- 1Department of Marine Biotechnology, National Kaohsiung University of Science and Technology, Kaohsiung, Taiwan
- 2The iEGG and Animal Biotechnology Center, National Chung Hsing University, Taichung, Taiwan
- 3Department of Life Sciences, National Chung Hsing University, Taichung, Taiwan
- 4Planning and Information Division, Fisheries Research Institute, Keelung, Taiwan
The dynamic regulation of ions and amino acids in the gills and mantle of the Asian hard clam, Meretrix lusoria, following the exposure to a hyperosmotic environment was hitherto unclear. The present study revealed that the osmolality as well as the Na+ and Cl– concentrations in the hemolymph were significantly increased 3 h after transferring the clams from an environment with the salinity of their natural habitat (brackish water; BW; 20‰) to one with hyperosmotic salinity (seawater; 35‰). In addition, we found that the specific activities of Na+/K+-ATPase, a key enzyme that plays a significant role in cell osmoregulation, in the gills and mantle of clams were significantly increased at 72 and 12 h post-transfer, respectively, during acclimation to hyperosmotic salinity. Similarly, the contents of free amino acids (FAAs) such as taurine, alanine, and glycine were significantly elevated during hyperosmotic salinity acclimation. Previous research indicates that taurine is the most abundant FAA in the gills and mantles of Asian hard clams and that the taurine transporter (TAUT) plays an important role in taurine accumulation. The present study showed that TAUT mRNA and protein expression were significantly and transiently increased in the mantle of Asian hard clams following exposure to seawater; although the expression of TAUT mRNA in the gills of Asian hard clams was also transiently stimulated by exposure to hyperosmotic salinity, the relative TAUT protein abundance decreased only at later stages. Accordingly, the findings of this study improve our understanding of the dynamic processes of ion and amino acid regulation in the peripheral tissues of bivalves under hyperosmotic stress.
Introduction
The Asian hard clam (Meretrix lusoria) is an important aquaculture species in Taiwan. This species naturally inhabits intertidal and estuarine areas, where the salinity is approximately 20‰ (the average of a tidal cycle) (Chen, 1976, 1990); however, this value varies immensely. In these areas, the salinity is very close to that of fresh water when there is abundant rain, but increases to high saline conditions when inundated by tides (Barnes, 1999). Since Asian hard clams are unable to move to different locations because of their poor locomotion abilities, they are frequently exposed to variable salinity challenges. This species does not possess an advanced systemic osmoregulatory mechanism, and thus the osmolality of its hemolymph conforms to the external salinity (Lin et al., 2016). As such, when the Asian hard clam is exposed to hyper or hypoosmotic salinity, its tissues are immediately exposed to extreme osmotic stress.
The mechanisms for maintaining cell volume are an important issue for cells facing osmotic challenges (Lang et al., 1998; Burg et al., 2007). Most cells respond to osmotic stress by triggering specific metabolic or membrane-transport processes that recover cell volume to a normal state (McManus et al., 1995). The movement of water across the cell membrane is constrained by the modulation of the content of inorganic and organic osmolytes in cells (McManus et al., 1995). The Na+/K+-ATPase (NKA) is a vital active transporter that transports Na+ and K+ into cells; its action provides a driving force for the secondary transport of other inorganic ions and modulates cellular osmolality (Morth et al., 2011; Toyoshima et al., 2011). Relatively abundant NKA activity has been detected in the gills and mantle of bivalves (Saintsing and Towle, 1978; Borgatti et al., 2003). The NKA activity in the mantle of the clam Rangia cuneata is induced under hyperosmotic conditions (Saintsing and Towle, 1978). Similarly, Borgatti et al. (2003) reported that the mantle NKA activity of Mediterranean mussels (Mytilus galloprovincialis) was significantly lower in 24‰ seawater (SW) than in 33‰ SW; however, the gill NKA activity did not differ. Notably, there was no difference between the mantle and gill NKA activity of ark shells (Scapharca inaequivalvis) under the same treatments (Borgatti et al., 2003). Our previous study indicated that Asian hard clams exposed to 10 and 20‰ brackish water (BW) and 35‰ SW had the same gill NKA activity. However, the mantle NKA activity of those exposed to SW was significantly upregulated compared to those exposed to 20‰ BW, while no differences were found between those exposed to 20‰ BW and 10‰ BW (Lin et al., 2016). Although mantle NKA activity of Asian hard clams did not change upon exposure to 10‰ BW, we found that it was temporarily increased after the individuals were transferred to this environment (Lin et al., 2021).
Although the modulation of inorganic ion content aids cellular osmoregulation, long-term changes in this content are deleterious (Yancey, 1994). Organic osmolytes (e.g., polyols, free amino acids (FAAs), and methylamines groups) are found in the cytosol of all animals and are compatible solutes to cells (Garcia-Perez and Burg, 1991; Yancey, 1994). As such, the accumulation of high concentrations of organic osmolytes does not have a harmful effect on cells (Yancey, 1994). FAAs are vital intracellular organic osmolytes in osmoconformer bivalves (Pourmozaffar et al., 2019). Among these FAAs, taurine is involved in many cell physiological functions, including volume regulation, maintaining the structural integrity of cell membranes, and stress responses (Huxtable et al., 2000; Lourenco and Camilo, 2002; Pourmozaffar et al., 2019). In many bivalve species, taurine is an abundant and important organic osmolyte that maintains intracellular osmolality in tissues (Lange, 1963; Gilles, 1972; Livingstone et al., 1979; Zurburg and DeZwaan, 1981; Deaton et al., 1985; Hosoi et al., 2003; Sokolowski et al., 2003; Toyohara and Hosoi, 2004; Kube et al., 2007). Many bivalves are adapted to hyperosmotic stress mainly through the accumulation of taurine in tissues (Pourmozaffar et al., 2019). Our previous study found that taurine is the predominant FAA in Asian hard clams (Lin et al., 2016). When Asian hard clams are transferred from 20‰ BW to 10‰ BW, the amino acid content (e.g., taurine, glutamate, glycine, and/or alanine) in their gills and mantle decreases (Lin et al., 2021). Moreover, the reduction of the taurine content in tissues is greater than that of the other amino acids (Lin et al., 2021). This indicates that the taurine content plays an important role in the osmoregulation of this species tissues after exposure to a low-salinity environment (Lin et al., 2021). Nevertheless, the change in taurine content in the gills and mantle of Asian hard clams after exposure to a high-salinity environment remains unclear.
The taurine transporter (TAUT) plays an important role in the intracellular accumulation of taurine (Lambert et al., 2015). For instance, the accumulation of taurine in cells is defective in TAUT knockout mice (Lotsch et al., 2014). The TAUT is Na+- and Cl–-dependent and its activity is acutely activated after exposure to hyperosmotic stress in a mammalian cell model (Hansen et al., 2012; Lambert et al., 2015). A functional analysis of the TAUT from giant Pacific oysters (Crassostrea gigas) and tilapia (Oreochromis mossambicus) in Xenopus laevis oocytes demonstrated that oyster and tilapia TAUT was related to taurine accumulation in cells and that its activity depended on the NaCl concentration (Hosoi et al., 2007). The function and properties of TAUT are conserved between bivalves and vertebrates. The TAUT gene and protein expression is stimulated after exposure to hyperosmotic mediums in both mammals and teleosts (Takeuchi et al., 2000, 2001; Shioda et al., 2002; Han et al., 2006; Lambert et al., 2015). The expression of TAUT mRNA in the gills and mantles of two marine bivalves, the giant Pacific oyster and Mediterranean mussel, is stimulated transiently after individuals are transferred from 30‰ SW to 15 and 60‰ SW, respectively (Hosoi et al., 2005; Hosoi et al., 2007). The short-term response of TAUT mRNA and protein expression in the gills and mantle of Asian hard clams to exposure to high-salinity SW is unknown. However, our previous study on this species revealed that gill TAUT mRNA expression was upregulated, whereas mantle TAUT mRNA expression was downregulated, and TAUT protein expression in the gills and mantle increased after the individuals were transferred from 20 to 10‰ BW (Lin et al., 2021). Accordingly, the transcription and/or translation of TAUT in bivalve tissues is regulated in response to salinity stress.
As an osmoconformer, the Asian hard clam conforms the osmolality of its body fluids to that of their external environment. We have previously compared the regulation of ions and amino acids in the mantle and gills of Asian hard clams in response to long-term exposure to 20‰ BW (the salinity of their natural habitat) and 35‰ SW (a hyperosmotic medium) (Lin et al., 2016). The short-term effects of hyperosmotic stress on the regulation of ions and amino acids in these tissues are yet to be determined. Moreover, no study has explored the regulation of ions and amino acids in the tissues of osmoconformer bivalves simultaneously after individuals are transferred to environments with hyperosmotic salinity. To further clarify the responses of Asian hard clams exposed to hyperosmotic stress, the present study explored the time-course changes in the regulation of ions and amino acids after individuals are transferred from 20‰ BW to 35‰ SW. For this, the time-course changes in osmolality and ionic levels of the hemolymph, as well as the content of taurine and other amino acids in the gills and mantle of Asian hard clams were measured. Additionally, the NKA activity and the TAUT mRNA and protein expression in the gills and mantle were analyzed. Considering our results, the present study can increase the understanding of the regulation of ions and amino acids in Asian hard clams transferred to a hyperosmotic environment. In addition, it provides useful information to enhance our knowledge of osmoregulation in osmoconformer bivalve tissues.
