- 1Laboratory of Ichthyology, Department of Oceanography and Ecology, Federal University of Espírito Santo, Vitória, Brazil
- 2Programa de Pós-Graduação em Oceanografia Ambiental, Department of Oceanography and Ecology, Federal University of Espírito Santo, Vitória, Brazil
- 3Research Institute for the Environment and Livelihoods, Charles Darwin University, Darwin, NT, Australia
- 4Reef Fish Ecology and Conservation Lab, Departamento de Biologia Marinha, Universidade Federal Fluminense, Niterói, Brazil
One of the most conspicuous marks of the Anthropocene worldwide is the ubiquitous pollution by long lifespan materials (e.g., plastic). In marine habitats, anthropogenic debris are observed from floating on the surface to deposited on the substrate or ingested by wildlife at different food web levels. However, the link between feeding strategy types and debris ingestion by reef fishes remains poorly explored. We analyzed the gut contents of three nominally herbivorous fishes along the Brazilian coast: the doctorfish Acanthurus chirurgus, the parrotfish, Sparisoma axillare, and the chub Kyphosus vaigiensis. Individual [i.e., total length (TL)] and species-level functional traits, as well sites with distinct environmental features (i.e., tourism activity intensity, fishing pressure, and distance from the coast), were tested as predictors of the concentration of debris found inside individual fish guts. Debris found were quantified, measured, and classified accordingly to color and shape. We found debris in 52.7% of individuals. Debris ranged from 0.10 to 11.75 mm, and the frequency of occurrence and ingestion rate (number of debris per individual) varied among species, being higher for the scraper species S. axillare (95.7% and 4.9 ± 1.2, respectively) and A. chirurgus (74.6% and 1.64 ± 0.34), than for the browser K. vaigiensis (55.8% and 0.83 ± 0.24). TL, scraping feeding mode, and the most impacted location were positively related to debris ingestion rate. Our work revealed a higher vulnerability of an ecologically important trophic group to debris ingestion and of an already threatened species according to Brazilian red list. Besides the increasing number of species contaminated by anthropogenic debris, its effect on fish biology and physiology remains poorly understood. Understanding these links would improve conservation planning as species contamination could act as a proxy for environmental pollution on marine habitats.
Introduction
The human footprints onto the planet are recent on a geological scale but already left such clear and distinct marks on land, oceans, and atmosphere that the present epoch is now known as the Anthropocene (Lewis and Maslin, 2015). One of the most conspicuous marks of the Anthropocene is pollution by long-lasting man-made materials (e.g., plastic), affecting terrestrial and aquatic environments (Derraik, 2002), spreading through food webs, and becoming a source of contamination even to humans (Rochman et al., 2015; Cox et al., 2019). Anthropogenic debris (henceforward “debris”) reaches the oceans directly (e.g., from ships) or indirectly, carried out by rivers and runoff after being wrongfully discarded by population on land (Rech et al., 2014; Jambeck et al., 2015). Different types of debris can be found and classified as “anthropogenic” from cellulose microfibers (Remy et al., 2015; Macieira et al., 2021) to hazardous man-made petroleum-based polymers such as plastics. The durability and slow degradation of plastics allow the fragmentation of this material into smaller pieces, facilitating dispersion throughout the environment. Moreover, smaller-sized fragments can be easily ingested by smaller species from lower trophic levels (Lusher et al., 2013) and become bioavailable to higher trophic level organisms (Carpenter and Smith, 1972; Wójcik-Fudalewska et al., 2016). The contamination by microplastic in species many times used for human consumption (e.g., seafood) (Rochman et al., 2015; Forrest and Hindell, 2018), or in the air and drinking water (Cox et al., 2019) represents now a common threat to human health. Urbanization level may also influence the distribution of debris, and the amount of it may be highly correlated to the human population (Barnes, 2005; Barnes et al., 2009). Although, in the marine environment, anthropogenic pollution can be perceived on continental shores (Andrades et al., 2020), seafloor (Galgani et al., 1996; Kane et al., 2020), and isolated oceanic islands (Andrades et al., 2018). A recent study on Brazilian beaches found that 97.7% of the stranded materials were plastic made (Andrades et al., 2020). On beaches, plastic debris reduce the esthetic value of beaches (Corraini et al., 2018), causing economic losses, besides affecting wildlife through contamination following its degradation.
Reports of debris ingestion by marine wildlife have long been known (Bjorndal et al., 1994; Robards et al., 1995). From invertebrates (Remy et al., 2015; Rotjan et al., 2019) to fishes (Rochman, 2013; Garnier et al., 2019; Macieira et al., 2021) and megafauna (Besseling et al., 2015; Santos et al., 2015; Germanov et al., 2018), varied groups and trophic levels are affected by the accumulation of man-made material in the marine environment (Farrell and Nelson, 2013; Gall and Thompson, 2015; Santos et al., 2021). Species’ ecological traits and their interaction with debris may influence their susceptibility to ingestion of debris (Covernton et al., 2021; Salerno et al., 2021). For example, filter-feeding animals accumulate high amounts of micro-debris due to their adaptation to feed on plankton (Germanov et al., 2018). Although, a recent study points out that plastic ingestion by this group is less common than by species with other feeding strategies (Savoca et al., 2021). Led by ecological traits, ingestion may yet influence the types of debris found into species’ digestive tracts. Although debris’ color and shape are less likely to affect passive filter-feeders ingestion once they are unselective non-visual feeding strategy (Collard et al., 2017), the angle of approach from active feeders and the color of debris may generate selection for specific type of debris. For example, drifting debris would look darker when approached from below or against the light (and paler from above or in favor of light), influencing the color of ingested particles according to species feeding (Santos et al., 2016) and swimming habits. Additionally, debris characteristics may play a role in such interaction. Rotjan et al. (2019) demonstrate through laboratory experiments that for the coral Astrangia poculata, fiber contamination predominates over microbeads, while in the Red Sea (Baalkhuyur et al., 2018) and the Southeastern Atlantic (Macieira et al., 2021), fibers are more common than fragments for reef fishes (Baalkhuyur et al., 2018; Macieira et al., 2021). But for seabirds, however, it does not seem to be relevant (Hidalgo-Ruz et al., 2021). Notwithstanding, fibers were the most common shape of debris found in different studies worldwide (Rochman et al., 2015; Savoca et al., 2019; Macieira et al., 2021) and may take longer to be egested after ingestion by fishes. Thus, the shape, color, and size of particles may represent different degrees of exposure risks for fishes (Xiong et al., 2019). The ingestion and retention of indigestible debris pose an important threat to individual health, once it may block nutrient absorption and cause physical damages to the digestive tract (Germanov et al., 2018; Rotjan et al., 2019).
Debris tend to sink due to the increasing weight of fouling by bacteria, algae, animals, or accumulated sediment (Barnes et al., 2009), which could greatly affect species feeding closer to the substrate. For example, in a recent review, Savoca et al. (2021) found that predators and benthic foragers are more prone to the ingestion of plastic debris, having active predators ingested plastic more frequently than grazers and filter-feeding species. Herbivorous fishes are conspicuous inhabitants of tropical and subtropical reef systems and are essential in the energy flux throughout the ecosystem trophodynamics (Choat and Clements, 1998; Poore et al., 2012). Although reports of debris ingestion are widespread (Garnier et al., 2019; Rotjan et al., 2019; Covernton et al., 2021; Macieira et al., 2021), including by commercially important species (Possatto et al., 2011; Avio et al., 2015; Rochman et al., 2015; Forrest and Hindell, 2018; Cox et al., 2019), studies characterizing ingestion by herbivorous reef fishes are still rare (Rochman et al., 2015; Jabeen et al., 2017; Markic et al., 2018; Garnier et al., 2019). Marine macroalgae are known to be a vector of microplastic into marine food webs (Carpenter and Smith, 1972; Gutow et al., 2016; Goss et al., 2018), and the susceptibility of herbivores to the ingestion of debris may be related to its accumulation on inert (e.g., sediment) or living substrate (i.e., deposited on or adhered to algae), but also depending on how species interact with this substrate while foraging.
Different species of nominally herbivorous reef fishes differ in behavior and diet but also pre- and post-ingestive processes (Choat et al., 2002, 2004). These differences help to define species nutrient assimilation (Clements et al., 2009). While some are territorial farming species (Ferreira et al., 1998), feeding behavior also varies from roving herbivores that crop frondose macroalgae (i.e., macroalgivores) (Clements and Choat, 1997) to species that target turf algae and imbued detritus (i.e., detritivores) or epilithic and/or endolithic photoautotroph microorganisms (i.e., microvores) (Ferreira and Gonçalves, 2006; Clements et al., 2017). Cropping and browsing, macroalgivores would ingest algae-adhered debris. On the other hand, scraping the substrate could yet induce the ingestion of sunk debris by scrapers detritivores and microvores. Targeting different food sources (i.e., the different diets among herbivorous fishes) could directly influence the susceptibility of species to debris ingestion once it is known to accumulate in different proportions on distinct substrata (Barnes et al., 2009; Maes et al., 2017). Yet, post-ingestive processing modes in this group could influence the size and shape of debris within the gut contents due to different abrasiveness. Food processing modes range from the presence of a pharyngeal mill that highly processes their food grinding the ingested material as in parrotfishes (Clements and Bellwood, 1988) to gizzard-like muscular stomachs in detritivorous acanthurids (Choat et al., 2004) and acid stomachs with hindgut fermentation in kyphosids (Clements and Choat, 1997). In the latter two, the physical processing of food is unlikely to have enough strength to break apart more resistant materials such as plastic. As mechanical erosion could determinate debris shape (Corcoran et al., 2009), more intense physical processing would result in smaller debris found within species’ tracts.
Understanding the functional traits linked to anthropogenic debris ingestion could reveal varying susceptibility to contamination of species and functions, providing indicators for establishment and monitoring threatening status. We determined the concentration of anthropogenic debris in the gut contents of three herbivorous reef fishes with different diets and food processing modes. Also, we correlate debris concentration with species’ functional and ecological traits and environmental traits characterizing the anthropogenic impact in three locations along the Brazilian coast. We hypothesized that (1) scraping species would present more debris within their guts due to a higher interaction with the substrate, (2) species with higher post-ingestive physical processing of food would present smaller debris in the guts, and (3) specimens from highly impacted locations would present higher debris ingestion.
Materials and Methods
Study Species and Sampling
One hundred and sixty-seven individuals distributed among the three species were sampled: the doctorfish Acanthurus chirurgus (Bloch, 1787) (n = 59) – Acanthuridae, the gray parrotfish Sparisoma axillare (Steindachner, 1878) (n = 56) – Labridae, and the brassy chub Kyphosus vaigiensis (Quoy & Gaimard, 1825) (n = 52) – Kyphosidae. Although broadly known as herbivores, these species present different diets and food processing modes (Choat et al., 2002, 2004; Ferreira and Gonçalves, 2006; Mendes et al., 2018). Grazing acanthurids usually ingest particulate material (Ferreira and Gonçalves, 2006), while parrotfishes grind their food using pharyngeal mills (Clements and Bellwood, 1988), and kyphosids crop large portions of macroalgae and rely on hindgut fermentation for digestion (Clements and Choat, 1997). Also, these species differ in foraging and swimming behavior. While A. chirurgus and S. axillare are reef dwellers that usually swim and forage closer to the substratum, scraping the surface in search of macroalgae, detritus, and microscopic photoautotrophs organisms (Francini-Filho et al., 2010; Clements et al., 2017), K. vaigiensis mainly crop brown and red macroalgae and usually swim higher in the water column, preferring highly hydrodynamic sites (Cordeiro et al., 2016).
