- 1Key Laboratory of Tropical Marine Bio-resources and Ecology, South China Sea Institute of Oceanology, Chinese Academy of Sciences, Guangzhou, China
- 2University of Chinese Academy of Sciences, Beijing, China
- 3Southern Marine Science and Engineering Guangdong Laboratory, Guangzhou, China
- 4Innovation Academy of South China Sea Ecology and Environmental Engineering, Chinese Academy of Sciences, Guangzhou, China
Seagrass meadows provide important habitats and rich organic carbon sources for consumers at different trophic levels but are threatened by accelerating eutrophication in coastal waters. Nevertheless, at present, carbon transfer processes throughout the food web and trophic pathways in eutrophic seagrass meadows are still poorly known. To resolve this issue, carbon sources of different trophic communities in a eutrophic tropical seagrass meadow [Xincun (XC) bay, South China Sea] under eutrophication were examined in summer and winter using dual stable isotopes. The δ13C value of omnivores and carnivores overlapped more with that of herbivores and planktivores/filter feeders, which mainly overlapped with that of epiphytes in summer and macroalgae in winter. Meanwhile, epiphytes and macroalgae exhibited high biomass and corresponding highest contribution to herbivores, omnivores, and carnivores in summer and winter, respectively. These results suggest that the grazing food chain was the main trophic pathway in this eutrophic seagrass meadow, and that the transfer of carbon flow in the grazing food chain was mainly dominated by the proliferating epiphytes or macroalgae carbon. In contrast, the contribution of seagrass to detritivores in both seasons was higher than that of other food sources. Our findings suggest that in eutrophic tropical seagrass meadows, the proliferation of epiphytes or macroalgae induced by high nutrient loading, as well as their seasonal changes, has a greater impact on the transfer of carbon in the grazing food chain than that in the detritus food chain, and the seagrass fueled the food web mainly through the detritus food chain.
Introduction
Seagrass meadows are highly complex and productive marine ecological systems that provide numerous ecological services. They not only provide spawning grounds (Jiang et al., 2020), nursery regions (Madi Moussa et al., 2020), habitat, and predation refuge areas for abundant fish, crabs, and other invertebrates (Barry et al., 2021; Tew et al., 2021), but they also provide nutrients and energy sources for the food web of the ecosystem (Park et al., 2013). Seagrass is not the only carbon source of this food web, which may also include significant contributions from other carbon sources, such as macroalgae, epiphyte, phytoplankton, and sediment microphytobenthos (Ouisse et al., 2012). However, the fate of these organic carbon sources remains controversial.
Seagrass is generally considered to have poor nutrition and low palatability for herbivores (Vergés et al., 2011; Boudouresque et al., 2016). Therefore, it is generally considered to be rarely ingested by herbivores (Nakamoto et al., 2019). However, mild seasonal variations in tropical seagrass meadows generally allow seagrass to remain abundant throughout the year (Nakamoto et al., 2019), which may increase the likelihood and intensity of seagrass consumption by herbivores (Heck et al., 2020). For example, previous reports have suggested that living seagrass provided main carbon sources for numerous herbivores and invertebrates in non-eutrophic tropical seagrass meadows (Burkholder et al., 2012; Chiu et al., 2013; Villa et al., 2019).
However, coastal seagrass meadows are threatened by accelerating eutrophication due to rapid economic development (Jiménez-Ramos et al., 2019; Kermagoret et al., 2019; Li et al., 2019). A vast amount of nutrient loading may cause the proliferation of phytoplankton, epiphytes, and opportunistic algae, which results in shading seagrass, eventually leading to the migration of primary producer structures from seagrass to phytoplankton and opportunistic algae-dominated communities in seagrass meadows (France, 2015; Barnes, 2019; Robertson and Savage, 2020). These variations would undoubtedly affect the feeding choices of consumers and the transfer pathway of carbon flow throughout the food web of seagrass meadows, but little is known about them. Previous studies on seagrass meadows have explored the spatial effects of eutrophication on the structure of primary producers (Han et al., 2016), trophic relations (Baden et al., 2010; Schmidt et al., 2017), and food web structure (Tewfik et al., 2007; Coll et al., 2011) from a spatial perspective. However, seasonal changes may also influence the structure of primary producer communities (Riegl et al., 2005; Qiu et al., 2017), and there is still little evidence of how temporal changes affect carbon transfer processes and carbon flow pathways of the food web in tropic eutrophic seagrass meadows.
Xincun bay is a tropical, nearly closed bay located in the southeast of Hainan island, China, and has a typical seagrass meadow (Huang et al., 2019). This seagrass meadow distributes abundant and diverse seagrass throughout the year (Huang et al., 2006), and has a long history of fish cage culture, which results in substantial nutrient loading (Liu et al., 2017). Meanwhile, the abundance of macroalgae Ulva pertusa fluctuates seasonally, with the highest and lowest abundance during winter and summer, respectively (Xu et al., 2009; Huang et al., 2019). Therefore, this study provides us with a natural experimental site to explore the key carbon transfer processes of the food web and trophic pathways in eutrophic tropical seagrass meadows.
In this study, we collected the main organic carbon sources, namely, seagrass, epiphytes, macroalgae, particulate organic matter (POM; mainly including phytoplankton) and sediment organic matter (SOM), and consumers in the summer and winter of the XC seagrass meadow to (1) explore the main carbon flow pathway of the food web in the eutrophic seagrass meadows, (2) study the influencing factors of carbon transfer in grazing and detritus food chain, and (3) assess the trophic role of seagrass in the food web of eutrophic seagrass meadows.
Materials and Methods
Study Area
This study was carried out in a tropical mixing seagrass meadow in XC (southeastern Hainan island, South China Sea, Figure 1), with Enhalus acoroides and Thalassia hemprichii as the dominant seagrass species (Huang et al., 2019). Seagrass mainly grows in a sandy substrate with an average water depth of <1.5 m from the southeast to the southwest of the bay (Huang et al., 2006). The total seagrass area is 175 ha (Huang et al., 2019). A vast amount of seagrass (E. acoroides and T. hemprichii) leaf detritus accumulated in the southern shallow waters of this bay.
Sample Collection, Processing, and Analysis
Samples were collected from four stations during low tide (Figure 1) in summer (August 2018) and winter (January 2019). One to three samples of each item were collected from each station. The POM samples were collected through filtering surface seawater samples onto pre-combusted Whatman GF/F filters (0.7 μm pore size, heated for 3 h at 450°C). The seawater nutrient samples were collected from previously filtered seawater and stored in bottles. The SOM was sampled by scraping the upper 1 cm layer using a spade. The samples of seagrass (E. acoroides and T. hemprichii) and macroalgae (Ulva pertusa) were collected and washed by hand. Meanwhile, in order to analyze the biomass of macroalgae, a 0.25-m2quadrat was used when the macroalgae samples were collected. The epiphyte samples were scraped from the surface of fresh seagrass leaves that had been measured in the area. The fish and crustacean samples were collected with a combination of trammel and fishing trap nets, while the other invertebrates were collected using a mesh screen (0.5 mm mesh). All the samples were stored at −20°C immediately after collection.
In the laboratory, the filters (POM samples) and epiphytes were acidified with 1 N HCl for 24 h to remove inorganic carbonates. The SOM samples were also acidified (1 N HCl) until the bubbling stopped to remove carbonates. All the acidified samples were washed with distilled water. The washed samples and seagrass, and macroalgae samples were dried at 60°C for 48 h. After weighing, the biomass of epiphytes and macroalgae was calculated and represented as the dry weight per square meter (g DW/m2). The dried samples, namely, seagrass, macroalgae, epiphyte, and SOM, were ground into a fine powder using a mortar and pestle. Muscle tissues were taken from the consumer samples and stored at −20°C after identification, weighing, and measurement. The frozen muscle sample was also ground into a fine powder using mortar and pestle after being dried. The POM and powdered samples were stored in a desiccator before analysis.
The dissolved inorganic nitrogen (DIN = ammonium + nitrate + nitrite) and dissolved inorganic phosphate (DIP) were measured following the methods developed by Grasshoff (2009) using a CANY 722s spectrophotometer. Stable isotope compositions of all the samples were analyzed using a continuous-flow isotope-ratio mass spectrometer (Delta V Advantage, Thermo Fisher Scientific, Waltham, MA, United States). Stable isotope ratios were calculated using the following formula:
where δ is the deviation from the standard reference material (Vienna Pee Dee Belemnite and atmospheric N2) in parts per mil (%), and R represents the ratio of 13C/12C or 15N/14N. In sample analysis, protein B2115-114859 (Elemental Microanalysis Ltd.) (δ13C = −26.98%, δ15N = 5.94%) was used as a working standard with an analytical uncertainty of <0.15% for stable isotope values.
