- 1Centro de Investigación Mariña, Laboratorio de Fisioloxía Animal, Departamento de Bioloxía Funcional e Ciencias da Saúde, Facultade de Bioloxía, Universidade de Vigo, Vigo, Spain
- 2Department of Biological Sciences, University of Bergen, Bergen, Norway
- 3Department of Biological and Environmental Sciences and Technologies, University of Salento, Lecce, Italy
- 4Institute of Marine Research, Bergen, Norway
Sensing of amino acids in fish brain, especially branched-chain amino acids (BCAA) like leucine, is involved in regulation of feed intake through different mechanisms. However, there is limited information regarding the possible involvement of mechanisms dependent on amino acid carriers of the solute carrier families (SLC) known to be key regulators of intracellular leucine concentration, namely L-type amino acid transporter 1 (LAT1), and sodium-dependent neutral amino acid transporter 2 (SNAT2) and 9,(SNAT9), for which evidence of their participation is available in mammals. Comparative analysis amongst sequences revealed a complex pattern of paralogues in Atlantic salmon, for LAT1 (slc7a5aa, slc7a5ab, slc7a5ba, slc7a5bb, slc7a5ca, and slc7a5cb), SNAT2 (slc38a2a and slc38a2b) and SNAT9 (slc38a9). After establishing phylogenetic relationships of the different paralogues evaluated, samples of the selected brain areas were taken from Atlantic salmon to assess tissue distribution of transcripts. In an additional experiment, fish were fed two diets with different levels of leucine (high leucine: 35 g/kg vs. control leucine: 27.3 g/kg). The high leucine diet resulted in lower feed intake and increased mRNA abundance of specific paralogues of LAT1 (slc7a5aa, slc7a5ab, and slc7a5bb) and SNAT2 (slc38a2a and slc38a2b) though apparently not for SNAT9 in brain areas like hypothalamus and telencephalon involved in food intake regulation. The results obtained suggest a role for members of the SLC family in the anorectic effect of leucine and thus their involvement as additional amino acid sensing mechanism not characterised so far in fish regulation of feed intake.
Introduction
In fish, feed intake is commonly regulated by both homeostatic and hedonic systems (Volkoff, 2016; Rønnestad et al., 2017; Soengas et al., 2018). While the homeostatic circuits control feed intake based on energetic information from nutrients and hormones levels and/or satiety signals from the gastrointestinal system to control the whole body energy balance, the non-homeostatic circuits are driven by the motivation to consume food independently of the energy status, but where reward is an important factor (Soengas et al., 2018). The sensing of various nutrients, including amino acids levels, is a fundamental process involved in the homeostatic regulation of feed intake and the hypothalamus has been described as the central core (Conde-Sieira and Soengas, 2017; Delgado et al., 2017). However, the mechanisms involved in regulation of feed intake by the hedonic system are scarcely known (Lutter and Nestler, 2009), but the telencephalon is the putative control centre (Mueller and Wullimann, 2009). The telencephalon also relates to the capacity of sensing levels of different nutrients including amino acids (Comesaña et al., 2018b; Otero-Rodiño et al., 2018).
Branched-chain amino acids (BCAA), especially leucine, are important amino acids in terms of regulation of feed intake and energy homoeostasis in mammals (Fromentin et al., 2012). Increased post-prandial circulating levels of leucine signals availability of protein/amino acids and cause a reduction in feed intake (Heeley and Blouet, 2016). This process occurs in the hypothalamus, and depends on several mechanisms not completely understood (Heeley and Blouet, 2016). These mechanisms include BCAA metabolism, glutamine metabolism, mechanistic target of rapamycin (mTOR), taste receptor signalling, general control non-derepressible 2 (GCN2) kinase signalling, and specific amino acid carriers (Efeyan et al., 2015; Heeley and Blouet, 2016). As for the latter, amino acid carriers play a pivotal role in amino acid sensing through binding of particular amino acid to their respective transporter protein (Hundal and Taylor, 2009). The carriers suggested to be involved in leucine sensing include several members of the solute carrier (SLC) gene superfamilies L-type amino acid transporter 1 (LAT1, encoded by gene Slc7a5), sodium-dependent neutral amino acid transporter 2 (SNAT2, encoded by gene Slc38a2), and sodium-dependent neutral amino acid transporter 9 (SNAT9, encoded by gene Slc38a9) (Taylor, 2014; Wyant et al., 2017). Although other leucine transport systems are present in blood brain barrier, these ones are required for mTOR signalling activation, which has a key role in integrating nutrient and endocrine signals (Dodd and Tee, 2012). In addition, these transporters are key regulators of the intracellular concentration of leucine (Dodd and Tee, 2012; Wyant et al., 2017).
In fish, available evidence regarding amino acid sensing capacity in brain is restricted to several studies in rainbow trout (Oncorhynchus mykiss) hypothalamus and telencephalon for which comparable mechanisms to those known in mammals are described for BCAA metabolism, glutamine metabolism, mTOR, GCN2 kinase, and taste receptor signalling (Conde-Sieira and Soengas, 2017; Soengas et al., 2018). These mechanisms displayed changes after raising leucine levels through intracerebroventricular (ICV, Comesaña et al., 2018a) or intraperitoneal (IP, Comesaña et al., 2018b) treatments. The activation of these mechanisms by leucine also resulted in regulation in the hypothalamus of the expression of neuropeptides down-regulating the orexigenic NPY and AgRP and up-regulating the anorexigenic POMC-A1 finally resulting in decreased feed intake (Comesaña et al., 2018a,b). There is some evidence that suggest that these carriers are involved in leucine transport in fish brain, including slc38a2 in rainbow trout (Comesaña et al., 2018a,b) and slc7a5 in grass carp (Yang et al., 2014). Moreover, increased expression of slc38a2 was observed in rainbow trout after increasing leucine levels through IP or ICV treatments (Comesaña et al., 2018a,b). There is no other evidence regarding the response to leucine of other putative carriers. The presence of specific carriers appears to be essential for leucine sensing capacity especially in those brain areas like the hypothalamus and the telencephalon involved in feed intake control in fish (Delgado et al., 2017; Soengas et al., 2018).
Therefore, using Atlantic salmon (Salmo salar) as experimental model, in the first experiment we identified and analysed the mRNA expression distribution pattern of the main amino acid carriers putatively involved in leucine transport such as slc7a5, slc38a2, and slc38a9, and analysed their mRNA expression distribution pattern in different regions of the brain of post-smolts. Teleosts have experienced an extra round of genome duplication compared with mammals (Meyer and Van de Peer, 2005), and this is further complicated in salmonids, which underwent an additional round of genome duplication (Pasquier et al., 2016) resulting in the possibility of having up to four isoforms of carriers that might have acquired different functions. Accordingly, in this study, we found six paralogues of slc7a5, two paralogues of slc38a2, and only one paralogue for slc38a9. We hypothesised that if these transporters relate to amino acid sensing and feed intake control, their expression must change in response to the dietary level of leucine. Therefore, in a second experiment, we assessed if the rise in dietary leucine levels resulting in decreased feed intake also induced changes in mRNA abundance of all these paralogues.
Materials and Methods
Ethical Standards
The animal handling and procedures described in this study complied with the guidelines of the Norwegian Regulation on Animal Experimentation and were approved by the National Animal Research Authority in Norway (reference FOTS ID 20799).
Experimental Design
4 weeks before the experiment, Atlantic salmon post-smolts (approx. 500 g) were randomly distributed in replicate tanks (volume ca. 470 L) of 50 fish each at Cargill Innovation Center (Dirdal, Norway). Fish were continuously fed with a commercial pellet (Adapt Marine 80, Cargill 35.6% crude protein, 11% carbohydrates, 33.5% crude fat, 13% ash, and 23.7 MJ/kg of feed) 4 times a day in periods of 70 min (19:00, 22:00, 01:00, and 06:00) by using an automatic feeder (Hølland Technology, Sandnes, Norway).
The tanks were maintained under controlled conditions with constant temperature (9 ± 0.01°C), salinity (28.39 ± 0.03‰), and oxygen levels (88 ± 0.42%). Constant light conditions (LD 24:0) was used to mimic standard commercial operational procedures and is known to stimulate growth in Atlantic salmon (Hansen et al., 1992). After the acclimation period, fish were pit-tagged and groups of fish were fed with either 27.3 g leucine/kg diet (control leucine; CL) or 35.0 g leucine/kg diet (high leucine; HL) during a period of 12 weeks. During the feeding period feed was offered in slight excess (10%), and about 0.78–1.48% of the biomass was consumed daily. The diets were formulated to be isoenergetic, isoproteic, and isolipidic, very close levels of amino acids and same levels of fish meal (∼15%). The diets ingredients and proximate composition of experimental diets are shown in Tables 1, 2. Plasma levels of amino acids in fish fed both diets are shown in Supplementary Table 1. Uneaten feed was collected to enable feed intake calculation. This marked the start point of the experiment, which lasted for 12 weeks in total. Feed intake were collected during 7 days before the sampling points at week 4 and week 12, and are shown as the mean + standard error of mean (S.E.M.).
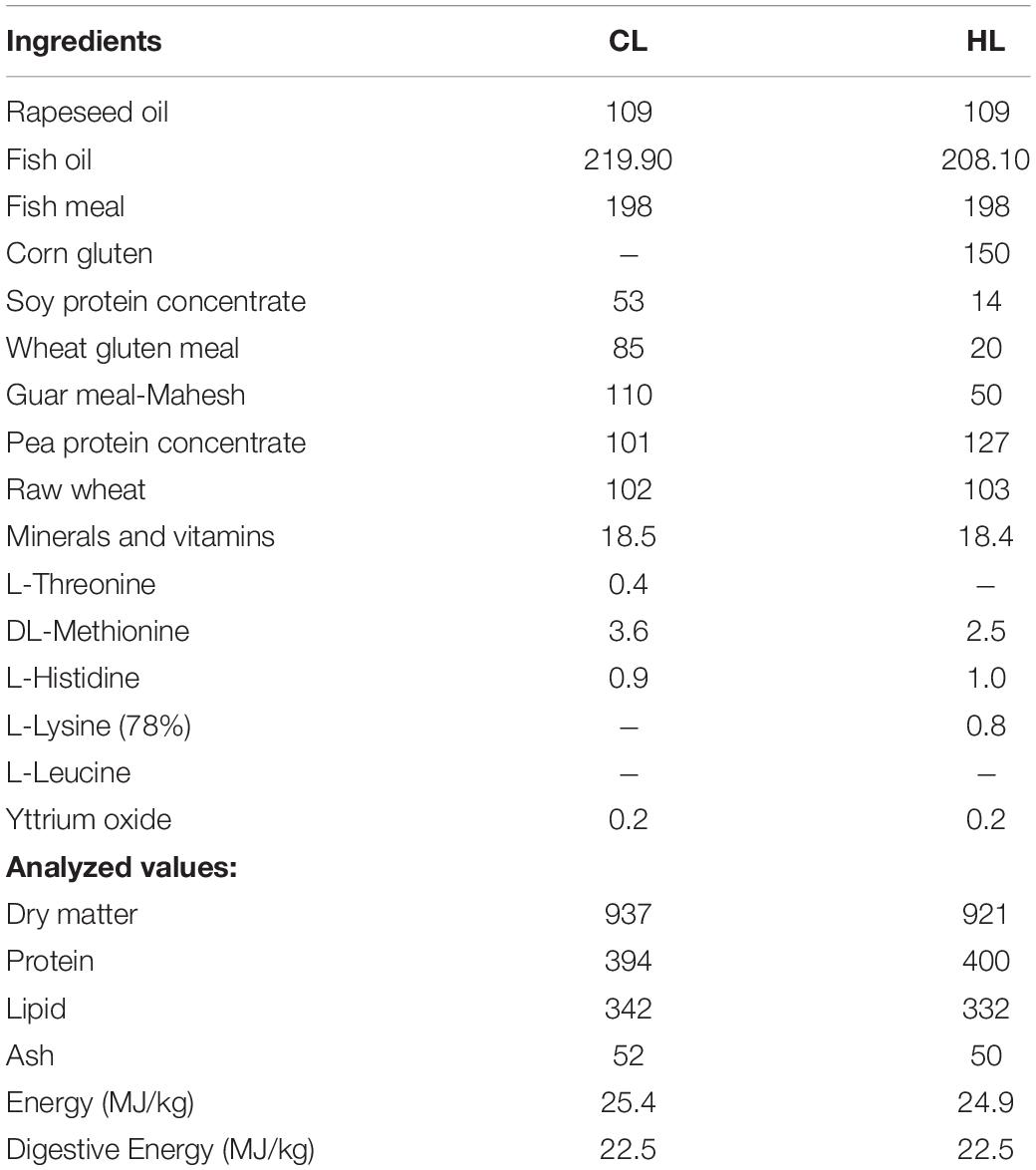
Table 1. The composition and analysed values (g/kg) of the two diets used with control leucine (CL; 27.3 g/kg) or high leucine (HL; 35 g/kg) levels.
