- 1Department of Marine Sciences and Convergent Technology, College of Science and Convergence Technology, Hanyang University, Ansan, South Korea
- 2NeoEnBiz Co., Bucheon, South Korea
Limited studies have been conducted on polychlorinated naphthalenes (PCNs) in the coastal environment worldwide. In this study, analytical methods were optimized for 18 PCN congeners in sediment using a multi-layer silica gel column and a gas chromatograph coupled to tandem mass spectrometry (GC-MS/MS). The optimized analytical methods of PCNs were employed for sediment samples from heavily industrialized bays of Korea to assess the occurrence, contamination, potential sources, and ecotoxicological concerns. PCNs were detected in all sediment samples, indicating ubiquitous contamination in industrialized coastal regions of Korea. Total concentrations and toxic equivalents (TEQs) of PCNs ranged from 0.99 to 21,500 (mean: 568) pg/g dry weight and from 1.72 × 10–5 to 18.8 (mean: 0.52) pg TEQ/g dry weight, respectively, which were within the ranges reported by other studies. A clear decreasing gradient was observed for the sedimentary PCNs from inner to outer parts of the bays, streams, and rivers. This result indicates that industrial activities are primary sources of PCNs. The highest PCN concentrations were observed in sediment close to non-ferrous and petrochemical industries, indicating potential sources. CNs 73 and 52 were predominant congeners of PCNs in all sediment samples. Diagnostic ratios and non-parametric multidimensional scaling analysis showed that the potential primary sources of PCNs are thermal-related emissions and the use of PCB technical mixtures. Although a few sediment samples exceeded the sediment quality guidelines of TEQs, the cumulative risks by dioxin-like contaminants may be caused for almost all coastal zones surveyed. This is the first report on PCNs in sediment from Korean coastal waters.
Introduction
Polychlorinated naphthalenes (PCNs) comprise 75 congeners from mono- to octa-CNs, with differing numbers and positions of chlorine substituents based on naphthalene structure. PCNs were extensively produced for applications including insulating oils for capacitors and flame retardants in electrical cables beginning in the 1950s (Odabasi et al., 2017; Agunbiade et al., 2020). The major PCN technical mixtures were branded as Halowax, Seekau, Clonacure waxes, and Wako-PCN, and were used in many countries (Falandysz et al., 2008). Although the consumption data of PCNs was not precisely recorded, the total PCN production from larger companies was estimated to be approximately 150,000 tons during the 1930s–1970s (Falandysz et al., 2014). Based on the structural similarities with polychlorinated biphenyls (PCBs) and polychlorinated dibenzo-p-dioxins and dibenzofurans (PCDD/Fs), PCNs showed similar dioxin-like toxicological effects binding with aryl-hydrocarbon (AhR) receptors (Falandysz et al., 2014). Previous in vivo and in vitro studies have reported that PCNs elicited mortality, embryotoxicity, hepatotoxicity, dermal lesions, teratogenicity, and carcinogenicity (Behnisch et al., 2003; Falandysz et al., 2014; Suzuki et al., 2020). The ecotoxicological data of PCNs have been reported for several species representing different trophic levels: algae, aquatic plants, invertebrates, fish, and birds (Noma et al., 2005; Helm et al., 2006). Because most studies used the Halowax technical mixtures, the ecotoxicological experiments could result in confusion due to possible toxicological interactions and dioxin impurities (Noma et al., 2005). Several acute toxicities of 1-chloronaphthalene have been reported on freshwater and saltwater species as 1,600, 2,300, and 690–2,500 μg/L for Daphnia magna, Lepomis macrochirus, and Cyprinodon variegatus, respectively (Falandysz, 1998; Helm et al., 2006). However, no data are available on ecotoxicological data for benthic organisms of PCNs.
Due to domestic and worldwide regulations on PCNs, the current PCN contamination is mostly emitted from thermal processes, accounting for over 80% of total PCN emissions in the environment (Bidleman et al., 2010). These include waste incineration, steel and coking industries, and chlor-alkali and copper smelting processes (Liu et al., 2010, 2014; Hu et al., 2013; Waheed et al., 2020). PCNs are also released from the use of PCB technical mixtures as impurities contained (Huang et al., 2015; Li et al., 2021). Although there has been no consumption of technical PCN mixtures (Park et al., 2010), several PCN congeners have been detected in multiple matrices, such as air, soil, freshwater biota, and human serum (Lee et al., 2007; Park et al., 2010; Kim et al., 2019a). However, no reports are available on the PCN contamination in the coastal environment of Korea.
Sediment acts as the last depositional reservoir for hydrophobic contaminants in the coastal environment (Liu et al., 2018; Dat et al., 2019; Lee et al., 2020). A previous study reported that PCNs are accumulated from sedimentary particles to marine species (Wang et al., 2012). It is prompted to survey the distribution and accumulation of organic contaminants in coastal sediments for evaluating the potential health risks of marine species (Moon et al., 2008a; Mahmood et al., 2014; Walker et al., 2015). Gu et al. (2021) predicted that PCN congeners are highly toxic at all the trophic positions in the food-web associated with joint toxicity mechanisms. To date, most previous studies have conducted the determination of PCN congeners in environmental and biota samples using a high-resolution mass spectrometer (HRMS) (Park et al., 2010, 2021; Liu et al., 2018; Kim et al., 2019a). Due to the enhanced sensitivity, chromatograph coupled to a tandem mass spectrometer (GC-MS/MS) has been partly utilized for PCN analysis in sediment samples (Li et al., 2016a; Wu et al., 2018). The challenge for an analytical method using a GC-MS/MS is the separation of individual PCN congeners because PCNs showed congener-specific toxicities (Falandysz et al., 2014; Suzuki et al., 2020). In our study, the analytical methods were optimized using a multi-layer silica gel column and a GC-MS/MS for the determination of major PCN congeners in sediment samples. Compared to the HRMS determination, the GC-MS/MS analysis could provide more cost-effective and comprehensive monitoring programs. In the present study, the PCN congeners were measured for the sediments collected from Ulsan and Onsan Bays surrounded by the “National Industrial Complexes” which have been developed since the 1970s. Our previous studies have reported severe pollution by unintentional and industrial contaminants, such as PCDD/Fs, dioxin-like PCBs, polybrominated diphenyl ethers (PBDEs), siloxanes, and plasticizers in the surveyed bays (Moon et al., 2007, 2008b; Lee et al., 2018, 2020; Kim et al., 2021). Therefore, the objectives of the present study are to assess the occurrence, contamination, source tracking, and ecotoxicological implications of PCNs in sediment from highly industrialized coastal waters. This is the first report on the congener-specific data on PCNs in Korean coastal waters.
Materials and Methods
Sample Collection
The Ulsan and Onsan Bays, located in the southeastern part of Korea, are surrounded by heavily industrialized complexes and the largest commercial harbors in Korea (Figure 1). The industrial complexes, comprising petrochemical, iron and non-ferrous industries, automobiles, and shipyards, have produced approximately 32% of the commercial and industrial products consumed in Korea (Lee et al., 2020). Surface sediments (4 cm depth), representing the sedimentation record for recent 4–6 years (Lee et al., 2018), were collected from 49 locations in April 2020 using a Van Veen grab sampler. Samples were wrapped in an aluminum foil that had been pre-washed with a solvent and transported to the laboratory. All sediment samples were stored in a –20°C freezer and then freeze-dried. In our study, the closest locations to industries around streams and rivers were chosen for tracking and characterizing the contamination sources of PCNs using congener-specific analysis. The sampling locations were divided into streams, rivers, and bays, such as Gosa (G1–G4), Yeocheon (Y1–Y2), and Daejeong (D1–D4) Streams, Taehwa (T1–T4) and Woihwang (W1–W7) Rivers, and Ulsan (U1–U18) and Onsan (O1–O10) Bays to investigate the spatial distribution of PCN contamination.
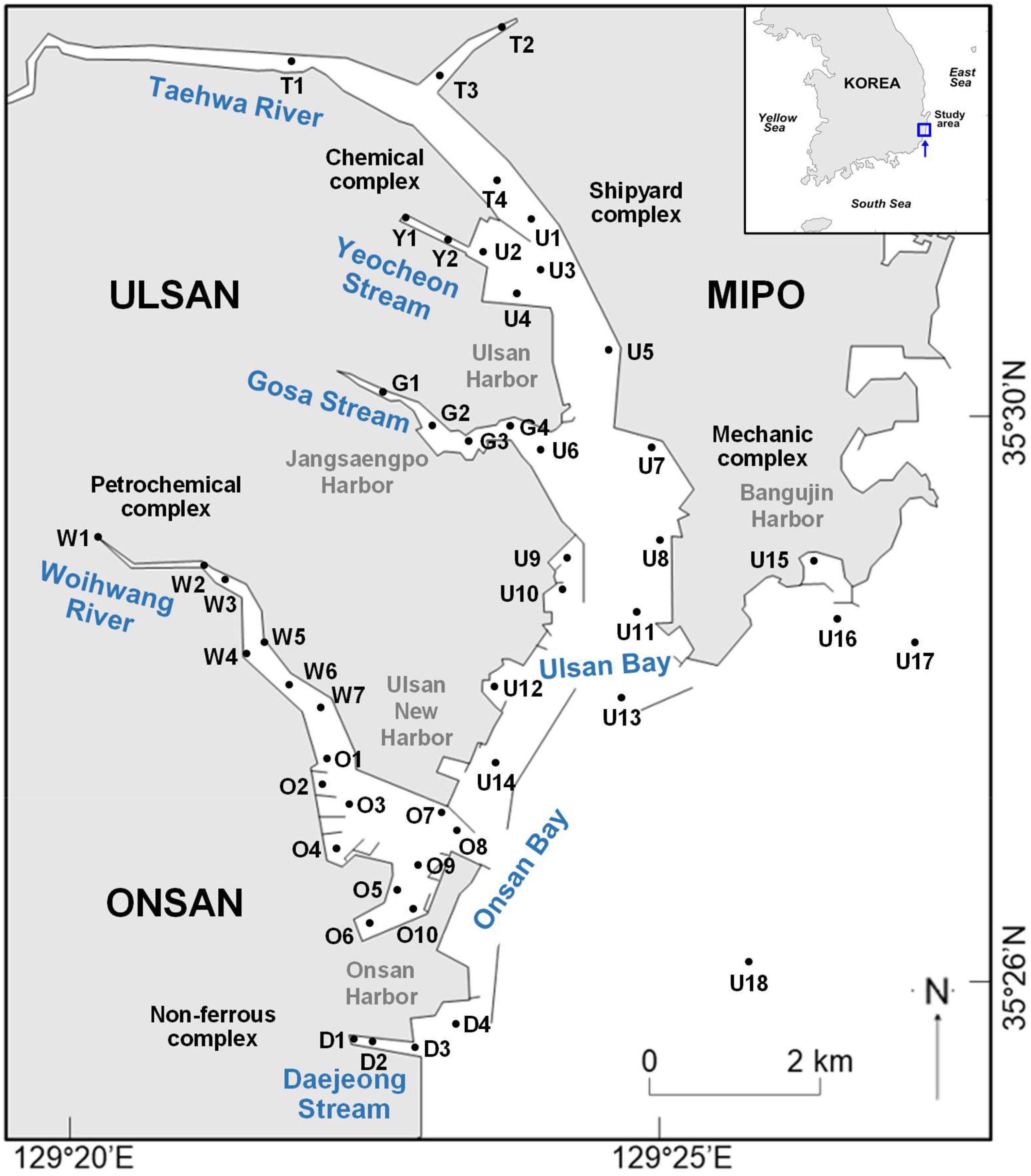
Figure 1. Sampling locations of sediment collected from Ulsan and Onsan Bays and their inflowing rivers and streams, Korea. Sampling stations T, Y, G, U, W, D, and O represent Taehwa River, Yeocheon Stream, Gosa Stream, Ulsan Bay, Woihwang Stream, Daejeong Stream, and Onsan Bay, respectively.
