- 1State Key Laboratory of Marine Resource Utilization in South China Sea, Hainan Aquaculture Breeding Engineering Research Center, Hainan University, Haikou, China
- 2Hainan Provincial Key Laboratory for Tropical Hydrobiology and Biotechnology, Department of Marine Sciences, College of Marine Science, Hainan University, Haikou, China
- 3Hainan Academy of Ocean and Fisheries Sciences, Haikou, China
- 4Department of Marine Sciences, University of Connecticut, Groton, CT, United States
The symbiosis of coral-Symbiodiniaceae is the quintessential basis of the coral reef ecosystem, and its breakdown results in coral bleaching, one of the most severe ecological catastrophes in the ocean. Critical to the establishment of the symbiosis is the host’s specific recognition of the symbionts through the binding of the coral host’s pattern recognition receptors (PRRs) to the symbiont cell surface’s glycoconjugates. However, the molecular basis for this recognition process is poorly understood. The present study investigated the binding affinities of the coral galectin PdGLT-1 to different symbiodiniacean species under different temperatures. At 25°C, the PdGLT-1 recombinant protein (rPdGLT-1) exhibited different binding affinities to different symbiodiniacean species from five genera, with a significantly higher binding affinity (p < 0.05) to Fugacium kawagutii (2.6-fold) and Cladocopium goreaui (1.9-fold) than Symbiodinium microadriaticum. The binding topology of rPdGLT-1 differed among the five symbiodiniacean species; for S. microadriaticum, Breviolum minutum, and Durusdinium trenchii, the binding was on some specific sites on the cell surface, whereas for C. goreaui and F. kawagutii, the binding signals were detected over the whole cell surface. Interestingly, PdGLT-1 binding induced agglutination of F. kawagutii cells but not of C. goreaui, explaining why C. goreaui was the most dominant symbiodiniacean symbionts in corals. Moreover, the affinity of rPdGLT-1 to Symbiodiniaceae was affected by temperature, and the highest binding affinities were observed at 30, 20, 30, 35, and 30°C for S. microadriaticum, B. minutum, C. goreaui, D. trenchii, and F. kawagutii, respectively. The optimal binding temperatures were consistent with the current understanding that D. trenchii was the most thermal resistant among these species. These results suggest that the binding affinity of the PRR PdGLT-1 may determine the specificity of host-symbiont pairing and explain why Cladocopium is the dominant symbionts of coral P. damicornis at normal temperature, and corals with Durusdinium symbionts may survive better at high temperature.
Introduction
Due to the unrelentless global warming, coral reefs are facing the danger of massive bleaching predicted for the end of this century (Hoegh-Guldberg et al., 2007; Magel et al., 2019; Weis, 2019). Thus, there is an urgent need to preserve the remaining reefs and help restore the lost or degraded ones. The establishment and maintenance of coral-Symbiodiniaceae symbiosis are of vital importance for the coral holobiont functioning, which is initiated by the chemical recognition between the coral host and the Symbiodiniaceae (Berkelmans and van Oppen, 2006; Iguchi et al., 2011; Bellantuono et al., 2012; Kita et al., 2015). The cell surface of Symbiodiniaceae is populated with glycoconjugates, such as mannose-mannose and galactose-β(1-4)-N-acetylglucosamine (Markell et al., 1992; Tortorelli et al., 2021). These glycoconjugates, which form the microbe-associated molecular patterns (MAMPs), can be recognized by the pattern recognition receptors (PRRs) such as lectins on the surface of coral cells (Wood-Charlson et al., 2006; Weis et al., 2008). Such glycan-lectin interaction is critical for initiating the coral-Symbiodiniaceae symbiosis (Tortorelli et al., 2021). The adaptive evolution of the symbiont’s glycan structure and the biochemical complementarity between the coral host and its symbionts are believed to determine the host specificity (Lin et al., 2015). For example, it has been shown that glycan ligands on the cell surface of Cladocopium C1f play a role in recognition during the initial contact at the onset of symbiosis with the coral Fungia scutaria larvae (Rodriguez-Lanetty et al., 2004; Wood-Charlson et al., 2006). Lectins in Sinularia lochmodes and Acropora millepora (Millectin) can recognize Symbiodiniaceae through the binding to their D-galactose and mannose (Kvennefors et al., 2008; Jimbo et al., 2013). Also, the recognition of coral host lectin induces the morphological transformation of Symbiodinium (Fransolet et al., 2012). Lectin in the coral Ctenactis echinata mediates the transformation of the flagellated motile form of Symbiodiniaceae into the non-motile coccoid form (Jimbo et al., 2010), a common form of coral symbionts. However, the molecular mechanisms and factors affecting the establishment and maintenance of the symbiosis between corals and Symbiodiniaceae are still poorly understood.
How lectins’ binding affinity to symbiont’s MAMPs differ among different species of Symbiodiniaceae is a fundamental question, and the answer can help address how the host corals selectively recruit symbionts from the surrounding environments. The symbiotic dinoflagellate algae in the family Symbiodiniaceae includes nine Clades named A-I (Pochon and Gates, 2010; LaJeunesse et al., 2018; Gonzalez-Pech et al., 2021). Out of the nine clades, Symbiodinium (Clade A) to Durusdinium (Clade D) are generally associated with the reef-building corals (Baker, 2003; Traylor-Knowles, 2021). Usually, there is one dominant species of Symbiodiniaceae in a host, which shapes the physiological performance of the corals under environmental stress (Silverstein et al., 2012). For instance, Clade D (Durusdinium) has been reported as a thermally tolerant symbionts. The thermal tolerance of coral Acropora millepora increases by 1–1.5°C when the dominant Clade C symbiont (Cladocopium) is replaced by Clade D (Berkelmans and van Oppen, 2006). Thus, to better protect or restore coral reefs under increasing seawater temperature, there is an urgent need to screen for the most heat-resistant coral-Symbiodiniaceae association, which requires a good understanding of the recognition process mediated by coral lectins.
Another critical issue is how environmental variables affect the establishment of the coral-Symbiodiniaceae symbiosis (Stat and Gates, 2011; Suggett and Smith, 2020). Seawater temperature, eutrophication, ocean acidification, and microplastic pollution are the most common factors affecting the health of corals (Hughes et al., 2003; Sully et al., 2019; Barott et al., 2021), among which heat stress is the most severe stressor. Recent studies have implicated lectins in the establishment of coral-Symbiodiniaceae symbiosis under heat stress. For instance, the C-type lectin from P. damicornis was found to be critical for the symbiont acquisition and sequestration under heat stress (Vidal-Dupiol et al., 2009). Furthermore, the galectin in P. damicornis (PdGLT-1) was shown to recognize both pathogenic bacteria and Symbiodiniaceae, and its binding activity was dramatically suppressed by high temperatures (>30°C) (Wu et al., 2019). However, how the binding activities of coral lectins to different genera of Symbiodiniaceae differ under heat stress has never been reported.
This study investigated the binding affinity of a previously identified coral lectin (PdGLT-1) (Wu et al., 2019) to five species from different major genera of the family Symbiodiniaceae. PdGLT-1 was a galectin from P. damicornis, a scleractinian coral belonging to Pocilloporidae, one of the most abundant and widespread corals (Schuttenberg and Hoegh-Guldberg, 2007). The five species of Symbiodiniaceae were Symbiodinium microadriaticum (Clade A), Breviolum minutum (Clade B), Cladocopium goreaui (Clade C), Durusdinium trenchii (Clade D), and Fugacium kawagutii (Clade F), which belong to the symbiont genera in P. damicornis (Cunning et al., 2018; Tang et al., 2018; Lin et al., 2019; Chankong et al., 2020; Li et al., 2021). The binding affinity of PdGLT-1 to these symbiodiniacean species was examined under a gradient of temperatures. Results in this study help us better understand the molecular mechanism underlying the initial establishment of the coral-Symbiodiniaceae symbiosis and lectins’ potential roles in coral survival or recovery after a heat stress-induced bleaching event.
Materials and Methods
Cultures of Symbiodiniacean Species
The five symbiodiniacean species, S. microadriaticum strain CCMP828, B. minutum strain CCMP830, C. goreaui strain CCMP2466, D. trenchii strain CCMP3428, and F. kawagutii strain CCMP2468, were provided by the National Center for Marine Algae and Microbiota (Bigelow Laboratory for Ocean Sciences, East Boothbay, Maine, United States). These strains were cultured in the L1 medium at 25°C in a light incubator (photon flux of 110 μmol photons m–2 s–1) at 12 h/12 h light-dark cycle until logarithmic growth stage.
