- 1Department of Animal Nutrition and Management, Swedish University of Agricultural Sciences, Uppsala, Sweden
- 2Swedish Center for Resource Recovery, University of Borås, Borås, Sweden
Changes in gut microbial composition over time in rainbow trout fed differentially processed diets supplemented with the filamentous fungi Neurospora intermedia were investigated in a 30-day feeding trial. Fish were fed a reference diet, non-preconditioned diet (NPD), or preconditioned (heat-treated) diet (PD), with the same inclusion level of N. intermedia in diets NPD and PD. Gut microbiota were analyzed on day 0, 10, 20, and 30. Gut microbial composition was similar for all diets on day 0, but was significantly different at day 10 and day 20. On day 30, the gut again contained similar communities irrespective of diet. The overall gut microbiota for each diet changed over time. Abundance of Peptostreptococcus and Streptococcus was higher in the initial days of feeding in fish fed on commercial diet, while a significant increase in lactic acid bacteria (Lactococcus lactis) was observed on day 30. Feed processing (preconditioning) did not contribute largely in shaping the gut microbiome. These results indicate that dietary manipulation and duration of feeding should be considered when evaluating gut microbial composition in cultured fish. A minimum 30-day feeding trial is suggested for gut microbiome, host and diet interaction studies.
Introduction
Single-cell proteins such as microalgae, bacteria, and fungi are microbial protein sources that represent potential alternatives as fish feed ingredients (Nalage et al., 2016). In particular, filamentous fungi are versatile microorganisms that can grow on a wide range of wastes, industrial by-products, and side-streams. The nutritional value of filamentous fungal biomass derives from its high protein content, fatty acid composition, and presence of other nutrients such as vitamins, minerals, anti-oxidants, and immune stimulant components (Karimi et al., 2019a). Despite these attractive nutritional properties of filamentous fungal biomass, few studies have explored its use as a fish feed ingredient. Using nuclear magnetic resonance (NMR) spectroscopy, Abro et al. (2014) investigated changes in the metabolism of Arctic charr (Salvelinus alpinus) fed with filamentous fungal species Rhizopus oryzae. In another study, Vidakovic et al. (2016) used intact and extracted baker’s yeast (Saccharomyces cerevisiae) and Rhizopus oryzae as separate diet ingredients and evaluated the effects on digestibility and intestinal barrier function in Arctic charr. Neurospora intermedia is a food-grade filamentous fungus isolated from traditional fermented food in Indonesia, and is therefore among the filamentous fungi species recognized as safe. Its nutritional properties and cultivation conditions have been extensively explored by our research group (University of Borås) and reported in previous studies (Ferreira et al., 2014, 2015; Gmoser et al., 2018; Karimi et al., 2019b). The high nutritional value of N. intermedia and its categorization as a dietary safe microorganism make it an ideal alternative ingredient for fish feed.
The gut microbiota is critical to fish nutrition as it produces several enzymes which help in digestion, transport of nutrients, direct protection from pathogens, and enhanced immunity (Austin, 2006; Merrifield et al., 2010; Camp et al., 2012; de Bruijn et al., 2018). Several studies have found that environmental (abiotic) and host (biotic) factors play important roles in shaping the gut community in fish. Gut microbial composition and diversity are influenced by genetics, sex, weight, age, rearing conditions, diet, and feeding habits (Hovda et al., 2012; Ingerslev et al., 2014a; Li et al., 2016; Ringø et al., 2016; Yan et al., 2016; Sun et al., 2020). High-throughput sequencing has been used previously to explore dietary effects on the gut microbiota of several fish species, such as rainbow trout, Atlantic salmon (Salmo salar), Arctic charr, sea bream (Sparus auratus), and channel catfish (Ictalurus punctatus) (Navarrete et al., 2013; Gajardo et al., 2017; Huyben et al., 2017; Nyman et al., 2017; Wang et al., 2019). Most of these studies have investigated the short- or long-term effect of diet on gut microbiota but, to our knowledge, none has investigated gradual changes in microbial communities over time. Diet can adversely modulate gut microbial composition in fish, leading to inflammation of the distal intestine, as demonstrated for Atlantic salmon fed high levels of soy protein (Gajardo et al., 2017). It has also been shown that Arctic charr fed filamentous fungi (Rhizopus oryzae) display higher frequency of diarrhea, despite high apparent digestibility coefficient (Langeland et al., 2016). Knowledge of the interactions between host, gut microbiota, diet, and feeding strategy is important when developing novel diets, in order to ensure better fish health and welfare. The present study sought to extend this knowledge by examining the role of novel filamentous fungi in modulating the intestinal microbiota of rainbow trout over successive 10-day feeding intervals and its efficiency as a fish feed ingredient.
Materials and Methods
Fish Husbandry
Juvenile rainbow trout were purchased from Vilstena Fiskodling AB, Fjärdhundra, Sweden, and the experiment was carried out in the Aquatic Facility, Center of Veterinary Medicine and Animal Science, Swedish University of Agricultural Sciences, Uppsala, Sweden. A total of 300 fish (average weight 127.8 ± 19.8 g) were randomly and evenly distributed between 15 oval experimental tanks (200 L) and reared in a 12-h light cycle (08.00–20.00 h). The experimental tanks were equipped with a partial recirculation system and supplied with fresh tap water at 3 L min–1. All fish were judged to be healthy, with no visible signs of injuries detected on skin, gills, or fins. Each experimental tank was connected to a waste feed and feces collection system. Temperature during the whole experiment was 11 ± 1°C and oxygen level was 8 ± 2 mg/L (HQ40D Portable Multi Meter, Hach, Loveland, CO, United States). The fish were acclimatized for 10 days on a commercial diet (Biomar EFICO ENVIRO 920 ADVANCE, 2% of body weight once a day prior to the experiment. The experiment was performed in compliance with laws and regulations on procedures and experiments on live animals in Sweden, which are overseen by the Swedish Board of Agriculture (diary number: 5.8.18-16347/2017).
Production of Neurospora intermedia Biomass
Fungal biomass of N. intermedia CBS 131.92 (Centraalbureau voor Schimmelcultures, Netherlands) was produced under semi-continuous cultivation condition at the Swedish Center for Resource Recovery, University of Borås. The fungus was cultivated on complex medium containing 30 g/L glucose and 5 g/L yeast extract as the major carbon and nitrogen source, respectively. Trace elements in the form of (NH4)2SO4, KH2PO4, CaCl2.2H2O, and MgSO4 x7H2O in concentrations of 7.5, 3.5, 1.0, and 0.75 g/L were added to the cultivation medium to support filamentous fungi growth, using a 26 L capacity bubble column bioreactor (airlift bioreactor converted to bubble column bioreactor by removing the internal loop tube) (Bioengineering, Switzerland). Cultivation was carried out at 35°C and 1 vvm (volume of air per volume of medium per minute). Cultivation condition parameters and sterilization method were according to Ferreira et al. (2015). To harvest biomass, 75% of the working volume of the reactor (15 L) was harvested twice per day, at 11.00 and 23.00 h. Fresh sterilized cultivation media was added to top up the cultivation broth after harvesting. Harvested broth, containing post-cultivation medium and biomass, was transferred to a cold room and stored at 4°C. After termination of cultivation, biomass was quickly separated from the culture media using a sieve, washed with distilled water, and dried in an air oven at 70°C.
Diets and Feeding
Feed preparation was carried out at the Swedish University of Agricultural Sciences, Uppsala, Sweden. Three experimental diets were prepared, a reference diet (RD), a non-preconditioned diet (NPD), and a preconditioned diet (PD). Diet RD was prepared with fishmeal as the major protein source. Diets NPD and PD were prepared by mixing 30% (by weight) of N. intermedia biomass with 70% of diet RD according to Cho (1979). The ingredients were mixed in a kitchen mixer, gelatin dissolved in hot water was added as a binder, and the ingredients were mixed again and pelleted through a meat grinder, using a 3.5 mm die (Nima Maskinteknik AB, Örebro, Sweden). The strings produced were dried in an air oven at 50°C for 12 h and cut into pellets with a twin blade blender (Kneubühler, Luzern, Germany).
Diets PD and NPD were formulated in the same way, but diet PD was preconditioned by heat-processing in a convection oven (Electrolux Professional, FCE061) at 105°C for 5 min, in order to increase the degree of gelatinization of starch and emulate temperature treatment during extrusion conditions. The prepared feed was stored at −20°C until it was fed to the fish (approximately 2 weeks). Data on feed composition and proximate analysis are presented in Tables 1, 2, respectively. Rainbow trout were fed twice a day throughout the 30-day feeding trial, using automatic belt feeders (Hølland teknologi, Sandnes, Norway). Feed was initially provided in excess (starting with a ration equal to 1.5% of initial body weight) and the ration was adjusted according to the feed waste in the tank.
