- 1Faculty of Environmental Management, Prince of Songkla University, Hat Yai, Thailand
- 2Coastal Oceanography and Climate Change Research Center, Prince of Songkla University, Hat Yai, Thailand
- 3Faculty of Science, School of Computational Science, Prince of Songkla University, Hat Yai, Thailand
- 4Climate Change Cluster, University of Technology Sydney, Ultimo, NSW, Australia
- 5Faculty of Environmental Management, Marine and Coastal Resources Institute, Prince of Songkla University, Hat Yai, Thailand
Marine debris has become a global problem affecting coral health around the globe. However, the photophysiological responses of corals to marine debris stress remain unclear. Therefore, this study firstly investigated transparent and opaque plastic bag shading and fishing nets directly contacting the coral. Photosynthetic performance, pigment content, symbiont density, and calcification rate of a branching coral Pocillopora acuta and a massive coral Porites lutea were investigated after 4 weeks of exposure to marine debris. The results show that the maximum quantum yield of PSII significantly decreased in P. lutea with all treatments, while P. acuta showed no effect on the maximum quantum yield of PSII from any treatments. Transparent plastic bag shading does not affect P. acuta, but significantly affected the maximum photochemical efficiency of P. lutea. Photoacclimation of cellular pigment content was also observed under opaque plastic bag shading for both species at week 2. Fishing nets had the strongest effect and resulted in P. acuta bleaching and P. lutea partial mortality as well as a decline in zooxanthellae density. Calcification rate of P. acuta significantly decreased with treatments using opaque plastic bag and fishing net, but for P. lutea only the treatment with fishing net gave any observable effects. This study suggests that the sensitivities of corals to marine debris differ strongly by species and morphology of the coral.
Introduction
Coral reefs are the most biologically diverse ecosystems in the world, and provide a variety of valuable ecosystem services to global communities (Moberg and Folke, 1999; Harrison and Booth, 2007). However, global warming, acidification of our oceans, and sea level rise can severely affect the survival of corals worldwide (Marshall and Baird, 2000; Orr et al., 2005; Anthony et al., 2008). Fishing gear found across shallow reefs can damage coral reef structure, destroying both benthic flora and fauna, as well as entangling macrofauna (Abu-Hilal and Al-Najjar, 2009). In addition, the anthropogenic activities over the last century have made marine debris a global problem (Ryan, 2015). It is estimated that about 4.8–12.7 MT of marine debris is discharged into the oceans every year (Jambeck et al., 2015), with 5.25 trillion plastic particles weighing 268,940 tons floating in the oceans (Eriksen et al., 2014). Plastic waste, fishing gear and metals are the dominant three types of debris observed in the Red Sea (Abu-Hilal and Al-Najjar, 2009). Marine debris can be found in every marine habitat, from surface water down to the deep sea (Dameron et al., 2007; Barnes et al., 2009; Buhl-Mortensen and Buhl-Mortensen, 2017), and it affects hundreds of marine species worldwide (Schipper et al., 2008; Barnes et al., 2009; Todd et al., 2010) as well as disrupts whole reef ecological systems (de Carvalho-Souza et al., 2018) and causes loss of coral structural complexity and diversity (Figueroa-Pico et al., 2020). Marine debris has detrimental effects on coral and coral reef ecosystems both directly and indirectly (Donohue et al., 2001). Large debris items physically damage corals through abrasion, suffocation, or starvation (Richards and Beger, 2011). Lamb et al. (2018) found more than 11 billion plastic items entangled with coral reefs in the Asia-Pacific, and suggested that coral disease outbreaks increase when corals are entangled with plastic. Decreased light intensity from being covered by plastic debris has been documented to effect branching coral health and lead to coral bleaching (Muhammad et al., 2021). Field research in Kho Tao, Thailand, found that coral underneath a fishing net suffered the most damage (Valderrama Ballesteros et al., 2018). Massive and branching coral types are the most susceptible when compared with colonies of other morphologies (Putra et al., 2021). In addition, corals are particularly sensitive to abrasion (Tanner, 1995) and abrasion responses in corals are common with plastics. Marine debris has the potential to accumulate bacteria (Zhang et al., 2017), and coral health is controlled by the microbiome within the muco-polysaccharide layer (Kline et al., 2006). Rapid changes in environmental conditions alter coral microbiome, causing bleaching, and disease susceptibility (Bourne et al., 2016). Coral requires carbon both as an autotrophic and a heterotrophic nutrition source (Anthony and Fabricius, 2000; Richards and Beger, 2011). However, macro- and microplastics affect cold-water coral skeleton growth rate, as microplastics interfere with polyps capturing food particles (Chapron et al., 2018). Microplastics are found wrapped in mesenterial tissue within the coral gut cavity, and ingesting high concentrations of microplastics could potentially damage the health of corals (Hall et al., 2015). A number of studies have confirmed that there is abundant marine debris in the oceans, and that it devastates coral reefs. However, there are limited studies quantifying the damage from different types of debris on photosynthesis and productivity of corals and zooxanthellae. Therefore, this study aimed to investigate how Pocillopora acuta and Porites lutea coral species respond to different types of marine debris.
Materials and Methods
Experimental Design
Six colonies each of the coral specie P. acuta and P. lutea were collected from Panwa Cape, Phuket Thailand (7°48′06.9″N 98°24′24.4″E) and transferred within 24 h to aquarium tanks at Prince of Songkla University, HatYai, Thailand, for acclimation. Temperature, salinity and light intensity from collection site were recorded. Every colony was acclimated in a holding tank for a week under 120 μmol photons m–2 s–1 light, 29°C, 33 psu salinity, and pH 8.20. After that, the coral colonies were cut into 192 nubbins (P. acuta with 3 cm length, P. lutea with size around 1.5 cm × 1.5 cm) and acclimated in holding tanks for another week, after which the nubbins were randomly placed to four sets (24 nubbins/specie/treatment) of experimental tanks and acclimated for 1 week. After acclimation, the tanks were subjected to four treatments. Control had no marine debris (Treatment 1); opaque plastic (High Density Polyethylene) bag-located 2 cm above nubbins (15% Light reduction, Treatment 2); transparent plastic (Low Density Polyethylene) bag-located 2 cm above nubbins (3% light reduction, Treatment 3); and Fishing net (13% light reduction and abrasion, Treatment 4). There were 6 replicate samples per treatment per species (Figure 1). The whole experiment was performed over 4 weeks to test the effects of marine debris on coral. We sampled nubbins for the zooxanthellae density and chlorophyll concentration analysis in week 2 and week 4. Heater chiller (JMC-02, JBA, China), COB light (TS-A600, Aquarium lamp, China), and LED light (A601, Chihiros, China) were, respectively, used to control water temperature and light intensity in the experimental tanks. Light intensity, temperature, salinity and pH in each experimental tank were set for 120 mmol photons m–2 s–1, 29°C, 33 psu and pH 8.20, respectively. Light was turned on at 10:00 and turned off at 22:00 (12:12 hr light:dark period). Nitrate and phosphate concentrations were controlled below 0 mg L–1 and 2 mg L–1, respectively. Aquariums were filled with natural seawater filtered with a nomex filter bag and treated with chlorine [50 mg chlorine L–1 (50 ppm)]. Thirty percent of the aquarium water was replaced every week, and all physical and chemical parameters were measured every 2 days. Photosynthetic performance of zooxanthellae and health of corals were monitored using chlorophyll a fluorescence, and this was randomly measured with the Diving-PAM at the top of nubbins. Pigment concentration and symbiotic cell density were investigated for supporting photosynthetic performance of zooxanthellae. Growth of corals was investigated using buoyant weight technique (Jokiel et al., 1978).
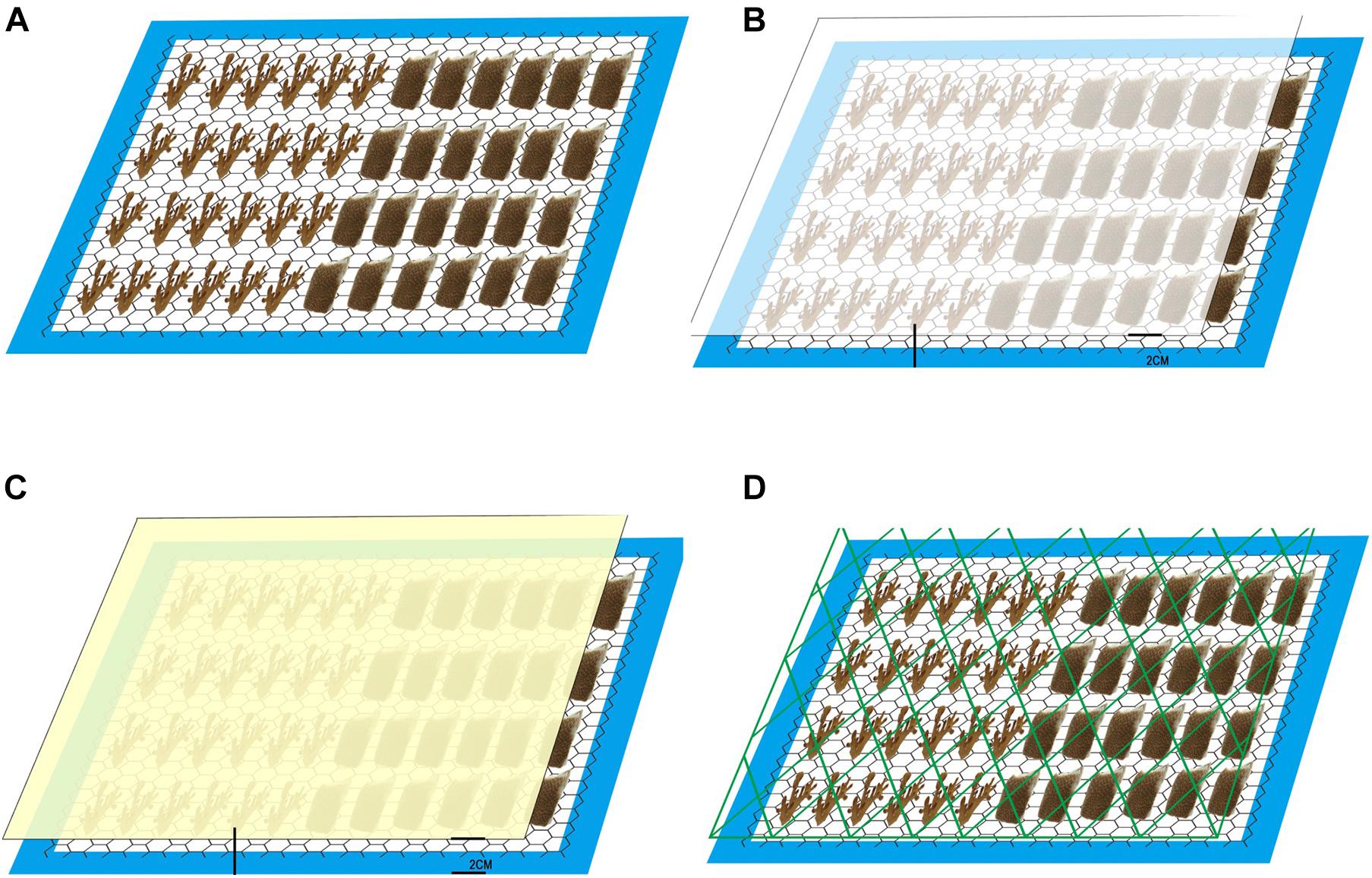
Figure 1. Images of the experiment tank design. (A) Control, (B) Transparent bag, (C) Opaque bag, and (D) Fishing net.
