- School of Marine and Atmospheric Science, Stony Brook University, Southampton, NY, United States
Many shallow coastal systems experience diel fluctuations in dissolved oxygen (DO) and pH that can intensify throughout the summer season and expose estuarine organisms to repeated episodes of coastal hypoxia and acidification. In temperate regions, larval release of the economically important blue crab Callinectes sapidus occurs in the summer, and while the earliest stage (zoea I) larvae are susceptible to persistent low DO and low pH conditions, their sensitivity to diel fluctuations is unknown. Here, a series of short-term (≤96 h) experiments were conducted to investigate the survival of C. sapidus zoea I larvae exposed to a range of diel cycling hypoxic and acidified conditions and durations. Two experiments comparing a diel cycling DO/pH treatment (fluctuating from ∼30% air saturation to ∼103% averaging ∼66%/and from pH ∼7.26 to ∼7.80 averaging ∼7.53) to a static low DO/pH treatment (∼43%/∼7.35), a static moderate DO/pH treatment (∼68%/∼7.59), and a static control treatment (∼106%/∼7.94) indicated that survival in the diel cycling treatment was significantly lower than the moderate treatment (p < 0.05) by 75 and 48% over 96 and 48 h, respectively, despite comparable mean experimental DO/pH values. Three other experiments aimed at identifying the effective minimum duration of low DO/low pH to significantly depress larval survival under diel cycling conditions revealed that 8 h of low DO/low pH (∼28%/∼7.43) over a 24-h diel cycle consistently decreased survival (p < 0.05) relative to control conditions by at least 55% regardless of experimental duration (72-, 48-, and 24-h experiments). An increase in DO beyond saturation to supersaturation (160%) and pH beyond normocapnic to highly basified (8.34) conditions during the day phase of the diel cycle did not improve survival of larvae exposed to nocturnal hypoxia and acidification. Collectively, these experiments demonstrate that diel cycling does not provide newly hatched C. sapidus larvae a temporal refuge capable of ameliorating low DO/pH stress, but rather is more lethal than chronic exposure to comparable average DO/pH conditions. Given that larvae exposed to a single nocturnal episode of moderate hypoxia and acidification experience significantly reduced survival, such occurrences may depress larval recruitment.
Introduction
Covarying, diel fluctuations of dissolved oxygen (DO) and pH commonly occur across shallow estuaries worldwide. Driven by the oscillating dominance of photosynthesis and respiration over a diel cycle, shallow productive coastal systems experience naturally dynamic DO, pH, and CO2 concentrations (Yates et al., 2007; Baumann et al., 2015; Baumann and Smith, 2017). Yet, many of these systems are additionally influenced by land-based practices that amplify the flux of nutrients and organic matter to coastal waters and accelerate metabolic processes (Nixon, 1995; Caffrey, 2004). Cultural eutrophication, or the increasing supply of organic matter to an ecosystem that is caused by anthropogenic influences such as nutrient enrichment (Malone and Newton, 2020), can intensify the amplitude of diel cycling and elicit repeated, severe episodes of nocturnal hypoxia as well as coastal acidification, particularly during warmer months when metabolic rates are maximal (Breitburg, 1990; Beck and Bruland, 2000; Baumann and Smith, 2017). Owing to the shallow nature of these systems, resident biota can be exposed to contemporaneous low DO and low pH conditions regardless of their position in the water column (Burnett, 1997; Tyler et al., 2009).
Biological responses to fluctuating hypoxia and/or acidification vary across estuarine taxa and life stages. Some early life stage fish species that utilize coastal habitat suffer reduced hatching success, growth, and/or survival, or engage in riskier behaviors that leave them more vulnerable to predation under diel cycling conditions relative to static ambient conditions (Davidson et al., 2016; Dixon et al., 2017; Morrell and Gobler, 2020), while others are quite tolerant (Lifavi et al., 2017; Targett et al., 2019) or only experience harmful effects under elevated temperatures (Targett et al., 2019). In some cases, diel cycling provides fish species a physiological refuge from, or reduction in negative behavioral effects compared to, chronically low DO and/or low pH conditions (Jarrold et al., 2017; Jarrold and Munday, 2018a; Cross et al., 2019), a benefit that can be temperature dependent (Laubenstein et al., 2020) or independent (Jarrold and Munday, 2018b). Field studies with oysters revealed significant negative differences in growth and immunocompromisation controlled by diel cycling hypoxia/acidification frequency and magnitude, respectively (Breitburg et al., 2015), and in situ measures of heartbeat rates indicated that the metabolic activity of bay scallops increases and becomes more dynamic under diel cycling hypoxia (Gurr et al., 2018). Lab studies suggest additional, negative outcomes of diel cycling hypoxia and acidification among many mollusks across larval and juvenile life stages with increased infection prevalence by parasitic protozoan Perkinsus spp. (Keppel et al., 2015), and increased percent of time spent closed (Porter and Breitburg, 2016), as well as reductions in growth and survival (Clark and Gobler, 2016; Gobler et al., 2017) relative to ambient conditions. Studies of diel cycling impacts among crustaceans are limited, but some effects can be deduced from short term exposures (<24 h) to hypoxia. Sensitivities to short term exposures vary at the species level among decapod crustaceans, with juveniles of some species exhibiting significant lethargy and mortality over 24-h (Eriksson and Baden, 1997) and 2-h exposures (Gravinese, 2020) to DO levels of ∼25% air saturation.
The Atlantic blue crab, Callinectes sapidus, a species comprising one of the largest fisheries for the eastern United States (National Marine Fisheries Service (NMFS), 2019), is sometimes considered a climate change “winner” under future global change (Glandon et al., 2019). Rising coastal temperatures increase juvenile growth rates in higher latitude temperate systems (Brylawski and Miller, 2006; Glandon and Miller, 2017) and are projected to benefit Chesapeake Bay populations due to reductions in the duration of winter and associated juvenile mortality (Glandon et al., 2019). Juvenile and adult animals both exhibit steady or increased calcification and growth under acidified conditions (Ries et al., 2009; Whiteley, 2011; Glandon and Miller, 2017) and are also considered moderately tolerant of hypoxia (deFur et al., 1990; Burnett, 1997; Mangum, 1997). Recent experiments with larvae, however, reveal sensitivities of early life stages to ocean acidification (Giltz and Taylor, 2017) and coastal hypoxia and acidification both individually and in combination (Tomasetti et al., 2018), further evidencing that crustacean tolerance to low DO- and/or low pH-stress varies throughout ontogeny often in association with changes in respiratory physiology (Whiteley, 2011; Alter et al., 2015; Leiva et al., 2018; Gravinese, 2020).
In temperate systems, the C. sapidus larval release coincides with the time of year when coastal hypoxia/acidification is the most persistent and severe (Dittel R and Epifanio, 1982; McConaugha et al., 1983; Breitburg, 1990; Wallace et al., 2014). Among crustaceans, the pelagic larval life stage is considered the most sensitive to low DO (Miller et al., 2002), particularly for C. sapidus, for which the 50% lethal DO threshold (LC50) is higher than the majority of values published for other larval crustacean species (Miller et al., 2002; Tomasetti et al., 2018). Predictably, low DO effects on C. sapidus are most acute in the larval stage, as crustacean sensitivities to hypoxia depend on their respiratory anatomy and habitat characteristics (Alter et al., 2015). While zoeal development of C. sapidus occurs primarily in oxygenated surface shelf waters (Epifanio and Garvine, 2001), newly hatched first stage (zoea I) larvae can spend their first hours to days in estuarine or nearshore coastal habitat (Tagatz, 1968; Sandifer, 1973; Provenzano et al., 1983; Roman and Boicourt, 1999), where they may be exposed to harmful conditions. Survival of zoea I larvae is reduced significantly under low DO and combined low DO/low pH conditions over static 4-day exposures, with nearly all larvae perishing at DO concentrations ≤ 2.5 mg L–1 or 35% (Tomasetti et al., 2018). However, many shallow eutrophic estuaries across the species’ geographic range commonly experience large fluctuations in DO and pH during summer months (Tyler et al., 2009; Baumann et al., 2015; Baumann and Smith, 2017), and the larval sensitivities to diel cycling low DO and low pH exposures are unknown.
Therefore, this study presents a series of short-term experiments to investigate the survival of C. sapidus zoea I larvae to diel cycling hypoxia and acidification over a range of magnitudes and durations. Specifically, varied quantities of air, CO2 and N2 were delivered either continuously to create distinct static DO and pH conditions or intermittently to create diel cycling DO and pH conditions and test our hypothesis that exposure to diel cycling hypoxia and acidification would negatively impact survival. Additional experiments altering the durations of hypoxia and acidification as well as the amplitudes of the changes in DO and pH under diel cycling were conducted to determine the relative importance of low DO/pH exposure durations and DO/pH fluctuations in driving larval mortality.
Materials and Methods
Animal Collection and Maintenance
The effects of diel cycling hypoxia and acidification were examined through laboratory experiments exposing zoea I larvae to a range of static or cycling DO and pH values. All experiments were completed during June–August of 2019 and 2020 at the Stony Brook Marine Science Center in Southampton, New York. Ovigerous female blue crabs were retrieved from Shinnecock Bay, NY, United States (40.869415, −72.489203) as granted by a Scientific Collectors Permit via the New York State Department of Environmental Conservation. Individual females were placed in separate 20-L polyethylene buckets located in an 1890-L tank with continuous flow of mechanically (foam fractionated) and biologically (via fixed bed aerated biotowers) filtered recirculating UV sterilized sea water. The 20-L buckets were outfitted with openings (∼7 cm in diameter) covered by 70 μm mesh to allow exchange with filtered sea water but retain larvae. Individual buckets were aerated with ambient air via plastic tubing (Tygon) and glass bonded silica air stones until larval hatching. Newly hatched larvae were concentrated in a 2-L polypropylene beaker by siphoning the filtered sea water and larvae out from the lower half of the 20-L polyethylene buckets with plastic tubing through 1,000 μm mesh that trapped egg mass strands and other particulates. Larvae were then distributed among experimental 1-L polypropylene vessels at densities that varied slightly between experiments but never exceeded 115 ind. L–1, as greater densities (>140 ind. L–1) can impair survival (Zmora et al., 2005) and are environmentally unrealistic (McConaugha et al., 1983). Larvae were fed live rotifers (50 ind. ml–1 day–1) enriched with the algae Nannochloropsis originally grown in f/2 media (Sulkin and Epifanio, 1975; Zmora et al., 2005).