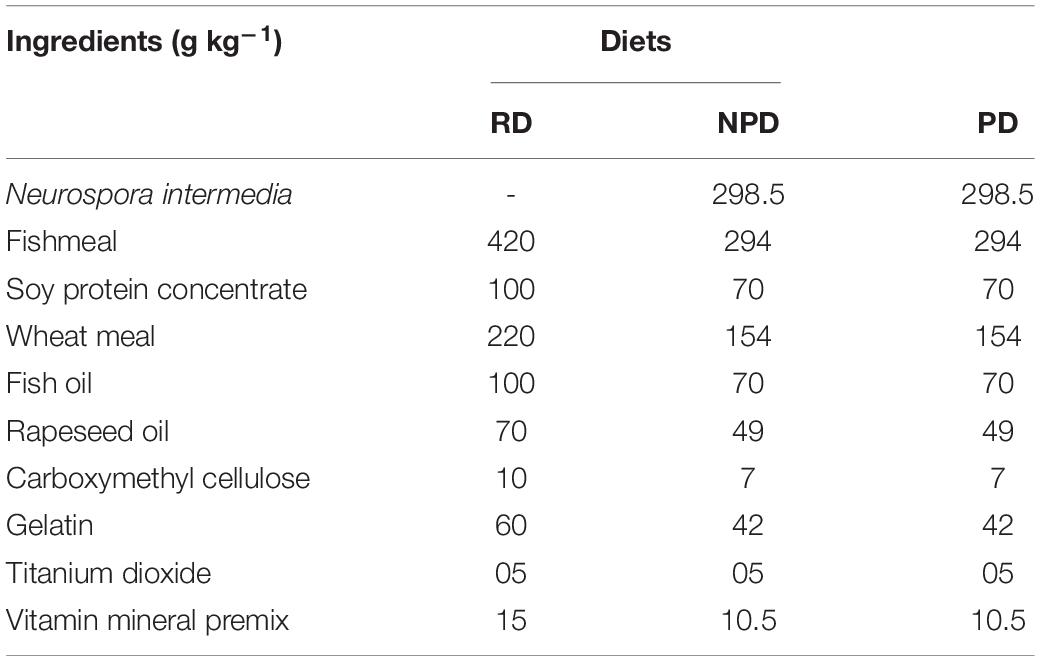
Table 1. Dietary composition (g kg–1 on dry matter basis) of the reference diet (RD), non-preconditioned diet (NPD), and preconditioned (heat-processed) diet (PD).
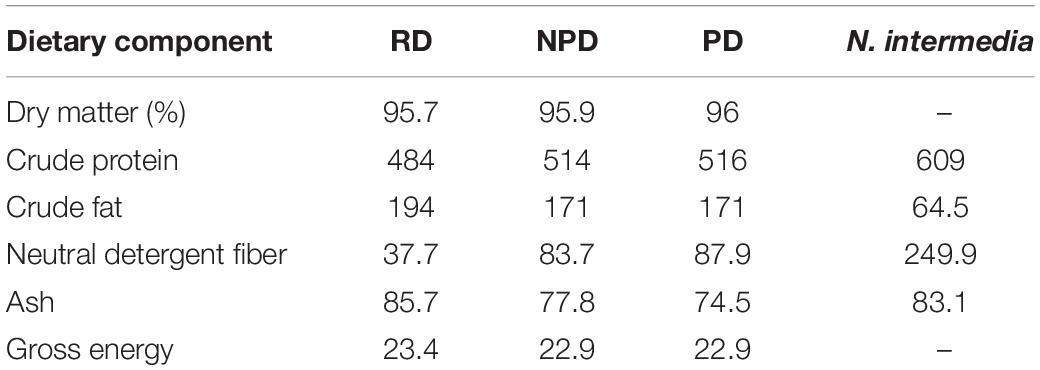
Table 2. Proximate composition [g kg–1 dry matter (DM)] and energy content (MJ kg–1 DM) of the reference diet (RD), non-preconditioned diet (NPD), preconditioned (heat-processed) diet (PD), and Neurospora intermedia fungal biomass.
Sample Collection
Fish were anesthetized with 80 mg/L tricaine methanesulfonate (MS-222, Western Chemical Inc., Ferndale, WA, United States) and weighed at the start and end of the trial, and growth performance was recorded. Sampling for gut microbiota was performed on five fish per treatment at 0, 10, 20, and 30 days of feeding. For this, euthanized fish were aseptically dissected from the ventral side after swabbing with ethanol (70% solution). The hindgut was dissected from the ileocecal valve to 0.5 cm above the anus, and digesta samples and mucosal scrapings were taken. These were snap-frozen in liquid nitrogen and stored at −80°C until DNA extraction.
Chemical Analysis
Experimental feeds were freeze-dried, milled, and stored at −20°C until analysis. In order to determine the dry matter content, the samples were dried in an oven for 16 h at 103°C and then cooled in a desiccator before weighing. Crude protein content (N × 6.25) (Nordic Committee on Food Analysis, 1976) was determined by the Kjeldahl method, using a 2020 Kjeltec digester and a 2400 Kjeltec Analyser unit (FOSS Analytical A/S, Hilleröd, Denmark). Crude lipid content was analyzed according to the Official Journal of the European Union (2009), using an extraction unit (1047 Hydrolysing Unit and a Soxtec System HT 1043; FOSS Analytical A/S). Neutral detergent fiber (NDF) was measured based on the method described by Chai and Udén (1998) using 100% neutral detergent solution, while amylase and sulphite were used for reduction of starch and protein. Gross energy (GE) content was determined in an isoperibol bomb calorimeter (Parr 6300, Parr Instrument Company, Moline, IL, United States). Dry matter, gross energy, and ash content were analyzed according to standard methods (AOAC, 1995).
Extraction of DNA
Intestinal samples (200 mg) were transferred to sterile cryotubes containing 1 mL InhibitEX buffer and 0.5 g of 0.1 mm silica beads, and homogenized at room temperature in a bead beater (Precellys Evolution, Bertin Technologies) for 2 × 1 min at 6,000 rpm, with a 5 min rest. DNA was extracted using the QIAamp Fast DNA Stool Mini Kit (Qiagen Gmbh, Hilden, Germany) according to the manufacturer’s instructions.
Library Preparation and Sequencing
The V4 region of the 16S rRNA gene was amplified from the extracted DNA using the primers 515F (5-GTGCCAGCMGCCGCGGTAA-3) and 805R (5-ACTACHVGGGTATCTAATCC-3). Polymerase chain reactions (PCR) were carried out using Phusion® High-Fidelity PCR Master Mix (New England Biolabs). PCR products were confirmed by gel electrophoresis and were purified with the Qiagen Gel Extraction Kit (Qiagen, Germany) and quantified by Qubit®3.0 Fluorometer (Invitrogen, Thermo Fisher Scientific). Final libraries including barcodes and adaptors were generated with the NEBNext® UltraTM DNA Library Prep Kit, and the amplicons were then sequenced using Illumina sequencing (NovaSeq 6000) at Novogene (Beijing, China). The BioProject accession number is PRJNA743247.
Bioinformatics Analysis
Paired-end reads were assigned to samples based on their unique barcode. These reads were merged after truncating off the barcode and primer sequence using FLASH (v1.2.71) (Magoč and Salzberg, 2011). Quality filtering on the raw sequence tags was performed using QIIME (v1.7.02) (Caporaso et al., 2010; Bokulich et al., 2013). Sequence analysis by clustering of operational taxonomic units (OTUs) was performed using Uparse software (Uparse v7.0.10013) (Edgar, 2013). Sequences with ≥ 97% homology were assigned to the same OTUs. Representative sequences for each OTU were screened for further annotation. For each representative sequence, Mothur software was applied to the SSU rRNA data in the SILVA Database4 for species annotation at each taxonomic rank (Wang et al., 2007; Quast et al., 2012).
Statistical Analysis
A linear mixed effect (LME) model (“nlme” package) was used to test for statistically significant differences between relative proportions of OTUs and diet, sampling day, and diet × day interactions. The LME model results were analyzed using R statistical software version 3.6 (Pinheiro et al., 2014; R Core Team, 2015), considering diet and day as fixed factors and tank as random factor. Data on bacterial OTUs were normalized by log transformation. LME comparison was conducted on OTUs with average abundance > 1%, followed by post hoc analysis of emmeans (“emmeans” package) with Tukey adjustment for multiple pairwise comparison. Similarity percentage analysis (SIMPER), analysis of similarity (ANOSIM), principal coordinate analysis (PCoA), principal component analysis (PCA), and Spearman correlation analysis were performed using Paleontological Statistics Software version 4.03 (PAST). Two-way ANOSIM was performed to investigate the effect of diet and day interval on beta diversity of gut microbial composition. The ANOSIM and SIMPER analyses were both based on Bray Curtis index, and Bonferroni correction was used to adjust for multiple pairwise comparisons to determine differences in gut microbial composition within and between time intervals for each diet. PCoA based on Bray Curtis and Jaccard dissimilarity was used to assess the overall clustering of samples according to microbial community composition based on diet and time interval.
Results
Gut Microbial Composition of Rainbow Trout
The overall gut microbial composition after 30 days of feeding showed high dominance of Tenericutes (84%), followed by Firmicutes (10%), and only very low relative abundance of other bacterial phyla. The high dominance of the Tenericutes phylum was due to two dominant Mycoplasma OTUs (Figure 1A). Assessment of the data did not reveal logical patterns, however, mainly since the two dominant Mycoplasma OTUs were not correlated to any of the parameters evaluated. Therefore, in further analyses on microbial composition the two dominant OTUs of Mycoplasma were excluded and relative abundance was recalculated, to discern effects on other bacterial taxa. In total, 4.8 million sequence reads of bacteria were obtained. The average number of sequence reads per sample without Mycoplasma was 13,244 and the lowest number obtained was 1,398. A total of 5,961 OTUs were obtained after excluding Mycoplasma OTUs, and bacterial OTU abundance was then dominated by two phyla, Firmicutes (58%) and Proteobacteria (15%) (Figure 1B). The overall trend in bacterial community composition from day 0 to day 30 was that Firmicutes ranged from 38 to 79% and Proteobacteria ranged from 8 to 24% for the different diets (Figure 2). Of the top 10 OTUs with abundance > 1% (Figure 3), Peptostreptococcus (9%), Lactococcus (L. lactis, 7%), Brevinema (6%), Streptococcus (5%), Deefgea (5%), and Anaerotruncus (4%) were the most abundant over the 30-day period.