Chlorophyll a Fluorescence
Chlorophyll a fluorescence of P. acuta and P. lutea in each treatment was quantified every 2 days over the 4 weeks of experiment by using a Diving-PAM fluorometer (Walz GmbH, Germany) connected to a 6-mm diameter fiber-optic probe (6 replicates per species per treatment per time). Photochemical efficiency of PSII was described by maximum quantum yield (Fv/Fm) or active PSII centers (Fv/F0) and measured at 08:00 (before light was turned on). Reduction of PSII reaction center number can provide photoinhibitory indication (Behrenfeld et al., 1998). Rapid light curves (RLCs) were measured at 13:00. Maximum relative electron transport rate (rETRmax), minimum saturating irradiance (Ek), and initial slope (alpha) were derived from curve fits (Platt et al., 1980; Ralph and Gademann, 2005).
Symbiotic Cell Density
Samples were collected every 2 weeks for zooxanthellae density analysis. The samples were water-picked into 50 mL of 0.2-μm-filtered seawater to remove the tissue from the skeleton. The slurry was centrifuged at 1,000 rpm for 10 min to produce a well-mixed sample (Hill and Ralph, 2007). Six replicates for each species and each experiment were counted using a haemocytometer under a light microscope. The cell density was determined per cm2 following coral surface area determinations used the paraffin wax technique (Stimson and Kinzie, 1991).
Photosynthetic Pigment Concentration
The slurry from determination of symbiotic cell density was also used in pigment concentration determinations. For photosynthetic pigment [chlorophyll (Chl) a and c2] analyses, the slurry was centrifuged for 5 min at 5,000 rpm. After that, the supernatant was discarded and algal pellets were re-suspended in 3 ml of 90% acetone and stored in darkness for 24 h at 4°C. After centrifugation, chlorophyll a and c2 (μg cm–2) concentrations were determined using the standard spectrophotometric method of Ritchie (2006) with absorbance measured at 630, 664, and 750 nm (Winters et al., 2009).
Calcification Rate
Buoyant weight technique was used for calculating calcification rate of the coral skeleton (Jokiel et al., 1978; Spencer Davies, 1989). In theory each sample is weighed in seawater of known density, and the dry weight of the sample in air is estimated by using Archimedes’ principle (Sinutok et al., 2011). Each replicate coral nubbin was weighted at the start of the experiment and the end of experiment (week 4) using a 4-digit precision balance (OHAUS, United States). Skeleton bulk densities used for P. lutea and P. acuta were 1.19 and 2.01 g cm–3, respectively (Tanzil et al., 2009; Ng et al., 2019). The temperature and salinity of seawater were measured first. Then the glass stopper was weighted both in air and in water for calculating the density of seawater (Spencer Davies, 1989). The skeleton growth rate was calculated from equation:
in which G = growth, a = weight at the end of experiment, b = weight at the start of experiment, and c = number of days between measuring a and b.
Statistical Analysis
For zooxanthellae density, pigment contents and calcification rate, one-way ANOVA was used to test for significant differences between the marine debris treatments. For chlorophyll fluorescence parameters, mixed repeated ANOVA was used to determine statistical differences among times of sampling, treatments and species. All tests used 95% confidence level threshold. Post hoc LSD test was used to determine statistical differences among the distinct groups. If the data distribution was not normal (Kolmogorov–Smirnov test) or not equal in variances (Levene’s test), the data were transformed with square-root or log10 before testing. If the transformed data did not meet the assumptions, non-parametric tests were used.
Results
Visual Appearances and Physicochemical Properties of Seawater
In transparent plastic bag treatment, the plastic gradually turned opaque due to biofouling from algae. The light reduction increased from 3% at the start to 76% at the end of experiment. However, with the opaque plastic bag treatment, light reduction increased from 15 to 89% during the same 4 weeks treatment. A few P. acuta fragments showed a slight color decrease during the experiment. Dissolved oxygen under opaque and transparent plastic covered area did not significantly decline when compared with the area above plastic, indicating no suffocation effects on the corals (Table 1). In the fishing net treatment, we observed the production of mucus. P. acuta showed significant bleaching after 2 weeks and death at the end of experiment (Figure 2D). P. lutea had partial tissue necrosis (Figure 2H) and swelling of the tissues as a result of the fishing net treatment (Figure 2I).
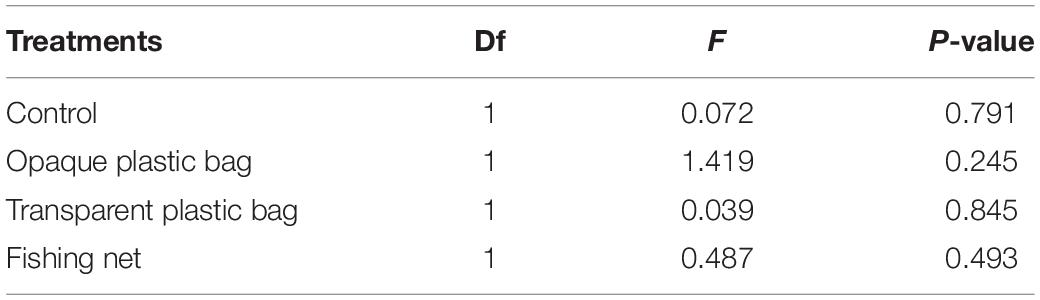
Table 1. Statistical analysis of dissolved oxygen above and below marine debris in each treatment tank.
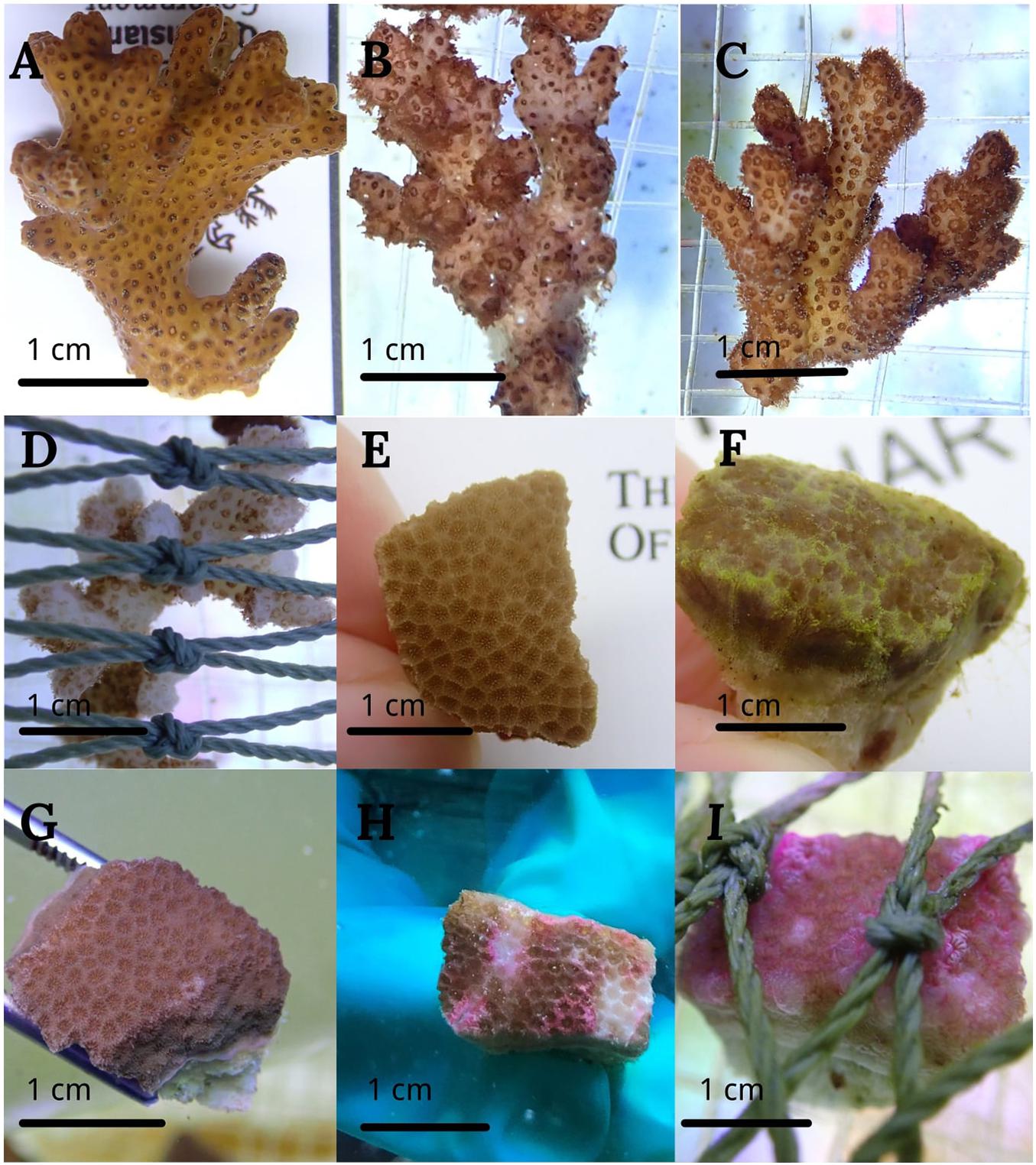
Figure 2. Images of the different corals subjected to treatments for 4 weeks. P. acuta and P. lutea in control (A,E), transparent bag (B,F), opaque bag (C,G), and fishing net treatments (D,H,I).
Chlorophyll a Fluorescence
Mixed repeated ANOVA showed that Fv/Fm of P. acuta had stabilized and remained mostly constant over the 4-week experiment (p = 0.115; Figure 3A), and there were no significant differences among treatments (p = 0.370; Figure 3A). Fv/Fm of P. lutea was more sensitive to marine debris stress than P. acuta (p < 0.05). Fv/Fm of P. lutea that was exposed to opaque bag, transparent bag or net treatment significantly declined from T7 (p < 0.05; LSD’s post hoc comparisons). At the end of the exposure period (week 4), Fv/Fm of P. lutea with opaque bag, transparent bag and net treatments were significantly lower than for the control (p = 0.001; Figure 3B). Fv/F0 had similar trend as Fv/Fm in both of the two species. P. lutea responded more to marine debris than P. acuta in all three treatments (p < 0.05; Figures 3C,D). There was no significant difference among treatments in P. acuta (p = 0.271; Figure 3C), but Fv/F0 in opaque bag, transparent bag and fishing net treatments were significantly lower than in control treatment of P. lutea (p < 0.001; Figure 3D) after 2 weeks (LSD post hoc comparisons) (Table 2).
