- 1CSIRO Oceans and Atmosphere, Hobart, TAS, Australia
- 2Centre for Marine Socioecology, University of Tasmania, Hobart, TAS, Australia
- 3CSIRO Data61, Hobart, TAS, Australia
- 4CSIRO Oceans and Atmosphere, Indian Ocean Marine Research Centre, Crawley, WA, Australia
When offshore oil and gas infrastructure is no longer needed, it is either removed, partially removed, left in place, or left in place but repurposed. These processes are collectively referred to as decommissioning. Australian legislation requires oil and gas companies to develop acceptable plans for the safe removal of all offshore infrastructure at the end of a project’s life. Over the next 50 years, the liability for this decommissioning in Australia is expected to exceed US$45 billion. Unlike countries such as Norway, the United Kingdom and the Netherlands, Australian decommissioning activities are in their infancy, with only three cases (to date) in Commonwealth waters where infrastructure has been left in place or partially removed as part of decommissioning. Differences between the Australian marine environment and that of other regions around the world where decommissioning-related research is better progressed include very low sedimentation rates, both tropical and temperate habitats, different species composition, low primary production, and frequent tropical cyclones, as well as unique sociodemographic and cultural characteristics. Accordingly, the outcomes of the decision support tools used in other regions to identify preferred decommissioning options may not be equally applicable in Australia. Here we describe research to support risk and impact assessment for offshore decommissioning in Australia, where full removal of infrastructure is the “base case” regulatory default, but other options including partial removal and/or repurposing might provide similar or better outcomes when environmental, social, economic and seafood safety aspects are considered. Based on our review we propose an integrated framework for research needs to meet legislative requirements for decommissioning and identify research gaps that need to be addressed to inform decision-making for decommissioning in the Australian context.
Introduction
Decommissioning is the process of plugging and abandoning oil and gas wells then removing or repurposing infrastructure at the end of its useful life. Decommissioning, as mandated in a range of national policies, should happen in a safe and environmentally and socially responsible manner. There has been very limited decommissioning in Australian Commonwealth waters (i.e., those that are more than 3 nautical miles offshore) to date, and almost no associated monitoring (Raitt et al., 2019). Australia has in the order of 3,500 km of pipeline, 57 platforms, and 11 floating facilities currently operating in Commonwealth waters (National Offshore Petroleum Safety and Environmental Management Authority [NOPSEMA], 2019a; Advisian, 2020), with additional pipelines and platforms in Western Australian and Victorian state waters. Australia’s National Offshore Petroleum Information Management System lists 1,648 wells drilled offshore since January 2001, with most activity concentrated in Australia’s north-west and the south-east regions (Figure 1). Few of Australia’s oil fields have been depleted to the point where decommissioning is required but more than half of Australia’s offshore petroleum assets are older than 20 years, with some exceeding 50 years, and as a consequence, would be expected to be approaching end of life soon. Estimates of Australia’s decommissioning liability over the next 50 years are between US$30 and 50 billion (National Energy Resources Australia [NERA], 2019; Advisian, 2020; Wood Mackenzie, 2020).
