave attack and cliff erosion.
Threats to the persistence and extent of mussel cover on intertidal platforms are varied and increasing in frequency and magnitude globally (e.g., human harvesting and trampling, storms, pollution, climate change, and heat waves; see Tsuchiya, 1983; Erlandsson et al., 2006, 2011; Micheli et al., 2016; Mendez et al., 2017; Seuront et al., 2019). In the light of our findings, these changes are expected to accelerate erosion rates on cohesive shore platforms along with the loss of potential protective functions for backing cliffs. Mussel bed conservation and enhancement measures could, therefore, contribute to coastline stability alongside the maintenance of associated biodiversity in the face of a range of anthropogenic threats including climate change and sea-level rise.
Data Availability Statement
The original contributions presented in the study are included in the article/Supplementary Material, further inquiries can be directed to the corresponding author.
Author Contributions
JLG, MC, MP, and JAG contributed to the conception and design of the study. JAG, JLG, and SS conducted the field research. JAG and MC analyzed substrate samples in the lab. JAG and JLG analyzed the data. JLG, JAG, MC, and FI wrote the original draft. All authors contributed to manuscript revision, read, and approved the submitted version.
Funding
This study was supported by the National Geographic Society (Grant No. CP-120R-17, to JLG, MC and MP) and Agencia Nacional de Promoción Científica y Tecnológica (PICT-2019-03904 to all authors).
Conflict of Interest
The authors declare that the research was conducted in the absence of any commercial or financial relationships that could be construed as a potential conflict of interest.
Publisher’s Note
All claims expressed in this article are solely those of the authors and do not necessarily represent those of their affiliated organizations, or those of the publisher, the editors and the reviewers. Any product that may be evaluated in this article, or claim that may be made by its manufacturer, is not guaranteed or endorsed by the publisher.
Acknowledgments
We thank María Bagur for feedback during the design of the project. We are also indebted to the Servicio de Hidrografía Naval – Sección Mareas (Ministerio de Defensa de la Nación) for providing tidal measurements from Mar del Plata port and the Servicio Meteorológico Nacional (Ministerio de Defensa de la Nación) for their publicly accessible local weather databases. We also thank Mona Edwards at the School of Geography and the Environment, University of Oxford, for assistance with the ion chromatography analysis. This is a contribution to the program of GrIETA.
Supplementary Material
The Supplementary Material for this article can be found online at: https://www.frontiersin.org/articles/10.3389/fmars.2021.756016/full#supplementary-material
Footnotes
References
Arribas, L. P., Bagur, M., Gutiérrez, J. L., and Palomo, M. G. (2015). Matching spatial scales of variation in mussel recruitment and adult densities across Southwestern Atlantic rocky shores. J. Sea Res. 95, 16–21. doi: 10.1016/j.seares.2014.10.015
Arribas, L. P., Bagur, M., Klein, E., Penchaszadeh, P., and Palomo, M. G. (2013). Geographic distribution of two mussel species and associated assemblages along the northern Argentinean coast. Aquat. Biol. 18, 91–103. doi: 10.3354/ab00495
Bagur, M., Gutiérrez, J. L., Arribas, L. P., and Palomo, M. G. (2014). Endolithic invertebrate communities and bioerosion rates in Southwestern Atlantic intertidal consolidated sediments. Mar. Biol. 161, 2279–2292. doi: 10.1007/s00227-014-2505-8
Bagur, M., Gutiérrez, J. L., Arribas, L. P., and Palomo, M. G. (2016). Complementary influences of co-occurring physical ecosystem engineers on species richness: insights from a Patagonian rocky shore. Biodivers. Conserv. 25, 2787–2802. doi: 10.1007/s10531-016-1203-x
Balson, P. S., Brew, D. S., Charman, R. O., Hobbs, P., Moses, C. A., Pearson, S., et al. (2006). “Coastal retreat: the role of eroding cohesive shore platforms,” in Proceedings of the Defra Conference on Flood and Coastal Erosion Risk Management (York, UK), 1–9. doi: 10.1007/978-3-319-48657-4_288-2
Bates, D., Mächler, M., Bolker, B., and Walker, S. (2015). Fitting linear mixed-effects models using lme4. J. Stat. Softw. 67, 1–48.
