- 1Department of Biology, College of Science, United Arab Emirates University, Al Ain, United Arab Emirates
- 2Harry Butler Institute, Murdoch University, Murdoch, WA, Australia
- 3Botany Department, Faculty of Science, Tanta University, Tanta, Egypt
- 4Department of Medical Education and Research, Dubai Health Authority, Dubai, United Arab Emirates
Gray mangrove (Avicennia marina) is the dominant vegetation distributed along the coast of the United Arab Emirates (UAE). Despite its performance as natural coastal guardians, very little is known about the reforestation projects to increase mangrove cover over the years in the UAE and in the Arabian Gulf. Plant growth-promoting actinobacteria (PGPA) were isolated from the mangrove rhizosphere sediments found in the UAE and were evaluated for their potential to produce plant growth regulators (PGRs) and to enhance mangrove growth under seawater irrigation conditions. In vitro screening identified nine rhizosphere-competent actinobacterial isolates, in a naturally competitive environment, of which Streptomyces coelicoflavus (Sc) showed a high phosphorus solubilizing activity. Moreover, Streptomyces polychromogenes (Sp), Streptomyces bacillaris (Sb), and Streptomyces ferrugineus (Sf) produced auxins, polyamines (PAs), and 1-aminocyclopropane-1-carboxylic acid (ACC) deaminase, respectively. Although sediment inoculation with single isolates significantly improved the dry biomass of mangrove shoots (43.2–74.0%) and roots (40.8–75.9%), the consortium of isolates (Sc/Sp/Sb/Sf) caused a greater increase in the dry weight of shoots (82.1%) and roots (81.6%) compared with seawater-irrigated plants (control). In our greenhouse experiments, the levels of photosynthetic pigments, in planta auxins, and PAs significantly increased in plant tissues inoculated with Sc/Sp/Sb/Sf; whereas ACC contents were reduced. This was also evident as the maximum velocity of rubisco carboxylation (Vcmax) increased four-fold in plants treated with the mixture of isolates over control. To the best of our knowledge, this is the first study reporting culturable halotolerant, rhizosphere-competent PGPA inhabiting salty and arid ecosystems applied individually or in combination to promote mangrove growth under harsh conditions such as those found in the Arabian coastal areas.
Introduction
Mangroves, also known as qurm, are one of the few woody ecosystems that grow along the arid coasts of the Arabian Gulf (Elmahdy et al., 2020). Avicennia marina (Forsk.) Vierh. (gray mangrove) is the most dominant mangrove species growing along the Arabian Gulf coast of the United Arab Emirates (UAE), Saudi Arabia, Bahrain, Qatar, and Iran (Almahasheer, 2018; Elmahdy et al., 2020). Mangrove vegetation forms a unique ecosystem that occupies the land–sea interphase of the tropics and subtropics. Mangrove trees act as a habitat for migratory birds and as a nursery base for important commercial fish species and can reduce coastal erosion. The trees also buffers adjacent marine ecosystems (often coral reefs) from terrestrial inputs (Spalding and Parrett, 2019). Mangrove forests are, however, under severe pressure worldwide. In the last 30 years, more than 50% of the mangroves in the world have been destroyed (Polidoro et al., 2010), mainly because of urban development, timber production, clearing for aquaculture, or for cultivation purposes (Primavera et al., 2004; Elmahdy et al., 2020). In the UAE, the major constraints affecting the growth pattern and distribution of mangrove trees are a wide seasonal variation in temperatures, poor soil conditions, and high seawater salinity (Barth and Khan, 2008; Samara et al., 2020).
Soil fertility and plant health depend largely on beneficial plant–microbe interactions present in the rhizosphere. For instance, free-living soil bacteria isolated from the rhizosphere, also referred to as plant growth-promoting (PGP) bacteria (PGPB), have been shown to improve plant growth or increase yield especially in nutrient-impoverished environments (Bashan and de-Bashan, 2005; Hassan et al., 2019; Kumar et al., 2019). Seed or root inoculation with PGPB is a common agricultural practice currently in use to improve the productivity and yields of many plants, including halophytes (El-Tarabily et al., 2019, 2020, 2021; Mathew et al., 2020; Moretti et al., 2020). PGPB may directly influence plant growth by enhancing the availability of essential nutrients such as phosphorous (P), nitrogen (N), and iron (Fe) (El-Tarabily and Youssef, 2010; Glick, 2015), stimulating transport in plants (Bertrand et al., 2000), and producing plant growth regulators (PGRs) such as auxins [e.g., indole-3-acetic acid (IAA)], cytokinins (CKs), gibberellins (GAs), and polyamines (PAs) (Bashan et al., 2004; Hassan et al., 2019). PGPB can also be involved in regulating stress hormonal levels (Glick et al., 2007; Glick, 2014). The enzyme 1-aminocyclopropane-1-carboxylic acid (ACC) deaminase (ACCD) produced by soil PGPB degrades ACC, thereby reducing the stress hormone ethylene (ET) in host plants (Glick, 2014; Acuna et al., 2019). Other PGPB exert their beneficial effects indirectly via the production of metabolites such as siderophores, or antifungal compounds to restrict the growth and control of plant pathogens (Glick et al., 2007). PGPB inoculants using either a single strain with multiple mechanisms or a group of isolates with each differing in activities could augment the positive impact to enhance plant growth (Glick, 2012; Mathew et al., 2020; Moretti et al., 2020).
In coastal wetlands, few studies have demonstrated the potential of using the PGPB isolated from mangrove roots and rhizosphere for arid mangrove reforestation (Bashan et al., 2009; El-Tarabily and Youssef, 2010; Castro et al., 2018; El-Tarabily et al., 2021). These reports have linked PGPB to the growth of mangroves through P-solubilization or N-fixation. There are only two reports so far that describe the inoculation of mangrove sediments with the IAA-producing Azotobacter spp. (Ravikumar et al., 2004; Kathiresan and Selvam, 2006). In addition, El-Tarabily and Youssef (2011) reported the ability of the ACCD-producing bacterium, Pseudoalteromonas maricaloris, to promote mangrove growth under greenhouse conditions. PGPB that may enhance mangrove growth through other mechanisms, such as the production of different PGRs, have not been explored yet.
The ability of PGPB to colonize the rhizosphere and/or the rhizoplane and create a favorable environment for development and function is essential to confer plant beneficial effects (Schreiter et al., 2018). Rhizosphere competency has been set as a main requirement for selecting effective PGPB (Hassan et al., 2019). Recently, actinobacteria isolated from marine environments were shown to promote the growth and seed yields of Salicornia bigelovii stimulating in planta levels of PAs and PGRs and reducing the effects of ET (El-Tarabily et al., 2020; Mathew et al., 2020). To our knowledge, no information is available on rhizosphere-competent PGPB competing with other microorganisms and colonizing mangrove roots and rhizosphere in sediments under natural competitive environments. Microcoleus chthonoplastes (Toledo et al., 1995a), two Azospirillum spp. (Puente et al., 1999), and Bacillus licheniformis and Phyllobacterium sp. (Rojas et al., 2001) have been reported to successfully colonize the roots of black mangrove (Avicennia germinans) in vitro in artificial seawater. The colonization of aerial roots (pneumatophores) of black mangrove trees by N-fixing cyanobacteria has also been evaluated in situ in Mexico (Toledo et al., 1995b).
Many existing reports have been dealt with actinobacterial communities living in mangrove sediments (Ruan et al., 2015; Kemung et al., 2020) and within mangrove roots (Jiang et al., 2018). Earlier, endophytic actinobacteria residing in the inner tissues of living plants have been isolated from the mangrove to study their antimicrobial activities (Gayathri and Muralikrishnan, 2013; Jiang et al., 2018). There are no studies so far in the literature that have reported actinobacteria isolated from mangrove sediments for their potential to promote the growth of mangrove seedlings either under naturally competitive environment or nursery/field conditions using seawater irrigation.
Current mangrove reforestation programs in the UAE may benefit from the deployment of PGPB (El-Tarabily and Youssef, 2010, 2011) or PGP actinobacteria (PGPA) (El-Tarabily et al., 2021). In the current study, actinobacteria were intentionally targeted because they are adapted to harsh desert environments of low rainfall, high temperature, and salinity found in the UAE and the Arabian Gulf region (Goodfellow and Williams, 1983; El-Tarabily et al., 2020). The objectives were to: (1) isolate potential PGPA native to the mangrove rhizosphere; (2) determine the ability of isolates to be rhizosphere competent under naturally competitive environments; (3) determine the PGPA attributes by evaluating the potential of isolates to either solubilize P, produce PGR (e.g., auxins, PAs), or ACCD in vitro; and (4) test the ability of the promising rhizosphere-competent PGPA to enhance the growth of mangrove seedlings under controlled greenhouse conditions using seawater irrigation. The best performing isolates were in vivo applied individually and in combination to assess whether mangrove growth can be enhanced by the different modes of action under greenhouse conditions.
Materials and Methods
Plant Materials and Sediment Characteristics
Viviparous seed capsules (propagules) of A. marina were collected from beneath healthy mangrove trees in the east coast of Abu Dhabi, UAE (24°26′48.5″ N and 54°26′40.6″ E). Propagules of almost similar dimensions were firstly rinsed in running tap water for 1 h followed by surface sterilization using 70% ethyl alcohol (EtOH; Sigma-Aldrich Chemie GmbH, Taufkirchen, Germany), 1.05% Clorox (20% household bleach), and two drops of Tween 20 (Sigma-Aldrich). Propagules were washed with 0.22 μm membrane filter-sterilized (Millipore Corporation, Burlington, MA, USA) full-strength seawater (salinity of 4.0%) and air-dried.
The dark grayish-black sediment chemical characteristics were: electrical conductivity = 4.81 dSm−1; pH = 8.36 (in 0.01 M CaCl2); organic carbon (C) 6.24%. Nutrients (mg kg−1 sediment) such as available and total P (8.83 and 85, respectively), N as nitrate (4) and ammonium (6.4), bicarbonate extractable potassium (K; 241), oxalate extractable amorphous Fe (331), and sulfate (414) were also detected.
Collection of Mangrove Rhizosphere Sediments
Rhizosphere sediments of eight small mangrove seedlings were collected from the same area described above. Rhizosphere sediments (maximum depth of 15 cm) were carefully collected by shaking roots into plastic bags. Each sample was passed through a 2-mm sieve to remove root fragments and small stones. The collected rhizosphere samples were air-dried at 25°C for 4 days to reduce the viable vegetative bacterial cells (Williams et al., 1972).
Quantification and Isolation of Actinobacteria From Mangrove Rhizosphere
All microbiological media in this study were prepared using millipore membrane filter-sterilized full-strength seawater. Actinobacterial populations of freshly sampled mangrove rhizosphere sediments were estimated using the soil dilution plate count method within 24 h of sampling. Three replicates of each rhizosphere sediment (20 g) were dispensed into 100 ml of filter-sterilized seawater, and the sediment suspension was placed in an ultrasonic cleaner at a frequency of 65,000 cycles s−1 for 20 s. The sediment suspension was then shaken on a rotary shaker Model G76 (New Brunswick Scientific, Edison, NJ, USA) at 250 rpm for 30 min at 28°C. Dilutions of 10−2–10−5 were made in membrane filter-sterilized full-strength seawater, and 0.2-ml aliquots were spread onto an inorganic salt starch agar (ISSA; Küster, 1959) in sterile petri dishes. After cooling ISSA medium to 45°C, cycloheximide and nystatin (50 μg ml−1 each; Sigma-Aldrich) were supplemented. Five plates per dilution were dried and incubated at 28°C ± 2°C in dark for 7 days. Colonies were counted as log10 colony-forming units (cfu) g−1 dry sediment. All actinobacteria were purified and stored on an oatmeal agar (ISP3 medium) supplemented with 0.1% yeast extract (OMYEA; Shirling and Gottlieb, 1966).
Preliminary identification of streptomycete and non-streptomycete actinobacteria was based on cultural characteristics such as color of aerial and substrate mycelia, and the production of diffusible pigments (Cross, 1989). In addition, the presence/absence of aerial mycelia, distribution of spores on both aerial/substrate mycelia, formation of sporangia, and stability/fragmentation of substrate mycelia were among the morphological features assessed.
Determination of Actinobacterial Tolerance to Different Concentrations of NaCl
Salt tolerance was evaluated by growing the selected isolates on ISSA medium made with deionized water and supplemented with NaCl concentrations: 0, 10, 20, 40, 60, and 80 g L−1. Isolates were streaked on ISSA plates and incubated at 28°C in dark for 7 days (Williams et al., 1972). Three replicates were used for each isolate. Isolates showing a strong growth and sporulation on ISSA supplemented with 80 g NaCl L−1 (8%) indicated high NaCl tolerance and were considered as halotolerant. Only these isolates were chosen for subsequent experiments.
Root Colonization and Rhizosphere-Competence Assays
The ability of the halotolerant actinobacterial isolates to be rhizosphere competent and to colonize mangrove rhizosphere was determined in two stages. A preliminary qualitative indicator root colonization jar bioassay (Silva et al., 2003) was carried out in vitro to determine whether mangrove root exudates acting as the sole C source could support the growth of each actinobacterial isolate.
Surface-sterilized mangrove propagules were soaked in 20 ml of a suspension of each actinobacterial isolate (108 cfu ml−1) for 24 h and then transferred into sterile 0.6% water-agar jars (300 mm × 40 mm in diameter) (Silva et al., 2003). Jars were incubated in a growth chamber (16-h photoperiod, 180–200 μmol m−2 s−1 fluorescent light; 25:20°C light:dark temperature). Root colonization by actinobacterial isolates was examined after 21 days and was recorded as a percentage of the total root length. Periodic visual inspections were performed daily to detect actinobacterial growth around arising roots (Silva et al., 2003). Assays were carried out two times with three replicates per culture.
Promising isolates from the in vitro jar bioassay were subjected to a qualitative and quantitative rhizosphere-competence assay using the non-sterilized soil tube assay (Ahmad and Baker, 1987) and modified by Nautiyal (1997) using rifampicin-resistant mutants (Misaghi and Donndelinger, 1990).
In the soil tube assay, a non-sterilized mangrove sediment was used. After 30 days, the first 14 cm of the propagule roots was retained, aspectically cut into 2 cm segments, and sequentially numbered and plated onto ISSA plates amended with rifampicin (Ahmad and Baker, 1987). The remaining roots were discarded. Loose soil particles on the remaining root segments were removed with forceps, air-dried for 24 h, shaken in sterile water for 1 h, and were studied individually. Soil particles were numbered according to the root segments from which they were recovered. Sediment dilutions (10−2–10−3) were prepared, and a 0.2-ml aliquot of each sediment dilution was inoculated onto ISSA plates supplemented with rifampicin and was incubated for 7 days at 28°C in dark. Root-colonization frequencies (R%) and the population densities (PD; log10 cfu g−1 dry sediment) of isolates in rhizosphere sediments (Ahmad and Baker, 1987) were assessed along the root length for all root segments and corresponding soil particles on which the actinobacterium could be recognized. The rhizosphere or the root segment was considered to be colonized when the actinobacterium was detected either on a root segment or in a corresponding rhizosphere soil sample or both. The percentage of the colonized root segments was calculated as the proportion of the total number of segments colonized to the total segments sampled at each distance. Rhizosphere-competence assays were independently repeated two times with eight replicates in each.
Nineteen isolates were selected from the obtained root colonization jar bioassay. Only nine isolates, which showed the strongest rhizosphere-competence ability and colonized the entire length of mangrove roots up to 14 cm in the rhizosphere-competence in vivo sediment tube assay, were selected to study their PGP characteristics.