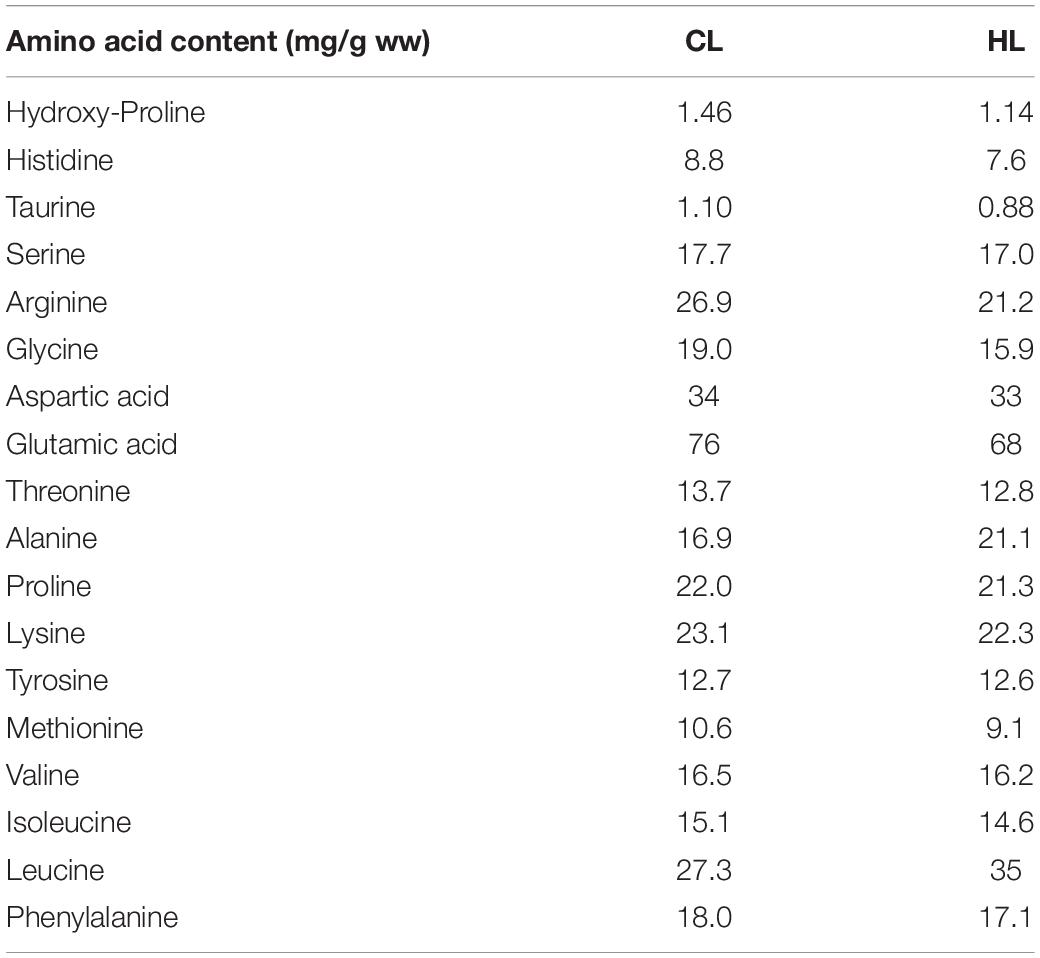
Table 2. Amino acid content (mg/g wet weight) within the experimental diets with control leucine (CL; 27.3 g/kg) or high leucine (HL; 35 g/kg) levels.
After 4 weeks of feeding fish with the experimental diets, 10 fish (769 ± 28 g) per tank (2 replicate tanks/diet) were euthanised with a lethal dose of Tricaine (PHARMAQ Ltd., Hampshire, United Kingdom) in 12 L of seawater. Fish standard length and weight were measured. The whole brain was collected in RNA later (Invitrogen, Carlsbad, CA, United States), left overnight at 4°C and stored at −80°C until RNA isolation was performed. The same experimental procedure was repeated on 10 fish (1446 ± 50 g) per tank (2 replicates/diet) at the end of the experiment on week 12. In addition, on week 12, blood was collected by a caudal venous puncture using a heparinized vacuum syringe and BD Vacutainer set (Becton Dickinson, Plymouth, United Kingdom). The blood was thereafter centrifuged at 1000 g for 10 min (4°C) (Hettich Zentrifugen Universal 320R, Tuttlingen, Germany) and stored at −80°C until further analysis. Three samples per tank were pooled and analysed for free amino acids on Biochrom 20 plus amino acid bioanalyzer (Amersham Pharmacia Biotech, Sweden) using post-column derivatisation with ninhydrin. Plasma amino acid composition is shown in Table 3.
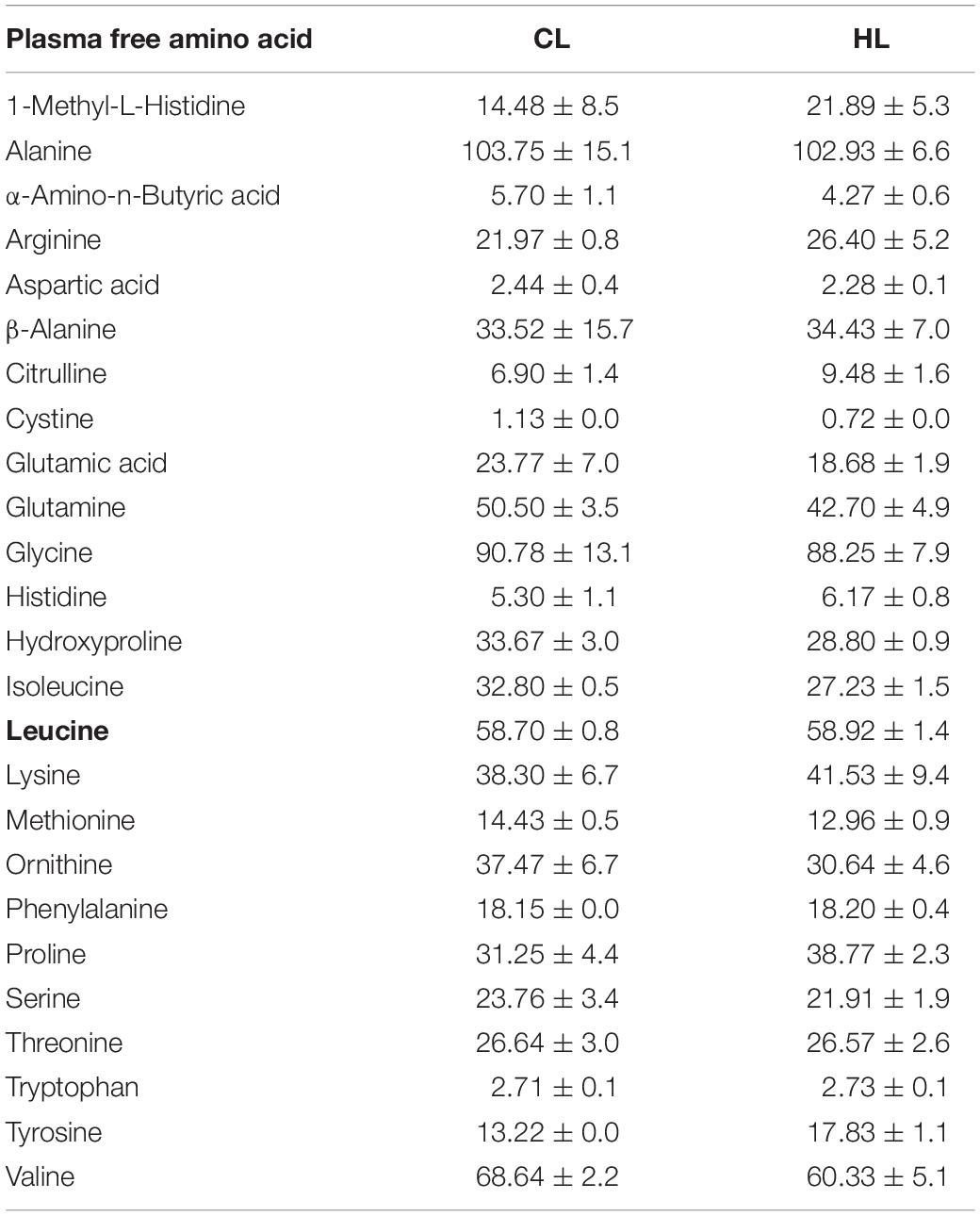
Table 3. Plasma free amino acid (μmol/100 mL) in fish fed diets with control (standard) leucine (CL; 27.3 g/kg) or high leucine (HL; 35 g/kg) levels.
Brain Dissection
The brain of 12 fish per diet and sampling time (n = 6 fish per tank/replicate) were randomly selected and dissected into five sections: telencephalon, midbrain, cerebellum, brain stem, and diencephalon. Diencephalic area included hypothalamus, part of the preoptic region and the thalamus, however, from this point we will name this section as hypothalamus as our region of interest in the control of feed intake. Six brains from fish fed with CL diet (n = 3 brains per tank/replicate) were used for analysis of the mRNA distribution level of the transporters in the five brain sections. Hypothalamus and telencephalon were used for the differential expression responsiveness to the dietary treatments after 4 and 12 weeks.
Analysis of mRNA Abundance by RT-qPCR
Total RNA of the five brain sections was extracted using TRI Reagent (Sigma-Aldrich, MO, United States) and subsequently treated with TURBO DNA-free kit (Ambion, Applied Biosystems, CA, United States) to remove any remnants of genomic DNA. RNA integrity number (RIN) of 45% of the samples was assessed by Agilent 2100 Bioanalyzer with RNA 6000 Nano Kit (Agilent Technologies, CA, United States), obtaining values higher than 8. Total RNA (3.6 μg in two batches of 1.8 μg) were reversed transcribed using SuperScript III Reverse Transcriptase (Invitrogen, CA, United States) and Oligo(dT)20 (50 μM) primers in a total reaction volume of 20 μL. The protocols were carried out accordingly to the manufacturer’s instructions.
We assessed the mRNA abundance of six paralogues of slc7a5 named as slc7a5aa, slc7a5ab, slc7a5ba, slc7a5bb, slc7a5ca, and slc7a5cb (one pair of primers amplify for paralogues ca and cb); two paralogues of slc38a2 named as slc38a2a and slc38a2b (the primers for this paralogue amplify two splicing variants); and slc38a9. For these transcripts, new primers were designed using NCBI primer designing tool from sequences available in GenBank database (Table 4). All primers were analysed for quantification cycle (Cq) and melting peak. Then, the qPCR products were run in a 2% agarose gel, purified using EZNA Gel Extraction kit (Omega Bio-tek, Norcross, GA, United States), and cloned into pCR4-TOPO vector (Thermo fisher Scientific, Waltham, MA, United States). Sequencing was performed at the University of Bergen Sequencing Facility (Bergen, Norway), and their identity was confirmed using blast analysis against the Atlantic salmon genome database in GenBank. Primers for a seventh paralogue of slc7a5 were designed though expression was only detected at very low level in gonads and not in other tissues like brain.
