- 1Department of Analytical Chemistry, University of Latvia, Riga, Latvia
- 2Marine Biology Station Piran, National Institute of Biology, Piran, Slovenia
- 3UCIBIO - Applied Molecular Biosciences Unit, Associate Laboratory i4HB - Institute for Health and Bioeconomy, Department of Chemistry, NOVA School of Science and Technology, NOVA University of Lisbon, Caparica, Portugal
- 4Scottish Association for Marine Science, Oban, United Kingdom
- 5Department of Molecular Biology and Genetic, Faculty of Science and Arts, Burdur Mehmet Akif Ersoy University, Burdur, Turkey
- 6Møreforsking AS, Ålesund, Norway
- 7Interdisciplinary Centre of Marine and Environmental Research, University of Porto, Matosinhos, Portugal
- 8Department of Engineering, Klaipeda University, Klaipeda, Lithuania
- 9Institute of Oceanography, University of Gdańsk, Gdynia, Poland
- 10Institute of Oceanology, Polish Academy of Sciences, Sopot, Poland
- 11Department of Separation Science, LUT School of Engineering Science, LUT University, Mikkeli, Finland
- 12Latvian State Institute of Wood Chemistry, Riga, Latvia
- 13Department of Environmental Sciences, University of Latvia, Riga, Latvia
- 14Institute of Biomedical Sciences Abel Salazar, University of Porto, Porto, Portugal
- 15ICBM-MPI Bridging Group for Marine Geochemistry, Institute for Chemistry and Biology of the Marine Environment (ICBM), University of Oldenburg, Oldenburg, Germany
- 16Rudolfs Cimdins Riga Biomaterials Innovations and Development Centre, Institute of General Chemical Engineering, Faculty of Materials Science and Applied Chemistry, Riga Technical University, Riga, Latvia
- 17Baltic Biomaterials Centre of Excellence, Riga Technical University, Riga, Latvia
- 18Ege University Application and Research Center for Testing and Analysis (EGE MATAL), İzmir, Turkey
- 19Department of Biology and Environmental Science, Linnaeus University, Kalmar, Sweden
- 20Institute of Environmental Sciences, Bogazici University, Istanbul, Turkey
- 21Waste Laboratory, University of Muhammadiyah Malang, Malang, Indonesia
- 22Laboratory of Forest and Water Resources, Latvia University of Life Sciences and Technologies, Jelgava, Latvia
- 23Section of Pharmacognosy and Chemistry of Natural Products, Department of Pharmacy, National and Kapodistrian University of Athens, Athens, Greece
- 24Department of Urban Studies/Environmental Science, Malmö University, Malmö, Sweden
- 25Marine Research Institute, Klaipėda University, Klaipėda, Lithuania
- 26Estonian Marine Institute, University of Tartu, Tallinn, Estonia
- 27Chair of Rural Building and Water Management, Estonian University of Life Sciences, Tartu, Estonia
- 28Department of Chemical and Environmental Engineering, University of the Basque Country, Donostia-San Sebastián, Spain
- 29Department of Biology, Faculty of Science, University of Sarajevo, Sarajevo, Bosnia and Herzegovina
- 30Institute of Food Science Research, CIAL (CSIC-UAM, CEI UAM+CSIC), Madrid, Spain
- 31Department of Nanobiotechnology, Biology Centre, ISB, CAS, České Budějovice, Czechia
- 32Regional Centre of Advanced Technologies and Materials, Czech Advanced Technology and Research Institute, Palacký University, Olomouc, Czechia
- 33Institute of Agrochemistry and Food Technology, IATA-CSIC, Valencia, Spain
- 34GEOMAR Centre for Marine Biotechnology, Research Unit Marine Natural Products Chemistry, GEOMAR Helmholtz Centre for Ocean Research, Kiel, Germany
- 35Faculty of Mathematics and Natural Sciences, Kiel University, Kiel, Germany
- 36Mycotheca Universitatis Taurinensis, Department of Life Sciences and Systems Biology, University of Torino, Turin, Italy
- 37Institute of Chemistry, University of Tartu, Tartu, Estonia
Biomass is defined as organic matter from living organisms represented in all kingdoms. It is recognized to be an excellent source of proteins, polysaccharides and lipids and, as such, embodies a tailored feedstock for new products and processes to apply in green industries. The industrial processes focused on the valorization of terrestrial biomass are well established, but marine sources still represent an untapped resource. Oceans and seas occupy over 70% of the Earth’s surface and are used intensively in worldwide economies through the fishery industry, as logistical routes, for mining ores and exploitation of fossil fuels, among others. All these activities produce waste. The other source of unused biomass derives from the beach wrack or washed-ashore organic material, especially in highly eutrophicated marine ecosystems. The development of high-added-value products from these side streams has been given priority in recent years due to the detection of a broad range of biopolymers, multiple nutrients and functional compounds that could find applications for human consumption or use in livestock/pet food, pharmaceutical and other industries. This review comprises a broad thematic approach in marine waste valorization, addressing the main achievements in marine biotechnology for advancing the circular economy, ranging from bioremediation applications for pollution treatment to energy and valorization for biomedical applications. It also includes a broad overview of the valorization of side streams in three selected case study areas: Norway, Scotland, and the Baltic Sea.
Introduction
Marine biomass represents a biotechnological resource with great diversity in composition and functional properties due to various bioactive compounds, from polyphenols and peptides to polysaccharides. Considering the shortage of terrestrial resources, the constantly increasing and aging population, there is an urgent need to propose alternative sources of food and novel medicine. There is a growing consumer awareness regarding the relationship between diet and health, resulting in elevated demand for new fish products with enhanced nutritional and functional properties (Al Khawli et al., 2019). Moreover, according to the World Health Organization (2021), musculoskeletal conditions are the main contributors that cause disability, thus personalized solutions, methods and materials for tissue regeneration are widely studied. Although often overlooked, marine waste can represent a practical alternative resource to address multiple societal challenges (Chubarenko et al., 2021). This review discusses mainly the state-of-the-art of valorization potential of two sources of marine wastes, (i) marine industries biowaste (focusing on fishery and aquaculture industries), as well as (ii) beach wrack. Indeed, the exploitation of marine biomass and valorization of seafood by-products either directly or by the extraction of biopolymers seems to be a promising alternative, leading to more environmentally sustainable uses of marine resources and higher economic benefits, in line with the circular economy and blue bioeconomy concepts. The realization of such developments is hampered by the lack of appropriate regulatory frames to enable the use of waste and by-products and to ensure the safety, quality, and acceptability of the product.
Beach Wrack
Beach-cast sea wrack or simply beach wrack is an organic material consisting of seagrass or seaweed biomass (Macreadie et al., 2017), various marine invertebrates, as well as human-made litter, mostly plastics (Oliveira et al., 2020), which accumulates on beaches due to the action of waves, tides, and aperiodical water level fluctuations (Suursaar et al., 2014). Despite the natural origin of most of this material and its significant ecological role (Dugan et al., 2003; Orr et al., 2005; Defeo et al., 2009; Nordstrom et al., 2011), beach wrack often becomes a social amenity (Kirkman and Kendrick, 1997; Macreadie et al., 2017) and/or presents environmental issues, if accumulated in excessive amounts (McGwynne et al., 1988; Macreadie et al., 2017). Anthropogenic pressure, such as shoreline reconfiguration (Macreadie et al., 2017), eutrophication (Risén et al., 2017) and climate change (Macreadie et al., 2011), stimulate the accumulation of wrack onshore and multiply the negative impacts on the environment as mentioned above. Likewise, marine eutrophication and climate change do not only affect the accumulation of sea wrack and its degradation, but this may be exacerbated in return by the products of aerobic decomposition as well. It is estimated that the annual global carbon flux from seagrass wrack to the atmosphere is between 1.31 and 19.04 Tg C/yr (Liu et al., 2019). Coordinated collection and valorization of beach wrack could be an intervention to mitigate the eutrophication processes by lowering the nutrient concentrations from the sea as well as lowering the nitrogen to phosphorus ratio. The collection and processing of beach wrack is in line with the European Union (EU) waste law (Regulation, 2008/98/EC), where its recycling is a priority. Importantly, the collection and removal of near-shore beach wrack is associated with estimated costs between 6 and 120 € per ton of disposed wrack or 38 € per meter of beach and an additional 85 € per ton for material drying (Mossbauer et al., 2012; Barbot et al., 2016). Hence, new value chains and business models should be developed to change the perspective of beach wrack from a cost-intensive to a profitable activity. Traditionally, this biomass was either composted (also with fresh-terrestrial green waste, thus contributing to the blue-green innovations) and utilized as a biofertilizer or for biomethane production (Barbot et al., 2016; Weinberger et al., 2020; Borchert et al., 2021). However, there are still unexploited opportunities to maximize its valorization potential in other industries, thus maintaining the circularity of financial and biological resources.
By-Products From Fishery and Aquaculture
According to the Food and Agriculture Organization (FAO), in 2018, about 88% of 179 million tons of total fish production was utilized for direct human consumption, while the remaining 12% was used for non-food purposes (FAO, 2020). Until now, all by-products of fish processing, estimated at up to 75% of the raw material (Rustad et al., 2011), were discarded or used directly as feed, in silage or fertilizers. Such fishery waste includes fish or by-catch species, having low or no commercial value, undersized or damaged commercial species, species of commercial value caught in insufficient amount to warrant a sale, as well as unused fish tissues, including fins, heads, skin and viscera (WRAP, 2012; Caruso, 2016). Nowadays, there is an increasing trend of using these by-products as materials to produce fishmeal and fish oil (currently estimated at 25–35%) (FAO, 2020). Moreover, other aquatic organisms, such as shellfish, seaweeds and aquatic plants, are being increasingly used in experimental projects for the production of food, feed, pharmaceutical and cosmetic products, as well as to produce biomaterials, biofuels and to improve the efficiency of water treatment (Barbot et al., 2016; Nisticò, 2017; Poblete-Castro et al., 2020). Nevertheless, the world’s fisheries discards are still high, exceeding 10%, equivalent to 9.1 million tons of the total production of marine fishery catch (as per 2014 data) and around 5.2 million tons/yr in the European Union (Pérez Roda et al., 2019). In fisheries and aquaculture combined, it is estimated that 35% of the global harvest is either lost or wasted every year (FAO, 2020). Therefore, additional valorization approaches are needed to minimize the amounts of discards and maintain the circularity of resources.
Since January 2019, discards at sea have become an illegal practice in the waters of the EU, increasing the need for a complete valorization of all landed fishery waste, both for large-scale and small fisheries operators. This creates a sociological and environmental imperative for the reduction of these discards and utilization of fishery waste as a potential resource (Rustad et al., 2011; Caruso, 2016). Regulatory frameworks, capacity building, services, infrastructure, as well as physical access to markets are therefore needed to reduce fish loss and waste. The above-mentioned measures can also contribute to improving resource sustainability and food security (FAO, 2020). Furthermore, the need for responsible fisheries and aquaculture practices to help preserve aquatic biodiversity has driven the search for alternative valorization routes for fish waste streams, such as heads, guts, skins, scales, and bones.
General Processing Aspects
When processing beach wrack or fishery by-products, it is crucial to start their processing as early as possible to minimize physical, chemical, and microbial degradation. To preserve the raw materials for as long as possible, chilling, freezing or acidification using organic acids is performed (Rustad et al., 2011). In general, by-products from side streams or waste can be valorized as they are, using appropriate extraction, purification and downstream processing methods (Figure 1). Alternative methods, such as hydrolysis, ensilaging, fermentation and gelation (surimi production from the fish protein fraction) with food-grade enzymes and/or microorganisms have been optimized for extraction and production of concentrated marine oils, functional protein food ingredients and products, as well as pharmaceutical-grade biopolymers and textiles (Kim, 2013; Lopez-Caballero et al., 2014). These methods are easily used to a wide range of industrial applications in the food, nutraceutical or biomedical sectors. This valorization approach favors the circular economy concept, providing an immediate solution for the reuse or recycling of materials. Biobased production of biopolymers can then be coupled with either sourcing the producer organism and its growth in bioreactors or microbial production in heterologous systems to guarantee a sustainable supply of the biopolymers. For example, hyaluronic acid production was demonstrated in Bacillus subtilis, Lactococcus lactis, and Pichia pastoris (de Oliveira et al., 2016; Badri et al., 2019). However, there are still challenges to successfully produce biopolymers using microorganisms, namely the complex regulatory mechanisms and in vivo polymerization. Nevertheless, fine-tuning of the expression of endogenous or heterologous genes has now advanced using molecular techniques, applying inducible and/or controllable genetic switches, such as CRISPR-Cas tools.
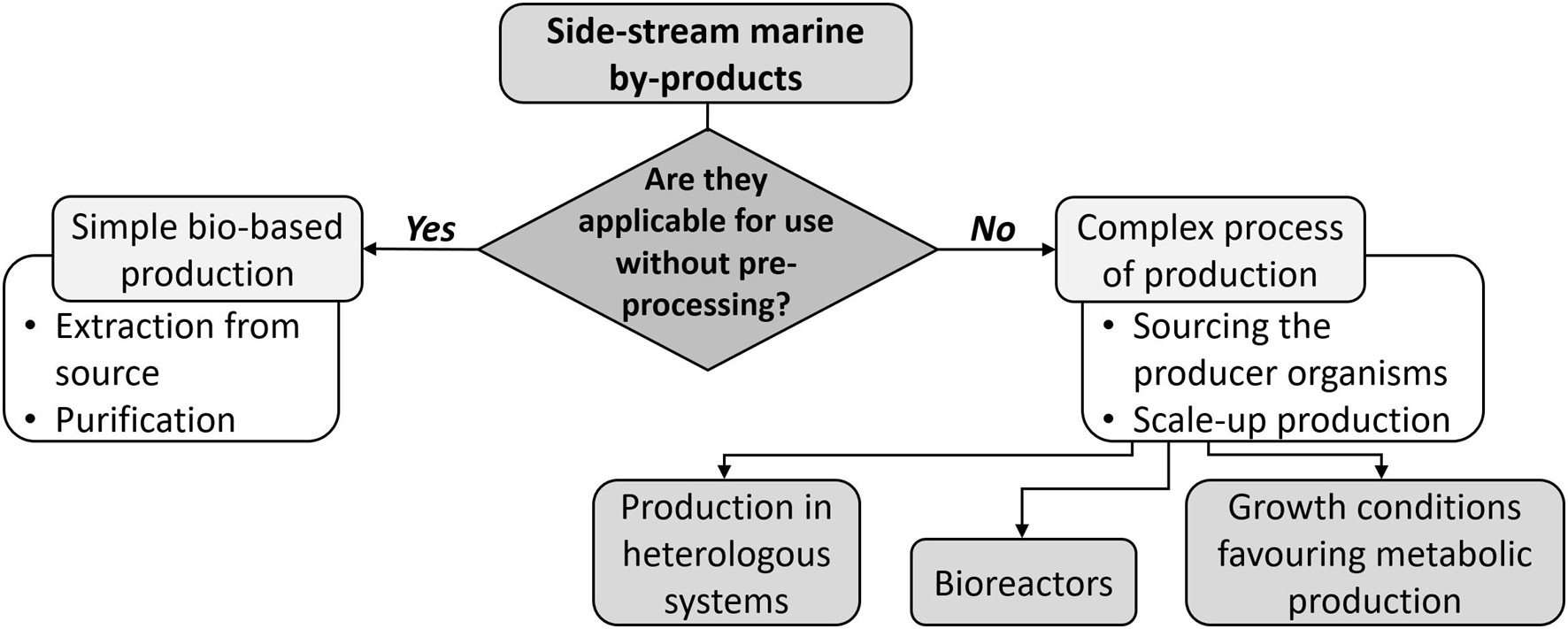
Figure 1. The options for utilization of side-stream marine by-products: simple production or combination of bio-based production and growth of producer species or production in heterologous systems, guaranteeing a sustainable sourcing (in case of bio-based production) or a sustainable supply (in case of the complex process of production) of marine biopolymers.
The remainder of this article is structured as follows. Section “Direct valorization of marine waste biomass” describes the alternatives for direct utilization of marine waste biomass. Section “Valorization of marine biopolymers” presents the potential applications of biopolymers from waste biomass, while the overarching European strategies are depicted in Section “Valorization of marine biomass as a European strategy.” Finally, Section “Case studies” describes selected case studies in Norway, Scotland and the Baltic Sea. These Northern European solutions could serve to provide future transfer of knowledge action plans toward Southern Europe and beyond.
Direct Valorization of Marine Waste Biomass
Biosorbents
The basic definition of biosorption is the removal of various contaminants using materials of biological origin (biomass). It is a process typically independent of energy, employing dead or waste biomass of low cost. Most biological waste materials can be efficiently and directly used as readily available biosorbents for removal of organic and inorganic pollutants and radionuclides from e.g., residual waters (de Freitas et al., 2019; Silva et al., 2019; Beni and Esmaeili, 2020; Fawzy and Gomaa, 2020; Magesh et al., 2020; Ubando et al., 2021; Table 1). Biosorption of pollutants on biosorbents usually includes several mechanisms based on the presence of appropriate functional groups (e.g., hydroxyl, carboxyl, amino, phosphate, sulfate, amide, imidazole, thiol, acetamide, etc.), which can interact with target pollutants. The adsorption efficiency can be increased through appropriate physical or chemical treatments (Bulgariu and Bulgariu, 2016; Safarik et al., 2018). The exhausted biosorbents have to be appropriately treated, including regeneration and reuse of biosorbents in multiple biosorption cycles. The totally exhausted biosorbents can then be used as fertilizers for soil remediation, or to form biochar through pyrolysis (Bãdescu et al., 2018). On the contrary, bioaccumulation employs living (micro-) organisms, and appropriate nutrients must be added continuously. Most pollutants are accumulated inside the cell, and thus the possibility of regeneration and repeated use is very limited (Beni and Esmaeili, 2020).
Seagrasses, macroalgae, as well as microalgae, have been extensively studied as biosorbents for the removal of various aquatic pollutants (e.g., metal ions, dyes, etc.) (Table 1). The algal cell wall is composed of polysaccharides (e.g., alginate), which have multiple functional groups that act as binding sites for metals and other pollutants. Brown algae, in particular, are very efficient biosorbents for the removal of different metal ions from water due to their unique features, such as high alginate content, higher uptake capacities, and their unlimited availability in the oceans (Davis et al., 2003). The biosorption capacity of algae for specific metals depends on several factors, such as the number of binding sites in the algae, the sites’ accessibility, the availability of the site and affinity between the binding site and the metal (Davis et al., 2003). For example, Saldarriaga-Hernandez et al. (2020) reviewed the bioremediation potential of Sargassum sp. in a circular economy approach. Brown marine macroalgae as natural cation exchangers for the removal of toxic metals were also reviewed (Figueira et al., 2000; Davis et al., 2003; Bilal et al., 2018; Mazur et al., 2018). He and Chen (2014) published a comprehensive review of heavy metal biosorption by algal biomass and discussed materials, performances, chemistry and modeling simulation tools. Biomass from marine macroalgae and seagrasses can be obtained in large quantities at a low price, provided that their harvesting is sustainable and does not affect the ecosystem balance in coastal areas. Different species of marine algae have been used to remove various metal ions, colorants (dyes) and other pollutants from water (Sheng et al., 2007; Bhatnagar et al., 2012; Aytas et al., 2014; Navarro et al., 2014; Rathod et al., 2014; Vijayaraghavan et al., 2015; Jerold and Sivasubramanian, 2016; Ungureanu et al., 2016; Mokhtar et al., 2017; Vigneshpriya et al., 2017; Arumugam et al., 2018; Kishore Kumar et al., 2018; Silva et al., 2019; Bouzikri et al., 2020; Coração et al., 2020; Fabre et al., 2020; Safarik et al., 2020a,b; Shobier et al., 2020; Table 1). Also, waste macroalgae biomass obtained after selected industrial processes can be employed for the same purposes (Safarik et al., 2018; Santos et al., 2018). Several commercially available biosorbents, such as Alga SORBTM, Bio-recovery Systems Inc., and B.V. Sorbex use marine algae. Despite the ease of the algal biosorption process, the technology is not yet recognized, and it requires further research and development efforts at a larger scale using industrial effluents.
Some studies attempted to modify algae to enhance their biosorption (Volesky, 2003), but this has not been considered favorable since it may increase the cost, both for the treatment onside and of the polluted biosorbent afterward, without always improving the sorption capacity. Moreover, active sorption sites are sometimes destroyed due to chemical treatment, resulting in lower biosorption than the untreated algal biosorbent. Despite these discouraging drawbacks, research is still progressing to modify specific properties of biosorbents to increase the biosorption capacities or simplify their recovery. An interesting strategy employs magnetic modification to obtain smart biomaterials, exhibiting several types of responses to an external magnetic field. Such materials can be easily and selectively separated from desired environments using permanent magnets, electromagnets or appropriate magnetic separators (Kanjilal and Bhattacharjee, 2018; Safarik et al., 2018; Hassan et al., 2020). Thus, magnetically responsive marine-derived biosorbents can find significant applications, especially for removing various inorganic and organic pollutants from wastewater. Several procedures can be employed for magnetic modification of marine-based biomass. Usually, magnetic iron oxide nano- and microparticles (Laurent et al., 2008) are used as magnetic labels for marine biomass modification (Safarik et al., 2016b, 2018, 2020a,b). Magnetically modified Sargassum horneri biomass was employed to remove several organic dyes (Angelova et al., 2016), while Sargassum swartzii modified with nanoscale zero-valence iron particles was used for crystal violet biosorption (Jerold et al., 2017). The brown alga Cystoseira barbata coated with magnetite particles was used for the removal of methylene blue from aqueous solution (Ozudogru et al., 2016), while the tropical marine green calcareous alga Cymopolia barbata, magnetically modified with microwave-synthesized iron oxide nano- and microparticles, was used for the removal of safranin O (Mullerova et al., 2019). The marine seagrass Posidonia oceanica was magnetically modified using three procedures and subsequently used to remove seven water-soluble organic dyes (Safarik et al., 2016a; Table 1).
Biochar is an intensively studied carbon-based material produced by pyrolysis of biomass in the absence of oxygen. The study of Macreadie et al. (2017) provided clear evidence that the conversion of beach wrack to biochar could be a viable environmental solution for dealing with unwanted wrack, offsetting carbon emissions and providing a commercially valuable product for agriculture and wastewater and its sludge treatment. The use of macroalgae biomass for biochar (charcoal) production, with energy co-generation potential, provides a value-driven model to sequester carbon and recycle nutrients (Bird et al., 2011). In specific cases, macroalgal biomass can be used as biochar and magnetic biochar precursor, as shown in several cases using brown or green macroalgae (Jung et al., 2016; Son et al., 2018a, b; Foroutan et al., 2019; Jung et al., 2019; Yang et al., 2019). The prepared magnetic biochars were employed as efficient adsorbents of acetylsalicylic acid, water-soluble organic dyes and copper ions (Jung et al., 2019).
Biofuels
Almost 80% of the world’s energy supply is provided by fossil fuels (Balachandar et al., 2013). Energy demands are increasing worldwide due to industrialization, population growth and modernization, leading to the over exploitation of limited available natural fossil fuel reserves (Kumar and Thakur, 2018; Kumar et al., 2020). These fuels represent a significant threat to the environment due to their greenhouse gases (GHG) emissions, which are the main cause of global warming. This stimulates the research on bioenergy production from biomass (Kumar and Thakur, 2018; Karkal and Kudre, 2020). Biofuel and related technologies are considered renewable alternatives to fossil-based fuels due to their sustainable features to overcome the global energy demand (Klavins et al., 2018). As biomass production can be quite expensive to meet the energy needs alone, energy production from waste biomass with the biorefinery approach is alternatively used. Waste biorefineries are attracting significant interest worldwide as sustainable waste management solutions (Khoo et al., 2019). In this case, both required energy needs are met, and a solution to the waste management problem is found in the circular economy context (Tuck et al., 2012; Ahrens et al., 2017).
Production of the first-generation of biofuels is mainly based on the biomass of terrestrial plants, such as corn, soybean, sugar cane, palm oil, among others (Chen et al., 2017; Yu and Tsang, 2017; Shuba and Kifle, 2018). However, their utilization also creates ecosystem damage, water shortage and food vs. fuel debate. Considering the problems related to the first-generation of biofuels, the second- and the third- generations have become alternative options, which are respectively produced from waste materials (plant and agricultural waste, municipal sludge) and microorganisms, without disrupting the environment and natural resources (Kumar et al., 2016, 2018; Shuba and Kifle, 2018).
The incorporation of wastewater treatment with microalgae for biofuel production has both environmental and economic benefits. In this process, microalgae are used as biosorbents before biofuel production. Different conversion technologies are used for the production of biofuels (Figure 2), such as biochemical – anaerobic digestion (biogas) and fermentation (bioethanol), and chemical conversion – extraction and transesterification (biodiesel) (Chen et al., 2015; Sikarwar et al., 2017; Kumar et al., 2020). In addition, several non-fermentation options for the production of energy from macroalgae are available, including direct combustion (heat energy), gasification (syngas for heat and power generation, liquefaction and production of hydrogen) and pyrolysis (production of liquid bio-oil, syngas and charcoal) (Bruhn et al., 2011; Luo and Zhou, 2012; Rowbotham et al., 2012).
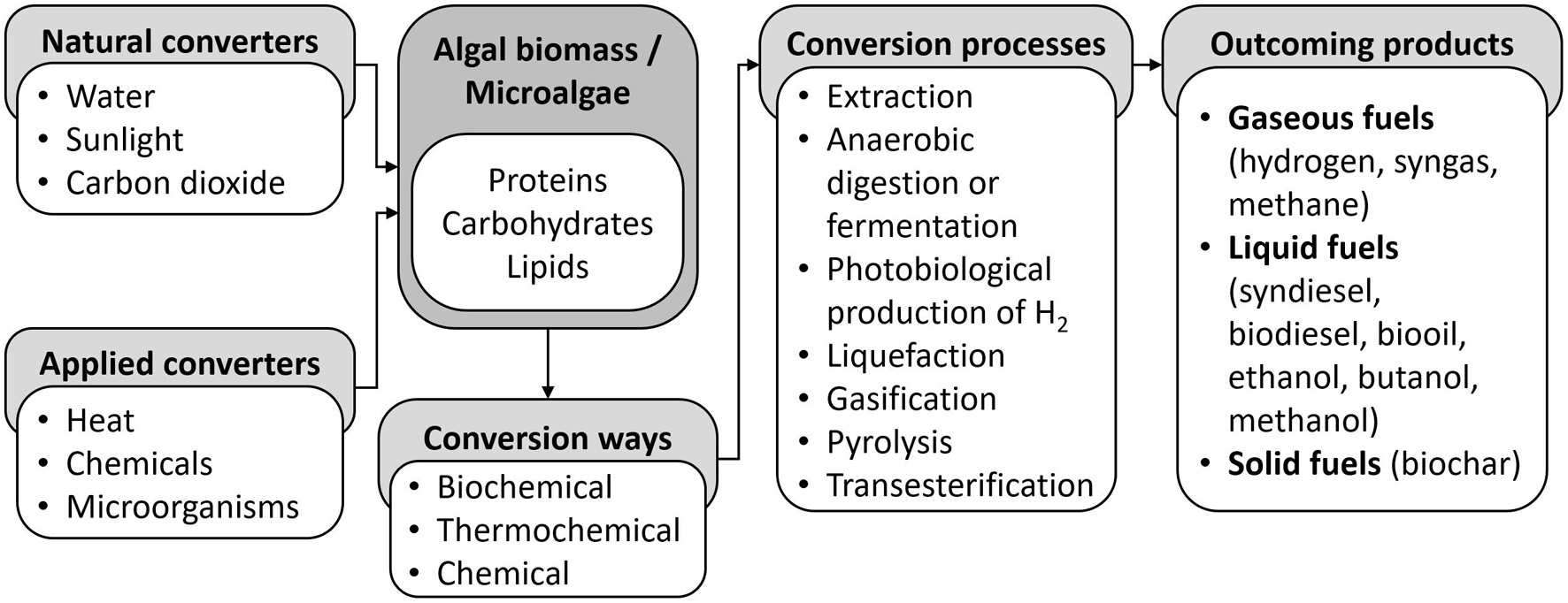
Figure 2. Schematic overview of conversion of algal biomass into economically significant products – biofuels, indicating the major converters, conversion ways and processes (Sikarwar et al., 2017; Kumar et al., 2020).
