- 1Marine Science Institute, University of the Philippines Diliman, Quezon City, Philippines
- 2Philippine Nuclear Research Institute, Department of Science and Technology, Diliman, Quezon City, Philippines
Coral reefs are typified by their benthic components, and reef diversity and productivity are traditionally ascribed to the symbiotic association between corals and zooxanthellae, and other macroalgal forms. Less understood is the role of plankton and adjacent pelagic areas in contributing to reef productivity. Half of the reef benthos are filter or particle feeders, while a significant proportion of reef fishes are planktivorous. These organisms can serve as bridges between adjacent oceanic areas to the reef proper, and the pelagic and benthic realm. Here, we investigate the plankton trophic dynamics in two reef systems in the West Philippine Sea. Physico-chemical data, phytoplankton and mesozooplankton samples were collected from stations spanning offshore to reef areas per site. These were subjected to microscopic and stable isotope analysis to determine variability in plankton distribution, phytoplankton and zooplankton interactions, and gain insights into the trophic dynamics and productivity of reefs. Results showed distinct variations in plankton biomass and assemblage from offshore to reef areas, as well as between the reef systems. Phytoplankton distributions pointed toward filtering out of cells across the fore reef and reef flat areas, while mesozooplankton distributions could be mediated more by other factors. Isotopic signatures of δ13C and δ15N indicated the influence of different nutrient sources for phytoplankton and that mesozooplankton relied only partly on phytoplankton for food in most areas of the reefs. The mesozooplankton likely also obtain food from other sources such as the microbial and detrital pathways. More in-depth spatio-temporal studies on these bentho-pelagic interactions are recommended, which can provide more robust estimates of the trophic dynamics of these reefs that are situated in important fishing grounds and key biodiversity areas.
Introduction
Coral reefs are one of the most biologically diverse and productive coastal ecosystems. They are geographically located in shallow oligotrophic waters in the inter-tropical regions at 30°N–30°S, and these reefs occupy less than 0.1% of the world’s oceans (Lesser, 2004). Within these restrictions, reefs are still able to support complex faunal and floral communities that are distinct in their taxonomic organization and spatial and temporal distribution. These marine ecosystems also play a role in the exchange of organic and non-organic matter with the adjacent ocean and lagoon (Hamner et al., 2007).
At the base of the marine food web, plankton support the functioning of the coral reef ecosystem by providing food for a wide array of coral reef-associated organisms. Half of the benthic fauna on coral reefs are estimated to be filter feeders or particle feeders; these animals feed on zooplankton and particulate organic matter (POM) (Yahel et al., 1998; Mayal et al., 2009). Many reef fish species larvae and juveniles rely on mesozooplankton and consequently the lower trophic levels for food. Despite occupying what is typically considered oligotrophic seas, the contribution of plankton to the productivity of coral reefs has long been recognized (Glynn, 1973; Hamner et al., 1998) though still less explored (Skinner et al., 2021). In one of the first studies to look into plankton on reefs, Glynn (1973) reported variations in plankton across a reef in Puerto Rico and estimated that over 91% of diatoms and 60% of zooplankton were removed from the water presumably by reef organisms. Hamner et al. (2007) illustrated the spatial variation of available zooplankton from the oceanic side to the lagoon in the barrier reef of Palau. This and other studies (Morales and Murillo, 1996; Gruber et al., 2018) also highlight the interaction between the open ocean and coral reefs through the import and export of plankton. More recent studies are demonstrating the significant contribution of oceanic processes to reef productivity (Genin et al., 2009; Wyatt et al., 2010, 2013; Leichter et al., 2013; McMahon et al., 2016; Morais and Bellwood, 2019; Fey et al., 2021). Wyatt et al. (2013) highlight that allochthonous oceanic particulate organic material (POM) comprised primarily of phytoplankton are important in providing nutrients at the fringing Ningaloo Reef. Morais and Bellwood (2019) estimated that 41% of the fish productivity in a windward reef on the Great Barrier Reef derived from pelagic subsidies, while McMahon et al. (2016) found that pelagic productivity can contribute greater than 70% to C consumed and assimilated by fish in oceanic reefs in the Red Sea.
Mesozooplankton are considered to be a key group bridging primary producers to benthos and fishes at the higher trophic levels in different marine ecosystems including coral reefs (Roman et al., 1990). This transfer of organic material through the mesozooplankton can occur through three possible pathways: grazing, microbial and detrital pathways (Gottfried and Roman, 1983; Morillo-Velarde et al., 2018). The grazing pathway is the classical concept of microphytoplankton as direct prey of zooplankton. The microbial pathway starts with heterotrophic bacteria which are consumed by nano- and microzooplankton such as protists which are then consumed by the mesozooplankton. In the detrital pathway, mesozooplankton consume non-living organic matter such as dead animals, plants, feces and other waste materials. The contribution of different trophic pathways in the system can vary depending on season (Nakajima et al., 2017) and location (McMahon et al., 2016; Skinner et al., 2021).
Measurement of stable isotopes has been widely used to provide insights on the trophic interactions both in freshwater and marine ecosystems (Fry and Sherr, 1984) specifically in a time-integrated manner (Vander Zanden and Rasmussen, 1999; Post, 2002). The pathways of energy flow through food webs can be traced and understood using carbon and nitrogen stable isotopes (Vander Zanden et al., 1999). The trophic positions of organisms, which reflect species’ long-term diet, are estimated by using the δ13C and δ15N signatures of the primary producers (e.g., phytoplankton) and various consumers (e.g., zooplankton and fish) (Popp et al., 2007). Moreover, stable isotopes analyses have been used to better understand the trophic interactions between planktonic communities (Basedow et al., 2009, 2016; Giering et al., 2019) in pelagic zones to trace sources and transport of nutrients.
The input of allochthonous material into coral reef productivity is generally recognized yet relatively unexplored. Through these pelagic subsidies, larger-scale oceanographic variabilities can influence reef resilience and/or vulnerability. In this study, we contribute information on this process in reefs in the Philippines, an area that is a global biodiversity hotspot and within a key fisheries region but with limited data. We investigated the interaction between the pelagic and benthic areas through the plankton, and the potential trophic paths in reefs in the West Philippine Sea. Specifically, we examined the plankton composition, abundance, and stable isotope signatures and investigated the variations in the distribution of microphytoplankton and mesozooplankton across and between two tropical reefs in the West Philippine Sea, and assessed the potential trophic pathway between phytoplankton and mesozooplankton within these reefs.
Materials and Methods
Study Sites
The Kalayaan Island Group (KIG) in the West Philippine Sea (WPS) is estimated to house approximately 30% of coral reefs in the Philippines and is recognized as a key biodiversity area (Ong et al., 2002; Arceo et al., 2020). These offshore reefs and surrounding pelagic areas also serve as important fishing grounds (Aliño and Quibilan, 2003). This area is embedded within the South China Sea (SCS) whose general circulation patterns are highly influenced by monsoonal wind systems (Wyrtki, 1961; Hu et al., 2000). During the northeast monsoon season (or winter time), overall circulation is cyclonic, with strong southward flows off the coast of Vietnam. During the southwest monsoon (summer), flow is primarily anticyclonic with weaker northward currents. Within the central area of the South China Sea, where the KIG is located, currents are weaker, and tides also have small amplitudes (Villanoy and Jacinto, 2017). The SCS is generally considered oligotrophic (Zhang and Yin, 2015), and the offshore and shelf waters of the WPS exhibit the Typical Tropical Structure with a thermocline, nutricline, a subsurface chlorophyll maximum found at around 40–75 m, and the peak primary production overlapping or just above the chlorophyll maximum (San Diego-McGlone et al., 1999). Previous work have focused on characterizing gross hydrodynamic features in the deeper waters of the WPS and SCS, and there are no studies that we are aware of specifically describing oceanographic patterns across time and space at a similar atoll scale featured in this study.
The research expedition in KIG was conducted from April 28, 2017 to May 18, 2017 on-board the BRP Velasquez. Two reef systems, Pag-asa (Thitu) Island and Sabina Shoal, within the KIG were the sites for this study (Figure 1). The samples were collected from at most six stations across two separate transects in Pag-asa Island on May 3 and 5, and one transect in Sabina Shoal on May 9. Small boats deployed from the ship were used to sample the reef areas. Supplementary Table S1 documents the details for the sampling stations.
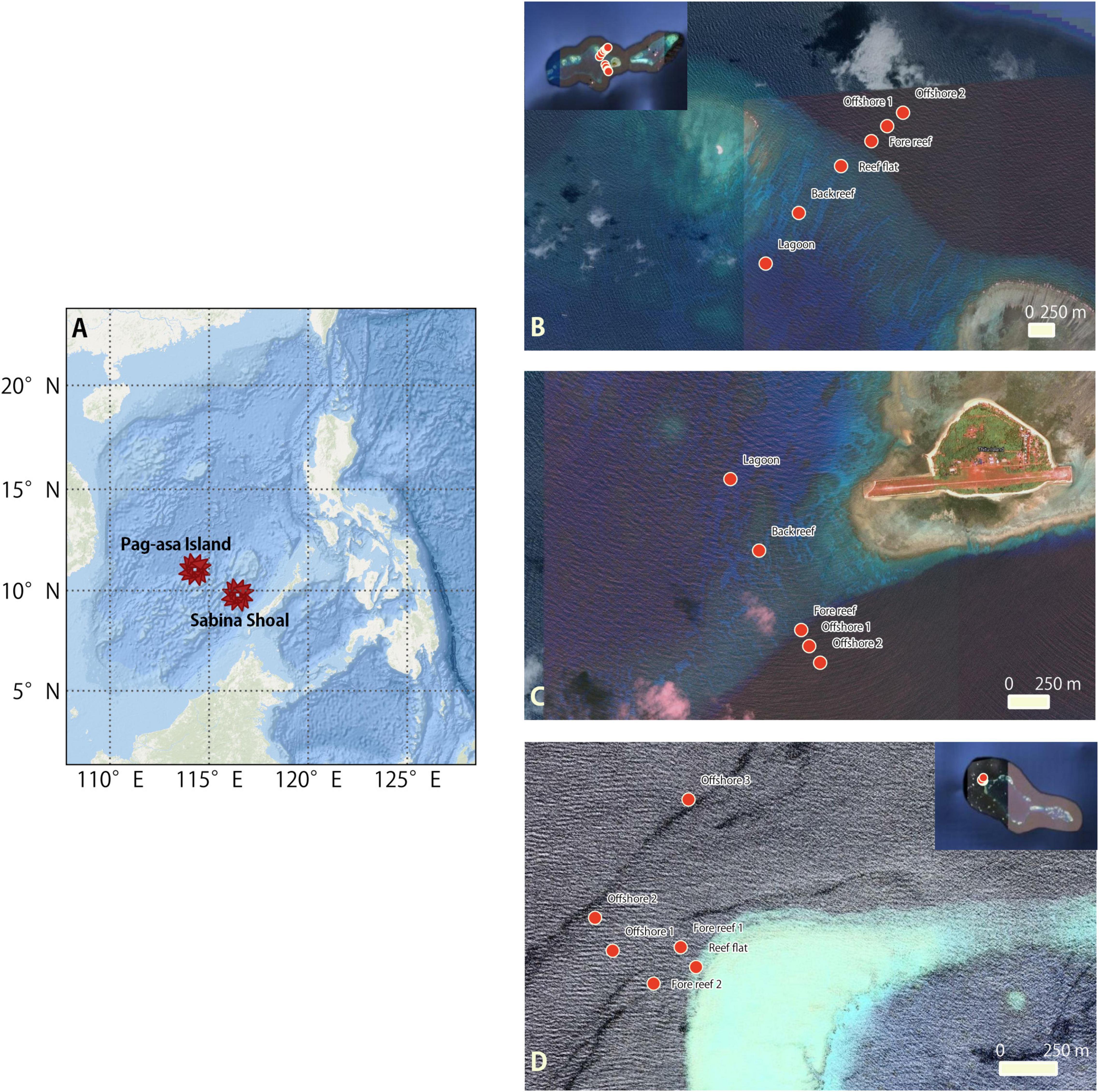
Figure 1. Maps of the study sites in the Kalayaan Island Group. (A) Philippine map showing the West Philippine Sea and islands; (B) Pag-asa Island Transect 1 with the larger Pag-asa Island perspective showing the 2 transects as inset; (C) Pag-asa Island Transect 2; and, (D) Sabina Shoal Transect and the whole Sabina Shoal perspective as inset.