Gene expression levels were determined by RT-qPCR using the CFX96 Real Time System (Bio-Rad Laboratories, CA, United States) in connection to CFX Manager Software version 3.1 (Bio-Rad, Laboratories, CA, United States). Analyses were performed on 2 μL cDNA (40 ng/reaction) using iTaq Universal SYBR Green Supermix (Bio-Rad, CA, United States), in a total PCR reaction volume of 20 μL, containing 0.6 μL of each primer (10 μM). Thermal cycling was initiated with incubation at 95°C for 30 s followed by 39 cycles, each one consisting of heating at 95°C for 5 s and annealing and extension at 60°C for 25 s. Following the final PCR cycle, melting curves were systematically performed and monitored (temperature gradient at 0.5°C for 2 s from 65 to 95°C) to be able to detect non-specific products and/or primer dimers. Standard curves generated from the target gene were cloned into pCR4-TOPO vector (Thermo Fisher Scientific) using a 10-fold dilution series and used to determine the qPCR efficiency for each assay (Table 2). No template control and no reverse transcriptase were run in each plate as negative controls. Relative expression of the target transcripts was calculated using qbase + software (Biogazelle, Zwijnaarde, Belgium) with actb (β-actin) as reference gene (stably expressed in this experiment).
Comparative Sequence Analysis and Evolutionary Relationships
Comparative sequence analysis was performed as previously outlined (Rønnestad et al., 2010; Verri and Werner, 2019; Gomes et al., 2020). Briefly, the paralogues of LAT1-, SNAT2-, and SNAT9-type proteins in Atlantic salmon, other salmoniforms (Salmo trutta, O. mykiss, Oncorhynchus kisutch, Oncorhynchus tshawytscha, Salvelinus alpinus, Salvelinus fontinalis, Salvelinus namaycush, Coregonus lavaretus, Coregonus sp. “balchen,” Thymallus thymallus, Hucho taimen) and an esociform (Esox lucius) were identified by BLAST against several gen(om)es using the National Center for Biotechnology Information (NCBI) databases (for details see Supplementary Table 1). Closer and more distant paralogues were identified and were included in the list of selected sequences. Only full-length sequences with high BLAST scores were considered (IDs reported in Figures 1–3).
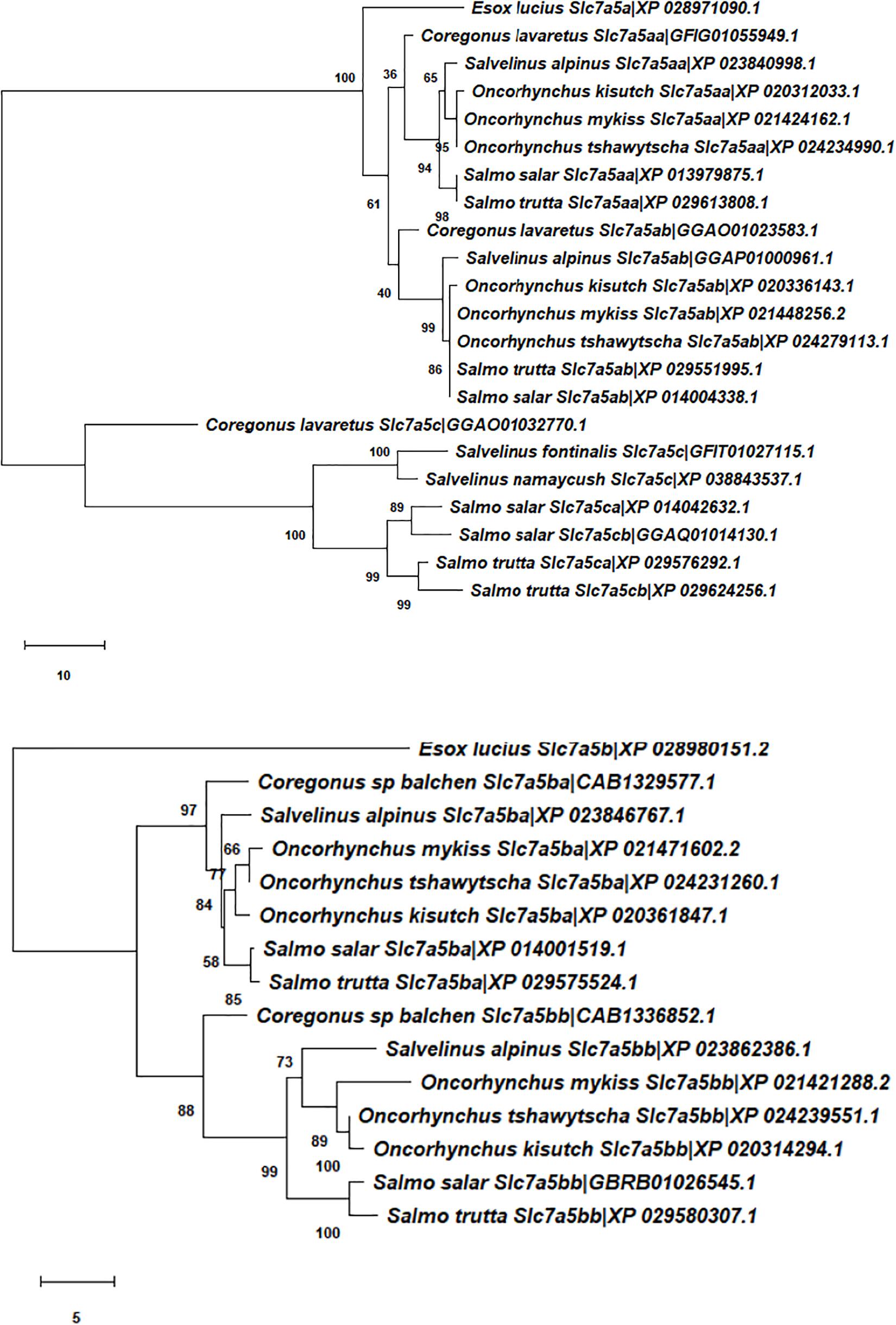
Figure 1. Unrooted phylogenetic trees depicting the evolutionary relationships of Atlantic salmon Slc7a5-type transporters in the context of the evolutionary relationships of Salmoniformes and Esociformes. Multiple protein sequence alignments were obtained by Clustal Omega (https://www.ebi.ac.uk/Tools/msa/clustalo/) using default parameters. The unrooted trees were constructed using the neighbour-joining method (Saitou and Nei, 1987) based on the alignment of the complete amino acid sequences of transporters. Bootstrap values (1000 replicates) indicating the occurrence of nodes are reported above each branch in the figure. The evolutionary distances were computed using the number of differences method (Nei and Kumar, 2000) and are in the units of the number of amino acid differences per sequence. Classical protein GenBank identification (ID) numbers are indicated for the canonically annotated amino acid sequences, while Transcriptome Shotgun Assembly (TSA) IDs are given for those amino acid sequences derived from (a) TSA project(s) by transcript-to-protein sequence translation (via ORFfinder; https://www.ncbi.nlm.nih.gov/orffinder/).
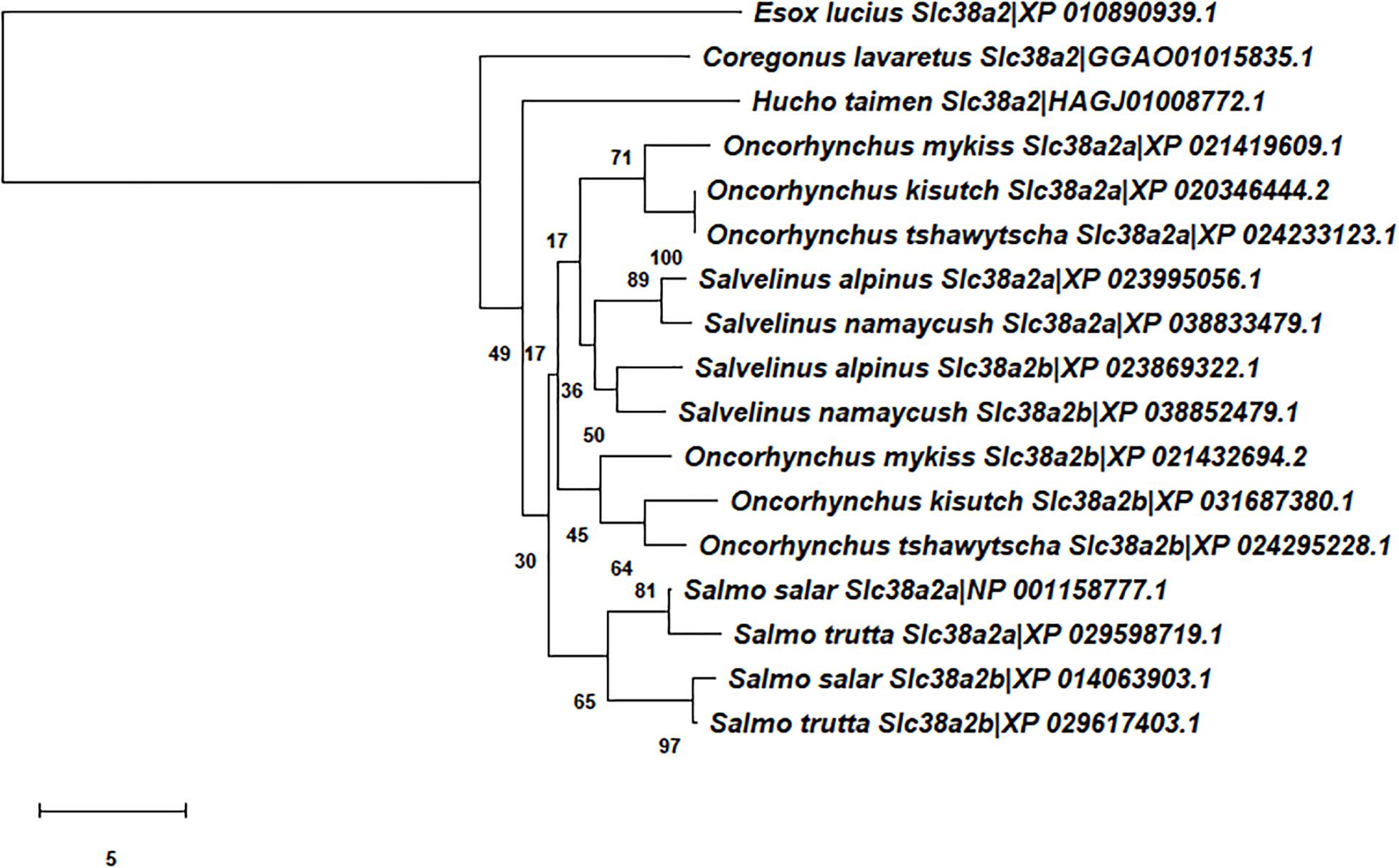
Figure 2. Unrooted phylogenetic tree depicting the evolutionary relationships of Atlantic salmon Slc38a2-type transporters in the context of the evolutionary relationships of Salmoniformes and Esociformes. See legend of Figure 1 for further details.