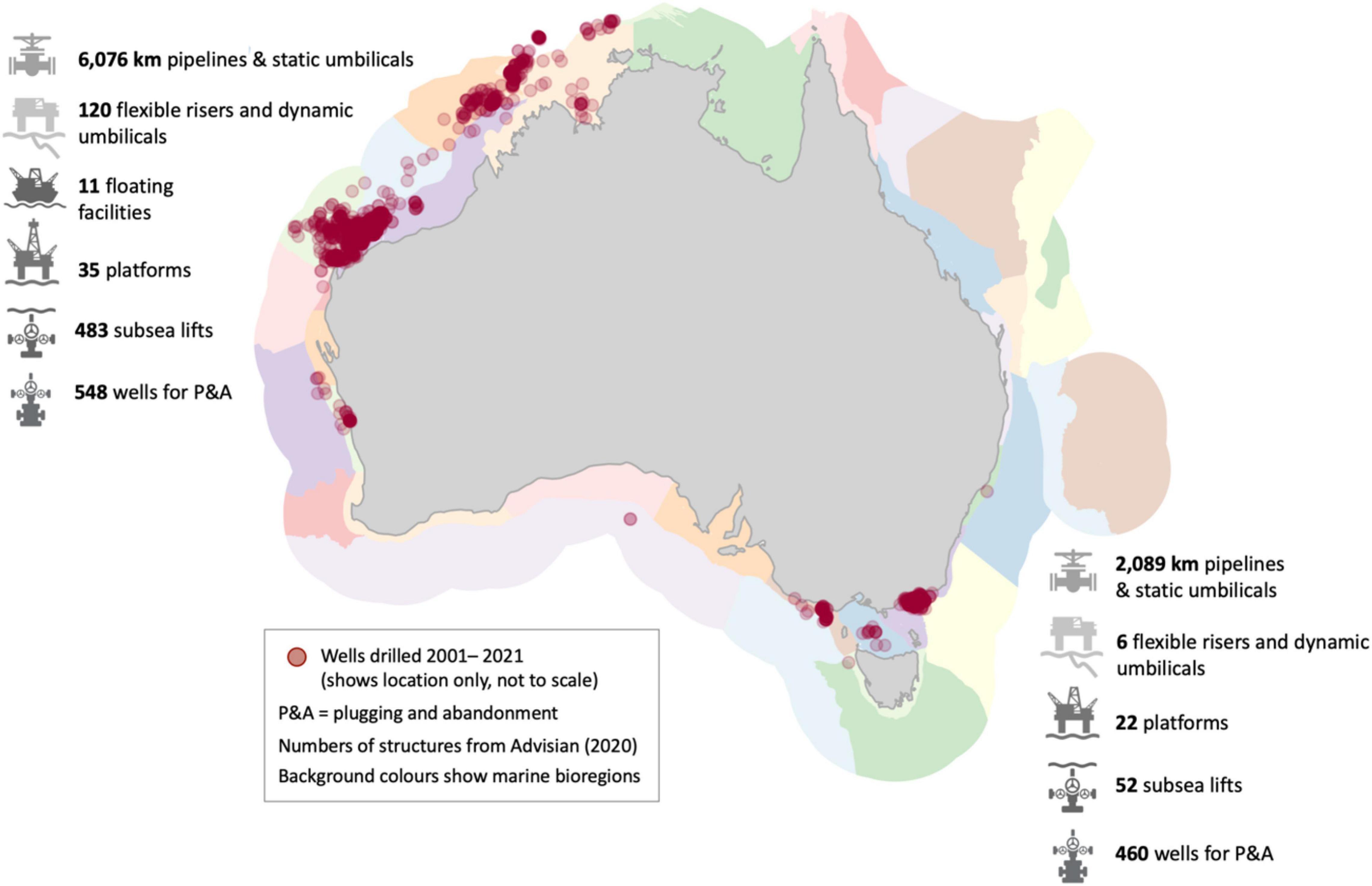
Figure 1. Locations of offshore oil and gas activity in Australia. Red circles show wells drilled by the oil and gas industry in the Australian marine environment between January 2001 and January 2021 (not to scale; data from https://nopims.dmp.wa.gov.au/Nopims/). Numbers of structures are indicative based on publicly available information and are derived from Advisian (2020). The diversity of marine environments in which these operations have occurred is shown by IMCRA v4.0 bioregions (pastel colours; Commonwealth of Australia, 2006). Commonwealth waters are those that are more than three nautical miles offshore.
The benefits and costs (particularly the environmental ones) associated with the operational phase of oil and gas infrastructure have been well established internationally (e.g., Schroeder and Love, 2004; Bernstein, 2015; Claisse et al., 2015; Cordes et al., 2016; Fowler et al., 2018; Bull and Love, 2019; Sommer et al., 2019; Beyer et al., 2020; Meyer-Gutbrod et al., 2020) and in Australia (e.g., Macreadie et al., 2011; McLean et al., 2017, 2018, 2021; Schramm et al., 2021). However, the environmental costs and benefits associated with decommissioning are less well studied, particularly in Australia because there is little precedent for the regulatory requirements for building an environmental case that justifies choosing a leave-in-place decommissioning option here. Moreover, the interdependence of the costs and benefits between the operational and decommissioning phases is relatively understudied. For example, during the operational phase, discharging produced water (PW; see Box 1 for acronyms and definitions of terms used in this paper) containing high concentrations of organic carbon and nutrients such as ammonia affects the productivity of the surrounding ecosystem. It is not certain how comparable the productivity will be once that discharge stops. There are also likely to be social consequences—or costs—to decommissioning decisions (discussed below).
BOX 1. Glossary and acronyms.
BPEO – Best Practicable Environmental Option Analysis; a type of decision support tool used in decommissioning
CA – Comparative Analysis; a type of decision support tool used in decommissioning
Commonwealth Waters – located more than three nautical miles offshore
Drill cuttings – the waste material, containing drilling muds, from drilling wellheads. Drill cuttings may have been in contact with the formation.
Drilling muds – fluids containing lubricants used when drilling wellheads
EP – Environment plans; documents required by NOPSEMA that describe the potential risks of an activity to the environment and how those risks will be mitigated.
Formation – in this case, the geological layer in the subsurface in which the petroleum formed and from which it was extracted
MCA – Multi Criteria Assessment; a type of decision support tool
Mattresses – subsea infrastructure supporting pipelines
NEBA – Net Environmental Benefit Analysis; a type of decision support tool
NOPSEMA – National Offshore Petroleum Safety and Environmental Management Authority; the Commonwealth agency with regulatory authority for the offshore oil and gas industry.