Experimental Design
Five separate larval survival experiments were conducted, two comparing static DO/pH treatments to a diel cycling treatment, one comparing different durations of low DO/pH over diel cycles, and two comparing different amplitudes of diel cycling as well as different durations of low DO/pH over diel cycles (Table 1). Experiments varied in length (experimental duration), experiment type, starting larval density, number of females providing larvae, and treatments as described in Table 1. All DO and pH values and diel cycling durations and amplitudes were based on conditions found in US east coast estuaries and for most cases directly measured in two shallow lagoonal estuaries along the coast of NY hosting ovigerous female blue crabs during the summer larval hatching season (Figure 1; Tyler et al., 2009; Baumann et al., 2015; Baumann and Smith, 2017). Briefly, multiparametic EXO2 sondes (Yellow Springs Instruments) were deployed during the summer of 2020 at two well-mixed estuaries with robust blue crab fisheries: Georgica Pond and Shinnecock Bay, to measure temperature, salinity, DO and pHNBS (NBS scale) at a frequency of 10 min, in addition to an Ion Sensitive Field Effect Transistor (ISFET)-based solid state pHT (total scale) SeaFet sensor (Satlantic) that was deployed alongside the multiparametric sonde in Shinnecock Bay to provide pHT measurements (Figure 1). In Georgica Pond the sensor array was deployed on a surface buoy platform and all observations were made at a depth of 0.7 m regardless of sea surface height. In Shinnecock Bay the multiparametric sonde and ISFET pH sensor were affixed atop a large cinder block and placed on the sandy sediment (∼0.3 m height above the bottom), but because Shinnecock Bay is a shallow, well-mixed tidal lagoon with an average depth of <2 m, observations were made at a depth of 1.0 ± 0.3 m (mean ± SD) and were representative of the pelagic environment. For all experiments, salinities were 27.6–29.9, and circulating water bath temperatures were maintained between 24 and 26°C (within the range of temperatures observed at Shinnecock Bay) by multiple heating wands (Finnex Aquarium Heaters) and an external water pump (Iwaki MD55RLT), conditions ideal for larval development (Costlow and Bookhout, 1959). When experiments exceeded 48 h, water changes occurred after 2 days, in which larvae were poured carefully onto a 250 μm sieve and transferred into a glass dish of filtered seawater, before being returned to clean experimental vessels with new filtered seawater. Survival was quantified at the end of each experiment and recovered individuals were considered dead if they had undergone decay and/or did not respond with any movement to light from a flashlight (larvae move toward the region of greatest light intensity) after periods of >30 s (Sandoz and Rogers, 1944).
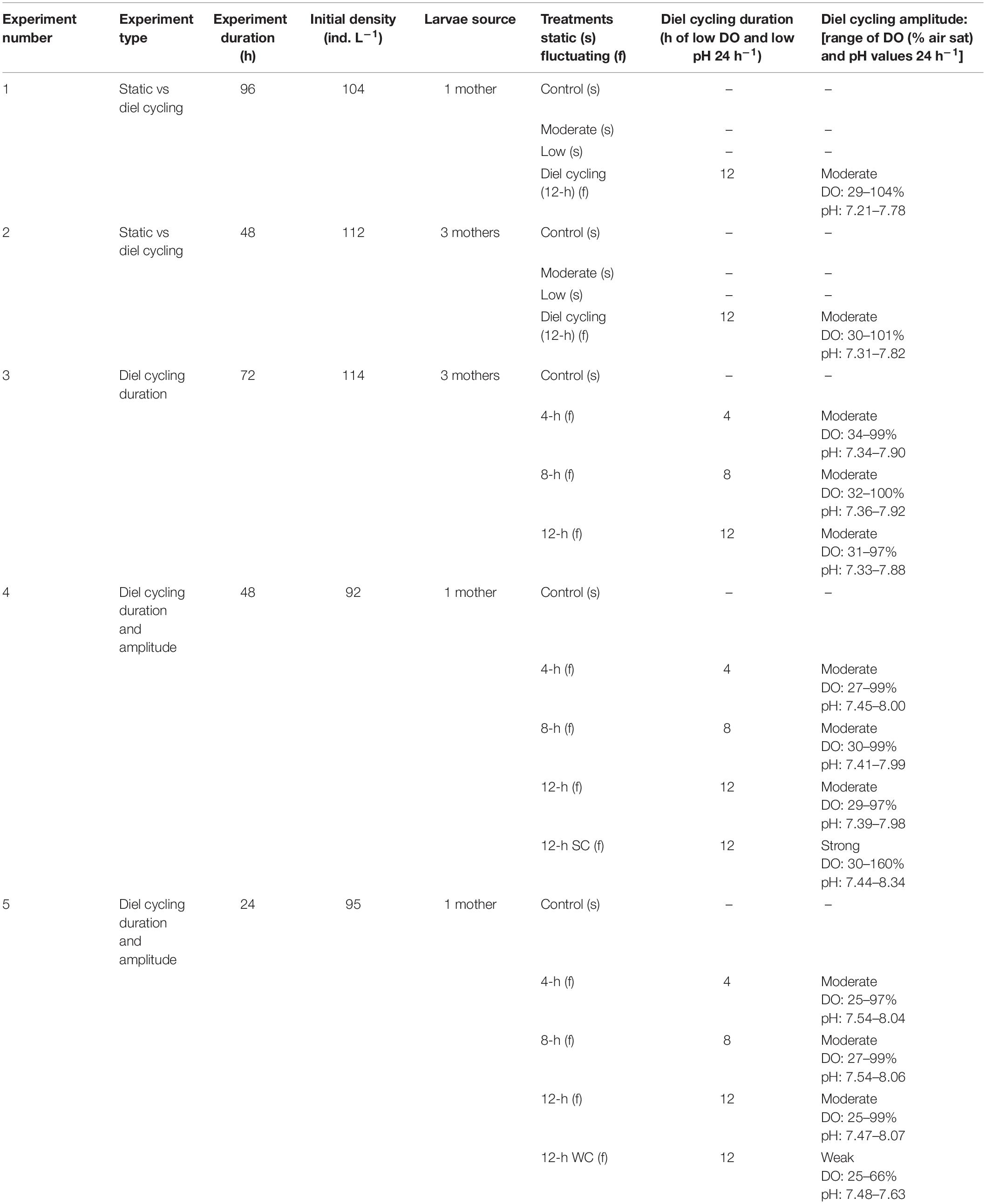
Table 1. Overview of experimental conditions for five experiments investigating the survival of Callinectes sapidus zoea I larvae to diel cycling hypoxia and acidification.
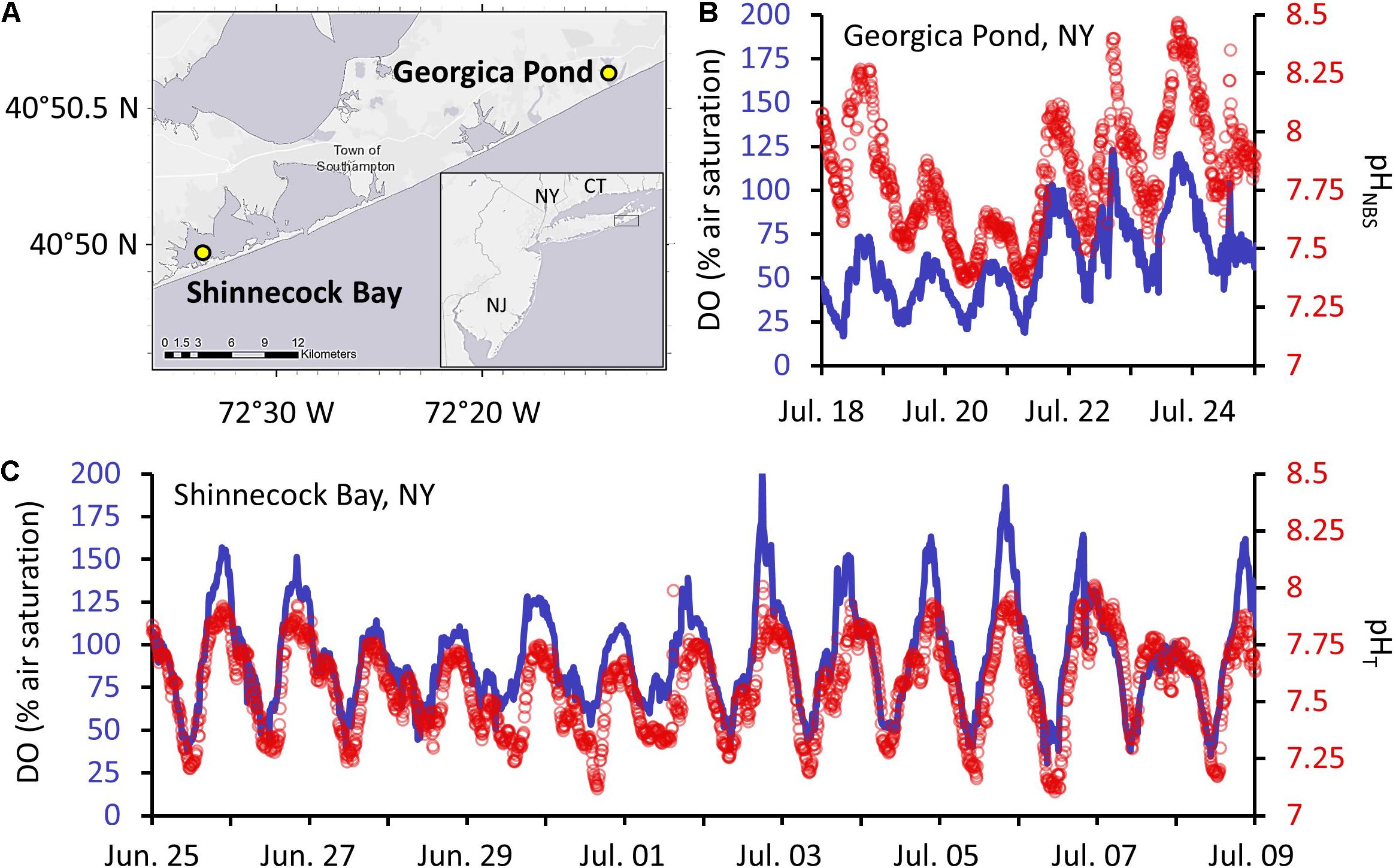
Figure 1. Daily fluctuations of dissolved oxygen (DO; line) and pH (circles) at two sites hosting ovigerous female blue crabs on Long Island, NY. (A) Locations of the two sites; (B) DO and pH fluctuations of Georgica Pond (temperature: 27.7 ± 1.1°C) a temporarily open estuary, and the site of one of the most robust blue crab fisheries in NY; (C) DO and pH fluctuations of Shinnecock Bay (temperature: 25.1 ± 1.2°C), the location where all ovigerous female blue crabs were sourced from for larval experiments.
