- 1Marine Data Technology Hub, College of Science and Engineering, James Cook University, Townsville, QLD, Australia
- 2Coastal and Marine Research Centre, Australian Rivers Institute, School of Environment and Science, Griffith University, Gold Coast, QLD, Australia
- 3Southern Seas Ecology Laboratories, School of Biological Sciences, The Environment Institute, The University of Adelaide, Adelaide, SA, Australia
- 4Department of Environment and Science, Brisbane, QLD, Australia
Despite genuine attempts, the history of marine and coastal ecosystem management is littered with examples of poor environmental, social and financial outcomes. Marine ecosystems are largely populated by species with open populations, and feature ecological processes that are driven by multiple, interwoven, dynamic causes and effects. This complexity limits the acquisition of relevant knowledge of habitat characteristics, species utilisation and ecosystem dynamics. The consequence of this lack of knowledge is uncertainty about the link between action taken and outcome achieved. Such uncertainty risks misdirected human and financial investment, and sometimes may even lead to perverse outcomes. Technological advances offer new data acquisition opportunities, but the diversity and complexity of the biological and ecological information needed to reduce uncertainty means the increase in knowledge will be slow unless it is undertaken in a structured and focussed way. We introduce “Ecological Constraint Mapping” – an approach that takes a “supply chain” point of view and focusses on identifying the principal factors that constrain life-history outcomes (success/productivity/resilience/fitness) for marine and coastal species, and ultimately the quality and resilience of the ecosystems they are components of, and the life-history supporting processes and values ecosystems provide. By providing a framework for the efficient development of actionable knowledge, Ecological Constraint Mapping can facilitate a move from paradigm-based to knowledge-informed decision-making on ecological issues. It is suitable for developing optimal solutions to a wide range of conservation and management problems, providing an organised framework that aligns with current perspectives on the complex nature of marine and coastal systems.
Introduction
Marine and coastal ecosystems are experiencing unprecedented pressures from human impacts (Carpenter et al., 2009; Waltham et al., 2020). Direct impacts from urban and industrial development, agriculture, fisheries and aquaculture are widespread, and impacts of climate change are emerging (Halpern et al., 2012). Unfortunately, the history of the management of activities affecting marine and coastal ecosystems and their component biota is littered with examples of poor environmental, social and financial outcomes. Despite genuine attempts at management, the direct and indirect effects of coastal development frequently result in unacceptable ecosystem outcomes (Peterson and Bishop, 2005), many fisheries restocking programs lack demonstrable success (Bell et al., 2006), habitat restoration often fails to achieve meaningful outcomes (Gilby et al., 2018; Sheaves et al., 2021), remediation actions result in unanticipated negative consequences (Hilderbrand et al., 2005), and there are significant difficulties in the identification of appropriate locations for offsets and in determining if offset programmes deliver real benefits (Vaissière et al., 2014). Although the blame for unrealised outcomes is often attributed to particular user groups (e.g., fishers or urban developers), the situation is rarely straightforward (Hawkins, 2011). In fact, the pervasiveness of these failings points to a significant overarching issue - that of unforeseen consequences – an issue often stemming from reliance on inappropriate knowledge (Sheaves, 2017; Sheaves et al., 2020a), a lack of whole-of-system understanding, or a paucity of appropriate knowledge of causal processes at appropriate spatial and temporal scales (Grubbs et al., 2016). This is exacerbated by the lack of a framework for conceptualising such knowledge and including it in environmental decision-making.
The knowledge necessary to maintain healthy, resilie nt marine and coastal ecosystems, and the biotic communities they comprise, is multifaceted (Ascough Ii et al., 2008), and includes information on exploitation rates, patterns of environmental change, habitat utilisation and so on. Even in the case of apparently simple parameters, such as animal-habitat relationships, the knowledge required for effective decision-making extends well beyond basic understanding of habitat occupancy. Most species require multiple habitats to fulfill their life-supporting requirements (e.g., food, refuge, breeding) at each life-history stage, so information is needed on how extensively each habitat contributes to the success of the various life-history stages (Kimirei et al., 2015), the nature and extent of connectivities among habitats (Van Lier et al., 2018), and the environmental requirements that enable or prevent habitat occupancy (Mattone and Sheaves, 2017; Dubuc et al., 2018). Such knowledge is fundamental to effective decision-making in fields as diverse as ecosystem-based fisheries management (Hilborn, 2011; Hill et al., 2020), coastal climate change adaptation (Sheaves et al., 2016) and the management of coastal ecosystems (DEHP, 2012). For instance, the inclusion of knowledge of the extent, composition, condition and utilisation of habitats by different life-history stages, and the connectivities among those habitat units, provides the key underpinning of modern wetland management (e.g., DEHP, 2012, 2017) such as the “Wetlands in the Great Barrier Reef Catchment: Management Strategy 2016–’21” (Figure 1, left panel). Unfortunately, detailed information on habitats, their values and connections between them is only available for a small proportion of species of marine fish, reptile and mammal species (Saenger et al., 2013; Newman et al., 2016). Indeed, even in a system of global importance like the Great Barrier Reef Catchment, details of habitat distributions and compositions are still incomplete (DEHP, 2017; Tarte and Yorkston, 2020).
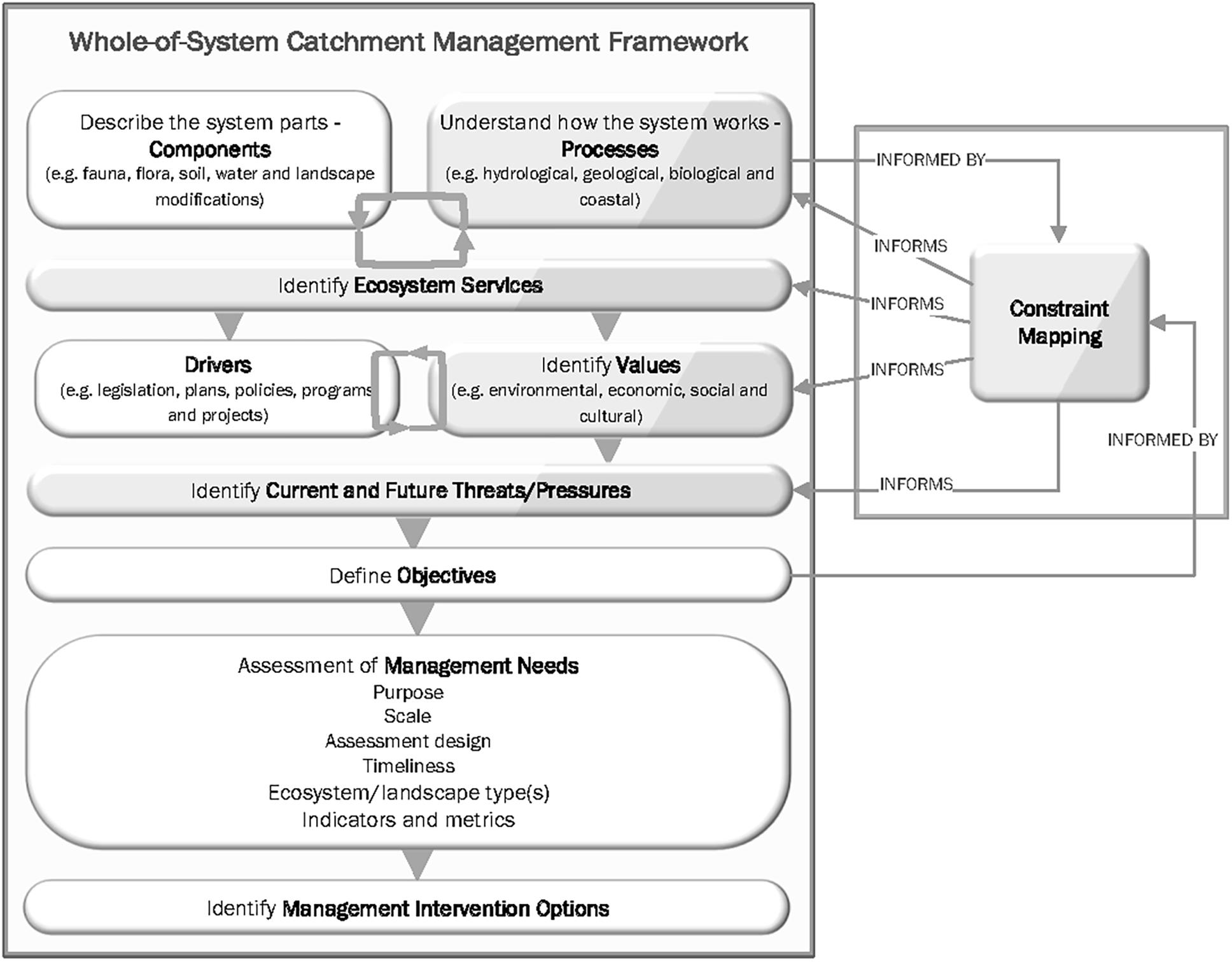
Figure 1. The relationship between Ecological Constraint Mapping and a whole-of-system catchment management framework. Left-hand side: Wetlands in the Great Barrier Reef Catchment: Management Strategy 2016–21, (modified from QldDEHP, 2016): Right-hand side: relationships to Constraint Mapping.