Preparation of PdGLT-1 Recombinant Protein and Determination of Binding Affinity of PdGLT-1 Recombinant Protein to Symbiodiniaceae
The expression and purification of the recombinant PdGLT-1 protein were carried out as previously reported (Wu et al., 2019). Briefly, the coding gene was isolated from P. damicornis and inserted in-frame into an expression plasmid to yield the recombinant construct PdGLT-1 (pEASY-E1-PdGLT-1), which was transformed into E. coli BL21 (DE3)-Transetta (TransGen, China). Positive transformants were screened using antibiotic resistance and sequencing, and one of them was selected and grown in the LB medium. The recombinant protein (designated rPdGLT-1) with 6 × His-tag was purified by a Ni2+ chelating Sepharose column (GE Healthcare).
In each binding affinity experiment, cells from the five symbiodiniacean species were harvested from 1 mL cultures by centrifugation at 5,000 g for 5 min at 4°C. The cell pellets were suspended in fresh medium and serially diluted to 105, 104, 103, 102, and 10 cells mL–1. From each of these cell suspensions, 300 μL of cell suspension was transferred to a fresh 1.5 mL microfuge tube and co-incubated with 300 μL of 0.1 mg mL–1 rPdGLT-1 at 25°C for 1 h. For the negative control, bovine serum albumin (BSA) was used in place of rPdGLT-1 to be co-incubated with each symbiodiniacean species. After incubation, the algal cells were pelleted by centrifugation at 1,600 g for 6 min to eliminate the unbound proteins. After washing with PBST three times to remove the non-specifically binding rPdGLT-1, the cells were pelleted and resuspended in PBS.
In order to compare the binding affinity of rPdGLT-1 to different symbiodiniacean species, 300 μL of algal cells (104 cells) for each species were co-incubated with 300 μL of 0.2 mg mL–1 rPdGLT-1 at 25°C for 1 h. For the negative control, BSA was used in co-incubation with an even mixture of five symbiodiniacean species. The washing and resuspension were conducted as mentioned above.
The bound rPdGLT-1 on the symbiodiniacean cell surface was dissociated in 50 μL loading buffer by boiling and the resulting protein solution was loaded to a SDS-PAGE gel. A blank control was set up using the loading buffer. After electrophoretic separation, the target protein was transferred to the PVDF membrane at 200 mA for 1.5 h. After blocked for 2 h at 25°C, PVDF membrane was incubated with His-tag mouse monoclonal antibody (1:1,000 dilution, AH367, Beyotime, China) at 4°C overnight, and then incubated with AP-labeled goat-anti-mouse antibody (1:5,000 dilution, A0258, Beyotime, China) at 25°C for 2 h. After each combination, the membrane was washed three times with PBST on a concentrator for 10 min, and the membrane was subjected to processing with BCIP/NBT Alkaline Phosphatase Color Development Kit (C3206, Beyotime, China) at 25°C for 5–30 min until clear bands were observed. The reaction was terminated by adding distilled water to the PVDF membrane, and each band’s optical density was measured using image processing software Image J (1.53c). In the comparison experiment of different symbiodiniacean species, incubation and western blotting were repeated three times (N = 3). The binding activity of rPdGLT-1 to a symbiodiniacean species was defined as the band intensity ratio of that species to S. microadriaticum.
Observation of Binding Site Topology of PdGLT-1 Recombinant Protein on Symbiodiniacean Cells
The binding area of rPdGLT-1 on the five symbiodiniacean species was observed using the immunofluorescence method. In brief, 105 algal cells from each species were resuspended in 200 μL of PBS. They were incubated with 200 μL of 0.1 mg mL–1 rPdGLT-1 at 25°C for 1 h. For negative and blank controls, BSA and PBS were used in place of rPdGLT-1, respectively. After incubation, the algae were washed by PBST three times and then centrifuged at 4,000 g for 5 min to eliminate the unbound protein. Twenty microliters of His-tag mouse monoclonal antibody (AH367, Beyotime, China) diluted at 1:200 was added to resuspend the algal cell pellets. After incubation at 25°C for 1 h and centrifugation at 1,600 g for 6 min, algal cells were washed three times with PBST. Next, algal cells were incubated with 20 μL of Alexa Fluor 488-labeled Goat Anti-Mouse IgG(H + L) diluted at 1:200 (A0428, Beyotime, China) in darkness at 25°C for 1 h. After triple washes with PBST, the algal cells were collected by centrifugation, resuspended in 20 μL of PBS, and observed under the IX71 Olympus fluorescence microscope.
Measurement of the Binding Activity of PdGLT-1 Recombinant Protein Under Different Temperatures
The binding activity of rPdGLT-1 to the five symbiodiniacean species was examined at the temperatures of 20, 25, 30, and 35°C. Briefly, each symbiodiniacean species from stock culture was split into 4 subsamples, and then 300 μL of 105 cells mL–1 of each symbiodiniacean species were incubated with 300 μL of 0.1 mg mL–1 rPdGLT-1 for 1 h at 20, 25, 30, and 35°C. For the negative control, BSA was used instead of rPdGLT-1. Each incubation was conducted in triplicate, and the subsequent SDS-PAGE and western blotting were performed as mentioned above. Loading buffer was used as the blank control. The band intensity of the incubation at a temperature was normalized to that of the incubation at 20°C.
Statistical Analysis
All data were expressed as mean ± standard deviation (SD). Before analysis, data were tested for normality using the Shapiro-Wilk test. When the variance was verified to be homogeneous, data were subjected to one-way analysis of variance (ANOVA) followed by multiple comparisons (S-N-K) to evaluate the significance of differences among different symbiodiniacean species or temperatures by using software IBM SPSS 20.0 (IBM, Inc.). Differences were considered significant at p < 0.05.
Results
Comparison of Binding Affinity of PdGLT-1 Recombinant Protein Among Symbiodiniacean Species
A binding band was observed for each of the five symbiodiniacean species examined at 25°C, and the band intensity increased with increasing algal concentration from 10 to 105 cells mL–1, especially for B. minutum and D. trenchii (Figure 1). The highest relative band intensity (2.6-fold) was observed in F. kawagutii, which exhibited a significantly higher binding affinity than other symbiodiniacean species (one-way ANOVA, F = 22.841, p < 0.05). C. goreaui exhibited the second highest band intensity, 1.9-fold higher than that of S. microadriaticum, and 1.4-fold higher than that of D. trenchii (p < 0.05 in both cases). No significant difference was found among S. microadriaticum, B. minutum, and D. trenchii. There were no detectable bands in the negative or blank control (Figure 2).
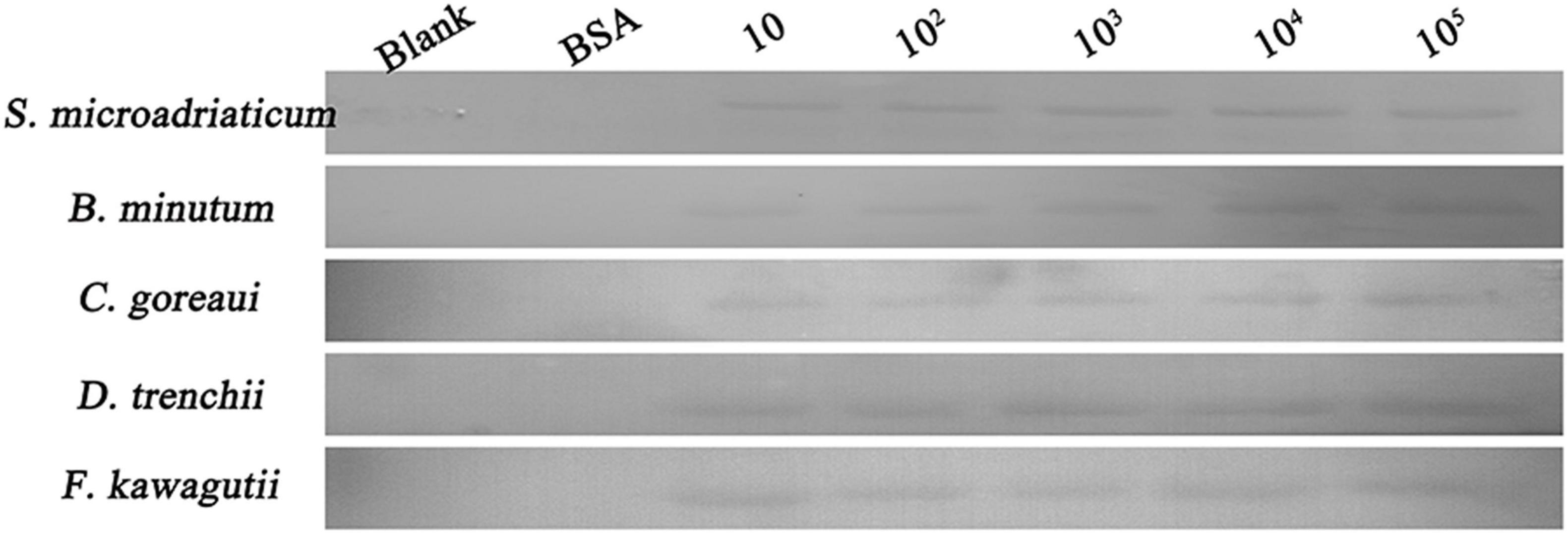
Figure 1. Binding activity of PdGLT-1 recombinant protein to five symbiodiniacean species of different concentrations (10, 102, 103, 104, and 105 cells mL–1) at 25°C, including S. microadriaticum, B. minutum, C. goreaui, D. trenchii, and F. kawagutii. BSA (bovine serum albumin) is employed as the negative control, while loading buffer is used as blank control.