Static vs Diel Cycling DO/pH Experiments
Two separate experiments comparing static DO/pH levels to diel cycling hypoxia/acidification were conducted that were similar in all aspects of experimental design but differed in experimental duration (experiment 1: 96-h total duration, experiment 2: 48-h total duration; Table 1). For both experiments, sixteen 1-L polyethylene vessels containing 0.2 μm filtered seawater from Shinnecock Bay were placed in a sea table temperature-controlled water bath. Replicate (n = 4) vessels received gas mixtures of ambient air, N2, and CO2 at different rates to create and maintain either normoxic and normocapnic conditions [DO: ∼106% air saturation (∼7.4 mg O2 L–1)/pH: ∼7.94; control treatment], moderately hypoxic and acidified conditions [∼68% (∼4.7 mg O2 L–1)/∼7.59; moderate treatment], hypoxic and acidified conditions [∼43% (∼3.0 mg O2 L–1)/∼7.35; low treatment] and diel cycling conditions (diel cycling treatment) that fluctuated between 12 h of hypoxic/acidified conditions [∼30% (∼2.0 mg O2 L–1)/∼7.26] at night and 12 h of normoxic/normocapnic conditions [∼103% (∼7.2 mg O2 L–1)/∼7.80] during the day, creating average DO and pH values [∼66% (∼4.6 mg O2 L–1)/∼7.53] comparable to the moderate treatment. The target levels in the static low treatment differed from the target low values in the diel cycling treatment because previous experiments established that 100% larval mortality was common at ∼30% (∼2.0 mg O2 L–1) over static 96-h exposures (Tomasetti et al., 2018) an outcome we tried to avoid. To achieve the diel cycling, solenoid valves were attached to a timer (Hunter) programed to regulate the flow of tanked gas mixtures bubbled into the seawater for 12 h at night followed by air for 12 h during the day (Clark and Gobler, 2016; Morrell and Gobler, 2020).
Diel Cycling Duration and Amplitude Experiments
Three additional larval survival experiments compared various durations of low DO/pH within diel cycling conditions (experiment 3: 72-h experiment) or compared both durations of low DO/pH and amplitudes of diel cycling (experiments 4 and 5: 48- and 24-h experiments, respectively; Table 1). For all experiments, replicate (n = 4) vessels were exposed to static normoxic and normocapnic conditions [DO: ∼99% (∼6.9 mg O2 L–1)/pH: ∼7.97; control treatment], or fluctuating low DO/pH exposures [∼28% (∼2.0 mg O2 L–1)/∼7.43] that had durations of low DO/pH lasting for 4 h (4-h treatment), 8 h (8-h treatment), or 12 h (12-h treatment) during the night, before cycling back to the day phase [∼99% (∼6.9 mg O2 L–1)/∼7.97] for the remainder of the daily 24-h cycle. All treatments with fluctuating DO/pH values were established through regulating gas mixtures through solenoid valves controlled by timers (Hunter) that varied in duration (4 vs 8 vs 12 h) based on the treatment.
The final two experiments investigating low DO/pH durations (experiments 4 and 5) also compared differences in amplitudes of diel cycling (Table 1). When exploring different amplitudes of diel cycling, we considered that intense photosynthetic activity in shallow environments can supply additional DO (increasing DO beyond saturated to supersaturated levels) and consume substantial quantities of CO2 (increasing pH beyond normocapnic to basified levels) in the day phase of a diel cycle (Breitburg, 1990; Tyler et al., 2009; Baumann et al., 2015). Alternatively, the day phase of diel cycling may only partially alleviate hypoxic/acidified conditions, meaning DO and pH values do not reach 100% saturation and normocapnia, respectively (Breitburg, 1992). To compare the effects of different amplitudes of diel cycling on larval survival, another treatment (n = 4 replicates) was added to simulate strong (12-h strong cycling amplitude treatment; experiment 4) or weak (12-h weak cycling amplitude treatment; experiment 5) diel cycling amplitudes (Table 1). To establish the 12-h strong cycling amplitude treatment in experiment 4, nitrox gas (65% N2, 35% O2) combined with CO2-stripped air (Keppel et al., 2016; Porter and Breitburg, 2016; Giomi et al., 2019) was introduced in place of ambient air for 12-h durations during the day phase of the 24-h diel cycle to create supersaturated and basified conditions [160% (11.3 mg O2 L–1)/8.34]. The 12-h weak cycling amplitude treatment of experiment 5, rather than receive solely ambient air or nitrox gas during the 12 h day phase, received a different combination of N2, CO2, and air for 12 h, to create DO/pH conditions of 66% (4.7 mg O2 L–1)/7.63. For both experiments, the night phase low DO/pH values remained identical across all diel cycling treatments (Table 1).
Chemistry
Temperature, DO, and pHT measurements were made twice daily for all static and fluctuating (once during the night phase and once during the day phase) treatments using a calibrated optical DO meter (Yellow Springs Instruments, ProODO) and a Honeywell Durafet ISFET-based pHT sensor calibrated with Dr. Andrew Dickson’s (Scripps Institute of Oceanography) seawater pH standard, respectively. To calculate the entire suite of carbonate chemistry parameters for each experiment, seawater samples were collected from replicates across each treatment at the beginning and/or end of the experiment in borosilicate, biological oxygen demand (BOD) bottles, preserved with 1% mercuric chloride, and sealed with a glass stopper using Apiezon L ultra-high vacuum grease and rubber bands, for dissolved inorganic carbon (DIC) analyses (Riebesell et al., 2011). A VINDTA 3D (Versatile Instrument for the Determination of Total Carbon) delivery system extracted DIC from samples which was released as CO2 to a UIC CM5017O coulometric titration unit that measured total DIC. Certified reference materials (CRM) provided by Dr. Andrew Dickson (Scripps Institute of Oceanography) were analyzed before each set of analyses, which only proceeded when recovery of CRM exceeded 99.6%. For each treatment, all carbonate chemistry parameters were calculated via the CO2SYS program1 using measured values of pH, DIC, temperature, salinity, pressure, and H2CO3 dissociation constants (Millero, 2010).
Statistical Analyses
RStudio statistical software (Version 1.3.959) was used for all statistical analyses, and the map was created with ArcMap (10.7.1). Differences in percent survival data were assessed using Analysis of Variance (ANOVA) tests with treatment as the explanatory variable, followed by post hoc Tukey Honest Significant Difference tests. Shapiro-Wilk tests of normality and Bartlett’s tests for homogeneity of variance were conducted to test ANOVA assumptions, and if an assumption was not met survival data was arc-sin square root transformed and retested. All survival values are reported as means ± standard error. Seawater chemistry values are reported as means ± standard deviations, with temperature, DO and pH values derived from twice daily measurements of these parameters in all replicates per treatment, and all other carbonate chemistry parameters derived from pH and DIC measurements from the replicates of each treatment at the start and/or end of experiments. Differences between average DO and pH levels in static and diel cycling treatments were assessed via Kruskal-Wallis ANOVAs followed by post hoc Wilcoxon rank sum tests, correcting for multiple comparisons through the Benjamini-Hochberg procedure.
Results
Static vs Diel Cycling DO/pH Experiments
In the first and second experiments, C. sapidus zoea I larval survival was compared across static control, static moderate, static low, and diel cycling treatments (Figure 2, Table 1, and see Supplementary Table 1 for detailed chemistry). For both experiments average DO levels were significantly different across all static and diel cycling treatments except for between the moderate and diel cycling treatments, as intended (p < 0.05, Wilcoxon test; Figure 2). The average pH in the diel cycling treatment of experiment 1 had a slightly lower average pH value (7.50 ± 0.05) than the moderate treatment (7.56 ± 0.02) but were still comparable given the range of average pH conditions among the other static treatments (7.93 and 7.31), and the average pH in experiment 2 was statistically indistinguishable from the moderate treatment, as intended.
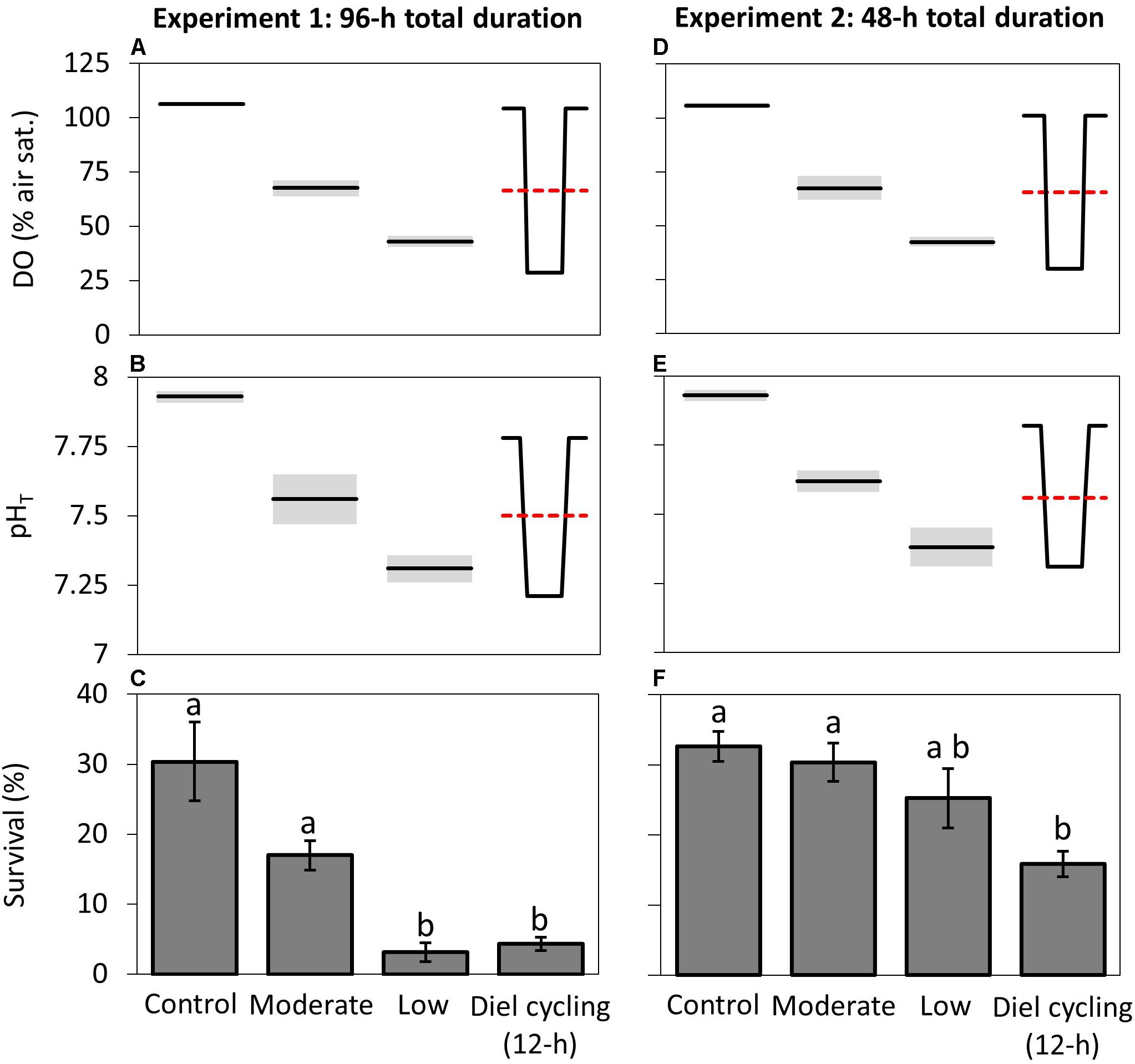
Figure 2. Survival of Callinectes sapidus zoea I larvae under static and diel cycling DO and pH regimes for experiments one (96-h experiment) and two (48-h experiment). DO (A,D) and pH (B,E) values (see Supplementary Table 1 for all chemical values) for experiment one and two, respectively, diagrammed over a 24-h interval from three static treatments and one diel cycling treatment (fluctuating between 12 h of hypoxic/acidified conditions and 12 h of normoxic/normocapnic conditions every 24 h), for which shading represents the S.D. of static treatments and dotted lines represent average values of the diel cycling treatment. Average larval survival for experiment one (C) and two (F), in which bars indicate means (±SE) and letters mark significant differences between treatments.