QRA – Quantitative Risk Assessment; a type of decision support tool
PAH – Polycyclic Aromatic Hydrocarbons; components within oil that confer toxicity
PRA – Probabilistic Risk Assessment; a type of decision support tool
PW – Produced water; water from the formation that is brought to the surface during the oil production process
Sediment infauna – animals that live in sediments
Topsides – the upper portion of an oil and gas platform (above the water line).
Generalisations about the ecological value of decommissioned oil and gas infrastructure from other well studied regions may manifest differently in Australian waters because: (1) Australia’s large marine estate contains a large number of different temperate and tropical marine habitats at the continental scale (Figure 1); (2) species assemblages in Australia are distinct from northern hemisphere regions which have been the focus of decommissioning research to date (notably the Gulf of Mexico, California, the North Sea, and the Adriatic Sea; see section “Status of Decommissioning Research Internationally and in Australia”); (3) the low rate of sedimentation in the Australian marine environment (e.g., Blom and Alsop, 1988; Baker et al., 2008), a consequence of comparatively low primary production and low riverine discharge, may affect the persistence of environmental contaminants around offshore infrastructure; and (4) finally, Australia has unique circumstances for social acceptability/social license in the context of decommissioning (see section “Socioeconomic Considerations”).
Here we provide an outline of research areas/needs that will help to ensure decommissioning in Australia builds on extant knowledge from other locations while recognising Australia’s specific and unique characteristics. The aims of this paper are to:
(i) consider research needs for evaluating environmental risk and impacts for decommissioning options in Australia, relative to the unique aspects of its biodiversity, marine environment and sociodemographic and cultural characteristics
(ii) develop a general framework that identifies the information needed to make environmentally sound decisions about decommissioning options (and that considers linkages between these aspects)
The Regulatory Context in Australia
The National Offshore Petroleum Safety and Environmental Management Authority (NOPSEMA) is Australia’s independent regulator for health and safety, structural (well) integrity and environmental management for oil and gas operations and greenhouse gas storage activities in Commonwealth waters, and in coastal waters where regulatory powers and functions have been conferred (National Offshore Petroleum Safety and Environmental Management Authority [NOPSEMA], 2019c). Current decommissioning legislation defines a “base case” expectation that oil and gas producers will remove all infrastructure prior to surrender of the petroleum title. While full removal of infrastructure is the default decommissioning option, other options include: (1) partial removal (this may range from almost complete removal where infrastructure is removed near to the sea-floor, but foundations are left in place, through to minimal removal where topsides are removed and any navigational risks addressed); (2) remain in situ (infrastructure is left in place, or toppled, after some level of removal); or (3) reefing (the process of using decommissioned infrastructure to form an artificial reef in the expectation of some environmental, social or economic value—infrastructure may be positioned in situ or placed elsewhere) (Shaw et al., 2018; see also Table 1). NOPSEMA’s view is that the option selected needs to provide equal or better environmental outcomes than the base case, and demonstrate that environmental risks can be managed to be at an ALARP (As Low As Reasonable Practicable) and acceptable level (see section “Assessing the Impacts and Risk of Different Decommissioning Options”). The mechanism for demonstrating that the environmental risks associated with a preferred decommissioning option are ALARP is via submission of an Environmental Plan. An Environment Plan will be rejected if it does not predict at least equal or better environmental outcomes for decommissioning in situ rather than the default base case. NOPSEMA recently released a new compliance strategy comprising objectives, action and targets to achieve the overarching goal that decommissioning is completed by titleholders in a timely, safe, and environmentally responsible manner (National Offshore Petroleum Safety and Environmental Management Authority [NOPSEMA], 2021). These updates identify triggers for decommissioning which include a pending sale, the time a facility has been in a non-production state, and/or an apparent lack of planning for decommissioning. Notably, current proposed changes to legislation also include so-called “trailing liability,” which would mean that under certain circumstances a company could remain liable to remediate an offshore petroleum asset and the immediate environment surrounding the infrastructure even after the title has been sold (Commonwealth of Australia, 2020).
Australian State- and Territory-level legislation that applies to offshore petroleum facilities in their jurisdictional waters (nearer than three nautical miles to shore) generally mirrors Commonwealth legislation. The Commonwealth Environment Protection and Biodiversity Conservation Act 1999 (EPBC Act) also applies in State or Northern Territory waters to activities that may affect a matter of national environmental significance. In these circumstances, there may be a bilateral agreement in place to allow the State assessment process to be relied upon by the Commonwealth.
Status of Decommissioning Research Internationally and in Australia
There have been a number of studies that show clear benefits of leaving oil and gas infrastructure in situ, and others that demonstrate how these structures pose a risk to the environment. Generally, the factors that influence these costs and benefits are considered to be: (i) the creation of habitat for managed or valued species, as well as the potential risk of oil and gas infrastructure aiding the spread of invasive species; (ii) the potential for impacts of legacy contaminants associated with either drilling or oil and gas production; and (iii) potential socioeconomic concerns relevant to stakeholders needs (including social acceptability of different decommissioning options). These factors are likely to influence each other (Figure 2; Schläppy et al., 2021) and should not be considered in isolation. In the sections below, we describe the lessons learned from the international literature for each of these factors, and also describe the circumstances that are unique to the Australian context.