Specimens from all three species were collected at three different locations along the Brazilian coast: Natal (5°47′ S; 35°11′W), Abrolhos Archipelago (17°20′S; 39°30′W), and Arraial do Cabo (22°58′S; 42°00′W) (Supplementary Figure 1) in the austral summer of 2016 (Abrolhos Archipelago and Arraial do Cabo) and 2017 (Natal). Benthic cover in reefs close to the city of Natal is mainly composed of small patches of large-bladed macroalgae (Dictyota spp. and Dictyopteris spp.), algal turfs, and sponges located ∼10 km from the coast (Aued et al., 2018; Roos et al., 2019). Abrolhos is an archipelago distant ∼70 km off the coast that harbors the largest coral reef system within the Southwest Atlantic Ocean, interspersed with algal turfs (Leão and Dominguez, 2000). The third location, Arraial do Cabo is a subtropical rocky reef with a marked seasonal temperature variation due to the occurrence of upwelling events (Valentin, 2001; Cordeiro et al., 2016) where sampling sites were located in a inlet distancing ∼3.5 km from the city harbor, the closest from a urban center when compared to the other locations. Besides these differences, the three locations present contrast levels of offshore distance, population size, tourism intensity, and fishing activity, characteristics that represent distinct impacts (Browne et al., 2015; Silva et al., 2018; Covernton et al., 2021) that likely contribute to debris accumulation in the subtidal zone. While the sampling reefs near the city of Natal fit into no category of protection with tourism activity being mostly from diving tourism, Arraial do Cabo is a sustainable use marine protected area (i.e., fishing and tourism are allowed under management rules), and the Abrolhos Archipelago is a Marine Park where fishing is prohibited but diving tourism is allowed although in a lower intensity than in Arraial do Cabo. Although protected, the proximity of the sampling sites to the highly populated urban center of Arraial do Cabo might pose a thread and a tendency for debris accumulation in the nearby rocky reefs and, therefore, ingestion by marine fauna (Peters and Bratton, 2016). The further offshore distance of Natal could, otherwise, provide a protection from the city pollution. Plastic pollution has been linked to fishing activity through lost fishing gears (e.g., causing ghost fishing) or derelict anchoring cables (Derraik, 2002; Browne et al., 2015), and also to tourism activity (Silva et al., 2018) and distance from urban vs. urbanized beaches across the Brazilian coast (Andrades et al., 2020), affecting the amount and type of debris found. To understand the relationship between fish contamination and the impact on the environmental characteristics, we used these locations as a predictive factor in our analysis and interpreted the results based on the combination of three characteristics of each location: offshore distance in kilometers, fishing pressure, and tourism activity.
Specimens were collected using spearfishing, under Brazilian environmental permits (SISBIO 48094 and 48112) and immediately put on ice for transportation to the laboratory. Total length (TL) and total weight (TW) were measured (Table 1), and gut contents removed, frozen, and stored in alcohol 70% for subsequent analysis. Stomach contents were removed from the stomach of A. chirurgus and K. vaigiensis. As parrotfishes lack a gastric stomach (Choat et al., 2002), the content of this species was removed from the foregut, as in previous works involving such group (Choat et al., 2002, 2004; Ferreira and Gonçalves, 2006; Mendes et al., 2018). Contents were assessed for the presence of anthropogenic debris through visual examination in a sterile Petri dish under a Leica MZ6 with transmitted-light base stereomicroscope (see section “Materials and Methods”; see Markic et al., 2020), 0.63–4.0× zoom range. Although we did not use laboratory blanks as suggested by recent literature to identify possible airborne contamination (Markic et al., 2020; Savoca et al., 2021), samples were always covered with sterile Petri dish caps (except during visual analysis) to avoid such contamination by external materials. No fibers resembling our laboratory coats were observed during the analysis as reported by Rochman et al. (2015). To avoid misidentification, each debris found was verified by at least two of the authors and confirmed not to be of natural origin. All debris were measured (maximum length) using a reticle ruler attached to the stereomicroscope lens, and classified as micro (<5 mm) and meso-debris (5–25 mm) (Ryan et al., 2009). Yet, it was also classified according to color and shape (fiber or fragment), as these characteristics are known to be important in determining exposure risks for fish (Xiong et al., 2019), and may provide insights on debris’ origin.
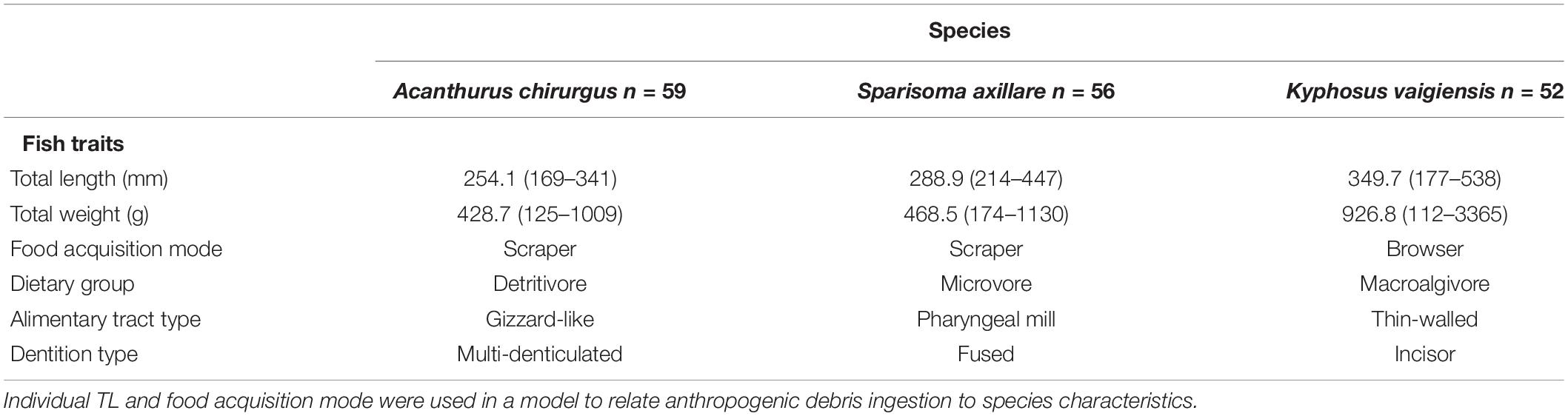
Table 1. Total (n) number of specimens, mean (range) species’ total length (TL) and weight, and ecological traits classification for each study species.
Species Traits
Individual TL, species-level functional trait food acquisition mode (scraper or browser), and species identity (ID) were tested against debris concentration inside species guts (Table 1). Study species can be classified into distinct categories according to dietary group, alimentary tract, and dentition type (Table 1). Analyzing and understanding differences among species may provide insights into the importance of functional traits among species ID.
Statistical Procedures
We used the non-parametric Kruskal–Wallis test (as data were not normally distributed) from the base R “stats” package (R Core Team, 2020), followed by Dunn’s multiple comparisons test (using Benjamin–Hochberg p-adjustment to avoid Type I errors) from “FSA” package (Ogle et al., 2019) to compare the difference in debris length among species.
The response variable in our analysis is the debris count taken from the guts of each individual fish sampled. As expected for counts, the frequency of our sample values were Poisson distributed (Supplementary Figure 2). However, prior to the application of the Poisson generalized linear model (GLM), a preliminary inspection of our data reveled that it is highly over-dispersed (dispersion parameter = 4.9) due to a large proportion of zero counts (47.3%) using “lme4” (Bates et al., 2015) and “MuMIn” (Barton, 2020) packages. Therefore, zero-inflated Poisson (ZIP) GLM were conducted using the “pscl” package (Zeileis et al., 2008) to estimate the influence of individual TL, location, and food acquisition mode on the number of debris ingested (M1) and individual TL, location, and species ID on the number of debris ingested (M2). ZIP models permit a mixture of causal factors to be evaluated and help better predict outcomes when there is a large number of zeros because of both the rarity of an event and false negatives. The ZIP models were fitted as a two-part modeling approach: one component assumes a Bernoulli distribution with a logit-link function and estimate the probability of observing a zero, and the other component assumes a Poisson distribution through a log-link function (Zuur et al., 2009). As species ID and functional trait “food acquisition mode” are collinear and precludes model convergence, we ran two different ZIP GLM model, both having the concentration of debris into species gut as the response variable. The first model (M1) compares debris concentration against food acquisition mode (two levels: scraper and browser), while the second model (M2) compares debris concentration against species (three levels: A. chirurgus, S. axillare, and K. vaigiensis).
We only included the variable location in the Bernoulli component of both models because variability in debris availability among locations is the only factor that can arguably explain the probability of false zeros. In the count component we included the body length, food acquisition mode/species ID, and location. Since all explanatory variables were significant at p < 0.05 in the M1 (Table 3), and the full model presented the best fit (Supplementary Table 1), all variables were retained in the final model. Whereas, in the M2, the explanatory variable body length was not significant at p > 0.05 (Table 4). The model selection was based on multimodel inference approach (Anderson, 2008), using the function “dredge” in the “MuMIn” R package (Barton, 2020). In multimodel inference, the models are ranked according to Akaike information criteria (AIC), delta AIC (Δi), and Akaike weights (wi) (Anderson, 2008). AIC considers not only the goodness-of-fit of a model, but also its complexity (number of parameters), and the models with the lowest AIC values are the most likely. We used the delta AIC (Δi) to select the most likely models, which were those with differences lesser than 3 among the AIC value of the given model i and the model with the lowest AIC (Δi < 3). The Akaike weights (wi) can be interpreted as probability that a certain model is the best among the set of models. The zero-inflated model was compared to ordinary Poisson and negative-binomial regression models using Vuong tests.
All statistical analysis were conducted with the R statistical language (R Core Team, 2020). Plots were produced using the base R, “ggplot2” (Wickham, 2016), “cowplot” (Wilke, 2020), and “colorspace” (Zeileis et al., 2020).
Results
Debris Abundance
From a total of the 167 specimens spanning the three species (Table 1), 88 (52.7%) contained anthropogenic debris in their digestive tracts. We found a total of 409 particles, either fibers (96.1%), or fragments (3.9%). Overall, S. axillare was the species that most ingested debris (n = 269 debris), followed by A. chirurgus (n = 97) and K. vaigiensis (n = 43). Throughout this study, we use the term “mean ingestion rate” as the number of debris per individual as in Crutchett et al. (2020). One of the main issues while comparing studies on anthropogenic debris ingestion is the wide array of units used (Collard et al., 2019). Therefore, besides mean ingestion rate, we chose to express the debris concentration into species gut contents also as the number of debris per gram of gut content and percentage of contaminated individuals (frequency of occurrence; Table 2) to facilitate future comparisons. Frequency (FO%) and mean ingestion rate (n debris per fish ± SE) were higher for S. axillare (95.7% and 4.9 ± 1.2, respectively) than A. chirurgus (74.6% and 1.64 ± 0.34) and K. vaigiensis (55.8% and 0.83 ± 0.24) (Figure 1 and Table 2).
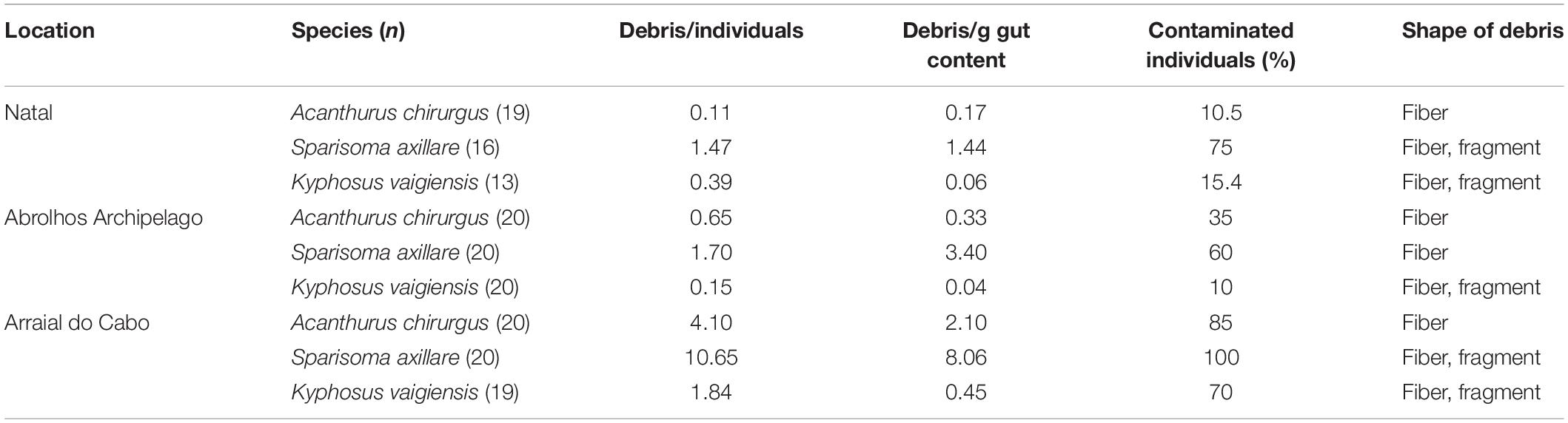
Table 2. Species and number (n) of collected individuals per location, debris per individual, debris per grams of gut content, percentage of contaminated individuals, and shape of debris found in each species gut content in each of the sampled sites.