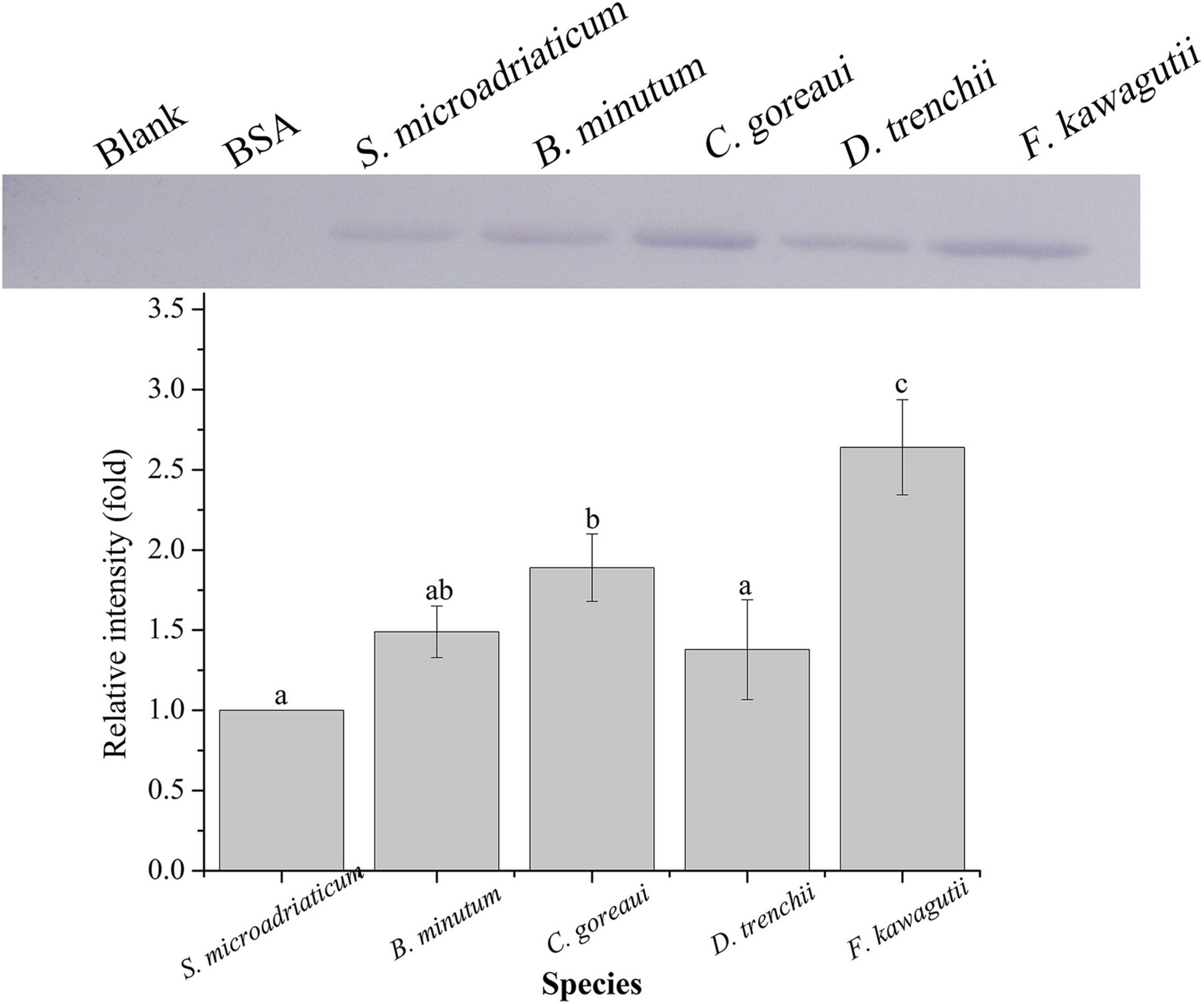
Figure 2. Binding of PdGLT-1 recombinant protein to S. microadriaticum, B. minutum, C. goreaui, D. trenchii, and F. kawagutii at 25°C. The figure above shows the binding band obtained by western blotting, and the figure below shows the gray scale ratio. The gray scale of the binding band of S. microadriaticum is used as the basis to calculate the gray scale ratio. The column represents mean ± standard deviation (N = 3), and the column with different letters represents significant difference (p < 0.05).
PdGLT-1 Recombinant Protein Binding Site Topology on Symbiodiniacean Cells
For C. goreaui and F. kawagutii, clear positive signals were widespread over the whole cell surface, and rPdGLT-1 bound F. kawagutii cells were agglutinated. In contrast, the positive signals were concentrated in smaller discrete regions on the cell surface of S. microadriaticum, B. minutum, and D. trenchii. No positive signals were observed in the negative (BSA) and blank (PBS) control (Figure 3).
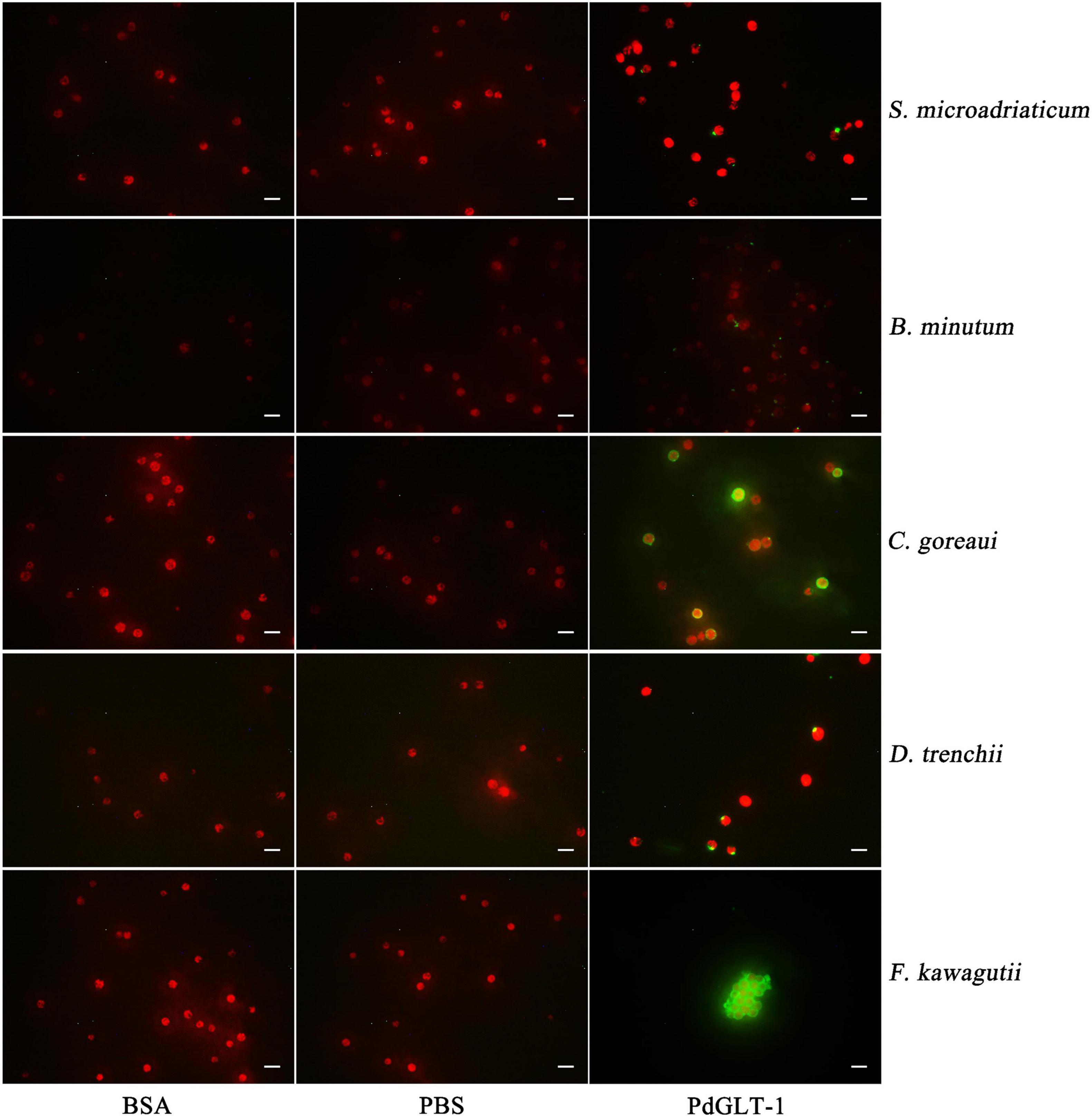
Figure 3. The binding region of rPdGLT-1 to S. microadriaticum, B. minutum, C. goreaui, D. trenchii, and F. kawagutii at 25°C through immunofluorescence method. His-tag mouse monoclonal antibody and Alexa Fluor 488-labeled Goat Anti-Mouse IgG(H + L) are used as primary and second antibodies, respectively. BSA is employed as negative control and PBS as blank control. Scale-bars are 20 μm.