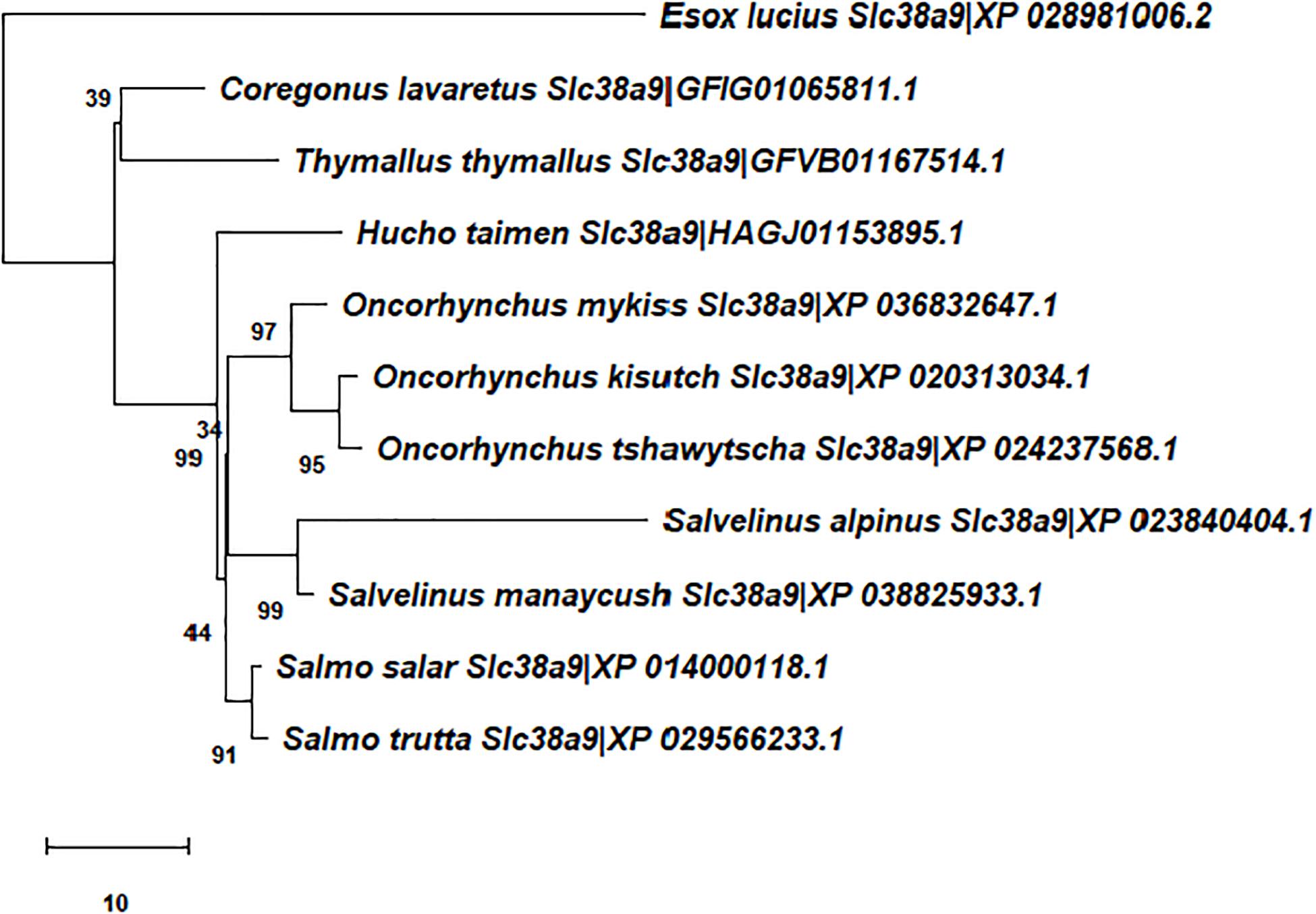
Figure 3. Unrooted phylogenetic tree depicting the evolutionary relationships of Atlantic salmon Slc38a9-type transporters in the context of the evolutionary relationships of Salmoniformes and Esociformes. See legend of Figure 1 for further details.
Multiple sequence alignments were performed on the selected paralogue proteins using Clustal Omega and neighbour-joining trees built using Molecular Evolutionary Genetics Analysis X (MEGAX) (Kumar et al., 2018).
Statistics
Statistical comparisons were done using SigmaStat (Systat Software, San José, CA, United States) statistical package. A two-tailed t-test was used to compare feed intake measurement and plasma AA levels from CL and HL groups. One-way ANOVA followed by Student-Newman-Keuls post-test was used for the comparison among the brain sections. In the diets experiment, comparisons were carried out using two-way ANOVA with time (4 and 12 weeks) and diets (CL and HL) as main factors. P-values of two-way ANOVA are displayed in Table 5. When a significant effect was noticed, post hoc comparisons were carried out by Bonferroni test. Data were transformed to logarithmic scale to fulfil conditions of normality and homoscedasticity (confirmed by Shapiro-Wilk and Levene’s test, respectively) but are shown in their decimal values for simplicity. Differences were considered statistically significant at P < 0.05.
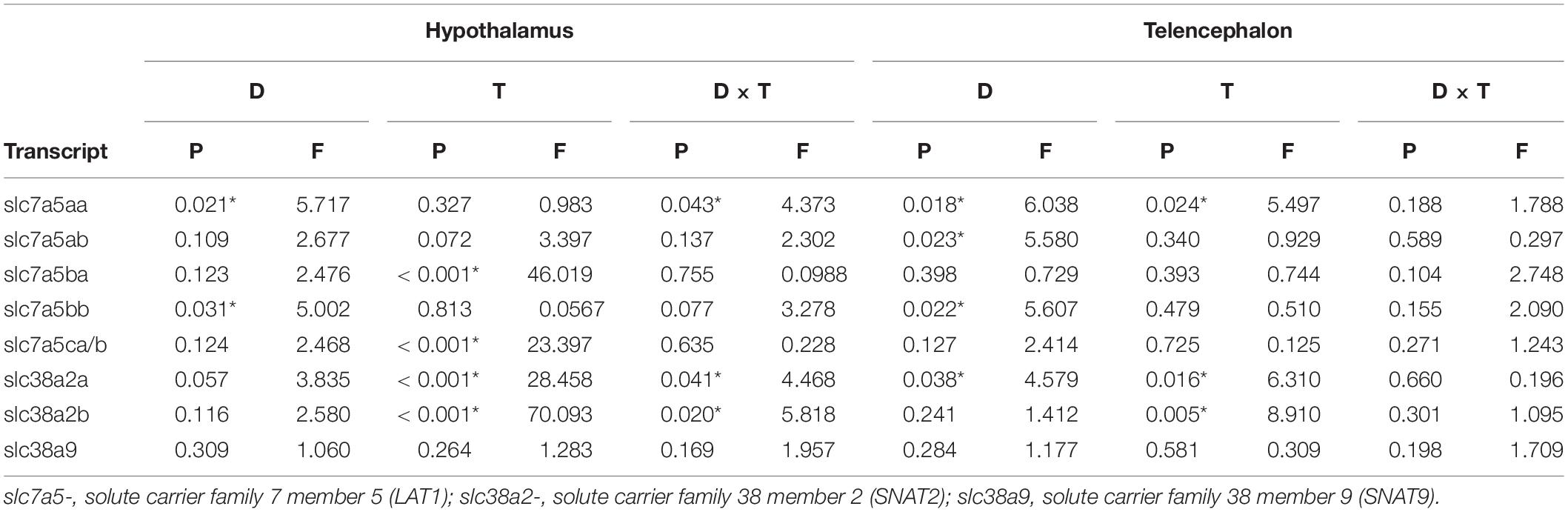
Table 5. P and F-values obtained after two-way analysis of variance of parameters assessed in hypothalamus and telencephalon of rainbow trout. Diet (D) and Time (T) were the main factors, and Diet × Time (D × T) is the first order interaction.
Results
Comparative Sequence Analysis and Evolutionary Relationships
Atlantic salmon Slc7a5-, Slc38a2-, and Slc38a9-type amino acid sequences were compared by multiple sequence alignment to their closest ortologues/paralogues from other salmoniformes, such as brown trout (S. trutta), rainbow trout (O. mykiss), coho salmon (O. kisutch), Chinook salmon (O. tshawytscha), Arctic char (S. alpinus), brook trout (S. fontinalis), lake trout (S. namaycush), common whitefish (C. lavaretus), Coregonus sp. “balchen,” grayling (T. thymallus), taimen (H. taimen), and one esociform, Northern pike (E. lucius). An optimal tree from the multiple alignment of the predicted amino acid sequences was generated for each Slc7a5- (Figure 1), Slc38a2- (Figure 2), and Slc38a9-type (Figure 3) amino acid sequence group.
For the Slc7a5-type amino acid sequences (Figure 1), three distinct clusters of salmonid sequences emerged in the tree. The first made of the Slc7a5aa and Slc7a5ab branches, the second of the Slc7a5ba and Slc7a5bb branches, and the third of the Slc7a5ca and Slc7a5cb branch of amino acid sequences. The Slc7a5aa and Slc7a5ab amino acid sequences, which shared high similarity, were present in all salmonid species. Analogously, the Slc7a5ba and Slc7a5bb amino acid sequences, which also shared high similarity, were present in all salmonid species. On the contrary, the Slc7a5ca and Slc7a5cb sequences associated to the genus Salmo only, which results in the presence of a duplicated gene in the Atlantic salmon and brown trout genomes, of a single gene in the genus Coregonus and Salvelinus, and in the absence of the gene in the genus Oncorhynchus. For the Slc38a2-type amino acid sequences (Figure 2), a single cluster of salmonid sequences emerged, made of the Slc38a2a and Slc38a2b only, whereas all the Slc38a9-type amino acid sequences (Figure 3) easily referred to a single gene. Notably, divergence of the Slc38a2-type sequences in its two Slc38a2a and Slc38a2b forms seems to have emerged as a block of two genes in the Salvelinus, Oncorhynchus and Salmo branches.
mRNA Abundance in Different Brain Areas
We assessed the expression of the different transporters in the brainstem, midbrain, cerebellum, telencephalon and hypothalamus as displayed in Figure 4.
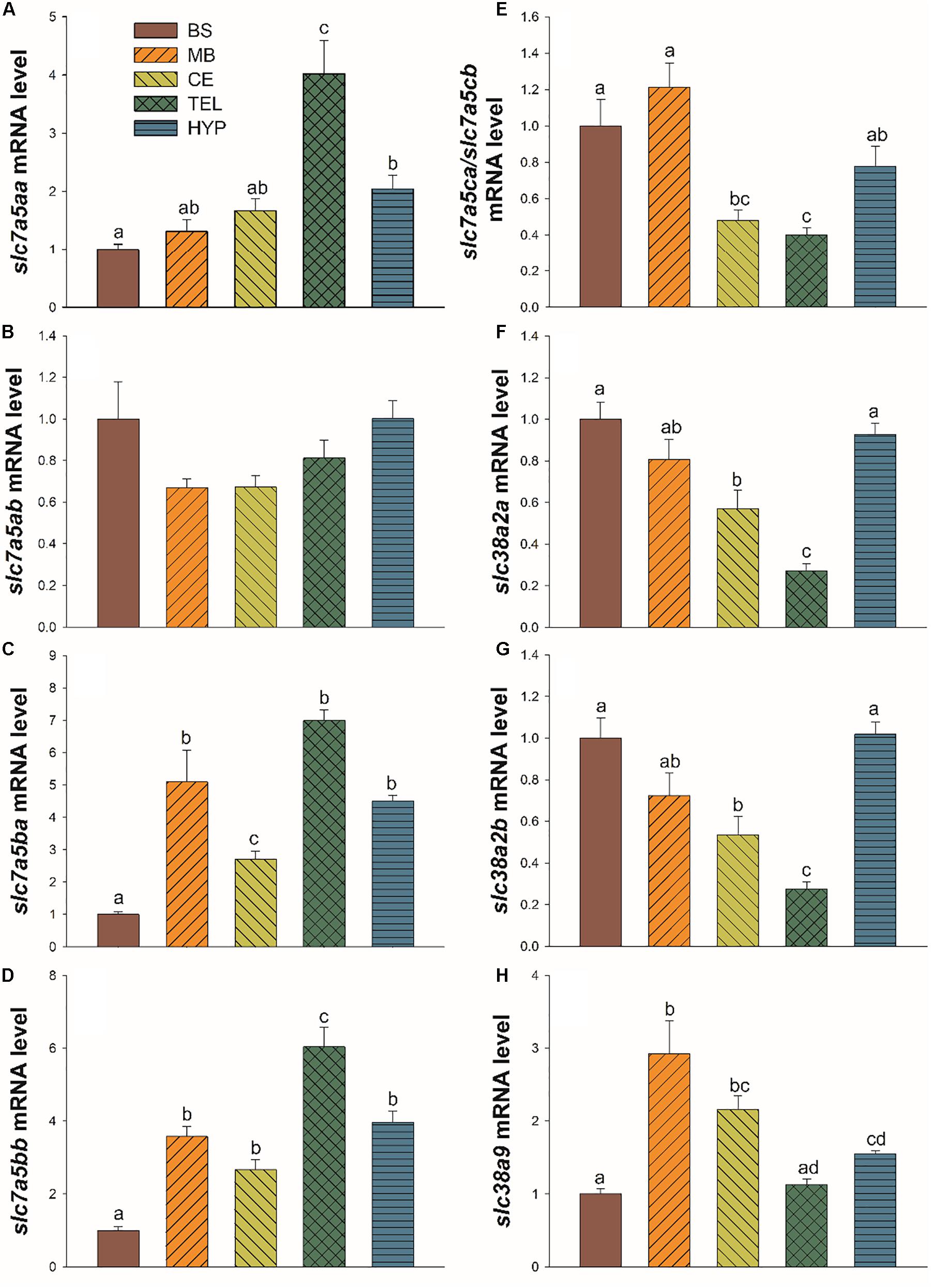
Figure 4. Brain distribution of mRNA level of slc7a5aa (A), slc7a5ab (B), slc7a5ba (C), slc7a5bb (D), slc7a5ca/slc7a5cb (E), slc38a2a (F), slc38a2b (G), and slc38a9 (H) in brainstem (BM), midbrain (MB), cerebellum (CE), telencephalon (TEL), and hypothalamus (HYP) of Atlantic salmon after 4 weeks of feeding with control leucine diet (CL; 27.3 g/kg of leucine). Each value is the mean ± SEM of n = 6 samples per tissue. Gene expression results are relative to brainstem (BM) and are normalised by actb (β-actin). Different letters indicate significant differences (P < 0.05) among different tissues.