For experiment 1 (96 h), among the static treatments, survival in the low treatment (3 ± 1%) was significantly lower (p < 0.005, Tukey HSD) than both the moderate (17 ± 2%) and control (30 ± 6%) treatments for which there were no significant differences in survival (Figure 2 and Supplementary Table 2). Exposure to diel cycling did not provide refuge from low DO/pH effects, as larval survival in the diel cycling treatment (4 ± 2%) was significantly lower than the moderate and control treatments (p < 0.05, Tukey HSD), and statistically indistinguishable from the low treatment (Figure 2 and Supplementary Table 2). Given the differences observed in the first experiment and the low survival in the low and diel cycling treatments, the second experiment sought to observe responses to similar chemistry over a shorter time frame, 48 h. Unlike the first experiment, there were no significant differences in larval survival among the static control (33 ± 2%), moderate (30 ± 3%), and low (25 ± 4%) treatments (Figure 2). The diel cycling treatment, however, exhibited significantly reduced survival (16 ± 2%) relative to the control and moderate treatments (p < 0.05, Tukey HSD; Supplementary Table 2), while remaining statistically indistinguishable from the low treatment (Figure 2). This further emphasizes the failure of diel cycling to provide refuge from hypoxic- and acidification-stress to blue crab larvae.
Diel Cycling Duration and Amplitude Experiments
Following the static vs diel cycling experiments presented above, three additional experiments of decreasing experimental duration (experiment 3: 72 h, experiment 4: 48 h, experiment 5: 24 h) were performed to identify the effective minimum duration of low DO/low pH needed to significantly depress larval survival (Table 1). These experiments compared the survival of C. sapidus zoea I larvae in static control conditions to that of diel cycling conditions that varied in the (i) duration of the low DO/pH conditions during the night phase (4 vs 8 vs 12 h), and for experiments four and five, (ii) the amplitude (moderate vs strong vs weak) of the diel cycling (Table 1 and Figure 3). For each experiment, the magnitude of the low DO/pH values were statistically indistinguishable across all diel cycling treatments, as intended (p > 0.05; Kruskal-Wallis ANOVAs).
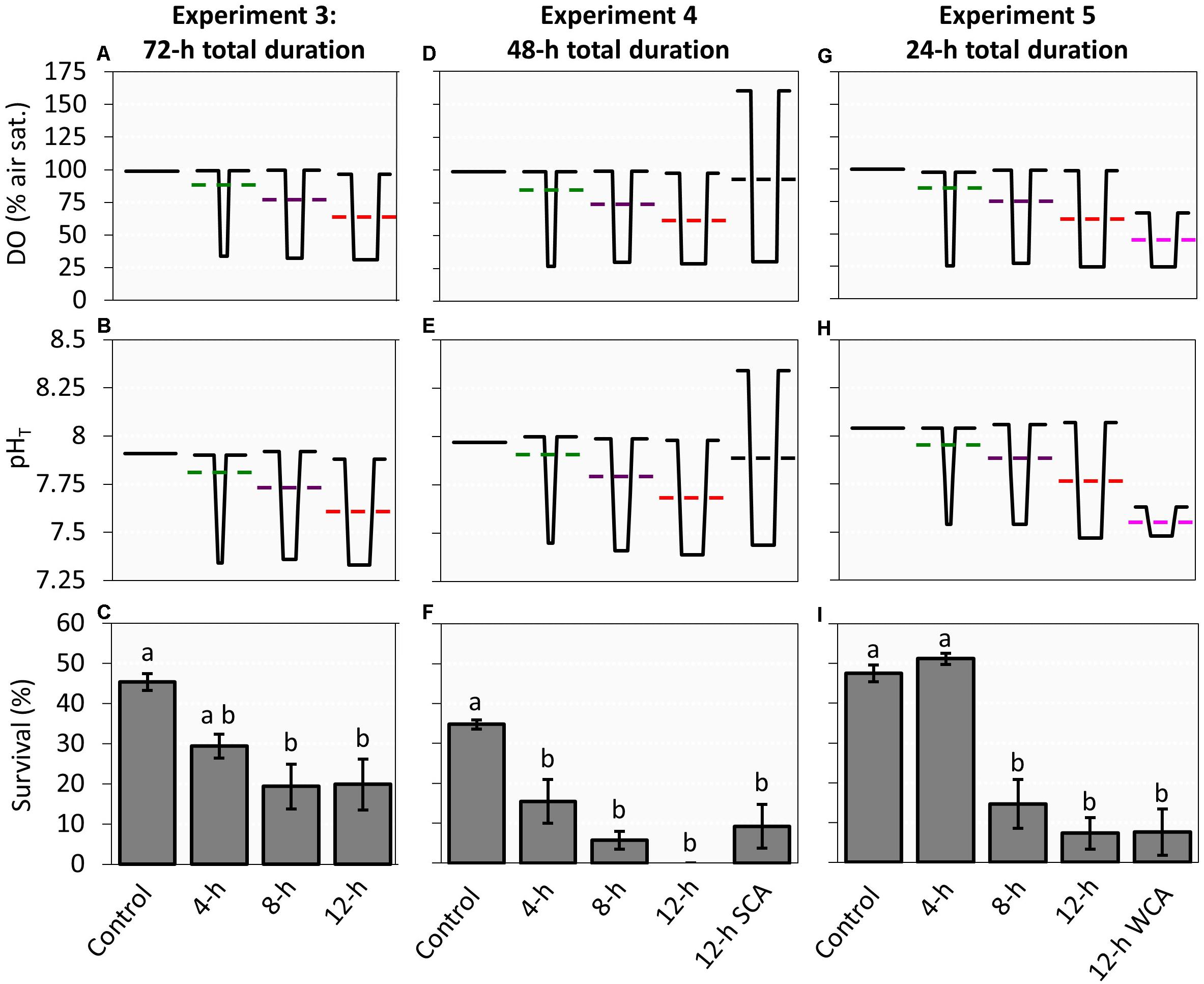
Figure 3. Survival of Callinectes sapidus zoea I larvae under varying durations and amplitudes of low DO/pH diel cycling for experiments three (72-h experiment), four (48-h experiment), and five (24-h experiment). DO (A,D,G) and pH (B,E,H) values, diagrammed over a 24-h interval from the static and cycling treatments, in which dotted lines represent average values of the cycling treatments (see Supplementary Tables 3, 5, 6 for all chemical values and Supplementary Table 7 for daily DO and pH averages of cycling treatments across experiments). Average larval survival (C,F,I), in which bars indicate means (±SE) and letters mark significant differences in survival; Captions SCA and WCA denote strong cycling amplitudes and weak cycling amplitudes, respectively.
In the third experiment (72 h), DO/pH conditions were static for the control treatment and fluctuating between a low DO/pH phase in the night that lasted for 4, 8, or 12 h, and a high DO/pH phase that lasted the remainder of the 24-h cycle (Figure 3 and see Supplementary Table 3 for detailed chemistry). Larval survivorship in the 8-h (19 ± 6%) and 12-h treatments (20 ± 6%), were significantly lower (p < 0.05, Tukey HSD) than the control treatment (45 ± 2%), while the 4-h treatment (29 ± 3%) was not (Figure 3 and Supplementary Table 4). There were no significant differences in larval survival among the cycling treatments.
During the fourth experiment (48 h), a 12-h strong cycling amplitude treatment was added, and the fourth experiment duration was also 24 h shorter (Figure 3, Table 1, and see Supplementary Table 5 for detailed chemistry). Fluctuating DO/pH significantly reduced larval survival across all treatments relative to the control (p < 0.05, Tukey HSD; Supplementary Table 4) with the survival of 4-h (15 ± 6%), 8-h (6 ± 2%), 12-h (0%), and 12-h strong cycling amplitude (9 ± 5%) treatments all <50% of the control (35 ± 1%) treatment (Figure 3). Like the prior experiment, there were no significant differences across cycling treatments (Figure 3). Additionally, the amplitude of the diel cycle did not significantly impact larval survival (Figure 3). Due to the complete mortality across all replicates of the 12-h treatment, the homogeneity of variance ANOVA assumption was not met, however, ANOVA is robust to violations of the equal variance assumption if the sample sizes are equal (Boneau, 1960).
In the final experiment which persisted for only 24 h (experiment 5), a new treatment–the 12-h weak cycling amplitude treatment–replaced the 12-h strong cycling amplitude treatment of the prior experiment (Figure 3, Table 1, and see Supplementary Table 6 for detailed chemistry). Larval survival in the 8-h (15 ± 6%), 12-h (7 ± 4%), and 12-h weak cycling amplitude (8 ± 6%) treatments were significantly lower than the control (48 ± 2%) treatments (p < 0.005, Tukey HSD), but contrary to the previous two experiments, were also significantly lower than the 4-h (51 ± 1%) treatment (p < 0.005, Tukey HSD; Figure 3 and Supplementary Table 4). There were no significant differences between the control and 4-h treatments, and again the amplitude of diel cycling did not impact larval survival (Figure 3).