Evaluation of PGP Activities
In this study, we aimed to isolate and characterize rhizosphere-competent actinobacteria from mangrove sediments in the UAE having single PGP activity (P-solubilization, auxins-, PAs-, or ACCD-production). The strongest nine rhizosphere-competent isolates were tested for their ability to solubilize P, to produce siderophores, NH3, and to fix N. The solubilization of P was determined using Pikovskaya's agar medium (Pikovskaya, 1948) amended with bromophenol blue as an indicator in which tricalcium phosphate was replaced with insoluble rock phosphate (RP; Tianjin Crown Champion International Co. Limited, Tianjin, China). The disappearance of the blue color and the production of a clear zone beneath the actinobacterial culture was an indicator of P-solubilization. All RP-solubilizing isolates showing large zones of clearing on Pikovskaya's agar were further tested quantitatively for their abilities to solubilize RP in the modified National Botanical Research Institute's phosphate broth (Nautiyal, 1997) containing 5 g L−1 RP. An increase in the released soluble P concentration and a drop in pH compared to non-inoculated control were taken as an indicator of the efficiency of isolates to be considered as a strong P solubilizer. For the determination of water-soluble P, colorimetric methods were developed using a molybdophosphoric acid blue complex (Murphy and Riley, 1962). The production of siderophores was monitored using chrome azurol S agar plates (Schwyn and Neilands, 1987). Isolates were considered as siderophores producers when a yellow-orange halo zone was observed around the colony. N-fixation of isolates was determined using the acetylene reduction assay (Holguin et al., 1992) and Varian 6000 gas chromatogram (Varian Instrument Group, Palo Alto, CA, USA). Ammonia production was determined using Nessler's reagent (Dye, 1962). The selected nine rhizosphere-competent isolates were also qualitatively tested for their ability to produce IAA, putrescine (Put), as the main PA, and ACCD in vitro.
For the detection of IAA, Erlenmeyer flasks containing 50 ml of sterile inorganic salt starch broth (ISSB; Küster, 1959) were supplied with 5 ml of 5% filter-sterilized L-tryptophan (Khalid et al., 2004). Flasks were inoculated with 2 ml of each isolate (108 cfu ml−1) and incubated at 28°C on an orbital shaker incubator (Thermo Fisher Scientific, Waltham, MA, USA) in dark at 250 rpm. Non-inoculated flasks were used as a control. After 7 days, suspensions from each flask were centrifuged at 15,000 × g for 30 min. The supernatant was filtered through millipore membranes and collected in sterile tubes. Culture supernatants (6 ml) were transferred into the sterilized McCartney glass bottles, and 4 ml of Salkowski reagent (2 ml of 0.5 M ferric chloride + 98 ml of 35% per chloric acid) were added as described by Gordon and Weber (1951). Bottles were left for 30 min; and the red color value/intensity was measured at 530 nm using a scanning spectrophotometer (UV-2101/3101 PC; Shimadzu Corporation, Analytical Instruments Division, Kyoto, Japan). Auxin compounds were quantitatively expressed as IAA-equivalents using a standard curve made from the standard solutions of IAA.
Preliminary qualitative testing of the nine actinobacterial isolates for Put production was performed in Moeller's decarboxylase agar medium (MDAM) supplemented with 2 g L−1 L-arginine-monohydrochloride (Sigma-Aldrich) and 0.02 g L−1 phenol red (Sigma-Aldrich) as a pH indicator (Arena and Manca de Nadra, 2001). Actinobacterial isolates were streaked on MDAM plates supplemented with arginine and incubated at 28°C in dark for 3 days. MDAM plates without arginine served as control. The production of a dark red halo beneath and around the colonies indicated the production of Put by the decarboxylating isolates.
The selected nine isolates were also screened for the production of ACCD. Isolates (5 days old) were streaked on N-free Dworkin and Foster's salts minimal agar medium (DF; Dworkin and Foster, 1958) amended with either ACC (3 mM; Sigma-Aldrich) or (NH4)2SO4 (2 g; control). Heat labile ACC was filtered-sterilized through a 0.22-μm millipore membrane; and the filtrate was added to DF medium after autoclaving. Strains were streaked on media and incubated at 28°C for 7 days. Growth and heavy sporulation on plates indicated positive for ACCD production. For the quantitative determination of ACCD activity, isolates were grown in ISSB at 28°C in dark for 5 days. Cells were centrifuged, washed with 0.1 M Tris-HCl (pH 8.5), and inoculated onto DF broth containing ACC on an orbital shaker incubator at 250 rpm. After 5 days, cells were resuspended in 0.1 M Tris-HCl and broken by three freezing/thawing cycles (Shah et al., 1998). The lysate was centrifuged at 80,000 × g for 1 h. The enzymatic activity of ACCD was assayed by monitoring the amount of α-ketobutyrate liberated (Honma and Shimomura, 1978). Protein concentrations were also determined (Bradford, 1976).
The selected isolates were further tested in vitro for their capability to produce PGRs: IAA and IPYA as auxins; isopentenyl adenine (iPa), isopentenyl adenoside (iPA), and zeatin (Z) as CKs; and GAs using reverse-phase high-performance liquid chromatography (HPLC; SpectraLab Scientific Inc., Ontario, Canada). For auxin detection, isolates were grown in glucose peptone broth (di Menna, 1957) supplemented with 5 ml of 5% L-tryptophan (Sigma-Aldrich). For the detection of GAs and CKs, isolates were cultivated in Strzelczyk and Pokojska-Burdziej (1984) medium. Sterilized flasks containing 200 ml of each medium were inoculated with 10 ml of each isolate (108 cfu ml−1), covered with an aluminum foil, and incubated in dark at 28°C on a rotary shaker at 250 rpm. After 10 days of incubation, the broth was centrifuged at 7,500 × g for 40 min. The supernatant was reduced to 50 ml by evaporation under vacuum, and the fluid was filtered through 0.22-μm millipore membranes. The filter-sterilized cell-free culture broth was acidified to pH = 3 and was shaken with ethyl acetate (Sigma-Aldrich) at 30 min intervals (Tien et al., 1979). The combined ethyl acetate fractions were collected for the identification of GAs as gibberellic acid (GA3) and auxins. The aqueous fraction was adjusted to pH = 7 and was shaken with water-saturated n-butanol (Sigma-Aldrich) at 30 min intervals, and the fractions were collected for the identification of CKs. Both ethyl acetate and n-butanol fractions were evaporated to dryness in a rotary evaporator under vacuum at 40°C, and the residues were redissolved in 1 ml of absolute methanol (Sigma-Aldrich). Methanol extracts were kept in dark at −20°C until further use for HPLC.
High-performance liquid chromatograms were produced by injecting 10 μl of the methanol dissolved sample onto a 10-μm reverse-phase column (Waters Associates μBondapak C18, 4 mm × 30 cm) in a Waters Associates liquid chromatography equipped with a differential UV detector. Two isocratic solvent systems were used to separate auxins, GA3, and CKs according to Tien et al. (1979). The concentrations of PGRs were obtained by comparing their respective peak areas in an unknown sample with their corresponding areas obtained with the authentic samples (Sigma-Aldrich) of a known concentration.
All isolates showing a large red halo color on MDAM plates were further examined for the quantitative determination of Put, spermidine (Spd), and spermine (Spm) in Moeller's decarboxylase broth medium (MDBM) amended with 2 g L−1 of L-arginine-monohydrochloride by reverse-phase HPLC (SpectraLab Scientific Inc., Ontario, Canada). Erlenmeyer flasks containing 50 ml of MDBM were inoculated with 3 ml of 20% glycerol suspension of each isolate (108 cfu ml−1) and incubated on an orbital shaker incubator at 250 rpm at 28°C. The suspension from each flask was centrifuged at 12,000 × g for 30 min after incubation for 10 days in dark. The internal PA standards and the derivatization of the membrane filter-sterilized cell-free filtrates were carried out as described by Marino et al. (2000). Aliquots of the filtrate (0.5 ml) were mixed with 0.5 ml of saturated sodium bicarbonate solution (Sigma-Aldrich) and 2 ml of dansyl chloride (Sigma-Aldrich) solution (10 mg ml−1 in acetone). The tube was incubated at 60°C for 20 min, and 50 μl of 28% ammonium hydroxide was added. After 30 min, the reaction mixture was filtered again through 0.22-μm millipore membranes and used for HPLC. The HPLC chromatograms were produced by injecting a 10-μl aliquot of the sample onto a 10-μm μBondapak C18 column (4 mm × 30 cm) in a liquid chromatograph (Waters Associates, Milford, MA, USA) equipped with a 254-nm UV detector (Smith and Davies, 1985).
All media related to PGP activities were prepared using millipore-membrane filter-sterilized full-strength seawater.
Identification of Actinobacterial Isolates
The four promising actinobacterial isolates were putatively identified according to their molecular, cultural, and morphological characteristics. The amplification of 16S ribosomal RNA (rRNA) gene using PCR was done by the Deutsche Sammlung von Mikroorganismen und Zellkulturen GmbH (DSMZ), Braunschweig, Germany. The primers 900R (5′-CCGTCAATTCATTTGAGTTT-3′), 357F (5′-TACGGGAGGCAGCAG-3′), and 800F (5′-ATTAGATACCCTGGTAG-3′) were used (Rainey et al., 1996), and the sequences were submitted in the National Center for Biotechnology Information (NCBI; https://www.ncbi.nlm.nih.gov/) with GenBank accession numbers MW577704-MW577707 for isolates #2, #15, #6, and #37, respectively.
For phylogenetic analyses, reference strains were selected from the top hits using the GenBank BLAST search. The sequences of the 16S rRNA gene amplificon (1,521 bp for isolate #2, 1,520 for isolate #6, and 1,517 for isolates #15 and #37) were aligned with the corresponding sequences from closely related Streptomyces spp. using CLUSTAL-X (Thompson et al., 1997) in the Molecular Evolutionary Genetics Analysis version X (MEGAX) software (Kumar et al., 2016). The neighbor-joining method (Saitou and Nei, 1987) was used to construct phylogenetic trees with the bootstrap values calculated based on 500 resamplings. For scanning electron microscopy (SEM), Philips XL-30 SEM (FEI Co., Eindhoven, The Netherlands) was used to examine the morphology of spore chains produced by the isolates.
In vitro Compatibility Test Among Actinobacterial Isolates
To determine the sensitivity of an isolate to diffusible antibacterial metabolites of other isolates, the spot-inoculated plate technique (de Boer et al., 1999) was used. For each isolate, an inoculum of ~108 cfu ml–1 cultured on OMYEA was used. Actinobacterial isolates were spot-inoculated on OMYEA by adding three droplets of 5 μl of the actinobacterial inoculum, and were incubated at 28°C in dark for 5 days. Later on, a suspension of the target isolate was sprayed over the surface of the spot-inoculated plates and were incubated in dark at 28°C for 4 days. Growth inhibition zones of the target isolate around the spot-inoculated isolates were evaluated if any (de Boer et al., 1999).
To determine whether the selected isolates do not inhibit each other by their antibacterial volatile compounds, the method described by Payne et al. (2000) was used. Fresh OMYEA plates were inoculated by spreading 0.2 ml of 108 cfu ml–1 of each isolate onto the surface of OMYEA, and plates were incubated at 28°C in dark for 5 days. After incubation, another fresh OMYEA plate was simultaneously inoculated with an actively growing culture of the tested isolate. Lids were removed, the plates containing the tested isolate were inverted over the previously grown isolate, and the two plate bases were taped together with Parafilm (American National Can TM, Greenwich, CT, USA). Non-inoculated plates were used as a control. The growth of the target isolate was compared to that of the control after 5 days of incubation at 28°C in dark (Payne et al., 2000). For each isolate, four independent replicates were used in these two tests.
Preparation of Actinobacterial Inoculum
Inocula of each isolate were prepared for sediment application by adding 300 g of oat bran as a food base in 500-ml Erlenmeyer flasks, which were autoclaved at 121°C for 20 min on 3 successive days. Oat bran was then aseptically inoculated with the spore suspensions (25 ml) of each isolate in 20% glycerol (~108 cfu ml−1) and incubated at 28°C in dark for 3 weeks. Non-colonized oat bran autoclaved two times served as a control (El-Tarabily et al., 2020). Oat bran colonized with each isolate (0.1 g colonized oat bran inoculum g−1 air-dried non-sterile sediment) was mixed thoroughly using a cement mixer in all aforementioned experiments.
Liquid inocula were also prepared for their application in the aforementioned greenhouse experiment. Isolates were grown in ISSB and shaken on an orbital shaker incubator at 250 rpm at 28°C in dark for 8 days. Each actinobacterial suspension was adjusted to be ~108 cfu ml−1.
Inoculation and Assessment of Plant Growth Characteristics in the Greenhouse
At low tide, surface mangrove sediments (20-cm depth) were collected with a clean spade at a distance of <25 cm near young mangrove trees (<70 cm tall). Sediments were air-dried, sieved, and stored in plastic bags in the greenhouse at 28 ± 2°C.
Oat bran colonized with each isolate (0.1 g colonized oat bran inoculum g−1 air-dried non-sterilized sediment) was mixed thoroughly using a cement mixer. In total, there were six treatment combinations: (1) control (C) autoclaved colonized oat bran without actinobacteria; individually inoculated with either (2) P-solubilizing isolate #2, (3) auxin-producing isolate #15, (4) PAs-producing isolate #6, or (5) ACCD-producing isolate #37; and (6) collectively inoculated with all four isolates as a consortium.
Surface-sterilized propagules of almost similar dimensions were germinated into free-draining pots (36 cm in diameter), filled with 14 kg of mangrove sediments containing six combinations, and irrigated with full-strength seawater (salinity of 4.0%) to container capacity.
Seedlings were maintained at 28 ± 2°C for 9 months under greenhouse conditions (mid-day photosynthetic photon flux density = 700 μmol m−2 s−1; average relative humidity = 60% ± 5%). In the treatments (2–6), additional applications (100 ml per pot) of actinobacterial inoculum suspended in ISSB (108 cfu ml−1) were made at the end of the 1st month and repeated bimonthly up to 9th month in a randomized complete block design (RCBD). Each treatment was replicated eight times, and each replicate was determined by a single pot containing one seedling. Greenhouse trials were conducted in 2018 and independently repeated in 2019.
The growth of 9-month-old plants was assessed at the end of greenhouse trials by recording the number of branches, total leaf areas, dry weights, and lengths of roots and shoots.
Determination of Photosynthetic Pigments and Gas Exchange
Contents of chlorophylls (chl a and b) and carotenoids (Davies, 1965; Holden, 1965) were determined in the leaves of 9-month-old plants. Sixteen replicate samples from two independently repeated experiments were analyzed for each treatment. Shimadzu UV-2101/3101 PC scanning spectrophotometer (Shimadzu Corporation Analytical Instruments Division, Kyoto, Japan) was used to measure the optical densities of chl a and b at 663 and 645 nm wave length, respectively (Holden, 1965).
Assimilation (A, μmol m−2 s−1) vs. sub-stomatal CO2 concentration (Ci, Pa) response curve (A:Ci) of leaves was measured for at least two leaves per replicate using portable photosynthesis open systems (Li-6400; Licor Inc., Lincoln, NE, USA; Youssef, 2007). Measurements for six replicates were recorded from two independently repeated experiments per treatment. The calculations of gas exchange parameters were performed (von Caemmerer and Farquhar, 1981). The data of each A:Ci curve were fitted into a non-rectangular hyperbola equation (Jones, 1992) using the Photosynthesis Assistant Software (v1.1, Dundee Scientific, Scotland, UK).
