- Department of Ecology, Faculty of Sciences, University of Málaga, Málaga, Spain
Continuous winter darkness at a latitude of 79°N was simulated in cultures of four species of Arctic seaweeds at 3 and 8°C. The laminarians Saccharina latissima and Alaria esculenta, and the rhodophytes Phycodrys rubens and Ptilota gunneri were monitored for 4 months in total darkness and after 1 week following light return in early spring, under controlled laboratory conditions. Biomass loss during darkness was enhanced by the high temperature in all species. At 8°C, the two laminarians were unable to resume growth upon re-illumination. Alaria esculenta showed new blade production by the end of the dark period, but only at 3°C. In all species, the photosynthetic ability was sustained, not suspended, during the whole dark period. P. rubens exhibited lower photosynthetic potential at 8°C than at 3°C during the darkness period, but it was able to recover its O2 evolving potential upon re-illumination, as P. gunneri and S. latissima did, but the latter only at 3°C. The reactivation of photosynthesis seemed to involve photosystem II over photosystem I, as 7 d of photoperiod after the prolonged darkness was not enough to fully recover the PAM-related photosynthetic parameters. Only small changes were recorded in the internal chemical composition (total C, total N, carbohydrates, proteins, and lipids), but species-specific differences were observed. Unlike subarctic areas with an operating photoperiod along the year, a warmer polar night might pose a limit to the ability of multi-year seaweeds to occupy the new ice-free illuminated areas of the Arctic coasts, so that newcomers will potentially be restricted to the spring-summer season.
Introduction
The Arctic is one of the regions of the planet where the undergoing rise in the mean annual temperature is more severe. In Kongsfjorden (western Svalbard archipelago, 79°N), winter is the season of the year most affected, suffering an increase of 3.0°C per decade in the air (Maturilli et al., 2019) and 1.6°C in the seawater (Hop et al., 2019), precluding sea-ice formation during the last 15 years. One of the major phenomena responsible for this warming is the increase in the amount of Atlantic water that has advected into Kongsfjorden by the West Spitsbergen current since 2005/2006, particularly during winter, both in surface and in deeper areas of the fjord (Cottier et al., 2007; Pavlov et al., 2013). These changes strongly influence the sea-ice dynamics, the hydrodynamics, and the biota. Kelp-dominated macroalgal communities are ecologically important primary producers and ecosystem engineers, structuring littoral habitats. They are a major component of nutrient cycling and play a key role in carbon capture leading to sequestration (Krausse-Jensen and Duarte, 2016). Macroalgae in the Arctic provide extensive substrata for colonizing organisms, and a three-dimensional habitat structure for a wide array of marine animals, including several commercially relevant species. The abundance and diversity of macroalga-associated fauna are highest in winter (Berge et al., 2015; Shuntanova et al., 2018), when many of these organisms reduce their reliance on spring-summer pelagic primary production by incorporating macroalgal biomass in their diet (Kortsch et al., 2012; Berge et al., 2015).
The kelps Saccharina latissima and Alaria esculenta are the dominant species of the upper sublittoral in Kongsfjorden between 4.5 and 14.5 m depth (Hop et al., 2012). In general, new kelp tissue in S. latissima is initiated in winter in cold-temperate regions, but it is restricted to spring in Kongsfjorden (Nielsen et al., 2014). The rhodophytes Ptilota gunneri and Phycodrys rubens are dominant in Kongsfjorden at 14.5–16.5 and 16.5–30 m, respectively, and subdominant from 8.5 to 14.5 m in the understory below Laminarians and Desmarestiales (Hop et al., 2012). Both red species also perform multiyear life spans of their macroscopic form. P. rubens blade length increases mainly in spring (Schoschina, 1996). Undoubtedly, winter is the season from which less information is available in polar habitats in general, and particularly in coastal marine organisms, despite being the one most impacted by global warming, as mentioned above.
Physiological studies of algae in Kongsfjorden have consistently reported low light compensation points for O2 production (LCP) of about 2–7 μmol m–2 s–1. Photosynthesis in Arctic kelps also shows low light requirements (Ik) for saturation of about 20–40 μmol m–2 s–1 (Latala, 1990; Krüger, 2016). These features constitute adaptations to the frequent low light environment and are considered a general characteristic of polar phototrophs (Gómez et al., 2009). However, these values change seasonally due to the light climate. Bischof et al. (2002) found that the maximum photosynthetic electron transport rates (ETRmax) in S. latissima and Saccorhiza dermatodea were reduced by one third at the end of the dark period (ice-covered early June) compared to values measured in mid-July with a clear water column. Moreover, significant drops in the optimum quantum yield (Fv/Fm) following ice breakup were recorded in the latter, but not in the former. Scheschonk et al. (2019) recently found that Fv/Fm and ETRmax values were higher in October than in mid-February in S. latissima.
As any enzyme-dependent reaction, the respiration rate is presumably stimulated by increasing temperatures, since the kinetics of enzymatic reactions speed up with the growth temperature in Laminariales (Davison, 1987). However, this was not the case for S. latissima from Kongsfjorden, which showed a reduction in the respiration rate of more than 50% at 9°C compared to 4°C in summer (Iñiguez et al., 2016). Higher respiration rates at low temperatures have been regarded as an adaptation to cope with cold in algae that are close to their lower temperature distribution limit (Iñiguez et al., 2016). The regulation of the respiration rate in macroalgae can account for changes in growth rates when photosynthesis is not affected (Gordillo et al., 2001; Iñiguez et al., 2015). The respiration rate during winter is of prime relevance since it imposes the rate at which C storage is being used, and then, potentially exhausted, so that both the respiration rates and the level of internal reserves will determine overwinter survival during the polar night.
At high nutrient and low light levels during late autumn and early winter, most Arctic kelps are presumed to build up N reserves, that are utilized in the following spring to initiate growth, once light is again available (Chapman and Craige, 1977). Photosynthesis drives growth during spring and early summer, but growth ceases in mid-late summer, so that photosynthetic C products are stored and used later during winter respiration (Scheschonk et al., 2019), ensuring survival. In kelps, mannitol is the photosynthetic product being stored in form of laminarin. Saccharina latissima is reported to decrease its laminarin content by as much as 96% over the winter period (mid-October to mid-February) in Kongsfjorden populations (Scheschonk et al., 2019). There is no such detail on the seasonal C and N utilization pattern in Arctic red algae, and the few reports available are restricted to the summer season (e.g., Gordillo et al., 2016).
Seasonal fluctuations of light climate and ambient nutrient levels are major drivers of the growth pattern of macroalgae, but less attention has been paid to water temperature, despite being the factor defining the biogeographical distribution of the species (Nielsen et al., 2014). The effects of an increase in water temperature in the physiology of A. esculenta, P. rubens, and Ptilota plumosa have been reported by Gordillo et al. (2016), while the temperature effects on S. latissima have been reported by Iñiguez et al. (2016), both in mid-summer. An increase of 5–6°C in the temperature stimulated growth in S. latissima and P. rubens, but it did not affect the growth rates of A. esculenta and P. plumosa, which already exhibited lower growth rates at the control temperature (4°C) than the former two species at that time of the year (July, in all cases). The summer increase in the growth rate due to warming in S. latissima and P. rubens led to reduced N reserves, so that an increase in the C:N ratio was observed in both species (Gordillo et al., 2016; Iñiguez et al., 2016). Unlike warming in summer, warming in winter will not affect an already ceased growth, but it may accelerate the rate of consumption of C reserves and could enhance thallus decay and erosion.
The relevance of temperature and light in the regulation of seasonal growth patterns and phenology suggests that warming may cause a disruption of both growth dynamics and survival, predictably affecting the large-scale ecosystem structure. Bartsch et al. (2016) recorded a complex pattern of change in the macroalgal community between 1996/1998 and 2012/2014, most likely due to co-acting environmental factors, such as lack of ice-scouring and the extension of the open water (ice-free) period, along with the increase in the mean water temperature. Overall algal biomass increased, including the four species studied here, and their peaks shifted to shallower depths. Changes also resulted in an altered seaweed community. Data on the impact of a warm winter scenario on macroalgal physiology during the polar night are scarce, but the consequences on the physiology of phototrophs are presumed to be severe. This lack of knowledge on the survival mechanisms and the resilience of habitat-forming macroalgal species weakens our ability to make predictions on the overall fate of Arctic littoral ecosystems.
The main hypothesis of the present study is that long periods of darkness under increased temperatures might compromise the thallus integrity, and the capacity to resume the photosynthetic activity in the following spring, compromising the interannual survival of overwintering Arctic seaweeds. According to this hypothesis, the relevant questions to be answered are: (1) Do biomass, respiration, and/or photosynthetic performance change at increased temperatures enough to compromise overwintering viability? (2) To what extent are interspecific differences in performance and composition observed? To tackle these relevant questions, we selected two kelp species (A. esculenta and S. latissima) and two red species (P. rubens and P. gunneri) representative of the Kongsfjord coastal marine ecosystem and cultured them in simulated winter conditions in the laboratory under complete darkness for about 4 months. We further studied the recovery of the photosynthetic performance upon illumination after the dark period.
Materials and Methods
Algal material was collected by scuba diving from Hansneset in Kongsfjorden (78°59′N, 11°59′E, Svalbard) at the end of September 2016. The Ochrophyceae Saccharina latissima (L.) Lane, Mayes, Druehl and Saunders, and Alaria esculenta (L.) Greville were collected from 5 to 8 m depths, while Phycodrys rubens (L.) Batters and Ptilota gunneri Silva, Maggs, and Irvine were collected from 9 to 10 m depths from the same spot. Young and visually healthy thalli between 20 and 50 cm in length were selected and transported to Malaga (Spain) in an electric-aided cool box with ice packs. For transportation, the thalli were placed stacked in paper towels soaked in seawater, and the cool box was powered at intervals. A thermometer with a wired temperature sensor inserted in the box showed that the temperature was always between 2 and 5°C. The total time in transit was one and a half days.
Plant Cultivation
Around 30 individual thalli of each species were placed in a 20 L acrylic tank (one tank per species). The incubation medium was prepared using the Instant Ocean salt mixture in distilled water (Instant Ocean, United States) enriched with Provasoli (1968), and bubbled with air pumps (four air spargers per tank) ensuring water motion. The medium was replaced every 2 weeks, while Provasoli was added weekly. A water tank for each species was placed in each temperature-controlled chamber. The low temperature (LT) chamber was set at 3.1 ± 0.4°C (3°C). The high temperature (HT) chamber was set at 8.0 ± 0.3°C (8°C). Typically, the water temperature in Kongsfjorden in the October–February period oscillates between ca. 0 and + 5°C according to the AWIPEV–COSYNA database1, so, 3°C was chosen as a representative average. Since Hop et al. (2019) proposed an average increase of 1.6°C per decade, the treatment at 8°C would represent a water temperature for the winter period expected in about three to four decades, i.e., before the end of this century.
During the acclimation phase (from September 25 to October 17), algae were cultured in their experimental tanks at the target temperature. Twenty μmol photons m–2 s–1 of white light were provided by neutral white LEDs, and the photoperiod was adjusted daily to fit the natural daylength in Svalbard, until complete darkness (mid-October), when the darkness treatment started. Initial measurements (T0) were taken in the final three to 5 days of the photoperiod. Normally, all measurements were taken every 4 weeks. After a period of nearly 4 months in darkness, light was switched on for 1 h on the first day (20 μmol photons m–2 s–1). For the next 7 days, the photoperiod was adjusted daily to resemble natural conditions in Kongfjorden to reach 5:19 h (L:D), and the irradiance was kept constant. At that point, a recovery P-I curve was taken by measuring the O2 evolution. Recovery measures were not taken for A. esculenta, since there were not enough flat thalli left at the end of the dark period. Only the midrib and a small area of new flat thalli (only at 3°C) remained at that point.
For the following measurements, three pieces from independent thalli of each species were taken from each of the tanks placed in the two different temperatures assayed, unless otherwise stated. Whenever possible, the same individuals were used for the different analyses for a given sampling time. Pieces from S. latissima and A. esculenta were taken from the meristematic zones using cork-borers 0.9 cm in diameter. Pieces from P. rubens consisted of the tip (2–3 cm) or total part (if small) of the leaves-like flat parts; and pieces from P. gunneri were taken from the tips (2–3 cm) of the branches collected using fine stainless steel tweezers and scissors. The weight of the pieces ranged from 20 to 60 mg for the kelps and from 80 to 150 for the reds, depending on thallus morphology.
Biomass
The loss of biomass was measured by weighing whole small thalli (about 20–30 cm length). Five individual thalli from each treatment were labeled with nylon thread and their fresh weight (FW) taken, after blotting them on paper towels, every 4 weeks.
Photosynthesis and Respiration Measurements
Oxygen evolution was measured in 7 mL custom-made acrylic chambers enclosed in a water recirculation, temperature-controlled system. The oxygen sensor was a Clark-type (model 5331, YSI, United States). The incubation time in light was typically 10 min, although it was extended to 30–45 min when the O2 evolution rates were low. In order to properly address the respiratory O2 consumption rates, total darkness was needed. All electronic LEDs, such as those in the stirring plates, were covered with black tape and the incubation chambers were covered with a black cloth. Dark incubation times elapsed 30 min to 1 h. After the O2 incubations, the absorptance and the chlorophyll a content of the thalli were measured.
Complete P-I curves (including respiration) for each species were performed in the O2 evolution chambers at the end of the 16 weeks dark incubation period, and after 1 week of progressively increasing the photoperiod. Irradiance was provided by white LED lamps and set to the desired level (0–300 μmol photons m–2s–1) by using neutral filters. Irradiance in the chambers was measured with a submersible spherical sensor (US-SQS/L, Walz, Germany) attached to a Li-250A light meter (Li-Cor, United States). Curves were fitted according to Jassby and Platt (1976), and the corresponding parameters were calculated: The light compensation point (LCP); the maximum net photosynthetic rate (Pmax); the photosynthetic efficiency (initial slope, α); and the saturation irradiance (Ik).