Materials and Methods
Study Animals and Experiments
The adult Asian clams used in this study were obtained from a local aquaculture farm with 20‰ BW. The clams had a body length of 30–50 mm and a weight of 16.5–18.5 g and were reared in 20‰ BW (pH 7.96 ± 0.02; Ammonia 0.011 ± 0.002 mg/L) for 15 days with a circulating system at 25°C under a 12:12 h light:dark photoperiod. During the acclimation, the clams were fed with commercial food (Shye Yih Feeding Co., Ltd., Kaohsiung, Taiwan) and 50% water was replaced once daily. The transferred hard clams were also hold under the same temperature, circulating system, photoperiod, and feed condition. The SW used in this study was prepared from aerated local tap water, to which appropriate amounts of Instant Ocean synthetic sea salt were added (Aquarium Systems, Blacksburg, VA, United States). For the salinity-transfer experiment, we followed the experimental design of Lin et al. (2021). The clams were transferred from 20‰ BW to 35‰ SW (pH 8.03 ± 0.01; Ammonia 0.014 ± 0.003 mg/L) and then sampled for further analyses at different time points over a period of 15 d (360 h). The Asian hard clams in the control group were transferred from 20 to 20‰ BW and sampled at 0, 24, and 360 h. These sampled time points showed acute (24 h) and chronic (360 h) responses in the Asian hard clams after transfer in the control group. All animals were randomly collected for sampling at different time points.
Hemolymph Analyses
We followed the methods of Lin et al. (2016) for sampling and analyzing the hemolymph of Asian hard clams. The hemolymph was collected from the heart or pericardial cavity of clam using a 23-G syringe and centrifuged at 14,000× g for 10 min at 4°C, and the resulting supernatant was used to measure the osmolality and Na+ and Cl– concentrations. Plasma osmolality was assessed using a WESCOR 5520 VAPRO osmometer (ELI Tech Group, South Logan, UT, United States). Na+ concentrations were measured using a Hitachi Z-8000 polarized Zeeman atomic absorption spectrophotometer (Tokyo, Japan), and Cl– concentrations were evaluated using the ferricyanide method. Photometric analysis was conducted using a Hitachi U-2001 spectrophotometer (Tokyo, Japan). The N-value for each time point was 8.
Content of Free Amino Acids in the Gills and Mantle
The content of FAAs in the gills and mantle of Asian hard clams was analyzed using the method described by Lin et al. (2016). The gills and mantles of clams were sampled and then freeze-dried (Osterode am Harz 3360; Germany) for 10 h. Dried samples were mixed and incubated with 1 mL 2% sulfosalicylic acid (Sigma, St. Louis, MO, United States) at 4°C for 48 h. The mixture was then centrifuged at 30,000× g at 4°C for 30 min. Thereafter, the supernatant was passed through a 0.22-μm filter (Millipore, Bedford, MA, United States). The filtrate was used to determine the FAA content using a Beckman 6,300 High Performance Amino Acid Analyzer (Palo Alto, CA, United States). The N-value for each time point was 5.
Specific Activity of Na+/K+-ATPase
The NKA activity in the gills and mantles of Asian hard clams was assayed according to the method described by Lin et al. (2016). The gills and mantles were first sampled and homogenized. The tissue homogenates were then suspended in a mixture of homogenization medium (100 mM imidazole-HCl, 5 mM Na2EDTA, 200 mM sucrose, and 0.1% sodium deoxycholate, pH 7.6) and proteinase inhibitor (10 mg antipain, 5 mg leupeptin, and 50 mg benzamidine dissolved in 5 mL aprotinin) (vol/vol: 100 L–1). Homogenization was performed using a motorized Teflon pestle at 600 rpm for 30 s. The homogenate was then centrifuged at 13,000× g at 4°C for 20 min, and the resulting supernatant was used to determine protein concentrations and enzyme activities. Protein concentrations were determined using a protein assay kit according to the manufacturer’s instructions (Bio-Rad, Hercules, CA, United States), using bovine serum albumin (Sigma) as a standard. The NKA activity was assayed by adding the supernatant to a reaction mixture (100 mM imidazole-HCl buffer, 125 mM NaCl, 75 mM KCl, 7.5 mM MgCl2, and 5 mM Na2ATP, pH 7.6). The reaction was run at 37°C for 30 min, after which 200 μL of ice-cold 30% trichloroacetic acid were added. The concentration of inorganic phosphate was determined according to the method described by Peterson (1978). The enzyme activity of NKA was defined as the difference between the inorganic phosphate liberated in the presence and absence of ouabain (3.75 mM) in the reaction mixture. Each sample was assayed in triplicate. The N-value for each time point was 5.
RNA Extraction and First-Strand Complementary DNA Synthesis
According to the manufacturer’s instructions, RNA-BeezTM (Tel-Test, Friendwood, TX, United States) was used to isolate total RNA from the gills and mantles of Asian hard clams. Genomic DNA was then eliminated from the isolated total RNA using the RNAspin Mini RNA Isolation Kit (GE Health Care, Piscataway, NJ, United States), according to the manufacturer’s instructions. Finally, the isolated RNA was dissolved in DEPC H2O. To verify RNA integrity, a 0.8% agarose gel electrophoresis was performed, and the extracted RNA samples were stored at −80°C. The first-strand cDNA was synthesized by reverse transcribing 5 μg of the total RNA using 1 μL of Oligo (dT) (0.5 μg μL–1) primer and 1 μL of PowerScript Reverse Transcriptase (Clontech, Mountain View, United States) according to the manufacturer’s instructions.
Quantitative Real-Time PCR
To quantify TAUT mRNA expression, a Mini Opticon real-time PCR system (Bio-Rad) was used to perform qPCR in a final reaction volume of 20 μL, containing 8 μL of cDNA (1,000×), 2 μL of either a 5-μM gene-specific primer mixture or a 5-μM β-actin primer mixture, and 10 μL of 2 × SYBR Green PCR Master Mix (Bio-Rad). The primer sequences used for TAUT gene amplification have been described by Lin et al. (2016). The mRNA values were normalized to the expression of β-actin mRNA from the same cDNA samples. The expression of β-actin mRNA was stable in the gills and mantle of Asian hard clams acclimated to different salinities. Therefore, it was used as the internal control. The N-value for each time point was 5.
Immunoblotting of the Taurine Transporter
Protein homogenates were prepared according to the method described by Lin et al. (2016). Protein homogenates (100 μg) of gills and mantles were heated at 100°C for 5 min and fractionated by electrophoresis on SDS-containing 10% polyacrylamide gels. Separated proteins were transferred from unstained gels to polyvinylidene fluoride membranes (Millipore). Blots were preincubated for 2 h in phosphate-buffered saline/Tween 20 buffer [PBST: 137 mM NaCl, 3 mM KCl, 10 mM Na2HPO4, and 2 mM KH2PO4, 0.2% (vol/vol) Tween 20, pH 7.4] containing 5% (wt/vol) non-fat dried milk to minimize non-specific binding, followed by overnight incubation at 4°C with a clam TAUT antibody diluted in PBST (1:1,500). The specificity of the TAUT antibody was confirmed in a previous study (Lin et al., 2016). After incubation with the TAUT antibody, the membranes were incubated with a goat anti-rabbit Ig GAP-conjugated secondary antibody (Chemicon, CA, United States) (1:2,500 dilution) for 2 h. The blots were developed using an NBT/BCIP kit (Sigma), and subsequent quantification of the immunoblots was performed using the method described by Lin et al. (2016). The immunoblots were photographed and imported as TIFF files into the ID image analysis software package (MCID Analysis Evaluation 7.0). To compare the relative intensities of the immunoreactive bands, the results were converted to numerical values. The N-value for each time point was 5.
Statistical Analysis
Comparisons between different time points were analyzed by Tukey’s pairwise test following one-way analysis of variance (ANOVA), the comparisons between various time points and 0 h were analyzed by one-way ANOVA and Dunnett’s test, and the level of statistical significance was set at 0.05. Different letters indicate significant differences between the different time points. The values in tables and figures are expressed as the mean ± SEM (standard error of the mean).
Results
Effects of High Salinity on the Osmolality and Ion Concentrations in Hemolymph
Following the transfer from 20‰ BW to 35‰ SW, we detected significant increase in the osmolality and ion concentrations in the hemolymph of Asian hard clams (Figure 1). After 3 h, there was a significant initial increase in hemolymph osmolality, which reached a stable level at 12 h post-transfer (Figure 1A). Similarly, the hemolymph concentrations of both Na+ (Figure 1B) and Cl– (Figure 1C) increased significantly within 3 h post-transfer (Figures 1B,C), reaching a stable level at 24 and 6 h post-transfer, respectively (Figures 1B,C). In the control group, we did not detect any changes in hemolymph osmolality or ion concentration during the experimental period (Figure 1).