Standards and Reagents
Among 75 PCN congeners, 18 congeners from mono- to octa-CNs (CNs 2, 6, 13, 27, 36/28, 46, 48, 50, 52, 53, 54, 63, 64/68, 66/67, 69, 72, 73, and 75) were measured for target contaminants, which were chosen based on toxic potency with AhR receptors and environmental occurrence (Falandysz et al., 2014; Pagano and Garner, 2021). All native standards of PCNs were purchased from Cambridge Isotope Laboratories (Andover, MA, United States) and Wellington Laboratories (Guelph, ON, Canada). Mass-labeled PCNs (13C10-CNs 27, 42, 52, 67, 73, and 75; ECN-5102, Wellington Laboratories) and native PCB congeners (CBs 103, 198, and 209) were used as internal and surrogate standards, respectively. Ultra-trace residue levels of hexane and dichloromethane (DCM) were purchased from J. T. Baker. Pesticide-analysis grade silica gel, nonane, anhydrous Na2SO4, and copper were purchased from GL Sciences, Kanto Chemical, Merck, and Sigma-Aldrich, respectively.
Optimization of a Clean-Up Method and Instrumental Parameters
Considering similar characteristics of PCNs with PCBs and PBDEs, a multi-layer silica gel column was applied for a clean-up method in the determination of PCN congeners in sediment samples (Moon et al., 2007, 2008a,b). A multi-layer silica column contained anhydrous Na2SO4 (0.5 g), activated silica gel (1 g), 22% H2SO4-impregnated silica gel (4 g), 44% H2SO4-impregnated silica gel (4 g), activated silica gel (1 g), 2% KOH-silica (3 g), and anhydrous Na2SO4 (0.5 g) from bottom to top in the class column (300 mm length, 15 mm inner diameter) (Supplementary Figure 1). Native and mass-labeled standards of PCNs were spiked into a sediment extractor to check for the efficacy and elution patterns of a multi-layer silica gel column. After the extractor was added to a multi-layer silica gel column, a mixture of 15% DCM in hexane (180 mL) was used as an elution solvent to remove the interferences and to fractionize the PCN congeners in sediment samples. The method optimized in our study is a minor modification of the method used for PCB and PBDE determinations in earlier studies (Moon et al., 2007, 2008a,b). Six eluted portions were fractionized every 30 mL of elution solvent. All 18 PCN congeners were eluted within 60 mL of a mixture of 15% DCM in hexane, which was used for a final cleanup method.
Sediment samples (∼10 g) were extracted using an accelerated solvent extractor (ASE 350, Dionex, Sunnyvale, CA, United States) with 60 mL of DCM and hexane (3:1) after spiking into surrogate standards (5 ng; CBs 103, 198, and 209), similar to the method for PCDD/Fs, PCBs, and PBDEs (Moon et al., 2007; Lee et al., 2020). Extracts were concentrated to 5 mL by TurboVap LV (Biotage, Uppsala, Sweden) and sulfur in extracts was removed using activated cooper. A multi-layer silica gel column optimized was used as a cleanup method with an elution with 60 mL of 15% DCM after spiking internal standards of mass-labeled PCNs (1 ng each). Eluant was concentrated and then dissolved in 100 μL of nonane.
A GC-MS/MS (7890GC/7000B, Agilent Technologies, Wilmington, DE, United States) and a DB-5MS UI capillary column (60 m length, 0.25 mm inner diameter, 0.25 μm film thickness; J&W Scientific, Palo Alto, CA, United States) were used for separation and quantification of 18 PCN congeners. The oven temperature was programed to increase from 90°C (1 min) to 160°C at 10°C/min (10 min), and then to 300°C (2 min). Inlet and ion source temperatures were maintained at 270°C and 330°C, respectively. Helium was used as a carrier gas at a constant flow rate of 1 mL/min. The MS/MS conditions were optimized for the accurate quantitation of PCN congeners under the multiple reaction monitoring (MRM) mode. Some congeners, such as CNs 36/28, 64/68, and 66/67, were co-eluted in our analytical method, similar to those described previously (Wu et al., 2018). Detailed information on quantification and confirmation m/z under the MRM mode and retention time for targeted PCN congeners are summarized in Supplementary Table 1.
Quality Control
In order to check for background contamination throughout whole experimental procedures, the procedural blank samples (n = 5; anhydrous Na2SO4) were processed every 10 samples as real samples. Only trace levels (CNs 2, 6, 13, 36/28, 46, and 48; 0.21 to 2.3 pg/g) were detected in the blank samples, which were subtracted from the measured concentrations in real samples. To check a crossover contamination of PCNs during a GC-MS/MS analysis, hexane was used before and after the injections of calibration standards and between every 15 samples. No crossover contamination was observed in our sample batch. The instrumental limit of quantifications (iLOQs) of 18 PCN congeners with a GC-MS/MS were calculated as ten times the standard deviation for seven replicate injections of the lowest acceptable level (0.1 ng/mL), which ranged from 0.1 to 1.4 pg/g dry weight (wt). All mass-labeled internal standards were detected without any interferences in all sediment samples. The recoveries of surrogate standards were 91% ± 11% (average ± standard deviation), 88% ± 14%, and 95% ± 8.3% for CBs 103, 198, and 209, respectively. Matrix-spiked samples were also employed to check for the influence of matrix effects for sediment samples. Recoveries of native and mass-labeled standards of PCNs for matrix-spiked samples ranged from 84 to 105% (mean: 93.6%) and 85 to 101% (mean: 95.3%), respectively. The detailed results of quality control conducted in our study are summarized in Supplementary Table 2. Total organic carbon (TOC) and nitrogen (TON) were measured using a CHN elemental analyzer. The accuracy and precision were 92 and 9.31% for TOC and 93 and 4.62% for TON, respectively.
Calculation of Toxic Equivalent Concentrations and Statistical Analysis
Unlike PCDD/Fs and dioxin-like PCBs, the toxic equivalent factors (TEFs) of PCNs are not provided from international agencies, such as the World Health Organization (WHO) (Van den Berg et al., 2006). In our study, the TEFs of PCN congeners were used from the values proposed by Falandysz et al. (2014), which was used for other studies (Pagano and Garner, 2021; Park et al., 2021). The toxic equivalent (TEQ) concentrations were calculated by multiplying the actual concentration of individual PCN congener and its TEF.
Total concentrations of PCNs (ΣPCN; the sum of 18 PCNs) and individual PCN detected > 60% in all sediment samples were only employed for statistical analyses. The concentration of individual PCN congener < iLOQ was treated as half iLOQ for the statistical analysis. For the calculation of ΣPCN concentrations, the concentration of individual PCN congener < iLOQ was treated as zero to avoid overestimation of the results. Because the PCN concentrations showed the log-normal distribution in our sediment samples, individual and total PCN concentrations were transformed to logarithm value for parametric analysis. Analysis of variance was used to assess differences in the concentrations and compositions of the sedimentary PCN congeners depending on the sampling locations. Pearson correlation analysis was conducted to assess the strength of relationships among PCN congeners or between PCN concentrations and TOC contents. All statistical analyses were performed using SPSS 18.0 software with a significance level at p < 0.05.
