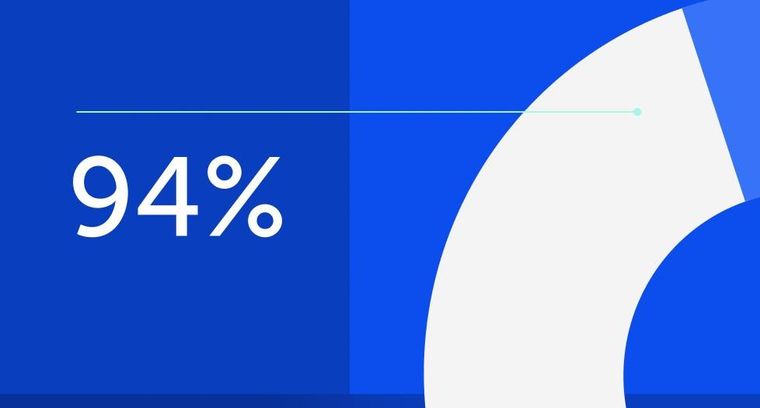
94% of researchers rate our articles as excellent or good
Learn more about the work of our research integrity team to safeguard the quality of each article we publish.
Find out more
ORIGINAL RESEARCH article
Front. Mar. Sci., 01 October 2021
Sec. Aquatic Physiology
Volume 8 - 2021 | https://doi.org/10.3389/fmars.2021.751401
Abalone (Haliotis spp.) are typical nocturnal creatures but Haliotis discus hannai is bold and active in the nighttime whereas H. gigantea tends to be timid and inactive. In this study, we quantified and compared differences in movement, feeding, and digestive physiology between H. discus hannai and H. gigantea as well as the potential molecular mechanisms on the basis of video observations and expression levels of genes related to feeding regulation. The feeding behaviors of both species were characterized by significant circadian rhythms (P < 0.05). However, the distance moved and the cumulative duration of movement were 2.61 and 1.94 times higher, respectively, in H. discus hannai than in H. gigantea over the 24-h cycle. The cumulative duration of feeding by H. discus hannai was only 1.15 times that by H. gigantea, but the feeding time as a percentage of the cumulative duration of movement (FTP) was up to 94.6% for H. gigantea and only 56.0% for H. discus hannai. The peaks for α-amylase activity and NPF expression levels in both species as well as the peak OX2R expression level in H. gigantea occurred during 20:00–00:00 h. By contrast, the peaks for alginate lyase activity and NPYR expression levels in H. discus hannai occurred at 16:00 h, when the FTP was significantly higher for H. discus hannai than for H. gigantea. These initial findings quantify specific behavior parameters and thus provide a reference for the selection of appropriate feeding strategies and the proliferation of abalone via bottom sowing.
Pacific abalone (Haliotis discus hannai) is one of the most economically important marine shellfish in China and is widely distributed in the Yellow Sea and along the coastline of the Northwest Pacific. In 2019, aquaculture production of abalone in China increased to 180,300 tons (China Bureau of Fisheries, 2020), accounting for approximately 90% of the world’s gross production. Owing to global climate change, summer heat-induced mortality of abalone is frequent in Fujian Province, which is the primary aquaculture region. To achieve healthy and sustainable development of the aquaculture industry, Haliotis gigantea, a species distributed along the coastline of Japan and South Korea, was introduced into China in 2003, which reduced the risk level for the national aquaculture industry (Luo et al., 2006). H. discus hannai is a bold and active nocturnal creature that rapidly crawls around after leaving the water before searching for a place to hide (Gao et al., 2020). By contrast, H. gigantea is a timid and inactive creature that closely adheres to the substrate instead of crawling around after leaving the water. The movement behaviors of the two species cannot be evaluated impartially via experience-based assessments and subjective descriptions. Thus, quantification of the behavioral characteristics and circadian feeding rhythms of nocturnal abalone is considered crucial for the development of a reasonable feeding strategy.
Determination of feeding rhythms is a critical part of feeding behavior research. The diel feeding peak of a farmed animal can be determined on the basis of behavioral observations. For example, the feeding peak for Dicentrarchus labrax occurs during 16:00–17:00 h (Azzaydi et al., 1998). Sun et al. (2015) observed the feeding rhythm of Apostichopus japonicus at three body sizes using an infrared photography system. They found that all three sizes took food during the night and the feeding peak occurred at 02:00–04:00 h, but large (length 13.6 ± 1.5 cm) and medium (8.4 ± 1.3 cm) sized individuals had a second feeding peak during the day. According to field observations, Trichodactylus borellianus adults have two feeding peaks, at noon and midnight (de Azevedo Carvalho et al., 2013). Lopez-Uriostegui et al. (2017) investigated the stomach repletion rhythms of caridean shrimp and showed that the feeding intensity of Macrobrachium americanum was higher during the day whereas that of Macrobrachium tenellum was higher at night. The feeding cycle of Anodonta anatina was found to cover 36 h, during which they keep their shells open for 20 h to filter food in the presence of algae, before closing the shells and then resting for 16 h (Hartmann et al., 2016). The feeding behaviors of Ruditapes philippinarum and Mytilus edulis are also characterized by circadian rhythms: they both open their shells at night to actively take in food over a cycle of 24 h (Robson et al., 2010; Houki et al., 2015). The diel feeding patterns of Laternula marilina and Meretrix meretrix can be divided into three stages: a high feeding proportion stage from 00:00 to 08:00, a low feeding proportion stage from 12:00 to 20:00, and another stage that varies between low and high feeding proportions (Zhuang, 2005, 2006). H. discus hannai and H. gigantea have been artificially reared for many years, but few studies have investigated the feeding and digestion physiology of the two species, although these basic data are important for the development of a reasonable feeding strategy.
Digestive enzyme activity can be used as a measure to indicate the level of digestive activity of an animal. Close relationships between the intensive period for digestive physiology and the peak period for feeding activities have been reported in previous studies. For example, Guerra-Santos et al. (2017) demonstrated synchronization of the diel feeding time and digestive enzyme rhythms in Oreochromis niloticus. Several studies of fish and marine invertebrates have suggested that secretion of digestive enzymes increased before the anticipated diel feeding peak in preparation for the upcoming feeding activity (Vera et al., 2007; Montoya et al., 2010; del Pozo et al., 2012; Sun et al., 2015). However, it is also possible that food may stimulate the digestive system to secrete more digestive enzymes after feeding, that is, the peak period for digestive enzymes may occur later than that for feeding (Bolasina et al., 2006). At present, it is not known whether the secretion of digestive enzymes exhibits diel periodicity in H. discus hannai and H. gigantea, or whether their secretion is associated with feeding behavior rhythms.
Appetite is another critical factor that can influence the feeding behavior of animals. Orexigenic and anorexigenic appetite-regulating factors can dynamically regulate the nervous system and digestive system. Neuropeptide F (NPF) is a homolog of the vertebrate neuropeptide Y (NPY) and has significant functions in feeding regulation in invertebrates (including mollusks) including stimulation of appetite and feeding behavior (de Bono and Bargmann, 1998; Wu et al., 2003, 2005; Nässel and Wegener, 2011). A study by Li et al. (2018) showed that injection of NPF double-stranded RNA into Acyrthosiphon pisum significantly suppressed expression levels of the gene and dramatically reduced the proportion of time spent feeding (PTSF). Orexin is a neurotransmitter secreted by the nerve center and it stimulates feeding activity and maintains the energy balance (Kukkonen, 2013). For example, Shimizu et al. (2014) showed that intracerebroventricular injection of orexin A into Rana catesbeiana larvae significantly increased PTSF. Thus, identification of how NPF and orexin are secreted as orexigenic factors during the day and night could provide insights into the mechanism responsible for the periodic feeding rhythm in abalone. Therefore, the aim of the present study was to quantify the movement characteristics and feeding rhythms of H. discus hannai and H. gigantea during the day and night on the basis of data acquired with a video surveillance system. We also compared diel variations in intestinal digestive enzyme activities and the expression levels of genes related to feeding activity in the two species to determine connections between their feeding behavior and physiology. The results obtained in this study enhance our basic biological knowledge regarding abalone, and could facilitate the development of a reasonable feeding strategy and improve the proliferation of abalone via bottom sowing.