Baxter, T. (2015). The Bioprotective Properties Of The Blue Mussel (Mytilus Edulis) On Rocky Shore Platforms. Undergraduate Dissertation. University of Oxford, UK.
Bell, E. C., and Gosline, J. M. (1997). Strategies for life in flow: tenacity, morphometry, and probability of dislodgment of two Mytilus species. Mar. Ecol. Prog. Ser. 159, 197–208. doi: 10.3354/meps159197
Borthagaray, A. I., and Carranza, A. (2007). Mussels as ecosystem engineers: their contribution to species richness in a rocky littoral community. Acta Oecol. 31, 243–250. doi: 10.1016/j.actao.2006.10.008
Brew, D. S. (2007). Understanding and Predicting Beach Morphological Change Processes Associated with the Erosion of Cohesive Foreshores. London: DEFRA.
Carrington, E., Moeser, G. M., Thompson, S. B., Coutts, L. C., and Craig, C. A. (2008). Mussel attachment on rocky shores: the effect of flow on byssus production. Integr. Comp. Biol. 48, 801–807. doi: 10.1093/icb/icn078
Carter, N. E. A., and Viles, H. A. (2005). Bioprotection explored: the story of a little known earth surface process. Geomorphology 67, 273–281. doi: 10.1016/j.geomorph.2004.10.004
Charman, R., Cane, T., Moses, C., and Williams, R. (2007). A device for measuring downwearing rates on cohesive shore platforms. Earth Surf. Process. Landf. 32, 2212–2221. doi: 10.1002/esp.1542
Coombes, M. A. (2011). Rock warming and drying under simulated intertidal conditions, part I: experimental procedures and comparisons with field data. Earth Surf. Process. Landf. 36, 2114–2121.
Coombes, M. A. (2014). “The rock coast of the British Isles: weathering and biogenic processes,” in Rocky Coasts: a Global Synthesis, Vol. 40, eds D. Kennedy, W. Stephenson, and L. Naylor (London: Geological Society), 57–76. doi: 10.1144/m40.5
Coombes, M. A., and Naylor, L. A. (2012). Rock warming and drying under simulated intertidal conditions, part II: weathering and biological influences on evaporative cooling and near-surface micro-climatic conditions as an example of biogeomorphic ecosystem engineering. Earth Surf. Process. Landf. 37, 100–118.
Coombes, M. A., Naylor, L. A., Viles, H. A., and Thompson, R. C. (2013). Bioprotection and disturbance: seaweed, microclimatic stability and conditions for mechanical weathering in the intertidal zone. Geomorphology 202, 4–14.
Coombes, M. A., Viles, H. A., Naylor, L. A., and La Marca, E. C. (2017). Cool barnacles: do common biogenic structures enhance or retard rates of deterioration of intertidal rocks and concrete? Sci. Total Environ. 580, 1034–1045. doi: 10.1016/j.scitotenv.2016.12.058
Desarnaud, J., Kiriyama, K., Bicer Simsir, B., Wilhelm, K., and Viles, H. (2019). A laboratory study of Equotip surface hardness measurements on a range of sandstones: what influences the values and what do they mean? Earth Surf. Process. Landf. 44, 1419–1429. doi: 10.1002/esp.4584
Dewez, T. J. B., Regard, V., Duperret, A., and Lasseur, E. (2015). Shore platform lowering due to frost shattering during the 2009 winter at Mesnil Val, English Channel coast, NW France. Earth Surf. Process. Landf. 40, 1688–1700. doi: 10.1002/esp.3760
Dodge-Wan, D., and Nagarajan, R. (2020). Boring of intertidal sandstones by isopod Sphaeroma triste in NW Borneo (Sarawak. Malaysia). J. Coast. Res. 36, 238–248. doi: 10.2112/jcoastres-d-19-00066.1
Erlandsson, J., McQuaid, C. D., and Stanczak, S. (2011). Recruit/algal interaction prevents recovery of overexploited mussel beds: indirect evidence that post-settlement mortality structures mussel populations. Estuar. Coast. Shelf Sci. 92, 132–139. doi: 10.1016/j.ecss.2010.12.028
Erlandsson, J., Pal, P., and McQuaid, C. D. (2006). Re-colonisation rate differs between co-existing indigenous and invasive intertidal mussels following major disturbance. Mar. Ecol. Prog. Ser. 320, 169–176. doi: 10.3354/meps320169
Fiore, M. M., D’Onofrio, E. E., Pousa, J. L., Schnack, E. J., and Bertola, G. R. (2009). Storm surges and coastal impacts at Mar del Plata, Argentina. Cont. Shelf Res. 29, 1643–1649. doi: 10.1016/j.csr.2009.05.004
Giampietri, L., and Piccolo, M. C. (2000). Diferencias climáticas en el área costera de la ciudad de Mar del Plata. Geoacta 25, 65–74.