Data Analysis
There remains a controversy about classifying consumers into the specific trophic group since the same consumer may have different dietary characteristics in different geographical environments. However, a discriminant function analysis (DFA) has been successfully conducted to classify samples into groups (Jepsen and Winemiller, 2002; Burress et al., 2013; Yoon et al., 2015). δ13C and δ15N values were used as variables to examine whether samples could be classified into predefined trophic groups. To determine the main carbon flow pathway in the seagrass meadow and the role of seagrass in the food web, we predefined trophic groups, based on the available literature, into herbivore, detritivore, planktivore/filter feeder, omnivore, and carnivore (Supplementary Table 1).
Additionally, Student's t-test was performed to test the seasonal differences in seawater nutrient, macroalgae, and epiphyte biomass, and the isotope ratios of food sources and consumers. Before this test, normality and homogeneity of the data were examined by the Shapiro–Wilk test and Levene's test, respectively. The non-parametric two-sample Mann–Whitney U-test was performed when the t-test assumptions failed. All the statistical analyses were performed using the IBM SPSS statistics 26 software, and a p < 0.05 indicated a statistically significant difference.
The contribution rate of potential food sources to consumers was estimated using the Bayesian model in the SIMMR package (Parnell and Inger, 2019), which was based on the SIAR package (Parnell et al., 2010), and implemented in the R 3.6.0 software (R Core Team, 2019). In order to reduce the number of food sources in the calculations, E. acoroides and T. hemprichii, and their epiphytes were considered as a single group called seagrass and epiphyte, respectively. Meanwhile, POM and SOM were not considered as potential food sources of herbivores based on the published literature (Du et al., 2019; Lin et al., 2021). In addition, considering that SOM is a mixture of other primary food sources (i.e., seagrass, epiphytes, macroalgae, and POM) (Carlier et al., 2009; Xu et al., 2018), we excluded it from the mixing model. Therefore, for herbivores, the potential food sources used in the SIMMR mixing model only included seagrass, epiphyte, and macroalgae. For the other trophic groups (i.e., planktivores, detritivores, omnivores, and carnivores), the potential food sources included seagrass, epiphytes, macroalgae, and POM. Except for the mean isotopic ratios (and SE) of each food source and the isotopic ratios of consumers, the SIMMR mixing model still required trophic enrichment factors (TEFs). Considering there are different trophic groups (see Section Data Analysis), we, therefore, used the widely accepted TEFs (Table 1) to estimate the direct contribution of food sources to herbivores, planktivores, detritivores, and omnivores, and the indirect contribution of food sources to carnivores. The SIMMR mixing model was not used to compute the contribution of low trophic level fauna (i.e., polychaetes, small crustaceans, and bivalves) to high trophic level consumers (i.e., omnivores and carnivores) because, in some cases, similar potential prey for high trophic level consumers had different isotope compositions. Therefore, such contributions were qualitatively determined by evaluating the isotope similarity between them and considering the TEFs (Abrantes and Sheaves, 2009). The outputs of the contribution rates in this model were expressed as 2., 25, 50, 75, and 97.5% credible interval (CI).

Table 1. Mean ± standard deviation (SD) of trophic enrichment factors (TEFs) used to calculate the contribution of potential food sources to the different trophic groups.
Results
Seawater Nutrients and Biomass of Macroalgae and Epiphytes
The concentrations of DIN and DIP in the seawater of XC bay ranged from 5.5 to 22 and 0.2 to 2.9 μM, respectively. The average concentrations of DIN and DIP were 9 and 5 μM in summer, and 16 and 1.6 μM in winter, respectively. A statistical analysis displayed significant differences in DIN and DIP between the seasons (p < 0.05), with higher concentrations in winter (Figure 2). Similarly, significant differences in the biomass of macroalgae and epiphytes were also observed between the seasons, with markedly higher and lower values in winter for macroalgae and epiphytes, respectively.
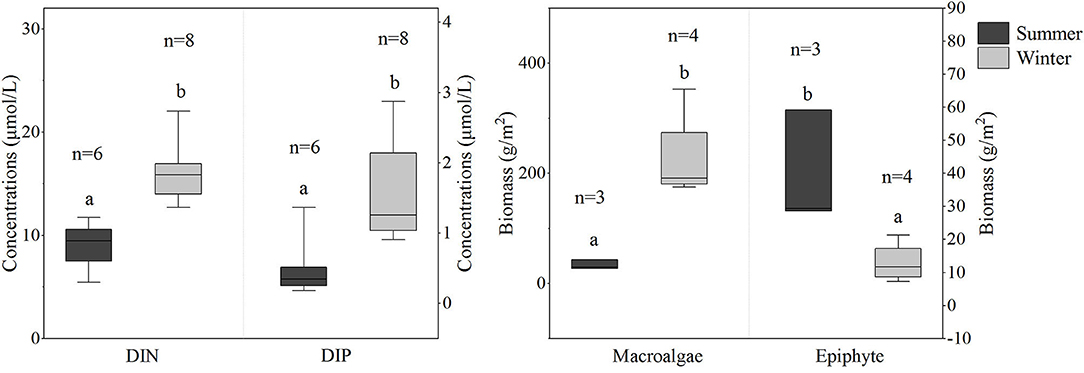
Figure 2. Concentrations of dissolved inorganic nitrogen (DIN) and dissolved inorganic phosphate (DIP), and biomass of macroalgae and epiphytes in summer and winter in Xincun bay meadow. Values are mean ± standard error (SE). The lower case letters (a and b) over the bars indicate significant differences between seasons (p < 0.05). The lower case letter (n) represents sample size. The detailed statistical analysis results are shown in Supplementary Tables 2,3.
Stable Isotope Composite of Food Sources
The δ13C values of each food source were similar between the two seasons, particularly for seagrass, epiphytes, and SOM, with annual average values of −9.7, −13.5, and −14.8%, respectively (Table 2). Significant differences were found in the δ13C values of macroalgae and POM between the two seasons, which both had higher values in summer. Among all the food sources, POM showed the lowest δ13C values in both summer (mean = −16%) and winter (mean = −19%), while the highest δ13C values were from macroalgae (mean = −7.3%) and seagrass (mean = −10.1%) in summer and winter, respectively.
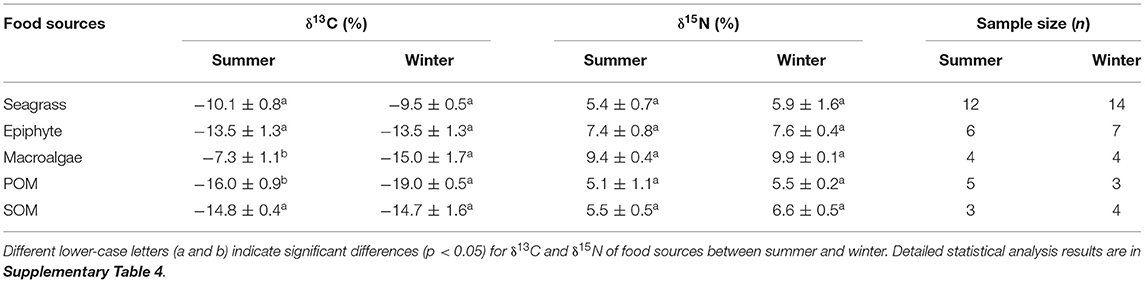
Table 2. The δ13C, δ15N values and sample size (mean ± SD) of food sources in summer and winter in Xincun bay.
The δ15N values of seagrass, epiphyte, macroalgae, POM, and SOM were also similar between summer and winter, with the corresponding annual average values of 5.6, 7.5, 9.6, 5.3, and 6.1%, respectively. In both seasons, POM and macroalgae had the minimum and maximum δ15N values, respectively (Table 2).
Isotope Values of Trophic Groups
A total of 74 and 78 species of consumers were collected in summer and winter, respectively (Figure 3). Based on the isotope ratios of the consumers, the DFA revealed that 93.8 and 87.5% of consumers could be successfully classified into the predefined trophic groups in summer and winter, respectively.
