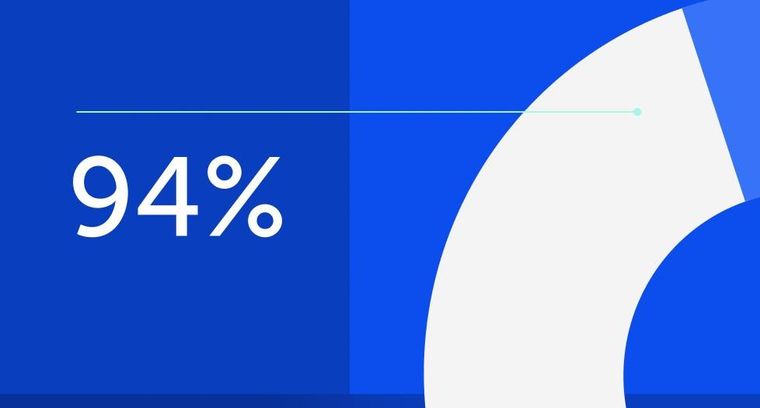
94% of researchers rate our articles as excellent or good
Learn more about the work of our research integrity team to safeguard the quality of each article we publish.
Find out more
ORIGINAL RESEARCH article
Front. Mar. Sci., 22 November 2021
Sec. Aquatic Physiology
Volume 8 - 2021 | https://doi.org/10.3389/fmars.2021.737926
The few hormone studies on bottlenose dolphin (Tursiops truncatus) pregnancy with different reproductive outcomes, e.g., normal birth, stillbirth and abortion, have mostly focused on progestagens or relaxin. However, recent analysis of androgens, glucocorticoids and estrogens has shown they are also biomarkers of cetacean pregnancy. Therefore, our objective was to examine circulating concentrations of androgens, glucocorticoids and estrogens during bottlenose dolphin pregnancies with different reproductive outcomes, including normal pregnancy (NORM, n = 27), failure to thrive (FTT, n = 17), perinatal loss (PNL, n = 20), early loss (EL, n = 12) and false pregnancy (FP, n = 16), to determine if they could be potential indicators of reproductive or fetal health. We analyzed longitudinal serum samples (n = 654) from 57 bottlenose dolphins and 92 reproductive events for testosterone, androstenedione, cortisol, estradiol and estrone conjugates. Testosterone concentrations were higher during EL compared to NORM and lower during FP at MID (day 121 – 240 post ovulation/conception) and LATE (day 241 – end of FP) stages (months post conception/ovulation [MPC, MPO] seven through ten, P < 0.05). During FTT, androstenedione concentrations were increased compared to NORM pregnancies in the EARLY and LATE stages (P ≤ 0.05), and concentrations were reduced during FP (P < 0.05). For cortisol, FTT pregnancies had higher concentrations compared to NORM during all stages (P < 0.05), while PNL had higher cortisol during EARLY and LATE stages (P < 0.05). Estradiol concentrations were lower for EL and FP compared to NORM (P < 0.05), while estrone conjugates were only reduced during FP (P < 0.05). Based on our results only cortisol may be a useful predictor of PNL, while both cortisol and androstenedione were useful for distinguishing FTT pregnancies. Similarly, both testosterone and estradiol during EL and FP were different from NORM. Our data indicate a suite of pregnancy specific hormone biomarkers to evaluate maternal and fetal health in bottlenose dolphins should include cortisol, androgens and estrogens. This research also highlights the importance on non-progestagen hormones as sentinels of cetacean pregnancy and fetal health.
Although there are several studies of hormone measurements during pregnancy in bottlenose dolphins (Tursiops truncatus), the existing data are mostly comprised of pregnancies with successful outcomes, i.e., a live birth and surviving calf. Within one zoo-based population of bottlenose dolphins, the pregnancy loss and stillbirth rates are reported to be 11.5 and 5.2%, respectively (Robeck et al., 2021). Although these numbers are similar to what has been reported for other zoo-based cetaceans and domestic species (Forar et al., 1995 [domestic, cattle]; Robeck et al., 2018 [cetacean, killer whale{Orcinus orca}]), the ability to predict reproductive outcome based on hormonal biomarkers of pregnancy and then be prepared for assistance and intervention with problematic pregnancies would be tremendously beneficial for animal care husbandry and management. Evaluations of normal and abnormal pregnancy via hormone analyses have mostly relied on measurements of circulating progesterone (P4) or progestagens (PG), relaxin and thyroid hormones (Bergfelt et al., 2011, 2017; O’Brien and Robeck, 2012; West et al., 2014; Robeck et al., 2021). These studies provide valuable information regarding pregnancy related hormone dynamics for successful pregnancies, stillbirths, abortions, and early embryonic loss. However, the study of other known pregnancy hormonal biomarkers, such as androgens and glucocorticoids (Steinman et al., 2016), during bottlenose dolphin pregnancies with different reproductive outcomes has yet to be performed and may shed more light on indicators that could identify poor reproductive outcomes.
Androgen analysis during pregnancy has been performed in a few cetacean species and is considered a consistent marker of pregnancy (Steinman et al., 2016 [bottlenose dolphin]; Robeck et al., 2017 [killer whale]; Wasser et al., 2017 [killer whale]; Boggs et al., 2019 [bottlenose dolphin]; Dalle Luche et al., 2020 [humpback whale, Megaptera novaeangliae]; Legacki et al., 2020 [killer whale, bottlenose dolphin, beluga, Delphinapterus leucas]). Previous analysis of circulating testosterone (T) during normal pregnancy in the bottlenose dolphin (pregnancies that result in the birth of a calf that survives longer than 30 days) has shown that T increases linearly, beginning in the third month of pregnancy, reaching significance during the fourth month and remains elevated above early pregnancy concentrations throughout gestation (Steinman et al., 2016), but androstenedione (A4), in serum, as measured using liquid chromatography tandem mass spectrometry (LCMS-MS), increases in a quadratic fashion, with concentrations highest during mid-gestation (Legacki et al., 2020). For bottlenose dolphins, Boggs et al. (2019) and Galligan et al. (2020) have both reported elevated androgen concentrations in blubber from pregnant animals. Elevated concentrations of androgens and/or androgen metabolites during pregnancy have been reported in North Atlantic right whales (Eubaleana glacialis, Hunt et al., 2006; Corkeron et al., 2017), beluga (Richard et al., 2017), Yangtze finless porpoise (Neophocaena asiaeorientalis, Hao et al., 2006), blue whales (Balaenoptera musculus, Melica et al., 2021b) and humpback whales (Hunt et al., 2019) but were not observed in gray whale (Eschrichtius robustus) blubber (Melica et al., 2021a). Dalle Luche et al. (2020) suggest that, during late term humpback whale pregnancy, A4 and T measurements may be more effective pregnancy biomarkers than P4. Despite sufficient evidence of increased androgen measurements during cetacean pregnancy, these analyses have yet to be incorporated on a larger scale into female reproductive analysis in both in situ and ex situ cetaceans. These observed elevations in androgens during cetacean pregnancy highlight the importance of integrating these measurements into reproductive hormone analysis and pregnancy assessments.
Analysis of circulating glucocorticoids (GCs) in bottlenose dolphins has occurred during and outside of pregnancy (Richkind and Ridgway, 1975; St. Aubin et al., 1996; Suzuki et al., 1998, 2003; Steinman et al., 2016). One study has shown GC concentrations remain unchanged during pregnancy (Richkind and Ridgway, 1975); however, this study had a limited number of animals and samples across pregnancy. Another, with a much larger sample size and monthly sample collection, has demonstrated that circulating cortisol increases significantly in the last month of gestation (Steinman et al., 2016). However, whether these observed late or near term increases in GCs are maternal or fetal-derived, or some combination of both is unknown. Elevated fecal GC metabolite concentrations during cetacean pregnancy have been observed in the North Atlantic right whale (Hunt et al., 2006), killer whale (Wasser et al., 2017), humpback whale (Hunt et al., 2019) and blue whale (Valenzuela-Molina et al., 2018). Thus, GC measurements may be able to distinguish between normal versus abnormal pregnancies, especially in late or near-term gestations.
Longitudinal measurements of circulating estrone/estrone conjugates (EC) concentrations during normal bottlenose dolphin pregnancy have been previously reported by our group (Steinman et al., 2016). Concentrations of EC increase significantly during the late stage of pregnancy in the bottlenose dolphin (Steinman et al., 2016). Another study has also shown an increase in estrogen concentrations across pregnancy (Richkind and Ridgway, 1975), although the specific estrogens measured were not reported. Circulating EC and estradiol (E2) also increase throughout killer whale pregnancy, peaking during the final month of gestation (Robeck et al., 2016). A longitudinal, quantitative analysis of circulating E2 throughout normal or abnormal bottlenose dolphin pregnancy has not been performed, to our knowledge.
In the killer whale, a study comparing circulating PGs and relaxin during pregnancies with different outcomes as well as false pregnancy and estrus represents one of the most thorough examinations of several different reproductive outcomes via longitudinal reproductive hormone monitoring in cetaceans (Robeck et al., 2018). We have recently conducted a similar study of P4 and PGs in the bottlenose dolphin (Robeck et al., 2021). Past studies of reproductive hormone profiles during normal bottlenose dolphin pregnancy have provided reference ranges that can be used to assess other pregnancy types, such as stillbirth, abortion or early embryonic loss (Bergfelt et al., 2011, 2017; O’Brien and Robeck, 2012; West et al., 2014).
Hormone concentrations outside of the normal physiologic range may have a negative influence on the developing fetus and, consequently, have been referred to as “endogenous functional teratogens” (Plagemann, 2005). Current management practices for evaluating animal and fetal health during pregnancy in zoo-based cetaceans typically includes serial ultrasound and progesterone monitoring (Ivancic et al., 2020; Saviano et al., 2020). Abnormal ultrasound or progesterone results necessitate further evaluation of the female and intervention if needed (Robeck et al., 2012). Comparisons of hormone profiles in bottlenose dolphin pregnancies with different reproductive outcomes may be able to identify a suite of hormone tests that could detect pregnancies that may be problematic and lead to improved pre-and post-natal care in cetaceans. In addition, combining a hormone profile evaluation with ultrasonic fetal age estimation in wild bottlenose dolphins during health assessments may help identify females with at risk pregnancies that could then be targeted for follow-up exams. Therefore, the overall goal of this study was to describe profiles of circulating androgens (T and A4), GCs (cortisol) and estrogens (E2 and EC) in abnormal pregnancies and false pregnancy (FP) and compare results against values from normal (NORM) pregnancies in the bottlenose dolphin. The specific objectives were to: (1) characterize profiles of circulating androgens, cortisol and estrogens during months and stages of pregnancies with different reproductive outcomes, including normal live birth, perinatal loss (PNL), early loss (EL), abortion (AB) and failure to thrive (FTT); and (2) investigate if any of the hormones tested could be predictors of a poor outcome or reproductive failure.
All samples were collected as part of routine husbandry procedures for bottlenose dolphins. All procedures described within were reviewed and approved by the SeaWorld Parks and Entertainment Incorporated Research Review Committee and were performed in accordance with the United States Animal Welfare Act for the care of marine mammals.