Gómez-Pujol, L., Pérez-Alberti, A., Blanco-Chao, R., Costa, S., Neves, M., and Del Río, L. (2014). “The rock coast of continental Europe in the Atlantic,” in Rocky Coasts: a Global Synthesis, Vol. 40, eds D. Kennedy, W. Stephenson, and L. Naylor (London: Geological Society), 77–88. doi: 10.1144/m40.6
Gowell, M., Coombes, M. A., and Viles, H. A. (2015). Rock-protecting seaweed? Experimental evidence of bioprotection in the intertidal zone. Earth Surf. Process. Landf. 40, 1364–1370. doi: 10.1002/esp.3736
Guichard, F., and Bourget, E. (1998). Topographic heterogeneity, hydrodynamics, and benthic community structure: a scale-dependent cascade. Mar. Ecol. Prog. Ser. 171, 59–70. doi: 10.3354/meps171059
Gutiérrez, J. L., Bagur, M., and Palomo, M. G. (2019). Algal epibionts as co-engineers in mussel beds: effects on abiotic conditions and mobile interstitial invertebrates. Diversity 11:17. doi: 10.3390/d11020017
Gutiérrez, J. L., Bagur, M., Arribas, L. P., and Palomo, M. G. (2018). Does rock type account for variation in mussel attachment strength? A test with Brachidontes rodriguezii in the southwestern Atlantic. Helgol. Mar. Res. 72:10.
Gutiérrez, J. L., Jones, C. G., Byers, J. E., Arkema, K. K., Berkenbusch, K., Committo, J. A., et al. (2011). “Physical ecosystem engineers and the functioning of estuaries and coasts,” in Functioning of Estuaries and Coastal Ecosystems,Treatise on Estuarine and Coastal Science, Vol. 7, eds C. H. R. Heip, C. J. M. Philippart, J. J. Middelburg, E. Wolanski, and D. McLusky (Amsterdam: Elsevier), 53–81. doi: 10.1016/b978-0-12-374711-2.00705-1
Gutiérrez, J. L., Jones, C. G., Strayer, D. L., and Iribarne, O. O. (2003). Mollusks as ecosystem engineers: the role of shell production in aquatic habitats. Oikos 101, 79–90. doi: 10.1034/j.1600-0706.2003.12322.x
Gutiérrez, J. L., Palomo, M. G., Bagur, M., Arribas, L. P., and Soria, S. A. (2015). Wave action limits crowding in an intertidal mussel. Mar. Ecol. Prog. Ser. 518, 153–163. doi: 10.3354/meps11086
Hall, K., and Thorn, C. E. (2014). Thermal fatigue and thermal shock in bedrock: an attempt to unravel the geomorphic processes and products. Geomorphology 206, 1–13. doi: 10.1016/j.geomorph.2013.09.022
Healy, T. R. (1968). Bioerosion on shore platforms developed in the Waitemata Formation, Auckland. Earth Sci. J. 2, 26–37.
Helmuth, B. S. (1998). Intertidal mussel microclimates: predicting the body temperature of a sessile invertebrate. Ecol. Monogr. 68, 51–74. doi: 10.1890/0012-9615(1998)068[0051:immptb]2.0.co;2
Isla, F. I., Cortizo, L., Merlotto, A., Bértola, G., Pontrelli Albisetti, M., and Finocchietti, C. (2018). Erosion in Buenos Aires province: coastal-management policy revisited. Ocean Coast. Manage. 156, 107–116. doi: 10.1016/j.ocecoaman.2017.09.008
Isla, F. I., Taglioretti, M., and Dondas, A. (2015). Revisión y nuevos aportes sobre la estratigrafía y sedimentología de los acantilados entre Mar de Cobo y Miramar, provincia de Buenos Aires. Rev. Asoc. Geol. Argent. 72, 235–250.