Mechanistic analysis of each A:Ci curve was performed using the model proposed by Farquhar et al. (1980) as subsequently modified by Harley et al. (1992). The model identifies the three critical phases in the A:Ci response curve. The first is the initial response below Ci concentration of ~20 Pa where ribulose 1,6-biphosphate (RuBP) is saturated and rubisco enzyme activity limits the carboxylation process. The slower rise of the curve beyond its inflection point represents the second phase. The higher Ci level within this phase results in the limiting factor being the supply of RuBP. The curve plateau represents the third phase, which indicates the availability of inorganic P for Calvin cycle. The model was used in this study to estimate the maximal rubisco-catalyzed carboxylation velocity (Vcmax), maximal electron transport rate (Jmax), and triose phosphate utilization rate (TPU).
Extraction and Measurements of Auxins, PAs, and ACC in Plant Tissues
Tissues of the terminal part of the root and shoot systems were used for the analysis of auxins, PAs, and ACC. At the end of the experiment, the extraction of IAA and IPYA from plant tissues was carried out according to Guinn et al. (1986), and the HPLC parameters were applied as described by Tien et al. (1979). The extraction of Put, Spd, and Spm was carried out using the method described by Flores and Galston (1982). The derivatization of the authentic PAs and plant extracts was carried out through the addition of benzoyl chloride (Sigma-Aldrich) (Redmond and Tseng, 1979), and HPLC chromatograms were produced according to Smith and Davies (1985).
In planta ACC was extracted according to Lizada and Yang (1979). The derivatization of ACC was performed by the addition of phenylisothiocyanate (Sigma-Aldrich), and the analytical HPLC method was used for a direct quantification of ACC (Lanneluc-Sanson et al., 1986). Sixteen replicate samples from two independently repeated experiments were analyzed for auxins, PAs, and ACC for each treatment.
Plant Nutrient Analyses
Shoot and root tissues were collected from 9-month-old plants by the end of the experiment, washed with deionized water, and oven-dried for 48 h at 60°C. For N measurements, the ground plant material was combusted at 950°C in oxygen using the LECO FP-428 Nitrogen Analyzer (Sweeney and Rexroad, 1987). N release from samples was measured using a thermal conductivity cell. For other minerals (P, K, S, Mg, Fe, Zn, and Cu), a plant material was digested in a mixture of nitric and perchloric acid (9:1) and measured by inductively coupled plasma atomic emission spectrometry (ICPAES; McQuaker et al., 1979). Sixteen replicate samples from two independently repeated experiments were analyzed.
Statistical Analyses
In all experiments, treatments were arranged in an RCBD. Eight independent replicates for each isolate were used for the in vitro PGP experiments. Experiments conducted in greenhouses were independently repeated two times with similar results, combined and analyzed. The data of PD were transformed into log10 cfu g−1 dry sediment. The R% were arc-sine transformed before ANOVA was carried out. To evaluate the effect of different actinobacterial isolates at the end of the greenhouse trials on plant growth, the levels of chl a, chl b, carotenoids, photosynthetic exchange characteristics, levels of in planta PGRs, and nutrient levels, data were subjected to ANOVA. Significant differences between the means were compared using Fisher's Protected LSD Test at p = 0.05. Data analyses were performed using SAS Software version 9 (SAS Institute Inc., Cary, NC, USA).
Results
Isolation and Screening of Halotolerant Actinobacterial Isolates
The populations of total aerobic actinobacteria in the mangrove rhizosphere were found to be 4.12 ± 1.71 log10 cfu g−1 dry sediment. According to their cultural and morphological characteristics, a total of 62 actinobacterial isolates were identified, purified on OMYEA plates, and stored at 4°C. All isolates grew well and sporulated heavily on ISSA medium supplied with 4% NaCl, but only 33 (53.23%) showed increased tolerance to 8% NaCl (Figure 1A) and were considered as true halotolerant isolates.
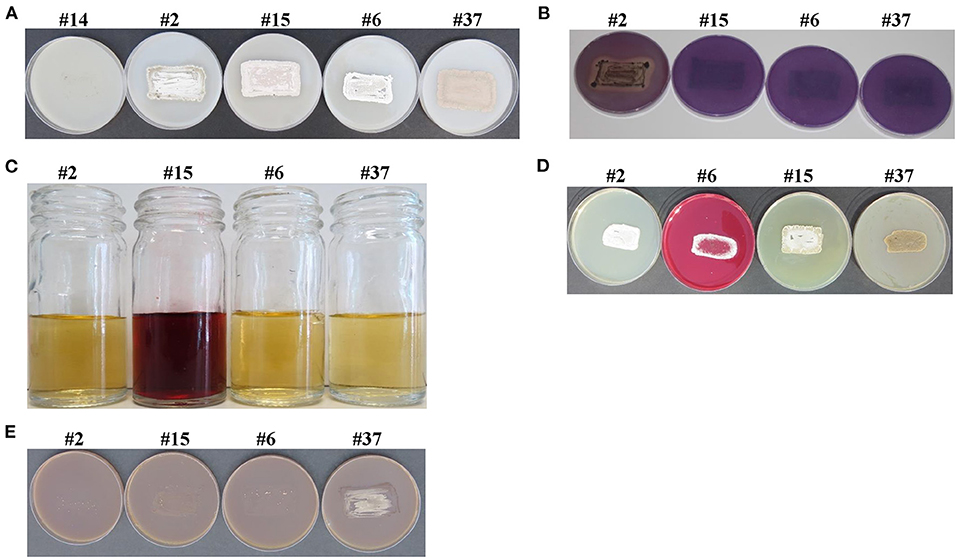
Figure 1. Salinity tolerance and in vitro plant growth-promoting characteristics of selected actinobacterial isolates. Selected actinobacterial isolates possessing (A) salinity tolerance; (B) the solubilization of P; the production of (C) IAA; (D) Put; and (E) ACCD. In (A), actinobacteria #2, #15, #6, and #37, but not #14 (control), were considered as salt-tolerant isolates due to growth and heavy sporulation on inorganic salt starch agar (ISSA) supplemented with 8% NaCl. Actinobacterial strain #14 was used as a control isolate. In (B), isolates were tested on Pikovskaya's agar medium amended with rock phosphate (RP) and bromophenol blue; and the production of clear zone surrounding the colony of isolate #2, but not #15, #6, or #37 was used as an indicator of P-solubilization. In (C), the addition of Salkowski reagent to cultures grown in inorganic salt starch broth (ISSB) amended with L-tryptophan; and the formation of red color in isolate #15, but not #2, #6, or #37, indicated the production of the auxin, IAA. In (D) isolates were tested on Moeller's decarboxylase agar medium (MDAM) amended with L-arginine-monohydrochloride; and the change from yellow to red color of the phenol red in isolate #6, but not #2, #15, or #37 indicated the production of the polyamine (PA), Put. In (E), isolates were inoculated on N-free Dworkin and Foster's salts minimal agar medium amended with ACC; where growth and heavy sporulation in isolate #37, but not #2, #15, or #6, indicated the efficiency to utilize ACC and the production of ACCD. P, phosphorus; IAA, indole-3-acetic acid; Put, putrescine; ACC, 1-aminocyclopropane-1-carboxylic; ACCD, 1-aminocyclopropane-1-carboxylic deaminase.
In addition, the 33 halotolerant actinobacterial isolates were in vitro screened, and 19 isolates (57.57%) were found to be the colonizers of mangrove roots after 21 days using the preliminary qualitative indicator root colonization jar bioassay. Isolates #2, #6, #15, #28, #33, #37, and #40 colonized up to 100% of the root length, whereas #9, #16, #21, and #35 colonized up to 85%. Three (#4, #19, and #23) and five (#11, #22, #31, #39, and #41) isolates colonized up to 70% and 50% of the roots after 21 days, respectively. The remaining 14 isolates were not included in the subsequent rhizosphere-competence assays as they failed to colonize the roots in vitro.
Under naturally competitive conditions, seven isolates (#11, #19, #22, #23, #31, #39, and #41), which colonized up to 50–70% in the in vitro jar bioassay, also completely failed to colonize roots or associated rhizosphere sediment in the mangrove sediment tube assay. The rhizosphere-competence, expressed as R%, of the other 12 root-colonizing isolates (#2, #4, #6, #9, #15, #16, #21, #28, #33, #35, #37, and #40) showed that roots and sediment particles attached to the roots of the 30-day-old mangrove seedlings were colonized to different degrees (Table 1).
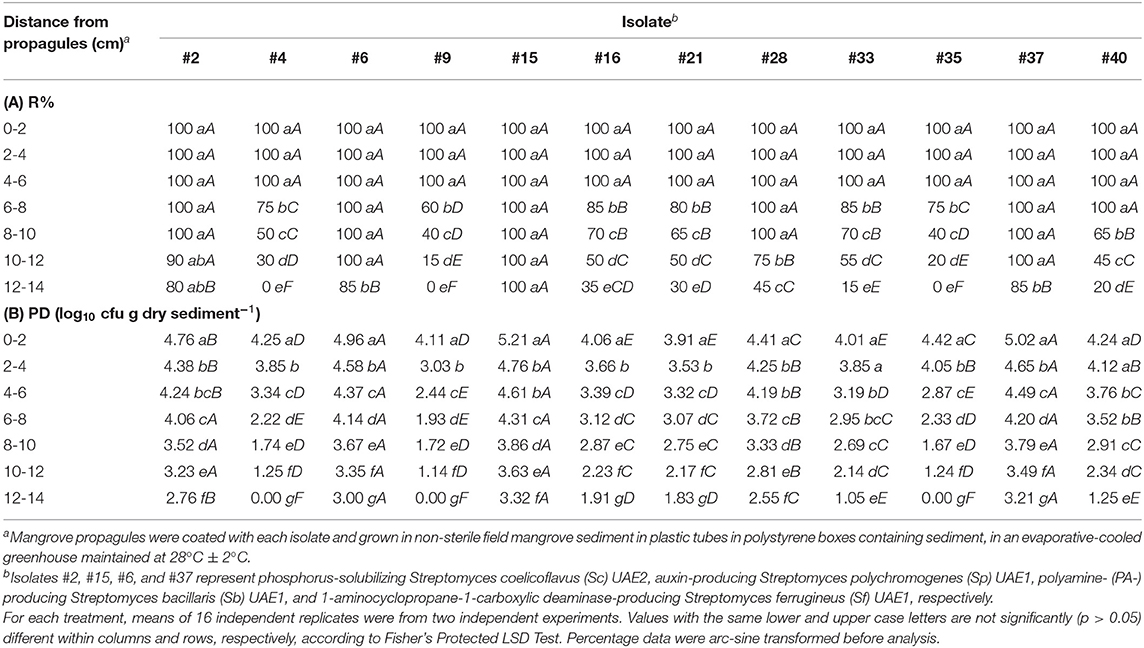
Table 1. Root-colonization frequencies (R%) of 12 halotolerant marine actinobacteria in the root segments of mangrove (Avicennia marina) and the population densities (PD) of the actinobacterial isolates in mangrove rhizosphere sediment 30 days after planting the treated mangrove propagules in the non-sterile sediment tube rhizosphere competence assay.
The PD also showed that the isolates were found in the rhizosphere at all depths of the roots but were significantly (p < 0.05) greater in the first 8 cm of the root system compared with other root depths (Table 1). The strongest 9 (out of 12) rhizosphere-competent isolates (#2, #6, #15, #16, #21, #28, #33, #37, and #40), which colonized the entire roots up to 14 cm depth (Table 1), were selected for further studies for their PGP characteristics. These nine isolates showed strong R% (≥65%) and PD (log10 cfu g−1 dry sediment >2.69) at the root depths of 8–10 cm (Table 1). The same isolates showed significantly (p < 0.05) higher R% and PD at depths of 8–10, 10–12, and 12–14 cm compared with the weak rhizosphere-competent isolates (#4, #9, and #35). Accordingly, these three isolates were not included because they showed poor R% (<50%) and PD (log10 cfu g−1 dry sediment <2) at the root depths of 8–10, 10–12, and 12–14 cm (Table 1).
In vitro Screening of the PGP Activities of the Isolates
The PGP activities of the best performing nine rhizosphere-competent isolates were also tested in vitro. P-solubilization abilities varied among isolates (Table 2). Four isolates (#2, #16, #21, and #40) were able to solubilize P (Table 2; Figure 1B). Isolates #16, #21, and #40 also produced other PGRs at high levels, whereas #2 produced no or negligible amounts of PGRs in vitro (Table 2; Figures 1C–E). A significant (p < 0.05) reduction in the pH and a concurrent significant (p < 0.05) increase in the concentration of the released P were also detected in culture broth of only isolates #2, #16, #21, and #40 compared with control or other isolates (Table 2). The highest percentage of P-solubilization and the most pronounced drop in medium pH were achieved by isolate #2 and was chosen for further experiments.
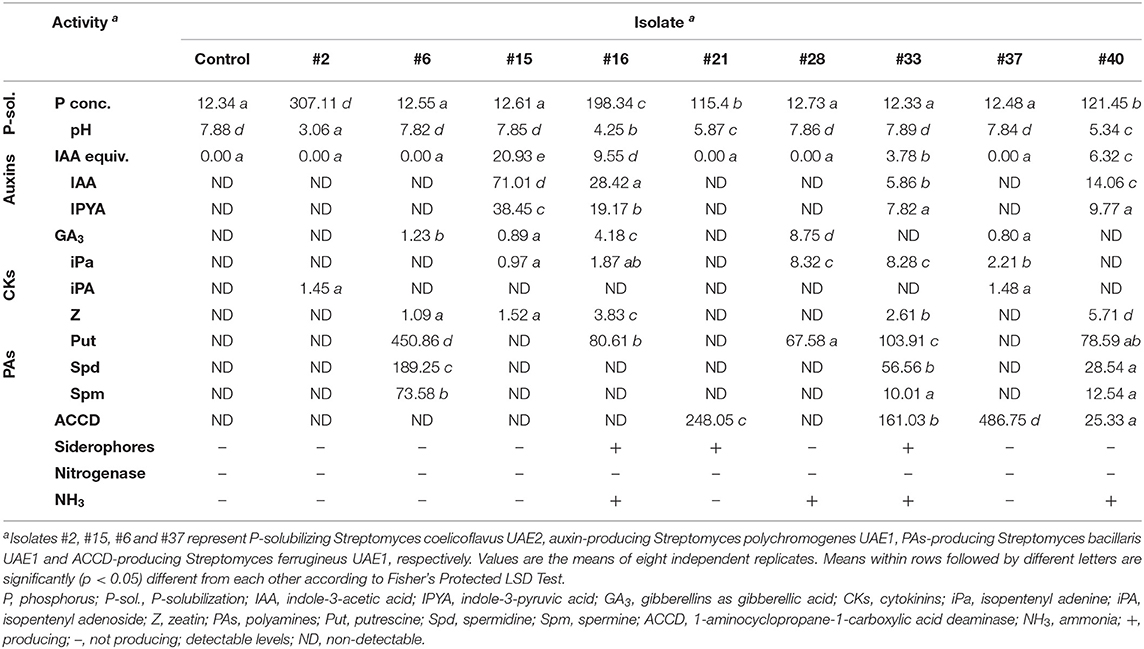
Table 2. In vitro production of plant growth regulators (PGRs) (μg ml−1), ACCD (nanomoles α-keto-butyrate mg−1 protein h−1), siderophores, nitrogenase enzyme, NH3; and the effect of inoculation with rock phosphate (RP) amended Pikovskaya's broth with the best performing rhizosphere-competent isolates obtained from the mangrove rhizosphere sediment on pH levels and concentrations of available P (mg L−1).