Pag-asa Island is at the eastern tip of an atoll in the northwestern portion of the KIG. It has an area of 37.2 hectares and is the largest Philippine-administered islands in the Kalayaan Island Group situated toward the west. It is occupied by military personnel and a civilian population of about 300. Transect 1 was located in the northwestern area, while Transect 2 was in the southwestern area. The first transect has a broad reef flat, a more pronounced spur and groove area, and a steeper reef slope than the second. Transect 2 is situated adjacent to the inhabited area of the island and the landing point for small boats is nearby. For both transects, Station 1 was in the lagoon area, Station 2 in the back reef, Station 3 in the reef flat (only for Transect 1), Station 4 on the fore reef slope and Stations 5 and 6 were offshore from the reef. We were unable to sample the targeted Station 3 at the reef flat area at Transect 2 due to a sudden squall and limited time at the island. In contrast to Pag-asa Island, Sabina Shoal is an uninhabited atoll at the eastern portion of the KIG. The transect is situated on the northwestern portion of the atoll and is characterized by a narrow and steep fore reef. Station 1 is in the reef flat area, while Station 2 is in the fore-reef area. Station 3 is in the fore-reef slope/deeper reef area. Stations 4 to 5 are sequentially further offshore from the reef. We were unable to access the lagoon here due to the extremely shallow and extensive reef flat.
Physico-Chemical Parameters
For each station, temperature, salinity, and density measurements were obtained using a CastAway CTD (YSI, United States) up to a maximum of 40 m or less, depending on the actual depth. A 5 L Niskin sampling bottle (General Oceanics Inc., United States) was deployed up to five depths per station to obtain samples for dissolved oxygen, nutrients, and chlorophyll a. Samples for dissolved oxygen were collected into BOD bottles and fixed with manganous chloride and alkali-iodide. Sub-samples for inorganic nutrients (phosphate, silicate, nitrite, and nitrate) were collected into 250 mL HDPE bottles, and water samples for chlorophyll a analysis were collected in 2.5 L book bottles. The inorganic nutrients and chlorophyll a samples were stored in an ice box until samples could be processed back on the ship.
On board the ship, samples for dissolved oxygen were immediately analyzed using a slight modification of the Winkler (1888) method by Carpenter (1965). Seawater samples for inorganic nutrients were transferred to centrifuge tubes and stored in a freezer at –20°C (4°C for silicate) before laboratory analyses on land. For chlorophyll a, 1000 mL of the sample was filtered through a 0.7 μm Whatman® GFF using a vacuum filter manifold. A few drops of magnesium carbonate (MgCO3) solution were added to the filter paper prior to filtration to preserve the samples. After filtration, the filter papers were placed in aluminum foil sheets and stored in a freezer (−20°C) until analysis back on land.
Plankton Collection
Collection and Processing of Phytoplankton and Zooplankton for Microscopy
Niskin bottles were deployed at discrete depths per station ranging from subsurface to a maximum of 85 m to gather phytoplankton samples. The number and actual depths sampled depended on the actual bottom depth (Supplementary Table S1). A known volume, typically 1 L was collected from the Niskin bottles, which were subsequently filtered through a 20 μm sieve, decanted into amber bottles, and fixed with 5 ml formalin. These were used to characterize the type and abundance of phytoplankton per station. For zooplankton composition and abundance, a plankton net with 200 μm mesh size and a flowmeter were vertically deployed and lowered until about a meter above the bottom. Samples from these vertical tows were filtered using a 200 μm sieve and fixed with ethanol.
Collection and Processing of Phytoplankton and Zooplankton for Stable Isotope Analysis
Phytoplankton for stable isotope analysis were obtained using vertical net tows (20 μm mesh size) deployed near the bottom or a maximum of 50 m. Collected samples were filtered using a 20 μm sieve into amber bottles which were stored in an ice chest until they could be processed on the ship. Zooplankton samples were collected using horizontal and vertical (depth-permitting) tows using 200 μm mesh size nets and a flowmeter. Vertical tows were deployed near the bottom or a maximum of 50 m, while for horizontal tows, the net was lowered just below the water surface and towed for 3 min while the boat was circling immediately around the station. Samples from the tows were filtered through a 200 μm sieve into amber bottles and stored in an ice chest as well. On board the ship, both phytoplankton and zooplankton samples were directly filtered through a pre-combusted GF/F Whatman filter (47 mm diameter) using a filtration manifold with a vacuum pump. The filters were filtered to refusal, folded into half and placed in pre-combusted aluminum foils, and stored in a −80°C freezer until further analysis.
Chemical and Chlorophyll Analysis
Phosphate, silicate, and nitrite concentrations were determined colorimetrically using the methods from Strickland and Parsons (1972) and Jones (1984) with the use of a UV-Vis spectrometer (Shimadzu UV Mini 1240, Japan). NOx (nitrate and nitrite) concentrations were measured using a modified shaking technique to reduce nitrate to nitrite using cadmium granules from Jones (1984), and then detected using the colorimetric method. Chlorophyll a (chl a) samples were analyzed fluorometrically using a Trilogy® laboratory fluorometer following the Intergovernmental Oceanographic Commission (Protocols for the Joint Global Ocean Flux Study (JGOFS), 1994).
Microscopy
Phytoplankton samples were analyzed under the HPO of a Carl Zeiss Axiovert 25 inverted microscope. One ml aliquot from each sample bottle was obtained using a micropipette and decanted onto a Sedgewick Rafter slide. Initially, the slide was viewed under the scanner objective to have an overview of the cell density of the sample. The cell counts were performed thrice per sample bottle, and were averaged for data analysis and interpretation. Identification was made up to the genus level based on Tomas (1997). Data were expressed as cell density per liter. Phytoplankton counts were averaged across depths per station to obtain cross-reef profiles.
Before microscopy, the zooplankton samples from the vertical tows were diluted to a volume of 200 ml and split using a Folsom plankton splitter. The zooplankton to be subsampled was poured into the splitter and rotated slowly back and forth. Internal partitions divided the samples into equal fractions. One half of the sample portion, approximately 100 ml, was placed in a petri dish with grids etched on the bottom, and fully counted. Counting was done using a stereomicroscope. Identification was made up to the order level, except for the groups of fish larvae, nauplius, polychaete worms and unidentified eggs. Data were expressed as individuals per cubic meter.
Stable Isotope Analysis
The phytoplankton and mesozooplankton samples for stable isotope analysis were dried at 60°C, rinsed with dilute HCl and ultrapure water, then dried again at 60°C. The samples were recovered from GF/F filters by scraping and were ground into a fine powder using an agate mortar and pestle before analysis. These were weighed and wrapped in tin capsules.
Measurement of bulk carbon and nitrogen isotopes in both phytoplankton and mesozooplankton samples were performed using a continuous flow IRMS (Delta V Advantage, Thermoscientific) interfaced with an elemental analyzer (ECS 4010, Costech, Inc.). The relative abundance of carbon and nitrogen isotopes were calculated using pre-calibrated amino acid standards (Tayasu et al., 2011): Glycine (δ13C = −34.92) and L-Threonine (δ13C = −9.45) for carbon and DL-Alanine (δ15N = −2.89) and L-Alanine (δ15N = 22.71) for nitrogen.
Stable isotope ratios were reported as delta (δ) notation:
where R is 15N/14N or 13C/12C. Isotope ratios are expressed in per mil (‰) relative to the ratio of international reference standards (Rstandard) which are Atmospheric Nitrogen and Vienna PeeDee Belemnite (VPDB) for nitrogen and carbon, respectively (Perkins et al., 2014).
Statistical Analysis
Principal Coordinate Analysis (PCoA) was used to analyze the multivariate distribution of the phytoplankton and mesozooplankton compositions, while Canonical Correspondence Analysis (CCA) was used to analyze the influence of environmental variables on the phytoplankton and mesozooplankton assemblages. The data were first transformed into distance measures using the Bray-Curtis method for the PCoA (Legendre and Anderson, 1999; Legendre and Legendre, 2012). For the CCA, data were standardized using the Hellinger method (Legendre and Legendre, 2012). Spearman’s correlation was employed to look at correlations and potential interactions between the phytoplankton and mesozooplankton taxa. Only taxa that were observed in at least half of the sampling stations per transect were included in the analysis. ANOVA was used to determine if there were any differences in the phytoplankton δ13C and δ15N between reef transects, followed by Tukey HSD to tease out specific differences. Data were assessed for conformity to the assumptions of normality and homogeneity of variances. The differences of the carbon and nitrogen isotopic values between the mesozooplankton and phytoplankton were calculated by obtaining the average δ13C and δ15N of the phytoplankton per transect or per reef zone, then subtracting the corresponding mesozooplankton average δ13C and δ15N for that transect or reef zone. All statistical analysis were done using R through the base package, as well as the vegan package.
Results
Water Column Conditions
The physico-chemical section profiles for the Pag-asa Island and Sabina Shoal transects are shown in Figure 2 in terms of temperature, salinity, density, chl a, phosphate (PO4), silicate (SiO3), and nitrate and nitrite (NOx). Generally, warm, stratified areas were evident in all transects with temperature values ranging from 28.76 to 31.70°C (Figure 2 and Supplementary Table S2). Salinity ranged from 33.32 to 33.73 psu. The Sabina Shoal site, though, showed much stronger stratification across the transect due to very warm surface waters and sharp thermocline (Figures 2C,F,I).