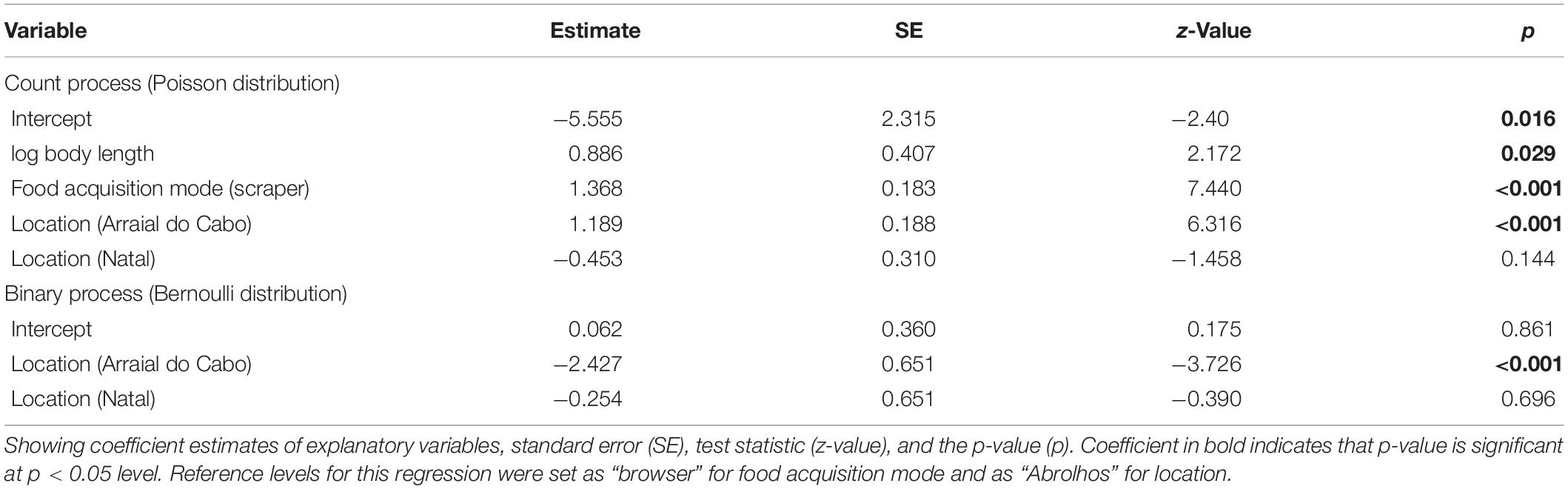
Table 3. Parameters estimates for the final zero-inflated Poisson generalized linear model for the association between traits of reef fishes, location, and number of debris ingested as the response variable.
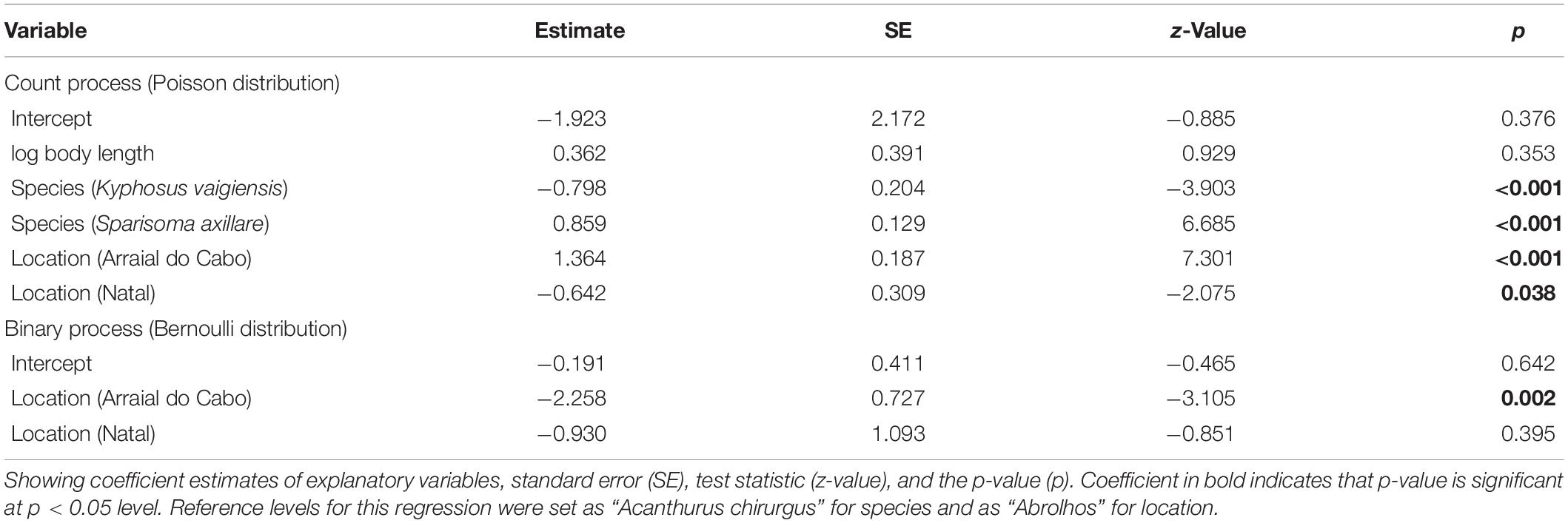
Table 4. Parameters estimates for the final zero-inflated Poisson generalized linear model for the association between different herbivorous reef fishes, location, and number of debris ingested as the response variable.
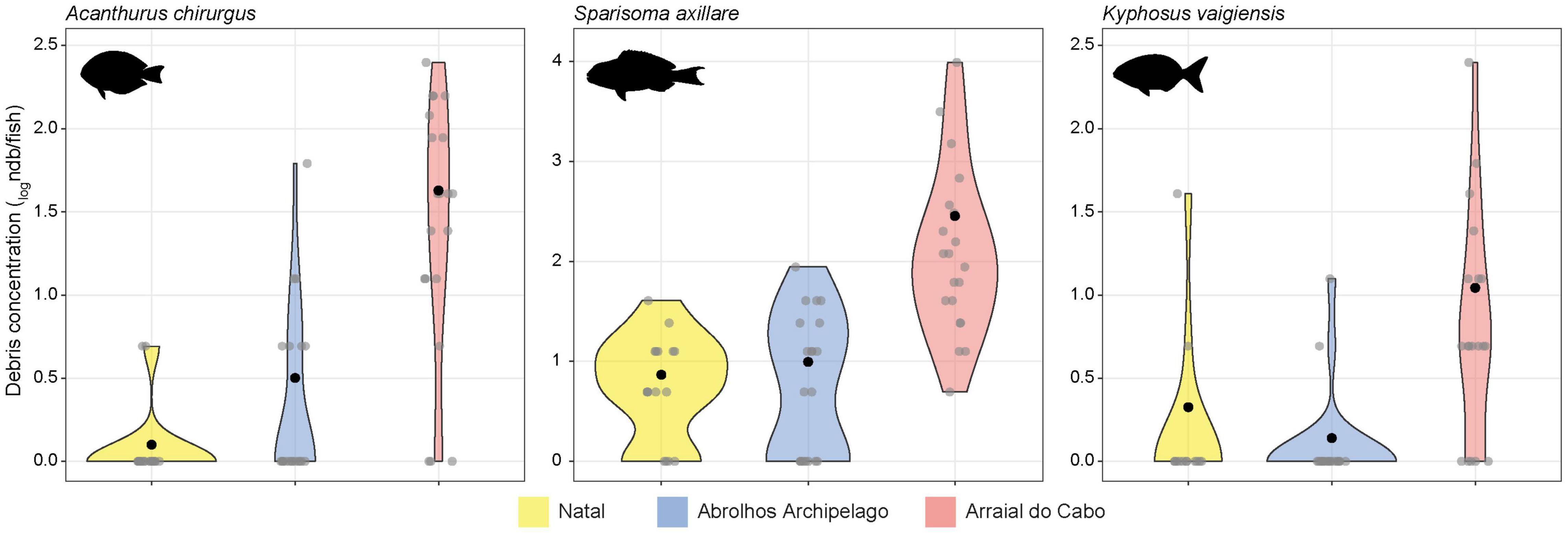
Figure 1. Comparison of debris concentration (debris per fish – ndb/fish) in the gut contents among locations for the three study species. Gray dots are each analyzed individual, and black dots are the mean for each species in each location. Values are in logarithmic scale for better visualization.
Debris Characterization
Micro- (92.4%) and meso-debris (7.6%) were found within species guts. Length of debris ranged from 0.15 to 8.5 mm (average ± SE: 1.72 ± 0.15) for A. chirurgus, 0.1–11.75 mm (1.94 ± 0.12) for S. axillare and 0.25–6.5 mm (2.26 ± 0.27) for K. vaigiensis (Figure 2). Although we expected to observe smaller debris in S. axillare due to its pharyngeal mill apparatus that grind ingested food, it seemed not to be able to break down ingested debris. No difference in debris length among species was found at any location (Kruskal–Wallis test: p > 0.05).
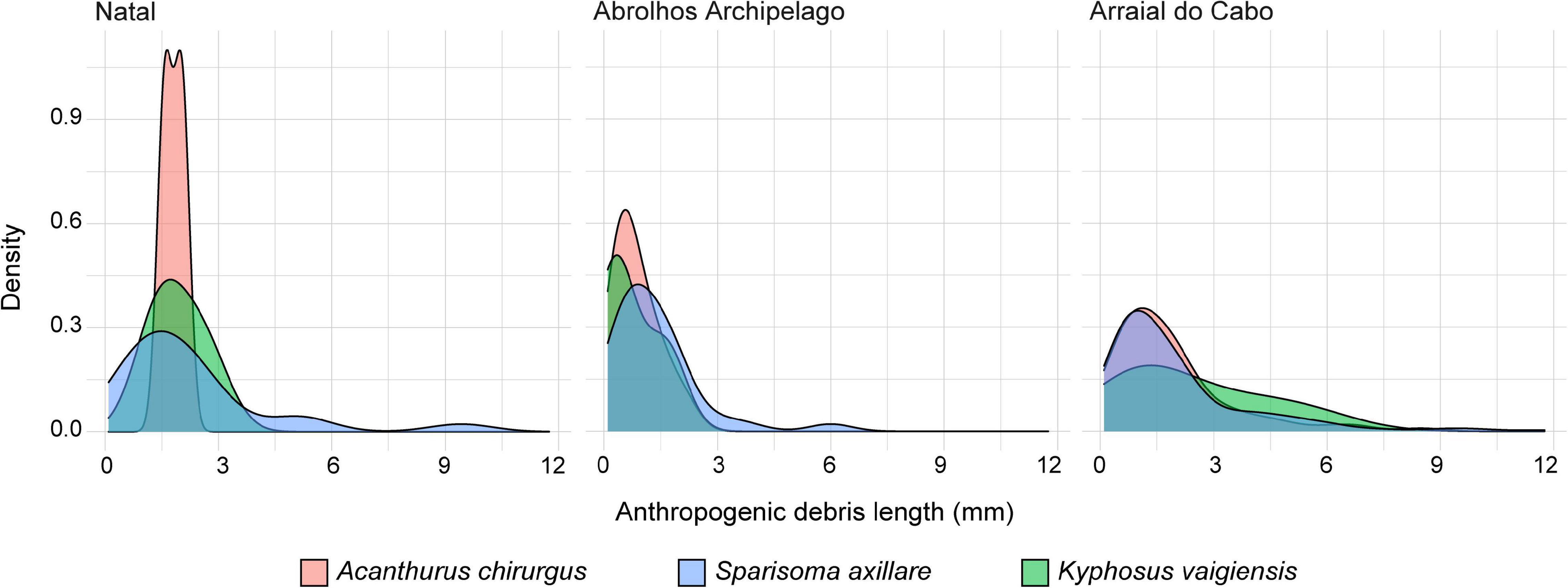
Figure 2. Density plot of the length of the anthropogenic debris found in the species’ gut contents sampled at each location.