Torrefaction, also known as destructive drying and slow pyrolysis, is a mild pyrolytic process that recently received wide attention from the scientific community, as both a method of pre-treatment and upgrade of low-quality fuels (Chew and Doshi, 2011; Chen et al., 2015), as well as for the production of biochar. This process may be organized at scales ranging from extensive industrial facilities down to the individual farms (Lehmann and Joseph, 2009) and even at the domestic level (Whitman and Lehmann, 2009), making it applicable to various socioeconomic situations.
Table 2 summarizes the most important algal biofuels, their production mechanisms and applications. Sustainability is the most important issue for biofuel production. Hence, currently, algae, especially microalgae are the most promising source for biofuels due to their availability and continuous supply. In addition, different biofuel production techniques can be applied depending on the type of algae and biofuel. Thus, the use of algae can still be regarded as a viable option for the next generation of biofuels.
Fertilizers and Soil Improvers
Beach wrack can be utilized as a biofertilizer for the cultivation and growth of plants. Nowadays, biofertilizers are preferred over chemical fertilizers due to their environmentally friendly and cost-effective nature. Biofertilizers contain microorganisms that can fix nitrogen, solubilize phosphate and promote plant growth. The shells of many bivalves, e.g., blue mussels and oysters, are rich in CaCO3, a mineral currently mined from limestone, representing a widely exploited resource for many industrial applications in agriculture, as a biofilter medium for wastewater treatment, or even cement production (Oso et al., 2011; Yao et al., 2014; Morris et al., 2019; Scialla et al., 2020). In Galicia (Spain), the second global largest aquaculture producer of blue mussels (Mytilus galloprovincialis), their shells are commonly used in agriculture for liming the acidified soil (Morris et al., 2019) or for the absorption of heavy metals (e.g., arsenic) to reduce soil pollution (Osorio-López et al., 2014). Marine organic waste, such as seagrasses washed ashore, can also be considered as an alternative and sustainable fertilizer source because of its content of essential macro- and microelements (Bãdescu et al., 2017; Emadodin et al., 2020). Algae contain regulatory macro- and micronutrients, plant hormones such as cytokines, auxins, gibberellins and betaines that can increase plant growth, as well as vitamins, amino acids and metabolites with antibacterial and antifungal activity, which improve productivity. However, the low concentration of phosphorus, the presence of litter or toxic materials in the biomass, especially if the sampling area is subjected to high levels of anthropogenic pressure, can be of concern for using beach wrack as fertilizer (Villares et al., 2016). The salinity of seaweed leachates can be another obstacle; thus, applying it at appropriate rates and leaching of salts before the application can be crucial to obtain an optimal beneficial effect on plant root development. Although already applied in practice, the use of marine algae as biofertilizers is an ongoing field of research. One of the remaining research questions is to understand what role fertilizers from seaweed play in marginal coastal conditions by stimulating the growth of terrestrial plants or for the provision of specific nutrient elements. As an example, beer barley, grown in Scotland, is traditionally fertilized with seaweed and is known for the ability to cope with marginal, high pH soils without inorganic fertilizer addition (Brown et al., 2020).
Biochar has demonstrated applications as a soil enhancer, capable of improving water holding capacity, nutrient status and microbial ecology of many soils (Lehmann et al., 2006; Lehmann and Joseph, 2009; Thies and Rillig, 2012). Bird et al. (2011) showed that macroalgal biochar has properties that provide direct nutrient benefits to soils and stimulate crop productivity and are especially useful for application on acidic soils. In contrast to bioenergy, in which all CO2 that is fixed in the biomass by photosynthesis is returned to the atmosphere quickly as fossil carbon emissions are offset, biochar has the potential for more significant impact on climate through its enhancement of the productivity of infertile soils and its effects on soil GHG fluxes (Woolf et al., 2010).
Feed
For decades, fishery waste has largely been used in fishmeal production (due to its high protein and lipid contents), but this application is no longer considered the only option. Vázquez et al. (2019a, b) described alternative processes to valorize fish discards and produce fish mince, gelatins, oils and fish protein hydrolysates to be used as aquaculture feed ingredients. Wastes generated from the industrial processing of various fish species can also be turned into peptones (water-soluble products of partial hydrolysis of proteins to be used as a liquid medium for growing bacteria) (Vázquez et al., 2020). Mussel meat is rich in protein, lipids, carbohydrates, minerals and carotenoid pigments (Grienke et al., 2014) with potential application as food/feed supplements, preservation agents and enzymes. Seaweeds were traditionally used for animal feeding, either as aquaculture or cattle feed (Araújo et al., 2021). The interest in their use as feed was increased after the 1960s when Norway started producing seaweed meal from kelp (Makkar et al., 2016). Nowadays, they are still used as additional feed for free-range ruminants grazing on beach cast seaweeds in the coastal areas (Bay-Larsen et al., 2016). Brown seaweeds are more often used as feed because of their large size and ease of harvesting (Makkar et al., 2016). Seaweeds can supply the rumen with high amounts of rumen-degradable protein or can be used as a source of digestible bypass protein (Tayyab et al., 2016; Molina-Alcaide et al., 2017). In remote regions, like the Arctic, seaweeds are considered as local protein sources for sustainable sheep farming to replace imported soya (Bay-Larsen et al., 2018). However, the latest research highlights the challenges when applying seaweed proteins in animal feed (Novoa-Garrido et al., 2017; Özkan Gülzari et al., 2019; Emblemsvåg et al., 2020; Koesling et al., 2021; Krogdahl et al., 2021). Some seaweed species, for example, Asparagopsis taxiformis, have antimethanogenic activity on fermentation and can inhibit methanogenesis in the rumen at very low inclusion levels (Machado et al., 2016). Hence, the most appropriate method for processing such seaweeds and feeding to livestock in systems with variable feed quality and content has not been determined yet (Kinley et al., 2016).
Seaweeds tend to accumulate heavy metals (e.g., arsenic) or iodine. Consequently, using polluted beach wrack for feeding could negatively affect animal health. The decomposition and pollution, together with variable and undefined composition, may make beach wrack unsuitable for feed, and considerable sorting and cleaning may be required. Moreover, to have a continuous supply for feeding purposes, industrial cultivation of algae might be considered.
Additional Direct Valorization of Side Streams
Shells can be further exploited as a useful source for the production of biocomposites (Gigante et al., 2020), bio-based insulation material in environmentally responsive building solutions (Martínez-García et al., 2020) or even as a substitute for concrete components to reduce the dependency on conventional natural materials and to decrease the emission of GHG (El Biriane and Barbachi, 2020). Furthermore, seashells can be used as a calcium source to produce bioactive materials in tissue engineering, such as hydroxyapatite, which is the main inorganic phase of the bone (Hart, 2020; Hembrick-Holloman et al., 2020). Finally, direct human patch applications (fish skin grafts) of high ω-3 rich fish skins, like cod or tilapia, are also used for tissue regeneration in chronic or trauma wounds (Lima-Junior et al., 2019).
Valorization of Marine Biopolymers
The valorization of side stream biopolymers into useful compounds can have positive environmental, economic, social and technical added values, contributing to the circular economy development. Marine polysaccharides that are present in various marine organisms have the broadest valorization potential. The molecular structure of marine polysaccharides is characterized by long molecular chains of repeating monosaccharide units linked together by glycosidic bonds (Nitta and Numata, 2013). Serving mostly as energy storage and with structural functions, they are derived from various marine resources, including crustaceans and marine algae (Raveendran et al., 2013). Marine polysaccharides are characterized by outstanding chemical and structural diversity, and due to their biocompatibility and biodegradability, they have been used as a material of choice in numerous biomedical applications (Table 3). Exhibiting a wide range of bioactivities (such as anticoagulant, antioxidant, antimicrobial, anticancer, immunomodulatory, or antiviral), they are ideal candidates as low-cost, renewable, non-toxic and abundant biomaterials for the development of novel biosystems, such as 3D scaffolds, nanofibers, membranes, hydrogels, and bioinks for tissue engineering, drug delivery and wound dressing applications (Manivasagan et al., 2017).
The major source of marine polysaccharides are algae, with carrageenans mainly present in red algae, alginates and fucoidans in brown algae and ulvans in green algae, ranging from 4 to 76% dry weight (Kraan, 2012). ι-Carrageenan, a sulfated polysaccharide from red seaweed, has been approved by FDA, as Carragelose®, for the treatment of viral and respiratory diseases (Lu et al., 2021). Moreover, many crustacean shells (e.g., those of shrimps) are composed of chitin, a nitrogen-containing linear polysaccharide with wide industrial use, for example, in drug delivery, cosmetics and food. Chitin is regarded as the second most abundant polysaccharide in nature, after cellulose, used for the commercial production of chitosan, a water-soluble derivative obtained by demineralization, deproteinization, and decolourization of chitin (Jiménez-Gómez and Cecilia, 2020).
Novel Materials for Biomedical Applications
The extraordinary biocompatibility, non-antigenicity, chelating ability and bioavailability of marine biopolymers make them suitable materials for biomedical applications. Due to the broad spectrum of reported bioactivities exhibited by marine polysaccharides, they are ideal candidates for novel biomedical systems and have been utilized in various formulations for drug delivery, wound healing and tissue engineering applications. The interest of the biomedical sector in marine polysaccharides is steadily increasing not only because of their natural origin and their unique biological and physicochemical properties, but also due to their stability, safety and high availability at a relatively low cost (Venkatesan et al., 2017; Choi and Ben-Nissan, 2019; Rahmati et al., 2019; Bilal and Iqbal, 2020).
In the pharmaceutical sector, marine polysaccharides have been used as binders, stabilizers, thickeners, matrix materials, emulsifiers, and suspending agents (Figure 3). Over the years, they have been utilized in various formulations, such as gels and hydrogels, micro/nanoparticles (MPs/NPs), films and membranes, nanofibers, as well as 3D porous structures, serving as drug release modifiers, bioadhesives, coatings, wound dressing materials and tissue engineering scaffolds for various biomedical applications (Ruocco et al., 2016; Vanparijs et al., 2017; Joshi et al., 2019).
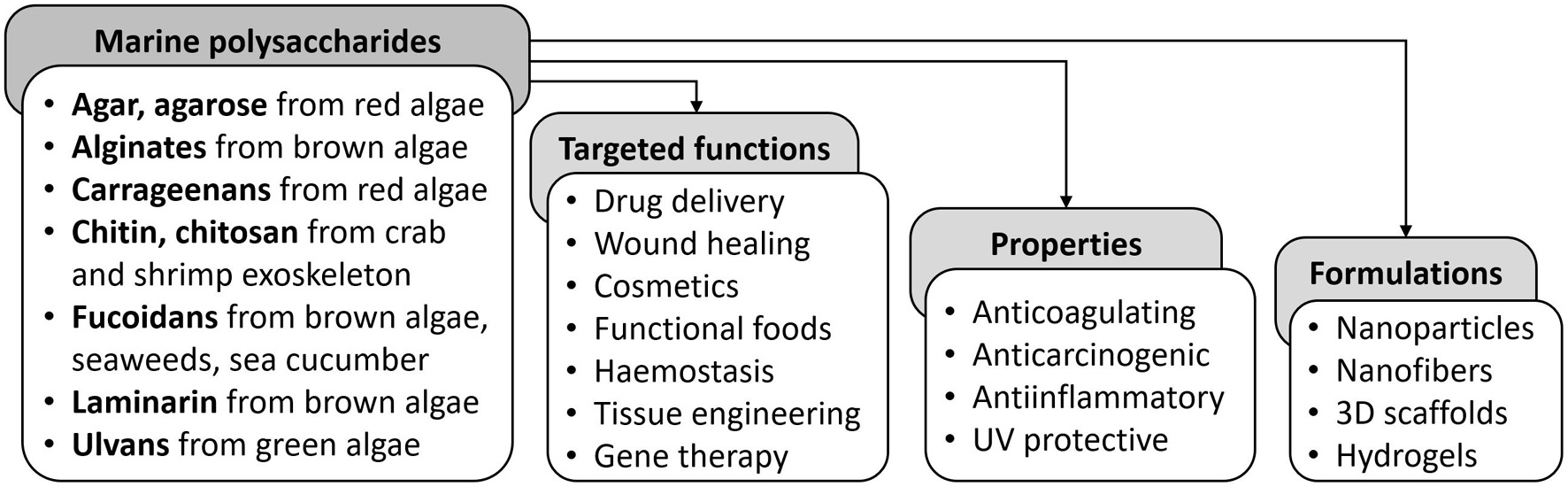
Figure 3. Examples of marine polysaccharides and their applicability for biomedical purposes (Ruocco et al., 2016; Joshi et al., 2019).
Mussel byssus contains high levels of collagen, another widely used raw material (Rodríguez et al., 2017). Collagen extracted from fishery resources is seen as a very promising direction of biotechnological valorization as it is available to a great extent, lacks toxicity and the sociocultural barriers are absent. Mussels easily attach to wet substrates or rocks in wave-battered seashores thanks to adhesive proteins and amino acids (e.g., 3,4-dihydroxyphenylalanine, DOPA). This fact has fueled research on mussel-inspired multifunctional coatings and bioadhesives for use on various surfaces (Lee et al., 2007; Shin et al., 2020). Shell extract of scallop (Pecten maximus) has been shown to stimulate the biosynthesis of extracellular matrix and both type I and type II collagen biosynthesis in primary cells, pointing out their potential in dermatology and cosmetic sectors (Latire et al., 2014).
Gels and Hydrogels
In the biomedical field, gels and hydrogels are recognized as promising biomaterials for drug delivery, tissue engineering, biosensors, self-healing and hemostasis systems due to their highly porous structure, tunable biodegradability and biocompatibility (Venkatesan et al., 2015; Chai et al., 2017). Hydrogels are gels that consist of hydrophilic polymer chains arranged in a 3D cross-linked network. This polymeric network can be controlled and easily manipulated for inclusion and, subsequently, the modified diffusion of various active ingredients (Domalik-Pyzik et al., 2019). Hydrogel scaffolds possess the ability to swell without dissolving in biological fluids; however, due to the fragility of their gel matrix, the need for novel and more stable hydrogel systems is still high (Hoare and Kohane, 2008).
In this respect, carrageenans possess enormous water retaining capacity and gelling properties and have been widely exploited to develop bio-hydrogels (Oun and Rhim, 2017). Alginate hydrogels have been widely used for wound dressing applications and other biomedical applications (Galli et al., 2018; Liao et al., 2018). When cross-linked with natural or synthetic components, they form soft or stiff gels with different physicochemical properties depending on the alginate mannuronic acid:guluronic acid ratio, the material composition and the degree of cross-linking (Gharazi et al., 2018). Chitosan-based hydrogels have been modified with catechol, hydrocaffeic acid, and poly(ethylene glycol) to enhance the bioadhesive, mechanical and antibacterial properties. The developed hydrogel patches and injectable gels can be used as soft tissue engineering materials (Du et al., 2020; Kim et al., 2020; Zheng et al., 2020).
Polymeric Micro- and Nanoparticles
In recent years, various delivery systems have attracted significant attention in the drug delivery sector. Owing to the benefits provided by their small sizes, the application of natural origin MPs/NPs has emerged as a very promising approach for targeted drug delivery. Polymeric MPs/NPs can be fabricated through different methods, such as polyelectrolyte complexation, emulsification and ionic gelation, exhibiting many advantages, such as improved drug solubility, distribution and bioavailability (Chifiriuc and Grumezescu, 2016). Due to their adjustable size and surface characteristics, they can be used as novel carriers for the controlled delivery of active pharmaceutical ingredients with improved pharmacokinetics and pharmacodynamics (Nikam et al., 2014; Manivasagan and Oh, 2016). Several studies have shown the collagen applications as a carrier in different drug delivery systems (Gu et al., 2019), having remarkable abilities and being the focus of extensive research efforts. In particular, collagens from a variety of marine sources have been used to produce micro (diameters between 0.1 and 100 μm) and nano (1–100 nm) bio-based drug delivery systems and are attractive and promising for applications in biomedical and pharmaceutical industries. Marine polysaccharides have been explored to design polymeric MPs/NPs, mainly because of their ionic nature. Oppositely charged polysaccharides can interact with ions, resulting in complex polyelectrolyte structures that can encapsulate various active compounds. The release of the embedded compounds from the complex can be controlled and achieved through various mechanisms, such as charge interactions, ion exchange mechanisms, polymer degradation or dissolution of the polyelectrolyte matrix (Venkatesan et al., 2016).
Numerous studies on the preparation of marine polymer-based nanoparticles have been reported over the years for targeted drug delivery (Bilal and Iqbal, 2020). In a recent report, hybrid alginate/chitosan nanoparticles were investigated for the in vitro release of lovastatin as promising new drug carriers (Thai et al., 2020). Ulvan/lysozyme nanoparticles have exhibited enhanced antibacterial activity against Staphylococcus aureus, while at the same time highlighting the potential of ulvan for the preparation of peptide/protein delivery systems (Tziveleka et al., 2018). The use of carrageenan in MPs/NPs-based drug delivery systems for various biomedical applications has also been actively explored. Insulin-loaded lectin-functionalized carboxymethylated κ-carrageenan microparticles were produced by ionic gelation technique, and their potential use as an improved carrier for the oral delivery of insulin was evaluated (Leong et al., 2011). Oral administration of the lectin-functionalized insulin-carrageenan microparticles (diameter 1273 ± 201 μm) in diabetic rats resulted in a sustained release of the insulin in the intestinal region and a prolonged duration of the hypoglycaemic effect, confirming their therapeutic efficacy. κ-Carrageenan extracted from the red algae Eucheuma cottonii was utilized to encapsulate the poorly soluble coenzyme Q10 (CoQ10) using the spray drying technique. The CoQ10-κ-carrageenan microparticles were shown to represent an efficient model to increase the water solubility of coenzyme Q10, creating a new water-based product for the food industry to be used either as a main ingredient or as an enriched additive (Chan et al., 2016). Microparticles synthesized using carrageenan with a different number of sulfate groups κ, ι, and λ, were prepared by microemulsion polymerization/crosslinking and were shown to include a wide range of particle sizes (0.5–100 μm). The particles and their modified forms were found to have broad biomedical applicability due to their drug delivery capability, antimicrobial activity, anticancer, high blood clotting effect, good biocompatibility, and cell viability (Sahiner et al., 2017).
Despite being one of the most widespread natural polysaccharides, chitin was for a long time considered as an intractable polymer due to its lack of solubility in common solvents, which limits its processing and practical use (Rinaudo, 2006). Recent studies have mainly focused on chitin NPs (Mincea et al., 2012; Zeng et al., 2012) and their applications in different fields. In nature, chitin occurs as micro/nanofibrils that form a composite together with proteins, pigments and calcium carbonate and has a structural role in the exoskeleton of crustaceans and insects (Chen et al., 2008). The unique properties of chitin NPs, such as their renewable and biodegradable characteristics, small size, low density, chemical stability, biological activity, biocompatibility and no cytotoxicity, make them excellent candidates for use in an extensive range of medical applications, nanocomposite fields, water treatment, cosmetics, electronics devices, etc. (Zeng et al., 2012). Several applications of chitin NPs have been developed during the last years in different fields; however, the application in the fields of materials science and health are the most predominant. The addition of nanochitin as a filler in the production of biocomposites enhances the physicochemical properties of the material in addition to its antifungal properties (Salaberria et al., 2014, 2015a, b; Herrera et al., 2017). Its biological activity and non-cytotoxicity have promoted the use of nanochitin for health care and medical applications, such as scaffold and tissue regeneration (Zubillaga et al., 2018; Danti et al., 2019; Smirnova et al., 2019; Zubillaga et al., 2020), drug and cosmetics (Mellou et al., 2019; Coltelli et al., 2020).
Chitosan, obtained from chitin available in the exoskeleton of crustaceans, is a cationic polymer described as an excellent material to design drug delivery systems due to its biocompatibility, biodegradability and non-toxicity. Chitosan NPs have a wide array of applications with excellent oral bioavailability for different biomolecules, such as hydrophobic drugs, nucleic acids, proteins and polysaccharides, retaining their bioactivity, improving stability and enhancing the therapeutic effect (Lang et al., 2020). Moreover, chitosan has mucoadhesive properties and a broad spectrum of bioactivities, namely antioxidant, anti-inflammatory and antimicrobial (Chan et al., 2016; Hafsa et al., 2016), which increase its potential interest for oral drug delivery applications. Chitosan NPs can be produced using different methods, although the most widely described ones are ionotropic gelation and polyelectrolyte complexation. These methods are simple, do not include organic solvents and provide an excellent opportunity to deliver large amounts of nanomaterial into desired products (Divya and Jisha, 2018). Other marine-derived polysaccharides, such as fucoidan, alginate, ulvan, carrageenan, and laminarin, commonly isolated from seaweeds, also have specific and interesting individual properties explored for potential application in drug delivery systems (Venkatesan et al., 2016). These natural anionic polymers can be used to produce NPs of different size, charge and shape for drug delivery applications using methods as emulsion, ionic gelation and polyelectrolyte complexing (Cardoso et al., 2016; Venkatesan et al., 2016). NPs made of marine polysaccharides have been exploited for oral delivery of active pharmaceutical drugs due to their increased stability and resistance to degradation under acidic gastrointestinal conditions leading to improved intestinal drug absorption. Insulin-loaded chitosan-alginate-pentasodium tripolyphosphate (TPP) NPs were produced by ionic gelation. The delivery by nasal administration in rabbits of this hybrid formulation showed enhanced systemic absorption demonstrating its potential in increasing nasal insulin absorption (Goycoolea et al., 2009). Fucoidan-chitosan NPs have been widely described as promising for application as carriers in oral drug delivery systems (Barbosa et al., 2019b). NPs resulting from the encapsulation of curcumin by O-carboxymethyl chitosan-fucoidan were shown to have lower toxicity in mouse fibroblasts when compared with the free form and to be efficiently internalized by Caco-2 cells, demonstrating its potential application for oral drug delivery (Huang et al., 2016). Chitosan-fucoidan NPs containing berberine were developed and shown by in vitro testing in Caco-2 cells/RAW264.7 cells co-culture to restore the barrier function in inflammatory and injured intestinal epithelial (Wu et al., 2014). Also, quercetin loaded fucoidan-chitosan NPs developed for application as a functional food were shown to be stable with controlled drug release under simulated gastrointestinal environment, while maintaining intense antioxidant activity (Barbosa et al., 2019a). Based on the known anticoagulation activity of fucoidan, NPs of chitosan-fucoidan were prepared to encapsulate red ginseng extract and improve its antithrombotic activity and physicochemical properties. Nanoencapsulation improved the ginsenoside solubility and decreased the effect of platelet aggregation in vitro. In vitro studies in the rat model also demonstrated that the NPs caused a significant reduction in thrombus formation when compared with the free red ginseng extract (Kim et al., 2016).
Production of chitosan-fucoidan NPs for pulmonary delivery of the antibiotic chitogentamicin has been described, and results indicate the improvement of antimicrobial efficacy and elimination of systemic toxicity when compared with the intravenous antibiotic administration, with great potential for pneumonia treatment (Huang et al., 2016). Marine-derived drug delivery systems based on chitosan-fucoidan NPs have been recently developed for drug delivery in cancer treatment. Gemcitabine-loaded NPs showed increased toxicity for human breast cancer cells without increasing toxic effects on endothelial cells when compared with free gemcitabine (Oliveira et al., 2018). Piperlongumine is a new class of pro-oxidant drugs with the potential for cancer-specific therapy. Encapsulation of this hydrophobic drug into chitosan-fucoidan NPs increased its solubility and bioavailability, enhancing its anticancer efficacy (Choi et al., 2019).
Studies on the production and application of marine collagen as drug delivery systems for biomedical or as supplements for the food industry are also available in the literature. A MPs protein delivery system was developed using an emulsification-gelation-solvent extraction method and a polymeric matrix of marine collagen extracted from the jellyfish Catostylus tagi. This collagen MPs system (median particle size 9.5 μm) showed promising and versatile results for the controlled release of therapeutic proteins with retained biological activity (Calejo et al., 2012). Collagen from a marine sponge (Porifera, Dictyoceratida) was used to produce a bio-based dressing for topical drug delivery able to absorb the excess wound exudate and at the same time release the drug regulating the healing process (Langasco et al., 2017). Also, collagen extracted from the marine sponge Chondrosia reniformis was used to develop collagen microspheres for dermal delivery of all-trans-retinol (Swatschek et al., 2002). Although the retinol loaded MPs showed a broad size distribution (ranging from 126 ± 2.9 nm to 2179 ± 42 nm), the dermal penetration of retinol-hydrogel-collagen MPs formulations was two-fold higher than when compared to retinol formulations without the MPs. NPs, produced with C. reniformis collagen (size 123 ± 5.5 nm), loaded with 17ß-estradiol-hemihydrate, for application in hormone replacement therapy, were shown to be a promising transdermal drug carrier system of estradiol with enhanced bioavailability, prolonged drug release and increased estradiol absorption compared to a commercial gel (Nicklas et al., 2009).
Marine collagen peptides obtained from Synodontidae fish scales were used to develop alginate NPs, loaded with collagen peptide chelated calcium (diameters approximately 400 nm). This in vivo study demonstrated that the core-shell NPs were able to improve calcium absorption and prevent calcium deficiency in rats treated with this novel biphasic material that could represent an improved calcium supplement for the food industry (Guo et al., 2015).
Polymeric Nanofibers
During the past years, polymeric nanofibers have gained considerable interest due to their unique properties, such as high surface-to-volume area, high and controlled porosity and mechanical flexibility (Kenry and Lim, 2017; Al-Enizi et al., 2018; Cheng et al., 2018). Marine biopolymers, due to their biocompatibility and biodegradability, are considered ideal candidates for the development of multifunctional non-wovens. Exhibiting high encapsulation efficacy and architectural analogy to the natural extracellular matrix, they can be easily produced through the electrospinning technique, which is the most widely used method for the production of polymeric nanofibers with tailor-made properties (Teo and Ramakrishna, 2006; Greiner and Wendorff, 2007; Bhardwaj and Kundu, 2010). Various natural and synthetic polymers can be utilized in nanofibrous matrices, incorporating numerous active agents for different biomedical applications.
In most cases, marine polysaccharides, often lacking chain entanglement, have been utilized in combination with other synthetic or natural biopolymers into hybrid polymeric nanofibrous systems that offer the advantageous properties of the combined ingredients (Zhao et al., 2016). Numerous synthetic polymers, such as polycaprolactone, polyethylene oxide, polyvinyl alcohol (Zia et al., 2017), polylactic acid and cellulose acetate, have been used in the development of such hybrid marine polymer-based electrospun patches. Various nanofibrous scaffolds of alginate, fucoidans, ulvan, chitosan and chitin and other biopolymers (e.g., gelatin, cellulose, hyaluronic acid, collagen and their derivatives) have been developed, exhibiting great potential in tissue regeneration, wound healing and controlled drug delivery (Kikionis et al., 2015; Mendes et al., 2017; Augustine et al., 2020). As a recent example, metformin-loaded polycaprolactone/chitosan nanofibrous patches were reported as potential guided bone regeneration membranes (Zhu et al., 2020), while in another work, electrospun alginate nanofibrous dressings loaded with the aqueous extract of Pinus halepensis bark displayed significant in vivo anti-inflammatory activity in mice (Kotroni et al., 2019).
Membranes and Films
While various membranes and films have been developed from marine biopolymers as wound healing or tissue engineering systems, it is alginate that has been most widely used in wound dressings, either alone or combined with other biomaterials. Due to its gelling and fluid-absorption ability, alginate can promote the skin recovery process, maintaining a physiological moist wound environment. It can be easily cross-linked via electrostatic, ionic interactions, covalent-like bonding, redox reactions and coordination with various metals and oppositely charged polysaccharides. The cation interaction of its guluronate blocks with calcium electrolyte into an egg-box-like structure (Goh et al., 2012) has been employed for various wound healing applications. Furthermore, its combination with other positively charged biopolymers, like chitosan in polyelectrolyte forms, has been shown to increase the mechanical stability of the wound dressing materials. In a similar approach, aiming to develop scaffolds for cell cultivation, anionic ulvan and cationic chitosan have been combined to form novel supramolecular structures of stabilized membranes through electrostatic interactions, showing excellent attachment and proliferation of 7F2 osteoblasts (Toskas et al., 2012). As mentioned before, chitosan has been used in many wound dressing and tissue engineering applications, dressing films and membranes (Khan et al., 2020).