The nature of marine and coastal systems places limits on the acquisition of relevant knowledge of habitat extent, composition and utilisation by animals. Populations are typically open (Bonhomme and Planes, 2000), and species utilise various habitats in different circumstances, such as at different depths and in different conditions of water clarity, current velocity, tidal range, salinity or dissolved oxygen. Additionally, ecosystems provide value to species in diverse ways (Barbier, 2017). Outcomes are driven by the interaction of multiple and dynamic causes and effects (Sheaves, 2016; Griffiths et al., 2019), organisms use their environment in complex ways (Nagelkerken et al., 2015; Lefcheck et al., 2019), and short- and medium-term species migrations and variations in environmental conditions, complicate the linking of faunal outcomes to habitats (Abrantes et al., 2019; Sievers et al., 2020). The lack of requisite knowledge constrains the development of useful criteria against which the success of interventions (such as management actions) can be judged (Loneragan et al., 2013), to objectively assess the need for intervention (Bell et al., 2006) or to determine the type of intervention that is needed (Bell et al., 2006; Loneragan et al., 2013). The consequences of this uncertainty about the link between action and outcome go beyond the failure to achieve useful goals, to the risk of misdirected investment and wasted resources (Bartley and Bell, 2008), and, perhaps more importantly, to ineffective decision-making (Figure 1).
There is an obvious need for fit-for-purpose data, but the amount and diversity of the data needed means that obtaining the requisite information, and converting it to actionable knowledge, is not simple. Indeed the scale of the problem is substantial because, even for important fisheries species, cross-life-cycle habitat utilisation, the spatial identification of these areas, and among-habitat connectivity, are poorly understood (Saenger et al., 2013; Brown et al., 2019), meaning that the specific values provided by habitats and connections among habitats are often unknown (Bradley et al., 2017). This knowledge deficit is difficult to overcome in marine and coastal environments owing to costly, remote and challenging research conditions, to the sheer extent of habitats requiring assessment, and because of the lack of underpinning information, such as the extent and composition of Habitat Features (see Box 1 for definitions of terms). The increasing availability of advanced technology, such as underwater video and sensor networks, offers new data acquisition opportunities (Christin et al., 2018; Konovalov et al., 2019; Brett et al., 2020; Sheaves et al., 2020b). However, the scale of the current knowledge deficit means that data capture will be slow unless it is undertaken in a structured way; with specific conceptualisation of what is to be achieved, of the causal links between data requirements, interventions and outcomes, and with the development of impactful output within a coherent knowledge synthesis framework. One logical approach is to take a “supply chain” point of view and identify the key bottlenecks in habitat and environmental requirements that constrain life-history outcomes. Knowledge of these constraints allows efficient, impact-relevant targeting of actions that provide the greatest likelihood of high-quality management and conservation outcomes.
BOX 1. Definition of terms.
Ecological Constraint Mapping: An approach that focusses on identifying the factors that constrain life-history outcomes (success/productivity/resilience/fitness) of species or faunal groups. (abridged to “Constraint Mapping” for brevity).
Constraint Network: A consolidation of information in the suite of Constraint Maps for an area to enable assessment of the key constraints on ecological outcomes relative to a specific issue or concern.
Habitat Features: The term “habitat” means different things to different people in different contexts (Hall et al., 1997). As a result, for clarity, we have used the term “habitat features” defined as areas of geological/geomorphological (e.g., boulders, gravel, rock, sand, clay) or biological (e.g., snags, in-water vegetation), hydrodynamic (e.g., plume front) components utilized by an organism, and defined at a scale relevant to the size and activity of the species in question. For example: a single mangrove root might provide usable habitat structure for a gastropod, a whole mangrove tree root complex for a crab, and a whole mangrove stand for a large (10-100 cm length) mangrove dwelling fish (e.g., Department of Environment and Science, Queensland, 2019).
Habitat Mosaics: Groups of Habitat Features, and the complex spatio-temporal connections among them, that support particular life-history stages.
Species Requirement Set: The complete set of requirements for a species to occur at a particular location based on all the situations and locations in which it occurs.
Structural Spatial Map: A geospatial representation of the habitat features in the area of interest for Constraint Mapping.
Under the logic that the most efficient use of management resources is to concentrate them on the key aspects that constrain and determine outcomes (e.g., biodiverse ecosystems, sustainable populations, fisheries productivity), we discuss Ecological Constraint Mapping (see Box 1 for definitions of terms) as an approach that focusses on identifying the factors that constrain life-history outcomes of particular species, faunal/floral groups, and provides a framework for the development of actionable knowledge and a basis for determining specific data needs.
Ecological Constraint Mapping
Risk-based approaches, such as Ecological Constraint Mapping (abridged for brevity to “Constraint Mapping”), are widely used in fields as diverse as business (Knechel, 2007) and law enforcement (Vander Beken, 2004), and form the basis of major environmental protocols such as the Red List of Ecosystems (Keith et al., 2013, 2015), where they provide a way of focussing on the information of greatest importance to decision-making. Risk-based approaches have been successfully applied to decisions around terrestrial renewable energy installations (Gove et al., 2016) and water resource planning (Mcgregor et al., 2018), and in the marine environment have been used to incorporate environmental, economic and social factors into restoration decision-making (Kennish et al., 2002).
Similar logic suggests that Constraint Mapping can be profitably applied to marine and coastal environmental problems, and can be relevant to a wide range of marine and coastal activities including fisheries management, coastal development, port infrastructure, and environmental protection. These disparate activities have diverse objectives and face different barriers to success (Stewart Sinclair et al., 2020), so Constraint Maps need to be context-specific to usefully inform specific outcomes based on questions and needs. Additionally, Constraint Maps need to be developed at scales relevant to ecosystem function, species life-histories and management requirements. Therefore, instead of simply detailing information about habitat extent, composition and occupancy, the focus is on identifying key habitat mosaic components and associated environmental requirements, over species life histories. Indeed, Constraint Maps need not relate to whole of a species life-history but can be developed at different conceptual scales (e.g., focussed on nursery ground utilisation) depending on relevance to the intended use.