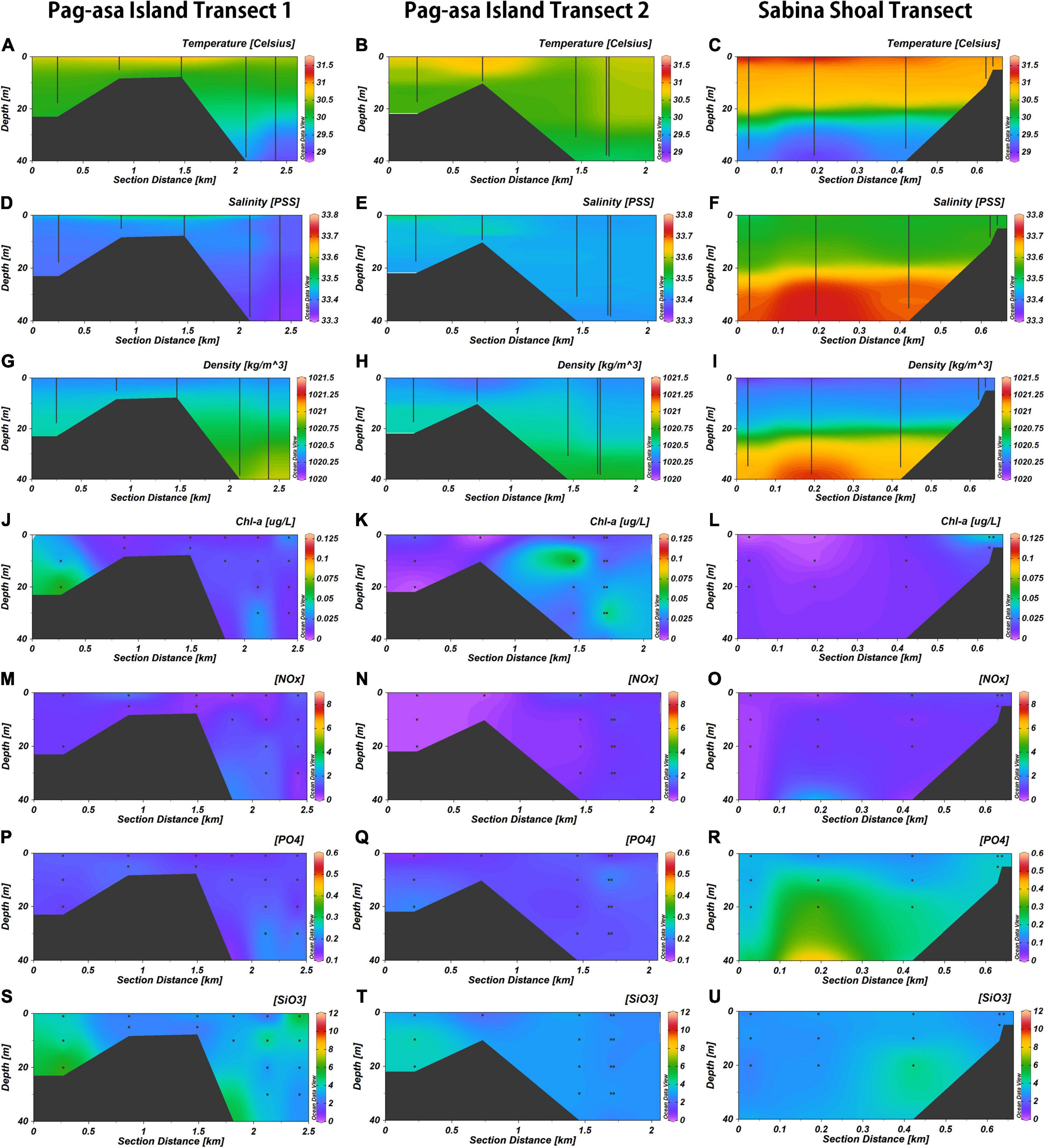
Figure 2. Section plots of the three transects Pag-asa Transect 1 (A,D,G,J,M,P,S), Pag-asa Transect 2 (B,E,H,K,N,Q,T), and Sabina Transect (C,F,I,L,O,R,U) with contour plots for temperature, salinity, density, chlorophyll a (chl a), phosphate (PO4), nitrate and nitrite (NOx), and silicate (SiO3) for each transect.
Phytoplankton biomass is considered to be generally low in waters surrounding coral reef ecosystems, characterized by typical concentrations of chl a at approximately 0.2–0.6 μg/L (Nakajima et al., 2016) owing to a low concentration of inorganic nutrients (Hearn et al., 2001). San Diego-McGlone et al. (1999) observed a maximum of 0.66 in their South China Sea sites. In the present study, relatively lower chl a values were observed with a maximum of 0.13 μg/L, where the Pag-asa transects (PT1 and PT2) had slightly higher average concentrations, and larger areas with higher concentrations compared to Sabina Shoal (Figures 2J,K,L and Supplementary Table S2). These high chl a concentrations at PT1 and PT 2 were found in the subsurface to deeper waters at the forereef and offshore zones. Relatively high chl a concentrations were also observed in the lagoon of PT1. The high chl a levels in Sabina Shoal were observed near the surface at the forereef zone. In general, nutrient concentrations ranged from 0.12 to 0.59 μM for phosphate, 1.93 to 11.92 μM for silicate, and 0.17 to 8.65 μM for NOx. Overall, all three transects tended to be depleted in nutrients at the surface, which increased going deeper (Figures 2M–U). There were some specific differences, though, between the three. Pag-asa transect 1 and Sabina Shoal had relatively higher nutrient concentrations than Pag-asa transect 2. These were situated in deeper areas offshore and even extending toward shallower depths in some offshore areas as at the forereef slopes. In contrast, Pag-asa transect 2 had lower phosphate and NOx concentrations, and did not exhibit any distinct increase at the forereef zone (Figure 2 and Supplementary Table S2). Silicate was also moderately high at the lagoon side of the two Pag-asa transects (Figures 2S,T,U and Supplementary Table S2).
Plankton Assemblages Across Reef Zones and Transects
A total of 48 phytoplankton genera belonging to 33 families were observed across the transects (Supplementary Table S3). Family Bacillariaceae was by far the most genera-abundant group, with four recorded genera each. Family Hemiaulaceae and Rhizosoleniaceae each had 3 genera, whereas families Chaetocerataceae, Dinophysaceae, Fragilariaceae, Skeletonemaceae, Thalassionemataceae, Thalassiosiraceae, and Triceratiaceae were represented by 2 genera each. All other remaining phytoplankton families had one representative genus only.
Phytoplankton abundances and assemblages exhibited variations between reef zones as well as between the three transects (Figures 3A, 4A, 5). Back reef and reef flat areas tended to have decreased phytoplankton abundances while those in the lagoon and fore-reef zones had higher abundances (Figure 5 and Supplementary Table S3). In Sabina Shoal, only the back reef and reef flat areas (Stations 1 and 2) were accessible, and these also had lower densities than fore-reef and deeper stations. The two Pag-asa Island transects had more similar phytoplankton compositions wherein diatoms of the Family Chaetoceraceae were the most abundant (dominated by the genus Chaetoceros), followed by cyanobacteria of the Family Phormidiaceae (Trichodesmium), and then diatoms of the Thalassionemataceae family (Thalassionema). Sabina Shoal was distinct from Pag-asa Island with much lower phytoplankton densities (except for the outer forereef), and its phytoplankton composition. The cyanobacteria Trichodesmium was the most dominant in Sabina Shoal, followed by the dinoflagellate Prorocentrum of family Prorocentraceae, and then diatoms of the Leptocylindraceae family (Leptocylindrus). The two axes of the CCA (Figure 4A) explained 47.42% of the variability in the phytoplankton. It highlighted the association of dinoflagellates from the Families Prorocentraceae and also Gonyaulacacea in Sabina Shoal, which exhibited low phytoplankton abundance, low chlorophyll, and higher nutrient concentrations, as also discussed above. Pagasa transects 1 and 2, on the other hand, clustered closer together and were associated more with higher chlorophyll, but relatively lower nutrient concentrations. Phytoplankton composition also appeared to have some variability within reef zones in a transect (Figure 5 and Supplementary Table S3) driven mainly by shifts in the dominance of Chaetoceros and Trichodesmium and increases in the contribution of dinoflagellates like Gymnodinium sp., Prorocentrum sp., and Protoperidinium sp., and other diatom species such as Thalassionema sp., Skeletonema sp., and Leptocylindrus sp., particularly in the lagoon, fore reef and offshore zones.
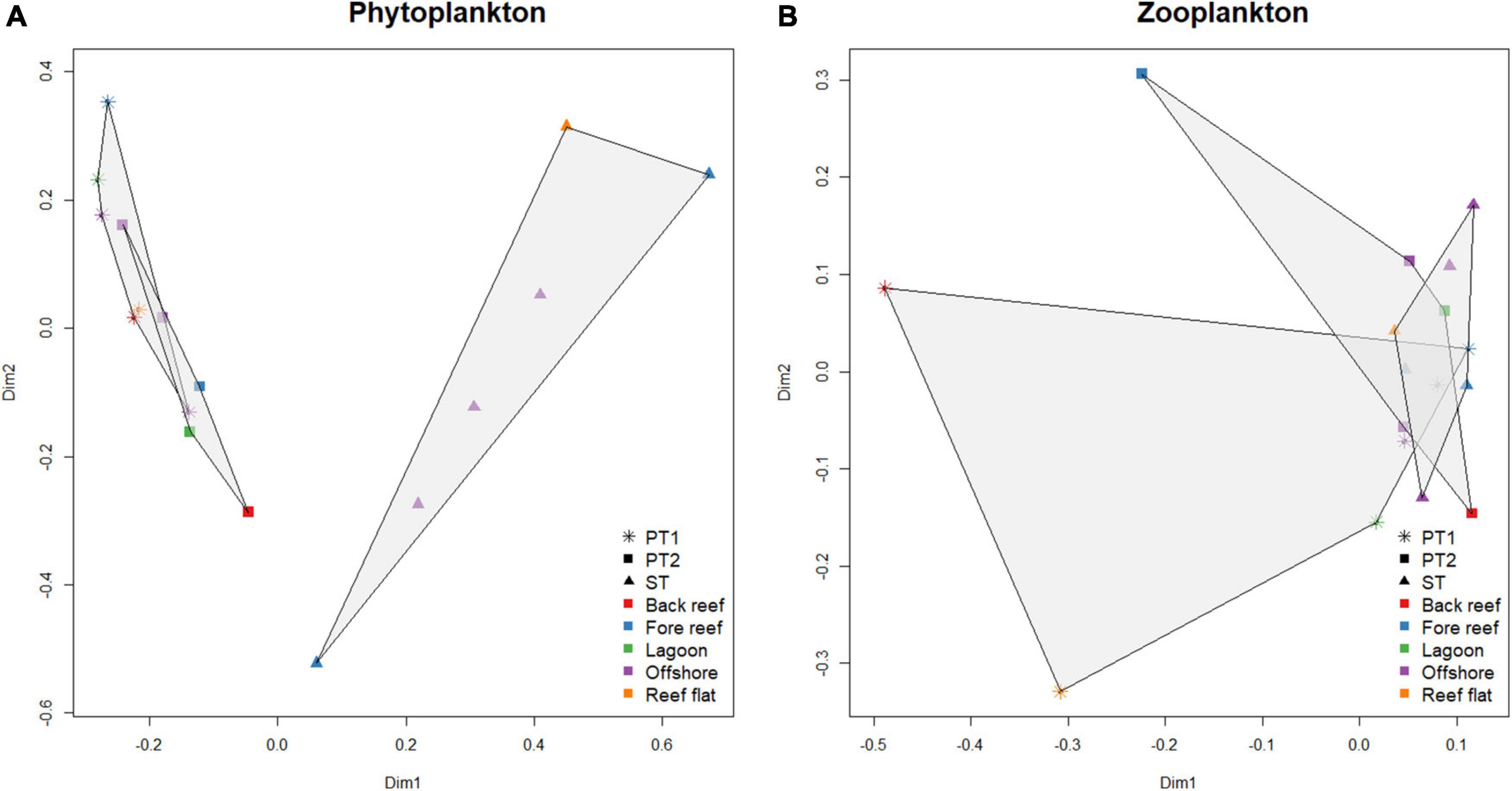
Figure 3. Principal Coordinate Analysis of the stations based on phytoplankton (A) and zooplankton (B) assemblages. Transects are represented by different symbols, with the gray areas showing the dispersion of one transect. The colors of the symbols represent the reef zone.