3D Structures
In recent years, 3D bioprinting has risen as a versatile tool in regenerative medicine. Therefore, the demand for suitable bioink materials with good printability, biocompatibility and mechanical integrity is apparent. Marine biopolymers, due to their chemical structures and biological functionalities, satisfy most requirements of 3D bioprinting. 3D bioprinting regenerative medicine techniques for human tissue and organ engineering and biofabrication currently apply to 4% chitosan, 10% gelatin, and 26% collagen from a marine origin in bioink formulations for cellular laying/encapsulation (Zhang et al., 2019). Marine polysaccharide hydrogels are naturally derived bioinks, demonstrating low immune response, sufficient biological cues and excellent biocompatibility for tissue engineering applications. Among various marine-origin macromolecules, alginate, carrageenan and chitosan have been widely used as hydrogels in 3D bioprinting for regenerative medicine, such as tissue repair and regeneration during recent years (Zhang et al., 2019). For example, the excellent biocompatibility and the thermogelation properties of κ-carrageenan and alginate have been used to fabricate the cell-laden scaffolds on alginate/carrageenan hydrogels in 3D bioprinting (Kim et al., 2019). In another approach, cells encapsulated within chitosan-based hydrogels demonstrated the printability and applicability of chitosan as a bioink for 3D bioprinting in bone tissue engineering (Demirtaş et al., 2017).
The freeze-drying technique has also been applied for the fabrication of 3D porous scaffolds. Freeze-dried scaffolds can be produced by removing the frozen solvent of a polymeric solution under vacuum, leaving empty spaces (pores) in the formed polymeric scaffold. The architectural characteristics of the produced scaffolds may be tuned by changing the freezing conditions, the polymer solution concentration and the polymer and solvent type (Reys et al., 2017). In this respect, chitosan freeze-dried sponge-like structures were obtained, exhibiting blood absorbing capacity suitable for haemostasis (Kavitha Sankar et al., 2017). Recently, the preparation of ulvan/gelatin hybrid sponge-like scaffolds was reported, exhibiting efficient mesenchymal stem cell adhesion and proliferation for bone tissue engineering applications (Tziveleka et al., 2020).
Novel Materials for Bio-Based Food Packaging
More than 380 million metric tons of plastics are produced worldwide (Ritchie and Roser, 2018). In Europe, 40% of produced plastic is used in packaging. Despite the tremendous benefits of using plastics for packaging, their single-use feature results in an enormous stream of waste with a significantly negative impact on the environment. Synthetic plastics are petroleum-based, hence consuming large amounts of fossil fuels for their production. Moreover, they are not biodegradable and, thus, after disposal, they can accumulate in natural ecosystems for up to several thousands of years. Consequently, more than 5 trillion plastic particles weighing over 250,000 tons are estimated to be floating in Earth’s oceans (Eriksen et al., 2014), posing a major threat to the trophic chain. Only 14% of plastic packaging is currently recycled (Ellen MacArthur Foundation, 2016), and there is a clear consensus that the industry needs to shift to biodegradable plastics from renewable resources (i.e., biopolymers) for a long-term solution to the current situation (Oliveira et al., 2020). Biopolyesters, such as polylactic acid (PLA), polyhydroxyalkanoates (PHAs) and thermoplastic starch, can be used for food packaging due to their relatively good processability using industrial techniques such as extrusion or thermoforming. However, their properties are still far from synthetic polymers (especially in terms of thermal resistance, barrier and mechanical performance), and their production costs are too high to compete on the market. Moreover, the raw materials typically used to produce biopolymers originate from land crops, whose primary use is the food sector. In this context, the packaging industry is looking for alternative biopolymers with enhanced properties that can be extracted from cheaper, sustainable resources. Given its abundance and interesting composition, marine biomass has received great intererest is being focused on marine biomass as one of the most promising alternative sources for the extraction of biopolymers for food packaging applications.
The structural polysaccharides found in the cell walls from seaweeds, such as cellulose and phycocolloids (Table 3), have excellent properties, which make them promising candidates for the development of bio-based and sustainable food packaging. Cellulose from land biomass has been widely used to produce food packaging materials and applied as a filler to improve the properties of other biopolymers (Ramamoorthy et al., 2015; Trache et al., 2016). In parallel, several recent studies have reported on the outstanding properties of cellulose extracted from marine biomass (Bettaieb et al., 2015; Khalil et al., 2017; Benito-González et al., 2018; Martínez-Sanz et al., 2020a). One particularly interesting approach, given the circular economy policies that are being promoted by the governing bodies, is the valorization of marine waste biomass. For instance, the residues generated after the accumulation of leaves from the seagrass Posidonia oceanica, found in the Mediterranean shores and the industrial waste produced after extraction of agar from red seaweeds have been used to extract cellulosic fractions with different degrees of purity (Benito-González et al., 2018; Benito-González et al., 2019a; Martínez-Sanz et al., 2020a). These cellulosic fractions can be used to produce films, employing a green method based on the production of aqueous suspensions. Even though the properties of the films may vary depending on the biomass source, commonly, the presence of other non-cellulosic components in the less purified fractions may improve the performance of the films (Benito-González et al., 2019a; Martínez-Sanz et al., 2020a). This is particularly interesting since high-performance cellulose-based packaging films could be produced utilizing simplified and more sustainable methods, thus reducing the production costs and the environmental impact. These cellulosic fractions can also be used as fillers to improve the properties of other biopolymers. One recent work has reported the capacity of cellulosic fractions from P. oceanica to produce biopolymeric films with improved mechanical and barrier performance, as well as with better stability upon storage when incorporated into thermoplastic starch by melt mixing (Benito-González et al., 2019b).
Cellulose from marine biomass can also be used in a particular type of structure known as aerogels. Aerogels are lightweight and highly porous structures, which present excellent sorption capacity and can be used in food packaging as absorbent pads (for fresh products such as meat and fish) and as components for the incorporation and sustained release of bioactive compounds in smart packaging, amongst others. Their high specific surface makes them also ideal materials for catalysis and other advanced applications. Many studies have reported the production of cellulose-based aerogels (Nguyen et al., 2014; Feng et al., 2015; Buchtová et al., 2019); however, the preparation methods are often quite complex, involving several steps (disruption of cellulose crystalline structure, gelation, cellulose regeneration, solvent exchange and a final drying step through supercritical CO2 or freeze-drying). Moreover, due to the highly hydrophilic characteristic of cellulose, hydrophobization treatments are usually required to improve the water-resistance of the aerogels. A simple freeze-drying method has been recently reported to yield high-performance aerogels from cellulosic fractions derived from aquatic biomass, and a very simple strategy has been developed for hydrophobization of cellulosic aerogels, making them stable in aqueous solutions (Fontes-Candia et al., 2019; Benito-González et al., 2020; Martínez-Sanz et al., 2020a,b). These materials display a highly porous structure, especially when using less purified cellulosic fractions, conferring a great sorption capacity when soaked in hydrophilic and/or hydrophobic liquids. This has been exploited to develop bioactive aerogels, incorporating an antioxidant extract that could be released upon contact with meat, thus reducing the oxidation processes taking place upon storage (Fontes-Candia et al., 2019).
Although cellulose is, without a doubt, the most widely exploited marine biomass biopolymer for the development of food packaging, other structural polysaccharides such as phycocolloids are currently being studied. In particular, agar (Sousa and Gonçalves, 2015; Malagurski et al., 2017; Martínez-Sanz et al., 2019), carrageenans (Choi et al., 2005; Vu and Won, 2014; Farhan and Hani, 2017) and alginate (Abdollahi et al., 2013a, b; Sirviö et al., 2014; Senturk Parreidt et al., 2018) have excellent potential for the production of food packaging films with interesting properties. The main disadvantages of phycocolloid films are their excessive rigidity (which is counteracted with the addition of plasticizing agents) and their low resistance to high relative humidity conditions (which can be improved by incorporating more hydrophobic fillers). Interestingly, less purified agar-based extracts have been shown to overcome these issues due to the positive effect of other compounds remaining from the native seaweeds, such as proteins and minerals (Martínez-Sanz et al., 2019). These phycocolloids can be used to produce porous aerogels (Quignard et al., 2008; Gonçalves et al., 2016; Manzocco et al., 2017), but similarly to cellulose, complex preparation methods are often required. Further research needs to be carried out to look for alternative manufacturing processes and to identify strategies to adjust the properties of the obtained aerogels to the requirements of different food packaging applications.
Even though there are no studies reported on marine collagen as an alternative food packaging material, there are some studies on bovine/porcine collagen that has already been tested to produce edible films for protection and extension of food products, such as sausages casings (Suurs and Barbut, 2020). Moreover, the addition of chitosan to gelatin films of cuttlefish skin improves the thermal stability of the polymer network and increases the antioxidant and antimicrobial activity against some Gram-positive and Gram-negative bacteria, which is a useful property in packaging production (Hajji et al., 2021). However, there are some restrains in a broad application of collagen as packaging material, as it is sensitive to moisture. Gelatin based packaging coatings have also been explored, but various additives (lysozyme, chitosan, chitin, essential oils, among others) need to be applied to achieve the desired properties (antimicrobial, antioxidative) (Chawla et al., 2021).
Use in the Leather Industry
Among the solutions for reducing fish waste, one potential option is utilizing fish skin to produce exotic leathers for accessories such as bags, gloves, or shoes. The valorization of any animal skin into leather materials involves tanning, a process that alters the skin protein structure, transforming the biodegradable skin into durable and flexible leather. Saranya et al. (2020) explored the potential of fish waste to produce fish oil, which could be used as a fat-liquoring agent in leather processing, and the results were better compared to those of a traditional commercial fat-liquoring agent. Thus, the fish oil fat-liquor produced from fish waste can be regarded as an eco-friendly alternative to lubricate leather as it allows to substantially reduce the sludge disposal issues in the tannery industry and reduce waste in the fisheries sector. To satisfy the trends for greener production, bio-tanning processes are being sought that replace chromium with plant-based tannins or the re-use of tanning floats, which can reduce the water consumption in the process up to 90%. An example of successful valorization of fish skin into leather is the Moroccan company SeaSkin, using a plant-based coloring system and a dry tanning process, thus reducing 95% of water in the process. Another valorization example is the use of salmon skin in leather straps for watches by the Norwegian company Berg Watches, in collaboration with the Icelandic Nordic Fishleather.
Use in Food/Feed Industries
Proteins are a highly valuable resource, present in several raw materials from plant and animal origin, available in different amounts and with the primary dietary function to provide essential amino acids and tissue building material to the human and animal body. Proteins of marine origin demonstrated higher quality due to high amounts of all essential amino acids (Abdul-Hamid et al., 2002; Prihanto et al., 2019). Several studies have also proven that the quality, digestibility and bioavailability of the essential amino acids in marine protein concentrates increase after food-grade hydrolysis processing (Faber et al., 2010; Kim, 2013). Marine protein hydrolysates can also contribute the necessary amounts of bioactive peptides with antioxidative, antihypertensive, metal-chelating, antimicrobial and anti-inflammatory/immunomodulatory properties to the human diet (Kim, 2013; Abdelhedi et al., 2018; Ediriweera et al., 2019).
Marine polysaccharides (e.g., alginates, carrageenans, agar), as well as gelatin, are used in food and feed industries as gelling agents, stabilizers and/or emulsifiers. Their addition can change the product’s viscosity, which impacts the transport of volatile components and affects flavor release (Liu et al., 2015). As an excellent source of proteins, lipids, vitamins and minerals, by-products as fish skin, viscera and blood, as well as crustacean and bivalve shells, are used in the pharmaceutical and agriculture industry and innovative food processing technologies (Beaulieu et al., 2013).
Use in Bioremediation
Several marine polysaccharides have also found useful applications in bioremediation, either as adsorbents of organic and inorganic pollutants or as suitable biopolymers for the immobilization of microbial species with relevant catabolic properties. For instance, chitosan and alginate-based membranes have shown to be highly efficient in the retention of industrial dyes and heavy metals, such as mercury, lead or nickel (Ngah et al., 2010), as well as in the removal of priority organic pollutants, including pesticides (Moraes et al., 2013), pharmaceutical drugs (Vassalini et al., 2020), phenolic compounds and other industry-borne pollutants (Vidal and Moraes, 2019; Vassalini et al., 2020). In fact, several membrane composites, containing these biopolymers, are now being regarded as suitable replacements of current adsorption technologies (e.g., activated carbon) for implementation in treatment plants, depuring industrial effluents (Vidal and Moraes, 2019). On the other hand, several marine biopolymers, including alginate, cellulose and chitosan, have also been widely used for the encapsulation of living cells for many purposes in biotechnology, including bioremediation (Wang et al., 2019). Cellular immobilization is of additional significance in bioremediation strategies that rely on the application of degrading microorganisms to the affected sites (i.e., bioaugmentation), as it maximizes cellular viability and stabilizes the metabolic performance, allowing to achieve productive biodegradation in biomes that often showcase inhospitable conditions for microbial development (e.g., extreme pH levels, low nutrient availability). For such ends, alginate beads, cellulose nanofibers or chitosan NPs have served as suitable microbial interfaces to facilitate the bioremediation of various organic pollutants and to mitigate the nutrient load of wastewaters (Eroglu et al., 2012; Sathishkumar et al., 2014; Khanpour-Alikelayeh et al., 2021), while also bearing minimal environmental impacts due to their biocompatibility and biodegradability.
Valorization of Marine Biomass as a European Strategy
The circular economy and bioeconomy are high on the EU policy making agenda. On the one hand, the Circular Economy Action Plan aims at reducing raw materials and associated environmental pressures, while on the other hand, the Bioeconomy Strategy promotes the exploitation of biomaterials in a sustainable manner. However, the development of novel products demands investment into the optimization of technical procedures, a clear cost-benefit and supply sustainability. That was also recognized by the European Commission that started to support this with new funding opportunities for research and innovation in the field of circular economy within marine biotechnology (Table 4). The first international EU-funded projects focused on exploiting marine organisms. They were financed within Framework Programme 7 - FP7 (2007-2013) and were mainly dedicated to innovative bioprospecting of marine microorganisms and the discovery of high value-added bioactive compounds. Some of these projects investigated the extraction and application potential of marine-derived biopolymers (polysaccharides, proteins, enzymes, among others) in the pharmaceutical, cosmeceutical and medical industries. Two projects within the EU FP7 SME (N-CHITOPACK and SEABIOPLAST) were financed for bioplastic production and food packaging from fish waste and seaweed biomass. At the end of FP7, an ERA-NET was launched for marine biotechnology (ERA-MBT) that funded 21 projects, from which eight were focused on marine biopolymers from a broad range of marine (micro)organisms (bacteria, cyanobacteria, macroalgae, shellfish, crustacean, fish). Polysaccharides, but mostly chitin, chitosan, alginate and laminarin, were the most investigated polymers within ERA-MBT funded projects. H2020 Framework Programme (2014–2020) proceeded with funding projects for marine biomass exploitation for new products. Two projects (PULMO and GoJelly) were focused on jellyfish biomass utilization (usually by-catch) for different applications, from which protein extraction (mainly collagen) was used in cosmeceutical, nutraceutical, medical and agricultural applications. A Public-Private Partnership between the EU and the Bio-based Industries Consortium was established under H2020 called Bio-Based Industries Joint Undertaking (BBI JU). The aim is to reduce Europe’s dependency on fossil-based products and to meet EU climate change goals that would result in greener and eco-friendlier growth. BBI JU funded two projects that foster a cost-effective marine biomass supply (micro- and macro- algae, aquaculture and fisheries side streams) and scale-up the production process of ingredients for further application. On the regional level, INTERREG Programmes also identified marine biomass (mostly seaweed, such as the Interreg Germany-Denmark project FucoSan on fucoidan) as a valuable feedstock for new value chains and biopolymer extraction.
Case Studies
Valorization of side streams (marine and terrestrial ones) could help transition to a more circular economy, where waste is minimized, and resources are used more efficiently by creating new value chains. This strategy is also stated in the EU Action Plan for the Circular Economy (COM/2015/0614), and there have been significant financial contributions to develop new, sustainable value chains, covering marine, and terrestrial sources. This section presents some of these value chains, categorized by geopolitical location: Norway, Scotland, and the Baltic Sea. Norway and Scotland are presented due to their traditional link with fisheries and aquaculture. Both countries have been introducing seaweed aquaculture in the past years, and the valorization potential of this side stream is also presented. Finally, we present the Baltic Sea, which has enormous potential for the valorization of beach wrack (Figure 4).
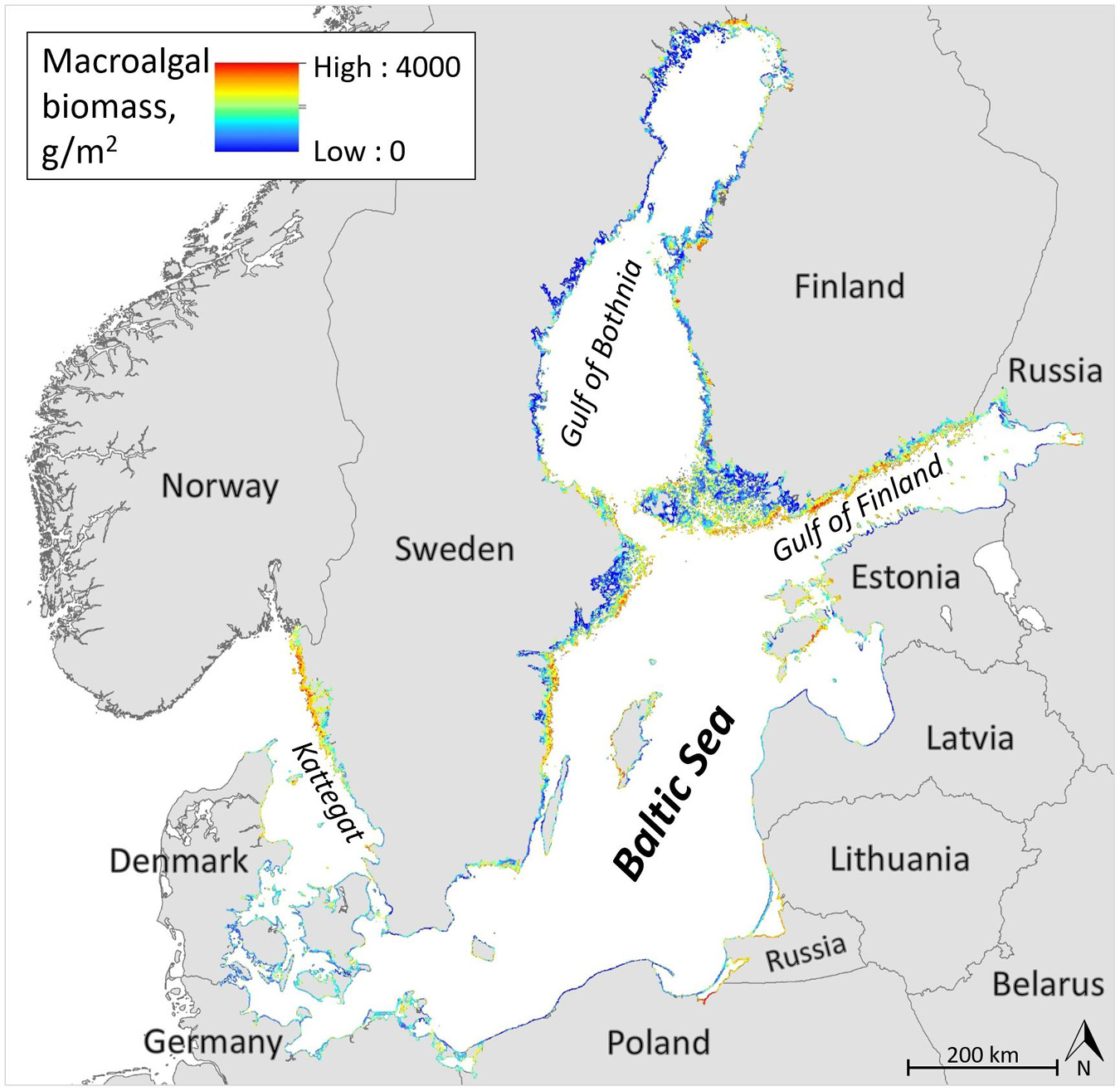
Figure 4. Monthly beach wrack growth production potential in the Baltic Sea by assessing macroalgal biomass amounts.
Status and Utilization of Marine Residual Raw Material in Norway
In Europe, Norway is the major producer of seafood and generates large quantities of residual raw material. Thus, ensuring sustainable harvesting and utilization of the marine resources is at the Norwegian governance strategic forefront (Ween et al., 2018; Ministry of Trade, Industry and Fisheries, 2019). Therefore, advances in the utilization of residual raw materials have been surveyed over several years under the Norwegian Seafood Research Fund (FHF) and earlier through the RUBIN Foundation (Recycling and Utilization of Organic By-Products in Norway). The surveys have given the industry players a better overview of the flow of goods and the possibilities for increased growth and value creation in the seafood sector.
The residual raw materials are defined as the non-primary products obtained from marine raw materials, which are fish and shellfish (crustaceans and molluscs), and seaweeds farmed and caught under Norwegian quotas in Norwegian waters. Opportunities and challenges of three specific sectors of marine waste (fish, shellfish, and seaweed waste) are presented in Table 5, as well as discussed in subparagraphs below.
Norwegian Fish and Shellfish Production Waste for Added Value Products
In Norway, most of the residual raw material from fish and shellfish is currently used, making an important contribution to value creation in the fisheries and aquaculture industry (Johansen et al., 2019). In 2020, about 861,000 tons (85%) of the available residual raw material was utilized to produce various products (Myhre et al., 2021). Large volumes are utilized to varying extents between the different sectors, from 62% in the shellfish industry to almost 100% in the pelagic sector. Norway is currently the largest producer of Atlantic salmon, yielding 93% of total Norwegian aquaculture production (FAO, 2016). In the aquaculture sector, there are strict rules for processing and handling waste in production, and in principle, all biological material is processed. The only fraction that is not being economically exploited is the blood from the slaughter process, for which there is still no usable technology. While in the other marine production sectors (e.g., fisheries), there are large seasonal fluctuations that give variation in access to residual raw material, the residues from the aquaculture industry arise as a relatively steady stream throughout the year. The growth in salmon aquaculture production and the increased use of recirculating aquaculture system (RAS) technology has led to an increased focus on the exploitation of solid waste (feed spill and faeces) from Norwegian aquaculture (Brod et al., 2017; Estevez et al., 2019; Meriac, 2019). The sludge from most land-based aquaculture and smolt production is commonly used for biogas production, and the remaining fraction after biogas production is used as a soil enhancer (Aas and Åsgård, 2017). The production of bioenergy from silage of dead fish from the Norwegian aquaculture industry has increased significantly in recent years. However, in open sea cages, no technology for sludge collection currently exists, and unless resource-efficient solutions like integrated multi-trophic aquaculture (IMTA) are applied, valuable resources are lost. Recently there has also been an increasing focus on producing organic fertilizers from fish waste from captured fish, promoting the recycling of nutrients from the sea and back to terrestrial environments (Ahuja et al., 2020).
Products based on marine residues are mainly used for the feed market (69%), direct and indirect human consumption (13%) and energy/biogas (19%) (SINTEF Ocean and Kontali Analyse, 2020; Myhre et al., 2021). Most marine residues from the production of pelagic fish and aquaculture are used as silage, fishmeal, fish oil and fish protein concentrate. The feed market is the most important application when it comes to volume. Consumer products also include flavorings in foods (extracts) and ingredients for functional food. Other products, for example, cosmetics, nutraceuticals and pharmaceutical products, are produced to a very modest extent from Norwegian based raw material (Pleym et al., 2019).
Norwegian Seaweed Production Waste for Added Value Products
In 2017, over 32.6 million tons of brown, red and green seaweed were produced from capture and aquaculture worldwide, with an annual increase of around 7% in the last 10 years and an average value of 400 USD/ton DW (dry weight) (Buschmann et al., 2017), mainly from the aquaculture sector. On the contrary, the European seaweed industry is mainly based on harvesting of natural resources, as the aquaculture of seaweed is still on the experimental and pilot-scale levels. The European seaweed capture has been stable with around 270,000 tons since 1960, in which Norway contributed with an average of 59.6% (FAO, 2021). Norwegian products from wild-harvested seaweed vary from meal or extracts to highly technological pharmaceutical products. The Norwegian seaweed industry has a long history and relies mostly on the wild harvest of two species: Laminaria hyperborea for alginates production and Ascophyllum nodosum for meal and extracts used for agricultural/horticultural and food or feed supplement purposes. The total harvest of A. nodosum and L. hyperborea combined in 2019 was 149,876 tons, with a first-hand value of 4.06 million EUR. Minor amounts of other seaweed species such as Ulva sp., Himanthalia elongata, Vertebrata lanosa, Palmaria palmata are harvested by hand and sold as a whole, locally to restaurants or directly to the consumer and result in almost no waste.
During the harvesting of A. nodosum, by-catches, mostly composed of Fucus species, are occasional and separated manually and processed further, mostly as seaweed extracts for agricultural use. During the A. nodosum biomass processing, low quantities of waste are produced. For example, gravel and sand are removed from the seaweed using high-pressure air and up to 82% of the moisture is removed by drying the wet biomass directly in a drum. After processing of A. nodosum into meal, 50% of the meal is further processed into extracts, together with fresh seaweed. The remaining material after extraction is used as fertilizer by local farmers (∼750 tons/yr). On the contrary, the industrial exploitation of L. hyperborea generates more waste. Alginates can constitute up to 40% of the dry weight of L. hyperborea (Gunn.) and are extracted in over a hundred different qualities for a broad range of applications. The factory DuPont is licensed for the production of 6,000 tons of alginate and has permission to release the liquid product containing stone dust and kelp dry matter into the seawater, while alginate dust is released to the air. Filtration of the production liquid to collect the rest of the material for soil improvement has been tested but not proven to be cost-effective.
As the aquaculture seaweed industry is still immature (Araújo et al., 2021), minimal investigation has been made to estimate the waste types (biomass or processing liquid). Current commercial seaweed farming in Norway is limited to the kelp species Saccharina latissima (sugar kelp) and Alaria esculenta (winged kelp) due to their ability to reach high biomass yields and a favorable content in nutritional and bioactive compounds with multiple industrial applications (Stévant et al., 2017; Broch et al., 2019). The loss during harvesting is estimated to be around 30% and measured losses of up to 40% when seaweed biomass is frozen and thawed (e.g., Emblemsvåg et al., 2020). To optimize the productions and have a minimum impact on the environment, various wastes generated during this novel type of production would need to be identified further to define the volume and develop innovative uses of these wastes.
Marine Waste in Scotland: Opportunities and Challenges
The Scottish aquaculture industry is dominated by farmed Atlantic salmon. However, rainbow trout, mussels and, more recently, seaweed are gaining attraction as well. Scottish government recognizes the importance of marine resources and has set a target to double the economic contribution of aquaculture from 1.8 billion GBP in 2016 to 3.6 billion GBP by 2030 and also to double the number of jobs to 18,000 by 2030 (Scotland Food and Drink, 2016).
However, aquaculture and fisheries also generate leftover residues and waste such as fish trimmings (guts, heads, tails, frames, and skin), by-catch, aquaculture mortalities, shells and various leftover biomass. This residual biomass can be divided into three categories: (a) co-products which contribute to the profit of the business; (b) by-products that do not generate substantial income but are cash-neutral when accounted for disposal costs; and (c) waste which costs business money to dispose of (Zero Waste Scotland, 2015). As with any market, the costs associated with the above categories fluctuate (e.g., cost of landfill) and are subject to policies and reforms.
In 2015, Zero Waste Scotland (a not-for-profit environmental organization funded by the Scottish Government and European Regional Development Fund) conducted a study, ‘Sector Study on Beer, Whisky, and Fish,’ to evaluate waste practices in Scotland. Their report identified several opportunities in the marine waste sector based on extracting value from leftover residue. However, the authors also highlighted a need for (a) coordinated and staged development of biorefinery strategy; (b) locally adapted innovative low-tech solutions, suitable for small scale and rural areas; (c) cross-sector awareness raising; (d) bioresources mapping; and (e) efficient data recording and sharing. Opportunities and challenges of three specific sectors of marine waste (fish, shellfish and seaweed waste) are presented in Table 6, as well as discussed in subparagraphs below.