Consequently, Constraint Mapping aligns with increasing understanding of the nuanced ways that organisms derive value from the environment (refuge, feeding areas), building on a spatially explicit conceptualisation of (nursery) habitat utilisation (Johnston and Sheaves, 2007; Nagelkerken et al., 2015). The viability, resistance and resilience of a species, population, community, food web or ecosystem depends on the complex interaction of habitat, connections, the physical environment, and the faunal components themselves, that successfully enable key life-supporting functions. Success and resilience are undermined when one or more of these key life-supporting functions is impaired for any life-history stage. Consequently, identifying (and understanding) the specific factors (habitat, connectivity, physical environmental requirements) constraining success in a particular situation is vital information needed to underpin optimal ecological outcomes, regardless of the outcome sought – whether that is, for example, attaining resilient fisheries (Hill et al., 2020), managing coastal wetlands (DEHP, 2012) or protecting endangered species. Indeed, the knowledge provided by Constraint Mapping is critical to understanding overall ecosystem functioning (Dobson et al., 2006), landscape resilience (Cumming, 2011; Olds et al., 2012), species and community responses to external pressures (Olden and Jackson, 2001; Villéger et al., 2010), and can serve as a key input into knowledge platforms for assessing the likely risks of climate change on species.
Important components of Constraint Mapping include: mapping of the existence, scale, extent and composition of habitat features; species use of habitat features specific to life-history stage, the services (particularly the unique values) that those habitats features provide to organisms (e.g., food, spawning sites, nursery grounds), key connections between the necessary habitat features, and environmental needs specific to life-history stage (e.g., water quality, sediment type, shelter). Together this body of information can be combined to provide a detailed assessment of the needs and requirements of species throughout their lives linked to the habitat mosaic – the Constraint Map. This, in turn, provides the base information for identifying the ecological and environmental factors that constrain or regulate ecological outcomes for a species (Figure 2).
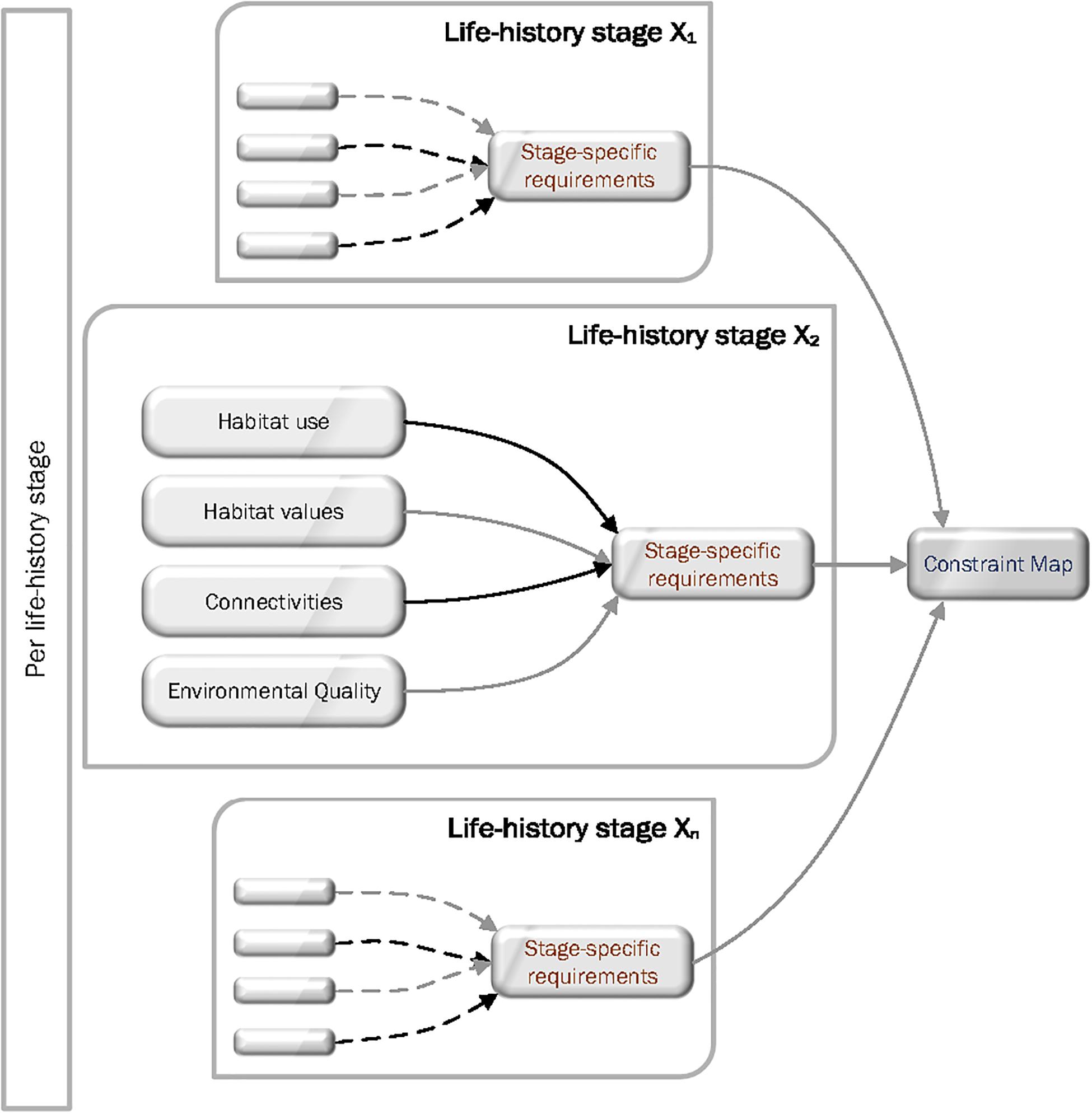
Figure 2. The Ecological Constraint Mapping framework that is repeated for each life-history stage of a species. Left-hand side box (per life-history stage) indicates the need for integration across life history stages. The more comprehensive the integration, the more likely true constraints will be detected. Life-history stage Xn indicates the framework applies to as many life-history stages as have been identified.
Fisheries management provides a useful case study when considering the utility of Ecological Constraint Mapping. A clear understanding of the functions regulating the productivity of each species or population within a fishery is the logical basis for many management decisions. For instance, it is vital in determining the extent to which management needs to focus on: (i) maintaining particular habitat features (e.g., a nursery habitat of limited extent or one where water quality is limiting for a species) vs. (ii) enhancing the availability of recruits (e.g., restocking), or (iii) regulating fishing pressure (e.g., catch quotas). Indeed, understanding the processes and aspects that constrain the success of species is a logical first step before undertaking potentially disruptive and costly action such as restocking (Loneragan et al., 2013). In a specific example in the context of marine and coastal management; by focussing on identifying the key bottlenecks, Constraint Mapping provides a focussed way to populate attribute-based mapping (e.g., intertidal and subtidal mapping: Department of Environment and Science, Queensland, 2019) with process level information relevant to species, life-history stage or community outcomes. In contrast, a comprehensive model of all ecological and biological aspect relevant to a species can be prohibitively expensive to obtain and extremely difficult to conceptualise.
Details of the Components of Ecological Constraint Mapping
The Constraint Map provides the basis for identifying and understanding the situation-specific constraints on outcomes and the critical knowledge required to inform environmental decision-making. The Constraint Map will indicate which components/qualities of the landscape/physical environment actively regulate a species’/habitat’s ability to meet its requirements. In turn, knowledge of these constraints provides the underpinning of high quality and efficient decision-making and priority setting.
Because Constraint Mapping aims to support decision-making of all types, it could be implemented to suit a spectrum of management purposes (e.g., whole fishery, habitat type), as well as promoting a standard language for quantitative models. Constraint Mapping is fundamentally an objective, bottom-up approach that focusses on habitats, individual species or populations, and on linking those species to habitat mapping applications or spatial decision tools. In most cases, it is logical to construct habitat-specific and species-by-species Constraint Maps, focussing first on priority species/habitats, with the aim of developing an increasingly comprehensive network of maps over time. The details of the Constraint Mapping procedure will vary from situation to situation, but, in general, can be thought of as a four-part procedure (Figure 3): (1) Identification of Taxa of Interest; (2) Definition of Habitat Features (standardised within- and, where possible, between-applications); (3) Identification of the Species Requirement Set; and (4) Situation-Specific Assessment. Additional, more complex steps are possible in data-rich situations [e.g., estimating the relative contributions of different habitat features to fisheries stocks (Fodrie et al., 2009)], however, the value of these depends on comprehensive understanding of all the factors contributing to the outcome of interest, meaning there will be relatively few situations where data-rich options can be employed without the need to amass a considerable body of additional information.