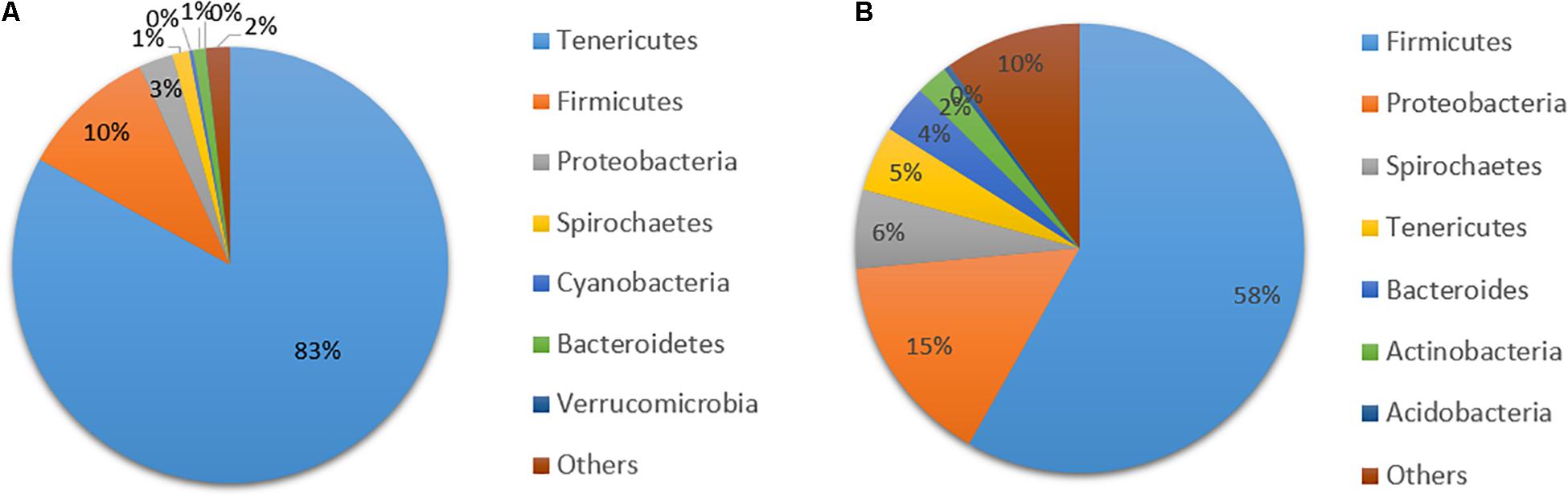
Figure 1. Mean relative abundance of bacterial taxa (phylum level) in the gut of rainbow trout fed the diets from 0 to day 30 (n = 60 samples), calculated with (A) with dominant Mycoplasma operational taxonomic units (OTUs) included and (B) without dominant Mycoplasma OTUs.
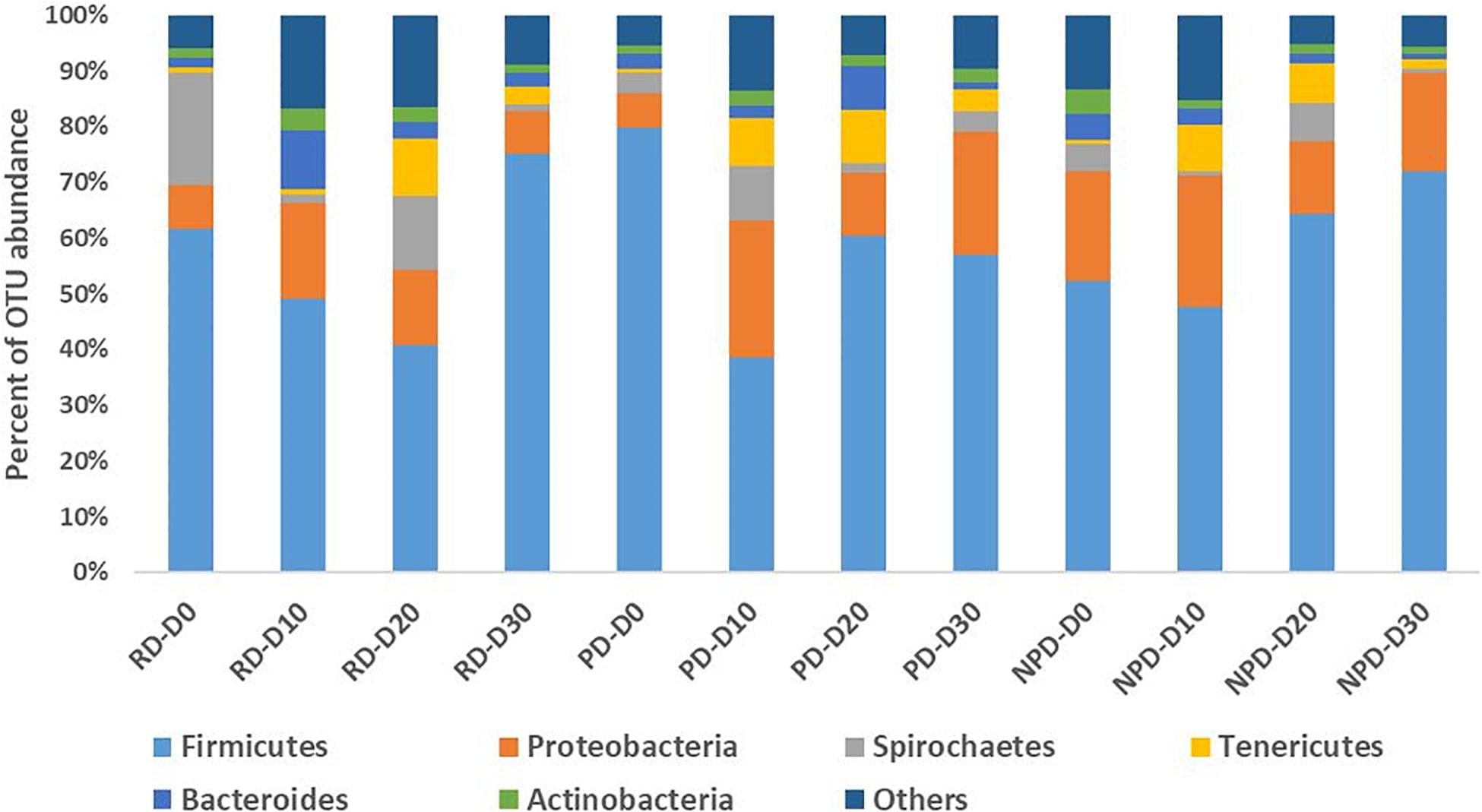
Figure 2. Relative abundance of bacterial taxa in the gut of rainbow trout at different time intervals (after excluding operational taxonomic unit OTU1 and OTU2, belonging to Mycoplasma) (n = 5). RD: reference diet, PD: preconditioned (heat-processed) diet, NPD: non-preconditioned diet. D0, D10, D20, and D30 refer to the 10-day time interval. Until day 0, all fish had been fed a commercial diet for 10 days.
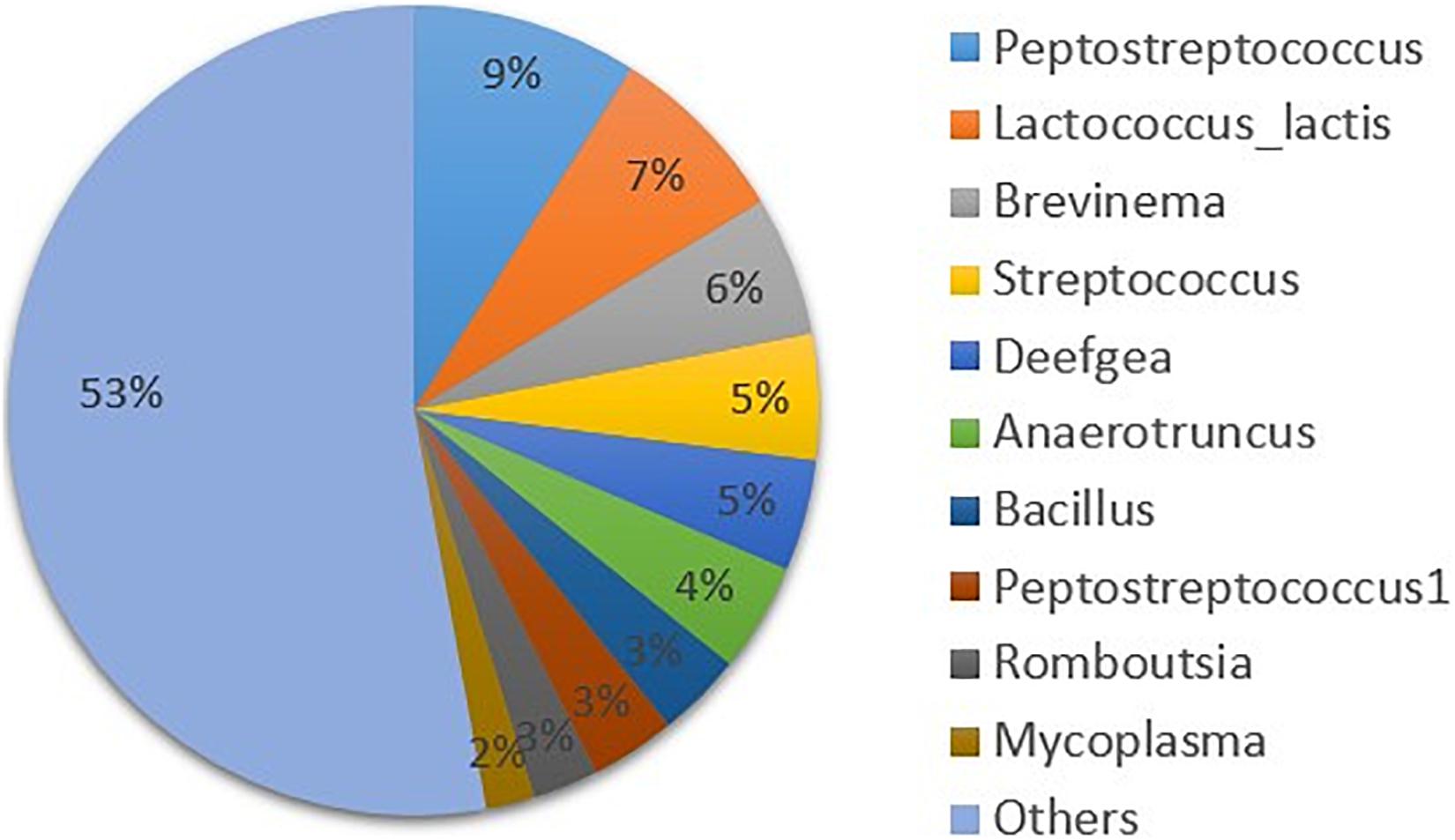
Figure 3. Mean relative abundance of the top 10 bacterial operational taxonomic units (OTUs) in the gut microbiota of rainbow trout from 0 to 30 days on the commercial and experimental diets (n = 60).