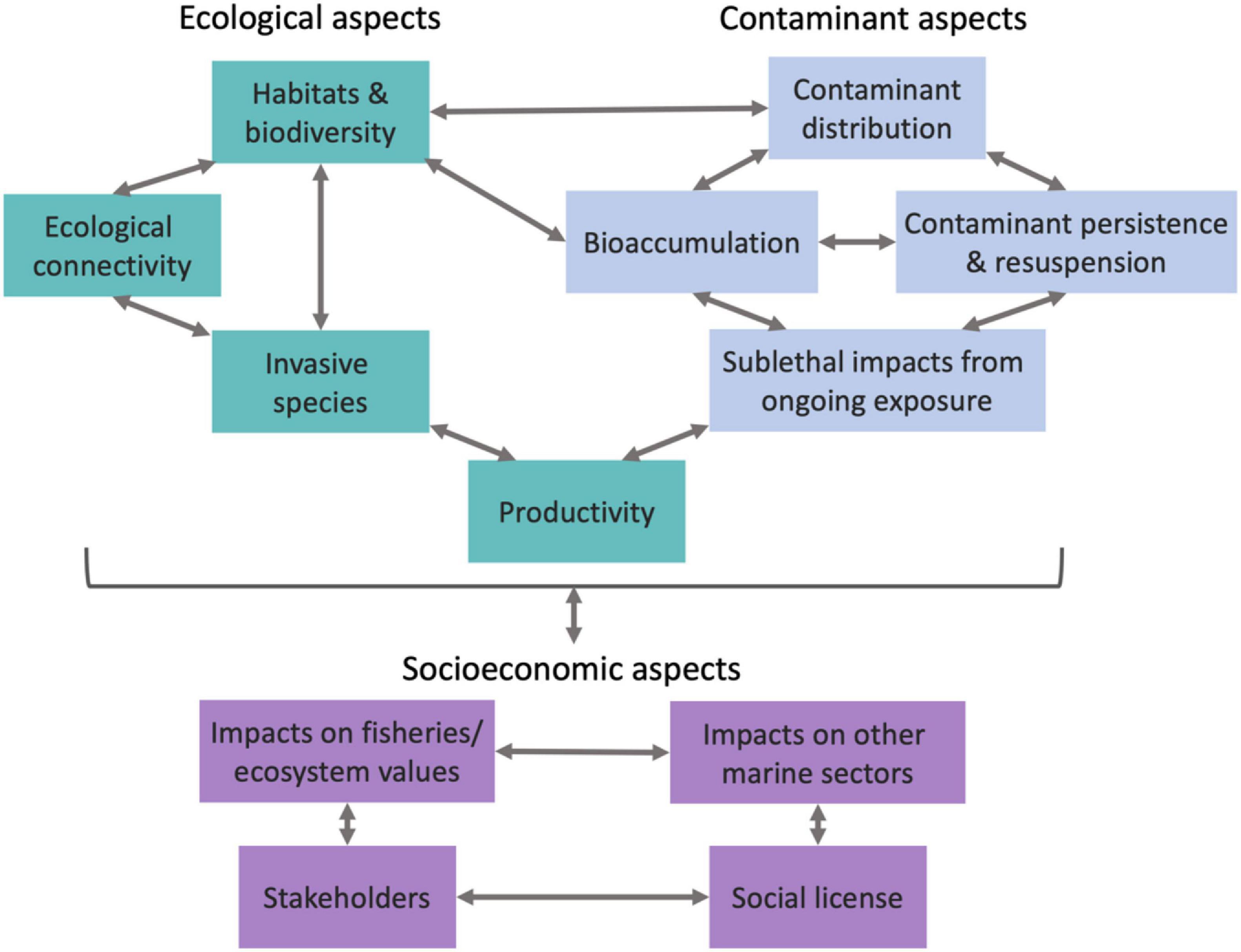
Figure 2. Summary of connections between different research elements needed to consider risk and impacts for decommissioning options. Boxes and arrows are colour coded as: ecological aspects (green), contaminant aspects (blue) and socioeconomic aspects (purple).
Ecological Considerations for Decommissioning
Habitats and Biodiversity
The positive effects of oil and gas structures on biodiversity and/or productivity are frequently cited to support a leave-in-place decommissioning decision. Biodiversity on and around infrastructure may increase due to the presence of hard, complex substrate, as well as enhanced recruitment and/or due to the ongoing attraction of fish and invertebrates (Macreadie et al., 2011; Love et al., 2012; McLean et al., 2017; Bull and Love, 2019; Claisse et al., 2019). Approval to leave-in-place requires operators to provide evidence that the infrastructure is of high conservation value with respect to habitats and biodiversity. Specifically, the biomass, community composition, productivity and health of the organisms inhabiting the oil and gas structures must be quantified to determine the conservation value of the habitat.