Chlorophyll fluorescence for photosynthetic performance was measured with a Mini-PAM (Walz, Germany) by generating rapid light curves, using actinic light intensities between 22 and 600 μmol photons m–2 s–1, measured with a Li-190R flat sensor attached to a Li-250A light meter (Li-Cor, United States). The optimum and effective quantum yields for the charge separation of photosystem II (PS II) reaction centers were measured for samples in the dark and under actinic illumination (Fv/Fm ΔF/Fm′, respectively). The electron transport rate (ETR) between PS II and PS I was calculated in short (30 s) incubations for each actinic irradiance as:
where ΔF/Fm′ is the effective quantum yield, 0.5 stands for the assumption of the even contribution of photons to PS II and PS I, PAR is the actinic irradiance (in μmol photons m–2 s–1), and A is the thallus absorptance.
The thallus absorptance (A) was estimated by placing the thalli between a white LED lamp and the flat light sensor, so that:
where I0 is the light irradiance reaching the sensor, and It is the irradiance transmitted through the thallus when this is placed covering the sensor, intercepting the light.
From rapid light curves (ETR vs. PAR) the following photosynthetic parameters were calculated according to Jassby and Platt (1976): the optimum quantum yield (Fv/Fm); the maximum electron transport rate (ETRmax); the quantum efficiency for linear electron transport (initial slope, αETR); and the subsaturating irradiance (Ek).
Biochemical Composition
The content of chlorophyll a was estimated from the same pieces of algae used for PAM and absorptance measurements. Chlorophyll was extracted overnight using dimethilformamide and quantified colorimetrically according to Wellburn (1994).
Total proteins, total lipids, and total carbohydrates were determined from freeze-dried material taken every 4 weeks and stored at −80°C. Total proteins were extracted in phosphate buffer (0.1 M, pH 6.5) with trichloroacetic acid 5% and incubated for 1 h. The samples were then subsequently washed with 100% acetone, and SDS (4%) was used to resuspend the protein extract. Proteins were estimated spectrophotometrically using bicinchoninic acid-copper sulfate for color development and bovine serum albumin as standard, and determined spectrophotometrically at 562 nm (Smith et al., 1985).
Total lipids were extracted using a chloroform:methanol (2:1) solution and incubated overnight in darkness (4°C). The solution was then evaporated with N2 before acid digestion using sulfuric acid. Lipids were estimated spectrophotometrically using phosphovanillin for color development and cholesterol as standard. Finally, the concentration of lipids was determined also spectrophotometrically at 520 nm (Barnes and Blackstock, 1973).
Total carbohydrates were extracted in hydrochloric acid (5%) and heated to 80°C for 2 h. Carbohydrates were estimated using phenol-sulfuric acid for color development and D-glucose as standard, and spectrophotometrically determined at 485 nm (Dubois et al., 1956).
The total C and N content was measured from freeze-dried material using a C:H:N elemental analyzer (Perkin–Elmer 2400CHN).
Statistical Analysis
The data are shown as the mean and standard deviation of three measurements of independent replicates (unless indicated otherwise, e.g., biomass, n = 5). The differences between 3 and 8°C and the effect of the time in darkness were tested using a two-factor ANOVA for each measured variable. For biomass, a Split-plot type of ANOVA was performed, since the same thalli were monitored throughout the experimental period. All the data used in the analyses were assayed for normality (Kolmogorov–Smirnov test) and subjected to Levene’s test to verify the homogeneity of variance. All analyses were performed using the software Graphpad Prism (v.9).
Results
The time spent in continuous darkness affected most of the variables studied in the four species, although species-specific responses were common. Warming produced significant effects in over 40% of the variables analyzed and influenced the pattern of changes observed during darkness (see Supplementary Table 1 for a summary of P-values of the ANOVA tests performed).
Biomass
Three of the four species lost biomass gradually during the dark incubation period at both temperatures. The other species, P. gunneri, lost biomass at 8°C but maintained it when cultured at 3°C (Figure 1). Weight losses of around 50% of the initial value were observed in A. esculenta, S. latissima, and P. rubens at 8°C, while they were only around 30, 40, and 30%, respectively, at 3°C. Thus, the increased temperature accelerated the weight loss in all species. By the end of the incubation period, the weight difference between the two temperatures was 21% in A. esculenta, 16% in S. latissima, 21% in P. rubens, and 14% in P. gunneri.
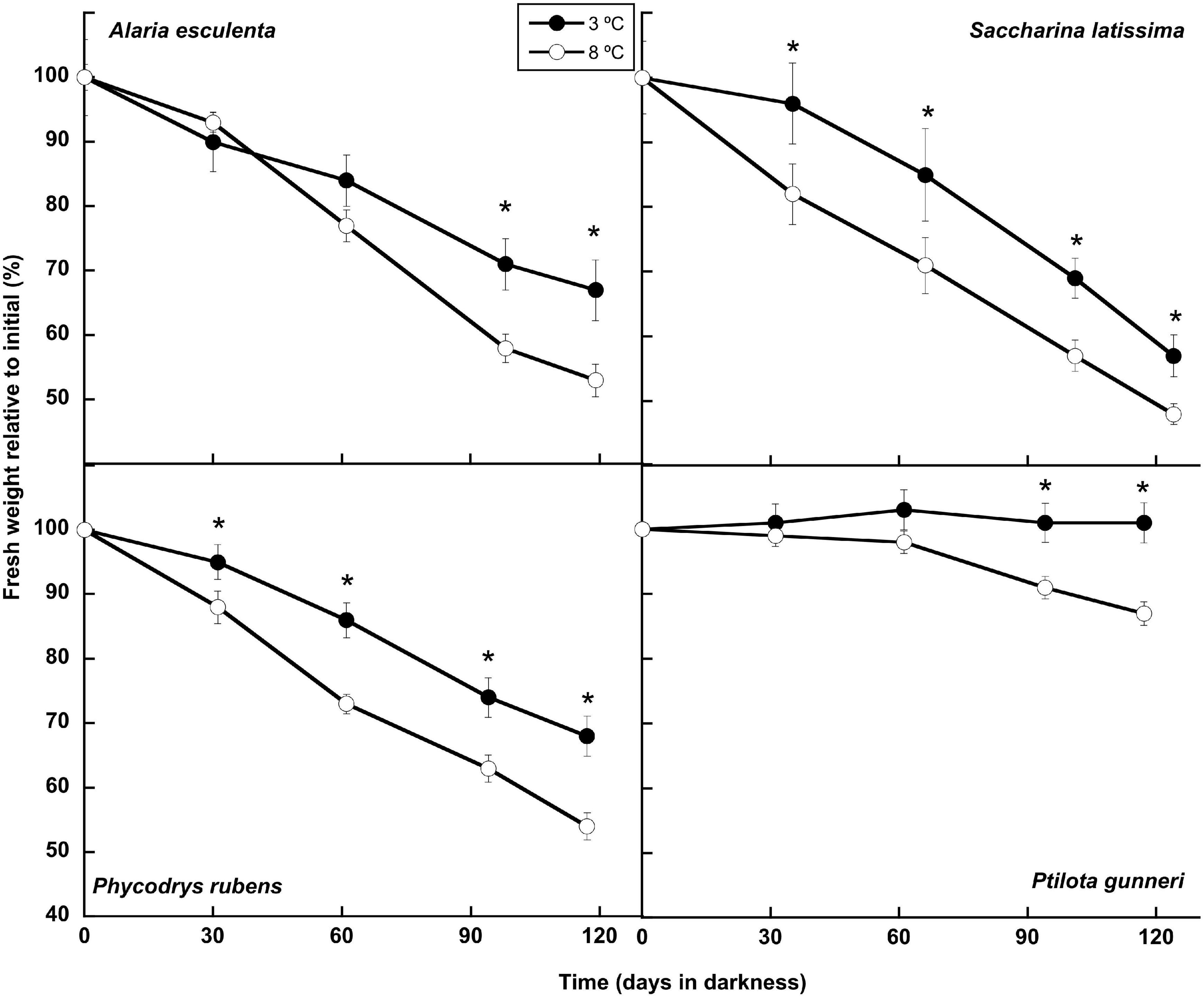
Figure 1. Biomass evolution during the 16 weeks of incubation in darkness of Alaria esculenta, Saccharina latissima, Phycodrys rubens, and Ptilota gunneri at two different temperatures. Data are mean of 5 replicates (error bars for standard deviation). Significant differences between temperatures marked with an asterisk.
Thallus Morphology
Visually, both Alaria esculenta and Saccharina latissima lost most of their tissue, with degradation being more obvious at 8°C than at 3°C (Figure 2). Remarkably, A. esculenta produced new blade tissue at the end of the dark period at 3°C, but not at 8°C. This new tissue continued growing for at least 2 months after the return of light. However, no new tissue was observed for this species at 8°C after the return of light. There was no blade growth in S. latissima in any of the temperatures assayed. Nonetheless, more production of new holdfast could be observed at 3°C compared to 8°C (Figures 2G,H, respectively). No visual differences were observed in red algae cultured at 3°C compared to 8°C. Marginally, some higher blade density was observed in Phycodrys rubens at 3°C (Figures 2I–L).
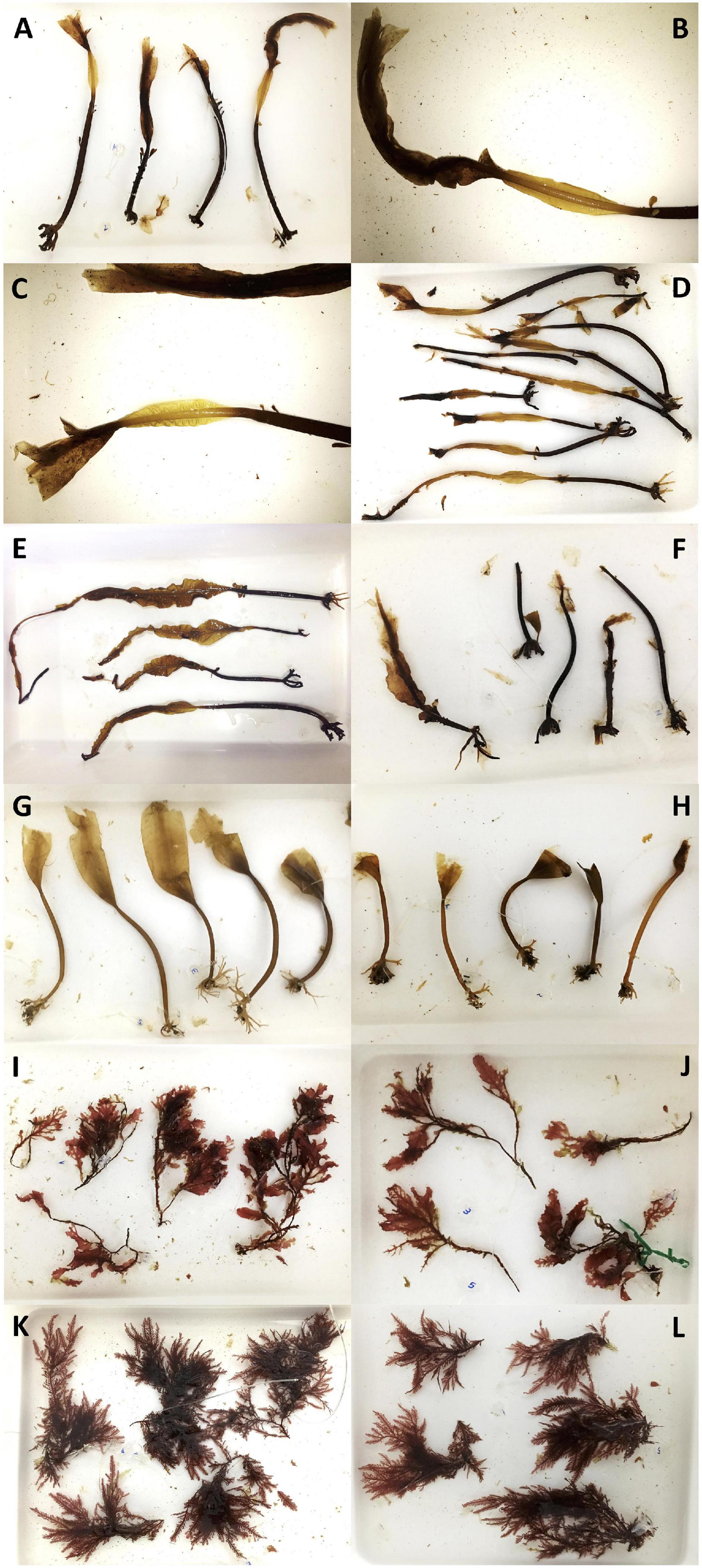
Figure 2. Pictures of thalli after 4 months in darkness at either 3 or 8°C. Alaria esculenta at 3°C the last day of darkness (A), [(B,C), detail] and after 1 week (D) and 2 months (E) of light return (D). Alaria esculenta at 8°C the last day of darkness (F). Saccharina latissima at 3°C (G) and 8°C (H) the last day of darkness. Phycodrys rubens at 3°C (I) and 8°C (J) the last day of darkness. Ptilota gunneri at 3°C (K) and 8°C (L) the last day of darkness.
Respiration and Photosynthesis
The respiration rates were measured monthly during the dark period and 7 days after light was switched on (Figure 3). The latter was not possible in A. esculenta, since only the holdfast and mid-rib remained by the end of the dark period at 8°C, and the new tissue that appeared at 3°C was not enough to carry out the measurements. During the dark months, A. esculenta gradually increased its respiration rate at 8°C, but it decreased at 3°C. A slight downregulation of the respiration at 3°C was also observed in all the other three species, but it was somewhat less clear, or absent, at 8°C. The onset of the photoperiod activated the metabolism in the three species measured, except in S. latissima at 8°C. At 3°C, the respiration rate was markedly higher than the initial values in S. latissima, while in the red species it reached values close to the initial ones at both temperatures.