Discussion
Complex interactions between global and smaller scale biogeochemical processes can create highly dynamic coastal environmental conditions (Tyler et al., 2009; Rabalais et al., 2010; Carstensen and Duarte, 2019; Rheuban et al., 2019). The dynamic range of DO, CO2, and pH in shallow eutrophic estuaries over diel cycles can force organisms to endure environmental extremes for multiple hours each day. This study revealed that brief exposures to coastal hypoxia and acidification under fluctuating DO/pH regimes consistently elicited significant mortality of C. sapidus zoea I larvae. Daily DO and pH values of ∼66% (∼4.6 mg O2 L–1) and ∼7.59, conditions that are commonly observed across eastern US estuaries (Baumann and Smith, 2017), while benign under static exposures, induced precipitous declines in survival under diel cycling exposures, decreasing survival by as much as 75% in the diel cycling treatment compared to the static moderate treatment over a 4-day period (experiment 1). Indeed, significant mortality (>90%) occurred in fluctuating treatments with daily DO and pH averages as high as 95% (6.7 mg O2 L–1) and 7.89, conditions common in most temperate and tropical estuaries. Despite the daily restoration of favorable environmental conditions, diel cycling did not provide larvae a refuge from the physiological stress associated with chronically low DO/pH conditions, even when daytime DO and pH levels were supersaturated and basified, respectively, and even when exposures were as short as one 8-h period.
The physiological capacity to maintain homeostasis in dynamic DO/CO2 environments likely depends on both the adaptive history and life stage of the animal (Waldbusser and Salisbury, 2014; Alter et al., 2015; Leiva et al., 2018). Many early-stage decapod planktonic larvae are unable to regulate their metabolism under declining DO (Belman and Childress, 1973; Spicer, 1995). Their rudimentary scaphognathites (appendages used to draw water toward the gills) and absence of functional gills (Costlow and Bookhout, 1959) suggests that gas exchange occurs across the body’s surface, severely restricting decapod larvae from altering the rate of O2 uptake and CO2 expulsion (Anger, 2001). Eight or twelve consecutive hours of hypoxia [∼28% (∼2.0 mg O2 L–1)] under diel cycling regimes induced significant mortality (>80%) across all experiments, regardless of the experimental duration, including over only 1 day. In another study, megalopae, which exhibit more developed respiratory morphology and a greater ability to oxyregulate, experienced >80% mortality when exposed to eighteen sustained hours of 20% air saturation (∼1.4 mg O2 L–1 at the temperature/salinity of our experiments), suggesting slightly improved tolerances compared to zoea I larvae (Tankersley and Wieber, 2000). Adults, by contrast, can endure over 350 h of hypoxia [34% (2.46 mg O2 L–1)] and experience no significant mortality relative to crabs in normoxic conditions (Brouwer et al., 2005; Brown-Peterson et al., 2005). Curiously, first-instar juveniles (J1s) were shown to deviate from the pattern of increased hypoxia tolerance with age, as J1 individuals were more vulnerable than megalopae (Tankersley and Wieber, 2000), perhaps as a result of the energetic costliness of the molting process associated with transition to the J1 stage.
Several behavioral, morphological, and physiological adaptations have been identified that support the general trend of increased hypoxia tolerance with age in the blue crab. Firstly, juvenile and adult blue crabs can simply relocate away from hypoxic habitat (Pihl et al., 1991). Additionally, the development of functional scaphognathites and structurally complex gills among benthic-stage blue crabs permits more efficient O2 extraction under declining DO conditions through hyperventilation (Batterton and Cameron, 1978; McMahon and Wilkens, 1983). And physiologically, hypoxia can induce increases in O2 affinity among adult blue crabs by increasing the amount of, and stimulating a structural change to, the respiratory protein hemocyanin (deFur et al., 1990; Mangum, 1997). Adaptations that benefit adult blue crabs under hypoxia can also increase tolerance to coastal acidification. Ion exchange across the gills, for example, allows for regulation of HCO3– in the hemolymph (Cameron, 1985) that, in addition to hemocyanin (Whiteley, 2011), buffers hemolymph pH (Melzner et al., 2009). Moreover, for adult blue crabs, the high CO2 concentrations commonly found in hypoxic environments can actually mitigate exercise fatigue (lengthening time until fatigue and reducing the number of turns and stops while walking) under hypoxia (Stover et al., 2013) by increasing hemocyanin oxygen binding affinity (Mangum and Burnett, 1986; Lehtonen and Burnett, 2016), an adaptation that improves blue crab mobility and the ability to ultimately escape low DO/low pH conditions (Lehtonen and Burnett, 2016).
Lacking many of these adaptations at the zoea I stage, C. sapidus larvae exhibited rapid mortality over relatively short (24–96 h) timescales. Consistent across all experiments, significant negative effects were detected in the diel cycling treatments with 8 or 12 h of low DO/pH, whereas effects of 4-h treatments varied depending on the experiment. Eight hours of stress over 1 day induced significantly increased mortality (experiment 5), for instance, yet 4 h of stress each day for three consecutive days did not (experiment 3). Hence, the lethal threshold for daily exposure to comparable levels of low DO/pH is likely somewhere between 4 and 8 h, above which the metabolic strains of the high O2 demand and limited supply overwhelm the larvae. Respiration rates of early stage decapod larvae often decrease substantially under low partial pressures of O2 (Anger, 2001), indicating that the low availability of O2 over 8-h or longer durations can limit energy metabolism and prevent larvae from maintaining energy balance. Yet 4-h exposures can significantly reduce larval survival at times, as demonstrated in experiment 4 (48-h total duration). Interestingly, the average larval survival of the ambient treatment in that experiment was the lowest of the three duration experiments, suggesting that larval health may have already been compromised or that maternal effects may contribute to short-term low DO/pH tolerance. Whenever possible we controlled for maternal effects by stocking single experiments with larvae from multiple mothers, however, when larval release was not coordinated or only one female was available the larvae were still used in experiments (Table 1).
While either nocturnal acidification, hypoxia, or a combination of the two could have contributed to decreased survival of crab larvae, we hypothesize that the low DO was the primary driver of mortality. Although the challenges of acid-base balance under low pH stress may act as a secondary stressor, Tomasetti et al. (2018) determined that significant acidification effects were not detected after 96 h of low pH similar to levels explored here but were detected after 14-day exposures. This was consistent with another study that found significant ocean acidification effects on C. sapidus larvae after periods exceeding 10 days and suggested that the energetic requirements of molting may have contributed to their sensitivity to low pH (Giltz and Taylor, 2017). Our short (≤96 h) experiments ensured that all effects occurred days before the first molt (Costlow and Bookhout, 1959) and were not a result of increased vulnerability or energetic strain associated with the molting process. Nonetheless, our experiments intentionally did not separate the two stressors seeking instead to mimic their covariance, so it is possible that the reported negative effects on larval survival are the result of a combination of low DO and low pH stress, especially since fluctuating co-stressors had not previously been tested.
Although 12-h durations of diel cycling hypoxia/acidification were not directly measured during our environmental monitoring, this severe scenario is not unrealistic for surface waters impacted by cultural eutrophication (Tyler et al., 2009; Wallace et al., 2014). This scenario may not apply to some systems such as some deep stratified systems or shallow oligotrophic systems, where shorter or less intense exposures may be more apt. Yet we note that for some systems, the true impacts of nocturnal hypoxia on C. sapidus larvae at times may be more severe than reported here as our experiments used low DO conditions ∼28% saturation or ∼2.0 mg L–1; shallow eutrophic estuaries can experience nocturnal DO concentrations near or at the surface that are much lower than this including anoxia (see Beck and Bruland, 2000, measured at a depth < 1.3 m; Tyler et al., 2009, measured throughout the entire water column; Wallace, 2020, measured at depths of 0.7–1.3 m at multiple locations). These findings have important implications for C. sapidus populations and fisheries/estuarine management, as even small reductions in larval mortality rates can markedly decrease post-dispersive recruitment (Underwood and Fairweather, 1989). Detection of zoea I larvae in waters with salinities as low as 16 (Sandifer, 1973) and more commonly in polyhaline salinity zones (Sandifer, 1975), as well as detection of zoea II larvae at the mouths of large embayments (Tagatz, 1968; Sandifer, 1973) suggests that larval release can sometimes occur upstream of estuary mouths, committing larvae to extended periods in the estuary. Weekly and/or monthly sampling in Delaware and Chesapeake Bays demonstrated that C. sapidus larval abundances peak in late summer (Sandifer, 1973; Dittel R and Epifanio, 1982; Epifanio et al., 1984) when coastal hypoxia/acidification is often most severe (Breitburg, 1990; Wallace et al., 2014). Moreover, larvae are released at night (Provenzano et al., 1983) or in the early morning (Tankersley et al., 2002), maximizing the likelihood of encountering the low DO/pH conditions associated with the most stressful phase of the diel cycle (Tyler et al., 2009; Baumann et al., 2015). Collectively, these observations suggest that estuarine DO and pH conditions could influence the success of larval recruitment to the juvenile stage.
Decades of larval transport research have established the general pattern of estuarine export and offshore development for C. sapidus larvae in the Mid-Atlantic (Epifanio, 1995, 2019; Epifanio and Garvine, 2001). Very little is known regarding patterns of larval release and transport north of Delaware Bay (e.g., for populations inhabiting NY and CT waters) where the coastal morphology, currents, and circulation patterns differ and where profits from blue crab landings have expanded in recent decades (National Marine Fisheries Service (NMFS), 2019). For example, NY and other northeast United States host a plethora of barrier island estuaries, some of which have ocean inlets that are entirely closed to the Atlantic Ocean during summer months (e.g., Mecox Bay, Sagaponack Pond, Georgica Pond, NY, United States; Gobler et al., 2005). In NY, these lagoonal estuaries host some of the most robust blue crab fisheries of the state. The life cycle of blue crabs in these systems is presently unknown as are the effects of the intense diel cycling hypoxia and acidification in these systems (Figure 1) on resident C. sapidus larvae. Additional environmental monitoring and larval transport research in these regions is needed to clarify the frequency and durations with which larvae of C. sapidus and other species encounter coastal hypoxia/acidification.
The acute sensitivities of C. sapidus larvae to coastal hypoxia revealed in our study are consistent with other meroplanktonic decapod species that inhabit well-oxygenated coastal surface waters for the majority of their larval development (Spicer, 1995; Alter et al., 2015). Specific adaptations such as catadromous migrations of adult females (Tankersley et al., 1998) and post-hatch upward swimming motion of larvae (Sulkin et al., 1980) likely make estuarine retention of C. sapidus over many diel cycles rare as larvae are eventually flushed out of estuaries to the shelf. Indeed, zoea I larvae outnumbered all other stages sampled in Chesapeake Bay, and are commonly found at more offshore stations in both Chesapeake and Delaware Bays (Sandifer, 1973, 1975; Epifanio et al., 1989). These patterns, however, may also reflect the challenges of surviving even short durations of estuarine transport, given the variable DO/pH regimes and high predation rates.