Shift in Gut Microbial Composition of Rainbow Trout With Diet and Days of Feeding
Principal coordinate analysis was performed to graphically explore the shift in community structure for different diets after different time intervals (Figures 4A,B). The percentage variation (PoV) explained for axis 1 and 2 when using Bray Curtis index was 25.5 and 10.3%, respectively. For the analysis based on Jaccard’s dissimilarity, PoV explained by axis 1 was 13% and by axis 2 was 6.68%. Differences in gut microbial composition at each day (within interval) were analyzed with one-way ANOSIM. The results confirmed that gut microbial composition for the different diets was similar at day 0 and day 30, but dissimilar at day 10 and day 20 (Supplementary Table 1). Pairwise comparison of the treatment groups showed that they were significantly dissimilar within and between 10-day intervals (Supplementary Table 2). At day 10, the overall microbial composition of the fish gut with diet PD and NPD was different from that with RD. At day 20 there was a difference in microbial composition between NPD and PD, but they did not differ from RD. Over 10-day intervals, there was a temporal change in gut microbial composition with all diets from day 0 to day 10, from day 10 to day 20, from day 20 to day 30, and from day 10 to day 30 (Supplementary Table 2). According to SIMPER analysis, the percentage dissimilarity for the pairwise-compared treatment groups ranged from 64.73 to 82.58%.
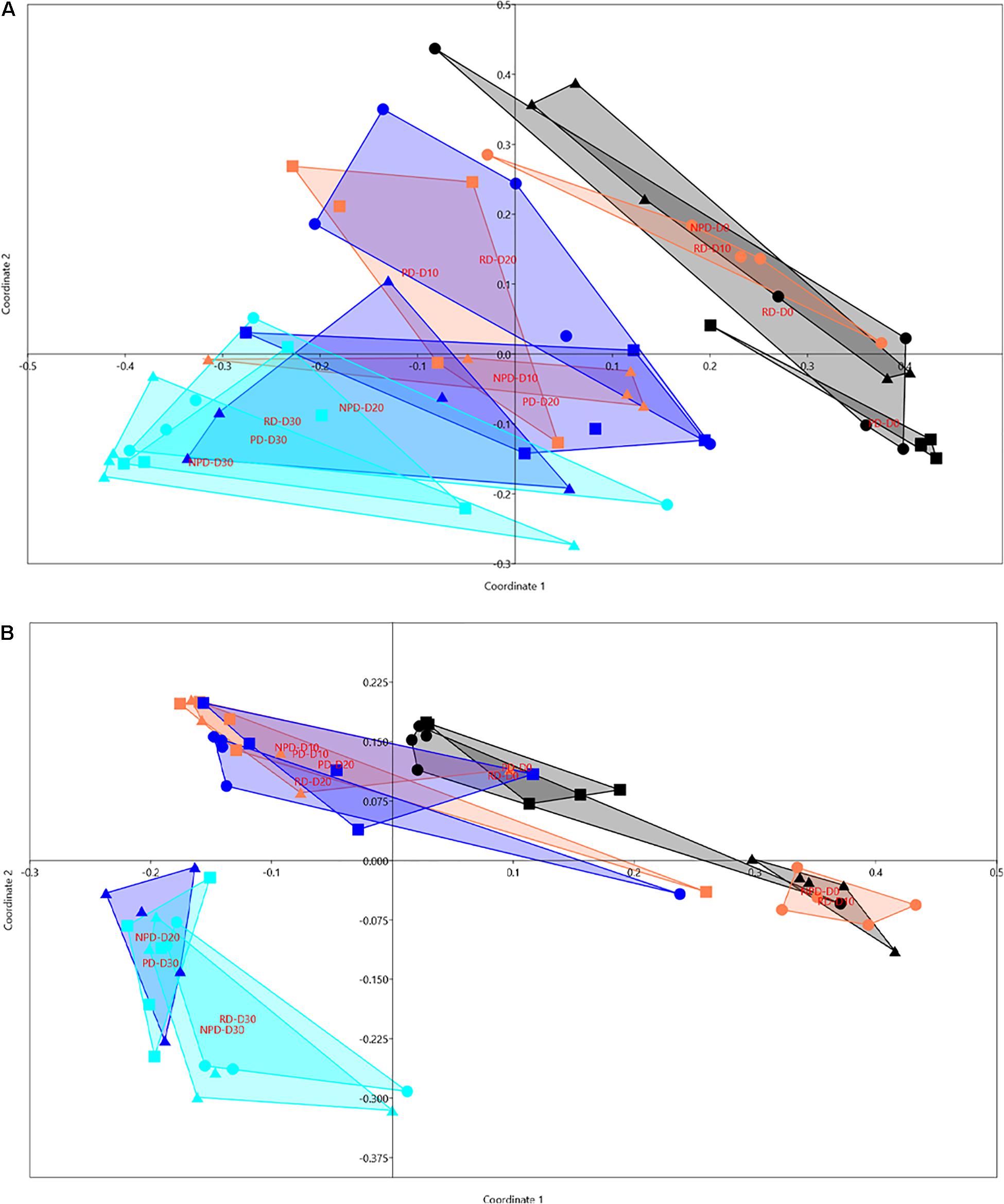
Figure 4. Principal coordinate analysis plot based on (A) Bray Curtis index and (B) Jaccard dissimilarity showing the shift in gut bacterial community of rainbow trout with diet and time. Reference diet (0), preconditioned diet (), non-preconditioned diet (Δ). Different colors represent day interval; days 0 (
), 10 (
), 20 (
) and 30 (
). Until day 0, all fish had been fed a commercial diet for 10 days.
Effect of Diet and Days of Feeding on Gut Microbial Composition of Rainbow Trout
Two-way ANOSIM revealed that diet and time had a significant influence in shaping the overall gut microbiota composition of trout (Supplementary Table 3). The results from the statistical analysis and interaction plot investigating the effect of treatments within and between 10-day intervals of feeding on the abundance of top six OTUs are shown in Figure 5. A more detailed description of these data can be found in Supplementary Tables 4–6. Day intervals had significant effects on the abundance of Peptostreptococcus and Streptococcus. Diet and day had significant effects on Lactococcus and Deefgea. The abundance of Anaerotruncus was significantly affected by diet. An interaction effect was observed only for Deefgea and Anaerotruncus. At day 30, the abundance of Streptococcus was significantly different between diets PD and NPD, while the abundance of Deefgea was significantly different for diet RD from NPD and PD (Supplementary Table 5). Significant increase in abundance from day 0 to day 30 for all diet namely RD, PD and NPD was only observed for Lactococcus (Supplementary Table 5). At day 0, Peptostreptococcus was the dominant taxon, but by day 30 Lactococcus was the most abundant taxon for all diets. The PCA results revealed that the occurrence of Peptostreptococcus and Streptococcus was positively and negatively correlated, respectively, with that of Lactococcus (Figure 6). These results were confirmed by Spearman correlation analysis (Supplementary Figure 1).
