- 1GeoAqua Consulting, Vineyard Haven, MA, United States
- 2Biology Department, Woods Hole Oceanographic Institution, Woods Hole, MA, United States
- 3MARUM – Center for Marine Environmental Sciences, University of Bremen, Bremen, Germany
Submarine fluids emissions in the form of geothermal vents are widespread in a variety of geological settings ranging from volcanic to tectonically active areas. This overview aims to describe representative examples of submarine vents in shallow-water areas around the globe. The areas described include: Iceland, Azores, Mediterranean Sea (Italy and Greece), Caribbean, Baja California, Japan, Papua, New Zealand, Taiwan. Common and divergent characteristics in terms of origin and geochemistry of the emitted fluids and their impact on the indigenous organisms and the surrounding environment have been identified. In the hottest vents seawater concentration is common as well as some water vapor phase separation. Carbon dioxide is the most common gas often associated with compounds of sulfur and methane. In several vents precipitation of minerals can be identified in the surrounding sediments. The analyses of the microbial communities often revealed putative chemoautotrophs, with Campylobacteria abundantly present at many vents where reduced sulfur compounds are available. The techniques that can be used for the detection and quantification of underwater vents are also described, including geophysical and geochemical tools. Finally, the main geobiological effects due to the presence of the hydrothermal activity and the induced changes in water chemistry are assessed.
Introduction
Submarine vents can be found worldwide in tectonically active environments ranging from volcanic areas to active tectonic lineaments. Each emission has specific geochemical signatures as a function of its origin (Dando, 2010), and even neighboring systems can vary significantly in terms of their geochemistry (Megalovasilis, 2014). Here we describe representative examples of such shallow-water vents (Figure 1).
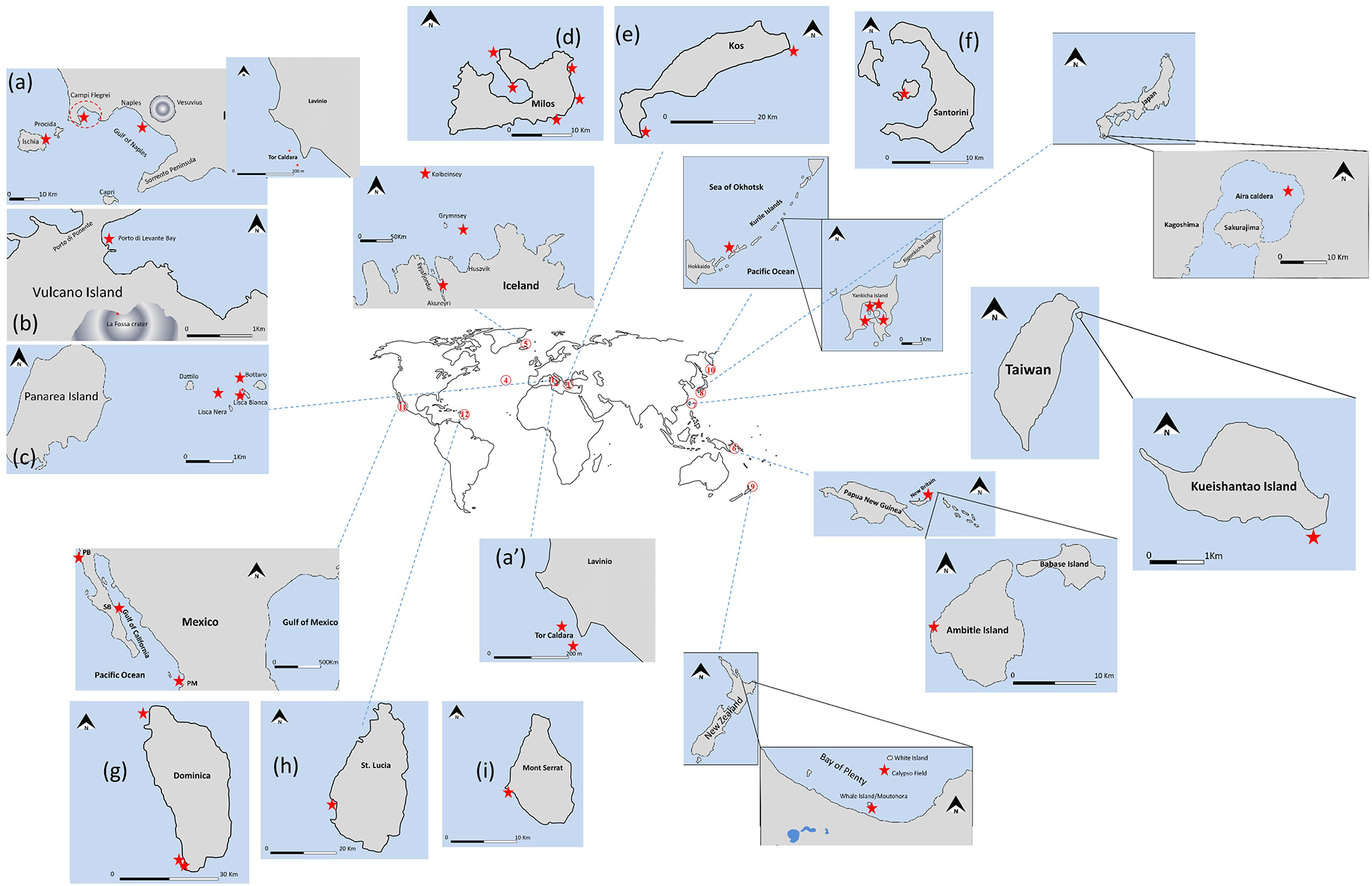
Figure 1. General location of the presented areas 1: Gulf of Naples (a), Tor Caldara (a’), 2: Aeolian Islands: Vulcano (b), Panarea (c), 3: Aegean Sea [Milos (d), Kos (e), Santorini (f)], 4: Azores Islands, 5: Iceland, 6: Papua New Guinea, 7: Taiwan, 8: Kagoshima Bay, 9: New Zealand, 10: Kurile Islands, 11: Baja California, 12: Lesser Antilles [Dominica (g), St. Lucia (h), Mont Serrat (i)].
Many of the vents are at depths that can be safely reached by scientific divers; allowing the scientists to directly study the phenomena with a far higher degree of detail than that achievable with any remotely operated system. The shallow-water setting is also useful for cost-effective testing and validation of sampling and monitoring techniques, which can then also be applied to deep-water environments.
Submarine hydrothermal vents have different chemical signatures and the effects on the surrounding environment are a function of the composition of the fluids, the volume of the seeps and the spatial and temporal distribution of the emissions. Figure 2 presents schematic diagrams of the fluids exchanges in geothermal vents. The fluids emitted from geothermal vents are generally characterized by low pH, negative redox potential and high temperatures. The gas phase has often high concentrations of CO2, H2S, CH4 of thermogenic origin and He from magmatic and mantle sources (Chiodini et al., 2006); steam is also occasionally present when the temperature is above the boiling point for the given depth. In the liquid phase, brines formed by phase separation of seawater, entrained seawater, and groundwater originating from coastal aquifers can be present in variable percentages (Dando et al., 2000; Tassi et al., 2009). The fluids, following the mixing with colder seawater and during the passage through the sedimentary layers, will also precipitate solid deposits including a variety of sulfosalts, metal sulfides, amorphous silica, Mn, Fe-oxyhydroxides and calcite; there is also an enrichment in potentially toxic elements such as Hg, As and heavy metals (Pichler and Veizer, 2004; Varnavas and Cronan, 2005; Conte and Caramanna, 2010). The emissions can also be associated with the deposition of large hydrothermal deposits (Binns and Scott, 1993; Savelli et al., 1999), some of which are considered to be at the origin of significant ore bodies such as the Kuroko-type deposits (Ohmoto and Skinner, 1983; Urabe and Kusakabe, 1990).
Submarine vents and the associated hydrothermal fluids play an important role in shaping the chemistry of seawater in terms of enrichment in specific ions, chemical compounds, dissolved gas concentration and induced pH and redox changes (Dittmar and Koch, 2006; Lang et al., 2006; Walker et al., 2008; Bennett et al., 2011; Gomez-Saez et al., 2016). Shallow-water hydrothermal systems often expel high concentrations of metal, with some of the metals serving as micronutrients in oligotrophic waters (e.g., Fe) while others, such as Cu, may be essential in lower concentrations, but toxic at higher concentrations. Three basic processes can occur to the dissolved metals under hydrothermal conditions: (1) oxidation and precipitation, (2) complexation by inorganic ligands, (3) complexation by organic ligands. A study by Kleint et al. (2015) indicated organic complexation of Cu in hydrothermal fluids of different shallow-water hydrothermal systems. Many microorganisms living in these habitats produce small organic molecules, ligands, that are able to form complexes with different metals, to either enhance their bioavailability or to decrease their toxicity (Klevenz et al., 2012). Arsenic (As) is another potentially toxic element that can be released in higher concentrations from hydrothermal vents, and organisms at vents have been shown to express detoxification mechanisms and specialized microbes exist at vents that can use it to generate energy (e.g., Ahmann et al., 1997; Price et al., 2013a; Callac et al., 2017). Iron is an essential micronutrient for all marine organisms, and it has been shown to be transported far away, i.e., thousands of km from deep-sea hydrothermal vents, even reaching the photic zone, due to dissolved-particulate exchange processes (Fitzsimmons et al., 2017). The transformation and distribution of soluble and complexed iron and other metals in shallow vent areas is a major gap in knowledge, which needs to be addressed as these compounds directly impact the photic zone of coastal oceans. The impact that these emissions have on the marine environment includes acidification, changes in dissolved gas concentration leading to potential hypoxic conditions, positive thermal anomalies and toxic metals accumulation in the sediments surrounding the vents which can alter the biological functions of marine organisms living close to the seepage (Tarasov et al., 1999; Cunha et al., 2008; Couto et al., 2010).
Some of the shallow-water vents with fluids that have a high percentage of CO2 have been considered as natural analogs for the study of the consequences of ocean acidification (Hall-Spencer et al., 2008; Vizzini et al., 2010) or as field-laboratories to develop detection and monitoring techniques for potential seepage from sub-seabed CO2 geological storage areas (Caramanna et al., 2005; Shitashima et al., 2008). The benthic communities of shallow-water venting areas have many characteristics in common with communities subject to anthropogenic impact (Tarasov, 2006). Shallow-water vents are frequently within the photic zone and the emitted hydrothermal fluids are often enriched in nutrients and reduced compounds, providing two energy sources for primary production: photosynthesis and chemosynthesis, using the energy generated through the oxidation of the reduced compounds to fix CO2. Hydrothermal systems harbor diverse microbial communities, with anaerobic thermophiles in particular thought to share characteristics with evolutionary early life forms on Earth (Stetter, 2006). The first isolated hyperthermophiles where obtained from shallow-water hydrothermal systems (Stetter, 2006) and to date a large number of extremophiles have been identified, able to utilize practically all reduced compounds of volcanic origin as electron donors in energy metabolism (Jochimsen et al., 1997; Sievert and Kuever, 2000; Oremland and Stolz, 2003; Handley et al., 2009; Price and Giovannelli, 2017). With the study of microbes from hydrothermal environments, many questions ranging from the adaptation to extreme conditions, microbe-metal interactions, to the origin of life might be answered.
This review provides an overview of submarine fluid emissions in shallow waters (< 200 m), their impact on the environment, the biota associated with these fluids, the techniques that can be applied for their detection and monitoring, and the insights that can be gained from their study. We intend this review as a reference work for the geology and geobiology of the different shallow-water venting sites that have been studied so far, rather than providing a comparative assessment of shallow-water and deep-sea hydrothermal systems, as for example given in the review by Price and Giovannelli (2017).
Geological Settings, Geographical Distribution, Geochemistry and Geomicrobiology of Submarine Shallow-Water Vents
Due to the presence of active plate margins, subduction zones, volcanic island arcs and hotspots, active volcanism is present in isolated islands, archipelagos, coastal zones and ocean ridges (Batiza, 1982; Simkin et al., 1989; Wilson, 1993; Siebert et al., 2011; Nomikou et al., 2013). Submarine hydrothermal vents associated with volcanically active areas emit fluids of variable composition and temperature with a chemical signature clearly different from that of the surrounding seawater (Price and Giovannelli, 2017). In this review, we present the characteristics of a selection of submarine fluid emissions in different volcanically and tectonically active areas.
Gulf of Naples (Italy)
The Gulf of Naples is a large bay along the southern Tyrrhenian shore of Italy. The gulf is delimited by Sorrento Peninsula and Capri Island on the south and by the Campi Flegrei, Pozzuoli Bay, Procida Mount and the islands of Procida and Ischia on the north. The dominant feature is the active Somma-Vesuvius stratovolcano along the shore a few km southeast of the city of Naples. The Somma-Vesuvius was originated by a series of effusive and Plinian explosive eruptions, while Pozzuoli Bay is the result of the collapse of a larger caldera following the eruption of the Campi Flegrei area 12 ka ago. There is strong geothermal activity with hot springs and gas emissions both onshore and offshore in the area (Scandone et al., 1991). Several gas vents are aligned along NW-SE tectonic structures on the seafloor of the Gulf of Naples about 2.5 km offshore the Vesuvius at depth ranging from 50 to 100 m. The acoustic signature of the emitted bubbles and the presence of sediments “charged” by gas below the seafloor is clearly visible along seismic profiles (Milia et al., 1998).
In the Pozzuoli Bay, other vents are present in water depth ranging from a few meters to about 30 m. The emitted gas is predominantly composed of CO2 (up to 985.7 μmol mol–1) with smaller amounts of H2S (up to 7.2 μmol mol–1) and CH4 (up to 5.9 μmol mol–1); the measured temperature of the emitted fluids is around 90°C. Based on the 3He/4He isotope ratio and geothermometry considerations, the source is likely to be a deep-seated hydrothermal/magmatic body with mantle contribution with T above 350°C (Tedesco et al., 1990; Orlando et al., 2011).
Another very active area in the Gulf of Naples is the island of Ischia. The island is part of the same volcanic district of Campi Flegrei and Somma-Vesuvius; a series of deposits formed by submarine explosive eruptions, aligned along a NE-SW main fault system (Paoletti et al., 2009). Several springs fed by thermal waters and fumaroles on the island are known and have been used since Roman times. The emitted fluid is represented by water vapor in equilibrium with CO2, H2S, CH4, H2 and Ar. The origin of such emissions is considered to be linked to two different sources: one aquifer at around 400 m of depth and with T around 250°C, and a deeper aquifer at about 900 m depth and at 300°C. The less deep aquifer is recharged by meteoric water with a component of seawater infiltrating along the shoreline as highlighted by an increase in Total Dissolved Solids (TDS) toward the coastal areas and by the Na/Cl ratio. The source of CO2 is a deep cooling magmatic body; the CO2 during its upward movement is modified by crustal input undergoing a δ13C differentiation through interaction with the aquifers (Inguaggiato et al., 2000; Chiodini et al., 2004; Di Napoli et al., 2009). A series of submarine vents are located in shallow-water (less than 10 m of depth) along the eastern shore of the island near the Castello Aragones. The vents release almost pure CO2 (90 to 95%) with N2 ranging from 3 to 6% and an absence of sulfuric compounds. The presence of CO2 reduces the seawater pH, and the absence of potentially toxic sulfur compounds makes this site a good area to study the effects of ocean acidification on marine biota (Hall-Spencer et al., 2008).
Macrofaunal benthic community patterns of the hydrothermal area of Secca Delle Fumose was studied by Donnarumma et al. (2019), revealing a preference by a few resistant, opportunistic species, such as the gastropod Tritia cuvierii and the polychete Capitella capitata. Low structural and functional diversity with high biomass and dominance of a few species was the result of investigating the nematofauna of the same site (Baldrighi et al., 2020). Combining investigations of macro and meiofauna, acidification was found to markedly affect β-diversity in the system (Appolloni et al., 2020). An increase in heterogeneity among sample replicates coupled to a decrease in number of taxa was argued as an indicator of redundancy loss and of resilience capacity.
Aeolian Islands (Italy)
The Aeolian Islands lie in the Southern Tyrrhenian Sea, twelve miles north of the coast of Sicily. The archipelago is composed of seven main islands (Alicudi, Filicudi, Lipari, Panarea, Salina, Stromboli, and Vulcano) and several islets and seamounts in a very active seismic and volcanic area along the south-eastern slope of the Tyrrhenian abyssal plain. The first volcanic events are dated to be 1.3 Ma old based on the age of the basalt emitted from Sisifo seamount, which is considered to be part of the submerged section of the archipelago, with the islands of Vulcano and Stromboli still hosting active volcanoes. Salina, Lipari and Vulcano islands are aligned along a NNW-SSE striking fault system and correspond to the outcrop of a volcanic ridge where activity began 188 ka in Lipari and moved toward Salina with deposits of 168 ka and then south to Vulcano Island where the current active system is located. On the eastern sector of the archipelago, the volcanism started in Panarea 10 ka ago and is now active on Stromboli (De Rosa et al., 2003 and references therein). Hydrothermal activity is present on the islands both onshore and offshore with fumaroles and fluids emissions. In particular, submarine vents can be found close to the coast of Vulcano Island in the bay of Porto di Levante and east of Panarea Island (Dando et al., 1999).
The activity on Vulcano Island following the last eruption in 1888-1890 is currently characterized by fumarolic emissions along the rim of the La Fossa crater and close to the shore of Porto di Levante with temperatures up to 500°C and up to 100°C, respectively (Capasso et al., 1999). Several vents are active in very shallow water (from less than 1 m to about 20 m) in the Porto di Levante bay area (Figure 1). The emitted fluids are characterized by low pH [below 6, (Aiuppa et al., 2021)], enrichment in Si, Fe, Mg and H2S and CO2 both as free and dissolved gases. The temperature is above the ambient water with values up to 65.5°C. The likely origin of the vents is the mixing between volcanic fluids and ambient seawater plus local contribution of groundwater in some of the vents (Sedwick and Stuben, 1996).
Another active area is located east of the island of Panarea close to the islets of Bottaro and Lisca bianca (Figure 1). This area was affected by a strong submarine eruption of gas in November 2002 (Chiodini et al., 2006); the most active vent generated a pseudo-crater structure on the seafloor at about 15 m of depth emitting gas flux estimated at 4.4 ∗ 108 l d–1 (Aliani et al., 2010). In subsequent years, the emission decreased, but several active vents still emit fluids at water depths ranging from 8 to 25 meters. The vents are aligned along active faults with N-S, E-W and NE-SW trend. The gas phase is prevalent, and it is mainly composed of CO2 (mean value of 94%), H2S (mean value 2%) and CH4 (mean value 120 ppm). The emitted gas generates plumes of bubbles creating pseudo-convective cells of high vorticity leading to turbulence and entrainment phenomena that affect the gas-water exchange (Espa et al., 2010; Caramanna et al., 2011).
Some of the vents emit hot (temperature ranging from 46 to 135°C), acidic water (pH ranging from 2.6 to 6.0), composed of a mix of deep fluids and seawater, including brines formed by phase separation in the hottest vents (Tassi et al., 2009). The area was sampled and surveyed by scientific divers in 2007 identifying a vent associated with deposition of Pb-As sulfosalts, Zn-Pb sulfides and Ba sulfates precipitating from a hot (T around 150°C) plume emitted from the seafloor at 25 m of depth (Conte and Caramanna, 2010). The precipitates originate from a flat structure outcropping from the surrounding sandy seafloor (Figure 3). Kadar et al. (2012) provided analytical evidence for the presence of a large fraction of seep derived elements in the water column (e.g., approximately 50% of iron, over 80% of Mn, 100% of Cr, S and Zn) in the form of nano sized particles (e.g., < 100 nm) even at typical open ocean pH.