Effects of Temperature on the Binding Affinity of PdGLT-1 Recombinant Protein
The relative band intensity of rPdGLT-1 bound to D. trenchii was highest at 35°C (1.5-fold), which was significantly higher than that at 30°C (1.4-fold; one-way ANOVA, F = 38.712, p < 0.05). The lowest intensities occurred at 20 and 25°C (1.0-fold, 1.0-fold, respectively), which were significantly lower (p < 0.05) than that at 30°C (Figure 4). The relative band intensity of rPdGLT-1 to S. microadriaticum was highest at 30°C (1.8-fold), slightly lower at 35°C (1.7-fold). The relative band intensities at 20 and 25°C were markedly lower, between which that at 25°C was significantly higher (1.4-fold; one-way ANOVA, F = 44.272, p < 0.05) than that at 20°C (Figure 5). Similar to S. microadriaticum, the highest band intensity of C. goreaui-bound rPdGLT-1 was detected at 30°C, which was 1.3-fold of that at 20°C, and significantly higher (one-way ANOVA, F = 9.062, p < 0.05) than those at 20, 25, and 35°C (1.1- and 1.0-fold; Figure 6). The highest relative band intensity of rPdGLT-1 bound to F. kawagutii also occurred at 30°C (1.5-fold), which was higher than that at 20°C (one-way ANOVA, F = 5.183, p < 0.05). The relative band intensities at 25 and 35°C (1.2- and 1.3-fold, respectively) were between that at 20 and 30°C, with no significant differences (Figure 7). In contrast, the highest relative band intensity of rPdGLT-1 bound to B. minutum was observed at 20°C, the lowest temperature among the five species examined. It was significantly higher than those at 25 and 35°C (one-way ANOVA, F = 4.555, p < 0.05). Furthermore, the band intensity at 30°C (0.8-fold) appeared to be higher than those at 25 and 35°C, but there were no significant differences among the three temperatures (Figure 8).
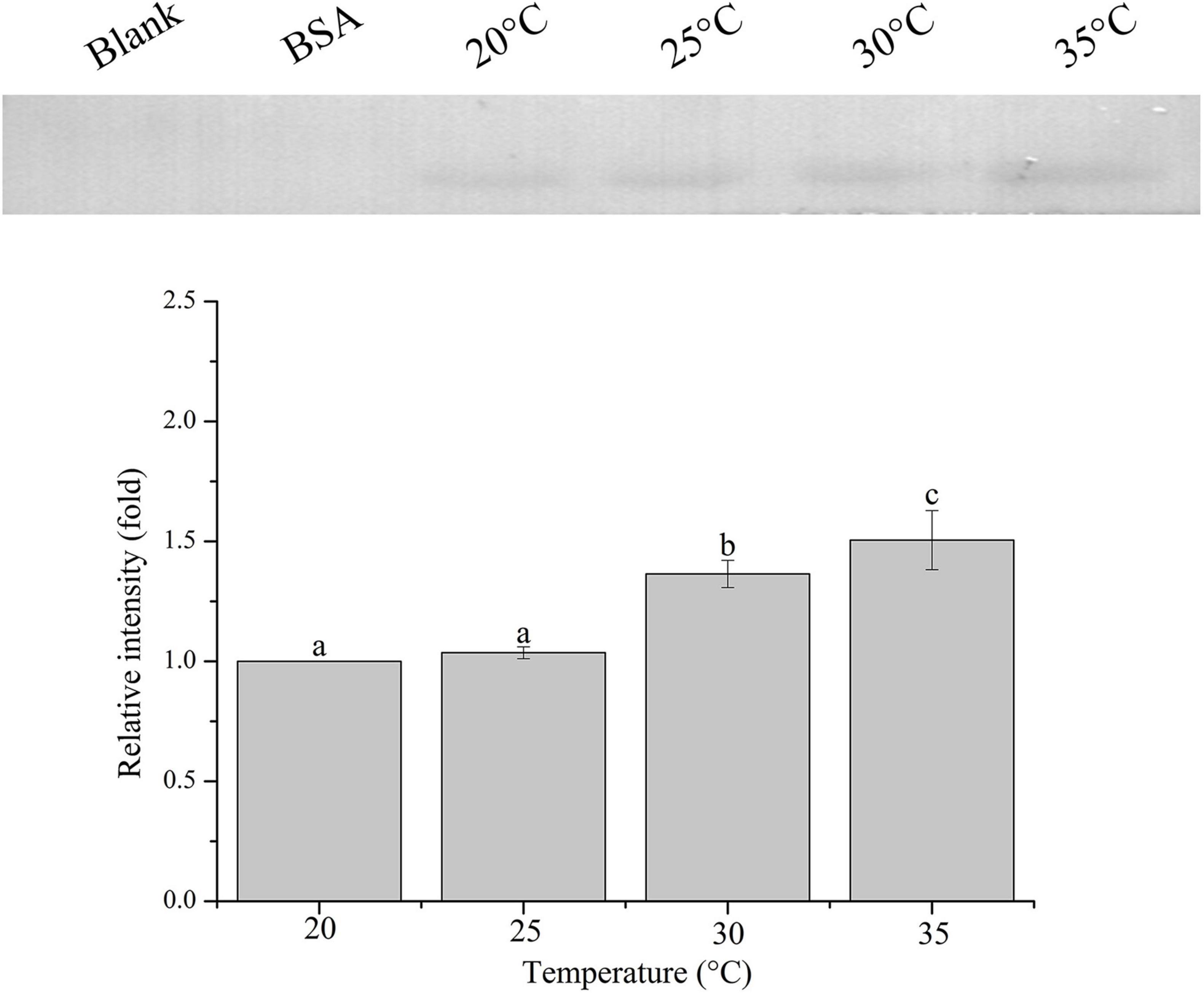
Figure 4. Binding of PdGLT-1 recombinant protein to D. trenchii at different temperatures. The figure above shows the binding band obtained by western blotting, and the figure below shows the gray ratio. The gray scale of the binding band of 20°C is used as the basis to calculate the gray scale ratio. The column represents mean ± standard deviation (N = 3), and the column with different letters represents significant difference (p < 0.05).