The production of auxins (e.g., IAA) significantly (p < 0.05) varied among actinobacterial species using the colorimetric analysis (Table 2). IAA was detected in the liquid cultures of isolates #15, #16, #33, and #40; other isolates did not produce IAA. The formation of the dark red color after the addition of the Salkowski reagent indicated that this is an IAA-producing isolate, i.e., #15 (Figure 1C). The three other isolates (#16, #33, and #40); however, showed other in vitro PGP characteristics (Table 2). Therefore, they were eliminated from subsequent experiments. The production of IAA and IPYA in the culture extracts of the selected nine isolates was further tested by HPLC analysis. The results showed variations among species in their ability to produce the IAA and IPYA (Table 2). The highest production of IAA and IPYA was detected by isolate #15.
We also screened the capability of isolates to produce Put on MDAM plates amended with L-arginine-monohydrochloride. We observed relatively moderate to large dark red halo around and beneath the colonies of isolates #6, #16, #28, #33, and #40; thus, indicating the production of Put (Table 2; Figure 1D). The remaining isolates did not produce Put because red halo was absent. HPLC analysis of the culture extracts of the isolates showed variations among species in their ability to produce the three types of PAs (Table 2). Isolates #6, #16, #28, #33, and #40 showed a range of Put production between 67.58 and 450.86 μg ml−1. On the other hand, isolates #6, #33, and #40 produced 28.54–189.25 μg ml−1 of Spd and 10.01–73.58 μg ml−1 of Spm, respectively (Table 2). Thus, we selected isolate #6 for further studies because this particular strain produced not only Put but also Spd and Spm at high levels in vitro.
On DF+ACC agar plates, only isolates #21, #33, #37, and #40 grew and sporulated. The rest grew on control medium only. This was confirmed by the qualitative estimation of ACCD activity among isolates, which exhibited moderate to high levels of ACCD activity (Table 2). Thus, isolate #37 was considered as an ACCD producer (Figure 1E) and was selected for further studies.
Isolates #16, #21, and #33 were able to produce siderophores (Table 2; Supplementary Figure 1). The production of GA3 was detected in five isolates (#6, #15, #16, #28, and #37; Table 2). The production of CKs (iPa, iPA, and Z) also varied among the nine tested isolates (Table 2). Isolates #15, #16, #28, #33, and #37 produced 0.97, 1.87, 8.32, 8.28, and 2.21 μg ml−1 of iPa, respectively. For iPA production, 1.45 μg ml−1 was detected in isolate #2 and 1.47 μg ml−1 in isolate #37. Isolates #6, #15, #16, #33, and #40; however, produced 1.09, 1.52, 3.83, 2.61, and 5.71 μg ml−1 of Z, respectively. None of the selected four isolates fixed N or produced NH3 (Table 2).
Isolates #2 (P-solubilizer), #15 (auxins-producer), #6 (PAs-producer), and #37 (ACCD-producer) were further included in the subsequent analyses/experiments for their single mode of action. Although isolates #16 and #40 showed strong rhizosphere-competence, they were excluded because they produced multiple PGRs.
Identification of Candidate PGPA Isolates
Nucleotide sequences of the 16S rRNA gene amplicons from the isolates were compared to those representing other actinobacterial species. Based on the evolutionary distance values using 16S rRNA gene sequences, the four actinobacterial candidates belonged to the genus Streptomyces species. The complete 16S rRNA gene sequence of isolate #2 (P-solubilizing) showed full 100% similarity with Streptomyces coelicoflavus NBRC 15399T (AB184650); the remaining Streptomyces spp. showed <99.5% similarities (Supplementary Figure 2). Gray aerial mycelia were produced, and yellow substrate (vegetative) mycelia were detected after 7 days of incubation on ISP3 medium with no diffusible pigment (Supplementary Figure 2). The configuration of the spore chains of isolate #2 belonged to the section spirales, and the strain formed open spiral chains consisting of 25–60 mature, smooth-surfaced spores on the aerial hyphae (Supplementary Figure 2). This suggests that isolate #2 can be regarded as S. coelicoflavus (Sc) (Gauze et al., 1983; Terekhova, 1986) strain UAE2.
Phylogenetic analysis of the auxin-producing strain #15 showed 99.8%, 99.7%, and 99.2% similarity to Streptomyces polychromogenes NBRC 13072T (NR043499), Streptomyces racemochromogenes NRRL B-5430T (NR043499), and Streptomyces katrae NBRC 13447T (NR041136), respectively. The other Streptomyces spp. showed <98.9% similarity with isolate #15 (Supplementary Figure 3). To recognize the exact species, cultural and morphological features were considered. The pure cultures produced grayish yellow substrate mycelia and yellowish-pink aerial mycelia after incubation for 7 days on ISP3 medium (Supplementary Figure 3). The reverse side of colony was with grayish yellow to light yellow pigment on ISP3 medium. Spore chains were long and straight with >50 spores per chain, which belonged to the section rectiflexibiles. In addition, the isolate possessed a smooth, nest-like tangled aerial mycelium (Supplementary Figure 3). These distinctive characteristics of isolate #15 are the common features of S. polychromogenes (Sp) Hagemann et al. (1964) strain UAE1.
The PAs-producing isolate #6 was phylogenetically grouped in the same clade as Streptomyces bacillaris NBRC 13487T (AB184439) with 100% similarity (Figure 2A). Yellow color of aerial mycelium and yellowish-brown color of substrate mycelium with a trace of yellow diffusible pigments were produced by isolate #6 on ISP3 medium (Figure 2B). When the strain was visualized using SEM, we observed smooth spores forming long chains (section rectiflexibiles) of 10–50 spores per chain (Figure 2C). This indicated that isolate #6 can most probably be S. bacillaris (Sb) (Krasil'nikov, 1958; Pridham, 1970) strain UAE1.
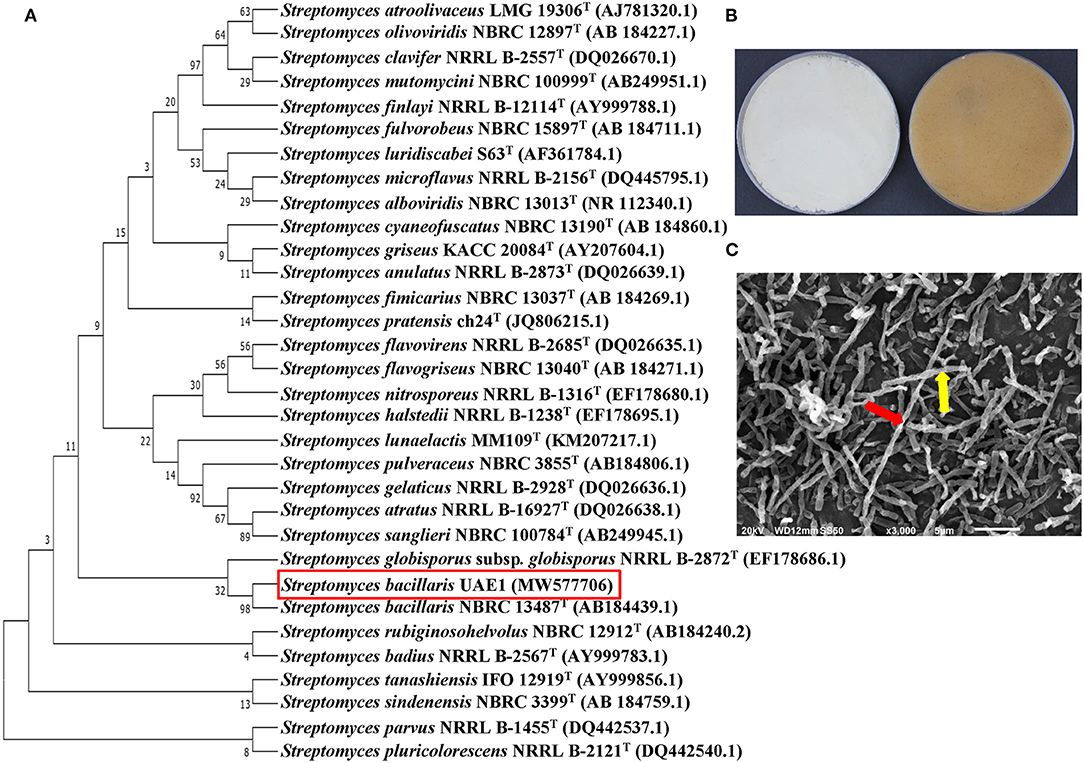
Figure 2. Taxonomic identification of the PAs-producing Streptomyces bacillaris (Sb) UAE1. (A) The dendrogram showing the phylogenetic relationships between Sb UAE1 (isolate #6; MW577706) and other members of Streptomyces spp. based on 16S ribosomal RNA (rRNA) sequences. (B) Yellow aerial (left) and yellowish-brown substrate (right) mycelia growing on ISP3 medium supplemented with yeast extract; and (C) scanning electron micrograph (3,000X) of long and rectiflexibiles spore chains (red arrow) and smooth-surfaced spores (yellow arrow) of Sb UAE1. In (A), numbers at nodes indicate the percentage levels of bootstrap support based on a neighbor-joining analysis of 500 resampled data sets. GenBank accession numbers are given in parentheses. PAs, polyamines.
In addition to its cultural and morphological characteristics, we phylogenetically analyzed ACCD-producing isolate #37 to distinguish it from the closely related species. This strain showed 99.6% similarity to Streptomyces ferrugineus HV38T, whereas the rest of Streptomyces spp. showed less than 98.5 (Figure 3A). On ISP3 medium, a whitish-greenish gray aerial mycelium, red substrate mycelium, and fuchsia-colored pigment were produced by isolate #37 (Figure 3B). This strain developed smooth spores (0.5–1.5 μm) (Figure 3C). Under SEM, spore chains appeared rectiflexibiles; each consisted of 10–20 spores per chain. This suggests that isolate #37 can be considered as S. ferrugineus (Sf) (Ruan et al., 2015) strain UAE1.
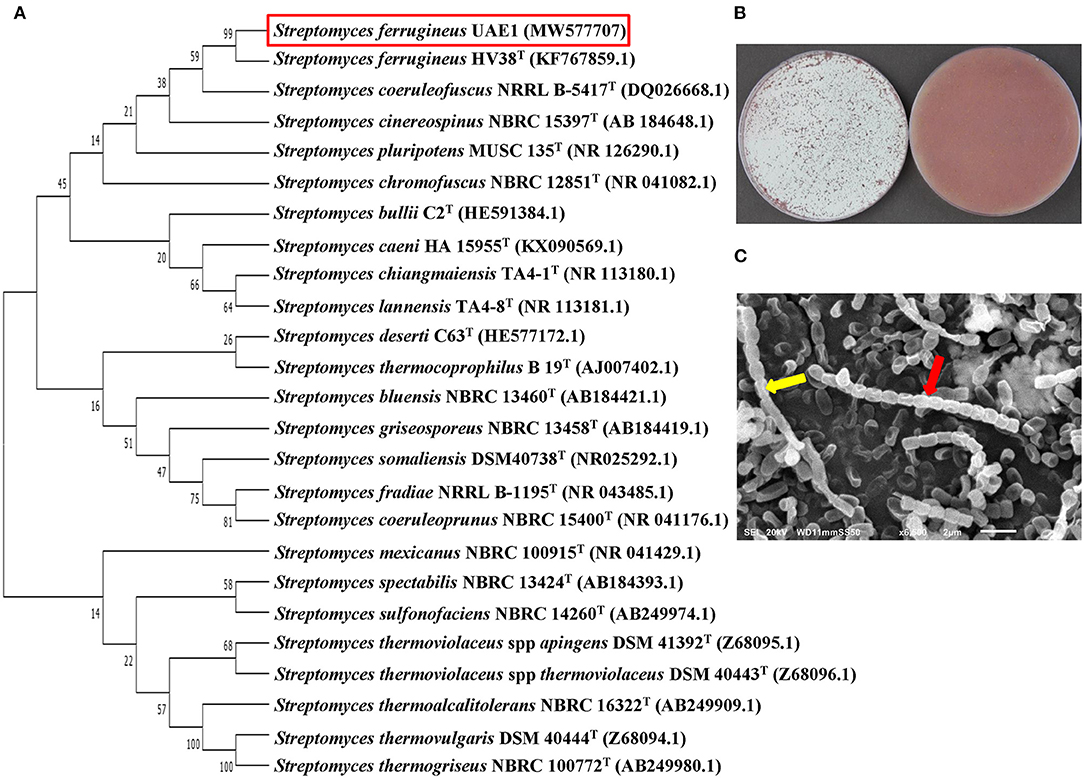
Figure 3. Taxonomic identification of the ACCD-producing Streptomyces ferrugineus (Sf) UAE1. (A) The dendrogram showing the phylogenetic relationships between Sf UAE1 (isolate #37; MW577707) and other members of Streptomyces spp. based on 16S ribosomal RNA (rRNA) sequences. (B) whitish-greenish gray aerial (left) and red substrate (right) mycelia growing on ISP3 medium supplemented with yeast extract; and (C) scanning electron micrograph (6,500X) of the rectiflexibiles spore chains (red arrow) and smooth-surfaced spores (yellow arrow) of Sf UAE1. In (A), numbers at nodes indicate the percentage levels of bootstrap support based on a neighbor-joining analysis of 500 resampled data sets. GenBank accession numbers are given in parentheses. ACCD, 1-aminocyclopropane-1-carboxylic deaminase.
Together, the promising rhizosphere-competent actinobacteria were identified as P-solubilizing Sc UAE2 (isolate #2), auxin-producing Sp UAE1 (isolate #15), PAs-producing Sb UAE1 (isolate #6), and ACCD-producing Sf UAE1 (isolate #37) isolates.
There was no evidence of inhibitory/killing effects of the volatile compounds of one isolate on the growth of others. Moreover, no diffusible antibacterial metabolites were produced by the four species of Streptomyces because the sprayed target isolates were not inhibited by the spot-inoculated isolate, indicating that these isolates were compatible and can be mixed safely as an actinobacterial consortium.
Evaluation of Mangrove Growth Promotion Under Greenhouse Conditions
In the greenhouse, we found that the application of the four isolates either individually or in a combination significantly (p < 0.05) promoted mangrove growth compared to the control (Figure 4A). Thus, the consortium of isolates (Sc/Sp/Sb/Sf) showed the best growth performance. Compared to non-inoculated control plants, individual treatments of Sc or Sp significantly (p < 0.05) increased the dry biomass of shoots and roots (Figure 4B); however, there was no significant (p > 0.05) difference in the tissue dry mass between the two actinobacterial treatments. Sb and Sf treatments increased the dry biomass of shoots by 58.9 and 74.0%, and roots by 60.1 and 75.9%, respectively, in comparison with mangrove control plants. On comparison of the treatment of mangrove seedlings with Sc/Sp/Sb/Sf to no treatment, significant (p < 0.05) increases in the tissue dry weights (82.1% in shoots and 81.6% in roots; Figure 4B) and lengths (77.3% in shoots and 81.0% in roots; Figure 4C) were observed.