Results and Discussion
Occurrence and Concentrations of Polychlorinated Naphthalenes in Industrialized Coastal Sediment
The sample characteristics and concentrations of PCN congeners detected in sediment from industrialized bays and their inflowing rivers and streams of Korea are summarized in Table 1 and Supplementary Table 3. Contents of TOC and TON in all of the sediment samples varied widely depending on the sampling locations, with ranges of 0.22–6.63% (mean: 1.78%) and 0.01–0.55% (mean: 0.15%) for TOC and TON, respectively. The highest TOC contents were observed at locations from streams, such as Gosa Stream (G2; 6.63%), Yeocheon Stream (Y1; 6.36%), and Daejeong Stream (D2; 5.95%), which are surrounded by heavily industrial complexes. The TOC contents in sediments from Taehwa River (0.94% ± 0.52%) passing through the urbanized region were significantly (p < 0.05) lower than those found in other coastal zones. Similar to TOC, the highest and lowest contents of TON were observed at stations collected from streams (G2 and D3) and Taehwa River (T1–T4; mean: 0.07%), respectively. Previous studies have reported that the molar ratio of C/N is a proxy for organic matter sources, which are 5–7 and > 15 for marine and terrestrial origins, respectively (Wu et al., 2020). In our study, the molar ration of C/N in sediment ranged from 6.88 to 36.1, with a mean value of 13.2, indicating that most sampling zones including the streams, rivers, and bays are influenced by the land-based organic matters. The highest C/N ratios were observed in sediments from inner part of streams and rivers, such as D1 (36.1), D2 (29.7), Y1 (27.6), and G1 (21.6) but were not significant (p > 0.05). Our findings suggest that industrial activities contributed to the high burden of organic matter into the bays through rivers and streams.
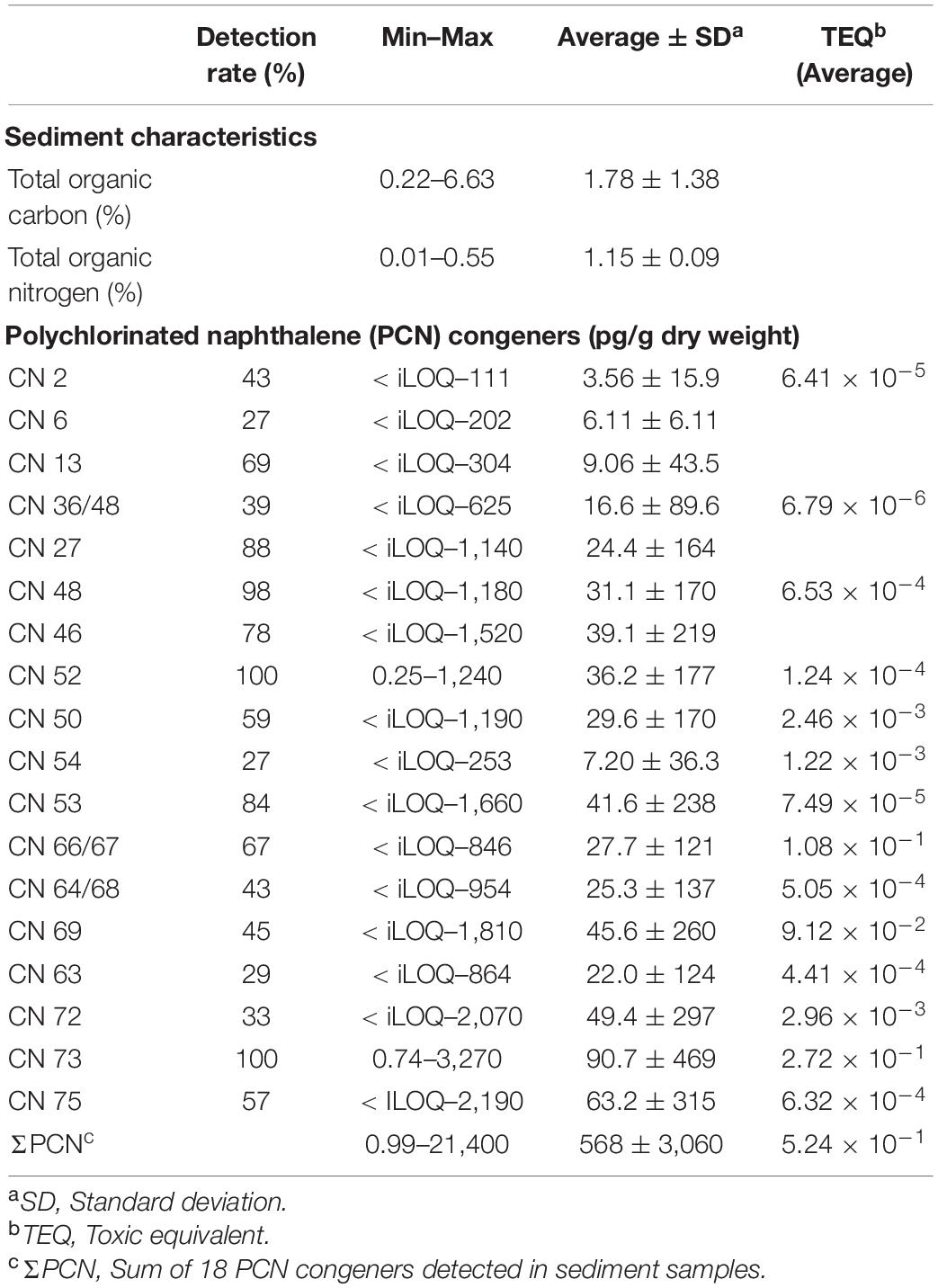
Table 1. Sediment characteristics and the concentrations of polychlorinated naphthalene (PCN) congeners in sediments collected from Ulsan and Onsan Bays and their inflowing streams and rivers, Korea.
Although the consumption of PCN technical mixtures was restricted in industrial markets of Korea, PCNs were detected in all sediment samples from industrialized coastal waters of Korea. This indicates the presence of ongoing sources of PCNs from industrialized complexes. The ΣPCN concentrations in all sediment samples ranged from 0.99 to 21,400 (mean: 568) pg/g dry wt. CNs 73 and 52 were detected in all sediment samples among 18 PCN congeners. CNs 13, 27, 36/28, 46, 48, 53, and 73 were detected at > 60% of all sediment samples. For homolog patterns, penta-CN was predominant, whereas mono- and di-CNs, except CN 13, were detected < 20% in all sediment samples. The highest concentration of PCN congeners was observed for CN 73, ranging from 0.74 to 3,270 (mean: 90.7) pg/g dry wt. The concentrations of highly chlorinated congeners, such as CNs 75 and 72, were also significantly (p < 0.05) higher than the concentrations of the remaining PCN congeners. The concentrations of CNs 75 and 72 ranged from < iLOQ to 2,190 (mean: 63.2) and < iLOQ to 2,070 (mean: 49.4) pg/g dry wt, respectively. Halowax (e.g., Halowax 1000), a representative PCN technical mixture, is primarily composed of mono- and di-CN congeners (Falandysz et al., 2006), which is inconsistent with the congener patterns of PCNs observed in our study. This implies that PCN technical mixtures are not major contamination sources in Korean coastal waters. The higher concentrations of CNs 75 and 72 in the sediment samples could be caused by a preferential adsorption of highly chlorinated contaminants to sedimentary particles (Moon et al., 2008a,b; Lee et al., 2020). In our study, the TEQ concentrations in all of the sediment samples ranged from 0.002 to 18.8 (mean: 0.52) pg TEQ/g dry wt. The highest TEQ concentrations were observed for CN 73 (mean: 0.27 pg TEQ/g dry wt), CN 66/67 (0.11 pg TEQ/g dry wt), and CN 69 (0.09 pg TEQ/g dry wt), which collectively accounted for over 90% of total TEQ concentrations.
The PCN concentrations observed in our sediment samples are compared to those reported for other locations or countries (Supplementary Table 4). The PCN concentrations in sediment samples collected after 2010 were only used to prevent the effectiveness of time trends. The ΣPCN concentrations in sediment from Ulsan Bay (mean: 42.3 pg/g dry wt), Onsan Bay (17.4 pg/g dry wt), and Taehwa River (19.6 pg/g dry wt) measured in our study were similar to those reported from Feitsui Reservoir, Taiwan (mean: 29.2 pg/g dry wt; Dat et al., 2019). The ΣPCN concentrations in sediment from Woihwang River (mean: 268 pg/g dry wt) and Yeocheon Stream (333 pg/g dry wt) were a similar order of magnitude to those reported for the Laojie River, Taiwan (980 pg/g dry wt; Dat et al., 2019). The highest ΣPCN concentrations found in Daejeong Stream (mean: 5,970 pg/g dry wt) were within the ranges of those reported for the Yangtze River, China (3,270 pg/g dry wt; Zhang et al., 2015), Liaohe River, China (4,400 pg/g dry wt; Li et al., 2016b), Yellow River, China (7,100 pg/g dry wt; Li et al., 2017), and Chenab River, Pakistan (8,930 pg/g dry wt; Mahmood et al., 2014). East China Sea (77,000 pg/g dry wt; Liu et al., 2018) and Detroit River, the United States (264,000 pg/g dry wt; McGoldrick et al., 2018) were approximately 2–4 orders of magnitude higher than those observed in our study. The Detroit River is located close to the largest industrial complexes in the United States, including steel, petrochemical, and automobile industries (McGoldrick et al., 2018).
Significant correlations (r = 0.315–0.950, p < 0.05) were observed among the concentrations of individual PCN congeners in our sediment samples (Supplementary Table 5), implying the presence of common sources and environmental behavior of PCN congeners in the coastal environment. The highest correlation coefficients were observed for CN 52 with other congeners, such as CN 73 (r = 0.923, p < 0.001), CN 46 (r = 0.861, p < 0.001), and CN 64/68 (r = 0.842, p < 0.001). In our study, a significant correlation coefficient was found between ΣPCN concentrations and TOC content (r = 0.409, p < 0.01). The higher correlation coefficients were observed for sediments collected from each coastal region, such as Gosa Stream (r = 0.541–0.990, p < 0.05), Daejeong Stream (r = 0.637–0.995, p < 0.05), and Woihwang River (r = 0.521–0.919, p < 0.05). These rivers and streams are surrounded by industrial complexes, which could expect the direct influences from industrial activities. In particular, the highest correlation coefficients were observed for sediments from Daejeong Stream, which is characterized by the highest contamination by PCNs observed in our study. This finding suggests that PCNs are primarily originated from the same sources, such as a specific industrial facility. Except for CNs 13 and 75, all PCN congeners showed significant correlations (r = 0.305–0.494, p < 0.05) with TOC content. This is likely due to greater hydrophobicity and Koc values of PCNs. This result suggests that organic carbon is to be one of the primary factors governing the sedimentary distribution of PCNs. Similar results were reported for other hydrophobic contaminants in the coastal environments of Korea (Lee et al., 2018, 2020; Kim et al., 2021).