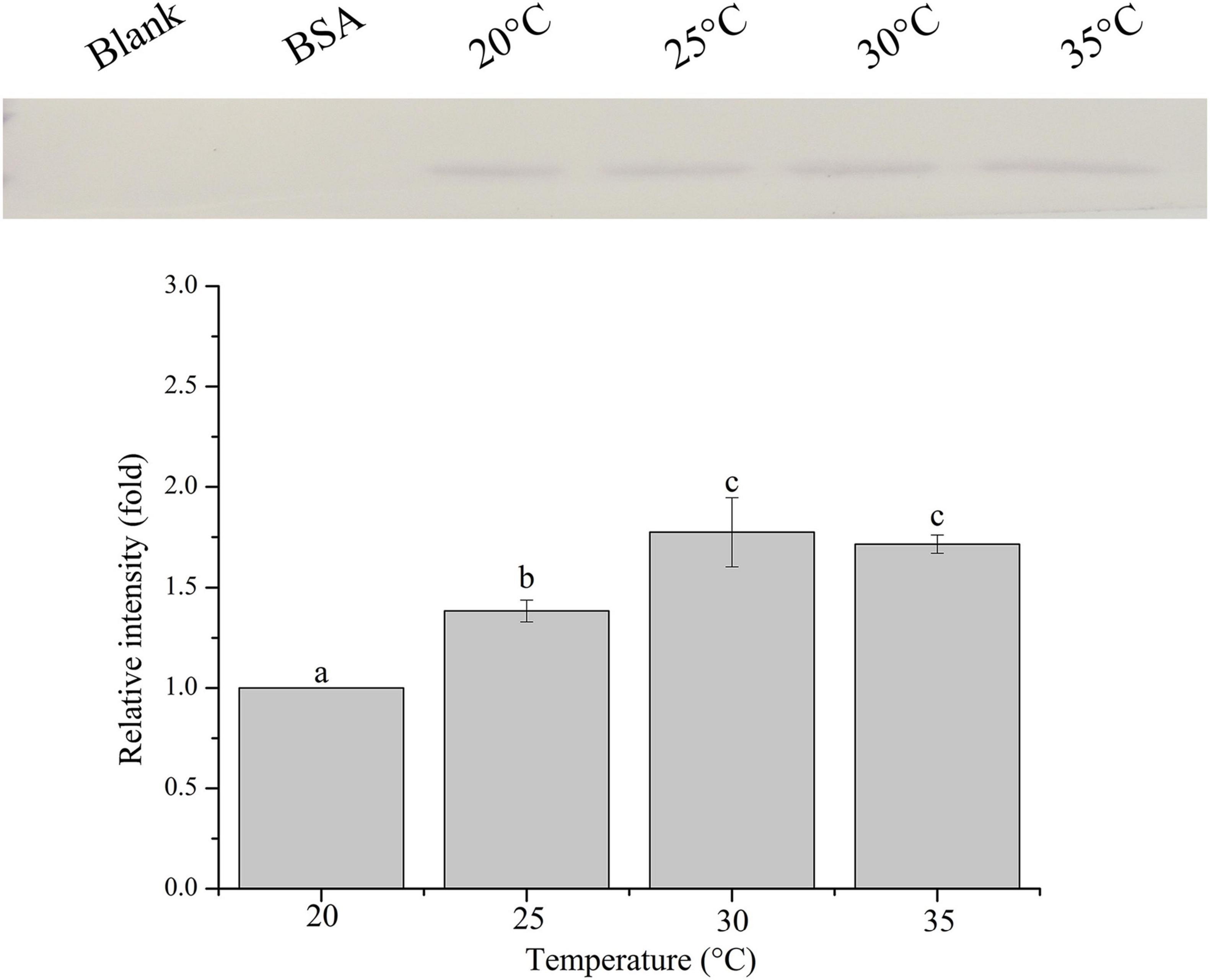
Figure 5. Binding of PdGLT-1 recombinant protein to S. microadriaticum at different temperatures. The figure above shows the binding band obtained by western blotting, and the figure below shows the gray ratio. The gray scale of the binding band of 20°C is used as the basis to calculate the gray scale ratio. The column represents mean ± standard deviation (N = 3), and the column with different letters represents significant difference (p < 0.05).
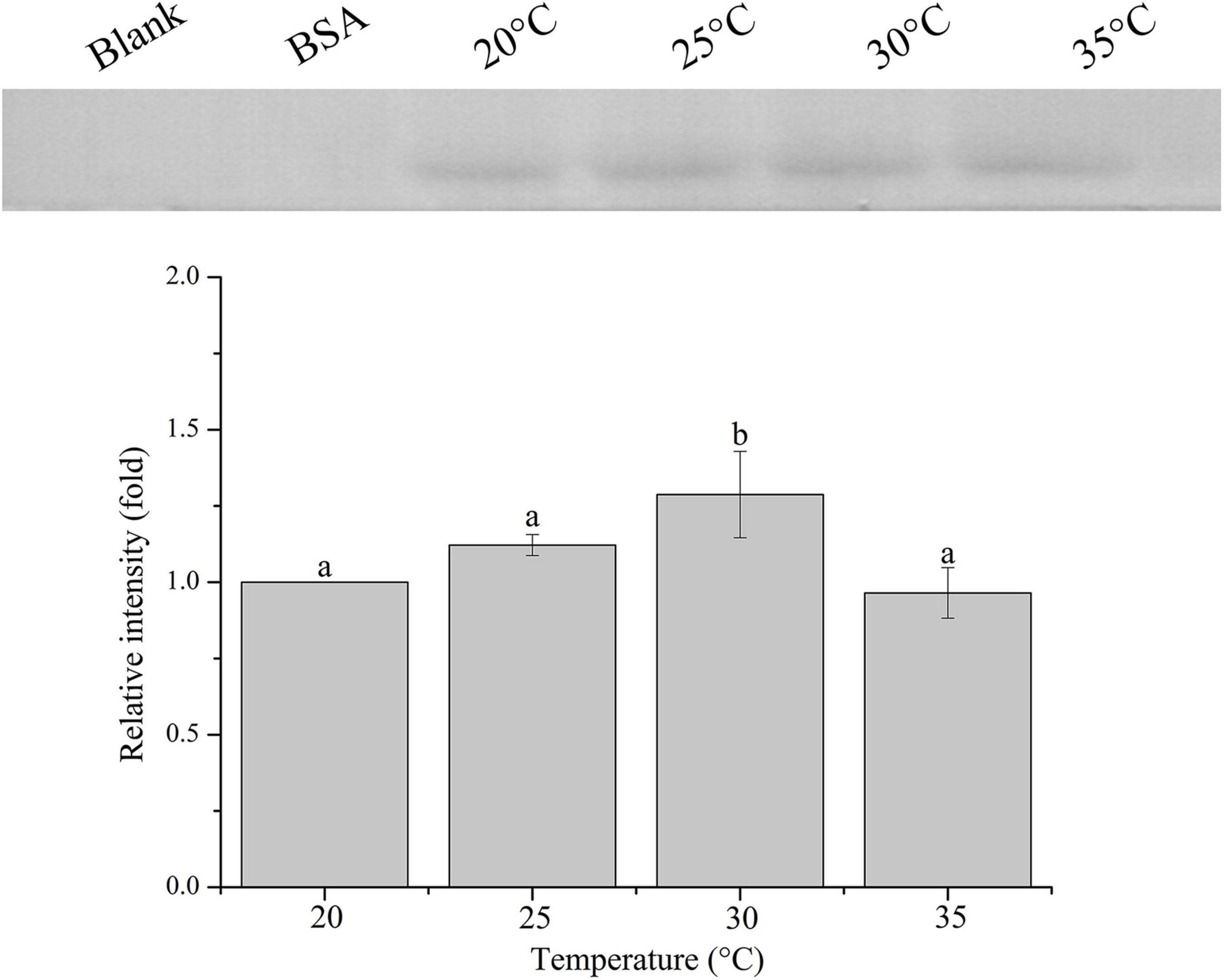
Figure 6. Binding of PdGLT-1 recombinant protein to C. goreaui at different temperatures. The figure above shows the binding band obtained by western blotting, and the figure below shows the gray ratio. The gray scale of the binding band of 20°C is used as the basis to calculate the gray scale ratio. The column represents mean ± standard deviation (N = 3), and the column with different letters represents significant difference (p < 0.05).
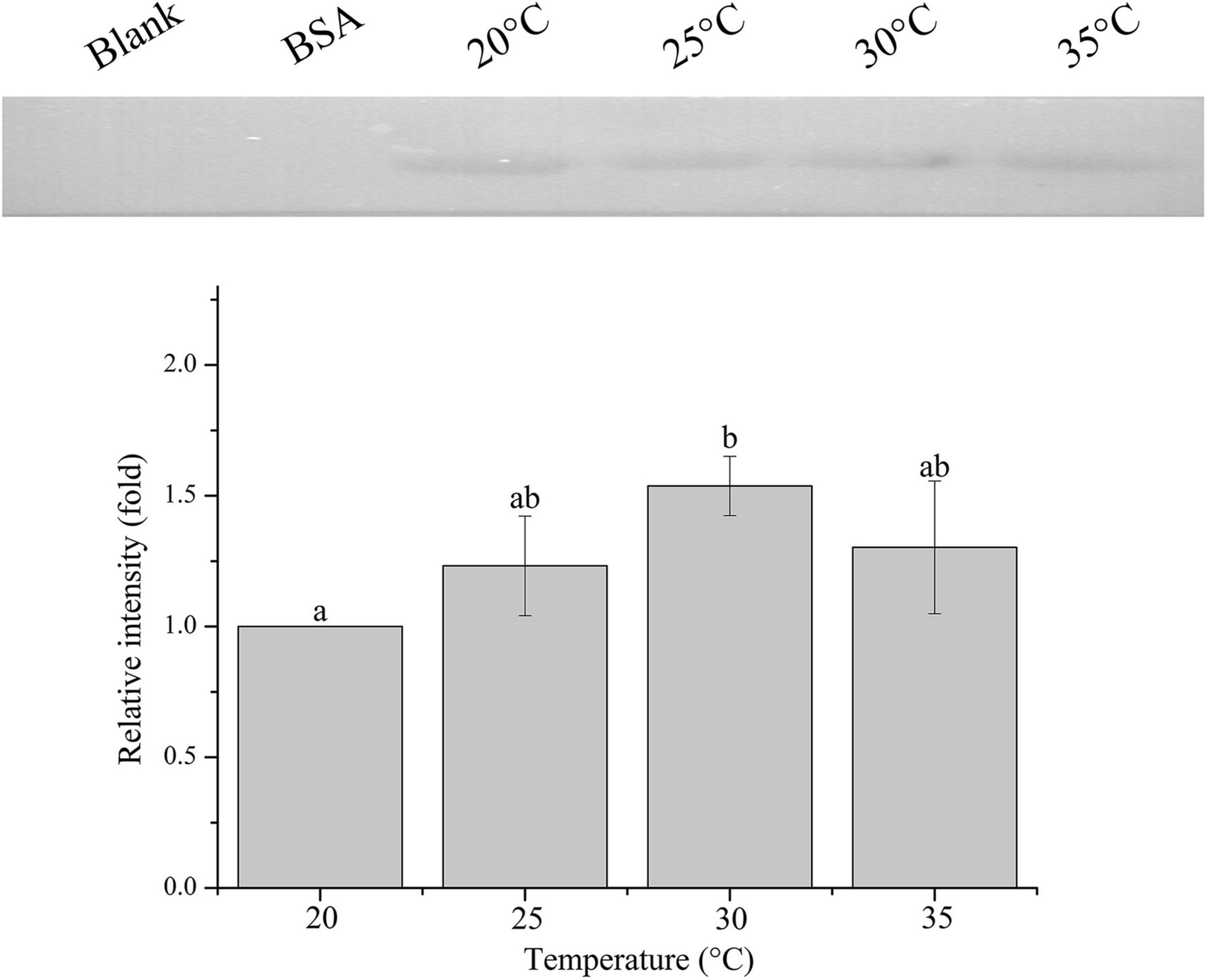
Figure 7. Binding of PdGLT-1 recombinant protein to F. kawagutii at different temperatures. The figure above shows the binding band obtained by western blotting, and the figure below shows the gray ratio. The gray scale of the binding band of 20°C is used as the basis to calculate the gray scale ratio. The column represents mean ± standard deviation (N = 3), and the column with different letters represents significant difference (p < 0.05).