Fish Waste
Some fish are immediately frozen upon arrival and exported as a whole, so the processing occurs elsewhere. Nevertheless, most fish are processed locally, which generates a significant amount of waste. During processing, some trimmings (guts, heads, tails, frames, and skin) are removed. Scotland-wide fish processing waste was estimated at 160,000 tons (Pitcairn et al., 2017). While there are well-established international markets for various fish parts in West Africa and East Asia, it is currently not cost-effective to transport fish waste long distances. However, some Scottish fish waste is exported shorter distances and processed into higher-value products (e.g., fish by-products processed in Norway) (Zero Waste Scotland, 2015).
Fish Waste Opportunities
Fish waste can be minced and sold as fish meal, used in animal feed. In Scotland, most fish waste (75%) that is being re-used is sold for blending into aquaculture feeds. Other lower value markets include pig and poultry feeds and pet food (Zero Waste Scotland, 2015). Aquaculture feed in Scotland is custom formulated to address the nutritional needs of specific fish species and their growth stages. Almost all feeds are a blend of materials. The main requirement is to feed with a high content of protein and ω-3 fatty acids. Physical properties are also considered, and losses during feeding are reduced by increasing pellet digestibility and decreasing the speed of sinking in the water. Intra-species recycling (e.g., salmon waste being recycled back into salmon feed) is not permitted under the EU Animal By-Product Regulation (ABPR); therefore, the dominant source of protein and oils in salmon feed is from other wild fish. Other cheaper feed materials (soy, corn, wheat, plant-based oils) are being blended to reduce the overall cost of fish feed, so the ratio of vegetable meal to fish meal increases. Currently, about 15% of fish feed is recycled into fish meal, and fish feed in Scotland remains more expensive (up to 40% in 2014) than in other countries such as Norway (Zero Waste Scotland, 2015).
Various innovative fish waste applications were identified in the ‘Sector Study on Beer, Whisky, and Fish’ report (Zero Waste Scotland, 2015). These include composting, land spreading and organic fertilizers (taking advantage of high nitrogen and phosphorus content), anaerobic digestion and biodiesel production (suitable for fish mortalities that cannot enter any other value chain), protein hydrolysis (protein and peptone refining techniques), a specialist market of manufacturing fish glue, extraction of collagen, guanine, enzymes, carotenoids, and hydroxyapatite. The number of these innovative applications suggests that there is an economic and environmental opportunity for utilizing fish waste in Scotland. However, these innovative uses have been tested only on a small scale in Scotland and generally have a low technology readiness level.
Currently, there are about 12 companies in Scotland focusing on recycling and use of fish waste. These include the production of fish meal, biofuel, fish leather, new foods, oils, and fertilizers. There is a growing demand for bio-based products and renewable energy. Naturally, the demand is driven by price and quality. In 2015, the price range for fish waste was 130–160 GBP/ton of pelagic waste (Zero Waste Scotland, 2015).
Fish Waste Challenges
There are many challenges associated with fish waste processing. Fish industries are often focused on their core and profitable business and lack the incentive to investigate other applications for their waste, especially if disposable is cheap and easy. Any new technology is often profitable only at a large scale, which is not suitable for small, disperse and rural fishing operations in Scotland. There is also an issue of seasonality and perishability (some products and wastes are available only in certain months), which lowers the steady input and consistency of the feedstock. Similarly, biological waste often consists of multiple residues and potential contamination, while it is cost-prohibitive to pretreat the waste before processing. For example, cod liver can be utilized to extract valuable oils, but is often damaged during processing. Similarly, fish skin used for gelatin extraction must be meat-free, which is not a priority during processing. Complicated waste regulation also presents another obstacle because businesses are reluctant to study different regulatory approaches, which is having a negative impact on their decision-making regarding fish waste.
Shellfish Waste
Shellfish production in Scotland is dominated by mussel (Mytilus spp.) and pacific oyster (Crassostrea gigas), with 6,699 tons and 4,610 tons produced, respectively. Scallop (Pecten maximus), queen scallop (Aequipecten opercularis) and native oyster (Ostrea edulis) are also produced, but at a smaller scale (not more than 150 tons combined). Cultivation of common periwinkle (Littorina littorea) was also recorded. In 2019, the total value for all species combined was approximately 7.9 million GBP (Munro, 2019).
Shellfish are shipped away as a whole, which practically eliminates any significant waste stream. Shellfish farmers have, therefore, a small incentive to even investigate any uses for shellfish waste and prefer local disposal.
Waste from crustacean (crabs, lobster, shrimp, etc.) is more dominant than the others. Shells and/or carapaces with no signs of diseases are often applied as organic land fertilizers. In 2008, Scotland produced 3,400–7,000 tons of crab waste and 6,500–13,000 tons of nephrops (Nephrops norvegicus) (Archer and Russel, 2008). The exact information is difficult to access; hence waste generations are largely broad estimates.
Innovative applications from shellfish waste include composting (Lanno et al., 2020), anaerobic digestion, processing into agricultural lime fertilizer, using shells as a calcium source for animal feed, enzyme extraction from viscera and using shells as aggregate for building applications and track surfacing. All the shells must be flesh-free, which is often difficult to achieve cost-effectively. Crustacean waste can be used to produce chitin (which is a well-established global industry), in fish feed, as an ingredient in pet food, to extract carotenoids, to produce stock for flavoring for human consumption, and use as fertilizer and a pesticide (e.g., reduce nematode presence) (Zero Waste Scotland, 2015).
Seaweed Waste
In Scotland, seaweeds are wild-harvested and have been used locally in small quantities for feed, food and fertilizers for centuries; however, large-scale seaweed cultivation is only recently being developed (Pitcairn et al., 2017). Several commercial and research farms (Hebridean Seaweed Company Ltd and Scottish Association for Marine Science) are actively pioneering the industry. Biorefinery report (Pitcairn et al., 2017) has estimated 8-10 million tons/yr of wild, easily and sustainably harvestable seaweed. Seaweed farming is also becoming more popular.
Overall, the most targeted species are S. latissima, A. esculenta, P. palmata, L. hyperborea, and A. nodosum. The seaweed industry creates significant opportunities in Scotland from seaweed applications in food, feed, fertilizers, anaerobic digestions, nutraceutical and pharmaceutical industry, while macroalgae benefit the environment by sequestering CO2 and absorbing nutrients from the water during growth. Similarly, microalgae have also been recognized for their potential in all application fields listed above and wastewater bioremediation (Scottish Enterprise, 2019). The benefits of IMTA, which includes seaweed, are also being investigated. The socio-economic opportunities and potential of the budding seaweed industry in Scotland are considerable and generally recognized. Scottish Enterprise (2019) estimated that high-value products from seaweed (specifically L. hyperborea) could contribute up to 300 million GBP/yr by 2030.
A recent review by Campbell et al. (2019) highlighted the environmental risks and knowledge gaps associated with large-scale seaweed farming in Europe. The authors identified several areas of concern, including facilitation of disease, alteration of population genetics and broader alterations to the local physiochemical environment (e.g., increased noise, altered nutrient fluxes and flow, impact on benthic species, etc.) (Campbell et al., 2019). Current small-scale farms in Scotland were identified as low risk; however, a transition to large-scale cultivation requires more research and monitoring efforts to fully understand the environmental implications and evaluate the balance between environmental risks and benefits of large-scale seaweed cultivation.
Side Stream Valorization in the Baltic Sea
Beach wrack has ecological functions such as providing food and habitat for sandy beach fauna, nutrients for dune vegetation, and protection for coastal dunes. In the framework of the INTERREG project GRASS (Table 7), existing environmental data and expert opinions were gathered to model beach wrack production potential in the Baltic Sea region. A higher amount of beach wrack is expected in the late autumn months and the early winter, along with the end of production season and the onset of heavier storms. High beach wrack production is predicted at shores that have a narrow photic zone (i.e., distance to the 10 m isobath less than 1 km) and are exposed to favorable wave direction. Moreover, higher solar radiance and water salinity are associated with elevated beach wrack.
Clear hotspots of beach wrack production and harvest emerged throughout the whole Baltic Sea area (including Kattegat, Figure 4). The highest production values (up to 4,000 g per m2 per month) were observed on the west and east coasts of Sweden, all along the southern coast of Finland, west coast of Estonia and in Gdansk Bay. However, some production hotspots were sporadically found even on the east coast of Finland, reaching northernmost parts of the Bothnian Bay as well as on the shores of St. Petersburg. The remaining parts of the Baltic Sea were characterized by lower beach wrack production potential (approximately 0–1,000 g per m2 per month).
Along most of the coast, the beach wrack that is deposited on the beaches does not unduly affect the people who live close by. However, in certain areas, a proportion of the wrack moving onshore is permanently trapped. It creates problems not only for inhabitants of those areas, local authorities responsible for maintaining the beaches or beach visitors (Kataržytė et al., 2019), but also for the local beach ecosystem. Seagrass and algae wrack, during decomposition, release several constituents, which alter the coastal biogeochemical cycles and influence organisms living there. These include nutrients and dissolved organic carbon, which affect flora and microbial activity, and heavy metals (in polluted systems), creating a risk for biota (Rudovica and Bartkevics, 2015). Also, the emission of volatile components from decaying plant material might represent a risk for human health (H2S, Hg0, Cs–137), as well as for climate change (by CH4 emission). Hence, beach wrack is the subject of several research projects (Table 7).
Pollution Prevention
Anthropogenic mercury release remains a problem in the aquatic environment and, based on the sedimentary records in the Baltic Sea, it exceeds Hg coming from natural sources (i.e., hydrothermal processes and rock weathering) by a factor of 5 on average. Recently, the emission of this metal to the environment has substantially decreased (Helsinki Commission, 2009, 2018; Kwasigroch et al., 2021). This has resulted in a noticeable decrease of mercury concentration in macrophytes in the Polish coastal zone of the southern Baltic (Bełdowska et al., 2015, 2016). In parallel, the intense growth of some macrophytobenthos on the sea bottom has been observed in many areas (Carmen et al., 2019; Sokołowski et al., 2021). This is stimulated by an improvement of environmental conditions and lengthening of the growing season. It leads to the rapid inclusion of mercury from the water column (which is introduced from natural and anthropogenic terrestrial sources) and from sediments (which was deposited in the past and can be considered retarded anthropogenic emission) (Bełdowska et al., 2015).
In many areas of the Baltic Sea, due to the pattern of currents and shape of the coastline, large quantities of macrophytobenthos gather in the coastal zone or end up as beach wrack. During the summer season in the Gulf of Gdansk, on 1 km of the beach, the amount of beached seagrass and algae wrack ranges from several dozens to 800 tons (Filipkowska et al., 2008; Weinberger et al., 2020). Considering median total Hg concentration (7.6 ng/g dry weight), it has been calculated that a beach segment that is 1 km long may receive 6 g of mercury per season. Analyses of coastal erosion in the Southern Baltic show that about 39% of the Polish coast is accumulative (Dubrawski et al., 2008). It means that about 200 km of coastline favors phytobenthic accumulation. During the summer season, benthic plants on Polish beaches alone may contain 0.05–1.2 kg of Hg (Bełdowska et al., 2015).
A recent study performed within the CONTRA project in the Puck Bay (sheltered part of Gulf of Gdańsk), Poland, indicate that the concentrations of Hg in the managed beach (P1), where live algae occur, were lower than those collected in the unmanaged site, where decomposing wrack was collected (sampling sites R1 and R2, Figure 5). However, in the unmanaged station, the concentrations of Hg in live algae (sampling site R3) were similar to those at the managed site. This indicates that although biological material from the bay accumulates Hg at the same rate and is characterized by the same mercury concentration in both sites, accumulation does not stop on landing. Decomposing beach wrack in the unmanaged site is rich in organic matter and continuously builds up Hg concentration. This is probably caused by the excellent sorption capabilities of decaying plants and algae material that may capture Hg from coastal water, acting as a filter for surface waters. Another explanation is the Hg capture from the atmosphere, where it originates in low emission from local sources (Bełdowska et al., 2014). This means that unmanaged beaches may not only transfer Hg from beach wrack via accumulation into live algae and subsequent release, but also enhance Hg flux to the beach from local sources.
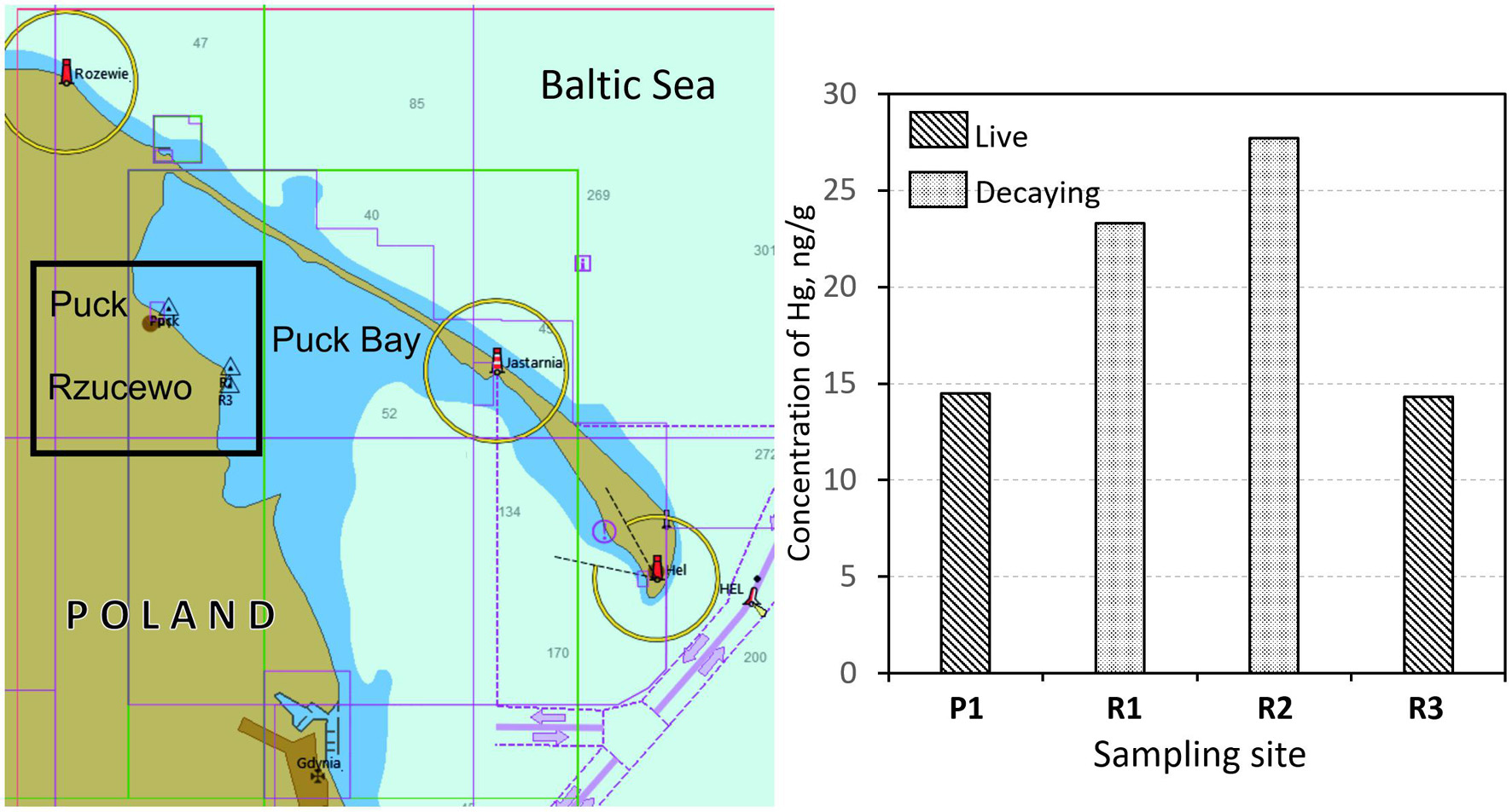
Figure 5. The study area (left) and total mercury concentration (right) in live algae at the managed site (P1) and unmanaged site (R3), decaying beach wrack at the unmanaged site (R1, R2).
Besides pollutants, nutrients are also removed from water by algae and marine plants, which are later released from decomposing beach wrack. Decomposition of organic matter at the bottom causes a higher concentration of phosphate and ammonia in porewaters than nearbottom waters (Graca et al., 2006). Porewaters, collected from areas beneath decaying beach wrack, had similar phosphate and ammonia concentrations (phosphate, p = 0.86; ammonia, p = 0.46) as porewaters in the coastal zone sediments, but higher than those in nearbottom waters (phosphate, p = 0.003; ammonia, p < 0.01) (Figures 6A,B).
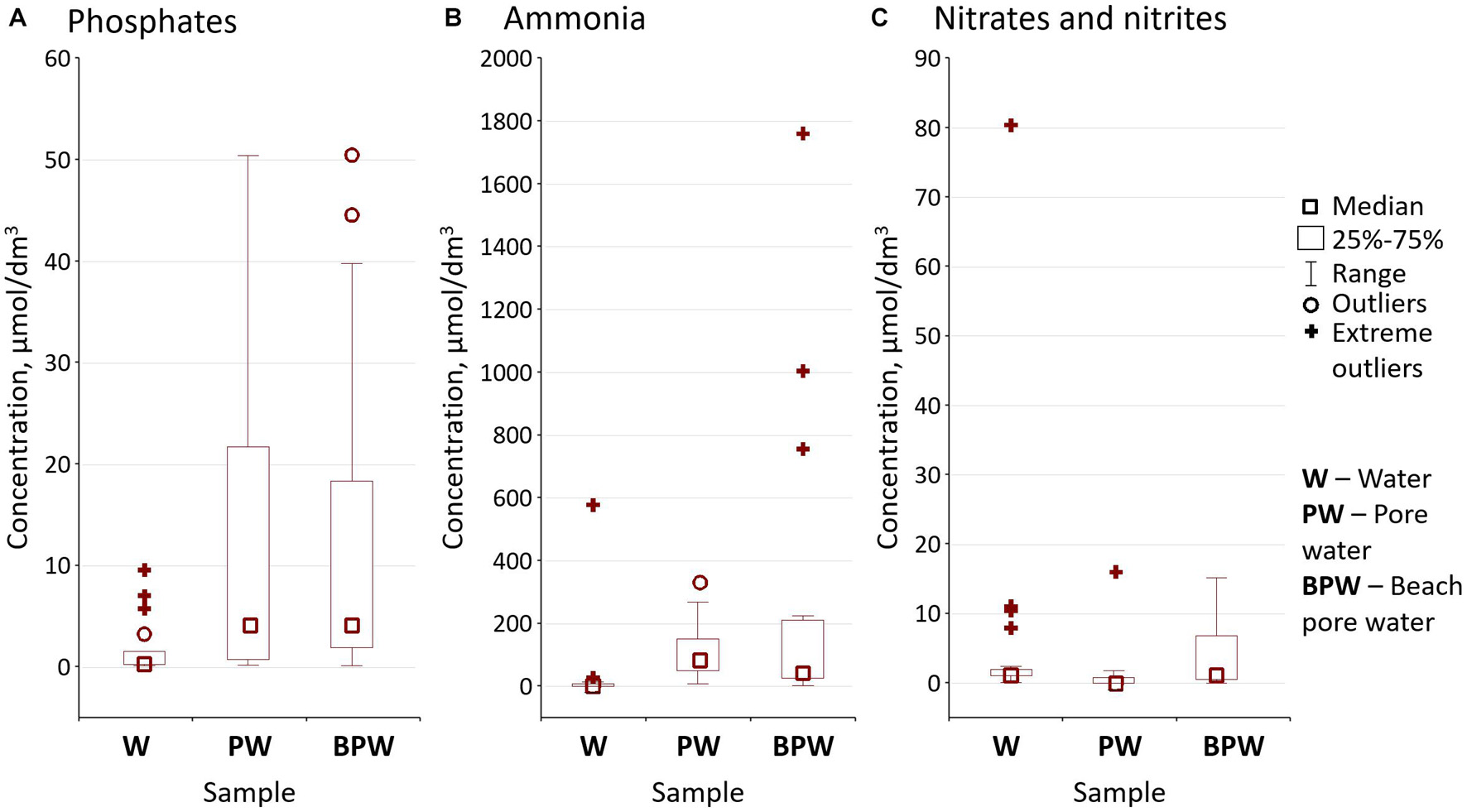
Figure 6. Concentration of (A) phosphates, (B) ammonia, and (C) nitrates + nitrites in water, porewater and beach porewater (sampled from under the detritus) from April (2019) to November (2019) at three sampling sites (P1, R1, R3) in the Bay of Puck (Poland).
Moreover, in porewaters collected beneath beach wrack in unmanaged beaches (sampling sites R1, R3), the median concentration of phosphate and ammonia equaled to 14.5 and 140.9 μmol/dm3, respectively. These concentrations were over three times higher than those from the managed beach in the same area (P1). This suggests that phosphates and ammonia released from decaying beach wrack are partially retained in the beach porewaters. However, in the longer perspective, they return to coastal waters, where they fuel primary production. One ton of dry beach detritus weight contains from 0.5 to slightly above 3 kg of phosphorus and from 5 to 32 kg of nitrogen. Such a load, delivered to sea water, is potentially responsible for the production of 0.5–3 tons of phytoplankton biomass.
Nitrates are also being produced in porewaters. They reach the highest concentration close to the surface, in oxygenated layers. In deeper layers, they are consumed by denitrification (Behrendt et al., 2013). In the study area, nitrate concentrations in porewaters collected beneath decaying beach wrack were similar to those observed in the water column (p = 0.811) and significantly higher than those in porewaters of coastal sediments (Figure 6C). The conditions below decaying beach wrack favor nitrification, which unfortunately reduces the nitrogen removal (in its gaseous form) from the water.
These results indicate that beach wrack removal from the beach can prevent both – pollutants and nutrients scavenged from plants and algae during their lifetime in the sea from re-entry to the coastal waters. Therefore, using beach wrack as a resource could contribute to the clean-up of the marine environment.
Gasification/Torrefaction
As a prospective solution for beach wrack from the Baltic Sea coast, processing, gasification and transformation to biochar has been demonstrated based on an experimental study and pilot-scale tests. Gasification is a chemical process that converts carbonaceous material, such as biomass and coal, into gaseous fuel or chemical feedstock (Basu, 2010), differing substantially from other thermal processes, such as incineration or pyrolysis (Porshnov et al., 2018). This gaseous fuel is known as producer gas or synthesis gas (syngas) containing CO2, H2, CO, H2O, CH4, and N2. Surplus char, formed from the pyrolysis process, is heated by supplying a limited amount of air in the gasifier. Beach wrack sampled from several sites along the Baltic Sea coastline contains relatively high amounts of plastic residues (up to 5%) and inorganic material (ash up to 30%). However, thermogravimetric and proximate/ultimate analysis on beach wrack demonstrates relatively high carbon amounts (up to 30%) with low organic chlorine and sulfur concentration. The highest heating value ranges 8–15 MJ/kg, thus proving the potential to use it for energy production purposes. Also, trace elements and heavy metal concentrations are low in beach wrack (Burlakovs et al., 2019).
The choice of thermochemical conversion technology was driven by the specific nature of the beach wrack. Beach wrack gasification tests were performed on an innovative gasification plant for pyrolysis of various wastes and the thermal cracking of pyrolysis gas products. The apparatus consists of an extruder-type pyrolizer/gasifier, a pyrolysis product separation chamber, a thermal cracker for gaseous pyrolysis products and a gas burning torch (Figure 7). The gasification process does not use air or oxygen as a gasification agent. The process is allothermal in nature, using an external heat source, and the system is used in continuous operation mode. In the extruder-type pyrolyzer (3), the fuel is compacted. The operating temperature of the extruder is set and automatically adjusted to 300–600°C. The primary reforming of the fuel into pyrolysis gas and coal is carried out in the extruder. In the pyrolysis product separation chamber (4), the pyrolysis gas is separated from the coal. The carbon is stored in an airtight container. After cooling, the coal is unloaded from the container and sent to a laboratory for analysis. The pyrolysis gas is fed to a secondary high-temperature reformer (6), where the pyrolysis gas is heated to 800–1200°C. At elevated temperatures, a high turbulent tar thermal cracking occurs, and heavy organic gaseous substances are reformed into the synthesis gas components, such as H2, CO, CO2. At the output of the secondary reformer, the gas is cooled, and the heat consumed in the process is recovered. The resulting synthesis gas has a high concentration of CH4 (up to 60%), but the obtained waste char can be used as biochar or as fuel to replace fossil analogs.
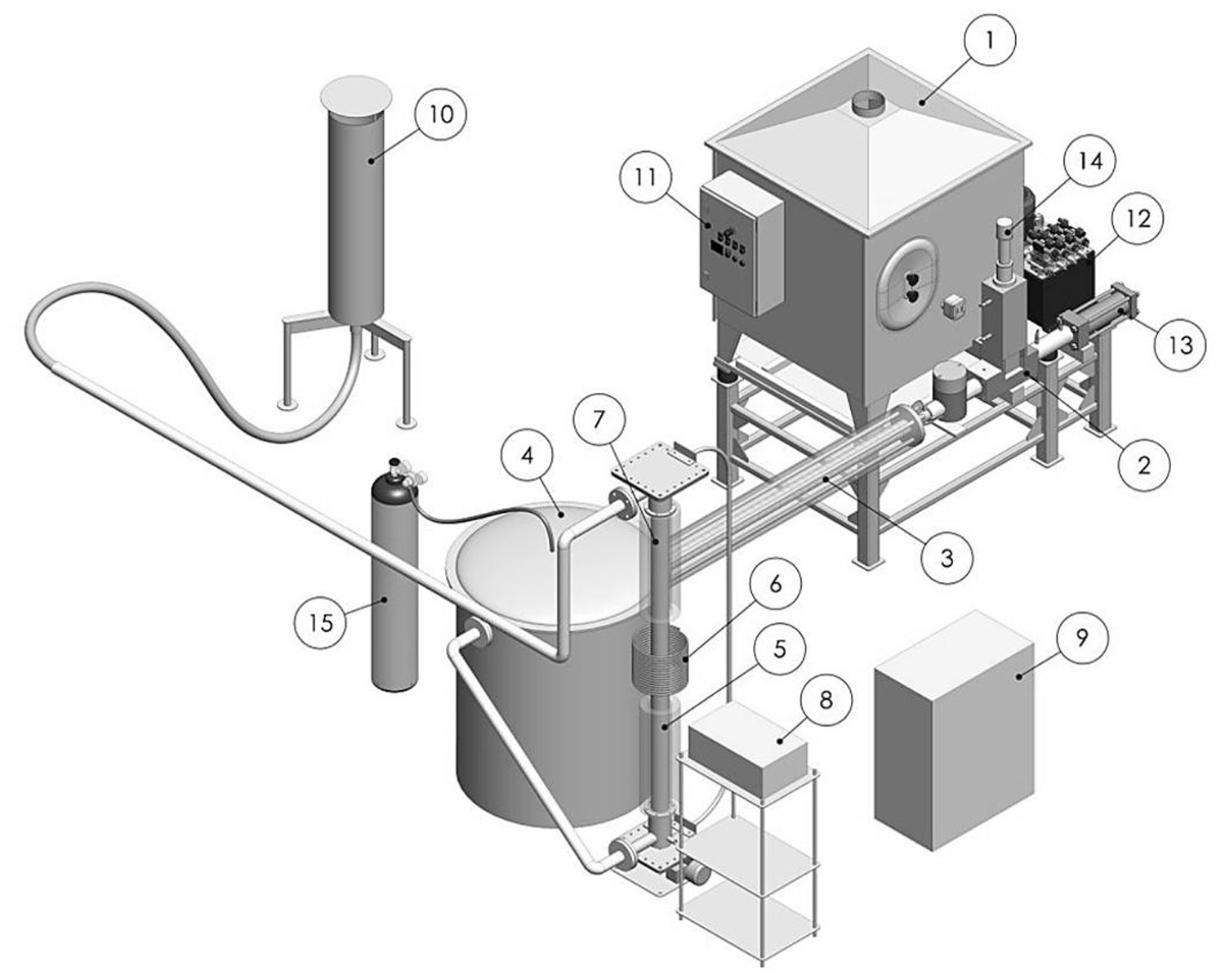
Figure 7. Construction of experimental gasification plant (Bisters et al., 2021): (1) feedstock bunker; (2) hydraulic press feeder; (3) extruder-type pyrolizer; (4) separation chamber – gas and char accumulation tank; (5) secondary gas cracking; (6) external inductive heater – temperature reformer; (7) gas cooler; (8) inductive heater resonator; (9) inductive heater power box; (10) flare; (11) control cabinet; (12) hydro-station; (13) hydro-cylinder; (14) prior hydro-presser box; (15) nitrogen balloon.