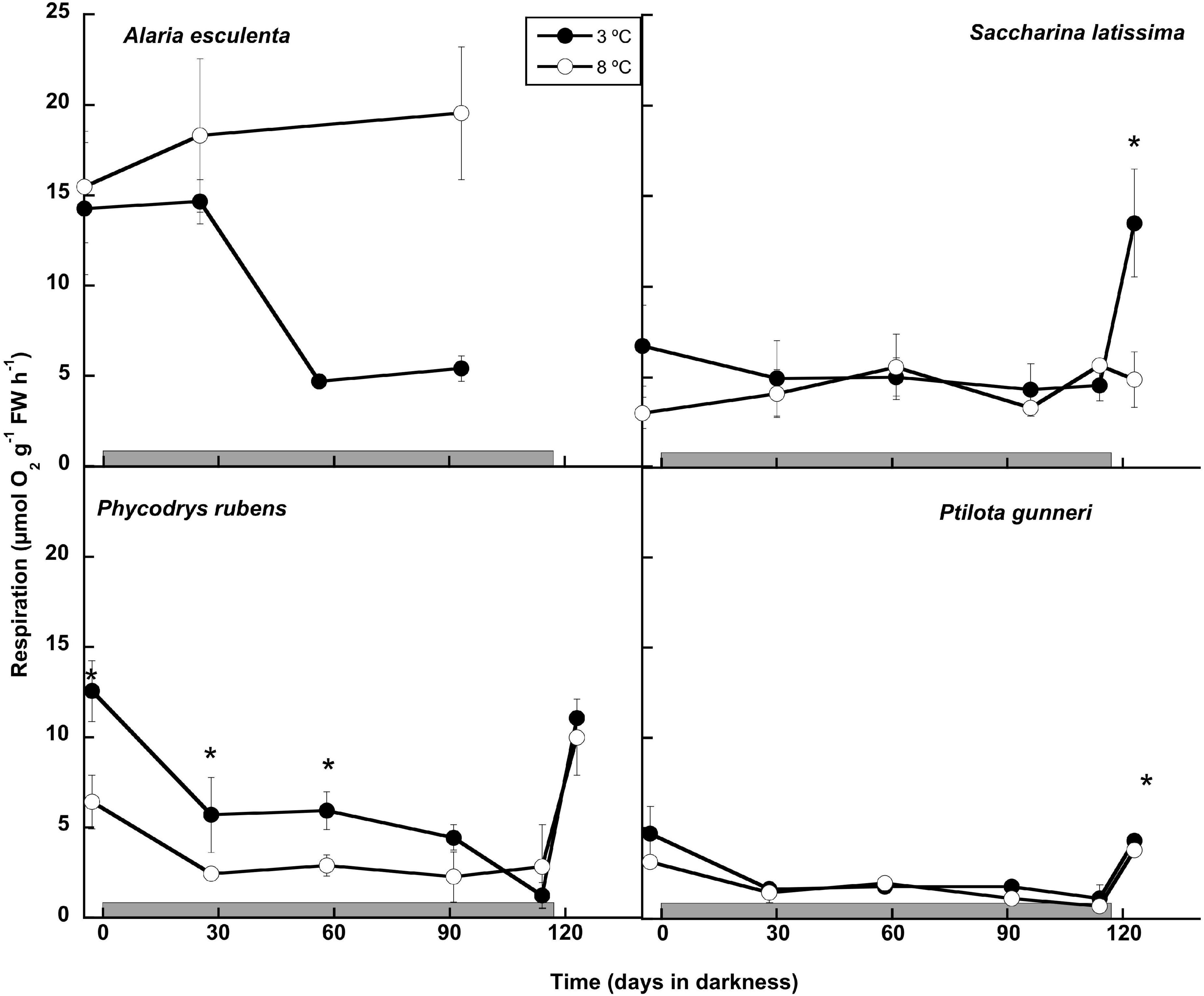
Figure 3. Respiration rate measured as O2 consumption during the 16 weeks of incubation in darkness (gray horizontal bar) of Alaria esculenta, Saccharina latissima, Phycodrys rubens, and Ptilota gunneri at two different temperatures. Data are mean of 3 replicates (error bars for standard deviation). Significant differences between temperatures marked with an asterisk.
The potential to maintain the capacity to perform photosynthetic activity during the dark period was analyzed both by O2 evolution (Figure 4), and by rapid light curves using PAM fluorometry (Table 1). All thalli started to evolve O2 immediately after illumination of the O2 chambers throughout the long dark period. The photosynthetic O2 evolution rates decreased gradually with time in most cases, and only P. gunneri retained values close to the initial ones at 3°C, but not at 8°C (Figure 4). Alaria esculenta and P. rubens showed a decrease in performance at high temperature from the beginning, pointing to an early detrimental effect during the previous temperature acclimation phase. Most cultures that survived the long dark period showed an ability to restore the photosynthetic O2 evolution to the initial levels after 1 week of the onset of a photoperiod. This was not the case for S. latissima at 8°C.
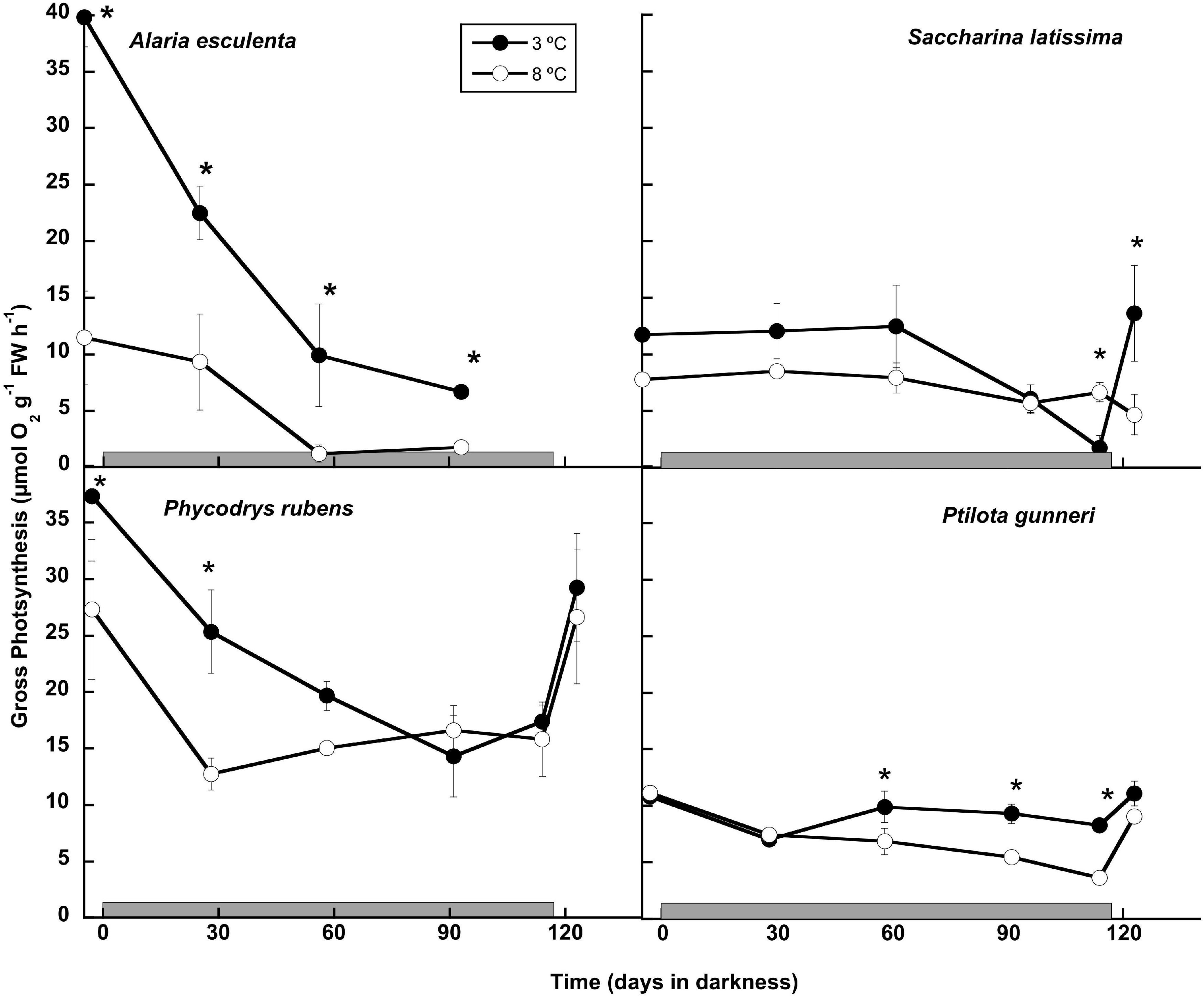
Figure 4. Gross photosynthetic rate measured as O2 production during the 16 weeks of incubation in darkness (gray horizontal bar) of Alaria esculenta, Saccharina latissima, Phycodrys rubens, and Ptilota gunneri at two different temperatures. Data are mean of 3 replicates (error bars for standard deviation). Significant differences between temperatures marked with an asterisk. See details for photoperiod in the text.
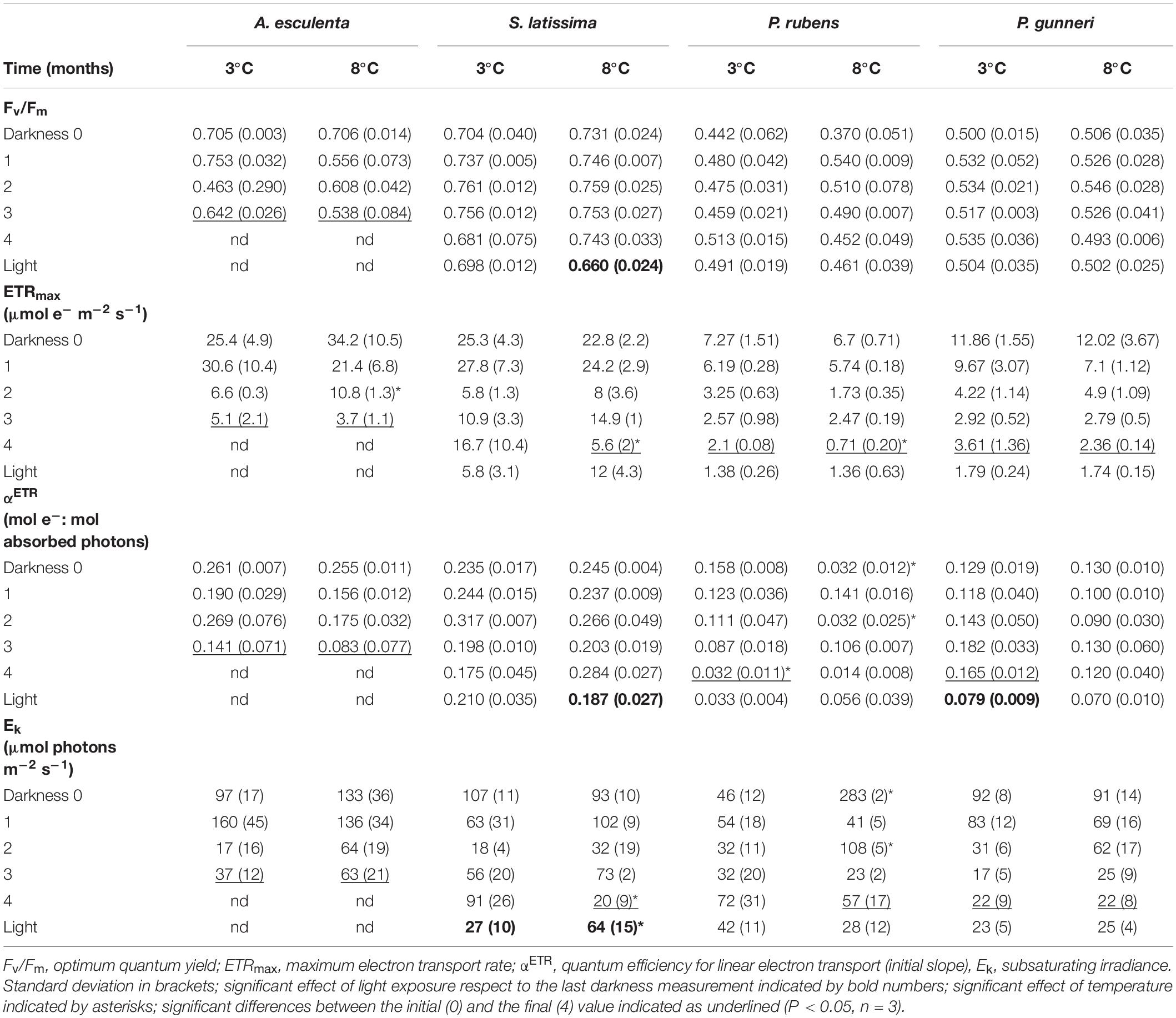
Table 1. Parameters from rapid light curves using PAM fluorometry fitted according to Jassby and Platt (1976) measured during the dark period (Darkness, months) and 7 days after light exposure at increasing photoperiod (Light).
The photosynthetic parameters derived from PAM chlorophyll fluorescence reflected the ability to perform photosynthetic electron transport throughout the darkness period. The initial values of the optimum quantum yield (Fv/Fm) were those commonly recorded in brown and red seaweeds, above 0.7 and around 0.5, respectively (Table 1). During the dark period, Fv/Fm did not show any significant decrease in any species except A. esculenta, which still showed values above 0.6 at 3°C but they decreased to 0.5 at 8°C, before disintegrating the blade (after 3 months). The return of light did not increase Fv/Fm in any species, and only a slight decrease was detected in S. latissima at 8°C.
The maximum electron transport rate (ETRmax) was the parameter most affected during winter. The initial values varied from around 7 μmol e– m–2 s–1 in the rhodophyte P. rubens to 34 μmol e–m–2 s–1 in S. latissima, with consistently higher values in brown compared to red species (Table 1). In all species, and in both temperature treatments, ETRmax values decreased with time, except S. latissima at 3°C, which was still able to transport ca. 16 μmol e– m–2 s–1 in the few thalli that remained healthy in the culture. The onset of light after the dark period did not promote a significant recovery in ETRmax in any situation.
The quantum efficiency of ETR (αETR) is the initial slope of the rapid light curves (Table 1). The initial values ranged from around 0.25 moles of transported electrons by mol of absorbed photons for the two brown species to values below 0.16 in the two red ones. The pattern of variation was unstable under all treatments, and the values at the end of the dark period had decreased compared to the initial ones in A. esculenta (regardless of the temperature), and in both red species at 3°C. The onset of light after the dark period did not produce any significant increase in αETR values, but a decrease in S. latissima at 8°C and in P. gunneri at 3°C was observed.
The subsaturating irradiance (Ek) was close to 100 μmol e–m–2 s–1 for all species at the beginning of the dark period, except for the rhodophyte P. rubens. In this species, an unusual high value of over 280 μmol e– m–2 s–1 at 8°C might indicate a transient acclimation to high temperatures that disappeared after 1 month in darkness. A general decrease in Ek was observed for all species, and values at the end of the dark period fell below 100 μmol e– m–2 s–1 in all cases. These values were particularly low in P. gunneri, being 22 μmol e– m–2 s–1 at both temperatures. Upon the return of light, Ek of S. latissima was the only significantly affected, showing a drop from 91 to 27 μmol e– m–2 s–1 at 3°C, but an increase from 20 to 64 μmol e– m–2 s–1 at 8°C (Table 1).