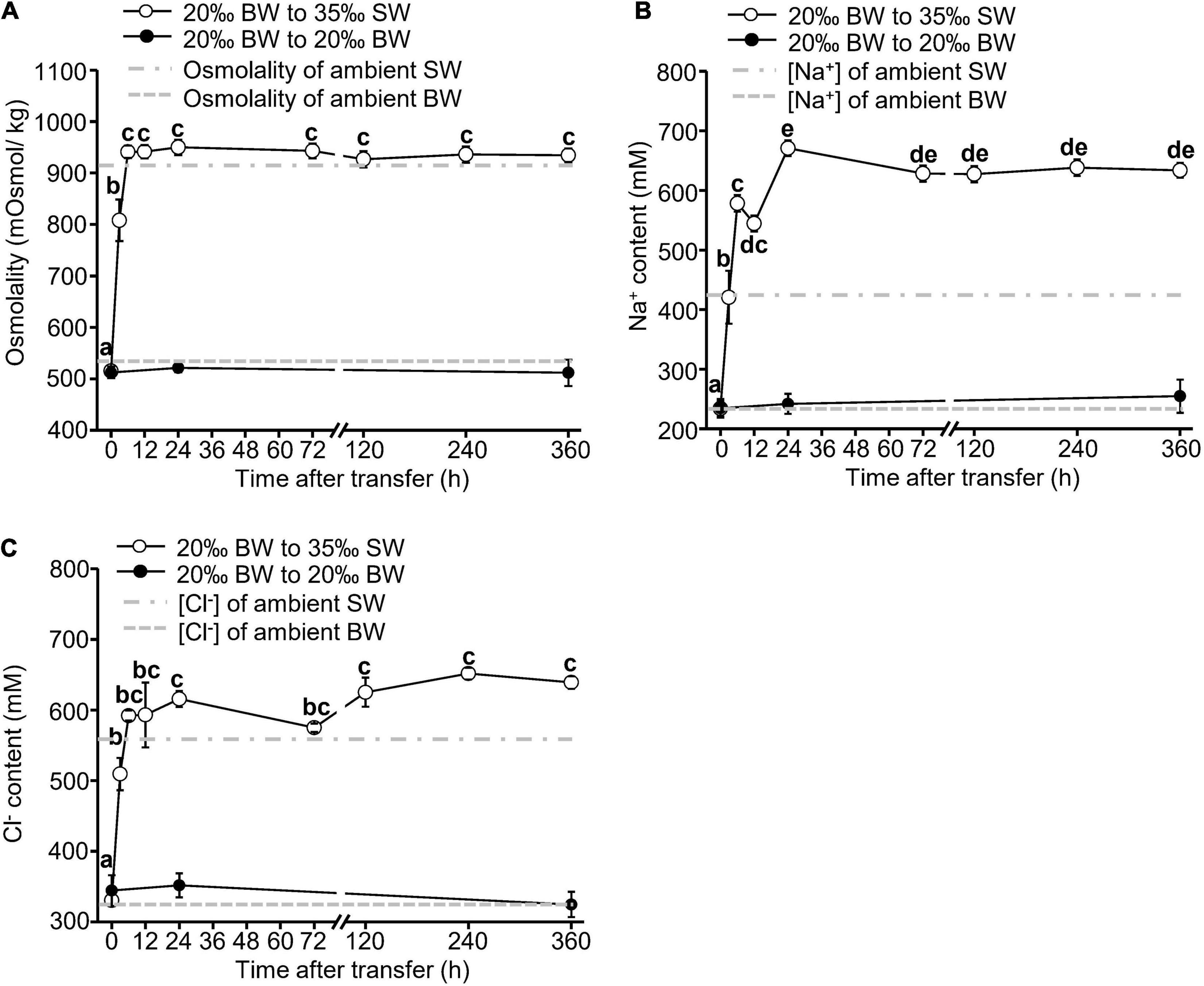
Figure 1. Changes in osmolality (A), sodium (B), and chloride (C) concentrations of the hemolymph of Asian hard clams transferred directly from 20‰ brackish water (BW) to 35‰ seawater (SW). Different letters indicate significant differences between different time points by the Tukey’s pairwise test following the one-way analysis of variance (ANOVA) (p < 0.05). No significant difference was found among time-points in the control group (from 20 to 20% BW). Values are the means ± SEM (N = 8).
Effects of High Salinity on Na+/K+-ATPase Activity in the Gills and Mantle
The NKA activity in the gills of Asian hard clams was significantly increased at 72 h (3 days) and 120 h (5 days) after the transfer from BW to SW (Figure 2A). Thereafter, the NKA activity decreased to its original level. In contrast, the mantle NKA activity was significantly upregulated at 12 h post-transfer but decreased to its original level after 120 h (5 days) (Figure 2B). In the control group, no significant difference was found between the NKA activity of the gills and mantle at different time points after transfer (Figure 2).
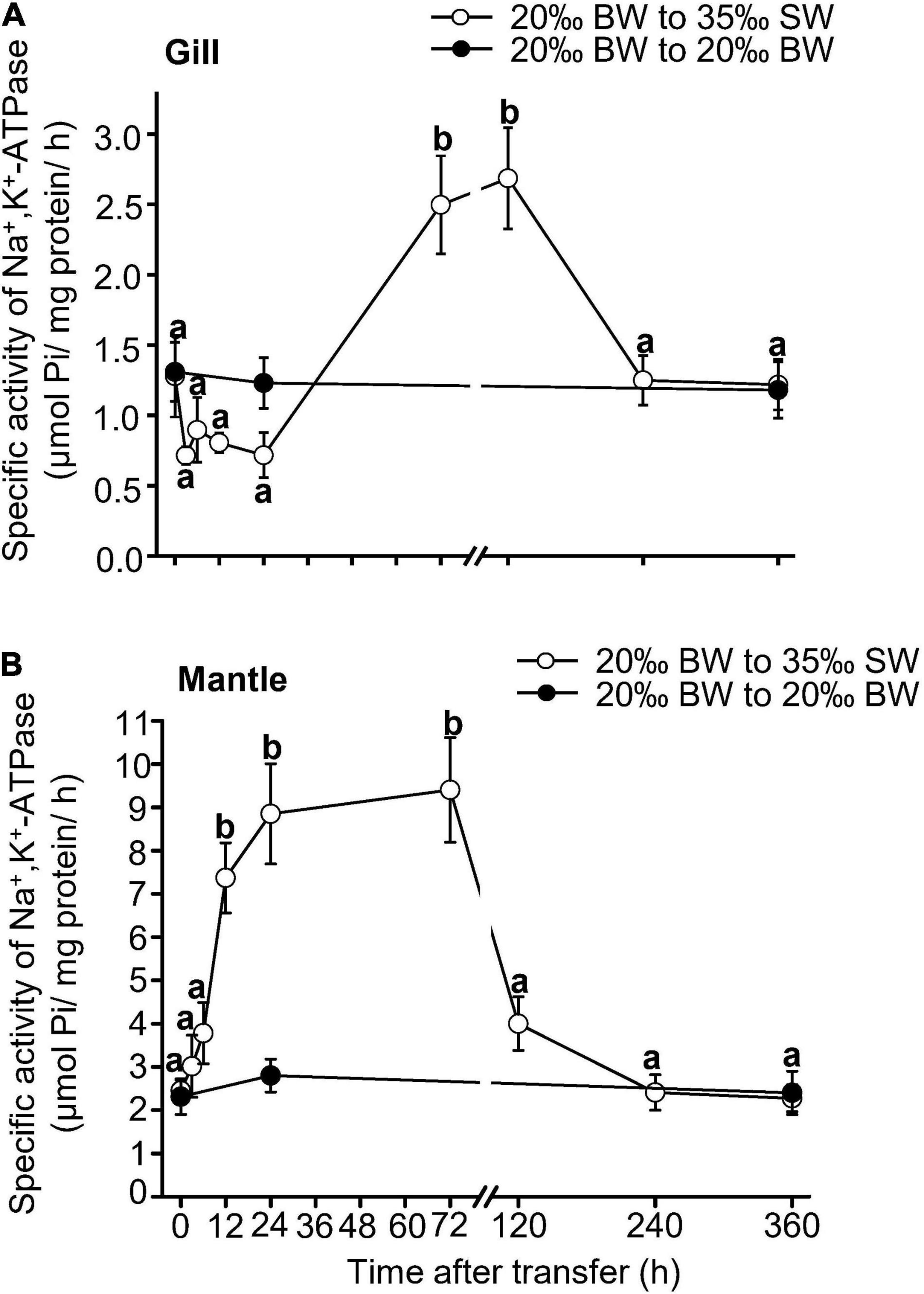
Figure 2. Changes in Na+/K+-ATPase activities in the gills (A) and mantle (B) of Asian hard clams following direct transfer from 20‰ brackish water (BW) to 35‰ seawater (SW). Different letters indicate significant differences between different time points by the Tukey’s pairwise test following the one-way analysis of variance (p < 0.05). No significant difference was found among time-points in the control group (from 20 to 20‰ BW). Values are the means ± SEM (N = 5).
Effects of High Salinity on Free Amino Acid Contents in the Gills and Mantle
The changes in the taurine content in the gills and mantle of Asian hard clams were in response to transfer from BW to SW, as no significant change was found in the control group after transfer (Figure 3). In the gills and mantle, the taurine content was significantly increased at 240 h (10 days) and 360 h (15 days) post-transfer (Figure 3 and Tables 1, 2). Similarly, the contents of glycine, alanine, and histidine in the gills, and those of aspartate, glutamate, glycine, alanine, and histidine in the mantle were also significantly upregulated after the transfer from BW to SW (Tables 1, 2). Briefly, the contents of alanine and glycine were upregulated earlier in the gills (6 h) than in the mantle (24 h) (Tables 1, 2). In contrast, the levels of aspartate and glutamate were increased in the mantle, but not in the gills (Tables 1, 2).