Abalone (Haliotis discus hannai Ino, shell length: 6.92 ± 0.37 cm, body weight: 46.14 ± 6.44 g; Haliotis gigantea Gmelin, shell length: 6.53 ± 0.28 cm, body weight: 31.88 ± 3.13 g) were purchased from Fuda Abalone Aquafarm (Jinjiang, Fujian, China). All experimental abalone were sourced from the same batch after artificial hatching. Before experiments, abalone were placed in four culture tanks (70 cm × 40 cm × 30 cm) with a predefined light cycle of 12 h light:12 h dark to acclimate for 10 days. The seawater was exchanged once a day and continuous aeration was provided. The tank conditions were as follows: water temperature = 18.7 ± 0.5°C; salinity = 30 ± 1; pH = 8.3; and dissolved oxygen concentration > 6 mg L–1. The culture water was sourced from a natural sea area and was used after sedimentation and sand filtration. Fresh Gracilariopsis lemaneiformis was provided as food every day at 5% of the wet weight of abalone to ensure that they reached a state of satiation.
Before behavioral experiments, a digital label was fixed to the shell of each abalone to allow differentiation. Abalone were placed in a cylindrical experiment tank (polyethylene material: diameter 40 cm, height 30 cm) 2 h before the start of the experiment to allow them to adapt to the experimental environment. Each experiment involved 10 abalone and was conducted for a duration of 24 h, starting at 12:00 h. We recorded the distance moved, cumulative duration of movement (CDM), mean velocity of movement (VMmean), and maximum velocity of movement (VMmax) for the abalone every 4 h (in the following, 12:00 is denoted as ZT0, and 16:00, 20:00, 00:00, and 4:00, 8:00, and 12:00 on the next day are denoted as ZT4, ZT8, ZT12, ZT16, ZT20, and ZT24, respectively). The experimental unit was covered with a blackout cloth to provide shade from external lighting. The light source was a built-in light-emitting diode and the light cycle was the same as that applied during acclimation. The rearing unit was equipped with a recirculating water system and supplied with continuous aeration. The dissolved oxygen concentration in the water was always maintained above 6 mg L–1. Fresh G. lemaneiformis was substituted at an amount equal to 3% of the wet weight of abalone at 17:00 pm daily. Ten abalone were used in each experiment, with each species tested in three repeated trials, for a total of 30 abalone. After each trial, the abalone were transferred to another aquarium bank for acclimation, and thus the experimental abalone were not reused in the behavioral experiments.
The movement behaviors of abalone were recorded with an infrared camera (HIKVISION, Hangzhou, China) located above the center of the experimental tank. The field of vision of the camera reached the bottom outer edge of the experimental tank. The light intensity at the bottom of the experimental tank was approximately 31.04 ± 9.73 lx. Experimental videos were collected and stored using a video surveillance unit. The light period was controlled with a clock controller at 12 h light:12 h dark. Video recordings of behaviors were analyzed using EthoVision XT 9.0 behavior analysis software (Noldus Information Technology, Wageningen, Netherlands). The video settings were as follows: total recording time = 24 h; time interval = 5 s; video format = AVI; video resolution = 680 × 480 pixels; and frame speed = 10 fps.
Ten abalone were selected and placed in the experimental tank described above for each experiment. PTSF and the cumulative duration of feeding (CDF) by H. discus hannai and H. gigantea were compared in a continuous 24-h period. These indexes were used to calculate the feeding rhythm percentage (day vs. dark) and the percentage CDF relative to the CDM. Each species was tested in three repeated trials, for a total of 30 abalone, fed in the same manner described above. We determined whether the abalone had fed or not according to their positions in the tank and the reduction in the proportion of food available from video data. The number of abalone that fed was recorded every 30 min, and eight consecutive feeding values based on 30-min intervals were used to generate a single mean value every 4 h. The feeding rhythm was assessed and analyzed according to the percentage of fed abalone (the number of abalone fed as a percentage of all abalone) in each period.
After the behavioral experiment, the abalone were fed for an extra week under the same settings used for acclimation. Next, from 12:00 onward, four abalone each for H. discus hannai and H. gigantea were taken every 4 h, transferred to an ice pan, and dissected to obtain their intestines. Residual food and feces were removed with tweezers, before separately placing the intestines of each abalone into a 2-mL freezer tube, which was stored under liquid nitrogen and transferred to an ultra-low temperature refrigerator (−80°C) for storage. The stored intestines were subsequently used to assay pepsase, α-amylase, and alginate lyase activity and the expression levels of feeding-related genes.
For assays, samples of the abalone intestines were weighed, homogenization buffer was added at nine times the mass of the sample, the tissue was processed at 4°C in a tissue homogenizer (DHS Life Science & Technology Co., Ltd., China), and the sample was then centrifuged (3,000 rpm, 10 min, 4°C). The supernatant was retained for testing. The total protein content of each homogenate was determined using a quantitative assay kit (code A045-2-2, Nanjing Jiancheng Bioengineering Institute, China) with Coomassie Brilliant Blue. The activities of pepsase, α-amylase, and alginate lyase were determined using a Micro Pepsase Assay Kit (code BC2325, Solarbio, China), α-Amylase Assay Kit (code C016-1-1, Nanjing Jiancheng Bioengineering Institute, China; based on iodine–starch colorimetry), and the 3,5-dinitrosalicylic acid method (Cao, 2016), respectively. The absorbance of the samples and standards was measured using a Tecan Infinite M200 Pro Multiscan Spectrum system. The activity unit for pepsase was defined as 1 μmol tyrosine generated by hydrolysis of hemoglobin catalyzed per mg of protein in 1 min at 37°C, which was denoted as 1 U mg prot–1. The activity unit for α-amylase was defined as 1 μmol starch generated by hydrolysis of hemoglobin catalyzed by 1 mg of protein in 3 min at 37°C, denoted as 1 U mg prot–1. The activity unit for alginate lyase was defined as 1 mg glucose generated by hydrolysis of sodium alginate catalyzed by 1 mg of protein in 6 min, denoted as 1 U mg prot–1. The enzyme activities and total protein content of all samples were determined for three technical replicates.
The remaining intestinal tissues stored at −80°C were used to determine the expression levels of feeding-related genes. First, 0.5–1 g of intestine sample from each abalone was transferred to 1 mL of TRIzolTM Reagent (Invitrogen, United States) for total RNA extraction. A NanoDrop 2000 system (Thermo Fisher Scientific, United States) was used to determine the RNA quality and concentration. Total RNA samples were subjected to gel electrophoresis analysis. The gels were photographed using a Bio-Rad Gel Doc XR + system and the quality of the sample extracts was confirmed according to the position and brightness of the bands. Any remaining DNA in the total RNA was removed with RNase-free DNase I (Thermo Fisher Scientific, United States). The RNA was then reverse transcribed to cDNA using a RevertAid First Strand cDNA Synthesis Kit (Thermo Fisher Scientific, United States). Real-time quantitative PCR was conducted with an ABI QuantStudio 6 Flex system. The 20-μL reaction system comprised 10 μL of Applied BiosystemsTM PowerUpTM SYBRTM Green Master Mix (Thermo Fisher Scientific, United States), 5 μL of cDNA template (diluted with double-distilled H2O to an appropriate multiple), 1 μL of forward primer (10 μM), 1 μL of reverse primer (10 μM), and 3 μL of double-distilled H2O. The primers used for fluorescence quantitative detection (Table 1) of NPF, NPYR, and orexin receptor type 2 (OX2R) were designed using the Primer3web website (version 4.1.0)1 by referring to relevant sequences, which we cloned and submitted to GenBank. All of the reaction components were added to a MicroAmpTM Fast Optical 96-Well Reaction Plate with Barcode (0.1 mL, Applied Biosystems, United States) and were evenly mixed and centrifuged. The fluorescence quantitative reaction process involved activation of uracil-DNA glycosylase at 50°C for 2 min, initial denaturation at 95°C for 2 min, and cycling conditions at 95°C for 15 s and 60°C for 1 min for 40 cycles in total. Each target gene in each sample was analyzed on the basis of three technical replicates. The mRNA levels of the target genes were calibrated using the real-time PCR Ct (2–ΔΔCt) relative quantitative method, with the reference gene 18S rRNA as the quantitative standard.