We found different colors of debris in the species gut contents: blue (52.6%), black (36.9%), red (5.9%), colorful (particles containing different colors in a single piece; 2.7%), transparent (1.5%), and green (0.5%). The distribution of the colors varied among locations (Supplementary Figure 2), being mostly black in Natal (55.2%) and Abrolhos (56%), but blue (54.9%) in Arraial do Cabo. The blue color also predominates in the contents of A. chirurgus (60.8%) and K. vaigiensis (88.4%), while both black and blue represent the same percentage in the gut contents of S. axillare (43.9%). The fibers and fragments found in the species from Arraial do Cabo had the highest color variation. S. axillare was the species with more different colors (blue, black, red, colorful, transparent, and green). Blue fibers found in the gut contents were similar to fishing/boating gears parts (e.g., ropes) and to debris found beached and on the reef bottom at one of the locations, Arraial do Cabo (Figure 3).
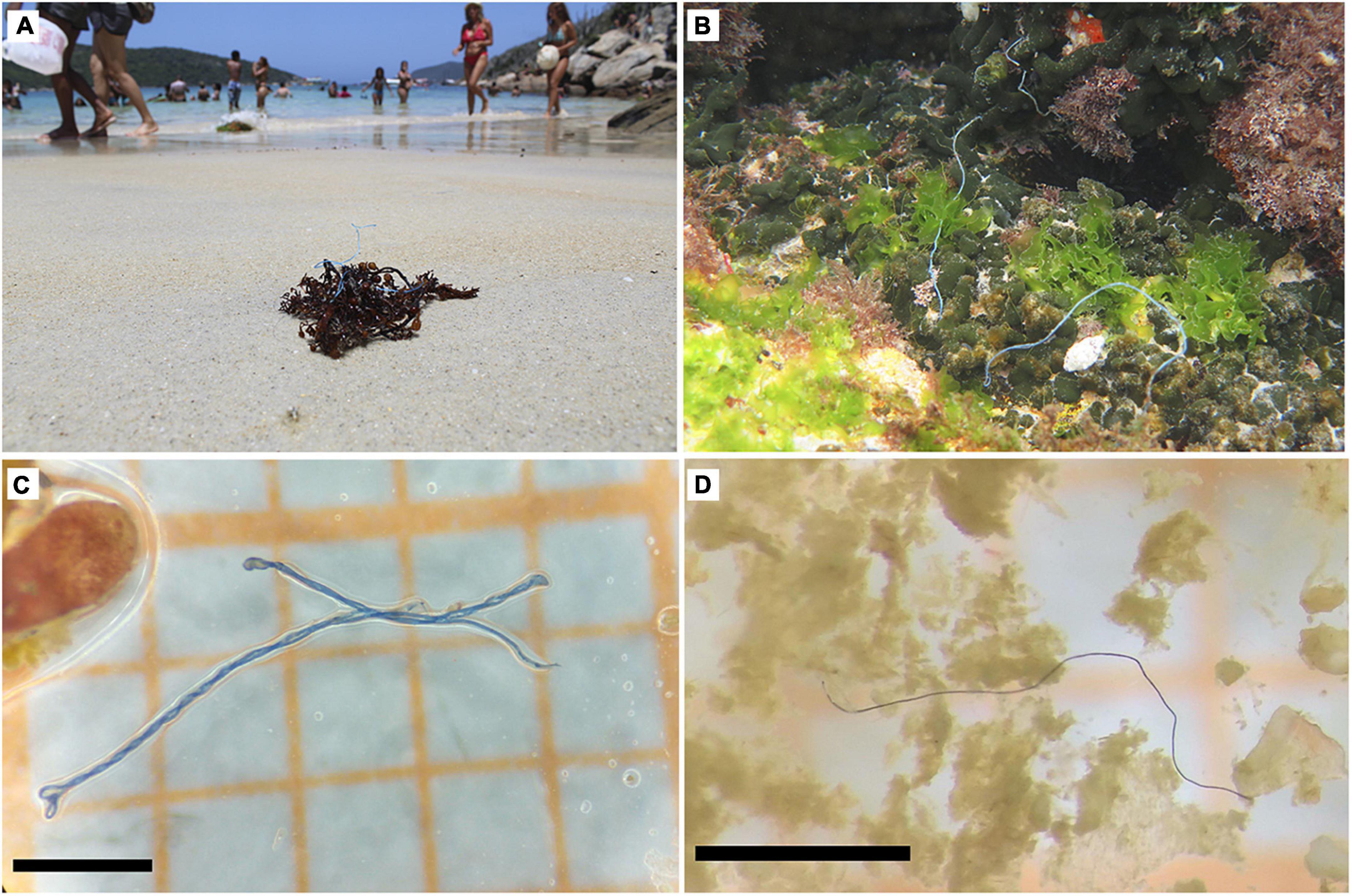
Figure 3. Similarity between fibers observed in the wild – attached to algae stranded on the beach (A) and on the reefs (B) of one of the sampling locations (Arraial do Cabo) – and found in the stomach contents of study species (C,D). Black scale bars in C,D represent 1 mm length. Photos by (A) Ryan Andrades and (B) Larissa J. Benevides.
Species and Environmental Traits vs. Debris Ingestion
Both the ZIP models were a significant improvement over the standard Poisson model (M1: Vuong test-statistic = 2.314, p = 0.01; M2: Vuong test-statistic = 1.922, p = 0.03) indicating statistically significant predictors in the part of the logit model predicting excessive zero. The ZIP GLM M1 revealed a positive and significant relationship between body length and scraper feeding mode with the number of debris ingested (Table 3 and Figure 4). The bigger the individual, higher was the number of debris found inside their guts. Moreover, species foraging closer to the substrate are likely to ingest more debris. In the ZIP GLM M2, the browser species K. vaigiensis was negatively significant with the number of debris, while the scraper S. axillare was positively related to debris concentration (Table 4 and Figure 5). However, body length did not show the same significance in the M2 as in the M1. Samples from Arraial do Cabo, where fishing pressure and tourism activity are more intense, had significant larger number of debris ingested at both models. The Bernoulli component of the model also indicated that Arraial do Cabo had a lower probability of zero values. Yet, in the M2, the location Natal showed a negative and significant relationship with debris concentration into species’ gut. Although we have not measured the amount of debris found in each site, this is likely a result of higher availability of debris in the subtidal habitats of Arraial do Cabo than in Natal and Abrolhos.
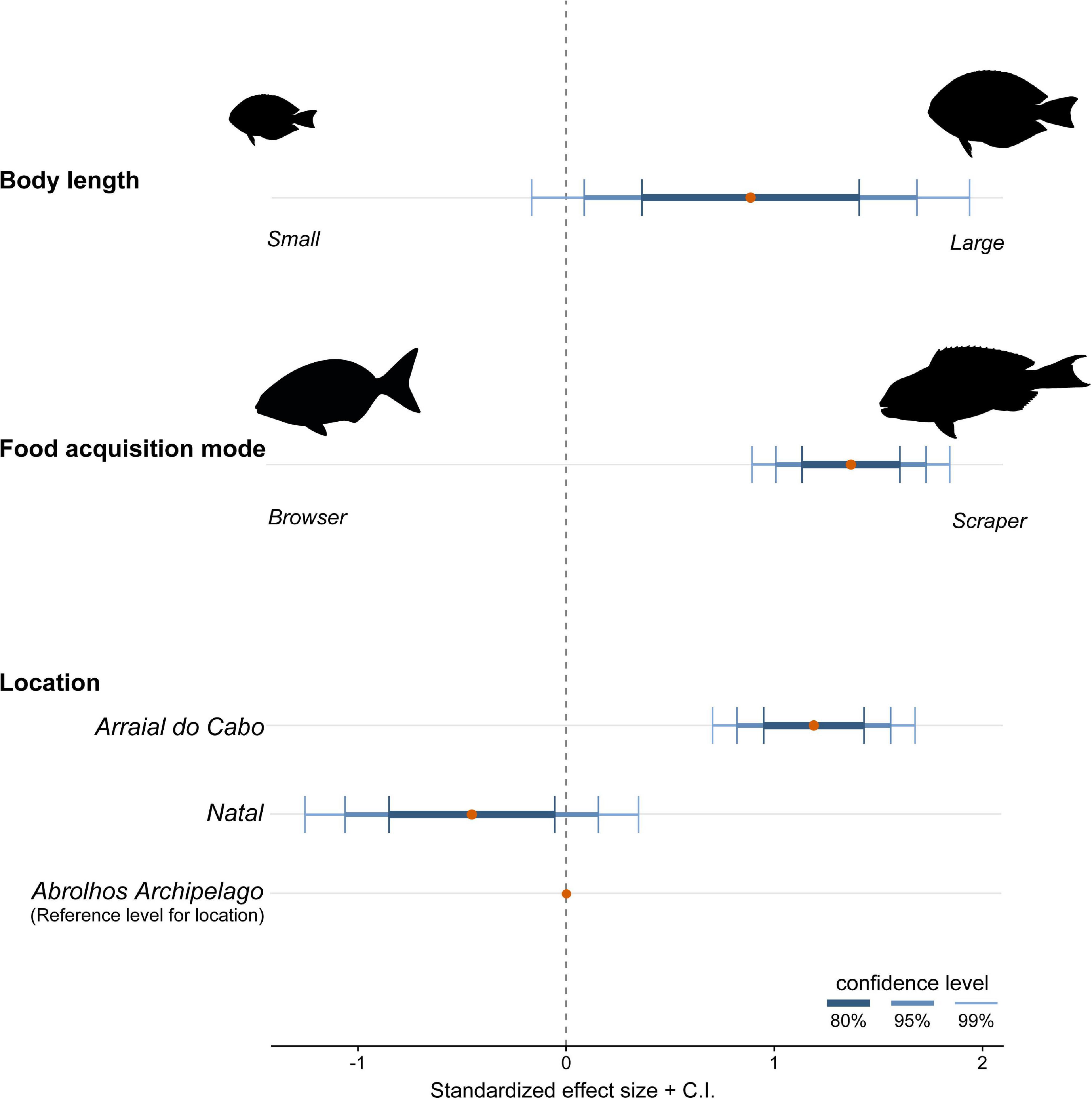
Figure 4. Effect sizes and confidence interval at three levels (80, 95, and 99%) of significance resulted from the zero-inflated Poisson generalized linear model testing the effect of body length, food acquisition mode, and location on the number of debris ingested.
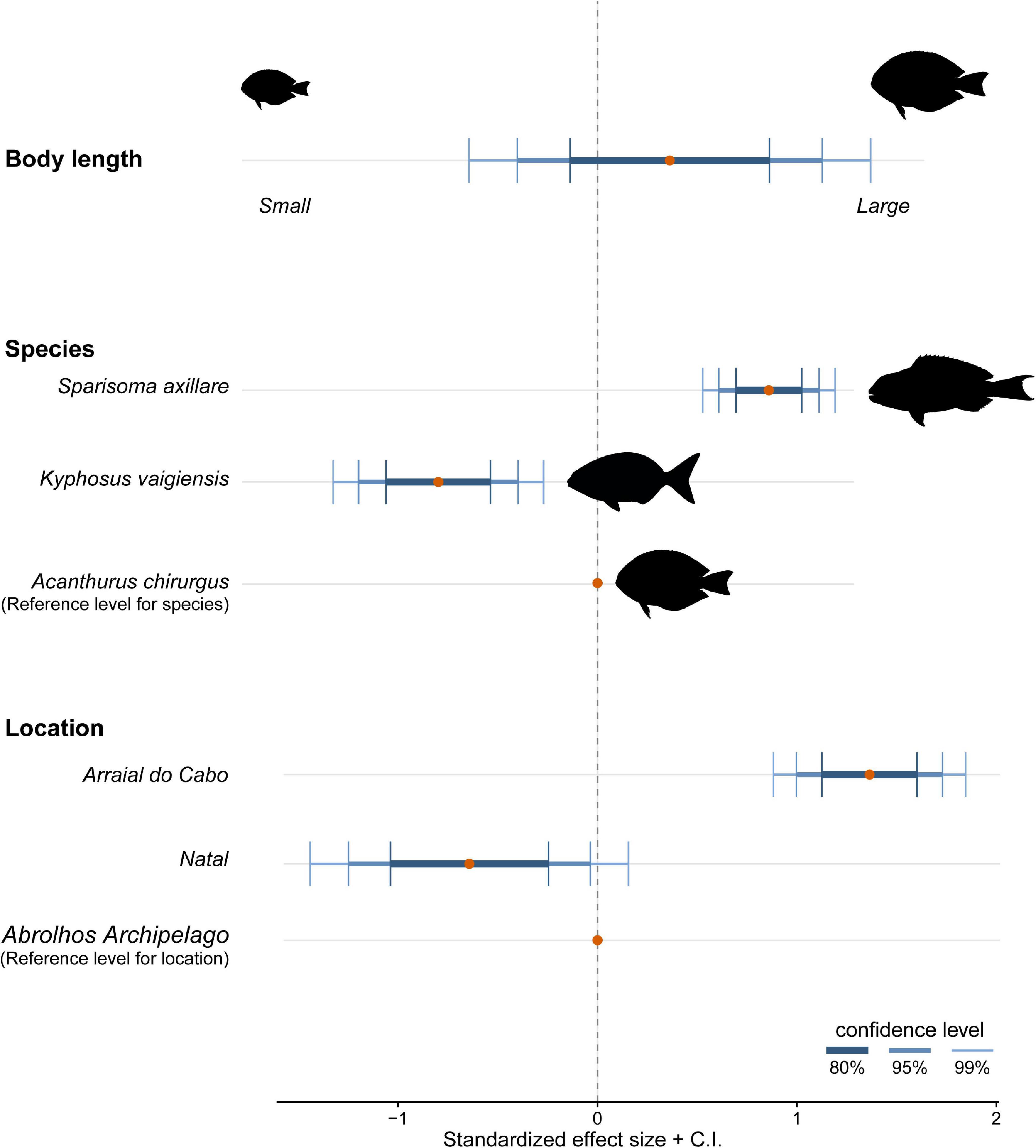
Figure 5. Effect sizes and confidence interval at three levels (80, 95, and 99%) of significance resulted from the zero-inflated Poisson generalized linear model testing the effect of body length, species ID, and location on the number of debris ingested.