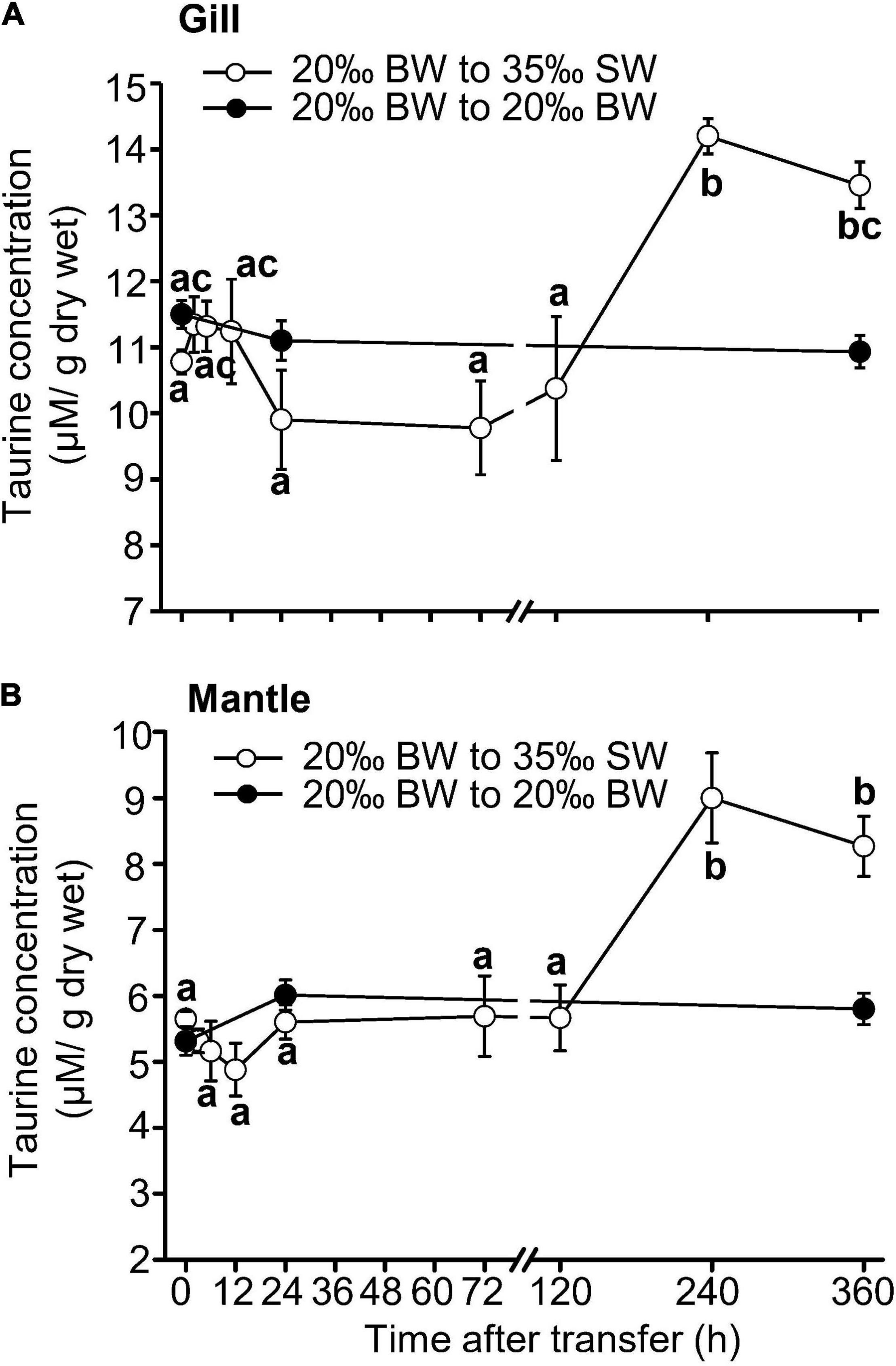
Figure 3. Changes in taurine content in the gills (A) and mantle (B) of Asian hard clams transferred directly from 20‰ brackish water (BW) to 35‰ seawater (SW). Different letters indicate significant differences between different time points, by the Tukey’s pairwise test following the one-way analysis of variance (ANOVA) (p < 0.05). No significant difference was found among time-points in the control group (from 20 to 20‰ BW). Values are the means ± SEM (N = 5).
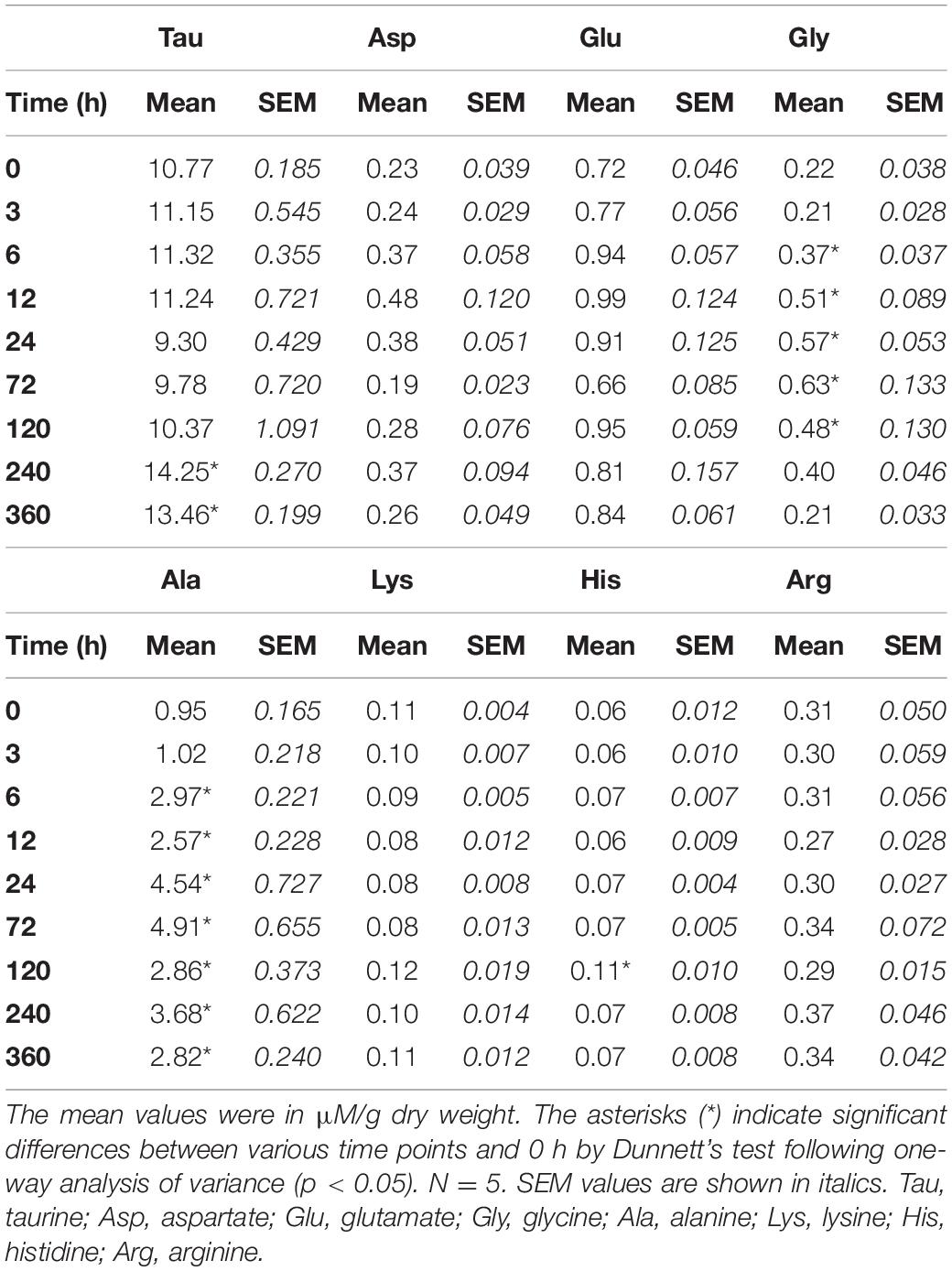
Table 1. Time-course changes in free amino acid (FAA) content of the gills of Asian hard clams transferred from brackish water (20‰) to seawater (35‰).