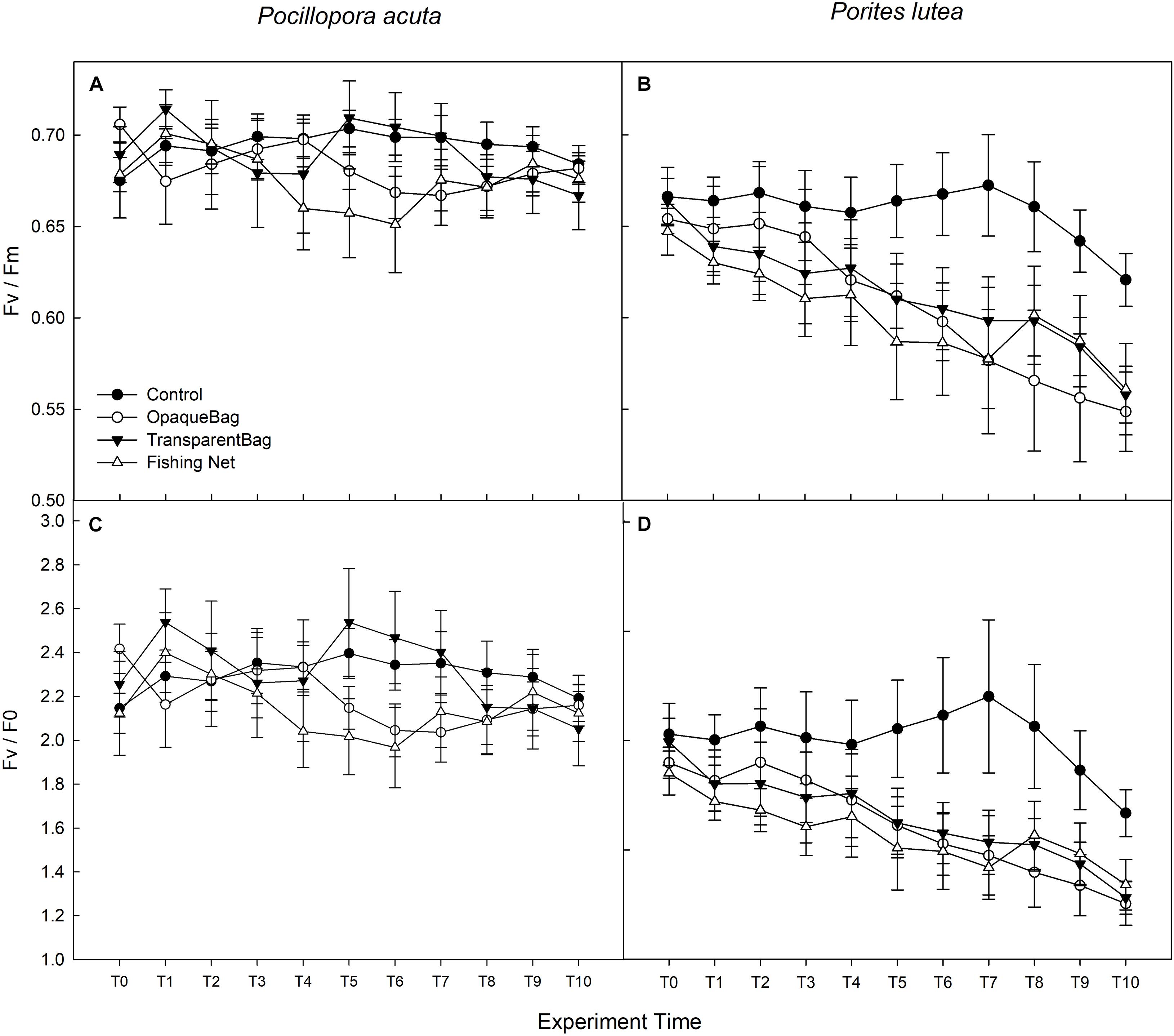
Figure 3. Maximum quantum yield of PSII (Fv/Fm; A,B) and Fv/F0 (C,D) in P. acuta (left column), and P. lutea (right column) from T0 to T10 (Graph treated with moving average, T1 in week 1, T4 in week 2, T7 in week 3, and T10 in week 4). Data are presented as Mean ± SE (n = 6).
Measurements of RLC were used to calculate alpha, rETRmax and Ek. Alpha of P. acuta had slightly changed but without significant differences over time (p = 0.672; Figure 4A) or among treatments (p = 0.927; Figure 4A). In P. lutea, alpha significantly decreased over the exposure period (p = 0.005; Figure 4B), but had no significant differences amongst treatments (p = 0.132; Figure 4B and Table 2).
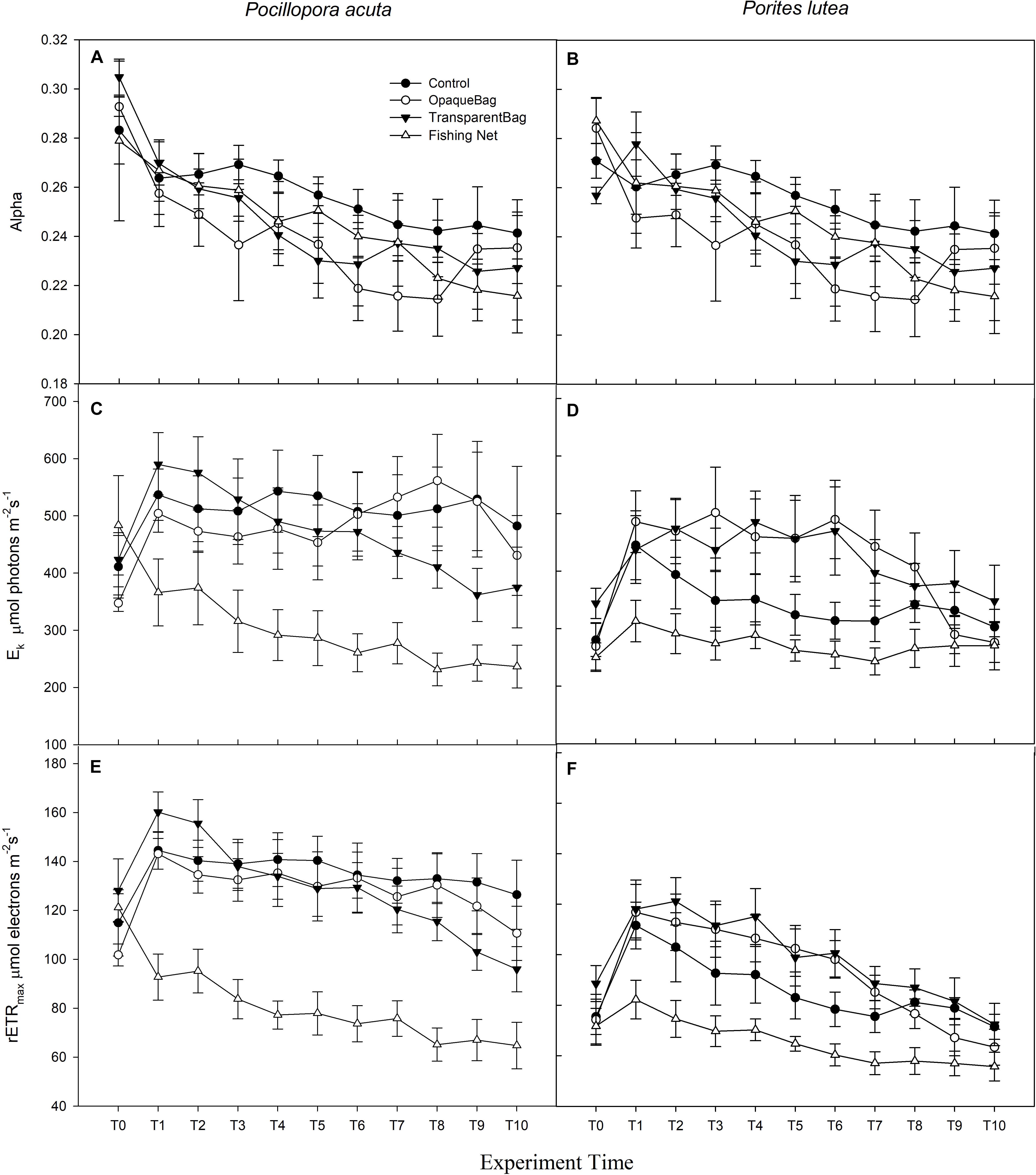
Figure 4. Alpha (A,B), Ek (C,D; μmol photons m–2 s–1), and rETRmax (E,F; μmol electrons m–2 s–1) of P. acuta (left column charts), and P. lutea (right column charts) from T0 to T10 (Graph treated with moving average, T1 in week 1, T4 in week 2, T7 in week 3, and T10 in week 4). Data are presented as Mean ± SE (n = 6).
Regarding Ek and rETRmax in P. acuta and P. lutea, the impact of marine debris was strong in the fishing net treatment. Both Ek and rETRmax of P. acuta (p < 0.001 and p < 0.001, respectively; Figures 4C,E) and P. lutea (p < 0.001 and p < 0.001, respectively; Figures 4D,F) in fishing net treatments were significantly lower than in the control, opaque bag or transparent bag treatments (Table 2).
Chlorophyll Concentration
After 2 weeks, there were no significant differences in chlorophyll a and c2 concentrations per surface area (μg cm–2) in P. acuta (p = 0.101 and p = 0.140, respectively; Figures 5A,C) or in P. lutea (p = 0.400 and p = 0.363, respectively; Figures 5B,D) among the treatments. However, cellular chlorophyll a concentration (pg cell–1) of P. acuta and P. lutea in opaque bag treatment were significantly higher than in control (p = 0.001 and p = 0.002, respectively; Figures 5E,F). When measured after 4 weeks, the cellular chlorophyll a concentration in both species had returned to the same level as the control (p = 0.680 and p = 0.230, respectively; Figures 5E,F).