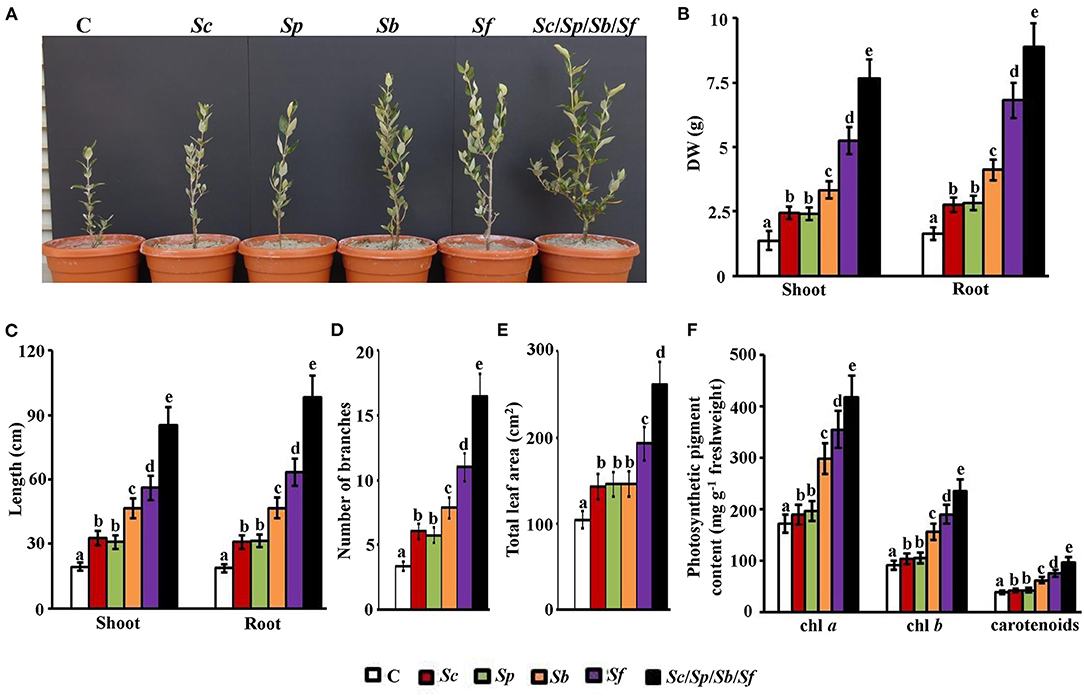
Figure 4. Effect of the actinobacterial isolates on the growth and development of mangrove. Effect of the treatment of the P-solubilizing, auxin-, PAs-, and ACCD-producing isolates on the (A) performance; (B) DW; and (C) length of shoot and root tissues; (D) number of branches, (E) total leaf area, and (F) photosynthetic pigment contents of chl a, chl b, and carotenoids on mangrove seedlings. In (A–F), the figure, dry weight and length of shoot and root tissues, number of branches, total leaf area, and photosynthetic pigments were measured at 9 months post planting the propagules of mangrove. Values are the means of 16 replicates for each sampling from two independent experiments. Mean values followed by different letters are significantly (p < 0.05) different from each other according to Fisher's Protected LSD Test. Bars represent SE. DW, dry weight; control (autoclaved non-inoculated oat bran); Sc, P-solubilizing isolate #2 (Streptomyces coelicoflavus UAE2); Sp, auxin-producing isolate #15 (Streptomyces polychromogenes UAE1); Sb, PAs-producing isolate #6 (Streptomyces bacillaris UAE1); Sf, ACCD-producing isolate #37 (Streptomyces ferrugineus UAE1); Sc/Sp/Sb/Sf, consortium of the four isolates. P, phosphorus; PAs, polyamines; ACCD, 1-aminocyclopropane-1-carboxylic deaminase; chl, chlorophyll.
On the treatment of seawater-irrigated plants with either Sc or Sp, there was a significant (p < 0.05) increase in the length of shoots (37.3–40.1%) and roots (39.6–40/6%) compared to the control treatment (Figure 4C). On the treatment of seedlings with Sb or Sf, we found that the length of both shoots and roots increased significantly (p < 0.05) compared to control. Thus, Sc/Sp/Sb/Sf revealed the greatest growth among all treatments. Similar observations were found in the number of the side branches of the vegetative growth of mangrove; where the treatment of mangrove with the four isolates resulted in the highest number of branches per plant (Figure 4D). Although the total leaf area was not significantly (p > 0.05) different among plants supplemented with either Sc, Sp, or Sb, the same treatments significantly (p < 0.05) increased leaf area compared to control plants (Figure 4E). The combination of the four isolates showed the largest increases in the total area of leaves (60.2%) compared to non-inoculated control plants. To a lesser extent, Sf treated plants showed an increase of 46.0%.
Except between Sc and Sp, there were significant (p < 0.05) differences in the contents of all photosynthetic pigments tested among treatments (Figure 4F). Thus, the plants grown in sediments amended with Sf had significantly (p < 0.05) higher levels of chlorophylls (a and b) and carotenoids than those plants grown in sediments amended with single inoculum of Sc, Sp, or Sb. Not surprisingly, the highest content of the three pigments was found in the plants treated with the group of isolates (Figure 4F).
Assessment of Photosynthetic Gas Exchange Parameters in Mangrove Plants
In general, all intrinsic photosynthetic gas exchange components were significantly (p < 0.05) enhanced by the application of the selected PGPA either individually or as a mixture compared to control plants (Figure 5). The particular strain of Sf played a dominant role in this photosynthetic enhancement of the three measured parameters (Vcmax, Jmax, and TPU) while Sc, Sp, and Sb were less effective. The PAs-producing isolate (Sb) significantly enhanced both Vcmax and Jmax compared to P-solubilizing (Sc) or auxin-producing (Sp) isolates while the values were significantly (p < 0.05) different from the control (Figures 5A,B). The Vcmax increased by more than three-fold in plants treated with the mixture of four actinobacteria over control (Figure 5A). The Vcmax, however, was insignificantly (p > 0.05) different between the plants treated with the mixture of PGPA (Sc/Sp/Sb/Sf) and ACCD-producing isolate (Sf); thus, these treatments attained higher Vcmax values than control plants (Figure 5A).
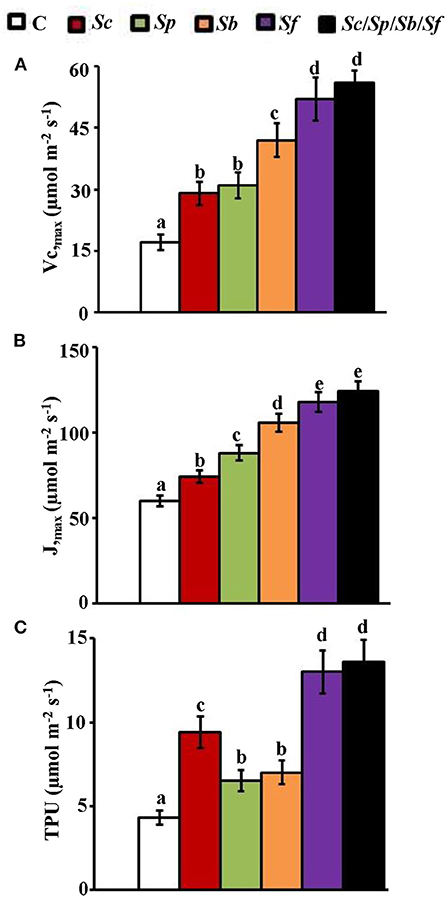
Figure 5. Estimation of photosynthetic parameters in mangroves after sediment inoculation with PGPA isolates. In planta contents of (A) Vcmax; (B) Jmax; and (C) TPU in mangrove shoot tissues after treatment with control, Sc, Sp, Sb, Sf, and Sc/Sp/Sb/Sf. Mangrove seedlings were grown in an evaporative-cooled greenhouse (28 ± 2°C). The values for each sampling are the means of six replicates from two independent experiments. Mean values followed by different letters are significantly (p < 0.05) different from each other according to Fisher's Protected LSD Test. Bars represent SE. Vcmax, Jmax, and TPU were measured at 9 months post planting the propagules of mangrove. Vcmax, maximal rubisco-catalyzed carboxylation velocity; Jmax, maximal electron transport rate; TPU, triose phosphate utilization rate; C, control (autoclaved non-inoculated oat bran); Sc, P-solubilizing isolate #2 (Streptomyces coelicoflavus UAE2); Sp, auxin-producing isolate #15 (Streptomyces polychromogenes UAE1); Sb, PAs-producing isolate #6 (Streptomyces bacillaris UAE1); Sf, ACCD-producing isolate #37 (Streptomyces ferrugineus UAE1); Sc/Sp/Sb/Sf, consortium of the four isolates; P, phosphorus; ACCD, 1-aminocyclopropane-1-carboxylic deaminase.
In addition, Jmax necessary for RuBP regeneration gradually increased from non-inoculated plants to plants treated with single or combined isolates (the order from the lowest to highest values of Jmax was: control < Sc<Sp<Sb<Sf =Sc/Sp/Sb/Sf; Figure 5B). The approach of using mixed inocula of actinobacteria drastically increased Jmax values by two-fold of that in control (Figure 5B). The TPU rate, which measures the availability of P to Calvin cycle, significantly (p < 0.05) increased in all treatments compared to control seedlings (Figure 5C); thus, appeared to be three-fold in plants treated with a mixture of isolates. None of the mangrove seedlings treated with Sp or Sb were significantly (p > 0.05) different from each other. Thus, the TPU of these plants was significantly (p < 0.05) lower compared to plants treated with Sc, Sf, or the consortium of isolates (Figure 5C).
Overall, our data indicated that the consortium of isolates greatly stimulated the growth of mangrove in comparison to any other single application of isolates. Thus, this could be attributed to the synergistic interactions of these isolates using multiple PGP mechanisms.
Determination of Hormonal and Nutrient Levels in Mangrove Tissues
To figure out the plausible cause of Sc, Sp, Sb, and Sf in the growth promotion of mangrove, we measured the levels of auxins, PAs, ACC, and nutrients in planta. We noticed that mangrove seedlings grown in sediments amended with Sp or a mixture of the four actinobacteria had significantly (p < 0.05) higher levels of IAA (Figure 6A) and IPYA (Figure 6B) in shoots and roots than in seedlings grown in sediments amended with Sc, Sb, Sf, or control treatment.
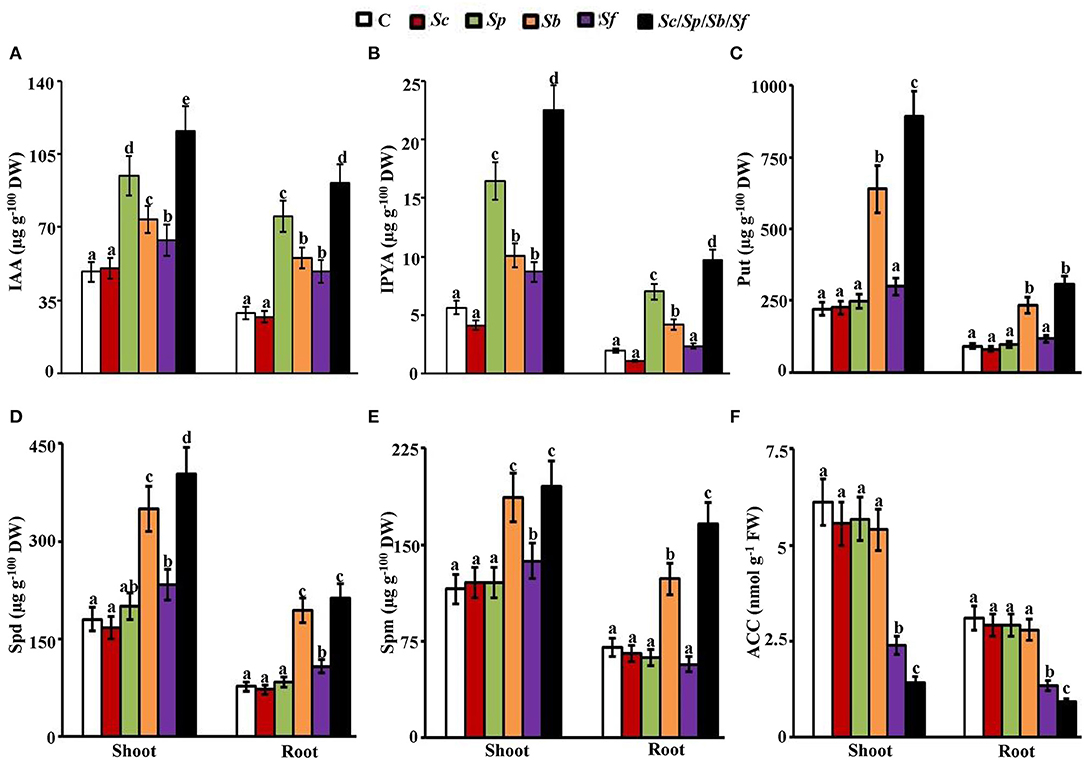
Figure 6. Effect of actinobacterial isolates on auxins, PAs, and ACC content of mangrove tissues. In planta contents of (A) IAA; (B) IPYA; (C) Put; (D) Spd; (E) Spm; and (F) ACC in mangrove shoot and root tissues after treatment with control, Sc, Sp, Sb, Sf, and Sc/Sp/Sb/Sf. Mangrove seedlings were grown in an evaporative-cooled greenhouse (28 ± 2°C). The values for each sampling are the means of 16 replicates from two independent experiments. Mean values followed by different letters are significantly (p < 0.05) different from each other according to Fisher's Protected LSD Test. Bars represent SE. In planta contents of IAA, IPYA, Put, Spd, Spm, and ACC were measured at 9 months post planting the propagules of mangrove. DW/FW, dry/fresh weight; C, control (autoclaved non-inoculated oat bran); Sc, P-solubilizing isolate #2 (Streptomyces coelicoflavus UAE2); Sp, auxin-producing isolate #15 (Streptomyces polychromogenes UAE1); Sb, PAs-producing isolate #6 (Streptomyces bacillaris UAE1); Sf, ACCD-producing isolate #37 (Streptomyces ferrugineus UAE1); Sc/Sp/Sb/Sf, consortium of the four isolates; P, phosphorus; IAA, indole-3-acetic acid; IPYA, indole-3-pyruvic acid; PAs, polyamines; Put, putrescine; Spd, spermidine, Spm, spermine; ACC, 1-aminocyclopropane-1-carboxylic; ACCD, ACC deaminase.
Applications of Sb or the four PGPA isolates significantly (p < 0.05) increased the levels of Put (Figure 6C), Spd (Figure 6D), and Spm (Figure 6E) in both shoots and roots, compared to those treatments of other single isolates of Sc, Sb, Sf, or control. Seedlings grown in sediments amended with Sf or the mixture of the tested isolates had significantly (p < 0.05) lower levels of ACC in both plant tissues than in the application of Sc, Sp, Sb, or the control (Figure 6F). In all treatments, auxins, PAs, and ACC measured in shoots were relatively higher than in roots (Figure 6). The overall IAA, IPYA, Put, Spd, and Spm levels were found to be the highest, but ACC content was the lowest in both tissues of the Sc/Sp/Sb/Sf treatment among other treatments (Figure 6). This suggests that the production of PGRs by individual actinobacterial isolates detected from the in vitro assays could possibly have positive effects on plant growth, thus the synergistic consortium of isolates might develop additive impact for better plant health.
To unravel how the P-solubilizing isolate potentially enhanced the growth of the treated mangrove, we analyzed the available P concentrations in sediments and the concentrations of P and other nutrients in the tissues of mangrove. In general, we did not find any significant (p > 0.05) difference in the contents of the major or minor nutrients in any of the tested tissues in plants treated with Sp, Sb, or Sf. This suggests that these isolates promoted the growth of plants via other mode of actions, i.e., the regulation of phytohormones/PAs.
We noticed that the isolate Sc amended in mangrove sediments significantly (p < 0.05) increased not only available P in sediments, but also in shoot and root tissues compared to control treatment (Table 3). In the treatment consisting of all four isolates combined, there was more P in sediments and plant tissues than in the control treatment. This suggests that P-solubilizing isolate Sc positively regulates the availability of P levels in sediments, thus increasing P content and probably other nutrients in planta to enhance growth characteristics.