Despite their differences from C. sapidus larvae, estuarine species with fully estuarine larval life history strategies may not necessarily be adapted to low DO/low pH. Rather, they may merely be closer to specific tolerance-limits that will potentially be encountered more frequently or for longer durations under continued climate change (Waldbusser and Salisbury, 2014; Breitburg et al., 2018; Grear et al., 2020). Larval Argopecten irradians, Mercenaria mercenaria, and Crassostrea virginica, for instance, exhibited significant reductions in survival and growth under diel cycling hypoxia and acidification that were no less severe than under chronic stress (Clark and Gobler, 2016). Such larval sensitivities to diel cycling hypoxic/acidified conditions likely contribute to the challenges associated with shellfish restoration in eutrophic environments for which shellfish populations often fail to recover to historical abundances (Jackson et al., 2001; Mann and Powell, 2007; Johnson et al., 2009).
Conclusion
Natural and direct anthropogenic pressures influence the dynamic range of coastal DO and pH conditions and can result in short, repeated exposure to environmental extremes over diel and/or tidal cycles. In coastal environments subject to high dynamicity, the extreme conditions in some cases may be more relevant to the ecology of the system than average conditions. Pooling percent survival data from this study and experimental data from Tomasetti et al. (2018) where larvae were exposed to a range of static low DO levels for periods ≤96 h (by first time point) further evidenced that at comparable average DO values, diel cycling hypoxic conditions often elicited more severe reductions in larval survival than static treatments (Figure 4 and Supplementary Table 8). For instance, static DO levels >50% air saturation never elicited declines in survival >50%, yet under diel cycling hypoxia >50% declines in survival were common at daily average DO levels ranging from 62 to 95%, occurring in 10 of 12 cases with the two exceptions involving 4-h exposures (Figure 4). Short (≤24 h) exposures to low DO have been shown to negatively impact the survival of C. sapidus megalopae and J1 individuals that may experience these conditions when they return and settle in estuarine habitat (Tankersley and Wieber, 2000). Our novel findings suggest that reductions in recruitment may similarly be influenced by coastal hypoxia/acidification encountered at the zoea I stage via a significant increase in mortality rates among larvae exposed briefly (8 h) to low DO/pH conditions during estuarine export. Management plans for coastal fisheries that consider fluctuating environmental conditions may better support their long-term sustainability, particularly for estuarine species, as exposures to low DO/pH over timescales shorter than one tidal period were proven to be lethal here. Advancements in autonomous in situ monitoring technology can now provide more cost-effective long term, high frequency environmental data and can reveal episodes of hypoxia/acidification that manual measurements made during the hours of daylight would miss. Our results highlight the value of integrating coastal DO and carbonate system variability into experimental designs as static scenarios can conceal adverse outcomes. Average environmental conditions do not always provide the most relevant information to assess vulnerability to stressors in fluctuating environments, especially given the high sensitivity and short timescales of biological processes such as early shell formation or larval development. Consequently, regulatory standards that simply rely on average DO and/or pH conditions rather than extremes or durations of extremes may leave aquatic life vulnerable to environmental harm, particularly under future coastal conditions (Tomasetti and Gobler, 2020). To address these uncertainties, additional studies examining the tolerances of coastal biota at early life stages to multiple stressors under various amplitudes and durations of fluctuating conditions are required.
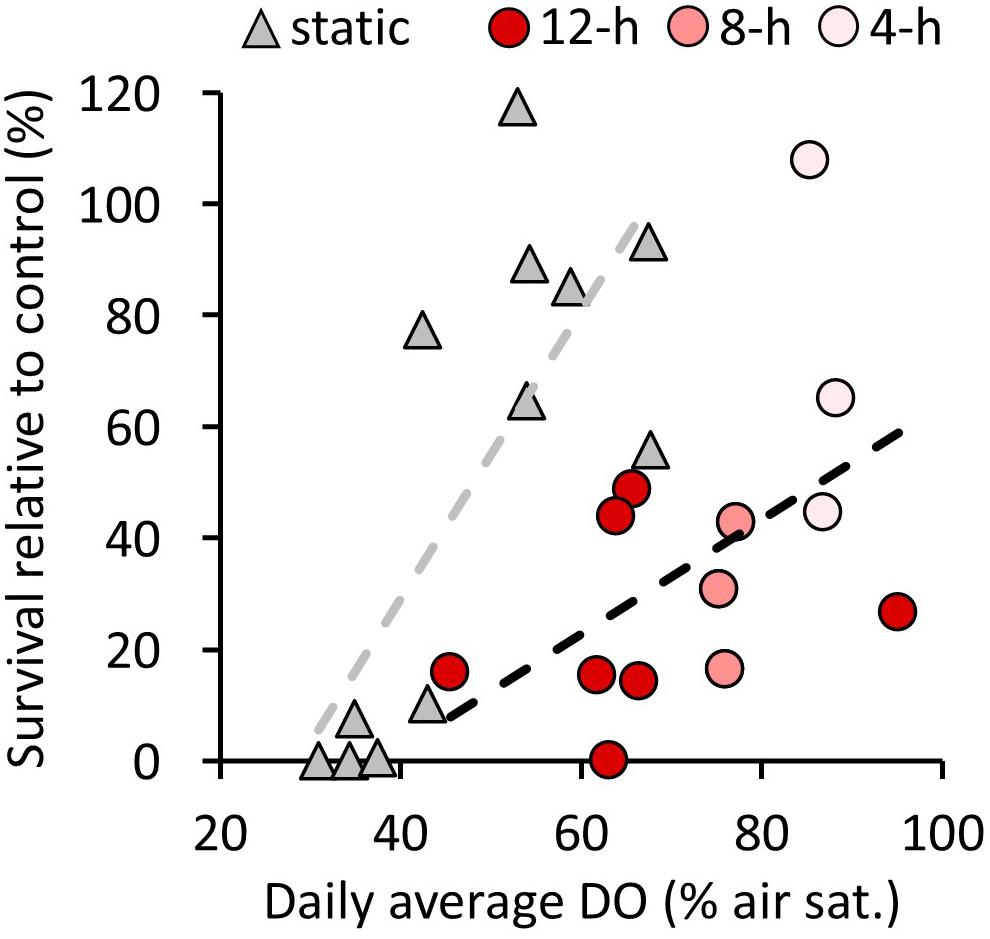
Figure 4. Survival of Callinectes sapidus zoea I larvae (relative to control treatments) as a function of daily average DO over periods ≤ 96 h of static (triangle) or fluctuating (circle) low DO conditions. Each point represents one experimental low DO treatment from the five experiments conducted in this study and four experiments by Tomasetti et al. (2018). The shade of red indicates the duration of hypoxia over a diel cycle within the fluctuating treatments. See Supplementary Table 8 for collated data.
Data Availability Statement
The original contributions presented in the study are included in the article/Supplementary Material, further inquiries can be directed to the corresponding author.
Author Contributions
ST, JK, and CG conceived and designed the experiments, analyzed the data, and revised the manuscript. ST and JK performed the experiments. CG contributed the reagents, materials, and analysis tools. ST and CG wrote the first draft of the manuscript. All authors contributed to the article and approved the submitted version.
Funding
We acknowledge support from the Stony Brook University Science Training and Research to Inform Decisions (STRIDE) Fellowship, the Renaissance Charitable Foundation, the Chicago Community Trust, and the Simons Foundation.
Conflict of Interest
The authors declare that the research was conducted in the absence of any commercial or financial relationships that could be construed as a potential conflict of interest.
Publisher’s Note
All claims expressed in this article are solely those of the authors and do not necessarily represent those of their affiliated organizations, or those of the publisher, the editors and the reviewers. Any product that may be evaluated in this article, or claim that may be made by its manufacturer, is not guaranteed or endorsed by the publisher.
Acknowledgments
We thank the Stony Brook Southampton captains Scott Convery and Brian Gagliardi for facilitating ovigerous blue crab retrievals, marine station manager Chris Paparo for help with female blue crab maintenance, undergraduate student Brendan Hallinan for assistance with experimental measurements, and research technician Andrew Lundstrom for analysis of samples for DIC.
Supplementary Material
The Supplementary Material for this article can be found online at: https://www.frontiersin.org/articles/10.3389/fmars.2021.720175/full#supplementary-material
Footnotes
References
Alter, K., Paschke, K., Gebauer, P., Cumillaf, J.-P., and Pörtner, H.-O. (2015). Differential physiological responses to oxygen availability in early life stages of decapods developing in distinct environments. Mar. Biol. 162, 1111–1124. doi: 10.1007/s00227-018-3406-z
Batterton, C. V., and Cameron, J. N. (1978). Characteristics of resting ventilation and response to hypoxia, hypercapnia, and emersion in the blue crab Callinectes sapidus (Rathbun). J. Exp. Zool. 203, 403–418. doi: 10.1002/jez.1402030308
Baumann, H., and Smith, E. M. (2017). Quantifying metabolically driven pH and Oxygen fluctuations in US nearshore habitats at diel to interannual time scales. Estuar. Coasts 41, 1102–1117. doi: 10.1007/s12237-017-0321-3
Baumann, H., Wallace, R. B., Tagliaferri, T., and Gobler, C. J. (2015). Large natural pH, CO2 and O2 fluctuations in a temperate tidal salt marsh on diel, seasonal, and interannual time scales. Estuar. Coasts 38, 220–231. doi: 10.1007/s12237-014-9800-y
Beck, N. G., and Bruland, K. W. (2000). Diel biogeochemical cycling in a hyperventilating shallow estuarine environment. Estuaries 23, 177–187. doi: 10.2307/1352825
Belman, B. W., and Childress, J. J. (1973). Oxygen consumption of the larvae of the lobster Panulirus interruptus (Randall) and the crab Cancer productus Randall. Comp. Biochem. Physiol. A Comp. Physiol. 44, 821–828. doi: 10.1016/0300-9629(73)90146-1
Boneau, C. A. (1960). The effects of violations of assumptions underlying the t test. Psychol. Bull. 57:49.
Breitburg, D. L. (1990). Near-shore hypoxia in the Chesapeake Bay: patterns and relationships among physical factors. Estuar. Coast. Shelf Sci. 30, 593–609. doi: 10.1016/0272-7714(90)90095-9
Breitburg, D. L. (1992). Episodic hypoxia in Chesapeake Bay: interacting effects of recruitment, behavior, and physical disturbance. Ecol. Monogr. 62, 525–546. doi: 10.2307/2937315
Breitburg, D. L., Hondorp, D., Audemard, C., Carnegie, R. B., Burrell, R. B., Trice, M., et al. (2015). Landscape-level variation in disease susceptibility related to shallow-water hypoxia. PLoS One 10:e0116223. doi: 10.1371/journal.pone.0116223
Breitburg, D., Levin, L. A., Oschlies, A., Grégoire, M., Chavez, F. P., Conley, D. J., et al. (2018). Declining oxygen in the global ocean and coastal waters. Science 359:eaam7240. doi: 10.1126/science.aam7240
Brouwer, M., Brown-Peterson, N. J., Larkin, P., Manning, S., Denslow, N., and Rose, K. (2005). “Molecular and organismal indicators of chronic and intermittent hypoxia in marine crustacea,” in Estuarine Indicators, ed. S. A. Bortone (Boca Raton, FL: CRC Press), 261–276.