A complete P-I curve was performed in the O2 evolution chambers at the end of the dark incubation period and 1 week after the onset of a photoperiod (Figure 5). The parameters from P-I curve fittings are shown in Table 2 (no data available for A. esculenta). Surprisingly, no photoinhibition was observed in any case even at an irradiance as high as 300 μmol photons m–2 s–1. None of the photosynthetic parameters derived from O2 evolution (LCP, Pmax, α, and IK) were significantly affected by the temperature by the end of the dark period. However, some differences appeared when the algae progressively returned to a photoperiod. In S. latissima, Pmax increased by 80% at 3°C after 1 week in a 5 h light/19 h dark photoperiod, while it decreased by 40% at 8°C. This meant that Pmax at 8°C was only 35% than that at 3°C. A similar behavior was found in the photosynthetic efficiency (α), increasing nearly four times after the return of the light at 3°C, accompanied by a small drop at 8°C. This behavior was very similar in both rhodophytes. Both Pmax and α increased at 3°C after returning to the photoperiod compared to the end of the dark period, but with little or no differences between 3 and 8°C. Finally, the saturation irradiance (Ik) was only affected by the photoperiod in S. latissima at 3°C. As shown in Table 2, photosynthesis was saturated at approximately half of the irradiance after 1 week under L/D cycles.
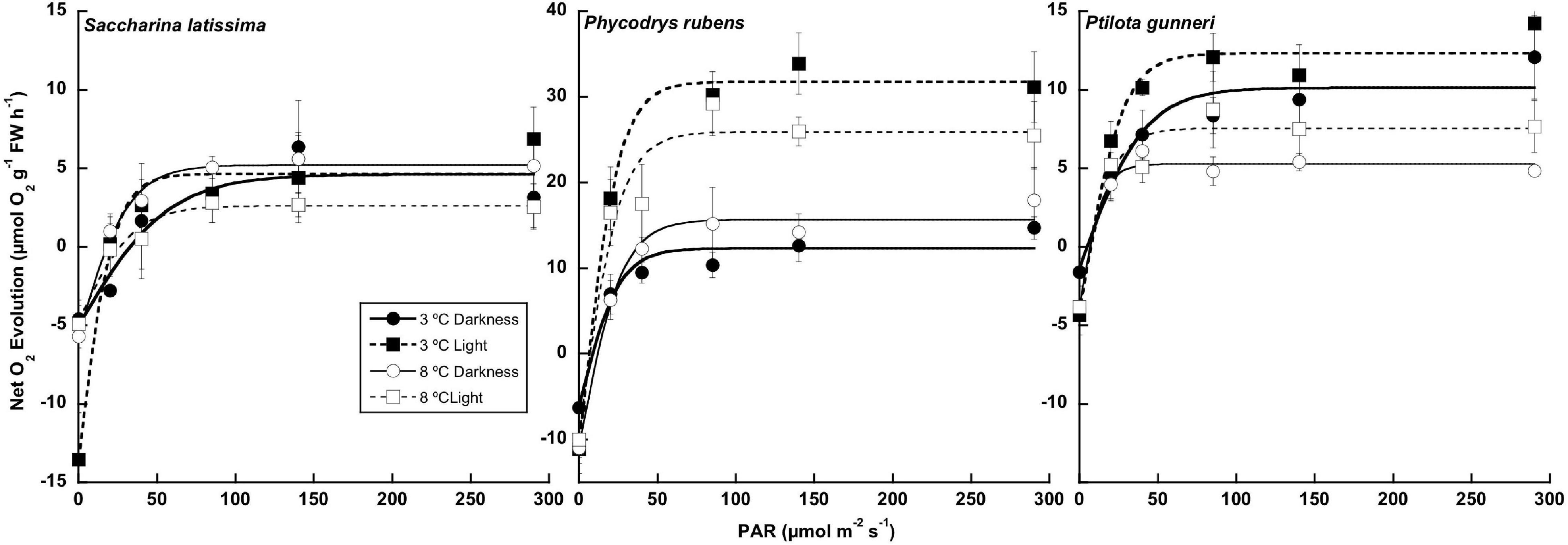
Figure 5. Net photosynthetic rate measured as O2 consumption at the end of the 16 weeks of incubation in darkness (Darkness), and 1 week after the onset of a photoperiod (Light) of Saccharina latissima, Phycodrys rubens, and Ptilota gunneri at two different temperatures. Data are mean of 3 replicates (error bars for standard deviation).
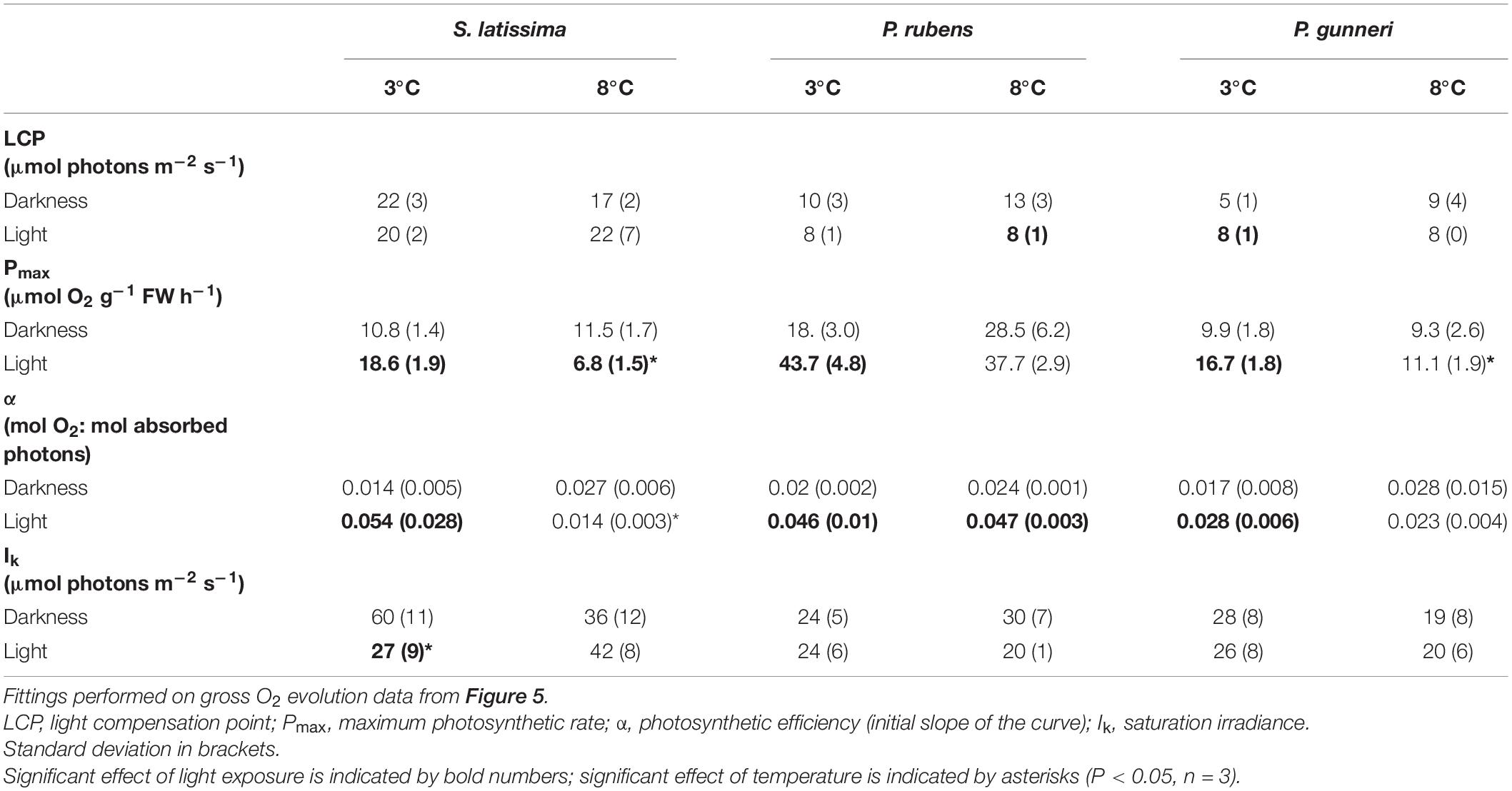
Table 2. Parameters from P-I curves fittings according to Jassby and Platt (1976) measured at the end of the dark period (darkness) and 7 days after light exposure at increasing photoperiod (light).
Composition
The concentration of chlorophyll a showed little change throughout the experiment, except for A. esculenta, in which it decreased gradually. No chlorophyll content could be measured after week 13 in this species as the blade disintegrated, with only the holdfast and the mid-rib remaining (Figures 2, 6). The temperature did not influence the chlorophyll a content, and significant differences were transitory, except in A. esculenta that already showed a lower concentration at the beginning of the dark period at 8°C, most likely due to some initial loss during the previous acclimation phase.
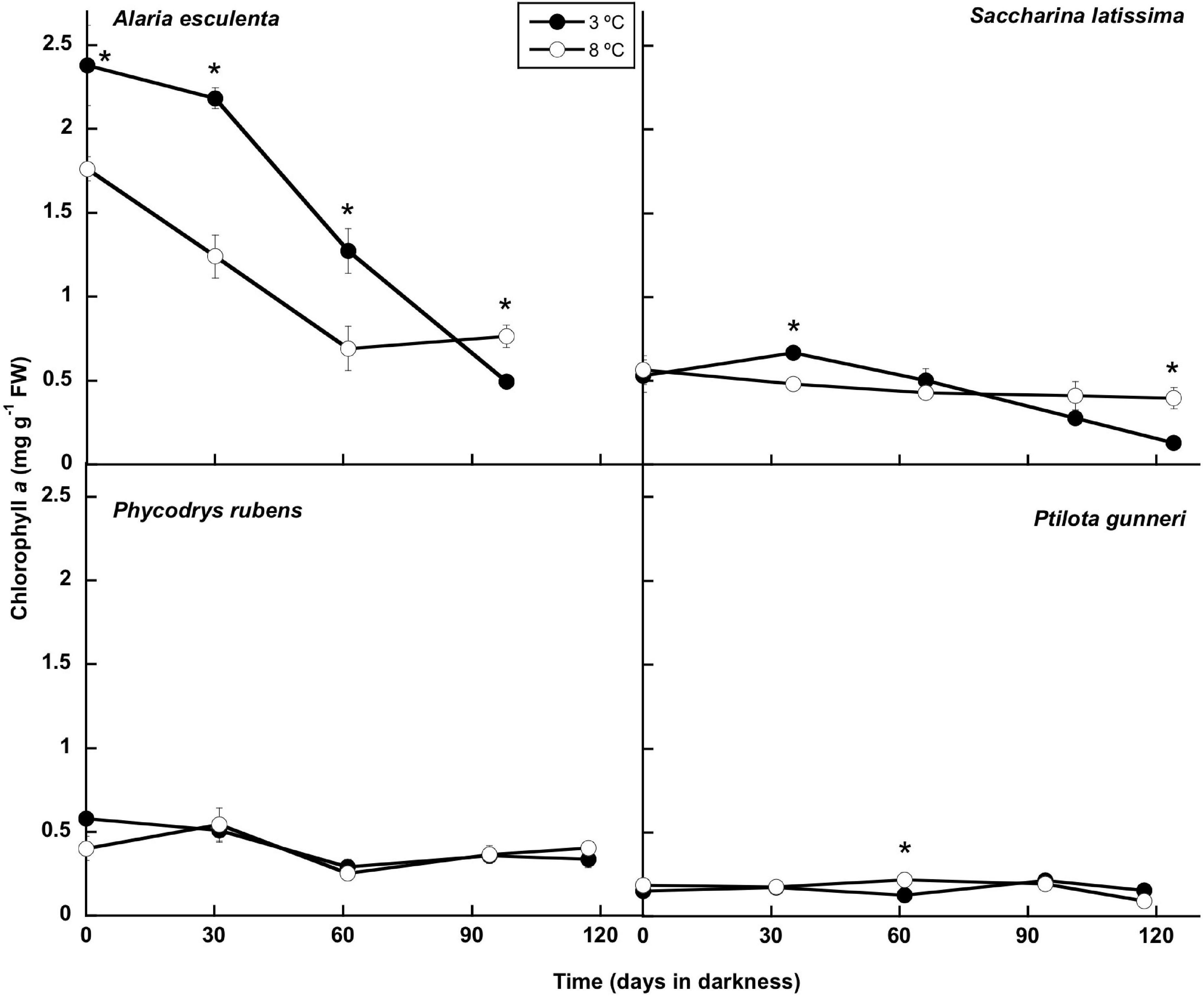
Figure 6. Chlorophyll evolution during the 16 weeks of incubation in darkness of Alaria esculenta, Saccharina latissima, Phycodrys rubens, and Ptilota gunneri at two different temperatures. Data are mean of 5 replicates (error bars for standard deviation). Significant differences between temperatures marked with an asterisk.
The composition of the thalli (total C and N, and total carbohydrates, lipids, and proteins content) was studied during the simulated winter period. The total C content of the thalli remained very stable during this period (Figure 7), and no significant differences were found between both temperatures. Only in A. esculenta, a small and progressive decrease of C was detected, most probably due to the disintegration of the old blades. This phenomenon occurred slightly faster at 8 than at 3°C.