Due to the ease of access and range of conditions, a number of studies have been conducted studying the geochemistry and microbiology of the vents of the Aeolian Islands, in particular around Volcano and Panarea (Gugliandolo and Maugeri, 1993, 2019; Gugliandolo et al., 1999; Manini et al., 2008; Rusch and Amend, 2008; Karuza et al., 2012; Price et al., 2015). These mostly cultivation- or total cell count-based studies showed the presence of various chemoautotrophs, predominantly sulfur-oxidizing bacteria, and heterotrophs, and could also demonstrate the influence of the vents on the distribution of viruses and prokaryotes. A combined approach of denaturant gradient gel electrophoresis (DGGE) and metagenomic sequencing revealed a high diversity of hyperthermophilic archaea (Antranikian et al., 2017). In particular, the Staphylothermus and Thermococcus, and strains of the aerobic hyperthermophilic genus Aeropyrum, were abundant. Regarding the bacterial community, Campylobacteria (formerly Epsilonproteobacteria; reclassified by Waite et al., 2017), especially the genera Sulfurimonas and Sulfurovum, were highly abundant. Amend et al. (2003) could show that oxidation reactions with O2 release significantly more energy than most anaerobic oxidation reactions, but the energy yield is comparable or even higher for several reactions in which NO3–, NO2– or Fe(III) serve as the terminal electron acceptors. Various hyperthermophiles have been isolated from Vulcano (e.g., Gugliandolo and Maugeri, 1993; Simmons and Norris, 2002; Stetter, 2006) as well as thermoactive enzymes for biotechnological applications (e.g., Suleiman et al., 2019).
Tor Caldara (Italy)
Tor Caldara is part of a natural park established in 1988 along the Tyrrenhian shore of southern Latium just south of the city of Rome and close to the village of Lavinio (Figure 1). The area is affected by active emission of endogenic fluids and in the past the sandy deposits that characterize the area were mined to extract the minerals, such as sulfur, precipitated from the fluids. Some geothermal springs were also used for bathing since Roman times (Mancinella et al., 2020).
This zone represents the southern boundaries of the Albani Hills volcanic complex. The Albano crater lake is at 24 km of distance from the area. As relic of the volcanic activity, a large discharge of endogenic gas is present (Barberi et al., 2019). The buried carbonatic Mesozoic basement is the main reservoir for the endogenous gases that rise through a network of faults and fractures mostly following N-S and W-E directions with NE-SW and NW-SE lineaments also well represented. A total release of 20 tons/day over an area of 2.93 km2 has been estimated for the Lavinio-Tor Caldara area (Carapezza et al., 2020). The main component of the gas is CO2 with values in the range of 93 to 99%, H2S is present with concentration of up to 6% (Barberi et al., 2019). The carbonatic basement also hosts the regional aquifer in which the gas dissolves and accumulates, the contribution of meteoric water prevents the fluid emission from becoming geothermic. Helium isotopic analysis suggests that the source of the gas is a deep magma affected by crustal contamination during its primary generation in a subduction process (Barberi et al., 2019). There is a slight correlation between seismic activity in the Albani Hills structure and CO2 degassing, suggesting a possible concause between gas release and stress-strain release at depth (Quattrocchi et al., 2009).
The underwater vents are located a few tens of meter offshore the cliff of Tor Caldara in shallow water (about 3.5 m) and are part of a series of vents aligned along the local fault system. Two areas have been identified: one is characterized by sand deposits covering the seafloor, the other is characterized by rocky outcrops and boulders. This latter is composed by lithified sandy clays of Pliocene age; the same deposits are also present at the foot of the cliff. The vents in the sand deposits are aligned along a distance of about 10 m showing rings of black deposits around the orifices likely due to precipitation of metal-sulfide minerals. The vents on the rocky bottom are colonized by filamentous sulfur-oxidizing bacteria, and recent studies have used these vents as a model system to study the succession and function of chemosynthetic microbial biofilms (Patwardhan et al., 2018, 2021). Part of the rocky outcrop has been formed through bioconstructions by Sabella alveolata (Mancinella et al., 2020). The gas emission is clearly observable in the form of bubble plumes that reach the surface of the sea. The gas is composed mainly of CO2 (76.7%), H2S (23.1%) and CH4 (0.18%); the relatively high concentration of H2S promotes the growth of sulfur-oxidizing bacteria (Patwardhan et al., 2018).
Aegean Islands (Greece)
Volcanism in the Aegean Sea, and more in general in the Hellenic Volcanic arc, is generated by the subduction of the African Plate below the European Plate. The high geothermal gradient in the area produces a strong hydrothermal circulation with a large amount of CO2 generated from degassing processes in the subducted slab coupled with mantle and magmatic contributes and thermal decomposition of the marine carbonates entrained during subduction (Dando et al., 1999 and references therein). The Hellenic volcanic arc has been a very active seismic and volcanic zone since Tertiary times; one of the strongest events was the Minoan eruption of 1400 B.C. that led to the formation of the central caldera of Santorini Island, which reaches about 400 m of depth. Post Minoan eruptions originated the Paleokameni and Neokameni islands in the middle of the caldera; these islands are characterized by the presence of hydrothermal vents in very shallow water (< 10 m), emitting Fe-enriched fluids with occurrence of Mn and As. Pyrite and iron oxy-hydroxides are highly abundant in the sediments, precipitating out upon contact with the cold oxygenated seawater (Varnavas and Cronan, 2005). Several submarine vents, emitting both geothermal waters and gases, are present along the shore of mainland Greece and close to many of the Aegean Islands at depths ranging from a few meters to less than 300 m. These vents are aligned along main tectonic fault lines, which, as in other similar situations, act as preferential paths for the deep-seated fluids to reach the surface. According to Dando et al. (2000), the sources of the fluids can be attributed to (1) magmatic input with mantle-derived He component, (2) seawater-rock interaction in the bedrock and deep crust, and (3) seawater-sediment-microbial interactions in the upper layers of the seafloor sedimentary cover.
The emissions are of geothermal origin with temperatures up to 115°C, which, due to the relatively shallow setting, is above the boiling point of the water, allowing for the generation of a vapor phase (Price et al., 2013b). The liquid phase has three end members: high-salinity brines of deep origin, low-salinity fluids and brines derived from the phase separation, and altered seawater. In Milos, Kos and Santorini islands (Figures 1d–f) the emission rates range between 0.7 and 124.3 l h–1 (at standard pressure and temperature, s. p. t.). It was observed that a large part of the liquid phase originates in seawater infiltrating the sedimentary cover of the seafloor by entrainment through convective cells generated by the rising fluids and gas. The gas phase is composed of CO2 (≤ 88-99%), H2 (∼ 3%), H2S (∼ 3%) and CH4 (∼ 1%) (Dando et al., 1995; Kyriaki et al., 2018). The emission rates range between 4.8 and 96.0 l h–1 (at s.p.t). The total estimates for CO2 emissions from the area are from 9 to 35 Mt year–1, which is considered influential for the global geochemical cycle (Dando et al., 2000). Mineralized deposits are often associated with the vents in form of sulfur deposits and amorphous silica with bacteria growing on the surface leading to biogeochemical processes affecting the chemistry of the emitted fluids (Fitzsimons et al., 1997).
A number of projects have focused on the shallow submarine hydrothermal systems around Milos over the past 20 years or so, making it arguably one of the best studied shallow-water vent areas (reviewed by Bühring and Sievert, 2017). This led to a comparatively good understanding of the geochemistry and geomicrobiology of these sites, particularly in Paleochori Bay. The western part of the Bay is described as being hydrothermally more active (Khimasia et al., 2020), with hydrothermal fluids percolating through permeable sediment, creating distinct zones on the sediment surface. Yellow-orange patches can be found in the center, surrounded by white and brown areas, often occurring in concentric circles of about 2 m in diameter (Sievert et al., 1999; Wenzhöfer et al., 2000; Khimasia et al., 2020). The yellow-orange precipitates are mainly composed of amorphous orpiment-like As-sulfides (As2S3) (Godelitsas et al., 2015), occurring in the hottest areas (≥ 85°C). Using multi-analyte voltammetric profiling in retrieved sediment cores, Yücel et al. (2013) showed that the sediment underlying the white mats are rich in dissolved Fe2+ and Mn2+ and free sulfide. In the lower temperature range (∼30–35°C) on the outer rim of the white patches, brown-colored manganese and iron oxide deposits are present (Yücel et al., 2013). These seafloor features highly depend on hydrodynamic conditions, being influenced by tides and subseafloor microearthquakes (Fitzsimons et al., 1997; Sievert et al., 1999; Aliani et al., 2004; Yücel et al., 2013). Hydrothermal particles from the submarine vents studied were dominated by Fe, Ca, Si and Al and strongly enriched in Mn, Cu, Pb, Ca and Ba (Megalovasilis, 2020). A fluid flux of 11,300 ± 1100 m3/day was calculated from porewater temperature profiles at 54 locations in the hydrothermal areas in Paleochori Bay, adding up to an annual arsenic input of approximately 1.5 × 104 kg into the Mediterranean Sea (Khimasia et al., 2021).
The fluids are acidic (pH∼5), hot (40–116°C), highly sulfidic (up to 3 mM H2S) and extremely enriched in arsenic (up to 78 μM, Price et al., 2013b). Arsenic is also present in the nanoparticulate phase of the hydrothermal fluids, summing up for up to 38% of the soluble arsenic (Durán-Toro et al., 2019) and the synthesis of these particles under simulated environmental hydrothermal conditions in the laboratory, elucidating the drivers of precipitation as a natural phenomenon occurring in marine environments at low pH (Durán-Toro et al., 2022). A number of bacteria and archaea were isolated from the Milos hydrothermal system, including the hyperthermophilic archaeon (Jochimsen et al., 1997; Dando et al., 1998; Arab et al., 2000), the thermophilic sulfate reducer Desulfacinum hydrothermale (Sievert and Kuever, 2000), the iron-reducer Deferrisoma palaeochoriense (Pérez-Rodríguez et al., 2016), and various chemolithoautotrophic sulfur- and iron-oxidizer (Brinkhoff et al., 1999; Sievert and Kuever, 2000; Giovannelli et al., 2012; Norris et al., 2020). Early studies on the bacterial diversity and community composition along the physicochemical gradients were conducted using Denaturing Gradient Gel Electrophoresis (DGGE) as a fingerprinting technique (Sievert et al., 1999, 2000a). These studies revealed a trend of decreased diversity with increasing temperature and also documented the effects of physical disturbances, such as sediment resuspension due to storm activity, on the bacterial community composition. Furthermore, it was shown that while Bacteria dominated the hydrothermal sediments, Archaea became more abundant with increasing temperature (Sievert et al., 2000b). By directly sequencing the 16S rRNA, Price et al. (2013b) investigated the microbial diversity at two sites with differing hydrothermal fluid composition caused by phase separation. A model that considers seawater mixing with hydrothermal fluids revealed that there is up to ∼50 times more energy available for microorganisms that can use S0 or H2S as electron donors and NO2– or O2 as electron acceptors compared to other reactions (Lu et al., 2020). Using sulfide and sulfate isotopic composition, Houghton et al. (2019) found sulfide oxidation predominating in hydrothermally affected areas, whereas active sulfate reduction was evident within the transition zone. The microbial communities of the surface sediments of the high salinity site were consequently dominated by sulfide-oxidizing Campylobacteria, whereas the low salinity site showed a predominance of Bacteroidetes (Flavobacteria) and Thermoplasmatales in the surface sediments, with a transition to Campylobacteria and Thermoproteales in the deeper layers. A study by Giovannelli et al. (2013), also revealed the overarching importance of Campylobacteria (60% of all sequences), in particular sequences related to the chemolithoautotrophic genus Sulfurovum. Gammaproteobacteria were found to increase in relative abundance with increasing distance from the vent center. A detailed study using next generation sequencing of 16S rRNA tags investigated changes in bacterial and archaeal diversity along a thermal gradient at a finer scale, identifying distinct zones corresponding to differences in community composition for both Bacteria and Archaea (Bühring and Sievert, 2017). Membrane lipid adaptations to the extreme conditions were assessed by Sollich et al. (2017). Based on intact polar lipids (IPL), archaea were found to dominate over bacteria in sediments with temperatures above 50°C, reflecting the low permeability of their ether-linked isoprenoidal lipids, which makes them more resistant to elevated temperatures.
Azores (Portugal)
The Azores Archipelago is composed of nine volcanic islands spread WNW-ESE along the 500 km outcrop of the Azores Plateau close to the triple junction of North America, Eurasian and African plates. The Mid-Atlantic Ridge divides the archipelago into three areas: Western Group, Central Group and Eastern Group. Geothermal and CO2-enriched waters and fumaroles are widespread on the islands both onshore and offshore (Cruz, 2003).
The dynamic of fluids is controlled by complex hydrogeological patterns with exchanges between a basal Na-Cl aquifer strongly influenced by seawater infiltration and perched aquifers characterized by Na-HCO3 and Na-HCO3-Cl waters, which can be divided in three groups as a function of temperature: cold (ambient temperature), thermal (up to 75°C) and boiling (up to 93°C). A further group of waters showing a Na-SO4 matrix with pH 2.02–2.27 are boiling pools from steam-heated perched aquifers. The water chemistry is also influenced by the weathering of the bedrock minerals and by entrainment of CO2 from the gas phase (Cruz and França, 2006). Proof that the geothermal aquifer is affected by seawater infiltration is the tidal influence on the CO2 concentration in the basal aquifer of Siete Ciddades volcano on S. Miguel Island. In this Na-Cl type aquifer, dissolved CO2 values range from 351 to 86 mg l–1 depending on the tidal regime; moreover, goethite and ferrihydrite with adsorbed As are precipitated from deep fluids enriched in Al, Fe and As when these are mixed with seawater (Carvalho et al., 2011). High values of dissolved CO2 (up to 365 mg l–1) and hydrothermal seepage are also observed in some of the 90 lakes that occupy volcanic craters, calderas and other tectonic and topographic depressions on the Azores (Cruz et al., 2006).
Within the Azores Archipelago, the D. Joao de Castro Seamount is an active underwater volcano; its first recorded eruption was in December 1720 and the most recent was in June-July 1997 at about 1,000 m of water depth. The top of the seamount is at around 13 m of depth and is occupied by a caldera with maximum depth of 40 m. Several active hydrothermal vents have been sampled in the shallow water. The vents are divided into two groups – “Yellow vents” and “White vents” – both types are aligned along fractures on the seafloor.
Nine other shallow-water hydrothermal sites have been identified (Cardigos et al., 2005) along the shores of Flores, Faial, Graciosa and S. Miguel islands (Aguiar and Costa, 2010). The emitted plumes influence the surrounding environment, originating up to 3°C higher temperatures and pH variations with a decrease of up to a few units within the water column mostly during low tide (Couto et al., 2010).
Up to this point, few studies, using both cultivation-based and cultivation-independent approaches, have been conducted to study the microbiology of the shallow-water vents around the Azores (Raghukumar et al., 2008; Mohandass et al., 2012; Rajasabapathy et al., 2014, 2018). Rajasabapathy et al. (2014, 2018) found that, in contrast to most other hydrothermal systems, which are dominated by ε-proteobacteria, the vents off Faial island were dominated by γ-proteobacteria. Ramasamy et al. (2020) was able to isolate an extremely copper tolerant Alcanivorax species (up to 600 μg mL–1).
Reykjanes Peninsula and Kolbeinsey (Iceland)
Iceland is a volcanic island in the Northern Atlantic; it is originated by the emersion of the Mid-Atlantic Ridge. Its origin is likely linked to a hotspot of upwelling from a relatively narrow mantle plume that is migrating eastwards (Shen et al., 1998). Other alternative origins need to be considered also due to the lack of definitive evidence on the presence of seismic anomalies in the lower mantle, which should be present in case of a plume (Foulger and Anderson, 2005). Widespread flood basalts are emitted by the intense volcanic activity along the rift zone with measured geothermal gradients of up to 150°C km–1 and with magma present between 10 to 30 km depth (Flóvenz and Saemundsson, 1993). These magmatic chambers are the heat source of a strong high-temperature hydrothermal activity along the rift where high-permeability areas (up to 150 millidarcies) are associated with sub-vertical faults and fractures. These faults and fractures allow for the formation of convective cells in the groundwater reservoirs fed mostly by meteoric water with local intrusion of seawater along some coastal areas such as in the Reykjanes Peninsula. The maximum temperature measured was 380°C down-hole a well at a depth of 2,200 m. The discharged water has Si, Na, Cl, SO4 as main ions and CO2, H2S and H2 as dissolved gases. The pH ranges from around 6 to 7 but, where hydrogen sulfide is oxidized by the contact with the atmospheric oxygen, sulfuric acid forms and drops the pH to 1 (Arnórsson, 1995). The large hydrothermal system associated with the volcanic rift shows 26 areas with high-temperature steam and 250 areas of lower temperatures with more than 600 hot springs along the flanks of the rift. The water temperature ranges between 62°C and 180°C and is largely used for heating purposes (Ragnarsson, 2000). Strong natural emissions of CO2 are detected in the geothermal areas with soil fluxes up to about 4∗103 t CO2 km–2 per year (Dereinda, 2008). The origin of CO2 is from mantle-derived degassing magma that is intruded below the rift at a rate of about 600 Mt a–1; the magma is able to generate a maximum CO2 emission of 1.3 Mt a–1 (Ármannsson et al., 2005). With this large onshore emission of fluids, it is not surprising that hydrothermal vents have also been identified offshore (Figure 1). The Reykjanes peninsula on the northwestern coast of Iceland harbors a series of freshwater, slightly alkaline, hot springs located on the seashore, from the tidal zone to about 100 m off the coast, with several springs emerging at low tide. The tides are as high as 4 m in this area, and therefore the organisms living in those hot springs can be subject to almost 100°C fluctuations twice per day (Hobel et al., 2005). A number of thermophilic, aerobic, heterotrophic bacteria of the genus Thermus were isolated (Kristjansson et al., 1986; Alfredsson et al., 1988). In 2005, the microbial ecology was studied by using diversity analysis of 16S rRNA, revealing the presence of clones related to both marine and terrestrial, thermophilic, mesophilic, and psychrophilic microorganisms scattered among 11 bacterial divisions, but no archaea (Hobel et al., 2005).
In the Eyjafjordur region offshore the northern coast of the island, submarine hydrothermal activity is present along NNE and NW trending faults on the basaltic seafloor. The NNE lineament follows the Kolbeinsey Ridge and a group of hydrothermal vents is found just south of Kolbeinsey Island at about 100 m of water depth. Fluids enriched in Ca, Cu and Mn are emitted from fractures and crater-like structures at temperature around 130°C thus allowing for phase separation due to boiling. In the gas phase, CO2 of magmatic and biogenic origin contributes are detected. The isotopic 3He/4He ratio is consistent with mantle plume origin of the gas. The vents are covered by highly altered basalts with formation of saponite, chlorite and chlorite-smectite clays and precipitation of calcite and Fe-hydroxides (Lackschewitz et al., 2006).
From hot marine sediments at 106 m depths at the Kolbeinsey Ridge, the hyperthermophilic hydrogen-oxidizing bacteria Aquifex pyrophilus has been isolated (Huber et al., 1992). Phylogenetic analyses show the Aq. pyrophilus lineage to be probably the deepest (earliest) in the bacterial tree, supporting the thermophilic ancestry of bacteria (Burggraf et al., 1992). Nanoarchaeum equitans, a hyperthermophilic virus-shaped Archaea, which represents a novel kingdom of Archaea, was isolated from Kolbeinsey Ridge as well (Stetter, 2006). With a cell diameter of only 400 nm, it is the smallest living organism known, which lives attached to the surface of a crenarchaeal host. Ignicoccus islandicus and I. pacificus are two more novel hyperthermophilic species of chemolithoautotrophic, sulfidogenic life-style that have been isolated from Kolbeinsey (Huber et al., 2000). Investigations by Fricke et al. (1989) revealed no vent-specific fauna at this hydrothermal site of medium depth, with recruitment apparently occurring from the surrounding non-vent environment.
Other hydrothermal vents are located inside the Grimsey Graben aligned along a NW trending fault system. It is an area of active submarine volcanism with the most recent eruption in 1867-1868; hyaloclastite deposits and glacial sediments are accumulated within the graben. More than 20 vents are distributed over a surface of 1 km2 on a structural high on the eastern side of the graben at about 400 m of water depth. The central part is the more active one, hosting 13 vents from anhydrite-talc enriched deposit mounds of about 10 m in diameter and rising 3 to 5 meters from the surrounding seafloor; 1 to 3 meter-tall anhydrite and talc chimneys are also emitting fluids. The sediments surrounding the vents are enriched in iron and sulfur. The emitted fluids have temperatures around 250°C which is above the boiling temperature for the depth, therefore inducing phase separation; the liquid phase has low salinity and is enriched in Mg and SO4 with pH from 5.9 to 6.8. A strong circulation of heated fluids is present in the sediments with temperatures of 50 to 80°C at the surface and up to 150°C 10 cm below. The gas phase is composed of up to 41% of CO2 of magmatic origin and up to 24% of CH4 derived from the thermal decomposition of the organic matter accumulated within the graben. Bubbles have been detected by echosounder almost up to the ocean surface (Hannington et al., 2001). Within the Eyjafjordur fjord, three conical chimneys of 25, 33, and 45 m of length have been discovered rising from the 100 m deep seafloor; their composition is mainly of amorphous silica with Mg-enriched clay minerals and minor carbonates. The 45 m chimney emits the majority of the fluids at temperatures ranging from 60 to 72°C and with fluxes around 50 liters per second. The fluids have low salinity with TDS 291 mg l–1 and pH 10. The main ion concentrations in the fluids are indicated in Table 1a. The source of the fluids is likely an onshore freshwater reservoir circulating within the heated bedrock. This hypothesis is supported by the fact that all 12 aerobic strains isolated from concentrated fluid grew at pH 10.0, but only one grew above 2.0% NaCl (Marteinsson et al., 2001).