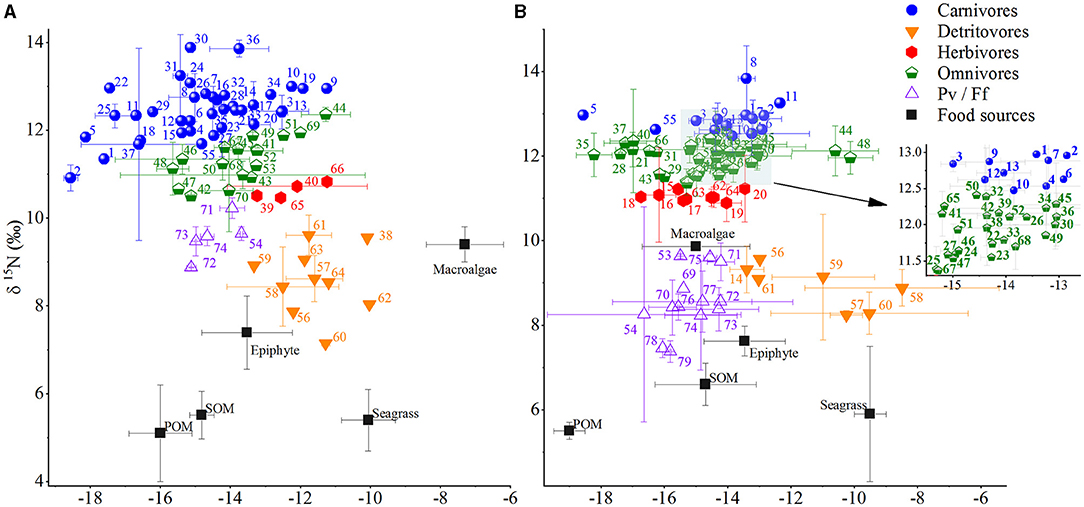
Figure 3. Plots of δ13C and δ15N values (mean ± SE) for consumers and food sources in (A) summer and (B) winter in Xincun bay. Sample sizes of consumers and food sources were 74 and 5 in summer, and 79 and 5 in winter, respectively. Pv represents planktivores, and Ff represents filter feeders. Legends for food sources and consumers are given in Table 2 and Supplementary Table 5, respectively.
The δ13C values of consumers showed a significant difference between seasons (U = 2,563.5, p = 0.01), with values ranging from −18.6 ± 0.2 (mean ± SE for carnivorous fish Abudefduf vaigiensis) to −10% (detritivorous gastropod Notosinistersubaura) in summer and from −18.6 (carnivorous fish Glossogobius aureus) to −8.5 ± 3.1% (mean ± SE for detritivorous echinoderm Archaster typicus) in winter, respectively. Among the trophic groups, planktivores/filter feeders displayed the smallest δ13C ranges in both summer (1.4%) and winter (1.4%), while the maximum δ13C ranges were displayed by carnivores (8.5%) and herbivores (82%) in summer and winter, respectively. Meanwhile, the average δ13C values of omnivores (t50 = 2.577, p = 0.013) and herbivores (U = 15, p = 0.019) varied significantly between seasons (Figure 3), with the values (mean ± SE, hereinafter the same) being −13.7 ± 1.2 and −12 ± 0.9%, respectively, in summer, and −14.8 ± 1.5 and −14.3 ± 2.2%, respectively, in winter.
Similar δ15N range of consumers was identified in both seasons, with values ranging from 7.1 for detritivorous echinoderm Hemicentrotus pulcherrimus to 13.9 ± 0.1% for carnivorous fish Scomberoides lysan in summer and from 7.4 ± 0.3 for planktivorous bivalve Trachycardium sp. to 13.8 ± 0.8% for carnivorous fish Lutjanus fulviflamma in winter, respectively. Among the trophic groups, the δ15N values of herbivores, detritivores, omnivores, and carnivores were all higher in winter than in summer, but they were inverse for planktivores/filter feeders. Meanwhile, the δ15N values of planktivores/filter feeders, omnivores, and carnivores varied significantly between seasons (Supplementary Table 6), with average values of 9.6, 11.3, and 12.5% in summer, and 8.5, 11.9, and 12.9% in winter, respectively.
Contribution of Food Sources to the Trophic Groups
The results of SIMMR mixing model suggested a clear seasonal distinction in the contribution of food sources to most consumers (Figure 4, Supplementary Table 7, Figure 1), especially for the contribution of food sources to omnivores and carnivores. In summer, epiphytes exhibited higher contribution to omnivores (83, 95% CI: 64–95%, Supplementary Figure 1a) and carnivores (85, 95% CI: 73–95%, Supplementary Figure 1b) compared with other food sources, while in winter, macroalgae contributed more to omnivores (55%, 95% CI: 46–62%, Supplementary Figure 1c) and carnivores (50%, 95% CI: 37–63%, Supplementary Figure 1d). On the contrary, the contribution of food sources to detritivores showed clear seasonal stability. In both seasons, seagrass contributed most to detritivores (mean > 53%, Supplementary Figures 1e,f).
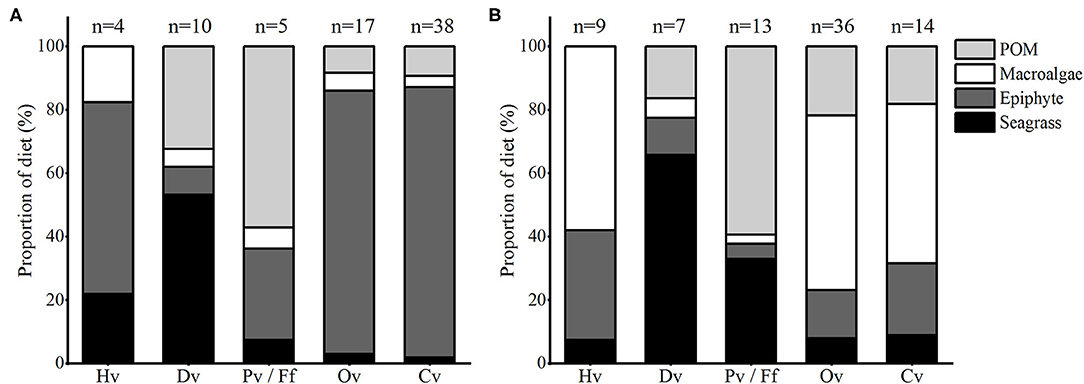
Figure 4. Stacked bar plots showing the mean proportion of the diet contribution of food sources to each trophic group in (A) summer and (B) winter in Xincun seagrass meadow. The lower case letter (n) represents statistical number of each trophic group. Hv, Dv, Pv, Ff, Ov, and Cv represent herbivores, detritivores, planktivores, filter feeders, omnivores and carnivores, respectively. The detailed summary of Bayesian mixing model (SIMMR) outputs and matrix plots of source contribution proportion are given in Supplementary Table 7, Figure 1, respectively.
For herbivores, large uncertainties seemed to hamper accurate quantifications. While in summer, the posterior probability distribution for epiphytes shifted strongly toward higher values (Supplementary Figure 1g), and the contribution proportion of epiphytes (61, 51–73%) was significantly higher than that of other food sources within the CI of 50% (Supplementary Table 7). On the contrary, in winter, the contribution of macroalgae (58, 51–65%) was significantly higher than that of epiphytes (35, 27–43%) and seagrass (8, 4–10%) within the CI of 50%. For planktivores, in summer, the contribution proportions of seagrass (8%, 95% CI: 1–19%) and macroalgae (7%, 95% CI: 1–16%) were the lowest, although the contribution range of epiphytes (95% CI: 6–53%) and POM (95% CI: 36–77%) overlapped a lot, the posterior probability distribution for POM shifted strongly toward higher values, and the contribution of POM was significantly higher than that of epiphytes within the CI of 50% (Supplementary Table 7). However, in winter, the contribution of POM was significantly higher than that of the other food sources (Supplementary Table 7).
Discussion
Isotope Ratios Characteristics of Food Sources
The δ13C and δ15N values of seagrass, epiphytes, and SOM fell within the range of previously reported values of the seagrass meadow distributed in the Indo-Pacific region (Du et al., 2016) and this study area (Fan et al., 2011; Liu et al., 2017). Higher δ15N values were observed for the five food sources in winter than in summer, which might be attributed to anthropogenic nitrogen sources (Arbi et al., 2018; Becherucci et al., 2019). In this study, this was evidenced by significantly high seawater nutrient concentration in winter. In contrast, a significant seasonal difference in δ13C values was only observed in POM and macroalgae, with higher values in summer. Similarly, substantial temporal variation in the stable isotopes of POM and macroalgae was also found in Zostera marina and Cymodocea nodosa seagrass meadows (Vizzini and Mazzola, 2003; Mittermayr et al., 2014), respectively. Such seasonal variations may be attributed to environmental factors and/or biotic physiological processes (Mittermayr et al., 2014; Jankowska et al., 2019; Nakamoto et al., 2019).