Jones, C. G., Lawton, J. H., and Shachak, M. (1994). Organisms as ecosystem engineers. Oikos 69, 373–386. doi: 10.2307/3545850
Kanyaya, J. I., and Trenhaile, A. S. (2005). Tidal wetting and drying on shore platforms: an experimental assessment. Geomorphology 70, 129–146. doi: 10.1016/j.geomorph.2005.04.005
Knight, J., and Burningham, H. (2020). Bedrock hardness values and morphological zonation of a shore platform in South Africa. Trans. Royal Soc. S. Afr. 75, 40–53. doi: 10.1080/0035919x.2019.1670751
La Marca, E. C., Coombes, M. A., Viles, H. A., and Naylor, L. A. (2014). The bio-protective role of a biological encrustation. Biol. Mar. Mediterr. 21, 345–346.
Lamarchina, S., Maenza, R. A., and Isla, F. I. (2021). Mixed sand and gravel beaches of Buenos Aires. Argentina. Morphodynamics and stability. J. Coast. Conserv. 25:41. doi: 10.1007/s11852-021-00830-7
Ledesma-Vázquez, J., and Johnson, M. E. (1994). Late pliocene abrasion platform from the cantil costero formation of Baja California. Cienc. Mar. 20, 139–157. doi: 10.7773/cm.v20i2.964
Mayaud, J. R., Viles, H. A., and Coombes, M. A. (2014). Exploring the influence of biofilm on short-term expansion and contraction of supratidal rock: an example from the Mediterranean. Earth Surf. Process. Landf 39, 1404–1412. doi: 10.1002/esp.3602
Mendez, M. M., Livore, J. P., Calcagno, J. A., and Bigatti, G. (2017). Effects of recreational activities on Patagonian rocky shores. Mar. Environ. Res. 130, 213–220.
Micheli, F., Heiman, K. W., Kappel, C. V., Martone, R. G., Sethi, S. A., Osio, G. C., et al. (2016). Combined impacts of natural and human disturbances on rocky shore communities. Ocean Coast. Manag. 126, 42–50.
Molaro, J. L., Walsh, K. J., Jawin, E. R., Ballouz, R. L., Bennett, C. A., DellaGiustina, D. N., et al. (2020). In situ evidence of thermally induced rock breakdown widespread on Bennu’s surface. Nat. Commun. 11:2913. doi: 10.1038/s41467-020-16528-7
Moses, C. (2014). “The rock coast of the British Isles: shore platforms,” in Rocky Coasts: a Global Synthesis, Vol. 40, eds D. Kennedy, W. Stephenson, and L. Naylor (London: Geological Society), 39–55. doi: 10.1144/M40.4
Mottershead, D. N. (1989). Rates and patterns of bedrock denudation by coastal salt spray weathering: a seven-year record. Earth Surf. Process. Landf. 14, 383–398. doi: 10.1002/esp.3290140504
Mottershead, D. N. (2013). “Coastal weathering,” in Treatise on Geomorphology, Volume 4, Weathering and Soils Geomorphology, ed. J. F. Shroder (San Diego, CA: Academic Press), 228–244. doi: 10.1016/b978-0-12-374739-6.00064-6
Moura, D., Gabriel, S., Gamito, S., Santos, R., Zugasti, E., Naylor, L., et al. (2012). Integrated assessment of bioerosion, biocover and downwearing rates of carbonate rock shore platforms in southern Portugal. Cont. Shelf Res. 38, 79–88. doi: 10.1016/j.csr.2012.03.003
Naylor, L. A., and Coombes, M. A. (2015). Bioprotection: working with nature to manage coastal hazards. Geogr. Rev. 28, 37–41.
Naylor, L. A., Coombes, M. A., and Viles, H. A. (2012). Reconceptualising the role of organisms in the erosion of rock coasts: a new model. Geomorphology 15, 17–30. doi: 10.1016/j.geomorph.2011.07.015
Neter, J., Wasserman, W., and Kutner, M. H. (1990). Applied Linear Statistical Models. Regression, Analysis of Variance, and Experimental Designs. Homewood, IL: Irwin.