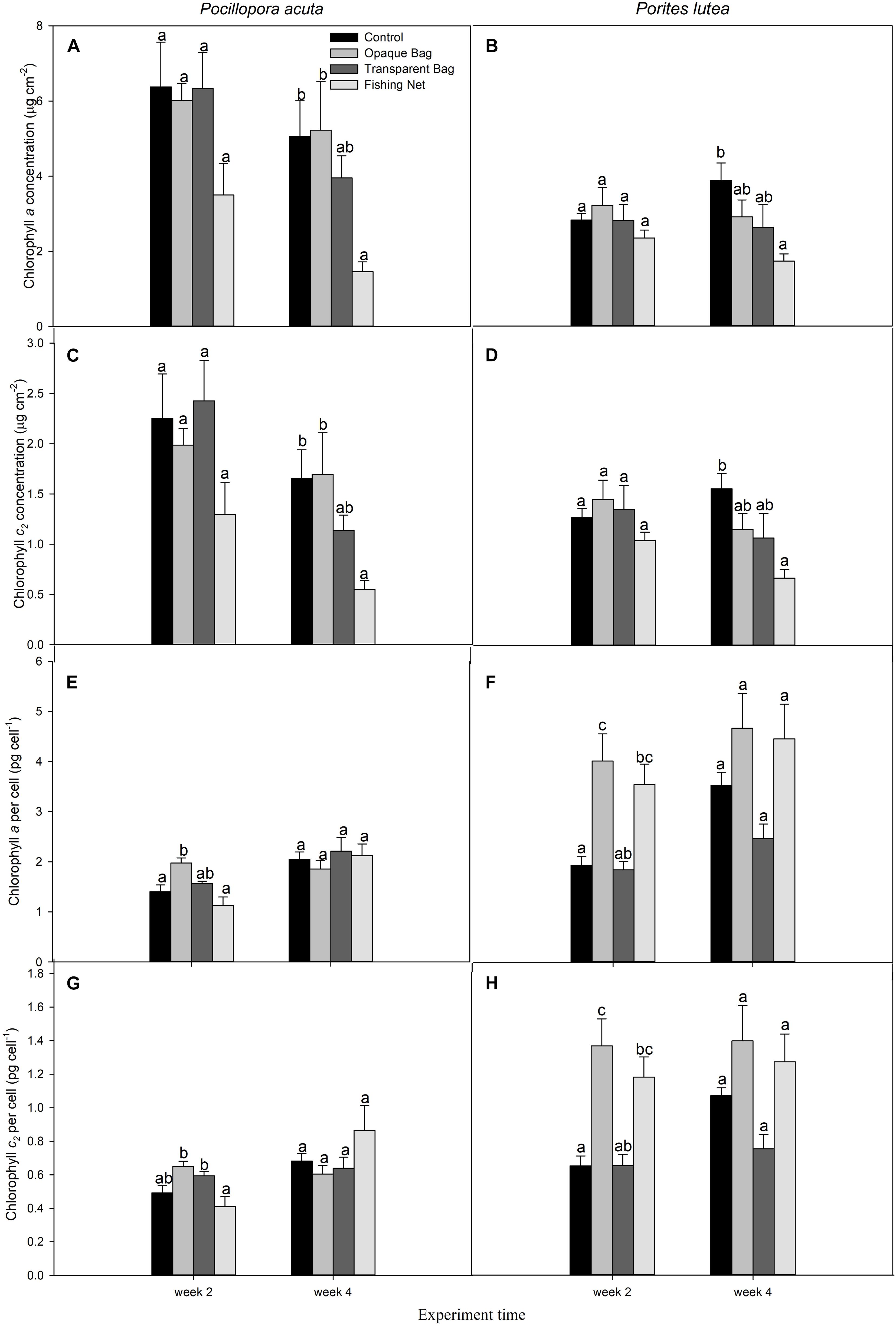
Figure 5. Chlorophyll a concentration (μg cm–2; A,B), chlorophyll c2 concentration (μg cm–2; C,D), cellular chlorophyll a concentration (pg cell–1; E, F), and cellular chlorophyll c2 concentration (pg cell–1; G,H) of P. acuta (left column chart) and P. lutea (right column chart) subjected to treatments, at week 2 and week 4. Data are presented as Mean ± SE (n = 6). a, b, and c indicate significant difference.
After 2 weeks, there were no changes in chlorophyll a and c2 concentrations in fishing net treated P. acuta (p > 0.05), but surface chlorophyll a and c2 concentrations decreased from control in week 4 (p = 0.021 and p = 0.021, respectively; Figures 5A,C,G). In P. lutea chlorophyll a and c2 concentrations per surface area declined in week 4 (p = 0.026 and p = 0.013, respectively; Figures 5B,D), but interestingly in week 2 chlorophyll a and c2 concentrations per cell had increased (p = 0.002 and p = 0.001, respectively; Figures 5F,H). At the end of treatment, the chlorophyll a and c2 concentrations per cell had the same levels as in control (p = 0.230 and p = 0.244, respectively; Figures 5F,H and Table 3).
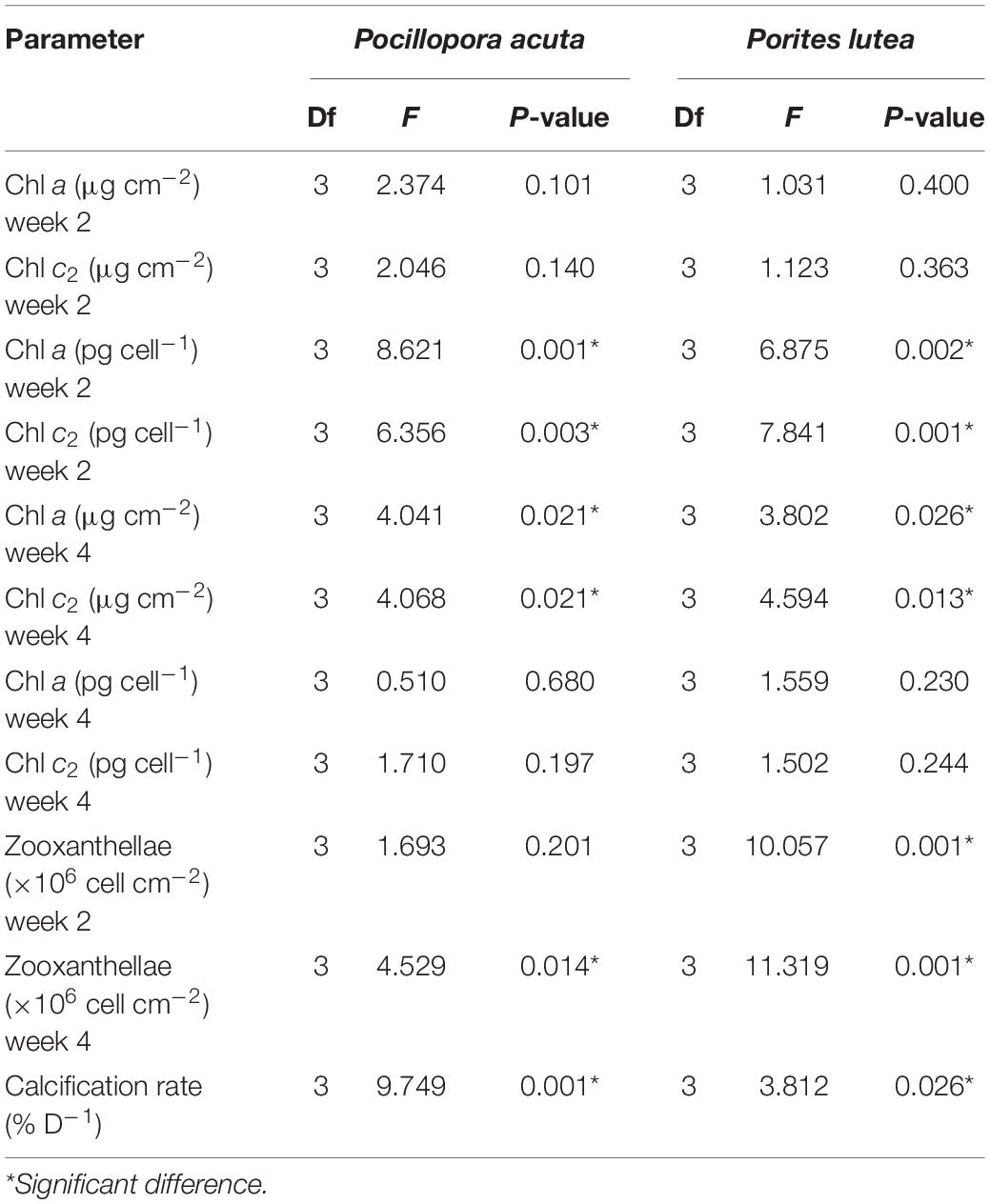
Table 3. Statistical analysis of chlorophyll a and c2, zooxanthellae density and calcification rate (One-way ANOVA).
Zooxanthellae Density
In P. acuta, there was no significant difference in symbiont density after 2 weeks of exposure to marine debris (p = 0.201; Figure 6A), while there was significantly lower symbiont density in the net treatment, compared to other treatments after 4 weeks of exposure (p = 0.014; Figure 6A). In P. lutea, significantly lower zooxanthellae densities in opaque bag and fishing net treatments were observed on weeks 2 and 4 of exposure (p < 0.001 and p < 0.001, respectively; Figure 6B and Table 3).