(1) Identification of Taxa of Interest: Many criteria could be used in identifying the taxa of interest, but selection would generally depend on factors such as: (a) government regulations and conservation agreements, (b) endangered status, (c) species or communities most vulnerable to extinction, (d) loss or damage economic value, (e) public interest (expert elicitation process) or (f) importance in maintaining key ecological processes (e.g., top-down control, herbivory, etc.). In some cases it may be a specific high value/threatened habitat or geographical feature that is of primary interest rather than a specific taxon. In this situation Ecological Constraint Mapping can be used to provide information on the relevance of the habitat feature to taxa and communities, with taxa of interest determined by their occurrence in the target habitat feature. Therefore, the assessment is focussed on the value of that habitat feature to the taxa occurring there relative to alternative habitat features available in the system.
(2) Definition of Habitat Features: The process involves the classification of the Habitat Features in areas utilised by each taxon of interest at scales relevant to the taxon (see Box 1). This provides the set of relevant habitat features against which constraints can be evaluated (Step 3), as well as enabling the development of the Structural Spatial Map that forms the physical basis for Situation-Specific Constraint Mapping (Step 4).
(3) Identification of the Species Requirement Set: This step involves assessment of the fundamental requirements of each species of concern, across all the situations and locations in which it occurs. There are four vital components: (a) Identifying potentially valuable Habitat Features. This includes identifying Stage-Specific Occupancy per Habitat Feature Type (i.e., what stages occupy each habitat feature type), assessing the Stage-Specific Density per Habitat Feature Type, and determining the Value of each Habitat Feature to each Life-History Stage. (b) Identifying Key Connectivities. Rarely does a single habitat feature provide all the requirements of a life-history stage, with different habitat features providing such things as food and refuge over various time scales (e.g., day/night, high/low tide) (Bacheler et al., 2009). As a result, determining the key connections is as important as identifying the habitat features themselves. Together, the habitat features and the connectivities define the life-history stage-specific Habitat Mosaic Components; the fundamental units that provide life and life-history supporting functions (e.g., feeding, refuge, spawning) (Nagelkerken et al., 2015). (c) Assess the Extent of Redundancy of Mosaic Components. Once identified, each of the Mosaic Components needs be assessed to determine the extent to which it provides unique life-history supporting functions (i.e., represents a fundamental requirement). If a unique service is present, then it has the potential to be a constraining factor. However, if the particular service is provided by several different mosaic components it affords possible redundancy and may be less constraining in particular situations. (d) Identify Environmental and Ecological Requirements. In addition to habitat features and connectivity, environmental and ecological requirements, such as temperature, salinity, dissolved oxygen, sediment chemistry, dietary requirements and interactions with other species, that can constrain life-history outcomes, need to be assessed. In terms of Constraint Mapping as a tool for identifying and understanding the situation-specific constraints on outcomes, it is important to separate local effects of environmental and ecological factors from the influence of the same factors at geographic or biogeographic scales (i.e., the Species Requirement Set). These large-scale factors limit the range over which a species can occur, but it is the local manifestation of these constraints that Constraint Mapping is concerned with.
Initially, identifying the Species Requirement Set will usually begin with a careful assessment of the body of knowledge already available on the species of interest. This needs to be an in-depth evaluation to ensure the information is strongly evidence based and reflects the most up to date understanding (Sheaves et al., 2020a). A full assessment of the current state of knowledge will reveal what information is already available, and indicate what additional information may be required for the specific Constraint Mapping application. Usually many aspects of the Species Requirement Set will be data deficient. Logically this gap identification should be followed by specific studies to address any key knowledge gaps highlighted. However, in reality this will rarely be possible at a whole-of-species scale; rather the gap identification will be used to direct Step 4, the Situation-Specific Assessment (see below), with the knowledge gained feeding back to refine the Species Requirement Set.
While the Species Requirement Set should ideally encompass the whole geographic range of the taxon, in reality, the management application will usually apply to a particular region. However, over time, the collection of compatible data across regions can be used to develop a comprehensive Species Requirement Set. Consequently, it is important to understand how much of the Species Requirement Set and the situation-specific constraints on outcomes are generic and to what extent they need to be nuanced for different contexts (Bradley et al., 2020).
(4) Situation-Specific Assessment: In this step, information from the Species Requirement Set is applied to a particular area of interest. Usually, specific studies will be required to develop a nuanced understanding accounting for local conditions, leading to knowledge of the constraints relative to particular types of local habitats. Critical issues assessed include: (1) the extent of critical Habitat Feature components relative to life-history stage needs, (2) identification of alternative structural components where they exist, (3) the intactness of key connections, and (4) the physical conditions prevailing in the habitat features relative to the Species Requirement Set and any natural or anthropogenic impediments. Ultimately, and more directly useful for management, situation-specific Constraint Mapping can be applied to a Structural Spatial Map – detailed habitat mapping to which the logic of Constraint Mapping can be applied – to develop a location-specific Structural Constraint Map. The existence of a Structural Spatial Map is a key pre-requisite for this process. Ideally, such maps need to be based on explicit and consistent frameworks, and while they exist or are under development for some systems (e.g., Department of Environment and Science, Queensland, 2019), they need to be a priority for others if the full benefits of Constraint Mapping are to be translated into management.
Example Case Study for a Constrain Map Approach and Outcome
The Department of Agriculture and Fisheries in Queensland, Australia, was interested in understanding the factors that determine nursery ground value for coastal fisheries species in upstream estuarine areas. To achieve this, Constraint Mapping was employed in estuaries in the Baffle Drainage Basin (23.94oS–24.67oS). The area is meso-tidal (range of 0–4 m) with highly seasonal rainfall, meaning smaller estuaries are only connected to freshwater intermittently (Sheaves, 1996). This results in strong tidal influence, with the frequency of connection to the ocean, and the duration of connection, decreasing toward upstream areas (See Supplementary Material for details). The area was of interest for decision-makers because of the potential for the removal of artificial bund walls and weirs in the upstream areas to restore natural hydrology, if this would result in a positive increase in habitat availability for fisheries species. To assessing the likely outcome of these interventions, the 4-step procedure was utilised to develop a constraint map.
(1) The Taxa of Interest identified was the mangrove red snapper, Lutjanus argentimaculatus, an important recreational and commercial species known to use estuarine and freshwater nurseries (Sheaves, 1995; Russell and McDougall, 2005), assessed as overfished in recent years in some areas of Queensland. (2) The Habitat Features were identified during site assessments when the upstream areas of the Baffle Drainage Basin were mapped. These sites consisted of a stream reach, usually composed of individual pools at low tide separated by rock and sand bars and featuring a range of complex habitats (e.g., snag, complex rock, flat bedrock). (3) The initial Species Requirement Set was determined following an extensive literature review of the species of interest, and this also provided key information on the ecology of the species and enabled analysis of critical knowledge gaps. It was determined that juvenile L. argentimaculatus has habitat requirements distinct from those of adults, and that upstream estuarine and downstream freshwater habitats comprised the most important habitats for early juveniles (e.g., Russell and McDougall, 2005). The final step was to make (4) Situation-Specific Assessments to the factors that constrain the occupation of upstream estuary habitats as nursery grounds for L. argentimaculatus.