The slc7a5 paralogues (Figures 4A–E) showed a differential expression among the brain sections analysed (p < 0,001, F = 12,472; p = 0.094, F = 2.243; p < 0.001, F = 21.708; p < 0.001, F = 30.682; p < 0.001, F = 15.809, for slc7a5aa, slc7a5ab, slc7a5ba, slc7a5bb, and slc7a5ca/slc7a5ab, respectively). Telencephalon is the area with the highest mRNA level in the case of slc7a5aa, slc7a5ba, and slc7a5bb (Figures 4A,C,D). In the case of hypothalamus, its mRNA level is in general in a medium range. In brainstem, expression was the lowest for slc7a5aa, slc7a5ba, and slc7a5bb. Midbrain and cerebellum mRNA abundance displayed average values. When comparing mRNA abundance within tissues (comparing Cts), values in hypothalamus and telencephalon of the paralogues assessed were higher for slc7a5ab and lower for slc7a5c while the other three paralogues displayed intermediate values (data not shown).
For slc38a2 (Figures 4F,G), both paralogues displayed the same distribution in the different brain areas (p < 0.001, F = 15.481; p < 0.001, F = 14.406, for slc38a2a and slc38a2b, respectively). The highest level of expression occurred in hypothalamus and brainstem, followed by midbrain, while expression was lowest in cerebellum and telencephalon. No significant differences occurred when comparing values of both paralogues in hypothalamus and telencephalon (data not shown).
In the case of slc38a9 (Figure 4H), mRNA level was the highest in midbrain followed by cerebellum, hypothalamus, telencephalon, and brainstem (p < 0.001, F = 12.240).
Effect of Feeding Fish With Diets Containing Different Leucine Levels
Feed intake significantly decreased in fish fed with HL diet (Figure 5). A decrease of 19.8% was observed from weeks 4–12. Compared to the CL diet, fish under HL diet showed a tendency for a decreased feed intake already at week 4, albeit not statistically significant. On week 12, HL fed fish showed a significant reduction (−17.8%) in feed intake compared with fish under CL diet (p = 0.0382, t = 4.969) (Figure 5).
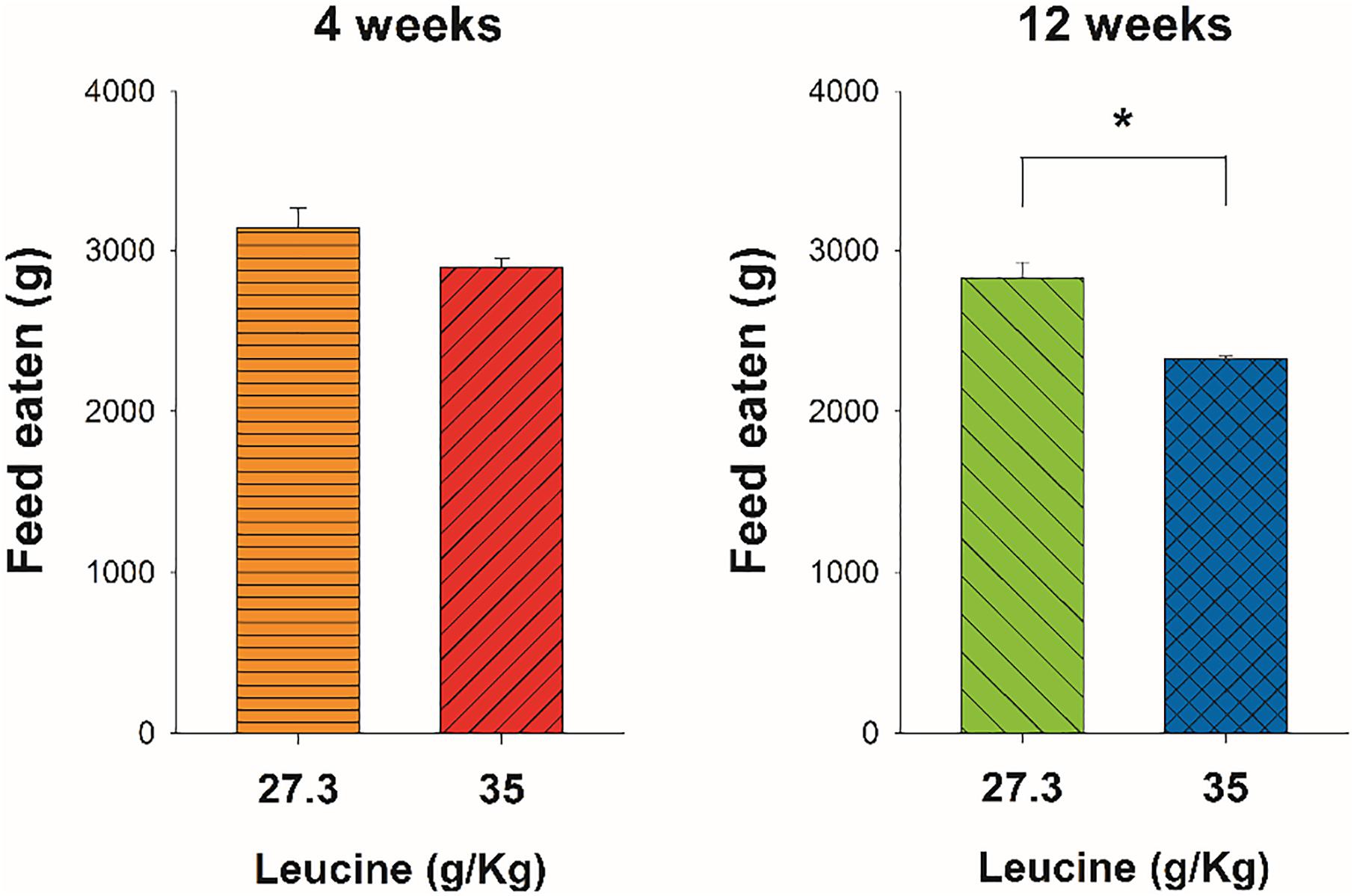
Figure 5. Food intake of Atlantic salmon after four (4w) or twelve (12w) weeks of feeding with control leucine diet (CL; 27.3 g/kg) or high leucine diet (HL; 35 g/kg). Results are shown as the mean + S.E.M. of the data obtained during the 7 days before the sampling points at week 4 and week 12. *Significantly different (p < 0.05) between diets.
In the hypothalamus, the expression of the two paralogues of slc7a5 (slc7a5aa and slc7a5bb; Figures 6A,D) and slc38a2 (slc38a2a and slc38a2b; Figures 6F,G, respectively) significantly increased in fish fed with HL for 12 weeks (and not four) compared with fish fed with the CL diet (p = 0.003, t = 3.343; p = 0.021, t = 2.503; p = 0.044, t = 2.819; p = 0.014, t = 2.694, for slc7a5aa, slc7a5bb slc38a2a, and slc38a2b, respectively). Moreover, independently of the diet, the expression of slc7a5ba, slc7a5ca/b, slc38a2a, and slc38a2b (Figures 6C,E–G, respectively) in the hypothalamus was lower at week 12 than the expression of the same paralogues observed at week 4 (CL diet: p < 0.001, t = 5.097; p = 0.003, t = 3.758; p < 0.001, t = 4.454; p < 0.001, t = 6.713 for slc7a5ba, slc7a5ca/b, slc38a2a, and slc38a2b, respectively; HL diet: p < 0.001, t = 4.507; p = 0.021, t = 3.082; p = 0.008, t = 2.935; p < 0.001, t = 5.002 for slc7a5ba, slc7a5ca/b, slc38a2a, and slc38a2b, respectively). No changes occurred for slc7a5ab (Figure 6B).
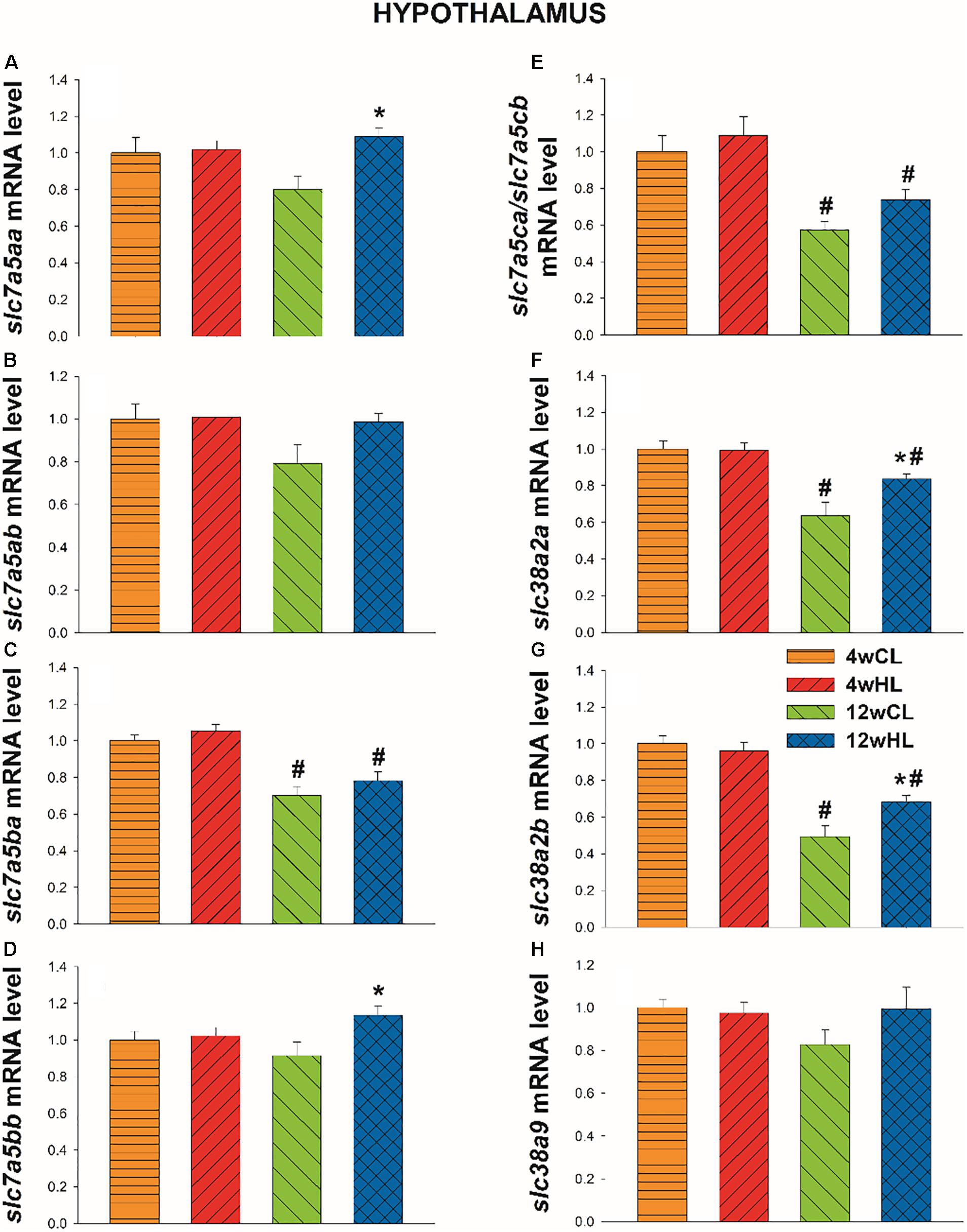
Figure 6. Hypothalamus mRNA level of slc7a5aa (A), slc7a5ab (B), slc7a5ba (C), slc7a5bb (D), slc7a5ca/slc7a5cb (E), slc38a2a (F), slc38a2b (G), and slc38a9 (H) of Atlantic salmon after four (4w) or twelve (12w) weeks of feeding with control leucine diet (CL; 27.3 g/kg) or high leucine diet (HL; 35 g/kg). Each value is the mean ± SEM of n = 12 samples per tissue. Gene expression results are relative to fish fed CL for 4w and are normalised by actb (β-actin). *, significantly different (P < 0.05) from fish fed with LL within the same time. #, significantly different (P < 0.05) from fish fed for 4 weeks with the same diet.