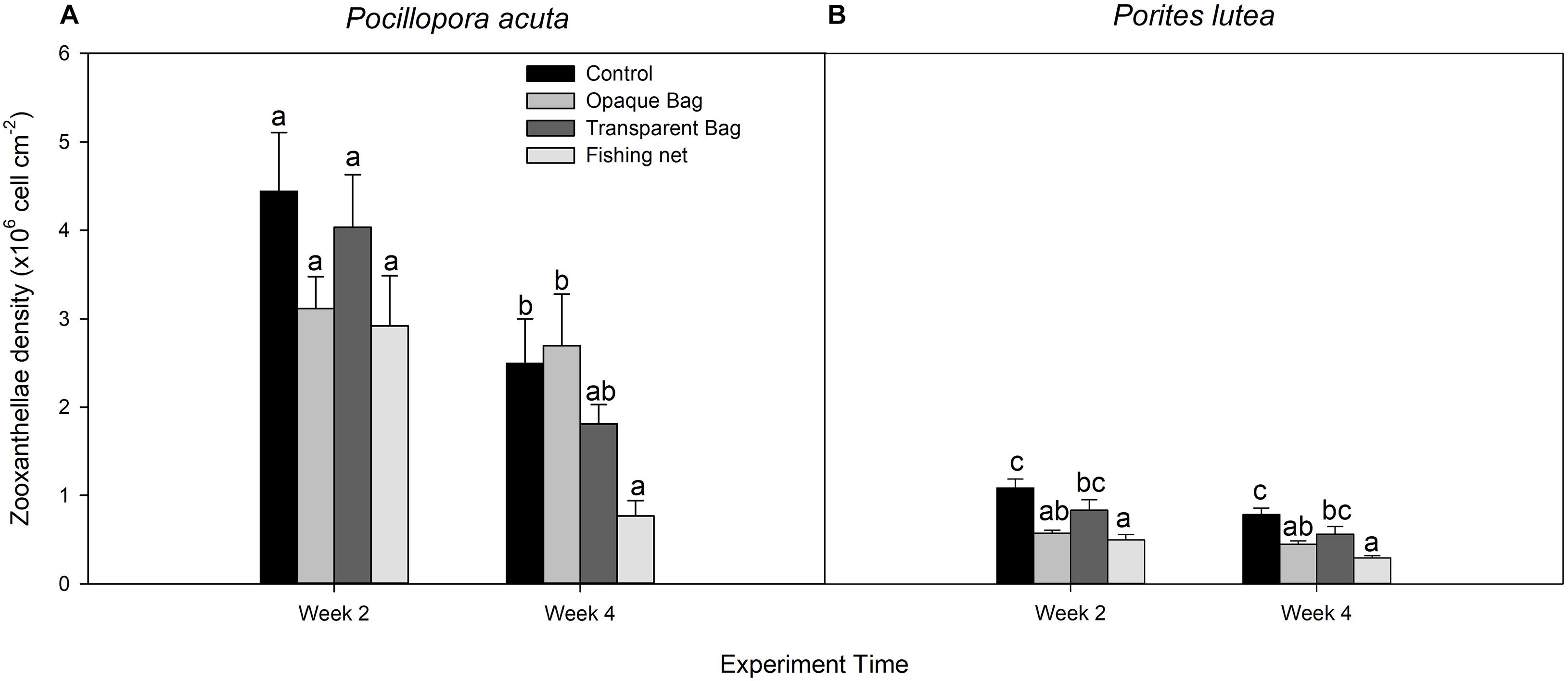
Figure 6. Zooxanthellae density of P. acuta (A) and P. lutea (B) in control, opaque bag, transparent bag, and fishing net treatments at week 2 and week 4 of experiment. Data are presented as Mean ± SE (n = 6). a, b, and c indicate significant difference.
Calcification Rate
Average calcification rate (% day–1) of both species in opaque bag, transparent bag and fishing net treatments was lower than in control treatment. Calcification rates of both species in fishing net treatment were significantly lower than in the other treatments (p < 0.001 and p = 0.026, respectively; Figures 7A,B and Table 3).
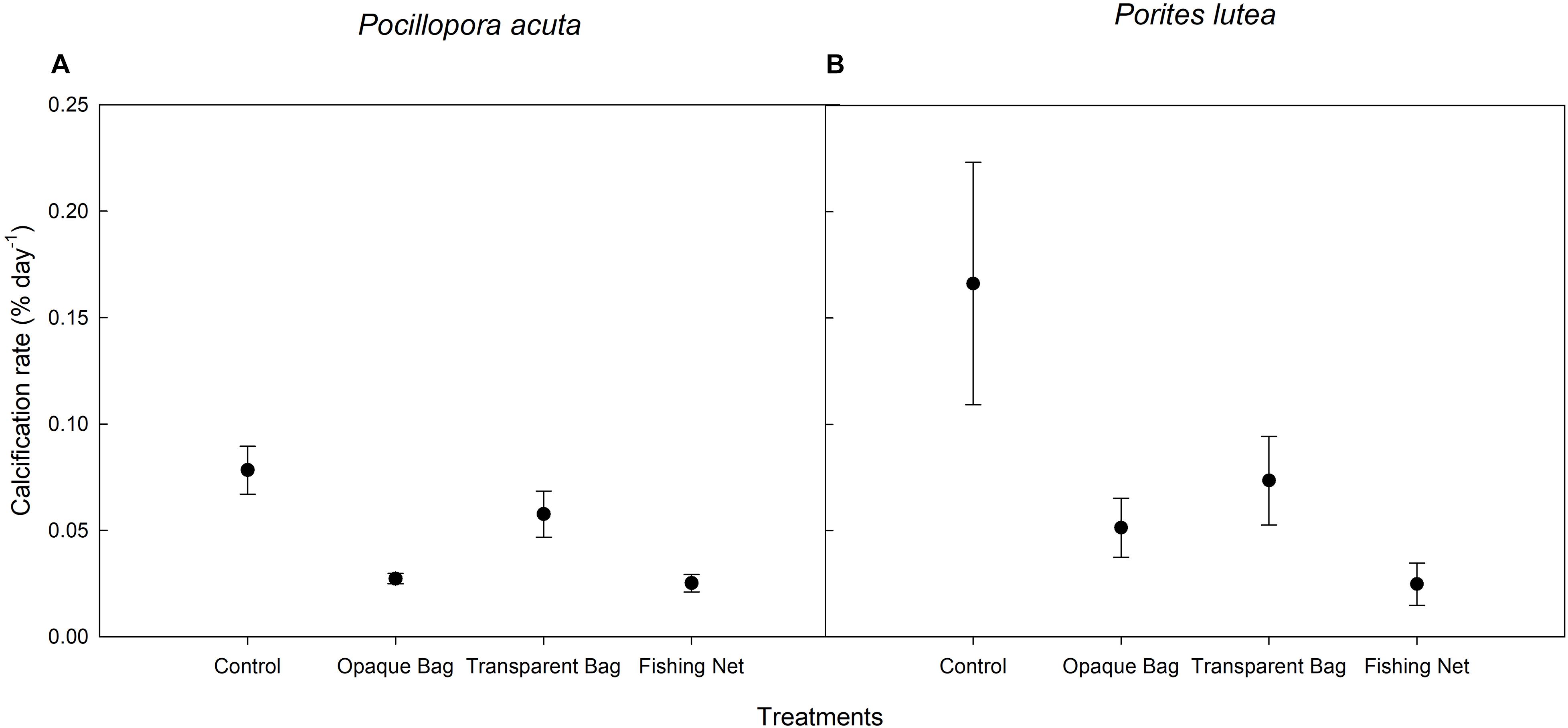
Figure 7. Calcification rate of P. acuta (A) and P. lutea (B) in control, opaque bag, transparent bag, and fishing net treatments. Data are presented as Mean ± SE (n = 6).
Discussion
Opaque Plastic Bag and Transparent Plastic Bag
Plastic bag debris can cause coral shading and suffocation resulting in bleaching as well as physical damage by abrasion, necrosis and ultimately mortality (Ryan, 2015; Valderrama Ballesteros et al., 2018). Indeed, shading directly decreases light availability for photosynthesis used by zooxanthellae, and we measured that response with opaque plastic bag and transparent plastic bag which, respectively, reduced light by 15 and 3% at the start of treatment. Although corals in close contact with plastic bags can suffer suffocation from oxygen depletion and lack of metabolite exchange (Ryan, 2015; Mouchi et al., 2019), in our experiments the plastic bag was located 2 cm above the coral, and the dissolved oxygen level under plastic covered area did not significantly decline relative to the area above the plastic bag, and so no suffocation was observed (Table 3). After 4 weeks of experimental treatment, the shading had increased to 89 and 76% for opaque plastic bag and transparent plastic bag cases, which was caused by algae covering the plastic bags. P. acuta showed no significant change in any indicator of chlorophyll fluorescence from opaque or transparent plastic bag stress. In contrast, photochemical efficiency (Fv/Fm) of P. lutea significantly declined in transparent and opaque plastic bag treatments, while efficiency of photosynthesis (alpha), saturating irradiance (Ek), and maximum rETRmax were not significantly different. This may indicate that P. lutea has less resilience to shading than P. acuta. Species-specific resilience to marine plastic debris has been found in scleractinian corals (Mouchi et al., 2019; Mueller and Schupp, 2020). A Fv/Fm decline can be caused by limited light availability, low density of symbionts and D1 protein degradation in PS II (Keren et al., 1997; Warner et al., 1999; Muller-Parker et al., 2015). As a result, the symbiont density decreased in the opaque plastic bag treatment in P. lutea, confirming the decline in maximum quantum yield. The study by Mueller and Schupp (2020) observed that Porites rus slightly paled in the first 21 days, then rapidly decreased when exposed to a transparent plastic bag, which might explain the fact that zooxanthellae density did not significantly decrease in our 4 weeks of experiment. Keshavmurthy et al. (2014) put forward the resistance mechanisms of hosts or the sustainable relationships with the clades or types of Symbiodiniaceae corals that can contend natural and anthropogenic stresses. Different coral species can associate with different symbiont compositions and the variety of components of Symbiodiniaceae provide unequal tolerances (Rouzé et al., 2016). Most of P. acuta in the sampling area associated with symbionts in Durusdinium spp. (D1) and Durusdinium sp. (D1-6; unpublicized report from Yucharoen, 2020), while P. lutea is commonly dominated by C15 (Cladocopium sp.; Chankong et al., 2018, 2020; Tan et al., 2020). However, there is no direct evidence to demonstrate that coral reactions to marine debris were affected by genus of zooxanthellae, while from the changed environmental factors, we hypothesized that types of Symbiodiniaceae might drive the corals to perform differently under marine debris stress.
Physella acuta in the opaque plastic bag treatment had an effect on its calcification rate. The skeleton growth of scleractinian corals is mainly influenced by light and water flow (Scoffin et al., 1992; Houlbrèque et al., 2003; Schutter et al., 2011; Browne, 2012; Wijgerde et al., 2012). Light motivates coral tissue extension and skeleton growth (Mass et al., 2007; Schutter et al., 2011; Cohen and Dubinsky, 2015; Eyal et al., 2019). During the decreased light condition in opaque plastic bag treatment, we considered that calcification rate got affected by declined advisability light. Furthermore, symbiotic zooxanthellae mainly affect hermatypic host calcification with photosynthesis, which supplies the inorganic carbon (Erez et al., 2011). Under lower light conditions, corals will reduce the growth rate due to less photosynthesis and calcification (Anthony and Hoegh-Guldberg, 2003b) Furthermore, decreased calcification may represent the photosynthetic performance of symbionts. In our study, the photosynthetic activity in P. acuta had no effect throughout, but the cellular chlorophyll a and c2 concentrations temporarily increased in week 2, which confirms the photoacclimation mechanisms of endosymbiotic dinoflagellates that adapt to reduced light conditions (Falkowski and Dubinsky, 1981; Jones and Yellowlees, 1997; Anthony and Hoegh-Guldberg, 2003a; Stambler and Dubinsky, 2005; Jones et al., 2020). P. lutea also increased cellular chlorophyll a and c2 concentrations in week 2, but continuous symbiont cell degradation was observed throughout. In some extreme low light conditions, decreased symbiont density also serves as photoacclimation of coral physiological responses to environmental conditions (Warner et al., 2002; Ulstrup et al., 2008).