Fish surveys were carried out using underwater video cameras throughout the estuarine and lower freshwater reaches of five systems, and physical and environmental characteristics assessed (Mattone et al., in review)1. Tidal connection patterns, dissolved oxygen (DO), temperature and salinity were recorded over the course of the study. The dominant habitat types in each site surveyed were assessed to characterise overall habitat complexity and utilisation (See Supplementary Material for details). The importance of various factors in predicting L. argentimaculatus occurrence was estimated and the constraints on the nursery habitat use determined (See Supplementary Material for analytical workflow sequence). The study identified that habitat use of juvenile L. argentimaculatus is constrained by 4 major factors: (a) pool size, (b) tidal connectivity, (c) DO dynamics, and (d) structural complexity (See Footnote 1). In fact, juveniles were mostly found in upstream areas that are tidally connected at least 60 days per year, and at least 0.5 m deep with area of 20 m2 at the lowest tidal level. Habitat occupancy of L. argentimaculatus juveniles was not constrained by salinity, as they were found across the full range of salinities encountered, including hypersaline conditions (>50 ‰). On the other hand, DO levels were likely to constrain juvenile habitat use, with L. argentimaculatus juveniles only observed in areas that only experienced short-term oxygen sags below >60% saturation. The proximity of complex structure (e.g., large woody debris, complex rock) was a very strong predictor of occurrence for the juveniles. By mapping these range of constraints spatially, a final Constraint Map was produced to inform and direct decision-making in this region (Figure 4).
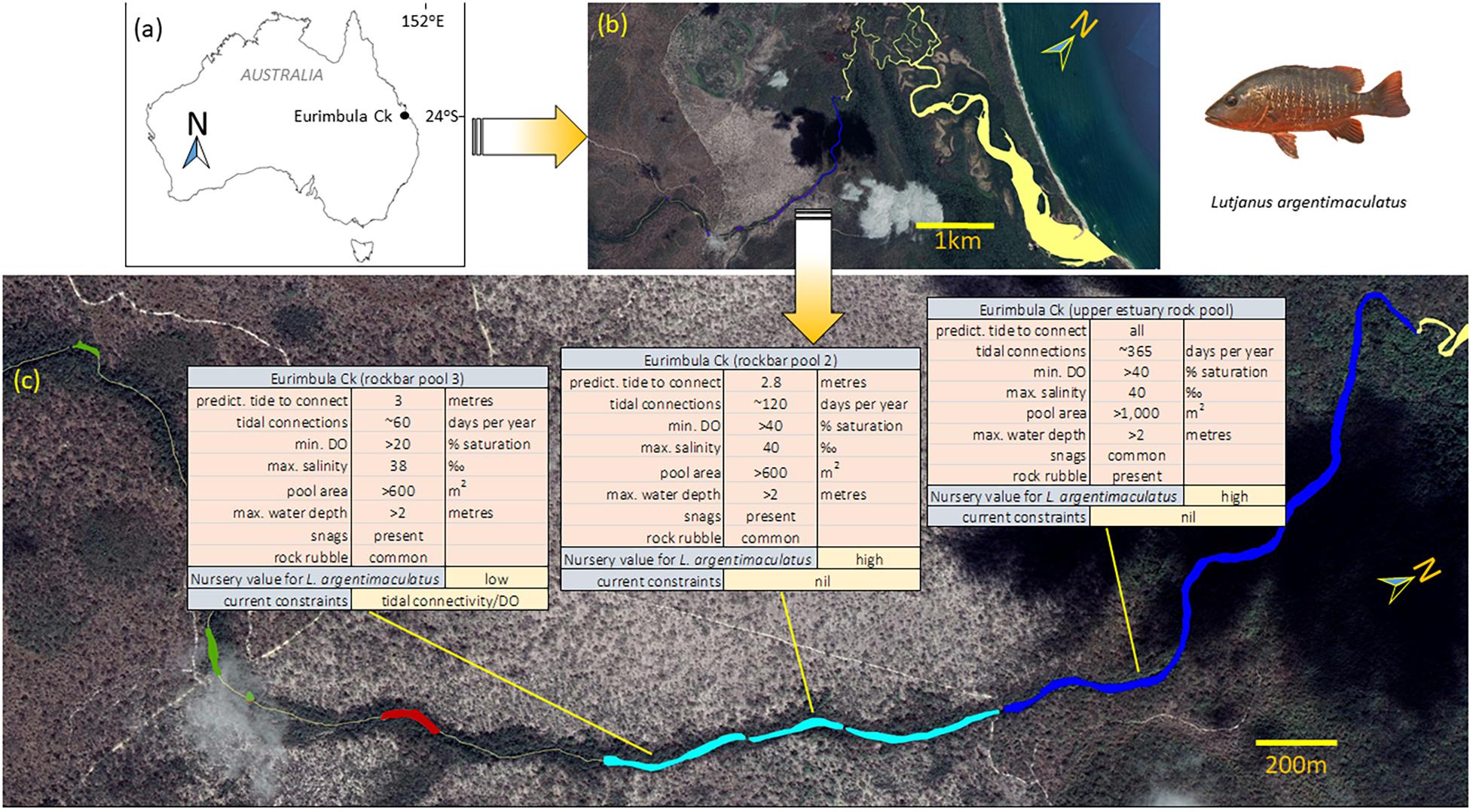
Figure 4. Example of a Constraint Map from a study by (See Footnote 1) aimed at improving the understanding of the factors that determine nursery usage at a species level, for three coastal fisheries species, in the case of this example the snapper Lutjananus argentimaculatus. Panels (a) and (b) location maps; (c) constraint tables for key constraints for nursery utilisation of three upper estuarine pools in Eurimbula Creek for L. argentimaculatus. The colour in the map indicate the level of tidal connectivity and salinity (dark blue = connected at every high tide, with marine to hypersaline conditions; light blue = connected during most high tide with marine to hypersaline conditions; Red = only connected on the highest spring tides with fresh to hyposaline conditions; Green = never tidally connected fully freshwater).
Output and Utilisation of Constraint Mapping
The basic output of the constraint mapping process is a knowledge-platform providing nuanced habitat requirement information for a species of interest that can be applied to a particular area. Preferably, this information should refer to the whole of a species life-cycle, but, at least initially, the constraint mapping is likely to be a situation- or need-specific (e.g., the case study presented). To be useful and accessible, the constraint mapping data layer needs to be displayed in a form that is useful to the end-user (generally government manager/policy maker, NGO, or industry), and which is easily integrated into existing spatial data repositories.
The information from a constraint map could be operationalised as site-specific data tables displayed on a mapping platform such as Google Earth, ESRI online, or remap, or used as the basis for decision support tools. Many other formats are possible. However, what is important is the detail and mode of the display relative to the intended purpose. For instance, it could be used as in Figure 4, to construct a specific assessment map that provides functional information relative to a particular focal issue (e.g., the nursery value of upstream estuary reaches to fisheries species) or alternatively as a basis for a Bayesian Belief Net predictive model. These could be used as simple decision-support tools, for instance, to enable coastal planners to make “green engineering” (Waltham and Sheaves, 2015) decisions on infrastructure that minimises ecological damage or optimises ecological outcomes (e.g., fine-tuning the design of a road culvert to enable ingress of a suitable number of tides per year to maintain connectivity). Similarly, the constraint information could allow regulators to make an informed decision on specific developments or barrier removal, or assist in the long-term planning for a region. By providing a clear exposition of the key information, the constraint map also provides for the focussed collection of information needed to extend understanding into other areas, and a way of identifying key knowledge gaps and filling them.
For instance, the information obtained in the case study, could be used to predict areas most likely to host juvenile L. argentimaculatus, information necessary for the prioritization of restoration efforts (e.g., bund removal, revegetation) or the minimization of the adverse impact of human development (e.g., roads). Additionally, the constraints on nursery ground value for L. argentimaculatus juveniles were assessed in light of a specific question and with certain knowledge gaps in mind. This means that, while relevant for that particular situation, results of the case study are likely to be fairly situation-specific, as well as specific to the particular aims of the study. As a result, the models developed in the case study would need to be tested and adjusted before they were applied to other areas or situations. These additional studies would also assist in the development of a more generally applicable understanding that provided more extensive geographic relevance. Tuning the models to different areas or situations will often require the collection of data in addition to those already available. This will particularly be the case where most available data come from fisheries-dependent sources because, by definition, juveniles will usually be poorly represented in fisheries-dependent studies. One important step will be to review the nature of the new area to be assessed and the scope, detail and quality of available information, in order to evaluate the extent to which the available model is likely to be appropriate, and to sharpen the focus of additional studies.