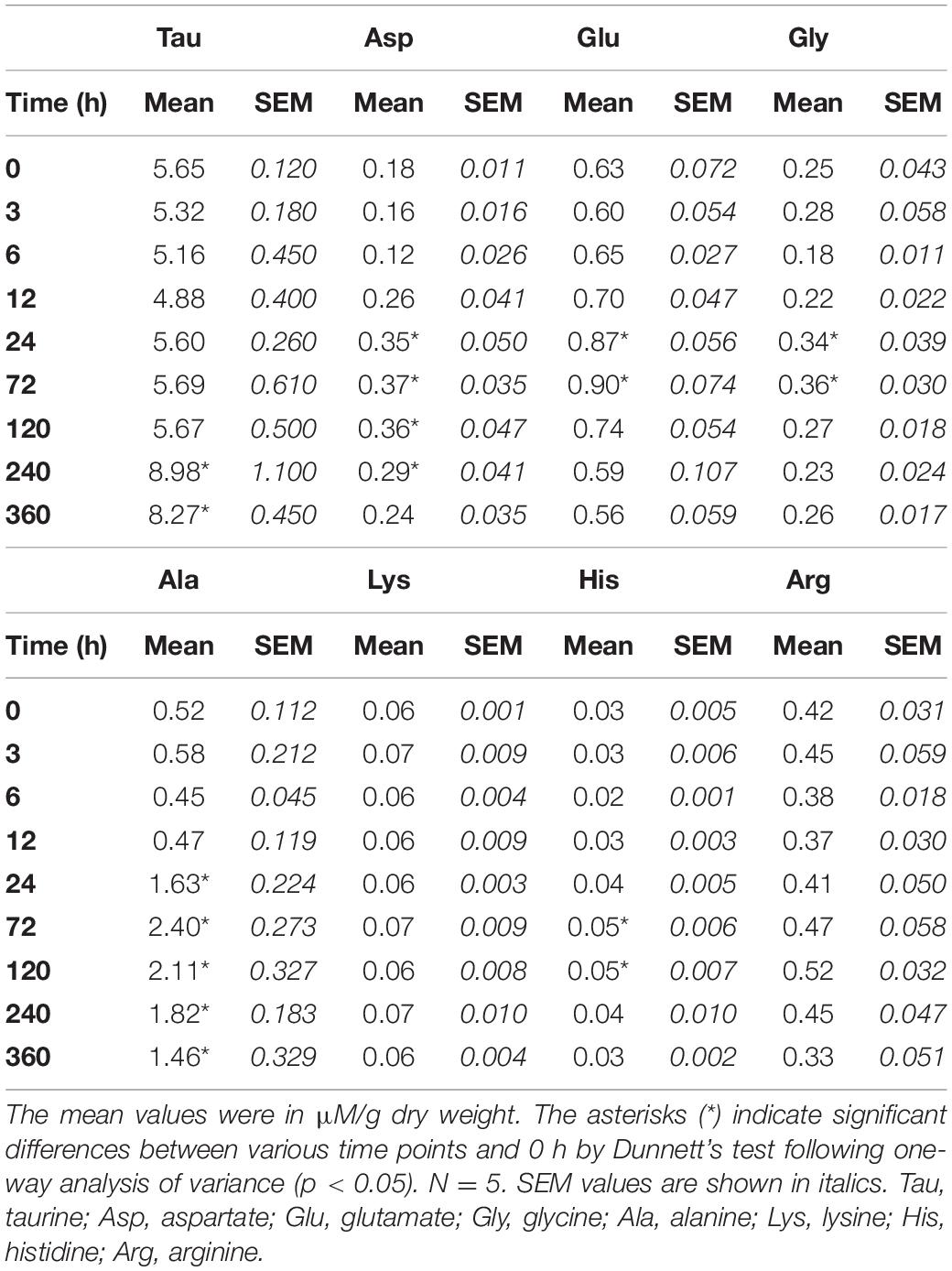
Table 2. Time-course changes in free amino acid (FAA) content of the mantle of Asian hard clams transferred from brackish water (20‰) to seawater (35‰).
Effects of High Salinity on Taurine Transporter mRNA and Protein Expression in the Gills and Mantle
The expression of TAUT mRNA in the gills and mantle of Asian hard clams was significantly increased at 6 h post-transfer and then declined to its original level at 24 and 72 h post-transfer, respectively (Figure 4). In addition, the relative abundance of the TAUT protein in the gills was significantly downregulated at 240 h (10 days) and 360 h (15 days) post-transfer (Figures 5A,B). However, the relative abundance of the TAUT protein in the mantle was significantly increased at 24 and 72 h post-transfer and then gradually decreased to its original level (Figures 5C,D). Notably, TAUT mRNA and protein expression in the gills and mantle did not significantly change in the control group after transfer.
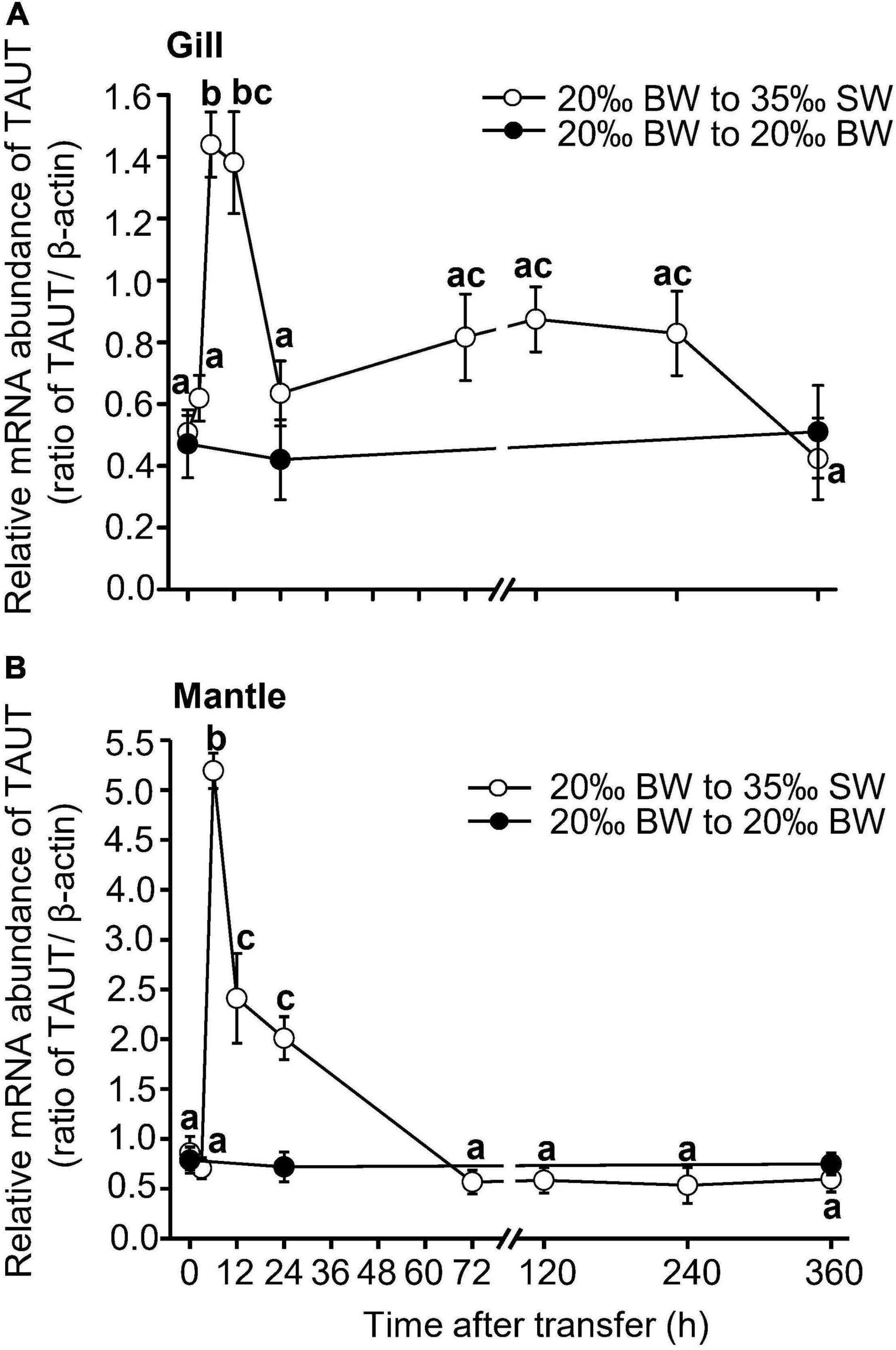
Figure 4. Changes in taurine transporter mRNA abundance in the gills (A) and mantle (B) of Asian hard clams following direct transfer from 20‰ brackish water (BW) to 35‰ seawater (SW). Different letters indicate significant differences between different time points, by the Tukey’s pairwise test following the one-way analysis of variance (ANOVA) (p < 0.05). No significant difference was found among time-points in the control group (from 20 to 20‰ SW). Values are the means ± SEM (N = 5).