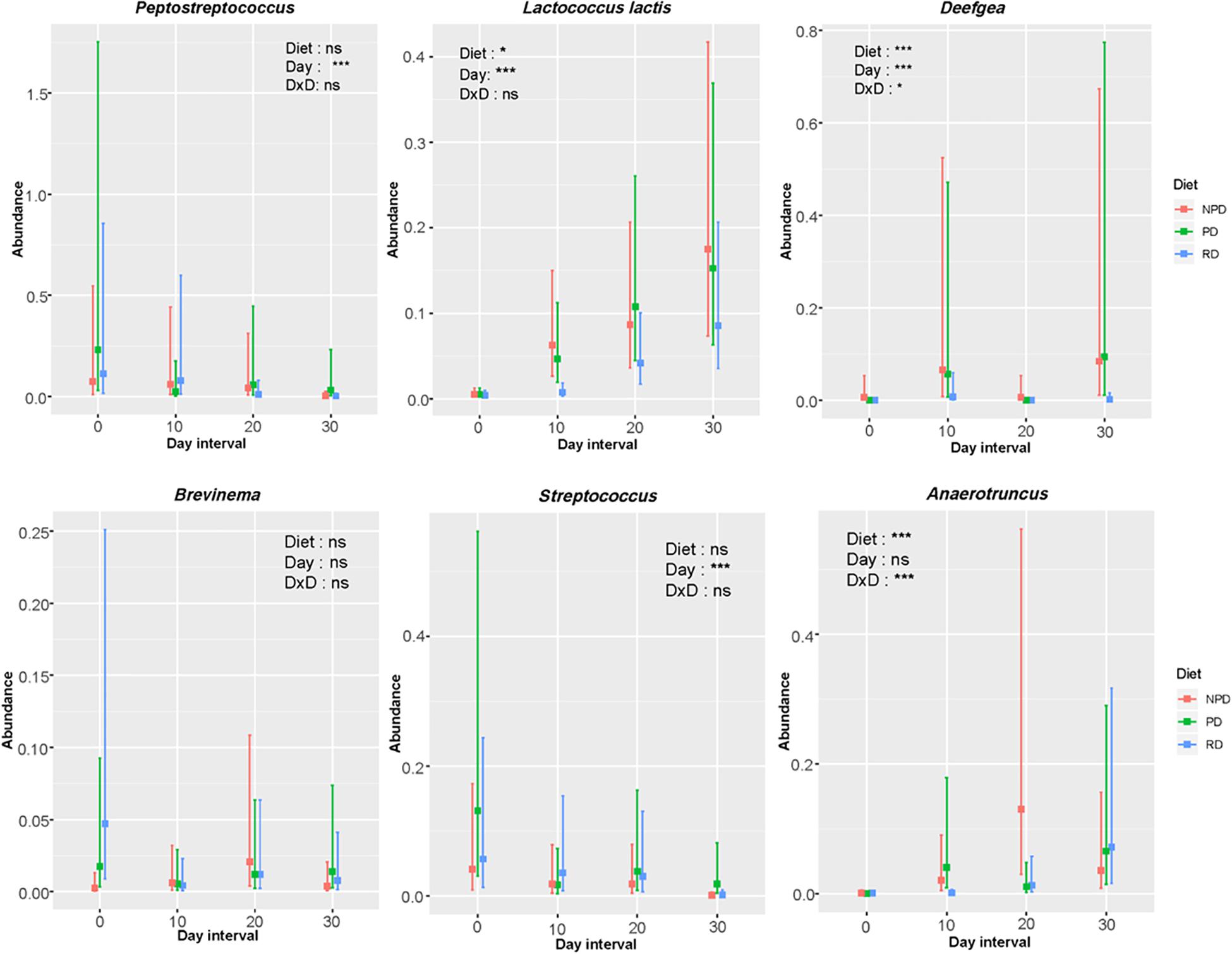
Figure 5. Interaction plot showing relative abundance of the top six bacterial taxa in the gut bacterial community of rainbow trout. RD: reference diet, PD: preconditioned (heat-processed) diet, NPD: non-preconditioned diet. Until day 0, all fish had been fed a commercial diet. The vertical bar in the plot represents confidence interval and overlapping bars means the difference is not significant. Significance: ***p < 0.001, ** p < 0.01, *p < 0.05, and ns p > 0.05.
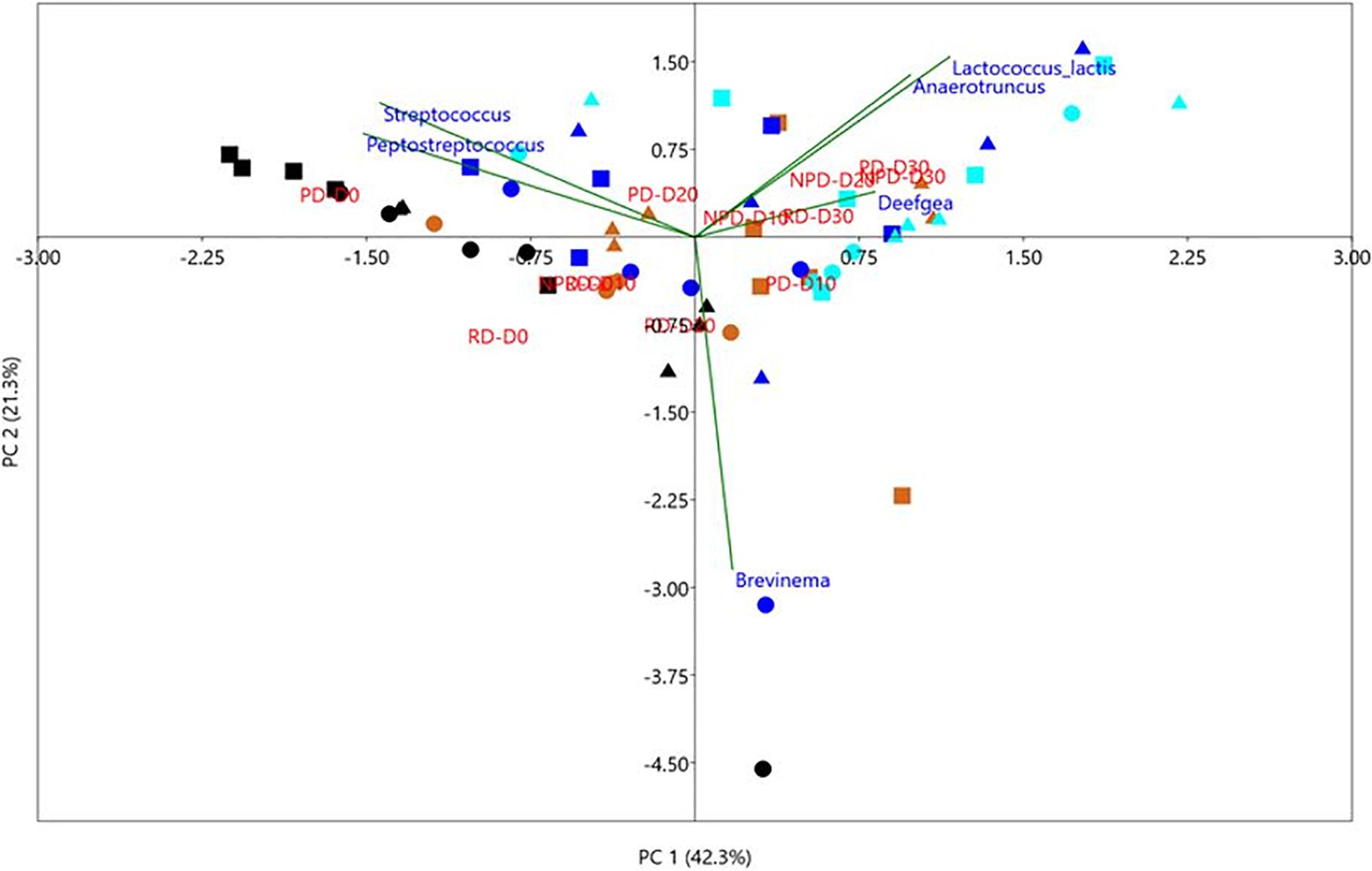
Figure 6. Principal component analysis (PCA) plot showing the correlation of the top six operational taxonomic units (OTUs) in the gut bacterial community of rainbow trout with diet and day interval. Reference diet (0), preconditioned diet (), non-preconditioned diet (Δ). Different colors represent day interval; days 0 (
), 10 (
), 20 (
), and 30 (
). Until day 0, all fish had been fed a commercial diet for 10 days.
Growth Performance
Over the 30-day study period, the fish achieved a mean weight gain of 45.6 ± 3.1%, 44.7 ± 1.5% and 45.1 ± 3.3% for diet RD, NPD, and PD, respectively. These values were not significantly different. All diets were consumed without obvious changes in the intake pattern and zero mortality was recorded during the experimental period.
Discussion
Shift in Overall Gut Microbial Composition With Diet and Time
The PCoA, ANOSIM, and SIMPER results demonstrated that the overall differences seen in gut microbial community were based on type of diet and feeding period. There was a gradual shift in bacterial communities between fish fed the commercial diet (day 0) and those fed the experimental diets (day 10–30) (Figures 4A,B and Supplementary Tables 1, 2). Bacterial composition was expected to be similar at day 0, since fish in all treatment tanks were fed the same commercial diet during the previous acclimatization period. Within 10 days of experimental diet feeding, microbial composition in the fish gut had changed significantly, indicating that all diets had a significant effect on microbial composition. Previous studies have also reported a change in gut microbiota following a change in diet for salmon, rainbow trout, and brown trout (Salmo trutta) after first feeding (Ingerslev et al., 2014b; Michl et al., 2017, 2019). In the studies by Michl and co-workers, trout were fed 0, 50, and 90% plant protein-based diets until 54 days after hatching and then fed a cross-over diet for another 39 days, and a change in microbiome was observed at both 54 and 93 days of feeding showing occurrence of gut microbiota is influenced diet and depend largely on time of sampling. In the present study, diet and 10-day period both had an effect in shaping the gut communities. However, Michl et al. (2017) observed no change in the gut microbiota over time, after a certain point or with longer feeding duration with the same diet, and concluded that microbiota composition depends largely on the actual diet fed at the time of sample collection. In the present study, the gut microbiota differed significantly at day 10 and day 20, but was similar at day 30 irrespective of different treatment diets, suggesting that obtained microbiota at this time point is not influenced by two of the environmental variations in this case heat processing of diet nor N. intermedia inclusion. However, temporal change for all diets from day 20–30 was evident. Until day 20, differences in microbial composition can be in order to adapt to the environment due to dietary intervention. Longer periods of study are needed to confirm this.