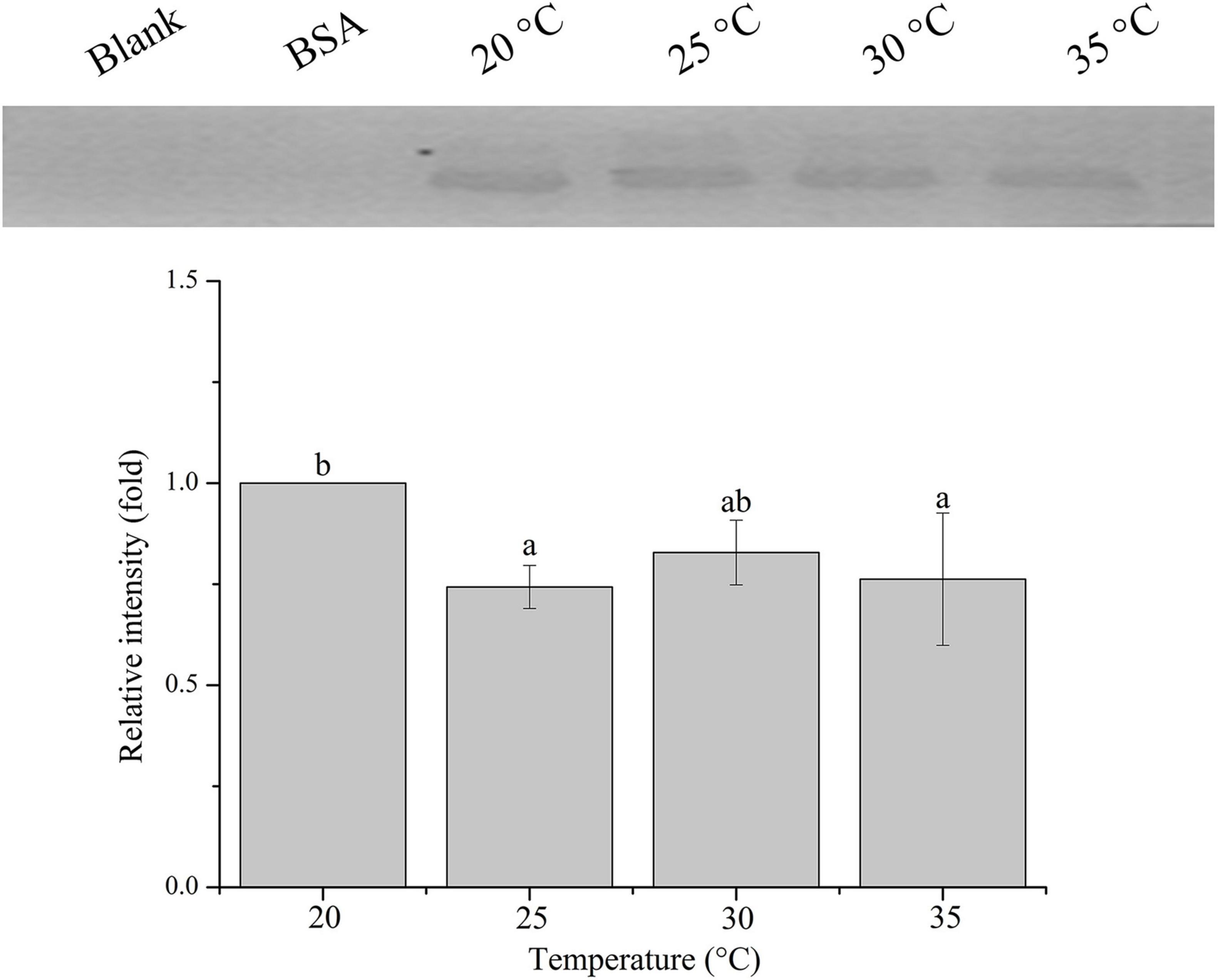
Figure 8. Binding of PdGLT-1 recombinant protein to B. minutum at different temperatures. The figure above shows the binding band obtained by western blotting, and the figure below shows the gray ratio. The gray scale of the binding band of 20°C is used as the basis to calculate the gray scale ratio. The column represents mean ± standard deviation (N = 3), and the column with different letters represents significant difference (p < 0.05).
Discussion
The establishment of the coral-Symbiodiniaceae symbiosis is essential to the prosperity of the coral reef ecosystem (Wood-Charlson et al., 2006). Recognition between corals and Symbiodiniaceae is the very first and critical step, which requires the involvement of corals’ lectins such as C-type lectins, galectins, rhamnose-binding lectins, and D-Galactose-binding lectins (Zhou et al., 2017, 2018; Weis, 2019; Wu et al., 2019). Besides, the binding of lectins to Symbiodiniaceae can be affected by temperature (Vidal-Dupiol et al., 2009; Bellantuono et al., 2012; Wu et al., 2019), and high temperature might decrease the binding affinity of coral lectins and further suppress their abilities to acquire and sequestrate the free Symbiodiniaceae to repopulate the bleached coral (Vidal-Dupiol et al., 2009). Therefore, information on the differential binding affinity of coral lectins to different genera of Symbiodiniaceae, and the differential effects of temperature on the binding capacity, is essential to understanding the underlying regulatory mechanism for the initial establishment of coral-Symbiodiniaceae symbiosis.
In the present study, a previously identified lectin (PdGLT-1) was expressed in E. coli, purified, and used to investigate the recognition of the coral P. damicornis to five species of Symbiodiniaceae. The results showed that rPdGLT-1 could bind to all five species of Symbiodiniaceae. Recognizable by PdGLT-1, these algae have the potential to establish symbiosis with P. damicornis. Consistent with this finding, high throughput sequencing studies on the symbiont communities of P. damicornis have indicated the presence of these five genera in this species of coral (Boulotte et al., 2016). Besides, the present study reported the differential binding affinities of PdGLT-1 to different symbiodiniacean species. A higher binding affinity was found to C. goreaui and F. kawagutii than S. microadriaticum, B. minutum, and D. trenchii. This result agrees with the mainstream academic view that clade C is the dominant Symbiodiniaceae in P. damicornis (Magalon et al., 2007).
To further explore the recognition characteristics between PdGLT-1 and Symbiodiniaceae, differences among five species of Symbiodiniaceae in the rPdGLT-1 binding pattern were carefully examined using immunofluorescence. Interestingly, rPdGLT-1 was generally bound to discrete loci on the surface of S. microadriaticum, B. minutum, and D. trenchii, while the binding sites of rPdGLT-1 to C. goreaui and F. kawagutii were widespread over the cell surface. These results were consistent with the higher binding affinity to C. goreaui and F. kawagutii measured by Western blotting. Intriguingly, rPdGLT-1 not only exhibited a higher binding affinity to F. kawagutii but also had an agglutinating effect (Figure 2). This finding suggests that PdGLT-1 is more likely to induce agglutination than endosymbiosis in F. kawagutii, which explains why F. kawagutii is not a dominant symbiont species in P. damicornis or other corals, and its role as an endosymbiont remains obscure. On the contrary, PdGLT-1 had high binding activity to C. goreaui but hardly with any agglutination effect. As such PdGLT-1 is more likely to induce symbiosis with C. goreaui than agglutination, consistent with the reports that Cladopodium spp. are dominant Symbiodiniaceae in P. damicornis (Magalon et al., 2007).