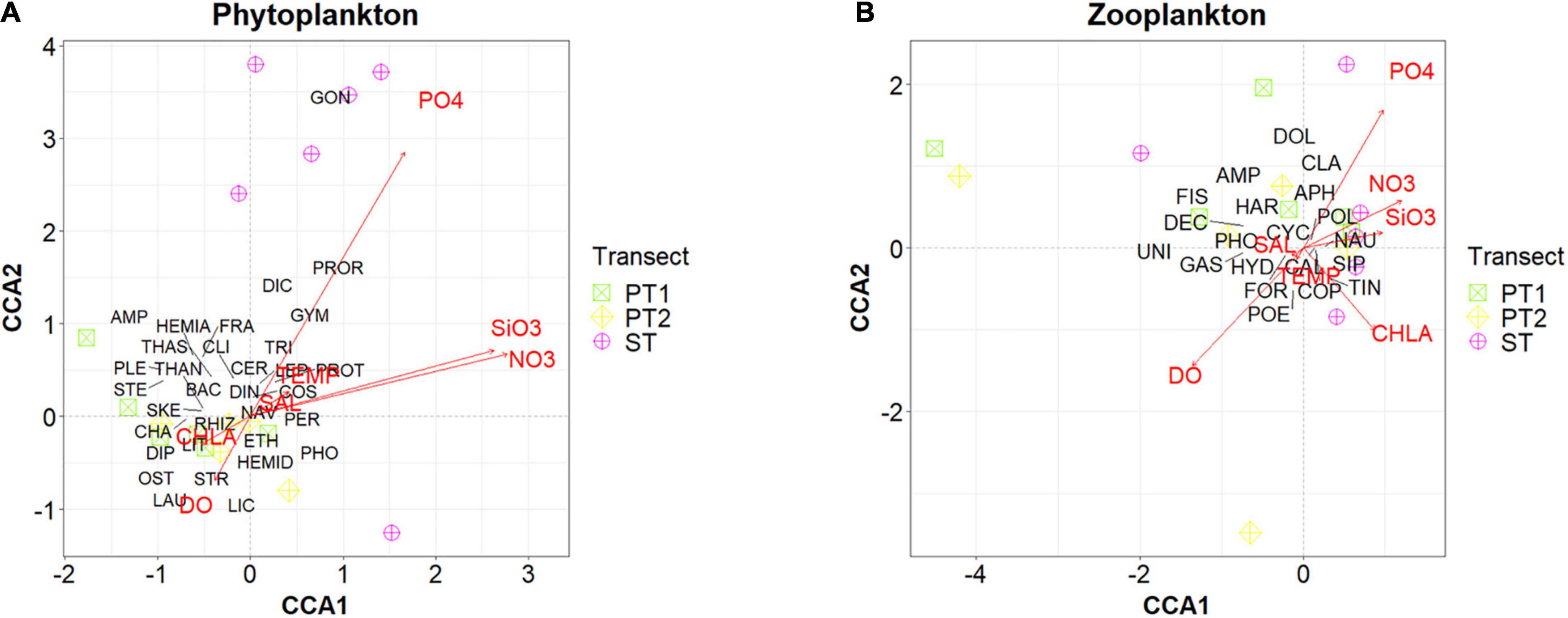
Figure 4. Canonical Correspondence Analysis of the transects based on phytoplankton (A) and zooplankton (B) assemblages constrained by physico-chemical parameters. Transects are represented by different symbols. Text labels represent taxa. For phytoplankton taxa - AMP, Amphisoleniaceae; BAC, Bacillariaceae; CER, Ceratiaceae; CHA, Chaetocerotaceae; CLI, Climacospheniaceae; COS, Coscinodiscaceae; DIC, Dictyochaceae; DIN, Dinophysaceae; DIP, Diploneidaceae; ETH, Ethmodiscaceae; FRA, Fragilariaceae; GON, Gonyaulacaceae; GYM, Gymnodiniaceae; HEMIA, Hemiaulaceae; HEMID, Hemidiscaceae; LAU, Lauderiaceae; LEP, Leptocylindraceae; LIC, Licmophoraceae; LIT, Lithodesmiaceae; NAV, Naviculaceae; OST, Ostreopsidaceae; PER, Peridiniaceae; PHO, Phormidiaceae; PLE, Pleurosigmataceae; PROR, Prorocentraceae; PROT, Protoperidiniaceae; RHIZ, Rhizosoleniaceae; SKE, Skeletonemaceae; STE, Stephanopyxidaceae; STR, Striatellaceae; THAN, Thalassionemataceae; THAS, Thalassiosiraceae; TRI, Triceratiaceae. For zooplankton taxa - AMP, Amphipoda; APH, Aphragmophora; CAL, Calanoida; CLA, Cladocera; COP, Copelata; CYC, Cyclopoida; DEC, Decapoda; DOL, Doliolida; FIS, Fish larvae; FOR, Forcipulatida; GAS, Gastropoda; HAR, Harpacticoida; HYD, Hydrozoa; NAU, Nauplius; PHO, Phoronida; POE, Poecilomastoida; POL, Polychaete worm; SIP, Siphonophora; TIN, Tintinnida; UNI, Unidentified egg.
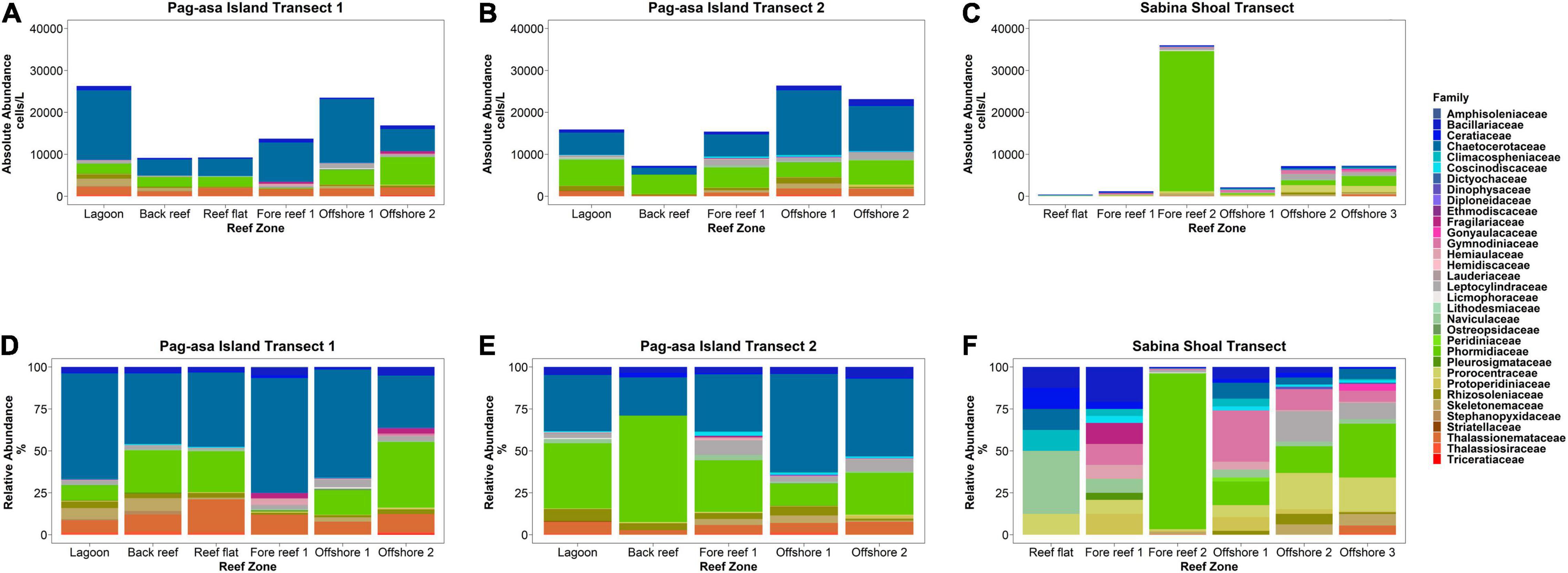
Figure 5. Phytoplankton absolute (A,B,C) and relative (D,E,F) abundances across the reef systems of Pag-asa Island and Sabina Shoal.
A total of 20 zooplankton orders were observed across the transects. Zooplankton abundances exhibited more variability within and between transects (Figures 3B, 4B, 6 and Supplementary Table S4) compared to phytoplankton. Zooplankton abundance tended to be higher in the inner reef areas compared to offshore, opposite the pattern observed for phytoplankton. Abundance was also the highest overall for Sabina Shoal and lowest for Pag-asa transect 1. Zooplankton composition had more overlaps between all three transects (Figure 3B) and was relatively more similar compared to patterns for the phytoplankton. The three transects were generally dominated by copepods (including their nauplii) under the orders Cyclopoida and Calanoida. Pag-asa Transect 2 and the Sabina transect had high abundances of copepods from the order Poecilostomatoida, while in Pag-asa transect 1 mesozooplankton under class Gastropoda were abundant. The CCA axes (Figure 4B) was able to explain 31.63% of the variance in zooplankton. It again highlighted the higher overlap in the mesozooplankton community composition across transects though there were variabilities in the environmental variables (Figure 4B). Though there are similarities between transects, the compositions still showed differences across reef zones where more types of mesozooplankton (e.g., fish larvae, Doliolida, Amphipoda, Hydrozoa) were found in the lagoon and/or back reef zones for the two Pag-asa transects, and offshore for Sabina transect (Figure 6 and Supplementary Table S4).
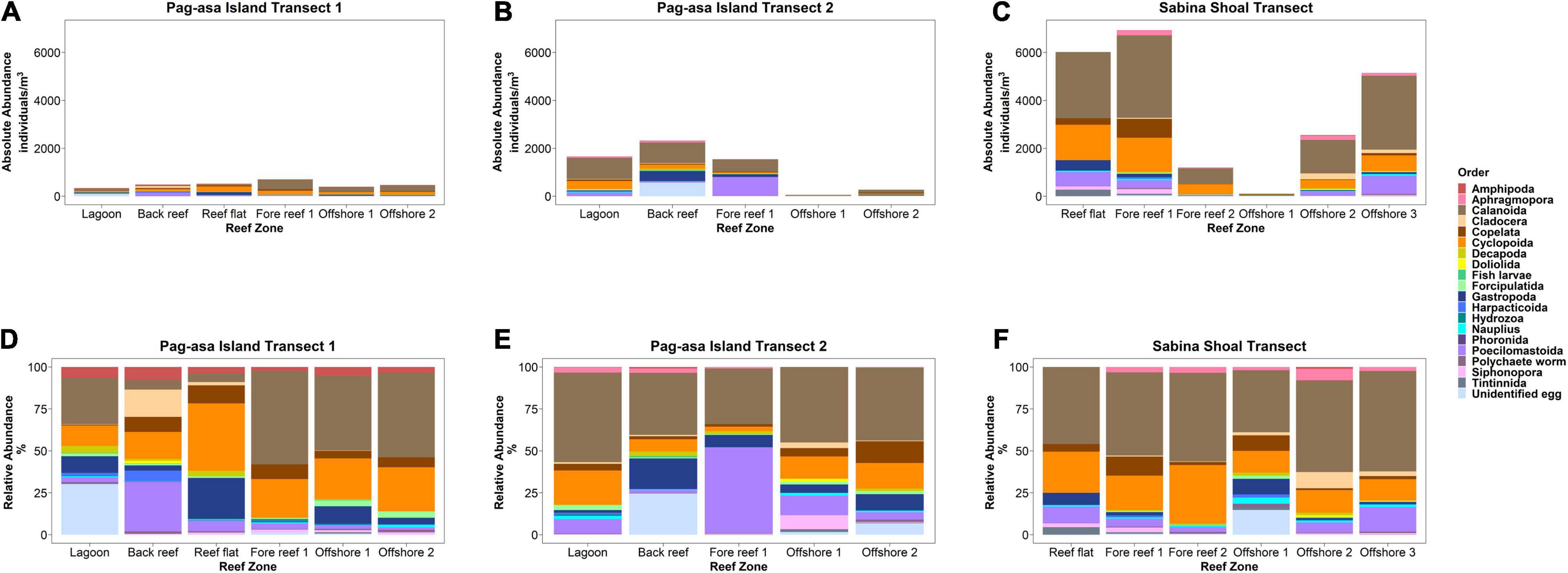
Figure 6. Zooplankton absolute (A,B,C) and relative (D,E,F) abundances across the reef systems of Pag-asa Island and Sabina Shoal.