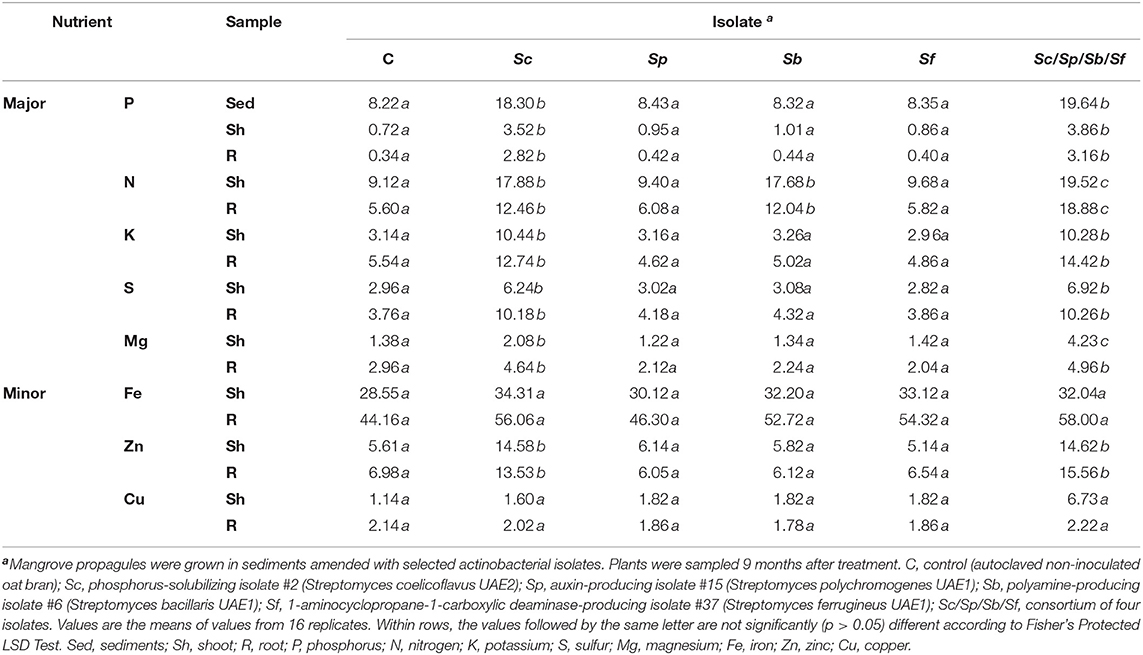
Table 3. Effect of plant growth-promoting actinobacterial isolates on available P concentration in sediments (mg kg−1) and major (g kg−1) and minor (mg kg−1) nutrients in the shoot and root tissues of mangrove (Avicennia marina) grown under greenhouse conditions at 28 ± 2°C.
Both Sc and Sb isolates significantly (p < 0.05) increased N in mangrove tissues compared to control plants, thus there was no significant (p > 0.05) difference in plant N when we compared the two treatments with each other (Table 3). It was also noted that the highest concentrations of N in shoots and roots were measured in plants treated with the combination of isolates. Plants amended with the single isolate Sc showed a significant (p < 0.05) increase in concentrations of K, S, Mg (major elements), and Zn (minor element) in shoot and root tissues compared to control or any other single PGPA isolate tested (Table 3). Thus, this increase of the same nutrients was comparable in the tissues of inoculated plants with the combined isolates. Our results indicate that the level of nutrient uptake in shoots and roots was most pronounced in the presence of Sc or the consortium of isolates applied to mangrove sediments.
On the other hand, there were no significant (p > 0.05) differences in Fe and Cu concentrations in the shoots or roots of plants grown in non-inoculated and inoculated sediments with single or combined Streptomyces isolate(s). This suggests that Sc does not have an effect on the uptake of these two elements. Taken together, treatments with Sc played a pivotal role in inducing P contents in plants. Thus, this might increase the levels of N, K, S, Mg, and Zn in mangrove seedlings directly or indirectly, which ultimately led to the growth promotion of mangrove.
Discussion
Mangroves can protect shorelines, mitigate climate change, and provide natural habitat for wildlife species and resources for local ecosystem at the Arabian Gulf (Li et al., 2019). Still, mangrove forests are greatly threatened by anthropogenic activities and natural pressure. In our efforts to enhance “mangrove reforestation,” we inoculated mangrove sediments with halotolerant PGPA isolated from local mangrove rhizosphere. Our aim was to increase mangrove productivity and performance and possibly expand nearby mangrove forests in the UAE and the Arabian Gulf. We hypothesized that the inoculation of mangrove sediments with a consortium of PGPA, each exhibiting a single PGP trait, would stimulate mangrove growth.
Investigations coming from different countries have indicated a diversity of actinobacteria isolated from mangrove rhizosphere, which have various biological activities. For instance, the isolated S. coelicoflavus BC 01 (Rao and Rao, 2013) and S. ferrugineus (Ruan et al., 2015) from mangrove sediments have been screened for their natural health products. Rhizosphere-competent S. bacilaris MAS 134 (Chakraborty et al., 2015) and endophytic S. polychromogens (Gohain et al., 2015) isolates have shown antagonistic activities against microbial pathogens. In the current study, the performance of four rhizosphere-competent PGPA isolates, namely Sc UAE2, Sp UAE1, Sb UAE1, and Sf UAE1, each possessing a sole mode of action, was investigated on mangrove plants under greenhouse conditions.
The distinct efficiencies in the performance of Sf and Sb over the other two isolates might be attributed to their abilities to produce ACCD and PAs, respectively. Such related activities of these isolates are notable because any relief from the inhospitable environments of waterlogged sediments and high salinity in mangrove ecosystem is essential for the normal growth of mangrove. However, there might be other mechanism(s) that were not investigated in the observed growth promotion.
Although P-solubilizing Sc and auxin-producing Sp were effective to a lesser extent, they were still able to significantly enhance seedling growth compared to control treatment. As suggested by Bashan and Holguin (2002), the current study highlights the potential of using a mixture of marine rhizosphere-competent PGPA as biological inoculants to enhance the growth and establishment of mangrove seedlings in nutrient-poor areas of coastal environments.
Mangrove forests occur in marine or brackish environments deficient in nutrients, mainly N and P (Naidoo, 2009). Therefore, researchers have focused their attention on naturally occurring soil PGPB capable of N-fixation and P-solubilization to determine their effects on mangrove growth (Bashan et al., 1998; Rojas et al., 2001; Galindo et al., 2006; El-Tarabily and Youssef, 2010). With the exception of testing the effects of P-solubilizing (Vazquez et al., 2000; Rojas et al., 2001; El-Tarabily and Youssef, 2010), N-fixing (Holguin et al., 1992; Bashan et al., 1998; Rojas et al., 2001), IAA-producing (Ravikumar et al., 2004; Kathiresan and Selvam, 2006), or ACCD-producing (El-Tarabily and Youssef, 2011) bacteria on mangrove growth under greenhouse conditions, no other reports have yet demonstrated if PGPB can stimulate mangrove growth via the production of other PGRs. Moreover, all these reports have dealt with non-actinobacterial isolates. Our study, however, demonstrated for the first time the potential of marine PGPA to promote the growth of mangrove using various PGP activities.
For successful plant growth promotion, the colonization of roots by the inoculated PGPB is a crucial step during the beneficial microbe–plant interaction (Hassan et al., 2019; Rilling et al., 2019). In addition, the introduced inoculum should enrich other microbial communities and adapt to a particular ecological niche. By assessing the growth promotion of the halophyte S. bigelovii, a recent study showed that rhizosphere-competent PGPA isolates producing IAA, PAs, and ACCD increased tissue biomass and lengths and seed production (Mathew et al., 2020). The potential isolates of PGPB were selected initially based on their ability to colonize roots in vitro using the qualitative primary root colonization bioassay (Silva et al., 2003). A rhizosphere-competence screening (Ahmad and Baker, 1987) in sediments under naturally competitive environment was followed. We did not find any report, so far, aiming at screening PGPB or PGPA to colonize mangrove rhizosphere and to show effective levels of rhizosphere competency under naturally competitive environments. Studies have so far tested the ability of the PGPB isolated from a native sediment to colonize mangrove roots in vitro using artificial seawater under non-competitive environments (Bashan et al., 1998; Puente et al., 1999; Rojas et al., 2001). The selection of promising PGPA from the mangrove rhizosphere herein was mainly based on their rhizosphere competency evaluated under a naturally competitive environment. Accordingly, the nine isolates recovered from the rhizosphere at all root depths (0–14 cm) were also supported by great R% and PD within the first 8-cm depth of roots. This indicated outstanding rhizosphere competence (Table 1) and could, therefore, be suitable for greenhouse/field inoculations.
In greenhouse experiments, four superior rhizosphere-competent isolates were chosen based on their in vitro PGR attributes. Several marine bacteria have been reported to produce auxins (Ravikumar et al., 2004; Mathew et al., 2020), CKs (Maruyama et al., 1986), GA3 (Sgroy et al., 2009), PAs (Suzuki et al., 1990; El-Tarabily et al., 2020), and ACCD (El-Tarabily and Youssef, 2011; Mathew et al., 2020). Despite great care being taken, GA3 and CK were eliminated due to the negligible amounts produced by PGPA in the current study (Table 2). None of the four isolates produced siderophores or NH3 or had the ability to fix N. Under greenhouse conditions, the applications of Oceanobacillus picturae obtained from the rhizosphere of mangrove ecosystem in the UAE (El-Tarabily and Youssef, 2010) match our results on Sc that both P-solubilizing isolates caused increases in the dry weight and length of tissues of mangrove. In this study, the consortium treatment, however, increased shoot and root lengths, shoot and root dry biomass, and total chlorophyll and carotenoids content of mangrove seedlings compared to those of P-solubilizing actinobacteria alone (Figure 4) or previously reported O. picturae treatments (El-Tarabily and Youssef, 2010). We argue that the mechanisms employed by the four PGPA to promote mangrove growth are significant compared to the application of actinobacteria that solubilized P only.
Streptomyces polychromogenes and Sb produced auxins (IAA and IPYA) and three PA types (Put, Spd, and Spm) in vitro, respectively; and promoted mangrove growth under greenhouse conditions. Consistent with that, Mathew et al. (2020) have reported an enhancement in the agronomic traits and biochemical properties of Salicornia tissues after inoculation with the auxin-producing isolate Streptomyces chartreusis in the UAE. In addition, the application of IAA-producing rhizosphere bacteria also increased the level of auxins in corn (Fallik et al., 1989) and Arabidopsis (Jiang et al., 2020). The inoculation of mangrove sediments with Sb resulted in increases in levels of the three PAs in shoots and roots, compared to control treatment in the greenhouse (Figure 6). It has been reported that Streptomyces griseoluteus producing Put, Spd, and Spm promoted the growth of bean plants by increasing endogenous PA levels under greenhouse conditions (Nassar et al., 2003). In addition, PAs produced by the rhizosphere-competent Streptomyces euryhalinus played a major role in S. bigelovii growth and development, and to a lesser extent by the non-rhizosphere-competent PAs-producing Actinoplanes deccanensis (El-Tarabily et al., 2020).
Streptomyces ferrugineus produced high levels of ACCD (Table 2). This isolate can cleave ACC (ET precursor) into NH3 and α-ketobutyrate (Glick et al., 2007). Sediment inoculation with Sf resulted in a significant reduction in the levels of ACC in mangrove root and shoot tissues, compared to control treatment (Figure 6). This, therefore, would reduce plant stress by lowering ET levels in plant tissues (Glick et al., 2007; El-Tarabily et al., 2019). This ultimately boosted growth parameters and photosynthetic performance in mangrove. Our results were in agreement with another study that the inoculation of mangrove sediments with P. maricaloris (an ACCD-producer) reduced ACC levels in shoots and roots and promoted the growth of mangrove (El-Tarabily and Youssef, 2011).
The inability of Sp, Sb, and Sf to solubilize P or fix N did not rule out a significant plant growth. Apparently, growth promotion was thus achieved by these PGPA via individual abilities to produce auxins, PAs, or ACCD, and possibly other PGRs on and/or near the root. It also seems that the production of ACCD and PAs by PGPA isolates are critical for plant growth promotion, especially in relation to the relief from environmental stresses. Similarly, Salicornia seedlings inoculated with either IAA-, PAs-, and ACCD-producing isolates positively regulated the activities of auxin and PAs with a concomitant decline in ACC levels in planta compared to individual isolate treatments (Mathew et al., 2020). It is worth mentioning that other PGP factors (e.g., the production of other PGRs), that were not assayed in this study, may also have a role in plant growth promotion.
The four isolates did not inhibit the activity of each other; instead the synergetic outcomes of the combined approach yielded the highest level of plant growth promotion in the greenhouse trials compared to individual or no isolate treatments. The maximum growth promotion by the mixture of four PGPA resulted in increases of about 82% in the dry weight of both shoots and roots, and 77% and 81% of the length of roots and shoots, respectively, compared to the untreated plants. Achieving such a considerable performance by the PGPA consortium on plant growth promotion can be explained by the synergetic interactions of the four isolates (Bashan et al., 2004; Bashan and de-Bashan, 2005; Mathew et al., 2020). Thus, this could be attributed to a significant increase in the levels of PGRs and nutrient uptake of mangrove. In studies related to bacteria associated with mangrove roots, mixed cultures of N-fixing Phyllobacterium sp. and P-solubilizing B. licheniformis doubled N incorporation and increased the development of black mangrove leaves compared to single cultures (Rojas et al., 2001). In addition, consortia of PGPB increased the growth parameters of corn compared to single inoculant treatments (Olanrewaju et al., 2019). Recently, three halotolerant marine rhizosphere-competent actinobacteria enhanced growth and increased the seed yield of S. bigelovii (Mathew et al., 2020). As such, similar observations in this study were demonstrated by the relative superiority in growth promotion when plants were inoculated with a combination of PGPA isolates.
Plant growth promotion reported in this study was clearly reflected in the enhanced photosynthetic gas exchange characteristics of the treated seedlings. Sf drastically improved all intrinsic components of photosynthesis, including the rate of rubisco catalytic activity, maximal rate of electron supply necessary for RUBP regeneration, and amounts of inorganic P available for utilization by Calvin cycle (TPU). The three other isolates Sc, Sp, and Sb also improved the above described components but to a lesser extent than that by ACCD-producing Sf (Figure 5). Here, an enhancement of the reported seedling growth parameters may be partially attributed to the improved rate of C assimilation, especially in mangrove plants inoculated with Sf or the consortium of isolates. Our data were in agreement with Ahmed et al. (2006) who found that the accumulation of ACC in mungbean (Vigna radiata) reduces the photosynthetic rate, and thus the produced ET reduces photosystem II activities. Together, the combined application of isolates was more effective in enhancing plant growth than treatments of individual actinobacteria. This was not only related to the decrease in ACC levels in planta but also to a significant induction of auxins, PAs, and nutrient uptake as well as the increase in photosynthetic gas exchange characteristics.
Our study suggest that Sc, Sp, Sb, and Sf can be added to the list of actinobacteria native to mangrove ecosystem, and highlight them as rhizosphere-competent PGP potentials derived from saline sediments in the UAE. Sowing propagules of mangrove in sediments amended with a consortium of four PGPA is expected to yield strong, well-developed mangrove seedlings in nurseries for permanent outplanting. The establishment of mangrove nurseries is in line with the efforts of the UAE government to rehabilitate the coastal and mangrove ecosystems in the Arabian Gulf. The present study can also improve the technology of marine agriculture using seawater irrigation practices. Thus, this provides a comprehensive assessment of growing mangroves locally, and provide knowledge for future research that can guide conservation and management in such harsh ecosystems in the Arabian Gulf.