In general, plastic shading affected more the response indicators for P. lutea than for P. acuta. This may be linked to the complex architecture of P. acuta, which provides light microhabitat diversification, thereby improving light utilization by the branching coral (Helmuth et al., 1997; Anthony and Hoegh-Guldberg, 2003b). Flow morphology like that of a massive coral has more work required for adaption to light-limited conditions, whereas species that have complex architectures, they are able to occupy extremely low or high-light regimes and consequently may have reduced competition in those habitats. Also, a greater physiological tolerance of light-habitat dynamics (e.g., after physical disturbances) will increase the physiological potential for colonization of newly formed light gaps.
The amount of biofouling by algae on plastic bags, which increased as the experiment proceeded, might also be a factor. Algal competition with coral has been proven, and it can affect corals via allelochemicals, abrasion, shading and overgrowth (McCook et al., 2001; Jompa and McCook, 2003; Rasher et al., 2011; Vieira et al., 2016). Swierts and Vermeij (2016) assumed that fast-growing corals (e.g., branching coral) suffer negative effects on growth from competition with epiphytic algae.
Fishing Net
In our experimental plan, the fishing net directly contacted coral fragments creating the potential for abrasion. Both species showed strong effects from fishing net stress, but different coral species respond to fishing nets differently. Heavy bleaching was observed in P. acuta after treatment with a fishing net for 2 weeks (Figure 2D). Bleaching is associated with coral health, because bleaching results from coral releasing symbiotic zooxanthellae (Douglas, 2003), making the coral more sensitive to diseases (Muller et al., 2008), and can negatively affect overall coral growth (Pratchett et al., 2015; McClanahan et al., 2018). Cell degradation occurred and surface chlorophyll concentration significantly declined after week 2, confirming this speculation. Tissue necrosis in P. lutea was observed under our treatments (Figure 2H), which can result in symbiont degradation and photosynthetic pigment decrease. Moreover, corals with small polyps have limited ability to physically clean up the foreign matter, leading to bleaching and tissue mortality (Philipp and Fabricius, 2003).
The significantly reduced rETRmax indicates the maximum photosynthetic capacity and this might affect growth of symbionts (Gao and Zheng, 2010; Xu et al., 2020). From prior research, abrasion of coral tissue by marine debris can result in disease outbreaks (Lamb et al., 2018), leading to decreased chlorophyll pigments and zooxanthellae cell degradation (Cervino et al., 2004). Therefore, we hypothesize that after 4 weeks coverage with fishing net, the reduced symbiont density and lower chlorophyll are related to coral disease. Furthermore, physical connection of nets to the corals affected heterotrophic energy acquisition, and increased energy consumption to produce mucus (Philipp and Fabricius, 2003). Producing the mucus required 35% of energy from an endosymbiotic (Riegl and Branch, 1995). Mainly, this energy source is used for host calcification and reproduction via symbionts synthesizing surplus carbon (Castrillón-Cifuentes et al., 2017), but under sedimentation conditions, the respiration from mucus production reversed the dominance and took up 65% from symbionts. Long-term energy cost combined with limited photosynthetic energy gain severely affected coral calcification (Anthony and Fabricius, 2000).
Porites trematodiasis is the most common disease caused by trematode larvae and manifests as pink swollen nodules, which we observed when P. lutea tissue contacted fishing nets (Figure 2I). Both biotic and abiotic stresses can cause coral disease outbreaks, and changes in environmental conditions can promote physiological stresses that impair host immunity (Jackson and Tinsley, 2002; Lafferty and Holt, 2003). Similarly, the red algal Corallophila huysmansii overgrowth on Porites cylindrica caused severe stress and mortality (Jompa and McCook, 2003).
Fishing net not only severely affected maximum quantum yield of PSII, chlorophyll a and c2 concentrations, symbiotic cell density and calcification rate, but also caused actual tissue necrosis in P. lutea under our laboratory conditions (Figure 2H). Instead, heavy bleaching was observed in P. acuta (Figure 2D). Corals with small polyps have limited ability to remove foreign matter and sediment particles, contributing to coral bleaching, and tissue mortality (Philipp and Fabricius, 2003). Significantly reduced rETRmax as well as the maximum photosynthetic capacity indicated the poor growth status of symbionts (Gao and Zheng, 2010; Xu et al., 2020). From previous research, marine debris abrasion on coral tissue induces disease outbreaks (Lamb et al., 2018), and diseases have led to a decrease in chlorophyll pigment and to zooxanthellae cell degradation (Cervino et al., 2004). Therefore, we hypothesized that after 4 weeks of covering with fishing net, the reduced symbionts and decreased chlorophyll were related to coral diseases. Interestingly, we also observed that cellular chlorophyll concentration increased in the second week, due to the photoacclimatization of living zooxanthellae that adapted to reduced light intensity and increased cellular chlorophyll to increase energy capture (Falkowski and Dubinsky, 1981; Jones and Yellowlees, 1997; Anthony and Hoegh-Guldberg, 2003a; Jones et al., 2020). Furthermore, exposure to fishing nets reduced energy acquired from photosynthesis and affected heterotrophic energy acquisition, increasing energy consumption to produce mucus (Philipp and Fabricius, 2003). Long-term negative energy costs combined with limited photosynthetic energy gains severely affected coral calcification (Anthony and Fabricius, 2000).
Conclusion
Different species of corals respond differently to various types of marine debris, differently. The responses may depend on the symbiont composition and density, coral morphology, and also on the resilience of the coral species. In this experiment, P. acuta showed less effects from each form of marine debris exposure than P. lutea, and a transparent plastic bag showed no effects on P. acuta. The different kinds of damage from different types of marine debris also make corals respond in different ways. Two types of plastic bag resulted in shading impacts on the corals in this experiment, whereas fishing net treatment affected corals with abrasion. The fishing net treatment had the strongest effects on both coral species tested. In conclusion, marine debris cover on coral causes stress responses in 2 weeks. We hope this work can help marine national parks and any related organizations to prioritize the clearing of marine debris. In aquarium experiments, we have separately assessed the shading and abrasion effects from marine debris, while in natural environment waves cause both shading and abrasion to happen simultaneously, which may accelerate the decline of coral health. The combined responses remain poorly understood and can be addressed in future work.
Data Availability Statement
The raw data supporting the conclusions of this article will be made available by the authors, without undue reservation.
Author Contributions
LY, SS, PA, PR, and PC contributed to conception and design of the study. LY, SS, PP, and PC processed the experiment. LY, SS, and PC organized the database, performed the statistical analysis, and wrote the first draft of the manuscript. All authors contributed to manuscript revision, read, and approved the submitted version.
Funding
This work is financially supported by The Institute for the Promotion of Teaching Science and Technology (IPST; DPST Graduate with First Placement; Grant Number: 006/2559), the Coastal Oceanography and Climate change Research Center, Prince of Songkla University (PSU), the Faculty of Environmental Management, PSU, and the Graduate School of PSU.
Conflict of Interest
The authors declare that the research was conducted in the absence of any commercial or financial relationships that could be construed as a potential conflict of interest.
Publisher’s Note
All claims expressed in this article are solely those of the authors and do not necessarily represent those of their affiliated organizations, or those of the publisher, the editors and the reviewers. Any product that may be evaluated in this article, or claim that may be made by its manufacturer, is not guaranteed or endorsed by the publisher.
Acknowledgments
We thank the Coastal Oceanography and Climate Change Research Center (COCC), the Marine and Coastal Resources Institute (MACORIN), and the Faculty of Environmental Management, Prince of Songkla University for research facilities. The authors thank Mathinee Yucharoen and Phatcharee Roekngandee for assistances in the laboratory. The authors thank Seppo Karrila and the Research and Development Office, PSU for assistance with proofing the English.
References
Abu-Hilal, A., and Al-Najjar, T. (2009). Marine litter in coral reef areas along the Jordan Gulf of Aqaba, Red Sea. J. Environ. Manag. 90, 1043–1049. doi: 10.1016/j.jenvman.2008.03.014
Anthony, K. R. N., and Hoegh-Guldberg, O. (2003a). Kinetics of photoacclimation in corals. Oecologia 134, 23–31. doi: 10.1007/s00442-002-1095-1
Anthony, K. R. N., and Hoegh-Guldberg, O. (2003b). Variation in coral photosynthesis, respiration and growth characteristics in contrasting light microhabitats: an analogue to plants in forest gaps and understoreys? Funct. Ecol. 17, 246–259. doi: 10.1046/j.1365-2435.2003.00731.x
Anthony, K. R. N., and Fabricius, K. E. (2000). Shifting roles of heterotrophy and autotrophy in coral energetics under varying turbidity. J. Exp. Mar. Biol. Ecol. 252, 221–253. doi: 10.1016/S0022-0981(00)00237-9
Anthony, K. R. N., Kline, D. I., Diaz-Pulido, G., Dove, S., and Hoegh-Guldberg, O. (2008). Ocean acidification causes bleaching and productivity loss in coral reef builders. Proc. Natl. Acad. Sci. U.S.A. 105, 17442–17446. doi: 10.1073/pnas.0804478105
Barnes, D. K. A., Galgani, F., Thompson, R. C., and Barlaz, M. (2009). Accumulation and fragmentation of plastic debris in global environments. Philos. Trans. R. Soc. B Biol. Sci. 364, 1985–1998. doi: 10.1098/rstb.2008.0205
Behrenfeld, M. J., Prasil, O., Kolber, Z. S., Babin, M., and Falkowski, P. G. (1998). Compensatory changes in Photosystem II electron turnover rates protect photosynthesis from photoinhibition. Photosynth. Res. 58, 259–268. doi: 10.1023/A:1006138630573
Bourne, D. G., Morrow, K. M., and Webster, N. S. (2016). Insights into the coral microbiome: underpinning the health and resilience of reef ecosystems. Annu. Rev. Microbiol. 70, 317–340. doi: 10.1146/annurev-micro-102215-095440
Browne, N. K. (2012). Spatial and temporal variations in coral growth on an inshore turbid reef subjected to multiple disturbances. Mar. Environ. Res. 77, 71–83. doi: 10.1016/j.marenvres.2012.02.005
Buhl-Mortensen, L., and Buhl-Mortensen, P. (2017). Marine litter in the Nordic Seas: distribution composition and abundance. Mar. Pollut. Bull. 125, 260–270. doi: 10.1016/j.marpolbul.2017.08.048
Castrillón-Cifuentes, A. L., Lozano-Cortés, D. F., and Zapata, F. A. (2017). Effect of short-term subaerial exposure on the cauliflower coral, Pocillopora damicornis, during a simulated extreme low-tide event. Coral Reefs 36, 401–414. doi: 10.1007/s00338-017-1552-2
Cervino, J. M., Hayes, R., Goreau, T., and Smith, G. (2004). Zooxanthellae regulation in yellow blotch/band and other coral diseases contrasted with temperature related bleaching: in situ destruction vs expulsion. Symbiosis 37, 63–85.