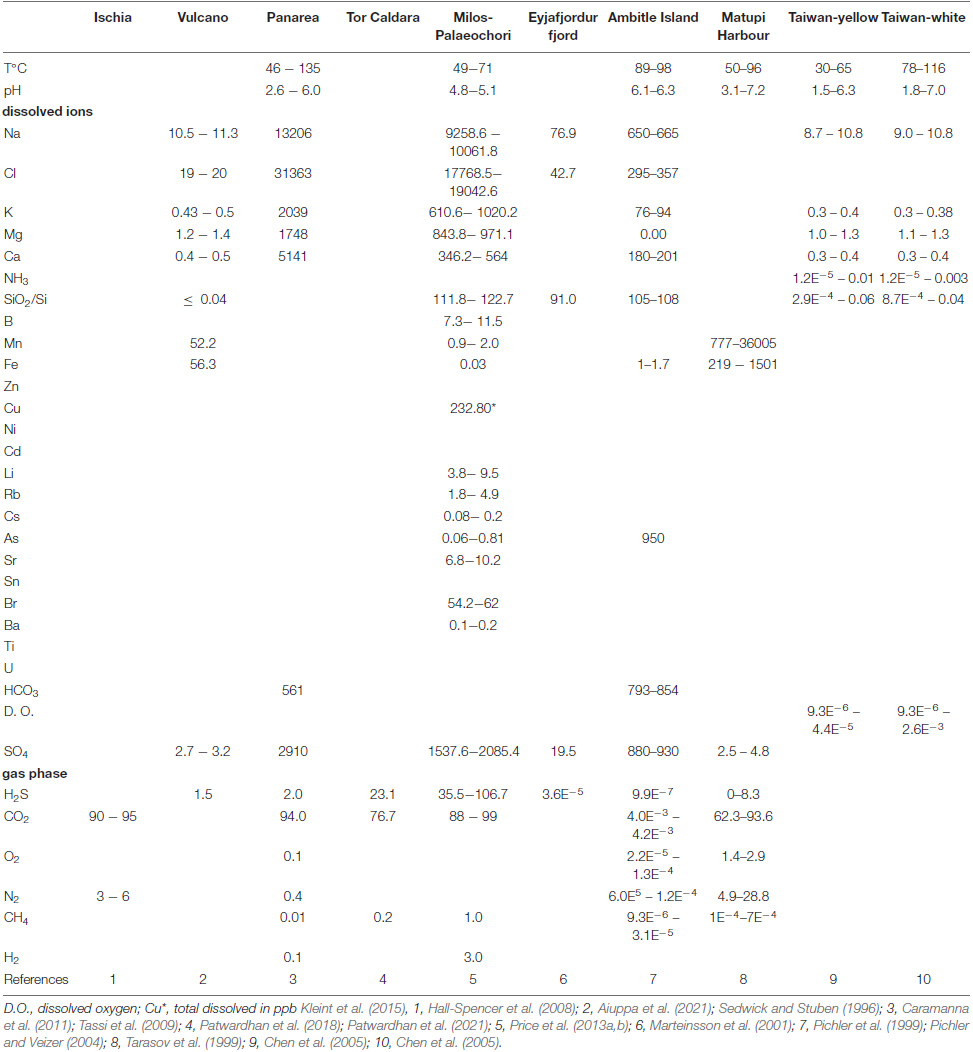
Table 1a. Main physical parameters and chemical composition of the emitted fluids (in ppm, Ba in ppb) and gases (in%) from Ischia, Volcano, Panarea, Tor Caldara, Milos, Eyjafjordur, Ambitle. Matupi, and Taiwan.
Ambitle Island and Matupi Harbour (Papua New Guinea)
Ambitle Island is part of Tabar-Feni Islands chain along the western shore of Papua New Guinea. These islands are the outcrop of a series of volcanoes that have been active since the Pliocene, emitting high k-alkaline lavas undersaturated in silica and originated from the melting of the subducing Pacific plate below the North Bismarck plate, due to adiabatic decompression following the current uplifting of the New Ireland forearc basin (McInnes and Cameron, 1994). Ambitle Island itself is a stratovolcano overlying marine limestone deposits of Oligocene age (Wallace et al., 1983). Geothermal activity is present in the western sector of the island originating hot mud pools, acid sulfate-chloride waters and low-T fumaroles (67 < T < 100°C). Along the western shore in Tutum Bay, a series of shallow-water (5–10 m) submarine vents are aligned NW-SE following fault lineaments (Figure 1). The seafloor is covered by medium-coarse sand and gravel composed of carbonates and volcanoclastic deposits; patches of corals and algal reefs characterize the area. Closer to the vents, the mineralogical composition of the sediments is mostly feldspar, hornblende, pyroxene and magnetite originated from the weathering of the andesite-dacite basalt of the bedrock by the acidic fluids; carbonate deposits increase only far (> 260 m) from the emissions, where the impact of the acidic fluids is reduced (Pichler and Veizer, 1999; Price and Pichler, 2005). The submarine emissions are divided into two different types: punctual vents emitting hot (89–98°C) clear mineralized, acidic (pH 6.1–6.3) and reducing (Redox −0.17 mV) fluids with a flow rate of 300–600 l min–1 from orifices of 10–15 cm in diameter and dispersed gas bubbling through the loose sedimentary cover (Table 1a). Shifting in position of the vents was observed and it is likely due to a channeling effect within the sediments (Pichler and Veizer, 1999, 2004). The fluids are enriched with CO2 and are close to the boiling point when emitted from the seafloor; a drop in temperature and the mixing with seawater causes a release of CO2, which triggers carbonate precipitation mostly in the form of aragonite crusts over boulders, pebbles and dead corals, filling part of the pore-space within the sedimentary cover (Pichler and Veizer, 2004). Fe-oxyhydroxides are also emitted from the vents in the form of amorphous colloidal fine grains precipitating as thin coating layers above the seafloor and as more massive deposits in the proximity of the vents. The estimated rate of precipitation is up to 1 cm a–1. Below the Fe-oxyhydroxides deposits, sulfide minerals are precipitated at depth inside the sedimentary cover and the fractured bedrock, following the mixing of the geothermal fluids with seawater (Pichler and Veizer, 1999). 87Sr/86Sr isotopic fraction gives a mixing fraction of seawater around 11% and calculated temperature of equilibrium for isotopic carbon is 78°C, close to the measured values (89–98°C) (Pichler and Veizer, 2004).
Arsenic is an important component of the emitted fluids with fluxes estimated up to 1,500 g day–1 for a 5,000 m2 area (Pichler et al., 1999). The observed arsenite (AsIII) concentration in the fluids is up to 950 μg l–1 compared with the 2.4 μg l–1 for seawater background (Price and Pichler, 2005; Akerman et al., 2011). AsIII is a mobile form of As and is very toxic for eukaryotes; concentrations of 3 μg l–1 are damaging for higher marine life forms and above 100 μg l–1 are harmful for humans (Price and Pichler, 2005). Despite the high values measured in Tutum Bay, AsIII is largely precipitated and trapped within the Fe-oxyhydroxides deposits with concentration up to 33,200 mg l–1 (Price and Pichler, 2005) and therefore the ecological effects are negligible (Pichler et al., 1999). Part of the AsIII is also conveyed toward the top layers of the water column by the buoyancy of the emitted fluids and there it is oxidized to the less reactive AsV (Price and Pichler, 2005). Bio-accumulation of As is recorded in organisms in Tutum Bay such as the soft coral Clavularia sp., calcareous algae Halimeda sp. and the tunicate Polycarpa sp. with measured concentration 2 to 20 times higher than the background values (Price et al., 2016). The source of AsIII is likely dissolution by the acidic fluids of arsenopyrite minerals within the bedrock (Pichler et al., 1999). The unique location at Tutum Bay makes it furthermore an ideal target for investigating the effect of ocean acidification on coral reefs (Pichler et al., 2019). The origin of the emissions is a mix between fluids from a deep geothermal reservoir with temperature of 257–291°C as inferred from the CH4/CO2 geothermometer leading to a depth of separation of about 1,000 m, forming CO2-rich water with low pH, which migrates toward the shallow-water table fed from the meteoric water. The resulting flow moves laterally, under gravitational control, where it mixes with marginal upwelling from the deeper reservoir producing diluted chloride water at 165°C and further mixing with shallow groundwater and seawater during the last phase of the ascent (Figure 4). It was estimated that about 10% of the deep fluids reach the surface (Pichler et al., 1999). Due to the high concentrations of arsenic, the vents off Ambitle Island have been used to investigate its effects on microbial diversity and to identify microorganisms able to cope with or even utilize arsenic (Pichler et al., 2006; Akerman et al., 2011; Meyer-Dombard et al., 2011, 2013).
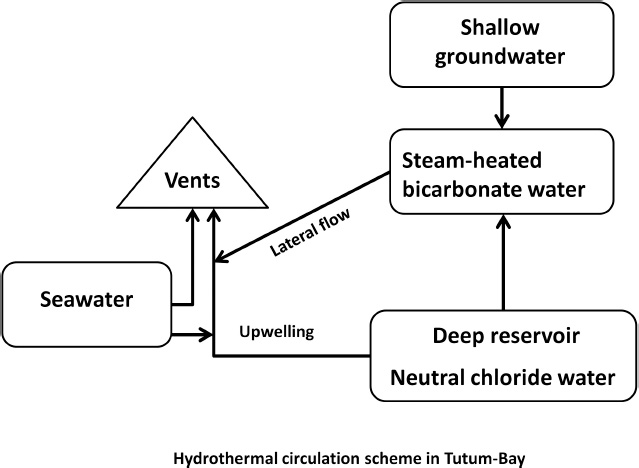
Figure 4. Simplified flow of the fluids in the vents in Tutum Bay (data from Pichler et al., 1999).
Another area where shallow-water geothermal fluids are emitted from the seafloor is Matupi Harbour on the NE of Rabaul caldera in the northern part of New Britain Island to the east of Papua New Guinea. The caldera was formed 1,400 year ago following a large explosion that displaced around 11 km3 of rock; several extinct and active volcanoes are aligned along the rim of the caldera. Matupi Harbour is a bay with maximum depth of 65 m with hot volcanic fluids seeping from the seafloor at 3 to 10 m of depth, forming small tubes of Fe-oxyhydroxide cement. Other emissions, both gas and liquid, are located along a steep slope (40–45° dip) from 1 to 10 m of depth creating crater-like structures, surrounded by bacterial mats. Table 1a shows the main chemical and physical parameters (Tarasov et al., 1999).
Kueishantao (Taiwan)
The island of Taiwan is close to the southern end of the Okinawa Trough, which is the back-arc basin of the Ryukyu subduction zone and part of the larger subduction of the Philippine Sea plate below the Eurasian plate, extending from southern Japan to Taiwan along about 1,000 km of a seismically and volcanically active area (Chen et al., 2005). The Tatun volcanic area is on the northern corner of Taiwan and was active until 200,000 years ago; nowadays intense geothermal activity is present with more than 200 springs emitting hot (120–293°C) acidic, sulfate-chloride water. About 1 km offshore this area, the islet of Kueishantao is surrounded by a submarine geothermal field with more than 30 vents emitting fluids from a rocky andesitic seafloor in shallow (10 to 30 m) water (Figure 1). The emissions are in both the gas and liquid phase and are divided into “yellow” and “white” (Table 1a). The yellow vents emit hotter fluids from chimneys and mounds of sulfur, in respect to the colder white vents, with an estimated annual total flux of 430-2600 kg Fe, 24-145 kg Mn, 5-32 kg Ba, 10-60 kg As, 03-1.9 kg Cd, and 2-10 kg Pb (Chen et al., 2018). The gas is CO2-dominant (up to 92%) with up to 8% of H2S (Chen et al., 2005; Yang et al., 2012). Sulfur and He isotopic composition is consistent with mantle origin of the deep end-member mixing with heated seawater and possible steam formation in the deepest plumbing (Chen et al., 2005; Yang et al., 2012).
Native sulfur deposits are found in the form of chimneys, sand and some nodules called “sulfur balls.” These balls are found nearby the vents, and samples up to 300 g in weight have been retrieved, showing growing rings consistent with the hypothesis that the nodules are formed from sulfur deposits by the rolling action of waves and currents. S is the main component, but other trace elements are: Li, Cr, Co, Ni, Rb, Sr, Cs, Fe, Zn, Cd, Pb, Mg, K, and Ca. Enrichment in Fe, Zn, Cd and Pb and depletion in Mg, K and Ca are observed in respect to the geothermal fluids composition. The source of these elements is deep magma with contribution from the weathering of andesite; seawater is providing Mn, Mg and K (Zeng et al., 2011). An increased concentration in boron is observed in the fluids (4.10–4.64 mg l–1) in respect to the seawater baseline (3.81 mg l–1). Isotopic measurements of δ11B in the emitted fluids indicated a relatively short-timed interaction between fluids and bedrock and the seawater as the main source for boron (Zeng et al., 2013). DOC values in the fluids are within the range of seawater suggesting that these vents are neither a source nor a sink for organic carbon. The fate of the vented CO2 was followed by Lin et al. (2019), generating a bubble-plume model, which provided tens of minutes as a conservative flushing time estimate. The depletion of total suspended matter, lower C/N ratios, and higher carbon isotopic values of particulate organic carbon (δ13CPOC) in the vertical plumes relative to values derived from Si-based models were attributed to the hydraulic sorting of the vented particles (Lin et al., 2020).
A study by Zhang et al. (2012) using ribosomal tag pyrosequencing showed that sulfur metabolism fuels microbial energy flow and element cycling in the water column over yellow and white vents off Kueishantao. The bacterial and archaeal communities from the white hydrothermal plume were dominated by the sulfur-reducing campylobacterium Nautilia and the archaeon Thermococcus, whereas the yellow plume was dominated by the sulfide-oxidizing gammaproteobacteria Thiomicrospira and by Marine Group II. Euryarchaeota. Functional metagenomic investigations by Tang et al. (2013) also revealed the dominance of Nauliliales-like Campylobacteria and Thiomicrospira-like Gammaproteobacteria in the vent and surface waters. The chemoautotrophic microorganisms in the vent and the surface water possess the reverse tricarboxylic acids cycle and the Calvin-Bassham-Benson cycle for carbon fixation (Li et al., 2018). The hydrothermal sediments around the vents revealed a dominance of Campylobacteria with decreasing abundance from the vent area (Wang et al., 2015, 2017), but in contrast to the fluids Sulfurovum and Sulfurimonas were the most dominant groups, using the reductive tricarboxylic acid (rTCA) cycle for chemoautrophic carbon fixation (Wang et al., 2017). A recent study using a combination of metagenomic and cultivation-based approached also found evidence for abundant heterotrophs, indicated by the presence of diverse genes that encode transporters, glycoside hydrolases, and peptidase involved in the degradation of organic matter and the isolation of a total of 408 heterotrophic strains (Tang et al., 2018).
The trophic structure within the vent system was investigated by using stable isotope analyses (Chang et al., 2018), which revealed a hydrothermal origin of the particulate organic matter (POM), which comprises up to 53% of the diet from zooplankton and epibenthic crustaceans.
Areas characterized by peculiar environmental conditions, such as those originated by the presence of mineralized acidic hydrothermal emissions, can develop endemic life forms. The crab Xenograpsus testudinatus has been found living nearby the vents and feeding on “oceanic snow” composed of zooplankton organisms killed by the toxic fluids that fall through the water column during slack water. Following its feeding habits, the crab is able to store a large accumulation of lipids (50–60% of dry mass) as survival strategy for low-food availability periods. Enzymes identified in the crab are stable at high temperature, variable pH and presence of elements known as inorganic inhibitors such as Cu2+, Fe2+, and Co2+ (Hu et al., 2012). A study by Zeng et al. (2018) showed the accumulation of Mn, Hg, and K within the shells of this crab. Bacteria residing in X. testudinatus were investigated using 16S ribosomal RNA gene amplicon pyrosequencing and revealed a dominance of γ- and ε-Proteobacteria (Yang et al., 2016). The low pH and high temperatures in the overlying waters may furthermore influence the zooplankton, by weakening the exoskeleton of copepods, which were found to harbor numerous abnormal protrusions (Mantha et al., 2013). Macrobiota species richness increased with horizontal distance from the vent, exerting a negative impact over several kilometers (Chan et al., 2016). The deeper vents off Kueishantao (200-300 m water depth) harbor Bathymodiolus taiwanensis (Wang et al., 2014), the smallest and shallowest-dwelling Bathymodiolinae (Cosel, 2008).
Kagoshima Bay (Japan)
Kagoshima Bay in southern Japan is a graben structure and hosts submarine hydrothermal vents emitting hot water and gas from the seafloor at about 200 m of water depth. The main feature of Kagoshima Bay is the active andesitic volcano Sakurajima that originated about 13,000 years ago and has been emitting clouds of ashes since 1955. During its eruption in 1914, lava flows created a bridge between the volcano and the eastern mainland and caused the deposition of a layer of pumice within the sedimentary cover of the seafloor. Sakurajima divides the bay in a northern and southern section separated by a shallow (30 m) and relatively narrow (3 km) channel between the west flank of the volcano and the western mainland. In the northern area in the Aira caldera (25 km in diameter), the sub-caldera Wakamiko hosts the main vents (Figure 1). This area is characterized by a flat muddy seafloor with depth around 200 m, which is mostly composed of silty clay and silty sand, unconsolidated sediments of approximately 80 m thickness. Low-gravity anomalies indicate that the bottom is filled with low-density sediments, which are likely pyroclastic deposits (Oki and Hayasaka, 1978; Yokoyama and Ohkawa, 1986; Ishibashi et al., 2008).
Hydrothermal fluids are emitted through the sedimentary cover generating small streams of bubbles. In 2007, during a survey by an autonomous underwater vehicle (AUV), a chimney rising about 3 m from the seafloor, composed of mineral deposits of native sulfur and sulfides, was discovered. The fluids are emitted from small orifices that are 15 cm in diameter; filamentous bacteria live around the emissions (Nakatani et al., 2008).
The gas-phase of the fluids is composed mostly of CO2 with elevated 3He/4He ratio indicating magmatic origin; Table 1b resumes the main physical and chemical parameters of the emissions. The measured temperature of the hydrothermal fluid is 215°C which is slightly above the 211°C boiling temperature of the water for that depth, thus some phase-separation with steam generation is also possible. The Na/Cl ratio is consistent with a component of the liquid originated from heated seawater, and the δD-δ18O isotopic values indicate also meteoric and magmatic water contributes (Ishibashi et al., 2008).