It is well documented that oil and gas infrastructure is used as habitat by a variety of marine species (Love et al., 2000, 2012, 2019a; Bull and Love, 2019). For example, oil and gas platforms offshore of Santa Barbara, California, host abundant, and productive fish communities (Love et al., 2012; Claisse et al., 2014; Pondella et al., 2015), with differences in the composition of invertebrate communities relative to nearby reefs (Love et al., 2019b), including a large number of introduced species at the oil platforms (Page et al., 2006). Studies in the Gulf of Mexico also found a higher diversity of target fish species (i.e., Lutjanus campechanus, Red Snapper) and deep sea coral communities associated with platforms than with comparable sites 2–3 km away (Ajemian et al., 2015; Benfield et al., 2019). These structures may also offer feeding benefits for apex predators such as seals (Russell et al., 2014).
Artificial hard structures are known to have different species assemblages than natural reefs (Carvalho et al., 2013). The (comparatively) smooth surfaces of oil and gas infrastructure may alter the species composition. Increased habitat complexity (3-D relief of a surface) generally increases the biodiversity and abundance of marine fouling communities (Strain et al., 2021), with platform complexity linked to enhanced diversity and abundance of fishes (e.g., Meyer-Gutbrod et al., 2019a). The smooth surfaces of oil and gas infrastructure may also alter the species composition of invertebrate communities, especially when they are “new” and the ecological succession process is just commencing (Sommer et al., 2019). Artificial structures are also frequently coated with antifoulants, which can favour the survival of weedy, generalist species (Piola et al., 2009). However, the longer infrastructure is immersed, the more similar species assemblages become with surrounding natural habitat (Sommer et al., 2019).
Communities with different composition than nearby hard substrate may still have conservation value. For instance, in the North Sea it was shown that oil and gas structures increased connectivity for threatened and endangered cold-water corals (Fowler et al., 2014). Similarly, widespread bottom trawling on Australia’s Northwest shelf in the 1970s and 1980s caused a change in the associated fish community, and it is possible that oil and gas infrastructure provided refuge for some species. In Australia, numerous studies have demonstrated that fish utilise oil and gas infrastructure as habitat (Macreadie et al., 2011, 2012; Pradella et al., 2014; McLean et al., 2017, 2018) and often support higher biomass and abundances of large, commercially important species than adjacent sandy habitats (Bond et al., 2020). However, the composition of communities at oil platforms and pipelines has generally not been compared to natural areas in Australia (e.g., Macreadie et al., 2011, 2012; Pradella et al., 2014).
There may be seasonal differences in the habitat value of oil and gas infrastructure. Geographical and seasonal variation in large-scale oceanographic currents and water temperatures present unique considerations when assessing habitat quality of infrastructure. For example, on Australia’s Northwest shelf, where there is considerable co-located infrastructure (Figure 1), environmental conditions are strongly influenced by large-scale oceanographic currents, including the south-westerly-flowing Indonesian Throughflow and Leeuwin currents. These currents transport warm, clear, oligotrophic waters to the outermost Northwest shelf between March and August (Cresswell and Peterson, 1993), resulting in strong seasonal variation in environmental conditions and larval connectivity across the shelf (Young et al., 1986; Beckley et al., 2009). Abundances of fish and invertebrate larvae in the water column have been shown to be highly variable across seasons (Young et al., 1986), being generally highest in August when the Leeuwin current is nearing the end of its seasonal peak (Beckley et al., 2009; Ridgway and Godfrey, 2015). Video-based observations of fish larvae on pipeline infrastructure across the Northwest shelf also supports the notion that larval distribution and connectivity is strongly influenced by large-scale oceanographic currents, with highest abundances and diversity of fish larvae also occurring in August when the Leeuwin current is nearing the end of its seasonal peak (McLean et al., 2017). Variation in large-scale oceanographic currents may therefore be an important consideration in assessing the quality of habitat of co-located infrastructure on Australia’s Northwest shelf. We recommend that in Australia, biological surveys be conducted in multiple seasons to account for these differences.
Productivity
Biological productivity at oil and gas infrastructure may justify leaving (partial) structures in situ. The wide depth range of oil and gas platforms can provide predator refuges at midwater depths, allowing for vertical separation between juvenile and larger fishes (Love et al., 2000; Pondella et al., 2015). Recent literature shows Californian oil platforms have amongst the highest production for fish of any system measured worldwide (Claisse et al., 2014), yet habitat values vary greatly among platforms, even among those located in similar ecological settings (Fowler et al., 2015). Productivity at one platform therefore cannot be used to infer the productivity of other platforms, especially across great distances (Fowler et al., 2015).