Anaerobic Digestion
To test the biogas potential obtained from different Baltic Sea coastal beach wrack, three experimental studies were conducted using anaerobic digestion methods. The first two tests were undertaken for three types of selected beach wrack algae from the Riga Gulf coast in Latvia and a mixed sample from the Kalmar coast in Sweden. Anaerobic fermentation (Figure 8) was applied for the samples without specific pre-treatment. In the first study, 16 bioreactors operated in batch mode at 38°C were used to ferment the three common algal types available as beach wrack in the Gulf of Riga. Testing samples were taken from the beach wrack piles in Jaunkemeri, Bigaunciems, and Ragaciems coastal areas in Latvia.
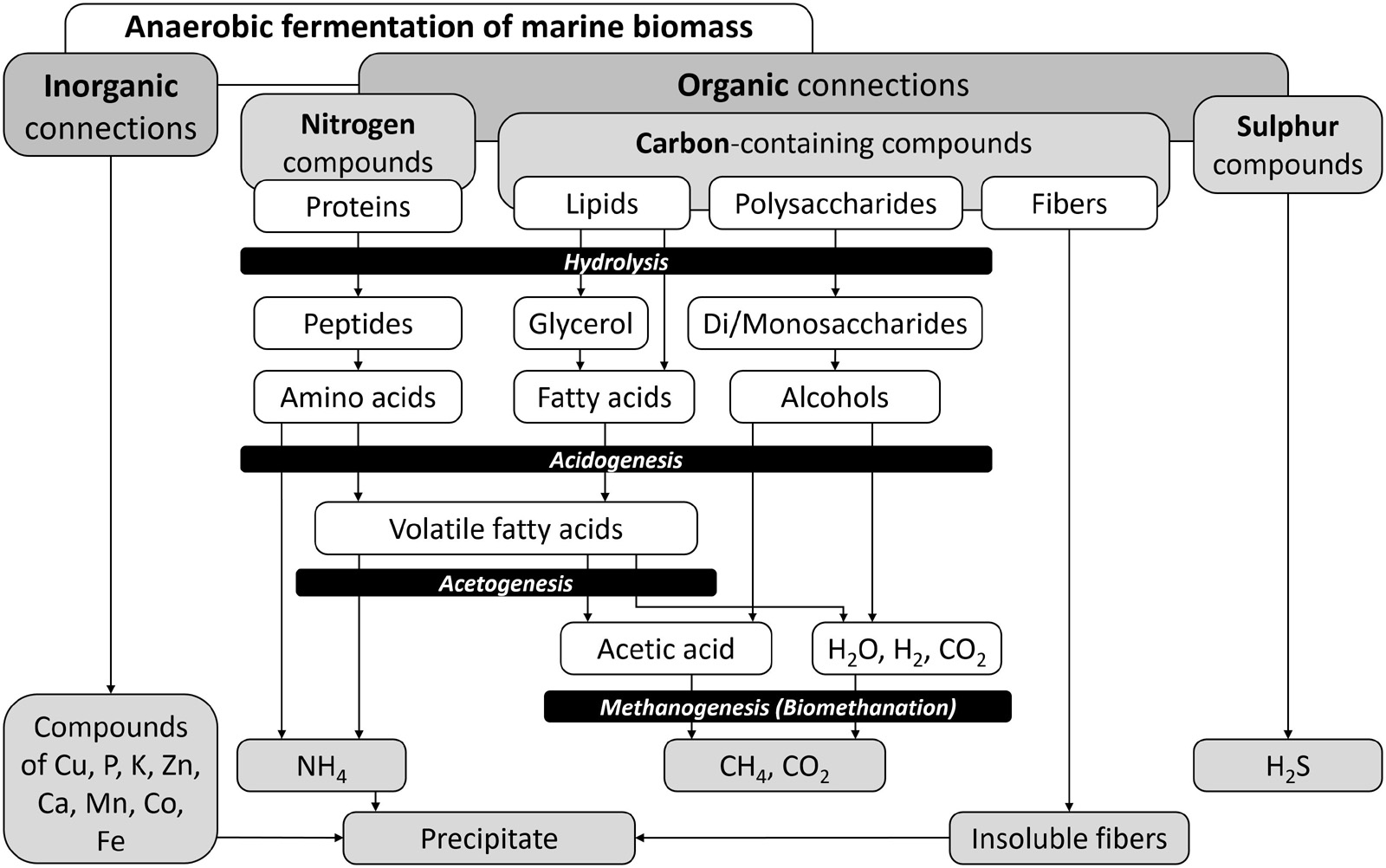
Figure 8. Schematic overview of the anaerobic fermentation of marine biomass, indicating organic and inorganic connections, the main chemical transformation of compounds and resulting intermediate and final products.
From fermentation of 32 days, 0.276 L/g DrOM (dry organic matter) biogas (0.046 L/g DrOM methane) from brown algae and 0.248 L/g DrOM biogas (0.027 L/g DrOM methane) from red algae were obtained. From green algae, 0.425 L/g DrOM biogas (0.071 L/g DrOM methane) were obtained on average. The study showed that from the coast-washed beach wrack, a small amount of methane can be generated per DrOM if there is no pre-treatment and conditioning of the samples. From the mixed sample, due to a higher presence of debris and lignocellulose feedstock, the biogas yield with applied anaerobic fermentation method also shows limited biogas potential.
Further study for measuring brown algae biogas production was tested with three pre-treatment methods: algae being kept for 24 h in tap water, washed for 1 h in a stream of running fresh water and dried. The resulting methane quantities were compared with those obtained from the raw brown algae. From algae that were kept in tap water for 24 h, 0.560 L/g DrOM biogas (0.198 L/g DrOM methane) was obtained, and from the ones washed 1 h in running water, 0.569 L/g DrOM biogas (0.211 L/g DrOM methane) was obtained, but from dried mass, only 0.164 L/g DrOM biogas (0.065 L/g DrOM methane) was gained. The study confirms that washing brown algae as a pre-treatment for anaerobic fermentation avoids salts inhibition and can perform better in biomethane production.
Biogas (methane), generated from brown algae in the study without special pre-treatment, is on average 0.276 L/g DrOM (0.046 L/g DrOM), which is a very low yield, and from red-brown algae in the study without special pre-treatment is on average 0.248 L/g DrOM (0.027 L/g DrOM). Red-green algae in the study without special pre-treatment acquired an average of 0.425 L/g DrOM (0.071 L/g DrOM), which is slightly better but still represents a relatively low result. Biogas (methane) generated from mixed algae with coastal reed mixture in the study without special pre-treatment on average shows 0.267 L/g DrOM (0.32 L/g DrOM), which is a comparably low yield. The retention of brown algae samples in water for 24 h resulted in about 63.6% more methane in the DrOM compared with unwashed brown algae. In the second brown algae study, sample pre-treatment in running water washed for 1 h gained about 74.4% more methane out of DrOM than units from unwashed brown algae. The test results have shown that rinsing seaweed before feeding into anaerobic digestion is preferable to achieve better yields. In cases where it is not possible to apply the described methodology, biogas/methane yields will be negligible, and recovery of the waste into biogas will not be economically feasible. With the pre-treatment of brown algae in running water, the feedstock is well suited for optimal volume biogas generation for energy recovery and use in other bio-SNG (synthetic natural gas) applications. With or without pre-treatment, seaweed biomass can be used in the co-fermentation of other waste streams like sewage sludge or manure. Such co-fermentation will optimize the C:N ratio and will neutralize the inhibiting effect. For clarification of the optimal process parameters, further studies and tests are recommended.
Conclusion
To effectively valorize marine waste, promising perspectives must be considered, namely: (a) the development of mechanical technology for the harvesting of the biomass; (b) the development of chemical and biological pipelines to conserve and/or process marine biomass; (c) the market search to maximize the potential use in the various industries; (d) the employment of communication strategies to raise awareness, increase consumers’ acceptance on the potential greener technologies or public health improvement through enhanced waste-originated food or feed ingredients as well as biomedicals; (e) the promotion of public and private funding toward innovation and technology development, while addressing the potential legislative bottlenecks; and (f) the application of eco-friendly principles to production systems. Even though significant progress has been made in marine-derived biomass research and innovation, there is still the unmet need for technology scale-up and the establishment of business opportunities to promote sustainability and circular economy. Hence, the valorization of waste into useful products entails a tight collaboration between industry and research sectors, as well as governance bodies. It is reflected in the governance promotion of resource efficiency in Europe by several European policy initiatives, the most recent one being the European Green Deal, which is Europe’s roadmap for a sustainable economy. The use of bioactive compounds, valorized from side streams and waste, will significantly contribute to global environmental sustainability as waste valorization companies contribute to a green, blue and circular economy. These companies should include a socially responsible connotation and provide an alternative source of income for communities that are heavily dependent on fisheries and aquaculture. Therefore, future research should, on the one hand, target the optimization of side streams processing into useful products, and guarantee a sustainable supply and product quality on the other hand, regardless of the season or geographical location.
Author Contributions
ViR, AR, and JuB designed the manuscript concept and drafted the manuscript. All authors contributed with their specific field of expertise, read, edited and approved the final version of the manuscript.
Funding
This publication is based upon work from COST Action CA18238 (Ocean4Biotech), supported by COST (European Cooperation in Science and Technology). AR and KK: this research was funded by the Slovenian Research Agency (research core funding P1-0245 and P1-0237). AR: this publication has been produced with financial assistance of the Interreg MED Programme, co-financed by the European Regional Development Fund (Project No. 8MED20_4.1_SP_001, internal ref. 8MED20_4.1_SP_001) – B-Blue project. SG, CT, and JO: this work is financed by national funds from FCT – Fundação para a Ciência e a Tecnologia, I.P., in the scope of the project UIDP/04378/2020 and UIDB/04378/2020 of the Research Unit on Applied Molecular Biosciences - UCIBIO and the project LA/P/0140/2020 of the Associate Laboratory Institute for Health and Bioeconomy – i4HB. JaB and WH: the preparation of the manuscript was supported by the Project CONTRA (Conversion of a Nuisance to a Resource and Asset #R090, 2018–2021) of the INTERREG Baltic Sea Region Program, and Polish Ministry of Science and Higher Education from the 2019–2021 science funding allocated for the implementation of international co-financed project W24/INTERREG BSR/2019. Research of Maris Klavins, VB, and LA was supported by ERDF project 1.1.1.1/16/A/050 “Variable fuel gasification for municipal solid waste recovery.” MC acknowledges the funding from CEEC program supported by FCT/MCTES (CEECIND/02968/2017) and Strategic Funding UIDB/04423/2020 and UIDP/04423/2020 supported by national funds provided by FCT and ERDF. AD acknowledges financial support provided by European Union’s Horizon 2020 research and innovation program under the grant agreement No 857287 and Latvian Council of Science research project No. lzp-2020/1-0054. MKa: the Interreg LAT_LIT Programme, co-financed by the European Regional Development Fund (LLI-525 ESMIC). LB acknowledges the funding from Erasmus + Project No. ECOBIAS 609967-EPP-1-2019-1-RS-EPPKA2-CBHE-JP; GA.2019-1991/001-001. Development of master curricula in ecological monitoring and aquatic bioassessment for Western Balkans HEIs/ECOBIAS. IS and KP acknowledge financial support provided by the projects CZ.02.1.01/0.0/0.0/17_048/0007323 and CZ.02.1.01/0.0/0.0/16_019/0000754 (Ministry of Education, Youth and Sports of the Czech Republic). ZV-G acknowledges support within the project No.1.1.1.2/VIAA/1/16/029 (Formula of peat-free soil conditioner with controlled-release fertilizing effect applicable for soil remediation and quality improvement of agricultural production). IZ: the projects SLTKT20427, KIK 17431 and SARASWATI 2.0. JuB: the project No.1.1.1.2/VIAA/3/19/531 (Innovative technologies for stabilization of landfills – diminishing of environmental impact and resources potential in frames of circular economy). The work conducted by CR, LA-H, and MA was fully financed by Møreforsking AS.
Conflict of Interest
The authors declare that the research was conducted in the absence of any commercial or financial relationships that could be construed as a potential conflict of interest.
Publisher’s Note
All claims expressed in this article are solely those of the authors and do not necessarily represent those of their affiliated organizations, or those of the publisher, the editors and the reviewers. Any product that may be evaluated in this article, or claim that may be made by its manufacturer, is not guaranteed or endorsed by the publisher.
Acknowledgments
Special thanks are addressed to Amanda Ingram (Zero Waste Scotland), Tracey Begg (Scottish Natural Heritage), Iona Campbell (SAMS) and Marine Scotland for the help with compiling the information regarding Scotland, as well as Maris Klavins for help with the gasification chapter.
References
Aas, T. S., and Åsgård, T. E. (2017). Estimated Content of Nutrients and Energy in Feed Spill and Faeces in Norwegian Salmon Culture. [Nofima Report 19/2017]. Tromsø: Nofima.
Abdelhedi, O., Nasri, R., Mora, L., Jridi, M., Toldrá, F., and Nasri, M. (2018). In silico analysis and molecular docking study of angiotensin I-converting enzyme inhibitory peptides from smooth-hound viscera protein hydrolysates fractionated by ultrafiltration. Food Chem. 239, 453–463. doi: 10.1016/j.foodchem.2017.06.112
Abdollahi, M., Alboofetileh, M., Behrooz, R., Rezaei, M., and Miraki, R. (2013a). Reducing water sensitivity of alginate bio-nanocomposite film using cellulose nanoparticles. Int. J. Biol. Macromol. 54, 166–173. doi: 10.1016/j.ijbiomac.2012.12.016
Abdollahi, M., Alboofetileh, M., Rezaei, M., and Behrooz, R. (2013b). Comparing physico-mechanical and thermal properties of alginate nanocomposite films reinforced with organic and/or inorganic nanofillers. Food Hydrocolloids 32, 416–424. doi: 10.1016/j.foodhyd.2013.02.006
Abdul-Hamid, A., Bakar, J., and Bee, G. H. (2002). Nutritional quality of spray dried protein hydrolysate from black tilapia (Oreochromis mossambicus). Food Chem. 78, 69–74. doi: 10.1016/S0308-8146(01)00380-6
Adeniyi, O. M., Azimov, U., and Burluka, A. (2018). Algae biofuel: current status and future applications. Renew. Sustain. Energy Rev. 90, 316–335. doi: 10.1016/j.rser.2018.03.067
Ahrens, T., Drescher-Hartung, S., and Anne, O. (2017). “Sustainability of the biowaste utilization for energy production,” in Biomass Volume Estimation and Valorization for Energy, ed. J. Tumuluru (London: IntechOpen), 165–187.
Ahuja, I., Dauksas, E., Remme, J. F., Richardsen, R., and Løes, A. K. (2020). Fish and fish waste-based fertilizers in organic farming – with status in norway: a review. Waste Manag. 115, 95–112. doi: 10.1016/j.wasman.2020.07.025
Al Khawli, F., Pateiro, M., Domínguez, R., Lorenzo, J. M., Gullón, P., Kousoulaki, K., et al. (2019). Innovative green technologies of intensification for valorization of seafood and their by-products. Mar. Drugs 17:689. doi: 10.3390/md17120689
Al-Enizi, A. M., Zagho, M. M., and Elzatahry, A. A. (2018). Polymer-based electrospun nanofibers for biomedical applications. Nanomaterials (Basel) 8:259. doi: 10.3390/nano8040259
Alfonsín, V., Maceiras, R., and Gutiérrez, C. (2019). Bioethanol production from industrial algae waste. Waste Manag. 87, 791–797. doi: 10.1016/j.wasman.2019.03.019
Angelova, R., Baldikova, E., Pospiskova, K., Maderova, Z., Safarikova, M., and Safarik, I. (2016). Magnetically modified Sargassum horneri biomass as an adsorbent for organic dye removal. J. Cleaner Prod. 137, 189–194. doi: 10.1016/j.jclepro.2016.07.068
Araújo, R., Vázquez Calderón, F., Sánchez López, J., Azevedo, I. C., Bruhn, A., Fluch, S., et al. (2021). Current status of the algae production industry in Europe: an emerging sector of the blue bioeconomy. Front. Mar. Sci. 7:626389. doi: 10.3389/fmars.2020.626389
Archer, M., and Russel, D. (2008). Crustacea Processing Waste Management. [Seafish Research Report SR593]. Edinburgh: Seafish.
Arumugam, N., Chelliapan, S., Kamyab, H., Thirugnana, S., Othman, N., and Nasri, N. S. (2018). Treatment of wastewater using seaweed: a review. Int. J. Environ. Res. Public Health 15:2851. doi: 10.3390/ijerph15122851
Augustine, R., Rehman, S. R. U., Ahmed, R., Zahid, A. A., Sharifi, M., Falahati, M., et al. (2020). Electrospun chitosan membranes containing bioactive and therapeutic agents for enhanced wound healing. Int. J. Biol. Macromol. 156, 153–170. doi: 10.1016/j.ijbiomac.2020.03.207
Aytas, S., Gunduz, E., and Gok, C. (2014). Biosorption of uranium ions by marine macroalga Padina pavonia. Clean Soil Air Water 42, 498–506. doi: 10.1002/clen.201300019
Bãdescu, I. S., Bulgariu, D., Ahmad, I., and Bulgariu, L. (2018). Valorisation possibilities of exhausted biosorbents loaded with metal ions – a review. J. Environ. Manag. 224, 288–297. doi: 10.1016/j.jenvman.2018.07.066
Bãdescu, I. S., Bulgariu, D., and Bulgariu, L. (2017). Alternative utilization of algal biomass (Ulva sp.) loaded with Zn(II) ions for improving of soil quality. J. Appl. Phycol. 29, 1069–1079. doi: 10.1007/s10811-016-0997-y
Badri, A., Raman, K., and Jayaraman, G. (2019). Uncovering novel pathways for enhancing hyaluronan synthesis in recombinant Lactococcus lactis: genome-scale metabolic modeling and experimental validation. Processes 7:43. doi: 10.3390/pr7060343
Balachandar, G., Khanna, N., and Das, D. (2013). “Biohydrogen production from organic wastes by dark fermentation,” in Biohydrogen, eds A. Pandey, J. S. Chang, P. C. Hallenbecka, and C. Larroche (Amsterdam: Elsevier), 103–144.
Barbosa, A. I., Costa Lima, S. A., and Reis, S. (2019a). Application of pH-responsive fucoidan/chitosan nanoparticles to improve oral quercetin delivery. Molecules 24:346. doi: 10.3390/molecules24020346
Barbosa, A. I., Coutinho, A. J., Costa Lima, S. A., and Reis, S. (2019b). Marine polysaccharides in pharmaceutical applications: fucoidan and chitosan as key players in the drug delivery match field. Mar. Drugs 17:654. doi: 10.3390/md17120654
Barbot, Y. N., Al-Ghaili, H., and Benz, R. (2016). A review on the valorization of macroalgal wastes for biomethane production. Mar. Drugs 14, 120. doi: 10.3390/md14060120
Basu, P. (2010). Biomass Gasification and Pyrolysis Practical Design and Theory. Cambridge: Academic Press.
Bay-Larsen, I., Risvoll, C., Vestrum, I., and Bjørkhaug, H. (2018). Local protein sources in animal feed – Perceptions among arctic sheep farmers. J. Rural Stud. 59, 98–110. doi: 10.1016/j.jrurstud.2018.02.004
Bay-Larsen, I., Vestrum, I. K., Lind, V., Risvoll, C., Novoa-Garrido, M., and Roleda, M. Y. (2016). “Traditional to commercial use of seaweeds: Cross-disciplinary perspectives in using local protein sources in Arctic sheep husbandry,” in Proceedings of the 26th General Meeting of the European Grassland Federation, Trondheim, 693–695.
Beaulieu, L., Thibodeau, J., Bonnet, C., Bryl, P., and Carbonneau, M. E. (2013). Evidence of anti-proliferative activities in blue mussel (Mytilus edulis) by-products. Mar. Drugs 11, 975–990. doi: 10.3390/md11040975
Behrendt, A., de Beer, D., and Stief, P. (2013). Vertical activity distribution of dissimilatory nitrate reduction in coastal marine sediments. Biogeosciences 10, 7509–7523. doi: 10.5194/bg-10-7509-2013
Bełdowska, M., Jędruch, A., Słupkowska, J., Saniewska, D., and Saniewski, M. (2015). Macrophyta as a vector of contemporary and historical mercury from the marine environment to the trophic web. Environ. Sci. Pollut. Res. 22, 5228–5240. doi: 10.1007/s11356-014-4003-4
Bełdowska, M., Jędruch, A., Zgrundo, A., Ziółkowska, M., Graca, B., and Gębka, K. (2016). The influence of cold season warming on the mercury pool in coastal benthic organisms. Estuar. Coast. Shelf Sci. 171, 99–105. doi: 10.1016/j.ecss.2016.01.033
Bełdowska, M., Saniewska, D., and Falkowska, L. (2014). Factors influencing variability of mercury input to the southern Baltic Sea. Mar. Pollut. Bull. 86, 283–290. doi: 10.1016/j.marpolbul.2014.07.004
Beni, A. A., and Esmaeili, A. (2020). Biosorption, an efficient method for removing heavy metals from industrial effluents: a review. Environ. Technol. Innov. 17:100503. doi: 10.1016/j.eti.2019.100503
Benito-González, I., López-Rubio, A., and Martínez-Sanz, M. (2018). Potential of lignocellulosic fractions from Posidonia oceanica to improve barrier and mechanical properties of bio-based packaging materials. Int. J. Biol. Macromol. 118, 542–551. doi: 10.1016/j.ijbiomac.2018.06.052
Benito-González, I., López-Rubio, A., Gavara, R., and Martínez-Sanz, M. (2019a). Cellulose nanocrystal-based films produced by more sustainable extraction protocols from Posidonia oceanica waste biomass. Cellulose 26, 8007–8024. doi: 10.1007/s10570-019-02641-4
Benito-González, I., López-Rubio, A., and Martínez-Sanz, M. (2019b). High-performance starch biocomposites with celullose from waste biomass: film properties and retrogradation behaviour. Carbohydr. Polym. 216, 180–188. doi: 10.1016/j.carbpol.2019.04.030
Benito-González, I., López-Rubio, A., Gómez-Mascaraque, L. G., and Martínez-Sanz, M. (2020). PLA coating improves the performance of renewable adsorbent pads based on cellulosic aerogels from aquatic waste biomass. Chem. Eng. J. 390:124607. doi: 10.1016/j.cej.2020.124607
Bettaieb, F., Khiari, R., Hassan, M. L., Belgacem, M. N., Bras, J., Dufresne, A., et al. (2015). Preparation and characterization of new cellulose nanocrystals from marine biomass Posidonia oceanica. Ind. Crops Prod. 72, 175–182. doi: 10.1016/j.indcrop.2014.12.038
Bhardwaj, N., and Kundu, S. C. (2010). Electrospinning: a fascinating fiber fabrication technique. Biotechnol. Adv. 28, 325–347. doi: 10.1016/j.biotechadv.2010.01.004
Bhatnagar, A., Vilar, V. J. P., Santos, J. C., Botelho, C. M. S., and Boaventura, R. A. R. (2012). Valorisation of marine Pelvetia canaliculata Ochrophyta for separation and recovery of nickel from water: equilibrium and kinetics modeling on Na-loaded algae. Chem. Eng. J. 200–202, 365–372. doi: 10.1016/j.cej.2012.06.071
Bilal, M., and Iqbal, H. M. N. (2020). Biologically active macromolecules: extraction strategies, therapeutic potential and biomedical perspective. Int. J. Biol. Macromol. 151, 1–18. doi: 10.1016/j.ijbiomac.2020.02.037
Bilal, M., Rasheed, T., Sosa-Hernández, J. E., Raza, A., Nabeel, F., and Iqbal, H. M. N. (2018). Biosorption: an interplay between marine algae and potentially toxic elements – a review. Mar. Drugs 16:65. doi: 10.3390/md16020065
Bird, M. I., Wurster, C. M., de Paula Silva, P. H., Bass, A. M., and de Nys, R. (2011). Algal biochar – production and properties. Bioresour. Technol. 102, 1886–1891. doi: 10.1016/j.biortech.2010.07.106
Bisters, V., Kalviss, J., Burlakovs, J., and Klavins, M. (2021). Algae processing for energy production: development of waste pyrolysis technology. Agron. Res. 19. doi: 10.15159/AR.21.010
Borchert, F., Emadodin, I., Kluß, C., Rotter, A., and Reinsch, T. (2021). Grass growth and N2O emissions from soil after application of jellyfish in coastal areas. Front. Mar. Sci. 8:711601. doi: 10.3389/fmars.2021.711601
Bouzikri, S., Ouasfi, N., Benzidia, N., Salhi, A., Bakkas, S., and Khamliche, L. (2020). Marine alga Bifurcaria bifurcata: biosorption of reactive Blue 19 and methylene blue from aqueous solutions. Environ. Sci. Pollut. Res. 27, 33636–33648. doi: 10.1007/s11356-020-07846-w
Broch, O. J., Alver, M. O., Bekkby, T., Gundersen, H., Forbord, S., Handå, A., et al. (2019). The kelp cultivation potential in coastal and offshore regions of Norway. Front. Mar. Sci. 5:529. doi: 10.3389/fmars.2018.00529
Brod, E., Oppen, J., Kristoffersen, A. Ø, Haraldsen, T. K., and Krogstad, T. (2017). Drying or anaerobic digestion of fish sludge: nitrogen fertilisation effects and logistics. Ambio 46, 852–864. doi: 10.1007/s13280-017-0927-5
Brown, L. K., Blanz, M., Wishart, J., Dieterich, B., Schmidt, S. B., Russell, J., et al. (2020). Is Bere barley specifically adapted to fertilisation with seaweed as a nutrient source? Nutr. Cycling Agroecosyst. 118, 149–163. doi: 10.1007/s10705-020-10090-w
Bruhn, A., Dahl, J., Nielsen, H. B., Nikolaisen, L., Rasmussen, M. B., Markager, S., et al. (2011). Bioenergy potential of Ulva lactuca: biomass yield, methane production and combustion. Bioresour. Technol. 102, 2595–2604. doi: 10.1016/j.biortech.2010.10.010
Buchtová, N., Pradille, C., Bouvard, J.-L., and Budtova, T. (2019). Mechanical properties of cellulose aerogels and cryogels. Soft Matter. 15, 7901–7908. doi: 10.1039/C9SM01028A
Bulgariu, D., and Bulgariu, L. (2016). Potential use of alkaline treated algae waste biomass as sustainable biosorbent for clean recovery of cadmium(II) from aqueous media: batch and column studies. J. Cleaner Prod. 112, 4525–4533. doi: 10.1016/j.jclepro.2015.05.124
Burlakovs, J., Kriipsalu, M., Porshnov, D., Jani, Y., Ozols, V., Pehme, K. M., et al. (2019). Gateway of landfilled plastic waste towards circular economy in Europe. Separations 6:6020025. doi: 10.3390/separations6020025
Buschmann, A. H., Camus, C., Infante, J., Neori, A., Israel, Á, Hernández-González, M. C., et al. (2017). Seaweed production: overview of the global state of exploitation, farming and emerging research activity. Eur. J. Phycol. 52, 391–406. doi: 10.1080/09670262.2017.1365175
Calejo, M. T., Almeida, A. J., and Fernandes, A. I. (2012). Exploring a new jellyfish collagen in the production of microparticles for protein delivery. J. Microencapsul. 29, 520–531. doi: 10.3109/02652048.2012.665089
Campbell, I., Macleod, A., Sahlmann, C., Neves, L., Funderud, J., Øverland, M., et al. (2019). The environmental risks associated with the development of seaweed farming in Europe – Prioritizing key knowledge gaps. Front. Mar. Sci. 6:107. doi: 10.3389/fmars.2019.00107
Cardoso, M. J., Costa, R. R., and Mano, J. F. (2016). Marine origin polysaccharides in drug delivery systems. Mar. Drugs 14:34. doi: 10.3390/md14020034
Cardoso, S. L., Costa, C. S. D., Nishikawa, E., da Silva, M. G. C., and Vieira, M. G. A. (2017). Biosorption of toxic metals using the alginate extraction residue from the brown algae Sargassum filipendula as a natural ion-exchanger. J. Cleaner Prod. 165, 491–499. doi: 10.1016/j.jclepro.2017.07.114
Carmen, B., Krause-Jensen, D., Alcoverro, T., Marbà, N., Duarte, C. M., Van Katwijk, M. M., et al. (2019). Recent trend reversal for declining European seagrass meadows. Nat. Commun. 10, 1–8. doi: 10.1038/s41467-019-11340-4
Caruso, G. (2016). Fishery wastes and by-products: a resource to be valorised. J. Fish. Sci. 10, 12–15.