Discussion
Species from multiple trophic levels, with different feeding behaviors and mobility, ingest or negatively interact with anthropogenic debris. In the marine environment, from sessile invertebrates such as corals (Hall et al., 2015; Rotjan et al., 2019) to megafauna as mobulid rays and whale sharks (Germanov et al., 2018), different species were reported to contain plastic debris within their digestive tracts. How wildlife interacts with litter in the oceans influences their susceptibility to ingest it (Wright et al., 2013; Collard et al., 2017). Here, we identified a link between the species’ functional traits and the anthropogenic debris ingestion by three species of herbivorous reef fishes. Both scraper species A. chirurgus and S. axillare, which feed scraping off the substrate while ingesting endolithic microalgae and a diversity of components from the epilithic algal matrix (detritus, sediment loads, and associated flora and fauna), showed higher ingestion of debris. The browser species K. vaigiensis differently, feed by cropping macroalgae and turfs, barely ingesting any detritus and sediment (Mendes et al., 2018), and ingested lower amount of debris. Comparatively, all specimens collected in Arraial do Cabo, a marine extractive reserve known to have areas of multiple use by tourism and fisheries, were the ones that showed higher ingestion of anthropogenic debris, likely because of higher availability (lower probability of zero counts). Although we have not accessed levels of contamination on each environment, the large proportion of ingested debris from all species and functional groups at a single location may be a proxy for a higher site-specific contamination. Thus, these species may act as bio-indicators for marine pollution, where the higher susceptibility of scrapers set them as better than browsers at this task, as expected.
Traits have become the central component of the growing area of functional or trait−based ecology, relating to the performance (growth rate, survival, reproduction) of an organism and/or its contribution to ecological processes (Luiz et al., 2019). Much of the popularity that trait−based ecology has gained is because it facilitates generalization across ecological communities with few species in common, but similar characteristics (McGill et al., 2006). For example, intrinsic species-level traits play an important role in species’ susceptibility to ingestion of debris (Salerno et al., 2021). Filter feeding strategies, for example, may expose animals to microplastic ingestion (Germanov et al., 2018, 2019). However, benthic-foraging species could be more susceptible to it as plastic fibers are known to be more common in the sediment than floating on surface waters due to biofouling (Barnes et al., 2009; Maes et al., 2017). Contamination typically occurs directly as particles are mistaken for food or indirectly by chance during ingestion of prey that consumed debris or is coated with them (Wieczorek et al., 2018). The latter process is most likely for herbivorous fishes, as they can ingest debris known to deposit or adhere to macroalgae (Gutow et al., 2016; Goss et al., 2018).
Species with different diets may exhibit different concentrations of ingested debris, once the alimentary tract could represent a secondary role in the ingested debris. Pharyngeal mill presence, gizzard-like, and thin-walled stomachs could represent different mechanical processing of ingested debris, reducing its size. Notwithstanding, species with thin-walled stomachs that feed exclusively cropping over macroalgae tend to have longer guts and lower feeding rates (Horn, 1989), which could explain a lower intake of debris in some groups such as our browser species K. vaigiensis (Table 4 and Figure 5). However, Macieira et al. (2021) found no debris within grazing parrotfishes in the southeastern Brazilian coast while invertebrates’ feeders were the ones with higher ingestion. In accordance, Savoca et al. (2021) found that grazing species (including acanthurids, parrotfishes, and kyphosids) ingested plastic less frequently than active predators. Meanwhile, Mizraji et al. (2017) found omnivores to have higher ingestion rates due to wider diet sources when compared to herbivores and carnivores. Although we haven’t compared different trophic categories, acanthurids, parrotfishes and kyphosids do differ in diet (Ferreira and Gonçalves, 2006; Mendes et al., 2018), and were expected to present different levels of debris ingestion.
Different feeding habits are likely to represent the major influence in debris ingestion by these groups. Morphological and physiological characteristics seem to play an important role in debris ingestion. For example, by cropping macroalgae while feeding (Clements and Choat, 1997), K. vaigiensis may have avoided higher concentrations of debris on the sediment, contrarily to A. chirurgus and S. axillare that scrape the substrate while feeding and have higher ingestion of debris possibly sunk and imbued within sediment and food sources. Yet, higher ingestion rates by S. axillare when compared to A. chirurgus might be carried by morphological differences such as dentition type. Beak-like morphology of parrotfishes’ dentition and mouth may propitiate higher ingestion of food-associated sediment and detritus, while some acanthurids have teeth that remove only detritus by brushing epilithic and turf algae (e.g., Ctenochaetus striatus; Purcell and Bellwood, 1993), others (as A. chirurgus) have multi-denticulated teeth that remove the turf algae as well (Mendes et al., 2018). Although we did not directly measured mouth size, it allometrically correlates with body size (Karpouzi and Stergiou, 2003), and species with wider mouth opening (e.g., larger individuals) is likely to reflect in different debris ingestion by species. Thus, larger individuals were expected to ingest more food per bit and, therefore, more anthropogenic debris (Figure 4). Notwithstanding, comparisons among species from different sites must be done carefully, as food targeting also differ. We recommend that further studies comparing debris concentration adhered or deposited among different substrata (i.e., frondose vs. turf algae and sediment) could better clarify the relationship between debris availability and ingestion by different herbivorous species and families.
Mostly, the ingestion rates found here for the three species (Table 2) were higher than for other marine herbivorous fishes reported from literature. For example, Siganus spp., a herbivore browser similar to kyphosids (Hoey et al., 2013), showed a concentration of 0.3–0.5 debris/fish in Indonesia (Rochman et al., 2015), but only of 0.15 debris/fish in French Polynesia (Garnier et al., 2019). Although our kyphosid showed lower ingestion rates comparing to the other species, these rates ranged from 0.15 to 1.84 debris/fish, mostly higher than previous registered. Contrarily, Markic et al. (2018) found ingestion rates higher in Kyphosus sandwicensis (junior synonym of K. elegans sensu Knudsen and Clements, 2013) than in acanthurids and parrotfishes in the South Pacific. However, the K. elegans individuals analyzed were present in a single location where acanthurids and parrotfishes were not collected, making difficult the comparison among species.
Fibers were the most common type found here and are also the most common shape type within microplastics (Remy et al., 2015) and fragments found in the marine environment or retrieved from examined species worldwide (Markic et al., 2020). Conversely, recent studies examining reef fishes gut contents commonly find higher amounts of fibers and artificial cellulose microfibers, possibly due to the proximity of reef environments to high populated areas (Macieira et al., 2021). These fibers may originate from washing machines that can produce more than 1900 particles per wash of a single garment of synthetic clothing (Browne et al., 2011), but also from fishing gears through lost and/or deterioration of these materials (Walker et al., 1997). Rochman et al. (2015) and Markic et al. (2018) argue that the difference in the shape of debris ingested by species among locations is linked to a more efficient wastewater management in more urbanized areas. The lack of proper waste collection and management increase solid waste disposal in the environment, being responsible for the higher number of fragments instead of fibers, which are more common in locations where treatment stations exist (Rochman et al., 2015; Markic et al., 2018), and could reflect on the debris found within species’ guts. However, monotony in debris color (black, blue, and a few red, almost exclusively of bright and vibrant whites, tones of gray, yellows, browns, and other colors typical of clothing) strongly argues against the household origin. In fact, black and blue are dominant in many other studies (Mizraji et al., 2017; Baalkhuyur et al., 2018) as were here. A recent study suggests that color selection, if any, carries deep explanations for the variation in color debris in marine species (Santos et al., 2016). The authors expand the theory of protective coloration (Thayer, 1896) and conclude that species approaching food from above (e.g., sea birds) would tend to ingest paler debris, while those reaching food from below (e.g., sea turtles) are likely to ingest darker ones. This is a neat idea that may explain much of what happens in open waters but fails at explaining benthic selection. In another hand, the dominance of clear blue fibers in our samples and of a downward approach of fishes to food and debris do not allow us to test Santos et al.’s (2016) concept. We advocate for an origin linked to boating and fishing, in particular, mooring and anchoring lines for which blue and black are dominant and the wear expressive (pers. obs.). However, because ground-trusted availability was not performed and plastic composition not accessed, determining fibers origin will require further investigation.
Many factors may influence the different concentration of debris among locations, such as environmental availability. For example, Markic et al. (2018) linked the higher debris ingestion of K. elegans to the pollution in one of their sampled locations, the island of Rapa Nui, situated within the South Pacific “garbage patch,” an area known for its high accumulation of marine debris (Eriksen et al., 2013). Among studied sites, fishing intensity, tourism activity, and distance from the coast are environmental traits that could relate to pollution and, therefore, debris ingestion. For three species analyzed, ingestion rate was higher in individuals from Arraial do Cabo. This location is the closest from a urban center within sampling locations, established as an extractive reserve with multiple use areas, with different fishing gears used, intense aquatic tourism, and being one of the most visited dive sites on the southeastern Brazilian coast (Giglio et al., 2017). Fishing is a well-known issue related to plastic pollution (Richards, 1994; Derraik, 2002; Copello and Quintana, 2003), contributing with lost fishing tackles that would harm wildlife by entanglement or ghost-fishing (Browne et al., 2015) but also by the deterioration of anchoring cables. The littering on beaches was already registered and linked to tourism activity in Arraial do Cabo (Silva et al., 2018), with plastic pollution representing the main source pollution at different urban and urbanized beaches across the Brazilian coast (Andrades et al., 2020). It poses a threat to wildlife in the region, as the reported debris ingestion by marine sea turtles (Awabdi et al., 2013; Lima et al., 2018), but also negatively impact the local community that economically relies on the scenic quality of beaches (Silva et al., 2018; Andrades et al., 2020).