Blood samples (n = 654: 576 samples from pregnancy or false pregnancy; 4 placental samples obtained from cord blood; and 74 samples from physiologic events) were collected from 57 animals during the period from 1983 through 2017 (Robeck et al., 2021; Table 1). These samples were collected as part of routine monitoring of animals for detection of reproductive events (n = 92). Reproductive events were defined as periods when serum P4 concentrations were increased above 1 ng/ml for longer than the normal luteal phase length of 21 days (O’Brien and Robeck, 2012). Physiologic events (n = 59 events) were comprised of samples collected during the following periods: FOLLICULAR (n = 16 samples from 12 animals and 12 events); OVULATION (n = 24 samples from 18 animals and 24 events); and LUTEAL (n = 34 samples from 15 animals and 23 events). Animals were housed at SeaWorld Parks in Orlando, San Antonio and San Diego. Animals were housed in enclosures containing ≥ 850 m3 of either natural processed (San Diego) or manufactured salt-water (Orlando, San Antonio).
Table 1. Classification of and sample collection characteristics, number of females within each group, mean ± sd (range) age at conception of animals within each group and number of previous calves prior to conception (parity).
Blood samples (n = 654 samples) were collected voluntarily (n = 509) or with manual restraint (n = 145) from animals ranging from weekly for peri-post ovulatory monitoring or monthly as part of routine husbandry management. Of these, there were 4 placental samples during normal pregnancy that were opportunistically collected from cord blood. Although most samples were collected using behavioral procedures without restraint, some of the historical samples collected prior to Jan 1, 2000, were collected using manual restraint. The exact number of these banked samples within this time period that were collected using restraint is unknown, but because the restraint method may have influenced GC sample concentration, the samples were further categorized as being either pre or post January 1, 2000, for the statistical analysis. Additionally, all ovulation samples (n = 24 samples/events from 18 animals) were collected as part of artificial insemination procedures and under restraint, thus these samples were also categorized accordingly (Robeck et al., 2013). Samples were collected from the ventral tail fluke using a 21-gauge winged blood collection set. Blood was collected by either the veterinary technician or attending veterinarian on staff and into BD Vacutainers (Becton Dickinson, Franklin Lakes, NJ, United States) containing activated thrombin. The thrombin-coagulated blood was centrifuged at 1500 rpm for 10 min, and the serum was decanted and frozen at −80°C for further testing. Although sampling time was not recorded for every sample, routine blood samples were typically collected in the mornings (before 12:00 h) as per standard husbandry procedure.
Bottlenose dolphin sera was extracted for use in the T and A4 hormone assays. Sample extractions were conducted identically to past hormone studies in killer whales (O’Brien et al., 2017; Robeck et al., 2017). Briefly, 3 ml of diethyl ether (Acros Organics, ThermoFisher, Waltham, MA, United States) was added to 0.15 ml of serum and vortexed at 1800 rpm for 5 min. The samples were then placed into an ultra-low (−80°C) freezer for 20 min to allow the aqueous layer to freeze. After, the solvent layer was poured off into a borosilicate glass tube and evaporated under compressed nitrogen gas. Samples were reconstituted with 0.3 ml of extraction buffer (0.2 M phosphate buffered saline, pH 7.5) and stored frozen at −20°C until assay. Mean ± sem extraction efficiency for this process (see aforementioned publications for description) was 93.7 ± 1.9% (n = 56).
All hormone concentrations were expressed as ng hormone per ml serum.
Testosterone concentrations were measured using a single antibody, direct enzyme immunoassay (EIA) as previously described (Munro and Lasley, 1988) and described in detail for use with bottlenose dolphin sera (Steinman et al., 2016). Assay validations for this species and sample matrix (parallelism, recovery) and antibody cross-reactivity and sensitivity information are described in detail in Steinman et al. (2016) and passed validity tests (parallelism, r = 0.994; recovery/accuracy, 87.22 ± 1.80%, linear regression, y = 0.98x – 7.02, r2 = 0.989, see Steinman et al., 2016). To check for intra-assay variation, samples were run in duplicate and any sample with a coefficient of variation (CV) > 10% between replicates was repeated. Intra-assay precision was previously tested in Steinman et al. (2016) by analyzing a single serum sample at different locations across the microtiter plate, and the CV was < 10%. Inter-assay CVs for two quality controls, with antibody binding at 30 and 70%, were 8.7 and 9.2%, respectively (n = 27 assays). A cetacean-specific biological control made from a pool of pregnant dolphin sera, with antibody binding at approximately 50%, was 11.3% (n = 27 assays).
Androstenedione concentrations were measured using a commercial, single antibody, direct EIA kit (40-056-205044, GenWay Biotech, Inc., San Diego, CA, United States) as previously described for use in the killer whale (O’Brien et al., 2017; Robeck et al., 2017). Aliquots (0.01 to 0.025 ml, depending on the sample concentration) of reconstituted extracted dolphin sera were analyzed, in duplicate, according to the kit instructions. Cross reactivity and sensitivity information can be found in the aforementioned publications and kit directional insert. Parallel displacement of dolphin serum compared to the standard curve was demonstrated (r = 0.972) and the recovery of known concentrations of standard to extracted serum was 119.4 ± 3.8% (linear regression, y = 1.21x – 1.03, r2 = 0.999), thereby demonstrating negligible matrix interference in the EIA. Samples were run in duplicate and any sample with a CV > 10% between replicates was repeated. To test for intra-assay precision, a single serum sample was tested at different locations (n = 24) across the microtiter plate, and the CV was 4.1%. Inter-assay CVs for a high and low control, with antibody binding at 20 and 60%, were 5.9 and 11.1%, respectively (n = 27 assays). The inter-assay CV for a cetacean-specific biological control made from a pool of pregnant dolphin sera, with antibody binding at approximately 65%, was 12.4% (n = 27 assays).
Cortisol concentrations were measured using a single antibody, direct EIA as previously described (Munro and Lasley, 1988) and described in detail for use with bottlenose dolphin sera (Steinman et al., 2016). Assay validations for this species and sample matrix (parallelism, recovery) and antibody cross-reactivity and sensitivity information are described in detail in Steinman et al. (2016) and passed validity tests (parallelism, r = 0.994; recovery/accuracy, 94.80 ± 3.24%, linear regression, y = 0.88x + 7, r2 = 0.988, see Steinman et al., 2016). To check for intra-assay variation, samples were run in duplicate and any sample with a CV > 10% between replicates was repeated. Intra-assay precision was previously tested in Steinman et al. (2016) by analyzing a single serum sample at different locations across the microtiter plate, and the CV was < 10%. Inter-assay CVs for two quality controls, with antibody binding at 30 and 70%, were 6.2 and 12%, respectively (n = 31 assays). The inter-assay CV for a cetacean-specific biological control made from a pool of pregnant dolphin sera, with binding at approximately 70%, was 11.4% (n = 31 assays).
Estradiol (E2) concentrations were measured using a single antibody, direct EIA as previously described for use with high performance liquid chromatography (HPLC) fractions for bottlenose dolphin sera (Steinman et al., 2016). Briefly, 0.002 to 0.05 ml (depending on the sample concentration) of bottlenose dolphin sera were analyzed, in duplicate, on the EIA. The remaining steps were the same as described in Steinman et al. (2016), and cross-reactivity and sensitivity information can also be found in this publication. Because we have only previously utilized this E2 assay to analyze bottlenose dolphin sera on HPLC fractions, we performed assay validations for this sample matrix in the present study. Parallel displacement of dolphin sera compared to the standard curve was demonstrated (r = 0.971), and the recovery of known concentrations of standard added to a pool of sera was 108.7 ± 6.1% (linear regression, y = 1.15x – 0.41, r2 = 0.998), thereby demonstrating negligible matrix interference in the EIA. To check for intra-assay variation, samples were run in duplicate and any sample with a CV > 10% between replicates was repeated. To test for intra-assay precision, a single serum sample was tested at different locations (n = 15) across the microtiter plate, and the CV was 9.6%. Inter-assay CVs for a high and low control, with antibody binding at 30 and 70%, were 10.6 and 11.3%, respectively (n = 35 assays). The inter-assay CV for a cetacean-specific biological control made from a pool of pregnant dolphin sera, with antibody binding at approximately 50%, was 11.9% (n = 35 assays).
Estrone and EC (estrone glucuronide and estrone sulfate) concentrations were measured using a single antibody, direct EIA as previously described (Munro et al., 1991) and described in detail for use with bottlenose dolphin sera (Steinman et al., 2016). Assay validations for this species and sample matrix (parallelism, recovery) and antibody cross-reactivity and sensitivity information are described in detail in the aforementioned study (Steinman et al., 2016) and passed validity tests (parallelism, r = 0.978; recovery/accuracy, 74.49 ± 3.08%, linear regression y = 0.84x – 9.89, r2 = 0.996, see Steinman et al., 2016). To check for intra-assay variation, samples were run in duplicate and any sample with a CV > 10% between replicates was repeated. Intra-assay precision was previously tested in Steinman et al. (2016) by analyzing a single serum sample at different locations across the microtiter plate, and the CV was < 10%. Inter-assay CVs for two quality controls, with antibody binding at 30 and 70%, were 9.4 and 12.4%, respectively (n = 37 assays). The inter-assay CV for a cetacean-specific biological control made from a pool of pregnant dolphin sera, with binding at approximately 50%, was 12.3% (n = 37 assays).
Within each animal and samples collected during a reproductive event, the date of ovulation was determined to align samples for analysis (Robeck et al., 2021). For this process, ovulation was determined by either knowing (n = 82) or estimating the date (n = 10). An ovulation date was considered “known” when: ovulation was determined by taking the midpoint between when samples were baseline and when they first became elevated post-ovulation and only relying on this estimation when the maximum time between these two samples was four weeks or less; based on observed estrus followed by elevated P4; ultrasonographic detection of ovulation; or daily urinary hormone analysis. Estimated ovulation dates were only done for normal births and were determined by subtracting the mean gestation length (376 days) for bottlenose dolphins from the date of parturition (O’Brien and Robeck, 2012). For abnormal pregnancies, only samples with known ovulation dates were included in the study (Table 1). Once the ovulation date was determined, the reproductive event period was divided based on stage or month post-ovulation (MPO). For non-pregnant animals, the follicular phase (FOLLICULAR) included any samples collected from one through five days before the day of ovulation (OVULATION) and luteal phase (LUTEAL) samples were samples collected during peak P4 which occurs between ten and 18 days post ovulation (O’Brien and Robeck, 2012).
Stage time periods were based on “trimesters” of a normal pregnancy and divided as follows: EARLY (days 1 to day 120 post-ovulation), MID (days 121 to 240), or LATE (days 241 until parturition). Reproductive event categories were defined as follows: Normal pregnancy (NORM) – a live calf that lived longer than 30 days (n = 217 samples within 27 pregnancies); Failure to thrive (FTT) – a live calf that lived from two to 30 days (n = 108 samples within 17 pregnancies); Perinatal loss (PNL) – a calf born either dead or alive but died prior to 24 h post-partum and had gestated for at least 352 days (the minimum gestation in a bottlenose dolphin that has resulted in the birth of a normal calf, E. Jensen, unpublished data, see Robeck et al., 2021, n = 93 samples within 20 pregnancies); Early loss (EL) – an ultrasound diagnosed, pregnant female that either reabsorbed or passed the conceptus or fetal tissue prior to day 121 (n = 58 samples within 12 pregnancies); and False pregnancy (FP) – a female with elevated P4 longer than a normal luteal phase (> 21 days; O’Brien and Robeck, 2012) without any ultrasonographic evidence of pregnancy (uterine membranes, fluid or conceptus) or was not in the presence of a breeding age male (n = 100 samples within 16 FPs).