The potential interactions between phytoplankton and mesozooplankton were evaluated per transect using Spearman correlation (Figure 7). There were only a few significant associations, and out of these, all showed a negative correlation between particular phytoplankton and mesozooplankton. In Pag-asa transect 1, four significant negative associations were observed: Cyclopoids and Skeletonema, Harpacticoids and Thalassionema, Poecilomastoida and Protoperidinium, and Siphonophore and Skeletonema. For Pag-asa transect 2, seven significant negative associations were observed, namely between Aphragmopora and Coscinodiscus as well as Cylindrotheca, Calanoida and Chaetoceros as well as Cylindrotheca, Decapoda and Bacteriastrum, Gastropoda and Bacteriastrum, and Polychaete worm and Chaetoceros. In the Sabina transect, only two significant negative associations were observed, namely between Cyclopoida and Gymnodinium, and Poecilomastoida and Protoperidinium.
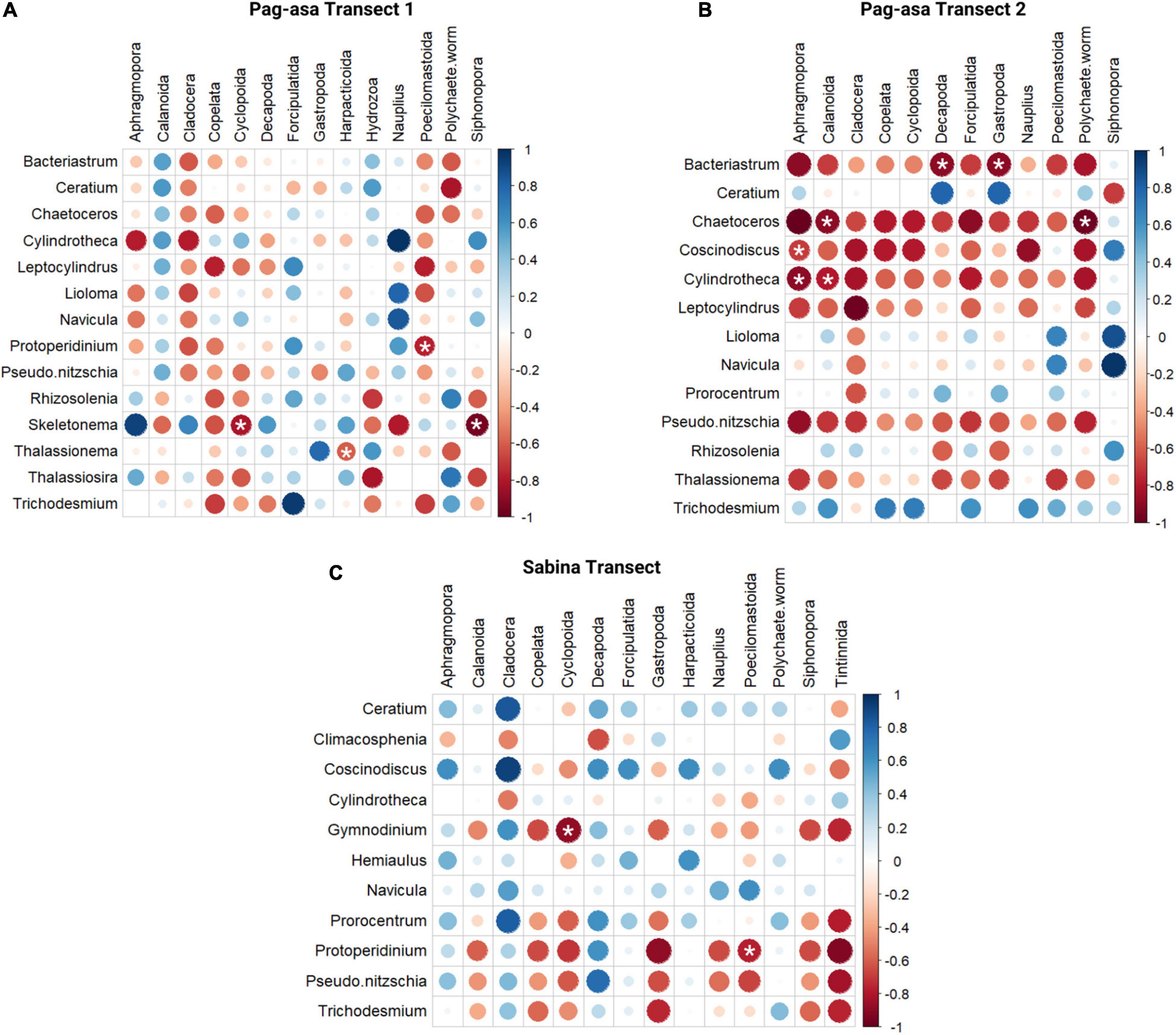
Figure 7. Spearman’s correlation between phytoplankton and zooplankton taxa per transect: (A) Pag-asa Transect 1, (B) Pag-asa Transect 2, and (C) Sabina Transect. Circles with a white asterisk are the only ones that are significant at p < 0.05.
Plankton Stable Isotope Analysis
The δ13C values obtained for the phytoplankton ranged from −23.9 to −17.5‰ (Table 1). δ15N values of phytoplankton were variable ranging from 3.1 to 11.4‰. There were no significant differences in the mean δ13C between transects; however, there were significant differences for mean δ15N. The two Pag-asa transects were significantly different from each other (p = 0.0002), as well as Pag-asa transect 2 and Sabina transect (p = 0.0135). There was no difference between Pag-asa transect 1 and Sabina transect (p = 0.0988) and both had higher δ15N values than Pag-asa transect 2.
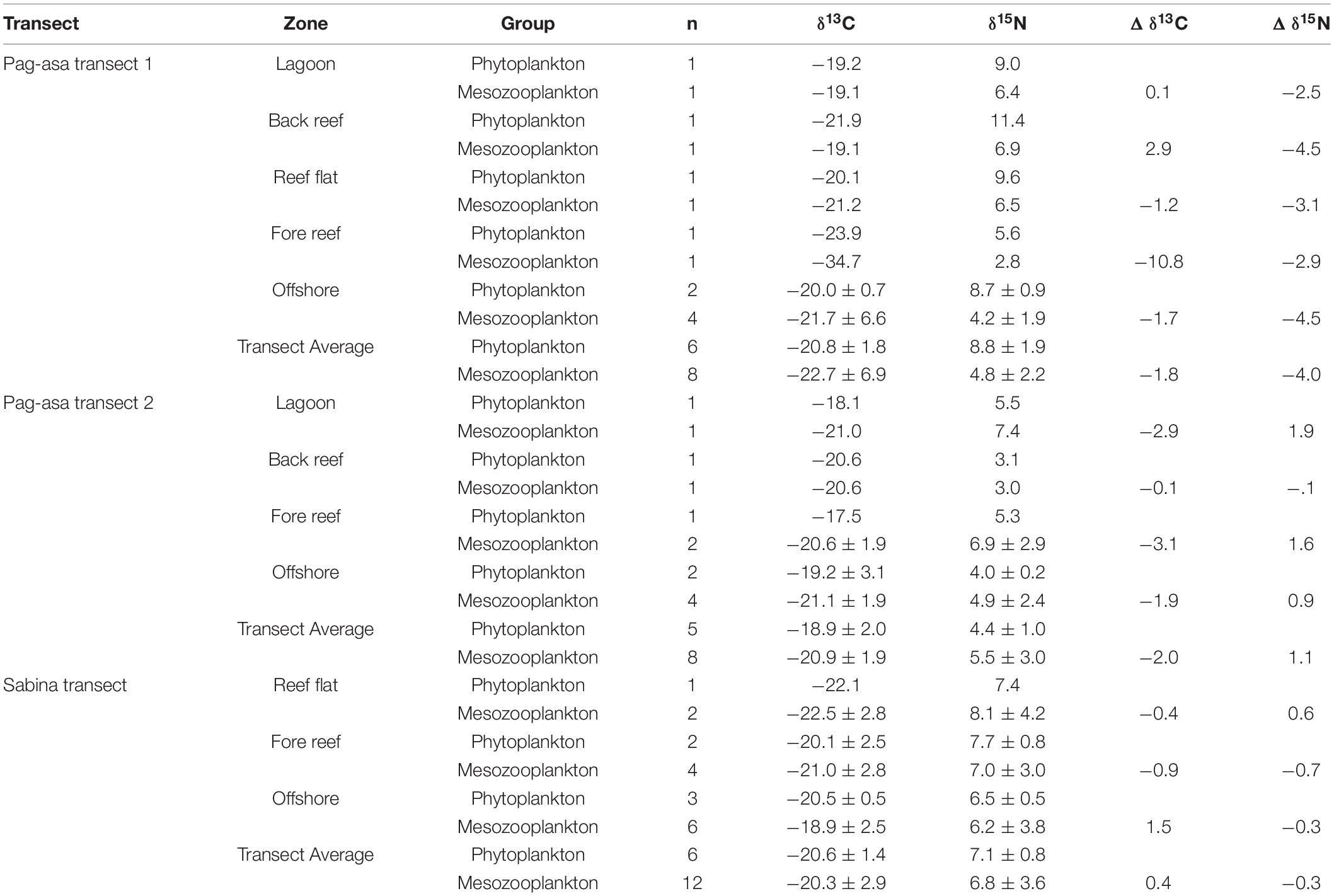
Table 1. Phytoplankton and mesozooplankton isotope values of δ13C and δ15N (mean ± SD; ‰), sample size and average differences in δ13C and δ15N between mesozooplankton relative to phytoplankton per zone or transect.
The mesozooplankton, on average in the Pag-asa transects, appeared to have relatively depleted 13C compared to the phytoplankton within each transect, while the Sabina Shoal transect showed slight enrichment (Table 1). There can be large variability though across reef zones. Within the Sabina transect, the offshore zone were enriched, while 15C values were slightly depleted in other zones. Within the Pag-asa transect 1, the enriched areas were the lagoon and back reef zones. All zones in Pag-asa transect 2 were consistently depleted. For δ15N of mesozooplankton relative to the corresponding putative phytoplankton δ15N per transect, only Pag-asa transect 2 on average appears to exhibit 15N enrichment by 1.1‰ (Table 1). The Sabina transect mesozooplankton was only slightly depleted especially compared to the Pag-asa transect 1 mesozooplankton. There are also a few variabilities across reef zones, where the reef flat in the Sabina transect was slightly enriched. Conversely, only the back reef in Pag-asa transect 2 was depleted. The biplots with convex hulls for phytoplankton and mesozooplankton in Figure 8 provide an indication of the breadth of their sources of nutrients and food. Pag-asa transect 1 and Sabina transect mesozooplankton potentially have other C sources apart from the phytoplankton in the transect. Pag-asa transect 2 mesozooplankton appears to have a less diverse source of food, which is more depleted than the phytoplankton. The larger range of δ15N in Sabina followed by Pag-asa transect 2 suggests that mesozooplankton in these areas have a wide range of feeding types, especially compared to the smaller range of Pag-asa transect 1.

Figure 8. δ15N: δ13C biplot with convex hulls per transect: (A) Pag-asa Island Transect 1, (B) Pag-asa Island Transect 2, and (C) Sabina Shoal Transect. Symbols represent different reef zones. Blue-yellow hulls represent phytoplankton samples, while brown hulls represent mesozooplankton.