In telencephalon, 4 weeks of feeding fish with HL diet increased mRNA abundance of slc7a5aa, slc7a5ab, and slc7a5bb (Figures 7A,B,D) in comparison with fish fed with CL diet (p = 0.014, t = 2.670; p = 0.023, t = 2.438; p = 0.007, t = 2.970, for slc7a5aa, slc7a5ab, and slc7a5bb, respectively). This effect did not occur after 12 weeks of feeding. Moreover, mRNA level observed after 12 weeks was lower than that observed after 4 weeks when comparing fish fed with HL diet in slc7a5aa and slc38a2a (Figures 7A,F) and when comparing CL diet in slc38a2b (Figure 7G) (p = 0.012, t = 2.754; p = 0.037, t = 2.221; p = 0.015, t = 2.640, for slc7a5aa, slc38a2a, and slc38a2b, respectively). No changes occurred for slc7a5ba (Figure 7C) and slc7a5ca (Figure 7E).
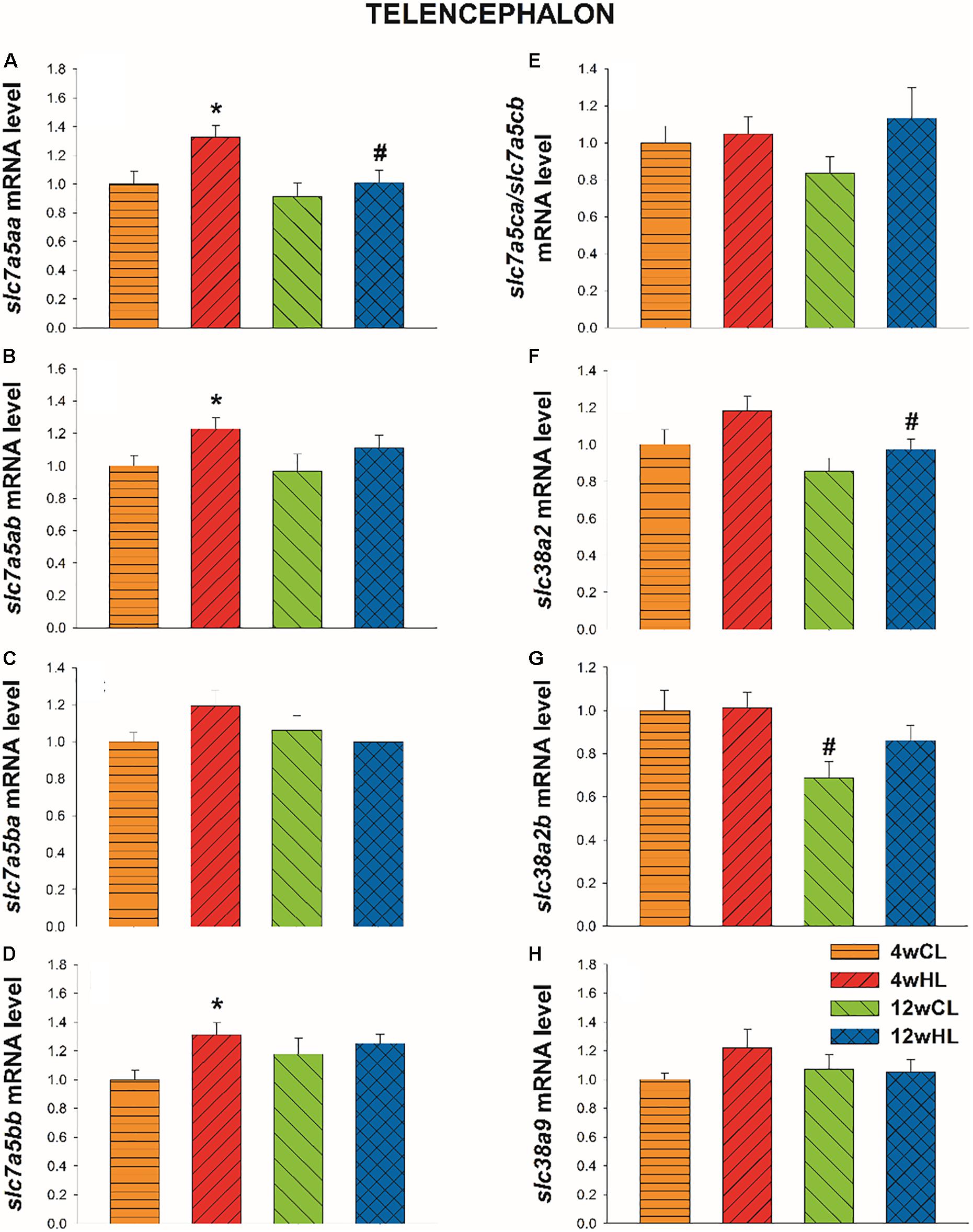
Figure 7. Telencephalon mRNA level of slc7a5aa (A), slc7a5ab (B), slc7a5ba (C), slc7a5bb (D), slc7a5ca/slc7a5cb (E), slc38a2a (F), slc38a2b (G), and slc38a9 (H) of Atlantic salmon after four (4w) or 12 weeks (12w) of feeding with control leucine diet (CL; 27.3 g/kg) or high leucine diet (HL; 35 g/kg). Each value is the mean ± SEM of n = 12 samples per tissue. Gene expression results are relative to fish fed CL for 4w and are normalised by actb (β-actin). *, significantly different (P < 0.05) from fish fed with LL within the same time. #, significantly different (P < 0.05) from fish fed for 4 weeks with the same diet.
Discussion
Phylogenetic Analysis of Carriers
Teleost fish are the largest and most diverse group of vertebrates (Betancur et al., 2017). This diversity is in part due to (a) specific gene duplication event(s) that occurred during the evolution of the actinopterygian lineage (Ravi and Venkatesh, 2018). In teleost genomes, the duplicated gene pairs provide a unique framework to study the diversification of gene functions and their impact on physiology and adaptation (Kassahn et al., 2009; Sato et al., 2009). Amongst teleost fish, salmonids are particularly interesting because of an additional gene duplication event that specifically occurred in a common ancestor of this group of teleosts (Pasquier et al., 2016).
The consequences of these gene duplication events are noticeable in the gene families here studied, with both slc7a5- and slc38a2-types widely appearing in duplicated forms. On the contrary, slc38a9 does not seem to be present in a duplicated form in the salmonid genomes.
The time frame involved enables tracking the consequences of the duplication events, and traces of the specific gene duplication of salmonids are clearly seen in the diversity of the Slc7a5-type transporters. In fact, the four teleost genes described above as slc7a5aa and slc7a5ab, on one hand, and slc7a5ba and slc7a5bb, on the other, are the result of two rounds of duplication that may have started to occur early during the phylogenetic evolution of salmonids. In fact, each salmonid species exhibits one Slc7a5aa, Slc7a5ab, Slc7a5ba, and Slc7a5bb member in each Slc7a5ab, Slc7a5ab, Slc7a5ba, and Slc7a5ebb branch. Conversely, the genome of the esociform species here described, i.e., the Northern pike E. lucius, which is from a phylogenetic point of view closely related to salmonids, displays two canonical forms only, one that roots to the salmonid Slc7a5aa and Slc7a5ab branches and the other to the Slc7a5ba and Slc7a5bb. Notably, another duplication event has lead, in an ancestor of salmoniformes to another slc7a5-type gene, here named slc7a5c, which underwent somehow another duplication event in the Atlantic salmon and of the closely related brown trout, which results in a slc7a5ca and a slc7a5cb gene couple. Notably, the genus Oncorhynchus does not seem to present such a gene (couple) in the genome. Moreover, the presence of Slc38a2-type (Slc38a2a and Slc38a2b) amino acid sequence clusters is clear in salmonids. These clusters are salmonid-specific, as it results also from the position of the Northern pike Slc38a2 sequence. Notably, in the case of the Slc38a2a and Slc38a2b it appears that the duplication event associates to the Salveninus, Oncorhynchus and Salmo lineages, which may be suggestive of a strict co-evolution process involving the slc38a2a and slc38a2b genes. The indication of a Slc38a2-type protein in the genus Coregonus and Hucho helped to balance the tree, and at the moment deeper studies are needed to fully assess whether or not Slc38a2 is duplicated also in these more basal groups of salmonids. In this context of complexity, the occurrence in salmonids of one slc38a9 gene only appears almost an exception and is very possibly due to gene loss in a multiply duplicated genome.
Taken together, lineage-specific sets of slc7a5, slc38a2, and slc38a9 genes have evolved in teleost fish through genome duplication events and gene loss. Studies need to address the phylogenetic relationships in the context of cotransporter-specific functions and expression patterns, as the repertoire of Slc7a5-, Slc28a2-, and Slc38a9-type proteins in teleost fish (and in particular in salmonids) is often too diverse to generalise the findings from one (group of) species.
Brain Distribution of Carriers
To our knowledge, expression analyses of amino acid carriers involved in leucine transport studied here have not been described in Atlantic salmon before. Previous studies focused on the presence of the slc7a5 carrier in the whole brain in grass carp Ctenopharingodon idellus (Yang et al., 2014) and the slc38a2 in two brain regions, hypothalamus and telencephalon, in rainbow trout (Comesaña et al., 2018a,b). Prior studies in fish described the presence of mRNA abundance of slc7a5 and slc38a2 in peripheral tissues such as turbot (Scophthalmus maximus) intestine (Xu et al., 2016), slc38a2 in rainbow trout liver (Skiba-Cassy et al., 2016), slc7a5 in rainbow trout intestine (To et al., 2019), slc7a5 in Japanese sea bass (Lateolabrax japonicus) intestine (Zhang et al., 2018), and slc7a5 and slc38a2 in grass carp intestine (Wang et al., 2018; Yuan et al., 2020).
In the current study, we first evaluated the distribution of the different paralogues of slc7a5, slc38a2, and slc38a9 in different brain areas, such as the brainstem, the midbrain, the cerebellum, the telencephalon, and the hypothalamus. The results showed that, in general, these genes are widely expressed in the brain, with differences among areas. We hypothesised that regions involved in amino acid-related control of feed intake such as the hypothalamus and the telencephalon (Soengas et al., 2018) would show the highest expression, providing that the transporters were involved in amino acid sensing. We can distinguish two trends among the transcripts evaluated. In the first trend, the expression of the different paralogues is highest in the telencephalon, followed by a high expression in the hypothalamus and a lower expression in the remaining areas. This trend includes several of the paralogues of slc7a5 (aa, ba, and bb). The other trend characterises by paralogues that have a low expression in the telencephalon but equally distributed in the other brain areas analysed. This response is present in slc38a2, slc38a9, and slc7a5ca/b. This would suggest a differential role of these carriers in feed intake regulation. However, although the expression is remarkable in these areas, regions not involved in this physiological process, such as the cerebellum and the midbrain (Soengas et al., 2018), also showed high levels of expression. These results demonstrate that these transporters have several roles depending on the brain area assessed, simply working like transporters to supply the cell with leucine or involved in nutrient sensing or other cellular pathways. The differences in distribution between slc38a2 and slc7a5 is noteworthy. Given that SNAT2 works in a coupled way with LAT1 (Dodd and Tee, 2012; Menchini and Chaudhry, 2019), we would expect a similar expression level of both in the same tissue.
Effects of Leucine on Carriers
Due to the additional rounds of genome duplication in salmonids (Pasquier et al., 2016), it is possible, as previously mentioned, that different paralogues of a specific transcript serve different physiological roles. Accordingly, in the present study we observed that of the six paralogues found for slc7a5, only forms aa, ab, and bb displayed mRNA expression changes in response to leucine-enriched diets suggesting that these forms would be those involved in leucine sensing. In contrast, no differences occurred between the two paralogues of slc38a2 under the same diet conditions.