Chai, Q., Jiao, Y., and Yu, X. (2017). Hydrogels for biomedical applications: their characteristics and the mechanisms behind them. Gels 3:6. doi: 10.3390/gels3010006
Chaiwong, K., Kiatsiriroat, T., Vorayos, N., and Thararax, C. (2013). Study of bio-oil and bio-char production from algae by slow pyrolysis. Biomass Bioenergy 56, 600–606. doi: 10.1016/j.biombioe.2013.05.035
Chan, S. W., Mirhosseini, H., Taip, F. S., Ling, T. C., Nehdi, I. A., and Tan, C. P. (2016). Emulsion formulation optimization and characterization of spray-dried κ-carrageenan microparticles for the encapsulation of CoQ10. Food Sci. Biotechnol. 25, 53–62. doi: 10.1007/s10068-016-0098-3
Chawla, R., Sivakumar, S., and Kaur, H. (2021). Antimicrobial edible films in food packaging: Current scenario and recent nanotechnological advancements- a review. Carbohydr. Polym. Technol. Appl. 2:100024. doi: 10.1016/j.carpta.2020.100024
Chemat, F., Rombaut, N., Sicaire, A. G., Meullemiestre, A., Fabiano-Tixier, A. S., and Abert-Vian, M. (2017). Ultrasound assisted extraction of food and natural products. Mechanisms, techniques, combinations, protocols and applications. A review. Ultrason. Sonochem. 34, 540–560. doi: 10.1016/j.ultsonch.2016.06.035
Chen, H., Zhou, D., Luo, G., Zhang, S., and Chen, J. (2015). Macroalgae for biofuels production: progress and perspectives. Renew. Sustain. Energy Rev. 47, 427–437. doi: 10.1016/j.rser.2015.03.086
Chen, P. Y., Lin, A. Y. M., McKittrick, J., and Meyers, M. A. (2008). Structure and mechanical properties of crab exoskeletons. Acta Biomater. 4, 587–596. doi: 10.1016/j.actbio.2007.12.010
Chen, S. S., Maneerung, T., Tsang, D. C. W., Ok, Y. S., and Wang, C. H. (2017). Valorization of biomass to hydroxymethylfurfural, levulinic acid, and fatty acid methyl ester by heterogeneous catalysts. Chem. Eng. J. 328, 246–273. doi: 10.1016/j.cej.2017.07.020
Cheng, H., Yang, X., Che, X., Yang, M., and Zhai, G. (2018). Biomedical application and controlled drug release of electrospun fibrous materials. Mater. Sci. Eng. C 90, 750–763. doi: 10.1016/j.msec.2018.05.007
Cheng, J., Yin, W., Chang, Z., Lundholm, N., and Jiang, Z. (2017). Biosorption capacity and kinetics of cadmium(II) on live and dead Chlorella vulgaris. J. Appl. Phycol. 29, 211–221. doi: 10.1007/s10811-016-0916-2
Chew, J. J., and Doshi, V. (2011). Recent advances in biomass pretreatment – Torrefaction fundamentals and technology. Renew. Sustain. Energy Rev. 15, 4212–4222. doi: 10.1016/j.rser.2011.09.017
Chifiriuc, M. C., and Grumezescu, A. M. (2016). “Iron oxide nanomaterials for functional imaging,” in Nanobiomaterials in Medical Imaging, Applications of Nanobiomaterials, Vol. 8, ed. A. M. Grumezescu (Norwich, NY: William Andrew Publishing), doi: 10.1016/B978-0-323-41736-5.00009-1
Choi, A. H., and Ben-Nissan, B. (eds) (2019). Marine-Derived Biomaterials for Tissue Engineering Applications, Springer Series in Biomaterials Science and Engineering 14. Singapore: Springer Singapore, doi: 10.1007/978-981-13-8855-2
Choi, D. G., Venkatesan, J., and Shim, M. S. (2019). Selective anticancer therapy using pro-oxidant drug-loaded chitosan–fucoidan nanoparticles. Int. J. Mol. Sci. 20:3220. doi: 10.3390/ijms20133220
Choi, J. H., Choi, W. Y., Cha, D. S., Chinnan, M. J., Park, H. J., Lee, D. S., et al. (2005). Diffusivity of potassium sorbate in κ-carrageenan based antimicrobial film. LWT Food Sci. Technol. 38, 417–423. doi: 10.1016/j.lwt.2004.07.004
Chubarenko, B., Woelfel, J., Hofmann, J., Aldag, S., Beldowski, J., Burlakovs, J., et al. (2021). Converting beach wrack into a resource as a challenge for the Baltic Sea (an overview). Ocean Coast. Manag. 200:105413. doi: 10.1016/j.ocecoaman.2020.105413
Cirne, D. G., Lehtomäki, A., Björnsson, L., and Blackall, L. L. (2007). Hydrolysis and microbial community analyses in two-stage anaerobic digestion of energy crops. J. Appl. Microbiol. 103, 516–527. doi: 10.1111/j.1365-2672.2006.03270.x
Coltelli, M.-B., Panariello, L., Morganti, P., Danti, S., Baroni, A., Lazzeri, A., et al. (2020). Skin-compatible biobased beauty masks prepared by extrusion. J. Funct. Biomater. 11:23. doi: 10.3390/jfb11020023
Coração, A. C., de, S., dos Santos, F. S., Duarte, J. A. D., Lopes-Filho, E. A. P., De-Paula, J. C., et al. (2020). What do we know about the utilization of the Sargassum species as biosorbents of trace metals in Brazil? J. Environ. Chem. Eng. 8:103941. doi: 10.1016/j.jece.2020.103941
Daneshvar, E., Sohrabi, M. S., Kousha, M., Bhatnagar, A., Aliakbarian, B., Converti, A., et al. (2014). Shrimp shell as an efficient bioadsorbent for Acid Blue 25 dye removal from aqueous solution. J. Taiwan Inst. Chem. Eng. 45, 2926–2934. doi: 10.1016/j.jtice.2014.09.019
Danti, S., Trombi, L., Fusco, A., Azimi, B., Lazzeri, A., Morganti, P., et al. (2019). Chitin nanofibrils and nanolignin as functional agents in skin regeneration. Int. J. Mol. Sci. 20:2669. doi: 10.3390/ijms20112669
Davis, T. A., Volesky, B., and Mucci, A. (2003). A review of the biochemistry of heavy metal biosorption by brown algae. Water Res. 37, 4311–4330. doi: 10.1016/S0043-1354(03)00293-8
de Freitas, G. R., da Silva, M. G. C., and Vieira, M. G. A. (2019). Biosorption technology for removal of toxic metals: a review of commercial biosorbents and patents. Environ. Sci. Pollut. Res. Int. 26, 19097–19118. doi: 10.1007/s11356-019-05330-8
de Godos, I., Muñoz, R., and Guieysse, B. (2012). Tetracycline removal during wastewater treatment in high-rate algal ponds. J. Hazard. Mater. 229, 446–449. doi: 10.1016/j.jhazmat.2012.05.106
de Oliveira, J. D., Carvalho, L. S., Gomes, A. M., Queiroz, L. R., Magalhães, B. S., and Parachin, N. S. (2016). Genetic basis for hyper production of hyaluronic acid in natural and engineered microorganisms. Microb. Cell Fact. 15:119. doi: 10.1186/s12934-016-0517-4
Defeo, O., McLachlan, A., Schoeman, D. S., Schlacher, T. A., Dugan, J., Jones, A., et al. (2009). Threats to sandy beach ecosystems: a review. Estuar. Coast. Shelf Sci. 81, 1–12. doi: 10.1016/j.ecss.2008.09.022
Demirtaş, T. T., Irmak, G., and Gümüşderelioğlu, M. (2017). A bioprintable form of chitosan hydrogel for bone tissue engineering. Biofabrication 9:35003. doi: 10.1088/1758-5090/aa7b1d
Divya, K., and Jisha, M. S. (2018). Chitosan nanoparticles preparation and applications. Environ. Chem. Lett. 16, 101–112. doi: 10.1007/s10311-017-0670-y
Dobrinčić, A., Balbino, S., Zorić, Z., Pedisić, S., Kovačević, D. B., Garofulić, I. E., et al. (2020). Advanced technologies for the extraction of marine brown algal polysaccharides. Mar. Drugs 18:168. doi: 10.3390/md18030168
Domalik-Pyzik, P., Chłopek, J., and Pielichowska, K. (2019). “Chitosan-based hydrogels: preparation, properties, and applications,” in Cellulose-Based Superabsorbent Hydrogels. Polymers and Polymeric Composites: A Reference Series, ed. I. H. Mondal (Cham: Springer), 1665–1693. doi: 10.1007/978-3-319-77830-3_55
Du, X., Liu, Y., Yan, H., Rafique, M., Li, S., Shan, X., et al. (2020). Anti-infective and pro-coagulant chitosan-based hydrogel tissue adhesive for sutureless wound closure. Biomacromolecules 21, 1243–1253. doi: 10.1021/acs.biomac.9b01707
Dubrawski, R., Zawadzka, E., Gawlik, W., and Bistram, K. (2008). Stan morskiej strefy brzegowej na podstawie wybranych wyników monitoringu brzegów morskich z lat 2004-2006 / The state of the coastal zone on the basis of selected results of the monitoring of sea coasts from 2004 to 2006. Inżynieria Morska I Geotechnika 1, 15–27.
Dugan, J. E., Hubbard, D. M., McCrary, M. D., and Pierson, M. O. (2003). The response of macrofauna communities and shorebirds to macrophyte wrack subsidies on exposed sandy beaches of southern California. Estuar. Coast. Shelf Sci. 58(Suppl.), 25–40. doi: 10.1016/S0272-7714(03)00045-3
Ediriweera, T. K., Aruppala, A. L. H., and Abeyrathne, E. D. N. S. (2019). Analysis of bioactive properties of fish protein hydrolysates from Scomber japonicus Fin wastes. J. Technol. Value Addition 1, 31–45.
El Biriane, M., and Barbachi, M. (2020). State-of-the-art review on recycled mussel shell waste in concrete and mortar. Innov. Infrastruct. Solut. 6:29. doi: 10.1007/s41062-020-00394-9
Ellen MacArthur Foundation (2016). The New Plastics Economy: Rethinking the Future of Plastics. Cowes: Ellen MacArthur Foundation.
Emadodin, I., Reinsch, T., Rotter, A., Orlando-Bonaca, M., Taube, F., and Javidpour, J. (2020). A perspective on the potential of using marine organic fertilizers for the sustainable management of coastal ecosystem services. Environ. Sustain. 3, 105–115. doi: 10.1007/s42398-020-00097-y
Emblemsvåg, J., Kvadsheim, N. P., Halfdanarson, J., Koesling, M., Nystrand, B. T., Sunde, J., et al. (2020). Strategic considerations for establishing a large-scale seaweed industry based on fish feed application: a Norwegian case study. J. Appl. Physiol. 32, 4159–4169. doi: 10.1007/s10811-020-02234-w
Eriksen, M., Lebreton, L. C. M., Carson, H. S., Thiel, M., Moore, C. J., Borerro, J. C., et al. (2014). Plastic pollution in the world’s oceans: more than 5 trillion plastic pieces weighing over 250,000 tons afloat at sea. PLoS One 9:e111913. doi: 10.1371/journal.pone.0111913
Eroglu, E., Agarwal, V., Bradshaw, M., Chem, X., Smith, S. M., Raston, C. L., et al. (2012). Nitrate removal from liquid effluents using microalgae immobilized on chitosan nanofiber mats. Green Chem. 14, 2682–2685. doi: 10.1039/C2GC35970G
Escapa, C., Coimbra, R. N., Nuevo, C., Vega, S., Paniagua, S., García, A. I., et al. (2017). Valorization of microalgae biomass by its use for the removal of paracetamol from contaminated water. Water 9:312. doi: 10.3390/w9050312
Esmaeli, A., Jokar, M., Kousha, M., Daneshvar, E., Zilouei, H., and Karimi, K. (2013). Acidic dye wastewater treatment onto a marine macroalga, Nizamuddina zanardini (Phylum: Ochrophyta). Chem. Eng. J. 217, 329–336. doi: 10.1016/j.cej.2012.11.038
Estevez, M. M., Tomczak-Wandzel, R., Akervold, K., and Tornes, O. (2019). Co-digestion of waste from the salmon aquaculture sector with regional sewage sludge: effects on methane yield and digestate nutrient content. Eco-Energetics 2, 29–34. doi: 10.24426/eco-energetics.v2i2.107
Faber, T. A., Bechtel, P. J., Hernot, D. C., Parsons, C. M., Swanson, K. S., Smiley, S., et al. (2010). Protein digestibility evaluations of meat and fish substrates using laboratory, avian, and ileally cannulated dog assays. J. Anim. Sci. 88, 1421–1432. doi: 10.2527/jas.2009-2140
Fabre, E., Dias, M., Costa, M., Henriques, B., Vale, C., Lopes, C. B., et al. (2020). Negligible effect of potentially toxic elements and rare earth elements on mercury removal from contaminated waters by green, brown and red living marine macroalgae. Sci. Total Environ. 724:138133. doi: 10.1016/j.scitotenv.2020.138133
Fan, Y., Saito, T., and Isogai, A. (2008). Preparation of chitin nanofibers from squid pen β-chitin by simple mechanical treatment under acid conditions. Biomacromolecules 9, 1919–1923. doi: 10.1021/bm800178b
FAO (2016). The State of the World Fisheries and Aquaculture. Contributing to Food Security and Nutrition For All. Rome: FAO.
FAO (2021). Fishery Statistical Collections. Global Aquaculture Production. Available online at: http://www.fao.org/fishery/statistics/global-aquaculture-production/en [Accessed January 22, 2021].
Farhan, A., and Hani, N. M. (2017). Characterization of edible packaging films based on semi-refined kappa-carrageenan plasticized with glycerol and sorbitol. Food Hydrocoll. 64, 48–58. doi: 10.1016/j.foodhyd.2016.10.034
Fawzy, M. A., and Gomaa, M. (2020). Use of algal biorefinery waste and waste office paper in the development of xerogels: a low cost and eco-friendly biosorbent for the effective removal of Congo red and Fe (II) from aqueous solutions. J. Environ. Manag. 262:110380. doi: 10.1016/j.jenvman.2020.110380
Feng, J., Nguyen, S. T., Fan, Z., and Duong, H. M. (2015). Advanced fabrication and oil absorption properties of super-hydrophobic recycled cellulose aerogels. Chem. Eng. J. 270, 168–175. doi: 10.1016/j.cej.2015.02.034
Figueira, M. M., Volesky, B., Ciminelli, V. S. T., and Roddick, F. A. (2000). Biosorption of metals in brown seaweed biomass. Water Res. 34, 196–204. doi: 10.1016/S0043-1354(99)00120-7
Filipkowska, A., Lubecki, L., Szymczak-Zyła, M., Kowalewska, G., Zbikowski, R., and Szefer, P. (2008). Utilisation of macroalgae from the Sopot beach (Baltic Sea). Oceanologia 50, 255–273.
Filote, C., Ungureanu, G., Boaventura, R., Santos, S., Volf, I., and Botelho, C. (2017). Green macroalgae from the Romanian coast of Black Sea: physico-chemical characterization and future perspectives on their use as metal anions biosorbents. Process Saf. Environ. Prot. 108, 34–43. doi: 10.1016/j.psep.2016.06.002
Flórez-Fernández, N., López-García, M., González-Muñoz, M. J., Vilariño, J. M. L., and Domínguez, H. (2017). Ultrasound-assisted extraction of fucoidan from Sargassum muticum. J. Appl. Phycol. 29, 1553–1561. doi: 10.1007/s10811-016-1043-9
Fontes-Candia, C., Erboz, E., Martínez-Abad, A., López-Rubio, A., and Martínez-Sanz, M. (2019). Superabsorbent food packaging bioactive cellulose-based aerogels from Arundo donax waste biomass. Food Hydrocoll. 96, 151–160. doi: 10.1016/j.foodhyd.2019.05.011
Foroutan, R., Mohammadi, R., Razeghi, J., and Ramavandi, B. (2019). Performance of algal activated carbon/Fe3O4 magnetic composite for cationic dyes removal from aqueous solutions. Algal Res. 40:101509. doi: 10.1016/j.algal.2019.101509
Galli, R., Sitoci-Ficici, K. H., Uckermann, O., Later, R., Marečková, M., Koch, M., et al. (2018). Label-free multiphoton microscopy reveals relevant tissue changes induced by alginate hydrogel implantation in rat spinal cord injury. Sci. Rep. 8:10841. doi: 10.1038/s41598-018-29140-z
Gang, L., Shunan, X., Fang, J., Yuguang, Z., and Zhigang, H. (2017). Thermal cracking products and bio-oil production from microalgae Desmodesmus sp. Int. J. Agric. Biol. Eng. 10, 198–206. doi: 10.25165/j.ijabe.20171004.3348
Gharazi, S., Zarket, B. C., DeMella, K. C., and Raghavan, S. R. (2018). Nature-inspired hydrogels with soft and stiff zones that exhibit a 100-fold difference in elastic modulus. ACS Appl. Mater. Interf. 10, 34664–34673. doi: 10.1021/acsami.8b14126
Gigante, V., Cinelli, P., Righetti, M. C., Sandroni, M., Tognotti, L., Seggiani, M., et al. (2020). Evaluation of mussel shells powder as reinforcement for PLA-based biocomposites. Int. J. Mol. Sci. 21:5364. doi: 10.3390/ijms21155364
Goh, C. H., Heng, P. W. S., and Chan, L. W. (2012). Alginates as a useful natural polymer for microencapsulation and therapeutic applications. Carbohydr. Polym. 88, 1–12. doi: 10.1016/j.carbpol.2011.11.012
Gonçalves, V. S. S., Gurikov, P., Poejo, J., Matias, A. A., Heinrich, S., Duarte, C. M. M., et al. (2016). Alginate-based hybrid aerogel microparticles for mucosal drug delivery. Eur. J. Pharm. Biopharm. 107, 160–170. doi: 10.1016/j.ejpb.2016.07.003
Goycoolea, F. M., Lollo, G., Remuñán-López, C., Quaglia, F., and Alonso, M. J. (2009). Chitosan-alginate blended nanoparticles as carriers for the transmucosal delivery of macromolecules. Biomacromolecules 10, 1736–1743. doi: 10.1021/bm9001377
Graca, B., Witek, Z., Burska, D., Białkowska, I., Łukawska-Matuszewska, K., and Bolałek, J. (2006). Pore water phosphate and ammonia below the permanent halocline in the south-eastern Baltic Sea and their benthic fluxes under anoxic conditions. J. Mar. Syst. 63, 141–154. doi: 10.1016/j.jmarsys.2006.06.003
Greiner, A., and Wendorff, J. H. (2007). Electrospinning: a fascinating method for the preparation of ultrathin fibers. Angew. Chem. 46, 5670–5703. doi: 10.1002/anie.200604646
Grienke, U., Silke, J., and Tasdemir, D. (2014). Bioactive compounds from marine mussels and their effects on human health. Food Chem. 142, 48–60. doi: 10.1016/j.foodchem.2013.07.027
Gu, L., Shan, T., Ma, Y.-X., Tay, F. R., and Niu, L. (2019). Novel biomedical applications of crosslinked collagen. Trends Biotechnol. 37, 464–491. doi: 10.1016/j.tibtech.2018.10.007
Guiry, M. D., and Blunden, G., eds (1991). Seaweed Resources in Europe: Uses and Potential. Chichester: John Wiley and Sons.
Guo, H., Hong, Z., and Yi, R. (2015). Core-shell collagen peptide chelated calcium/calcium alginate nanoparticles from fish scales for calcium supplementation. J. Food Sci. 80, 1595–1601. doi: 10.1111/1750-3841.12912
Hafsa, J., Smach, M. A., Charfeddine, B., Limem, K., Majdoub, H., and Rouatbi, S. (2016). Antioxidant and antimicrobial proprieties of chitin and chitosan extracted from Parapenaeus longirostris shrimp shell waste. Ann. Pharm. Fr. 74, 27–33. doi: 10.1016/j.pharma.2015.07.005
Hajji, S., Kchaou, H., Bkhairia, I., Salem, R. B. S. B., Boufi, S., Debeaufort, F., et al. (2021). Conception of active food packaging films based on crab chitosan and gelatin enriched with crustacean protein hydrolysates with improved functional and biological properties. Food Hydrocoll. 116:106639. doi: 10.1016/j.foodhyd.2021.106639
Hart, A. (2020). Mini-review of waste shell-derived materials’ applications. Waste Manag. Res. 38, 514–527. doi: 10.1177/0734242X19897812
Hassan, M., Naidu, R., Du, J., Liu, Y., and Qi, F. (2020). Critical review of magnetic biosorbents: their preparation, application, and regeneration for wastewater treatment. Sci. Total Environ. 702:134893. doi: 10.1016/j.scitotenv.2019.134893
He, J., and Chen, J. P. (2014). A comprehensive review on biosorption of heavy metals by algal biomass: Materials, performances, chemistry, and modeling simulation tools. Bioresour. Technol. 160, 67–78. doi: 10.1016/j.biortech.2014.01.068
Helsinki Commission (2009). Waterborne Inputs of Heavy Metals to the Baltic Sea. HELCOM Indicator Fact Sheets. Available online at: http://archive.iwlearn.net/helcom.fi/environment2/ifs/ifs2009/en_GB/waterborne_hm/index.html [Accessed January 22, 2021].
Helsinki Commission (2018). Metals (Lead, Cadmium And Mercury). HELCOM core indicator report. Available online at: https://www.helcom.fi/wp-content/uploads/2019/08/Metals-HELCOM-core-indicator-2018.pdf [Accessed May 31, 2021].
Hembrick-Holloman, V., Samuel, T., Mohammed, Z., Jeelani, S., and Rangari, V. K. (2020). Ecofriendly production of bioactive tissue engineering scaffolds derived from egg- and sea-shells. J. Mater. Res. Technol. 9, 13729–13739. doi: 10.1016/j.jmrt.2020.09.093
Hernández-Estévez, A., and Cristiani-Urbina, E. (2014). Nickel (II) biosorption from aqueous solutions by shrimp head biomass. Environ. Monit. Assess. 186, 7987–7998. doi: 10.1007/s10661-014-3981-5
Herrera, N., Singh, A. A., Salaberria, A. M., Labidi, J., Mathew, A. P., and Oksman, K. (2017). Triethyl citrate (TEC) as a dispersing aid in polylactic acid/chitin nanocomposites prepared via liquid-assisted extrusion. Polymers (Basel) 9:406. doi: 10.3390/polym9090406
Hoare, T. R., and Kohane, D. S. (2008). Hydrogels in drug delivery: progress and challenges. Polymer 49, 1993–2007. doi: 10.1016/j.polymer.2008.01.027
Huang, Y. C., Li, R. Y., Chen, J. Y., and Chen, J. K. (2016). Biphasic release of gentamicin from chitosan/fucoidan nanoparticles for pulmonary delivery. Carbohydr. Polym. 138, 114–122. doi: 10.1016/j.carbpol.2015.11.072
Ifuku, S., Nogi, M., Abe, K., Yoshioka, M., Morimoto, M., Saimoto, H., et al. (2009). Preparation of chitin nanofibers with a uniform width as α-chitin from crab shells. Biomacromolecules 10, 1584–1588. doi: 10.1021/bm900163d
Jerold, M., and Sivasubramanian, V. (2016). Biosorption of malachite green from aqueous solution using brown marine macro algae Sargassum swartzii. Desalination Water Treat. 57, 25288–25300. doi: 10.1080/19443994.2016.1156582
Jerold, M., Vasantharaj, K., Joseph, D., and Sivasubramanian, V. (2017). Fabrication of hybrid biosorbent nanoscale zero-valent iron-Sargassum swartzii biocomposite for the removal of crystal violet from aqueous solution. Int. J. Phytoremed. 19, 214–224. doi: 10.1080/15226514.2016.120760
Jiménez-Gómez, C. P., and Cecilia, J. A. (2020). Chitosan: a natural biopolymer with a wide and varied range of applications. Molecules 25:3981. doi: 10.3390/molecules25173981
Johansen, U., Bull-Berg, H., Vik, L. H., Stokka, A. M., Richardsen, R., and Winther, U. (2019). The Norwegian seafood industry – importance for the national economy. Mar. Policy 110:103561. doi: 10.1016/j.marpol.2019.103561
Jönsson, M., Allahgholi, L., Sardari, R. R. R., Hreggviosson, G. O., and Karlsson, E. N. (2020). Extraction and modification of macroalgal polysaccharides for current and next-generation applications. Molecules 25:930. doi: 10.3390/molecules25040930
Joshi, S., Eshwar, S., and Jain, V. (2019). “Marine polysaccharides: biomedical and tissue engineering applications,” in Marine-Derived Biomaterials for Tissue Engineering Applications, Springer Series in Biomaterials Science and Engineering, Vol. 14, eds A. Choi and B. Ben-Nissan (Singapore: Springer), 443–487. doi: 10.1007/978-981-13-8855-2_19
Jung, K. W., Choi, B. H., Jeong, T. U., and Ahn, K. H. (2016). Facile synthesis of magnetic biochar/Fe3O4 nanocomposites using electro-magnetization technique and its application on the removal of acid orange 7 from aqueous media. Bioresour. Technol. 220, 672–676. doi: 10.1016/j.biortech.2016.09.035
Jung, K. W., Choi, B. H., Song, K. G., and Choi, J. W. (2019). Statistical optimization of preparing marine macroalgae derived activated carbon/iron oxide magnetic composites for sequestering acetylsalicylic acid from aqueous media using response surface methodologys. Chemosphere 215, 432–443. doi: 10.1016/j.chemosphere.2018.10.069
Kadam, S. U., Tiwari, B. K., and O’Donnell, C. P. (2015). Extraction, structure and biofunctional activities of laminarin from brown algae. Int. J. Food Sci. Technol. 50, 24–31. doi: 10.1111/ijfs.12692
Kanjilal, T., and Bhattacharjee, C. (2018). “Green applications of magnetic sorbents for environmental remediation,” in Organic Pollutants in Wastewater I. Methods of Analysis, Removal and Trearment, eds I. A. M. Asiri and A. Mohammad (Millersville: Materials Research Forum LLC), 1–41. doi: 10.21741/9781945291630
Karkal, S. S., and Kudre, T. G. (2020). Valorization of fish discards for the sustainable production of renewable fuels. J. Cleaner Prod. 275:122985. doi: 10.1016/j.jclepro.2020.122985
Karunanithi, P., Murali, M. R., Samuel, S., Raghavendran, H. R. B., Abbas, A. A., and Kamarul, T. (2016). Three dimensional alginate-fucoidan composite hydrogel augments the chondrogenic differentiation of mesenchymal stromal cells. Carbohydr. Polym. 147, 294–303. doi: 10.1016/j.carbpol.2016.03.102
Kataržytė, M., Vaičiūtė, D., and Nasvytis, P. (2019). Excellent bathing waters in coastal areas: is microbial pollution the only important parameter? Ocean Coast. Manag. 182:104922. doi: 10.1016/j.ocecoaman.2019.104922
Kavitha Sankar, P. C., Rajmohan, G., and Rosemary, M. J. (2017). Physico-chemical characterisation and biological evaluation of freeze dried chitosan sponge for wound care. Mater. Lett. 208, 130–132. doi: 10.1016/j.matlet.2017.05.010
Kenry and Lim, C. T. (2017). Nanofiber technology: current status and emerging developments. Prog. Polym. Sci. 70, 1–17. doi: 10.1016/j.progpolymsci.2017.03.002
Khalil, A. H., Tye, Y. Y., Saurabh, C. K., Leh, C., Lai, T. K., Chong, E., et al. (2017). Biodegradable polymer films from seaweed polysaccharides: a review on cellulose as a reinforcement material. Exp. Polym. Lett. 11, 244–265. doi: 10.3144/expresspolymlett.2017.26
Khan, A. Z., Jamil, S., Akhtar, A., Bashir, M. M., and Yar, M. (2020). Chitosan based hybrid materials used for wound healing applications – a short review. Int. J. Polym. Mater. Polym. Biomater. 69, 419–436. doi: 10.1080/00914037.2019.1575828
Khan, M. I., Shin, J. H., and Kim, J. D. (2018). The promising future of microalgae: current status, challenges, and optimization of a sustainable and renewable industry for biofuels, feed, and other products. Microb. Cell Fact. 17:36. doi: 10.1186/s12934-018-0879-x
Khanpour-Alikelayeh, E., Partovinia, A., Talebi, A., and Kermanian, H. (2021). Enhanced biodegradation of light crude oil by immobilized Bacillus licheniformis in fabricated alginate beads through electrospray technique. Environ. Monit. Assess. 193:328. doi: 10.1007/s10661-021-09104-z
Khoo, C. G., Dasan, Y. K., Lam, M. K., and Lee, K. T. (2019). Algae biorefinery: Review on a broad spectrum of downstream processes and products. Bioresour. Technol. 292:121964. doi: 10.1016/j.biortech.2019.121964
Kikionis, S., Ioannou, E., Toskas, G., and Roussis, V. (2015). Electrospun biocomposite nanofibers of ulvan/PCL and ulvan/PEO. J. Appl. Polym. Sci. 132:42153. doi: 10.1002/app.42153
Kim, E. S., Lee, J.-S., and Lee, H. G. (2016). Nanoencapsulation of red ginseng extracts using chitosan with polyglutamic acid or fucoidan for improving antithrombotic activities. J. Agric. Food Chem. 64, 4765–4771. doi: 10.1021/acs.jafc.6b00911
Kim, M. H., Lee, Y. W., Jung, W. K., Oh, J., and Nam, S. Y. (2019). Enhanced rheological behaviors of alginate hydrogels with carrageenan for extrusion-based bioprinting. J. Mech. Behav. Biomed. Mater. 98, 187–194. doi: 10.1016/j.jmbbm.2019.06.014
Kim, M., Ahn, Y., Lee, K., Jung, W., and Cha, C. (2020). In situ facile-forming chitosan hydrogels with tunable physicomechanical and tissue adhesive properties by polymer graft architecture. Carbohydr. Polym. 229:115538. doi: 10.1016/j.carbpol.2019.115538
Kim, S.-K., ed (2013). Marine Proteins and Peptides: Biological Activities and Applications. Chichester: John Wiley and Sons, doi: 10.1002/9781118375082
Kinley, R. D., de Nys, R., Vucko, M. J., Machado, L., and Tomkins, N. W. (2016). The red macroalgae Asparagopsis taxiformis is a potent natural antimethanogenic that reduces methane production during in vitro fermentation with rumen fluid. Anim. Prod. Sci. 56, 282–289. doi: 10.1071/AN15576
Kirkman, H., and Kendrick, G. A. (1997). Ecological significance and commercial harvesting of drifting and beach-cast macro-algae and seagrasses in Australia: a review. J. Appl. Phycol. 9, 311–326. doi: 10.1023/A:1007965506873
Kishore Kumar, K., Prasad, M. K., Baburao, G., Sudhakar, M., Sivajyothi, J., Sathish, T., et al. (2018). A fortunate marine algae biomass, sargassum cinereum for removal of pb(Ii): Studies on thermodynamics, kinetics and characterization. Desalination Water Treat. 116, 179–186. doi: 10.5004/dwt.2018.22544
Klavins, M., Bisters, V., and Burlakovs, J. (2018). Small scale gasification application and perspectives in circular economy. Environ. Clim. Technol. 22, 42–54. doi: 10.2478/rtuect-2018-0003
Koçer, A. T., and Özçimen, D. (2018). Investigation of the biogas production potential from algal wastes. Waste Manag. Res. 36, 1100–1105.