Spatial Distribution of Polychlorinated Naphthalenes in Sedimentary Environment
The spatial distribution of the actual and TEQ concentrations of ΣPCN in sediment collected from Ulsan and Onsan Bays and their inflowing rivers and streams are presented in Figure 2. The overall contamination of ΣPCN in sediment was higher in Ulsan Bay (42.7 ± 61.1 pg/g dry wt; mean ± standard deviation) than in Onsan Bay (17.6 ± 12.7 pg/g dry wt) without significance (p > 0.05). Our previous studies have reported the higher sedimentary contamination by flame retardants and plasticizers in Onsan Bay than Ulsan Bay (Lee et al., 2020; Kim et al., 2021), which was inconsistent with those observed in our study. This implies that sources and environmental behaviors are dependent on types of organic contaminants in the coastal environment. Sediment from Taehwa River showed relatively lower ΣPCN concentrations (22.3 ± 30.6 pg/g dry wt) than those found in Ulsan Bay, suggesting that urbanized activities are not a major contamination source of PCNs. However, the higher ΣPCN concentrations were observed in sediment from Yeocheon Stream (Y1–Y2; 350 ± 493 pg/g dry wt), close to non-ferrous industries (e.g., aluminum). Our findings indicate that the sedimentary contamination by PCNs is associated with the proximity to the industry producing PCNs. For Ulsan Bay, the ΣPCN concentrations in sediment collected from the inner part (U1–U4; 90.7 ± 65.7 pg/g dry wt) were significantly (p < 0.05) higher than those observed in the outer part of the bay (U5–U14; 12.9 ± 6.92 pg/g dry wt). Moreover, a clear decreasing trend was observed in the ΣPCN concentrations from inner to outer parts of the bay. Similarly, a clear decreasing trend in the ΣPCN concentrations was observed in sediment from inner to outer part of Gosa Stream. These results suggest that contamination sources of PCNs are present in inner parts of the bays and streams close to industrial complexes and harbors. In our study, the ΣPCN concentrations in sediment from Gosa Stream (G1–G4; 83.9 ± 63.6 pg/g dry wt) were significantly (p < 0.05) lower than those found in Yeocheon Stream. Unlike our findings, the highest contamination by siloxanes were observed in sediment from Gosa Stream (Lee et al., 2018), suggesting both chemical groups seem to have distinct contamination sources.
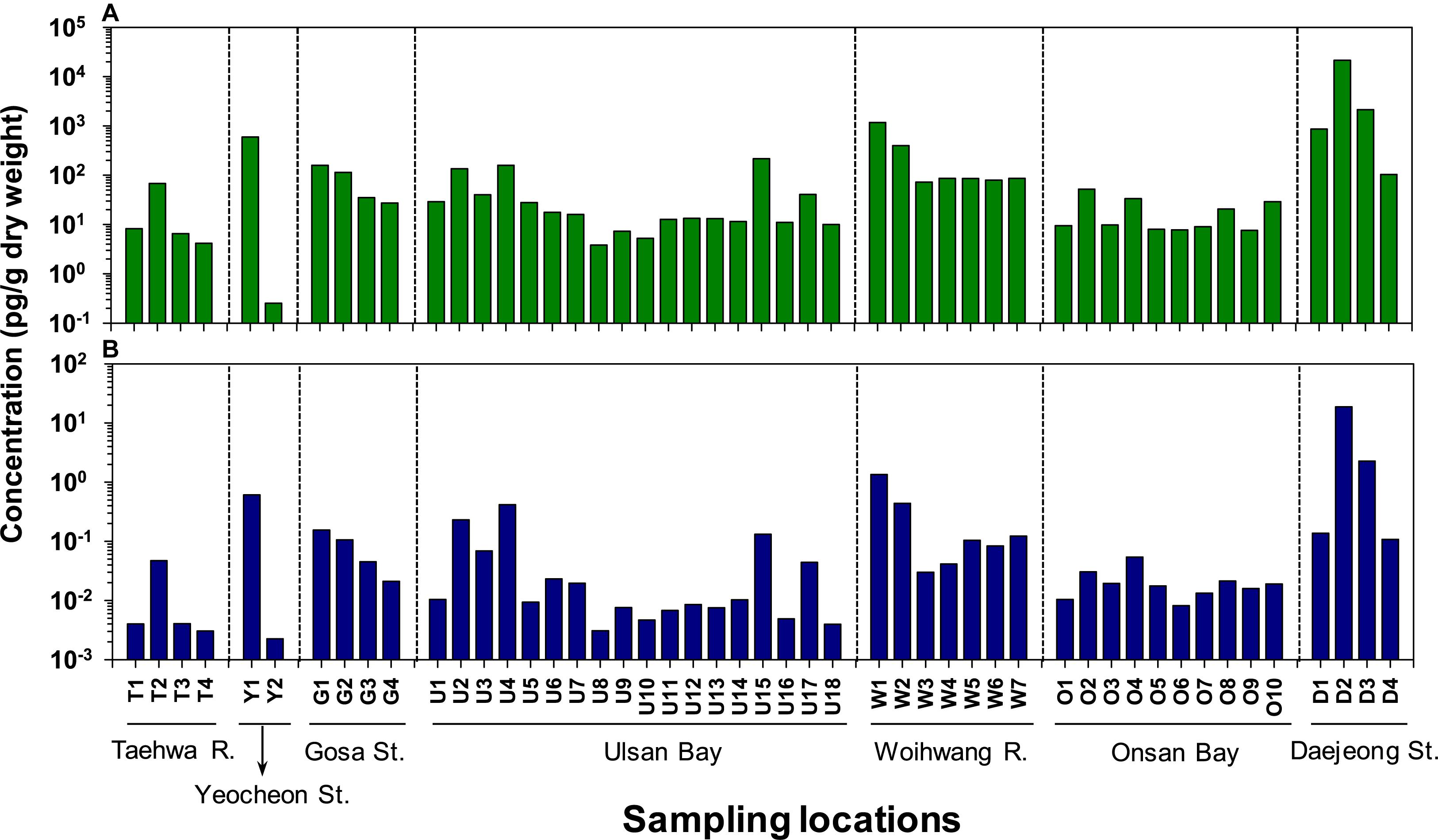
Figure 2. Spatial distribution of (A) total and (B) toxic equivalent (TEQ) concentrations of polychlorinated naphthalenes (PCNs) in sediment collected from Ulsan and Onsan Bays and their inflowing rivers and streams, Korea.
Although the ΣPCN concentrations in sediment from Onsan Bay were relatively lower than those observed in other coastal regions surveyed, the sediment from Woihwang River (W1–W7; 273 ± 417 pg/g dry wt) was highly contaminated by PCNs. In particular, the sediment from Station W1 close to non-ferrous and petrochemical industries showed higher concentration of ΣPCN (1,175 pg/g dry wt). This indicates that these industries could be primary sources of PCN contamination. Previous studies have reported that the metal and non-ferrous smelting processes are major contamination sources of PCNs in the environment (Yamamoto et al., 2018; Hu et al., 2021; Shen et al., 2021). In our study, the highest ΣPCN concentrations were observed in sediment from Daejeong Stream (D1–D4; 6,000 ± 10,400 pg/g dry wt), surrounded by non-ferrous (zinc, copper, and aluminum) industries. In particular, the sediment from Station D2 showed the highest ΣPCN concentration (21,000 pg/g dry wt) in all sediment samples. During the smelting processes of iron and non-ferrous materials (e.g., copper and aluminum), cutting oils could contain PCNs as impurities (Agunbiade et al., 2020; Shen et al., 2021).
The overall spatial distribution of TEQ concentrations in sediment was similar to those observed in the actual ΣPCN concentrations (Figure 2B). The highest TEQ concentrations were found in sediment from Daejeong Stream (5.32 ± 9.03 pg TEQ/g dry wt), which were 1–2 orders of magnitude higher (p < 0.05) than those observed in other coastal regions. Sediment from Station D2 showed the highest TEQ concentration (18.8 pg TEQ/g dry wt), followed by Station D3 (2.27 pg TEQ/g dry wt) from Daejeong Stream. Sediments from Yeocheon Stream (0.31 ± 0.43 pg TEQ/g dry wt) and Woihwang River (0.31 ± 0.48 pg TEQ/g dry wt) had higher TEQ concentrations than those observed in other regions.
Congener-Specific Profiles and Potential Sources of Polychlorinated Naphthalenes
The congener profiles of actual and TEQ concentrations of PCNs in sediment from Ulsan and Onsan Bays and their inflowing rivers and streams are presented Figure 3. For actual PCN concentrations, the predominant congeners were CNs 73 and 52 in all sediment samples, which accounted for 31 and 19% of the ΣPCN concentrations, respectively. The next highly contributed congeners were CNs 53 and 66/67, collectively accounting for 22% of the ΣPCN concentrations. The other PCN congeners contributed to < 10% of the ΣPCN concentrations. Previous studies reported the predominance of CNs 73, 52, and 60 in sediment samples (Castells et al., 2008; Zhao et al., 2011), consistent with those found in our study. Penta- and hexa-CNs were major homolog groups rather than less-chlorinated PCNs due to their hydrophobic characters in aquatic environment (Meijer et al., 2001). For TEQ concentrations, CNs 73 (mean: 74%) and 66/67 (24%) only showed contributions of total TEQs. This could be explained by higher concentrations and 1–5 orders of magnitude higher TEFs of these congeners than those of the others. The congener patterns of predominant PCNs in our sediment samples were similar to those observed in marine biota. Reported data showed that highly toxic congeners of PCNs (CNs 66, 67, and 73) are prevalent in marine fish and mammals (e.g., polar bears) across the globe (Bidleman et al., 2010; Agunbiade et al., 2020). This similarity of congener patterns for PCNs in marine sediment and biota could be based on their persistence and bioaccumulation potentials. Comprehensive bioaccumulation studies are needed for protecting marine organisms and humans from the exposure of PCNs.