Table 1. Real-time quantitative PCR primers used for feeding-related genes in Haliotis discus hannai and Haliotis gigantea.
All data were statistically analyzed using SPSS version 22.0. Before analyzing the data, their conformance to a normal distribution and homogeneity of variance were validated using the Kolmogorov-Smirnov test and Levene’s test. Differences in movement behavior, digestive enzyme activities, and expression levels of NPF, NPYR, and OX2R between the two species at different sampling time points were analyzed using two-way analysis of variance and Tukey’s multiple comparisons test. Data are expressed as the mean ± standard error (SE). P < 0.05 indicates a significant difference and P < 0.01 a highly significant difference. Data obtained from the analyses were plotted using Microsoft Excel.
Cosine fitting was performed using data for digestive enzyme activities and the expression levels of NPF, NPYR, and OX2R with the Cosinor program package in Matlab. The model used for the calculations is Y = M + Acos (ωt + Φ), where M (MESO) represents the median value of the fitted cosine curve; A, ω, and Φ are the amplitude, angular frequency, and peak value of the cosine fitting, respectively; t denotes the circadian time points around 24 h; and Y represents the digestive enzyme activity or expression level of the corresponding gene measured at each point every 4 h. The values of M, A, and Φ in the cosine fitting curves were calculated after fitting (Hoogerwerf et al., 2007). The significance level was set at P < 0.05.
There were significant differences in the distance moved between the two species (Supplementary Table 1, P < 0.01). The distance moved by H. gigantea was significantly lower than that moved by H. discus hannai (P < 0.01) at all-time points except for ZT24. Over 24 h, the summed distance moved by H. discus hannai was 2.61 times the distance moved by H. gigantea. For H. discus hannai, the distance moved at ZT24 was significantly lower than the distances moved at ZT8, ZT12, and ZT16 (P < 0.05), but not significantly different from the distances moved at ZT0, ZT4, and ZT20 (Figure 1A). At ZT0, ZT4, and ZT24, none of the H. gigantea individuals moved, and the distance moved at ZT12 was significantly higher than that at any other time point (Figure 1A). The cosine analysis results suggest that the distances moved by H. discus hannai and H. gigantea exhibited significant circadian rhythms (Table 2, P < 0.05).
Figure 1. Distance moved (A), cumulative duration of movement (B), mean velocity of movement (C), and maximum velocity of movement (D) by Haliotis discus hannai and Haliotis gigantea. Values are expressed as mean ± SE, sample size = 30. Treatments with different letters are significantly different at different sampling times in the same species (P < 0.05). “*” and “**” indicate significant differences (P < 0.05 and P < 0.01, respectively) in movement parameters at the same sampling time in different species. No label indicates no significant difference (P < 0.05) in movement parameters at the same sampling time in the same species.
There were significant differences in CDM between the two species (Supplementary Table 1, P < 0.01). CDM was significantly lower for H. gigantea than for H. discus hannai at all-time points (P < 0.01). There were also significant differences in the cumulative duration of movement at all-time points (Supplementary Table 1, P < 0.01). For H. discus hannai, the CDM at ZT24 was significantly lower than at ZT8, ZT12, ZT16, and ZT20 (P < 0.05). The CDM at ZT12 was significantly higher than at any other time point (Figure 1B). For H. gigantea, no individual moved at ZT0, ZT4, and ZT24 (Figure 1B). The cosine analysis results suggest that the cumulative duration of movement by H. discus hannai and H. gigantea exhibited significant circadian rhythms (Table 2, P < 0.05).
At ZT0, ZT4, ZT8, ZT16, and ZT24, VMmean was significantly lower for H. gigantea than for H. discus hannai (Supplementary Table 1, P < 0.01). At ZT12 and ZT20, VMmean was significantly higher for H. gigantea than for H. discus hannai (P < 0.05). At ZT20, VMmean for H. discus hannai was significantly lower than at any other time point (Figure 1C, P < 0.05). For H. gigantea, VMmean at ZT0, ZT4, and ZT24 was significantly lower than at any other time point (P < 0.05). The maximum VMmean occurred at ZT12 (Figure 1C).
There were significant differences in VMmax between the two species (Supplementary Table 1, P < 0.01). At ZT0, ZT4, ZT8, ZT12, ZT20, and ZT24, VMmax was significantly lower for H. gigantea than for H. discus hannai (P < 0.01). For H. discus hannai, VMmax was at a minimum at ZT24 and at a maximum at ZT12 (Figure 1D). For H. gigantea, VMmax at ZT0, ZT4, and ZT24 was significantly lower than at any other time points (P < 0.05), and was at a maximum at ZT12 (Figure 1D). The cosine analysis results suggest that VMmax for H. discus hannai and H. gigantea exhibited significant circadian rhythms (Table 2, P < 0.05).
There were significant differences in PTSF between the two species (Supplementary Table 1, P < 0.01). At ZT4, ZT8, ZT12, ZT16, and ZT20, PTSF was significantly lower for H. gigantea than for H. discus hannai (Figure 2A, P < 0.01). At ZT0 and ZT24, no H. discus hannai individuals exhibited feeding behavior, but PTSF at ZT12 was significantly higher than at any other time (Figure 2A). For H. gigantea, PTSF at ZT12 was significantly higher than at any other time (Figure 2A). In general, PTSF for H. discus hannai and H. gigantea tended to increase during ZT0–ZT12, but decreased continuously during ZT12–ZT24. PTSF during the day and PTSF at night were significantly higher for H. discus hannai than for H. gigantea (P < 0.01), and PTSF was significantly higher at night than during the day for the two species (P < 0.01; Figure 2B).
Figure 2. Proportion of time spent feeding (A,B), cumulative duration of feeding (C), and percentage of cumulative duration of feeding relative to cumulative duration of movement (D) in Haliotis discus hannai and Haliotis gigantea. Values are expressed as mean ± SE, sample size = 30. Treatments with different letters are significantly different at different sampling times in the same species (P < 0.05). “*” and “**” indicate significant differences (P < 0.05 and P < 0.01, respectively) in the feeding parameters at the same sampling time in different species. No label indicates no significant difference (P < 0.05) in the feeding parameters at the same sampling time in the same species.
There were significant differences in CDF between the two species (Supplementary Table 1, P < 0.01). At ZT8 and ZT12, CDF was significantly lower for H. gigantea than for H. discus hannai (Figure 2C, P < 0.01). Over 24 h, the summed CDF for H. discus hannai was 1.15 times the value for H. gigantea.
There were significant differences in CDF at different sampling times (Supplementary Table 1, P < 0.01). For H. discus hannai, CDF was significantly shorter at ZT0, ZT4, and ZT24 than at any of the other time points (P < 0.05); the maximum value occurred at ZT12 (Figure 2C). For H. gigantea, CDF was significantly longer at ZT12 than at any other time point (Figure 2C). The cosine analysis results suggest that CDF for H. discus hannai and H. gigantea exhibited significant circadian rhythms (Table 2, P < 0.05). The percentage CDF relative to CDM was significantly higher for H. gigantea (94.6%) than for H. discus hannai (P < 0.01; Figure 2D).
There were significant differences in pepsase activity at different sampling times between the two species (Supplementary Table 2, P < 0.01). At ZT4, ZT8, and ZT24, pepsase activity in H. gigantea was significantly higher than in H. discus hannai (P < 0.05). However, there were no significant differences in pepsase activity between the species at ZT0, ZT12, or ZT20 (Figure 3A). The pepsase activity in H. discus hannai at ZT4 was significantly lower than at ZT0, ZT12, ZT16, and ZT20 (P < 0.05), but the activity at each of these time points did not differ significantly from the activity at ZT8 and ZT24 (Figure 3A). In H. gigantea, the pepsase activity at ZT12 was significantly lower than at ZT20, but was not different from the activity at any other time. The cosine analysis results suggest that pepsase activities in H. discus hannai and H. gigantea did not exhibit a circadian rhythm during the day or at night (Table 3, P > 0.05).