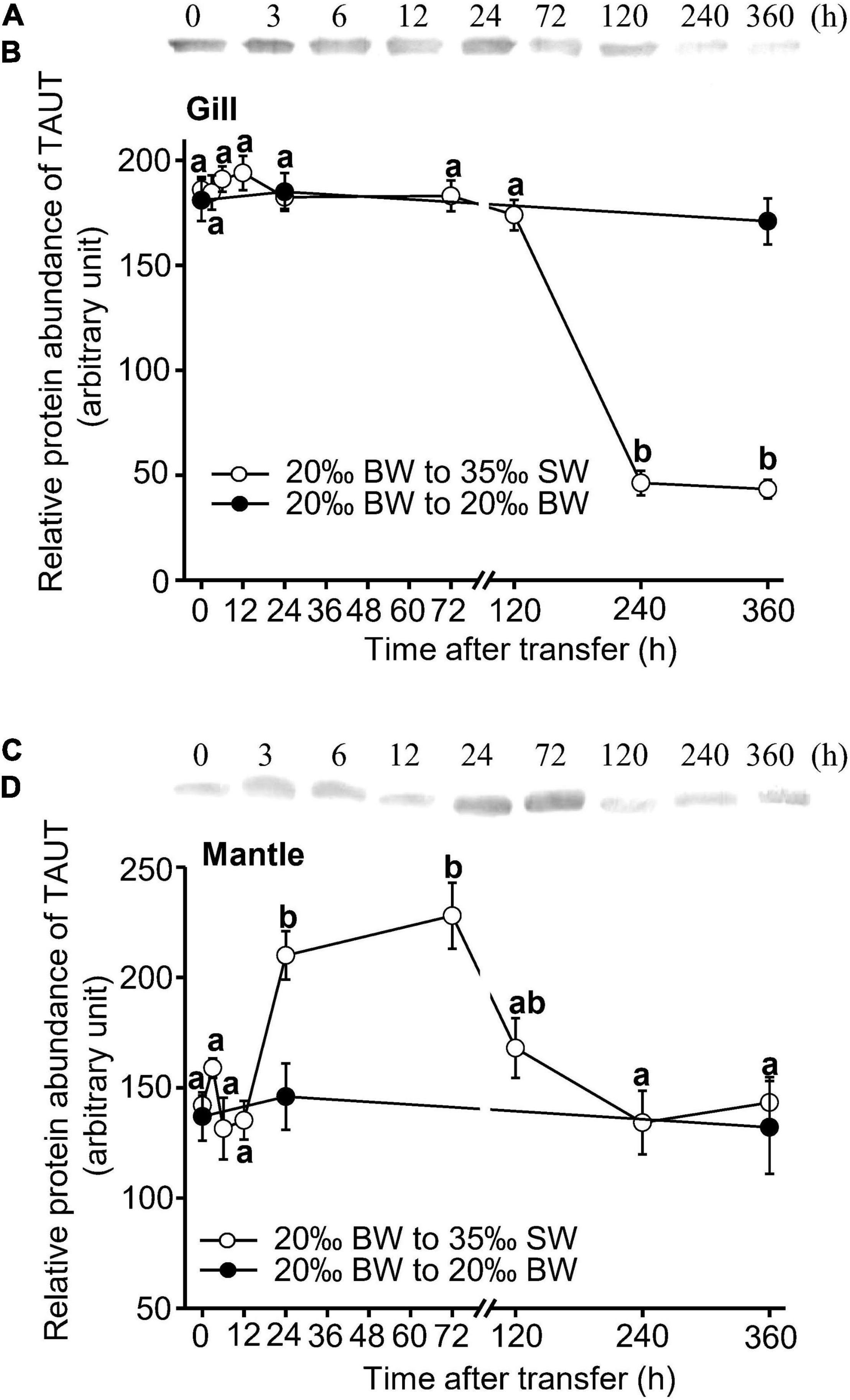
Figure 5. Representative immunoblots (A,C) and changes in relative protein abundance of gill and mantle taurine transporter (B,D) in Asian hard clams following transfer from 20‰ brackish water (BW) to 35‰ seawater (SW). Different letters indicate significant differences between different time points, by the Tukey’s pairwise test following the one-way analysis of variance (ANOVA) (p < 0.05). No significant difference was found among time-points in the control group (from 20 to 20‰ BW). Values are the means ± SEM (N = 5).
Discussion
Our previous research demonstrated that Asian hard clams are osmoconformer bivalves, as the osmolality of their body fluids conforms to that of their environment after long-term exposure (Lin et al., 2016). In the present study, we further revealed the time-course changes in hemolymph osmolality in the Asian hard clam after transfer from 20‰ BW, the salinity of their natural habitat, to 35‰ SW, an hyperosmotic salinity. We found that the hemolymph osmolality started increasing at 3 h post-transfer and stabilized at 6 h post-transfer. In addition, we found that the concentrations of both Na+ and Cl– in the hemolymph were significantly increased at 3 h post-transfer and gradually stabilized after 24 h. This indicates that the hemolymph osmolality of the Asian hard clam quickly conforms to hyperosmotic salinity. A similar phenomenon has been observed in other marine osmoconformers. When the giant Pacific oyster was transferred from 380 to 800 mOsm/kg SW, its hemolymph osmolality increased rapidly at 2 h post-transfer, reaching an equilibrium with the ambient osmolality at 8 h post-transfer (Hosoi et al., 2003). In the Pacific abalone Haliotis discus hannai, the levels of Na+ and K+ in the hemolymph were significantly elevated and stabilized at 0.25 h post-transfer after being transferred from 30 to 40‰ SW (Jia and Liu, 2018). However, Lin et al. (2021) reported that the osmolality, Na+, and Cl– levels in the hemolymph of Asian hard clams that were transferred from 20 to 10‰ BW, a hypoosmotic salinity, were significantly decreased after 24 h and stabilized at 72 h post-transfer. These results suggest that the changes in osmolality and Na+ and Cl– levels in the hemolymph of Asian hard clams under hypoosmotic conditions are slower than under hyperosmotic conditions.
The NKA belongs to the P-type ATPase family and is essential for the regulation of cell osmolality (Lang et al., 1998; Li and Langhans, 2015). Saintsing and Towle (1978) revealed that the acclimation of the Atlantic rangia Rangia cuneata, a freshwater bivalve, to a low-salinity environment was accompanied by an adaptive increase in NKA activity in the mantle. In the Pacific abalone, the NKA activity of both the gills and mantle was significantly upregulated after individuals were transferred from 30 to 40‰ SW, which is a hyperosmotic salinity (Jia and Liu, 2018). This change was suggested to be involved in the osmoregulation of these bivalves. In the present study, we found that the NKA activity in the gills and mantle of Asian hard clams transferred from 20‰ BW to 35‰ SW was significantly and transiently elevated. This increase in NKA activity after transfer to the hyperosmotic environment may also reflect the osmoregulatory responses of these tissues. In contrast to its response to hyperosmotic salinity exposure, Lin et al. (2021) demonstrated that the NKA activity in the gills and mantle of Asian hard clams did not change after being transferred from 20 to 10‰ BW, an hypoosmotic environment, compared to the original level at 0 h. The hemolymph osmolality when this species is exposed to 10, 20, and 35‰ environments is approximately 230, 520, and 950 mOsmol/kg, respectively (Lin et al., 2016), and declines to approximately 230 mOsmol/kg 72 h after being transferred from 20 to 10‰ BW (Lin et al., 2021). The present study revealed that when the Asian hard clams were transferred from 20‰ BW to 35‰ SW, the osmolality of hemolymph was increased to approximately 950 mOsmol/kg 6 h after the transfer. The change in hemolymph osmolality was more acute and larger in individuals transferred to 35‰ SW than those transferred to 10‰ BW. Different changes in hemolymph osmolality may cause different responses of NKA activity in the gills and mantle of the Asian hard clam under high and low salinity exposure.
In the present study, the mantle NKA activity after the Asian hard clams were transferred to 35‰ SW was found to increase greatly from 12 to 72 h post-transfer and then returned to its original level 120 h after the transfer. The gill NKA activity significantly increased from 72 to 120 h post-transfer. Additionally, the increase in NKA activity was approximately four and two times its original level in the mantle and gills, respectively. These results revealed that the mantle NKA activity was more sensitive to hyperosmotic salinity stress than the gill NKA activity. Lin et al. (2016) demonstrated that the NKA activity was higher in the mantle than in the gills (over approximately 2.5–5 times) when Asian hard clams were exposed to 10, 20, and 35‰ environments. Furthermore, the mantle NKA activity of Asian hard calms exposed to 35‰ SW was significantly higher than that those exposed to 20‰ BW. In contrast, there were no differences between the gill NKA activities of individuals exposed to different salinity levels (Lin et al., 2016). In Rangia cuneata, the NKA activity was also higher in the mantle than in the gills (over approximately three times) (Saintsing and Towle, 1978). The mantle NKA activity, but not the gill NKA activity, was adaptively increased when Rangia cuneata was exposed to a relatively hypoosmotic environment (Saintsing and Towle, 1978). Lin et al. (2021) stated that the NKA activity was transiently changed in the mantle, but not in the gills of Asian hard clams under hypoosmotic salinity. According to these studies, higher NKA activity in the mantle may enable it to rely more on the NKA than the gills for osmoregulation under certain types of osmotic stress. This may explain the greater and faster change in the mantle NKA activity than that in the gills under hyperosmotic salinity.
Furthermore, FAAs are vital organic osmolytes in bivalves (Pourmozaffar et al., 2019). For instance, alanine, glutamate, glycine, and taurine have been identified as important FAAs for maintaining intracellular osmolality in different marine bivalve species (Sokolowski et al., 2003; Pourmozaffar et al., 2019). In the Asian hard clam, taurine is the most abundant FAA and plays an important role in tissues under osmotic challenge (Lin et al., 2016; Lin et al., 2021). The present study indicated that the ratio of taurine content in the total amount of measured amino acids in the gills and mantle of Asian hard clams before being transferred from 20‰ BW to 35‰ SW was approximately 70–75%. In addition, the taurine content of the gills was approximately two times higher than that of the mantle. Hence, the gills may have greater buffering capacity than the mantle when individuals are exposed to 35‰ SW. In addition, alanine is the second most dominant FAA in the gills and mantles of the Asian hard clam (Lin et al., 2016). The present study showed that the ratio of alanine content was approximately 7 and 10% in the measured FAAs contents in the gill and mantle of the hard clam acclimated to 20‰ BW, respectively. After the Asian hard clam was exposed to 35‰ SW, the ratio of alanine content was significantly elevated up to approximately 15% within 6 and 24 h post-transfer in the gills and mantle, respectively. Thus, different taurine content and the time-course change in alanine content may also result in variable changes in NKA activity between the gills and mantle of Asian hard clams after transfer from 20‰ BW to 35‰ SW.