Conclusion and Recommendations
Ecological Constraint Mapping provides a vehicle to facilitate a move from paradigm-based decision making, that risks reliance on poorly supported ideas (Sheaves et al., 2020a), to knowledge-informed decision making. Without knowledge of the critical functional bottlenecks that constrain anticipated ecological outcomes, environmental decision-making will continue to provide sub-optimal, and sometimes perverse outcomes and result in wasted resources; an increasingly unsustainable situation in a world where ecosystems are increasingly impacted by anthropogenic activities and climate change (Waltham et al., 2020). Consequently, Constraint Mapping provides a key tool for the effective translation of general scientific ecological information to actionable knowledge suitable for use for specific management purposes (Enquist et al., 2017). By identifying the particular landscape and seascape components that constrain optimal outcomes, Constraint Mapping provides information vital to directing actions efficiently and effectively.
In providing a framework that aligns with current perspectives on the complex nature of marine and coastal systems, Constraint Mapping can enable the efficient development of a general functional understanding of the requirements critical for species viability. This, in itself, provides a tactical advantage; if the key constraints can be identified at large scale (e.g., regional or even global), local data collection can focus cost-effectively on addressing key knowledge gaps specific to the local situation. Consequently, Ecological Constraint Mapping can help achieve that most elusive of goals: properly addressing identified knowledge gaps. In addition, unlike other decision-support tools, fundamental characteristics of Constraint Mapping are its scalability and explicit focus on the extent to which the included knowledge is transferable to other areas and situations (considering local-specific constraints in terms of a broad understanding of species needs). Consequently, Constraint Mapping is suitable for developing optimal solutions to key resource and conservation applications in marine and coastal realms, in areas as diverse as fisheries productivity and resilience, species persistence, local biodiversity, overall ecosystem health, environmental offsetting, habitat restoration, monitoring and assessment, and resilient food webs.
The discussion of Constraint Mapping has concentrated on species interaction-based questions, a focus that is particularly pertinent to species-by-species management, the level at which large scale marine biological resource management is still primarily conducted (Hilborn, 2011). However, a logical extension is to develop Constraint Mapping to support whole-of-ecosystem management by overlaying single species Constraints Maps into Constraint Networks, allowing identification of process hotspots and coldspots (areas that constrain outcomes for sets of species). The identification of the process hotspots and coldspots in Constraint Networks would enable improved targeting of actions enabling more effective management at species community levels.
It is easy to dismiss the development of a constraint-based understanding of marine and coastal ecosystems as an unachievable goal because it is data-intensive and difficult. Realistically, Constraint Mapping provides a cost-effective approach to achieving management goals. Because amassing the necessary knowledge is expensive, developing a Constraint Map will be most achievable in situations where the cost of perverse outcomes are highest or most obvious, or where the incentives for knowledge-rich outcomes are substantial. For example, detailed and defensible understanding can enable retiring risk associated with the operation of marine renewable energy infrastructure, significantly reducing the need for expensive intensive monitoring (Copping et al., 2019). However, there is an urgent need to extend Constraint Mapping even to resource-poor areas because these are areas where resource dependency is often highest, and because of the resource savings and management efficiencies made possible by knowledge-informed decision-making. A Constraint Mapping approach offers considerable opportunities because the understanding developed in identifying the Species Requirement Set can direct the key research needed to construct a nuanced Constraint Map for resource-poor contexts. More broadly, the lack of such functional understanding is a major inhibitor to achieving the triple-bottom-line of the blue economy [sustainable use of marine resources to optimise joint economic, social and ecosystem outcomes (Silver et al., 2015)], because a well-functioning and sustainable blue-economy are vital for both developing and developed economies in increasingly stressed marine and coastal environments (Roberts and Ali, 2016).
Constraint Mapping provides only one component of the information needed for holistic decision-making. The nuanced habitat value layer provided by constraint mapping still needs to be combined and integrated with other situation-specific information (Ascough Ii et al., 2008), such as fisheries data on recruitment and growth rates and stock levels, future climate change predictions, patterns of coastal development, details of dredging programs, social-economic factors, etc. Indeed, Constraint Mapping should not be seen as an end to itself but rather a key component that informs and is informed by, broader management frameworks (e.g., Figure 1). Because Constraint Mapping aims to identify and understand the specific biological and biophysical bottlenecks inhibiting species viability, the information from Constraint Mapping provides the opportunity to make more precise, knowledge-informed decisions that rapidly produce desired environmental outcomes and minimise the costs of misdirected actions.
Author Contributions
MS, CM, RC, MB, and NW conceived the idea. All authors contributed to writing and editing the manuscript.
Conflict of Interest
The authors declare that the research was conducted in the absence of any commercial or financial relationships that could be construed as a potential conflict of interest.
Publisher’s Note
All claims expressed in this article are solely those of the authors and do not necessarily represent those of their affiliated organizations, or those of the publisher, the editors and the reviewers. Any product that may be evaluated in this article, or claim that may be made by its manufacturer, is not guaranteed or endorsed by the publisher.
Supplementary Material
The Supplementary Material for this article can be found online at: https://www.frontiersin.org/articles/10.3389/fmars.2021.717448/full#supplementary-material
Footnotes
- ^ Mattone, C., Bradley, M., Barnett, A., Konovalov, D., and Sheaves, M. (in review). Species-specific constraints on the nursery value of sub-tropical estuarine habitats. Ecosystems.
References
Abrantes, K. G., Sheaves, M., and Fries, J. (2019). Estimating the value of tropical coastal wetland habitats to fisheries: caveats and assumptions. PLoS ONE 14:e0215350. doi: 10.1371/journal.pone.0215350
Ascough Ii, J., Maier, H., Ravalico, J., and Strudley, M. (2008). Future research challenges for incorporation of uncertainty in environmental and ecological decision-making. Ecol. Modell. 219, 383–399. doi: 10.1016/j.ecolmodel.2008.07.015
Bacheler, N. M., Paramore, L. M., Buckel, J. A., and Hightower, J. E. (2009). Abiotic and biotic factors influence the habitat use of an estuarine fish. Mar. Ecol. Prog. Ser. 377, 263–277. doi: 10.3354/meps07805
Bartley, D. M., and Bell, J. D. (2008). Restocking, stock enhancement, and sea ranching: Arenas of progress. Rev. Fish. Sci. 16, 357–365. doi: 10.1080/10641260701678058
Bell, J. D., Bartley, D. M., Lorenzen, K., and Loneragan, N. R. (2006). Restocking and stock enhancement of coastal fisheries: potential, problems and progress. Fish. Res. 80, 1–8. doi: 10.1016/j.fishres.2006.03.008
Bonhomme, F., and Planes, S. (2000). Some evolutionary arguments about what maintains the pelagic interval in reef fishes. Environ. Biol. Fish. 59, 365–383. doi: 10.1023/a:1026508715631
Bradley, M., Baker, R., and Sheaves, M. (2017). Hidden components in tropical seascapes: deep-estuary habitats support unique fish assemblages. Estuar. Coasts 40, 1195–1206. doi: 10.1007/s12237-016-0192-z
Bradley, M., Nagelkerken, I., Baker, R., and Sheaves, M. (2020). Context-dependence: a conceptual approach for understanding the habitat relationships of coastal marine fauna. BioScience 70, 986–1004.
Brett, A., Leape, J., Abbott, M., Sakaguchi, H., Cao, L., Chand, K., et al. (2020). Ocean data need a sea change to help navigate the warming world. Nature 582, 181–183. doi: 10.1038/d41586-020-01668-z
Brown, C. J., Broadley, A., Adame, M. F., Branch, T. A., Turschwell, M. P., and Connolly, R. M. (2019). The assessment of fishery status depends on fish habitats. Fish Fish. 20, 1–14. doi: 10.1111/faf.12318
Carpenter, S. R., Mooney, H. A., Agard, J., Capistrano, D., DeFries, R. S., Díaz, S., et al. (2009). Science for managing ecosystem services: beyond the millennium ecosystem assessment. Proc. Nat. Acad. Sci. 106, 1305–1312.