Figure 3. Activities of pepsase (A), α-amylase (B), and alginate lyase (C) in Haliotis discus hannai and Haliotis gigantea. Values are expressed as mean ± SE (n = 4). Treatments with different letters are significantly different at different sampling times in the same species (P < 0.05). “*” and “**” indicate significant differences at P < 0.05 and P < 0.01, respectively, in the digestive enzyme activity levels at the same sampling time in different species. No label indicates no significant difference at P < 0.05 in the digestive enzyme activity levels at the same sampling time in the same species.
The α-amylase activity in H. gigantea was significantly lower than in H. discus hannai at ZT12 and ZT16 (P < 0.05), but was significantly higher than in H. discus hannai at ZT24 (Figure 3B, P < 0.01). There were significant differences in α-amylase activity at different time points (Supplementary Table 2, P < 0.01). In H. discus hannai, α-amylase activity at ZT0 and ZT24 was significantly lower than at ZT8, ZT12, and ZT16 (Figure 3B, P < 0.05). The cosine analysis results suggest that the α-amylase activity in H. discus hannai exhibited a circadian rhythm (Table 3, P < 0.05). In H. gigantea, the α-amylase activity at ZT0 was significantly lower than that at any other time point (Figure 3B).
There were significant differences in alginate lyase activity between the two species (Supplementary Table 2, P < 0.01). At ZT8, ZT12, ZT16, and ZT20, the alginate lyase activity in H. gigantea was significantly higher than in H. discus hannai (Figure 3C, P < 0.01). The lowest alginate lyase activity in H. discus hannai occurred at ZT16, but it was not significantly different from the activity at ZT0, ZT12, or ZT20. In H. gigantea, the alginate lyase activity at ZT0 was significantly lower than at ZT8, ZT12, ZT16, and ZT20, but it was not significantly different from the activity at ZT4 or ZT24 (Figure 3C). The cosine analysis results suggest that the alginate lyase activity in H. gigantea exhibited a circadian rhythm (Table 3, P < 0.05).
There were significant differences in NPF expression levels between the two species at different sampling times (Supplementary Table 3, P < 0.01). At ZT0, ZT8, and ZT12, NPF expression in H. gigantea was significantly lower than in H. discus hannai (P < 0.01). At ZT4, NPF expression in H. gigantea was significantly higher than in H. discus hannai (Figure 4A, P < 0.01). In H. discus hannai, the NPF expression level was lowest at ZT16, and the level at ZT8 was significantly higher than that at any other time point (Figure 4A), but NPF expression did not exhibit a significant circadian rhythm. NPF expression in H. gigantea was lowest at ZT12 but it was not significantly different from the level at ZT0, ZT16, or ZT20, and NPF expression did not exhibit a circadian rhythm (Table 4, P > 0.05).
Figure 4. Expression levels of NPF (A), NPYR (B), and OX2R (C) in Haliotis discus hannai and Haliotis gigantea. Values are expressed as mean ± SE (n = 4). Treatments with different letters are significantly different at different sampling times in the same species (P < 0.05). “*” and “**” indicate significant differences at P < 0.05 and P < 0.01, respectively, in the relative expression levels of feeding-related genes at the same sampling time in different species. No label indicates no significant difference at P < 0.05 in the relative expression levels of feeding-related genes at the same sampling time in the same species.
Table 4. Cosinor analysis results obtained based on the relative expression levels of feeding-related genes.
There were significant differences in NPYR expression levels between the two species (Supplementary Table 3, P < 0.01). At ZT12 and ZT16, NPYR expression levels were significantly lower in H. gigantea than in H. discus hannai (P < 0.01). At ZT8, NPYR expression was significantly higher in H. gigantea than in H. discus hannai (P < 0.01), but there were no significant differences in expression at ZT0, ZT20, or ZT24 (Figure 4B). NPYR expression in H. discus hannai was significantly lower at ZT8 than that at any other time point (P < 0.05) except for ZT24, but the expression level at ZT4 was significantly higher than at any other time point except for ZT16 (Figure 4B). In H. gigantea, NPYR expression was lowest at ZT12, but the level at ZT4 was significantly higher than at ZT8, ZT12, ZT16, or ZT20 (Figure 4B), and the expression levels exhibited a significant circadian rhythm (Table 4, P < 0.05).
There were significant differences in OX2R expression between the two species (Supplementary Table 3, P < 0.01). At ZT0, ZT4, ZT8, ZT20, and ZT24, relative expression levels of OX2R were significantly higher in H. gigantea than in H. discus hannai (P < 0.05). At ZT16, OX2R expression in H. gigantea was significantly lower than in H. discus hannai (Figure 4C, P < 0.01). In H. discus hannai, the relative expression level of OX2R at ZT4 was significantly lower than at ZT16, ZT20, or ZT24 (P < 0.05), but was not significantly different from the level at ZT0, ZT8, or ZT12 (Figure 4C). The relative expression level of OX2R in H. gigantea was lowest at ZT12 but did not significantly differ from the level at ZT0 or ZT4. The cosine analysis results suggest that the relative expression levels of OX2R in H. discus hannai and H. gigantea did not exhibit circadian cosine rhythms (Table 4, P > 0.05).
In this study, we found that the feeding behaviors of H. discus hannai and H. gigantea exhibited significant circadian rhythms according to video analysis. The feeding peaks for H. discus hannai and H. gigantea were concentrated at ZT12 (00:00 h), whereas there was barely any feeding at ZT0 (12:00 h) and ZT24 (12:00 h), suggesting that the two species are typical nocturnal animals. We demonstrated the inactivity of H. gigantea from visual observations of the distance moved (the cumulative distance moved by H. discus hannai within 24 h was 2.61 times that moved by H. gigantea) and CDM (CDM by H. discus hannai within 24 h was 1.94 times the CDM by H. gigantea). The crawling distance and duration of movement for H. gigantea were also less than those for H. discus hannai. However, the CDF by H. discus hannai was only 1.15 times the CDF by H. gigantea, confirming that H. discus hannai tended to be more active than H. gigantea.
The feeding rhythms of H. discus hannai and H. gigantea were determined according to the PTSF at each time point via video analysis. Bansemer et al. (2015) investigated the diel feeding rhythms of Haliotis laevigata and H. laevigata × Haliotis rubra by determining their food intake. In particular, under a 12 h light:12 h dark photoperiod, excess food was provided at 16:00 h every day and the abalone food intake was then determined at 19:00, 22:00, 01:00, 04:00, and 08:00 h. After an excess amount of Ulva sp. was added to the tank at 16:00 h, H. laevigata and H. laevigata × H. rubra immediately responded by feeding. From 16:00 to 08:00 h, the two species consumed Ulva sp. at a linear rate. Tahil and Juinio-Menez (1999) used a similar method based on food intake measurement and found that Haliotis asinina also exhibited a significant nocturnal feeding habit: feeding activity appeared to be intense during 18:00–02:00 h, when food (mixed fragments of fresh red algae) was consumed at a relatively stable rate. We found that the nocturnal feeding activities of H. discus hannai and H. gigantea had an obvious peak instead of being evenly distributed across the dark period, and thus their activities appear to be slightly different from those of H. laevigata, H. laevigata × H. rubra, and H. asinina. Other field and laboratory studies of abalone behavior observed every few hours also suggest that the peak feeding periods for Haliotis roei and Haliotis tuberculata occur at night (Wells and Keesing, 1989; Roussel et al., 2020).
The feeding rhythms of H. discus hannai and H. gigantea are similar to those of Archachatina marginata and Achatina achatina, which are herbivorous terrestrial gastropods. In a previous study, Ademolu et al. (2011) investigated A. marginata and A. achatina in an artificial ecosystem and found that their feeding activities mostly occurred during 21:00–02:00 h at night and reached a peak at 00:00 h, whereas both species barely fed during 04:00–20:00 h, and thus both are typical nocturnal animals. Similarly, other herbivorous gastropods (e.g., Turbo chrysostomus and Cerithium tenellum) feed actively at night (Klumpp and Pulfrich, 1989; Klumpp et al., 1992), possibly because of evolution under predation stress (Hahn, 1989) and to avoid competition with herbivores that feed during the day. In comparison to the swimming ability of herbivorous fish, gastropods move at a lower velocity during food competition, so foraging at night would save energy, which could be distributed throughout the day to evade predators as well, and allow accumulation of more food at night (Carefoot and Taylor, 1989). The feeding behavior of abalone may involve a trade-off involving the best foraging time, attempting to avoid competition, and reducing the risk of predation (de Azevedo Carvalho et al., 2013). Paramisgurnus dabryanus is a typical nocturnal feeding species and has two clear feeding peaks during the day at 04:00–05:00 h and 20:00–21:00 h. This fish has underdeveloped vision but has five pairs of barbels that facilitate highly developed olfaction, which might explain its adaptation to feeding at night (Yuan et al., 2018). Similar to P. dabryanus, abalone also has underdeveloped vision, but little is known about any olfaction-mediated feeding mechanism in abalone.