12 weeks of diets supplemented with leucine (and not 4 weeks) significantly increased the mRNA abundance of slc7a5 (aa/bb) and slc38a2 (a/b) in the Atlantic salmon hypothalamus. In the case of the telencephalon, after 4 weeks (and not twelve) slc7a5 (aa, ab, and bb) the mRNA abundance also increased in fish fed HL diet in comparison with those fed with CL diet. This led us to hypothesise that LAT1 and SNAT2 transporters might be involved in leucine sensing in the brain of Atlantic salmon. This is the first time in which this kind of amino sensing mechanisms is suggested to exist in the brain of a teleost fish since in available studies in rainbow trout other mechanisms (BCAA metabolism, glutamine metabolism, mTOR, taste receptor signalling, and GCN2 kinase signalling) had been suggested (Soengas et al., 2018). These mechanisms related to carrier function would match with the reduced feed intake observed in fish fed with the HL diet compared with fish fed with the CL diet in the present study as well as with (minor) changes in mRNA of some of the neuropeptides involved in control of food intake assessed in week 12 (not shown). This in agreement with a similar anorectic response observed in another salmonid species (rainbow trout) treated with leucine where the activation of amino acid sensing mechanisms occurred in the hypothalamus and telencephalon (Comesaña et al., 2018a,b). Thus, we are providing evidence for an additional amino acid sensing mechanism involved in the inhibition of feed intake observed in salmonids fed elevated levels of leucine. However, it is interesting to mention that the high levels of leucine in HL diet did not reflect in differences in plasma levels of leucine after 12 weeks. Since clear effects occurred both in feed intake and in carrier mRNA abundance in fish fed HL diet we suggest that a fast uptake and metabolic use of leucine is responsible for the lack of changes in plasma. The increase in slc38a2 values is comparable to that observed in rainbow trout in the hypothalamus and the telencephalon after leucine treatment (Comesaña et al., 2018a,b). This fish response to leucine observed in this and other studies (Comesaña et al., 2018a,b) was different than in mammalian brain where these transporters are up regulated (mRNA abundance) but under conditions of amino acid deprivation (Hyde et al., 2007; Hellsten et al., 2017). However, there is no clear information in mammals regarding the impact of the presence of BCAA on the expression of these carriers in brain, but in other tissues, like skeletal muscle essential amino acid ingestion is known to stimulate SNAT2 and LAT1 mRNA abundance (Drummond et al., 2010; Rundqvist et al., 2017). Another interesting aspect of the amino acid uptake is that LAT1 and SNAT2 are exchanging leucine in a coupled way with glutamine and in previous studies it was observed that glutamine is also involved in amino acid sensing in fish (Comesaña et al., 2018a,b). This reinforces the importance of glutamine as part of amino acid sensing. Interestingly, a decrease (though not significant, p = 0.3267, t = 1.288) occurred in plasma levels of glutamine in fish fed the HL diet. Therefore, the regulation of these transporters is complex and dependent on many factors such as tissue or organism status but seems that different amino acids regulate differentially.
From data of two-way ANOVA we did not observe significant changes in mRNA abundance of scl38a9 in fish fed with HL diet either in hypothalamus or in telencephalon suggesting that SNAT9 is not relevant in terms of leucine sensing in Atlantic salmon brain. However, when the time factor is not considered, a significant increase (one-way ANOVA) occurred in fish fed HL when compared with fish fed CL after 4 weeks in telencephalon and after 12 weeks in hypothalamus. These trends are the same as those observed for LAT1 and SNAT2 in this study reinforcing the relationship among them. In any case, we cannot exclude the possibility of a different interpretation as suggested by two-way ANOVA. In that case, one reason for the lack of changes might relate to its location in the lysosome membrane (Menchini and Chaudhry, 2019) while LAT1 and SNAT2 appear to facilitate transport for extracellular space into the cell. Another explanation may relate to its putative role as an arginine sensor, since deprivation of this amino acid changed mRNA abundance of Slc38a9 in mammals (Wyant et al., 2017). Again, the absence of studies dealing with increased amount of specific amino acid do not allow proper comparisons among fish and mammalian models.
We chose the transporters assessed (LAT1, SNAT2, and SNAT9) since they relate to leucine detection through mTOR signalling (Wyant et al., 2017). However, we cannot discard that other transporters could be also involved in feed intake regulation in brain since in mammals both SLC36 and SLC1 have been implicated in mTOR signalling as well (Dodd and Tee, 2012; Taylor, 2014).
A higher concentration of leucine in the diet upregulated mRNA abundance of slc7a5 and slc38a2 in the hypothalamus and the telencephalon after 12 and 4 weeks, respectively, and probably something similar occurred for slc38a9. This difference in the time needed to exert this effect between both areas highlights the different role of these brain areas in the regulation of feed intake (Soengas et al., 2018). Hedonic regulation of feed intake in fish apparently takes place in the telencephalon (Soengas et al., 2018). In this sense, although no studies have directly addressed taste preference of leucine in Atlantic salmon, it has been suggested to putatively act together with some other free amino acids to stimulate feed intake (Kousoulaki et al., 2018) and it is known to serve as an attractant in other salmonids (Kasumyan, 2016; Comesaña et al., 2018b). Therefore, the effects observed in the telencephalon may link leucine sensing to hedonic regulation of feed intake. On the other hand, homeostatic regulation, that takes place in the hypothalamus, works at short and long-term in order to maintain energy homoeostasis (Soengas et al., 2018). This kind of long-term homeostatic regulation usually relates to an adiposity signal through leptin in mammals. However, it is not completely defined how this hormone works in fish (Rønnestad et al., 2017; Blanco and Soengas, 2021), but clearly it cannot be considered as a lipostat (Michel et al., 2016). Considering the carnivore nature of many fish species, including salmonids, the possibility of leucine acting as a protein availability signal in fish is not unlikely (Soengas et al., 2018). The upregulation of LAT1 and SNAT2 by leucine might act as a signal of amino acid sensing regulating energy homoeostasis and feed intake directly. However, we cannot discard that these transporters might act just as a carrier to rise intracellular levels of leucine. Once leucine levels rise, other mechanisms that detect amino acids would sense them. These will likely include mTOR, known to be activated in fish brain (Comesaña et al., 2018a,b) whose integration would result in modulation of feed intake (Delgado et al., 2017). Since we assessed mRNA abundance and not protein levels caution must be taken when interpreting results regarding regulatory functions of the carriers.
Conclusion
The amino acid transporters LAT1, SNAT2, and SNAT9 are present in brain areas of Atlantic salmon. Elevated leucine level in the diet, resulted in decreased feed intake, and this occurred in parallel in the hypothalamus and the telencephalon (brain areas involved in the regulation of feed intake) with the up regulation of mRNA abundance of specific paralogues of LAT1 (slc7a5aa, slc7a5ab, and slc7a5bb) and SNAT2 (slc38a2a and slc38a2b) though apparently not for SNAT9. This upregulation might act as a signal of leucine presence thus providing support for the existence in central brain areas involved in feed intake regulation of an additional amino acid sensing mechanism not characterised yet in fish. However, we cannot exclude that these transporters act as a carrier to rise intracellular levels of leucine to be sense by other mechanisms of amino acid sensing. Accordingly, further studies are needed to establish the relation of these carriers with other mechanisms of amino sensing like mTOR, and thus shed light on the relation with feed intake. The difference in time required to observe effects of leucine between the telencephalon and the hypothalamus might relate to the different feed intake regulation primarily occurring in both brain areas (hedonic in the telencephalon and homeostatic in the hypothalamus). Finally, since we evaluated leucine concentrations in the range of commercial diets, the present results remark the importance of choosing the best and the most suitable diet for fish. Thus, different commercial diets, with an apparently smaller difference in the concentration of a specific key amino acid like leucine, may cause significant differences in regulation of signalling pathways resulting in substantial differences in feed intake.
Data Availability Statement
The raw data supporting the conclusions of this article will be made available by the authors, without undue reservation.
Ethics Statement
The animal study was reviewed and approved by National Animal Research Authority in Norway (reference FOTS ID 20799).
Author Contributions
SC, FL, JS, and IR conceived and designed the research. SC, FL, A-EO, and ME performed the experiments. SC, FL, and TV analysed data and prepared the figures. FL, JS, and IR supervised the project. All authors interpreted results of the experiments, edited and revised the manuscript, with JS and IR had the main contribution, and approved the final version of manuscript.
Funding
This study was supported by the Research Council of Norway (RCN) (LeuSENSE, No. 267626 and Gut2Brain 2020, No. 311627) to IR, and the Spanish Agencia Estatal de Investigación and European Fund of Regional Development (PID2019-103969RB-C31) and Xunta de Galicia (Consolidación e estructuración de unidades de investigación competitivas do SUG, ED431B 2019/37) to JS. SC was recipient of a predoctoral fellowship (Programme FPU) from Spanish Ministerio de Educación, Cultura y Deporte (FPU16/00045) and a mobility fellowship for predoctoral researchers of University of Vigo. TV received support from the University of Salento (Fondi ex-60%) and from the Apulian Region (Project 78M4CM5 and Project 0001/ASA/19/PU).
Conflict of Interest
The authors declare that the research was conducted in the absence of any commercial or financial relationships that could be construed as a potential conflict of interest.
Publisher’s Note
All claims expressed in this article are solely those of the authors and do not necessarily represent those of their affiliated organizations, or those of the publisher, the editors and the reviewers. Any product that may be evaluated in this article, or claim that may be made by its manufacturer, is not guaranteed or endorsed by the publisher.
Acknowledgments
We thank Cargill Innovation Center and its entire staff for fish rearing, assistance with sampling, and water chemistry. In particular, we are grateful for the technical assistance provided by Mali Bjerkhaug Hartviksen and Christian De Santis during the planning of the experiment at the facility. We are also grateful for assistance during sampling by Ana da Silva Gomes, Ingvill Tolås, Virginie Gelebart, Tharmini Kalananthan, Daniel Flatejord, and Magnus Mangersnes.
Supplementary Material
The Supplementary Material for this article can be found online at: https://www.frontiersin.org/articles/10.3389/fmars.2021.711508/full#supplementary-material
References
Betancur, R. R., Wiley, E. O., Arratia, G., Acero, A., Bailly, N., Miya, M., et al. (2017). Phylogenetic classification of bony fishes. BMC Evol. Biol. 17:162. doi: 10.1186/s12862-017-0958-3
Blanco, A. M., and Soengas, J. L. (2021). Leptin signalling in teleost fish with emphasis in food intake regulation. Mol. Cell. Endocrinol. 526:111209. doi: 10.1016/j.mce.2021.111209
Comesaña, S., Velasco, C., Ceinos, R. M., López Patiño, M. A., Míguez, J. M., Morais, S., et al. (2018a). Evidence for the presence in rainbow trout brain of amino acid-sensing systems involved in the control of food intake. Am. J. Physiol. Regul. Integr. Comp. Physiol. 314, R201–R215. doi: 10.1152/ajpregu.00283.2017
Comesaña, S., Velasco, C., Conde-Sieira, M., Míguez, J. M., Soengas, J. L., and Morais, S. (2018b). Feeding stimulation ability and central effects of intraperitoneal treatment of L-leucine, L-valine, and L-proline on amino acid sensing systems in rainbow trout: implication in food intake control. Front. Physiol. 9:1209. doi: 10.3389/fphys.2018.01209
Conde-Sieira, M., and Soengas, J. L. (2017). Nutrient sensing systems in fish: impact on food intake regulation and energy homeostasis. Front. Neurosci. 10:603. doi: 10.3389/fnins.2016.00603
Delgado, M. J., Cerdá-Reverter, J. M., and Soengas, J. L. (2017). Hypothalamic integration of metabolic, endocrine, and circadian signals in fish: involvement in the control of food intake. Front. Neurosci. 11:354. doi: 10.3389/fnins.2017.00354
Dodd, K. M., and Tee, A. R. (2012). Leucine and mTORC1: a complex relationship. Am. J. Physiol. Endocrinol. Metab. 302, E1329–E1342. doi: 10.1152/ajpendo.00525.2011
Drummond, M. J., Glynn, E. L., Fry, C. S., Timmerman, K. L., Volpi, E., and Rasmussen, B. B. (2010). An increase in essential amino acid availability upregulates amino acid transporter expression in human skeletal muscle. Am. J. Physiol. Endocrinol. Metab. 298, E1011–E1018.