The long-term perturbation of ion levels in cells is harmful. Therefore, cells survive under long-term osmotic stress by modulating their organic osmolyte content (Kwon and Handler, 1995; Yancey, 2005), such as FAAs, which are extremely important in osmoconformer bivalves. The accumulation of FAAs in the tissues of bivalves is increased under hyperosmotic conditions (Yancey, 2005; Pourmozaffar et al., 2019). Taurine is the most abundant and important FAA for the osmoregulation of tissues in most osmoconformer bivalves (Lange, 1963; Gilles, 1972; Livingstone et al., 1979; Zurburg and DeZwaan, 1981; Deaton et al., 1985; Hosoi et al., 2003; Sokolowski et al., 2003; Toyohara and Hosoi, 2004; Kube et al., 2007). In the present study, we found that the taurine contents of the gills and mantle of Asian hard clams were significantly increased at 240 and 360 h (10 and 15 days) after transfer to 35‰ SW. This means that the accumulation of taurine in these tissues occurred as a late response to hyperosmotic salinity exposure. A similar phenomenon was found in the giant Pacific oyster, whose mantle taurine content was upregulated at the last time point of the experiment after transfer to a hyperosmotic environment (from 15 to 30‰ SW; Hosoi et al., 2003). Our results also revealed that the contents of other FAAs were upregulated in the gills and mantle of the Asian hard clam after transfer to 35‰ SW. Briefly, the glycine and alanine contents were significantly upregulated at 6 h post-transfer, while the content of histidine only increased at 120 h post-transfer in the gills of Asian hard clams. In the mantle, the aspartate, glutamate, glycine, and alanine contents began to be upregulated at 24 h post-transfer, and the histidine content was increased at 72 h post-transfer. Notably, the level and amplitude of the alanine content, the second most abundant FAA in the gills and mantle of Asian hard clams, were higher than those of other FAAs. The alanine content in the gills and mantle increased at 6 and 24 h post-transfer, respectively, until the end of the experiment. Moreover, the ratio of alanine content in the total content of measured FAAs in the gills increased from approximately 7 to 15%, and it increased from 10 to 15% in the mantle. The change in alanine content is suggested to partially increase the ability of these tissues to respond faster when faced with a hyperosmotic salinity. In the giant Pacific oyster, the alanine content of the mantle also increased faster than the taurine content, and the increased amplitude of alanine content was far higher than that of the other FAAs after transfer from 15 to 30‰ SW (Hosoi et al., 2003). Therefore, the increased alanine content may partially contribute to the osmoregulation of tissues in osmoconformer bivalves under hyperosmotic stress at an early stage.
The present study demonstrated that the taurine content of the gills and mantle of Asian hard clams predominantly increased at 240 and 360 h (10 and 15 days) post-transfer. Even though the alanine content increased during hyperosmotic acclimation, taurine was still the most abundant FAA in the gills and mantle, accounting for approximately 70–75% of the measured FAAs content. The TAUT is vital for taurine accumulation in cells (Hosoi et al., 2005, 2007; Lambert et al., 2015). The expression of TAUT has been detected in gills and mantles of bivalves, such as deep-sea mussels, Mediterranean blue mussels, giant Pacific oysters, and Asian hard clams (Hosoi et al., 2005, 2007; Inoue et al., 2008; Lin et al., 2016). The present study found that TAUT mRNA expression in the gills and mantle of Asian hard clams was acutely activated, peaked at 6 h post-transfer from 10‰ BW to 35‰ SW, and returned to its original level at 24 and 72 h post-transfer, respectively. Similar results were reported for other bivalves under high salinity stress. The expression of TAUT mRNA in the gills and mantle of the Mediterranean blue mussel increased at 2 h post-transfer until 24 h post-transfer (the final time point of the experiment) after transfer from 30 to 60‰ SW (Hosoi et al., 2005). The expression of TAUT mRNA in the mantle of the giant Pacific oyster was also quickly induced, reached a maximum level at 6 h post-transfer, and then declined to its original level after transfer from 30 to 60‰ SW (Hosoi et al., 2007). These results indicated that TAUT mRNA expression in the gills and mantle of bivalves is acutely and transiently induced by the hyperosmotic medium. Han et al. (2006) reported that hyperosmotic mediums could induce the activity of tonicity-responsive enhancer binding protein (TonEBP/NFAT5) to bind and activate the tonicity response element in the promoter region to the TAUT gene in mammalian cells. Therefore, TAUT gene expression in the tissues of bivalves upon high salinity challenge may be induced through the same pathway.
Similar to mRNA expression, the TAUT protein expression in the mantle of Asian hard clams was upregulated after transfer to 35‰ SW in the present study. The corresponding increase in TAUT protein abundance was also observed in human corneal epithelial cells after exposure to a hyperosmotic medium (Shioda et al., 2002). The increased protein expression in the mantle may be a result of upregulated TAUT mRNA expression. In contrast, relative TAUT protein levels in the gills did not change before 120 h post-transfer, but decreased after 240 h post-transfer. The taurine content of the gills was higher than that of the mantle (approximately two times higher at 0 h), and the alanine content increased faster in the gills than in the mantle (6 vs. 24 h post-transfer). Previous studies have reported that increased Na+ and Cl– concentrations could activate the TAUT activity of bivalves (Hosoi et al., 2005, 2007; Inoue et al., 2008). Therefore, the increased TAUT activity in the gills of Asian hard clams after transfer to 35‰ SW may be sufficient to increase the accumulation of taurine by enhancing TAUT protein translation, although it may not be strictly necessary. The TAUT protein abundance in the gills and mantle of Asian hard clams was greatly downregulated and returned to the original level, respectively, after 240 h post-transfer in the present study. The taurine content in these tissues was significantly increased. Han et al. (1997) reported that the TAUT abundance was lower in cells cultured in a medium containing extra taurine than in cells cultured in a taurine-free medium. The increased taurine content may cause a negative feedback on TAUT protein expression in the gills and mantle of the Asian hard clam at the later stages of the response to being transferred to 35‰ SW.
In summary, the present study reported the dynamic physiological responses and regulation of the gills and mantle of Asian hard clams after transfer to hyperosmotic salinity from the salinity of their natural habitats. The findings of this study enhance our understanding of the regulatory mechanisms underlying the response of hard clams to hyperosmotic salinity stress.
Data Availability Statement
The original contributions presented in the study are included in the article/supplementary material, further inquiries can be directed to the corresponding author/s.
Author Contributions
C-HL, P-LY, and T-HL wrote the manuscript, analyzed the results, conceived, and designed the research and the experiments. C-HL and T-HL secured funding for this research and for all reagents and materials. C-HL, P-LY, and Y-CW performed the experiments. All authors contributed to the article and approved the submitted version.
Funding
This study was financially supported by grants from the Ministry of Science and Technology (MOST) of Taiwan (89-2313- B-025-334, 90-2313-B-005-065, 91-2313-B-005-053, and 109-2313-B-992- 003-MY3).
Conflict of Interest
The authors declare that the research was conducted in the absence of any commercial or financial relationships that could be construed as a potential conflict of interest.
Publisher’s Note
All claims expressed in this article are solely those of the authors and do not necessarily represent those of their affiliated organizations, or those of the publisher, the editors and the reviewers. Any product that may be evaluated in this article, or claim that may be made by its manufacturer, is not guaranteed or endorsed by the publisher.
References
Barnes, D. K. A. (1999). High diversity of tropical intertidal zone sponges in temperature, salinity and current extremes. Afr. J. Ecol. 37, 424–434. doi: 10.1046/j.1365-2028.1999.00197.x
Borgatti, A. R., Pagliarani, A., Ventrella, V., Manuzzi, M. P., Trombetti, F., Pirini, M., et al. (2003). Na+, K+-ATPase and other parameters in bivalve mollusks from the Adriatic sea under different environmental conditions. Vet. Res. Commun. 1, 207–210. doi: 10.1023/b:verc.0000014141.63067.6b
Burg, M. B., Ferraris, J. D., and Dmitrieva, N. I. (2007). Cellular response to hyperosmotic stresses. Physiol. Rev. 87, 1441–1474. doi: 10.1152/physrev.00056.2006
L. C. Chen (ed.) (1990). “Hard clam culture,” in Aquaculture in Taiwan (Cambridge: Blackwell Scientific Publications), 223–227.
T. P. Chen (ed.) (1976). “Culture of clam, Meretrix lusoria,” in Aquaculture Practices in Taiwan (Norwich: Page Bors, Ltd).