Furthermore, the establishment and maintenance of the coral-Symbiodiniaceae symbiosis are strongly influenced by temperature, the severe coral bleaching in recent years have been attributed to heat stress (Sampayo et al., 2008; Stat and Gates, 2011; Klepac and Barshis, 2020). From the PdGLT-1 binding in five temperature (20, 25, 30, and 35°C) experiments, clear species-specific optimal PdGLT-1 binding temperature was noted. Interestingly, the highest optimal temperature (35°C) was found in D. trenchii (Clade D), the second highest (30°C) in S. microadriaticum (Clade A), C. goreaui (Clade C), and F. kawagutii (Clade F), and the lowest (20°C) was found in B. minutum (Clade B). These results suggest that coral lectins have variable potentials to acquire and sequestrate Symbiodiniaceae in a temperature-dependent manner. This might affect the differential repopulation of the symbionts in the bleached corals.
Accumulating evidence has indicated that a higher relative abundance of Clade D Symbiodiniaceae in corals contributes to the heat resistance of corals, and Clade D Symbiodiniaceae are regarded as the as most heat-tolerant clade among all Symbiodiniaceae clades (Abrego et al., 2009; Keshavmurthy et al., 2017; Thinesh et al., 2019). Moreover, symbionts type-switching from Clades A, B, C, or F to Clade D following a bleaching event is believed to be the strategy of corals to recover from bleaching (Thinesh et al., 2019). Results in the present study show that the binding affinity of PdGLT-1 to D. trenchii remains relatively high under thermal stress (35°C). This property may facilitate symbiosis establishment of P. damicornis with Clade D Symbiodiniaceae under high temperatures and the survival of the holobiont after thermal stress. This should be verified in the future using infection experiments.
In summary, the binding affinity of coral lectin PdGLT-1 to five genera of Symbiodiniaceae was investigated to shed light on the molecular recognition for symbiosis establishment. PdGLT-1 exhibits a greater binding affinity to C. goreaui and F. kawagutii than B. minutum, D. trenchii, and S. microadriaticum, implying a higher likelihood for those two species to be recruited by P. damicornis as symbionts. However, as PdGLT-1 binding induces cell agglutination in F. kawagutii, the potential of F. kawagutii to become symbionts is undermined. These findings explain why C. goreaui is the most dominant Symbiodiniaceae in P. damicornis. Furthermore, we find that the PdGLT-1’s binding affinity to symbiodiniacean cells was affected by temperature, and the optimal binding temperature differed with species. The high binding affinity of PdGLT-1 to D. trenchii (thermal-tolerant Symbiodiniaceae) under heat stress (35°C) can explain why corals with D. trenchii as dominant symbionts are more heat-resistant.
Data Availability Statement
The original contributions presented in the study are included in the article/supplementary material, further inquiries can be directed to the corresponding author/s.
Author Contributions
XW and ZZ conceived, designed the experiments, and analyzed the data. XW, YW, and MA performed the experiments. ZW and ZZ contributed to the reagents, materials, and analysis tools. XW, ZZ, SL, and ZW contributed to the discussion and wrote the manuscript. All authors read and approved the final manuscript.
Funding
This research was supported by grants from the Hainan Provincial Natural Science Foundation of China (Grant Nos. 2019RC067 and 420CXTD432), the National Natural Science Foundation of China (Grant Nos. 31772460 and 42076145), and the Major Science and Technology Program of Hainan Province (Grant No. ZDKJ2019011).
Conflict of Interest
The authors declare that the research was conducted in the absence of any commercial or financial relationships that could be construed as a potential conflict of interest.
Publisher’s Note
All claims expressed in this article are solely those of the authors and do not necessarily represent those of their affiliated organizations, or those of the publisher, the editors and the reviewers. Any product that may be evaluated in this article, or claim that may be made by its manufacturer, is not guaranteed or endorsed by the publisher.
Acknowledgments
We were grateful to all of the laboratory members for their continuous technical advice and helpful discussions.
References
Abrego, D., van Oppen, M. J. H., and Willis, B. L. (2009). Onset of algal endosymbiont specificity varies among closely related species of Acropora corals during early ontogeny. Mol. Ecol. 18, 3532–3543. doi: 10.1111/j.1365-294X.2009.04276.x
Baker, A. C. (2003). Flexibility and specificity in coral-algal symbiosis: diversity, ecology, and biogeography of Symbiodinium. Annu. Rev. Ecol. Evol. Syst. 34, 661–689.
Barott, K. L., Huffmyer, A. S., Davidson, J. M., Lenz, E. A., Matsuda, S. B., Hancock, J. R., et al. (2021). Coral bleaching response is unaltered following acclimatization to reefs with distinct environmental conditions. Proc. Natl. Acad. Sci. U.S.A. 118:e2025435118. doi: 10.1073/pnas.2025435118
Bellantuono, A. J., Granados-Cifuentes, C., Miller, D. J., Hoegh-Guldberg, O., and Rodriguez-Lanetty, M. (2012). Coral thermal tolerance: tuning gene expression to resist thermal stress. PLoS One 7:e50685. doi: 10.1371/journal.pone.0050685
Berkelmans, R., and van Oppen, M. J. (2006). The role of zooxanthellae in the thermal tolerance of corals: a ‘nugget of hope’ for coral reefs in an era of climate change. Proc. Biol. Sci. 273, 2305–2312. doi: 10.1098/rspb.2006.3567
Boulotte, N. M., Dalton, S. J., Carroll, A. G., Harrison, P. L., Putnam, H. M., Peplow, L. M., et al. (2016). Exploring the Symbiodinium rare biosphere provides evidence for symbiont switching in reef-building corals. ISME J. 10, 2693–2701. doi: 10.1038/ismej.2016.54
Chankong, A., Kongjandtre, N., Senanan, W., and Manthachitra, V. (2020). Community composition of Symbiodiniaceae among four scleractinian corals in the eastern Gulf of Thailand. Reg. Stud. Mar. Sci. 33:100918.
Cunning, R., Bay, R. A., Gillette, P., Baker, A. C., and Traylor-Knowles, N. (2018). Comparative analysis of the Pocillopora damicornis genome highlights role of immune system in coral evolution. Sci. Rep. 8:16134. doi: 10.1038/s41598-018-34459-8
Fransolet, D., Roberty, S., and Plumier, J.-C. (2012). Establishment of endosymbiosis: the case of cnidarians and Symbiodinium. J. Exp. Mar. Biol. 42, 1–7.