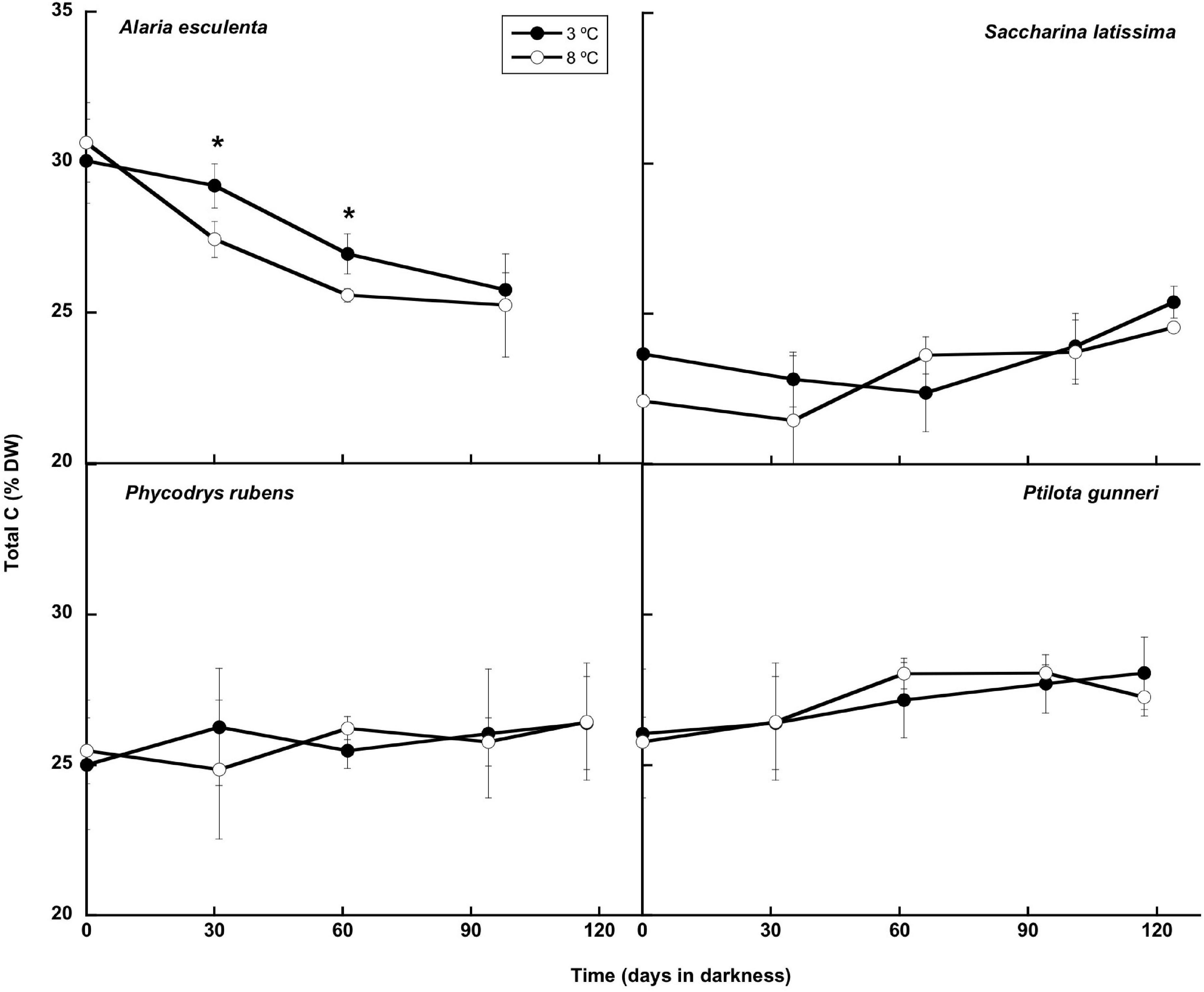
Figure 7. Total C during the 16 weeks of incubation in darkness of Alaria esculenta, Saccharina latissima, Phycodrys rubens, and Ptilota gunneri at two different temperatures. Data are mean of 5 replicates (error bars for standard deviation). Significant differences between temperatures marked with an asterisk.
The total nitrogen content (Figure 8) presented small oscillations around the initial value in A. esculenta, P. rubens, and P. gunneri at 3 and 8°C, and in S. latissima at 8°C. In this latter species, a small increase of the N content was found at 3°C at the end of the dark period. When the contents of C and N were considered together (C:N molar ratio: Figure 9), the behavior differed from one species to another. The C:N ratio did not vary in P. rubens during the experiment at any temperature, with values around 8, while in the other rhodophyte, P. gunneri, it increased from 8 to 10 at both temperatures after the first month of darkness, due to the drop in the N content. In the ochrophytes, the initial C:N values were higher (around 12), and generally decreased to values between 9 and 10, except in L. saccharina at 8°C, that remained unchanged. In A. esculenta, this drop was due to the loss of C, while in S. latissima at 3°C, it was due to the increase of N.
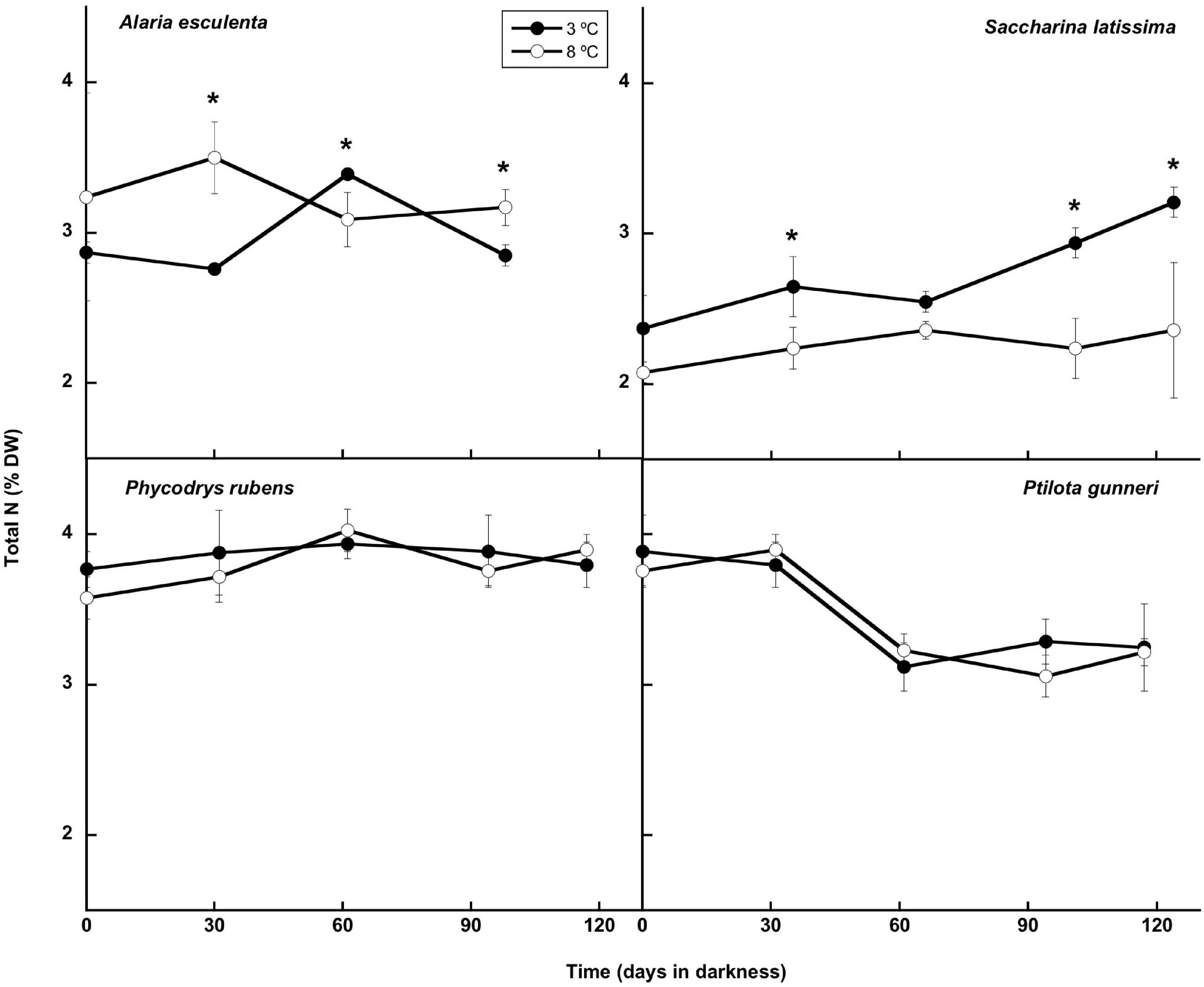
Figure 8. Total N during the 16 weeks of incubation in darkness of Alaria esculenta, Saccharina latissima, Phycodrys rubens, and Ptilota gunneri at two different temperatures. Data are mean of 5 replicates (error bars for standard deviation). Significant differences between temperatures marked with an asterisk.
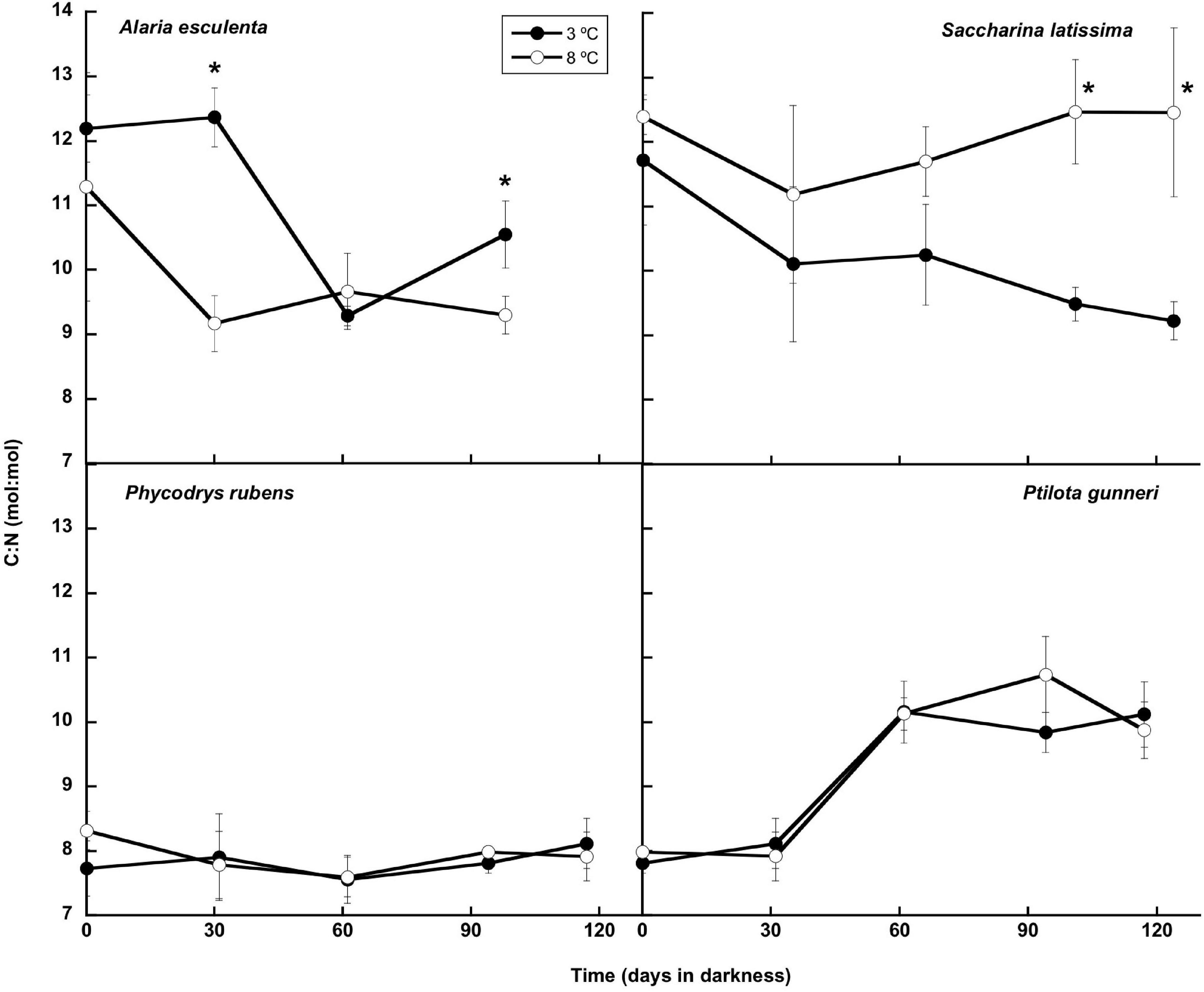
Figure 9. C:N molar ratio during the 16 weeks of incubation in darkness of Alaria esculenta, Saccharina latissima, Phycodrys rubens, and Ptilota gunneri at two different temperatures. Data are mean of 5 replicates (error bars for standard deviation). Significant differences between temperatures marked with an asterisk.
The biochemical composition (carbohydrates, proteins, and lipids) of the four species of macroalgae is shown in Table 3. In A. esculenta, the carbohydrate content significantly dropped at the end of the experiment at 8°C, while proteins did not change at any temperature. On the contrary, lipids decreased by nearly 50%, without differences between 3 and 8°C. In S. latissima, both carbohydrates and proteins significantly increased between day 0 and week 16, as lipids did at 3°C (the increase in lipids at 8°C was not significant). Additionally, the carbohydrate content was higher at 8 than at 3°C. Phycodrys rubens presented the highest concentration of carbohydrates, proteins, and lipids of all the species analyzed, and their content was very stable during the dark incubation period. Only the lipid content was significantly higher in the last measurements. For this species, some temporary effects of warming also occurred. The protein content was slightly higher at 8 than at 3°C at the end of the experiment, as well as that of lipids at the onset of the dark incubation. Finally, P. gunneri showed only a significant decrease of carbohydrates at the end of the dark period, independently of the temperature. Additionally, as in the case of P. rubens, the initial lipid concentration was higher at 8 than at 3°C.