Koesling, M., Kvadsheim, N. P., Halfdanarson, J., Emblemsvåg, J., and Rebours, C. (2021). Environmental impacts of protein-production from farmed seaweed: comparison of possible scenarios in Norway. J. Clean. Prod. 307:127301. doi: 10.1016/j.jclepro.2021.127301
Kotroni, E., Simirioti, E., Kikionis, S., Sfiniadakis, I., Siamidi, A., Karalis, V., et al. (2019). In vivo evaluation of the anti-inflammatory activity of electrospun micro/nanofibrous patches loaded with Pinus halepensis bark extract on hairless mice skin. Materials 12:2596. doi: 10.3390/ma12162596
Kraan, S. (2012). “Algal polysaccharides, novel applications and outlook,” in Carbohydrates. Comprehensive Studies on Glycobiology and Glycotechnology, ed. C.-F. Chang (London: IntechOpen), 489–532.
Krogdahl, Å, Jaramillo-Torres, A., Ahlstrøm, Ø, Chikwati, E., Aasen, I. M., and Kortner, T. M. (2021). Protein value and health aspects of the seaweeds Saccharina latissima and Palmaria palmata evaluated with mink as model for monogastric animals. Anim. Feed Sci. Tech. 276:114902. doi: 10.1016/j.anifeedsci.2021.114902
Kumar, M. N. R. V. (2000). A review of chitin and chitosan applications. React. Funct. Polym. 46, 1–27. doi: 10.1016/S1381-5148(00)00038-9
Kumar, M., and Thakur, I. S. (2018). Municipal secondary sludge as carbon source for production and characterization of biodiesel from oleaginous bacteria. Bioresour. Technol. Rep. 4, 106–113. doi: 10.1016/j.biteb.2018.09.011
Kumar, M., Ghosh, P., Khosla, K., and Thakur, I. S. (2016). Biodiesel production from municipal secondary sludge. Bioresour. Technol. 216, 165–171. doi: 10.1016/J.BIORTECH.2016.05.078
Kumar, M., Sun, Y., Rathour, R., Pandey, A., Thakur, I. S., and Tsang, D. C. W. (2020). Algae as potential feedstock for the production of biofuels and value-added products: opportunities and challenges. Sci. Total Environ. 716:137116. doi: 10.1016/j.scitotenv.2020.137116
Kumar, M., Sundaram, S., Gnansounou, E., Larroche, C., and Thakur, I. S. (2018). Carbon dioxide capture, storage and production of biofuel and biomaterials by bacteria: a review. Bioresour. Technol. 247, 1059–1068. doi: 10.1016/j.biortech.2017.09.050
Kwasigroch, U., Bełdowska, M., Jędruch, A., and Łukawska-Matuszewska, K. (2021). Distribution and bioavailability of mercury in the surface sediments of the Baltic Sea. Environ. Sci. Pollut. Res. 28, 35690–35708. doi: 10.1007/s11356-021-13023-4
Lahaye, M., and Robic, A. (2007). Structure and functional properties of ulvan, a polysaccharide from green seaweeds. Biomacromolecules 8, 1765–1774. doi: 10.1021/bm061185q
Lang, X., Wang, T., Sun, M., Chen, X., and Liu, Y. (2020). Advances and applications of chitosan-based nanomaterials as oral delivery carriers: a review. Int. J. Biol. Macromol. 154, 433–445. doi: 10.1016/j.ijbiomac.2020.03.148
Langasco, R., Cadeddu, B., Formato, M., Lepedda, A. J., Cossu, M., Giunchedi, P., et al. (2017). Natural collagenic skeleton of marine sponges in pharmaceutics: innovative biomaterial for topical drug delivery. Mater. Sci. Eng. 70, 710–720. doi: 10.1016/j.msec.2016.09.041
Lanno, M., Silm, M., Shanskiy, M., Kisand, A., Orupõld, K., and Kriipsalu, M. (2020). Open windrow composting of fish waste in Estonia. Agron. Res. 18, 2465–2477. doi: 10.15159/AR.20.194
Latire, T., Legendre, F., Bigot, N., Carduner, L., Kellouche, S., Bouyoucef, M., et al. (2014). Shell extracts from the marine bivalve Pecten maximus regulate the synthesis of extracellular matrix in primary cultured human skin fibroblasts. PLoS One 9:e99931. doi: 10.1371/journal.pone.0099931
Laurent, S., Forge, D., Port, M., Roch, A., Robic, C., Vander Elst, L., et al. (2008). Magnetic iron oxide nanoparticles: synthesis, stabilization, vectorization, physicochemical characterizations, and biological applications. Chem. Rev. 108, 2064–2110. doi: 10.1021/cr068445e
Lee, H., Dellatore, S. M., Miller, W. M., and Messersmith, P. B. (2007). Mussel-inspired surface chemistry for multifunctional coatings. Science 318, 426–430. doi: 10.1126/science.1147241
Lehmann, J., and Joseph, S. (2009). Biochar for Environmental Management. Science, Technology and Implementation. London: Routlege.
Lehmann, J., Gaunt, J., and Rondon, M. (2006). Bio-char sequestration in terrestrial ecosystems – a review. Mitig. Adapt. Strateg. Glob. Change 11, 403–427. doi: 10.1007/s11027-005-9006-5
Leong, K. H., Chung, L. Y., Noordin, M. I., Onuki, Y., Morishita, M., and Takayama, K. (2011). Lectin-functionalized carboxymethylated kappa-carrageenan microparticles for oral insulin delivery. Carbohydr. Polym. 86, 555–565. doi: 10.1016/j.carbpol.2011.04.070
León-López, L., Bañuelos-Piña, A. M., Reyes-Moreno, C., Milán-Carrillo, J., Contreras-Andrade, I., Sánchez-Magaña, L. M., et al. (2019). Optimisation of temperature and time for the dark germination bioprocess of Moringa oleifera seeds to boost nutritional value, total phenolic content and antioxidant activity. Int. Food Res. J. 26, 831–839.
Liao, J., Jia, Y., Wang, B., Shi, K., and Qian, Z. (2018). Injectable hybrid poly(ε-caprolactone)-b-poly(ethylene glycol)-b-poly(ε-caprolactone) porous microspheres/alginate hydrogel cross-linked by calcium gluconate crystals deposited in the pores of microspheres improved skin wound healing. ACS Biomater. Sci. Eng. 4, 1029–1036. doi: 10.1021/acsbiomaterials.7b00860
Lima-Junior, E. M., de Moraes Filho, M. O., Costa, B. A., Fechine, F. V., de Moraes, M. E. A., Silva-Junior, F. R., et al. (2019). Innovative treatment using tilapia skin as a xenograft for partial thickness burns after a gunpowder explosion. J. Surg. Case Rep. 2019:rjz181. doi: 10.1093/jscr/rjz181
Liu, G., Zhou, Y., Liu, D., Wang, Q., Ruan, Z., He, Q., et al. (2015). Global transcriptome analysis of the tentacle of the jellyfish Cyanea capillata using deep sequencing and expressed sequence tags: Insight into the toxin-and degenerative disease-related transcripts. PLoS One 10:e0142680. doi: 10.1371/journal.pone.0142680
Liu, S., Trevathan-Tackett, S. M., Ewers Lewis, C. J., Ollivier, Q. R., Jiang, Z., Huang, X., et al. (2019). Beach-cast seagrass wrack contributes substantially to global greenhouse gas emissions. J. Environ. Manag. 231, 329–335. doi: 10.1016/j.jenvman.2018.10.047
Lopez-Caballero, M. E., Gimenez, B., Gomez-Guillen, M. C., and Montero, P. (2014). “Valorization and integral use and of seafood by-products,” in Engineering Aspects of Food Biotechnology, eds J. Texeira and A. Vicente (Boca Raton, FL: CRC Press), 367–413.
Lu, W. Y., Li, H. J., Li, Q. Y., and Wu, Y. C. (2021). Application of marine natural products in drug research. Bioorgan. Med. Chem. 35:116058. doi: 10.1016/j.bmc.2021.116058
Luo, Z., and Zhou, J. (2012). “Thermal conversion of biomass,” in Handbook of Climate Change Mitigation, eds W.-Y. Chen, J. Seiner, T. Suzuki, and M. Lackner (New York, NY: Springer), 1001–1042. doi: 10.1007/978-1-4419-7991-9_27
Machado, L., Magnusson, M., Paul, N. A., Kinley, R., de Nys, R., and Tomkins, N. (2016). Identification of bioactives from the red seaweed Asparagopsis taxiformis that promote antimethanogenic activity in vitro. J. Appl. Phycol. 28, 3117–3126. doi: 10.1007/s10811-016-0830-7
Macreadie, P. I., Bishop, M. J., and Booth, D. J. (2011). Implications of climate change for macrophytic rafts and their hitchhikers. Mar. Ecol. Prog. Ser. 443, 285–292. doi: 10.3354/meps09529
Macreadie, P. I., Trevathan-Tackett, S. M., Baldock, J. A., and Kelleway, J. J. (2017). Converting beach-cast seagrass wrack into biochar: a climate-friendly solution to a coastal problem. Sci. Total Environ. 574, 90–94. doi: 10.1016/j.scitotenv.2016.09.021
Magesh, N., Annam Renita, A., and Senthil Kumar, P. (2020). Practice on treating pharmaceutical compounds (antibiotics) present in wastewater using biosorption techniques with different biowaste compounds. A review. Environ. Prog. Sustain. Energy 39:e13429. doi: 10.1002/ep.13429
Makkar, H. P. S., Tran, G., Heuzé, V., Giger-Reverdin, S., Lessire, M., Lebas, F., et al. (2016). Seaweeds for livestock diets: a review. Anim. Feed Sci. Technol. 212, 1–17. doi: 10.1016/j.anifeedsci.2015.09.018
Malagurski, I., Levic, S., Nesic, A., Mitric, M., Pavlovic, V., and Dimitrijevic-Brankovic, S. (2017). Mineralized agar-based nanocomposite films: Potential food packaging materials with antimicrobial properties. Carbohydr. Polym. 175, 55–62. doi: 10.1016/j.carbpol.2017.07.064
Manivasagan, P., and Oh, J. (2016). Marine polysaccharide-based nanomaterials as a novel source of nanobiotechnological applications. Int. J. Biol. Macromol. 82, 315–327. doi: 10.1016/j.ijbiomac.2015.10.081
Manivasagan, P., Bharathiraja, S., Moorthy, M. S., Oh, Y.-O., Seo, H., and Oh, J. (2017). Marine biopolymer-based nanomaterials as a novel platform for theranostic applications. Polym. Rev. 57, 631–667. doi: 10.1080/15583724.2017.1311914
Manzocco, L., Valoppi, F., Calligaris, S., Andreatta, F., Spilimbergo, S., and Nicoli, M. C. (2017). Exploitation of κ-carrageenan aerogels as template for edible oleogel preparation. Food Hydrocoll. 71, 68–75. doi: 10.1016/j.foodhyd.2017.04.021
Marinho-Soriano, E., Nunes, S. O., Carneiro, M. A. A., and Pereira, D. C. (2009). Nutrients’ removal from aquaculture wastewater using the macroalgae Gracilaria birdiae. Biomass Bioenergy 33, 327–331. doi: 10.1016/j.biombioe.2008.07.002
Martínez-García, C., González-Fonteboa, B., Carro-López, D., and Pérez-Ordóñez, J. L. (2020). Mussel shells: A canning industry by-product converted into a bio-based insulation material. J. Cleaner Prod. 269:122343. doi: 10.1016/j.jclepro.2020.122343
Martínez-Sanz, M., Cebrián-Lloret, V., Mazarro-Ruiz, J., and López-Rubio, A. (2020a). Improved performance of less purified cellulosic films obtained from agar waste biomass. Carbohydr. Polym. 233:115887. doi: 10.1016/j.carbpol.2020.115887
Martínez-Sanz, M., Gómez-Mascaraque, L. G., Ballester, A., Martínez-Abad, A., Brodkorb, A., and López-Rubio, A. (2019). Production of unpurified agar-based extracts from red seaweed Gelidium sesquipedale by means of simplified extraction protocols. Algal Res. 38:101420. doi: 10.1016/j.algal.2019.101420
Martínez-Sanz, M., Ström, A., Lopez-Sanchez, P., Knutsen, S. H., Ballance, S., Zobel, H. K., et al. (2020b). Advanced structural characterisation of agar-based hydrogels: Rheological and small angle scattering studies. Carbohydr. Polym. 236:115655. doi: 10.1016/j.carbpol.2019.115655
Marungrueng, K., and Pavasant, P. (2007). High performance biosorbent (Caulerpa lentillifera) for basic dye removal. Bioresour. Technol. 98, 1567–1572. doi: 10.1016/j.biortech.2006.06.010
Mazur, L. P., Cechinel, M. A. P., de Souza, S. M. A. G. U., Boaventura, R. A. R., and Vilar, V. J. P. (2018). Brown marine macroalgae as natural cation exchangers for toxic metal removal from industrial wastewaters: a review. J. Environ. Manag. 223, 215–253. doi: 10.1016/j.jenvman.2018.05.086
McGwynne, L. E., McLachlan, A., and Furstenberg, J. P. (1988). Wrack breakdown on sandy beaches – Its impact on interstitial meiofauna. Mar. Environ. Res. 25, 213–232. doi: 10.1016/0141-1136(88)90004-9
Mellou, F., Varvaresou, A., and Papageorgiou, S. (2019). Renewable sources: applications in personal care formulations. Int. J. Cosmet. Sci. 41, 517–525. doi: 10.1111/ics.12564
Mendes, A. C., Stephansen, K., and Chronakis, I. S. (2017). Electrospinning of food proteins and polysaccharides. Food Hydrocoll. 68, 53–68. doi: 10.1016/j.foodhyd.2016.10.022
Meriac, A. (2019). Smolt Production and the Potential for Solid Waste Collection in Norway. [Nofima Report 25/2019]. Tromsø: Nofima.
Mincea, M., Negrulescu, A., and Ostafe, V. (2012). Preparation, modification, and applications of chitin nanowhiskers: a review. Rev. Adv. Mater. Sci. 30, 225–242.
Ministry of Trade, Industry and Fisheries (2019). Regjeringa sin strategi for auka verdiskaping frå marint restråstoff / The Government’s Strategy for Increasing Value Creation from Marine Residual Raw Materials. [Strategy W-0029 N]. Oslo: Ministry of Trade, Industry and Fisheries.
Mokhtar, N., Aziz, E. A., Aris, A., Ishak, W. F. W., and Ali, N. S. M. (2017). Biosorption of azo-dye using marine macro-alga of Euchema spinosum. J. Environ. Chem. Eng. 5, 5721–5731. doi: 10.1016/j.jece.2017.10.043
Molina-Alcaide, E., Carro, M. D., Roleda, M. Y., Weisbjerg, M. R., Lind, V., and Novoa-Garrido, M. (2017). In vitro ruminal fermentation and methane production of different seaweed species. Anim. Feed Sci. Technol. 228, 1–12. doi: 10.1016/j.anifeedsci.2017.03.012
Moraes, M. A., Cocenza, D. S., Vasconcellos, F. C., Fraceto, L. F., and Beppu, M. M. (2013). Chitosan and alginate biopolymer membranes for remediation of contaminated water with herbicides. J. Environ. Manag. 131, 222–227. doi: 10.1016/j.jenvman.2013.09.028
Morris, J. P., Backeljau, T., and Chapelle, G. (2019). Shells from aquaculture: a valuable biomaterial, not a nuisance waste product. Rev. Aquac. 11, 42–57. doi: 10.1111/raq.12225
Mossbauer, M., Haller, I., Dahlke, S., and Schernewski, G. (2012). Management of stranded eelgrass and macroalgae along the German Baltic coastline. Ocean Coast. Manag. 57, 1–9. doi: 10.1016/j.ocecoaman.2011.10.012
Mullerova, S., Baldikova, E., Prochazkova, J., Pospiskova, K., and Safarik, I. (2019). Magnetically modified macroalgae Cymopolia barbata biomass as an adsorbent for safranin O removal. Mater. Chem. Phys. 225, 174–180. doi: 10.1016/j.matchemphys.2018.12.074
Munro, L. (2019). Scottish Fish Farm Production Survey 2018. [Marine Scotland Science Report]. Edinburgh: Scottish Government.
Myhre, M., Richardsen, R., Nystøyl, R., and Strandheim, G. (2021). Analyse marint restråstoff 2020-2022. Tilgjengelighet og anvendelse av marint restråstoff i fra norsk fiskeri- og havbruksnćring. Report number: 2021:00633. Trondheim: SINTEF Ocean Rapporte.
Navarro, A. E., Lim, H., Chang, E., Lee, Y., and Manrique, A. S. (2014). Uptake of sulfa drugs from aqueous solutions by marine algae. Sep. Sci. Technol. 49, 2175–2181. doi: 10.1080/01496395.2014.926930
Ngah, W. S., Teong, L. C., and Hanafiah, M. A. K. M. (2010). Adsorption of dyes and heavy metal ions by chitosan composites: a review. Carbohydr. Polym. 83, 1446–1456. doi: 10.1016/j.carbpol.2010.11.004
Nguyen, S. T., Feng, J., Ng, S. K., Wong, J. P. W., Tan, V. B. C., and Duong, H. M. (2014). Advanced thermal insulation and absorption properties of recycled cellulose aerogels. Coll. Surf. A 445, 128–134. doi: 10.1016/j.colsurfa.2014.01.015
Nicklas, M., Schatton, W., Heinemann, S., Hanke, T., and Kreuter, J. (2009). Preparation and characterization of marine sponge collagen nanoparticles and employment for the transdermal delivery of 17β-estradiol-hemihydrate. Drug Dev. Ind. Pharm. 35, 1035–1042. doi: 10.1080/03639040902755213
Nikam, A. P., Mukesh, P. R., and Haudhary, S. P. (2014). Nanoparticles – an overview. Int. J. Res. Dev. Pharm. Life Sci. 3, 1121–1127.
Nisticò, R. (2017). Aquatic-derived biomaterials for a sustainable future: a European opportunity. Resources 6:65. doi: 10.3390/resources6040065
Nitta, S. K., and Numata, K. (2013). Biopolymer-based nanoparticles for drug/gene delivery and tissue engineering. Int. J. Mol. Sci. 14, 1629–1654. doi: 10.3390/ijms14011629
Nordstrom, K. F., Jackson, N. L., Korotky, K. H., and Puleo, J. A. (2011). Aeolian transport rates across raked and unraked beaches on a developed coast. Earth Surf. Processes Landf. 36, 779–789. doi: 10.1002/esp.2105
Novoa-Garrido, M., Rebours, C., Aanensen, L., Torp, T., Lind, V., and Steinshamn, H. (2017). Effect of seaweed on gastrointestinal microbiota isolated from Norwegian White sheep. Acta Agric. Scand. A Anim. Sci. 66, 152–160. doi: 10.1080/09064702.2017.1310287
Oliveira, C., Neves, N. M., Reis, R. L., Martins, A., and Silva, T. H. (2018). Gemcitabine delivered by fucoidan/chitosan nanoparticles presents increased toxicity over human breast cancer cells. Nanomedicine (London, England) 13, 2037–2050. doi: 10.2217/nnm-2018-0004
Oliveira, J., Belchior, A., da Silva, V. D., Rotter, A., Petrovski, Ž., Almeida, P. L., et al. (2020). Marine environmental plastic pollution: mitigation by microorganism degradation and recycling valorization. Front. Mar. Sci. 7:567126. doi: 10.3389/fmars.2020.567126
Orr, M., Zimmer, M., Jelinski, D. E., and Mews, M. (2005). Wrack deposition on different beach types: Spatial and temporal variation in the pattern of subsidy. Ecology 86, 1496–1507. doi: 10.1890/04-1486
Oso, A. O., Idowu, A. A., and Niameh, O. T. (2011). Growth response, nutrient and mineral retention, bone mineralisation and walking ability of broiler chickens fed with dietary inclusion of various unconventional mineral sources. J. Anim. Physiol. Anim. Nutr. 95, 461–467. doi: 10.1111/j.1439
Osorio-López, C., Seco-Reigosa, N., Garrido-Rodríguez, B., Cutillas-Barreiro, L., Arias-Estévez, M., Fernández-Sanjurjo, M. J., et al. (2014). As(V) adsorption on forest and vineyard soils and pyritic material with or without mussel shell: kinetics and fractionation. J. Taiwan Inst. Chem. Eng. 45, 1007–1014. doi: 10.1016/j.jtice.2013.10.001
Oun, A. A., and Rhim, J. W. (2017). Carrageenan-based hydrogels and films: Effect of ZnO and CuO nanoparticles on the physical, mechanical, and antimicrobial properties. Food Hydrocoll. 67, 45–53. doi: 10.1016/j.foodhyd.2016.12.040
Özkan Gülzari, Ş, Lind, V., Aasen, I. M., and Steinshamn, H. (2019). Effect of supplementing sheep diets with macroalgae species on in vivo nutrient digestibility, rumen fermentation and blood amino acid profile. Animal 13, 2792–2801. doi: 10.1017/S1751731119001502
Ozudogru, Y., Merdivan, M., and Göksan, T. (2016). Biosorption of methylene blue from an aqueous solution by iron oxide-coated Cystoseira barbata. J. Turkish Chem. Soc. Sec. A 3, 551–564. doi: 10.18596/jotcsa.40601
Peng, F. Q., Ying, G. G., Yang, B., Liu, S., Lai, H. J., Liu, Y. S., et al. (2014). Biotransformation of progesterone and norgestrel by two freshwater microalgae (Scenedesmus obliquus and Chlorella pyrenoidosa): transformation kinetics and products identification. Chemosphere 95, 581–588. doi: 10.1016/j.chemosphere.2013.10.013
Pérez Roda, A., Gilman, E., Huntington, T., Kennelly, S. J., Suuronen, P., Chaloupka, M., et al. (2019). A Third Assessment of Global Marine Fisheries Discards. [FAO Fisheries and Aquaculture Technical Paper No. 633]. Rome: FAO.
Pitcairn, J., Warmington, J., Gandy, S., Deswarte, F., and Bell, J. (2017). Biorefining potential for Scotland: mapping bioresource arisings across Scotland. Ind. Biotechnol. 13, 301–305. doi: 10.1089/ind.2017.29111.jpi
Pleym, I. E., Svorken, M., and Birthe, V. (2019). Verdifulle rester. Muligheter for norsk marint restråstoff / Valuable Waste. Opportunities for Norwegian Marine Raw Material. [Nofima Report 9/2019]. Tromsø: Nofima.
Poblete-Castro, I., Hoffmann, S. L., Becker, J., and Wittmann, C. (2020). Cascaded valorization of seaweed using microbial cell factories. Curr. Opin. Biotechnol. 65, 102–113. doi: 10.1016/j.copbio.2020.02.008
Porshnov, D., Ozols, V., Ansone-Bertina, L., Burlakovs, J., and Klavins, M. (2018). Thermal decomposition study of major refuse derived fuel components. Energy Proc. 147, 48–53. doi: 10.1016/j.egypro.2018.07.032
Prihanto, A. A., Nurdiani, R., and Bagus, A. D. (2019). Production and characteristics of fish protein hydrolysate from parrotfish (Chlorurus sordidus) head. PeerJ 7:e8297. doi: 10.7717/peerj.8297
Quignard, F., Valentin, R., and Di Renzo, F. (2008). Aerogel materials from marine polysaccharides. N. J. Chem. 32, 1300–1310. doi: 10.1039/B808218A
Rae, I. B., Gibb, S. W., and Lu, S. (2009). Biosorption of Hg from aqueous solutions by crab carapace. J. Hazard. Mater. 164, 1601–1604. doi: 10.1016/j.jhazmat.2008.09.052
Rahmati, M., Alipanahi, Z., and Mozafari, M. (2019). Emerging biomedical applications of algal polysaccharides. Curr. Pharm. Design 25, 1335–1344. doi: 10.2174/1381612825666190423160357
Ramamoorthy, S. K., Skrifvars, M., and Persson, A. (2015). A review of natural fibers used in biocomposites: Plant, animal and regenerated cellulose fibers. Polym. Rev. 55, 107–162. doi: 10.1080/15583724.2014.971124
Rathod, M., Mody, K., and Basha, S. (2014). Efficient removal of phosphate from aqueous solutions by red seaweed Kappaphycus alverezii. J. Cleaner Prod. 84, 484–493. doi: 10.1016/j.jclepro.2014.03.064
Raveendran, S., Yoshida, Y., Maekawa, T., and Kumar, D. S. (2013). Pharmaceutically versatile sulfated polysaccharide based bionano platforms. Nanomedicine 9, 605–626. doi: 10.1016/j.nano.2012.12.006
Reys, L. L., Silva, S. S., Pirraco, R. P., Marques, A. P., Mano, J. F., Silva, T. H., et al. (2017). Influence of freezing temperature and deacetylation degree on the performance of freeze-dried chitosan scaffolds towards cartilage tissue engineering. Eur. Polym. J. 95, 232–240. doi: 10.1016/j.eurpolymj.2017.08.017
Rinaudo, M. (2006). Chitin and chitosan: properties and applications. Progress Polym. Sci. 31, 603–632. doi: 10.1016/j.progpolymsci.2006.06.001
Risén, E., Nordström, J., Malmström, M. E., and Gröndahl, F. (2017). Non-market values of algae beach-cast management – study site Trelleborg, Sweden. Ocean Coast. Manag. 140, 59–67. doi: 10.1016/j.ocecoaman.2017.02.009
Ritchie, H., and Roser, M. (2018). “Plastic Pollution”. Published online at OurWorldInData.org. Available online at: ‘https://ourworldindata.org/plastic-pollution’ (Accessed May 3, 2021).