Discussion
Variability of Plankton Across the Reefs
Coral reefs are dynamic environments influenced by tides and waves, yet distinct patterns in the plankton across the scale of the reef zones can be distinguished. The phytoplankton abundance and assemblage showed a distinct pattern of variation from offshore to the reef or lagoon side. Phytoplankton were abundant offshore, becomes depleted in the fore-reef and reef flat areas that harbor the most benthic and associated organisms, then increases again in the reefs with lagoons. This pattern was robust across the three reefs investigated. This supports the concept of the “wall of mouths” where plankton are removed from the water column by planktivores aggregated in the fore-reef area (Hamner et al., 1998; Morais and Bellwood, 2019). Benthic and suspension feeders are also potential consumers of phytoplankton in reefs in addition to planktivorous fish; these include bivalves (Klumpp et al., 1992), gastropods (Lesser et al., 1992), and polychaetes (Vedel and Riisgard, 1993). Previous observations such as by Glynn (1973) in a Caribbean coral reef, Moriarty et al. (1985) in Lizard Reef, GBR, Sorokin (1991) in Vietnam, and Wyatt et al. (2010) in Ningaloo Reef, highlight that the filtering out of phytoplankton and microbial communities from flowing water across reefs can be commonly seen. The lagoon areas typically have longer residence times, are deeper, and have fewer organisms, thus providing an environment where the phytoplankton can accumulate (Lowe and Falter, 2015; Pagano et al., 2017). This cross-reef pattern indicates that input of allochthonous phytoplankton from offshore can occur within different coral reefs, and can be one of the mechanisms by which reefs here in the West Philippine Sea obtain and retain nutrients in these low nutrient environments.
Interestingly, the mesozooplankton pattern was different from that of the phytoplankton. They were relatively more abundant in the fore reef and reef flat zones, but their distribution was also more variable. This can be due to differences in the planktivores and benthic suspension feeders present in the reefs. Different taxa feed on particular components of the plankton. Tunicates, sponges, and molluscs primarily incorporate dissolved organic matter (DOM), bacteria, and phytoplankton, whereas cnidarians also ingest zooplankton (Gili and Coma, 1998). Glynn (1973) also observed that diatoms were 31% more efficiently filtered out of the water column than zooplankton in a Puerto Rican reef. The distribution of mesozooplankton, particularly those of reef-associated or resident zooplankton, is also likely mediated by their swimming behavior (Emery, 1968; Ohlhorst, 1982). Increased abundances of zooplankton at the surface of a shallow back reef in Moorea, French Polynesia were attributed to their upward swimming behavior (Alldredge and King, 2009).
Potential Trophic Relationships Between Phytoplankton and Mesozooplankton
The C isotopic signature across the three reefs was similar even if there were differences in the phytoplankton assemblages between Sabina Shoal and Pag-asa Island. These differences were not enough to shift the δ13C signatures, possibly since all three generally had abundant diatoms and cyanobacteria. There do appear to be variations in the nitrogen source for the reefs even within Pag-asa Island. Pag-asa Island transect 1 and Sabina Shoal have more enriched δ15N that is potentially due to uptake of NOx from deeper upwelled waters (Loick et al., 2007; Michener and Kaufman, 2007; Henschke et al., 2015; Kürten et al., 2016). These transects had distinctly higher nutrient concentrations at the deeper portions up to the fore reef slope and mid-water column. Although for the Sabina Shoal transect, the stronger stratification possibly still limited the concentration, thus cell abundances were low. The more depleted δ15N signature of Pag-asa transect 2 could then be due to the greater contribution of recycled nitrogen in the form of ammonium or urea under more oligotrophic conditions (Loick et al., 2007; Henschke et al., 2015).
There is a wide range of isotopic signatures observed in coral reefs even within the Pacific. For phytoplankton or POM, values from −22 to −18 δ13C are measured on average (e.g., Yamamuro et al., 1995; Letourneur et al., 2013; Wyatt et al., 2013; Briand et al., 2015; and Fey et al., 2021), while for zooplankton values of −20.5 to −13 δ13C on average (e.g., Yamamuro et al., 1995; Wyatt et al., 2013; and Fey et al., 2021). The δ15N values are even wider at −0.14 for Trichodesmium at a New Caledonian reef (Briand et al., 2015) through 15 for phytoplankton at Marquesas (Fey et al., 2021). For zooplankton, δ15N values ranging from about 5 to 17 on average have been observed on Pacific reefs (Yamamuro et al., 1995; Wyatt et al., 2013; Fey et al., 2021). The values obtained in this study lie within these typical observed values.
Mesozooplankton in the studied reefs likely have other food sources apart from phytoplankton since most of their δ13C were depleted relative to the phytoplankton, and there were only a few signs of potential grazing interactions between them based on the correlations. The carbon isotopic composition of consumers is typically within ± 1‰ of their food source, with a small (about 0.5-1‰) enrichment (Peterson and Fry, 1987; Michener and Kaufman, 2007). Only three stations showed some level of 13C enrichment where some areas in Pag-asa transect 1 and Sabina could have mesozooplankton more directly linked to the phytoplankton through the grazing pathway. For the mesozooplankton in other areas and Pag-asa transect 2 in particular, the microbial and detrital pathways could be the main sources of food. Smaller components of the plankton (i.e., nanoplankton and picoplankton) have been found to significantly contribute to the productivity of coral reefs (Sorokin, 1995; Ferrier-Pagès and Gattuso, 1998; Wyatt et al., 2010). Mesozooplankton have broad and flexible feeding strategies. Picoplankton are grazed by mesozooplankton such as cladocerans (Lipej et al., 1997), rotifers (Sanders et al., 1989), barnacle larvae and larvaceans (Scheinberg et al., 2005; Vargas et al., 2006), and appendicularians (Gorsky et al., 1999). Pico-sized prey is generally viewed as inefficiently ingested by most mesozooplankton, including copepods, as exhibited by experiments using natural phytoplankton assemblages and culture set-ups (Frost, 1972), since they cannot be effectively retained by the grazer’s feeding appendages (Kiørboe, 2011). However, field and laboratory studies have recently shown direct consumption of substantial concentrations of picoplankton by mesozooplankton (Wilson and Steinberg, 2010; Zhao et al., 2020). For instance, diet analysis exhibited the gut presence of Synechococcus in zooplankters, even in the presence of abundant alternative food sources (Motwani and Gorokhova, 2013). Picoplankton can form aggregates with detrital material and bacteria, thereby increasing accessibility to consumers (Heinle et al., 1977), and reports have now indicated that large zooplankton can also ingest relatively large quantities of picoplankton in the form of micro-colonies and loose agglomerates (Cruz and Neuer, 2019). Nanoplankton such as nanoflagellates are grazed on by marine cladocerans and veliger larvae (Katechakis and Stibor, 2004; Vargas et al., 2006). A mesocosm experiment by Uitto and Hällfors (1997) allowed the investigation of zooplankton grazing on nanoplankton. Meso- (copepods and cladocerans) and microzooplankton (mostly copepod nauplii) were able to ingest 3–70% and 1–6% of nanoplankton daily, with specific rates varying between 3 and 9% for mesozooplankton, and from 4 to 130% for microzooplankton. Increasing evidence from more recent research also revealed detrital remains of macrophytes and terrestrial plants can likewise contribute to organic matter and consequently subsidize the zooplankton diet (Harfmann et al., 2019; Tang et al., 2021). In this study, these smaller plankton were not sampled but could be contributing to the more depleted 13C signature (Kukert and Riebesell, 1998; Rolff, 2000) as part of the microbial pathway. The other likely food source for zooplankton on these reefs is detrital material from dead plants, animals, feces, and other non-living organic material. The contribution of detritus to particulate organic carbon (POC) from different coral reefs in the Pacific range from 52 to 88% on average (Nakajima et al., 2017). In a coral reef in Okinawa, Japan, Nakajima et al. (2017) calculated the role of grazing and microbial food webs in sustaining the mesozooplankton community at an average of 38.7% and 37.2%, respectively, with the remaining amount filled in by detritus. These proportional contributions varied depending on the season, and in this study, the influence of these pathways could also shift depending on the reef zone and system.
The variations we have observed across and between reefs have been associated with the observed covariation in physico-chemical parameters, and possible biological interactions. However, other physical factors that we were unable to measure can also possibly influence the phytoplankton and mesozooplankton patterns. Local-scale circulation and the consequent advection of plankton can also contribute to their retention or dispersal in specific areas of the reef, and was, as mentioned above, one possible factor in the higher phytoplankton abundances observed in the lagoons (Lowe and Falter, 2015; Pagano et al., 2017). Tidal currents can impact the patterns of plankton abundances in reefs (Hamner et al., 2007). In this study though, we were unable to track the tides and sample across a spectrum of tides due to logistical constraints. We recommend that future work conduct longer observations, if possible, preferably with fixed stations across the reefs monitored for a variety of physico-chemical parameters, including hydrodynamic conditions. This would allow better resolution of the roles of different factors on the phytoplankton and zooplankton distributions and interactions.
Coral reefs are sentinel ecosystems that provide us with a slew of ecosystem services. Unfortunately, they are highly threatened by global and local stressors, most especially within the Coral Triangle biodiversity hotspot (Burke et al., 2012). Understanding the functioning and productivity of these ecosystems are important if we want to conserve better and manage them. Recent work has highlighted the significant role of pelagic subsidies in coral reef fish productivity (Skinner et al., 2021), despite declines in coral cover and lower topography (Morais and Bellwood, 2019). This also points to the potential influence of larger-scale oceanographic productivity variations on reefs. There has been very limited information in the Philippines, an archipelagic country, on the coupling of benthic reefs with the surrounding pelagic waters and tracing such trophic pathways. In this study, we present new information on the interaction of pelagic waters with offshore reefs through the phytoplankton and mesozooplankton communities. Spatially distinct patterns were apparent across reef zones and reef systems, likely mediated by water column conditions, filter-feeding of reef organisms as waters flow across the reefs, and mesozooplankton behavior. The phytoplankton on these reefs are also influenced by the different nutrient sources present. The trophic pathways for mesozooplankton in the reefs are possibly a combination of the grazing, microbial and detrital pathways, given that the stable isotope signatures and correlation analysis show limited signals from the direct grazing of the phytoplankton. More comprehensive sampling of different food web components and environmental conditions at different time points together with bulk and/or compound-specific stable isotopes (McMahon et al., 2016) would help provide a clearer picture of these trophic relationships. This first look into the interaction of pelagic plankton with coral reefs in the Philippines highlights the need to further explore the significance of pelagic contributions to the benthos across the different areas of the country, from offshore atolls to fringing reefs.
Data Availability Statement
The raw data supporting the conclusions of this article will be made available by the authors, without undue reservation.
Author Contributions
AY, GJ, and GA conceived of and designed the study. AY, GA, NG, and GJ conducted the field surveys. NM, GA, and NG conducted the laboratory analysis. AY, GA, NM, and NG analyzed the data and prepared the figures and tables. All authors contributed to writing and revising the manuscript.
Funding
This study was funded by the Philippine Department of Environment and Natural Resources through the program entitled “Coastal Assessment for Rehabilitation Enhancement: Capability Development and Resiliency of Ecosystems (CARE-CaDRES)”. The Marine Science Institute, University of the Philippines provided partial support for the publication fee through the project entitled “Upgrading capacity, infrastructure, and assets for marine scientific research in the Philippines”.
Conflict of Interest
The authors declare that the research was conducted in the absence of any commercial or financial relationships that could be construed as a potential conflict of interest.
Publisher’s Note
All claims expressed in this article are solely those of the authors and do not necessarily represent those of their affiliated organizations, or those of the publisher, the editors and the reviewers. Any product that may be evaluated in this article, or claim that may be made by its manufacturer, is not guaranteed or endorsed by the publisher.
Acknowledgments
We thank Cesar Villanoy and the Physical Oceanography laboratory for organizing the research cruise, the Philippine Navy and the BRP Gregorio Velasquez (AGR 702) for the help in sample collection during the research expedition conducted in the Kalayaan Group of Islands in 2017. We also thank the Department of Science and Technology – Philippine Nuclear Research Institute for the collaboration in conducting the stable isotope analyses, John Kristoffer Q. Andres for identifying the zooplankton samples, and John Michael N. Aguilar for analyzing the samples for chemical parameters.