Unless stated otherwise, statistical analyses were performed using Stata statistical software (version 16; StataCorp LP, College Station, TX, United States). We initially compared gestation length, age and parity across status categories. These comparisons were made using a two-level restricted maximum likelihood (REML) linear mixed model (LLM) with the dependent variables for each analysis being gestation length, age and parity, and with status as a categorical fixed variable and animal id as a random effects (level 2) variable. A post hoc marginal mean comparison was then made across status categories using a Šidák correction. Additionally, because earlier work indicated that age and parity were highly correlated (O’Brien and Robeck, 2012), we analyzed our data set to determine if this trend continued by performing a pairwise comparison across all data between age and parity using a REML LMM with id set as the random variable. Degrees of freedom adjustments for the small sample size were performed using the Kenward–Roger approximation (Kenward and Roger, 1997).
Hormone concentration comparisons were only made between NORM and one of the other abnormal reproductive events (FTT, PNL, EL, AB, or FP) and the analysis repeated until each potential paired comparison was completed. To compare hormone concentrations during either different stages or months post-conception between NORM and one of the other reproductive events, we used a two (animal id) or three level random effects (pregnancy id) LMM REML regression model (Cnaan et al., 1997; West et al., 2015; Robeck et al., 2017). Two or three level random effects models were compared using the likelihood ratio test and three level models (pregnancies within each animal) were used only if they provided significant improvement over 2 level models using animal ID only (West et al., 2015). Degrees of freedom adjustments for the small sample size were performed using the Kenward–Roger approximation (Kenward and Roger, 1997). For the REML regression models, the dependent variable was hormone and the fixed effect variables (level 1) were status (NORM and one of the other reproductive events) and pregnancy time period (either stage or month), animal age, season and method. Season was defined as samples collected during winter (December through February), spring (March through May), summer (June through August) or fall (September through November). Animal age and season were included as covariates to control for the effect that these variables may have on the different hormone concentrations being evaluated (Steinman et al., 2016; O’Brien et al., 2017; Robeck et al., 2017). In addition to age being previously identified as influencing hormone concentrations in normal pregnancies (O’Brien and Robeck, 2012; Steinman et al., 2016), and despite no apparent differences between animal age within each status group (Table 1), previous research indicated that animal age and not parity was a significant variable associated with pregnancy loss (O’Brien and Robeck, 2012). Although parity could have been added as covariate, its collinearity with age made it inappropriate to include in the model with age and, again, based on previously published results, we decided that age was the more appropriate variable to include in our analysis. We also included a categorical variable “method” which divided sample collection based on date of collection between pre and post Jan 1, 2000. This was an attempt to control for potential differences that may have occurred between collection methods (restraint versus behavioral) and the influence these methods may have had on hormone concentrations. All final mixed effects models were checked for normality using quantile plots of the standard residuals. If quantile-quantile (qnorm) plots of standardized residuals exhibited non-normal distribution then data were log transformed or square root transformed as predicted by the Shapiro–Wilk test (Ladder command, STATA) until residuals were normalized. Paired comparisons of the dependent variable marginal (predicted) means within each reproductive event against normal for each time category were made at a significance of P < 0.05. If appropriate, multiple comparisons of marginal means were performed using Bonferroni corrections at P < 0.05. For text, tables and graphs any transformed data were first back-transformed, and then, all data were presented as marginal means with 95% confidence intervals (CI) unless noted otherwise.
A total of 92 reproductive events and 59 physiologic events (FOLLICULAR, OVULATION or LUTEAL) were identified within 57 females (Tables 1, 2). Some animals had more than one type of reproductive event and, as a result, the total number of animals in Table 1 is greater than the actual number of animals in the study. No significant differences were detected between the mean age or parity of the females within each category of reproductive events and the length of time for EL and FP were significantly reduced compared to each of the other reproductive events (Table 1, Robeck et al., 2021). As would be expected, animal age was significantly (F1,52 = 124, P < 0.0001) associated with animal parity with age increasing at 2.63 ± 0.24 years for each successive reproductive event.
Table 2. Marginal mean (95% CI) hormone concentration (ng/ml) of testosterone (T), androstenedione (A4), cortisol, estradiol (E2) and estrone conjugates (EC) during each stage (EARLY, MID, LATE) of normal pregnancies, the follicular phase (FOLLICULAR), OVULATION and the luteal phase (LUTEAL) in bottlenose dolphins.
All final mixed model statistics for each hormone by time group (stage or month post-conception [MPC]) are presented in Supplementary Tables 1, 2 and statistics for significant variables for analysis by either stage or MPC are listed in Supplementary Tables 3, 4. Significant post hoc marginal mean comparisons are presented within text. Based on standardized residual analysis, all final models used the square-root of T and the logs of A4, cortisol, E2 and EC to provide the best residual fit.
Post hoc marginal mean comparisons of T concentrations between all time periods, pre or post pregnancy, indicated that FOLLICULAR and OVULATION were reduced compared to EARLY pregnancy (Table 2). All three gestational stages increased successively (P < 0.05) over the preceding stage (Table 2) and placental T concentrations were significantly greater than all stages and reproductive states (Table 2). Seasonal differences occurred between winter (1.59 ng/ml, 95% CI = 1.24 – 1.98 ng/ml) and summer (1.05 ng/ml, 95% CI = 0.78 – 1.58 ng/ml) concentrations. For analysis by MPC, T concentrations increased during pregnancy, and peak concentrations occurred during MPC 8 (3.33 ng/ml, 95% CI = 2.51 – 4.27 ng/ml) and 9 (3.96 ng/ml, 95% CI = 3.02 – 5.01 ng/ml, Figure 1).
Figure 1. The marginal predicted 95% CI for testosterone during each month post-conception (MPC) of normal pregnancies as represented by the dark gray shaded portion of the graph and the mean by a solid black line. Line graphs with data points represent each abnormal pregnancy (marginal mean). MPC of abnormal pregnancies (or month post ovulation for false pregnancies) that have mean concentrations that are significantly different from normal are highlighted with an asterisk (*). Data were square root transformed for analysis and then back-transformed for data presentation.
EARLY was reduced (P < 0.05) compared to MID and LATE (Table 2). Placental A4 concentrations were less than EARLY, FOLLICULAR and LUTEAL (Table 2). For analysis by MPC, A4 concentrations increased throughout each MPC, and peak concentrations occurred during MPC 7 (15.3 ng/ml, 95% CI = 11.4 – 20.4 ng/ml) and 8 (18.2 ng/ml, 95% CI = 13.3 – 24.9 ng/ml, Figure 2). Concentrations of A4 collected while under restraint (5.8 ng/ml, 95% CI 4.5 to 7.6 ng/ml) were higher compared to behavioral (4.2 ng/ml, 95% CI 3.7 to 4.7 ng/ml).
Figure 2. The marginal predicted 95% CI for androstenedione during each month post-conception (MPC) of normal pregnancies as represented by the dark gray shaded portion of the graph and the mean by a solid black line. Line graphs with data points represent each abnormal pregnancy (marginal mean). MPC of abnormal pregnancies which have mean concentrations that are significantly different from normal are highlighted with an asterisk (*). Data were log transformed for analysis and then back-transformed for data presentation.
Within NORM, cortisol during EARLY was lower (P < 0.05) compared to LATE (Table 2), and within MPC concentrations peaked in MPC 11 (8.1 ng/ml, 95% CI = 4.6 to 14.0 ng/ml, Figure 3).
Figure 3. The marginal predicted 95% CI for cortisol during each month post-conception (MPC) of normal pregnancies as represented by the dark gray shaded portion of the graph and the mean by a solid black line. Line graphs with data points represent each abnormal pregnancy (marginal mean). MPC of abnormal pregnancies which have mean concentrations that are significantly different from normal are highlighted with an asterisk (*). Data were log transformed for analysis and then back-transformed for data presentation.
Post hoc marginal mean comparisons of E2 concentrations between all time periods, pre or post pregnancy, indicated that placenta was significantly increased compared to all other groups or stages (Table 2). Within NORM, EARLY was reduced (P < 0.05) compared to LATE (Table 2). For seasonal differences, E2 during winter (0.59 ng/ml, 95% CI = 0.41 – 0.85 ng/ml) was significantly higher compared to summer (0.47 ng/ml, 95% CI = 0.33 – 0.67 ng/ml). For data analysis by MPC, no individual MPC was significantly higher (Figure 4).
Figure 4. The marginal predicted 95% CI for estradiol during each month post-conception (MPC) of normal pregnancies as represented by the dark gray shaded portion of the graph and the mean by a solid black line. Line graphs with data points represent each abnormal pregnancy (marginal mean). MPC of abnormal pregnancies which have mean concentrations that are significantly different from normal are highlighted with an asterisk (*). Data were log transformed for analysis and then back-transformed for data presentation.
Post hoc marginal mean comparisons of EC concentrations between all time periods, pre or post pregnancy, indicated LUTEAL was significantly lower compared to MID and LATE, and placental EC was significantly increased compared to all other groups or stages (Table 2). Within NORM, EARLY was reduced (P < 0.05) compared to MID and LATE (Table 2). For analysis by MPC, EC peaked during MPC 10 (Figure 5, Supplementary Table 7).
Figure 5. The marginal predicted 95% CI for estrone conjugates during each month post-conception (MPC) of normal pregnancies as represented by the dark gray shaded portion of the graph and the mean by a solid black line. Line graphs with data points represent each abnormal pregnancy (marginal mean). MPC of abnormal pregnancies which have mean concentrations that are significantly different from normal are highlighted with an asterisk (*). Data were log transformed for analysis and then back-transformed for data presentation.
Marginal effects of T concentrations in FTT animals indicated that EARLY was significantly reduced compared to MID and LATE (Table 3). For season, summer (1.35 ng/ml, 95% CI = 1.01 – 1.73 ng/ml) T concentrations were significantly lower compared to winter (1.99 ng/ml, 95% CI = 1.56 – 2.48 ng/ml). No intra-MPC differences were detected between FTT and NORM (Figure 1).
Table 3. Marginal mean (95% CI) testosterone and androstenedione concentrations (ng/ml) during each stage of normal (live birth) and abnormal bottlenose dolphin pregnancies.
For status across pregnancy, marginal mean A4 concentrations for FTT (6.8 ng/ml, 95% CI = 5.5 to 8.4 ng/ml) were higher (P < 0.05) than NORM (4.5 ng/ml, 95% CI = 3.8 to 45.2 ng/ml). Marginal effects of FTT animals indicated that A4 during EARLY was significantly reduced compared to MID and LATE (Table 3). Intra-stage differences demonstrated that, within EARLY (P = 0.05) and LATE (P = 0.048), A4 concentrations were increased compared to NORM (Table 3). The only difference between FTT and NORM occurred in MPC 9 (Figure 2, Supplementary Table 5).