Christin, S., Hervet, E., and Lecomte, N. (2018). Applications for deep learning in ecology. Methods Ecol. Evol. 10, 1632–1644.
Copping, A. E., Freeman, M. C., Gorton, A. M., and Hemery, L. G. (2019). A Risk Retirement Pathway for Potential Effects of Underwater Noise and Electromagnetic Fields for Marine Renewable Energy. Paper presented at the OCEANS 2019. SEATTLE: IEEE, doi: 10.23919/OCEANS40490.2019.8962841
Cumming, G. S. (2011). Spatial resilience: integrating landscape ecology, resilience, and sustainability. Landsc. Ecol. 26, 899–909. doi: 10.1007/s10980-011-9623-1
DEHP. (2017). Queensland Intertidal and Subtidal ecosystem classification scheme Version 1.0: Module 1—Introduction and implementation of intertidal and subtidal ecosystem classification. Retrieved from Brisbane, QLD.
Department of Environment and Science, Queensland (2019). Queensland Wetland Classification Method, WetlandInfo Website. Available online at: https://wetlandinfo.des.qld.gov.au/wetlands/what-are-wetlands/definitions-classification/classification-systems-background/typology.html (accessed August 2, 2021).
Dobson, A., Lodge, D., Alder, J., Cumming, G. S., Keymer, J., McGlade, J., et al. (2006). Habitat loss, trophic collapse, and the decline of ecosystem services. Ecology 87, 1915–1924. doi: 10.1890/0012-9658(2006)87[1915:hltcat]2.0.co;2
Dubuc, A., Waltham, N., Baker, R., Marchand, C., and Sheaves, M. (2018). Patterns of fish utilisation in a tropical Indo-Pacific mangrove-coral seascape, New Caledonia. Plos one 14:e0207168. doi: 10.1371/journal.pone.0207168
Enquist, C. A., Jackson, S. T., Garfin, G. M., Davis, F. W., Gerber, L. R., Littell, J. A., et al. (2017). Foundations of translational ecology. Front. Ecol. Environ. 15, 541–550. doi: 10.1002/fee.1733
Fodrie, F. J., Levin, L. A., and Lucas, A. J. (2009). Use of population fitness to evaluate the nursery function of juvenile habitats. Mar. Ecol. Prog. Ser. 385, 39–49. doi: 10.3354/meps08069
Gilby, B. L., Olds, A. D., Connolly, R. M., Henderson, C. J., and Schlacher, T. A. (2018). Spatial restoration ecology: placing restoration in a landscape context. Bioscience 68, 1007–1019. doi: 10.1093/biosci/biy126
Gove, B., Williams, L. J., Beresford, A. E., Roddis, P., Campbell, C., Teuten, E., et al. (2016). Reconciling biodiversity conservation and widespread deployment of renewable energy technologies in the UK. PloS one 11:e0150956. doi: 10.1371/journal.pone.0150956
Griffiths, L. L., Connolly, R. M., and Brown, C. J. (2019). Critical gaps in seagrass protection reveal the need to address multiple pressures and cumulative impacts. Ocean Coast. Manage. 183:104946. doi: 10.1016/j.ocecoaman.2019.104946
Grubbs, R. D., Carlson, J. K., Romine, J. G., Curtis, T. H., McElroy, W. D., McCandless, C. T., et al. (2016). Critical assessment and ramifications of a purported marine trophic cascade. Sci. Rep. 6:20970. doi: 10.1038/srep20970
Hall, L. S., Krausman, P. R., and Morrison, M. L. (1997). The habitat concept and a plea for standard terminology. Wildl. Soc. Bull. 25:173–182.
Halpern, B. S., Longo, C., Hardy, D., McLeod, K. L., Samhouri, J. F., Katona, S. K., et al. (2012). An index to assess the health and benefits of the global ocean. Nature 488:615.
Hawkins, C. T. (2011). Finding blame for environmental outcomes: a cognitive style approach to understanding stakeholder attributions, attitudes, and values. Univ. Massachusetts Amherst 1–110.
Hilborn, R. (2011). Future directions in ecosystem based fisheries management: a personal perspective. Fish. Res. 108, 235–239. doi: 10.1016/j.fishres.2010.12.030
Hilderbrand, R. H., Watts, A. C., and Randle, A. M. (2005). The myths of restoration ecology. Ecol. Soc. 10:100119,
Hill, S. L., Hinke, J., Bertrand, S., Fritz, L., Furness, R. W., Ianelli, J. N., et al. (2020). Reference points for predators will progress ecosystem−based management of fisheries. Fish Fish. 21, 368–378. doi: 10.1111/faf.12434
Johnston, R., and Sheaves, M. (2007). Small fish and crustaceans demonstrate a preference for particular small-scale habitats when mangrove forests are not accessible. J. Exp. Mar. Biol. Ecol 353, 164–179. doi: 10.1016/j.jembe.2007.05.039
Keith, D. A., Rodriguez, J. P., Brooks, T. M., Burgman, M. A., Barrow, E. G., Bland, L., et al. (2015). The IUCN red list of ecosystems: motivations, challenges, and applications. Conserv. Lett. 8, 214–226. doi: 10.1111/conl.12167
Keith, D. A., Rodriguez, J. P., Rodriguez-Clark, K. M., Nicholson, E., Aapala, K., Alonso, A., et al. (2013). Scientific foundations for an IUCN red list of ecosystems. PloS one 8:e62111. doi: 10.1371/journal.pone.0062111
Kennish, R., Wilson, K. D., Lo, J., Clarke, S. C., and Laister, S. (2002). Selecting sites for large-scale deployment of artificial reefs in Hong Kong: constraint mapping and prioritization techniques. ICES J. Mar. Sci. 59, S164–S170.
Kimirei, I. A., Nagelkerken, I., Slooter, N., Gonzalez, E., Huijbers, C. M., Mgaya, Y. D., et al. (2015). Demography of fish populations reveals new challenges in appraising juvenile habitat values. Mar. Ecol. Prog. Ser. 518, 225–237. doi: 10.3354/meps11059
Knechel, W. R. (2007). The business risk audit: origins, obstacles and opportunities. Account. Org. Soc. 32, 383–408. doi: 10.1016/j.aos.2006.09.005
Konovalov, D. A., Saleh, A., Bradley, M., Sankupellay, M., Marini, S., and Sheaves, M. (2019). Underwater Fish Detection with Weak Multi-Domain Supervision. Paper presented at the 2019 International Joint Conference on Neural Networks. Budapest: IJCNN.