Worldwide records of debris ingestion by fishes vary in concentration according to study sites, feeding strategy, and the methodology used (Markic et al., 2020; Covernton et al., 2021). Although few studies had demonstrated debris ingestion by reef fishes (Rochman et al., 2015; Mizraji et al., 2017; Forrest and Hindell, 2018; Markic et al., 2018; van der Hal et al., 2018; Garnier et al., 2019), this contamination is worrisome for an environment already heavily threatened by anthropogenic impacts (Hughes et al., 2017). Markic et al. (2018) rises the possibility of kyphosids being an important group to be considered in biomonitoring programs and that it should not be pooled with other species in debris’ ingestion studies. We agree that not only families but species should be considered as a single unit in such studies, and not be pooled. However, worth to highlight that generalizations around Kyphosidae family from Markic et al. (2018) must be analyzed carefully, as the species Girella tricuspidata (classified by them as a herbivore grazer from the Kyphosidae family) actually belongs to the Girellidae family (Knudsen and Clements, 2013; Knudsen et al., 2019) and is an omnivore that feeds mainly on a mixture of algae, detritus, and small crustaceans (Clements and Choat, 1997). We did not find higher ingestion rate for kyphosids. Instead, the species with higher debris ingestion was the gray parrotfish S. axillare, an already threatened Brazilian endemic species (VU – ICMBIO, 2021). Feeding on photoautotroph microorganisms, plus their adaptation to retain such smaller food items (Clements et al., 2017) while also ingesting high sediment loads, are indicatives that parrotfishes could be a priority group for biomonitoring debris contamination. When debris contamination are soon detected in vulnerable species, it might help monitoring programs to prevent drastic demographic effects (i.e., a reduction in population size) due long-term effects of debris ingestion (Critchell and Hoogenboom, 2018).
The consequences of anthropogenic debris ingestion go from intestinal lesions and obstruction (Ahrendt et al., 2020) to intoxication (Rochman et al., 2013). It might lead to decreasing strength and death by predation or starvation to contamination by external microbes from biofouling and missing nutritional opportunities (Boerger et al., 2010; Rotjan et al., 2019; Machovsky-Capuska et al., 2020; Santos et al., 2020). Thus, the ingestion of indigestible debris may block nutrient absorption and cause mechanical damage to the intestinal tract in fishes (Germanov et al., 2018), especially those with sharp instead of rounded edges (Pirsaheb et al., 2020). Yet, anthropogenic debris may sorb and accumulate contaminants and become highly toxic (Barnes et al., 2009; Cole et al., 2013; Setälä et al., 2014), acting as disruptors of the endocrine system through plastic-derived estrogen mimics released from aqueous systems into the environment (LaFleur and Schug, 2011). However, bioaccumulation and biomagnification studies yield controversial results. While bioaccumulation within trophic levels seem to occur more commonly (Collard et al., 2017; Miller et al., 2020), and is possible through different pathways (Santos et al., 2021), the biomagnification across food webs remains to be confirmed (Miller et al., 2020). Hence, careful must be taken while analyzing results of biomagnification and trophic transfer, preferably using realistic contaminants concentrations as could be found in the wild (Miller et al., 2020; Savoca et al., 2021). Whether biomagnification occurs or not, identifying community traits linked to debris concentration is urgent for understanding contamination throughout food webs and the different susceptibility of wildlife taxa to litter ingestion (McNeish et al., 2018).
Here we compared for the first time the debris ingestion rate among species with similar but different diets, and identified ecological traits positively related to higher number of debris (i.e., scraping food acquisition mode). Our results indicate that feeding strategy do influence species’ susceptibility to debris ingestion and support previous studies with similar results (e.g., Mizraji et al., 2017; Germanov et al., 2018), but also highlight the importance of species-specific trait analyses in order to improve and better design conservation planning while identifying vulnerable groups to anthropogenic litter ingestion (Santos et al., 2016). Additionally, studies embracing a wider number of taxa with distinctive assemblages and traits will provide a full comprehension of how and which groups are more susceptible to debris ingestion. Understanding these links would help to improve the conservation planning for the marine environment as some groups act better than others as biomonitoring tools.
Data Availability Statement
The raw data supporting the conclusions of this article will be made available by the authors, without undue reservation.
Ethics Statement
The animal study was reviewed and approved by SISBIO – Sistema de Autorização e Informação em Biodiversidade.
Author Contributions
GC-F designed the study. GC-F, LB, and CF conducted the fieldwork. TC and GC-F performed the laboratory analysis. GC-F and OL performed the statistical analysis. CF and J-CJ provided the financial support. All authors contributed to data interpretation, critical revision, and approved the submitted version.
Funding
This work was supported through grant from CNPq (401908/2013-5) to CF. TC was supported by undergraduate from CNPq. CF was continuing supported by grants from CNPq and FAPERJ.
Conflict of Interest
The authors declare that the research was conducted in the absence of any commercial or financial relationships that could be construed as a potential conflict of interest.
Publisher’s Note
All claims expressed in this article are solely those of the authors and do not necessarily represent those of their affiliated organizations, or those of the publisher, the editors and the reviewers. Any product that may be evaluated in this article, or claim that may be made by its manufacturer, is not guaranteed or endorsed by the publisher.
Supplementary Material
The Supplementary Material for this article can be found online at: https://www.frontiersin.org/articles/10.3389/fmars.2021.717435/full#supplementary-material
References
Ahrendt, C., Perez-Venegas, D. J., Urbina, M., Gonzalez, C., Echeveste, P., Aldana, M., et al. (2020). Microplastic ingestion cause intestinal lesions in the intertidal fish Girella laevifrons. Mar. Pollut. Bull. 151:110795. doi: 10.1016/j.marpolbul.2019.110795
Anderson, D. R. (2008). in Model Based Inference in the Life Sciences: a Primer on Evidence, 1st Edn. New York: Springer Science & Business Media.
Andrades, R., Pegado, T., Godoy, B. S., Reis-Filho, J. A., Nunes, J. L. S., Grillo, A. C., et al. (2020). Anthropogenic litter on Brazilian beaches: baseline, trends and recommendations for future approaches. Mar. Pollut. Bull. 151:110842. doi: 10.1016/j.marpolbul.2019.110842
Andrades, R., Santos, R. G., Joyeux, J., Chelazzi, D., Cincinelli, A., and Giarrizzo, T. (2018). Marine debris in Trindade Island, a remote island of the South Atlantic. Mar. Pollut. Bull. 137, 180–184. doi: 10.1016/j.marpolbul.2018.10.003
Aued, A. W., Smith, F., Quimbayo, J. P., Cândido, D. V., Longo, G. O., Ferreira, C. E. L., et al. (2018). Large-scale patterns of benthic marine communities in the Brazilian Province. PLoS One 13:e0198452. doi: 10.1371/journal.pone.0198452
Avio, C. G., Gorbi, S., and Regoli, F. (2015). Experimental development of a new protocol for extraction and characterization of microplastics in fish tissues: first observations in commercial species from Adriatic Sea. Mar. Environ. Res. 111, 18–26. doi: 10.1016/j.marenvres.2015.06.014
Awabdi, D. R., Siciliano, S., and Di Beneditto, A. P. M. (2013). First record of killer whales (Orcinus orca) contents of juvenile green turtles, Chelonia mydas, in Rio de Janeiro, south-eastern Brazil. Mar. Biodivers. Rec. 6, 1–6. doi: 10.1017/S1755267212001029
Baalkhuyur, F. M., Bin Dohaish, E.-J. A., Elhalwagy, M. E. A., Alikunhi, N. M., AlSuwailem, A. M., Røstad, A., et al. (2018). Microplastic in the gastrointestinal tract of fishes along the Saudi Arabian Red Sea coast. Mar. Pollut. Bull. 131, 407–415. doi: 10.1016/j.marpolbul.2018.04.040
Barnes, D. K. A. (2005). Remote islands reveal rapid rise of southern hemisphere sea debris. Sci. World J. 5, 915–921. doi: 10.1100/tsw.2005.120
Barnes, D. K. A., Galgani, F. F., Thompson, R. C., and Barlaz, M. (2009). Accumulation and fragmentation of plastic debris in global environments. Philos. Trans. R. Soc. Lond. B. Biol. Sci. 364, 1985–1998. doi: 10.1098/rstb.2008.0205
Barton, K. (2020). MuMIn: multi-Model Inference. R package version 1.43.17. Version 1, 18. Available online at: https://r-forge.r-project.org/R/?group_id=346 (accessed July 14, 2021).
Bates, D., Mächler, M., Bolker, B., and Walker, S. (2015). Fitting linear mixed-effects models using lme4. J. Stat. Softw. 67:115. doi: 10.18637/jss.v067.i01
Besseling, E., Foekema, E. M., Van Franeker, J. A., Leopold, M. F., Kühn, S., Bravo Rebolledo, E. L., et al. (2015). Microplastic in a macro filter feeder: humpback whale Megaptera novaeangliae. Mar. Pollut. Bull. 95, 248–252. doi: 10.1016/j.marpolbul.2015.04.007
Bjorndal, K. A., Bolten, A. B., and Lagueux, C. J. (1994). Ingestion of marine debris by juvenile sea turtles in coastal Florida habitats. Mar. Pollut. Bull. 28, 154–158. doi: 10.1016/0025-326X(94)90391-3
Boerger, C. M., Lattin, G. L., Moore, S. L., and Moore, C. J. (2010). Plastic ingestion by planktivorous fishes in the North Pacific Central Gyre. Mar. Pollut. Bull. 60, 2275–2278. doi: 10.1016/j.marpolbul.2010.08.007
Browne, M. A., Crump, P., Niven, S. J., Teuten, E. L., Tonkin, A., Galloway, T., et al. (2011). Accumulation of microplastic on shorelines woldwide: sources and sinks. Environ. Sci. Technol. 45, 9175–9179. doi: 10.1021/es201811s
Browne, M. A., Underwood, A. J., Chapman, M. G., Williams, R., Thompson, R. C., and Van Franeker, J. A. (2015). Linking effects of anthropogenic debris to ecological impacts. Proc. R. Soc. B Biol. Sci. 282. doi: 10.1098/rspb.2014.2929
Carpenter, E. J., and Smith, K. L. (1972). Plastics on the Sargasso Sea surface. Science 175, 1240–1241. doi: 10.1126/science.175.4027.1240
Choat, J. H., and Clements, K. D. (1998). Vertebrate herbivores in marine and terrestrial environments: a nutritional ecology perspective. Annu. Rev. Ecol. Syst. 29, 375–403. doi: 10.1146/annurev.ecolsys.29.1.375
Choat, J. H., Clements, K. D., and Robbins, W. D. (2002). The trophic status of herbivorous fishes on coral reefs: 1. Dietary analyses. Mar. Biol. 140, 613–623. doi: 10.1007/s00227-001-0715-3
Choat, J. H., Robbins, W. D., and Clements, K. D. (2004). The trophic status of herbivorous fishes on coral reefs: II. Food processing modes and trophodynamics. Mar. Biol. 145, 445–454. doi: 10.1007/s00227-004-1341-7
Clements, K. D., and Bellwood, D. R. (1988). A comparison of the feeding mechanisms of two herbivorous labroid fishes, the temperate Odax pullus, and the tropical Scarus rubroviolaceus. Aust. J. Mar. Freshw. Res. 39, 87–107. doi: 10.1071/MF9880087
Clements, K. D., and Choat, J. H. (1997). Comparison of herbivory in the closely-related marine fish genera Girella and Kyphosus. Mar. Biol. 127, 579–586. doi: 10.1007/s002270050048
Clements, K. D., German, D. P., Piché, J., Tribollet, A., and Choat, J. H. (2017). Integrating ecological roles and trophic diversification on coral reefs: multiple lines of evidence identify parrotfishes as microphages. Biol. J. Linn. Soc. 120, 729–751. doi: 10.1111/bij.12914
Clements, K. D., Raubenheimer, D., and Choat, J. H. (2009). Nutritional ecology of marine herbivorous fishes: ten years on. Funct. Ecol. 23, 79–92. doi: 10.1111/j.1365-2435.2008.01524.x