Supplementary Material
The Supplementary Material for this article can be found online at: https://www.frontiersin.org/articles/10.3389/fmars.2021.724504/full#supplementary-material
References
Aliño, P. M., and Quibilan, M. C. C. (2003). The Kalayaan Islands: Our Natural Heritage. Marine Science Institute. Quezon City: University of the Philippines.
Alldredge, A. L., and King, J. M. (2009). Near-surface enrichment of zooplankton over a shallow back reef: implications for coral reef food webs. Coral Reefs 28, 895–908. doi: 10.1007/s00338-009-0534-4
Arceo, H. O., Cabasan, J. P., Luciano, R. M. A., Heyres, L. J. D., Mamauag, S. S., and Aliño, P. M. (2020). Estimating the potential fisheries production of three offshore reefs in the West Philippine Sea, Philippines. Philipp. J. Sci. 149, 647–658.
Basedow, S. L., De Silva, N. A. L., Bode, A., and Van Beusekorn, J. (2016). Trophic positions of mesozooplankton across the North Atlantic: estimates derived from biovolume spectrum theories and stable isotope analyses. J. Plankton Res. 38, 1364–1378. doi: 10.1093/plankt/fbw070
Basedow, S. L., Tande, K. S., and Zhou, M. (2009). Biovolume spectrum theories applied: spatial patterns of trophic levels within a mesozooplankton community at the polar front. J. Plankton Res. 32, 1105–1119. doi: 10.1093/plankt/fbp110
Briand, M. J., Bonnet, X., Goiran, C., Guillou, G., and Letourneur, Y. (2015). Major sources of organic matter in a complex coral reef lagoon: identification from isotopic signatures (δ13C and δ15N). PLoS One 10:e0131555. doi: 10.1371/journal.pone.0131555
Burke, L., Reytar, K., Spalding, M., and Perry, A. (2012). Reefs At Risk Revisited in the Coral Triangle. Washington, DC: World Resources Institute.
Carpenter, J. H. (1965). The chesapeake bay institute technique for the winkler dissolved oxygen method. Limnol. Oceanogr. 10, 141–143. doi: 10.4319/lo.1965.10.1.0141
Cruz, B. N., and Neuer, S. (2019). Heterotrophic bacteria enhance the aggregation of the marine picocyanobacteria Prochlorococcus and Synechococcus. Front. Microbiol. 10:1864. doi: 10.3389/fmicb.2019.01864
Emery, A. R. (1968). Preliminary observations on coral reef plankton 1. Limnol. Oceanogr. 13, 293–303.
Ferrier-Pagès, C., and Gattuso, J. P. (1998). Biomass, production and grazing rates of pico-and nanoplankton in coral reef waters (Miyako Island. Japan). Microb. Ecol. 35, 46–57. doi: 10.1007/s002489900059
Fey, P., Parravicini, V., Bãnaru, D., Dierking, J., Galzin, R., Lebreton, B., et al. (2021). Multi-trophic markers illuminate the understanding of the functioning of a remote, low coral cover Marquesan coral reef food web. Sci. Rep. 11, 1–14.
Frost, B. W. (1972). Effect of size and concentration of food particles on the feeding behavior of the marine planktonic copepod Calanus finmarchicus. Limnol. Oceanogr. 17, 805–815. doi: 10.4319/lo.1972.17.6.0805
Fry, B., and Sherr, E. B. (1984). δ13C Measurements as indicators of carbon flow in marine and freshwater ecosystems. Contrib. Mar. Sci. 27, 13–47.
Genin, A., Monismith, S. G., Reidenbach, M. A., Yahel, G., and Koseff, J. R. (2009). Intense benthic grazing of phytoplankton in a coral reef. Limnol. Oceanogr. 54, 938–951. doi: 10.4319/lo.2009.54.3.0938
Giering, S. L., Wells, S. R., Mayers, K. M. J., Schuster, H., Cornwell, L., Fileman, E. S., et al. (2019). Seasonal variation of zooplankton community structure and trophic position in the Celtic Sea: a stable isotope and biovolume spectrum approach. Prog. Oceanogr. 177:101943. doi: 10.1016/j.pocean.2018.03.012
Gili, J. M., and Coma, R. (1998). Benthic suspension feeders: their paramount role in littoral marine food webs. Trends Ecol. Evol. 13, 316–321. doi: 10.1016/s0169-5347(98)01365-2
Glynn, P. W. (1973). Ecology of a Caribbean Reef. The Porites reef flat biotope. II. Plankton community with evidence for depletion. Mar. Biol. 22, 1–21. doi: 10.1007/bf00388905
Gorsky, G., Chre ìtiennot-Dinet, M. J., Blanchot, J., and Palazzoli, I. (1999). Picoplankton and nanoplankton aggregation by appendicularians: Fecal pellet contents of Megalocercus huxleyi in the equatorial Pacific. J. Geophys. Res. Oceans 104, 3381–3390.
Gottfried, M., and Roman, M. R. (1983). Ingestion and incorporation of coral-mucus detritus by reef zooplankton. Mar. Biol. 72, 211–218. doi: 10.1007/bf00396825
Gruber, R. K., Lowe, R. J., and Falter, J. L. (2018). Benthic uptake of phytoplankton and ocean-reef exchange of particulate nutrients on a tide-dominated reef. Limnol. Oceanogr. 63, 1–17. doi: 10.1002/lno.10790
Hamner, W. M., Colin, P. L., and Hamner, P. P. (2007). Export-import dynamics of zooplankton on a coral reef in Palau. Mar. Ecol. Prog. Ser. 334, 83–92. doi: 10.3354/meps334083
Hamner, W. M., Jones, M. S., Carleton, J. H., Hauri, I. R., and Williams, D. (1998). Zooplankton, planktivorous fish, and water currents on a windward reef face: great Barrier Reef, Australia. Bull. Mar. Sci. 42, 459–479.
Harfmann, J., Kurobe, T., Bergamaschi, B., Teh, S., and Hernes, P. (2019). Plant detritus is selectively consumed by estuarine copepods and can augment their survival. Sci. Rep. 9:9076. doi: 10.1038/s41598-019-45503-6
Hearn, C., Atkinson, M., and Falter, J. (2001). A physical derivation of nutrient-uptake rates in coral reefs: effects of roughness and waves. Coral Reefs 20, 347–356.
Heinle, D. R., Harris, R. P., Ustach, J. F., and Flemer, D. A. (1977). Detritus as food for estuarine copepods. Mar. Biol. 40, 341–353. doi: 10.1007/bf00395727
Henschke, N., Everett, J. D., Suthers, I. M., Smith, J. A., Hunt, B. P. V., Doblin, M. A., et al. (2015). Zooplankton trophic niches respond to different water types of the western Tasman Sea: a stable isotope analysis. Deep Sea Res. I 104, 1–8. doi: 10.1016/j.dsr.2015.06.010
Hu, J., Kawamura, H., Hong, H., and Qi, Y. (2000). A review on the currents in the South China Sea: seasonal circulation, South China Sea warm current and Kuroshio intrusion. J. Oceanogr. 56, 607–624. doi: 10.1023/A:1011117531252
Jones, M. N. (1984). Nitrate reduction by shaking with cadmium: alternative to cadmium columns. Water Re. 18, 643–646. doi: 10.1016/0043-1354(84)90215-X
Katechakis, A., and Stibor, H. (2004). Feeding selectivities of the marine cladocerans Penilia avirostris, Podon intermedius and Evadne nordmanni. Mar. Biol. 145, 529–539.
Kiørboe, T. (2011). How zooplankton feed: mechanisms, traits and trade-offs. Biol. Rev. 86, 311–339. doi: 10.1111/j.1469-185X.2010.00148.x
Klumpp, D. W., Bayne, B. L., and Hawkins, A. J. S. (1992). Nutrition of the giant clam Tridacna gigas (L.). 1. Contribution of filter feeding and photosynthates to respiration and growth. J. Exp. Mar. Biol. Ecol. 155, 105–122.
Kukert, H., and Riebesell, U. (1998). Phytoplankton carbon isotope fractionation during a diatom spring bloom in a Norwegian fjord. Mar. Ecol. Prog. Ser. 173, 127–138.
Kürten, B., Al-Aidaroos, A. M., Kürten, S., El-Sherbiny, M. M., Devassy, R. P., Struck, U., et al. (2016). Carbon and nitrogen stable isotope ratios of pelagic zooplankton elucidate ecohydrographic features in the oligotrophic Red Sea. Prog. Oceanogr. 140, 69–90. doi: 10.1016/j.pocean.2015.11.003
Legendre, P., and Anderson, M. J. (1999). Distance-based redundancy analysis: testing multispecies responses in multifactorial ecological experiments. Ecol. Monogr. 69, 1–24.
Leichter, J. J., Alldredge, A. L., Bernardi, G., Brooks, A. J., Carlson, C. A., Carpenter, R. C., et al. (2013). Biological and physical interactions on a tropical island coral reef: transport and retention processes on Moorea, French Polynesia. Oceanography 26, 52–63. doi: 10.5670/oceanog.2013.45
Lesser, M. P. (2004). Experimental biology of coral reef systems. J. Exp. Mar. Biol. Ecol. 300, 217–252. doi: 10.1016/j.jembe.2003.12.027
Lesser, M. P., Shumway, S. E., Cucci, T., and Smith, J. (1992). Impact of fouling organisms on mussel rope culture: interspecific competition for food among suspension-feeding invertebrates. J. Exp. Mar. Biol. Ecol. 165, 91–102. doi: 10.1016/0022-0981(92)90291-h
Letourneur, Y., De Loma, T. L., Richard, P., Harmelin-Vivien, M. L., Cresson, P., Banaru, D., et al. (2013). Identifying carbon sources and trophic position of coral reef fishes using diet and stable isotope (δ 15 N and δ 13 C) analyses in two contrasted bays in Moorea, French Polynesia. Coral Reefs 32, 1091–1102.
Lipej, L., Mozetic, P., Turk, V., and Malej, A. (1997). The trophic role of the marine cladoceran Penilia avirostris in the Gulf of Trieste. Hydrobiologia 360, 197–203.
Loick, N., Dippner, J., Doan, H. N., Liskow, I., and Voss, M. (2007). Pelagic nitrogen dynamics in the Vietnamese upwelling area according to stable nitrogen and carbon isotope data. Deep Sea Res. Part I 54, 596–607. doi: 10.1016/j.dsr.2006.12.009
Lowe, R. J., and Falter, J. L. (2015). Oceanic forcing of coral reefs. Annu. Rev. Mar. Sci. 7, 43–66. doi: 10.1146/annurev-marine-010814-015834
Mayal, E. M., Neumann-Leitao, S., Feitosa, F., Schwamborn, R., Silva, T., and da Silva-Cunha, M. (2009). Hydrology, plankton, and corals of the Maracajaú reefs (Northeastern Brazil) - an ecosystem under severe thermal stress. Braz. Arch. Biol. Technol. 52, 665–678. doi: 10.1590/S1516-89132009000300019
McMahon, K. W., Thorrold, S. R., Houghton, L. A., and Berumen, M. L. (2016). Tracing carbon flow through coral reef food webs using a compound-specific stable isotope approach. Oecologia 180, 809–821. doi: 10.1007/s00442-015-3475-3
Michener, R. H., and Kaufman, L. (2007). Stable isotope ratios as tracers in marine food webs: an update. Stable Isotopes Ecol. Environ. Sci. 2, 238–282. doi: 10.1002/9780470691854.ch9
Morais, R. A., and Bellwood, D. R. (2019). Pelagic subsidies underpin fish productivity on a degraded coral reef. Curr. Biol. 29, 1521–1527. doi: 10.1016/j.cub.2019.03.044
Morales, A. R., and Murillo, M. M. (1996). Distribution, abundance and composition of coral reef zooplankton, Cahuita National Park, Limon, Costa Rica. Rev. Biol. Trop. 44, 619–630.