The existing literature shows that the decomposition of macroalgae is one of the main reasons for the increase in their δ13C values (Corbisier et al., 2004). Although high temperatures in summer usually result in the death and decomposition of macroalgae (Guo et al., 2006), this might not be the main reason for the extremely high isotope values of macroalgae in summer, since macroalgae decomposition usually causes small δ13C variations (within 1%) (Raven et al., 2002). Wang and Yeh (2003) suggested that organisms with δ13C values >-10% must use bicarbonate (HC). In this site, the pH in summer was usually higher than that in winter (http://hnsthb.hainan.gov.cn), indicating that CO2 in the seawater might be limited in summer. A previous study has proved that macroalgae are capable of both C3 and C4 photosynthesis and can take up HC, which has higher δ13C values than CO2 (Wang and Yeh, 2003; Xu et al., 2012; Valiela et al., 2018). The limited CO2 concentration resulted in higher HC absorption, which might cause macroalgae to have higher δ13C values in summer. In addition, higher irradiance and growth rate can also affect the isotope composition of primary producers. It has been reported that macroalgae have higher δ13C values with increased photon fluence rates and growth rate, where photon fluence rate acts as a major factor between the two, and 10 to 25% 13C can accumulate in macroalgae (Wiencke and Fischer, 1990; Wang and Yeh, 2003). Therefore, the higher δ13C values of macroalgae in summer might result from mixed factors, but this requires further research.
In contrast, δ13C variations in POM are often attributed to differences in growth rate (Gu et al., 2006) and species composition (Sun et al., 2012). Previous studies have shown that a high growth rate of phytoplankton could lead to strong 13C enrichment (Gu et al., 2006), and that there was no obvious change in the composition of species in this site (Wei et al., 2014). Therefore, the significantly high δ13C of POM in summer may be mainly due to its high growth rate. This was further proved by the high summer biomass of POM in this site (Wei et al., 2014). In addition, decomposed macroalgae were observed in this site in summer, which might also result in higher δ13C values for POM (Malet et al., 2008; Schaal et al., 2010). It is interesting that the δ13C values for POM in this study were close to the values previously measured in the same site (Fan et al., 2011) and around the mid south coast of Korea (Kang et al., 2009), but higher than values dominated by marine phytoplankton (Schaal et al., 2010; Du et al., 2019). Such higher δ13C values for POM may be attributed to the specific taxonomic composition of phytoplankton in this site. The δ13C values of the diatom-dominated coarse POM were generally higher than those of the nanoplankton-dominated POM (e.g., nano-flagellates) (Kang et al., 2009). A previous study has proved that diatoms are the dominant species of phytoplankton in present study area (Wei et al., 2014). Therefore, the high contribution of diatoms to the phytoplankton biomass might be the main reason for the higher δ13C values of POM in this site.
Key Carbon Transfer Processes and Major Trophic Pathway in the Seagrass Meadow
This study showed that herbivores have a wide range of δ13C values, which overlaps rarely with that of seagrass, but wider than that of epiphytes in summer and macroalgae in winter. This indicates that macroalgae or epiphytes would be preferentially fed upon. A previous study has suggested that herbivores prefer to consume high-protein primary producers with lower C/N ratios (Macreadie et al., 2017). Enhalus acoroides and T. hemprichii, which are dominated in the investigated area, have richer lignocellulose tissue and lower nitrogen content than macroalgae and epiphytes, making them less nutritious and energetically favorable for consumption by herbivores (Enríquez et al., 1993; Macreadie et al., 2017). Therefore, the seagrass meadow in XC bay might mainly provide a habitat and shelter for herbivores, while the food was mainly supplied by macroalgae and epiphytes. Meanwhile, a large number of epiphytes consumed by herbivores may also cause seagrass leaves to fall on the sediment floor, thus indirectly providing seagrass carbon sources for detritivores and decomposers (Hily et al., 2004; Ouisse et al., 2012).
The δ13C values of detritivores in the XC meadow were close to those of epiphytes and seagrass. However, the more depleted δ15N values in detritivores indicated that seagrass was their main food source, since the enrichment factors of detritivores that were the main benthic invertebrates in our study ranged from 2.7‰ to 3.6‰ (average 3.2‰) (Zanden and Rasmussen, 2001; Parker et al., 2008; Caut et al., 2009; Taylor et al., 2017). Detritivores are known to feed on decomposed plant materials rather than living plants (Platell et al., 2009; Lebreton et al., 2012; Ouisse et al., 2012), and previous studies in this study area (Fan et al., 2011) and other temperate seagrass meadows (Mascart et al., 2018) have shown that there was no obvious difference in the isotope composition between living seagrass leaves and its detritus. Therefore, seagrass carbon in the study area probably passes into the food web mainly through the detritus pathway. Similarly, seagrass was rarely consumed directly by herbivores and was passed into the food web more through detritivores feeding on dead seagrass laves, which was also found in temperate seagrass meadows (Boudouresque et al., 2016; François et al., 2018).
In addition, previous studies have shown that seagrass could also provide food sources for planktivores/filter feeders through small seagrass detrital particles (POM and SOM) formed by microbial degradation (Thresher et al., 1992; Vizzini et al., 2002). In this study, the food sources of planktivores/filter feeders were indeed POM and SOM, as evidenced by the more depleted δ13C values for planktivores/filter feeders, POM, and SOM. However, the main component of POM was phytoplankton (see Isotope Ratios Characteristics of Food Sources), and macroalgae and epiphytes had more contribution than seagrass to SOM in this site (Liu et al., 2016a). Therefore, the carbon sources of planktivores/filter feeders might originate more from phytoplankton, macroalgae, and epiphytes. Seagrass might play a greater role in the collection of food for filter feeders and planktivores by decreasing water flow to enhance the sedimentation of suspended particulate matter (Larkum et al., 2006) and capturing particulates on its leaves by the seagrass canopy and plants (Kennedy et al., 2004), respectively.
The δ13C values of predators (omnivores and carnivores) exhibited a characteristic of overlapping more with those of epiphytes in summer, and macroalgae and epiphytes in winter. This indicated that the main trophic base of high trophic level consumers originated from macroalgae or epiphytes. This result is consistent with other studies on eutrophic temperate seagrass meadows with abundant macroalgae or epiphytes (Hyndes and Lavery, 2005). However, they are different from previous studies on non-eutrophic tropical seagrass meadows, where most high trophic level consumers were supported mainly by carbon that originated from seagrass and epiphytes (Connolly and Waltham, 2015; Du et al., 2016, 2019), which might be attributed to the lack of or low biomass of macroalgae there. Furthermore, the δ13C values of most predators were relatively concentrated and overlapped more with those of herbivores and planktivores/filter feeders rather than detritivores, suggesting that the main trophic pathway of the food web was the grazing pathway (involving a combination of phytoplankton, macroalgae, epiphytes, herbivores, planktivores/filter feeders, and their predators) in the XC seagrass meadow.
Flow of Seagrass Carbon in Food Web and Its Influencing Factors
In this study, the eutrophication-induced proliferation of macroalgae and epiphytes, as well as their seasonal changes, significantly affected their contribution to the diet of consumers and carbon transfer in the food chain, but this effect was observed only on specific species/groups. According to the SIMMR result (Figure 4, Supplementary Table 7), the transfer of seagrass carbon in the grazing food chain pathway was markedly limited by the abundance of epiphytes and macroalgae, at least compared with the situation of overgrazing by large grazers (Valentine and Duffy, 2006; Hyndes et al., 2014). In summer, epiphytes with higher biomass contributed significantly to the diets of herbivores and omnivores. In winter, epiphytes still contributed to the diet of herbivores to some extent, which might be attributed to the relatively conservative feeding habits of some herbivores (Bremm et al., 2016), while herbivores and omnivores mainly relied upon palatable and abundant macroalgae (see Contribution of Food Sources to the Trophic Groups). Meanwhile, the predators mainly preyed on herbivores rather than detritivores in both seasons (see Key Carbon Transfer Processes and Major Trophic Pathway in the Seagrass Meadow). These indicated that the carbon flow pathway of this seagrass meadow food web was affected by the seasonal abundance of epiphytes and macroalgae, and that epiphyte- and macroalgae-derived carbon was mainly transmitted through herbivores/omnivores to predators in summer and in winter. These were also proved by the higher contribution of epiphytes in summer and macroalgae in winter to carnivores.