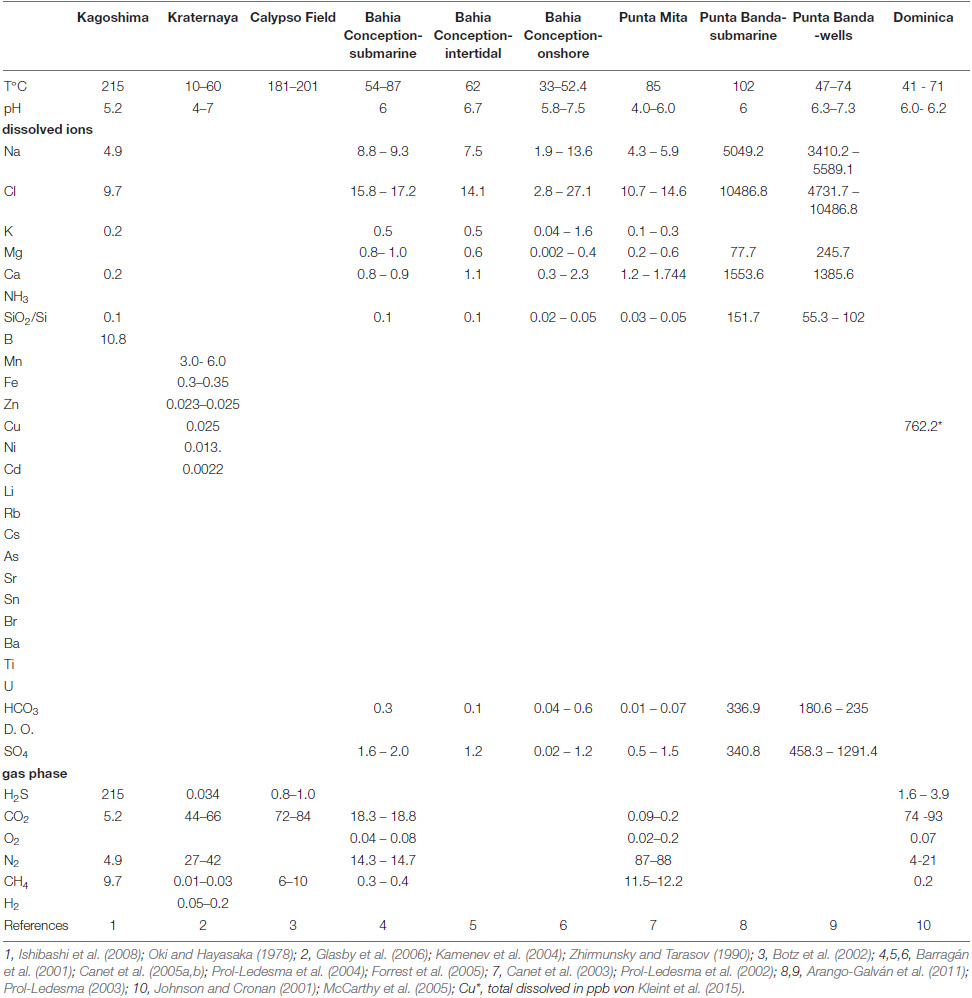
Table 1b. Main physical parameters and chemical composition of the emitted fluids (in ppm, Ba in ppb) and gases (in%) from Kagoshima, Kraternaya, Calypso, Bahia Conception, Punta Mita and Punta Banda, as well as Dominica.
Fluids collected within the pore-space of the sediments nearby the vents show the presence of hydrocarbons enriched in aromatic compounds of pyrolytic origin with different maturation stages from kerogen. The origin of this petroleum is supposed to be linked to the interaction of the hot geothermal fluids (around 300°C) with thick accumulation of organic matter on the seafloor of the caldera; the presence of high concentration of B, which is supposed to be mobilized from organic-rich sediments at T > 100°C, also supports this hypothesis (Yamanaka et al., 1999; Ishibashi et al., 2008). A similar thermogenic origin is proposed for the emitted methane considering the CH4/3He ratio (Craig and Horibe, 1994).
Deposits of Kuroko-type sulfides and sulfates are present in the sediments; they originate in mineral precipitation once the hydrothermal fluids mix with the surrounding seawater. The presence of barite, stibnite, realgar and pyrite with up to 2% of As and the absence of Fe-oxyhydroxides suggests a reducing environment within the sediments. The association of petroleum and Kuroko-type deposits is observed in other areas of Japan such as in the Green Tuff region (Yamanaka et al., 1999; Ishibashi et al., 2008). High levels of Hg in the sediments close to the vents have been measured with concentrations up to 257 mg kg–1; more than 80% is present as HgS indicating a reaction between Hg and hydrogen sulfide under anoxic conditions within the sediments. High organic mercury concentration up to 0.9 mg kg–1 suggests that organic-Hg can be formed from submarine fumaroles when the total Hg availability is high. An ecological implication is that in the long-term, a progressive accumulation within the sediments of Kagoshima Bay may cause toxicity effects on the biota (Sakamoto et al., 1995). The impact of the volcanic vents on the marine environment of the bay is also augmented by the fact that the bottom layers of the water column, mostly in summer when the thermal stratification is more evident, are almost stagnant due to the presence of the shallow and narrow channel between Sakurajima and the mainland; this reduces the circulation between the water inside the caldera and the open sea. As a consequence, the bottom layers are hypoxic (dissolved oxygen below 0.5 ppm) and acidic (pH 6.5) due to the presence of low-pH emissions; this affects the development of calcareous life forms such as some species of foraminifera, which are almost absent from the sediments close to the vents (Oki and Hayasaka, 1978; Yamanaka et al., 1999). The primary chemical form of arsenic in the deep water layer is As(III) and during summer time, due to biological activity, the As(V) in the surface waters gets reduced to As(III) as well (Tomiyasu et al., 2021).
Early studies of the microbial communities by Hoaki et al. (1995) using a most-probable-number approach found a strong dominance of hyperthermophilic sulfur-dependent heterotrophs, followed by sulfur-dependent autotrophs. The heterotrophs most likely require the abundantly present amino acids for their growth. The endosymbiontic bacteria in the vestimentiferan tubeworm Lamellibrachia satsuma was characterized as belonging to the Deltaproteobacteria (Yamamoto et al., 2002). Tanaka et al. (2006) isolated a strictly aerobic and thermophilic bacterium (Thermaerobacter litoralis) from a coastal hydrothermal field on the Satsuma Peninsula, Kagoshima Prefecture. Efforts by Takeuchi et al. (2014) led to the isolation of Methyloceanibacter caenitepidi, a facultatively methylotrophic bacterium and Methylocaldum marinum, a thermotolerant methane-oxidizing bacterium, respectively. Nakagawa et al. (2004) isolated Hydrogenivirga caldilitoris, which is extremely thermophilic and which can oxidize hydrogen and sulfur. From the phylum Chloroflexi, the ferric iron- and nitrate-reducing bacterium Ardenticatena maritima has been isolated by Kawaichi et al. (2013a).
The bacterial and archaeal diversity was determined within the iron-rich coastal hydrothermal field of Yamagana beach (Kawaichi et al., 2013b). The results of clone analyses based on 16S rRNA genes revealed chemolithoautotrophic thermophiles, primarily within the bacterial order Aquificales and chemoheterotrophic thermophiles from the order Thermales and the family Desulfococcaceae. A microbial community analyses by Nishiyama et al. (2013) using clone libraries revealed Proteobacteria, Bacteroidetes, and Firmicutes, sharing only 92% sequence similarity with their closest sequences in public databases, suggesting a unique and novel bacterial community. The archaeal library contained only three OTUs, most of which were affiliated with Thaumarchaeota.
Kurile Islands (Japan)
The Kurile archipelago is composed of a series of volcanic islands stretching on a 600 km long arc in the Western Pacific from Hokkaido Island to the south to the Kamchatka peninsula to the north. Nineteen of these islands host 44 volcanoes and hydrothermal fields; moreover, about 100 submarine volcanoes and 5 submerged calderas are present along the arc. The origin of such intense seismic and volcanic activity is linked to the subduction of the Pacific Plate and the Philippine Plate below the Okhotsk Plate and the Eurasian Plate, respectively. The islands are distributed in an arc-trench system along two parallel arcs; the frontal arc hosts 55% of the onshore volcanoes while the submarine volcanoes are mostly in the rear arc. A tectonic system of faults and fractures controls part of the volcanism with emission of andesitic lava in the Quaternary-Neogene. In the central part of the Kurile Islands arc, the islands of Riponkicha and Yankicha, are the outcrops of the Ushishir Volcano; the last eruption took place in 1884 (Glasby et al., 2006).
Kraternaya Bay on Yankicha Island is the flooded crater of the Ushishir Volcano formed in the Late Pliocene–Holocene, forming a relatively shallow caldera similar in its shape to Kagoshima Bay in Japan. It is characterized by a narrow and shallow inlet (0.2–0.4 m at low tide), two small islets that divide the bay in a western deeper part (63 m depth), and a shallower eastern section with maximum depth of 49 m (Figure 1). Steep slopes (40 to 60° dip) mark the rim of the bay with only the slope opposite to the inlet being more gradual with only 20 to 30° of incline. The bottom in the deepest parts is covered by clay silt; silty sand is present below 30 m, and boulders and gravel cover the slopes. The thickness of the sedimentary cover is 80–120 m (Kamenev et al., 2004; Glasby et al., 2006).
Submarine geothermal vents, from intertidal to 30 m of depth, are aligned along fractures in the bedrock creating a ring that follows the perimeter of the bay (Tarasov and Zhirmunskaya, 1989; Zhirmunsky and Tarasov, 1990). An estimated 22,000 m3 day–1 of geothermal water flows in the bay from the submarine vents and from an onshore boiling pond (Kamenev et al., 2004; Glasby et al., 2006). This input of water also affects the bay due to its limited circulation and exchange with the open ocean creating a stratification of the water column with surface temperatures inside the bay of 9 to 12°C compared with the 2.5 to 3.5°C of the ocean outside (Zhirmunsky and Tarasov, 1990). The emitted acidic hot water (10 to 60°C) is Cl-Na-Ca type enriched in sulfur compounds and heavy metals. The gas-phase is CO2 dominated with N2 as the other main component (Table 1b).
Accumulation of Zn was observed in the soft tissues of the bivalve mollusks Macoma calcarea and Macoma golikovi which inhabit all the zones of underwater volcanic activity, with settlements of lesser density within vents containing hydrogen sulfide (Kamenev et al., 2004). Mats of bacteria including sulfur-oxidizing bacteria and thermophilic archaea develop around the vents below elemental sulfur deposits leading to high rates of CO2 fixation and primary production (Zhirmunsky and Tarasov, 1990). Sediments on the seafloor are covered by Fe-P crusts with up to 5% P. In the sediments, Fe is the main element with concentration up to 11%. Fe and P originate from the hydrothermal fluids. The presence of the hydrothermal vents and the associated chemical environment allowed for the development of new species of macrofauna including holothurians, bivalves and polychetes. The first 10 to 13 m of the water column is affected by high abundances of the ciliate Mesodinium rubrum, which generates large “red tides” within the bay (Tarasov and Zhirmunskaya, 1989; Kamenev et al., 2004; Glasby et al., 2006). The physiological state of microbial communities within the hydrothermal sediments of the Bay were investigated using lipid biomarkers. The ratio of trans/cis in the ω7 isomers of monoenoic fatty acids significantly exceeded 0.1, which is indicative of physiological stress in bacteria (Kharlamenko et al., 2009). A study by Nikiforov (2001) on the allozyme variability of different polymorphic loci in the sea urchin Strongylocentrotus droebachiensis from hydrothermally influenced Kraternaya Bay and an unaffected site, revealed statistically significant differences in the genotypic allele frequencies and in level of heterozygosity. They argue for a selective significance of this allozyme polymorphism under environmental stress conditions. Miroshnichenko et al. (1989) isolated Thermococcus stetteri from the vents of Kraternaya. Th. stetteri is an extremely thermophilic marine sulfur-metabolizing archaeon, which grows at temperatures up to 98°C. Submarine hydrothermal deposits are also present off the west shore of the island of Iturup close to Hokkaido. The deposits are in the form of Fe-Mn nodules and crusts and Mn-oxyhydroxides cement on the seafloor at about 40 m depth (Glasby et al., 2006).
White Island (New Zealand)
The Taupo Volcanic Zone (TVZ) is a very active ryolitic-dacitic back-arc volcanic and geothermal area in New Zealand in the central part of the North Island. The TVZ is associated with the subduction of the Pacific Plate below the Australian Plate originating a graben structure 150 km long and 40 km wide along a NE-SW direction. High heat-flow (4,200 ± 500 MW), twenty geothermal sites and eight calderas, formed during the last 2 Ma, characterize the area with CO2 emissions from magmatic sources and metamorphic degassing aligned along local and regional faults (Pantin and Wright, 1994; Kerrick et al., 1995; Horwell et al., 2005; Werner and Cardellini, 2006). The sources of the heat-flow and of the deep-seated fluids rising in the geothermal areas are a shallow magmatic reservoir at about 500 m of depth and two other deeper chambers at 1–2 km and 2–7 km respectively (Peltier et al., 2009). Submarine vents have been identified in the Bay of Plenty mostly between the two volcanic islands of Mothuora Island (Whale Island) and White Island within the Whakatane Graben in water depth ranging from 30 to 350 m (Figure 1). The graben is filled with up to 2.5 km thick pyroclastic deposits originated by the eruption which formed a caldera, now largely submerged, about 65 Ka ago; volcanism was active up to 18 Ka ago. Bubbles can be seen at the sea surface; pockmarks, cones and mounds have been observed on the seafloor in a 144 km2 area. The cones and the mounds are composed mostly of crystalline anhydrite and bubbles are emitted from small orifices a few centimeters in diameter. Clay deposits surrounding the cones are enriched in As (530 ppm), Sb (150 ppm), Hg (90 ppm), Tl (500 ppm), U (138 ppm) and Mo (1000 ppm); amorphous material is also precipitated enriched up to 1% in Cu, Pb and Zn, several hundreds of ppm of Ag and about 10 ppm Au. The mineral assemblage is consistent with fluid temperatures of around 200°C at the moment of their emission from the seafloor. This value for the fluid’s temperature is also in good agreement with the proposed maximum temperature of 320–340°C of the deep aquifer composed of dilute Na-Cl solution fed by meteoric water with a contribution of seawater heated by the shallow magmatic body (Pantin and Wright, 1994; Seward and Kerrick, 1996; Hocking et al., 2010).
One of the most active submarine venting areas is the so-called “Calypso Field”, which was explored by a team from Cousteau’s research vessel Calypso in the 1980s. About twenty vents discharge fluids from the seafloor at a water depth between 170 and 200 m. The emissions are aligned NE-SW and clustered in two areas, 10 and 15 km south of White Island; each area covers 1–1.5 km2. The gas-phase is CO2 prevalent with CH4 and H2S; polynuclear aromatic hydrocarbons (PAH) of thermogenic origin are emitted from the interaction between the hot fluids and organic material sourced as terrestrial detritus, and near-coastal vegetation remains (Table 1b). δ13C isotopic composition of the CO2 is between −1.7 ‰ and −4.2 ‰ indicating mantel origin plus some contribution from magmatic sources and thermal dissociation of marine carbonates (Botz et al., 2002). Zitoun et al. (2020) suggested to be careful if using White Islands as model for ‘future ocean’ scenarios. The seawater carbonate chemistry gets altered in only small areas around the vents, showing furthermore substantial variability and Hg reaches toxic concentrations.
The vents show discontinuous activity, which is likely due to three causes: localized reduction of pressure within the geothermal reservoir, clogging of the smaller hydrothermal plumbing system by the deposition of minerals, and collapse of the larger conduits eroded by the aggressive rising fluids (Pantin and Wright, 1994). Two end-members for the emissions can be identified: a fast-rising magmatic component and a slower flux of hydrothermal origin including meteoric and seawater contributes in analogy with the fumaroles active onshore White Island (Giggenbach, 1987; Sarano et al., 1989). Close to the hot emissions, filamentous white bacteria have been observed (Sarano et al., 1989; Pantin and Wright, 1994; Hocking et al., 2010). So far, no designated geomicrobiological investigation of the submarine hydrothermal vents has been performed, only the hot vents on White island have been investigated (Donachie et al., 2002). A study by Kamenev et al. (1993) investigated the macro- and meiofauna and found the pogonophore Siboglinum around gas-hydrothermal vents at temperatures around 65°C. The white mats were described as containing thiobacilli and colorless filamentous sulfur oxidizing bacteria of the genera Thiodendron and Thiothrix (Sorokin, 1991). Close to White island, Beggiatoa mats have been described at moderate temperatures on sediment composed of coarse sand mixed with gravel (Sorokin, 1991).
Bahía Conception, Punta Mita, Punta Santa Barbara and Punta Banda (Mexico)
The Baja California Peninsula (BCP) extends NW-SE on the western side of the Gulf of California between Mexico and the Pacific Ocean. The tectonic setting of the area is controlled by NW-SE strike-slip faults linking the North American and the Pacific plates allowing for the formation of extensional rift systems and pull-apart basins (Barragán et al., 2001). Geothermal activity is diffuse along the BCP, originating springs and groundwater of high and low enthalpy allowing for 76% of the total geothermal electricity generated in Mexico to be produced here. The main reservoir is composed of heated meteoric water circulating through the regional faults, which act as preferential paths for the endogen fluids (Arango-Galván et al., 2011).
Bahia Conception is a NW-SE 40 km long bay within the Gulf of California along the eastern shore of BCP. At least five areas of interest for the development of geothermal power plants have been identified in the bay area highlighting the presence of strong hydrothermal circulation (Villanueva-Estrada et al., 2013). Cretaceous granites are covered with andesite lava flows and breccias of the tertiary Comondu’ Group produced by forearc magmatic activity during a pre-rift phase. Late Pliocene conglomerates and cherts of the Infierno Formation indicate that hydrothermal circulation was active in shallow-water carbonate mud. Early Pleistocene basaltic flows and pyroclastic deposits are the latest volcanic events in the area (Canet et al., 2005b).
The bay is bordered by direct faults originating a semi-graben structure developed during the late Miocene extension phase of the Gulf of California. The Conception Fault Zone caused an uplift of up to 600 m along the eastern border of the bay. El Requeson fault on the western border is the main tectonic feature acting as preferential path for the geothermal fluids emitted from intertidal and submarine vents (Barragán et al., 2001; Prol-Ledesma et al., 2004; Canet et al., 2005a, b).
Three main areas with submarine vents have been identified around Punta Santa Barbara, Punta Mita and Punta Banda (Figure 1). Along the El Requeson fault near Punta Santa Barbara, hydrothermal fluids are emitted along fractures on the seabed for over 750 m from the coastline in shallow-water up to 13 m of depth. The vents emit a mix of seawater and hydrothermal fluids of meteoric origin. The recorded temperature is 54°C at the seafloor and 87°C within the sediments around the vents. Na/Li, Na-K-Ca, and Si geothermometers indicate a reservoir temperature around 200°C. Amorphous opal-A is the main component of the mineral deposits originated from the fluids. Crystalline calcite with up to 50.8% in CaO is also present with Ba enrichment up to 921 ppm. Fe-oxyhydroxides with up to 5,112 ppm of As cover the volcanic boulders on the seafloor and are associated with orange-yellow biofilms. The main source of As is likely the andesite volcanic bedrock from where it is mobilized by the action of neutral Na-Cl hot water and it is then transported in reduced state AsIII. Once the hot fluids mix with the colder and oxygen enriched seawater, As is oxidized and adsorbed in the Fe-oxyhydroxides. Pyrite and micro-particles of cinnabar are also present. A 75 m long fossil reef of silica-carbonate stromatolites lies along the intertidal vents. The δ13C (+ 9.3‰) of carbonates is consistent with Ca precipitation caused by CO2 degassing. The intertidal fluids produce precipitates in the form of manganese oxides, opal, barite and calcite cements; biological activity is likely involved in the formation of MnO2 (Canet et al., 2005a, b; Villanueva-Estrada et al., 2013).
The emitted gas is dominated by N2 and CO2, with CH4 being also present. The temperature of the emitted fluids from the submarine vents ranges from between about 54 and 87°C showing a negative relation with the tidal cycle with lower T during high tide (Table 1b). δ13C (−34.3‰) of CH4 is consistent with thermogenic origin from algal kerogen. δ13C (−5.85‰) of CO2 indicates a mantle origin but the CO2/3He ratio (1.83 ∗ 108) is one order of magnitude lower than the usual values for mantle-derived gas; therefore, a contribute from the thermal decomposition of organic sediments is likely. Geothermometers indicate a temperature for the reservoir of 180–200°C (Prol-Ledesma et al., 2004; Forrest et al., 2005). The emissions originate from meteoric water infiltrating onshore within the deep sub-surface and heated by a granodiorite magmatic body. The fluids then circulate within the faults network interacting with the quaternary sediments before emerging from the seafloor and mixing with the surrounding seawater.