High fishery productivity on Californian oil platforms is strongly linked to high biomasses of structural epifauna (“biofouling communities”) and high nutrients. Abundant biofouling communities, including barnacles and blue mussels, are fuelled by the primary production that occurs in the nutrient rich environments of the Southern California coast and also in the North Sea. These abundant biofouling communities can contribute to fish production via three mechanisms. First, increased physical complexity greatly increases surface area of hard substrate available to larval fish (Love et al., 2019a), promoting enhanced larval recruitment (Emery et al., 2006). Second, the larvae produced by fouling communities provides food for juvenile fish that recruit as larvae and utilise the structures as a predator refuge (Bull and Love, 2019). Third, organisms (e.g., blue mussels) grow on platforms until their body weight exceeds their capacity to remain attached, at which point they fall to the base of the platform, creating shell mounds at rates of up to 70 kg of shell per day (Love et al., 1999; see Figure 3). These shell mounds host a rich and diverse community of invertebrates and fishes, which provide food for large, predatory fishes at the base of the platform (Love et al., 1999; Page et al., 2005; Meyer-Gutbrod et al., 2019b). In the Gulf of Mexico, by contrast, where shell mounds are comparatively rare, there is not a comparable invertebrate community and, as a consequence, there are fewer large predatory fish (reviewed in Goddard and Love, 2010). In Australian waters, primary productivity is relatively low, and Australian oil and gas infrastructure is not typically associated with shell mounds (Figure 3). The fish productivity at Australian platforms needs to be determined for productivity to be used as a justification for decommissioning infrastructure in situ.
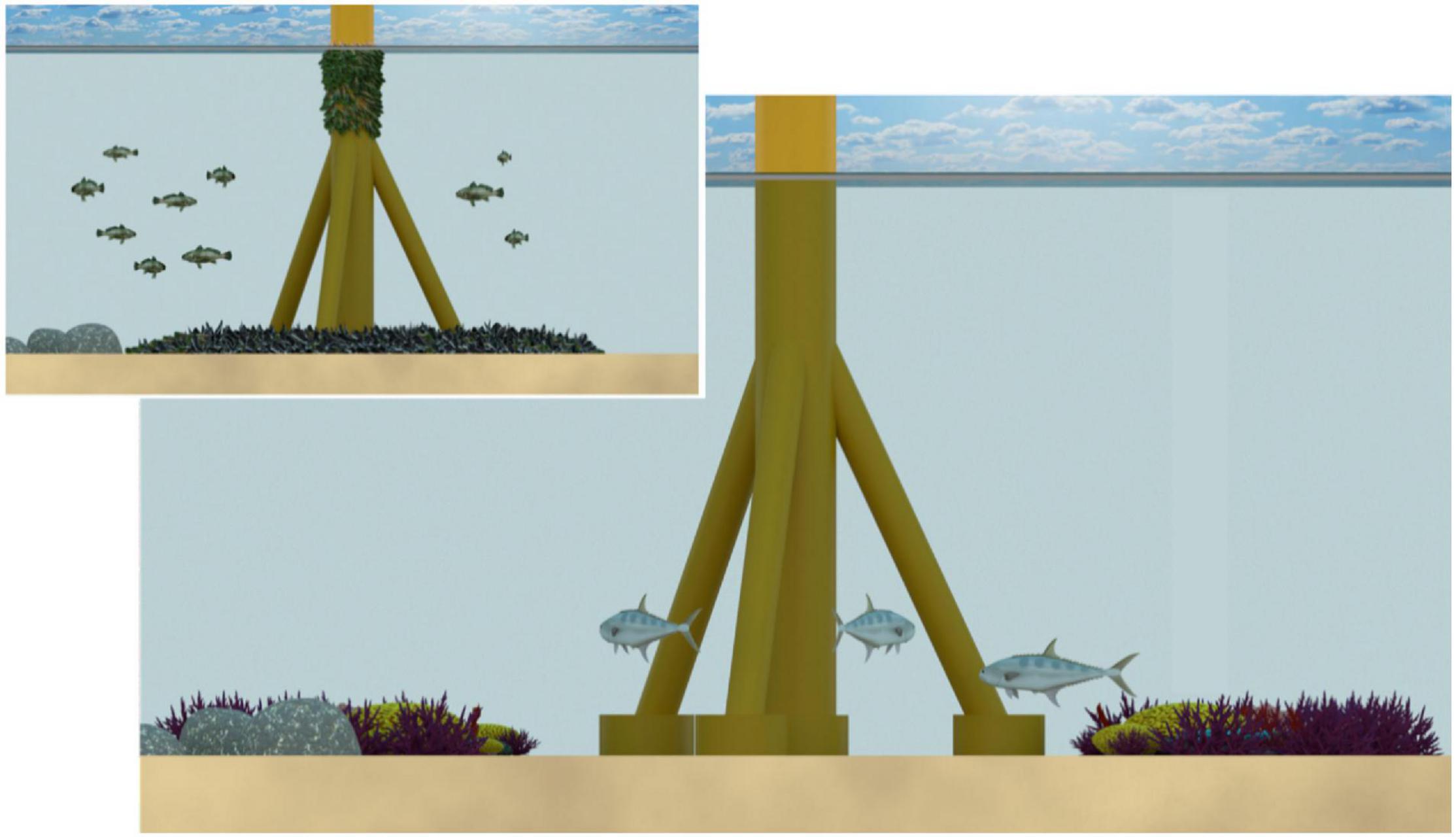
Figure 3. Shell mounds in California (inset) compared with the Australian context (no shell mounds). For structures in Californian environments there is a high level of growth of mussels on infrastructure, which deposits shell mounds to the seafloor (and then provides habitat and a food source for fish and invertebrates). By contrast, Australian oil and gas infrastructure is not typically associated with shell mounds, and as a consequence, may not support similar levels of fish productivity to California.