Cole, M., Lindeque, P., Fileman, E., Halsband, C., Goodhead, R., Moger, J., et al. (2013). Microplastic ingestion by zooplankton. Environ. Sci. Technol. 47, 6646–6655. doi: 10.1021/es400663f
Collard, F., Gasperi, J., Gabrielsen, G. W., and Tassin, B. (2019). Plastic Particle Ingestion by Wild Freshwater Fish: a Critical Review. Environ. Sci. Technol. 53, 12974–12988. doi: 10.1021/acs.est.9b03083
Collard, F., Gilbert, B., Eppe, G., Roos, L., Compère, P., Das, K., et al. (2017). Morphology of the filtration apparatus of three planktivorous fishes and relation with ingested anthropogenic particles. Mar. Pollut. Bull. 116, 182–191. doi: 10.1016/j.marpolbul.2016.12.067
Copello, S., and Quintana, F. (2003). Marine debris ingestion by Southern Giant Petrels and its potential relationships with fisheries in the Southern Atlantic Ocean. Mar. Pollut. Bull. 46, 1513–1515. doi: 10.1016/S0025-326X(03)00312-6
Corcoran, P. L., Biesinger, M. C., and Grifi, M. (2009). Plastics and beaches: a degrading relationship. Mar. Pollut. Bull. 58, 80–84. doi: 10.1016/j.marpolbul.2008.08.022
Cordeiro, C. A. M. M., Mendes, T. C., Harborne, A. R., and Ferreira, C. E. L. (2016). Spatial distribution of nominally herbivorous fishes across environmental gradients on Brazilian rocky reefs. J. Fish Biol. 89, 939–958. doi: 10.1111/jfb.12849
Corraini, N. R., de Souza de Lima, A., Bonetti, J., and Rangel-Buitrago, N. (2018). Troubles in the paradise: litter and its scenic impact on the North Santa Catarina island beaches. Brazil. Mar. Pollut. Bull. 131, 572–579. doi: 10.1016/j.marpolbul.2018.04.061
Covernton, G. A., Davies, H. L., Cox, K. D., El-Sabaawi, R., Juanes, F., Dudas, S. E., et al. (2021). A Bayesian analysis of the factors determining microplastics ingestion in fishes. J. Hazard. Mater. 413:125405. doi: 10.1016/j.jhazmat.2021.125405
Cox, K. D., Covernton, G. A., Davies, H. L., Dower, J. F., Juanes, F., and Dudas, S. E. (2019). Human Consumption of Microplastics. Environ. Sci. Technol. 53, 7068–7074. doi: 10.1021/acs.est.9b01517
Critchell, K., and Hoogenboom, M. O. (2018). Effects of microplastic exposure on the body condition and behaviour of planktivorous reef fish (Acanthochromis polyacanthus). PLoS One 13:e0193308. doi: 10.1371/journal.pone.0193308
Crutchett, T., Paterson, H., Ford, B. M., and Speldewinde, P. (2020). Plastic ingestion in sardines (Sardinops sagax) from Frenchman Bay, Western Australia, highlights a problem in a ubiquitous fish. Front. Mar. Sci. 7, 1–13. doi: 10.3389/fmars.2020.00526
Derraik, J. G. (2002). The pollution of the marine environment by plastic debris: a review. Mar. Pollut. Bull. 44, 842–852. doi: 10.1016/S0025-326X(02)00220-5
Eriksen, M., Maximenko, N., Thiel, M., Cummins, A., Lattin, G., Wilson, S., et al. (2013). Plastic pollution in the South Pacific subtropical gyre. Mar. Pollut. Bull. 68, 71–76. doi: 10.1016/j.marpolbul.2012.12.021
Farrell, P., and Nelson, K. (2013). Trophic level transfer of microplastic: mytilus edulis (L.) to Carcinus maenas (L.). Environ. Pollut. 177, 1–3. doi: 10.1016/j.envpol.2013.01.046
Ferreira, C. E. L., and Gonçalves, J. E. A. (2006). Community structure and diet of roving herbivorous reef fishes in the Abrolhos Archipelago, south-western Atlantic. J. Fish Biol. 69, 1533–1551. doi: 10.1111/j.1095-8649.2006.01220.x
Ferreira, C. E. L., Peret, A. C., and Coutinho, R. (1998). Seasonal grazing rates and food processing by tropical herbivorous fishes. J. Fish Biol. 53, 222–235. doi: 10.1111/J.1095-8649.1998.TB01029.X
Forrest, A. K., and Hindell, M. (2018). Ingestion of plastic by fish destined for human consumption in remote South Pacific Islands. Aust. J. Marit. Ocean Aff. 10, 81–97. doi: 10.1080/18366503.2018.1460945
Francini-Filho, R. B., Ferreira, C. M., Coni, E. O. C., de Moura, R. L., and Kaufman, L. (2010). Foraging activity of roving herbivorous reef fish (Acanthuridae and Scaridae) in eastern Brazil: influence of resource availability and interference competition. J. Mar. Biol. Assoc. U. K. 90, 481–492. doi: 10.1017/S0025315409991147
Galgani, F., Souplet, A., and Cadiou, Y. (1996). Accumulation of debris on the deep sea floor off the French Mediterranean coast. Mar. Ecol. Prog. Ser. 142, 225–234. doi: 10.3354/meps142225
Gall, S. C., and Thompson, R. C. (2015). The impact of debris on marine life. Mar. Pollut. Bull. 92, 170–179. doi: 10.1016/j.marpolbul.2014.12.041
Garnier, Y., Jacob, H., Guerra, A. S., Bertucci, F., and Lecchini, D. (2019). Evaluation of microplastic ingestion by tropical fish from Moorea Island. French Polynesia. Mar. Pollut. Bull. 140, 165–170. doi: 10.1016/j.marpolbul.2019.01.038
Germanov, E. S., Marshall, A. D., Bejder, L., Fossi, M. C., and Loneragan, N. R. (2018). Microplastics: no small problem for filter-feeding megafauna. Trends Ecol. Evol. 33, 227–232. doi: 10.1016/j.tree.2018.01.005
Germanov, E. S., Marshall, A. D., Hendrawan, I. G., Admiraal, R., Rohner, C. A., Argeswara, J., et al. (2019). Microplastics on the menu: plastics pollute Indonesian manta ray and whale shark feeding grounds. Front. Mar. Sci. 6:679. doi: 10.3389/fmars.2019.00679
Giglio, V. J., Ternes, M. L. F., Mendes, T. C., Cordeiro, C. A. M. M., and Ferreira, C. E. L. (2017). Anchoring damages to benthic organisms in a subtropical scuba dive hotspot. J. Coast. Conserv. 21, 311–316. doi: 10.1007/s11852-017-0507-7
Goss, H., Jaskiel, J., and Rotjan, R. D. (2018). Thalassia testudinum as a potential vector for incorporating microplastics into benthic marine food webs. Mar. Pollut. Bull. 135, 1085–1089. doi: 10.1016/j.marpolbul.2018.08.024
Gutow, L., Eckerlebe, A., Giménez, L., and Saborowski, R. (2016). Experimental evaluation of seaweeds as a vector for microplastics into marine food webs. Environ. Sci. Technol. 50, 915–923. doi: 10.1021/acs.est.5b02431
Hall, N. M., Berry, K. L. E., Rintoul, L., and Hoogenboom, M. O. (2015). Microplastic ingestion by scleractinian corals. Mar. Biol. 162, 725–732. doi: 10.1007/s00227-015-2619-7
Hidalgo-Ruz, V., Luna-Jorquera, G., Eriksen, M., Frick, H., Miranda-Urbina, D., Portflitt-Toro, M., et al. (2021). Factors (type, colour, density, and shape) determining the removal of marine plastic debris by seabirds from the South Pacific Ocean: is there a pattern? Aquat. Conserv. Mar. Freshw. Ecosyst. 31, 389–407. doi: 10.1002/aqc.3453
Hoey, A. S., Brandl, S. J., and Bellwood, D. R. (2013). Diet and cross-shelf distribution of rabbitfishes (f. Siganidae) on the northern Great Barrier Reef: implications for ecosystem function. Coral Reefs 32, 973–984. doi: 10.1007/s00338-013-1043-z
Hughes, T. P., Barnes, M. L., Bellwood, D. R., Cinner, J. E., Cumming, G. S., Jackson, J. B. C., et al. (2017). Coral reefs in the Anthropocene. Nature 546, 82–90. doi: 10.1038/nature22901
Jabeen, K., Su, L., Li, J., Yang, D., Tong, C., Mu, J., et al. (2017). Microplastics and mesoplastics in fish from coastal and fresh waters of China. Environ. Pollut. 221, 141–149. doi: 10.1016/j.envpol.2016.11.055
Jambeck, J. R., Geyer, R., Wilcox, C., Siegler, T. R., Perryman, M., Andrady, A., et al. (2015). Plastic waste inputs from land into the ocean. Science 347, 768–771. doi: 10.1126/science.1260352
Kane, I. A., Clare, M. A., Miramontes, E., Wogelius, R., Rothwell, J. J., Garreau, P., et al. (2020). Seafloor microplastic hotspots controlled by deep-sea circulation. Science 368, 1140–1145. doi: 10.1126/science.aba5899
Karpouzi, V. S., and Stergiou, K. I. (2003). The relationships between mouth size and shape and body length for 18 species of marine fishes and their trophic implications. J. Fish Biol. 62, 1353–1365. doi: 10.1046/j.1095-8649.2003.00118.x
Knudsen, S. W., Choat, J. H., and Clements, K. D. (2019). The herbivorous fish family Kyphosidae (Teleostei: Perciformes) represents a recent radiation from higher latitudes. J. Biogeogr. 46, 2067–2080. doi: 10.1111/jbi.13634
Knudsen, S. W., and Clements, K. D. (2013). Revision of the fish family Kyphosidae (Teleostei: Perciformes). Zootaxa 3751, 1–101. doi: 10.11646/zootaxa.3751.1.1
LaFleur, A. D., and Schug, K. A. (2011). A review of separation methods for the determination of estrogens and plastics-derived estrogen mimics from aqueous systems. Anal. Chim. Acta 696, 6–26. doi: 10.1016/j.aca.2011.03.054
Leão, Z. M. A. N., and Dominguez, J. M. L. (2000). Tropical coast of Brazil. Mar. Pollut. Bull. 41, 112–122. doi: 10.1016/S0025-326X(00)00105-3
Lewis, S. L., and Maslin, M. A. (2015). Defining the Anthropocene. Nature 519, 171–180. doi: 10.1038/nature14258
Lima, S. R., Barbosa, J. M., da, S., Padilha, F. G. F., Saracchini, P. G. V., Braga, M., et al. (2018). Physical characteristics of free-living sea turtles that had and had not ingested debris in Microregion of the Lakes. Brazil. Mar. Pollut. Bull. 137, 723–727. doi: 10.1016/j.marpolbul.2018.10.032
Luiz, O. J., Olden, J. D., Kennard, M. J., Crook, D. A., Douglas, M. M., Saunders, T. M., et al. (2019). Trait-based ecology of fishes: a quantitative assessment of literature trends and knowledge gaps using topic modelling. Fish Fish. 20, 1100–1110. doi: 10.1111/faf.12399
Lusher, A. L., McHugh, M., and Thompson, R. C. (2013). Occurrence of microplastics in the gastrointestinal tract of pelagic and demersal fish from the English Channel. Mar. Pollut. Bull. 67, 94–99. doi: 10.1016/j.marpolbul.2012.11.028
Machovsky-Capuska, G. E., Andrades, R., and Santos, R. G. (2020). Debris ingestion and nutritional niches in estuarine and reef green turtles. Mar. Pollut. Bull. 153:110943. doi: 10.1016/j.marpolbul.2020.110943
Macieira, R. M., Oliveira, L. A. S., Cardozo-Ferreira, G. C., Pimentel, C. R., Andrades, R., Gasparini, J. L., et al. (2021). Microplastic and artificial cellulose microfibers ingestion by reef fishes in the Guarapari Islands, southwestern Atlantic. Mar. Pollut. Bull. 167:112371. doi: 10.1016/j.marpolbul.2021.112371
Maes, T., Van der Meulen, M. D., Devriese, L. I., Leslie, H. A., Huvet, A., Frère, L., et al. (2017). Microplastics baseline surveys at the water surface and in sediments of the North-East Atlantic. Front. Mar. Sci. 4, 1–13. doi: 10.3389/fmars.2017.00135
Markic, A., Gaertner, J. C., Gaertner-Mazouni, N., and Koelmans, A. A. (2020). Plastic ingestion by marine fish in the wild. Crit. Rev. Environ. Sci. Technol. 50, 657–697. doi: 10.1080/10643389.2019.1631990
Markic, A., Niemand, C., Bridson, J. H., Mazouni-Gaertner, N., Gaertner, J. C., Eriksen, M., et al. (2018). Double trouble in the South Pacific subtropical gyre: increased plastic ingestion by fish in the oceanic accumulation zone. Mar. Pollut. Bull. 136, 547–564. doi: 10.1016/j.marpolbul.2018.09.031
McGill, B. J., Enquist, B. J., Weiher, E., and Westoby, M. (2006). Rebuilding community ecology from functional traits. Trends Ecol. Evol. 21, 178–185. doi: 10.1016/j.tree.2006.02.002
McNeish, R. E., Kim, L. H., Barrett, H. A., Mason, S. A., Kelly, J. J., and Hoellein, T. J. (2018). Microplastic in riverine fish is connected to species traits. Sci. Rep. 8, 1–12. doi: 10.1038/s41598-018-29980-9
Mendes, T. C., Ferreira, C. E. L., and Clements, K. D. (2018). Discordance between diet analysis and dietary macronutrient content in four nominally herbivorous fishes from the Southwestern Atlantic. Mar. Biol. 165:180. doi: 10.1007/s00227-018-3438-4
Miller, M. E., Hamann, M., and Kroon, F. J. (2020). Bioaccumulation and biomagnification of microplastics in marine organisms: a review and meta-analysis of current data. PLoS One 15, 1–25. doi: 10.1371/journal.pone.0240792
Mizraji, R., Ahrendt, C., Perez-Venegas, D., Vargas, J., Pulgar, J., Aldana, M., et al. (2017). Is the feeding type related with the content of microplastics in intertidal fish gut? Mar. Pollut. Bull. 116, 498–500. doi: 10.1016/j.marpolbul.2017.01.008
Ogle, D. H., Wheeler, P., and Dinno, A. (2019). FSA: fisheries Stock Analysis. R package version 0.8. 26. Available online at: https://github.com/droglenc/FSA (accessed July 17, 2021).