Little information is available on the effect of thermal processing of feed on the fish gut microbiome. In the presented study, there was no difference in overall microbial composition between the preconditioned (thermal-processed) diet (PD) and the non-preconditioned diet (NPD) at day 30. However, Zhang and Li (2018) observed a decrease in gut microbiota at taxonomic and OTU levels when catfish were fed thermal-processed fish as food (steam, 100°C for 15 min) compared with non-processed food or feed.
Effect of Diet and Duration of Feeding on Core Gut Microbiome
In the present study, high ubiquitous abundance of Tenericutes, dominated by two Mycoplasma OTUs, was found which is similar to findings in previous studies on rainbow trout (Lowrey et al., 2015; Lyons et al., 2017a,b; Huyben et al., 2018). According to Holben et al. (2002), Mycoplasma can be a natural resident in the gut of both farmed and wild salmon. As the biological function of Mycoplasma is not known and very high dominance of the two otus took over the statistical analyses, thus it reduced chance to identify associations among the other microbes possibly associated with the diets.
Data analysis revealed that the next most common phylum in the core gut microbiota after Tenericutes was Firmicutes, folowed by Proteobacteria (Figure 1B), as found in other studies on salmonids (Nayak, 2010; Gajardo et al., 2017). One previous study has found that the core gut microbial composition of rainbow trout is resistant to change due to diet type, but that conclusion was reached by comparing data at phylum level (Wong et al., 2013). Another study suggested that there might be differences at lower taxonomic ranks, particularly at species or genus level, rather than at higher taxonomic ranks (Michl et al., 2017). This was the case in the present study, where the abundance of Lactococcus (Lactobacillales), Deefgea (Neisseriales), and Anaerotruncus (Clostridiales) was significantly enriched from day 0 to day 30 and fish on the preconditioned diet (PD) had higher abundance of Streptococcus than those on the non-preconditioned diet (NPD). Lower abundance of bacteria of the genera Deefgea and Anaerotruncus was observed, as also found in the gut microbiota of humans, rainbow trout, and Atlantic salmon isolated through 16s sequencing (Namsolleck et al., 2004; Perez-Fuentes et al., 2018; Ricaud et al., 2018). Peptostreptococcus and Streptococcus are generally present in high abundance in protein-rich environments, and play an important role in amino acid catabolism and absorption in the gut (Dai et al., 2011; Davila et al., 2013; Neis et al., 2015). The abundance of one taxa can suppress that of another depending on nutrient availability for growth. A shift in microbial composition from Streptococcus to Lactobacillus has been reported in Atlantic salmon fed fishmeal-free diets or diets with fishmeal replaced with plant protein (Hartviksen et al., 2014). This is comparable to the results in the present study, where Peptostreptococcus, the dominant taxon at day 0 (all fish fed the commercial diet) decreased in abundance with time, whereas abundance of Lactococcus increased conferring the change due to the substrate exchange.
Diet and Duration of Feeding Promotes Abundance and Dominance of Intestinal Lactococcus lactis
Gut bacterial composition in rainbow trout fed plant protein-based diets and Atlantic salmon fed a fishmeal-free diet is reported to show an increase in abundance of Lactobacillales (Schmidt et al., 2016; Michl et al., 2017). A study using PCR-TTGE-dependent bacterial quantification showed an increase in Lactobacillus and Lactococcus in Atlantic salmon fed diets in which 30% of the fishmeal was replaced with fermented soy meal (Catalán et al., 2018). Lactic acid bacteria are natural inhabitants of the fish gut and have the ability to adhere and colonize and play a beneficial role in the gut (Seppola et al., 2006; Gatesoupe, 2008). Additionally, growth of L. lactis is highly substrate-dependent (Rombouts et al., 2020). It is possible that components in the cell wall of N. intermedia, such as beta glucan, chitin, and glycoproteins, act as fermentable substrate for Lactobacillales. Increased Lactobacillales abundance has been observed in Artic charr and rainbow trout fed yeast (Huyben et al., 2017; Nyman et al., 2017). Lactobacillales from fish is known to be slow-growing and the recommended growth period on agar media at low temperatures is up to 4 weeks (Ringø and Gatesoupe, 1998), which is in line with the findings in this study of highest Lactobacillales abundance on day 30. Studies have shown that use of L. lactis as a probiotic can enhance weight, immunity, and disease resistance in fish (Sun et al., 2012; Heo et al., 2013; Xia et al., 2018). Lactobacillus lactis has also been shown to improve the gut architecture and modulate the intestinal microbial composition in fish (Dawood et al., 2016; Xia et al., 2019; Won et al., 2020).
Conclusion
The filamentous fungi Neurospora intermedia has a good nutritional profile with high protein content and healthy gut microbiota profile with dominance of lactic acid bacteria. It can be advocated as a protein source to replace fishmeal in the diet of cultured fish, for sustainable feed production and aquaculture. Changes due to environmental interventions, in this case diet and feeding duration were more pronounced for modulating the fish gut microbes at overall and at lower taxonomic levels than feed preprocessing. Preconditioning (steam-processing) of the diet had no effect on shaping the overall microbial gut composition as they were similar on day 30. Diets containing N. intermedia promoted abundance of Lactococcus compared with the commercial diet. Thus duration of feeding should be taken into account when studying changes in the gut microbial community in rainbow trout following diet manipulation. Based on our findings, a minimum 30-day feeding period is recommended in studies on feed-host interactions. Since, the overall gut microbiota continuously changed until day 30, a future research should investigate the further trends of their occurrence.
Data Availability Statement
The datasets presented in this study can be found in online repositories. The names of the repository/repositories and accession number(s) can be found below: www.ncbi.nlm.nih.gov/bioproject, PRJNA743247.
Ethics Statement
The animal study was reviewed and approved by Swedish Board of Agriculture (diary number: 5.8.18-16347/2017).
Author Contributions
TL and AK conceived the study and experimental design. AS and SK carried out the experimental trial, participated in sampling of the fish material, and wrote the first draft of the manuscript. AS, SK, AV, and ML were performed the feed optimization and feed production. AS and JD were responsible for the DNA analysis and performed the data analysis. TL, AV, and JD participated in editing the final manuscript. SK, JF, and MT were involved in fungus production. All authors contributed to manuscript revision, and read and approved the submitted version.
Conflict of Interest
The authors declare that the research was conducted in the absence of any commercial or financial relationships that could be construed as a potential conflict of interest.
Publisher’s Note
All claims expressed in this article are solely those of the authors and do not necessarily represent those of their affiliated organizations, or those of the publisher, the editors and the reviewers. Any product that may be evaluated in this article, or claim that may be made by its manufacturer, is not guaranteed or endorsed by the publisher.
Acknowledgments
We would like to thank Indian Council of Agricultural Research for providing fellowship for AS, and laboratory staff at the Swedish University of Agricultural Sciences for practical support. We would also like to thank the Swedish Agency for Economic and Regional Growth (Tillväxtverket) for providing financial support through a European Regional Development Fund.
Supplementary Material
The Supplementary Material for this article can be found online at: https://www.frontiersin.org/articles/10.3389/fmars.2021.728569/full#supplementary-material
Footnotes
- ^ http://ccb.jhu.edu/software/FLASH/
- ^ http://qiime.org/scripts/split_libraries_fastq.html
- ^ http://drive5.com/uparse/
- ^ http://www.arb-silva.de/
References
Abro, R., Moazzami, A. A., Lindberg, J. E., and Lundh, T. (2014). Metabolic insights in Arctic charr (Salvelinus alpinus) fed with zygomycetes and fish meal diets as assessed in liver using nuclear magnetic resonance (NMR) spectroscopy. Int. Aquat. Res. 6, 1–11. doi: 10.1007/s40071-014-0063-9
AOAC (1995). Official Methods of Analysis of AOAC International. Washington, DC: Association of Official Analytical Chemists.
Austin, B. (2006). The bacterial microflora of fish, revised. Sci. World J. 6, 931–945. doi: 10.1100/tsw.2006.181
Bokulich, N. A., Subramanian, S., Faith, J. J., Gevers, D., Gordon, J. I., Knight, R., et al. (2013). Quality-filtering vastly improves diversity estimates from Illumina amplicon sequencing. Nat. Methods 10, 57–59. doi: 10.1038/nmeth.2276
Camp, J. G., Jazwa, A. L., Trent, C. M., and Rawls, J. F. (2012). Intronic cis-regulatory modules mediate tissue-specific and microbial control of angptl4/fiaf transcription. PLoS Genet. 8:e1002585. doi: 10.1371/journal.pgen.1002585
Caporaso, J. G., Kuczynski, J., Stombaugh, J., Bittinger, K., Bushman, F. D., Costello, E. K., et al. (2010). QIIME allows analysis of high-throughput community sequencing data. Nat. Methods 7:335. doi: 10.1038/nmeth.f.303
Catalán, N., Villasante, A., Wacyk, J., Ramírez, C., and Romero, J. (2018). Fermented soybean meal increases lactic acid bacteria in gut microbiota of Atlantic Salmon (Salmo salar). Probiotics Antimicrob. Proteins 10, 566–576. doi: 10.1007/s12602-017-9366-7
Chai, W., and Udén, P. (1998). An alternative oven method combined with different detergent strengths in the analysis of neutral detergent fibre. Anim. Feed Sci. Technol. 74, 281–288. doi: 10.1016/S0377-8401(98)00187-4
Cho, C. (1979). “Apparent digestibility measurements in feedstuffs for rainbow trout,” in Proceedings of The World Symposium On Finfish Nutrition And Fishfeed Technology, eds J. Halver and K. Tiews (Berlin: Heenemann), 239–247.