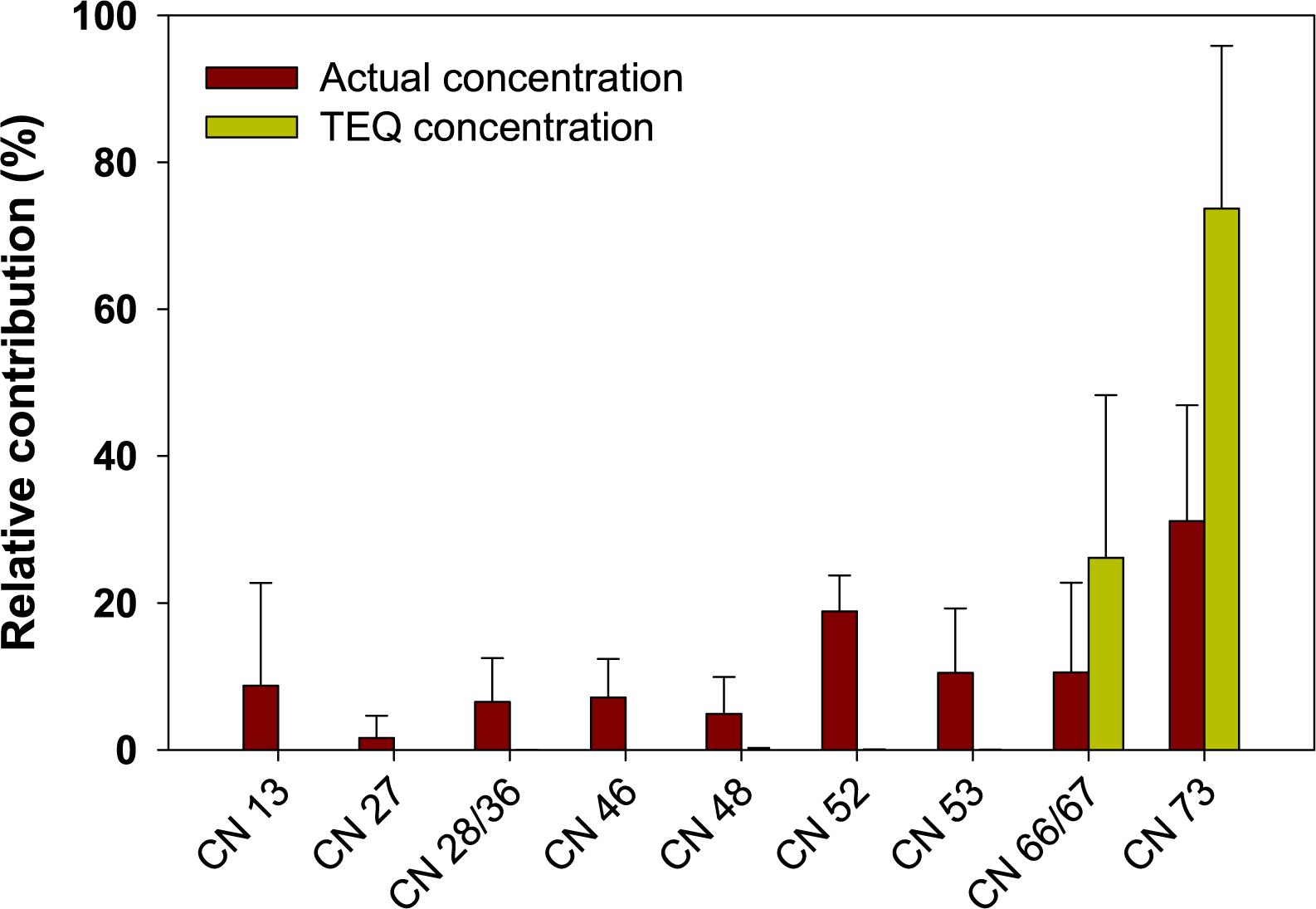
Figure 3. Average relative contribution of PCN congeners for actual and TEQ concentrations in sediment from Ulsan and Onsan Bays and their inflowing rivers and streams, Korea. Vertical bars represent standard deviation.
Earlier studies have utilized the diagnostic ratios of PCN congeners to track the potential sources of PCNs in sediment samples (Lee et al., 2007; Wang et al., 2012; Dat et al., 2019). The diagnostic ratio of CNs 66/67 to 71/72 has been applied to differentiate typical contamination sources of PCNs, such as thermal-related emission, technical PCB mixtures, and technical PCN mixtures (Dat et al., 2019). Ratio of CNs 66/67 and 71/72 > 2.5 indicate thermal-related emissions, while that < 2.5 indicates the consumption of PCB or PCN technical mixtures (Dat et al., 2019). The concentration ratio of ΣPCN with thermal-related congeners (CNs 13, 27, 36, 50, 52, 54, 66, 67, and 73) and ΣPCN was also employed to distinguish the contamination sources, which are > 0.5 for thermal-related emissions and < 0.11 for the use of technical mixtures (Lee et al., 2007). Some sediment samples (D1, W2, W4, W7, U2, G1, and T2) exceeded 2.5 for the diagnostic ratio of CNs 66/67 to 71/72 (Figure 4), indicating thermal-related emissions of PCNs. In contrast, sediments from W1, Y1, D2, D3, D4, G2, and U17 indicated the consumption of PCB or PCN technical mixtures for PCN contamination. In our study, most sediment samples from Ulsan and Onsan Bays did not contain CNs 71/72, precluding calculation of the diagnostic ratio of CNs 66/67 and 71/72. The diagnostic ratio between ΣPCN with thermal-related congeners and ΣPCN exceeded 0.5, indicating thermal-related contamination of PCNs. However, no sediment samples were included as an indication for the use of technical mixtures. Our findings suggest that the sedimentary contamination by PCNs in our study primarily originates from thermal-related emissions with a minor contribution from technical mixtures of PCBs as impurities. Considering no consumption of PCN technical mixtures in Korea, technical mixtures could be associated with the consumption of PCBs. Ulsan and Onsan industrial complexes are surrounded by industrial complexes for petrochemicals, ferrous and non-ferrous products, paints, plasticizers, and automobiles. These industries include the combustion processes and the use of industrial products containing PCBs, which are supported by the severe contamination by PCBs in Ulsan and Onsan Bays (Moon et al., 2008b).
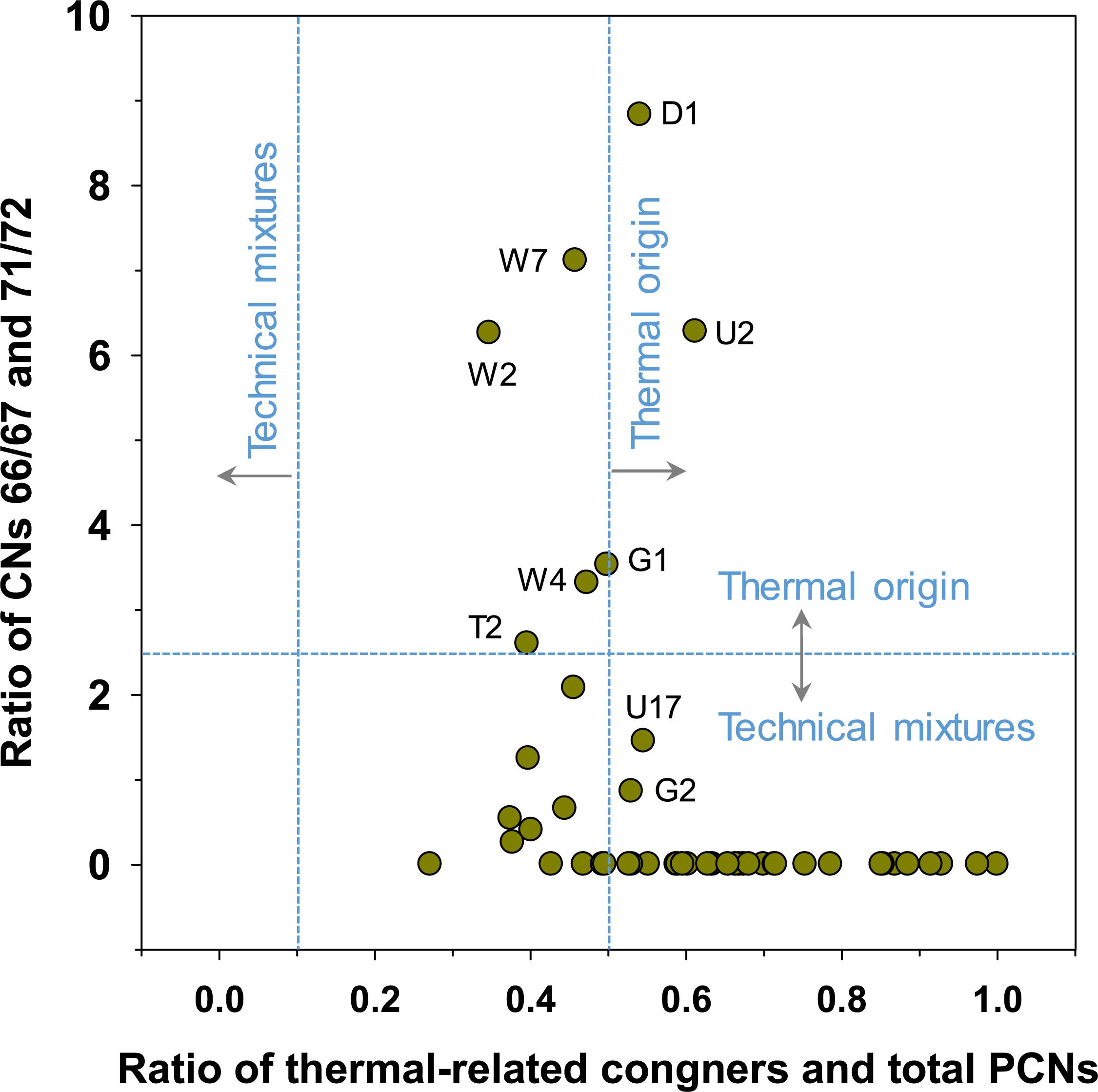
Figure 4. Diagnostic ratios of the concentrations of PCN congeners in sediment from Ulsan and Onsan Bays and their inflowing streams and rivers, Korea.