However, the transfer of seagrass carbon in the detrital food chain was still relatively stable, even in the presence of abundant macroalgae. The mixing model indicated that the contribution of seagrass to detritivores was higher than that of other food sources, with a high average value (>53%) in both summer and winter (Figure 4). This result is higher than that of Park et al. (2013) in temperate eutrophic Z. marina meadow, but similar to the results of Sepulveda-lozada et al. (2015) in tropical eutrophic seagrass meadow. It is possible, because the absolute decomposition of seagrass dead leaves takes longer compared with macroalgae (Lavery et al., 2013; Liu et al., 2016b; Trevathan-Tackett et al., 2020), which might accumulate adequate food for the diet of detritivores. Meanwhile, seagrass meadows in tropical regions may accumulate more seagrass debris than those in temperate regions as a result of relatively mild seasonal changes (Nakamoto et al., 2019). Therefore, adequate seagrass detritus throughout the year in tropical seagrass meadows might keep the transfer pathway of carbon derived from seagrass stable along the detritus food chain (Figure 5).
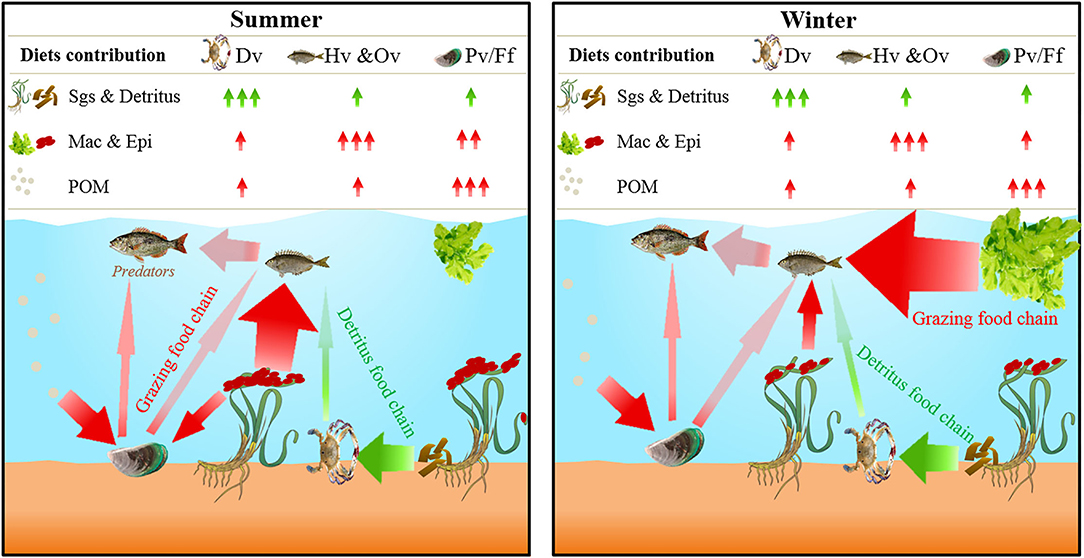
Figure 5. Conceptual summary of findings for the carbon transfer process of food web and trophic pathway in a eutrophic tropical seagrass meadow.
Overall, our study clarified the transfer process of each carbon source in the XC seagrass meadow food web by identifying the carbon sources of each trophic group, and identified its main trophic pathway (grazing food chain), influencing factors (seasonal variations), and the trophic role of seagrass (provide carbon source through the detritus food chain). However, our results explored the process of carbon transfer in a specific tropical seagrass meadow. In further studies, spatial comparisons are also necessary to enhance our understanding of the effect of different primary producer structures on the process of carbon transfer in seagrass meadows.
Conclusions
Our study demonstrated that grazing food chain was the main trophic pathway in eutrophic tropical seagrass meadows. Meanwhile, seasonal variations could result in the seasonal proliferation of macroalgae and epiphytes, which could obviously affect carbon transfer processes and trophic pathways in the food web. In summer, abundant epiphytes, rather than seagrass, chiefly fueled the food web through the grazing food chain. In winter, the food web is mainly fueled by prolific macroalgae through grazing food chain. In contrast, seagrass was relatively abundant throughout the year, and fueled the food web mainly through the detritus food chain in both seasons. In addition, although the proliferation of macroalgae and epiphytes seems to limit the transfer of seagrass carbon in the grazing food chain, seagrass trapped particles into sediment and on seagrass leaves by meadow architecture, which could also be used in the food web. Hence, seagrass could also play an important role in the carbon flow transfer of the food web in eutrophic tropical seagrass meadows.
Data Availability Statement
The datasets presented in this study can be found in online repositories. The names of the repository/repositories and accession number(s) can be found in the article/Supplementary Material.
Ethics Statement
The animal study was reviewed and approved by South China Sea Institute of Oceanology, Chinese Academy of Sciences.
Author Contributions
LC: conceptualization, methodology, investigation, formal analysis, visualization, and writing—original draft. ZJ: conceptualization, investigation, and writing—review and editing. XH: supervision, conceptualization, writing—review and editing, and funding acquisition. YW: investigation and writing—review. SL, JL, QC, and JH: investigation. All authors contributed to the article and approved the submitted version.
Funding
This research was supported by the National Natural Science Foundation of China (Grant Nos: 41730529, U1901221), Key Special Project for Introduced Talents Team of Southern Marine Science and Engineering Guangdong Laboratory (Guangzhou) (GML2019ZD0405), the Natural Science Fund of Guangdong (2019A1515010552, 2020A1515010907, 2018A030310043), and the Innovation Academy of South China Sea Ecology and Environmental Engineering, Chinese Academy of Sciences (ISEE2021PY06, ISEE2021ZD03).
Conflict of Interest
The authors declare that the research was conducted in the absence of any commercial or financial relationships that could be construed as a potential conflict of interest.
Publisher's Note
All claims expressed in this article are solely those of the authors and do not necessarily represent those of their affiliated organizations, or those of the publisher, the editors and the reviewers. Any product that may be evaluated in this article, or claim that may be made by its manufacturer, is not guaranteed or endorsed by the publisher.
Acknowledgments
The authors thank Shouhui Dai for performing the stable isotope analyses.