Deaton, L. E., Hilbish, T. J., and Koehn, R. K. (1985). Protein as a sourceof amino nitrogen during hyperosmotic volume regulation in the mussel, Mytilus edulis. Physiol. Zool. 57, 609–619. doi: 10.1086/physzool.57.6.30155987
Garcia-Perez, A., and Burg, M. B. (1991). Renal medullary organic osmolytes. Physiol. Rev 71, 1081–1115. doi: 10.1152/physrev.1991.71.4.1081
Gilles, R. (1972). Osmoregulation in three molluscs: Acanthochitona discrepans (Brown), Glycymeris glycymeris (L.) and Mytilus edulis (L). Biol. Bulle. 142, 25–35. doi: 10.2307/1540243
Han, X., Budreau, A. M., and Chesney, R. W. (1997). Adaptive regulation of MDCK cell taurine transporter (pNCT) mRNA: transcription of pNCT gene is regulated by external taurine concentration. Biochim. Biophys. Acta 10351, 296–304. doi: 10.1016/s0167-4781(96)00217-5
Han, X., Patters, A. B., Jones, D. P., Zelikovic, I., and Chesney, R. W. (2006). The taurine transporter: mechanisms of regulation. Acta Physiol (Oxf). 187, 61–73. doi: 10.1111/j.1748-1716.2006.01573.x
Hansen, D. B., Friis, M. B., Hoffmann, E. K., and Lambert, I. H. (2012). Down-regulation of the taurine transporter TauT during hypoosmotic stress in NIH3T3 mouse fibroblasts. J. Membr. Biol. 245, 77–87. doi: 10.1007/s00232-012-9416-8
Hosoi, M., Kubota, S., Toyohara, M., and Toyohara, H. (2003). Effect of salinity change on free amino acid content in Pacific oyster. Fish. Sci. 69, 395–400. doi: 10.1046/j.1444-2906.2003.00634.x
Hosoi, M., Shinzato, C., Takagi, M., Hosoi-Tanabe, S., Sawada, H., Terasawa, E., et al. (2007). Taurine transporter from the giant Pacific oyster Crassostrea gigas: function and expression in response to hyper- and hypo-osmotic stress. Fish. Sci. 73, 385–394. doi: 10.1111/j.1444-2906.2007.01346.x
Hosoi, M., Takeuchi, K., Sawada, H., and Toyohara, H. (2005). Expression and functional analysis of mussel taurine transporter, as a key molecule in cellular osmoconforming. J. Exp. Biol. 208, 4203–4211. doi: 10.1242/jeb.01868
Huxtable, R. J., Pierno, S., De Luca, A., Camerino, C., and Camerino, D. C. (2000). Expanding the circle 1975–1999: sulfur biochemistry and insights on the biological functions of taurine. Adv. Exp. Med. Biol. 483, 1–25. doi: 10.1007/0-306-46838-7_1
Inoue, K., Tsukuda, K., Koito, T., Miyazaki, Y., Hosoi, M., Kado, R., et al. (2008). Possible role of a taurine transporter in the deep-sea mussel Bathymodiolus septemdierum in adaptation to hydrothermal vents. FEBS Lett. 582, 1542–1546. doi: 10.1016/j.febslet.2008.03.052
Jia, Y., and Liu, X. (2018). Expression of Na+/K+-ATPase was affected by salinity change in Pacific abalone Haliotis discus hannai. Front. Physiol. 7:1244. doi: 10.3389/fphys.2018.01244
Kube, S., Sokolowski, A., Jansen, J. M., and Schiedek, D. (2007). Seasonal variability of free amino acids in two marine bivalves, Macoma balthica and Mytilus spp., in relation to environmental and physiological factors. Comp. Biochem. Physiol. 147, 1015–1027. doi: 10.1016/j.cbpa.2007.03.012
Kwon, H. M., and Handler, J. S. (1995). Cell volume regulated transporters of compatible osmolytes. Curr. Opin. Cell. Biol. 7, 465–471. doi: 10.1016/0955-0674(95)80002-6
Lambert, I. H., Kristensen, D. M., Holm, J. B., and Mortensen, O. H. (2015). Physiological role of taurine–from organism to organelle. Acta Physiol (Oxf). 213, 191–212. doi: 10.1111/apha.12365
Lang, L., Busch, G. L., Ritter, M., Völkl, H., Waldegger, S., Gulbins, E., et al. (1998). Functional significance of cell volume regulatory mechanisms. Physiol. Rev. 78, 247–306. doi: 10.1152/physrev.1998.78.1.247
Lange, R. (1963). The osmotic function of amino acids and taurine in the mussel, Mytilus edulis. Comp. Biochem. Physiol. 10, 172–179.
Li, Z., and Langhans, S. A. (2015). Transcriptional regulators of Na+, K+-ATPase subunits. Front. Cell. Dev. Biol. 3:66. doi: 10.3389/fcell.2015.00066
Lin, C. H., Yeh, P. L., and Lee, T. H. (2016). Ionic and amino acid regulation in hard clam (Meretrix lusoria) in response to salinity challenges. Front. Physiol. 25:368. doi: 10.3389/fphys.2016.00368
Lin, C. H., Yeh, P. L., and Lee, T. H. (2021). Time-course changes in the regulation of ions and amino acids in the hard clam Meretrix lusoria upon lower salinity challenge. J. Exp. Zool. A Ecol. Integr. Physiol. 335, 602–613. doi: 10.1002/jez.2503
Livingstone, D. R., Widdows, J., and Fieth, P. (1979). Aspects of nitrogen metabolism of the common mussel Mytilus edulis: adaptation to abrupt and fluctuating change in salinity. Mar. Biol. 53, 41–55. doi: 10.1007/BF00386528
Lotsch, J., Hummel, T., Warskulat, U., Coste, O., Haussinger, D., Geisslinger, G., et al. (2014). Congenital taurine deficiency in mice is associated with reduced sensitivity to nociceptive chemical stimulation. Neuroscience 259, 63–70. doi: 10.1016/j.neuroscience.2013.11.037
Lourenco, R., and Camilo, M. E. (2002). Taurine: a conditionally essential amino acid in humans? An overview in health and disease. Nutr. Hosp. 17, 262–270.
McManus, M. L., Churchwell, K. B., and Strange, K. (1995). Regulation of cell volume in health and disease. N. Engl. J. Med. 333, 1260–1266. doi: 10.1056/NEJM199511093331906
Morth, J. P., Pedersen, B. P., Buch-Pedersen, M. J., Andersen, J. P., Vilsen, B., Palmgren, M. G., et al. (2011). A structural overview of the plasma membrane Na+,K+-ATPase and H+-ATPase ion pumps. Nat. Rev. Mol. Cell. Biol. 12, 60–70. doi: 10.1038/nrm3031
Peterson, G. L. (1978). A simplified method for analysis of inorganic phosphate in the presence of interfering substances. Anal. Biochem. 84, 164–172. doi: 10.1016/0003-2697(78)90495-5
Pourmozaffar, S., Tamadoni Jahromi, S., Rameshi, H., Sadeghi, A., Bagheri, T., Behzadi, S., et al. (2019). The role of salinity in physiological responses of bivalves. Rev. Aqua 12, 1548–1566. doi: 10.1111/raq.12397
Saintsing, D. G., and Towle, D. W. (1978). Na+, K+-ATPase in the osmoregulation Clam Rangia cuneata. J. Exp. Zool. 206, 435–442. doi: 10.1002/jez.1402060312
Shioda, R., Reinach, P. S., Hisatsune, T., and Miyamoto, Y. (2002). Osmosensitive taurine transporter expression and activity in human corneal epithelial cells. Invest. Ophthalmol. Vis. Sci. 43, 2916–2922.
Sokolowski, A., Wolowicz, M., and Hummel, H. (2003). Free amino acids in the clam Macoma balthica L (Bivalvia Mollusca) from brackish waters of the southern Baltic Sea. Comp. Biochem. Physiol. A Mol. Integr. Physiol. 134, 579–592. doi: 10.1016/s1095-6433(02)00360-4
Takeuchi, K., Toyohara, H., and Sakaguchi, M. (2000). A hyperosmotic stress induced mRNA of carp cell encodes Na+-and Cl–-dependent high affinity taurine transporter. Biochim. Biophys. Acta. 1464, 219–230. doi: 10.1016/S0005-2736(00)00158-9
Takeuchi, K., Toyohara, H., Kinoshita, M., and Sakaguchi, M. (2001). Ubiquitous increase in taurine transporter mRNA in tissues of tilapia (Oreochromis mossambicus) during high-salinity adaptation. Fish. Physiol. Biochem. 23, 173–182. doi: 10.1023/A:1007889725718
Toyohara, H., and Hosoi, M. (2004). The role of taurine in the osmotic adaptation in the marine mussel Mytilus galloprovincialis. Mar. Biotech. 6, S51 1–S516.
Toyoshima, C., Kanai, R., and Cornelius, F. (2011). First crystal structures of Na+,K+-ATPase: new light on the oldest ion pump. Structure 19, 1732–1738. doi: 10.1016/j.str.2011.10.016
Yancey, P. H. (1994). “Compatible and counteracting solutes,” in Cellular and Molecular Physiology of Cell Volume Regulation, ed. K. Strange (Boca Raton, FL: CRC Press), 81–109. doi: 10.1201/9780367812140-7
Yancey, P. H. (2005). Organic osmolytes as compatible, metabolic and counteracting cytoprotectants in high osmolarity and other stresses. J. Exp. Biol. 208, 2819–2830. doi: 10.1242/jeb.01730
Keywords: hard clam, osmoregulation, taurine transporter, free amino acid, Na+/K+ ATPase
Citation: Lin C-H, Yeh P-L, Wang Y-C and Lee T-H (2021) Dynamic Regulation of Ions and Amino Acids in Adult Asian Hard Clams Meretrix lusoria Upon Hyperosmotic Salinity. Front. Mar. Sci. 8:749418. doi: 10.3389/fmars.2021.749418
Received: 29 July 2021; Accepted: 17 September 2021;
Published: 14 October 2021.
Edited by:
Xiaodan Wang, East China Normal University, ChinaReviewed by:
Zongli Yao, East China Sea Fisheries Research Institute, Chinese Academy of Fishery Sciences, ChinaPedro Miguel Guerreiro, University of Algarve, Portugal
Copyright © 2021 Lin, Yeh, Wang and Lee. This is an open-access article distributed under the terms of the Creative Commons Attribution License (CC BY). The use, distribution or reproduction in other forums is permitted, provided the original author(s) and the copyright owner(s) are credited and that the original publication in this journal is cited, in accordance with accepted academic practice. No use, distribution or reproduction is permitted which does not comply with these terms.
*Correspondence: Tsung-Han Lee, dGhsZWVAZW1haWwubmNodS5lZHUudHc=