In order to further characterize the contamination sources of PCNs, non-parametric multidimensional scaling (nMDS) analysis was performed with sedimentary PCN congeners using a PRIMER software (Plymouth, United Kingdom) (Figure 5). Four groups were clustered based on cluster analysis and a stress value of 0.01, indicating significance. All groups showed higher contribution of CN 73, which is an indicator for thermal emission of PCNs (Liu et al., 2018). This result indicates that thermal processes primarily contributed to PCN contamination in the coastal zones surveyed, consistent with the results from diagnostic ratios. Group A included almost all of the sampling locations. It was characterized by the dominance of CNs 73 and 52, collectively accounting for > 50% of the ΣPCN concentrations. These PCN congeners primarily originate from thermal-related activities (Lee et al., 2007; Liu et al., 2018). This suggests that PCNs are derived from thermal emission associated with industrial activities. As mentioned above, the Ulsan and Onsan industrial complexes are characterized by thermal-related activities, such as petrochemical and non-ferrous industries. Thus, thermal-related industrial activities could primarily contribute to sedimentary contamination by PCNs. Park et al. (2021) reported that combustion-related congeners are predominantly detected in agricultural soils near industrial cities of Korea. Group B, an innermost location (Station W1) from Woihwang River, was characterized by a higher contribution of tetra-CNs (44% of ΣPCN), such as CNs 28/36, 46, and 48, to the ΣPCN concentrations. Nadal et al. (2007, 2011) reported the dominance of tetra-CNs in environmental samples collected from chemical/petrochemical complexes. Woihwang River is surrounded by the largest petrochemical and non-ferrous industries, which could significantly affect PCN contamination in sediment. Although Groups C and D include the stations (D2 and D3) from Daejeong Stream, the congener profiles of PCNs were different. Group C (Station D3) was characterized by the dominance of CN 73 (> 40% of ΣPCN) and similar contributions (∼10% of each) of CNs 46, 48, 52, 53, and 66/67 of the ΣPCN concentrations. Group D (Station D2), the highest PCN concentration (21,000 pg/g dry wt and 18.8 pg TEQ/g dry wt) recorded in our study, was characterized by similar contributions (10% ± 3.1%) for all tetra- to hexa-CN congeners (CNs 28/36, 46, 48, 52, 53, and 66/67) of the ΣPCN concentrations. Considering the location close to non-ferrous industries, discharges of cutting oils containing PCNs as impurities during non-ferrous smelting processes may affect the severe contamination by PCNs. Shen et al. (2021) reported that smelting processes are major sources of PCNs in the environment. Although the specific industrial facilities or processes were not suggested for PCN contamination sources in our study due to the limited accessibility for sample collection, the comprehensive survey on PCN contamination sources is required for effluents from different types of industrial complexes. Moreover, the additional analytical tools, such as stable carbon isotope fractionation, are needed to accurately identify the specific sources of PCNs in the sedimentary environment (Horii et al., 2005; Huang et al., 2020).
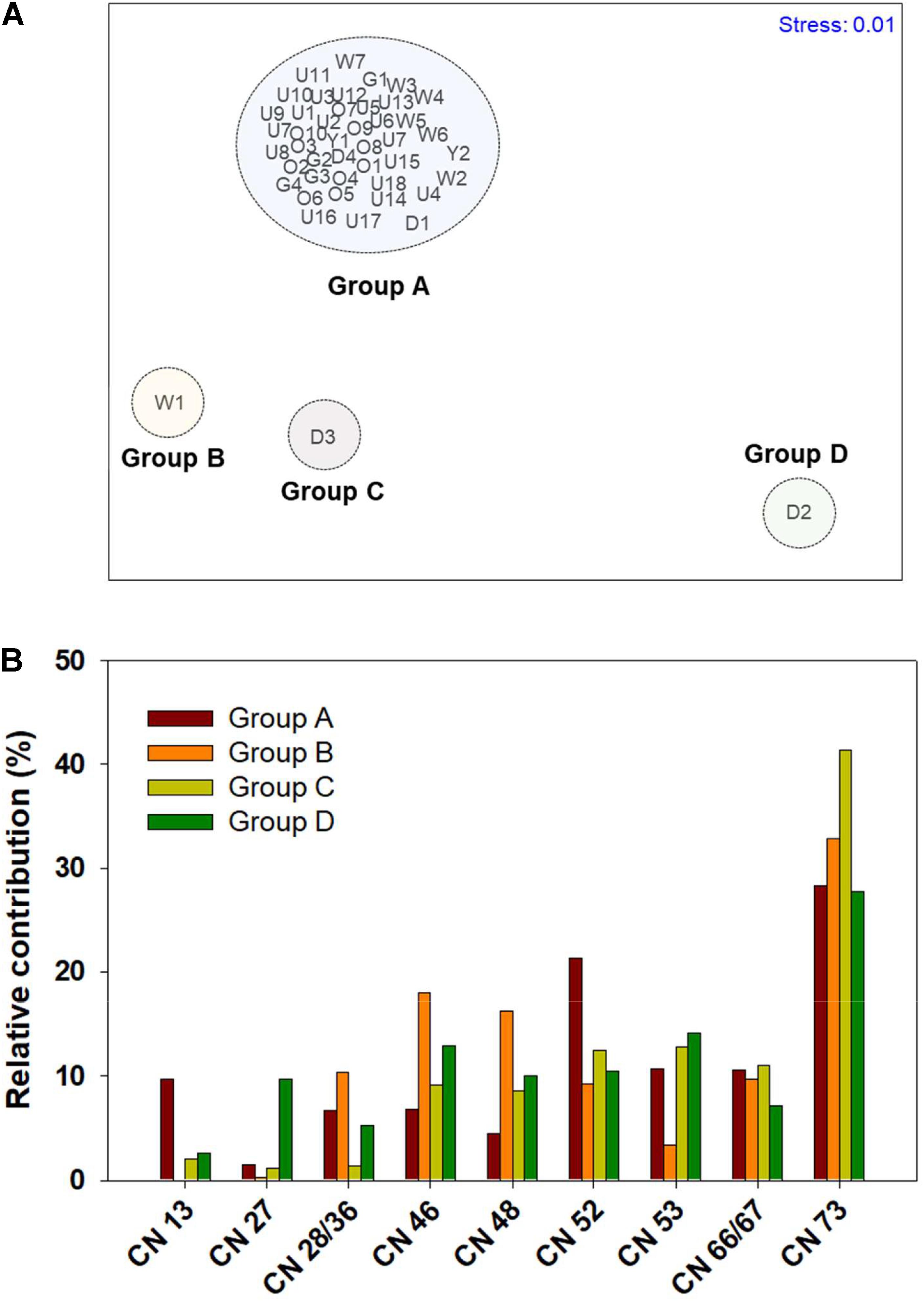
Figure 5. (A) Plot of non-parametric multidimensional scaling (nMDS) ordination for polychlorinated naphthalene (PCN) congeners in sediment from industrialized bays and their surrounding rivers and streams of Korea. (B) Comparison of relative congener profiles of PCNs for each group (Groups A, B, C, and D) clustered by nMDS ordination.
Ecotoxicological Implications
Several sediment quality guidelines (SGQs) have been developed for assessing the association between dioxin-like contaminant levels and biological significance (Canadian Council of Ministers of the Environment, 2014; Walker et al., 2015). The Canadian Council of Ministers of the Environment (CCME) has proposed the interim SQGs (ISQGs; 0.85 pg TEQ/g dry wt) and probable effect levels (PELs; 21.5 pg TEQ/g dry wt) as screening tools to assess the potential health risks to aquatic life derived from dioxin-like contaminants (Zhao et al., 2011; Walker et al., 2015). Due to the lack of available SQGs for only PCNs, the SQGs of PCDD/Fs expressed as TEQs were employed for the assessment of ecotoxicological concerns of PCNs. In our study, only three sediment samples from Daejeong Streams (D2; 18.6 and D3: 2.27 pg TEQ/g dry wt) and Woihwang River (W1; 1.35 pg TEQ/g dry wt) exceeded the ISQGs, implying limited health risks to benthic organisms (Supplementary Figure 2). Moreover, no sediments exceeded the PELs. Despite this, our previous studies have reported higher levels of TEQs by PCDD/Fs and dioxin-like PCBs in Ulsan Bay sediments, which showed 3.01 ± 0.95 and 5.40 ± 8.11 pg TEQ/g dry wt for PCDD/Fs and dioxin-like PCBs, respectively (Moon et al., 2008b). The study surveyed the dioxin-like contaminants in the sediments from only central parts of Ulsan Bay. Thus, the higher concentrations of PCDD/Fs and dioxin-like PCBs are expected in sediments from inner parts of bays, rivers, and streams. Kim et al. (2019b) also reported the newly identified dioxin-like contaminants, such as new polycyclic aromatic hydrocarbons, in sediments from tributaries of Ulsan and Onsan Bays. Our findings indicate the cumulative risks of dioxin-like contaminants to inhabiting species in the sedimentary environments surveyed.
Conclusion
Analytical methods for the determination of 18 PCN congeners in sediment samples were optimized with a multi-layer silica gel column and a GC-MS/MS. PCNs were detectable in all sediment samples from the industrialized coastal zone, indicating widespread contamination by PCNs in the coastal environments. The concentrations of PCNs and TEQs were within the ranges of those reported for other countries. Gradual decrease in concentrations of PCNs were observed from inner to outer parts of the streams, rivers, and bays, indicating that sedimentary PCN distribution is influenced by proximity to industrial complexes. The highest concentrations of PCNs were observed in sediment close to non-ferrous and petrochemical industries. Predominant congeners, diagnostic ratios, and multivariate statistical analysis showed that PCNs primarily originate from thermal-related emission and the use of PCB technical mixtures. The concentrations of PCNs in sediment could be contributed to the cumulative risks by dioxin-like contaminants, which may exceed the SQGs. A comprehensive ecotoxicological investigation on dioxin-like toxicity is required to protect inhabiting species in the coastal environments surveyed.