Lefcheck, J. S., Hughes, B. B., Johnson, A. J., Pfirrmann, B. W., Rasher, D. B., Smyth, A. R., et al. (2019). Are coastal habitats important nurseries? a meta−analysis. Conserv. Lett. 12:e12645. doi: 10.1111/conl.12645
Loneragan, N. R., Jenkins, G. I., and Taylor, M. D. (2013). Marine stock enhancement, restocking, and sea ranching in Australia: future directions and a synthesis of two decades of research and development. Rev. Fish. Sci. 21, 222–236. doi: 10.1080/10641262.2013.796810
Mattone, C., and Sheaves, M. (2017). Patterns, drivers and implications of dissolved oxygen dynamics in tropical mangrove forests. Estuarine Coast. Shelf Sci. 197, 205–213. doi: 10.1016/j.ecss.2017.08.028
Mcgregor, G. B., Marshall, J. C., Lobegeiger, J. S., Holloway, D., Menke, N., and Coysh, J. (2018). A risk-based ecohydrological approach to assessing environmental flow regimes. Environ. Manag. 61, 358–374. doi: 10.1007/s00267-017-0850-3
Nagelkerken, I., Sheaves, M., Baker, R., and Connolly, R. M. (2015). The seascape nursery: a novel spatial approach to identify and manage nurseries for coastal marine fauna. Fish Fish. 16, 362–371. doi: 10.1111/faf.12057
Newman, S. J., Williams, A. J., Wakefield, C. B., Nicol, S. J., Taylor, B. M., and O’Malley, J. M. (2016). Review of the life history characteristics, ecology and fisheries for deep-water tropical demersal fish in the Indo-Pacific region. Rev. Fish Biol. Fish. 26, 537–562. doi: 10.1007/s11160-016-9442-1
Olden, J. D., and Jackson, D. A. (2001). Fish-habitat relationships in lakes: Gaining predictive and explanatory insight by using artificial neural networks. Trans. Am. Fish. Soc. 130, 878–897. doi: 10.1577/1548-8659(2001)130<0878:fhrilg>2.0.co;2
Olds, A. D., Pitt, K. A., Maxwell, P. S., and Connolly, R. M. (2012). Synergistic effects of reserves and connectivity on ecological resilience. J. Appl. Ecol. 49, 1195–1203. doi: 10.1111/jpe.12002
Peterson, C. H., and Bishop, M. J. (2005). Assessing the environmental impacts of beach nourishment. Bioscience 55, 887–896. doi: 10.1641/0006-3568(2005)055[0887:ateiob]2.0.co;2
QldDEHP (2016). Wetlands in the Great Barrier Reef Catchments: Management Strategy 2016-2021. Brisbane, QLD: DEHP
Russell, D. J., and McDougall, A. J. (2005). Movement and juvenile recruitment of mangrove jack, Lutjanus argentimaculatus (Forsskål), in northern Australia. Mar. Freshw. Res. 56, 465–475. doi: 10.1071/MF04222
Saenger, P., Gartside, D., and Funge-Smith, S. (2013). A review of mangrove and seagrass ecosystems and their linkage to fisheries and fisheries management. Report to Food and Agriculture Organization of the United Nations Regional Office for Asia and the Pacific. Bangkok.
Sheaves, M. (1995). Large lutjanid and serranid fishes in tropical estuaries: are they adults or juveniles? Mar. Ecol. Progr. Ser. 129, 31–40. doi: 10.3354/meps129031
Sheaves, M. (1996). Do spatial differences in the abundance of two serranid fishes in estuaries of tropical Australia reflect longterm salinity patterns? Mar. Ecol. Prog. Ser. 137, 39–49. doi: 10.3354/MEPS137039
Sheaves, M. (2016). Simple processes drive unpredictable differences in estuarine fish assemblages: baselines for understanding site-specific ecological and anthropogenic impacts. Estuarine Coast. Shelf Sci. 170, 61–69. doi: 10.1016/j.ecss.2015.12.025
Sheaves, M. (2017). How many fish use mangroves? The 75% rule an ill−defined and poorly validated concept. Fish Fish. 18, 778–789. doi: 10.1111/faf.12213
Sheaves, M., Abrantes, K., Barnett, A., Benham, C., Dale, P., Mattone, C., et al. (2020a). The consequences of paradigm change and poorly validated science: the example of the value of mangroves to fisheries. Fish Fish. 21:12479. doi: 10.1111/faf.12479
Sheaves, M., Bradley, M., Herrera, C., Mattone, C., Lennard, C., Sheaves, J., et al. (2020b). Optimising video sampling for juvenile fish surveys: using deep learning and evaluation of assumptions to produce critical fisheries parameters. Fish. Fish. 21, 1259–1276. doi: 10.1111/faf.12501
Sheaves, M., Sporne, I., Dichmont, C. M., Bustamante, R. H., Dale, P., Deng, R., et al. (2016). Principles for operationalizing climate change adaptation strategies to support the resilience of estuarine and coastal ecosystems: an Australian perspective. Mar. Policy 68, 229–240. doi: 10.1016/j.marpol.2016.03.014
Sheaves, M., Waltham, N. J., Benham, C., Bradley, M., Mattone, C., Diedrich, A., et al. (2021). Restoration of marine ecosystems: Understanding possible futures for optimal outcomes. Sci. Total Environ. 2021:148845. doi: 10.1016/j.scitotenv.2021.148845
Sievers, K. T., McClure, E. C., Abesamis, R. A., and Russ, G. R. (2020). Non−reef habitats in a tropical seascape affect density and biomass of fishes on coral reefs. Ecol. Evol. 10, 13673–13686. doi: 10.1002/ece3.6940
Silver, J. J., Gray, N. J., Campbell, L. M., Fairbanks, L. W., and Gruby, R. L. (2015). Blue economy and competing discourses in international oceans governance. J. Environ. Dev. 24, 135–160. doi: 10.1177/1070496515580797
Stewart Sinclair, P., Purandare, J., Bayraktarov, E., Waltham, N., Reeves, S., Statton, J., et al. (2020). Blue restoration – building confidence and overcoming barriers. Front. Mar. Sci. 7:748. doi: 10.3389/fmars.2020.541700
Tarte, D., and Yorkston, H. (2020). Monitoring estuarine wetlands within the Reef 2050 Integrated Monitoring and Reporting Program: Final Report of the Wetlands Expert Group. Townsville, QLD: Great Barrier Reef Marine Park Authority
Vaissière, A. C., Levrel, H., Pioch, S., and Carlier, A. (2014). Biodiversity offsets for offshore wind farm projects: the current situation in Europe. Mar. Policy 48, 172–183. doi: 10.1016/j.marpol.2014.03.023
Van Lier, J. R., Wilson, S. K., Depczynski, M., Wenger, L. N., and Fulton, C. J. (2018). Habitat connectivity and complexity underpin fish community structure across a seascape of tropical macroalgae meadows. Landsc. Ecol. 33, 1287–1300. doi: 10.1007/s10980-018-0682-4
Vander Beken, T. (2004). Risky business: A risk-based methodology to measure organized crime. Crime Law Soc. Change 41, 471–516. doi: 10.1023/b:cris.0000039599.73924.af
Villéger, S., Miranda, J. R., Hernandez, D. F., and Mouillot, D. (2010). Contrasting changes in taxonomic vs. functional diversity of tropical fish communities after habitat degradation. Ecol. Appl. 20, 1512–1522. doi: 10.1890/09-1310.1
Waltham, N. J., Elliott, M., Lee, S. Y., Lovelock, C., Duarte, C. M., Buelow, C., et al. (2020). UN decade on ecosystem restoration 2021–2030—what chance for success in restoring coastal ecosystems? Front. Mar. Sci. 7:71. doi: 10.3389/fmars.2020.00071
Keywords: coastal, decision-making, estuary, fish, framework, habitat
Citation: Sheaves M, Mattone C, Connolly RM, Hernandez S, Nagelkerken I, Murray N, Ronan M, Waltham NJ and Bradley M (2021) Ecological Constraint Mapping: Understanding Outcome-Limiting Bottlenecks for Improved Environmental Decision-Making in Marine and Coastal Environments. Front. Mar. Sci. 8:717448. doi: 10.3389/fmars.2021.717448
Received: 31 May 2021; Accepted: 26 July 2021;
Published: 20 August 2021.
Edited by:
Stefano Cannicci, The University of Hong Kong, Hong Kong, SAR ChinaReviewed by:
Francesca Porri, South African Institute for Aquatic Biodiversity, South AfricaMarilia Bueno, State University of Campinas, Brazil
Copyright © 2021 Sheaves, Mattone, Connolly, Hernandez, Nagelkerken, Murray, Ronan, Waltham and Bradley. This is an open-access article distributed under the terms of the Creative Commons Attribution License (CC BY). The use, distribution or reproduction in other forums is permitted, provided the original author(s) and the copyright owner(s) are credited and that the original publication in this journal is cited, in accordance with accepted academic practice. No use, distribution or reproduction is permitted which does not comply with these terms.
*Correspondence: Marcus Sheaves, TWFyY3VzLnNoZWF2ZXNAamN1LmVkdS5hdQ==