Pappalardo, M., and D’Olivo, M. (2019). Testing a methodology to assess fluctuations of coastal rocks surface temperature. J. Mar. Sci. Eng. 7:315. doi: 10.3390/jmse7090315
Payo, A., Hall, J. W., Dickson, M. E., and Walkden, M. J. (2015). Feedback structure of cliff and shore platform morphodynamics. J. Coast. Conserv. 19, 847–859. doi: 10.1007/s11852-014-0342-z
Pinn, E. H., Richardson, C. A., Thompson, R. C., and Hawkins, S. J. (2005). Burrow morphology, biometry, age and growth of piddocks (Mollusca: Bivalvia: Pholadidae) on the south coast of England. Mar. Biol. 147, 943–953. doi: 10.1007/s00227-005-1582-0
Porter, N. J., and Trenhaile, A. S. (2007). Short-term rock surface expansion and contraction in the intertidal zone. Earth Surf. Process. Landf. 32, 1379–1397. doi: 10.1002/esp.1479
R Core Team (2020). R: A Language And Environment For Statistical Computing v 3.4.1. (Vienna: R Foundation for Statistical Computing).
Rico, Y., Gómez Samus, L., and Bidegain, J. C. (2020). Magnetoestratigrafía y parámetros magnéticos de los acantilados de San Eduardo del Mar. Buenos Aires, Argentina. Rev. Asoc. Geol. Argent. 77, 104–131.
Robinson, D. A., and Jerwood, L. C. (1987). Sub-aerial weathering of chalk shore platforms during harsh winters in southeast England. Mar. Geol. 77, 1–14. doi: 10.1016/0025-3227(87)90080-6
Santos, A. R., Veiga, M. D. R., Matias, L., Santos Silva, A., and De Brito, J. (2018). Durability and compatibility of lime-based mortars: the effect of aggregates. Infrastructures 3:34.
Santos, T., Gomes, M. I., Silva, A. S., Ferraz, E., and Faria, P. (2020). Comparison of mineralogical, mechanical and hygroscopic characteristic of earthen, gypsum and cement-based plasters. Constr. Build. Mater. 254:119222. doi: 10.1016/j.conbuildmat.2020.119222
Scrosati, R. A., and Ellrich, J. A. (2018). Thermal moderation of the intertidal zone by seaweed canopies in winter. Mar. Biol. 165:115. doi: 10.1007/s00227-018-3374-3
Seuront, L., Nicastro, K. R., Zardi, G. I., and Goberville, E. (2019). Decreased thermal tolerance under recurrent heat stress conditions explains summer mass mortality of the blue mussel Mytilus edulis. Sci. Rep. 9:17498. doi: 10.1038/s41598-019-53580-w
Silliman, B. R., Bertness, M. D., Altieri, A. H., Griffin, J. N., Bazterrica, M. C., Hidalgo, F. J., et al. (2011). Whole-community facilitation regulates biodiversity on Patagonian rocky shores. PLoS One 6:e24502. doi: 10.1371/journal.pone.0024502
Smith, B. J., Gomez-Heras, M., and Viles, H. A. (2010). “Underlying issues on the selection, use and conservation of building limestone,” in Limestone in the Built Environment: Present-Day Challenges for the Preservation of the Past, eds B.J. Smith, M. Gomez-Heras, H.A. Viles, and J. Cassar (London: Geological Society) 331, 1–11. doi: 10.1144/SP331.1
Soria, S. A. (2020). Efectos de la Fragmentación De Bancos De Mejillín, Brachidontes Rodriguezii, Sobre Las Comunidades De Ambientes Rocosos Intermareales. Doctoral Thesis. Universidad de Buenos Aires, Argentina.