The options chosen for decommissioning may affect the productivity and conservation value of the system. For instance, the fisheries productivity at a platform may change with depth (Love et al., 2000; Pondella et al., 2015). Partial removal would likely result in the loss of fish biomass and production for species typically occupying the shallow portions of the platform structure. In California, it has been estimated that complete removal of a platform would result in 95% or more reduction in the average fish biomass and production, while losses due to partial removal would average 10% or less (Meyer-Gutbrod et al., 2020). We are unaware of similar studies having been conducted in Australia. If the value of oil and gas infrastructure as habitat is used to justify leaving the platforms partially in place, we recommend determining how the removal of the upper portions of the structure will change the habitat value.
Invasive Species
Subsea infrastructure may act as a conduit to facilitate the spread of invasive marine species by providing suitable habitat in areas where it does not exist naturally. The pipelines that interconnect oil platforms could provide a connecting pathway for dispersal of mobile non-indigenous species. The organisms that encrust oil platforms are generally distinct from those in surrounding communities and the densities of fish and invertebrates on pipelines generally higher than surrounding areas (Love and York, 2005). In Brazil, the servicing of platforms by industry vessels is thought to be a major source of invasive (exotic) species introductions, potentially endangering nearby natural environments (Braga et al., 2021).
In general, a high abundance of exotic species (some of which are cryptic) has been found on platforms off the Santa Barbara coast (Page et al., 2006). The exotic species can reach densities of up to 40% cover and may have very different compositions between different structures. The exotic species are absent or rare on natural reefs in the area. Platforms can enhance connectivity (see also the section “Ecological Connectivity and Ocean Environment”) and so contribute to the spread of invasive species (e.g., Watersipora, an invasive bryozoan; Page, 2019). These differences in species composition are not limited to invertebrates—in the Gulf of Mexico, fish species composition around platforms differs from the surrounding area, and it has been suggested that fish “followed” the platforms as they were towed in from South America (reviewed in Bull and Love, 2019). Although invasive species are comparatively rare in tropical marine waters of the Indo-Pacific (Wells, 2018; Wells et al., 2019) for unknown reasons, the one documented invasive species in Australia’s north-west (the ascidian Didemnum perlucidum) was recorded on artificial structures associated with oil and gas activity (Wells, 2018).
Nuisance species (e.g., those that can grow to unwanted abundance) in the pelagic environment may increase because of oil and gas infrastructure. Oil and gas infrastructure can also contribute to jellyfish and Harmful Algal Blooms (Duarte et al., 2013; Schulze et al., 2020). The surge in numbers of moon jellyfish in Adriatic Sea was positively correlated with propagation of offshore marine constructions including oil and gas platforms (Vodopivec et al., 2017) as artificial substrates are preferred settling surfaces for polyps—the juvenile life stage (Holst and Jarms, 2007). Encrusting communities represent a potential habitat for the dinoflagellate Gambierdiscus toxicus that causes ciguatera fish poisoning. This dinoflagellate was found in all the platforms (n = 6) surveyed in Gulf of Mexico (Villareal et al., 2007). Availability of substrate in combination with climate change that has been linked to expansion in the range of Gambierdiscus spp. (Villareal et al., 2007) and jellyfish (Purcell, 2005) makes the risk likely to increase in future.
We are not aware of studies which quantify the proportion of exotic sessile epifauna on and around Australian oil and gas infrastructure. The relative contribution of exotic species, and the consequences both for indigenous organisms and biological communities, needs to be assessed in determining the value of oil and gas infrastructure as habitat for conservation purposes in Australia. The biofouling communities on oil and gas infrastructure should be characterised to determine whether the species they contain have conservation value, or alternatively, include invasive pests. Depending on local concerns, these surveys could be extended to the pelagic environment.