Dai, Z.-L., Wu, G., and Zhu, W.-Y. (2011). Amino acid metabolism in intestinal bacteria: links between gut ecology and host health. Front. Biosci. 16:1768–1786. doi: 10.2741/3820
Davila, A.-M., Blachier, F., Gotteland, M., Andriamihaja, M., Benetti, P.-H., Sanz, Y., et al. (2013). Re-print of “Intestinal luminal nitrogen metabolism: role of the gut microbiota and consequences for the host”. Pharmacol. Res. 69, 114–126. doi: 10.1016/j.phrs.2013.01.003
Dawood, M. A., Koshio, S., Ishikawa, M., Yokoyama, S., El Basuini, M. F., Hossain, M. S., et al. (2016). Effects of dietary supplementation of Lactobacillus rhamnosus or/and Lactococcus lactis on the growth, gut microbiota and immune responses of red sea bream, Pagrus major. Fish Shellfish Immunol. 49, 275–285. doi: 10.1016/j.fsi.2015.12.047
de Bruijn, I., Liu, Y., Wiegertjes, G. F., and Raaijmakers, J. M. (2018). Exploring fish microbial communities to mitigate emerging diseases in aquaculture. FEMS Microbiol. Ecol. 94:fix161. doi: 10.1093/femsec/fix161
Edgar, R. C. (2013). UPARSE: highly accurate OTU sequences from microbial amplicon reads. Nat. Methods 10:996. doi: 10.1038/nmeth.2604
Ferreira, J. A., Lennartsson, P. R., and Taherzadeh, M. J. (2014). Production of ethanol and biomass from thin stillage using food-grade zygomycetes and ascomycetes filamentous fungi (vol 7, pg 3872, 2014). Energies 7, 4199–4201. doi: 10.3390/en7074199
Ferreira, J. A., Lennartsson, P. R., and Taherzadeh, M. J. (2015). Production of ethanol and biomass from thin stillage by Neurospora intermedia: a pilot study for process diversification. Eng. Life Sci. 15, 751–759. doi: 10.1002/elsc.201400213
Gajardo, K., Jaramillo-Torres, A., Kortner, T. M., Merrifield, D. L., Tinsley, J., Bakke, A. M., et al. (2017). Alternative protein sources in the diet modulate microbiota and functionality in the distal intestine of Atlantic salmon (Salmo salar). Appl. Environ. Microbiol. 83, e2615–e2616. doi: 10.1128/AEM.02615-16
Gatesoupe, F.-J. (2008). Updating the importance of lactic acid bacteria in fish farming: natural occurrence and probiotic treatments. J. Mol. Microbiol. Biotechnol. 14, 107–114. doi: 10.1159/000106089
Gmoser, R., Ferreira, J. A., Lundin, M., Taherzadeh, M., and Lennartsson, P. (2018). Pigment production by the edible filamentous fungus Neurospora intermedia. Fermentation 4:11. doi: 10.3390/fermentation4010011
Hartviksen, M., Vecino, J., Ringø, E., Bakke, A. M., Wadsworth, S., and Krogdahl, Å, et al. (2014). Alternative dietary protein sources for Atlantic salmon (Salmo salar L.) effect on intestinal microbiota, intestinal and liver histology and growth. Aquacult. Nutr. 20, 381–398. doi: 10.1111/anu.12087
Heo, W.-S., Kim, Y.-R., Kim, E.-Y., Bai, S. C., and Kong, I.-S. (2013). Effects of dietary probiotic, Lactococcus lactis subsp. lactis I2, supplementation on the growth and immune response of olive flounder (Paralichthys olivaceus). Aquaculture 376, 20–24. doi: 10.1016/j.aquaculture.2012.11.009
Holben, W., Williams, P., Saarinen, M., Särkilahti, L., and Apajalahti, J. (2002). Phylogenetic analysis of intestinal microflora indicates a novel Mycoplasma phylotype in farmed and wild salmon. Microb. Ecol. 44, 175–185. doi: 10.1007/s00248-002-1011-6
Hovda, M. B., Fontanillas, R., McGurk, C., Obach, A., and Rosnes, J. T. (2012). Seasonal variations in the intestinal microbiota of farmed Atlantic salmon (Salmo salar L.). Aquacult. Res. 43, 154–159. doi: 10.1111/j.1365-2109.2011.02805.x
Huyben, D., Nyman, A., Vidaković, A., Passoth, V., Moccia, R., Kiessling, A., et al. (2017). Effects of dietary inclusion of the yeasts Saccharomyces cerevisiae and Wickerhamomyces anomalus on gut microbiota of rainbow trout. Aquaculture 473, 528–537. doi: 10.1016/j.aquaculture.2017.03.024
Huyben, D., Sun, L., Moccia, R., Kiessling, A., Dicksved, J., and Lundh, T. (2018). Dietary live yeast and increased water temperature influence the gut microbiota of rainbow trout. J. Appl. Microbiol. 124, 1377–1392. doi: 10.1111/jam.13738
Ingerslev, H.-C., Strube, M. L., von Gersdorff Jørgensen, L., Dalsgaard, I., Boye, M., and Madsen, L. (2014a). Diet type dictates the gut microbiota and the immune response against Yersinia ruckeri in rainbow trout (Oncorhynchus mykiss). Fish Shellfish Immunol. 40, 624–633. doi: 10.1016/j.fsi.2014.08.021
Ingerslev, H.-C., von Gersdorff Jørgensen, L., Strube, M. L., Larsen, N., Dalsgaard, I., Boye, M., et al. (2014b). The development of the gut microbiota in rainbow trout (Oncorhynchus mykiss) is affected by first feeding and diet type. Aquaculture 424, 24–34. doi: 10.1016/j.aquaculture.2013.12.032
Karimi, S., Mahboobi Soofiani, N., Lundh, T., Mahboubi, A., Kiessling, A., and Taherzadeh, M. J. (2019a). Evaluation of filamentous fungal biomass cultivated on Vinasse as an alternative nutrient source of fish feed: protein, lipid, and mineral composition. Fermentation 5:99. doi: 10.3390/fermentation5040099
Karimi, S., Soofiani, N. M., Lundh, T., Mahboubi, A., Kiessling, A., and Taherzadeh, M. J. (2019b). Evaluation of filamentous fungal biomass cultivated on vinasse as an alternative nutrient source of fish feed: protein, lipid, and mineral composition. Ferment. Basel 5:99.
Langeland, M., Vidakovic, A., Vielma, J., Lindberg, J., Kiessling, A., and Lundh, T. (2016). Digestibility of microbial and mussel meal for Arctic charr (Salvelinus alpinus) and Eurasian perch (Perca fluviatilis). Aquacult. Nutr. 22, 485–495. doi: 10.1111/anu.12268
Li, X., Yan, Q., Ringø, E., Wu, X., He, Y., and Yang, D. (2016). The influence of weight and gender on intestinal bacterial community of wild largemouth bronze gudgeon (Coreius guichenoti, 1874). BMC Microbiol. 16:191. doi: 10.1186/s12866-016-0809-1
Lowrey, L., Woodhams, D. C., Tacchi, L., and Salinas, I. (2015). Topographical mapping of the rainbow trout (Oncorhynchus mykiss) microbiome reveals a diverse bacterial community with antifungal properties in the skin. Appl. Environ. Microbiol. 81, 6915–6925. doi: 10.1128/AEM.01826-15
Lyons, P. P., Turnbull, J. F., Dawson, K. A., and Crumlish, M. (2017b). Phylogenetic and functional characterization of the distal intestinal microbiome of rainbow trout Oncorhynchus mykiss from both farm and aquarium settings. J. Appl. Microbiol. 122, 347–363. doi: 10.1111/jam.13347
Lyons, P. P., Turnbull, J. F., Dawson, K. A., and Crumlish, M. (2017a). Exploring the microbial diversity of the distal intestinal lumen and mucosa of farmed rainbow trout O ncorhynchus mykiss (Walbaum) using next generation sequencing (NGS). Aquacult. Res. 48, 77–91. doi: 10.1111/are.12863
Magoč, T., and Salzberg, S. L. (2011). FLASH: fast length adjustment of short reads to improve genome assemblies. Bioinformatics 27, 2957–2963. doi: 10.1093/bioinformatics/btr507
Merrifield, D., Bradley, G., Baker, R., and Davies, S. (2010). Probiotic applications for rainbow trout (Oncorhynchus mykiss Walbaum) II. Effects on growth performance, feed utilization, intestinal microbiota and related health criteria postantibiotic treatment. Aquacult. Nutr. 16, 496–503. doi: 10.1111/j.1365-2095.2009.00688.x
Michl, S. C., Beyer, M., Ratten, J.-M., Hasler, M., LaRoche, J., and Schulz, C. (2019). A diet-change modulates the previously established bacterial gut community in juvenile brown trout (Salmo trutta). Sci. Rep. 9, 1–12. doi: 10.1038/s41598-019-38800-7
Michl, S. C., Ratten, J.-M., Beyer, M., Hasler, M., LaRoche, J., and Schulz, C. (2017). The malleable gut microbiome of juvenile rainbow trout (Oncorhynchus mykiss): diet-dependent shifts of bacterial community structures. PLoS One 12:e0177735. doi: 10.1371/journal.pone.0177735
Nalage, D., Khedkar, G., Kalyankar, A., Sarkate, A. P. Sr., Vb, B., and Khedkar, C. (2016). “Single cell proteins,” in The Encyclopedia of Food and Health, eds B. Caballero, F. Toldra, and P. Finglas (London: Academic Press), 790–794. doi: 10.1016/B978-0-12-384947-2.00628-0
Namsolleck, P., Thiel, R., Lawson, P., Holmstrøm, K., Rajilic, M., Vaughan, E. E., et al. (2004). Molecular methods for the analysis of gut microbiota. Microb. Ecol. Health Dis. 16, 71–85. doi: 10.1080/08910600410032367
Navarrete, P., Fuentes, P., De la Fuente, L., Barros, L., Magne, F., Opazo, R., et al. (2013). Short-term effects of dietary soybean meal and lactic acid bacteria on the intestinal morphology and microbiota of Atlantic salmon (Salmo salar). Aquacult. Nutr. 19, 827–836. doi: 10.1111/anu.12047
Nayak, S. K. (2010). Role of gastrointestinal microbiota in fish. Aquacult. Res. 41, 1553–1573. doi: 10.1111/j.1365-2109.2010.02546.x
Neis, E. P., Dejong, C. H., and Rensen, S. S. (2015). The role of microbial amino acid metabolism in host metabolism. Nutrients 7, 2930–2946. doi: 10.3390/nu7042930
Nordic Committee on Food Analysis (1976). Nitrogen Determination in Food and Feed According to Kjeldahl. No 6, 3rd Edn. Esbo: Nordic committee on food analysis.