Spencer, T. (1988). Limestone coastal morphology: the biological contribution. Prog. Phys. Geogr. 12, 66–101. doi: 10.1177/030913338801200103
Stephenson, W. J., and Finlayson, B. L. (2009). Measuring erosion with the micro-erosion meter-Contributions to understanding landform evolution. Earth Sci. Rev. 95, 53–62. doi: 10.1016/j.earscirev.2009.03.006
Stephenson, W. J., and Kirk, R. M. (2000). Development of shore platforms on Kaikoura Peninsula. South Island, New Zealand: II: the role of subaerial weathering. Geomorphology 32, 43–56. doi: 10.1016/S0169-555X(99)00062-8
Thyrring, J., Tremblay, R., and Sejr, M. K. (2020). Local cold adaption increases the thermal window of temperate mussels in the Arctic. Conserv. Physiol. 7:coz098. doi: 10.1093/conphys/coz098
Trenhaile, A. S. (2002). Rock coasts, with particular emphasis on shore platforms. Geomorphology 48, 7–22. doi: 10.1016/S0169-555X(02)00173-3
Trenhaile, A. S. (2006). Tidal wetting and drying on shore platforms: an experimental study of surface expansion and contraction. Geomorphology 76, 316–331. doi: 10.1016/j.geomorph.2005.11.006
Trenhaile, A. S., and Mercan, D. W. (1984). Frost weathering and the saturation of coastal rocks. Earth Surf. Process. Landf. 9, 321–331. doi: 10.1002/esp.3290090405
Trenhaile, A. S., and Rudakas, P. (1981). Freeze-thaw and shore platform development in Gaspé. Québec. Geogr. Phys. Quat. 35, 171–181. doi: 10.7202/1000435ar
Trovant, B., Orensanz, J. L., Ruzzante, D. E., Stotz, W., and Basso, N. G. (2015). Scorched mussels (Bivalvia: Mytilidae: Brachidontinae) from the temperate coasts of South America: phylogenetic relationships, trans-Pacific connections and the footprints of Quaternary glaciations. Mol. Phylogenet. Evol. 82, 60–74. doi: 10.1016/j.ympev.2014.10.002
Trudgill, S. T. (1987). Bioerosion of intertidal limestone, Co. Clare, Eire 3: zonation, process and form. Mar. Geol. 74, 111–121. doi: 10.1016/0025-3227(87)90009-0
Tsuchiya, M. (1983). Mass mortality in a population of the mussel Mytilus edulis L. caused by high temperature on rocky shores. J. Exp. Mar. Biol. Ecol. 66, 101–111. doi: 10.1016/0022-0981(83)90032-1
Turowski, J. M., and Cook, K. L. (2017). Field techniques for measuring bedrock erosion and denudation. Earth Surf. Process. Landf. 42, 109–127.
Viles, H., Goudie, A., Grab, S., and Lalley, J. (2011). The use of the Schmidt Hammer and Equotip for rock hardness assessment in geomorphology and heritage science: a comparative analysis. Earth Surf. Process. Landf. 36, 320–333. doi: 10.1002/esp.2040
Wang, Z. F., Shen, S. L., Yin, Z. Y., and Xu, Y. S. (2015). Rapid field evaluation of the strength of cement-stabilized clayey soil. Bull. Eng. Geol. Environ. 74, 991–999. doi: 10.1007/s10064-014-0643-3
Wilhelm, K., Viles, H., and Burke, Ó (2016). Low impact surface hardness testing (Equotip) on porous surfaces–advances in methodology with implications for rock weathering and stone deterioration research. Earth Surf. Process. Landf. 41, 1027–1038. doi: 10.1002/esp.3882
Keywords: bioprotection, biotic cover, ecosystem engineering, intertidal, sessile organisms, biophysical interactions, mussel
Citation: Gonzalez JA, Coombes MA, Palomo MG, Isla FI, Soria SA and Gutiérrez JL (2021) Enhanced Weathering and Erosion of a Cohesive Shore Platform Following the Experimental Removal of Mussels. Front. Mar. Sci. 8:756016. doi: 10.3389/fmars.2021.756016
Received: 09 August 2021; Accepted: 27 September 2021;
Published: 21 October 2021.
Edited by:
Zhan Hu, Sun Yat-sen University, ChinaReviewed by:
Francesco Cozzoli, National Research Council, Consiglio Nazionale delle Ricerche (CNR), ItalyHelena Granja, University of Minho, Portugal
Copyright © 2021 Gonzalez, Coombes, Palomo, Isla, Soria and Gutiérrez. This is an open-access article distributed under the terms of the Creative Commons Attribution License (CC BY). The use, distribution or reproduction in other forums is permitted, provided the original author(s) and the copyright owner(s) are credited and that the original publication in this journal is cited, in accordance with accepted academic practice. No use, distribution or reproduction is permitted which does not comply with these terms.
*Correspondence: Jorge L. Gutiérrez, amd1dGllcnJlekBncmlldGEub3JnLmFy