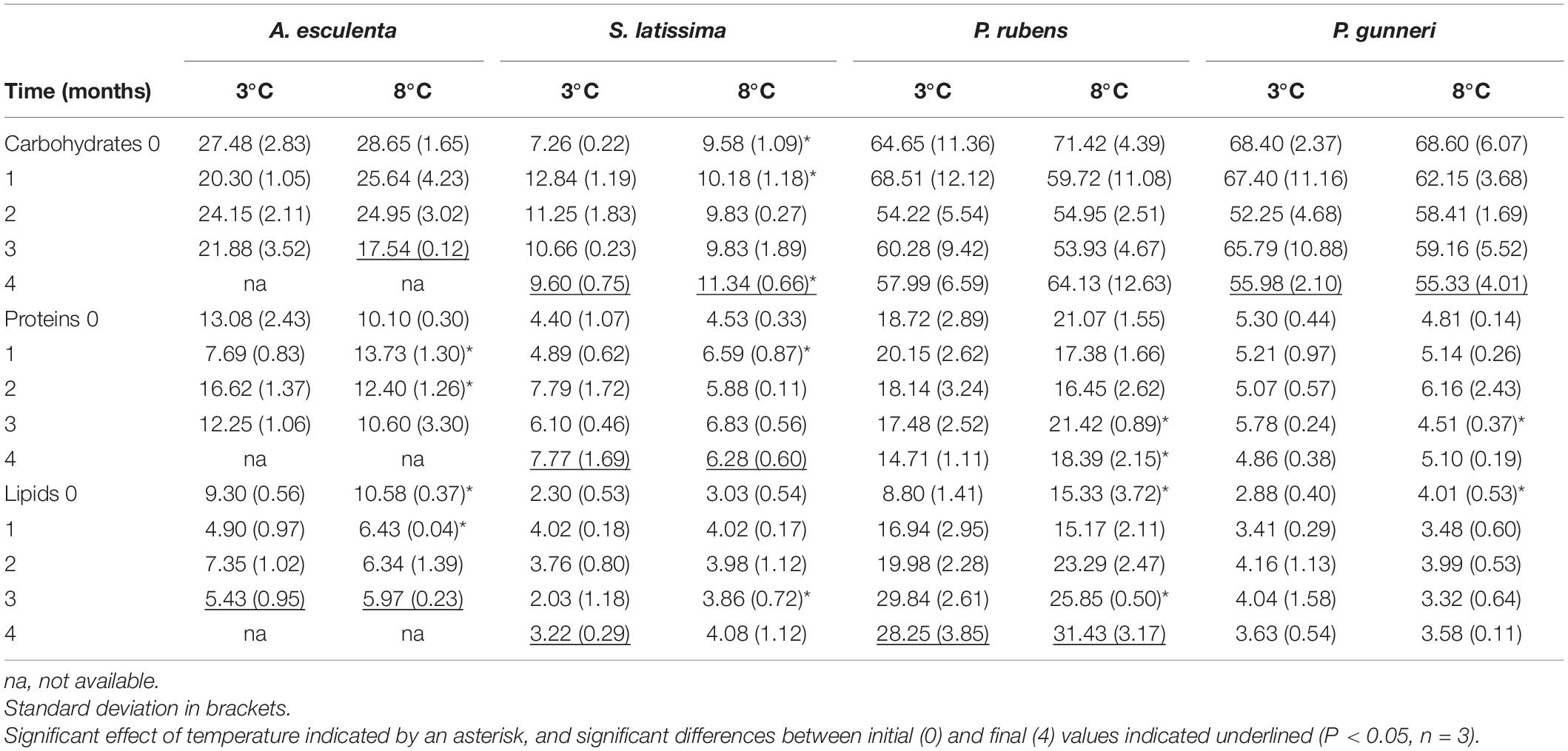
Table 3. Total carbohydrates, proteins and lipid content (in mg g–1 FW) measured during the dark period (months).
Discussion
In Kongsfjorden, the absence of ice cover has shortened the polar night, so that light penetrating the water column occurs as soon as the sun is above the horizon, while the coldest seawater temperatures are recorded by the end of March or the beginning of April (COSYNA underwater observatory2). In this sense, in our experiments, we followed the natural photoperiod by exposing algae to complete darkness in accordance with the time the sun is below the horizon in Kongsfjorden (i.e., 112 days). This is the minimum duration of the polar night at this latitude as defined for ice algae photosynthetic ability (Berge et al., 2020), so that, under natural conditions, darkness could be even longer than in this study, at least for ice-covered areas.
Polar macrophytes must show a high potential for dark survival, as they must live up to 4 months (or even more) in complete darkness (Schlie et al., 2011), but the physiological, biochemical, and molecular mechanisms behind this adaptation for dark survival are still poorly understood (Karsten et al., 2019). Some mechanisms for long-term dark survival have been described in polar diatoms (McMinn and Martin, 2013), including the utilization of stored products (Palmisano and Sullivan, 1983; Schaub et al., 2017), the reduction of respiration and metabolic rates (Peters and Thomas, 1996), the formation of resting stages (reviewed by McQuoid and Hobson, 1996), or facultative heterotrophy (Hellebust and Lewin, 1977; Armbrust et al., 2004). The utilization of storage products by macroalgae during long periods of darkness has also been reported (Weykam et al., 1997; Scheschonk et al., 2019).
In the present work, a laboratory simulation of Arctic polar nights for 16 weeks showed that all four species were more resilient at 3 than at 8°C, and that both kelps were unable to resume growth after being cultivated at 8°C. At low temperatures, the appearance of new tissue in A. esculenta by the end of the dark period partially resembles the life strategy of the endemic kelp Laminaria solidungula. In this species, new blade formation begins in early winter (mid-November to early December; Chapman and Lindley, 1980), when the sun remains entirely below the horizon for 24 h a day. Blade elongation continues through winter with maximal rates in late winter to early spring, even under thick ice. This behavior has been called “season anticipator” (Kain, 1989), in contrast to “season responder,” which encompasses the majority of species that sustain their fastest growth in spring and early summer according to light penetration in the water column. To our knowledge, this is the first time that new tissue production under complete darkness has been reported for A. esculenta. Similarly, we also observed some new tissue in S. latissima, but this was restricted to the holdfast. In both species, a warmer dark period precluded new tissue formation, thus compromising interannual growth and survival. In the case of the rhodophytes, even though a significant loss of thallus weight was detected in P. rubens at both temperatures and in P. gunneri at 8°C, no morphological damage was apparent, and photosynthetic O2 evolution was immediately resumed in all cases after the return of the photoperiod. This indicates a high resilience of both rhodophytes to warming during the polar night. Warmer temperatures during early summer enhanced the growth rate of S. latissima (Iñiguez et al., 2016) and P. rubens (Gordillo et al., 2016), but not significantly in A. esculenta and P. gunneri (formerly named as P. plumosa) (Gordillo et al., 2016). Hence, the effect of increased temperature is season dependent, i.e., there is no correspondence between the response to warming during the photosynthetically active growing season and their ability to overcome long periods of darkness. Since winter survival seems to rely on the accumulation of carbohydrates during the end of the summer and early autumn, one may argue that warming may favor carbohydrate accumulation, balancing out the overall effect of warming in a per year basis. However, recent data from our group (Jiménez et al., unpublished) from experiments performed in summer and early autumn incubating these species at 3 and 8°C proved that there were not significant differences in neither carbohydrates nor total C content between the two temperatures. Then, it is not expected that warming during summer and early autumn will compensate for the higher biomass degradation observed during the polar night.
Despite the high relevance of the respiration rate for surviving long dark periods in the cold, there is very little information on Arctic macroalgae. The increase in the respiration rate at 8°C seemed to have been responsible for the faster degradation of the thalli of A. esculenta, but it does not explain the faster degradation of S. latissima and P. rubens, both showing lower respiration rates at 8°C at the beginning of the dark period. S. latissima is known to have its temperature optimum well above 3°C, even in Kongsfjorden populations (Olischläger et al., 2014), so, 3°C is a more stressful temperature than 8°C in summer (Parages et al., 2013). Nevertheless, low water temperature seems to be a prerequisite in this species to overcome the long dark period during the polar night. Similarly, P. rubens benefits from higher temperatures in summer (Gordillo et al., 2016), and lower respiration rates at 8°C were also recorded at the beginning of the dark period, despite biomass degradation happening at a higher rate than at 3°C. In other words, higher respiration rates as a response to low temperatures are observed when S. latissima and P. rubens perform a photosynthetic activity (under a photoperiod), but they are not sustained during prolonged periods of time in darkness. Scheschonk et al. (2019) found no difference in the respiration rates in S. latissima in October compared to February. However, we did observe a reactivation of respiration in February, but only 1 week after re-illumination at 3°C. At 8°C, this reactivation did not occur. The reactivation of respiration upon illumination was also observed in the two red species at similar levels under both temperatures, emphasizing the resilient character of these rhodophytes to a warm polar night.
It has been commonly observed that polar microalgae (both planktonic and benthic) can reactivate photosynthesis upon re-illumination during the polar night (Wulff et al., 2008; Schaub et al., 2017; Kvernvik et al., 2018). To our knowledge, there is no information available on macroalgae to this respect. Scheschonk et al. (2019) found no difference in the photosynthetic performance in S. latissima and L. solidungula in February compared to the previous October in the same fjord of our study. However, their measurement in February was not carried out in total darkness, so some reactivation during the previous illuminated days could have occurred. This seems not to be the case, since in our experiment, all four species were able to respond immediately to illumination at any stage during the darkness period, evolving O2 in the photosynthetic chambers without any noticeable lag. Nonetheless, warming affected the four species, although at different times during darkness, evidencing species-specific traits in photosynthetic abilities during darkness. As reported for S. latissima by Scheschonk et al. (2019), some photo-physiological parameters remained largely unaffected over the course of the polar night. However, that was not the case for the other kelp, A. esculenta, and the two red species, which progressively lost some ability to perform electron transport at both temperatures. To some extent, these drops were also reflected in the chlorophyll content, so that some pigment restructuration must have taken place. The fact that Fv/Fm was more stable than ETRmax may indicate that the reorganization was affecting PS I more than PS II, although gross O2 evolution also diminished. A decrease in photosynthetic electron transport efficiency, along with a decrease in the pigment content and a shrinkage of the chloroplasts is a well-known, long-term response to extended dark periods in microalgae (Wulff et al., 2008; Reeves et al., 2011; Veuger and van Oevelen, 2011; Kvernvik et al., 2018).
Warming also affected the way macroalgae recovered from darkness upon re-illumination. The improvement in photosynthetic performance measured as O2 evolution, particularly its Pmax, was not reflected in the equivalent parameters from PAM fluorimetry. This may indicate that recovery relies more on cyclic electron transport in PS II than in linear electron transport between PS II and PS I. A further study on the specific activity of each photosystem is required to elucidate this issue. Bischof et al. (2002) found that ETRmax took 2 weeks for recovery after ice breakup in late spring in brown and red seaweeds sampled directly from Kongsfjorden. In the Antarctic rhodophyte Palmaria decipiens, Lüder et al. (2002) found that the recovery of ETRmax after 6 months of darkness was small upon 7 days of re-illumination, and it took over 2 weeks to get close to full recovery. In contrast, photosynthetic O2 production was detected after only 3 days of re-illumination in the red species Delesseria sanguinea, incubated for 9 months in darkness (Lüning and Schmitz, 1988).
In the present work, the total Chl a content was also very stable throughout the whole dark period in both rhodophytes, P. rubens and P. gunneri, as well as in S. latissima at 8°C—a small decrease occurred in this last species at 3°C after 2 months in complete darkness. However, a significant increase in the Chl a content was found in this species after 1 month, in agreement with the results of Lacour et al. (2019) in the Arctic diatom Chaetoceros neogracilis. This might be an acclimation response to reduced irradiance at the onset of the dark period. Similarly, Graiff et al. (2021) reported that the maximum Chl a in the ochrophyte Fucus vesiculosus from the Baltic Sea was found in winter. A different behavior appeared in A. esculenta, showing a very significant decrease of Chl a during the first weeks of dark incubation. This differential response of A. esculenta could be due to the degradation of the old blade during the winter. Then, most probably, none of these species used pigments during the polar night as reserve products to survive darkness. Additionally, a high pigment content might ensure a rapid and efficient response at the onset of the photoperiod in mid-February. Scheschonk et al. (2019) did not find any significant variation in the photosynthetic pigment content (Chl a, fucoxanthin, violaxanthin, antheraxanthin, and zeaxanthin) when comparing S. latissima and L. solidungula from Kongsfjorden in October (before the polar night) and February (at the beginning of the illuminated period). Lüder et al. (2002) described that in the Antarctic-endemic rhodophyte Palmaria decipiens, phycobiliproteins (considered as N reserve in red algae) started to decrease only after 4 months in darkness, while no decrease in the Chl a content occurred before the fifth month in the absence of light. In contrast, Wulff et al. (2008) found degradation of pigments from a natural benthic diatom community from Antarctica after “only” 64 days in darkness. Similarly, Karsten et al. (2019) reported that pigments in the benthic diatom Surirella cf. minuta from Kongsfjorden began to degrade after a few days in darkness. The reduction in the pigment content might be the result of acclimation to dark conditions, in which pigments are not already needed. However, a possible use of the carbon skeletons and of N, (in the case of phycobiliproteins) for the maintenance of basal metabolism during prolonged darkness cannot be discarded.
Generally, no carbon was lost during dark incubation, either at 3 or 8°C, except in A. esculenta. Previous reports (Scheschonk et al., 2019) concluded that the ability of Arctic kelps to persist under polar night conditions correlates with the consumption of stored laminarin, whose content was reduced by 96% in S. latissima and by 90% in L. solidungula during the polar night. The total C content followed this same trend, decaying from October to February. This behavior, in which the tissue content of carbon storage products peaks in late summer and declines to a minimum in late winter/early spring, was already described by Black (1950) for the Laminariaceae of the British Islands and by Mann (1973). Nielsen et al. (2014) found a similar behavior of the C content in S. latissima from Aarhus Bay (Denmark, 56°N), with maximum levels in November and minimum in March. Nevertheless, the differences were very subtle. However, in the present work, a significant drop of C during winter was found only in A. esculenta, but not in S. latissima, nor in the rhodophytes. Carbon losses during winter may occur by respiration (consumption) of stored carbohydrates and/or by blade erosion. In the case of S. latissima, no change in respiration occurred during winter, as already reported by Scheschonk et al. (2019), while in the rhodophytes, a significant decrease was found. Another mechanism for keeping a stable C content in S. latissima might be an efficient translocation of C from the center and distal regions of the blade to the meristem via the sieve tube/trumpet cells (Küppers and Kremer, 1978; Kremer, 1981; Johnston and Raven, 1986). Finally, some dark carbon uptake during winter cannot be discarded (Johnston and Raven, 1986).