South of the Gulf of California, along the Pacific shore of Mexico, Punta Mita is another area of shallow-water geothermal activity linked to the Mexican Volcanic Belt in a regional extension area with high heat flow. The basement is of Paleozoic age and it is composed of metamorphic and calcareous schists. Cretaceous limestone and sandstone deposits unconformably overly the basement; granodiorite intrusions intercept both series. Tertiary discordant volcanic and conglomerate sequences are followed by quaternary unconsolidated sandstone covers. A series of submarine vents are aligned NW-SE along a 100 m long fracture in the basaltic bedrock at 10 m depth about 500 m offshore forming small chimneys and calcareous mounds composed of 95% CaCO3 and 5% MgCO3 with Ba, Hg, sulfides and phosphates mineralization. δ34S values in pyrite crystals are between −4.9‰ and −13.3‰ indicating a biogenic origin of the deposits. δ13C values in calcite (−39 ‰ to + 0.9‰) are consistent with precipitation from a mix of marine bicarbonate and oxidation of methane by microbial activity (Núñez Cornú et al., 2000; Canet et al., 2003; Alfonso et al., 2005). The emitted gas is N2 prevalent with methane and oxygen; δD and δ18O suggest that the hydrothermal fluids are a mix of seawater and meteoric water (Table 1b) with an infiltration altitude of about 500 m (Prol-Ledesma et al., 2002; Taran et al., 2002; Canet et al., 2003).
A study by Dávila-Ramos et al. (2015) comparably investigated the bacterial communities from Bahía Conception (BC) and Punta Mita (PM), which mainly differ in terms of redox potential (0.5 V in BC and −0.3 V in PM) and pH (6.2 in BC and 4.5 in PM). The community from BC was dominated by gamma- and deltaproteobacteria, Campylobacteria, as well as Bacteroidetes, whereas the low redox potential from PM favored the development of Thermotogae, Aquificae, and Planctomycetes. The high concentrations of methane in PM lead to the discovery of bacteria related to Methylothermus thermalis, which is a moderately thermophilic methanotroph.
On the northern Pacific shore of BCP, the Punta Banda peninsula hosts geothermal emissions along the NW trending strike-slip Agua Blanca fault. The vents are both intertidal and submarine and the emitted fluids are a mix of meteoric geothermal water and seawater with the geothermal end member concentration between 60 to 100% of the emissions (Table 1b). Silica geothermometers indicate reservoir temperature from 110 to 137°C. Precipitates of pyrite and gypsum enriched in As, Hg, Sb and Tl are generated from the cooling of the geothermal fluids mixing with the surrounding colder seawater. The main gas phase components are 51% CH4 and 44% N2 (Prol-Ledesma, 2003; Arango-Galván et al., 2011).
Dominica, St. Lucia, Mont Serrat (Lesser Antilles Volcanic Arc)
The Caribbean Sea is a tectonically active region, as the Caribbean Plate moves eastward with respect to the North and South American Plates and it collides with the Atlantic Plate, which is then subducted beneath the Caribbean Sea producing the active Lesser Antilles Volcanic Arc (Johnson and Cronan, 2001), giving rise to a series of islands stretching from Grenada in the South to Saba in the North. The younger arc is made up of the volcanic islands of Dominica, Guadeloupe, Montserrat, Nevis, St. Kitts, St. Eustatius and Saba, all with histories of late Tertiary and Quaternary volcanism. Several of the islands host underwater vents (Figures 1g–i).
On Dominica Island the volcanic activity is relatively recent being restricted to Pliocene-Quaternary periods. Several Pleistocene composite andesitic volcanoes are superimposed upon older Middle Pliocene shield volcanoes creating a N-S trend. About 30 Kyr ago the Roseau Ignimbrite eruption marked a shift toward effusive volcanism with formation of pelean dome complexes composed by abdesite/dacite lava (McCarthy, 2004).
The Plat Pays volcanic complex on the southern tip of the island is characterized by shallow seismicity and intense geothermal activity both on-shore and off-shore. Two submarine hydrothermal areas are located along the south-western coast of Dominica: Champagne Hot Springs (CHS) and Soufriere (SOU). The submarine activity is represented by punctual vents emitting clear fluids and by diffuse bubbling through sand and fractured volcanic rocks (Kleint et al., 2017).
The CHS are aligned along two E-W trending fissures in the bedrock composed by medium-K calc-andesites lava. More than 50 vents have been identified at 3-4 m of water depth. Hydrous ferric oxide precipitates in layers up to 5 cm thick are present around the vents caused by a strong enrichment in Fe (13-fold with values up to 12% in weight) of the sediments compared to the usual concentrations in the Caribbean sediments. Elevated values in AsIII (up to 311 ppm) are also measured in the sediments likely due to As adsorption by the hydrous ferric oxide. The main source of the fluids is identified in a meteoretically derived hydrothermal member with variable percentage of seawater entrained in the flow (Johnson and Cronan, 2001; McCarthy et al., 2005). This hypothesis is supported by the values of salinity (17.3‰ for CHS and 10.9‰ for SOU) that are consistently lower than the surrounding seawater. Chemical data are presented in Table 1b. The SOU site is composed by more than 100 vents in lineaments up to 10 m long in water depth up to 10 m. The sediments are enriched in Fe (29.8%) and As (477 ppm) forming halos of alteration around the vents (Johnson and Cronan, 2001). Other vents are present in St. Kitts and Toucary Bay in similarly shallow water (1 – 5 m) creating Fe enrichment of the sediments of 12.1% and 23. 5% respectively (Johnson and Cronan, 2001).
Kleint et al. (2017) investigated the concentration, size fraction distribution (colloidal and soluble) as well as redox speciation and labile concentrations of the micronutrient iron (Fe) in the fluids of the hydrothermal system off Dominica and found them highly enriched in Fe(II), indicating a strong complexation of Fe(II), strong enough to prevent oxidation and precipitation. The direct release of stabilized Fe(II) into the photic zone might influence production in the coastal waters. A study by Gomez-Saez et al. (2015) investigated the hydrothermal fluids of the hydrothermal vents off Dominica as a source of dissolved organic matter and its interactions with the abundantly present dissolved iron. The study provides evidence of co-precipitation of DOM with iron at hydrothermal systems as a selective process, which characteristically alters the molecular composition of DOM released with the hydrothermal fluids.
Bacterial community structure analysis revealed a significantly lower alpha diversity in the hydrothermally impacted sites, with the presence of key players in iron cycling generally known from deep-sea vents (e.g., Zetaproteobacteria, Deferribacteres), supporting the potential importance of microbes performing iron redox processes. The microbial community composition has been characterized using next generation sequencing, revealing Gammaproteobacteria as the most abundant class in vent sediments (Pop Ristova et al., 2017). Microbial carbon fixation was investigated using stable isotope probing experiments with 13C-bicarbonate under light and dark conditions (Gomez-Saez et al., 2017). The authors found that chemoautotrophy was responsible for up to 65% of the total carbon fixation, emphasizing dark carbon fixation as a prominent process for biomass production in marine coastal environments influenced by hydrothermalism.
Submarine vents of St. Lucia are present in the Qualibou caldera along the south-western coast of the island at about 5m of depth. The emitted fluids have temperature up to 35°C and the surrounding sediments are enriched in Fe (17.4%) and As (243 ppm) due to the precipitates from the vents (Johnson and Cronan, 2001).
On the west coast of the island of Mont Serrat an area of intermittent bubbling from the seafloor in shallow water (2- 5 m) is present, with up to 100 vents active at one time. The temperature of the fluids went up to 57°C after the onset of a major eruption on the island. The sediments surrounding the vents are enriched in Fe (8.1%) and As (7,060 ppm). The shallow-water settings create a high-energy and high-oxidizing environment thus preventing substantial building-up of mineral deposits but sub-surface phase-separation could generate sizeable mineralization at shallow depth below the venting field (Johnson and Cronan, 2001).
Technologies for Sampling, Monitoring, and Detection
Different methods can be used to identify the presence of geothermal vents on the seafloor including geochemical, geophysical and visual tools.
Fluid and Sediment Sampling
The shallow-water vents are often within the range of scuba activity and therefore can be studied by means of scientific divers. Fluids sampling techniques have been developed for the geochemical monitoring of volcanic seeps (Tarasov and Zhirmunskaya, 1989; Marteinsson et al., 2001; Caramanna et al., 2005; Chen et al., 2005). Devices can be deployed for measuring the fluxes of the emitted fluids (Bradley et al., 2010) and current meters have been positioned by divers to quantify the water entrainment in gas plumes (Aliani et al., 2010). In situ studies of the impact of the fluids on the marine biota have been conducted by divers in several of the seepage areas (Hall-Spencer et al., 2008; Manini et al., 2008).
The microbial communities within hydrothermally impacted sediments can be studied by diver-operated sampling of surface sediment or by using push cores (e.g., Sievert et al., 1999; Giovannelli et al., 2013; Pop Ristova et al., 2017). Microbially mediated processes have been studied by mimicking natural conditions in laboratory incubations (e.g., Gomez-Saez et al., 2017).
Acoustic Detection
The presence of gas bubbles within the water column above the seepages can be detected by acoustic methods. The gas-phase represents a very strong target for sonar with its sharp difference in density when compared with the surrounding liquid environment; it is therefore possible to track the bubbles plume during its rising through the water column (von Deimling et al., 2010; Rovere et al., 2014). Gas-charged sediments show a characteristic acoustic turbidity creating blank volumes when sounded by sub-bottom profilers and seismic methods (Hovland, 1991; Fleischer et al., 2001; Schroot and Schüttenhelm, 2003; Passaro et al., 2014). Other acoustic signatures of gas in the sub-bottom are the evidence of pipes and conduits that can be still active or relics of a former plumbing system for the seeping fluids (Orange et al., 2005; Schroot et al., 2005).
Morphological Indicators
Morphological features on the seafloor that are associated with the current or past presence of fluids emissions and that can be used to identify areas of seepage include:
• Chimneys: geothermal fluids are often vented from the seafloor through chimneys made of precipitate minerals such as carbonates, silicates and sulfurs often acting as cement of sediments or pebbles (Hannington et al., 2011; Zeng et al., 2011).
• Carbonate outcrops: deposition of carbonates are linked to the fast degassing of CO2 and are found around both cold seeps and geothermal vents. Some microbial activity is also associated with the formation of authigenic carbonates (Conti et al., 2002; Capozzi et al., 2012).
• Local fault and fracture system: the presence of a fault system in the bedrock is often associated with emission of fluids from the seafloor. The fractures representing a preferential path for the fluids (Chiodini et al., 2006; Khimasia et al., 2021).
Geochemical Signatures
Emissions of acidic fluids can generate plumes with pH values far below the normal levels of the oceans. Measuring the water pH in vertical logs and horizontal transects is a method for identifying seepage areas (Le Bris et al., 2001). Microsensor profiling can also be used to monitor sub-millimeter changes of other parameters in hydrothermally influenced sediments, like temperature, oxygen, and total sulfide (Wenzhöfer et al., 2000; Yücel et al., 2013; Sollich et al., 2017). Positive anomalies in dissolved gases such as CO2 and CH4 are indicative of the presence of submarine fluids both as geothermal vents or cold seeps. Autonomous underwater vehicles (AUV) can be equipped with specific suites of chemical sensors including pH meters and PCO2 sensors; they can be used for underwater surveys aimed at the identification of seeping areas (Brewer et al., 2004; Shitashima et al., 2013).
Conclusion and Future Research Directions
Shallow-water vents are ideal targets to study the interplay between the geological forces, environmental stressors and their imprint on life (Bühring and Sievert, 2017; Price and Giovannelli, 2017). Interdisciplinary research approaches targeting shallow hydrothermal systems worldwide have the potential to transform our knowledge from seemingly isolated extreme environments toward a general understanding of global element cycling and transport, microbial adaptation, and evolution.
Overall, the large scatter in the environmental data compiled within this review prevents a convincing, unambiguous and holistic comparison between the different vent systems. Taken together, the results suggest a more standardized sampling and analytical procedure for shallow water hydrothermal systems in order to reach a global understanding. Besides the detection of major and minor elements of the fluid, we suggest to include the analyses of the gas composition, completed by the detection of temperature and pH along vertical and horizontal gradients. To quantify the hydrothermal input from shallow hydrothermal system to coastal oceans, a holistic study of the transport of hydrothermal elements is necessary, following the example of Khimasia et al. (2021), but also taking into consideration the different phases and exchanges between dissolved, colloidal, and nanoparticles as well as complexation with organic material.
The analysis of microbial diversity and function allows further understanding of the underlying geobiological potential. Especially the application of different -omics techniques, like functional metagenomics to study gene and protein expression and function of the whole community or metabolomics to identify unique chemical fingerprints that cellular processes leave behind, are very promising approaches that can lead to an in depth understanding of ecosystem functioning, in particular when they are coupled to detailed geochemical characterization and rate measurements of relevant processes.
Finally, shallow-water geothermal vents emitting CO2 can be used as a “natural laboratory” for the study of the potential impact of ocean acidification and global warming on marine life. Moreover, a variety of techniques for gas and liquid emission detection and monitoring can be developed and tested in these scenarios with a cost-effective attitude before being deployed in larger or deeper areas.
Author Contributions
GC conceived the idea for the review. GC, SMS, and SIB wrote the manuscript. All authors contributed to the article and approved the submitted version.
Funding
SMS was supported by the United States National Science Foundation (OCE-1124272) and the WHOI Investment in Science Fund and SIB by the Deutsche Forschungsgemeinschaft (Emmy Noether grant BU 2606/1).
Conflict of Interest
GC is employed by GeoAqua Consulting.
The remaining authors declare that the research was conducted in the absence of any commercial or financial relationships that could be construed as a potential conflict of interest.
Publisher’s Note
All claims expressed in this article are solely those of the authors and do not necessarily represent those of their affiliated organizations, or those of the publisher, the editors and the reviewers. Any product that may be evaluated in this article, or claim that may be made by its manufacturer, is not guaranteed or endorsed by the publisher.
Acknowledgments
We would like to acknowledge our collaborators in shallow-water hydrothermal vent research: Jan Amend, Dionysis I. Foustoukos, Donato Giovannelli, Athanasios Godelitsas, Andrea Koschinsky, Nadine Le Bris, Thomas Pichler, Petra Pop Ristova, Roy Price, Costantino Vetriani, and Mustafa Yücel. Further thanks to the reviewers for their valuable comments.
Supplementary Material
The Supplementary Material for this article can be found online at: https://www.frontiersin.org/articles/10.3389/fmars.2021.727199/full#supplementary-material
Supplementary Table 1 | Location, depth and distance from shore of the shallow water hydrothermal systems. Coordinates calculated from Google Earth.
References
Aguiar, P., and Costa, A. C. (2010). “Shallow hydrothermal vents and marine protected areas within the Azores Archipelago,” in Geographic Technologies Applied to Marine Spatial Planning and Integrated Coastal Zone Management, eds H. Calado and A. Gil (Ponta Delgada: Universidade dos Acores).
Ahmann, D., Krumholz, L. R., Hemond, H. F., Lovley, D. R., and Morel, F. M. M. (1997). Microbial mobilization of arsenic from sediments of the Aberjona Watershed. Environ. Sci. Technol. 31, 2923–2930.
Aiuppa, A., Hall-Spencer, J. M., Milazzo, M., Turco, G., Caliro, S., and Di Napoli, R. (2021). Volcanic CO2 seep geochemistry and use in understanding ocean acidification. Biogeochemistry 152, 93–115.
Akerman, N. H., Price, R. E., Pichler, T., and Amend, J. P. (2011). Energy sources for chemolithotrophs in an arsenic- and iron-rich shallow-sea hydrothermal system. Geobiology 9, 436–445. doi: 10.1111/j.1472-4669.2011.00291.x
Alfonso, P., Prol-Ledesma, R. M., Canet, C., Melgarejo, J. C., and Fallick, A. E. (2005). Isotopic evidence for biogenic precipitation as a principal mineralization process in coastal gasohydrothermal vents, Punta Mita, Mexico. Chem. Geol. 224, 113–121.
Alfredsson, G. A., Kristjansson, J. K., Hjörleifsdottir, S., and Stetter, K. O. (1988). Rhodothermus marinus, gen. nov., sp. nov., a Thermophilic, Halophilic Bacterium from Submarine Hot Springs in Iceland. Microbiology 134, 299–306.
Aliani, S., Bortoluzzi, G., Caramanna, G., and Raffa, F. (2010). Seawater dynamics and environmental settings after November 2002 gas eruption off Bottaro (Panarea, Aeolian Islands, Mediterranean Sea). Cont. Shelf Res. 30, 1338–1348.
Aliani, S., Meloni, R., and Dando, P. R. (2004). Periodicities in sediment temperature time-series at a marine shallow water hydrothermal vent in Milos Island (Aegean Volcanic arc, Eastern Mediterranean). J. Mar. Syst. 46, 109–119.
Amend, J. P., Rogers, K. L., Shock, E. L., Gurrieri, S., and Inguaggiato, S. (2003). Energetics of chemolithoautotrophy in the hydrothermal system of Vulcano Island, southern Italy. Geobiology 1, 37–58.
Antranikian, G., Suleiman, M., Schäfers, C., Adams, M. W. W., Bartolucci, S., Blamey, J. M., et al. (2017). Diversity of bacteria and archaea from two shallow marine hydrothermal vents from Vulcano Island. Extremophiles 21, 733–742. doi: 10.1007/s00792-017-0938-y
Appolloni, L., Zeppilli, D., Donnarumma, L., Baldrighi, E., Chianese, E., Russo, G. F., et al. (2020). Seawater Acidification Affects Beta-Diversity of Benthic Communities at a Shallow Hydrothermal Vent in a Mediterranean Marine Protected Area (Underwater Archaeological Park of Baia, Naples, Italy). Diversity 12:464.
Arab, H., Volker, H., and Thomm, M. (2000). Thermococcus aegaeicus sp nov and Staphylothermus hellenicus sp nov., two novel hyperthermophilic archaea isolated from geothermally heated vents off Palaeochori Bay, Milos, Greece. Int. J. Syst. Evol. Microbiol. 50, 2101–2108. doi: 10.1099/00207713-50-6-2101
Arango-Galván, C., Prol-Ledesma, R. M., Flores-Márquez, E. L., Canet, C., and Villanueva Estrada, R. E. (2011). Shallow submarine and subaerial, low-enthalpy hydrothermal manifestations in Punta Banda, Baja California, Mexico: geophysical and geochemical characterization. Geothermics 40, 102–111.
Ármannsson, H., Fridriksson, T., and Kristjánsson, B. R. (2005). CO2 emissions from geothermal power plants and natural geothermal activity in Iceland. Geothermics 34, 286–296. doi: 10.1016/j.dib.2019.104339
Arnórsson, S. (1995). Geothermal systems in Iceland: structure and conceptual models—I. High-temperature areas. Geothermics 24, 561–602.
Baldrighi, E., Zeppille, D., Appolloni, L., Donnarumma, L., Chianese, E., Russo, G. F., et al. (2020). Meiofaunal communities and nematode diversity characterizing the Secca delle Fumose shallow vent area (Gulf of Naples, Italy). PeerJ 8:e9058. doi: 10.7717/peerj.9058
Barberi, F., Carapezza, M. L., Tarchini, L., Ranaldi, M., Ricci, T., and Gattuso, A. (2019). Anomalous Discharge of Endogenous Gas at Lavinio (Rome, Italy) and the Lethal Accident of 5 September 2011. GeoHealth 3, 407–422. doi: 10.1029/2019GH000211
Barragán, R., Birkle, P., Portugal, M. E., Arellano, G., and Alvarez, R. (2001). Geochemical survey of medium temperature geothermal resources from the Baja California Peninsula and Sonora, México. J. Volcanol. Geotherm. Res. 110, 101–119.
Batiza, R. (1982). Abundances, distribution and sizes of Volcanos in the pacific-ocean and implications for the origin of non-hotspot Volcanos. Earth Planet. Sci. Lett. 60, 195–206.
Bennett, S. A., Statham, P. J., Green, D. R. H., Le Bris, N., Mcdermott, J. M., Prado, F., et al. (2011). Dissolved and particulate organic carbon in hydrothermal plumes from the East Pacific Rise, 9°50′N. Deep Sea Res. I Oceanogr. Res. Pap. 58, 922–931.
Binns, R. A., and Scott, S. D. (1993). Actively forming polymetallic sulphide deposits associated with felsic volcanic rocks in the eastern Manus back-arc basin, Papua New Guinea. Econ. Geol. 88, 2226–2236.
Botz, R., Wehner, H., Schmitt, M., Worthington, T. J., Schmidt, M., and Stoffers, P. (2002). Thermogenic hydrocarbons from the offshore Calypso hydrothermal field, Bay of Plenty, New Zealand. Chem. Geol. 186, 235–248.