For status across pregnancy, marginal mean cortisol concentrations for FTT pregnancies (7.2 ng/ml, 95% CI = 4.9 to 10.5 ng/ml) were higher compared to NORM (3.1 ng/ml, 95% CI = 2.3 to 4.3 ng/ml). Marginal effects of FTT animals indicated that cortisol during EARLY was significantly lower compared to LATE (Table 4). Also for FTT, intra-stage differences indicated that cortisol was significantly higher compared to NORM for all gestational stages (Table 4). For seasonal differences, spring (3.29 ng/ml, 95% CI = 2.36 – 4.58 ng/ml) cortisol concentrations were marginally, significantly lower (P = 0.057) compared to winter (5.11 ng/ml, 95% CI = 3.61 – 7.26 ng/ml). Within FTT, cortisol significantly peaked during MPC 12 (Figure 3, Supplementary Table 6). During MPC 7, significant intra-MPC differences occurred between FTT and NORM (Figure 3, Supplementary Table 6).
Table 4. Marginal mean (95% CI) cortisol concentrations (ng/ml) during each stage of normal (live birth) and abnormal bottlenose dolphin pregnancies.
No intra or inter stage differences in E2 concentrations were detected within FTT and between FTT and NORM for both stage and MPC (Table 5 and Figure 4).
Table 5. Marginal mean (95% CI) estradiol and estrone conjugate concentrations (ng/ml) during each stage of normal (live birth) and abnormal bottlenose dolphin pregnancies.
Marginal effects of FTT animals indicated that EC during EARLY was significantly lower compared to LATE (Table 5). No significant intra-stage differences between FTT and NORM were detected for stage and MPC (Table 5 and Figure 5) and within FTT no difference in MPC was found for EC (Figure 5).
Marginal mean concentrations of T during EARLY were lower compared to MID and LATE. No intra-stage or intra-MPC differences were detected between NORM and PNL (Table 3 and Figure 1).
EARLY A4 was significantly lower compared to MID and LATE (Table 3). No intra-stage or intra-MPC differences were detected between NORM and PNL (Table 3 and Figure 2).
For status across pregnancy, marginal mean cortisol during PNL (9.5 ng/ml, 95% CI = 6.2 to 14.5 ng/ml) was increased compared to NORM (3.2 ng/ml, 95% CI = 2.3 to 4.5 ng/ml). Within PNL, marginal effects indicated that EARLY and MID were significantly reduced compared to LATE (Table 4). Intra-stage differences indicated that, for PNL, cortisol concentrations during EARLY and LATE were significantly increased compared to NORM (Table 4). Although season was significant (Supplementary Table 3), no marginal mean seasonal differences were detected. Within PNL, cortisol significantly peaked during MPC 12 (Figure 3, Supplementary Table 6). Significant intra-MPC differences occurred in cortisol concentrations between PNL and NORM during MPC 1 and MPC 10 (Figure 3, Supplementary Table 6).
Across pregnancy, PNL (0.66 ng/ml, 95% CI = 0.46 – 0.96 ng/ml) was increased compared with NORM (0.48 ng/ml, 95% CI = 0.35 – 0.67 ng/ml). Age was found to influence E2, where concentrations decreased at a rate of 1.04 pg/year of age. Concentrations of E2 were increased by 0.21 ng for behavioral sampling versus restraint. Marginal effects for PNL did not detect any differences between each stage or MPC (Table 5) or intra-stage or intra-MPC differences between PNL and NORM (Table 5 and Figure 4).
Across pregnancy, within PNL, EC was increased (0.78 ng/ml, 95% CI = 0.52 – 1.15 ng/ml) compared with NORM (0.56 ng/ml, 95% CI = 0.39 – 0.80 ng/ml). EC concentrations decreased at a rate of 1.03 pg/year of age. Marginal effects for PNL indicated that stage EARLY was significantly reduced compared to stage LATE (Table 5), but there were no intra-stage or intra-MPC differences between PNL and NORM (Table 5 and Figure 5).
For EL, T concentrations were higher during EARLY compared to NORM (Table 3). However, within EARLY, no significant differences were detected for any independent variables. Although T had a significant peak within EL during MPC 1 (Supplementary Table 4), no intra-month differences were detected between EL and NORM (Figure 1).
No significant inter-MPC changes in A4 were detected within EL, and no intra-month differences between EL and NORM were detected (Figure 2).
For age, cortisol concentrations decreased by 0.05 ng/year of age. Within method, restraint resulted in a significant increase in cortisol concentrations (5.72 ng/ml, 95% CI = 3.6 to 12.4 ng/ml) compared to behavioral collection methods (2.5 ng/ml, 95% CI = 1.7 to 3.7 ng/ml). For MPC, no significant effects were detected (Figure 3).
Within EARLY stage, EL was decreased compared to NORM (Table 5). For MPC, no significant effects were detected (Figure 4).
Within stage EARLY, no variables were significant (Table 5, Supplementary Table 4). For MPC, no intra-month comparisons were different (Figure 5).
Both MID and LATE FP stages were significantly reduced compared to NORM (Table 3). T concentrations in FP were significantly reduced from NORM by MPO/MPC 7 and beyond (Figure 1, Supplementary Table 5).
For method, A4 concentrations from samples collected during restraint (4.3 ng/ml, 95% CI = 3.3 to 5.6 ng/ml) were increased compared to behavioral sample collection (3.1 ng/ml, 95% CI = 2.8 to 3.5 ng/ml). Concentrations of A4 during MID and LATE stages were significantly reduced compared to NORM (Table 3). For MPO comparisons, A4 was significantly higher for NORM from MPO 6 through 9 compared to FP (Figure 2, Supplementary Table 5).
Post hoc marginal mean comparisons of cortisol concentrations within FP or between FP and NORM did not detect any differences between stages or MPO (Table 4 and Figure 3).
Post hoc marginal mean analysis indicated that E2 concentrations were lower (P ≤ 0.05) across FP (0.34 ng/ml, 95% CI = 0.24 to 0.48 ng/ml) compared to NORM (0.53 ng/ml, 95% CI = 0.39 to 0.72 ng/ml) and within MID and LATE stages. Concentrations during summer (0.41 ng/ml, 95% CI = 0.30 to 0.56 ng/ml) were lower (P ≤ 0.05) compared to winter (0.54 ng/ml, 95% CI = 0.4 to 0.74 ng/ml). Both MID and LATE stages were significantly lower during FP compared to NORM (Table 5). Significant differences between NORM and FP were detected by MPC 9 (Figure 4, Supplementary Table 7).
Post hoc marginal mean analysis indicated that EC concentrations were lower (P ≤ 0.05) across FP (0.38 ng/ml, 95% CI = 0.26 to 0.55 ng/ml) compared to NORM (0.69 ng/ml, 95% CI = 0.49 to 0.98 ng/ml). All stages of FP were significantly reduced compared to NORM (Table 5). Post hoc marginal analysis indicated overall FP was lower (P < 0.0001) compared to NORM. MPO months 1, 3 and 5 were (P ≤ 0.05) reduced during FP compared to NORM (Figure 5, Supplementary Table 7).
Our results show that, during abnormal pregnancy, there are some deviations in hormone concentrations (elevated or reduced) from normal pregnancy ranges for androgens, cortisol and estrogens. There were significant differences in androgen concentrations for some pregnancies with poor reproductive outcomes, including FTT and EL as well as FP, when data were analyzed by stage and/or month post-conception. Differences in cortisol measurements from normal pregnancy were only apparent in PNL during the EARLY and LATE stages and at all stages in FTT. Estrogen concentrations were different from normal pregnancy during EL and FP only. Seasonal influences on hormone concentrations were evident for T and E2 and, to a lesser degree, cortisol. Age primarily influenced estrogens during PNL and cortisol during EL, whereby hormone concentrations decreased with age. Our results demonstrate a more extensive panel of hormone tests, and not just progesterone, can provide more information about the overall health of pregnancy in the bottlenose dolphin.
Longitudinal patterns of circulating T, cortisol and EC during normal pregnancies were similar to our prior results (Steinman et al., 2016). Our results demonstrated that A4 had a similar trend to T during normal pregnancies with MID and LATE stages higher compared to EARLY, peaked during MPCs 7 and 8 then decreased until parturition. Our results support past research using LCMS-MS that has shown that A4 increases in a quadratic fashion with concentrations similar in MID and LATE stage during normal pregnancy and concentrations for both stages elevated compared to EARLY (Legacki et al., 2020). Increases of A4 during pregnancy have also been observed in the killer whale (Robeck et al., 2017; Legacki et al., 2020) and the beluga (Legacki et al., 2020), as well as humans (see review in Kuijper et al., 2013).
The longitudinal gestational profile of A4 was visually similar to T. In the killer whale, the peak in A4 concentrations precedes the T peak (MPCs 13 and 14, respectively) and is suggested to be related to their metabolic relationship where A4 is converted to T and estrogens via aromatization in the ovary or placenta (Robeck et al., 2017). Similarly, in our study, peak A4 (MPCs 7 and 8) preceded peak T (MPC 8 and 9). Concentrations of A4 were higher than T during all stages of normal pregnancy despite the larger cross-reactivity with other androgens (in particular dihydrotestosterone) for our T EIA. The same was observed when analyzed by LCMS-MS (Legacki et al., 2020). Because estrogens increase in late gestation in the bottlenose dolphin (Steinman et al., 2016), it is possible that more A4 would need to be biologically available for conversion to these other reproductive hormones and could explain the higher concentrations of A4 compared to T (Steinman et al., 2016).
Elevated androgen concentrations during pregnancy have been reported in the bottlenose dolphin (Boggs et al., 2019; Galligan et al., 2020) as well as other cetacean species (Robeck et al., 2017 and Wasser et al., 2017 [killer whale]; Richard et al., 2017 and Legacki et al., 2020 [beluga]; Rolland et al., 2005 and Corkeron et al., 2017 [North Atlantic right whale]; Hunt et al., 2019 [humpback whale]). Whether the source of androgens during bottlenose dolphin pregnancy is maternal or fetal-placental derived is unknown. However, the lower androgen concentrations we observed during FP compared to normal pregnancy indicates that these increases in androgens are pregnancy specific. In the killer whale, comparison of maternal serum and cord blood (representative of the placenta) has demonstrated that T, but not A4, is significantly higher in placental versus maternal sources (Robeck et al., 2017). This alone could imply that the major source of T is the fetus or placenta, while A4 is maternally derived. However, hormones may accumulate on one side of the fetal-maternal barrier due to preferential binding with proteins (Silberzahn et al., 1984). For the bottlenose dolphin, we found similar concentrations of both placental T and A4 as compared to maternal sources during LATE pregnancy. Despite this, we do not know for certain if androgens concentrations are higher in maternal versus placental sources or whether they concentrate in certain areas of the fetal-maternal unit due to other reasons.
The present study confirmed our previous findings of increased cortisol during late pregnancy in the bottlenose dolphin during NORM (Steinman et al., 2016). Increases in circulating GC or excretory metabolite concentrations during pregnancy have been documented in killer whales (Robeck et al., 2017), North Atlantic right whales (Hunt et al., 2006; Corkeron et al., 2017), humpback whales (Hunt et al., 2019) and blue whales (Valenzuela-Molina et al., 2018). Increases in circulating or excreted GC measures during pregnancy have been reported in several other wildlife species, including New and Old World primates, spotted hyenas (Crocuta crocuta), African elephants (Loxodonta africana) and dugongs (see review in Edwards and Boonstra, 2018). Glucocorticoid increases during late gestation are essential for maturation of the fetus in preparation for survival outside the uterine environment (Fisher, 1986), especially respiratory system development and maturation. Additionally, GC increases are also expected as part of the cascade of events required for induction of parturition in the cow (Adams and Wagner, 1970) and elephants (Meyer et al., 2004). Thus, it is unsurprising to see elevations of circulating GCs during LATE pregnancy in the bottlenose dolphin.