Data Availability Statement
The datasets presented in this study can be found in online repositories. The names of the repository/repositories and accession number(s) can be found in the article/Supplementary Material.
Author Contributions
KE-T and SA designed the research, supervised the study, and wrote the manuscript. KE-T, AS, AE, and AH performed greenhouse experiments. KE-T, AS, and SA developed the phylogenetic analyses. KE-T and AE performed the microscopic experiments. KE-T and SA analyzed the data. AS, AE, AH, BA, ME-E, and AK assisted with experiments and/or data evaluation. All authors critically revised the manuscript and approved the final version.
Funding
This project was funded by Abu Dhabi Research Award (AARE2019) for Research Excellence-Department of Education and Knowledge (ADEK-007; Grant #: 21S105) to KE-T and Khalifa Center for Biotechnology and Genetic Engineering-UAEU (Grant #: 12R028) to SA.
Conflict of Interest
The authors declare that the research was conducted in the absence of any commercial or financial relationships that could be construed as a potential conflict of interest.
Publisher's Note
All claims expressed in this article are solely those of the authors and do not necessarily represent those of their affiliated organizations, or those of the publisher, the editors and the reviewers. Any product that may be evaluated in this article, or claim that may be made by its manufacturer, is not guaranteed or endorsed by the publisher.
Acknowledgments
KE-T would like to thank the library at Murdoch University, Australia for the valuable online resources and comprehensive databases.
Supplementary Material
The Supplementary Material for this article can be found online at: https://www.frontiersin.org/articles/10.3389/fmars.2021.715123/full#supplementary-material
References
Acuna, J. J., Campos, M., Mora, M. L., Jaisi, D. P., and Jorquera, M. A. (2019). ACCD-producing rhizobacteria from an Andean Altiplano native plant (Parastrephia quadrangularis) and their potential to alleviate salt stress in wheat seedlings. Appl. Soil Ecol. 136, 184–190. doi: 10.1016/j.apsoil.2019.01.005
Ahmad, J. S., and Baker, R. (1987). Rhizosphere competence of Trichoderma harzianum. Phytopathology 77, 182–189. doi: 10.1094/Phyto-77-182
Ahmed, S., Nawata, E., and Sakuratani, T. (2006). Changes of endogenous ABA and ACC, and their correlations to photosynthesis and water relations in mungbean (Vigna radiata (L.) Wilczak cv. KPS1) during waterlogging. Environ. Exper. Bot. 57, 278–284. doi: 10.1016/j.envexpbot.2005.06.006
Almahasheer, H. (2018). Spatial coverage of mangrove communities in the Arabian Gulf. Environ. Monit. Assess. 190:85. doi: 10.1007/s10661-018-6472-2
Arena, M. E., and Manca de Nadra, M. C. (2001). Biogenic amine production by Lactobacillus. J. Appl. Microbiol. 90, 158–162. doi: 10.1046/j.1365-2672.2001.01223.x
Barth, H., and Khan, N. (2008). Biogeophysical setting of the Gulf, in Protecting the Gulf's Marine Ecosystems from Pollution, ed A. Abuzinada, H. Barth, F. Krupp, B. Boer, and T. Abdessalam (Basel: Brikhauser Verlag), 1–21.
Bashan, Y., and de-Bashan, L. E. (2005). Bacteria/plant growth-promotion, in Encyclopedia of Soils in the Environment, ed D. Hillel (Oxford: Elsevier), 103–115.
Bashan, Y., and Holguin, G. (2002). Plant growth-promoting bacteria: a potential tool for arid mangrove reforestation. Trees. 16, 159–166. doi: 10.1007/s00468-001-0152-4
Bashan, Y., Holguin, G., and de-Bashan, L. E. (2004). Azospirillum-plant relationships: physiological, molecular, agricultural, and environmental advances (1997–2003). Can. J. Microbiol. 50, 521–577. doi: 10.1139/w04-035
Bashan, Y., Puente, M. E., Myrold, D. D., and Toledo, G. (1998). In vitro transfer of fixed nitrogen from diazotrophic filamentous cyanobacteria to black mangrove seedlings. FEMS Microbiol. Ecol. 26, 165–170. doi: 10.1111/j.1574-6941.1998.tb00502.x
Bashan, Y., Salazar, B., Puente, M. E., Bacilio, M., and Linderman, R. (2009). Enhanced establishment and growth of giant cardon cactus in an eroded field in the Sonoran Desert using native legume trees as nurse plants aided by plant growth-promoting microorganisms and compost. Biol. Fert. Soils 6, 585–594. doi: 10.1007/s00374-009-0367-x
Bertrand, H., Plassard, C., Pinochet, X., Touraine, B., Normand, P., and Cleyet-Marel, J. C. (2000). Stimulation of the ionic transport system in Brassica napus by a plant growth-promoting rhizobacterium (Achromobacter sp.). Can. J. Microbiol. 46, 229–236. doi: 10.1139/w99-137
Bradford, M. (1976). A rapid and sensitive method for the quantitation of microgram quantities of protein utilizing the principle of protein-dye binding. Anal. Biochem. 72, 248–258. doi: 10.1016/0003-2697(76)90527-3
Castro, R. A., Dourado, M. N., de Almeida, J. R., Lacava, P. T., Nave, A., de Melo, I. S., et al. (2018). Mangrove endophyte promotes reforestation tree (Acacia polyphylla) growth. Braz. J. Microbiol. 49, 59–66. doi: 10.1016/j.bjm.2017.04.002
Chakraborty, R. V., Chakraborty, K., and Thilakan, B. (2015). Isolation and characterization of antagonistic Streptomyces spp. from marine sediments along the southwest coast of India. Indian J. Mar. Sci. 44, 1742–1749.
Cross, T. (1989). Growth and examination of actinomycete-some guidelines, in Bergey's Manual of Systematic Bacteriology, eds S. T. Williams, M. E. Sharpe, and J. G. Holt, J.G. (Baltimore: Williams and Wilkins), 2340–2343.
Davies, B. H. (1965). Analysis of carotenoid pigments, in Chemistry and Biochemistry of Plant Pigments, ed T.W. Goodwin (London: Academic Press), 489–532.
de Boer, M., Van der Sluis, I., Van Loon, L. C., and Bakker, P. A. H. M. (1999). Combining fluorescent Pseudomonas sp. strains to enhance suppression of Fusarium wilt of radish. Eur. J. Plant Pathol. 105, 201–210. doi: 10.1023/A:1008761729073
di Menna, M. E. (1957). The isolation of yeasts from soil. J. Gen. Microbiol. 17, 678–688. doi: 10.1099/00221287-17-3-678
Dworkin, M., and Foster, J. (1958). Experiments with some microorganisms which utilize ethane and hydrogen. J. Bacteriol. 75, 592–601. doi: 10.1128/jb.75.5.592-603.1958
Dye, D. W. (1962). The inadequacy of the usual determinative tests for identification of Xanthomonas spp. NZT Sci. 5, 393–416.
Elmahdy, S. I., Ali, T. A., Mohamed, M. M., Howari, F. M., Abouleish, M., and Simonet, D. (2020). Spatiotemporal mapping and monitoring of mangrove forests changes from 1990 to 2019 in the Northern Emirates, UAE using random forest, kernel logistic regression and naive bayes tree models. Front. Environ. Sci. 8:102. doi: 10.3389/fenvs.2020.00102
El-Tarabily, K., and Youssef, T. (2010). Enhancement of morphological, anatomical and physiological characteristics of seedlings of the mangrove Avicennia marina inoculated with a native phosphate-solubilizing isolate of Oceanobacillus picturae under greenhouse conditions. Plant Soil 332, 147–162. doi: 10.1007/s11104-010-0280-y
El-Tarabily, K., and Youssef, T. (2011). Improved growth performance of the mangrove Avicennia marina seedlings using a 1-aminocyclopropane-1-carboxylic acid deaminase-producing isolate of Pseudoalteromonas maricaloris. Plant Growth Regul. 65, 473–483. doi: 10.1007/s10725-011-9618-6
El-Tarabily, K. A., AlKhajeh, A. S., Ayyash, M. M., Alnuaimi, L. H., Sham, A., ElBaghdady, K. Z., et al. (2019). Growth promotion of Salicornia bigelovii by Micromonospora chalcea UAE1, an endophytic 1-aminocyclopropane-1-carboxylic acid deaminase-producing actinobacterial isolate. Front. Microbiol. 10:1694. doi: 10.3389/fmicb.2019.01694
El-Tarabily, K. A., ElBaghdady, K. Z., AlKhajeh, A. S., Ayyash, M. M., Aljneibi, R. S., El-Keblawy, A., et al. (2020). Polyamine-producing actinobacteria enhance biomass production and seed yield in Salicornia bigelovii. Biol. Fertil. Soils 56, 499–519. doi: 10.1007/s00374-020-01450-3
El-Tarabily, K. A., Ramadan, G. A., Elbadawi, A. A., Hassan, A. H., Tariq, S., Ghazal, E. W., et al. (2021). The marine endophytic polyamine-producing Streptomyces mutabilis UAE1 isolated from extreme niches in the Arabian Gulf promotes the performance of mangrove (Avicennia marina) seedlings under greenhouse conditions. Front. Mar. Sci. 13:710200. doi: 10.3389/fmars.2021.710200
Fallik, E., Okon, Y., Epstein, E., Goldman, A., and Fischer, M. (1989). Identification and quantification of IAA and IBA in Azospirillum brasilense-inoculated maize roots. Soil Biol. Biochem. 21, 147–153. doi: 10.1016/0038-0717(89)90024-2
Farquhar, G., von Caemmerer, S., and Berry, J. (1980). A biochemical model of photosynthetic CO2 assimilation in leaves of C3 species. Planta 149, 78–90. doi: 10.1007/BF00386231
Flores, H. E., and Galston, A. W. (1982). Analysis of polyamines in higher plants by high performance liquid chromatography. Plant Physiol. 69, 701–706. doi: 10.1104/pp.69.3.701
Galindo, T., Polania, J., Sanchez, J., Moreno, N., Vanegas, J., and Holguin, G. (2006). Microbial inoculants effects on growth promotion of mangrove and Citrullus vulgaris San Andres Isla, Colombia. Acta Biologica Colombiana 11, 83–97.
Gauze, G. F., Preobrazhenskaya, T. P., Sveshnikova, M. A., Terekhova, L. P., and Maximova, T. S. (1983). A guide for the determination of actinomycetes. Genera Streptomyces, Streptoverticillium, and Chainia. Nauka, Moscow, URSS.
Gayathri, P., and Muralikrishnan, V. (2013). Isolation and characterization of endophytic actinomycetes from mangrove plant for antimicrobial activity. Int. J. Curr. Microbiol. App. Sci. 2, 78–89.
Glick, B. R. (2012). Plant growth-promoting bacteria: mechanisms and applications. Scientifica. 2012:963401. doi: 10.6064/2012/963401
Glick, B. R. (2014). Bacteria with ACC deaminase can promote plant growth and help to feed the world. Microbiol. Res. 169, 30–39. doi: 10.1016/j.micres.2013.09.009
Glick, B. R. (2015). Introduction to plant growth promoting bacteria, in: Beneficial Plant-Bacterial Interactions, ed B. R. Glick (Switzerland: Springer International Publishing), 1–28.
Glick, B. R., Todorovic, B., Czarny, J., Cheng, Z., Duan, J., and McConkey, B. (2007). Promotion of plant growth by bacterial ACC deaminase. Crit. Rev. Plant Sci. 26, 227–242. doi: 10.1080/07352680701572966
Gohain, A., Gogoi, A., Debnath, R., Yadav, A., Singh, B. P., Gupta, V. K., et al. (2015). Antimicrobial biosynthetic potential and genetic diversity of endophytic actinomycetes associated with medicinal plants. FEMS Microbiol. Lett. 362:fnv158. doi: 10.1093/femsle/fnv158
Goodfellow, M., and Williams, S. (1983). Ecology of actinomycetes. Annu. Rev. Microbiol. 37, 189–216. doi: 10.1146/annurev.mi.37.100183.001201
Gordon, S. A., and Weber, R. P. (1951). Colorimetric estimation of indoleacetic acid. Plant Physiol. 26, 192–195. doi: 10.1104/pp.26.1.192
Guinn, G., Brummett, D. L., and Beier, R. C. (1986). Purification and measurement of abscisic acid and indole-acetic acid by high performance liquid chromatography. Plant Physiol. 81, 997–1002. doi: 10.1104/pp.81.4.997
Hagemann, G., Pénasse, L., and Teillon, J. (1964). Zur Systematik der Actinomyceten. 9. Streptomyceten mit cinnamoneus Luftmycel. In Zentralbl. Bakteriol. Parasitenkd. Infekt.
Harley, P., Thomas, R., Reynolds, J., and Strain, B. (1992). Modeling photosynthesis of cotton grown in elevated CO2. Plant Cell Environ. 15, 271–282. doi: 10.1111/j.1365-3040.1992.tb00974.x
Hassan, M. K., McInroy, J. A., and Kloepper, J. W. (2019). The interactions of rhizodeposits with plant growth-promoting rhizobacteria in the rhizosphere: a review. Agriculture 9:142. doi: 10.3390/agriculture9070142
Holden, M. (1965). Chlorophylls, in Chemistry and Biochemistry of Plant Pigments, ed T. W. Goodwin (London: Academic Press), 462–488.
Holguin, G., Guzman, M. A., and Bashan, Y. (1992). Two new nitrogen-fixing bacteria from the rhizosphere of mangrove trees: their isolation, identification and in vitro interaction with rhizosphere Staphylococcus sp. FEMS Microbiol. Ecol. 101, 207–216. doi: 10.1016/0168-6445(92)90017-P
Honma, M., and Shimomura, T. (1978). Metabolism of 1-aminocyclopropane-1-carboxylic acid. Agric. Biol. Chem. 42, 1825–1831. doi: 10.1080/00021369.1978.10863261
Jiang, Y., Wu, Y., Hu, N., Li, H., and Jiao, J. (2020). Interactions of bacterial-feeding nematodes and indole-3-acetic acid (IAA)-producing bacteria promotes growth of Arabidopsis thaliana by regulating soil auxin status. Appl. Soil. Ecol. 147:103447. doi: 10.1016/j.apsoil.2019.103447
Jiang, Z. -k., Tuo, L., Huang, D. -l., Osterman, I. A., Tyurin, A. P., Liu, S. -w., et al. (2018). Diversity, novelty, and antimicrobial activity of endophytic actinobacteria from mangrove plants in Beilun Estuary National Nature Reserve of Guangxi, China. Front. Microbiol. 9:868. doi: 10.3389/fmicb.2018.00868
Jones, H. G. (1992). Plant and Microclimate: A Quantitative Approach to Environmental Plant Physiology. Cambridge, UK: Cambridge University Press, 428.
Kathiresan, K., and Selvam, M. M. (2006). Evaluation of beneficial bacteria from mangrove soil. Bot. Mar. 49, 86–88. doi: 10.1515/BOT.2006.011
Kemung, H. M., Tan, L. T.-H., Chan, K.- G., Ser, H. -L., Law, J. W. -F., Lee, L. -H., et al. (2020). Streptomyces sp. strain MUSC 125 from mangrove soil in Malaysia with anti-MRSA, anti-biofilm and antioxidant activities. Molecules 25:3545. doi: 10.3390/molecules25153545
Khalid, A., Arshad, M., and Zahir, Z. A. (2004). Screening plant growth-promoting rhizobacteria for improving growth and yield of wheat. J. Appl. Microbiol. 96, 473–480. doi: 10.1046/j.1365-2672.2003.02161.x
Krasil'nikov, N. A. (1958). The significance of antibiotics as specific characteristics of actinomycetes, and their determination by the method of experimental transformation. Folia Biologica 4, 257–265.