Peters, C. A., and Bratton, S. P. (2016). Urbanization is a major influence on microplastic ingestion by sunfish in the Brazos River Basin. Central Texas, USA. Environ. Pollut. 210, 380–387. doi: 10.1016/j.envpol.2016.01.018
Pirsaheb, M., Hossini, H., and Makhdoumi, P. (2020). Review of microplastic occurrence and toxicological effects in marine environment: experimental evidence of inflammation. Process Saf. Environ. Prot. 142, 1–14. doi: 10.1016/j.psep.2020.05.050
Poore, A. G. B., Campbell, A. H., Coleman, R. A., Edgar, G. J., Jormalainen, V., Reynolds, P. L., et al. (2012). Global patterns in the impact of marine herbivores on benthic primary producers. Ecol. Lett. 15, 912–922. doi: 10.1111/j.1461-0248.2012.01804.x
Possatto, F. E., Barletta, M., Costa, M. F., Ivar do Sul, J. A., and Dantas, D. V. (2011). Plastic debris ingestion by marine catfish: an unexpected fisheries impact. Mar. Pollut. Bull. 62, 1098–1102. doi: 10.1016/j.marpolbul.2011.01.036
Purcell, S. W., and Bellwood, D. R. (1993). A functional analysis of food procurement in two surgeonfish species, Acanthurus nigrofuscus and Ctenochaetus striatus (Acanthuridae). Environ. Biol. Fish. 37, 139–159. doi: 10.1007/BF00000589
R Core Team, R. (2020). The R Project for Statistical Computing. Available online at: https://www.r-project.org/ (accessed February 15, 2021).
Rech, S., Macaya-Caquilpán, V., Pantoja, J. F., Rivadeneira, M. M., Jofre Madariaga, D., and Thiel, M. (2014). Rivers as a source of marine litter – A study from the SE Pacific. Mar. Pollut. Bull. 82, 66–75. doi: 10.1016/j.marpolbul.2014.03.019
Remy, F., Collard, F., Gilbert, B., Compère, P., Eppe, G., and Lepoint, G. (2015). When microplastic is not plastic: the ingestion of artificial cellulose fibers by macrofauna living in seagrass macrophytodetritus. Environ. Sci. Technol. 49, 11158–11166. doi: 10.1021/acs.est.5b02005
Richards, A. H. (1994). Problems of drift-net fisheries in the South Pacific. Mar. Pollut. Bull. 29, 106–111. doi: 10.1016/0025-326X(94)90433-2
Robards, M. D., Piatt, J. F., and Wohl, K. D. (1995). Increasing frequency of plastic particles ingested by seabirds in the subarctic North Pacific. Mar. Pollut. Bull. 30, 151–157. doi: 10.1016/0025-326X(94)00121-O
Rochman, C. M. (2013). Plastics and priority pollutants: a multiple stressor in aquatic habitats. Environ. Sci. Technol. 47, 2439–2440. doi: 10.1021/es400748b
Rochman, C. M., Hoh, E., Kurobe, T., and Teh, S. J. (2013). Ingested plastic transfers hazardous chemicals to fish and induces hepatic stress. Sci. Rep. 3:3263. doi: 10.1038/srep03263
Rochman, C. M., Tahir, A., Williams, S. L., Baxa, D. V., Lam, R., Miller, J. T., et al. (2015). Anthropogenic debris in seafood: plastic debris and fibers from textiles in fish and bivalves sold for human consumption. Sci. Rep. 5:14340. doi: 10.1038/srep14340
Roos, N. C., Pennino, M., Carvalho, A., and Longo, G. O. (2019). Drivers of abundance and biomass of Brazilian parrotfishes. Mar. Ecol. Prog. Ser. 623, 117–130. doi: 10.3354/meps13005
Rotjan, R. D., Sharp, K. H., Gauthier, A. E., Yelton, R., Baron Lopez, E. M., Carilli, J., et al. (2019). Patterns, dynamics and consequences of microplastic ingestion by the temperate coral, Astrangia poculata. Proc. R. Soc. B Biol. Sci. 286, 1–9. doi: 10.1098/rspb.2019.0726
Ryan, P. G., Moore, C. J., van Franeker, J. A., and Moloney, C. L. (2009). Monitoring the abundance of plastic debris in the marine environment. Philos. Trans. R. Soc. Lond. B. Biol. Sci. 364, 1999–2012. doi: 10.1098/rstb.2008.0207
Salerno, M., Berlino, M., Mangano, M. C., and Sarà, G. (2021). Microplastics and the functional traits of fishes: a global meta-analysis. Glob. Chang. Biol. 27, 2645–2655. doi: 10.1111/gcb.15570
Santos, R. G., Andrades, R., Boldrini, M. A., and Martins, A. S. (2015). Debris ingestion by juvenile marine turtles: an underestimated problem. Mar. Pollut. Bull. 93, 37–43. doi: 10.1016/j.marpolbul.2015.02.022
Santos, R. G., Andrades, R., Demetrio, G. R., Kuwai, G. M., Sobral, M. F., Vieira, J., et al. (2020). Exploring plastic-induced satiety in foraging green turtles. Environ. Pollut. 265:114918. doi: 10.1016/j.envpol.2020.114918
Santos, R. G., Andrades, R., Fardim, L. M., and Martins, A. S. (2016). Marine debris ingestion and Thayer’s law – The importance of plastic color. Environ. Pollut. 214, 585–588. doi: 10.1016/j.envpol.2016.04.024
Santos, R. G., Machovsky-Capuska, G. E., and Andrades, R. (2021). Plastic ingestion as an evolutionary trap: toward a holistic understanding. Science 373, 56–60. doi: 10.1126/science.abh0945
Savoca, M. S., McInturf, A. G., and Hazen, E. L. (2021). Plastic ingestion by marine fish is widespread and increasing. Glob. Chang. Biol. 27, 2188–2199. doi: 10.1111/gcb.15533
Savoca, S., Capillo, G., Mancuso, M., Faggio, C., Panarello, G., Crupi, R., et al. (2019). Detection of artificial cellulose microfibers in Boops boops from the northern coasts of Sicily (Central Mediterranean). Sci. Total Environ. 691, 455–465. doi: 10.1016/j.scitotenv.2019.07.148
Setälä, O., Fleming-Lehtinen, V., and Lehtiniemi, M. (2014). Ingestion and transfer of microplastics in the planktonic food web. Environ. Pollut. 185, 77–83. doi: 10.1016/j.envpol.2013.10.013
Silva, M. L., da Castro, R. O., Sales, A. S., Araújo, F. V., and de. (2018). Marine debris on beaches of Arraial do Cabo, RJ, Brazil: an important coastal tourist destination. Mar. Pollut. Bull. 130, 153–158. doi: 10.1016/j.marpolbul.2018.03.026
Thayer, A. H. (1896). The law which underlies protective coloration. Auk 13, 124–129. doi: 10.2307/4068693
Valentin, J. L. (2001). “The Cabo Frio upwelling system, Brazil” in Coastal Marine Ecosystems of Latin America. eds U. Seeliger and B. Kjerfve (Germany: Springer-Verlag). 97–105. doi: 10.1007/978-3-662-04482-7_8
van der Hal, N., Yeruham, E., and Angel, D. L. (2018). “Dynamics in Microplastic Ingestion During the Past Six Decades in Herbivorous Fish on the Mediterranean Israeli Coast” in Proceedings of the International Conference on Microplastic Pollution in the Mediterranean Sea. eds M. Cocca, E. Di Pace, M. Errico, G. Gentile, A. Montarsolo, and R. Mossotti (Switzerland: Springer, Cham). 159–165. doi: 10.1007/978-3-319-71279-6_21
Walker, T. R., Reid, K., Arnould, J. P. Y., and Croxall, J. P. (1997). Marine debris surveys at Bird Island, South Georgia 1990-1995. Mar. Pollut. Bull. 34, 61–65. doi: 10.1016/S0025-326X(96)00053-7
Wickham, H. (2016). ggplot2: elegant Graphics for Data Analysis. New York: Springer-Verlag. doi: 10.1093/bioinformatics/btr406
Wieczorek, A. M., Morrison, L., Croot, P. L., Allcock, A. L., MacLoughlin, E., Savard, O., et al. (2018). Frequency of microplastics in mesopelagic fishes from the Northwest Atlantic. Front. Mar. Sci. 5:1–9. doi: 10.3389/fmars.2018.00039
Wilke, C. O. (2020). cowplot: streamlined Plot Theme and Plot Annotations for “ggplot2.” R Packag. Version 1.1.1. Available online at: https://cran.r-project.org/package=cowplot (accessed 15 June, 2021).
Wójcik-Fudalewska, D., Normant-Saremba, M., and Anastácio, P. (2016). Occurrence of plastic debris in the stomach of the invasive crab Eriocheir sinensis. Mar. Pollut. Bull. 113, 306–311. doi: 10.1016/j.marpolbul.2016.09.059
Wright, S. L., Thompson, R. C., and Galloway, T. S. (2013). The physical impacts of microplastics on marine organisms: a review. Environ. Pollut. 178, 483–492. doi: 10.1016/j.envpol.2013.02.031
Xiong, X., Tu, Y., Chen, X., Jiang, X., Shi, H., Wu, C., et al. (2019). Ingestion and egestion of polyethylene microplastics by goldfish (Carassius auratus): influence of color and morphological features. Heliyon 5:e03063. doi: 10.1016/j.heliyon.2019.e03063
Zeileis, A., Fisher, J. C., Hornik, K., Ihaka, R., McWhite, C. D., Murrell, P., et al. (2020). Colorspace: a toolbox for manipulating and assessing colors and palettes. J. Stat. Softw. 96, 1–49. doi: 10.18637/jss.v096.i01
Zeileis, A., Kleiber, C., and Jackman, S. (2008). Regression models for count data in R. J. Stat. Softw. 27. doi: 10.18637/jss.v027.i08
Keywords: marine pollution, anthropogenic debris, ingestion, herbivorous fishes, functional traits
Citation: Cardozo-Ferreira GC, Calazans TL, Benevides LJ, Luiz OJ, Ferreira CEL and Joyeux J-C (2021) Ecological Traits Influencing Anthropogenic Debris Ingestion by Herbivorous Reef Fishes. Front. Mar. Sci. 8:717435. doi: 10.3389/fmars.2021.717435
Received: 30 May 2021; Accepted: 13 August 2021;
Published: 03 September 2021.
Edited by:
Matthew Savoca, Stanford University, United StatesReviewed by:
Alexandra McInturf, University of California, Davis, United StatesGarth Aidan Covernton, University of Victoria, Canada
Copyright © 2021 Cardozo-Ferreira, Calazans, Benevides, Luiz, Ferreira and Joyeux. This is an open-access article distributed under the terms of the Creative Commons Attribution License (CC BY). The use, distribution or reproduction in other forums is permitted, provided the original author(s) and the copyright owner(s) are credited and that the original publication in this journal is cited, in accordance with accepted academic practice. No use, distribution or reproduction is permitted which does not comply with these terms.
*Correspondence: Gabriel C. Cardozo-Ferreira, Z2FicmllbGNjZkBnbWFpbC5jb20=