The behaviors and physiological activities of organisms are closely associated with periodic variations in the surrounding environment. Most organisms exhibit endogenous rhythms in order to cope with future cycle events (Tran et al., 2011) and they can adapt their behavior and physiological cycles to the environmental cycle, so their feeding behaviors will occur when they are likely to succeed (Connor and Gracey, 2011; López-Olmeda and Sánchez-Vázquez, 2011; Houki and Kawamura, 2020). Feeding duration as a percentage of CDM was up to 94.6% in H. gigantea but only 56.0% in H. discus hannai. In addition, CDM was much shorter for H. gigantea than for H. discus hannai, indicating that H. gigantea made full use of its limited movement time when actively feeding. Under the experimental rearing conditions, H. gigantea did not have to spend time finding food because an adequate food supply was provided, which was supported by the experimental results (feeding duration as a percentage of CDM was up to 94.6%). In addition to its feeding activities, H. discus hannai made other intense movements, and crawling would have consumed energy. There is no definitive explanation for this movement behavior apart from feeding, and it is probably due to interspecific differences in “character” or the need to consume additional food by discontinuous movement during the feeding process. In their natural environment, abalone prefer to hide underneath rocks and other shelters. The foot muscle of H. gigantea can be completely covered by the shell, but that of H. discus hannai is excessively thick and the external part of the food muscles cannot be completely covered, resulting in a higher predation risk. Therefore, we consider that H. discus hannai has a greater need to find shelter in comparison to H. gigantea, which may explain why the former moved frequently in the experimental tank without any shelter in order to search for somewhere to hide. H. gigantea has a smaller and thinner foot muscle area, and its physiology involves a smaller condition factor than that of H. discus hannai at the same size, while its ability to store energy is relatively poor. Therefore, its behavior can be characterized as “remain still unless it is necessary to move, and eat food once movement commences,” which may help to reduce its energy consumption to the greatest extent possible.
The activity levels of digestive enzymes can reflect the digestive ability of an animal (Bansemer et al., 2016; Ma et al., 2020). Among the three digestive enzymes tested in the two species in this study, only α-amylase activity in H. discus hannai and alginate lyase activity in H. gigantea exhibited significant circadian rhythms. The activity peaks for pepsase and α-amylase in H. discus hannai coincided with its feeding peak at ZT12 (00:00 h), while the activity of alginate lyase had two peaks at ZT4 (16:00 h) and ZT24 (12:00 h). The highest pepsase activity in H. gigantea occurred at ZT20 (08:00 h), but the activities of both α-amylase and alginate lyase were maximum at ZT8 (20:00 h), that is, 4 h earlier than the feeding peak. Apostichopus japonicus (Sun et al., 2015), Eriocheir sinensis (Jia et al., 2018), and Colossoma macropomum (Reis et al., 2019) also have peak periods for digestive enzyme activity that coincide with the peak period for feeding. The secretion of digestive enzymes is generally rhythmic in animals with a daily feeding rhythm. However, food intake may be an even more important stimulus than the effects of changes in the light cycle (Rodríguez et al., 2021). The feeding rhythm of Dicentrarchus labrax exhibited seasonal changes whereby feeding activities occurred during the day in spring, summer, and autumn, but at night in winter (Sánchez-Vázquez et al., 1998). Irrespective of whether D. labrax consumed food at night or during the day, maximum α-amylase activity occurred during the feeding period. For individuals that exhibited feeding behavior at night, maximum α-amylase activity occurred at 18:00 h, whereas the peak enzyme activity in individuals with feeding behavior during the day occurred at 03:39 h, indicating that feeding time could regulate α-amylase secretion rhythm. Therefore, we consider that the secretion of digestive enzymes in abalone is consistent with the feeding period and follows an endogenous rhythm on the whole, but it is also closely associated with the specific feeding behavior of an individual animal. Thus, intake of food will increase the secretion of digestive enzymes in abalone (Britz et al., 1996), so the statistical results might not exhibit a cosine rhythm.
In a previous study, Montoya et al. (2010) allowed Sparus aurata access to periodic feeding at a fixed time each day, and found that α-amylase and alkaline protease activities increased a few hours before feeding. By contrast, the subjects in a random feeding group did not exhibit this pattern and their α-amylase activity did not increase until 1 h after feeding. These findings suggest that S. aurata can prepare for upcoming food activities. In another study, Vera et al. (2007) fed Carassius auratus fed at regular intervals and found that movements and α-amylase activity increased a few hours before feeding to coincide with the expected feeding time. In contrast to these experimental animals subjected to regular feeding, food was readily available for the abalone in our experiments, which may explain the non-rhythmic secretion of digestive enzymes, although the enzyme activity was still generally greater at night than during the day. Some of the digestive enzymes produced by animals may actually be secreted by bacteria in the intestines. The bacterium Enterobacter ludwigii, which can produce alginate lyase, was isolated from the intestines of H. rubra × H. laevigata (Amin et al., 2017). Thus, the digestive enzymes might not have been secreted just by the abalone in our experiments; further research is required to determine whether the enzymes secreted by intestinal flora exhibit rhythms, which may also be associated with the rhythmic secretion of digestive enzymes in the intestines of abalone.
NPF plays a crucial role in the regulation of invertebrate feeding (Nässel and Wegener, 2011). NPF directly regulates filter feeding by Ruditapes philippinarum and a study showed that injection of 5 μg g–1 rp-NPF into its hemocoel significantly increased the filtration rate by 23% within 2 h and by 65% after 8 h. Furthermore, rp-NPF mRNA expression in the ganglia splanchnicum of R. philippinarum increased after a short fasting period, with the highest level reached after 72 h of starvation before dropping immediately after 2 h of feeding (Wang et al., 2017). Similarly, after fasting for 24 h, LmiNPF1 expression in the brain of Locusta migratoria increased significantly before it was significantly downregulated after refeeding, which directly demonstrated that NPF can regulate feeding behavior (Tan et al., 2019). We found that the peak expression levels of NPF in H. discus hannai and H. gigantea occurred at ZT8 (20:00 h), which was 4 h earlier than the feeding behavior peak, possibly because of the role of NPF in facilitating the feeding behavior of abalone. The amino acid sequence of the neuropeptide group of NPF in H. discus hannai has been determined (Kim et al., 2019). Thus, in future studies, the role of NPF in facilitating the feeding behavior of H. discus hannai could be validated by directly injecting Hdh-NPF. The expression level of LmiNPF1 in the brain of L. migratoria was higher during the day than at night: it increased continuously during 07:00–17:00 h and the highest expression occurred at 17:00 before a continuous decrease to the lowest level during 17:00–07:00 on the following day, and the rate of the decrease was faster at night than during the day (08:30–00:30 h). Thus, it is considered that NPF–NPFR signaling may be crucial in ensuring lower activity of L. migratoria at night (Tan et al., 2019). In H. discus hannai and H. gigantea, the highest NPYR expression levels occurred at ZT4 (16:00 h) and in each species was earlier than the peak NPF expression level. Therefore, we suggest that the earlier occurrence of the peak NPYR expression level may help to prepare for an increase in NPF expression, thereby playing a role in regulating the periodic feeding behavior of abalone.