Brown-Peterson, N. J., Larkin, P., Denslow, N., King, C., Manning, S., and Brouwer, M. (2005). Molecular indicators of hypoxia in the blue crab Callinectes sapidus. Mar. Ecol. Prog. Ser. 286, 203–215. doi: 10.3354/meps286203
Brylawski, B. J., and Miller, T. J. (2006). Temperature-dependent growth of the blue crab (Callinectes sapidus): a molt process approach. Can. J. Fish. Aquat. Sci. 63, 1298–1308. doi: 10.1139/f06-011
Burnett, L. E. (1997). The challenges of living in hypoxic and hypercapnic aquatic environments. Am. Zool. 37, 633–640.
Caffrey, J. M. (2004). Factors controlling net ecosystem metabolism in U.S. estuaries. Estuaries 27, 90–101. doi: 10.1007/bf02803563
Cameron, J. N. (1985). Compensation of hypercapnic acidosis in the aquatic blue crab, Callinectes Sapidus: the predominance of external sea water over carapace carbonate as the proton sink. J. Exp. Biol. 114, 197–206. doi: 10.1242/jeb.114.1.197
Carstensen, J., and Duarte, C. M. (2019). Drivers of pH variability in coastal ecosystems. Environ. Sci. Technol. 53, 4020–4029. doi: 10.1021/acs.est.8b03655
Clark, H. R., and Gobler, C. J. (2016). Diurnal fluctuations in CO2 and dissolved oxygen concentrations do not provide a refuge from hypoxia and acidification for early-life-stage bivalves. Mar. Ecol. Prog. Ser. 558, 1–14. doi: 10.3389/fmars.2016.00282
Costlow, J. D., and Bookhout, C. G. (1959). The larval development of Callinectes sapidus Rathbun reared in the laboratory. Biol. Bull. 116, 373–396.
Cross, E. L., Murray, C. S., and Baumann, H. (2019). Diel and tidal pCO2 × O2 fluctuations provide physiological refuge to early life stages of a coastal forage fish. Sci. Rep. 9:18146. doi: 10.1038/s41598-019-53930-8
Davidson, M. I., Targett, T. E., and Grecay, P. A. (2016). Evaluating the effects of diel-cycling hypoxia and pH on growth and survival of juvenile summer flounder Paralichthys dentatus. Mar. Ecol. Prog. Ser. 556, 223–235. doi: 10.3354/meps11817
deFur, P. L., Mangum, C. P., and Reese, J. E. (1990). Respiratory responses of the blue crab Callinectes sapidus to long-term hypoxia. Biol. Bull. 178, 46–54.
Dittel R, A. I, and Epifanio, C. E. (1982). Seasonal abundance and vertical distribution of crab larvae in Delaware Bay. Estuaries 5, 197–202. doi: 10.2307/1351835
Dixon, R. L., Grecay, P. A., and Targett, T. E. (2017). Responses of juvenile Atlantic silverside, striped killifish, mummichog, and striped bass to acute hypoxia and acidification: aquatic surface respiration and survival. J. Exp. Mar. Bio. Ecol. 493, 20–30. doi: 10.1016/j.jembe.2017.04.001
Epifanio, C. E. (1995). Transport of blue crab (Callinectes Sapidus) larvae in the waters off mid-Atlantic states. Bull. Mar. Sci. 57, 713–725.
Epifanio, C. E. (2019). Early life history of the blue crab Callinectes sapidus: a review. J. Shellfish Res. 38, 1–22. doi: 10.2983/035.038.0101
Epifanio, C. E., and Garvine, R. W. (2001). Larval transport on the Atlantic continental shelf of North America: a review. Estuar. Coast. Shelf Sci. 52, 51–77. doi: 10.1006/ecss.2000.0727
Epifanio, C. E., Masse, A. K., and Garvine, R. W. (1989). Transport of blue crab larvae by surface currents off Delaware Bay, USA. Mar. Ecol. Prog. Ser. 54, 35–41. doi: 10.3354/meps054035
Epifanio, C. E., Valenti, C. C., and Pembroke, A. E. (1984). Dispersal and recruitment of blue crab larvae in Delaware Bay, USA. Estuar. Coast. Shelf Sci. 18, 1–12.
Eriksson, S. P., and Baden, S. P. (1997). Behaviour and tolerance to hypoxia in juvenile Norway lobster (Nephrops norvegicus) of different ages. Mar. Biol. 128, 49–54. doi: 10.1007/s002270050067
Giltz, S. M., and Taylor, C. M. (2017). Reduced growth and survival in the larval blue crab Callinectes sapidus Under predicted ocean acidification. J. Shellfish Res. 36, 481–485.
Giomi, F., Barausse, A., Duarte, C. M., Booth, J., Agusti, S., Saderne, V., et al. (2019). Oxygen supersaturation protects coastal marine fauna from ocean warming. Sci. Adv. 5:eaax1814. doi: 10.1126/sciadv.aax1814
Glandon, H. L., and Miller, T. J. (2017). No effect of high pCO2 on juvenile blue crab, Callinectes sapidus, growth and consumption despite positive responses to concurrent warming. ICES J. Mar. Sci. 74, 1201–1209. doi: 10.1093/icesjms/fsw171
Glandon, H. L., Kilbourne, K. H., and Miller, T. J. (2019). Winter is (not) coming: warming temperatures will affect the overwinter behavior and survival of blue crab. PLoS One 14:e0219555. doi: 10.1371/journal.pone.0219555
Gobler, C. J., Clark, H. R., Griffith, A. W., and Lusty, M. W. (2017). Diurnal Fluctuations in Acidification and Hypoxia Reduce Growth and Survival of Larval and Juvenile Bay Scallops (Argopecten irradians) and Hard Clams (Mercenaria mercenaria). Front. Mar. Sci. 3:282.
Gobler, C. J., Cullison, L. A., Koch, F., Harder, T. M., and Krause, J. W. (2005). Influence of freshwater flow, ocean exchange, and seasonal cycles on phytoplankton – nutrient dynamics in a temporarily open estuary. Estuar. Coast. Shelf Sci. 65, 275–288. doi: 10.1016/j.ecss.2005.05.016
Gravinese, P. M. (2020). The tolerance of juvenile stone crabs to hypoxia: size matters. J. Exp. Mar. Bio. Ecol. 523:151269. doi: 10.1016/j.jembe.2019.151269
Grear, J. S., O’Leary, C. A., Nye, J. A., Tettelbach, S. T., and Gobler, C. J. (2020). Effects of coastal acidification on North Atlantic bivalves: interpreting laboratory responses in the context of in situ populations. Mar. Ecol. Prog. Ser. 633, 89–104. doi: 10.3354/meps13140
Gurr, S. J., Goleski, J., Lima, F. P., Seabra, R., Gobler, C. J., and Volkenborn, N. (2018). Cardiac responses of the bay scallop Argopecten irradians to diel-cycling hypoxia. J. Exp. Mar. Bio. Ecol. 500, 18–29. doi: 10.1016/j.jembe.2017.12.011
Jackson, J. B. C., Kirby, M. X., Berger, W. H., Bjorndal, K. A., Botsford, L. W., Bourque, B. J., et al. (2001). Historical overfishing and the recent collapse of coastal ecosystems. Science 293, 629–637. doi: 10.1126/science.1059199
Jarrold, M. D., and Munday, P. L. (2018a). Diel CO2 cycles do not modify juvenile growth, survival and otolith development in two coral reef fish under ocean acidification. Mar. Biol. 165:49. doi: 10.1007/s00227-018-3311-5
Jarrold, M. D., and Munday, P. L. (2018b). Elevated temperature does not substantially modify the interactive effects between elevated CO2 and diel CO2 cycles on the survival, growth and behavior of a coral reef fish. Front. Mar. Sci. 5:458. doi: 10.3389/fmars.2018.00458
Jarrold, M. D., Humphrey, C., McCormick, M. I., and Munday, P. L. (2017). Diel CO2 cycles reduce severity of behavioural abnormalities in coral reef fish under ocean acidification. Sci. Rep. 7:10153. doi: 10.1038/s41598-017-10378-y
Johnson, M. W., Powers, S. P., Senne, J., and Park, K. (2009). Assessing in situ tolerances of eastern oysters (Crassostrea virginica) under moderate hypoxic regimes: implications for restoration. J. Shellfish Res. 28, 185–192. doi: 10.2983/035.028.0202
Keppel, A. G., Breitburg, D. L., and Burrell, R. B. (2016). Effects of co-varying diel-cycling hypoxia and pH on growth in the juvenile eastern oyster, Crassostrea virginica. PLoS One 11:e0161088. doi: 10.1371/journal.pone.0161088
Keppel, A. G., Breitburg, D. L., Wikfors, G. H., Burrell, R. B., and Clark, V. M. (2015). Effects of co-varying diel-cycling hypoxia and pH on disease susceptibility in the eastern oyster Crassostrea virginica. Mar. Ecol. Prog. Ser. 538, 169–183. doi: 10.3354/meps11479
Laubenstein, T. D., Jarrold, M. D., Rummer, J. L., and Munday, P. L. (2020). Beneficial effects of diel CO2 cycles on reef fish metabolic performance are diminished under elevated temperature. Sci. Total Environ. 735:139084. doi: 10.1016/j.scitotenv.2020.139084
Lehtonen, M. P., and Burnett, L. E. (2016). Effects of hypoxia and hypercapnic hypoxia on oxygen transport and acid–base status in the atlantic blue crab, Callinectes sapidus, during exercise. J. Exp. Zool. 325, 598–609. doi: 10.1002/jez.2054
Leiva, F. P., Garcés, C., Verberk, W. C. E. P., Care, M., Paschke, K., and Gebauer, P. (2018). Differences in the respiratory response to temperature and hypoxia across four life-stages of the intertidal porcelain crab Petrolisthes laevigatus. Mar. Biol. 165:146.
Lifavi, D. M., Targett, T. E., and Grecay, P. A. (2017). Effects of diel-cycling hypoxia and acidification on juvenile weakfish Cynoscion regalis growth, survival, and activity. Mar. Ecol. Prog. Ser. 564, 163–174. doi: 10.3354/meps11966
Malone, T. C., and Newton, A. (2020). The globalization of cultural eutrophication in the coastal ocean: causes and consequences. Front. Mar. Sci. 7:670. doi: 10.3389/fmars.2020.00670
Mangum, C. P. (1997). Adaptation of the oxygen transport system to hypoxia in the blue crab Callinectes sapidus. Am. Zool. 37, 604–611.