Supplementary Material
The Supplementary Material for this article can be found online at: https://www.frontiersin.org/articles/10.3389/fmars.2021.725282/full#supplementary-material
References
Abrantes, K., and Sheaves, M. (2009). Food web structure in a near-pristine mangrove area of the Australian Wet Tropics. Estuar. Coast. Shelf Sci. 82, 597–607. doi: 10.1016/j.ecss.2009.02.021
Arbi, I., Liu, S., Zhang, J., Wu, Y., and Huang, X. (2018). Detection of terrigenous and marine organic matter flow into a eutrophic semi-enclosed bay by δ13C and δ15N of intertidal macrobenthos and basal food sources. Sci. Total Environ. 613–614, 847–860. doi: 10.1016/j.scitotenv.2017.09.143
Baden, S., Boström, C., Tobiasson, S., Arponen, H., and Moksnes, P. O. (2010). Relative importance of trophic interactions and nutrient enrichment in seagrass ecosystems: a broad-scale field experiment in the Baltic-Skagerrak area. Limnol. Oceanogr. 55, 1435–1448. doi: 10.4319/lo.2010.55.3.1435
Barnes, R. S. K. (2019). Context dependency in the effect of Ulva-induced loss of seagrass cover on estuarine macrobenthic abundance and biodiversity. Aquat. Conserv. Mar. Freshw. Ecosyst. 29, 163–174. doi: 10.1002/aqc.2977
Barry, S. C., Hyman, A. C., Jacoby, C. A., Reynolds, L. K., Kowalewski, M., and Frazer, T. K. (2021). Variation in seagrass-associated macroinvertebrate communities along the Gulf Coast of Peninsular Florida: an exploration of patterns and ecological consequences. Front. Mar. Sci. 8:596966. doi: 10.3389/fmars.2021.596966
Becherucci, M. E., Alvarez, M. F., Iribarne, O., and Martinetto, P. (2019). Eutrophication in a semi-desert coastal ecosystem promotes increases in N and C isotopic signatures and changes in primary sources. Mar. Environ. Res. 146, 71–79. doi: 10.1016/j.marenvres.2019.03.004
Beesley, L. S., Pusey, B. J., Douglas, M. M., Gwinn, D. C., Canham, C. A., Keogh, C. S., et al. (2020). New insights into the food web of an Australian tropical river to inform water resource management. Sci. Rep. 10, 1–12. doi: 10.1038/s41598-020-71331-0
Boudouresque, C. F., Pergent, G., Pergent-Martini, C., Ruitton, S., Thibaut, T., and Verlaque, M. (2016). The necromass of the Posidonia oceanica seagrass meadow: fate, role, ecosystem services and vulnerability. Hydrobiologia 781, 25–42. doi: 10.1007/s10750-015-2333-y
Bremm, C., Carvalho, P. C. F., Fonseca, L., Amaral, G. A., Mezzalira, J. C., Perez, N. B., et al. (2016). Diet switching by mammalian herbivores in response to exotic grass invasion. PLoS ONE 11, 1–16. doi: 10.1371/journal.pone.0150167
Burkholder, D. A., Heithaus, M. R., and Fourqurean, J. W. (2012). Feeding preferences of herbivores in a relatively pristine subtropical seagrass ecosystem. Mar. Freshw. Res. 63, 1051–1058. doi: 10.1071/MF12029
Burress, E. D., Duarte, A., Gangloff, M. M., and Siefferman, L. (2013). Isotopic trophic guild structure of a diverse subtropical South American fish community. Ecol. Freshw. Fish 22, 66–72. doi: 10.1111/eff.12002
Carlier, A., Riera, P., Amouroux, J., Bodiou, J., Desmalades, M., and Grémare, A. (2009). Spatial heterogeneity in the food web of a heavily modified Mediterranean coastal lagoon: stable isotope evidence. Aquat. Biol. 5, 167–179. doi: 10.3354/ab00147
Carvalho, D. R., de, Alves, C. B. M., Moreira, M. Z., and Pompeu, P. S. (2020). Trophic diversity and carbon sources supporting fish communities along a pollution gradient in a tropical river. Sci. Total Environ. 738:139878. doi: 10.1016/j.scitotenv.2020.139878
Caut, S., Angulo, E., and Courchamp, F. (2009). Variation in discrimination factors (Δ15N and Δ13C): the effect of diet isotopic values and applications for diet reconstruction. J. Appl. Ecol. 46, 443–453. doi: 10.1111/j.1365-2664.2009.01620.x
Chiu, S. H., Huang, Y. H., and Lin, H. J. (2013). Carbon budget of leaves of the tropical intertidal seagrass Thalassia hemprichii. Estuar. Coast. Shelf Sci. 125, 27–35. doi: 10.1016/j.ecss.2013.03.026
Coll, M., Schmidt, A., Romanuk, T., and Lotze, H. K. (2011). Food-web structure of seagrass communities across different spatial scales and human impacts. PLoS ONE 6:e22591. doi: 10.1371/journal.pone.0022591
Connolly, R. M., and Waltham, N. J. (2015). Spatial analysis of carbon isotopes reveals seagrass contribution to fishery food web. Ecosphere 6, 1–12. doi: 10.1890/ES14-00243.1
Corbisier, T. N., Petti, M. A. V., Skowronski, R. S. P., and Brito, T. A. S. (2004). Trophic relationships in the nearshore zone of Martel Inlet (King George Island, Antarctica): δ13C stable-isotope analysis. Polar Biol. 27, 75–82. doi: 10.1007/s00300-003-0567-z
Dézerald, O., Srivastava, D. S., Céréghino, R., Carrias, J. F., Corbara, B., Farjalla, V. F., et al. (2018). Functional traits and environmental conditions predict community isotopic niches and energy pathways across spatial scales. Funct. Ecol. 32, 2423–2434. doi: 10.1111/1365-2435.13142
Du, J., Chen, Z., Xie, M., Chen, M., Zheng, X., Liao, J., et al. (2019). Analysis of organic carbon sources in tropical seagrass fish: a case study of the east coast of Hainan Province. Mar. Biol. Res. 15, 513–522. doi: 10.1080/17451000.2019.1673896
Du, J., Zheng, X., Peristiwady, T., Liao, J., Ch. Makatipu, P., Yin, X., et al. (2016). Food sources and trophic structure of fishes and benthic macroinvertebrates in a tropical seagrass meadow revealed by stable isotope analysis. Mar. Biol. Res. 12, 748–757. doi: 10.1080/17451000.2016.1183791
Enríquez, S., Duarte, C. M., and Sand-Jensen, K. (1993). Patterns in decomposition rates among photosynthetic organisms: the importance of detritus C:N:P content. Oecologia 94, 457–471. doi: 10.1007/BF00566960
Fan, M., Huang, X., Zhang, D., Zhang, J., Jiang, Z., and Zeng, Y. (2011). Food sources of fish and macro-invertebrates in a tropical seagrass bed at Xincun Bay, Southern China. Shengtai Xuebao Acta Ecol. Sin. 31, 31–38.
France, R. L. (2015). Feeding on the edge: δ13C analysis of a macrofauna food web from disappearing seagrass beds in a polluted urban harbour - implications for biomonitoring and restoration efforts. Aquat. Conserv. Mar. Freshw. Ecosyst. 25, 81–90. doi: 10.1002/aqc.2353
François, R., Thibaud, M., Marleen, D. T., Michel, L. N., and Gilles, L. (2018). Seagrass organic matter transfer in Posidonia oceanica macrophytodetritus accumulations. Estuar. Coast. Shelf Sci. 212, 73–79. doi: 10.1016/j.ecss.2018.07.001
Grasshoff, K. (2009). Methods of Seawater Analysis, eds K. Grasshoff, K. Kremling, and M. Ehrhardt. Weinheim: Wiley-VCH Verlag GmbH.
Gu, B., Chapman, A. D., and Schelske, C. L. (2006). Factors controlling seasonal variations in stable isotope composition of particulate organic matter in a soft water eutrophic lake. Limnol. Oceanogr. 51, 2837–2848. doi: 10.4319/lo.2006.51.6.2837
Guo, G., Dong, S., and Dong, Y. (2006). Effects of constant and diel fluctuating temperatures on the growth and photosynthesis of the macroalgae Ulva pertusa Kjellm. Period. Ocean Univ. China 36, 941–945. doi: 10.16441/j.cnki.hdxb.2006.06.019
Han, Q., Soissons, L. M., Bouma, T. J., van Katwijk, M. M., and Liu, D. (2016). Combined nutrient and macroalgae loads lead to response in seagrass indicator properties. Mar. Pollut. Bull. 106, 174–182. doi: 10.1016/j.marpolbul.2016.03.004
Heck, K. L., Samsonova, M., Poore, A. G. B., and Hyndes, G. A. (2020). Global patterns in seagrass herbivory: why, despite existing evidence, there are solid arguments in favor of latitudinal gradients in seagrass herbivory. Estuar. Coasts 44, 481–490. doi: 10.1007/s12237-020-00833-x
Hily, C., Connan, S., Raffin, C., and Wyllie-Echeverria, S. (2004). In vitro experimental assessment of the grazing pressure of two gastropods on Zostera marina L. ephiphytic algae. Aquat Bot. 78, 183–195. doi: 10.1016/j.aquabot.2003.10.001
Huang, X., Huang, L., Li, Y., Xu, Z., Fong, C. W., Huang, D., et al. (2006). Main seagrass beds and threats to their habitats in the coastal sea of South China. Chinese Sci. Bull. 51, 136–142. doi: 10.1007/s11434-006-9136-5
Huang, X., Jiang, Z., Liu, S., Yu, S., Wu, Y., Zhang, J., et al. (2019). Study on Ecology of Tropical Seagrass in China. Beijing: Science China Press.