Bradley, E., Leifer, I., and Roberts, D. (2010). Long-term monitoring of a marine geologic hydrocarbon source by a coastal air pollution station in Southern California. Atmos. Environ. 44, 4973–4981.
Brewer, P. G., Peltzer, E., Aya, I., Haugan, P., Bellerby, R., Yamane, K., et al. (2004). Small scale field study of an ocean CO2 Plume. J. Oceanogr. 60, 751–758.
Brinkhoff, T., Sievert, S. M., Kuever, J., and Muyzer, G. (1999). Distribution and diversity of sulfur-oxidizing Thiomicrospira spp. at a shallow-water hydrothermal vent in the Aegean Sea (Milos, Greece). Appl. Environ. Microbiol. 65, 3843–3849. doi: 10.1128/AEM.65.9.3843-3849.1999
Bühring, S. I., and Sievert, S. M. (2017). “The shallow submarine hot vent system off Milos (Greece) – a natural laboratory to study hydrothermal geomicrobiology,” in Life at Vents and Seeps (Life in Extreme Environments), ed. J. Kallmeyer (Berlin: De Gruyter).
Burggraf, S., Olsen, G. J., Stetter, K. O., and Woese, C. R. (1992). A phylogenetic analysis of Aquifex pyrophilus. Syst. Appl. Microbiol. 15, 352–356. doi: 10.1016/S0723-2020(11)80207-9
Callac, N., Posth, N. R., Rattray, J. E., Yamoah, K. K. Y., Wiech, A., Ivarsson, M., et al. (2017). Modes of carbon fixation in an arsenic and CO2-rich shallow hydrothermal ecosystem. Sci. Rep. 7:14708. doi: 10.1038/s41598-017-13910-2
Canet, C., Marı, X., Prol-Ledesma, R. M., Melgarejo, J.-C., and Reyes, A. (2003). Methane-related carbonates formed at submarine hydrothermal springs: A new setting for microbially-derived carbonates? Mar. Geol. 199, 245–261.
Canet, C., Prol-Ledesma, R. M., Torres-Alvarado, I., Gilg, H. A., Villanueva, R. E., and Cruz, R. L.-S. (2005b). Silica-carbonate stromatolites related to coastal hydrothermal venting in Bahía Concepción, Baja California Sur, Mexico. Sediment. Geol. 174, 97–113.
Canet, C., Prol-Ledesma, R. M., Proenza, J. A., Rubio-Ramos, M. A., Forrest, M. J., Torres-Vera, M. A., et al. (2005a). Mn–Ba–Hg mineralization at shallow submarine hydrothermal vents in Bahía Concepción, Baja California Sur, Mexico. Chem. Geol. 224, 96–112.
Capasso, G., Favara, R., Francofonte, S., and Inguaggiato, S. (1999). Chemical and isotopic variations in fumarolic discharge and thermal waters at Vulcano Island (Aeolian Islands, Italy) during 1996: evidence of resumed volcanic activity. J. Volcanol. Geotherm. Res. 88, 167–175.
Capozzi, R., Guido, F. L., Oppo, D., and Gabbianelli, G. (2012). Methane-Derived Authigenic Carbonates (MDAC) in northern-central Adriatic Sea: relationships between reservoir and methane seepages. Mar. Geol. 332-334, 174–188.
Caramanna, G., Voltattorni, N., and Maroto-Valer, M. M. (2011). Is Panarea Island (Italy) a valid and cost-effective natural laboratory for the development of detection and monitoring techniques for submarine CO2 seepage? Greenhouse Gas. Sci. Technol. 1, 200–210.
Caramanna, G., Voltattorni, N., Caramanna, L., Cinti, D., Galli, G., Pizzino, L., et al. (2005). “Scientific diving techniques applied to the geomorphological and geochemical study of some submarine volcanic gas vents (Aeolian Islands, Southern Tyrrhenian Sea, Italy),” in Proceedings of the 2005 American Academy of Underwater Sciences Symposium, Groton, CT.
Carapezza, M. L., Ranaldi, M., Tarchini, L., Ricci, T., and Barberi, F. (2020). Origin and hazard of CO2 and H2S emissions in the Lavinio-Tor Caldara zone (Metropolitan City of Rome Capital, Italy). J. Volcanol. Geotherm. Res. 402:106985.
Cardigos, F., Colaco, A., Dando, P. R., Avila, S. P., Sarradin, P. M., Tempera, F., et al. (2005). Shallow water hydrothermal vent field fluids and communities of the D. Joao de Castro Seamount (Azores). Chem. Geol. 224, 153–168.
Carvalho, M. D. R., Mateus, A., Nunes, J. C., and Carvalho, J. M. (2011). Chemistry of the Ferraria thermal water, S. Miguel Island, Azores: mixing and precipitation processes. Environ. Earth Sci. 64, 539–547.
Chan, B. K. K., Wang, T.-W., Chen, P.-C., Lin, C.-W., Chan, T.-Y., and Tsang, L. M. (2016). Community structure of Macrobiota and environmental parameters in shallow water hydrothermal vents off Kueishan Island, Taiwan. PLoS One 11:e0148675. doi: 10.1371/journal.pone.0148675
Chang, N.-N., Lin, L.-H., Tu, T.-H., Jeng, M.-S., Chikaraishi, Y., and Wang, P.-L. (2018). Trophic structure and energy flow in a shallow-water hydrothermal vent: insights from a stable isotope approach. PLoS One 13:e0204753. doi: 10.1371/journal.pone.0204753
Chen, C. T. A., Zeng, Z. G., Kuo, F. W., Yang, T. Y. F., Wang, B. J., and Tu, Y. Y. (2005). Tide-influenced acidic hydrothermal system offshore NE Taiwan. Chem. Geol. 224, 69–81.
Chen, X. G., Lyu, S. S., Garbe-Schonberg, D., Lebrato, M., Li, X. H., Zhang, H. Y., et al. (2018). Heavy metals from Kueishantao shallow-sea hydrothermal vents, offshore northeast Taiwan. J. Mar. Syst. 180, 211–219.
Chiodini, G., Avino, R., Brombach, T., Caliro, S., Cardellini, C., De Vita, S., et al. (2004). Fumarolic and diffuse soil degassing west of Mount Epomeo, Ischia, Italy. J. Volcanol. Geotherm. Res. 133, 291–309.
Chiodini, G., Caliro, S., Caramanna, G., Granieri, D., Minopoli, C., Moretti, R., et al. (2006). Geochemistry of the submarine gaseous emissions of panarea (Aeolian Islands, Southern Italy): magmatic vs. hydrothermal origin and implications for volcanic surveillance. Pure Appl. Geophys. 163, 759–780.
Conte, A. M., and Caramanna, G. (2010). Preliminary characterisation of a shallow water hydrothermal sulphide deposit recovered by scientific divers (Aeolian Islands, southern Tyrrhenian Sea). Underwater Technol. 29, 109–115.
Conti, A., Stefanon, A., and Zuppi, G. M. (2002). Gas seeps and rock formation in the northern Adriatic Sea. Cont. Shelf Res. 22, 2333–2344.
Cosel, V. (2008). A new Bathymodioline mussel (Bivalvia: Mytiloidea: Mytilidae: Bathymodiolinae) from vent sites near Kueishan Island, north east Taiwan. Raffles Bull. Zool. 19, 105–114.
Couto, R. P., Neto, A. I., and Rodrigues, A. S. (2010). Metal concentration and structural changes in Corallina elongata (Corallinales, Rhodophyta) from hydrothermal vents. Mar. Pollut. Bull. 60, 509–514. doi: 10.1016/j.marpolbul.2009.11.014
Craig, H., and Horibe, Y. (1994). 3He and methane in Sakurajima Caldera, Kagoshima Bay, Japan. Earth Planet. Sci. Lett. 123, 221–226.
Cruz, J. V. (2003). Volcanoes geochemical monitoring in the Azores Archipelago: overview and prospect for the future. Vulcanica 1, 7–21.
Cruz, J. V., and França, Z. (2006). Hydrogeochemistry of thermal and mineral water springs of the Azores archipelago (Portugal). J. Volcanol. Geotherm. Res. 151, 382–398.
Cruz, J. V., Antunes, P., Amaral, C., França, Z., and Nunes, J. C. (2006). Volcanic lakes of the Azores archipelago (Portugal): geological setting and geochemical characterization. J. Volcanol. Geotherm. Res. 156, 135–157.
Cunha, L., Amaral, A., Medeiros, V., Martins, G. M., Wallenstein, F. F. M. M., Couto, R. P., et al. (2008). Bioavailable metals and cellular effects in the digestive gland of marine limpets living close to shallow water hydrothermal vents. Chemosphere 71, 1356–1362. doi: 10.1016/j.chemosphere.2007.11.022
Dando, P. R. (2010). “Biological Communities at Marine Shallow-Water Vent and Seep Sites,” in The Vent and Seep Biota: Aspects from Microbes to Ecosystems, ed. S. Kiel (Dordrecht: Springer), 333–378. doi: 10.1016/B978-0-12-381015-1.00001-0
Dando, P. R., Aliani, S., Arab, H., Bianchi, C. N., Brehmer, M., Cocito, S., et al. (2000). Hydrothermal studies in the Aegean Sea. Phys. Chem. Earth B Hydrol. Oceans Atmos. 25, 1–8.
Dando, P. R., Hughes, J. A., Leahy, Y., Niven, S. J., Taylor, L. J., and Smith, C. (1995). Gas venting rates from submarine hydrothermal areas around the island of Milos, Hellenic volcanic arc. Cont. Shelf Res. 15, 913–929.
Dando, P. R., Stuben, D., and Varnavas, S. P. (1999). Hydrothermalism in the Mediterranean Sea. Prog. Oceanogr. 44, 333–367.
Dando, P. R., Thomm, M., Arab, H., Brehmer, M., Hooper, L. E., Jochimsen, B., et al. (1998). Microbiology of shallow hydrothermal sites off Palaeochori Bay, Milos (Hellenic Volcanic Arc). Cah. Biol. Mar. 39, 369–372.
Dávila-Ramos, S., Estradas-Romero, A., Prol-Ledesma, R. M., and Juárez-López, K. (2015). Bacterial Populations (First Record) at Two Shallow Hydrothermal Vents of the Mexican Pacific West Coast. Geomicrobiol. J. 32, 657–665.
De Rosa, R., Guillou, H., Mazzuoli, R., and Ventura, G. (2003). New unspiked K–Ar ages of volcanic rocks of the central and western sector of the Aeolian Islands: reconstruction of the volcanic stages. J. Volcanol. Geotherm. Res. 120, 161–178.
Dereinda, F. (2008). CO2 Emissions from the Krafla geothermal Area, Iceland. (Reykjavik: United Nations University).
Di Napoli, R., Aiuppa, A., Bellomo, S., Brusca, L., D’alessandro, W., Candela, E. G., et al. (2009). A model for Ischia hydrothermal system: evidences from the chemistry of thermal groundwaters. J. Volcanol. Geotherm. Res. 186, 133–159.
Dittmar, T., and Koch, B. P. (2006). Thermogenic organic matter dissolved in the abyssal ocean. Mar. Chem. 102, 208–217.
Donachie, S. P., Christenson, B. W., Kunkel, D. D., Malahoff, A., and Alam, M. (2002). Microbial community in acidic hydrothermal waters of volcanically active White Island, New Zealand. Extremophiles 6, 419–425. doi: 10.1007/s00792-002-0274-7
Donnarumma, L., Appolloni, L., Chianese, E., Bruno, R., Baldrighi, E., Guglielmo, R., et al. (2019). Environmental and Benthic Community Patterns of the Shallow Hydrothermal Area of Secca Delle Fumose (Baia, Naples, Italy). Front. Mar. Sci. 6:685. doi: 10.3389/fmars.2019.00685
Durán-Toro, V. M., Price, R. E., Maas, M., Brombach, C. C., Pichler, T., Rezwan, K., et al. (2019). Amorphous arsenic sulfide nanoparticles in a shallow water hydrothermal system. Mar. Chem. 211, 25–36.
Durán-Toro, V., Rezwan, K., Bühring, S. I., and Maas, M. (2022). Arsenic and sulfur nanoparticle synthesis mimicking environmental conditions of submarine shallow-water hydrothermal vents. J. Environ. Sci. 111, 301–312.
Espa, S., Caramanna, G., and Bouché, V. (2010). Field study and laboratory experiments of bubble plumes in shallow seas as analogues of sub-seabed CO2 leakages. Appl. Geochem. 25, 696–704.
Fitzsimmons, J. N., John, S. G., Marsay, C. M., Hoffman, C. L., Nicholas, S. L., Toner, B. M., et al. (2017). Iron persistence in a distal hydrothermal plume supported by dissolved-particulate exchange. Nat. Geosci. 10, 195–201.
Fitzsimons, M. F., Dando, P. R., Hughes, J. A., Thiermann, F., Akoumianaki, I., and Pratt, S. M. (1997). Submarine hydrothermal brine seeps off Milos, Greece: observations and geochemistry. Mar. Chem. 57, 325–340.
Fleischer, P., Orsi, T. H., Richardson, M. D., and Anerson, A. L. (2001). Distribution of free gas in marine sediments: a global overview. Geo Mar. Lett. 21, 103–122.
Flóvenz, Ó. G., and Saemundsson, K. (1993). Heat flow and geothermal processes in Iceland. Tectonophysics 225, 123–138. doi: 10.1111/jiec.12100
Forrest, M. J., Ledesma-Vázquez, J., Ussler, W., Kulongoski, J. T., Hilton, D. R., and Greene, H. G. (2005). Gas geochemistry of a shallow submarine hydrothermal vent associated with the El Requesón fault zone, Bahía Concepción, Baja California Sur, México. Chem. Geol. 224, 82–95.
Foulger, G. R., and Anderson, D. L. (2005). A cool model for the Iceland hotspot. J. Volcanol. Geotherm. Res. 141, 1–22.
Fricke, H., Giere, O., Stetter, K., Alfredsson, G. A., Kristjansson, J. K., Stoffers, P., et al. (1989). Hydrothermal vent communities at the shallow subpolar Mid-Atlantic ridge. Mar. Biol. 102, 425–429.
Giggenbach, W. F. (1987). Redox processes governing the chemistry of fumarolic gas discharges from White Island, New Zealand. Appl. Geochem. 2, 143–161.
Giovannelli, D., D’errico, G., Manini, E., Yakimov, M., and Vetriani, C. (2013). Diversity and phylogenetic analyses of bacteria from a shallow-water hydrothermal vent in Milos island (Greece). Front. Microbiol. 4:184. doi: 10.3389/fmicb.2013.00184
Giovannelli, D., Grosche, A., Starovoytov, V., Yakimov, M., Manini, E., and Vetriani, C. (2012). Galenea microaerophila gen. nov., sp. nov., a mesophilic, microaerophilic, chemosynthetic, thiosulfate-oxidizing bacterium isolated from a shallow water hydrothermal vent. Int. J. Syst. Evol. Microbiol. 62(Pt 12), 3060–3066. doi: 10.1099/ijs.0.040808-0
Glasby, G. P., Cherkashov, G. A., Gavrilenko, G. M., Rashidov, V. A., and Slovtsov, I. B. (2006). Submarine hydrothermal activity and mineralization on the Kurile and western Aleutian island arcs, N.W. Pacific. Mar. Geol. 231, 163–180.
Godelitsas, A., Price, R. E., Pichler, T., Amend, J., Gamaletsos, P., and Göttlicher, J. (2015). Amorphous As-sulfide precipitates from the shallow-water hydrothermal vents off Milos Island (Greece). Mar. Chem. 177(Part 5), 687–696.
Gomez-Saez, G. V., Niggemann, J., Dittmar, T., Pohlabeln, A. M., Lang, S. Q., Noowong, A., et al. (2016). Molecular evidence for abiotic sulfurization of dissolved organic matter in marine shallow hydrothermal systems. Geochim. Cosmochim. Acta 190, 35–52.
Gomez-Saez, G. V., Pop Ristova, P., Sievert, S. M., Elvert, M., Hinrichs, K.-U., and Bühring, S. I. (2017). Relative importance of chemoautotrophy for primary production in a light exposed marine shallow hydrothermal system. Front. Microbiol. 8:702. doi: 10.3389/fmicb.2017.00702
Gomez-Saez, G. V., Riedel, T., Niggemann, J., Pichler, T., Dittmar, T., and Bühring, S. I. (2015). Interaction between iron and dissolved organic matter in a marine shallow hydrothermal system off Dominica Island (Lesser Antilles). Mar. Chem. 177(Part 4), 677–686.
Gugliandolo, C., and Maugeri, T. L. (1993). Chemolithotrophic, sulfur-oxidizing bacteria from a marine, shallow hydrothermal vent of Vulcano (Italy). Geomicrobiol. J. 11, 109–120.
Gugliandolo, C., and Maugeri, T. L. (2019). Phylogenetic Diversity of Archaea in Shallow Hydrothermal Vents of Eolian Islands, Italy. Diversity 11:156.
Gugliandolo, C., Italiano, F., Maugeri, T. L., Inguaggiato, S., Caccamo, D., and Amend, J. P. (1999). Submarine hydrothermal vents of the Aeolian Islands: relationship between microbial communities and thermal fluids. Geomicrobiol. J. 16, 105–117.
Hall-Spencer, J. M., Rodolfo-Metalpa, R., Martin, S., Ransome, E., Fine, M., Turner, S. M., et al. (2008). Volcanic carbon dioxide vents show ecosystem effects of ocean acidification. Nature 454, 96–99.
Handley, K. M., Hery, M., and Lloyd, J. R. (2009). Redox cycling of arsenic by the hydrothermal marine bacterium Marinobacter santoriniensis. Environ. Microbiol. 11, 1601–1611. doi: 10.1111/j.1462-2920.2009.01890.x
Hannington, M., Herzig, P., Stoffers, P., Scholten, J., Botz, R., Garbe-Schönberg, D., et al. (2001). First observations of high-temperature submarine hydrothermal vents and massive anhydrite deposits off the north coast of Iceland. Mar. Geol. 177, 199–220.
Hannington, M., Jamieson, J., Monecke, T., Petersen, S., and Beaulieu, S. (2011). The abundance of seafloor massive sulfide deposits. Geology 39, 1155–1158. doi: 10.1128/mBio.00279-11
Hoaki, T., Nishijima, M., Miyashita, H., and Maruyama, T. (1995). Dense Community of Hyperthermophilic Sulfur-Dependent Heterotrophs in a Geothermally Heated Shallow Submarine Biotope near Kodakara-Jima Island, Kagoshima, Japan. Appl. Environ. Microbiol. 61, 1931–1937. doi: 10.1128/aem.61.5.1931-1937.1995
Hobel, C. F. V., Marteinsson, V. T., Hreggvidsson, G. Ó., and Kristjánsson, J. K. (2005). Investigation of the microbial ecology of intertidal hot springs by using diversity analysis of 16S rRNA and chitinase genes. Appl. Environ. Microbiol. 71, 2771–2776. doi: 10.1128/AEM.71.5.2771-2776.2005
Hocking, M. W. A., Hannington, M. D., Percival, J. B., Stoffers, P., Schwarz-Schampera, U., and De Ronde, C. E. J. (2010). Clay alteration of volcaniclastic material in a submarine geothermal system, Bay of Plenty, New Zealand. J. Volcanol. Geotherm. Res. 191, 180–192.
Horwell, C. J., Patterson, J. E., Gamble, J. A., and Allen, A. G. (2005). Monitoring and mapping of hydrogen sulphide emissions across an active geothermal field: Rotorua, New Zealand. J. Volcanol. Geotherm. Res. 139, 259–269.
Houghton, J. L., Gilhooly, W. P., Kafantaris, F.-C. A., Druschel, G. K., Lu, G.-S., Amend, J. P., et al. (2019). Spatially and temporally variable sulfur cycling in shallow-sea hydrothermal vents, Milos, Greece. Mar. Chem. 208, 83–94.
Hovland, M. (1991). Large pockmarks, gas-charged sediments and possible clay diapirs in the Skagerrak. Mar. Pet. Geol. 8, 311–316.
Hu, M. Y. A., Hagen, W., Jeng, M. S., and Saborowski, R. (2012). Metabolic energy demand and food utilization of the hydrothermal vent crab Xenograpsus testudinatus (Crustacea: Brachyura). Aquat. Biol. 15, 11–25.