Mangum, C. P., and Burnett, L. E. (1986). The CO2 sensitivity of the hemocyanins and its relationship to Cl– sensitivity. Biol. Bull. 171, 248–263. doi: 10.2307/1541921
Mann, R., and Powell, E. N. (2007). Why oyster restoration goals in the Chesapeake Bay are not and probably cannot be achieved. J. Shellfish Res. 26, 905–917.
McConaugha, J. R., Johnson, D. F., Provenzano, A. J., and Maris, R. C. (1983). Seasonal distribution of larvae of Callinectes sapidus (Crustacea: Decapoda) in the waters adjacent to Chesapeake bay. J. Crustacean Biol. 3, 582–591. doi: 10.2307/1547953
McMahon, B. R., and Wilkens, J. L. (1983). “Ventilation, perfusion and oxygen uptake,” in Internal Anatomy And Physiological Regulation, ed. L. H. Mantel (New York, NY: Academic Press), 289–372.
Melzner, F., Gutowska, M. A., Langenbuch, M., Dupont, S., Lucassen, M., Thorndyke, M. C., et al. (2009). Physiological basis for high CO2 tolerance in marine ectothermic animals: pre-adaptation through lifestyle and ontogeny? Biogeosciences 6, 2313–2331.
Miller, D., Poucher, S., and Coiro, L. (2002). Determination of lethal dissolved oxygen levels for selected marine and estuarine fishes, crustaceans, and a bivalve. Mar. Biol. 140, 287–296.
Millero, F. J. (2010). Carbonate constants for estuarine waters. Mar. Freshwater Res. 61, 139–142. doi: 10.1071/MF09254
Morrell, B. K., and Gobler, C. J. (2020). Negative effects of diurnal changes in acidification and hypoxia on early-life stage estuarine fishes. Diversity 12:25. doi: 10.3390/d12010025
National Marine Fisheries Service (NMFS) (2019). Personal Communication. National Marine Fisheries Service, Fisheries Statistics Division. Silver Spring, MY: National Marine Fisheries Service (NMFS).
Nixon, S. W. (1995). Coastal marine eutrophication: a definition, social causes, and future concerns. Ophelia 41, 199–219.
Pihl, L., Baden, S. P., and Diaz, R. J. (1991). Effects of periodic hypoxia on distribution of demersal fish and crustaceans. Mar. Biol. 108, 349–360. doi: 10.1007/BF01313644
Porter, E. T., and Breitburg, D. L. (2016). Eastern oyster, Crassostrea virginica, valve gape behavior under diel-cycling hypoxia. Mar. Biol. 163:218. doi: 10.1007/s00227-016-2980-1
Provenzano, A. J., McConaugha, J. R., Philips, K. B., Johnson, D. F., and Clark, J. (1983). Vertical distribution of first stage larvae of the blue crab, Callinectes sapidus, at the mouth of Chesapeake Bay. Estuar. Coast. Shelf Sci. 16, 489–499. doi: 10.1016/0272-7714(83)90081-1
Rabalais, N. N., Diaz, R. J., Levin, L. A., Turner, R. E., Gilbert, D., and Zhang, J. (2010). Dynamics and distribution of natural and human-caused hypoxia. Biogeosciences 7, 585–619. doi: 10.5194/bg-7-585-2010
Rheuban, J. E., Doney, S. C., McCorkle, D. C., and Jakuba, R. W. (2019). Quantifying the effects of nutrient enrichment and freshwater mixing on coastal ocean acidification. J. Geophys. Res. C Oceans 124, 9085–9100. doi: 10.1029/2019JC015556
Riebesell, U., Fabry, V. J., Hansson, L., and Gattuso, J.-P. (2011). Guide To Best Practices For Ocean Acidification Research And Data Reporting. Luxembourg: Office for Official Publications of the European Communities. Reprint.
Ries, J. B., Cohen, A. L., and McCorkle, D. C. (2009). Marine calcifiers exhibit mixed responses to CO2-induced ocean acidification. Geology 37, 1131–1134. doi: 10.1130/G30210A.1
Roman, M. R., and Boicourt, W. C. (1999). Dispersion and recruitment of crab larvae in the Chesapeake Bay plume: physical and biological controls. Estuaries 22, 563–574. doi: 10.2307/1353044
Sandifer, P. A. (1973). Distribution and abundance of decapod crustacean larvae in the York River estuary and adjacent lower Cheaspeake Bay, Virginia, 1968–1969. Chesapeake Science 14, 235–257.
Sandifer, P. A. (1975). The role of pelagic larvae in recruitment to populations of adult decapod crustaceans in the York River estuary and adjacent lower Chesapeake Bay, Virginia. Estuar. Coast. Mar. Sci. 3, 269–279. doi: 10.1016/0302-3524(75)90028-6
Sandoz, M., and Rogers, R. (1944). The effect of environmental factors on hatching, moulting, and survival of zoea larvae of the blue crab Callinectes sapidus Rathbun. Ecology 25, 216–228.
Spicer, J. I. (1995). Ontogeny of respiratory function in crustaceans exhibiting either direct or indirect development. J. Exp. Zool. 272, 413–418. doi: 10.1002/jez.1402720602
Stover, K. K., Burnett, K. G., McElroy, E. J., and Burnett, L. E. (2013). Locomotory fatigue during moderate and severe hypoxia and hypercapnia in the Atlantic blue crab, Callinectes sapidus. Biol. Bull. 224, 68–78. doi: 10.1086/BBLv224n2p68
Sulkin, S. D., and Epifanio, C. E. (1975). Comparison of rotifers and other diets for rearing early larvae of the blue crab, Callinectes sapidus Rathbun. Estuar. Coast. Mar. Sci. 3, 109–113. doi: 10.1016/0302-3524(75)90011-0
Sulkin, S. D., Van Heukelem, W., Kelly, P., and Van Heukelem, L. (1980). The behavioral basis of larval recruitment in the crab Callinectes sapidus Rathbun: a laboratory investigation of ontogenetic changes in geotaxis and barokinesis. Biol. Bull. 159, 402–417.
Tagatz, M. E. (1968). Biology of the Blue Crab, Callinectes Sapidus Rathbun in the St. Johns River, Florida. U. S. Fish Wildl. Serv. Fish. Bull. 67, 17–33.
Tankersley, R. A., and Wieber, M. G. (2000). Physiological responses of postlarval and juvenile blue crabs Callinectes sapidus to hypoxia and anoxia. Mar. Ecol. Prog. Ser. 194, 179–191. doi: 10.3354/meps194179
Tankersley, R. A., Bullock, T. M., Forward, R. B., and Rittschof, D. (2002). Larval release behaviors in the blue crab Callinectes sapidus: role of chemical cues. J. Exp. Mar. Bio. Ecol. 273, 1–14. doi: 10.1016/S0022-0981(02)00135-1
Tankersley, R. A., Wieber, M. G., Sigala, M. A., and Kachurak, K. A. (1998). Migratory behavior of ovigerous blue crabs Callinectes sapidus: evidence for selective tidal-stream transport. Biol. Bull. 195, 168–173. doi: 10.2307/1542824
Targett, T. E., Grecay, P. A., and Dixon, R. L. (2019). Growth of the estuarine fish Fundulus heteroclitus in response to diel-cycling hypoxia and acidification: interaction with temperature. Can. J. Fish. Aquat. Sci. 76, 1295–1304. doi: 10.1139/cjfas-2018-0216
Tomasetti, S. J., and Gobler, C. J. (2020). Dissolved oxygen and pH criteria leave fisheries at risk. Science 368, 372–373. doi: 10.1126/science.aba4896
Tomasetti, S. J., Morrell, B. K., Merlo, L. R., and Gobler, C. J. (2018). Individual and combined effects of low dissolved oxygen and low pH on survival of early stage larval blue crabs Callinectes sapidus. PLoS One 13:e0208629. doi: 10.1371/journal.pone.0208629
Tyler, R. M., Brady, D. C., and Targett, T. E. (2009). Temporal and spatial dynamics of diel-cycling hypoxia in estuarine tributaries. Estuar. Coasts 32, 123–145. doi: 10.1007/s12237-008-9108-x
Underwood, A. J., and Fairweather, P. G. (1989). Supply-side ecology and benthic marine assemblages. Trends Ecol. Evol. 4, 16–20.
Waldbusser, G. G., and Salisbury, J. E. (2014). Ocean acidification in the coastal zone from an organism’s perspective: multiple system parameters, frequency domains, and habitats. Ann. Rev. Mar. Sci. 6, 221–247. doi: 10.1146/annurev-marine-121211-172238
Wallace, R. B. (2020). Coastal Ocean Acidification: Dynamics And Potential To Affect Marine Mollusks. Stony Brook: State University of New York. Ph.D. thesis.
Wallace, R. B., Baumann, H., Grear, J. S., Aller, R. C., and Gobler, C. J. (2014). Coastal ocean acidification: the other eutrophication problem. Estuar. Coast. Shelf Sci. 148, 1–13. doi: 10.1016/j.ecss.2014.05.027
Whiteley, N. M. (2011). Physiological and ecological responses of crustaceans to ocean acidification. Mar. Ecol. Prog. Ser. 430, 257–272.
Yates, K. K., Dufore, C., Smiley, N., Jackson, C., and Halley, R. B. (2007). Diurnal variation of oxygen and carbonate system parameters in Tampa Bay and Florida Bay. Mar. Chem. 104, 110–124. doi: 10.1016/j.marchem.2006.12.008
Keywords: hypoxia, coastal acidification, diel cycling, coastal change, larval release, blue crab, Callinectes sapidus
Citation: Tomasetti SJ, Kraemer JR Jr and Gobler CJ (2021) Brief Episodes of Nocturnal Hypoxia and Acidification Reduce Survival of Economically Important Blue Crab (Callinectes sapidus) Larvae. Front. Mar. Sci. 8:720175. doi: 10.3389/fmars.2021.720175
Received: 03 June 2021; Accepted: 02 August 2021;
Published: 20 August 2021.
Edited by:
Rachel Collin, Smithsonian Tropical Research Institute (SI), United StatesReviewed by:
Noelle Marie Lucey, Smithsonian Tropical Research Institute, PanamaLouis Burnett, College of Charleston, United States
Copyright © 2021 Tomasetti, Kraemer and Gobler. This is an open-access article distributed under the terms of the Creative Commons Attribution License (CC BY). The use, distribution or reproduction in other forums is permitted, provided the original author(s) and the copyright owner(s) are credited and that the original publication in this journal is cited, in accordance with accepted academic practice. No use, distribution or reproduction is permitted which does not comply with these terms.
*Correspondence: Christopher J. Gobler, Y2hyaXN0b3BoZXIuZ29ibGVyQHN0b255YnJvb2suZWR1