Rodríguez, F., Morán, L., González, G., Troncoso, E., and Zúñiga, R. N. (2017). Collagen extraction from mussel byssus: a new marine collagen source with physicochemical properties of industrial interest. J. Food Sci. Technol. 54, 1228–1238.
Rowbotham, J. S., Dyer, P. W., Greenwell, H. C., and Theodorou, M. K. (2012). Thermochemical processing of macroalgae: a late bloomer in the development of third-generation biofuels? Biofuels 3, 441–461. doi: 10.4155/bfs.12.29
Rudovica, V., and Bartkevics, V. (2015). Chemical elements in the muscle tissues of European eel (Anguilla anguilla) from selected lakes in Latvia. Environ. Monit. Assess. 187:608. doi: 10.1007/s10661-015-4832-8
Ruocco, N., Costantini, S., Guariniello, S., and Costantini, M. (2016). Polysaccharides from the marine environment with pharmacological, cosmeceutical and nutraceutical potential. Molecules 21:551. doi: 10.3390/molecules21050551
Rustad, T., Storrø, I., and Slizyte, R. (2011). Possibilities for the utilisation of marine by-products. Int. J. Food Sci. Technol. 46, 2001–2014. doi: 10.1111/j.1365-2621.2011.02736.x
Safarik, I., Ashoura, N., Maderova, Z., Pospiskova, K., Baldikova, E., and Safarikova, M. (2016a). Magnetically modified Posidonia oceanica biomass as an adsorbent for organic dyes removal. Mediterr. Mar. Sci. 17, 351–358. doi: 10.12681/mms.1549
Safarik, I., Baldikova, E., Pospiskova, K., and Safarikova, M. (2016b). Magnetic modification of diamagnetic agglomerate forming powder materials. Particuology 29, 169–171. doi: 10.1016/j.partic.2016.05.002
Safarik, I., Baldikova, E., Prochazkova, J., and Pospiskova, K. (2020a). Magnetic particles in algae biotechnology: recent updates. J. Appl. Phycol. 32, 1743–1753. doi: 10.1007/s10811-020-02109-0
Safarik, I., Baldikova, E., Prochazkova, J., Safarikova, M., and Pospiskova, K. (2018). Magnetically modified agricultural and food waste: preparation and application. J. Agric. Food Chem. 66, 2538–2552. doi: 10.1021/acs.jafc.7b06105
Safarik, I., Prochazkova, J., Baldikova, E., and Pospiskova, K. (2020b). “Magnetically responsive algae and seagrass derivatives for pollutant removal,” in Wastes: Solutions, Treatments and Opportunities III, eds C. Vilarinho, F. Castro, M. Goncalves, and A. L. Fernando (Boca Raton, FL: CRC Press), 131–136.
Sahiner, N., Sagbas, S., and Yılmaz, S. (2017). Microgels derived from different forms of carrageenans, kappa, iota, and lambda for biomedical applications. MRS Adv. 2, 2521–2527. doi: 10.1557/adv.2017.415
Salaberria, A. M., Fernandes, S. C. M., Diaz, R. H., and Labidi, J. (2015a). Processing of α-chitin nanofibers by dynamic high pressure homogenization: characterization and antifungal activity against A. niger. Carbohydr. Polym. 116, 286–291. doi: 10.1016/j.carbpol.2014.04.047
Salaberria, A. M., Labidi, J., and Fernandes, S. C. M. (2014). Chitin nanocrystals and nanofibers as nano-sized fillers into thermoplastic starch-based biocomposites processed by melt-mixing. Chem. Eng. J. 256, 356–364. doi: 10.1016/j.cej.2014.07.009
Salaberria, A. M., Labidi, J., and Fernandes, S. C. M. (2015b). Different routes to turn chitin into stunning nano-objects. Eur. Polym. J. 68, 503–515. doi: 10.1016/j.eurpolymj.2015.03.005
Saldarriaga-Hernandez, S., Hernandez-Vargas, G., Iqbal, H. M. N., Barceló, D., and Parra-Saldívar, R. (2020). Bioremediation potential of Sargassum sp. biomass to tackle pollution in coastal ecosystems: circular economy approach. Sci. Total Environ. 715:136978. doi: 10.1016/j.scitotenv.2020.136978
Santos, S. C. R., Ungureanu, G., Volf, I., Boaventura, R. A. R., and Botelho, C. M. S. (2018). “Macroalgae biomass as sorbent for metal ions,” in Biomass as Renewable Raw Material to Obtain Bioproducts of High-Tech Value, eds V. Popa and I. Volf (Amsterdam: Elsevier), 69–112. doi: 10.1016/B978-0-444-63774-1.00003-X
Saranya, R., Tamil Selvi, A., Jayapriya, J., and Aravindhan, R. (2020). Synthesis of fat liquor through fish waste valorization, characterization and applications in tannery industry. Waste Biomass Valorization 11, 6637–6647. doi: 10.1007/s12649-020-00944-3
Sathishkumar, P., Kamala-Kannan, S., Cho, M., Kim, J. S., Hadibarata, T., Salim, M. R., et al. (2014). Laccase immobilization on cellulose nanofiber: the catalytic efficiency and recyclic application for simulated dye effluent treatment. J. Mol. Catal. B 100, 111–120. doi: 10.1016/j.molcatb.2013.12.008
Scialla, S., Carella, F., Dapporto, M., Sprio, S., Piancastelli, A., Palazzo, B., et al. (2020). Mussel shell-derived macroporous 3D scaffold: characterization and optimization study of a bioceramic from the circular economy. Mar. Drugs 18:309. doi: 10.3390/md18060309
Scotland Food and Drink (2016). Aquaculture Growth to 2030. A Strategic Plan for Farming Scotland’s Seas. Edinburgh: Scotland Food and Drink.
Scottish Enterprise (2019). Biorefinery roadmap for scotland: building a sustainable future. Ind. Biotechnol. 15, 284–289. doi: 10.1089/ind.2019.29188.sen
Senturk Parreidt, T., Müller, K., and Schmid, M. (2018). Alginate-based edible films and coatings for food packaging applications. Foods (Basel) 7:170. doi: 10.3390/foods7100170
Sheng, P. X., Ting, Y.-P., and Chen, J. P. (2007). Biosorption of heavy metal ions (Pb, Cu, and Cd) from aqueous solutions by the marine alga Sargassum sp. in single- and multiple-metal systems. Ind. Eng. Chem. Res. 46, 2438–2444. doi: 10.1021/ie0615786
Shin, M., Shin, J. Y., Kim, K., Yang, B., Han, J. W., Kim, N.-K., et al. (2020). The position of lysine controls the catechol-mediated surface adhesion and cohesion in underwater mussel adhesion. J. Coll. Interface Sci. 563, 168–176. doi: 10.1016/j.jcis.2019.12.082
Shobier, A. H., El-Sadaawy, M. M., and El-Said, G. F. (2020). Removal of hexavalent chromium by ecofriendly raw marine green alga Ulva fasciata: kinetic, thermodynamic and isotherm studies. Egypt. J. Aquat. Res. 46, 325–331. doi: 10.1016/j.ejar.2020.09.003
Shuba, E. S., and Kifle, D. (2018). Microalgae to biofuels: ‘Promising’ alternative and renewable energy, review. Renew. Sustain. Energy Rev. 81(Part 1), 743–755. doi: 10.1016/j.rser.2017.08.042
Sikarwar, V. S., Zhao, M., Fennell, P. S., Shah, N., and Anthony, E. J. (2017). Progress in biofuel production from gasification. Progress Energy Combust. Sci. 61, 189–248. doi: 10.1016/j.pecs.2017.04.001
Silva, A., Delerue-Matos, C., Figueiredo, S. A., and Freitas, O. M. (2019). The use of algae and fungi for removal of pharmaceuticals by bioremediation and biosorption processes: a review. Water 11:1555. doi: 10.3390/w11081555
SINTEF Ocean and Kontali Analyse (2020). Marint Restråstoff – Historisk Database. Available online at: https://www.marintrestrastoff.no/ [Accessed July 16, 2021].
Sirviö, J. A., Kolehmainen, A., Liimatainen, H., Niinimäki, J., and Hormi, O. E. O. (2014). Biocomposite cellulose-alginate films: promising packaging materials. Food Chem. 151, 343–351. doi: 10.1016/j.foodchem.2013.11.037
Smirnova, N. V., Kolbe, K. A., Dresvyanina, E. N., Grebennikov, S. F., Dobrovolskaya, I. P., Yudin, V. E., et al. (2019). Effect of chitin nanofibrils on biocompatibility and bioactivity of the chitosan-based composite. Materials (Basel) 12:1874. doi: 10.3390/ma12111874
Sokołowski, A., Jankowska, E., Bałazy, P., and Jędruch, A. (2021). Distribution and extent of benthic habitats in Puck Bay (Gulf of Gdañsk, southern Baltic Sea). Oceanologia 63, 301–320. doi: 10.1016/j.oceano.2021.03.001
Son, E. B., Poo, K. M., Chang, J. S., and Chae, K. J. (2018a). Heavy metal removal from aqueous solutions using engineered magnetic biochars derived from waste marine macro-algal biomass. Sci. Total Environ. 615, 161–168. doi: 10.1016/j.scitotenv.2017.09.171
Son, E. B., Poo, K. M., Mohamed, H. O., Choi, Y. J., Cho, W. C., and Chae, K. J. (2018b). A novel approach to developing a reusable marine macro-algae adsorbent with chitosan and ferric oxide for simultaneous efficient heavy metal removal and easy magnetic separation. Bioresour. Technol. 259, 381–387. doi: 10.1016/j.biortech.2018.03.077
Sousa, A. M. M., and Gonçalves, M. P. (2015). Strategies to improve the mechanical strength and water resistance of agar films for food packaging applications. Carbohydr. Polym. 132, 196–204. doi: 10.1016/j.carbpol.2015.06.022
Stévant, P., Rebours, C., and Chapman, A. (2017). Seaweed aquaculture in Norway: recent industrial developments and future perspectives. Aquac. Int. 25, 1373–1390. doi: 10.1007/s10499-017-0120-7
Suurs, P., and Barbut, S. (2020). Collagen use for co-extruded sausage casings–a review. Trends Food Sci. Technol. 102, 91–101. doi: 10.1016/j.tifs.2020.06.011
Suursaar, Ü, Torn, K., Martin, G., Herkül, K., and Tiit, K. (2014). Formation and species composition of stormcast beach wrack in the Gulf of Riga, Baltic Sea. Oceanologia 56, 673–695. doi: 10.5697/oc.56-4.673
Swatschek, D., Schatton, W., Müller, W., and Kreuter, J. (2002). Microparticles derived from marine sponge collagen (SCMPs): preparation, characterization and suitability for dermal delivery of all-trans retinol. Eur. J. Pharm. Biopharm. 54, 125–133. doi: 10.1016/s0939-6411(02)00046-2
Tayyab, U., Novoa-Garrido, M., Roleda, M. Y., Lind, V., and Weisbjerg, M. R. (2016). Ruminal and intestinal protein degradability of various seaweed species measured in situ in dairy cows. Anim. Feed Sci. Technol. 213, 44–54. doi: 10.1016/j.anifeedsci.2016.01.003
Teo, W. E., and Ramakrishna, S. (2006). A review on electrospinning design and nanofibre assemblies. Nanotechnology 17, R89–R106. doi: 10.1088/0957-4484/17/14/R01
Thai, H., Nguyen, C. T., Thach, L. T., Tran, M. T., Mai, H. D., Nguyen, T. T. T., et al. (2020). Characterization of chitosan/alginate/lovastatin nanoparticles and investigation of their toxic effects in vitro and in vivo. Sci. Rep. 10:909. doi: 10.1038/s41598-020-57666-8
Thies, J. E., and Rillig, M. C. (2012). “Characteristics of biochar: biological properties,” in Biochar for Environmental Management, eds J. Lehmann and S. Joseph (London: Routledge), 119–138.
Toskas, G., Heinemann, S., Heinemann, C., Cherif, C., Hund, R. D., Roussis, V., et al. (2012). Ulvan and ulvan/chitosan polyelectrolyte nanofibrous membranes as a potential substrate material for the cultivation of osteoblasts. Carbohydr. Polym. 89, 997–1002. doi: 10.1016/j.carbpol.2012.04.045
Trache, D., Hussin, M. H., Hui Chuin, C. T., Sabar, S., Fazita, M. R. N., Taiwo, O. F. A., et al. (2016). Microcrystalline cellulose: isolation, characterization and bio-composites application – a review. Int. J. Biol. Macromol. 93, 789–804. doi: 10.1016/j.ijbiomac.2016.09.056
Tuck, C. O., Pérez, E., Horváth, I. T., Sheldon, R. A., and Poliakoff, M. (2012). Valorization of biomass: Deriving more value from waste. Science (New York) 337, 695–699. doi: 10.1126/science.1218930
Tziveleka, L. A., Pippa, N., Georgantea, P., Ioannou, E., Demetzos, C., and Roussis, V. (2018). Marine sulfated polysaccharides as versatile polyelectrolytes for the development of drug delivery nanoplatforms: Complexation of ulvan with lysozyme. Int. J. Biol. Macromol. 118, 69–75. doi: 10.1016/j.ijbiomac.2018.06.050
Tziveleka, L. A., Sapalidis, A., Kikionis, S., Aggelidou, E., Demiri, E., Kritis, A., et al. (2020). Hybrid sponge-like scaffolds based on ulvan and gelatin: design, characterization and evaluation of their potential use in bone tissue engineering. Materials (Basel) 13:1763. doi: 10.3390/ma13071763
Ubando, A. T., Africa, A. D. M., Maniquiz-Redillas, M. C., Culaba, A. B., Chen, W.-H., and Chang, J.-S. (2021). Microalgal biosorption of heavy metals: a comprehensive bibliometric review. J. Hazard. Mater. 402:123431. doi: 10.1016/j.jhazmat.2020.123431
Ungureanu, G., Filote, C., Santos, S. C. R., Boaventura, R. A. R., Volf, I., and Botelho, C. M. S. (2016). Antimony oxyanions uptake by green marine macroalgae. J. Environ. Chem. Eng. 4, 3441–3450. doi: 10.1016/j.jece.2016.07.023
Vanparijs, N., Nuhn, L., and De Geest, B. G. (2017). Transiently thermoresponsive polymers and their applications in biomedicine. Chem. Soc. Rev. 46, 1193–1239. doi: 10.1039/C6CS00748A
Varaprasad, K., Jayaramudu, T., Kanikireddy, V., Toro, C., and Sadiku, E. R. (2020). Alginate-based composite materials for wound dressing application: a mini review. Carbohydr. Polym. 236:116025. doi: 10.1016/j.carbpol.2020.116025
Vassalini, I., Gjipalaj, J., Crespi, S., Gianocelli, A., Mella, M., Ferroni, M., et al. (2020). Alginate-derived active blend enhances adsorption and photocatalytic removal of organic pollutants in water. Adv. Sustain. Syst. 4:1900112. doi: 10.1002/adsu.201900112
Vázquez, J. A., Durán, A. I., Menduíña, A., and Nogueira, M. (2020). Biotechnological valorization of food marine wastes: microbial productions on peptones obtained from aquaculture by-products. Biomolecules 10:1184. doi: 10.3390/biom10081184
Vázquez, J. A., Fernández-Compás, A., Blanco, M., Rodríguez-Amado, I., Moreno, H., Borderías, J., et al. (2019a). Development of bioprocesses for the integral valorisation of fish discards. Biochem. Eng. J. 144, 198–208. doi: 10.1016/j.bej.2019.02.004
Vázquez, J. A., Sotelo, C. G., Sanz, N., Pérez-Martín, R. I., Rodríguez-Amado, I., and Valcarcel, J. (2019b). Valorization of aquaculture by-products of salmonids to produce enzymatic hydrolysates: process optimization, chemical characterization and evaluation of bioactives. Mar. Drugs 17:676. doi: 10.3390/md17120676
Venkatesan, J., Anil, S., and Kim, S.-K. (2017). Seaweed Polysaccharides. Isolation, Biological and Biomedical Applications. Amsterdam: Elsevier.
Venkatesan, J., Anil, S., Kim, S. K., and Shim, M. S. (2016). Seaweed polysaccharide-based nanoparticles: preparation and applications for drug delivery. Polymers (Basel) 8:30. doi: 10.3390/polym8020030
Venkatesan, J., Lowe, B., Anil, S., Manivasagan, P., Kheraif, A. A. A., Kang, K. H., et al. (2015). Seaweed polysaccharides and their potential biomedical applications. Starch 67, 381–390. doi: 10.1002/star.201400127
Vidal, R. R. L., and Moraes, J. S. (2019). Removal of organic pollutants from wastewater using chitosan: a literature review. Int. J. Environ. Sci. Technol. 16, 1741–1754. doi: 10.1007/s13762-018-2061-8
Vigneshpriya, D., Krishnaveni, N., and Renganathan, S. (2017). Marine brown macroalga Sargassum wightii as a novel biosorbent for removal of brilliant green dye from aqueous solution: Kinetics, equilibrium isotherm modeling and phytotoxicity of treated and untreated dye. Desalination Water Treat. 78, 300–312. doi: 10.5004/dwt.2017.20884
Vijayaraghavan, J., Bhagavathi Pushpa, T., Sardhar Basha, S. J., Vijayaraghavan, K., and Jegan, J. (2015). Evaluation of red marine alga Kappaphycus alvarezii as biosorbent for methylene blue: isotherm, kinetic, and mechanism studies. Sep. Sci. Technol. 50, 1120–1126. doi: 10.1080/01496395.2014.965260
Villares, R., Fernández-Lema, E., and López-Mosquera, M. E. (2016). Evaluation of beach wrack for use as an organic fertilizer: temporal survey in different areas. Thalassas 32, 19–36. doi: 10.1007/s41208-015-0003-5
Vu, C. H. T., and Won, K. (2014). Leaching-resistant carrageenan-based colorimetric oxygen indicator films for intelligent food packaging. J.Agric. Food Chem. 62, 7263–7267. doi: 10.1021/jf5014764
Wang, B., Wan, Y., Zheng, Y., Lee, X., Liu, T., Yu, Z., et al. (2019). Alginate-based composites for environmental applications: a critical review. Crit. Rev. Environ. Sci. Technol. 49, 318–356. doi: 10.1080/10643389.2018.1547621
Wang, S., Wang, X., Poon, K., Wang, Y., Li, S., Liu, H., et al. (2013). Removal and reductive dechlorination of triclosan by Chlorella pyrenoidosa. Chemosphere 92, 1498–1505. doi: 10.1016/j.chemosphere.2013.03.067
Ween, O., Kjerstad, M., and Stangeland, J. (2018). “Strategies for shifting marine proteins up the value chain to develop added-value ingredients,” in Blue Growth: Aquaculture, Fisheries, Market and Health Perspectives, eds B. J. Thu and L. K. Akslen-Hoel (Oslo: Orkana Akademisk).
Weinberger, F., Paalme, T., and Wikström, S. A. (2020). Seaweed resources of the Baltic Sea, Kattegat and German and Danish North Sea coasts. Bot. Mar. 63, 61–72. doi: 10.1515/bot-2019-0019
Whitman, T., and Lehmann, J. (2009). Biochar – one way forward for soil carbon in offset mechanisms in Africa? Environ. Sci. Policy 12, 1024–1027. doi: 10.1016/j.envsci.2009.07.013
Woolf, D., Amonette, J. E., Street-Perrott, F. A., Lehmann, J., and Joseph, S. (2010). Sustainable biochar to mitigate global climate change. Nat. Commun. 1:56. doi: 10.1038/ncomms1053
World Health Organization (2021). Musculoskeletal Conditions. Available online at: https://www.who.int/news-room/fact-sheets/detail/musculoskeletal-conditions [Accessed May 7, 2021].
WRAP (2012). Sector Guidance Note: Preventing Waste in the Fish Processing Supply Chain. Banbury: Waste and Resources Action Programme (WRAP).
Wu, S. J., Don, T. M., Lin, C. W., and Mi, F. L. (2014). Delivery of berberine using chitosan/fucoidan-taurine conjugate nanoparticles for treatment of defective intestinal epithelial tight junction barrier. Mar. Drugs 12, 5677–5697. doi: 10.3390/md12115677
Yadav, M., Goswami, P., Paritosh, K., Kumar, M., Pareek, N., and Vivekanand, V. (2019). Seafood waste: a source for preparation of commercially employable chitin/chitosan materials. Bioresour. Bioprocess. 6:8. doi: 10.1186/s40643-019-0243-y
Yang, L., Wang, Y., Liu, A., and Zhang, Y. (2019). CoOx/MoOy-anchored multi-wrinkled biomass carbon as a promising material for rapidly selective methyl blue removal. J. Mater. Sci. 54, 11024–11036. doi: 10.1007/s10853-019-03612-7
Yao, Z., Xia, M., Li, H., Chen, T., Ye, Y., and Zheng, H. (2014). Bivalve shell: not an abundant useless waste but a functional and versatile biomaterial. Crit. Rev. Environ. Sci. Technol. 44, 2502–2530. doi: 10.1080/10643389.2013.829763
Yu, I. K., and Tsang, D. C. W. (2017). Conversion of biomass to hydroxymethylfurfural: a review of catalytic systems and underlying mechanisms. Bioresour. Technol. 238, 716–732. doi: 10.1016/j.biortech.2017.04.026
Zeng, J.-B., He, Y.-S., Li, S.-L., and Wang, Y.-Z. (2012). Chitin whiskers: an overview. Biomacromolecules 13, 1–11. doi: 10.1021/bm201564a
Zero Waste Scotland (2015). Sector Study on Beer, Whisky and Fish. [Final Report]. Harwell: Ricardo-AEA Ltd.
Zhang, M., Zhao, Y., Qin, X., Jia, W., Chai, L., Huang, M., et al. (2019). Microplastics from mulching film is a distinct habitat for bacteria in farmland soil. Sci. Total Environ. 688, 470–478. doi: 10.1016/j.scitotenv.2019.06.108
Zhao, Y., Qiu, Y., Wang, H., Chen, Y., Jin, S., and Chen, S. (2016). Preparation of nanofibers with renewable polymers and their application in wound dressing. Int. J. Polym. Sci. 2016:4672839. doi: 10.1155/2016/4672839
Zheng, Z., Bian, S., Li, Z., Zhang, Z., Liu, Y., Zhai, X., et al. (2020). Catechol modified quaternized chitosan enhanced wet adhesive and antibacterial properties of injectable thermo-sensitive hydrogel for wound healing. Carbohydr. Polym. 249:116826. doi: 10.1016/j.carbpol.2020.116826
Zhong, Q.-Q., Zhao, Y. Q., Shen, L., Hao, B., Xu, X., Gao, B.-Y., et al. (2020). Single and binary competitive adsorption of cobalt and nickel onto novel magnetic composites derived from green macroalgae. Environ. Eng. Sci. 37, 1–13. doi: 10.1089/ees.2019.0305
Zhou, C., Gong, X., Han, J., and Guo, R. (2016). Removal of Pb (II) and Zn (II) from aqueous solutions by raw crab shell: a comparative study. Water Environ. Res. 88, 374–383. doi: 10.2175/106143016X14504669768174
Zhu, J., Ye, H., Deng, D., Li, J., and Wu, Y. (2020). Electrospun metformin-loaded polycaprolactone/chitosan nanofibrous membranes as promoting guided bone regeneration membranes: Preparation and characterization of fibers, drug release, and osteogenic activity in vitro. J. Biomater. Appl. 34, 1282–1293. doi: 10.1177/0885328220901807
Zia, F., Anjum, M. N., Saif, M. J., Jamil, T., Malik, K., Anjum, S., et al. (2017). “Alginate-poly(ethylene) glycol and poly(ethylene) oxide blend materials,” in Algae Based Polymers, Blends, and Composites, eds K. M. Zia, M. Zuber, and M. Ali (Amsterdam: Elsevier), 581–601. doi: 10.1016/B978-0-12-812360-7.00016-1
Zubillaga, V., Alonso-Varona, A., Fernandes, S. C. M., Salaberria, A. M., and Palomares, T. (2020). Adipose-derived mesenchymal stem cell chondrospheroids cultured in hypoxia and a 3D porous chitosan/chitin nanocrystal scaffold as a platform for cartilage tissue engineering. Int. J. Mol. Sci. 21:1004. doi: 10.3390/ijms21031004
Keywords: marine waste, marine industrial by-products, marine biopolymers, marine biomass, waste valorization, circular economy, blue biotechnology, beach wrack
Citation: Rudovica V, Rotter A, Gaudêncio SP, Novoveská L, Akgül F, Akslen-Hoel LK, Alexandrino DAM, Anne O, Arbidans L, Atanassova M, Bełdowska M, Bełdowski J, Bhatnagar A, Bikovens O, Bisters V, Carvalho MF, Catalá TS, Dubnika A, Erdoğan A, Ferrans L, Haznedaroglu BZ, Setyobudi RH, Graca B, Grinfelde I, Hogland W, Ioannou E, Jani Y, Kataržytė M, Kikionis S, Klun K, Kotta J, Kriipsalu M, Labidi J, Lukić Bilela L, Martínez-Sanz M, Oliveira J, Ozola-Davidane R, Pilecka-Ulcugaceva J, Pospiskova K, Rebours C, Roussis V, López-Rubio A, Safarik I, Schmieder F, Stankevica K, Tamm T, Tasdemir D, Torres C, Varese GC, Vincevica-Gaile Z, Zekker I and Burlakovs J (2021) Valorization of Marine Waste: Use of Industrial By-Products and Beach Wrack Towards the Production of High Added-Value Products. Front. Mar. Sci. 8:723333. doi: 10.3389/fmars.2021.723333
Received: 10 June 2021; Accepted: 30 August 2021;
Published: 20 October 2021.
Edited by:
Sachin Kumar, Sardar Swaran Singh National Institute of Renewable Energy, IndiaReviewed by:
Bulgariu Laura, Gheorghe Asachi Technical University of Iaşi, RomaniaDaniela Giordano, Institute of Bioscience and Bioresources, Italian National Research Council, Italy
Copyright © 2021 Rudovica, Rotter, Gaudêncio, Novoveská, Akgül, Akslen-Hoel, Alexandrino, Anne, Arbidans, Atanassova, Bełdowska, Bełdowski, Bhatnagar, Bikovens, Bisters, Carvalho, Catalá, Dubnika, Erdoğan, Ferrans, Haznedaroglu, Setyobudi, Graca, Grinfelde, Hogland, Ioannou, Jani, Kataržytė, Kikionis, Klun, Kotta, Kriipsalu, Labidi, Lukić Bilela, Martínez-Sanz, Oliveira, Ozola-Davidane, Pilecka-Ulcugaceva, Pospiskova, Rebours, Roussis, López-Rubio, Safarik, Schmieder, Stankevica, Tamm, Tasdemir, Torres, Varese, Vincevica-Gaile, Zekker and Burlakovs. This is an open-access article distributed under the terms of the Creative Commons Attribution License (CC BY). The use, distribution or reproduction in other forums is permitted, provided the original author(s) and the copyright owner(s) are credited and that the original publication in this journal is cited, in accordance with accepted academic practice. No use, distribution or reproduction is permitted which does not comply with these terms.
*Correspondence: Ana Rotter, YW5hLnJvdHRlckBuaWIuc2k=
†These authors share first authorship