Data Availability Statement
The original contributions presented in the study are included in the article/Supplementary Material, further inquiries can be directed to the corresponding author/s.
Author Contributions
H-HL collected the sediment samples in the field, measured target contaminants in the samples, and wrote the draft of the manuscript. SL developed and validated the analytical methods of target contaminants. JSL processed and analyzed the chemical data and critically reviewed the draft of the manuscript. H-BM processed all analytical data and edited the manuscript for journal submission. All authors contributed to the article and approved the submitted version.
Funding
This study was supported by the projects entitled “Development of Techniques for Assessment and Management of Hazardous Chemicals in the Marine Environment” and “Development of Technology for Impact Assessment and Management of HNS Discharged from Marine Industrial Facilities” funded by the Ministry of Oceans and Fisheries (MOF), Korea.
Conflict of Interest
JSL was employed by the company NeoEnBiz Co.
The remaining authors declare that the research was conducted in the absence of any commercial or financial relationships that could be construed as a potential conflict of interest.
Publisher’s Note
All claims expressed in this article are solely those of the authors and do not necessarily represent those of their affiliated organizations, or those of the publisher, the editors and the reviewers. Any product that may be evaluated in this article, or claim that may be made by its manufacturer, is not guaranteed or endorsed by the publisher.
Supplementary Material
The Supplementary Material for this article can be found online at: https://www.frontiersin.org/articles/10.3389/fmars.2021.754278/full#supplementary-material
References
Agunbiade, I. V., Adeniji, A. O., Okoh, A. I., and Okoh, O. O. (2020). A review on occurrence and analytical procedures for the evaluation of polychlorinated naphthalenes in human and environmental matrices. Environ. Pollut. Bioavailab. 32, 154–174.
Behnisch, P. A., Hosoe, K., and Sakai, S. I. (2003). Brominated dioxin-like compounds: In vitro assessment in comparison to classical dioxin-like compounds and other polyaromatic compounds. Environ. Int. 29, 861–877. doi: 10.1016/s0160-4120(03)00105-3
Bidleman, T. F., Helm, P. A., Braune, B. M., and Gabrielsen, G. W. (2010). Polychlorinated naphthalenes in polar environments–A review. Sci. Total Environ. 408, 2919–2935. doi: 10.1016/j.scitotenv.2009.09.013
Canadian Council of Ministers of the Environment (2014). Sediment quality guidelines for the protection of aquatic life. Winnipeg: Canadian Council of Ministers of the Environment.
Castells, P., Parera, J., Santos, F. J., and Galceran, M. T. (2008). Occurrence of polychlorinated naphthalenes, polychlorinated biphenyls and short-chain chlorinated paraffins in marine sediments from Barcelona (Spain). Chemosphere 70, 1552–1562. doi: 10.1016/j.chemosphere.2007.08.034
Dat, N. D., Chang, K. S., Wu, C. P., Chen, Y. J., Tsai, C. L., Chi, K. H., et al. (2019). Measurement of PCNs in sediments collected from reservoir and river in northern Taiwan. Ecotoxicol. Environ. Saf. 174, 384–389. doi: 10.1016/j.ecoenv.2019.02.087
Falandysz, J. (1998). Polychlorinated naphthalenes: An environmental update. Environ. Pollut. 101, 77–90. doi: 10.1016/s0269-7491(98)00023-2
Falandysz, J., Chudzyński, K., Takekuma, M., Yamamoto, T., Noma, Y., Hanari, N., et al. (2008). Multivariate analysis of identity of imported technical PCN formulation. J. Environ. Sci. Health C 43, 1381–1390. doi: 10.1080/10934520802232022
Falandysz, J., Fernandes, A., Gregoraszczuk, E., and Rose, M. (2014). The toxicological effects of halogenated naphthalenes: a review of aryl hydrocarbon receptor-mediated (dioxin-like) relative potency factors. J. Environ. Sci. Health. C 32, 239–272. doi: 10.1080/10590501.2014.938945
Falandysz, J., Nose, K., Ishikawa, Y., Łukaszewicz, E., Yamashita, N., and Noma, Y. (2006). HRGC/HRMS analysis of chloronaphthalenes in several batches of Halowax 1000, 1001, 1013, 1014 and 1099. J. Environ. Sci. Health A 41, 2237–2255. doi: 10.1080/10934520600872748
Gu, W., Li, X., Du, M., Ren, Z., Li, Q., and Li, Y. (2021). Identification and regulation of ecotoxicity of polychlorinated naphthalenes to aquatic food Chain (green algae-Daphnia magna-fish). Aquat. Toxicol. 233:105774. doi: 10.1016/j.aquatox.2021.105774
Helm, P. A., Kannan, K., and Bidleman, T. F. (2006). “Polychlorinated naphthalenes in the Great Lakes,” in Persistent Organic Pollutants in the Great Lakes, ed. R. A. Hites (Berlin: Springer), 267–306. doi: 10.1007/698_5_043
Horii, Y., Kannan, K., Petrick, G., Gamo, T., Falandysz, J., and Yamashita, N. (2005). Congener-specific carbon isotopic analysis of technical PCB and PCN mixtures using two-dimensional gas chromatography-isotope ratio mass spectrometry. Environ. Sci. Technol. 39, 4206–4212. doi: 10.1021/es050133q
Hu, J. C., Wu, J., Xu, C. Y., and Jin, J. (2021). Characterization and health risks of PCDD/Fs, PCBs, and PCNs in the soil around a typical secondary copper smelter. Huan. Jing. Ke. Xue. 42, 1141–1151. doi: 10.13227/j.hjkx.202009052
Hu, J., Zheng, M., Liu, W., Li, C., Nie, Z., Liu, G., et al. (2013). Characterization of polychlorinated naphthalenes in stack gas emissions from waste incinerators. Environ. Sci. Pollut. Res. 20, 2905–2911. doi: 10.1007/s11356-012-1218-0
Huang, C., Zeng, Y., Luo, X., Ren, Z., Lu, Q., Tian, Y., et al. (2020). Tracing the sources and microbial degradation of PCBs in field sediments by a multiple-line-of-evidence approach including compound-specific stable isotope analysis. Wat. Res. 182:115977. doi: 10.1016/j.watres.2020.115977
Huang, J., Yu, G., Yamaguchi, M., Matsumura, T., Yamazaki, N., and Weber, R. (2015). Congener-specific analysis of polychlorinated naphthalenes (PCNs) in the major Chinese technical PCB formulation from a stored Chinese electrrical capacitor. Envrion. Sci. Pollut. Res. 22, 14471–14477. doi: 10.1007/s11356-014-3677-y
Kim, J., Hong, S., Cha, J., Lee, J., Kim, T., Lee, S., et al. (2019b). Newly identified AhR-active compounds in the sediments of an industrial area using effect-directed analysis. Environ. Sci. Technol. 53, 10043–10052. doi: 10.1021/acs.est.9b02166
Kim, K. W., Choo, G., Cho, H. S., Lee, B. C., Park, K., and Oh, J. E. (2019a). The occurrence and distribution of polychlorinated naphthalenes (PCNs), focusing on tissue-specific bioaccumulation in crucian carp in South Korea. Sci. Total Environ. 665, 484–491. doi: 10.1016/j.scitotenv.2019.01.238
Kim, S., Kim, Y., and Moon, H.-B. (2021). Contamination and historical trends of legacy and emerging plasticizers in sediment from highly industrialized bays of Korea. Sci. Total Environ. 765:142751. doi: 10.1016/j.scitotenv.2020.142751
Lee, H.-K., Lee, S., Lim, J.-E., and Moon, H.-B. (2020). Legacy and novel flame retardants in water and sediment from highly industrialized bays of Korea: Occurrence, source tracking, decadal time trend, and ecological risks. Mar. Pollut. Bull. 160:111639. doi: 10.1016/j.marpolbul.2020.111639
Lee, S. C., Harner, T., Pozo, K., Shoeib, M., Wania, F., Muir, D. C., et al. (2007). Polychlorinated naphthalenes in the global atmospheric passive sampling (GAPS) study. Environ. Sci. Technol. 41, 2680–2687. doi: 10.1021/es062352x
Lee, S.-Y., Lee, S., Choi, M., Kannan, K., and Moon, H.-B. (2018). An optimized method for the analysis of cyclic and linear siloxanes and their distribution in surface and core sediments from industrialized bays in Korea. Environ. Pollut. 236, 111–118. doi: 10.1016/j.envpol.2018.01.051
Li, C., Li, J., Lyu, B., Wu, Y., Yang, L., Zheng, M., et al. (2021). Burden and risk of polychlorinated naphthalenes in Chinese human milk and a global comparison of human exposure. Environ. Sci. Technol. 55, 6804–6813. doi: 10.1021/acs.est.1c00605
Li, F., Jin, J., Gao, Y., Geng, N., Tan, D., Zhang, H., et al. (2016b). Occurrence, distribution and source apportionment of polychlorinated naphthalenes (PCNs) in sediments and soils from the Liaohe River Basin. China Environ. Pollut. 211, 226–232. doi: 10.1016/j.envpol.2015.09.055
Li, F., Jin, J., Tan, D., Xu, J., Dhanjai, N. Y., Zhang, H., et al. (2016a). High performance solid-phase extraction cleanup method coupled with gas chromatography-triple quadrupole mass spectrometry for analysis of polychlorinated naphthalenes and dioxin-like polychlorinated biphenyls in complex samples. J. Chromato. A 1448, 1–8. doi: 10.1016/j.chroma.2016.04.037