Several studies have shown that orexin can enhance the feeding and movement behaviors of fish (Rønnestad et al., 2017). For example, the feeding behaviors of Astyanax mexicanus (Penney and Volkoff, 2014) and Carassius auratus (Mandic and Volkoff, 2018) were stimulated after injection with orexin. In vertebrates, activation of OXR stimulates feeding and gastrointestinal movements, and OXR expression levels are also closely associated with feeding behavior (Wong, 2011; Volkoff, 2016; Baldascino et al., 2017). After 24 h of fasting, OX1R and OX2R mRNA levels increased in the hypothalamus of male Wistar rats (Rattus norvegicus) 2.5- and 2-fold, respectively (Karteris et al., 2005). After overnight fasting, OX1R and OX2R expression levels decreased in the duodenum of R. norvegicus (Flemström et al., 2010). It has been shown that the expression levels of orexin in other animals exhibit circadian rhythms. Analysis of prepro-orexin expression in the hypothalamus of Epinephelus coioides showed that it was higher in the light phase than the dark phase that and it increased significantly during feeding activity (Yan et al., 2011). Prepro-orexin mRNA expression increased significantly at 10:00 h, which corresponded to the daily feeding time of 10:00 h, before subsequently dropping rapidly to the pre-feeding level by 10:30 h. The expression level remained at a low level for the rest of the light phase, with the lowest level occurring during 23:00–05:00 h. At feeding time, prepro-orexin expression increased, but then dropped after feeding, suggesting that orexin might activate feeding behavior as a signal of starvation. In another study involving night feeding, the relative expression of orexin in the hypothalamus of Mus musculus exhibited an obvious diurnal distribution, with the lowest point in the mid-phase of the light period, before increasing in the dark period and peaking in the mid-phase of the dark period. Orexin expression decreased again in the later phase of the dark period (Fenzl et al., 2009). In the present study, OX2R expression in H. gigantea increased significantly before the feeding peak (ZT8, 20:00 h), which may be an indicator of orexin-mediated feeding behavior. The peak for OX2R expression levels in H. discus hannai occurred following the feeding peak (ZT16, 04:00 h). Thus, feeding behavior might be controlled by the interaction of a variety of neuropeptides, and orexin may not be the dominant factor in feeding regulation.
In this study, we compared the movements, feeding behaviors, and digestive physiology of H. discus hannai and H. gigantea, and showed that both species exhibit significant circadian rhythms, with a feeding peak at ZT12 (00:00 h). H. discus hannai tended to be bolder than H. gigantea, but H. gigantea spent 94.6% of the duration of its movement in feeding activities, whereas the duration of feeding by H. discus hannai only accounted for 56.0% of its CDM. The maximum α-amylase activity and NPF expression in both species and maximum OX2R expression in H. gigantea occurred between ZT8 (20:00 h) and ZT12 (00:00 h), and PTSF in this period also reached the maximum value. Feeding rhythms provide a biological basis for developing scientific feeding practices. Thus, an understanding of the characteristic feeding behaviors of abalone species may help to maximize economic benefits according to the feeding duration and frequency as well as other rearing strategies. The peak alginate lyase activity and peak NPYR expression level in H. discus hannai occurred at ZT4 (16:00 h), and PTSF for H. discus hannai was significantly higher than for H. gigantea, so we recommend feeding of H. discus hannai at 16:00 h, but H. gigantea should not be fed until 20:00 h. Staggered peak feeding can avoid food competition between species and may match the outcomes of adaptive selection during long-term evolution for the two species. An understanding of the physiological characteristics in terms of feeding and digestion in these species may contribute to the development of reasonable feeding strategies to improve the proliferation of abalone via bottom sowing, with further potential benefits from aiding in harvesting and protecting biodiversity.
The data presented in the study are deposited in the National Center for Biotechnology Information repository (https://www.ncbi.nlm.nih.gov/nucleotide/), accession numbers DQ845483.1, MZ389085, MW386999, MZ389087, MZ363638, MZ389086, AY319437.1, and D88574.1.
This study of animals was reviewed and approved by the Animal Welfare Committee of the College of Ocean and Earth Sciences, Xiamen University (permit no. COES-0001).
XG and CK conceptualized the study and had primary responsibility for the final content. ML, MZ, and SL conducted the research and collected the data. XL and WY provided materials and interpreted the data. ML and XG wrote the manuscript. All authors read and approved the final manuscript.
This research was supported by grants from the Chinese Ministry of Science and Technology through the National Key Research and Development Program of China (2018YFD0901400), the Fujian Ocean and Fisheries Research Grand (FJHJF-L-2020-7), Earmarked fund for the Modern Agro-industry Technology Research System (CARS-49), the China Postdoctoral Science Foundation Grant (2019M650153 and 2021T140393), and the Outstanding Postdoctoral Scholarship from the State Key Laboratory of Marine Environmental Science at Xiamen University.
The authors declare that the research was conducted in the absence of any commercial or financial relationships that could be construed as a potential conflict of interest.
All claims expressed in this article are solely those of the authors and do not necessarily represent those of their affiliated organizations, or those of the publisher, the editors and the reviewers. Any product that may be evaluated in this article, or claim that may be made by its manufacturer, is not guaranteed or endorsed by the publisher.
We would like to thank Chengyun Li and Jianxiong Li for their assistance with abalone collection and rearing.
The Supplementary Material for this article can be found online at: https://www.frontiersin.org/articles/10.3389/fmars.2021.751401/full#supplementary-material
Ademolu, K. O., Jayeola, O. A., Idowu, A. B., and Elemide, I. O. (2011). Circadian variation in locomotor and feeding periods of two land snail species. Arch. Zootec. 60, 1323–1326. doi: 10.4321/S0004-05922011000400048
Amin, M., Bolch, C. C. J., Adams, M. B., and Burke, C. M. (2017). Isolation of alginate lyase-producing bacteria and screening for their potential characteristics as abalone probionts. Aquacult. Res. 48, 5614–5623. doi: 10.1111/are.13383
Azzaydi, M., Madrid, J. A., Zamora, S., Sánchez-Vázquez, F. J., and Martıìnez, F. J. (1998). Effect of three feeding strategies (automatic, ad libitum demand-feeding and time-restricted demand-feeding) on feeding rhythms and growth in European sea bass (Dicentrarchus labrax L.). Aquaculture 163, 285–296. doi: 10.1016/S0044-8486(98)00238-5
Baldascino, E., Di Cristina, G., Tedesco, P., Hobbs, C., Shaw, T. J., Ponte, G., et al. (2017). The gastric ganglion of Octopus vulgaris: preliminary characterization of gene-and putative neurochemical-complexity, and the effect of Aggregata octopiana digestive tract infection on gene expression. Front. Physiol. 8:1001. doi: 10.3389/fphys.2017.01001
Bansemer, M. S., Qin, J. G., Currie, K.-L., and Stone, D. A. J. (2015). Temperature-dependent feed consumption patterns for greenlip (Haliotis laevigata) and hybrid (H. laevigata× Haliotis rubra) abalone fed fresh macroalgae or a formulated diet. J. Shellfish Res. 34, 885–892. doi: 10.2983/035.034.0318
Bansemer, M. S., Qin, J. G., Harris, J. O., Schaefer, E. N., Wang, H., Mercer, G. J., et al. (2016). Age-dependent response of digestive enzyme activities to dietary protein level and water temperature in greenlip abalone (Haliotis laevigata). Aquaculture 451, 451–456. doi: 10.1016/j.aquaculture.2015.10.013
Bolasina, S., Tagawa, M., Yamashita, Y., and Tanaka, M. (2006). Effect of stocking density on growth, digestive enzyme activity and cortisol level in larvae and juveniles of Japanese flounder, Paralichthys olivaceus. Aquaculture 259, 432–443. doi: 10.1016/j.aquaculture.2006.05.021
Britz, P. J., Hecht, T., and Knauer, J. (1996). Gastric evacuation time and digestive enzyme activity in abalone Haliotis midae fed a formulated diet. S. Afr. J. Mar. Sci. 17, 297–303. doi: 10.2989/025776196784158581
Cao, H. (2016). The cloning, functional analysis and identification of SNPs associated with growth traits of three digestive enzymes in Haliotis diversicolor. Ph.D. thesis. Xiamen: Xiamen University.
Carefoot, T. H., and Taylor, B. E. (1989). “Sea hares in coral rubbles: the significance of nocturnal grazing,” in Proceedings of the Sixth International Coral Reef Symposium, eds J. H. Choat (Townsville: International Coral Reef Symposium Executive Committee), 20–28.