Concentrations of E2 increased throughout pregnancy, and placental E2 and EC measurements were the highest compared to all other maternal estrogen sources and reproductive stages (Table 2). Estrogens play an important role in the initiation and regulation of labor (Gibb et al., 2006). Thus, the late stage increases in estrogens that we observed may also be important for these processes. However, we did not have many samples within the immediate peri-parturient window that may have revealed more information regarding estrogen dynamics and its relationship with parturition. Our results in the present study with respect to EC also corroborated our previous findings (Steinman et al., 2016). We further were able to determine that the placenta is a major source of estrogens in bottlenose dolphin pregnancy. In primates, estrogens are necessary for stimulation of vascular endometrial growth factor and blood vessel growth in the placenta (Albrecht and Pepe, 2010). Hence, it is possible that the placental estrogens play a similar role in cetaceans.
Testosterone concentrations and patterns were similar between FTT and normal pregnancy (Tables 2, 3 and Figures 1, 2), but within A4 measurements, concentrations during EARLY and LATE were higher during FTT pregnancies. In humans, the influence of androgens on birth weight is more pronounced during the second trimester, where observations of elevated T during week 17 of gestation are associated with intrauterine growth restriction (Carlsen et al., 2006) and elevated A4 during the first trimester of pregnancy is associated with pre-eclampsia (pregnancy induced hypertension, see review in Kuijper et al., 2013). However, spontaneous pre-eclampsia is believed to be limited to human and non-human primate pregnancies and does not occur in other mammals (Berkane et al., 2017). Whether the differences in A4 concentrations we observed were an indicator of a potential problem or a result of chance remains to be determined. Evidence suggests that the majority (71%) of calf deaths after the first 24 h are related to failure of passive transfer and malnutrition, both of which are typically associated with poor or no nursing (Sweeney et al., 2010). Although the inability of a calf to nurse is multifactorial and can be influenced by dam abnormalities (e.g., due to illness or inability to aid the calf in learning to nurse), physically weak or immature calves due to gestational growth restriction (Osborn et al., 2012; Robeck et al., 2012) could potentially fall into this abnormal reproductive outcome group.
Cortisol was increased during all stages of FTT pregnancies, with the marginal mean concentrations two to three-fold higher than normal pregnancy. In wild killer whales, fecal GC metabolites are increased in unsuccessful pregnancies compared to successful ones (Wasser et al., 2017). Cortisol increases during the LATE stage of pregnancy play a role in maturation of the fetus for its survival outside the uterine environment (Fisher, 1986). However, excessive cortisol may be detrimental. In humans, increased cortisol during pregnancy is associated with early onset of labor and low birth weight (Austin and Leader, 2000). In the present study, there was no difference in the gestation lengths of FTT pregnancies compared to normal pregnancies (Table 1) suggesting that premature labor was likely not, or only minimally, a contributing factor to neonatal survival rates in the bottlenose dolphin. Birth weight and length are typically not determined in bottlenose dolphin calves so post-natal weight and length are only estimates, thus, it is unknown if there is an association with low birth weight and FTT pregnancies. Increased maternal cortisol in human pregnancy has also been shown to affect infant cognitive development suggesting that excess cortisol may have a programing influence on fetal development (Davis and Sandman, 2010). The placental enzyme 11β-hydroxysteroid dehydrogenase type 2 (11β-HSD2), regulates cortisol influence on the fetus by converting it to its inactive form, cortisone (Beitens et al., 1973). This enzyme increases during pregnancy then decreases toward the end of gestation to permit passage of more cortisol to the fetus to aid in late term organ development (Austin and Leader, 2000). However, 11β-HSD2 is only a partial barrier, and fetal cortisol measures are closely correlated with maternal concentrations (Gitau et al., 1998, 2001); consequently, excess maternal cortisol could have detrimental effects on the developing fetus. Investigations of 11β-HSD2 during pregnancy is limited to humans and rats (see review in Edwards and Boonstra, 2018), and any gestational effects of 11β-HSD2 regulation on other mammalian and wildlife species is unknown. Nonetheless, it is possible that the excess maternal cortisol we observed during FTT pregnancies had a negative influence on the developing fetus that did not affect the length of gestation but instead manifested negatively in neonate health. In laboratory rodent models, maternal stress negatively impacts both offspring growth and behavior (Meaney et al., 2007) while in snowshoe hares (Lepus americanus), elevated maternal fecal GC metabolites due to heightened predation risk results in lower reproductive output (less offspring) as well as lower quality offspring (Sheriff et al., 2009). In meerkats who have a cooperative breeding-social hierarchy structure, subordinate females who have been evicted from their social group while pregnant demonstrate elevated fecal GC metabolites. These subordinate females have lower reproductive function and are more likely to abort pregnancies (Young et al., 2006). As mentioned before with androgens, it is difficult to determine if poor nursing is due to maternal or calf causes, or a combination thereof. However, possible detrimental cognitive developmental effects on fetal programing associated with elevated maternal cortisol (Davis and Sandman, 2010) could explain poor nursing behavior on part of the calf.
For this study, we had a sufficient number of pregnancies and reproductive outcomes to be able to separate FTT pregnancies into its own category. Although there could be many different causes for neonatal mortality in the bottlenose dolphin, cortisol and possibly A4 analysis may be able to identify pregnancies with calves that are at risk. This information could improve calf survival by increasing post-natal monitoring of at risk calves to allow for rapid medical intervention if necessary. Furthermore, in the larger context of analyzing causes of reproductive failure in the bottlenose dolphin on a population level (e.g., Deepwater Horizon oil spill, Kellar et al., 2017), recognition of possible FTT pregnancies via hormone analysis may provide more insight into the possibility of differing effects of the environment or anthropogenic influences, both short and long term, on bottlenose dolphin pregnancy. The ability to identify at risk or compromised pregnancies over the short term can possibly be linked to long term reproductive failure and the overall health status of a population. Hence, when possible, reproductive studies should include FTT as a separate analytical category.
Perinatal loss included stillborn calves (calves born dead) as well as calves born live but died within 24 h. For bottlenose dolphins under human care, PNL rates of 5.2% and 11.5% have been reported (Sweeney et al., 2010; Robeck et al., 2021). During PNL in bottlenose dolphins, reduced concentrations of hormones have been reported for P4 (Bergfelt et al., 2011), relaxin (Bergfelt et al., 2017), total and free thyroxine (West et al., 2014) and PGs (Robeck et al., 2021). Across pregnancy, we found increased concentrations of cortisol and estrogens for PNL. When analyzed by stage, cortisol was four and three-fold higher during EARLY and LATE stages, respectively, but estrogens were not statistically different at the stage or MPC level. In pregnant ewes administered corticosteroids, a trend of increased circulating estrone has been demonstrated and indicates increased cortisol may stimulate placental production of estrogens (Keller-Wood et al., 2014). This may explain our observations of significant (P = 0.044 and 0.049 for E2 and EC, respectively) increased estrogens across PNL pregnancies.
In contrast with our findings, Kellar et al. (2017) has shown that blubber cortisol had no influence on the reproductive success rate on a population of bottlenose dolphins following the Deepwater Horizon event. However, that study only relied on single sample analysis and the timing of sample collection could have affected their results. Furthermore, cortisol concentrations may have been elevated for the population as a whole because of the effects of the oil spill, and discrete changes in cortisol concentrations may have been missed or dampened as a result. It has been reported that in pregnant ewes administered hydrocortisone to increase circulating maternal cortisol concentrations, there is a higher incidence of stillbirth and fetal death but no evidence of changes to uterine blood flow, placental hormone concentrations or birth weight (Keller-Wood et al., 2014). They suggest the relationship between poor reproductive outcome and increased maternal cortisol may be a result of cortisol metabolism by the dam and/or fetus (Keller-Wood et al., 2014). Additionally, in humans, late term fetal loss associated with elevated cortisol may result in changes to fetal cardiac function or size (Trainer, 2002). Our findings indicate there are potential negative influences of elevated cortisol outside of the normal pregnancy reference range on the feto-placental unit during pregnancy.
Like FTT, there was no difference in mean gestation length for PNL compared to normal pregnancy, so it is unlikely that increased cortisol resulted in premature labor for PNL in our study. We also assume that the major contributing factor in bottlenose dolphin stillbirth is dystocia or a prolonged labor (Robeck et al., 2021), and that dystocia may be associated with calf size (Robeck et al., 2012). Although dystocia is associated with increased cortisol at parturition in buffaloes (Sathya et al., 2007), we did not have many samples collected immediately prior to parturition (n = 3 and 5 for normal and PNL, respectively), so the elevated cortisol concentrations we observed during the late stage with respect to PNL were most likely not associated with any possible dystocia related influences. Continued efforts to collect and analyze samples in close temporal relationship to parturition would help answer some of these questions.
Like what has been reported with P4, where concentrations are increased above normal pregnancy concentrations during the EARLY stage in EL (Robeck et al., 2021), we also observed an increase above normal pregnancy for T. Hyperandrogenemia, especially during early pregnancy, is a contributor to recurrent pregnancy loss in humans (Okon et al., 1998), and elevated T and A4 are associated with preeclampsia, a condition in which the placenta may be small or damaged most likely due to abnormal blood vessel development in the placenta during early pregnancy (Troisi et al., 2003). Elevated androgens may be responsible for miscarriages due to implantational defects, possibly, because T can prevent endometrial estrogen-related gene expression (Kowalski et al., 2004). This may explain our observations of reduced estradiol in EL. Because androgens may be placental or fetal-derived, perhaps, the earlier than expected increase in T during EL, in combination with or separate from increased P4 during the same time, may be a signal of compromised placental or fetal health, e.g., chromosomal defects that can be maternally recognized. This maternal recognition may then lead to termination of a pregnancy with a high likelihood of a poor outcome. Causes of EL are largely unknown in the bottlenose dolphin, and in most instances, no placental or fetal tissue that can be examined for defects is expelled. Consequently, there is little that can be done to prevent this occurrence or examine it in detail.
The phenomenon of FP in cetaceans has not been well documented. Diagnosis of FP has relied on consistent (monthly or semi-monthly) monitoring of serum P4/PGs in combination with ovarian and uterine ultrasonography once elevations in these concentrations have exceeded the known luteal phase length for the species. To our knowledge, incidences of FP have been reported in three cetacean species: bottlenose dolphin (Sawyer-Steffan et al., 1983; Kirby and Ridgway, 1984; Yoshioka et al., 1986; Kirby, 1990; Robeck et al., 2021); killer whale (Robeck et al., 2018); and the false killer whale (Pseudorca crassidens, Robeck et al., 1994; Atkinson et al., 1999). Although FP has been previously reported in the bottlenose dolphin, the frequency of this occurrence is unknown. This highlights the importance of routine sampling and hormone monitoring with follow up ultrasonography in cases where the duration of P4/PG elevation exceeds the known luteal phase interval to provide more data and, hence, insight into this reproductive phenomenon.