Huber, H., Burggraf, S., Mayer, T., Wyschkony, I., Rachel, R., and Stetter, K. O. (2000). Ignicoccus gen. nov., a novel genus of hyperthermophilic, chemolithoautotrophic Archaea, represented by two new species, Ignicoccus islandicus sp nov and Ignicoccus pacificus sp nov. and Ignicoccus pacificus sp. nov. Int. J. Syst. Evol. Microbiol. 50, 2093–2100. doi: 10.1099/00207713-50-6-2093
Huber, R., Wilharm, T., Huber, D., Trincone, A., Burggraf, S., König, H., et al. (1992). Aquifex pyrophilus gen. nov. sp. nov., Represents a Novel Group of Marine Hyperthermophilic Hydrogen-Oxidizing Bacteria. Syst. Appl. Microbiol. 15, 340–351.
Inguaggiato, S., Pecoraino, G., and D’amore, F. (2000). Chemical and isotopical characterisation of fluid manifestations of Ischia Island (Italy). J. Volcanol. Geotherm. Res. 99, 151–178.
Ishibashi, J.-I., Nakaseama, M., Seguchi, M., Yamashita, T., Doi, S., Sakamoto, T., et al. (2008). Marine shallow-water hydrothermal activity and mineralization at the Wakamiko crater in Kagoshima bay, south Kyushu, Japan. J. Volcanol. Geotherm. Res. 173, 84–98.
Jochimsen, B., Peinemann-Simon, S., Volker, H., Stuben, D., Botz, R., Stoffers, P., et al. (1997). Stetteria hydrogenophila, gen. nov. and sp. nov., a novel mixotrophic sulfur-dependent crenarchaeote isolated from Milos, Greece. Extremophiles 1, 67–73. doi: 10.1007/s007920050016
Johnson, A., and Cronan, D. S. (2001). Hydrothermal metalliferous sediments and waters off the Lesser Antilles. Mar. Georesour. Geotechnol. 19, 65–83.
Kadar, E., Fisher, A., Stolpe, B., Harrison, R. M., Parello, F., and Lead, J. (2012). Metallic nanoparticle enrichment at low temperature, shallow CO2 seeps in Southern Italy. Mar. Chem. 140-141, 24–32.
Kamenev, G. M., Fadeev, V. I., Selin, N. I., Tarasov, V. G., and Malakhov, V. V. (1993). Composition and distribution of macro- and meiobenthos around sublittoral hydrothermal vents in the Bay of Plenty, New Zealand. N.Z. J. Mar. Freshw. Res. 27, 407–418.
Kamenev, G. M., Kavun, V. Y., Tarasov, V. G., and Fadeev, V. I. (2004). Distribution of bivalve mollusks Macoma golikovi Scarlato and Kafanov, 1988 and Macoma calcarea (Gmelin, 1791) in the shallow-water hydrothermal ecosystem of Kraternaya Bight (Yankich Island, Kuril Islands): connection with feeding type and hydrothermal activity of Ushishir Volcano. Cont. Shelf Res. 24, 75–95.
Karuza, A., Celussi, M., Cibic, T., Del Negro, P., and De Vittor, C. (2012). Virioplankton and bacterioplankton in a shallow CO2-dominated hydrothermal vent (Panarea Island, Tyrrhenian Sea). Estuar. Coast. Shelf Sci. 97, 10–18.
Kawaichi, S., Ito, N., Kamikawa, R., Sugawara, T., Yoshida, T., and Sako, Y. (2013a). Ardenticatena maritima gen. nov., sp. nov., a ferric iron- and nitrate-reducing bacterium of the phylum ‘Chloroflexi’ isolated from an iron-rich coastal hydrothermal field, and description of Ardenticatenia classis nov. Int. J. Syst. Evol. Microbiol. 63, 2992–3002. doi: 10.1099/ijs.0.046532-0
Kawaichi, S., Ito, N., Yoshida, T., and Sako, Y. (2013b). Bacterial and Archaeal Diversity in an Iron-Rich Coastal Hydrothermal Field in Yamagawa, Kagoshima, Japan. Microbes Environ. 28, 405–413. doi: 10.1264/jsme2.me13048
Kerrick, D. M., Mckibben, M. A., Seward, T. M., and Caldeira, K. (1995). Convective hydrothermal C02 emission from high heat flow regions. Chem. Geol. 121, 285–293.
Kharlamenko, V. I., Imbs, A. B., and Tarasov, V. G. (2009). Physiological state of microbial communities and mats of the bottom sediments in the marine shallow-water hydrothermal ecosystem of Kraternaya Bight (Kurile Islands). Russ. J. Mar. Biol. 35, 323–330.
Khimasia, A., Renshaw, C. E., Price, R. E., and Pichler, T. (2021). Hydrothermal flux and porewater geochemistry in Paleochori Bay, Milos, Greece. Chem. Geol. 571:120188.
Khimasia, A., Rovere, A., and Pichler, T. (2020). Hydrothermal areas, microbial mats and sea grass in Paleochori Bay, Milos, Greece. J. Maps 16, 348–356.
Kleint, C., Kuzmanovski, S., Powell, Z., Bühring, S. I., Sander, S. G., and Koschinsky, A. (2015). Organic Cu-complexation at the shallow marine hydrothermal vent fields off the coast of Milos (Greece), Dominica (Lesser Antilles) and the Bay of Plenty (New Zealand). Mar. Chem. 173, 244–252.
Kleint, C., Pichler, T., and Koschinsky, A. (2017). Geochemical characteristics, speciation and size-fractionation of iron (Fe) in two marine shallow-water hydrothermal systems, Dominica, Lesser Antilles. Chem. Geol. 454, 44–53.
Klevenz, V., Sander, S. G., Perner, M., and Koschinsky, A. (2012). Amelioration of free copper by hydrothermal vent microbes as a response to high copper concentrations. Chem. Ecol. 28, 405–420.
Kristjansson, J. K., Hreggvidsson, G. O., and Alfredsson, G. A. (1986). Isolation of Halotolerant Thermus spp. from Submarine Hot Springs in Iceland. Appl. Environ. Microbiol. 52, 1313–1316. doi: 10.1128/aem.52.6.1313-1316.1986
Kyriaki, D., Lisa, G. A., Sergio, C., Manfredi, L., Konstantinos, H., Konstantinos, K., et al. (2018). Gas geochemistry and CO2 output estimation at the island of Milos, Greece. J. Volcanol. Geotherm. Res. 365, 13–22.
Lackschewitz, K. S., Botz, R., Garbe-Schönberg, D., Scholten, J., and Stoffers, P. (2006). Mineralogy and geochemistry of clay samples from active hydrothermal vents off the north coast of Iceland. Mar. Geol. 225, 177–190.
Lang, S. Q., Butterfield, D. A., Lilley, M. D., Paul Johnson, H., and Hedges, J. I. (2006). Dissolved organic carbon in ridge-axis and ridge-flank hydrothermal systems. Geochim. Cosmochim. Acta 70, 3830–3842.
Le Bris, N., Sarradin, P.-M., and Pennec, S. (2001). A new deep-sea probe for in situ pH measurement in the environment of hydrothermal vent biological communities. Deep Sea Res. I Oceanogr. Res. Pap. 48, 1941–1951.
Li, Y., Tang, K., Zhang, L., Zhao, Z., Xie, X., Chen, C.-T. A., et al. (2018). Coupled carbon, sulfur, and nitrogen cycles mediated by microorganisms in the water column of a shallow-water hydrothermal ecosystem. Front. Microbiol. 9:2718. doi: 10.3389/fmicb.2018.02718
Lin, Y. S., Lee, J., Lin, L. H., Fu, K. H., Chen, C. T. A., Wang, Y. H., et al. (2020). Biogeochemistry and dynamics of particulate organic matter in a shallow-water hydrothermal field (Kueishantao Islet, NE Taiwan). Mar. Geol. 422:106121.
Lin, Y.-S., Lui, H.-K., Lee, J., Chen, C.-T. A., Burr, G. S., Chou, W.-C., et al. (2019). Fates of vent CO2 and its impact on carbonate chemistry in the shallow-water hydrothermal field offshore Kueishantao Islet, NE Taiwan. Mar. Chem. 210, 1–12.
Lu, G.-S., Larowe, D. E., Fike, D. A., Druschel, G. K., Gilhooly, W. P. III, Price, R. E., et al. (2020). Bioenergetic characterization of a shallow-sea hydrothermal vent system: Milos Island, Greece. PLoS One 15:e0234175. doi: 10.1371/journal.pone.0234175
Mancinella, D., Mantelo, D., and M, T. (2020). Emissioni gassose sottomarine nel tratto costiero prospicente Tor Caldara (Lazio meridionale, Italia). Mem. Descr. Carta Geol. D’It. 105, 29–33.
Manini, E., Luna, G. M., Corinaldesi, C., Zeppilli, D., Bortoluzzi, G., Caramanna, G., et al. (2008). Prokaryote diversity and virus abundance in shallow hydrothermal vents of the Mediterranean sea (Panarea island) and the pacific ocean (North Sulawesi-Indonesia). Microb. Ecol. 55, 626–639. doi: 10.1007/s00248-007-9306-2
Mantha, G., Awasthi, A. K., Al-Aidaroos, A. M., and Hwang, J. S. (2013). Diversity and abnormalities of cyclopoid copepods around hydrothermal vent fluids, Kueishantao Island, north-eastern Taiwan. J. Nat. History 47, 685–697.
Marteinsson, V. T., Kristjánsson, J. K., Kristmannsdóttir, H., Dahlkvist, M., Saemundsson, K., Hannington, M., et al. (2001). Discovery and Description of Giant Submarine Smectite Cones on the Seafloor in Eyjafjordur, Northern Iceland, and a Novel Thermal Microbial Habitat. Appl. Environ. Microbiol. 67, 827–833. doi: 10.1128/AEM.67.2.827-833.2001
McCarthy, K. T. (2004). A Geochemical and Spatial Characterization of the Champagne Hot Springs Shallow Hydrothermal Vent Field, Dominica, Lesser Antilles. Master’s thesis. Tampa, FL: University of South Florida.
McCarthy, K. T., Pichler, T., and Price, R. E. (2005). Geochemistry of Champagne Hot Springs shallow hydrothermal vent field and associated sediments, Dominica, Lesser Antilles. Chem. Geol. 224, 55–68.
McInnes, B. I. A., and Cameron, E. M. (1994). Carbonated, alkaline hybridizing melts from a sub-arc environment: mantle wedge samples from the Tabar-Lihir-Tanga-Feni arc, Papua New Guinea. Earth Planet. Sci. Lett. 122, 125–141.
Megalovasilis, P. (2014). Partition geochemistry of hydrothermal precipitates from submarine hydrothermal fields in the Hellenic Volcanic Island Arc. Geochem. Int. 52, 992–1010.
Megalovasilis, P. (2020). Geochemistry of Hydrothermal Particles in Shallow Submarine Hydrothermal Vents on Milos Island, Aegean Sea East Mediterranean. Geochem. Int. 58, 151–181.
Meyer-Dombard, D. A. R., Amend, J. P., and Osburn, M. R. (2013). Microbial diversity and potential for arsenic and iron biogeochemical cycling at an arsenic rich, shallow-sea hydrothermal vent (Tutum Bay, Papua New Guinea). Chem. Geol. 348, 37–47.
Meyer-Dombard, D. R., Price, R. E., Pichler, T., and Amend, J. P. (2011). Prokaryotic Populations in Arsenic-Rich Shallow-Sea Hydrothermal Sediments of Ambitle Island, Papua New Guinea. Geomicrobiol. J. 29, 1–17.
Milia, A., Mirabile, L., Torrente, M. M., and Dvorak, J. J. (1998). Volcanism offshore of Vesuvius Volcano in Naples Bay. Bull. Volcanol. 59, 404–413.
Miroshnichenko, M. L., Bonch-Osmolovskaya, E. A., Neuner, A., Kostrikina, N. A., Chernych, N. A., and Alekseev, V. A. (1989). Thermococcus stetteri sp. nov., a New Extremely Thermophilic Marine Sulfur-Metabolizing Archaebacterium. Syst. Appl. Microbiol. 12, 257–262.
Mohandass, C., Rajasabapathy, R., Ravindran, C., Colaco, A., Santos, R. S., and Meena, R. M. (2012). Bacterial diversity and their adaptations in the shallow water hydrothermal vent at D. Joao de Castro Seamount (DJCS), Azores, Portugal. Cah. Biol. Mar. 53, 65–76.
Nakagawa, S., Nakamura, S., Inagaki, F., Takai, K., Shirai, N., and Sako, Y. (2004). Hydrogenivirga caldilitoris gen. nov., sp. nov., a novel extremely thermophilic, hydrogen- and sulfur-oxidizing bacterium from a coastal hydrothermal field. Int. J. Syst. Evol. Microbiol. 54, 2079–2084. doi: 10.1099/ijs.0.03031-0
Nakatani, T., Ura, T., Ito, Y., Kojima, J., Tamura, K., Sakamaki, T., et al. (2008). “AUV “TUNA-SAND” and its Exploration of hydrothermal vents at Kagoshima Bay,” in Proceedings of the OCEANS 2008 - MTS/IEEE Kobe Techno-Ocean, Kobe, 1–5.
Nikiforov, S. M. (2001). Allozyme Variability of the Sea Urchin Strongylocentrotus droebachiensis in the Area of Volcanic Hydrotherms. Russ. J. Mar. Biol. 27, 383–391.
Nishiyama, M., Yamamoto, S., and Kurosawa, N. (2013). Microbial community analysis of a coastal hot spring in Kagoshima, Japan, using molecular- and culture-based approaches. J. Microbiol. 51, 413–422. doi: 10.1007/s12275-013-2419-z
Nomikou, P., Papanikolaou, D., Alexandri, M., Sakellariou, D., and Rousakis, G. (2013). Submarine volcanoes along the Aegean volcanic arc. Tectonophysics 597-598, 123–146.
Norris, P. R., Davis-Belmar, C. S., Calvo-Bado, L. A., and Ogden, T. J. (2020). Salt-tolerant Acidihalobacter and Acidithiobacillus species from Vulcano (Italy) and Milos (Greece). Extremophiles 24, 593–602. doi: 10.1007/s00792-020-01178-w
Núñez Cornú, F. J., Prol-Ledesma, R. M., Cupul Magaña, A., and Suárez Plascencia, C. (2000). Near shore submarine hydrothermal activity in Bahia Banderas, western Mexico. Geofísica Int. 39, 171–178.
Ohmoto, H., and Skinner, J. (1983). The Kuroko and Related Volcanogenic Massive Sulphide Deposits. New Haven, CT: Economic Geology Publishing.
Oki, K., and Hayasaka, S. (1978). Geological study on Kagoshima Bay, south Kyushu, Japan. Part IV – A note on the peculiar mode of occurrence of foraminifers in the bottom sediments of the bay-head area. Sci. Rep. 11, 1–11.
Orange, D., García-García, A., Lorenson, T., Nittrouer, C., Milligan, T., Miserocchi, S., et al. (2005). Shallow gas and flood deposition on the Po Delta. Mar. Geol. 22, 159–177.
Orlando, V., Franco, T., Dario, T., Robert, P. J., and Antonio, C. (2011). Submarine and Inland Gas Discharges from the Campi Flegrei (Southern Italy) and the Pozzuoli Bay: geochemical Clues for a Common Hydrothermal-Magmatic Source. Procedia Earth Planet. Sci. 4, 57–73.
Pantin, H. M., and Wright, I. C. (1994). Submarine hydrothermal activity within the offshore Taupo Volcanic Zone, Bay of Plenty continental shelf, New Zealand. Cont. Shelf Res. 14, 1411–1438.
Paoletti, V., Di Maio, R., Cella, F., Florio, G., Motschka, K., Roberti, N., et al. (2009). The Ischia volcanic island (Southern Italy): inferences from potential field data interpretation. J. Volcanol. Geotherm. Res. 179, 69–86.
Passaro, S., Genovese, S., Sacchi, M., Barra, M., Rumolo, P., Tamburrino, S., et al. (2014). First hydroacoustic evidence of marine, active fluid vents in the Naples Bay continental shelf (Southern Italy). J. Volcanol. Geotherm. Res. 285, 29–35.
Patwardhan, S., Foustoukos, D. I., Giovannelli, D., Yucel, M., and Vetriani, C. (2018). Ecological Succession of Sulfur-Oxidizing Epsilon- and Gammaproteobacteria During Colonization of a Shallow-Water Gas Vent. Front. Microbiol. 9:2970. doi: 10.3389/fmicb.2018.02970
Patwardhan, S., Smedile, F., Giovannelli, D., and Vetriani, C. (2021). Metaproteogenomic Profiling of Chemosynthetic Microbial Biofilms Reveals Metabolic Flexibility During Colonization of a Shallow-Water Gas Vent. Front. Microbiol. 12:638300. doi: 10.3389/fmicb.2021.638300
Peltier, A., Scott, B., and Hurst, T. (2009). Ground deformation patterns at White Island volcano (New Zealand) between 1967 and 2008 deduced from levelling data. J. Volcanol. Geotherm. Res. 181, 207–218.
Pérez-Rodríguez, I., Rawls, M., Coykendall, D. K., and Foustoukos, D. I. (2016). Deferrisoma palaeochoriense sp. nov., a thermophilic, iron(III)-reducing bacterium from a shallow-water hydrothermal vent in the Mediterranean Sea. Int. J. Syst. Evol. Microbiol. 66, 830–836. doi: 10.1099/ijsem.0.000798
Pichler, T., Amend, J. P., Garey, J., Hallock, P., Hsia, N. P., Karlen, D. J., et al. (2006). A natural laboratory to study arsenic geobiocomplexity. Eos Trans. Am. Geophys. Union 87, 221–225.
Pichler, T., and Veizer, J. (1999). Precipitation of Fe(III) oxyhydroxide deposits from shallow-water hydrothermal fluids in Tutum Bay, Ambitle Island, Papua New Guinea. Chem. Geol. 162, 15–31.
Pichler, T., and Veizer, J. (2004). The precipitation of aragonite from shallow-water hydrothermal fluids in a coral reef, Tutum Bay, Ambitle Island, Papua New Guinea. Chem. Geol. 207, 31–45.
Pichler, T., Biscere, T., Kinch, J., Zampighi, M., Houlbreque, F., and Rodolfo-Metalpa, R. (2019). Suitability of the shallow water hydrothermal system at Ambitle Island (Papua New Guinea) to study the effect of high pCO(2) on coral reefs. Mar. Pollut. Bull. 138, 148–158. doi: 10.1016/j.marpolbul.2018.11.003
Pichler, T., Veizer, J., and Hall, G. E. M. (1999). The chemical composition of shallow-water hydrothermal fluids in Tutum Bay, Ambitle Island, Papua New Guinea and their effect on ambient seawater. Mar. Chem. 64, 229–252.
Pop Ristova, P., Pichler, T., Friedrich, M. W., and Bühring, S. I. (2017). Bacterial Diversity and Biogeochemistry of Two Marine Shallow-Water Hydrothermal Systems off Dominica (Lesser Antilles). Front. Microbiol. 8:2400. doi: 10.3389/fmicb.2017.02400
Price, R. E., and Giovannelli, D. (2017). “A review of the geochemistry and microbiology of marine shallow-water hydrothermal vents,” in Reference Module in Earth Systems and Environmental Sciences, eds J. K.Cochran, H. J., Bokuniewicz, and P. L. Yager (Amsterdam: Elsevier), 1–29. doi: 10.1111/j.1365-2672.1998.tb05289.x
Price, R. E., and Pichler, T. (2005). Distribution, speciation and bioavailability of arsenic in a shallow-water submarine hydrothermal system, Tutum Bay, Ambitle Island, PNG. Chem. Geol. 224, 122–135.
Price, R. E., Breuer, C., Reeves, E., Bach, W., and Pichler, T. (2016). Arsenic bioaccumulation and biotransformation in deep-sea hydrothermal vent organisms from the PACMANUS hydrothermal field, Manus Basin, PNG. Deep Sea Res. I Oceanogr. Res. Pap. 117, 95–106.
Price, R. E., Larowe, D. E., Italiano, F., Savov, I., Pichler, T., and Amend, J. P. (2015). Subsurface hydrothermal processes and the bioenergetics of chemolithoautotrophy at the shallow-sea vents off Panarea Island (Italy). Chem. Geol. 407-408, 21–45.