Gonzalez-Pech, R. A., Stephens, T. G., Chen, Y., Mohamed, A. R., Cheng, Y., Shah, S., et al. (2021). Comparison of 15 dinoflagellate genomes reveals extensive sequence and structural divergence in family Symbiodiniaceae and genus Symbiodinium. BMC Biol. 19:73. doi: 10.1186/s12915-021-00994-6
Hoegh-Guldberg, O., Mumby, P. J., Hooten, A. J., Steneck, R. S., Greenfield, P., Gomez, E., et al. (2007). Coral reefs under rapid climate change and ocean acidification. Science 318, 1737–1742. doi: 10.1126/science.1152509
Hughes, T. P., Baird, A. H., Bellwood, D. R., Card, M., Connolly, S. R., Folke, C., et al. (2003). Climate change, human impacts, and the resilience of coral reefs. Science 301, 929–933. doi: 10.1126/science.1085046
Iguchi, A., Shinzato, C., Foret, S., and Miller, D. J. (2011). Identification of fast-evolving genes in the scleractinian coral Acropora using comparative EST analysis. PLoS One 6:e20140. doi: 10.1371/journal.pone.0020140
Jimbo, M., Suda, Y., Koike, K., Nakamura-Tsuruta, S., Kominami, J., Kamei, M., et al. (2013). Possible involvement of glycolipids in lectin-mediated cellular transformation of symbiotic microalgae in corals. J. Exp. Mar. Biol. Ecol. 439, 129–135. doi: 10.1016/j.jembe.2012.10.022
Jimbo, M., Yamashita, H., Koike, K., Sakai, R., and Kamiya, H. (2010). Effects of lectin in the scleractinian coral Ctenactis echinata on symbiotic zooxanthellae. Fish. Sci. 76, 355–363. doi: 10.1007/s12562-009-0204-z
Keshavmurthy, S., Tang, K. H., Hsu, C. M., Gan, C. H., Kuo, C. Y., Soong, K., et al. (2017). Symbiodinium spp. associated with scleractinian corals from Dongsha Atoll (Pratas), Taiwan, in the South China Sea. PeerJ 5:e2871. doi: 10.7717/peerj.2871
Kita, A., Jimbo, M., Sakai, R., Morimoto, Y., and Miki, K. (2015). Crystal structure of a symbiosis-related lectin from octocoral. Glycobiology 25, 1016–1023. doi: 10.1093/glycob/cwv033
Klepac, C. N., and Barshis, D. J. (2020). Reduced thermal tolerance of massive coral species in a highly variable environment. Proc. Biol. Sci. 287:20201379. doi: 10.1098/rspb.2020.1379
Kvennefors, E. C., Leggat, W., Hoegh-Guldberg, O., Degnan, B. M., and Barnes, A. C. (2008). An ancient and variable mannose-binding lectin from the coral Acropora millepora binds both pathogens and symbionts. Dev. Comp. Immunol. 32, 1582–1592. doi: 10.1016/j.dci.2008.05.010
LaJeunesse, T. C., Parkinson, J. E., Gabrielson, P. W., Jeong, H. J., Reimer, J. D., Voolstra, C. R., et al. (2018). Systematic revision of Symbiodiniaceae highlights the antiquity and diversity of coral endosymbionts. Curr. Biol. 28, 2570–2580e2576. doi: 10.1016/j.cub.2018.07.008
Li, J., Long, L., Zou, Y., and Zhang, S. (2021). Microbial community and transcriptional responses to increased temperatures in coral Pocillopora damicornis holobiont. Environ. Microbiol. 23, 826–843. doi: 10.1111/1462-2920.15168
Lin, S., Cheng, S., Song, B., Zhong, X., Lin, X., Li, W., et al. (2015). The genome of Symbiodinium kawagutii illuminates dinoflagellate gene expression and coral symbiosis. Science 350, 691–694. doi: 10.1126/science.aad0408
Lin, S., Yu, L., and Zhang, H. (2019). Transcriptomic responses to thermal stress and varied phosphorus conditions in Fugacium kawagutii. Microorganisms 7:96. doi: 10.3390/microorganisms7040096
Magalon, H., Flot, J. F., and Baudry, E. (2007). Molecular identification of symbiotic dinoflagellates in Pacific corals in the genus Pocillopora. Coral Reefs 26, 551–558. doi: 10.1007/s00338-007-0215-0
Magel, J. M. T., Burns, J. H. R., Gates, R. D., and Baum, J. K. (2019). Effects of bleaching-associated mass coral mortality on reef structural complexity across a gradient of local disturbance. Sci. Rep. 9:2512. doi: 10.1038/s41598-018-37713-1
Markell, D. A., Trench, R. K., and Iglesias-Prieto, R. (1992). Macromolecules associated with the cell walls of symbiotic dinoflagellates. Symbiosis 12, 19–31.
Pochon, X., and Gates, R. D. (2010). A new Symbiodinium clade (Dinophyceae) from soritid foraminifera in Hawai’i. Mol. Phylogenet. Evol. 56, 492–497. doi: 10.1016/j.ympev.2010.03.040
Rodriguez-Lanetty, M., Krupp, D. A., and Weis, V. M. (2004). Distinct ITS types of Symbiodinium in Clade C correlate with cnidarian/dinoflagellate specificity during onset of symbiosis. Mar. Ecol. Prog. Ser. 275, 97–102.
Sampayo, E. M., Ridgway, T., Bongaerts, P., and Hoegh-Guldberg, O. (2008). Bleaching susceptibility and mortality of corals are determined by fine-scale differences in symbiont type. Proc. Natl. Acad. Sci. U.S.A. 105, 10444–10449. doi: 10.1073/pnas.0708049105
Schuttenberg, H., and Hoegh-Guldberg, O. (2007). A world with corals: what will it take? Science 318:42. doi: 10.1126/science.318.5847.42b
Silverstein, R. N., Correa, A. M., and Baker, A. C. (2012). Specificity is rarely absolute in coral-algal symbiosis: implications for coral response to climate change. Proc. Biol. Sci. 279, 2609–2618. doi: 10.1098/rspb.2012.0055
Stat, M., and Gates, R. D. (2011). Clade D Symbiodinium in scleractinian corals: a “Nugget” of hope, a selfish opportunist, an ominous sign, or all of the above? J. Mar. Sci. 2011:730715.
Suggett, D. J., and Smith, D. J. (2020). Coral bleaching patterns are the outcome of complex biological and environmental networking. Glob. Change Biol. 26, 68–79. doi: 10.1111/gcb.14871
Sully, S., Burkepile, D. E., Donovan, M. K., Hodgson, G., and van Woesik, R. (2019). A global analysis of coral bleaching over the past two decades. Nat. Commun. 10:1264. doi: 10.1038/s41467-019-09238-2
Tang, J., Ni, X., Zhou, Z., Wang, L., and Lin, S. (2018). Acute microplastic exposure raises stress response and suppresses detoxification and immune capacities in the scleractinian coral Pocillopora damicornis. Environ. Pollut. 243(Pt A), 66–74. doi: 10.1016/j.envpol.2018.08.045
Thinesh, T., Meenatchi, R., Jose, P. A., Kiran, G. S., and Selvin, J. (2019). Differential bleaching and recovery pattern of southeast Indian coral reef to 2016 global mass bleaching event: occurrence of stress-tolerant symbiont Durusdinium (Clade D) in corals of Palk Bay. Mar. Pollut. Bull. 145, 287–294. doi: 10.1016/j.marpolbul.2019.05.033
Tortorelli, G., Rautengarten, C., Bacic, A., Segal, G., Ebert, B., Davy, S. K., et al. (2021). Cell surface carbohydrates of symbiotic dinoflagellates and their role in the establishment of cnidarian-dinoflagellate symbiosis. ISME J. 1–10. doi: 10.1038/s41396-021-01059-w
Traylor-Knowles, N. (2021). Unlocking the single-cell mysteries of a reef-building coral. Cell 184, 2802–2804. doi: 10.1016/j.cell.2021.05.007
Vidal-Dupiol, J., Adjeroud, M., Roger, E., Foure, L., Duval, D., Mone, Y., et al. (2009). Coral bleaching under thermal stress: putative involvement of host/symbiont recognition mechanisms. BMC Physiol. 9:14. doi: 10.1186/1472-6793-9-14
Weis, V. M. (2019). Cell biology of coral symbiosis: foundational study can inform solutions to the coral reef crisis. Integr. Comp. Biol. 59, 845–855. doi: 10.1093/icb/icz067
Weis, V. M., Davy, S. K., Hoegh-Guldberg, O., Rodriguez-Lanetty, M., and Pringle, J. R. (2008). Cell biology in model systems as the key to understanding corals. Trends Ecol. Evol. 23, 369–376. doi: 10.1016/j.tree.2008.03.004
Wood-Charlson, E. M., Hollingsworth, L. L., Krupp, D. A., and Weis, V. M. (2006). Lectin/glycan interactions play a role in recognition in a coral/dinoflagellate symbiosis. Cell. Microbiol. 8, 1985–1993. doi: 10.1111/j.1462-5822.2006.00765.x
Wu, Y., Zhou, Z., Wang, J., Luo, J., Wang, L., and Zhang, Y. (2019). Temperature regulates the recognition activities of a galectin to pathogen and symbiont in the scleractinian coral Pocillopora damicornis. Dev. Comp. Immunol. 96, 103–110. doi: 10.1016/j.dci.2019.03.003
Zhou, Z., Yu, X., Tang, J., Zhu, Y., Chen, G., Guo, L., et al. (2017). Dual recognition activity of a rhamnose-binding lectin to pathogenic bacteria and zooxanthellae in stony coral Pocillopora damicornis. Dev. Comp. Immunol. 70, 88–93. doi: 10.1016/j.dci.2017.01.009
Keywords: scleractinian coral, symbiosis establishment, Symbiodiniaceae, heat stress, galectin
Citation: Wang X, Wu Z, Wu Y, An M, Zhou Z and Lin S (2021) Differential Affinities of a Pocillopora damicornis Galectin to Five Genera of Symbiodiniaceae at Different Temperatures. Front. Mar. Sci. 8:754808. doi: 10.3389/fmars.2021.754808
Received: 07 August 2021; Accepted: 06 December 2021;
Published: 24 December 2021.
Edited by:
Ranjeet Bhagooli, University of Mauritius, MauritiusReviewed by:
Beatriz Casareto, Shizuoka University, JapanWalter Dellisanti, Hong Kong Polytechnic University, Hong Kong SAR, China
Copyright © 2021 Wang, Wu, Wu, An, Zhou and Lin. This is an open-access article distributed under the terms of the Creative Commons Attribution License (CC BY). The use, distribution or reproduction in other forums is permitted, provided the original author(s) and the copyright owner(s) are credited and that the original publication in this journal is cited, in accordance with accepted academic practice. No use, distribution or reproduction is permitted which does not comply with these terms.
*Correspondence: Zhongjie Wu, d3V6akBobmhreS5jbg==; Zhi Zhou, emhvdXpoaUBoYWluYW51LmVkdS5jbg==