Hyndes, G. A., and Lavery, P. S. (2005). Does transported seagrass provide an important trophic link in unvegetated, nearshore areas? Estuar. Coast Shelf Sci. 63, 633–643. doi: 10.1016/j.ecss.2005.01.008
Hyndes, G. A., Nagelkerken, I., Mcleod, R. J., Connolly, R. M., Lavery, P. S., and Vanderklift, M. A. (2014). Mechanisms and ecological role of carbon transfer within coastal seascapes. Biol. Rev. 89, 232–254. doi: 10.1111/brv.12055
Jankowska, E., Michel, L. N., Lepoint, G., and Włodarska-Kowalczuk, M. (2019). Stabilizing effects of seagrass meadows on coastal water benthic food webs. J. Exp. Mar. Bio. Ecol. 510, 54–63. doi: 10.1016/j.jembe.2018.10.004
Jepsen, D. B., and Winemiller, K. O. (2002). Structure of tropical river food webs revealed by stable isotope ratios. Oikos 96, 46–55. doi: 10.1034/j.1600-0706.2002.960105.x
Jiang, Z., Huang, D., Fang, Y., Cui, L., and Zhao, C. (2020). Home for marine species : seagrass leaves as vital spawning grounds and food source. Front. Mar. Sci. 7:194. doi: 10.3389/fmars.2020.00194
Jiménez-Ramos, R., Egea, L. G., Vergara, J. J., Bouma, T. J., and Brun, F. G. (2019). The role of flow velocity combined with habitat complexity as a top–down regulator in seagrass meadows. Oikos 128, 64–76. doi: 10.1111/oik.05452
Kang, C. K., Choy, E. J., Hur, Y. B., and Myeong, J. I. (2009). Isotopic evidence of particle size-dependent food partitioning in cocultured sea squirt Halocynthia roretzi and Pacific oyster Crassostrea gigas. Aquat. Biol. 6, 289–302. doi: 10.3354/ab00126
Kaymak, N., Winemiller, K. O., Akin, S., Altuner, Z., Polat, F., and Dal, T. (2018). Spatial and temporal variation in food web structure of an impounded river in Anatolia. Mar. Freshw. Res. 69, 1453–1471. doi: 10.1071/MF17270
Kennedy, H., Gacia, E., Kennedy, D. P., Papadimitriou, S., and Duarte, C. M. (2004). Organic carbon sources to SE Asian coastal sediments. Estuar. Coast. Shelf Sci. 60, 59–68. doi: 10.1016/j.ecss.2003.11.019
Kermagoret, C., Claudet, J., Derolez, V., Nugues, M. M., Ouisse, V., Quillien, N., et al. (2019). How does eutrophication impact bundles of ecosystem services in multiple coastal habitats using state-and-transition models. Ocean Coast. Manag. 174, 144–153. doi: 10.1016/j.ocecoaman.2019.03.028
Larkum, A. W. D., Orth, R. J., and Duarte, C. M. (2006). Seagrasses: Biology, Ecology and Conservation. Dordrecht: Springer.
Lavery, P. S., McMahon, K., Weyers, J., Boyce, M. C., and Oldham, C. E. (2013). Release of dissolved organic carbon from seagrass wrack and its implications for trophic connectivity. Mar. Ecol. Prog. Ser. 494, 121–133. doi: 10.3354/meps10554
Lebreton, B., Richard, P., Galois, R., Radenac, G., Brahmia, A., Colli, G., et al. (2012). Food sources used by sediment meiofauna in an intertidal Zostera noltii seagrass bed: a seasonal stable isotope study. Mar. Biol. 159, 1537–1550. doi: 10.1007/s00227-012-1940-7
Li, M., Lundquist, C. J., Pilditch, C. A., Rees, T. A. V., and Ellis, J. (2019). Implications of nutrient enrichment for the conservation and management of seagrass Zostera muelleri meadows. Aquat. Conserv. Mar. Freshw. Ecosyst. 29, 1484–1502. doi: 10.1002/aqc.3141
Lin, J., Liu, X., Lai, T., He, B., Du, J., and Zheng, X. (2021). Trophic importance of the seagrass Halophila ovalis in the food web of a Hepu seagrass bed and adjacent waters, Beihai, China. Ecol. Indic. 125:107607. doi: 10.1016/j.ecolind.2021.107607
Liu, S., Jiang, Z., Wu, Y., Zhang, J., Arbi, I., Ye, F., et al. (2017). Effects of nutrient load on microbial activities within a seagrass-dominated ecosystem: implications of changes in seagrass blue carbon. Mar. Pollut. Bull. 117, 214–221. doi: 10.1016/j.marpolbul.2017.01.056
Liu, S., Jiang, Z., Zhang, J., Wu, Y., Lian, Z., and Huang, X. (2016a). Effect of nutrient enrichment on the source and composition of sediment organic carbon in tropical seagrass beds in the South China Sea. Mar. Pollut. Bull. 110, 274–280. doi: 10.1016/j.marpolbul.2016.06.054
Liu, Y., Zhang, S., Zhou, X., Zhao, X., Xu, S., and Xing, C. (2016b). C/N stable isotope analysis of macro algae litters in kelp bed in Gouqi Island. J. Shanghai Oecan Univ. 25, 438–444. doi: 10.12024/jsou.20160101633
Macreadie, P. I., Jarvis, J., Trevathan-Tackett, S. M., and Bellgrove, A. (2017). “Seagrasses and macroalgae: importance, vulnerability and impacts,” in Climate Change Impacts on Fisheries and Aquaculture: A Global Analysis Volume II, eds B. F. Phillips and M. Perez-Ramirez (West Sussex, UK: John Wiley & Sons, Ltd), 729–770.
Madi Moussa, R., Bertucci, F., Jorissen, H., Gache, C., Waqalevu, V. P., Parravicini, V., et al. (2020). Importance of intertidal seagrass beds as nursery area for coral reef fish juveniles (Mayotte, Indian Ocean): nursery areas in a tropical island. Reg. Stud. Mar. Sci. 33:100965. doi: 10.1016/j.rsma.2019.100965
Malet, N., Sauriau, P. G., Ryckaert, M., Malestroit, P., and Guillou, G. (2008). Dynamics and sources of suspended particulate organic matter in the Marennes-Oléron oyster farming bay: insights from stable isotopes and microalgae ecology. Estuar. Coast. Shelf Sci. 78, 576–586. doi: 10.1016/j.ecss.2007.11.001
Mascart, T., De Troch, M., Remy, F., Loïc, N. M., and Lepoint, G. (2018). Seasonal dependence on seagrass detritus and trophic niche partitioning in four copepod eco-morphotypes. Food Webs 16:e00086. doi: 10.1016/j.fooweb.2018.e00086
Mittermayr, A., Fox, S. E., and Sommer, U. (2014). Temporal variation in stable isotope composition (δ13C, δ15N and δ34S) of a temperate Zostera marina food web. Mar. Ecol. Prog. Ser. 505, 95–105. doi: 10.3354/meps10797
Nakamoto, K., Hayakawa, J., Kawamura, T., Ohtsuchi, N., Yamada, H., Kitagawa, T., et al. (2019). Seasonal fluctuation in food sources of herbivorous gastropods in a subtropical seagrass bed estimated by stable isotope analysis. J. Mar. Biol. Assoc. United Kingdom 99, 1119–1125. doi: 10.1017/S0025315418001108
Ouisse, V., Riera, P., Migné, A., Leroux, C., and Davoult, D. (2012). Food web analysis in intertidal Zostera marina and Zostera noltii communities in winter and summer. Mar. Biol. 159, 165–175. doi: 10.1007/s00227-011-1796-2
Park, H. J., Choy, E. J., Lee, K., and Kang, C. (2013). Trophic transfer between coastal habitats in a seagrass-dominated macrotidal embayment system as determined by stable isotope and fatty acid signatures. Mar. Freshw. Res. 64, 1169–1183. doi: 10.1071/MF12327
Parker, J. D., Montoya, J. P., and Hay, M. E. (2008). A specialist detritivore links Spartina alterniflora to salt marsh food webs. Mar. Ecol. Prog. Ser. 364, 87–95. doi: 10.3354/meps07504
Parnell, A., and Inger, R. (2019). Stable Isotope Mixing Models in R With Simmr. Available online at: https://cran.r-project.org/web/packages/simmr/vignettes/simmr.html
Parnell, A. C., Inger, R., Bearhop, S., and Jackson, A. L. (2010). Source partitioning using stable isotopes: coping with too much variation. PLoS ONE 5:e9672. doi: 10.1371/journal.pone.0009672
Platell, M., Wildsmith, M., Potter, I., and Valensini, F. (2009). Fish diets and food webs in the Swan – Canning estuary. River Sci. 28, 1–12.