Efeyan, A., Comb, W. C., and Sabatini, D. M. (2015). Nutrient-sensing mechanisms and pathways. Nature 517, 302–310. doi: 10.1038/nature14190
Fromentin, G., Darcel, N., Chaumontet, C., Marsset-Baglieri, A., Nadkarni, N., and Tomé, D. (2012). Peripheral and central mechanisms involved in the control of food intake by dietary amino acids and proteins. Nutr. Res. Rev. 25, 29–39. doi: 10.1017/S0954422411000175
Gomes, A. S., Vacca, F., Cinquetti, R., Murashita, K., Barca, A., Bossi, E., et al. (2020). Identification and characterization of the Atlantic salmon peptide transporter 1a. Am. J. Physiol. Cell. Physiol. 318, C191–C204. doi: 10.1152/ajpcell.00360.2019
Hansen, T., Stefansson, S., and Taranger, G. L. (1992). Growth and sexual maturation in Atlantic salmon, Salmo salar L., reared in sea cages at two different light regimes. Aquaculture Res. 23, 275–280. doi: 10.1111/j.1365-2109.1992.tb00770.x
Heeley, N., and Blouet, C. (2016). Central amino acid sensing in the control of feeding behavior. Front. Endocrinol. 7:148. doi: 10.3389/fendo.2016.00148
Hellsten, S. V., Lekholm, E., Ahmad, T., and Frediksson, R. (2017). The gene expression of numerous SLC transporters is altered in the immortalized hypothalamic cell line N25/2 following amino acid starvation. FEBS Open Biol. 7, 249–264. doi: 10.1002/2211-5463.12181
Hundal, H. S., and Taylor, P. M. (2009). Amino acid transceptors: gate keepers of nutrient exchange and regulators of nutrient signaling. Am. J. Physiol. Endocrinol. Metab. 296, E603–E613. doi: 10.1152/ajpendo.91002.2008
Hyde, R., Cwiklinski, E. L., MacAulay, K., Taylor, P. M., and Hundal, H. S. (2007). Distinct sensor pathways in the hierarchical control of SNAT2, a putative amino acid transceptor, by amino acid availability. J. Biol. Chem. 282, 19788–19798. doi: 10.1074/jbc.M611520200
Kassahn, K. S., Dang, V. T., Wilkins, S. J., Perkins, A. C., and Ragan, M. A. (2009). Evolution of gene function and regulatory control after whole-genome duplication: comparative analyses in vertebrates. Genome Res. 19, 1404–1418. doi: 10.1101/gr.086827.108
Kasumyan, A. O. (2016). Taste attractiveness of free amino acids and their physicochemical and biological properties (as exemplified by fishes). J. Evol. Biochem. Physiol. 52, 271–281. doi: 10.1134/S0022093016040013
Kousoulaki, K., Rønnestad, I., Rathore, R., Sixten, H. J., Campbell, P., Nordrum, S., et al. (2018). Physiological responses of Atlantic salmon (Salmo salar L.) fed very low (3%) fishmeal diets supplemented with feeding-modulating crystalline amino acid mixes as identified in krill hydrolysate. Aquaculture 486, 184–196. doi: 10.1016/j.aquaculture.2017.12.011
Kumar, S., Stecher, G., Li, M., Knyaz, C., and Tamura, K. (2018). MEGA X: Molecular evolutionary genetics analysis across computing platforms. Mol. Biol. Evol. 35, 1547–1549. doi: 10.1093/molbev/msy096
Lutter, M., and Nestler, E. J. (2009). Homeostatic and hedonic signals interact in the regulation of food Intake. J. Nutr. 139, 629–632. doi: 10.3945/jn.108.097618
Menchini, R. J., and Chaudhry, F. A. (2019). Multifaceted regulation of the system A transporter Slc38a2 suggests nanoscale regulation of amino acid metabolism and cellular signalling. Neuropharmacol. 161:107789. doi: 10.1016/j.neuropharm.2019.107789
Meyer, A., and Van de Peer, Y. (2005). From 2R to 3R: evidence for a fish-specific genome duplication (FSGD). Bioessays 27, 937–945. doi: 10.1002/bies.20293
Michel, M., Page-McCaw, P. S., Chen, W., and Cone, R. D. (2016). Leptin signaling regulates glucose homeostasis, but not adipostasis, in the zebrafish. Proc. Natl. Acad. Sci. USA 113, 3084–3089.
Mueller, T., and Wullimann, M. F. (2009). An evolutionary interpretation of teleostean forebrain anatomy. Brain Behav. Evol. 74, 30–42. doi: 10.1073/pnas.1513212113
Nei, M., and Kumar, S. (2000). Molecular evolution and phylogenetics. New York, NY: Oxford University Press.
Otero-Rodiño, C., Rocha, A., Álvarez-Otero, R., Ceinos, R. M., López-Patiño, M. A., Míguez, J. M., et al. (2018). Glucosensing capacity of rainbow trout telencephalon. J. Neuroendocrinol. 30:e12583. doi: 10.1111/jne.12583
Pasquier, J., Cabau, C., Nguyen, T., Jouanno, E., Severac, D., Braasch, I., et al. (2016). Gene evolution and gene expression after whole genome duplication in fish: the PhyloFish database. BMC Genom. 17:368. doi: 10.1186/s12864-016-2709-z
Ravi, V., and Venkatesh, B. (2018). Rapidly evolving fish genomes and teleost diversity. Curr. Opin. Genet. Dev. 18, 544–550. doi: 10.1016/j.gde.2008.11.001
Rønnestad, I., Gomes, A. S., Murashita, K., Angotzi, R., Jönsson, E., and Volkoff, H. (2017). Appetite-controlling endocrine systems in teleosts. Front. Endocrinol. 8:661. doi: 10.3389/fendo.2017.00073
Rønnestad, I., Murashita, K., Kottra, G., Jordal, A. E., Narawane, S., Jolly, C., et al. (2010). Molecular cloning and functional expression of Atlantic salmon peptide transporter 1 in Xenopus oocytes reveals efficient intestinal uptake of lysine-containing and other bioactive di- and tripeptides in teleost fish. J. Nutr. 140, 893–900. doi: 10.3945/jn.109.118240
Rundqvist, H. C., Esbjörnsson, M., Rooyackers, O., Österlund, T., Moberg, M., Apro, W., et al. (2017). Influence of nutrient ingestion on amino acid transporters and protein synthesis in human skeletal muscle after sprint exercise. J. Appl. Physiol. 123, 1501–1515. doi: 10.1152/japplphysiol.00244.2017
Saitou, N., and Nei, M. (1987). The neighbor-joining method: a new method for reconstructing phylogenetic trees. Mol. Biol. Evol. 4, 406–425. doi: 10.1093/oxfordjournals.molbev.a040454
Sato, Y., Hashiguchi, Y., and Nishida, M. (2009). Temporal pattern of loss/persistence of duplicate genes involved in signal transduction and metabolic pathways after teleost-specific genome duplication. BMC Evol. Biol. 9:127. doi: 10.1186/1471-2148-9-127
Skiba-Cassy, S., Geurden, I., Panserat, S., and Seiliez, I. (2016). Dietary methionine imbalance alters the transcriptional regulation of genes involved in glucose, lipid and amino acid metabolismin the liver of rainbow trout (Oncorhynchus mykiss). Aquaculture 454, 56–65. doi: 10.1016/j.aquaculture.2015.12.015
Soengas, J. L., Cerdá-Reverter, J. M., and Delgado, M. J. (2018). Central regulation of food intake in fish: an evolutionary perspective. J. Mol. Endocrinol. 60, R171–R199. doi: 10.1530/JME-17-0320
Taylor, P. M. (2014). Role of amino acid transporters in amino acid sensing. Am. J. Clin. Nutr. 99, 223S–230S. doi: 10.3945/ajcn.113.070086
To, V. P. T. H., Masagounder, K., and Loewen, M. E. (2019). SLC transporters ASCT2, B0AT1-like, y+LAT1, and LAT4-like associate with methionine electrogenic and radio-isotope flux kinetics in rainbow trout intestine. Physiol. Rep. 7:e14274. doi: 10.14814/phy2.14274
Verri, T., and Werner, A. (2019). Type II Na+-phosphate cotransporters and phosphate balance in teleost fish. Pflugers Arch. 471, 193–212. doi: 10.1007/s00424-018-2239-4
Volkoff, H. (2016). The neuroendocrine regulation of food intake in fish: a review of current knowledge. Front. Neurosci. 10:540. doi: 10.3389/fnins.2016.00540
Wang, K.-Z., Jiang, W.-D., Wu, P., Liu, Y., Jiang, J., Kuang, S.-Y., et al. (2018). Gossypol reduced the intestinal amino acid absorption capacity of young grass carp (Ctenopharyngodon idella). Aquaculture 492, 46–58. doi: 10.1016/j.aquaculture.2018.03.061
Wyant, G. A., Abu-Remaileh, M., Wolfson, R. L., Chen, W. W., Freinkman, E., Danai, L. V., et al. (2017). mTORC1 activator SLC38A9 is required to efflux essential amino acid from lysosomes and use protein as a nutrient. Cell 171, 642–654. doi: 10.1016/j.cell.2017.09.046
Xu, D., He, G., Mai, K., Xu, W., and Song, F. (2016). Postprandial nutrient-sensing and metabolic responses after partial dietary replacement by soyabean meal in turbot (Scophthalmus maximus L.). Brit. J. Nutr. 115, 379–388. doi: 10.1017/S0007114515004535
Yang, J., Tan, Q., Zhu, W., Chen, C., Liang, X., and Pan, L. (2014). Cloning and molecular characterization of cationic amino acid transporter y+LAT1 in grass carp (Ctenopharingodon idellus). Fish Physiol. Biochem. 40, 93–104. doi: 10.1007/s10695-013-9827-1
Yuan, Z.-H., Feng, L., Jiang, W.-D., Wu, P., Liu, Y., Jiang, J., et al. (2020). Choline deficiency decreased the growth performances and damaged the amino acid absorption capacity in juvenile grass carp (Ctenopharyngodon idella). Aquaculture 518:734829. doi: 10.1016/j.aquaculture.2019.734829
Zhang, C., Rahimnejad, S., Wang, Y.-R., Lu, K., Song, K., Wang, L., et al. (2018). Substituting fish meal with soybean meal in diets for Japanese seabass (Lateolabrax japonicus): Effects on growth, digestive enzymes activity, gut histology, and expression of gut inflammatory and transporter genes. Aquaculture 483, 173–182. doi: 10.1016/j.aquaculture.2017.10.029
Keywords: amino acid sensing, leucine, amino acid carriers, Atlantic salmon, hypothalamus, telencephalon
Citation: Comesaña S, Lai F, Olderbakk Jordal A-E, Verri T, Espe M, Soengas JL and Rønnestad I (2021) Amino Acid Carriers of the Solute Carrier Families 7 (SLC7) and 38 (SLC38) Are Involved in Leucine Sensing in the Brain of Atlantic Salmon (Salmo salar). Front. Mar. Sci. 8:711508. doi: 10.3389/fmars.2021.711508
Received: 18 May 2021; Accepted: 16 July 2021;
Published: 02 August 2021.
Edited by:
Enric Gisbert, Institute of Agrifood Research and Technology (IRTA), SpainReviewed by:
Zélia Cristina Pereira Velez, University of Algarve, PortugalMansour Torfi Mozanzadeh, South Iran Aquaculture Research Center, Iran
Copyright © 2021 Comesaña, Lai, Olderbakk Jordal, Verri, Espe, Soengas and Rønnestad. This is an open-access article distributed under the terms of the Creative Commons Attribution License (CC BY). The use, distribution or reproduction in other forums is permitted, provided the original author(s) and the copyright owner(s) are credited and that the original publication in this journal is cited, in accordance with accepted academic practice. No use, distribution or reproduction is permitted which does not comply with these terms.
*Correspondence: José L. Soengas, anNvZW5nYXNAdXZpZ28uZXM=