Chankong, A., Kongjandtre, N., Senanan, W., and Manthachitra, V. (2018). Genetic diversity of symbiodiniaceae associated with Porites lutea and Pocillopora damicornis in the Gulf of Thailand Inferred from nucleotide sequences of internal transcribed spacer-2. J. Fish. Environ. 42, 66–79.
Chankong, A., Kongjandtre, N., Senanan, W., and Manthachitra, V. (2020). Community composition of Symbiodiniaceae among four scleractinian corals in the eastern Gulf of Thailand. Reg. Stud. Mar. Sci. 33:100918. doi: 10.1016/j.rsma.2019.100918
Chapron, L., Peru, E., Engler, A., Ghiglione, J. F., Meistertzheim, A. L., Pruski, A. M., et al. (2018). Macro- and microplastics affect cold-water corals growth, feeding and behaviour. Sci. Rep. 8:15299. doi: 10.1038/s41598-018-33683-6
Cohen, I., and Dubinsky, Z. (2015). Long term photoacclimation responses of the coral Stylophora pistillata to reciprocal deep to shallow transplantation: photosynthesis and calcification. Front. Mar. Sci. 2:45. doi: 10.3389/fmars.2015.00045
Dameron, O. J., Parke, M., Albins, M. A., and Brainard, R. (2007). Marine debris accumulation in the Northwestern Hawaiian Islands: an examination of rates and processes. Mar. Pollut. Bull. 54, 423–433. doi: 10.1016/j.marpolbul.2006.11.019
de Carvalho-Souza, G. F., Llope, M., Tinôco, M. S., Medeiros, D. V., Maia-Nogueira, R., and Sampaio, C. L. S. (2018). Marine litter disrupts ecological processes in reef systems. Mar. Pollut. Bull. 133, 464–471. doi: 10.1016/j.marpolbul.2018.05.049
Donohue, M. J., Boland, R. C., Sramek, C. M., and Antonelis, G. A. (2001). Derelict fishing gear in the Northwestern Hawaiian Islands: diving survey and debris removal in 1999 confirm threat to coral ecosystems. Mar. Pollut. Bull. 42, 1301–1312. doi: 10.1016/s0025-326x(01)00139-4
Douglas, A. E. (2003). Coral bleaching—how and why? Mar. Pollut. Bull. 46, 385–392. doi: 10.1016/S0025-326X(03)00037-7
Erez, J., Reynaud, S., Silverman, J., Schneider, K., and Allemand, D. (2011). “Coral calcification under ocean acidification and global change,” in Coral Reefs: An Ecosystem in Transition, eds Z. Dubinsky and N. Stambler (Dordrecht: Springer), 151–176. doi: 10.1007/978-94-007-0114-4_10
Eriksen, M., Lebreton, L. C. M., Carson, H. S., Thiel, M., Moore, C. J., Borerro, J. C., et al. (2014). Plastic pollution in the world’s oceans: more than 5 trillion plastic pieces weighing over 250,000 tons afloat at sea. PLoS One 9:e111913. doi: 10.1371/journal.pone.0111913
Eyal, G., Cohen, I., Eyal-Shaham, L., Ben-Zvi, O., Tikochinski, Y., and Loya, Y. (2019). Photoacclimation and induction of light-enhanced calcification in the mesophotic coral Euphyllia paradivisa. R. Soc. Open Sci. 6:180527. doi: 10.1098/rsos.180527
Falkowski, P. G., and Dubinsky, Z. (1981). Light-shade adaptation of Stylophora pistillata, a hermatypic coral from the Gulf of Eilat. Nature 289, 172–174. doi: 10.1038/289172a0
Figueroa-Pico, J., Tortosa, F. S., and Carpio, A. J. (2020). Coral fracture by derelict fishing gear affects the sustainability of the marginal reefs of Ecuador. Coral Reefs 39, 819–827. doi: 10.1007/s00338-020-01926-6
Gao, K., and Zheng, Y. (2010). Combined effects of ocean acidification and solar UV radiation on photosynthesis, growth, pigmentation and calcification of the coralline alga Corallina sessilis (Rhodophyta). Glob. Chang. Biol. 16, 2388–2398. doi: 10.1111/j.1365-2486.2009.02113.x
Hall, N. M., Berry, K. L. E., Rintoul, L., and Hoogenboom, M. O. (2015). Microplastic ingestion by scleractinian corals. Mar. Biol. 162, 725–732. doi: 10.1007/s00227-015-2619-7
Harrison, P. L., and Booth, D. J. (2007). Coral reefs: naturally dynamic and increasingly disturbed ecosystems. Mar. Ecol. 1, 316–377.
Helmuth, B. S. T., Timmerman, B. E. H., and Sebens, K. P. (1997). Interplay of host morphology and symbiont microhabitat in coral aggregations. Mar. Biol. 130, 1–10. doi: 10.1007/s002270050219
Hill, R., and Ralph, P. J. (2007). Post-bleaching viability of expelled zooxanthellae from the scleractinian coral Pocillopora damicornis. Mar. Ecol. Prog. Ser. 352, 137–144. doi: 10.3354/meps07159
Houlbrèque, F., Tambutté, E., and Ferrier-Pagès, C. (2003). Effect of zooplankton availability on the rates of photosynthesis, and tissue and skeletal growth in the scleractinian coral Stylophora pistillata. J. Exp. Mar. Biol. Ecol. 296, 145–166. doi: 10.1016/S0022-0981(03)00259-4
Jackson, J., and Tinsley, R. (2002). Effects of environmental temperature on the susceptibility of Xenopus laevis and X. wittei (Anura) to Protopolystoma xenopodis (Monogenea). Parasitol. Res. 88, 632–638. doi: 10.1007/s00436-002-0629-0
Jambeck, J. R., Geyer, R., Wilcox, C., Siegler, T. R., Perryman, M., Andrady, A., et al. (2015). Plastic waste inputs from land into the ocean. Science 347, 768–771. doi: 10.1126/science.1260352
Jokiel, P. L., Maragos, J. W., and Franzisket, L. (1978). “Coral growth buoyant weight technique,” in Coral Reefs: Research Methods, eds D. R. Stoddart and R. E. Johannes (Paris: UNESCO), 529–542.
Jompa, J., and McCook, L. (2003). Coral-algal competition: macroalgae with different properties have different effects on corals. Mar. Ecol. Prog. Ser. 258, 87–95. doi: 10.3354/meps258087
Jones, R., Giofre, N., Luter, H. M., Neoh, T. L., Fisher, R., and Duckworth, A. (2020). Responses of corals to chronic turbidity. Sci. Rep. 10:4762. doi: 10.1038/s41598-020-61712-w
Jones, R. J., and Yellowlees, D. (1997). Regulation and control of intracellular algae (= zooxanthellae) in hard corals. Philos. Trans. R. Soc. Lond. Ser. B Biol. Sci. 352, 457–468. doi: 10.1098/rstb.1997.0033
Keren, N., Berg, A., van Kan, P. J. M., Levanon, H., and Ohad, I. (1997). Mechanism of photosystem II photoinactivation and D1 protein degradation at low light: the role of back electron flow. Proc. Natl. Acad. Sci. U.S.A. 94, 1579–1584. doi: 10.1073/pnas.94.4.1579
Keshavmurthy, S., Meng, P. J., Wang, J. T., Kuo, C. Y., Yang, S. Y., Hsu, C. M., et al. (2014). Can resistant coral-Symbiodinium associations enable coral communities to survive climate change? A study of a site exposed to long-term hot water input. PeerJ 2:e327. doi: 10.7717/peerj.327
Kline, D., Kuntz, N., Breitbart, M., Knowlton, N., and Rohwer, F. (2006). Role of elevated organic carbon levels and microbial activity in coral mortality. Mar. Ecol. Prog. Ser. 314, 119–125. doi: 10.3354/meps314119
Lafferty, K. D., and Holt, R. D. (2003). How should environmental stress affect the population dynamics of disease? Ecol. Lett. 6, 654–664. doi: 10.1046/j.1461-0248.2003.00480.x
Lamb, J. B., Willis, B. L., Fiorenza, E. A., Couch, C. S., Howard, R., Rader, D. N., et al. (2018). Plastic waste associated with disease on coral reefs. Science 359, 460–462. doi: 10.1126/science.aar3320
Marshall, P. A., and Baird, A. H. (2000). Bleaching of corals on the Great Barrier Reef: differential susceptibilities among taxa. Coral Reefs 19, 155–163. doi: 10.1007/s003380000086
Mass, T., Einbinder, S., Brokovich, E., Shashar, N., Vago, R., Erez, J., et al. (2007). Photoacclimation of Stylophora pistillata to light extremes: metabolism and calcification. Mar. Ecol. Prog. Ser. 334, 93–102. doi: 10.3354/meps334093
McClanahan, T. R., Weil, E., and Baird, A. H. (2018). “Consequences of coral bleaching for sessile reef organisms,” in Coral Bleaching: Patterns, Processes, Causes and Consequences, eds M. J. H. van Oppen and J. M. Lough (Heidelberg: Springer International Publishing), 231–263. doi: 10.1007/978-3-319-75393-5_10
McCook, L., Jompa, J., and Diaz-Pulido, G. (2001). Competition between corals and algae on coral reefs: a review of evidence and mechanisms. Coral Reefs 19, 400–417. doi: 10.1007/s003380000129
Moberg, F., and Folke, C. (1999). Ecological goods and services of coral reef ecosystems. Ecol. Econ. 29, 215–233. doi: 10.1016/S0921-8009(99)00009-9
Mouchi, V., Chapron, L., Peru, E., Pruski, A. M., Meistertzheim, A. L., Vétion, G., et al. (2019). Long-term aquaria study suggests species-specific responses of two cold-water corals to macro-and microplastics exposure. Environ. Pollut. 253, 322–329. doi: 10.1016/j.envpol.2019.07.024
Mueller, J. S., and Schupp, P. J. (2020). Shading by marine litter impairs the health of the two Indo-Pacific scleractinian corals Porites rus and Pavona cactus. Mar. Pollut. Bull. 158:111429. doi: 10.1016/j.marpolbul.2020.111429
Muhammad, F., Zamani, N. P., and Ismet, M. S. (2021). The effect of plastic debris attachment to the health of branching corals in Kelapa Dua Island, Thousand Islands. IOP Conf. Ser. Earth Environ. Sci. 771:012017. doi: 10.1088/1755-1315/771/1/012017
Muller, E. M., Rogers, C. S., Spitzack, A. S., and van Woesik, R. (2008). Bleaching increases likelihood of disease on Acropora palmata (Lamarck) in Hawksnest Bay, St John, US Virgin Islands. Coral Reefs 27, 191–195. doi: 10.1007/s00338-007-0310-2
Muller-Parker, G., D’Elia, C. F., and Cook, C. B. (2015). “Interactions between corals and their symbiotic algae,” in Coral Reefs in the Anthropocene, ed. C. Birkeland (Dordrecht: Springer Netherlands), 99–116. doi: 10.1007/978-94-017-7249-5_5
Ng, C. S. L., Lim, J., Sam, S. Q., Kikuzawa, Y., Toh, T. C., Wee, T., et al. (2019). Variability in skeletal bulk densities of common hard corals in Southeast Asia. Coral Reefs 38, 1133–1143. doi: 10.1007/s00338-019-01852-2
Orr, J. C., Fabry, V. J., Aumont, O., Bopp, L., Doney, S. C., Feely, R. A., et al. (2005). Anthropogenic ocean acidification over the twenty-first century and its impact on calcifying organisms. Nature 437, 681–686. doi: 10.1038/nature04095
Philipp, E., and Fabricius, K. (2003). Photophysiological stress in scleractinian corals in response to short-term sedimentation. J. Exp. Mar. Biol. Ecol. 287, 57–78. doi: 10.1016/S0022-0981(02)00495-1
Platt, T., Gallegos, C. L., and Harrison, W. G. (1980). Photoinhibition of photosynthesis in natural assemblages of marine phytoplankton. J. Mar. Res. 38, 687–701.