Nitrogen in Arctic waters is predominantly available during winter and early spring mostly in the form of nitrate, being almost completely depleted in summer (Piquet et al., 2014; van De Poll et al., 2016). Kelps are able to internally store inorganic nitrogen during the high-availability season (Korb and Gerard, 2000; Gevaert et al., 2002), to be used for growth during spring and early summer. In this work, different responses of the N content during prolonged darkness were found. Scheschonk et al. (2019) did not find any change in the nitrogen concentration in L. solidungula between October and February—according to these authors, most probably, no nitrogen demand existed during the polar night due to a low protein synthesis in the dark. However, in contrast to this Arctic-endemic species, the N content increased in the meristem and center regions of the blades of S. latissima during the dark period, as we also found at 3°C in this work. Nielsen et al. (2014) reported data of the N content in the blades of S. latissima in Denmark of 3.16–3.48% DW, while Scheschonk et al. (2019) gave values close to 2.5% DW in February 2017 in S. latissima from Kongsfjorden. In the present study, the N concentrations in February, after 16 weeks in darkness, were around 2.3% DW at 8°C and 3.2% DW at 3°C, very similar to previous reports. Both rhodophytes had an N content between 3 and 4% DW during the whole experiment, while in A. esculenta, the concentrations oscillated around 3% DW. These values are higher than those reported by Gordillo et al. (2006) in mid-summer, indicating that some N uptake and storage must have taken place at some point from late summer to late winter.
Scheschonk et al. (2019) reported a significant decrease in the C:N ratio in S. latissima during the dark period from ∼28 to ∼11 in the meristem, due to the decrease in internal C and the increase in internal N. C:N values in October in the meristem were around 28, much higher than the ones reported in the present study, most probably due to our preincubation with nutrient-enriched seawater before laboratory dark treatment, and the use of older plants. Nielsen et al. (2014) also found values of C:N in September in the natural medium of ∼25, sharply decreasing to 5.6–9.4 in March. In our case, the minimum C:N was around 9, similar to the values reported by these authors in March 2000. The C:N ratio in P. rubens showed a behavior similar to the Arctic-endemic kelp L. solidungula (Scheschonk et al., 2019), i.e., without any significant change during the dark period. Apparently, neither the C nor N content significantly varied in any of these species.
The biochemical composition was affected by darkness and temperature, depending on the species. Major changes in the biochemical composition were found in S. latissima. In all cases, the highest increases occurred at 3°C compared to 8°C. These increases in macromolecules correlated with a higher C content in this species at the end of the experiment. It is generally accepted that the carbohydrate content decreases in Arctic kelps during the polar night, however, in this study, this only happened in A. esculenta at 8°C. This might indicate a very efficient translocation of cell components from the distal to central and meristematic zones during darkness in S. latissima, as already proposed by Nielsen et al. (2014) and Scheschonk et al. (2019), among others.
According to IPCC (2014), the surface temperature in the Arctic seawater is predicted to rise between 2 and 5°C, or even more, until the end of this century. In the case of kelps, Lüning (1990) already pointed that, since temperature is a major environmental factor that controls their development during all life-history stages, including germination, gametogenesis, and recruitment, changing temperatures may not only affect the development of single species, but also alter the species’ interactions and, consequently, the ecosystem’s structure (Nabivailo and Titlyanov, 2006; Lathlean et al., 2017; Brooks and Crowe, 2018; Griffith et al., 2018; Liesner et al., 2020). Some authors propose that in kelp species with temperature-mediated sex ratios, increasing temperatures might lead to population declines (Lee and Brinkhuis, 1988; Izquierdo et al., 2002; Nelson, 2005; Oppliger et al., 2011; Monteiro et al., 2019), especially at sites where species are close to their upper thermal tolerance limit (Ospina-Alvarez and Piferrer, 2008; Hays et al., 2017). Also, the strong decrease in sea-ice cover in Kongsfjorden is probably one of the major factors behind the increase of seaweed biomass off Hansneset between 1996–98 and 2012–13, particularly in the intertidal and upper subtidal zones (Bartsch et al., 2016). In addition, it is commonly accepted that the increasing seawater temperature is influencing the growth of many marine species, thus altering species composition and relative abundances, potentially changing the food web structures (Yletyinen, 2019). In agreement with Coello-Camba and Agustiì (2017), when growth is affected by warming, substantial alterations in phytoplankton responses may be expected and, therefore, in the whole polar ecosystem. Our data, among others, indicate that these presumed changes might be expected in polar phototrophs in general, now including macroalgae, and in overwintering species in particular. In combination with other environmental drivers, these changes can decrease the community resilience, and food webs may abruptly shift to a new state with different species interactions, ecosystems diversity, and ecological services (Coello-Camba and Agustiì, 2017).
Conclusion
A warmer polar night might pose a limit to the occurrence of multiyear individuals of some species, especially some common kelps. In contrast, red species showed higher resilience to warming during the polar night. Although a warmer and less ice-covered Arctic may potentially favor macroalgal expansion at a pan-Arctic level, some uncertainty still exists (Krause-Jensen et al., 2020). On one side, the suspended sediment from glacial run-off significantly reduces light to the seabed, effectively nullifying the benefits of an increased ice-free season on annual macroalgal growth (Bonsell and Dunton, 2018). On the other hand, unlike subarctic areas with an operating photoperiod the whole year (Husa et al., 2014), a warmer and long polar night restricts the possibility for newcomers to thrive interannually, so that their presence will potentially be restricted to the spring-summer season.
Data Availability Statement
The original contributions presented in the study are included in the article/Supplementary Material, further inquiries can be directed to the corresponding author/s.
Author Contributions
All authors contributed equally to the different stages of the research and approved the final version.
Funding
Financial support for this research was provided by the Spanish Ministry of Science, Innovation and Universities (project CGL2015-67014R).
Conflict of Interest
The authors declare that the research was conducted in the absence of any commercial or financial relationships that could be construed as a potential conflict of interest.
Publisher’s Note
All claims expressed in this article are solely those of the authors and do not necessarily represent those of their affiliated organizations, or those of the publisher, the editors and the reviewers. Any product that may be evaluated in this article, or claim that may be made by its manufacturer, is not guaranteed or endorsed by the publisher.
Acknowledgments
We would like to thank Manuel Macías (financed by the regional government, Junta de Andalucía) for technical assistance. We would also like to thank Inka Bartsch and Kai Bischof for logistical assistance. We would also further like to thank the diving team of the Alfred Wegener Institut at Bremerhaven (Germany) headed by Max Schwanitz.
Supplementary Material
The Supplementary Material for this article can be found online at: https://www.frontiersin.org/articles/10.3389/fmars.2021.750209/full#supplementary-material
Footnotes
- ^ https://dashboard.awi.de/?dashboard=2847
- ^ www.awipev.eu/awipev-observatories/underwater-observatory/
References
Armbrust, E. V., Berges, J., Bowler, C., Green, B. R., Martinez, D., Putnam, N. H., et al. (2004). The genome of the diatom Thalassiosira pseudonana: ecology, evolution, and metabolism. Science 306, 79–86. doi: 10.1126/science.1101156
Barnes, H., and Blackstock, J. (1973). Estimation of lipids in marine animals and tissues: detailed investigation of the sulphophosphovanilun method for ‘total’ lipids. J. Exp. Mar. Biol. Ecol. 12, 103–118. doi: 10.1016/0022-0981(73)90040-3
Bartsch, I., Paar, M., Fredriksen, S., Schwanitz, M., Daniel, C., Hop, H., et al. (2016). Changes in kelp forest biomass and depth distribution in Kongsfjorden, Svalbard, between 1996–1998 and 2012–2014 reflect Arctic warming. Polar Biol. 39, 2021–2036.
Berge, J., Renaud, P. E., Darnis, G., Cottier, F., Lastd, K., Gabrielsenb, T. M., et al. (2015). In the dark: a review of ecosystem processes during the Arctic polar night. Prog. Oceanogr. 139, 258–271. doi: 10.1016/j.pocean.2015.08.005
Berge, J., Johnsen, G., and Cohen, J. H. (2020). “Polar night marine ecology,” in Advances in Polar Ecology, Vol. 4, eds J. Berge, G. Johnsen, and J. H. Cohen (Cham: Springer).
Bischof, K., Hanelt, D., Aguilera, J., Karsten, U., Karsten, U., and Vögele, B. (2002). Seasonal variation in ecophysiological patterns in macroalgae from an Arctic fjord. I. Sensitivity of photosynthesis to ultraviolet radiation. Mar. Biol. 140, 1097–1106.
Black, W. P. (1950). Seasonal variation in weight and chemical com- position of the common British laminariaceae. J. Mar. Biol. Assoc. UK 29, 45–72.
Bonsell, C., and Dunton, K. H. (2018). Long-term patterns of benthic irradiance and kelp production in the central Beaufort sea reveal implications of warming for Arctic inner shelves. Progr. Oceanog. 162, 160–170. doi: 10.1016/j.pocean.2018.02.016
Brooks, P. R., and Crowe, T. P. (2018). Density and biotic interactions modify the combined effects of global and local stressors. Oikos 127, 1746–1758. doi: 10.1111/oik.04459
Chapman, A. R. O., and Craige, J. S. (1977). Seasonal growth in Laminaria longicruris: relations with dissolved inorganic nutrients and internal reserves of nitrogen. Mar. Biol. 40, 197–205. doi: 10.1007/bf00390875
Chapman, A. R. O., and Lindley, J. E. (1980). Seasonal growth of Laminaria longicruris in the high Arctic in relation to irradiance and dissolved nutrient concentration. Mar. Biol. 57, 197-205.
Coello-Camba, A., and Agustiì, S. (2017). Thermal thresholds of phytoplankton growth in polar waters and their consequences for a warming polar ocean. Front. Mar. Sci 4:168. doi: 10.3389/fmars.2017.00168
Cottier, F. R., Nilsen, F., Enall, M. E., Gerland, S., Tverberg, V., and Svendsen, H. (2007). Wintertime warming of an Arctic shelf in response to large-scale atmospheric circulation. Geophys. Res. Lett. 34. doi: 10.1029/2007GL029948
Davison, I. R. (1987). Adaptation of photosynthesis in Laminaria saccharina (Phaeophyta) to changes in growth temperature. J. Phycol. 23, 273–283. doi: 10.1111/j.1529-8817.1987.tb04135.x
Dubois, M., Gilles, K. A., Hamilton, J. K., Rebers, P. A., and Smith, F. (1956). Colorimetric method for determination of sugars and related substances. Anal. Chem. 28, 350–356. doi: 10.1021/ac60111a017
Gevaert, F., Creach, A., Davoult, D., Hol, A. C., Seuront, L., and Lemoine, Y. (2002). Photoinhibition and seasonal photosynthetic performance of the seaweed Laminaria saccharina during a simulated tidal cycle: chlorophyll fluorescence measurements and pigment analysis. Plant Cell Environ. 25, 859–872.
Gómez, I., Wulff, A., Roleda, M., Huovinen, P., Karsten, U., Quartino, M. L., et al. (2009). Light and temperature demands of marine benthic microalgae and seaweeds in polar regions. Bot. Mar. 52, 593–608.
Gordillo, F. J., Niell, F. X., and Figueroa, F. L. (2001). Non-photosynthetic enhancement of growth by high CO2 level in the nitrophilic seaweed Ulva rigida C. Agardh (Chlorophyta). Planta 213, 64–70. doi: 10.1007/s004250000468
Gordillo, F. J., Carmona, R., Viñegla, B., Wiencke, C., and Jiménez, C. (2016). Effects of simultaneous increase in temperature and ocean acidification on biochemical composition and photosynthetic performance of common macroalgae from Kongsfjorden (Svalbard). Polar Biol. 39, 1993–2007. doi: 10.1007/s00300-016-1897-y
Gordillo, F. J. L., and Aguilera, J.Jiménez, C. (2006). The response of nutrient assimilation and biochemical composition of Arctic seaweeds to a nutrient input in summer. J. Exp. Bot. 57, 2661–2671. doi: 10.1093/jxb/erl029
Graiff, A., Bartsch, I., Glaser, K., and Karsten, U. (2021). Seasonal photophysiological performance of adult western Baltic Fucus vesiculosus (Phaeophyceae) under ocean warming and acidification. Front. Mar. Sci. 8:666493. doi: 10.3389/fmars.2021.666493
Griffith, G. P., Hop, H., Vihtakari, M., Wold, A., Kalhagen, K., and Gabrielsen, G. W. (2018). Ecological resilience of Arctic marine food webs to climate change. Nat. Clim. Change 9, 868–872. doi: 10.1038/s41558-019-0601-y
Hays, G. C., Mazaris, A. D., Schofield, G., and Laloë, J. O. (2017). Population viability at extreme sex-ratio skews produced by temperature- dependent sex determination. Proc. R. Soc. B 284:20162576. doi: 10.1098/rspb.2016.2576
Hellebust, J. A., and Lewin, J. (1977). “Heterotrophic nutrition,” in The Biology of Diatoms, ed. D. Werner (Berkeley, CA: University of California Press), 169–197.
Hop, H., Wiencke, C., Vögele, B., and Kovaltchouk, N. A. (2012). Species composition, zonation, and biomass of marine benthic macroalgae in Kongsfjorden, Svalbard. Bot. Mar. 55, 399–414. doi: 10.1515/bot-2012-0097
Hop, H., Cottier, F., and Berge, J. (2019). “Autonomous marine observatories in Kongsfjorden, Svalbard,” in Advances in Polar Ecology: Ecosystem Kongsfjorden, Svalbard, eds H. Hop and C. Wiencke (Berlin: Springer International Publishing), 515–533. doi: 10.1007/978-3-319-46425-1_13
Husa, V., Steen, H., and Sjøtun, K. (2014). Historical changes in macroalgal communities in Hardangerfjord (Norway). Mar. Biol. Res. 10, 226–240. doi: 10.1080/17451000.2013.810751
Iñiguez, C., Carmona, R., Lorenzo, M. R., Niell, F. X., Wiencke, C., and Gordillo, F. J. (2015). Increased CO2 modifies the carbon balance and the photosynthetic yield of two common Arctic brown seaweeds: desmarestia aculeata and Alaria esculenta. Polar Biol. 39, 1979–1991. doi: 10.1007/s00300-015-1724-x
Iñiguez, C., Carmona, R., Lorenzo, M. R., Niell, F. X., Wiencke, C., and Gordillo, F. J. (2016). Increased temperature, rather than elevated CO2, modulates the carbon assimilation of the Arctic kelps Saccharina latissima and Laminaria solidungula. Mar. Biol. 163, 248–265. doi: 10.1007/s00227-016-3024-6
IPCC (2014). “Climate change 2014: synthesis report,” in Contribution of Working Groups I, II and III to the 5th Assessment Report of the Intergovernmental Panel on Climate Change, eds R. K. Pachauri and L. A. Meyer (Geneva: IPCC), 151.