China Bureau of Fisheries (2020). China Fishery Statistical Yearbook 2020. Beijing: China Agriculture Press.
Connor, K. M., and Gracey, A. Y. (2011). Circadian cycles are the dominant transcriptional rhythm in the intertidal mussel Mytilus californianus. Proc. Natl. Acad. Sci. U. S. A. 108, 16110–16115. doi: 10.1073/pnas.1111076108
de Azevedo Carvalho, D., Collins, P. A., and de Bonis, C. J. (2013). The diel feeding rhythm of the freshwater crab Trichodactylus borellianus (Decapoda: brachyura) in mesocosm and natural conditions. Mar. Freshwater Behav. Physiol. 46, 89–104. doi: 10.1080/10236244.2013.800758
de Bono, M., and Bargmann, C. I. (1998). Natural variation in a neuropeptide Y receptor homolog modifies social behavior and food response in C. elegans. Cell 94, 679–689. doi: 10.1016/s0092-8674(00)81609-8
del Pozo, A., Montoya, A., Vera, L. M., and Sánchez-Vázquez, F. J. (2012). Daily rhythms of clock gene expression, glycaemia and digestive physiology in diurnal/nocturnal European seabass. Physiol. Behav. 106, 446–450. doi: 10.1016/j.physbeh.2012.03.006
Fenzl, T., Flachskamm, C., Rossbauer, M., Deussing, J. M., and Kimura, M. (2009). Circadian rhythms of basal orexin levels in the hypothalamus are not influenced by an impaired corticotropin-releasing hormone receptor type 1 system. Behav. Brain Res. 203, 143–145. doi: 10.1016/j.bbr.2009.04.012
Flemström, G., Bengtsson, M. W., Mäkelä, K., and Herzig, K. H. (2010). Effects of short-term food deprivation on orexin-A-induced intestinal bicarbonate secretion in comparison with related secretagogues. Acta Physiol. 198, 373–380. doi: 10.1111/j.1748-1716.2009.02067.x
Gao, X., Luo, X., You, W., and Ke, C. (2020). Circadian movement behaviours and metabolism differences of the Pacific abalone Haliotis discus hannai. J. Photochem. Photobiol. B 211:111994. doi: 10.1016/j.jphotobiol.2020.111994
Guerra-Santos, B., López-Olmeda, J. F., de Mattos, B. O., Baião, A. B., Pereira, D. S. P., Sánchez-Vázquez, F. J., et al. (2017). Synchronization to light and mealtime of daily rhythms of locomotor activity, plasma glucose and digestive enzymes in the Nile tilapia (Oreochromis niloticus). Comp. Biochem. Physiol. A Mol. Integr. Physiol. 204, 40–47. doi: 10.1016/j.cbpa.2016.11.006
Hahn, K. O. (1989). “Biotic and abiotic factors affecting the culture of abalone,” in Handbook of Culture of Abalone and Other Marine Gastropods, ed. K. O. Hahn (Boca Raton: CRC Press), 113–134.
Hartmann, J. T., Beggel, S., Auerswald, K., and Geist, J. (2016). Determination of the most suitable adhesive for tagging freshwater mussels and its use in an experimental study of filtration behaviour and biological rhythm. J. Molluscan Stud. 82, 415–421. doi: 10.1093/mollus/eyw003
Hoogerwerf, W. A., Hellmich, H. L., Cornélissen, G., Halberg, F., Shahinian, V. B., Bostwick, J., et al. (2007). Clock gene expression in the murine gastrointestinal tract: endogenous rhythmicity and effects of a feeding regimen. Gastroenterology 133, 1250–1260. doi: 10.1053/j.gastro.2007.07.009
Houki, S., and Kawamura, T. (2020). Feeding and digestion periodicity of Manila clam Ruditapes philippinarum in natural intertidal and subtidal zones estimated from the morphological condition of the crystalline style. J. Molluscan Stud. 86, 361–371. doi: 10.1093/mollus/eyaa027
Houki, S., Kawamura, T., Irie, T., Won, N.-I., and Watanabe, Y. (2015). The daily cycle of siphon extension behavior in the Manila clam controlled by endogenous rhythm. Fish. Sci. 81, 453–461. doi: 10.1007/s12562-015-0859-6
Jia, E., Yan, M., Lai, Q., Luo, W., Jiang, G., Liu, W., et al. (2018). Feeding rhythm of the Chinese mitten crab (Eriocheir sinensis). J. Fish. Sci. China 25, 546–554.
Karteris, E., Machado, R. J., Chen, J., Zervou, S., Hillhouse, E. W., and Randeva, H. S. (2005). Food deprivation differentially modulates orexin receptor expression and signaling in rat hypothalamus and adrenal cortex. Am. J. Physiol. Endocrinol. Metab. 288, E1089–E1100. doi: 10.1152/ajpendo.00351.2004
Kim, M. A., Markkandan, K., Han, N.-Y., Park, J.-M., Lee, J. S., Lee, H., et al. (2019). Neural ganglia transcriptome and peptidome associated with sexual maturation in female Pacific abalone (Haliotis discus hannai). Genes 10:268. doi: 10.3390/genes10040268
Klumpp, D. W., and Pulfrich, A. (1989). Trophic significance of herbivorous macroinvertebrates on the central great barrier reef. Coral Reefs 8, 135–144. doi: 10.1007/BF00338269
Klumpp, D. W., Salita-Espinosa, J. S., and Fortes, M. D. (1992). The role of epiphytic periphyton and macroinvertebrate grazers in the trophic flux of a tropical seagrass community. Aquat. Biol. 43, 327–349. doi: 10.1016/0304-3770(92)90046-L
Kukkonen, J. P. (2013). Physiology of the orexinergic/hypocretinergic system: a revisit in 2012. Am. J. Physiol. Cell Physiol. 304, C2–C32. doi: 10.1152/ajpcell.00227.2012
Li, X., Qu, M.-J., Zhang, Y., Li, J.-W., and Liu, T.-X. (2018). Expression of neuropeptide F gene and its regulation of feeding behavior in the pea aphid, Acyrthosiphon pisum. Front. Physiol. 9:87. doi: 10.3389/fphys.2018.00087
López-Olmeda, J. F., and Sánchez-Vázquez, F. J. (2011). Thermal biology of zebrafish (Danio rerio). J. Therm. Biol. 36, 91–104. doi: 10.1016/j.jtherbio.2010.12.005
Lopez-Uriostegui, F., Ponce-Palafox, J. T., Lango-Reynoso, F., Castañeda-Chavez, M. D. R., Arenas-Fuentes, V. E., and Vega-Villasante, F. (2017). Stomach repletion rhythms of the caridean shrimps, Macrobrachium americanum and M. tenellum (Crustacea: decapoda) in a caged-pond system. Pakistan J. Zool. 49, 973–977. doi: 10.17582/journal.pjz/2017.49.3.973.977
Luo, X., Ke, C., You, W., Yang, J., and Wu, J. (2006). Preliminary studies on hybridization between the abalones Haliotis sieboldii and H.discus discus. J. Xiamen Univ. 45, 602–605.