Nyman, A., Huyben, D., Lundh, T., and Dicksved, J. (2017). Effects of microbe-and mussel-based diets on the gut microbiota in Arctic charr (Salvelinus alpinus). Aquacult. Rep. 5, 34–40. doi: 10.1016/j.aqrep.2016.12.003
Official Journal of the European Union (2009). Determination of Crude Oils and Fat No 152/2009. Method B.
Perez-Fuentes, J. A., Perez-Rostro, C. I., Hernandez-Vergara, M. P., and Monroy-Dosta, M. D. (2018). Variation of the bacterial composition of biofloc and the intestine of Nile tilapia Oreochromis niloticus, cultivated using biofloc technology, supplied different feed rations. Aquacult. Res. 49, 3658–3668. doi: 10.1111/are.13834
Pinheiro, J., Bates, D., DebRoy, S., Sarkar, D., and R Core Team (2014). nlme: Linear and Nonlinear Mixed Effects Models. R Package Version 3.1-117. Available online at http://CRAN.R-project.org/package=nlme (accessed May 21, 2020).
Quast, C., Pruesse, E., Yilmaz, P., Gerken, J., Schweer, T., Yarza, P., et al. (2012). The SILVA ribosomal RNA gene database project: improved data processing and web-based tools. Nucleic Acids Res. 41, D590–D596. doi: 10.1093/nar/gks1219
R Core Team (2015). R: A Language And Environment For Statistical Computing. Vienna: R Foundation for Statistical Computing.
Ricaud, K., Rey, M., Plagnes-Juan, E., Larroquet, L., Even, M., Quillet, E., et al. (2018). Composition of intestinal microbiota in two lines of rainbow trout (Oncorhynchus Mykiss) divergently selected for muscle fat content. Open Microbiol. J. 12:308. doi: 10.2174/1874285801812010308
Ringø, E., and Gatesoupe, F.-J. (1998). Lactic acid bacteria in fish: a review. Aquaculture 160, 177–203. doi: 10.1016/S0044-8486(97)00299-8
Ringø, E., Zhou, Z., Vecino, J. G., Wadsworth, S., Romero, J., and Krogdahl, Å, et al. (2016). Effect of dietary components on the gut microbiota of aquatic animals. A never-ending story? Aquacult. Nutr. 22, 219–282. doi: 10.1111/anu.12346
Rombouts, J. L., Kranendonk, E. M. M., Regueira, A., Weissbrodt, D. G., Kleerebezem, R., and van Loosdrecht, M. C. M. (2020). Selecting for lactic acid producing and utilising bacteria in anaerobic enrichment cultures. Biotechnol. Bioeng. 117, 1281–1293. doi: 10.1002/bit.27301
Schmidt, V., Amaral-Zettler, L., Davidson, J., Summerfelt, S., and Good, C. (2016). Influence of fishmeal-free diets on microbial communities in Atlantic salmon (Salmo salar) recirculation aquaculture systems. Appl. Environ. Microbiol. 82, 4470–4481. doi: 10.1128/AEM.00902-16
Seppola, M., Olsen, R. E., Sandaker, E., Kanapathippillai, P., Holzapfel, W., and Ringø, E. (2006). Random amplification of polymorphic DNA (RAPD) typing of carnobacteria isolated from hindgut chamber and large intestine of Atlantic cod (Gadus morhua L.). Syst. Appl. Microbiol. 29, 131–137. doi: 10.1016/j.syapm.2005.07.006
Sun, S., Su, Y., Yu, H., Ge, X., and Zhang, C. (2020). Starvation affects the intestinal microbiota structure and the expression of inflammatory-related genes of the juvenile blunt snout bream, Megalobrama amblycephala. Aquaculture 517:734764. doi: 10.1016/j.aquaculture.2019.734764
Sun, Y. Z., Yang, H. L., Ma, R. L., Song, K., and Li, J. S. (2012). Effect of Lactococcus lactis and Enterococcus faecium on growth performance, digestive enzymes and immune response of grouper Epinephelus coioides. Aquacult. Nutr. 18, 281–289. doi: 10.1111/j.1365-2095.2011.00894.x
Vidakovic, A., Langeland, M., Sundh, H., Sundell, K., Olstorpe, M., Vielma, J., et al. (2016). Evaluation of growth performance and intestinal barrier function in Arctic Charr (Salvelinus alpinus) fed yeast (Saccharomyces cerevisiae), fungi (Rhizopus oryzae) and blue mussel (Mytilus edulis). Aquacult. Nutr. 22, 1348–1360. doi: 10.1111/anu.12344
Wang, E., Yuan, Z., Wang, K., Gao, D., Liu, Z., and Liles, M. R. (2019). Consumption of florfenicol-medicated feed alters the composition of the channel catfish intestinal microbiota including enriching the relative abundance of opportunistic pathogens. Aquaculture 501, 111–118. doi: 10.1016/j.aquaculture.2018.11.019
Wang, Q., Garrity, G. M., Tiedje, J. M., and Cole, J. R. (2007). Naive Bayesian classifier for rapid assignment of rRNA sequences into the new bacterial taxonomy. Appl. Environ. Microbiol. 73, 5261–5267. doi: 10.1128/AEM.00062-07
Won, S., Hamidoghli, A., Choi, W., Park, Y., Jang, W. J., Kong, I.-S., et al. (2020). Effects of Bacillus subtilis WB60 and Lactococcus lactis on growth, immune responses, histology and gene expression in Nile Tilapia, Oreochromis niloticus. Microorganisms 8:67. doi: 10.3390/microorganisms8010067
Wong, S., Waldrop, T., Summerfelt, S., Davidson, J., Barrows, F., Kenney, P. B., et al. (2013). Aquacultured rainbow trout (Oncorhynchus mykiss) possess a large core intestinal microbiota that is resistant to variation in diet and rearing density. Appl. Environ. Microbiol. 79, 4974–4984. doi: 10.1128/AEM.00924-13
Xia, Y., Cao, J., Wang, M., Lu, M., Chen, G., Gao, F., et al. (2019). Effects of Lactococcus lactis subsp. lactis JCM5805 on colonization dynamics of gut microbiota and regulation of immunity in early ontogenetic stages of tilapia. Fish Shellfish Immunol. 86, 53–63. doi: 10.1016/j.fsi.2018.11.022
Xia, Y., Lu, M., Chen, G., Cao, J., Gao, F., Wang, M., et al. (2018). Effects of dietary Lactobacillus rhamnosus JCM1136 and Lactococcus lactis subsp. lactis JCM5805 on the growth, intestinal microbiota, morphology, immune response and disease resistance of juvenile Nile tilapia, Oreochromis niloticus. Fish Shellfish Immunol. 76, 368–379. doi: 10.1016/j.fsi.2018.03.020
Yan, Q., Li, J., Yu, Y., Wang, J., He, Z., Van Nostrand, J. D., et al. (2016). Environmental filtering decreases with fish development for the assembly of gut microbiota. Environ. Microbiol. 18, 4739–4754. doi: 10.1111/1462-2920.13365
Keywords: rainbow trout, filamentous fungi, duration of feeding, gut microbiome, fish, Lactococcus, amplicon sequencing
Citation: Singh A, Karimi S, Vidakovic A, Dicksved J, Langeland M, Ferreira JA, Taherzadeh MJ, Kiessling A and Lundh T (2021) Dietary Filamentous Fungi and Duration of Feeding Modulates Gut Microbial Composition in Rainbow Trout (Oncorhynchus mykiss). Front. Mar. Sci. 8:728569. doi: 10.3389/fmars.2021.728569
Received: 21 June 2021; Accepted: 27 September 2021;
Published: 21 October 2021.
Edited by:
Fotini Kokou, Wageningen University and Research, NetherlandsReviewed by:
Alma Hernández De Rojas, Spanish Institute of Oceanography (IEO), SpainSven Wuertz, Leibniz-Institute of Freshwater Ecology and Inland Fisheries (IGB), Germany
Copyright © 2021 Singh, Karimi, Vidakovic, Dicksved, Langeland, Ferreira, Taherzadeh, Kiessling and Lundh. This is an open-access article distributed under the terms of the Creative Commons Attribution License (CC BY). The use, distribution or reproduction in other forums is permitted, provided the original author(s) and the copyright owner(s) are credited and that the original publication in this journal is cited, in accordance with accepted academic practice. No use, distribution or reproduction is permitted which does not comply with these terms.
*Correspondence: Torbjörn Lundh, VG9yYmomI3gwMEY2O3JuLmx1bmRoQHNsdS5zZQ==
†These authors have contributed equally to this work and share first authorship