Li, Q., Cheng, X., Wang, Y., Cheng, Z., Guo, L., Li, K., et al. (2017). Impacts of human activities on the spatial distribution and sources of polychlorinated naphthalenes in the middle and lower reaches of the Yellow River. Chemosphere 176, 369–377. doi: 10.1016/j.chemosphere.2017.02.130
Liu, A., Jia, J., Lan, J., Zhao, Z., and Yao, P. (2018). Distribution, composition, and ecological risk of surface sedimental polychlorinated naphthalenes in the East China Sea. Mar. Pollut. Bull. 135, 90–94. doi: 10.1016/j.marpolbul.2018.07.017
Liu, G., Cai, Z., and Zheng, M. (2014). Sources of unintentionally produced polychlorinated naphthalenes. Chemosphere 94, 1–12. doi: 10.1016/j.chemosphere.2013.09.021
Liu, G., Zheng, M., Lv, P., Liu, W., Wang, C., Zhang, B., et al. (2010). Estimation and characterization of polychlorinated naphthalene emission from coking industries. Environ. Sci. Technol. 44, 8156–8161. doi: 10.1021/es102474w
Mahmood, A., Malik, R. N., Li, J., and Zhang, G. (2014). Congener specific analysis, spatial distribution and screening-level risk assessment of polychlorinated naphthalenes in water and sediments from two tributaries of the River Chenab. Pakis. Sci. Total Environ. 485, 693–700. doi: 10.1016/j.scitotenv.2014.03.118
McGoldrick, D. J., Pelletier, M., De Solla, S. R., Marvin, C. H., and Martin, P. A. (2018). Legacy of legacies: Chlorinated naphthalenes in Lake Trout, Walleye, Herring Gull eggs and sediments from the Laurentian Great Lakes indicate possible resuspension during contaminated sediment remediation. Sci. Total Environ. 634, 1424–1434. doi: 10.1016/j.scitotenv.2018.04.077
Meijer, S. N., Harner, T., Helm, P. A., Halsall, C. J., Johnston, A. E., and Jones, K. C. (2001). Polychlorinated naphthalenes in UK soils: time trends, markers of source, and equilibrium status. Environ. Sci. Technol. 35, 4205–4213. doi: 10.1021/es010071d
Moon, H.-B., Choi, H.-G., Lee, P.-Y., and Ok, G. (2008b). Congener-specific characterization and sources of polychlorinated dibenzo-p-dioxins, dibenzofurans and dioxin-like polychlorinated biphenyls in marine sediments from industrialized bays of Korea. Environ. Toxicol. Chem. 27, 323–333. doi: 10.1897/07-337R.1
Moon, H.-B., Kannan, K., Lee, S.-J., and Choi, M. (2007). Polybrominated diphenyl ethers (PBDEs) in marine sediments from industrialized bays of Korea. Mar. Pollut. Bull. 54, 1402–1412. doi: 10.1016/j.marpolbul.2007.05.024
Moon, H.-B., Yoon, S.-P., Jung, R.-H., and Choi, M. (2008a). Wastewater treatment plants (WWTPs) as a source of sediment contamination by toxic organic pollutants and fecal sterols in a semi-enclosed bay in Korea. Chemosphere 73, 880–889. doi: 10.1016/j.chemosphere.2008.07.038
Nadal, M., Schuhmacher, M., and Domingo, J. L. (2007). Levels of metals, PCBs, PCNs and PAHs in soils of a highly industrialized chemical/petrochemical area: Temporal trend. Chemosphere 66, 267–276. doi: 10.1016/j.chemosphere.2006.05.020
Nadal, M., Schuhmacher, M., and Domingo, J. L. (2011). Long-term environmental monitoring of persistent organic pollutants and metals in a chemical/petrochemical area: Human health risks. Environ. Pollut. 159, 1769–1777. doi: 10.1016/j.envpol.2011.04.007
Noma, Y., Minetomatsu, K., Falandysz, J., Swietojańska, A., Flisak, M., Miyaji, K., et al. (2005). By-side impurities in chloronaphthalene mixtures of the Halowax series: All 75 chlorodibenzo-p-dioxins. J. Environ. Sci. Health A Toxicol. Hazard. Subst. Environ. Eng. 40, 77–89. doi: 10.1081/ese-200033581
Odabasi, M., Dumanoglu, Y., Kara, M., Altiok, H., Elbir, T., and Bayram, A. (2017). Polychlorinated naphthalene (PCN) emissions from scrap processing steel plants with electric-arc furnaces. Sci. Total Environ. 574, 1305–1312. doi: 10.1016/j.scitotenv.2016.08.028
Pagano, J. J., and Garner, A. J. (2021). Polychlorinated naphthalenes across the great lakes: Lake trout and walleye concentrations, trends, and teq assessment-2004-2018. Environ. Sci. Technol. 55, 2411–2421. doi: 10.1021/acs.est.0c07507
Park, H., Kang, J.-H., Baek, S.-Y., and Chang, Y.-S. (2010). Relative importance of polychlorinated naphthalenes compared to dioxins, and polychlorinated biphenyls in human serum from Korea: Contribution to TEQ and potential sources. Environ. Pollut. 158, 1420–1427. doi: 10.1016/j.envpol.2009.12.039
Park, M.-K., Cho, H.-K., Cho, I.-G., Lee, S.-E., and Choi, S.-D. (2021). Contamination characteristics of polychlorinated naphthalenes in the agricultural soil of two industrial cities in South Korea. Chemosphere 273:129721. doi: 10.1016/j.chemosphere.2021.129721
Shen, J., Yang, L., Liu, G., Zhao, X., and Zheng, M. (2021). Occurrence, profiles, and control of unintentional POPs in the steelmaking industry: A review. Sci. Total Environ. 773:145692. doi: 10.1016/j.scitotenv.2021.145692
Suzuki, G., Michinaka, C., Matsukami, H., Noma, Y., and Kajiwara, N. (2020). Validity of using a relative potency factor approach for the risk management of dioxin-like polychlorinated naphthalenes. Chemosphere 244:125448. doi: 10.1016/j.chemosphere.2019.125448
Van den Berg, M., Birnbaum, L. S., Denison, M., De Vito, M., Farland, W., Feeley, M., et al. (2006). The 2005 World Health Organization reevaluation of human and mammalian toxic equivalency factors for dioxins and dioxin-like compounds. Toxicol. Sci. 93, 223–241. doi: 10.1093/toxsci/kfl055
Waheed, S., Khan, M. U., Sweetman, A. J., Jones, K. C., Moon, H.-B., and Malik, R. N. (2020). Exposure of polychlorinated naphthalenes (PCNs) to Pakistani populations via non-dietary sources from neglected e-waste hubs: A problem of high health concern. Environ. Pollut. 259:113838. doi: 10.1016/j.envpol.2019.113838
Walker, T. R., Willis, R., Gray, T., McClean, B., McMillan, S., Leroy, M., et al. (2015). Ecological risk assessment of sediments in Sydney Harbour, Nova Scotia, Canada. Soil Sed. Contam. 24, 471–493. doi: 10.1080/15320383.2015.982244
Wang, Y., Cheng, Z., Li, J., Luo, C., Xu, Y., Li, Q., et al. (2012). Polychlorinated naphthalenes (PCNs) in the surface soils of the Pearl River Delta, South China: Distribution, sources, and air-soil exchange. Environ. Pollut. 170, 1–7. doi: 10.1016/j.envpol.2012.06.008
Wu, J., Hu, J., Wang, S., Jin, J., Wang, R., Wang, Y., et al. (2018). Levels, sources, and potential human health risks of PCNs, PCDD/Fs, and PCBs in an industrial area of Shandong Province, China. Chemosphere 199, 382–389. doi: 10.1016/j.chemosphere.2018.02.039
Wu, X., Wu, B., Jiang, M., Chang, F., Nan, Q., Yu, X., et al. (2020). Distribution, sources and burial flux of sedimentary organic matter in the East China Sea. J. Oceanol. Limnol. 38, 1488–1501. doi: 10.1007/s00343-020-0037-2
Yamamoto, T., Higashino, K., Abe, T., Takasuga, T., Takemori, H., Weber, R., et al. (2018). PCDD/PCDF formation in the chlor-alkali process-Laboratory study and comparion with patterns from contaminated sites. Envrion. Sci. Pollut. Res. 25, 31874–31884. doi: 10.1007/s11356-017-0777-5
Zhang, L., Zhang, L., Dong, L., Huang, Y., and Li, X. (2015). Concentrations and patterns of polychlorinated naphthalenes in surface sediment samples from Wuxi, Suzhou, and Nantong, in East China. Chemosphere 138, 668–674. doi: 10.1016/j.chemosphere.2015.07.045
Keywords: TEQ, non-ferrous, sediment quality, dioxin-like, cumulative risk, PCB
Citation: Lee H-H, Lee S, Lee JS and Moon H-B (2021) Distribution of Polychlorinated Naphthalenes in Sediment From Industrialized Coastal Waters of Korea With the Optimized Cleanup and GC-MS/MS Methods. Front. Mar. Sci. 8:754278. doi: 10.3389/fmars.2021.754278
Received: 06 August 2021; Accepted: 11 November 2021;
Published: 07 December 2021.
Edited by:
Kenneth Mei Yee Leung, City University of Hong Kong, Hong Kong SAR, ChinaReviewed by:
Guijin Su, Research Center for Eco-Environmental Sciences, Chinese Academy of Sciences (CAS), ChinaJinping Cheng, Hong Kong University of Science and Technology, Hong Kong SAR, China
Copyright © 2021 Lee, Lee, Lee and Moon. This is an open-access article distributed under the terms of the Creative Commons Attribution License (CC BY). The use, distribution or reproduction in other forums is permitted, provided the original author(s) and the copyright owner(s) are credited and that the original publication in this journal is cited, in accordance with accepted academic practice. No use, distribution or reproduction is permitted which does not comply with these terms.
*Correspondence: Hyo-Bang Moon, aGJtb29uQGhhbnlhbmcuYWMua3I=