Ma, S., Guo, Y., Sun, L., Fan, W., Liu, Y., Liu, D., et al. (2020). Over high or low dietary protein levels depressed the growth, TOR signaling, apoptosis, immune and anti-stress of abalone Haliotis discus hannai. Fish Shellfish Immunol. 106, 241–251. doi: 10.1016/j.fsi.2020.08.004
Mandic, S., and Volkoff, H. (2018). The effects of fasting and appetite regulators on catecholamine and serotonin synthesis pathways in goldfish (Carassius auratus). Comp. Biochem. Physiol. A Mol. Integr. Physiol. 223, 1–9. doi: 10.1016/j.cbpa.2018.04.017
Montoya, A., López-Olmeda, J. F., Yúfera, M., Sánchez-Muros, M. J., and Sánchez-Vázquez, F. J. (2010). Feeding time synchronises daily rhythms of behaviour and digestive physiology in gilthead seabream (Sparus aurata). Aquaculture 306, 315–321. doi: 10.1016/j.aquaculture.2010.06.023
Nässel, D. R., and Wegener, C. (2011). A comparative review of short and long neuropeptide F signaling in invertebrates: any similarities to vertebrate neuropeptide Y signaling? Peptides 32, 1335–1355. doi: 10.1016/j.peptides.2011.03.013
Penney, C. C., and Volkoff, H. (2014). Peripheral injections of cholecystokinin, apelin, ghrelin and orexin in cavefish (Astyanax fasciatus mexicanus): effects on feeding and on the brain expression levels of tyrosine hydroxylase, mechanistic target of rapamycin and appetite-related hormones. Gen. Comp. Endocrinol. 196, 34–40. doi: 10.1016/j.ygcen.2013.11.015
Reis, Y. S., Leite, J. L. R., de Almeida, C. A. L., Pereira, D. S. P., Vidal, L. V. O., de Araujo, F. G., et al. (2019). New insights into tambaqui (Colossoma macropomum) feeding behavior and digestive physiology by the self-feeding approach: effects on growth, dial patterns of food digestibility, amylase activity and gastrointestinal transit time. Aquaculture 498, 116–122. doi: 10.1016/j.aquaculture.2018.08.054
Robson, A. A., de Leaniz, C. G., Wilson, R. P., and Halsey, L. G. (2010). Effect of anthropogenic feeding regimes on activity rhythms of laboratory mussels exposed to natural light. Hydrobiologia 655, 197–204. doi: 10.1007/s10750-010-0449-7
Rodríguez, I., Betancor, M. B., López-Jiménez, J. Á, Esteban, M. Á, Sánchez-Vázquez, F. J., and López-Olmeda, J. F. (2021). Daily rhythms in the morphometric parameters of hepatocytes and intestine of the European sea bass (Dicentrarchus labrax): influence of feeding time and hepatic zonation. J. Comp. Physiol. B 191, 503–515. doi: 10.1007/s00360-020-01334-w
Rønnestad, I., Gomes, A. S., Murashita, K., Angotzi, R., Jönsson, E., and Volkoff, H. (2017). Appetite-controlling endocrine systems in teleosts. Front. Endocrinol. 8:73. doi: 10.3389/fendo.2017.00073
Roussel, S., Poitevin, P., Day, R., Le Grand, F., Stiger-Pouvreau, V., Leblanc, C., et al. (2020). Haliotis tuberculata, a generalist marine herbivore that prefers a mixed diet, but with consistent individual foraging activity. Ethology 126, 716–726. doi: 10.1111/eth.13020
Sánchez-Vázquez, F. J., Azzaydi, M., Martínez, F. J., Zamora, S., and Madrid, J. A. (1998). Annual rhythms of demand-feeding activity in sea bass: evidence of a seasonal phase inversion of the diel feeding pattern. Chronobiol. Int. 15, 607–622. doi: 10.3109/07420529808993197
Shimizu, S., Nakamachi, T., Konno, N., and Matsuda, K. (2014). Orexin A enhances food intake in bullfrog larvae. Peptides 59, 79–82. doi: 10.1016/j.peptides.2014.07.013
Sun, J., Zhang, L., Pan, Y., Lin, C., Wang, F., Kan, R., et al. (2015). Feeding behavior and digestive physiology in sea cucumber Apostichopus japonicus. Physiol. Behav. 139, 336–343. doi: 10.1016/j.physbeh.2014.11.051
Tahil, A. S., and Juinio-Menez, M. A. (1999). Natural diet, feeding periodicity and functional response to food density of the abalone, Haliotis asinina L., (Gastropoda). Aquacult. Res. 30, 95–107. doi: 10.1046/j.1365-2109.1999.00294.x
Tan, S., Li, A., Wang, Y., and Shi, W. (2019). Role of the neuropeptide F 1 in regulating the appetite for food in Locusta migratoria. Pest Manage. Sci. 75, 1304–1309. doi: 10.1002/ps.5244
Tran, D., Nadau, A., Durrieu, G., Ciret, P., Parisot, J.-P., and Massabuau, J.-C. (2011). Field chronobiology of a molluscan bivalve: how the moon and sun cycles interact to drive oyster activity rhythms. Chronobiol. Int. 28, 307–317. doi: 10.3109/07420528.2011.565897
Vera, L. M., De Pedro, N., Gómez-Milán, E., Delgado, M. J., Sánchez-Muros, M. J., Madrid, J. A., et al. (2007). Feeding entrainment of locomotor activity rhythms, digestive enzymes and neuroendocrine factors in goldfish. Physiol. Behav. 90, 518–524. doi: 10.1016/j.physbeh.2006.10.017
Volkoff, H. (2016). The neuroendocrine regulation of food intake in fish: a review of current knowledge. Front. Neurosci. 10:540. doi: 10.3389/fnins.2016.00540
Wang, X., Miao, J., Liu, P., and Pan, L. (2017). Role of neuropeptide F in regulating filter feeding of Manila clam, Ruditapes philippinarum. Comp. Biochem. Physiol. B Biochem. Mol. Biol. 205, 30–38. doi: 10.1016/j.cbpb.2016.12.001
Wells, F. E., and Keesing, J. K. (1989). Reproduction and feeding in the abalone Haliotis Roei Gray. Aust. J. Mar. Freshwater Res. 40, 187–197. doi: 10.1071/MF9890187
Wu, Q., Wen, T., Lee, G., Park, J. H., Cai, H. N., and Shen, P. (2003). Developmental control of foraging and social behavior by the Drosophila neuropeptide Y-like system. Neuron 39, 147–161. doi: 10.1016/s0896-6273(03)00396-9
Wu, Q., Zhang, Y., Xu, J., and Shen, P. (2005). Regulation of hunger-driven behaviors by neural ribosomal S6 kinase in Drosophila. Proc. Natl. Acad. Sci. U. S. A. 102, 13289–13294. doi: 10.1073/pnas.0501914102
Yan, A., Zhang, L., Tang, Z., Zhang, Y., Qin, C., Li, B., et al. (2011). Orange-spotted grouper (Epinephelus coioides) orexin: molecular cloning, tissue expression, ontogeny, daily rhythm and regulation of NPY gene expression. Peptides 32, 1363–1370. doi: 10.1016/j.peptides.2011.05.004
Yuan, Q., Lü, W., Tang, W., Yue, M., and Zhou, W. (2018). Study on feeding rhythm and daily feed intake of Paramisgurnus dabryanus. S. China Fish. Sci. 14, 115–120.
Zhuang, S. (2005). Influence of salinity, diurnal rhythm and daylength on feeding in Laternula marilina Reeve. Aquacult. Res. 36, 130–136. doi: 10.1111/j.1365-2109.2004.01195.x
Keywords: abalone, digestive enzyme, feeding behavior, neuropeptide F, orexin receptor
Citation: Lyu M, Gao X, Zhang M, Lin S, Luo X, You W and Ke C (2021) Comparative Study of the Feeding Characteristics and Digestion Physiology of Haliotis discus hannai and Haliotis gigantea. Front. Mar. Sci. 8:751401. doi: 10.3389/fmars.2021.751401
Received: 01 August 2021; Accepted: 13 September 2021;
Published: 01 October 2021.
Edited by:
Youji Wang, Shanghai Ocean University, ChinaCopyright © 2021 Lyu, Gao, Zhang, Lin, Luo, You and Ke. This is an open-access article distributed under the terms of the Creative Commons Attribution License (CC BY). The use, distribution or reproduction in other forums is permitted, provided the original author(s) and the copyright owner(s) are credited and that the original publication in this journal is cited, in accordance with accepted academic practice. No use, distribution or reproduction is permitted which does not comply with these terms.
*Correspondence: Xiaolong Gao, eGxnYW9AeG11LmVkdS5jbg==; Caihuan Ke, Y2hrZUB4bXUuZWR1LmNu
Disclaimer: All claims expressed in this article are solely those of the authors and do not necessarily represent those of their affiliated organizations, or those of the publisher, the editors and the reviewers. Any product that may be evaluated in this article or claim that may be made by its manufacturer is not guaranteed or endorsed by the publisher.
Research integrity at Frontiers
Learn more about the work of our research integrity team to safeguard the quality of each article we publish.