Kumar, A., Patel, J. S., Meena, V. S., and Ramteke, P. W. (2019). Plant growth-promoting rhizobacteria: strategies to improve abiotic stresses under sustainable agriculture. J. Plant Nutr. 42, 1402–1415. doi: 10.1080/01904167.2019.1616757
Kumar, S., Stecher, G., and Tamura, K. (2016). MEGA7: Molecular evolutionary genetics analysis version 7.0 for bigger datasets. Mol. Biol. Evol. 33, 1870–1874. doi: 10.1093/molbev/msw054
Küster, E. (1959). Outline of a comparative study of criteria used in characterization of the actinomycetes. Int. Bull. Bact. Nomen. Taxon. 9, 97–104. doi: 10.1099/0096266X-9-2-97
Lanneluc-Sanson, D., Phan, C. T., and Granger, R. L. (1986). Analysis by reverse-phase high-pressure liquid chromatography of phenylisothiocyanate-derivatized 1-aminocyclopropane-1-carboxylic acid in apple extracts. Anal. Biochem. 155, 322–327. doi: 10.1016/0003-2697(86)90441-0
Li, W., El-Askary, H., Qurban, M. A., Li, J., ManiKandan, K. P., and Piechota, T. (2019). Using multi-indices approach to quantify mangrove changes over the Western Arabian Gulf along Saudi Arabia coast. Ecol. Indic. 102, 734–745. doi: 10.1016/j.ecolind.2019.03.047
Lizada, M. C., and Yang, S. F. (1979). A simple and sensitive assay for 1-aminocyclopropane-1-carboxylic acid. Anal. Biochem. 100, 140–145. doi: 10.1016/0003-2697(79)90123-4
Marino, M., Maifreni, M., Moret, S., and Rondinini, G. (2000). The capacity of Enterobacteriaceae species to produce biogenic amines in cheese. Lett. Appl. Microbiol. 31, 169–173. doi: 10.1046/j.1365-2672.2000.00783.x
Maruyama, A., Maeda, M., and Simidu, U. (1986). Occurrence of plant hormone (cytokinin)-producing bacteria in the sea. J. Appl. Bacteriol. 61, 569–574. doi: 10.1111/j.1365-2672.1986.tb01731.x
Mathew, B. T., Torky, Y., Amin, A., Mourad, A. I., Ayyash, M. M., El-Keblawy, A., et al. (2020). Halotolerant marine rhizosphere-competent actinobacteria promote Salicornia bigelovii growth and seed production using seawater irrigation. Front. Microbiol. 11:552. doi: 10.3389/fmicb.2020.00552
McQuaker, N. R., Brown, D. F., and Kluckner, P. D. (1979). Digestion of environmental materials for analysis by inductively coupled plasma-atomic emission spectrometry. Anal. Chem. 51, 1082–1084. doi: 10.1021/ac50043a071
Misaghi, I. J., and Donndelinger, I. J. (1990). Endophytic bacteria in symptom-free cotton plants. Phytopathology 80, 808–811. doi: 10.1094/Phyto-80-808
Moretti, L. G., Crusciol, C. A., Kuramae, E. E., Bossolani, J. W., Moreira, A., Costa, N. R., et al. (2020). Effects of growth-promoting bacteria on soybean root activity, plant development, and yield. Agronomy J. 112, 418–428. doi: 10.1002/agj2.20010
Murphy, J., and Riley, J. P. (1962). A modified single solution method for the determination of phosphate in natural waters. Anal. Chim. Acta 27, 31–36. doi: 10.1016/S0003-2670(00)88444-5
Naidoo, G. (2009). Differential effects of nitrogen and phosphorus enrichment on growth of dwarf Avicennia marina mangroves. Aquat. Bot. 90, 184–190. doi: 10.1016/j.aquabot.2008.10.001
Nassar, A. H., El-Tarabily, K. A., and Sivasithamparam, K. (2003). Growth promotion of bean (Phaseolus vulgaris L.) by a polyamine-producing isolate of Streptomyces griseoluteus. Plant Growth Regul. 40, 97–106. doi: 10.1023/A:1024233303526
Nautiyal, C. S. (1997). A method for selection and characterization of rhizosphere-competent bacteria of chickpea. Curr. Microbiol. 34, 12–17. doi: 10.1007/s002849900136
Olanrewaju, O. S., Glick, B. R., and Babalola, O. O. (2019). Bacterial consortium for improved maize (Zea mays L.) production. Microorganisms 7:519. doi: 10.3390/microorganisms7110519
Payne, C., Bruce, A., and Staines, H. (2000). Yeast and bacteria as biological control agents against fungal discolouration of Pinus sylvestris blocks in laboratory-based tests and the role of antifungal volatiles. Holzforschung 54, 563–569. doi: 10.1515/HF.2000.096
Pikovskaya, R. I. (1948). Mobilization of phosphorus in soil in connection with the vital activity of some microbial species. Microbiology 17, 362–370.
Polidoro, B., Carpenter, K., Collins, L., Duke, N., Ellison, A., Ellison, J., et al. (2010). The loss of species: Mangrove extinction risk and failure of critical ecosystem services. PLoS ONE 5:e10095. doi: 10.1371/journal.pone.0010095
Pridham, T. G. (1970). New names and new combinations in the order Actinomycetales Buchanan 1917. US. Dept. Agric. Tech. Bull. 1424, 1–55.
Primavera, J. H., Sadaba, R. B., Lebata, M. J. H. L., and Altamirano, J. P. (2004). Handjournal of Mangroves in the Philippines-Panay. Iloilo, Philippines: SEAFDEC Aquaculture Department.
Puente, M. E., Holguin, G., Glick, B. R., and Bashan, Y. (1999). Root-surface colonization of black mangrove seedling by Azospirillum halopraeferens and Azospirillum brasilense in sea water. FEMS Microbiol. Ecol. 29, 283–292. doi: 10.1111/j.1574-6941.1999.tb00619.x
Rainey, F. A., Ward-Rainey, N., Kroppenstedt, R. M., and Stackebrandt, E. (1996). The genus Nocardiopsis represents a phylogenetically coherent taxon and a distinct actinomycete lineage: Proposal of Nocardiopsaceae fam. nov. Int. J. Syst. Bacteriol. 46, 1088–1092. doi: 10.1099/00207713-46-4-1088
Rao, K. V. R., and Rao, T. R. (2013). Molecular characterization and its antioxidant activity of a newly isolated Streptomyces coelicoflavus BC 01 from mangrove soil. J. Young Pharm. 5, 121–126. doi: 10.1016/j.jyp.2013.10.002
Ravikumar, S., Kathiresan, K., Ignatiammal, S. T., Selvam, M. B., and Shanthy, S. (2004). Nitrogen-fixing azotobacters from mangrove habitat and their utility as marine biofertilizers. J. Exp. Mar. Biol. Ecol. 312, 5–17. doi: 10.1016/j.jembe.2004.05.020
Redmond, J. W., and Tseng, A. (1979). High-pressure liquid chromatographic determination of putrescine, spermidine and spermine. J. Chromatogr. 170, 479–481. doi: 10.1016/S0021-9673(00)95481-5
Rilling, J. I., Acuna, J. J., Nannipieri, P., Cassan, F., Maruyama, F., and Jorquera, M. A. (2019). Current opinion and perspectives on the methods for tracking and monitoring plant growth promoting bacteria. Soil Biol. Biochem. 130, 205–219. doi: 10.1016/j.soilbio.2018.12.012
Rojas, A., Holguin, G., Glick, B. R., and Bashan, Y. (2001). Synergism between Phyllobacterium sp. (N2-fixer) and Bacillus licheniformis (P-solubilizer), both from a semiarid mangrove rhizosphere. FEMS Microbiol. Ecol. 35, 181–187. doi: 10.1111/j.1574-6941.2001.tb00802.x
Ruan, C.-y., Zhang, L., Ye, W.-w., Xie, X.-c., Srivibool, R., Duangmal, K., et al. (2015). Streptomyces ferrugineus sp. nov., isolated from mangrove soil in Thailand. Antonie van Leeuwenhoek 107, 39–45. doi: 10.1007/s10482-014-0301-6
Saitou, N., and Nei, M. (1987). The neighbor-joining method: a new method for reconstructing phylogenetic trees. Mol. Biol. Evol. 4, 406–425.
Samara, F., Solovieva, N., Ghalayini, T., Nasrallah, Z. A., and Saburova, M. (2020). Assessment of the environmental status of the mangrove ecosystem in the United Arab Emirates. Water 12:1623. doi: 10.3390/w12061623
Schreiter, S., Babin, D., Smalla, K., and Grosch, R. (2018). Rhizosphere competence and biocontrol effect of Pseudomonas sp. RU47 independent from plant species and soil type at the field scale. Front. Microbiol. 9:97. doi: 10.3389/fmicb.2018.00097
Schwyn, B., and Neilands, J. B. (1987). Universal chemical assay for the detection and determination of siderophores. Anal. Biochem. 160, 47–56. doi: 10.1016/0003-2697(87)90612-9
Sgroy, V., Cassan, F., Masciarelli, O., Del Papa, M. F., Lagares, A., and Luna, V. (2009). Isolation and characterization of endophytic plant growth-promoting (PGPB) or stress homeostasis-regulating (PSHB) bacteria associated to the halophyte Prosopis strombulifera. Appl. Microbiol. Biotechnol. 85, 371–381. doi: 10.1007/s00253-009-2116-3
Shah, S., Li, J., Moffatt, B. A., and Glick, B. R. (1998). Isolation and characterization of ACC deaminase gene from two different plant growth-promoting rhizobacteria. Can. J. Microbiol. 44, 833–843. doi: 10.1139/w98-074
Shirling, E. B., and Gottlieb, D. (1966). Methods for characterization of Streptomyces species. Int. J. Syst. Bacteriol. 16, 313–340. doi: 10.1099/00207713-16-3-313
Silva, H. S. A., Romeiro, R. S. A., and Mounteer, A. (2003). Development of a root colonization bioassays for rapid screening of rhizobacteria for potential biocontrol agents. J. Phytopathol. 151, 42–46. doi: 10.1046/j.1439-0434.2003.00678.x
Smith, M. A., and Davies, P. J. (1985). Separation and quantitation of polyamines in plant tissue by high performance liquid chromatography of their dansyl derivatives. Plant Physiol. 78, 89–91. doi: 10.1104/pp.78.1.89
Spalding, M., and Parrett, C. L. (2019). Global patterns in mangrove recreation and tourism. Mar. Policy 110:103540. doi: 10.1016/j.marpol.2019.103540
Strzelczyk, E., and Pokojska-Burdziej, A. (1984). Production of auxins and gibberellin-like substances by mycorrhizal fungi, bacteria and actinomycetes isolated from the soil and the mycorrhizosphere of pine (Pinus silvestris L.). Plant Soil 81, 185–194. doi: 10.1007/BF02197150
Suzuki, S., Noda, J., and Takama, K. (1990). Growth and polyamine production of Alteromonas spp. in fish meat extracts under modified atmosphere. Bull. Fac. Fish. Hokkaido Univ. 41, 213–220.
Sweeney, R. A., and Rexroad, P. R. (1987). Comparison of LECO FP228 ‘nitrogen determinator’ with AOAC copper catalyst Kjeldahl method for crude protein. J. Assoc. Off. Anal. Chem. 70, 1028–1030. doi: 10.1093/jaoac/70.6.1028
Terekhova, L. P. (1986). Footnote f. In Validation of the publication of new names and new combinations previously effectively published outside the IJSB. List number 22. Int. J. Syst. Bacteriol. 36, 573–576. doi: 10.1099/00207713-36-4-573
Thompson, J. D., Gibson, T. J., Plewniak, F., Jeanmougin, F., and Higgins, D. G. (1997). The CLUSTAL_X windows interface: flexible strategies for multiple sequence alignment aided by quality analysis tools. Nucleic Acids Res. 25, 4876–4882. doi: 10.1093/nar/25.24.4876
Tien, T. M., Gaskings, M. H., and Hubbell, D. H. (1979). Plant growth substances produced by Azospirillum brasilense and their effect on the growth of pearl millet (Pennisetum americanum L.). Appl. Environ. Microbiol. 37, 1016–1024. doi: 10.1128/aem.37.5.1016-1024.1979
Toledo, G., Bashan, Y., and Soeldner, A. (1995a). In vitro colonization and increase in nitrogen fixation of seedling roots of black mangrove inoculated by a filamentous cyanobacteria. Can. J. Microbiol. 41, 1012–1020. doi: 10.1139/m95-140
Toledo, G., Bashan, Y., and Soeldner, A. (1995b). Cyanobacteria and black mangroves in Northwestern Mexico: colonization, and diurnal and seasonal nitrogen fixation on aerial roots. Can. J. Microbiol. 41, 999–1011. doi: 10.1139/m95-139
Vazquez, P., Holguin, G., Puente, M., ELopez Cortes, A., and Bashan, Y. (2000). Phosphate solubilizing microorganisms associated with the rhizosphere of mangroves in a semi arid coastal lagoon. Biol. Fert. Soils 30, 460–468. doi: 10.1007/s003740050024
von Caemmerer, S., and Farquhar, G. D. (1981). Some relationships between the biochemistry of photosynthesis and the gas exchange of leaves. Planta 153, 376–387. doi: 10.1007/BF00384257
Williams, S. T., Shameemullah, M., Watson, E. T., and Mayfield, C. I. (1972). Studies on the ecology of actinomycetes in soil. VI. The influence of moisture tension on growth and survival. Soil Biol. Biochem. 4, 215–225. doi: 10.1016/0038-0717(72)90014-4
Keywords: actinobacteria, Arabian Gulf, consortium, halophyte, mangrove, marine agriculture, plant growth promotion
Citation: El-Tarabily KA, Sham A, Elbadawi AA, Hassan AH, Alhosani BKK, El-Esawi MA, AlKhajeh AS and AbuQamar SF (2021) A Consortium of Rhizosphere-Competent Actinobacteria Exhibiting Multiple Plant Growth-Promoting Traits Improves the Growth of Avicennia marina in the United Arab Emirates. Front. Mar. Sci. 8:715123. doi: 10.3389/fmars.2021.715123
Received: 26 May 2021; Accepted: 29 July 2021;
Published: 13 September 2021.
Edited by:
Wael Ahmed Ismail, Arabian Gulf University, BahrainReviewed by:
Tahar Mechichi, University of Sfax, TunisiaMoez Hanin, University of Sfax, Tunisia
Luigi Lucini, Catholic University of the Sacred Heart, Italy
Copyright © 2021 El-Tarabily, Sham, Elbadawi, Hassan, Alhosani, El-Esawi, AlKhajeh and AbuQamar. This is an open-access article distributed under the terms of the Creative Commons Attribution License (CC BY). The use, distribution or reproduction in other forums is permitted, provided the original author(s) and the copyright owner(s) are credited and that the original publication in this journal is cited, in accordance with accepted academic practice. No use, distribution or reproduction is permitted which does not comply with these terms.
*Correspondence: Khaled A. El-Tarabily, a3RhcmFiaWx5JiN4MDAwNDA7dWFldS5hYy5hZQ==; Synan F. AbuQamar, c2FidXFhbWFyJiN4MDAwNDA7dWFldS5hYy5hZQ==