Qiu, G., Short, F. T., Fan, H., and Liu, G. (2017). Temporal variation of intertidal seagrass in southern China (2008–2014). Ocean Sci. J. 52, 397–410. doi: 10.1007/s12601-017-0039-y
R Core Team (2019). R: A Language and Environment for Statistical Computing. R Foundation for Statistical Computing, Vienna. Available online at: http://www.r-project.org
Raven, J. A., Johnston, A. M., Kübler, J. E., Korb, R., Mcinroy, S. G., Handley, L. L., et al. (2002). Mechanistic interpretation of carbon isotope discrimination by marine macroalgae and seagrasses. Funct. Plant Biol. 29, 355–378. doi: 10.1071/PP01201
Riccialdelli, L., Newsome, S. D., Fogel, M. L., and Fernández, D. A. (2017). Trophic interactions and food web structure of a subantarctic marine food web in the Beagle Channel: Bahía Lapataia, Argentina. Polar Biol. 40, 807–821. doi: 10.1007/s00300-016-2007-x
Riegl, B. M., Moyer, R. P., Morris, L. J., Virnstein, R. W., and Purkis, S. J. (2005). Distribution and seasonal biomass of drift macroalgae in the Indian River Lagoon (Florida, USA) estimated with acoustic seafloor classification (QTCView, Echoplus). J. Exp. Mar. Bio. Ecol. 326, 89–104. doi: 10.1016/j.jembe.2005.05.009
Robertson, B. P., and Savage, C. (2020). Thresholds in catchment nitrogen load for shifts from seagrass to nuisance macroalgae in shallow intertidal estuaries. Limnol. Oceanogr. 66, 1–14. doi: 10.1002/lno.11689
Schaal, G., Riera, P., and Leroux, C. (2010). Trophic ecology in a Northern Brittany (Batz Island, France) kelp (Laminaria digitata) forest, as investigated through stable isotopes and chemical assays. J. Sea Res. 63, 24–35. doi: 10.1016/j.seares.2009.09.002
Schmidt, A. L., Coll, M., and Lotze, H. K. (2017). Regional-scale differences in eutrophication effects on eelgrass-associated (Zostera marina) macrofauna. Estuar. Coasts 40, 1096–1112. doi: 10.1007/s12237-016-0204-z
Sepulveda-lozada, A., Mendoza-Carranza, W.olff, M., Saint-Paul, U., and Ponce-Mendoza, A. (2015). Differences in food web structure of mangroves and freshwater marshes: evidence from stable isotope studies in the Southern Gulf of Mexico. Wetl. Ecol. Manag. 23, 293–314. doi: 10.1007/s11273-014-9382-2
Sun, Z., Gao, Q., Dong, S., Shin, P. K. S., and Wang, F. (2012). Seasonal changes in food uptake by the sea cucumber Apostichopus japonicus in a farm pond: evidence from C and N stable isotopes. J. Ocean Univ. China. 12, 160–168. doi: 10.1007/s11802-013-1952-z
Taylor, G. C., Hill, J. M., Jackson, M. C., Peel, R. A., and Weyl, O. L. F. (2017). Estimating δ15N fractionation and adjusting the lipid correction equation using Southern African freshwater fishes. PLoS ONE 12:e0178047. doi: 10.1371/journal.pone.0178047
Tew, K. S., Kuo, J., Cheng, J.-O., Ko, F.-C., Meng, P.-J., Mayfield, A. B., et al. (2021). Impacts of seagrass on benthic microalgae and phytoplankton communities in an experimentally warmed coral reef mesocosm. Front. Mar. Sci. 8:679683. doi: 10.3389/fmars.2021.679683
Tewfik, A., Rasmussen, J. B., and McCann, K. S. (2007). Simplification of seagrass food webs across a gradient of nutrient enrichment. Can. J. Fish. Aquat. Sci. 64, 956–967. doi: 10.1139/f07-071
Thresher, R. E., Nichols, P. D., Gunn, J. S., Bruce, B. D., and Furlani, D. M. (1992). Seagrass detritus as the basis of a coastal planktonic food chain. Limnol. Oceanogr. 37, 1754–1758. doi: 10.4319/lo.1992.37.8.1754
Trevathan-Tackett, S. M., Jeffries, T. C., Macreadie, P. I., Manojlovic, B., and Ralph, P. (2020). Long-term decomposition captures key steps in microbial breakdown of seagrass litter. Sci. Total Environ. 705:135806. doi: 10.1016/j.scitotenv.2019.135806
Valentine, J. F., and Duffy, J. E. (2006). “The central role of grazing in seagrass ecology,” in Seagrasses: Biology, Ecology and Conservation (Dordrecht: Springer), 463–501. doi: 10.1007/978-1-4020-2983-7_20
Valiela, I., Liu, D., Lloret, J., Chenoweth, K., and Hanacek, D. (2018). Stable isotopic evidence of nitrogen sources and C4 metabolism driving the world's largest macroalgal green tides in the Yellow Sea. Sci. Rep. 8, 1–12. doi: 10.1038/s41598-018-35309-3
Vergés, A., Alcoverro, T., and Romero, J. (2011). Plant defences and the role of epibiosis in mediating within-plant feeding choices of seagrass consumers. Oecologia 166, 381–390. doi: 10.1007/s00442-010-1830-y
Villa, C. A., Bell, I., Madden Hof, C., Limpus, C. J., and Gaus, C. (2019). Elucidating temporal trends in trace element exposure of green turtles (Chelonia mydas) using the toxicokinetic differences of blood and scute samples. Sci. Total Environ. 651, 2450–2459. doi: 10.1016/j.scitotenv.2018.10.092
Vizzini, S., and Mazzola, A. (2003). Seasonal variations in the stable carbon and nitrogen isotope ratios (13C/12C and 15N/14N) of primary producers and consumers in a western Mediterranean coastal lagoon. Mar. Biol. 142, 1009–1018. doi: 10.1007/s00227-003-1027-6
Vizzini, S., Sara, G., Michener, R. H., and Mazzola, A. (2002). The trophic role of the macrophyte Cymodocea nodosa (Ucria) Asch. in a Mediterranean saltworks: evidence from carbon and nitrogen stable isotope ratios. Bull. Mar. Sci. 71, 1369–1378. doi: 10.1515/BOT.2002.063
Wang, W. L., and Yeh, H. W. (2003). δ13C values of marine macroalgae from Taiwan. Bot. Bull. Acad. Sin. 44, 107–112.
Wei, C., Wang, D., Zhang, Y., Yang, Q., and Li, M. (2014). Investigation and analysis of phytoplankton in pearl oyster culture area of Xincun bay. J. South. Agric. 45, 1286–1290. doi: 10.3969/j:issn.2095-1191.2014.7.1286
Wiencke, C., and Fischer, G. (1990). Growth and stable carbon isotope composition of cold-water macroalgae in relation to light and temperature. Mar. Ecol. Prog. Ser. 65, 283–292. doi: 10.3354/meps065283
Xu, J., Fan, X., Zhang, X., Xu, D., Mou, S., Cao, S., et al. (2012). Evidence of coexistence of C3 and C4 photosynthetic pathways in a green-tide-forming alga, ulva prolifera. PLoS ONE 7:e37438. doi: 10.1371/journal.pone.0037438
Xu, W. Z., Cheung, S. G., Nan, Z., and Paul, Z. (2018). Dual isotope assessment of trophic dynamics of an intertidal infaunal community with seasonal shifts in food sources. Mar. Biol. 165, 1–13. doi: 10.1007/s00227-017-3278-7
Xu, Z., Huang, L., and Huang, X. (2009). Growth strategy of seagrass Thalassia hemprichii in Xincun Bay near Hainan Island of China. Mar. Sci. Bull. 11, 15–19.
Yoon, J.-D., Park, S.-H., Chang, K.-H., Choi, J.-Y., Joo, G.-J., Nam, G.-S., et al. (2015). Characteristics of fish fauna in the lower Geum river and identification of trophic guilds using stable isotopes analysis. Korean J. Environ. Biol. 33, 34–44. doi: 10.11626/KJEB.2015.33.1.034
Keywords: seagrass meadow, eutrophication, carbon sources, carbon transfer process, grazing pathway, detritus pathway
Citation: Cui L, Jiang Z, Huang X, Wu Y, Liu S, Chen Q, Li J and He J (2021) Carbon Transfer Processes of Food Web and Trophic Pathways in a Tropical Eutrophic Seagrass Meadow. Front. Mar. Sci. 8:725282. doi: 10.3389/fmars.2021.725282
Received: 15 June 2021; Accepted: 27 September 2021;
Published: 29 October 2021.
Edited by:
Oscar Serrano, Edith Cowan University, AustraliaReviewed by:
Kenta Watanabe, Port and Airport Research Institute (PARI), JapanAlexandra Kinnby, University of Gothenburg, Sweden
Copyright © 2021 Cui, Jiang, Huang, Wu, Liu, Chen, Li and He. This is an open-access article distributed under the terms of the Creative Commons Attribution License (CC BY). The use, distribution or reproduction in other forums is permitted, provided the original author(s) and the copyright owner(s) are credited and that the original publication in this journal is cited, in accordance with accepted academic practice. No use, distribution or reproduction is permitted which does not comply with these terms.
*Correspondence: Zhijian Jiang, amlhbmd6ajE5ODJAc2NzaW8uYWMuY24=; Xiaoping Huang, eHBodWFuZ0BzY3Npby5hYy5jbg==