Androgen and estrogen concentrations were remarkably different for FP compared to normal pregnancy with concentrations lower during MID and LATE stages and some corresponding MPCs for both T and A4. For the reproductive outcomes analyzed, hormone concentration differences from normal pregnancy were most evident for FP. The mean length of FP in the present study was 178 days, approximately 6 months. This is in agreement with past studies in bottlenose dolphins that have reported extended luteal phases/FPs lasting 5 to 6 months without evidence of pregnancy (Sawyer-Steffan et al., 1983; Kirby and Ridgway, 1984; Yoshioka et al., 1986; Kirby, 1990). As a result of this shortened length of FP compared to normal pregnancy, any influence of FP on androgen and estrogen concentrations would be expected to be minimal beyond this period, i.e., MID stage, after termination of the FP. Fecal androgen metabolite analysis in combination with pregnanediol glucuronide measures have been able to differentiate between pregnancy and FP in polar bears (Ursus maritimus, Stoops et al., 2012). In dogs, elevated E2 and reduced EC concentrations compared to pregnancy have been observed for FP (Chakraborty, 1987), but androgens were not distinguishable between the two states (Concannon and Castracane, 1985). However, unlike the bottlenose dolphin, in dogs, androgens peak in early pregnancy (within the first 20 days of a 60–65 days of gestation), so discrete changes between the two reproductive states may be missed during such a small window of time (Concannon and Castracane, 1985). The differences in androgens and estrogens we observed between normal pregnancy and FP indicates testing for these reproductive hormone classes may help distinguish between the two reproductive outcomes. Furthermore, because of the absence of a placenta and fetus in a FP, any androgen measurements during the FP would mainly be of CL or ovarian origin and supports the theory that the source of increased androgens we measured during actual pregnancies are most likely placental or fetal, and not maternally derived. When compared to FP, the estrogen increase observed during MID and LATE stages in normal pregnancy as well as placental concentrations suggests these increases are largely due to pregnancy and, as mentioned previously, are a good measure for distinguishing between the reproductive conditions.
Past work by our group has demonstrated that PG concentrations were better at differentiating between the two conditions compared to P4; during FP, PG concentrations were reduced compared to normal pregnancy by three months post-ovulation, whereas P4 was not until month nine (Robeck et al., 2021). Based on our past and present results, for pregnancy/false pregnancy confirmation, a hormone panel should include P4, PG, androgens and estrogens. The ability to discriminate between pregnancy and false pregnancy using a suite of hormone tests would be extremely useful for health assessments in wild populations where only a single sample may be collected and would decrease incorrect diagnoses of animals as pregnant. Pregnancy diagnosis based solely on P4 analysis is likely not able to detect differences between the two reproductive states.
Little to no seasonal effect was noted across all hormones and reproductive states. The exceptions within these hormones and reproductive states were found with testosterone (normal and FTT), estradiol (normal and false pregnancy) and marginally for cortisol (FTT). This supports previous studies that have also found little to no influence of season on delphinid hormone concentrations during and outside of pregnancy (St. Aubin et al., 1996; Steinman et al., 2016; Biancani et al., 2017; Robeck et al., 2017). We found no influence of age on reproductive outcome (Table 1). This was unexpected because earlier work in bottlenose dolphins demonstrated that animals older than 25 had higher incidences of failed pregnancies (AB) (O’Brien and Robeck, 2012). However, the previous study focused primarily on early loss (EL, < 120 days of pregnancy) and, therefore, the results and distribution of animals is not directly comparable to this study. Nonetheless, age did have some effect on hormone concentrations in the present study, including E2 and cortisol, but only during abnormal pregnancies. For cortisol, only concentrations during EL decreased with age. Additionally, and similar to our previous work (Steinman et al., 2016), we also found an association of age and estrogen concentrations whereby both E2 and EC decreased with age during PNL. However, in the killer whale, a closely related delphinid, no association of age and estrogen measurement during normal pregnancies has been found (Robeck et al., 2016). Whether the influence of age on estrogens is exclusively limited to PNL in the bottlenose dolphin or was an anomaly within our subjects remains to be determined and should be studied further. Apart from studies by our group in the bottlenose dolphin (O’Brien and Robeck, 2012; Steinman et al., 2016) and the killer whale (Robeck et al., 2016, 2017, 2018), the influence of age within pregnancy has not been reported. Accurate ages may be difficult to obtain in in situ settings but should be included in data analyses when available. Although bottlenose dolphins can give birth into their 40s (Dudley, 2008), most do not, and increased EL may be evidence of declining fertility with age or reproductive senescence. The various mechanisms for increased EL in the bottlenose dolphin are unknown but have been hypothesized to be related to a decrease in oocyte quality and numbers as has been observed in other mammalian species (O’Brien and Robeck, 2012; Agenor and Battacharya, 2015; Satué and Gardon, 2016; Cimadomo et al., 2018). Nonetheless, because population recruitment relies largely on the fitness of the dam, the influence of age on reproductive success needs to be evaluated. Because age and parity were correlated but parity was not associated with early loss in a previous study (O’Brien and Robeck, 2012) and because parity’s collinearity with age was not well-suited to our model, we did not include parity as covariate. As a result, although unlikely, parity may still have had an influence on hormone concentrations that went undetected.
Sample collection method (under restraint versus behavioral) had limited influence on hormone concentrations. Because it was impossible to know if samples collected prior to Jan 1, 2000, were collected with manual restraint or behavioral conditioning, despite our best efforts to uncover this information, we do not know for certain if all samples designated as manual restraint were actually collected under those circumstances. As a result, it is likely that some samples designated as restraint were collected behaviorally. Our intention with examining collection method as a variable was to determine if this could have influenced hormone concentrations. Our results indicated that cortisol concentrations increased under restraint during EL only, while A4 measurements were influenced during FP. The increase in A4 could be a result of androgen production by the adrenal glands, as has been observed in non-human primates (Möhle et al., 2002). Regardless, it appears that collection method had minimal effect on our results, but sample collection methods, if potentially stressful, should be included as an analytical variable, especially for in situ studies where animals are not habituated to restraint, but restraint is likely the only option for blood collection.
Based on our results, a suite of pregnancy specific hormone biomarkers for bottlenose dolphin pregnancy should include cortisol, androgens and estrogens. Cortisol measurements may be used to identify FTT and PNL pregnancies during EARLY (MPC 1) and LATE (MPC 10) stages, while A4 analysis may also be able to identify FTT outcomes during EARLY and LATE (MPC 9). For EL, T analysis during EARLY pregnancy may be able to identify EL before it occurs, and steps can be taken to increase monitoring the health of a female. And for FP, co-measurements of androgens (T and A4) and estrogens (E2 and EC), especially during MID (MPO 7, 8) and LATE (MPO 9) stages should be able to distinguish FP from normal pregnancy. These results also highlight the need for consistent, serial sampling during cetacean pregnancy when possible. Increased serial, longitudinal hormone monitoring throughout gestation in the bottlenose dolphin could possibly identify problematic pregnancies and increased observations and study of these animals could also provide more insight into poor reproductive outcomes in this species. Significant changes in hormone concentrations were also revealed when data were analyzed by MPC/MPO in addition to pregnancy stage. These discrete changes may have been missed without the frequent sampling that occurred within our subjects. Finally, this study illustrates the importance of investigating other non-progestagen biomarkers of pregnancy in cetaceans.
The original contributions presented in the study are included in the article/Supplementary Material, further inquiries can be directed to the corresponding author/s.
The animal study was reviewed and approved by SeaWorld Parks and Entertainment Incorporated Research Review Committee. Written informed consent was obtained from the owners for the participation of their animals in this study.
KS and TR contributed equally to the manuscript. GM contributed to sample collection, manuscript preparation, writing and editing. All authors contributed to the article and approved the submitted version.
This project was funded by SeaWorld Parks and Entertainment, Inc. The funder had no role in study design, data collection and analysis, decision to publish or preparation of manuscript.
The authors were employed by SeaWorld Parks and Entertainment Inc.
All claims expressed in this article are solely those of the authors and do not necessarily represent those of their affiliated organizations, or those of the publisher, the editors and the reviewers. Any product that may be evaluated in this article, or claim that may be made by its manufacturer, is not guaranteed or endorsed by the publisher.
The authors wish to thank the SeaWorld trainers, veterinary and animal care staff who helped facilitate this study. Technical assistance for hormone assays was provided by Species Preservation Laboratory research technicians Amanda McDonnell, Jacqueline Posy, and intern Miranda Neumann. This is a SeaWorld Parks and Entertainment contribution number 2021-09.