Izquierdo, J., Peìrez-Ruzafa, I. M., and Gallardo, T. (2002). Effect of temperature and photon fluence rate on gametophytes and young sporophytes of Laminaria ochroleuca Pylaie. Helgoland Mar. Res. 55, 285–292.
Jassby, A. D., and Platt, T. (1976). Mathematical formulation of the relationship between photosynthesis and light for phytoplankton. Limnol. Oceanogr. 21, 540–547. doi: 10.4319/lo.1976.21.4.0540
Johnston, A. M., and Raven, J. A. (1986). Dark carbon fixation studies on the intertidal macroalga Ascophyllum nodosum (Phaeophyta). J. Phycol. 2, 78–83. doi: 10.1111/j.1529-8817.1986.tb02518.x
Kain, J. M. (1989). The seasons in the subtidal. Br. Phycol. J. 24, 203–215. doi: 10.1080/00071618900650221
Karsten, U., Schaub, I., Woelfel, J., Sevilgen, D. S., Schlie, C., Becker, B., et al. (2019). “Living on cold substrata: new insights and approaches in the study of microphytobenthos ecophysiology and ecology in Kongsfjorden,” in The Ecosystem of Kongsfjorden, Svalbard, eds H. Hop and C. Wiencke (Berlin: Springer International Publishing), 303–338. doi: 10.1007/978-3-319-46425-1_8
Korb, R. E., and Gerard, V. A. (2000). Effects of concurrent low temperature and low nitrogen supply on polar and temperate seaweeds. Mar. Ecol. Prog. Ser. 198, 73–82. doi: 10.3354/meps198073
Kortsch, S., Primicerio, R., Beuchel, F., Renaud, P. E., Rodrigue, J., Lønne, O. J., et al. (2012). Climate-driven regime shifts in Arctic marine benthos. Proc. Natl. Acad. Sci. U. S. A 109, 14052–14057. doi: 10.1073/pnas.1207509109
Krausse-Jensen, D., and Duarte, C. (2016). Substantial role of macroalgae in marine carbon sequestration. Nat. Geosci. 9, 737–742. doi: 10.1038/ngeo2790
Krause-Jensen, D., Archambault, P., Assis, J., Bartsch, I., Bischof, K., Filbee-Dexter, K., et al. (2020). Imprint of climate change on pan-Arctic marine vegetation. Front. Mar. Sci. 7:617324. doi: 10.3389/fmars.2020.617324
Kremer, B. P. (1981). Metabolic implications of non-photosynthetic carbon fixation in brown macroalgae. Phycologia 20, 242–250.
Krüger, M. (2016). Photosynthese-Lichtkurven ausgewählter Makroalgenarten des Kongsfjords (Spitzbergen, Norwegen) als Grundlage für Abschätzungen der Produktivität des arktischen Kelpwaldes. Ph. D. Thesis. Germany: Technical University of Freiberg.
Küppers, U., and Kremer, B. P. (1978). Longitudinal profiles of carbon dioxide fixation capacities in marine macroalgae. Plant Physiol. 62, 49–53. doi: 10.1104/pp.62.1.49
Kvernvik, A. C., Hoppe, C. J. M., Lawrenz, E., Prasi, O., Greenacre, M., Wiktor, J. M., et al. (2018). Fast reactivation of photosynthesis in arctic phytoplankton during the polar night. J. Phycol. 54, 461–470. doi: 10.1111/jpy.12750
Lacour, T., Morin, P.-I., Sciandra, T., Onaher, N., Campbell, D. A., Ferland, J., et al. (2019). Decoupling light harvesting, electron transport and carbon fixation during prolonged darkness supports rapid recovery upon re-illumination in the Arctic diatom Chaetoceros neogracilis. Polar Biol. 42, 1787–1799. doi: 10.1007/s00300-019-02507-2
Latala, A. (1990). Photosynthesis and respiration of some marine benthic algae from Spitsbergen. Polar Res. 8, 303–307. doi: 10.3402/polar.v8i2.6822
Lathlean, J., McWilliam, R. A., Pankhurst, J., and Minchinton, T. E. (2017). Altering species interactions outweighs the effects of experimental warming in structuring rocky shore community. J. Exp. Mar. Biol. Ecol. 496, 22–28.
Lee, J. A., and Brinkhuis, B. H. (1988). Seasonal light and temperature interaction effects on development of Laminaria saccharina (Phaeophyta) gametophytes and juvenile sporophytes. J. Phycol. 24, 181–191.
Liesner, D., Shama, L. N. S., Diehl, N., Valentin, K., and Bartsch, I. (2020). Thermal plasticity of the kelp Laminaria digitata (Phaeophyceae) across life cycle stages reveals the importance of cold seasons for marine forests. Front. Mar. Sci. 7:456. doi: 10.3389/fmars.2020.00456
Lüder, U. H., Wiencke, C., and Knoetzel, J. (2002). Acclimation of photosynthesis and pigments during and after six months of darkness in Palmaria decipiens (Rhodophyta): a study to simulate Antarctic winter sea ice cover. J. Phycol. 38, 904–913. doi: 10.1046/j.1529-8817.2002.t01-1-01071.x
Lüning, K., and Schmitz, K. (1988). Dark growth of the red alga Delesseria sanguinea (Ceramiales): lack of chlorophyll, photosynthetic capability and phycobilisomes. Phycologia 27, 72–77.
Lüning, K. (1990). Seaweeds: their Environment, Biogeography and Ecophysiology. New York, NY: John Wiley and Sons.
Mann, K. H. (1973). Seaweeds: their productivity and strategy for growth. Science 182, 975–981. doi: 10.1126/science.182.4116.975
Maturilli, M., Hanssen-Bauer, I., Neuber, R., Rex, M., and Edvarsen, K. (2019). “The atmosphere above Ny-Ålesund: Climate and global warming, ozone and surface UV radiation,” in The Ecosystem of Kongsfjorden, Svalbard, eds H. Hop and C. Wiencke (Berlin: Springer International Publishing), 23–46.
McMinn, A., and Martin, A. (2013). Dark survival in a warming world. Proc. R. Soc. B 280:20122909. doi: 10.1098/rspb.2012.2909
McQuoid, M. R., and Hobson, L. A. (1996). Diatom resting stages. J. Phycol. 32, 889–902. doi: 10.1111/j.0022-3646.1996.00889.x
Monteiro, C., Heinrich, S., Bartsch, I., Valentin, K., Corre, E., Colleìn, J., et al. (2019). Temperature modulates sex-biased gene expression in the gametophytes of the kelp Saccharina latissima. Front. Mar. Sci 6:769. doi: 10.3389/fmars.2019.007697487
Nabivailo, Y. V., and Titlyanov, E. A. (2006). Competitive relationships in natural and artificial algal communities. Russ. J. Mar. Biol. 32, 21–31.
Nelson, W. (2005). Life history and growth in culture of the endemic New Zealand kelp Lessonia variegata J. Agardh in response to differing regimes of temperature, photoperiod and light. J. Appl. Phycol 17, 23–28.
Nielsen, M. M., Krause-Jensen, D., Olesen, B., Thinggaard, R., Christensen, P. B., and Bruhn, A. (2014). Growth dynamics of Saccharina latissima (Laminariales, Phaeophyceae) in Aarhus Bay, Denmark, and along the species’ distribution range. Mar. Biol. 161, 2011–2022. doi: 10.1007/s00227-014-2482-y
Olischläger, M., Iñigu, C., Gordillo, F. J. L., and Wiencke, C. (2014). Biochemical composition of temperate and Arctic populations of Saccharina latissima after exposure to increased pCO2 and temperature reveals ecotypic variation. Planta 240, 1213–1224. doi: 10.1007/s00425-014-2143-x
Oppliger, L. V., Correa, J. A., Faugeron, S., Beltraìn, J., Tellier, F., Valero, M., et al. (2011). Sex ratio variation in the Lessonia nigrescens complex (Laminariales, Phaeophyceae): effect of latitude, temperature and marginality. J. Phycol. 47, 5–12. doi: 10.1111/j.1529-8817.2010.00930.x
Ospina-Alvarez, N., and Piferrer, F. (2008). Temperature-dependent sex determination in fish revisited: prevalence, a single sex ratio response pattern, and possible effects of climate change. PLoS One 3:e2837. doi: 10.1371/journal.pone.0002837
Palmisano, A. C., and Sullivan, C. W. (1983). Physiology of sea ice diatoms. II. Dark survival of three polar diatoms. Can. J. Microbiol. 29, 157–160. doi: 10.1139/m83-026
Parages, M., Heinrich, S., Wiencke, C., and Jiménez, C. (2013). Rapid phosphorylation of MAP kinase-like proteins in two species of Arctic kelps in response to temperature and UV radiation stress. Environ. Exp. Bot. 91, 30–37. doi: 10.1016/j.envexpbot.2013.02.005
Pavlov, A., Tverberg, V., Ivanov, B., Nilsen, F. Falk-Petersen, S., and Granskog, M. (2013). Warming of Atlantic water in two West Spitsbergen fjords over the last century (1912–2009). Polar Res. 32:11206. doi: 10.3402/polar.v32i0.11206
Peters, E., and Thomas, D. N. (1996). Prolonged darkness and diatom mortality I: marine Antarctic species. J. Exp. Mar. Biol. Ecol. 207, 25–41. doi: 10.1016/s0022-0981(96)02520-8
Piquet, A. M. T., van de Poll, W. H., Visser, R. J. W., Wiencke, C., Bolhuis, H., and Buma, A. G. J. (2014). Springtime phytoplankton dynamics in Arctic Krossfjorden and Kongsfjorden (Spitsbergen) as a function of glacier proximity. Biogeoscience 11, 2263–2279. doi: 10.5194/bg-11-2263-2014
Provasoli, L. (1968). “Media and prospects for the cultivation of marine algae,” in Cultures and Collections of Algae, in Proceedings of the U.S.-Japan Conference, Hakone September 1966, eds A. Watanabe and A. Hattori (Tokyo: Japan Society of Plant Physiology), 63–75.
Reeves, S., McMinn, A., and Martin, A. (2011). The effect of prolonged darkness on the growth, recovery and survival of Antarctic sea ice diatoms. Polar Biol. 34, 1019–1032. doi: 10.1111/nph.15843
Schaub, I., Wagner, H., Graeve, M., and Karsten, U. (2017). Effects of prolonged darkness and temperature on the lipid metabolism in the benthic diatom Navicula perminuta from the Arctic Adventfjorden, Svalbard. Polar Biol. 40, 1425–1439. doi: 10.1007/s00300-016-2067-y
Scheschonk, L., Becker, S., Hehemann, J. H., Diehl, N., Karsten, U., and Bischof, K. (2019). Arctic kelp eco-physiology during the polar night in the face of global warming: a crucial role for laminarin. Mar. Ecol. Prog. Ser. 611, 59–74. doi: 10.3354/meps12860
Schlie, C., Woelfel, J., Rüdiger, F., Schumann, R., and Karsten, U. (2011). “Ecophysiological performance of benthic diatoms from arctic waters,” in The Diatom World. Cellular Origin, Life in Extreme Habitats and Astrobiology, Vol. 19, eds J. Seckbach and P. Kociolek (Berlin: Springer), 425–436.
Schoschina, E. V. (1996). Seasonal and age dynamics of growth and reproduction of Phycodrys rubens (Rhodophyta) in the Barents and White Seas. Aquat. Bot. 5, 13–30. doi: 10.1016/0304-3770(96)01055-8
Shuntanova, N., Nikishina, D., Ivanov, M., Berge, J., Renaud, P. E., Ivanova, T., et al. (2018). The longer the better: the effect of substrate on sessile biota in Arctic kelp forest. Polar Biol. 41:993. doi: 10.1007/s00300-018-2263-z
Smith, P. K., Krohn, R. I., Hermanson, T. I., Mallia, A. K., Gartner, F. H., Provenzano, M. D., et al. (1985). Measurement of protein using bicinchoninic acid. Analyt. Biochem. 150, 76–85.
van De Poll, W. H., Maat, D. S., Fischer, P., Rozema, P. D., Daly, O. B., Koppelle, S., et al. (2016). Atlantic advection driven changes in glacial melt- water: effects on phytoplankton chlorophyll a and taxonomic composition in Kongsfjorden, Spitsbergen. Front. Mar. Sci. 3:200. doi: 10.3389/fmars.2016.00200
Veuger, B., and van Oevelen, D. (2011). Long-term pigment dynamics and diatom survival in dark sediment. Limnol. Oceanogr. 56, 1065–1074. doi: 10.4319/lo.2011.56.3.1065
Wellburn, A. R. (1994). The spectral determination of chlorophylls a and b, as well as total carotenoids, using various solvents with spectrophotometers of different resolution. J. Plant Physiol. 144, 307–313. doi: 10.1016/S0176-1617(11)81192-2
Weykam, G., Thomas, D. N., and Wiencke, C. (1997). Growth and photosynthesis of the Antarctic red algae Palmaria decipiens (Palmariales) and Iridaea cordata (Gigartinales) during and following extended periods of darkness. Phycologia 36, 395–405.
Wulff, A., Roleda, M. Y., Zacher, K., and Wiencke, C. (2008). Exposure to sudden light burst after prolonged darkness—a case study on benthic diatoms in Antarctica. Diatom Res. 23, 519–532. doi: 10.1080/0269249x.2008.9705774
Keywords: biochemical composition, darkness survival, global warming, kelp, photosynthetic performance, polar night, respiration, seasonality
Citation: Gordillo FJL, Carmona R and Jiménez C (2022) A Warmer Arctic Compromises Winter Survival of Habitat-Forming Seaweeds. Front. Mar. Sci. 8:750209. doi: 10.3389/fmars.2021.750209
Received: 30 July 2021; Accepted: 14 December 2021;
Published: 13 January 2022.
Edited by:
Satya Panigrahi, Indira Gandhi Centre for Atomic Research (IGCAR), IndiaReviewed by:
Simon Morley, British Antarctic Survey (BAS), United KingdomLadd Johnson, Laval University, Canada
Copyright © 2022 Gordillo, Carmona and Jiménez. This is an open-access article distributed under the terms of the Creative Commons Attribution License (CC BY). The use, distribution or reproduction in other forums is permitted, provided the original author(s) and the copyright owner(s) are credited and that the original publication in this journal is cited, in accordance with accepted academic practice. No use, distribution or reproduction is permitted which does not comply with these terms.
*Correspondence: Francisco J. L. Gordillo, Z29yZGlsbG9AdW1hLmVz