Pratchett, M., Anderson, K. D., Hoogenboom, M. O., Widman, E., Baird, A., Pandolfi, J., et al. (2015). Spatial, temporal and taxonomic variation in coral growth—implications for the structure and function of coral reef ecosystems. Oceanogr. Mar. Biol. 53, 215–295. doi: 10.1201/b18733-7
Putra, M. G. A., Zamani, N. P., Natih, N. M., and Harahap, S. A. (2021). Relationship between characteristics of marine debris and impact to coral reef. J. Ilmiah Perikanan Dan Kelautan 13, 11–19. doi: 10.20473/jipk.v13i1.18896
Ralph, P. J., and Gademann, R. (2005). Rapid light curves: a powerful tool to assess photosynthetic activity. Aquat. Bot. 82, 222–237. doi: 10.1016/j.aquabot.2005.02.006
Rasher, D., Stout, E., Engel, S., Kubanek, J., and Hay, M. (2011). Macroalgal terpenes function as allelopathic agents against reef corals. Proc. Natl. Acad. Sci. U.S.A. 108, 17726–17731. doi: 10.1073/pnas.1108628108
Richards, Z. T., and Beger, M. (2011). A quantification of the standing stock of macro-debris in Majuro lagoon and its effect on hard coral communities. Mar. Pollut. Bull. 62, 1693–1701. doi: 10.1016/j.marpolbul.2011.06.003
Riegl, B., and Branch, G. M. (1995). Effects of sediment on the energy budgets of four scleractinian (Bourne 1900) and five alcyonacean (Lamouroux 1816) corals. J. Exp. Mar. Biol. Ecol. 186, 259–275. doi: 10.1016/0022-0981(94)00164-9
Ritchie, R. J. (2006). Consistent sets of spectrophotometric chlorophyll equations for acetone, methanol and ethanol solvents. Photosynth. Res. 89, 27–41. doi: 10.1007/s11120-006-9065-9
Rouzé, H., Lecellier, G., Saulnier, D., and Berteaux-Lecellier, V. (2016). Symbiodinium clades A and D differentially predispose Acropora cytherea to disease and Vibrio spp. Colonization. Ecol. Evol. 6, 560–572.
Ryan, P. G. (2015). “A brief history of marine litter research,” in Marine Anthropogenic Litter, eds M. Bergmann, L. Gutow, and M. Klages (Cham: Springer), 1–25. doi: 10.1007/978-3-319-16510-3_1
Schipper, J., Chanson, J. S., Chiozza, F., Cox, N. A., Hoffmann, M., Katariya, V., et al. (2008). The status of the world’s land and marine mammals: diversity, threat, and knowledge. Science 322, 225–230. doi: 10.1126/science.1165115
Schutter, M., Kranenbarg, S., Wijffels, R. H., Verreth, J., and Osinga, R. (2011). Modification of light utilization for skeletal growth by water flow in the scleractinian coral Galaxea fascicularis. Mar. Biol. 158, 769–777. doi: 10.1007/s00227-010-1605-3
Scoffin, T. P., Tudhope, A. W., Brown, B. E., Chansang, H., and Cheeney, R. F. (1992). Patterns and possible environmental controls of skeletogenesis of Porites lutea, South Thailand. Coral Reefs 11, 1–11. doi: 10.1007/BF00291929
Sinutok, S., Hill, R., Doblin, M. A., Wuhrer, R., and Ralph, P. J. (2011). Warmer more acidic conditions cause decreased productivity and calcification in subtropical coral reef sediment-dwelling calcifiers. Limnol. Oceanogr. 56, 1200–1212. doi: 10.4319/lo.2011.56.4.1200
Spencer Davies, P. (1989). Short-term growth measurements of corals using an accurate buoyant weighing technique. Mar. Biol. 101, 389–395. doi: 10.1007/BF00428135
Stambler, N., and Dubinsky, Z. (2005). Corals as light collectors: an integrating sphere approach. Coral Reefs 24, 1–9. doi: 10.1007/s00338-004-0452-4
Stimson, J., and Kinzie, R. A. (1991). The temporal pattern and rate of release of zooxanthellae from the reef coral Pocillopora damicornis (Linnaeus) under nitrogen-enrichment and control conditions. J. Exp. Mar. Biol. Ecol. 153, 63–74. doi: 10.1016/S0022-0981(05)80006-1
Swierts, T., and Vermeij, M. J. (2016). Competitive interactions between corals and turf algae depend on coral colony form. PeerJ 4:e1984. doi: 10.7717/peerj.1984
Tan, Y. T. R., Wainwright, B. J., Afiq-Rosli, L., Ip, Y. C. A., Lee, J. N., Nguyen, N. T. H., et al. (2020). Endosymbiont diversity and community structure in Porites lutea from Southeast Asia are driven by a suite of environmental variables. Symbiosis 80, 269–277. doi: 10.1007/s13199-020-00671-2
Tanner, J. E. (1995). Competition between scleractinian corals and macroalgae an experimental investigation of coral growth, survival and reproduction. J. Exp. Mar. Biol. Ecol. 190, 151–168. doi: 10.1016/0022-0981(95)00027-O
Tanzil, J. T. I., Brown, B. E., Tudhope, A. W., and Dunne, R. P. (2009). Decline in skeletal growth of the coral Porites lutea from the Andaman Sea, South Thailand between 1984 and 2005. Coral Reefs 28, 519–528. doi: 10.1007/s00338-008-0457-5
Todd, P. A., Ong, X., and Chou, L. M. (2010). Impacts of pollution on marine life in Southeast Asia. Biodivers. Conserv. 19, 1063–1082. doi: 10.1007/s10531-010-9778-0
Ulstrup, K. E., Hill, R., van Oppen, M. J. H., Larkum, A. W. D., and Ralph, P. J. (2008). Seasonal variation in the photo-physiology of homogeneous and heterogeneous Symbiodinium consortia in two scleractinian corals. Mar. Ecol. Prog. Ser. 361, 139–150. doi: 10.3354/meps07360
Valderrama Ballesteros, L., Matthews, J. L., and Hoeksema, B. W. (2018). Pollution and coral damage caused by derelict fishing gear on coral reefs around Koh Tao, Gulf of Thailand. Mar. Pollut. Bull. 135, 1107–1116. doi: 10.1016/j.marpolbul.2018.08.033
Vieira, C., Thomas, O. P., Culioli, G., Genta-Jouve, G., Houlbreque, F., Gaubert, J., et al. (2016). Allelopathic interactions between the brown algal genus Lobophora (Dictyotales, Phaeophyceae) and scleractinian corals. Sci. Rep. 6:18637. doi: 10.1038/srep18637
Warner, M., Chilcoat, G., McFarland, F., and Fitt, W. (2002). Seasonal fluctuations in the photosynthetic capacity of photosystem II in symbiotic dinoflagellates in the Caribbean reef-building coral Montastraea. Mar. Biol. 141, 31–38. doi: 10.1007/s00227-002-0807-8
Warner, M. E., Fitt, W. K., and Schmidt, G. W. (1999). Damage to photosystem II in symbiotic dinoflagellates: a determinant of coral bleaching. Proc. Natl. Acad. Sci. U.S.A. 96, 8007–8012. doi: 10.1073/pnas.96.14.8007
Wijgerde, T., Jurriaans, S., Hoofd, M., Verreth, J. A. J., and Osinga, R. (2012). Oxygen and heterotrophy affect calcification of the scleractinian coral Galaxea fascicularis. PLoS One 7:e52702. doi: 10.1371/journal.pone.0052702
Winters, G., Holzman, R., Blekhman, A., Beer, S., and Loya, Y. (2009). Photographic assessment of coral chlorophyll contents: implications for ecophysiological studies and coral monitoring. J. Exp. Mar. Biol. Ecol. 380, 25–35. doi: 10.1016/j.jembe.2009.09.004
Xu, H., Feng, B., Xie, M., Ren, Y., Xia, J., Zhang, Y., et al. (2020). Physiological characteristics and environment adaptability of reef-building corals at the Wuzhizhou Island of South China Sea. Front. Physiol. 11:390. doi: 10.3389/fphys.2020.00390
Yucharoen, M. (2020). Stress Indicator for Coral and Zooxanthellae at the Southern Reef of Phuket for Resistance to Coral Bleaching. NSTDA. P-17-52222 Report. Thailand: National Science and Technology Development Agency.
Keywords: marine debris, plastic litter, fishing nets, ecophysiology, zooxanthellae, photoacclimation, corals
Citation: Ying L, Sinutok S, Pramneechote P, Aiyarak P, Ralph PJ and Chotikarn P (2021) Physiological Responses of Pocillopora acuta and Porites lutea Under Plastic and Fishing Net Stress. Front. Mar. Sci. 8:712214. doi: 10.3389/fmars.2021.712214
Received: 20 May 2021; Accepted: 30 August 2021;
Published: 08 October 2021.
Edited by:
Ranjeet Bhagooli, University of Mauritius, MauritiusReviewed by:
Hiroya Yamano, National Institute for Environmental Studies (NIES), JapanSuchana Chavanich, Chulalongkorn University, Thailand
Copyright © 2021 Ying, Sinutok, Pramneechote, Aiyarak, Ralph and Chotikarn. This is an open-access article distributed under the terms of the Creative Commons Attribution License (CC BY). The use, distribution or reproduction in other forums is permitted, provided the original author(s) and the copyright owner(s) are credited and that the original publication in this journal is cited, in accordance with accepted academic practice. No use, distribution or reproduction is permitted which does not comply with these terms.
*Correspondence: Ponlachart Chotikarn, cG9ubGFjaGFydC5jQHBzdS5hYy50aA==