The Supplementary Material for this article can be found online at: https://www.frontiersin.org/articles/10.3389/fmars.2021.737926/full#supplementary-material
Adams, W. M., and Wagner, W. C. (1970). The role of corticoids in parturition. Biol. Reprod. 3, 223–227. doi: 10.1093/biolreprod/3.2.223
Agenor, A., and Battacharya, S. (2015). Infertility and miscarriage: common pathways in manifestation and management. Womens Health 11, 527–541. doi: 10.2217/whe.15.19
Albrecht, E. D., and Pepe, G. J. (2010). Estrogen regulation of placental angiogenesis and fetal ovarian development during primate pregnancy. Int. J. Dev. Biol. 54, 397–408. doi: 10.1387/ijdb.082758ea
Atkinson, S., Combelles, C., Vincent, D., Nachtigall, P., Pawloski, J., and Breese, M. (1999). Monitoring of progesterone in captive female false killer whales, Pseudorca crassidens. Gen. Comp. Endocrinol. 115, 323–332. doi: 10.1006/gcen.1999.7319
Austin, M. P., and Leader, L. (2000). Maternal stress and obstetric and infant outcomes: epidemiological findings and neuroendocrine mechanisms. Aust. N. Z. J. Obstet. Gynaecol. 40, 331–337. doi: 10.1111/j.1479-828x.2000.tb03344.x
Beitens, I. Z., Bayard, F., Ances, I. G., Kowarski, A., and Migeon, C. J. (1973). The metabolic clearance rate, blood production, interconversion and transplacental passage of cortisol and cortisone in pregnancy near term. Pediatr. Res. 7, 509–519. doi: 10.1203/00006450-197305000-00004
Bergfelt, D. R., Blum, J. L., Steinetz, B. G., Steinman, K. J., O’Brien, J. K., and Robeck, T. R. (2017). Relaxin as a hormonal aid to evaluate pregnancy and pregnancy loss in bottlenose dolphins (Tursiops truncatus). Gen. Comp. Endocrinol. 242, 24–29. doi: 10.1016/j.ygcen.2015.12.024
Bergfelt, D. R., Steinetz, B. G., Lasano, S., West, K. L., Campbell, M., and Adams, G. P. (2011). Relaxin and progesterone during pregnancy and the post-partum period in association with live and stillborn calves in bottlenose dolphins (Tursiops truncatus). Gen. Comp. Endocrinol. 170, 650–656. doi: 10.1016/j.ygcen.2010.12.002
Berkane, N., Liere, P., Oudinet, J. P., Hertig, A., Lefevre, G., and Pluchino, N. (2017). From pregnancy to preeclampsia: a key role for estrogens. Endocr. Rev. 38, 123–144. doi: 10.1210/er.2016-1065
Biancani, B., DaDalt, L., Gallina, G., Capolongo, F., and Gabai, G. (2017). Fecal cortisol radioimmunoassay to monitor adrenal gland activity in the bottlenose dolphin (Tursiops truncates) under human care. Mar. Mamm. Sci. 33, 1014–1034. doi: 10.1111/mms.12424
Boggs, A. S. P., Ragland, J. M., Zolman, E. S., Schock, T. B., Morey, J. S., Galligan, T. M., et al. (2019). Remote blubber sampling paired with liquid chromatography tandem mass spectrometry for steroidal endocrinology in free-ranging bottlenose dolphins (Tursiops truncatus). Gen. Comp. Endocrinol. 281, 164–172. doi: 10.1016/j.ygcen.2019.06.006
Carlsen, S., Jacobsen, G., and Romundstad, P. (2006). Maternal testosterone levels during pregnancy are associated with offspring size at birth. Eur. J. Endocrinol. 155, 365–370. doi: 10.1530/eje.1.02200
Chakraborty, P. K. (1987). Reproductive hormone concentrations during estrus, pregnancy, and pseudopregnancy in the Labrador bitch. Theriogenology 27, 827–870. doi: 10.1016/0093-691X(87)90205-6
Cimadomo, D., Fabozzi, G., Vaiarelli, A., Ubaldi, N., Ubaldi, F. M., and Rienzi, L. (2018). Impact of maternal age on oocyte and embryo competence. Front. Endocrinol. 9:327. doi: 10.3389/fendo.2018.00327
Cnaan, A., Laird, N. M., and Slasor, P. (1997). Using the general linear mixed model to analyze unbalanced repeated measures and longitudinal data. Stat. Med. 16, 2349–2380. doi: 10.1002/(sici)1097-0258(19971030)16:20<2349::aid-sim667>3.0.co;2-e
Concannon, P. W., and Castracane, V. D. (1985). Serum androstenedione and testosterone concentrations during pregnancy and nonpregnant cycles in dogs. Biol. Reprod. 33, 1078–1083. doi: 10.1095/biolreprod33.5.1078
Corkeron, P., Rolland, R. M., Hunt, K. E., and Kraus, S. D. (2017). A right whale poo-tree: classification trees of faecal hormones identify reproductive states in North Atlantic right whales (Eubalaena glacialis). Conserv. Physiol. 5:cox006. doi: 10.1093/conphys/cox006
Dalle Luche, G. D., Boggs, A. S. P., Kucklick, J. R., Groß, J., Hawker, D. W., and Nash, S. B. (2020). Androstenedione and testosterone but not progesterone are potential biomarkers of pregnancy in humpback whales (Megaptera novaeangliae) approaching parturition. Sci. Rep. 10:2954. doi: 10.1038/s41598-020-58933-4
Davis, E. P., and Sandman, C. A. (2010). The timing of prenatal exposure to maternal cortisol and psychosocial stress is associated with human infant cognitive development. Child Dev. 81, 131–148. doi: 10.1111/j.1467-8624.2009.01385.x
Dudley, M. (2008). Association of Zoos and Aquariums, North America Region Bottlenose Dolphin Studbook. San Diego, CA: SeaWorld.
Edwards, P. D., and Boonstra, R. (2018). Glucocorticoids and CBG during pregnancy in Mmammals: diversity, pattern, and function. Gen. Comp. Endocrinol. 259, 122–130. doi: 10.1016/j.ygcen.2017.11.012
Fisher, D. A. (1986). The unique endocrine milieu of the fetus. J. Clin. Invest. 78, 603–611. doi: 10.1172/JCI112616
Forar, A. L., Gay, J. M., and Hancock, D. D. (1995). The frequency of endemic fetal loss in dairy cattle: a review. Theriogenology 43, 989–1000. doi: 10.1016/0093-691x(95)00063-e
Galligan, T. M., Boggs, A. S. P., Balmer, B. C., Rowles, T., Smith, C. R., Townsend, F., et al. (2020). Blubber steroid hormone profiles as indicators of physiological state in free-ranging common bottlenose dolphins (Tursiops truncatus). Comp. Biochem. Physiol. A Physiol. 239:110583. doi: 10.1016/j.cbpa.2019.110583
Gibb, W., Lye, S. J., and Challis, J. R. G. (2006). “Parturition,” in Physiology of Reproduction, ed. J. D. Neill (Cambridge, MA: Academic Press), 2925–2974.
Gitau, R., Cameron, A., Fisk, N., and Glover, V. (1998). Fetal exposure to maternal cortisol. Lancet 352, 707–708. doi: 10.1016/s0140-6736(05)60824-0
Gitau, R., Fisk, N., Teixerira, J., Cameron, A., and Glover, V. (2001). Fetal hypothalamic- pituitary-adrenal stress responses to invasive procedures are independent of maternal responses. J. Clin. Endocrinol. Metab. 86, 104–109. doi: 10.1210/jcem.86.1.7090
Hao, Y. J., Chen, D. Q., Zhao, Q. Z., and Wang, D. (2006). Serum concentrations of gonadotropins and steroid hormones of Neophocaena phocaenoides asiaeorientalis in middle and lower regions of the Yangtze river. Theriogenology 67, 673–680. doi: 10.1016/j.theriogenology.2006.06.014
Hunt, K. E., Robbins, J., Buck, C. L., Bérubé, M., and Rolland, R. M. (2019). Evaluation of fecal hormones for noninvasive research on reproduction and stress in humpback whales (Megaptera novaeangliae). Gen. Comp. Endocrinol. 280, 24–34. doi: 10.1016/j.ygcen.2019.04.004
Hunt, K. E., Rolland, R. M., Kraus, S. D., and Wasser, S. K. (2006). Analysis of fecal glucocorticoids in the North Atlantic right whale (Eubalaena glacialis). Gen. Comp. Endocrinol. 148, 260–272. doi: 10.1016/j.ygcen.2006.03.012
Ivancic, M., Gomez, F. M., Musser, W. B., Barratclough, A., Meegen, J. M., Waitt, S. M., et al. (2020). Ultrasonographic findings associated with normal pregnancy and fetal well-being in the bottlenose dolphin (Tursiops truncatus). Vet. Radiol. Ultrasound 61, 215–226. doi: 10.1111/vru.12835
Kellar, N. M., Speakman, T. R., Smith, C. R., Lane, S. M., Balmer, B. C., Trego, M. L., et al. (2017). Low reproductive success rates of common bottlenose dolphins Tursiops truncatus in the northern Gulf of Mexico following the Deepwater Horizon disaster (2010–2015). Endang. Species Res. 33, 43–158. doi: 10.3354/esr00775
Keller-Wood, M., Feng, X., Wood, C. E., Richards, E., Anthony, R. V., Dahl, G. E., et al. (2014). Elevated maternal cortisol leads to relative maternal hyperglycemia and increased stillbirth in ovine pregnancy. Am. J. Physiol. Regul. Integr. Comp. Physiol. 307, 405–413. doi: 10.1152/ajpregu.00530.2013
Kenward, M. G., and Roger, J. H. (1997). Small sample inference for fixed effects from restricted maximum likelihood. Biometrics 5, 983–997. doi: 10.2307/2533558
Kirby, V. L. (1990). “Endocrinology of marine mammals,” in CRC Handbook of Marine Mammal Medicine: Health, Disease, and Rehabilitation, 1st Edn, ed. L. A. Dierauf (Boca Raton, FL: CRC Press), 303–351.
Kirby, V. L., and Ridgway, S. H. (1984). Hormonal evidence of spontaneous ovulation in captive dolphins, Tursiops truncatus and Delphinus delphis. Rep. Intl. Whaling Commission 6, 459–464.
Kowalski, A. A., Vale-Cruz, D. S., Simmen, F. A., and Simmen, R. C. (2004). Uterine androgen receptors: roles in estrogen-mediated gene expression and DNA synthesis. Biol. Reprod. 70, 1349–1357. doi: 10.1095/biolreprod.103.024786
Kuijper, E. A. M., Ket, J. C. F., Caanen, M. R., and Lambalk, C. B. (2013). Reproductive hormone concentratrions in pregnancy and neonates: a systematic review. Reprod. Biomed. Online 27, 33–63. doi: 10.1016/j.rmbo.2013.03.009
Legacki, E. L., Robeck, T. R., Steinman, K. J., and Conley, A. J. (2020). Comparative analysis of steroids in cyclic and pregnant killer whales, beluga whales and bottlenose dolphins using liquid chromatography tandem mass spectrometry. Gen. Comp. Endocrinol. 285:113273. doi: 10.1016/j.ygcen.2019.113273
Meaney, M. J., Syzf, M., and Seckl, J. R. (2007). Epigenetic mechanisms of perinatal programming of hypothalamic-pituitary-adrenal function and health. Trends Mol. Med. 13, 269–277. doi: 10.1016/j.molmed.2007.05.003
Melica, V., Atkinson, S., Calambokidis, J., Lang, A., Scordino, J., and Mueter, F. (2021a). Application of endocrine biomarkers to upadte information on reproductive physiology in gray whales (Eschrichtius robustus). PLoS ONE 16:e0255368. doi: 10.1371/journal.pone.0255368
Melica, V., Atkinson, S., Gendron, D., Calmbokidis, J., and Mueter, F. (2021b). Blubber Endocrine profiles provide insights into reproductive biology in blue whales from the eastern NorthPacific Ocean. Gen. Comp. Endocrinol. 310:113830. doi: 10.1016/j.ygcen.2021.113830
Meyer, J. M., Walker, S. L., Freeman, E. W., Steinetz, B. G., and Brown, J. L. (2004). Species and fetal gender effects on the endocrinology of pregnancy in elephants. Gen. Comp. Endocrinol. 138, 263–270. doi: 10.1016/j.ygcen.2004.06.010
Möhle, U., Heistermann, M., Palme, R., and Hodges, J. K. (2002). Characterization of urinary and fecal metabolites of testosterone and their measurement for assessing gonadal endocrine function in male nonhuman primates. Gen. Comp. Endocrinol. 129, 135–145. doi: 10.1016/s0016-6480(02)00525-7
Munro, C., Stabenfeldt, G., Cragun, J., Addiego, L., Overstreet, J., and Lasley, B. (1991). Relationship of serum estradiol and progesterone concentrations to the excretion profile of their major urinary metabolites as measured by enzyme immunoassay and radioimmunoassay. Clin. Chem. 37, 838–844. doi: 10.1093/clinchem/37.6.838
Munro, C. J., and Lasley, B. L. (1988). “Non-radiometric methods for immunoassay of steroid hormones,” in Non-Radiometric Assays: Technology and Application in Polypeptide and Steroid Hormone Detection, eds B. D. Albertson and F. P. Haseltine (New York, NY: Alan R. Liss), 289–329.
O’Brien, J. K., and Robeck, T. R. (2012). The relationship of maternal characteristics and circulating progesterone concentrations with reproductive outcome in the bottlenose dolphin (Tursiops truncatus) after artificial insemination, with and without ovulation induction, and natural breeding. Theriogenology 78, 469–482. doi: 10.1016/j.theriogenology.2012.02.011
O’Brien, J. K., Steinman, K. J., Fetter, G. A., and Robeck, T. R. (2017). Androgen and glucocorticoid production in the male killer whale (Orcinus orca): influence of age, maturity, and environmental factors. Andrology 5, 180–190. doi: 10.1111/andr.12254
Okon, M. A., Laird, S. M., Tuckermann, E. M., and Liu, T. C. (1998). Serum androgen levels in women who have recurrent miscarriages and their correlation with markers of endometrial function. Fertil. Steril. 69, 682–690. doi: 10.1016/s0015-0282(98)00007-7
Osborn, S., Dalton, L. M., Dold, C., and Robeck, T. R. (2012). Management of twin pregnancy and neonatal concerns in a beluga (Delphinapterus leucas). J. Zoo Wild. Med. 43, 193–196.