Price, R. E., Lesniewski, R., Nitzsche, K. S., Meyerdierks, A., Saltikov, C., Pichler, T., et al. (2013a). Archaeal and bacterial diversity in an arsenic-rich shallow-sea hydrothermal system undergoing phase separation. Front. Microbiol. 4:158. doi: 10.3389/fmicb.2013.00158
Price, R. E., Savov, I., Planer-Friedrich, B., Bühring, S. I., Amend, J., and Pichler, T. (2013b). Processes influencing extreme As enrichment in shallow-sea hydrothermal fluids of Milos Island, Greece. Chem. Geol. 348, 15–26.
Prol-Ledesma, R., Canet, C., Armienta, M. A., and Solís, G. (2002). “Vent fluid in the Punta Mita coastal submarine hydrothermal system, Mexico,” in Proceedings of the 2002 GSA Annual Meeting, Las Vegas, NV.
Prol-Ledesma, R. M. (2003). Similarities in the chemistry of shallow submarine hydrothermal vents. Geothermics 32, 639–644.
Prol-Ledesma, R. M., Canet, C., Torres-Vera, M. A., Forrest, M. J., and Armienta, M. A. (2004). Vent fluid chemistry in Bahía Concepción coastal submarine hydrothermal system, Baja California Sur, Mexico. J. Volcanol. Geotherm. Res. 137, 311–328.
Quattrocchi, F., Cantucci, B., Cinti, D., Galli, G., Pizzino, L., Sciarra, A., et al. (2009). Continuous/discrete geochemical monitoring of CO2 natural analogues and of Diffuse Degassing Structures (DDS): hints for CO2 storage sites geochemical monitoring protocol. Energy Procedia 1, 2135–2142.
Raghukumar, C., Mohandass, C., Cardigos, F., D’costa, P. M., Santos, R. S., and Colaco, A. (2008). Assemblage of benthic diatoms and culturable heterotrophs in shallow-water hydrothermal vent of the D. Joao de Castro Seamount, Azores in the Atlantic Ocean. Curr. Sci. 95, 1715–1723.
Ragnarsson, A. (2000). “Geothermal development in Iceland 1995-1999,” in Proceedings of the World Geothermal Congress 2000, Tohoku.
Rajasabapathy, R., Mohandass, C., Bettencourt, R., Colaco, A., Goulart, J., and Meena, R. M. (2018). Bacterial diversity at a shallow-water hydrothermal vent (Espalamaca) in Azores Island. Curr. Sci. 115, 2110–2121.
Rajasabapathy, R., Mohandass, C., Colaco, A., Dastager, S. G., Santos, R. S., and Meena, R. M. (2014). Culturable bacterial phylogeny from a shallow water hydrothermal vent of Espalamaca (Faial, Azores) reveals a variety of novel taxa. Curr. Sci. 106, 58–69.
Ramasamy, K. P., Rajasabapathy, R., Lips, I., Mohandass, C., and James, R. A. (2020). Genomic features and copper biosorption potential of a new Alcanivorax sp. VBW004 isolated from the shallow hydrothermal vent (Azores, Portugal). Genomics 112, 3268–3273. doi: 10.1016/j.ygeno.2020.06.015
Rovere, M., Gamberi, F., Mercorella, A., Rashed, H., Gallerani, A., Leidi, E., et al. (2014). Venting and seepage systems associated with mud volcanoes and mud diapirs in the southern Tyrrhenian Sea. Mar. Geol. 347, 153–171.
Rusch, A., and Amend, J. P. (2008). Functional characterization of the microbial community in geothermally heated marine sediments. Microb. Ecol. 55, 723–736.
Sakamoto, H., Tomiyasu, T., and Yonehara, N. (1995). The contents and chemical forms of mercury in sediments from Kagoshima Bay, in comparison with Minamata Bay and Yatsushiro Sea, southwestern Japan. Geochem. J. 29, 97–105.
Sarano, F., Murphy, R. C., Houghton, B. F., and Hedenquist, J. W. (1989). Preliminary observations of submarine geothermal activity in the vicinity of White Island Volcano, Taupo Volcanic Zone, New Zealand. J. R. Soc. N. Z. 19, 449–459.
Savelli, C., Marani, M., and Gamberi, F. (1999). Geochemistry of metalliferous, hydrothermal deposits in the Aeolian arc (Tyrrhenian Sea). J. Volcanol. Geotherm. Res. 88, 305–323.
Scandone, R., Bellucci, F., Lirer, L., and Rolandi, G. (1991). The structure of the campanian plain and the activity of the neapolitan volcanos (Italy). J. Volcanol. Geotherm. Res. 48, 1–31.
Schroot, B. M., and Schüttenhelm, R. T. E. (2003). Shallow gas and gas seepage: expressions on seismic and otheracoustic data from the Netherlands North Sea. J. Geochem. Explor. 78-79, 305–309.
Schroot, B. M., Klaver, G. T., and Schüttenhelm, R. T. E. (2005). Surface and subsurface expressions of gas seepage to the seabed—examples from the Southern North Sea. Mar. Pet. Geol. 22, 499–515.
Sedwick, P., and Stuben, D. (1996). Chemistry of shallow submarine warm springs in an arc-volcanic setting: Vulcano Island, Aeolian Archipelago, Italy. Mar. Chem. 53, 147–161.
Seward, T. M., and Kerrick, D. M. (1996). Hydrothermal CO2 emission from the Taupo Volcanic Zone, New Zealand. Earth Planet. Sci. Lett. 139, 105–113.
Shen, Y., Solomon, S. C., Bjarnason, I. T., and Wolfe, C. J. (1998). Seismic evidence for a lower-mantle origin of the Iceland plume. Nature 395, 62–65. doi: 10.1038/35016054
Shitashima, K., Maeda, Y., and Ohsumi, T. (2013). Strategies for Detection and Monitoring of CO2 Leakage in Sub-seabed CCS. Energy Procedia 37, 4283–4290.
Shitashima, K., Maeda, Y., Koike, Y., and Ohsumi, T. (2008). Natural analogue of the rise and dissolution of liquid CO2 in the ocean. Int. J. Greenhouse Gas Control 2, 95–104.
Siebert, L., Simkin, T., and Kimberly, P. (2011). Volcanoes of the World, 3rd Edn. Berkeley, CA: University of California Press.
Sievert, S. M., and Kuever, J. (2000). Desulfacinum hydrothermale sp nov., a thermophilic, sulfate-reducing bacterium from geothermally heated sediments near Milos Island (Greece). Int. J. Syst. Evol. Microbiol. 50, 1239–1246. doi: 10.1099/00207713-50-3-1239
Sievert, S. M., Brinkhoff, T., Muyzer, G., Ziebis, V., and Kuever, J. (1999). Spatial heterogeneity of bacterial populations along an environmental gradient at a shallow submarine hydrothermal vent near Milos Island (Greece). Appl. Environ. Microbiol. 65, 3834–3842. doi: 10.1128/AEM.65.9.3834-3842.1999
Sievert, S. M., Kuever, J., and Muyzer, G. (2000a). Identification of 16S ribosomal DNA-defined bacterial populations at a shallow submarine hydrothermal vent near Milos Island (Greece). Appl. Environ. Microbiol. 66, 3102–3109. doi: 10.1128/AEM.66.7.3102-3109.2000
Sievert, S. M., Ziebis, W., Kuever, J., and Sahm, K. (2000b). Relative abundance of Archaea and Bacteria along a thermal gradient of a shallow-water hydrothermal vent quantified by rRNA slot-blot hybridization. Microbiology 146, 1287–1293. doi: 10.1099/00221287-146-6-1287
Simkin, T., Tilling, R. I., Taggert, J. N., Jones, W. J., and Spall, H. (1989). This Dynamic Planet: Map of Volcanoes, Earthquakes and Plate Tectonics. Reston, VA: US Geological Survey.
Simmons, S., and Norris, P. R. (2002). Acidophiles of saline water at thermal vents of Vulcano, Italy. Extremophiles 6, 201–207. doi: 10.1007/s007920100242
Sollich, M., Yoshinaga, M. Y., Häusler, S., Price, R. E., Hinrichs, K.-U., and Bühring, S. I. (2017). Heat stress dictates microbial lipid composition along a thermal gradient in marine sediments. Front. Microbiol. 8:1550. doi: 10.3389/fmicb.2017.01550
Sorokin, D. Y. (1991). Oxidation of reduced sulfur-compounds in volcanic active regions of Plenty Gulf (New-Zealand) and Matupy-Harbor (New Britain, Papua-New-Guinea). Izvestiya Akad. Nauk Ser. Biol. 376–387.
Stetter, K. O. (2006). History of discovery of the first hyperthermophiles. Extremophiles 10, 357–362.
Suleiman, M., Schroder, C., Klippel, B., Schafers, C., Kruger, A., and Antranikian, G. (2019). Extremely thermoactive archaeal endoglucanase from a shallow marine hydrothermal vent from Vulcano Island. Appl. Microbiol. Biotechnol. 103, 1267–1274. doi: 10.1007/s00253-018-9542-z
Takeuchi, M., Katayama, T., Yamagishi, T., Hanada, S., Tamaki, H., Kamagata, Y., et al. (2014). Methyloceanibacter caenitepidi gen. nov., sp. nov., a facultatively methylotrophic bacterium isolated from marine sediments near a hydrothermal vent. Int. J. Syst. Evol. Microbiol. 64, 462–468. doi: 10.1099/ijs.0.053397-0
Tanaka, R., Kawaichi, S., Nishimura, H., and Sako, Y. (2006). Thermaerobacter litoralis sp. nov., a strictly aerobic and thermophilic bacterium isolated from a coastal hydrothermal field. Int. J. Syst. Evol. Microbiol. 56, 1531–1534. doi: 10.1099/ijs.0.64203-0
Tang, K., Liu, K., Jiao, N., Zhang, Y., and Chen, C.-T. A. (2013). Functional Metagenomic Investigations of Microbial Communities in a Shallow-Sea Hydrothermal System. PLoS One 8:e72958. doi: 10.1371/journal.pone.0072958
Tang, K., Zhang, Y., Lin, D., Han, Y., Chen, C.-T. A., Wang, D., et al. (2018). Cultivation-Independent and Cultivation-Dependent Analysis of Microbes in the Shallow-Sea Hydrothermal System Off Kueishantao Island, Taiwan: unmasking Heterotrophic Bacterial Diversity and Functional Capacity. Front. Microbiol. 9:279. doi: 10.3389/fmicb.2018.00279
Taran, Y. A., Inguaggiato, S., Marin, M., and Yurova, L. M. (2002). Geochemistry of fluids from submarine hot springs at Punta de Mita, Nayarit, Mexico. J. Volcanol. Geotherm. Res. 115, 329–338.
Tarasov, V. G. (2006). Effects of shallow-water hydrothermal venting on biological communities of coastal marine ecosystems of the western Pacific. Adv. Mar. Biol. 50, 267–421. doi: 10.1016/S0065-2881(05)50004-X
Tarasov, V. G., and Zhirmunskaya, A. V. (1989). Investigation of the ecosystem of Kraternaya Bight (Kurile Islands). Biol. Morya 3, 4–12.
Tarasov, V. G., Gebruk, A. V., Shulkin, V. M., Kamenev, G. M., Fadeev, V. I., Kosmynin, V. N., et al. (1999). Effect of shallow-water hydrothermal venting on the biota of Matupi Harbour (Rabaul Caldera, New Britain Island, Papua New Guinea). Cont. Shelf Res. 19, 79–116.
Tassi, F., Capaccioni, B., Caramanna, G., Cinti, D., Montegrossi, G., Pizzino, L., et al. (2009). Low-pH waters discharging from submarine vents at Panarea Island (Aeolian Islands, southern Italy) after the 2002 gas blast: origin of hydrothermal fluids and implications for volcanic surveillance. Appl. Geochem. 24, 246–254.
Tedesco, D., Allard, P., Sano, Y., Wakita, H., and Pece, R. (1990). Helium-3 in subaerial and submarine fumaroles of Campi Flegrei caldera, Italy. Geochim. Cosmochim. Acta 54, 1105–1116.
Tomiyasu, T., Nakagawa, M., Kodamatani, H., and Kanzaki, R. (2021). The influence of submarine volcano on seasonal changes in arsenic in the waters of Kagoshima Bay, southwestern Japan. Environ. Earth Sci. 80:331.
Urabe, T., and Kusakabe, M. (1990). Barite silica chimneys from the Sumisu Rift, Izu-Bonin Arc: possible analog to hematitic chert associated with Kuroko deposits. Earth Planet. Sci. Lett. 100, 283–290.
Varnavas, S. P., and Cronan, D. S. (2005). Submarine hydrothermal activity off Santorini and Milos in the Central Hellenic Volcanic Arc: a synthesis. Chem. Geol. 224, 40–54.
Villanueva-Estrada, R. E., Prol-Ledesma, R. M., Rodríguez-Díaz, A. A., Canet, C., and Armienta, M. A. (2013). Arsenic in hot springs of Bahía Concepción, Baja California Peninsula, México. Chem. Geol. 348, 27–36.
Vizzini, S., Tomasello, A., Maida, G. D., Pirrotta, M., Mazzola, A., and Calvo, S. (2010). Effect of explosive shallow hydrothermal vents on δ13C and growth performance in the seagrass Posidonia oceanica. J. Ecol. 98, 1284–1291.
von Deimling, J. S., Greinert, J., Chapman, N. R., Rabbel, W., and Linke, P. (2010). Acoustic imaging of natural gas seepage in the North Sea: sensing bubbles controlled by variable currents. Limnol. Oceanogr. Methods 8, 155–171.
Waite, D. W., Vanwonterghem, I., Rinke, C., Parks, D. H., Zhang, Y., Takai, K., et al. (2017). Comparative Genomic Analysis of the Class Epsilonproteobacteria and Proposed Reclassification to Epsilonbacteraeota (phyl. nov.). Front. Microbiol. 8:682. doi: 10.3389/fmicb.2017.00682
Walker, B. D., Mccarthy, M. D., Fisher, A. T., and Guilderson, T. P. (2008). Dissolved inorganic carbon isotopic composition of low-temperature axial and ridge-flank hydrothermal fluids of the Juan de Fuca Ridge. Mar. Chem. 108, 123–136.
Wallace, D. A., Johnson, R. W., Chappell, B. W., Arculus, R. J., Perfit, M. R., and Crick, I. H. (1983). Cainozoic volcanism of the Tabar, Lihir, Tanga, and Feni Islands, Papua New Guinea: geology, whole-rock analyses, and rock-forming mineral compositions. Rep. Bur. Min. Resour. 243, 1–62.
Wang, L., Cheung, M. K., Kwan, H. S., Hwang, J.-S., and Wong, C. K. (2015). Microbial diversity in shallow-water hydrothermal sediments of Kueishan Island, Taiwan as revealed by pyrosequencing. J. Basic Microbiol. 55, 1308–1318. doi: 10.1002/jobm.201400811
Wang, L., Cheung, M. K., Liu, R., Wong, C. K., Kwan, H. S., and Hwang, J.-S. (2017). Diversity of Total Bacterial Communities and Chemoautotrophic Populations in Sulfur-Rich Sediments of Shallow-Water Hydrothermal Vents off Kueishan Island, Taiwan. Microb. Ecol. 73, 571–582. doi: 10.1007/s00248-016-0898-2
Wang, T.-W., Chan, T.-Y., and Chan, B. K. K. (2014). Trophic relationships of hydrothermal vent and non-vent communities in the upper sublittoral and upper bathyal zones off Kueishan Island, Taiwan: a combined morphological, gut content analysis and stable isotope approach. Mar. Biol. 161, 2447–2463.
Wenzhöfer, F., Holby, O., Glud, R. N., Nielsen, H. K., and Gundersen, J. K. (2000). In situ microsensor studies of a shallow water hydrothermal vent at Milos, Greece. Mar. Chem. 69, 43–54.
Werner, C., and Cardellini, C. (2006). Comparison of carbon dioxide emissions with fluid upflow, chemistry, and geologic structures at the Rotorua geothermal system, New Zealand. Geothermics 35, 221–238.
Wilson, L. (1993). Volcanos - a Planetary Perspective. Nature 364, 498–498. doi: 10.1089/ast.2019.2107
Yamamoto, H., Fujikura, K., Hiraishi, A., Kato, K., and Maki, Y. (2002). Phylogenetic characterization and biomass estimation of bacterial endosymbionts associated with invertebrates dwelling in chemosynthetic communities of hydrothermal vent and cold seep fields. Marine Ecol. Prog. Ser. 245, 61–67.
Yamanaka, T., Mizota, C., Murae, T., and Hashimoto, J. (1999). A currently forming petroleum associated with hydrothermal mineralization in a submarine caldera, Kagoshima Bay, Japan. Geochem. J. 33, 355–367.
Yang, L., Hong, H., Guo, W., Chen, C.-T. A., Pan, P.-I., and Feng, C.-C. (2012). Absorption and fluorescence of dissolved organic matter in submarine hydrothermal vents off NE Taiwan. Mar. Chem. 128-129, 64–71.
Yang, S.-H., Chiang, P.-W., Hsu, T.-C., Kao, S.-J., and Tang, S.-L. (2016). Bacterial Community Associated with Organs of Shallow Hydrothermal Vent Crab Xenograpsus testudinatus near Kuishan Island, Taiwan. PLoS One 11:e0150597. doi: 10.1371/journal.pone.0150597
Yokoyama, I., and Ohkawa, S. (1986). The subsurface structure of the AIRA caldera and its vicinity in Southern Kyushu, Japan. J. Volcanol. Geotherm. Res. 30, 253–282.
Yücel, M., Sievert, S. M., Vetriani, C., Foustoukos, D. I., Giovannelli, D., and Le Bris, N. (2013). Eco-geochemical dynamics of a shallow-water hydrothermal vent system at Milos Island, Aegean Sea (Eastern Mediterranean). Chem. Geol. 356, 11–20.
Zeng, Z., Chen, C.-T. A., Yin, X., Zhang, X., Wang, X., Zhang, G.-L., et al. (2011). Origin of native sulfur ball from the Kueishantao hydrothermal field offshore northeast Taiwan: evidence from trace and rare earth element composition. J. Asian Earth Sci. 40, 661–671.
Zeng, Z., Ma, Y., Wang, X., Chen, C.-T. A., Yin, X., Zhang, S., et al. (2018). Elemental compositions of crab and snail shells from the Kueishantao hydrothermal field in the southwestern Okinawa Trough. J. Mar. Syst. 90–101.
Zeng, Z., Wang, X., Chen, C.-T. A., Yin, X., Chen, S., Ma, Y., et al. (2013). Boron isotope compositions of fluids and plumes from the Kueishantao hydrothermal field off northeastern Taiwan: implications for fluid origin and hydrothermal processes. Mar. Chem. 157, 59–66.
Zhang, Y., Zhao, Z., Chen, C.-T. A., Tang, K., Su, J., and Jiao, N. (2012). Sulfur Metabolizing Microbes Dominate Microbial Communities in Andesite-Hosted Shallow-Sea Hydrothermal Systems. PLoS One 7:e44593. doi: 10.1371/journal.pone.0044593
Zhirmunsky, A. V., and Tarasov, V. G. (1990). Unusual marine ecosystem in the flooded crater of Ushisher volcano. Mar. Ecol. Prog. Ser. 65, 95–102.
Keywords: shallow-water fluid emissions, hydrothermal systems, hydrothermal geomicrobiology, hydrothermal geochemistry, geology of hydrothermal systems
Citation: Caramanna G, Sievert SM and Bühring SI (2021) Submarine Shallow-Water Fluid Emissions and Their Geomicrobiological Imprint: A Global Overview. Front. Mar. Sci. 8:727199. doi: 10.3389/fmars.2021.727199
Received: 18 June 2021; Accepted: 20 September 2021;
Published: 25 October 2021.
Edited by:
Stefano Aliani, National Research Council (CNR), ItalyReviewed by:
Pavlos Megalovasilis, University of Patras, GreeceDonato Giovannelli, University of Naples Federico II, Italy
Copyright © 2021 Caramanna, Sievert and Bühring. This is an open-access article distributed under the terms of the Creative Commons Attribution License (CC BY). The use, distribution or reproduction in other forums is permitted, provided the original author(s) and the copyright owner(s) are credited and that the original publication in this journal is cited, in accordance with accepted academic practice. No use, distribution or reproduction is permitted which does not comply with these terms.
*Correspondence: Solveig I. Bühring, c2J1ZWhyaW5nQG1hcnVtLmRl