Moriarty, D. J. W., Pollard, P. C., and Hunt, W. G. (1985). Temporal and spatial variation in bacterial production in the water column over a coral reef. Mar. Biol. 85, 285–292.
Morillo-Velarde, P. S., Briones-Fourzan, P., Alvarez-Filip, L., Aguiniga-Garcia, S., Sanchez-Gonzalez, A., and Lozano-Alvarez, E. (2018). Habitat degradation alters trophic pathways but not food chain length on shallow Caribbean coral reefs. Nat. Sci. Rep. 8, 1–12. doi: 10.1038/s41598-018-22463-x
Motwani, N. H., and Gorokhova, E. (2013). Mesozooplankton grazing on picocyanobacteria in the Baltic Sea as inferred from molecular diet analysis. PLoS One 8:e79230. doi: 10.1371/journal.pone.0079230
Nakajima, R., Nakatomi, N., Kurihara, H., Fox, M. D., Smith, J. E., and Okaji, K. (2016). Crown-of-thorns starfish larvae can feed on organic matter released from corals. Diversity 8:18.
Nakajima, R., Yamazaki, H., Lewis, L., Khen, A., Smith, J., Nakatomi, N., et al. (2017). Planktonic trophic structure in a coral reef ecosystem – grazing versus microbial food webs and the production of mesozooplankton. Prog. Oceanogr. 156, 104–120. doi: 10.1016/j.pocean.2017.06.007
Ohlhorst, S. L. (1982). Diel migration patterns of demersal reef zooplankton. J. Exp. Mar. Biol. Ecol. 60, 1–15. doi: 10.1016/0022-0981(81)90176-3
Ong, P. S. Afuang, L. E., and Rosell-Ambal, R. G. (eds.) (2002). Philippine Biodiversity Conservation Priorities: A Second Iteration of the National Biodiversity Strategy and action Plan. Quezon City: Department of Environment and Natural Resources, 113.
Pagano, M., Rodier, M., Guillaumot, C., Thomas, Y., Henry, K., and Andréfouët, S. (2017). Ocean-lagoon water and plankton exchanges in a semi-closed pearl farming atoll lagoon (Ahe, Tuamotu archipelago, French Polynesia). Estuar. Coast. Shelf Sci. 191, 60–73. doi: 10.1016/j.ecss.2017.04.017
Perkins, M. J., McDonald, R. A., van Veen, F. J. F., Kelley, S., Rees, G., and Bearhop, S. (2014). Application of nitrogen and carbon stable isotopes (δ 15N and δ 13C) to quantify food chain length and trophic structure. PLoS One 9:e0093281. doi: 10.1371/journal.pone.0093281
Peterson, B. J., and Fry, B. (1987). Stable isotopes in ecosystem studies. Annu. Rev. Ecol. Syst. 18, 293–320.
Popp, B. N., Graham, B. S., Olson, R. J., Hannides, C. C. S., Lott, M. J., Lopez-Ibarra, G. A., et al. (2007). “Insight into the trophic ecology of yellowfin tuna, Thunnus albacares, from compound-specific nitrogen isotope analysis of proteinaceous amino acids,” in Stable Isotopes as Indicators of Ecological Change, eds T. Dawson and R. Siegwolf (Amsterdam: Elsevier Academic Press), 173–190. doi: 10.1016/s1936-7961(07)01012-3
Post, D. M. (2002). Using stable isotopes to estimate trophic position: models, methods, and assumptions. Ecology 83, 703–718. doi: 10.1111/j.1095-8649.2012.03251.x
Protocols for the Joint Global Ocean Flux Study (JGOFS) (1994). Core Measurements. Intergovernmental Oceanographic Commission. Scientific Committee on Oceanic Research, Manual and Guides 29. Paris: JGOFS, 97–100.
Rolff, C. (2000). Seasonal variation in δ13C and δ15N of size-fractionated plankton at a coastal station in the northern Baltic proper. Mar. Ecol. Prog. Ser. 203, 47–65.
Roman, M. R., Furnas, M. J., and Mullin, M. M. (1990). Zooplankton abundance and grazing at Davies Reef, Great Barrier Reef, Australia. Mar. Biol. 105, 73–82. doi: 10.1007/bf01344272
San Diego-McGlone, M. L., Jacinto, G. S., Dupra, V. C., Narcise, I. S., Padayao, D. O., and Velasquez, I. B. (1999). A comparison of nutrient characteristics and primary productivity in the Sulu Sea and South China Sea. Acta Oceanogr. Taiwanica 37, 219–229.
Sanders, R. W., Porter, K. G., Bennet, S. J., and DeBiase, A. E. (1989). Seasonal patterns of bacterivory by flagellates, ciliates, rotifers and cladocerans in a freshwater plankton community. Limnol. Oceanogr. 34, 673–687. doi: 10.4319/lo.1989.34.4.0673
Scheinberg, R. D., Landry, M. R., and Calbet, A. (2005). Grazing of two common appendicularians on the natural prey assemblage of a tropical coastal ecosystem. Mar. Ecol. Prog. Ser. 294, 201–212. doi: 10.3354/meps294201
Skinner, C., Mill, A. C., Fox, M. D., Newman, S. P., Zhu, Y., Kuhl, A., et al. (2021). Offshore pelagic subsidies dominate carbon inputs to coral reef predators. Sci. Adv. 7, 1–13. doi: 10.1126/sciadv.abf3792
Sorokin, Y. I. (1991). Parameters of productivity and metabolism of coral reef ecosystems off central Vietnam. Est. Coast Shelf Sci. 33, 259–280. doi: 10.1016/0272-7714(91)90056-H
Sorokin, Y. I. (1995). Role of plankton in the turnover of organic matter on the Great Barrier Reef, Australia. Hydrobiologia 308, 35–44. doi: 10.1007/bf00037785
Strickland, J. D. H., and Parsons, T. R. (1972). A Practical Handbook of Seawater Analysis. Fisheries Research Board of Canada Bulletin 167. Ottawa, ON: Fisheries Research Board of Canada.
Tang, Y., Zhou, D., Su, L., Liu, Z., Zhang, X., and Dumont, H. J. (2021). Vallisneria natans detritus supports Daphnia magna somatic growth and reproduction under addition of periphyton. Aquat. Ecol. 55, 579–588. doi: 10.1007/s10452-021-09846-5
Tayasu, I., Hirasawa, R., Ogawa, N. O., Ohkouchi, N., and Yamada, K. (2011). New organic reference materials for carbon-and nitrogen-stable isotope ratio measurements provided by Center for Ecological Research, Kyoto University, and Institute of Biogeosciences, Japan Agency for Marine-Earth Science and Technology. Limnology 12, 261–266. doi: 10.1007/s10201-011-0345-5
Tomas, C. R. (1997). Identifying Marine Phytoplankton. Florida Department of Environmental Protection. St. Petersburg, FL: Florida Marine Research Institute.
Uitto, A., and Hällfors, S. (1997). Grazing by mesozooplankton and metazoan microplankton on nanophytoplankton in a mesocosm experiment in the northern Baltic. J. Plankton Res. 19, 655–673. doi: 10.1093/plankt/19.6.655
Vander Zanden, M. J., Casselman, J. M., and Rasmussen, J. B. (1999). Stable isotope evidence for the food web consequences of species invasions in lakes. Nature 401, 464–467. doi: 10.1371/journal.pone.0211870
Vander Zanden, M. J., and Rasmussen, J. B. (1999). Primary consumer δ13C and δ15N and the trophic position of aquatic consumers. Ecology 80, 1395–1404. doi: 10.1890/0012-9658(1999)080[1395:pccana]2.0.co;2
Vargas, C. A., Manríquez, P. H., and Navarrete, S. A. (2006). Feeding by larvae of intertidal invertebrates: assessing their position in pelagic food webs. Ecology 87, 444–457. doi: 10.1890/05-0265
Vedel, A., and Riisgard, H. U. (1993). Filter-feeding in the polychaete Nereis diversicolor: growth and bioenergetics. Mar. Ecol. Prog. Ser. 100, 145–152. doi: 10.3354/meps100145
Villanoy, C. L., and Jacinto, G. S. (2017). Component 1. Oceanography of KIG in Predicting Responses Between Ocean Transport and Ecological Connectivity of Threatened Ecosystems in the West Philippine Sea (PROTECT WPS) Terminal Report. Quezon City: Marine Science Institute of the University of the Philippines (UP MSI), 137.
Wilson, S. E., and Steinberg, D. K. (2010). Autotrophic picoplankton in mesozooplankton guts: evidence of aggregate feeding in the mesopelagic zone and export of small phytoplankton. Mar. Ecol. Prog. Ser. 412, 11–27. doi: 10.3354/meps08648
Winkler, L. W. (1888). Die Bestimmung des in Wasser gelösten Sauerstoffen. Berichte Deutschen Chem. Gesellschaft 21, 2843–2855.
Wyatt, A. S., Lowe, R. J., Humphries, S., and Waite, A. M. (2010). Particulate nutrient fluxes over a fringing coral reef: relevant scales of phytoplankton production and mechanisms of supply. Mar. Ecol. Prog. Ser. 405, 113–130. doi: 10.3354/meps08508
Wyatt, A. S., Lowe, R. J., Humphries, S., and Waite, A. M. (2013). Particulate nutrient fluxes over a fringing coral reef: source-sink dynamics inferred from carbon to nitrogen ratios and stable isotopes. Limnol. Oceanogr. 58, 409–427. doi: 10.4319/lo.2013.58.1.0409
Wyrtki, K. (1961). The thermohaline circulation in relation to the general circulation in the oceans. Deep Sea Res. 8, 39–64. doi: 10.1016/0146-6313(61)90014-4
Yahel, G., Post, A. F., Fabricius, K. D. M., Vaulot, D., and Genin, A. (1998). Phytoplankton distribution and grazing near coral reefs. Limnol. Oceanogr. 43, 551–563. doi: 10.4319/lo.1998.43.4.0551
Yamamuro, M., Kayanne, H., and Minagawao, M. (1995). Carbon and nitrogen stable isotopes of primary producers in coral reef ecosystems. Limnol. Oceanogr. 40, 617–621.
Zhang, Y., and Yin, K. (2015). “Fish catch and nutrient limitation in the South China Sea,” in Proceedings of the International Conference on Plant, Marine and Environmental Sciences (PMES-2015) Jan. 1-2, 2015 Kuala Lumpur, (Malaysia), doi: 10.15242/IICBE.C0115030
Keywords: benthic-pelagic coupling, plankton trophic dynamics, reef, stable isotope, West Philippine Sea
Citation: Yñiguez AT, Apego GCM, Mendoza N, Gomez NC and Jacinto GS (2022) Nearshore to Offshore Trends in Plankton Assemblage and Stable Isotopes in Reefs of the West Philippine Sea. Front. Mar. Sci. 8:724504. doi: 10.3389/fmars.2021.724504
Received: 13 June 2021; Accepted: 27 December 2021;
Published: 25 January 2022.
Edited by:
Yehuda Benayahu, Tel Aviv University, IsraelReviewed by:
Kusum Komal Karati, Centre for Marine Living Resources and Ecology (CMLRE), IndiaVladimir G. Dvoretsky, Murmansk Marine Biological Institute, Russia
Copyright © 2022 Yñiguez, Apego, Mendoza, Gomez and Jacinto. This is an open-access article distributed under the terms of the Creative Commons Attribution License (CC BY). The use, distribution or reproduction in other forums is permitted, provided the original author(s) and the copyright owner(s) are credited and that the original publication in this journal is cited, in accordance with accepted academic practice. No use, distribution or reproduction is permitted which does not comply with these terms.
*Correspondence: Aletta T. Yñiguez, YXR5bmlndWV6QG1zaS51cGQuZWR1LnBo