Plagemann, A. (2005). Perinatal programming and functional teratogenesis: impact on body weight regulation and obesity. Physiol. Behav. 86, 661–668. doi: 10.1016/j.physbeh.2005.08.065
Richard, J. T., Robeck, T. R., Osborn, S. D., Naples, L., McDermott, A., LaForge, R., et al. (2017). Testosterone and progesterone concentrations in blow samples are biologically relevant in belugas (Delphinapterus leucas). Gen. Comp. Endocrinol. 246, 183–193. doi: 10.1016/j.ygcen.2016.12.006
Richkind, M., and Ridgway, S. H. (1975). Estrogens, corticosteroids and progestagen patterns in the pregnant and non-pregnant bottle-nosed dolphin Tursiops truncatus following the intramuscular administration of NIH-FSH-OVINE-S9. J. Steroid Biochem. 6, 15–20.
Robeck, T., Gross, T., Walsh, M., Campbell, T., and McBain, J. (1994). “Preliminary results on radioimmunoassay determination post enzyme hydrolysis urinary progestin concentrations in the false killer whale (Pseudorca crassidnes),” in Proceedings of the 25th Annual Conference for the International Association of Aquatic Animals, Vallejo, CA, 156.
Robeck, T. R., Blum, J. L., Steinman, K. J., Ratner, J. R., Bergfelt, D. R., and O’Brien, J. K. (2018). Longitudinal profiles of relaxin and progestagens during pregnancy, pregnancy loss and false pregnancy in the killer whale (Orcinus orca). Gen. Comp. Endocinol. 267, 98–108. doi: 10.1016/j.ygcen.2018.06.008
Robeck, T. R., Gili, C., Doescher, B. M., Sweeney, J., DeLaender, P., Van Elk, C. E., et al. (2012). Altrenogest and progesterone therapy during pregnancy in bottlenose dolphins (Tursiops truncatus) with progesterone insufficiency. J. Zoo Wildl. Med. 43, 296–308. doi: 10.1638/2011-0166.1
Robeck, T. R., Montano, G. A., Steinman, K. J., Smolensky, P., Sweeney, J., Osborn, S., et al. (2013). Development and evaluation of deep intra-uterine artificial insemination using cryopreserved sexed spermatozoa in the bottlenose dolphin (Tursiops truncatus). Anim. Reprod. Sci. 139, 168—-181. doi: 10.1016/j.anireprosci.2013.04.004
Robeck, T. R., Steinman, K. J., and O’Brien, J. K. (2016). Characterization and longitudinal monitoring of serum progestagens and estrogens during normal pregnancy in the killer whale (Orcinus orca). Gen. Comp. Endocrinol. 236, 83–97. doi: 10.1016/j.ygcen.2016.07.010
Robeck, T. R., Steinman, K. J., and O’Brien, J. K. (2017). Characterization and longitudinal monitoring of serum androgens and glucocorticoids during normal pregnancy in the killer whale (Orcinus orca). Gen. Comp. Endocrinol. 247, 116–129. doi: 10.1016/j.ygcen.2017.01.023
Robeck, T. R., Steinman, K. J., Parry, C. B., Gomez, F. M., and Jensen, E. D. (2021). Comparisons of serum progesterone and progestagen concentrations in normal and abnormal bottlenose dolphin (Tursiops truncatus) pregnancy. Front. Mar. Sci. 8:630563. doi: 10.3389/fmars.2021.630563
Rolland, R. M., Hunt, K. E., Kraus, S. D., and Wasser, S. K. (2005). Assessing reproductive status of right whales (Eubalaena glacialis) using fecal hormone metabolites. Gen. Comp. Endocrinol. 142, 308–317. doi: 10.1016/ygcen.2005.02.002
Sathya, A., Prabhakar, S., Sangha, S. P. S., and Ghuman, S. P. S. (2007). Vitamin E and selenium supplementation reduces plasma cortisol and oxidative stress in dystocia- affected buffaloes. Vet. Res. Commun. 31, 809–818. doi: 10.1007/s11259-007-0116-2
Satué, K., and Gardon, J. C. (2016). “Pregnancy loss in mares,” in Genital Infections and Infertility, ed. A. M. Darwish (London: Intech Open), doi: 10.5772/673742
Saviano, P., Fiorucci, L., Grande, F., Macrelli, R., Troisi, A., Polisca, A., et al. (2020). Pregnancy and fetal development: cepalic presentation and other descriptive ultrasonographic findings from clinically healthy bottlenose dolphins (Tursiops truncatus) under human care. Animals 10:908. doi: 10.3390/ani10050908
Sawyer-Steffan, J. E., Kirby, V. L., and Gilmartin, W. C. (1983). Progesterone and estrogens in the pregnant and non-pregnant dolphin, Tursiops truncatus, and the effects of induced ovulation. Biol. Reprod. 28, 897–901. doi: 10.1095/biolreprod28.4.897
Sheriff, M. J., Krebs, C. J., and Boonstra, R. (2009). The sensitive hare: sublethal effects of predator stress on reproduction in snowshoe hares. J. Anim. Ecol. 78, 1249–1288. doi: 10.1111/j.1365-2656.2009.01552x
Silberzahn, P., Zwain, I., and Martin, B. (1984). Concentration increase of unbound testosterone in plasma of the mare throughout pregnancy. Endocrinology 115, 416–419. doi: 10.1210/endo-115-1-416
St. Aubin, D. J., Ridgway, S. H., and Wells, R. S. (1996). Dolphin thyroid and adrenal hormones: circulating levels in wild and semi-domesticated Tursiops truncatus, and influence of sex, age and season. Mar. Mamm. Sci. 12, 1–13. doi: 10.1111/j.1748-7692.1996.tb00301.x
Steinman, K. J., Robeck, T. R., and O’Brien, J. K. (2016). Characterization of estrogens, testosterone, and cortisol in normal bottlenose dolphin (Tursiops truncatus) pregnancy. Gen. Comp. Endocrinol. 226, 102–112. doi: 10.1016/j.ygcen.2015.12.019
Stoops, M. A., MacKinnon, K. M., and Roth, T. L. (2012). Longitudinal fecal hormone analysis For monitoring reproductive activity in the female polar bear (Ursus maritimus). Theriogenology 78, 1977–1986. doi: 10.1016/j.theriogenology.2012.07.005
Suzuki, M., Tobayama, T., Katsumata, E., Yoshioka, M., and Aidia, K. (1998). Serum cortisol levels in captive killer whale and bottlenose dolphin. Fish. Sci. 64, 643–647. doi: 10.2331/fishsci.64.643
Suzuki, M., Uchida, S., Ueda, K., Tobayama, T., Katsumata, E., Yoshioka, M., et al. (2003). Diurnal and annual changes in serum and cortisol concentrations in Indo-Pacific bottlenose dolphins (Tursiops aduncus) and killer whales (Orcinus orca). Gen. Comp. Endocrinol. 132, 427–433. doi: 10.1016/s0016-6480(03)00100-x
Sweeney, J. C., Stone, R., Campbell, M., McBain, J., St. Leger, J., Xitco, M., et al. (2010). Comparative survivability for Tursiops neonates from three U.S. institutions for the decades 1990–1999 and 2000 to 2009. Aquat. Mamm. 36, 248–261. doi: 10.1578/AM.36.3.2010.248
Trainer, P. J. (2002). Corticosteroids and pregnancy. Semin. Reprod. Med. 20, 375–380. doi: 10.1055/s-2002-36710
Troisi, R., Ptischman, N., Roberts, J., Siiteri, P., Daftary, A., Sims, C., et al. (2003). Associations of maternal and umbilical cord hormone concentrations with maternal, gestational, and neonatal factors (United States). Cancer Causes Control 14, 347–355. doi: 10.1023/a:1023934518975
Valenzuela-Molina, M., Atkinson, S., Mashburn, K., Gendron, D., and Brownell, R. L. Jr. (2018). Fecal steroid hormones reveal reproductive state in female blue whales sampled in the Gulf of California, Mexico. Gen. Comp. Endocrinol. 261, 127–135. doi: 10.1016/j.ygcen.2018.02.015
Wasser, S. K., Lundin, J. I., Ayres, K., Seely, E., Giles, D., Balcomb, K., et al. (2017). Population growth is limited by nutritional impacts on pregnancy success in endangered Southern Resident killer whales (Orcinus orca). PLoS One 12:e0179824. doi: 10.1371/journal.pone.0179824
West, B. T., Welch, K. B., and Galecki, A. T. (2015). Linear Mixed Models: A Practical Guide Using Statistical Software, 2nd Edn. (Boca Raton, FL: CRC Press), 199–248.
West, K. L., Ramer, J., Brown, J. L., Sweeney, J., Hanahoe, E. M., Reidarson, T., et al. (2014). Thyroid hormone concentrations in relation to age, sex, pregnancy, and perinatal loss in bottlenose dolphins (Tursiops truncatus). Gen. Comp. Endocrinol. 197, 73–81. doi: 10.1016/j.ygcen.2013.11.021
Yoshioka, M., Mohri, E., Tobayama, T., Aida, K., and Hanyu, I. (1986). Annual changes in serum reproductive hormone levels in the captive bottlenosed dolphins. Bull. Jpn. Soc. Sci. Fish. 52, 1939–1946. doi: 10.1292/jvms.16-0544
Keywords: bottlenose dolphins, pregnancy, abnormal pregnancy, androgens, estrogens, cortisol
Citation: Steinman KJ, Montano GA and Robeck TR (2021) Characterization of Circulating Androgens, Cortisol and Estrogens During Normal, Abnormal and False Pregnancy in Bottlenose Dolphins (Tursiops truncatus) Under Managed Care. Front. Mar. Sci. 8:737926. doi: 10.3389/fmars.2021.737926
Received: 07 July 2021; Accepted: 18 October 2021;
Published: 22 November 2021.
Edited by:
Jose Fernando Lopez-Olmeda, University of Murcia, SpainReviewed by:
Kelly J. Robinson, University of St Andrews, United KingdomCopyright © 2021 Steinman, Montano and Robeck. This is an open-access article distributed under the terms of the Creative Commons Attribution License (CC BY). The use, distribution or reproduction in other forums is permitted, provided the original author(s) and the copyright owner(s) are credited and that the original publication in this journal is cited, in accordance with accepted academic practice. No use, distribution or reproduction is permitted which does not comply with these terms.
*Correspondence: K.J. Steinman, S2FyZW4uU3RlaW5tYW5AU2VhV29ybGQuY29t
Disclaimer: All claims expressed in this article are solely those of the authors and do not necessarily represent those of their affiliated organizations, or those of the publisher, the editors and the reviewers. Any product that may be evaluated in this article or claim that may be made by its manufacturer is not guaranteed or endorsed by the publisher.
Research integrity at Frontiers
Learn more about the work of our research integrity team to safeguard the quality of each article we publish.