Ecological Connectivity and Ocean Environment
Most prior ecological studies have examined platforms in isolation (e.g., Love et al., 2005; Benfield et al., 2019) and have not measured the ecological connectivity among structures. Determining this conservation value is especially important as oil platforms, which are grouped around petroleum deposits (e.g., Figures 1, 4), are unlikely to be ecologically isolated. Similar to reefs, oil and gas structures represent interconnected networks of hard structures which larvae can pass between. Connectivity of oil and gas infrastructure may allow for associated fish to “seed” natural areas elsewhere, or for introduced species and other marine pests to proliferate (Nishimoto et al., 2019). Local hydrodynamic conditions may affect the connectivity of infrastructure with nearby reefs or fishing areas, also altering the value of the area as habitat. Decommissioning research should therefore consider how well these structures are connected, and what the implications of removing one or more structures from the network would be. In addition, the value of structures may change over time. As described above (see section “Habitats and Biodiversity”), platforms which have been in place for a long time could have undergone colonisation and succession, leading to the formation of diverse and stable communities. As a consequence, these older platforms may be disproportionally valuable as sources of larvae/juveniles to neighbouring areas, so therefore provide higher preservation value, i.e., “the spillover effect” (e.g., Palumbi, 2003).
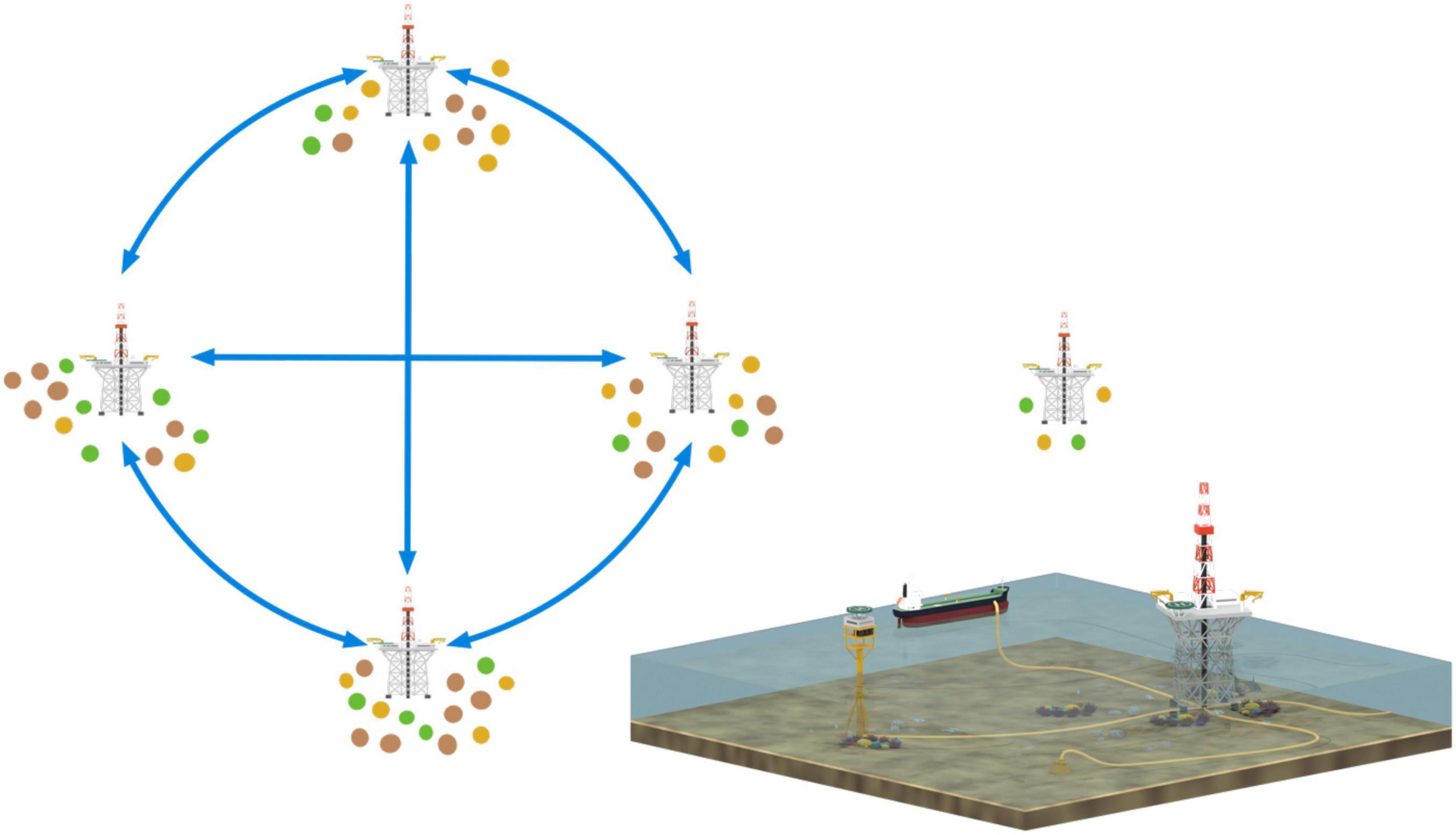
Figure 4. Connectivity of biological particles (e.g., larvae) between oil and gas infrastructure in offshore environments depends on the proximity of structures to each other, pathways for connections (such as pipelines), pelagic larval duration (and behaviour), as well as patterns of oceanographic currents. Inset image shows a visual rendering of different types of co-located structures.
Large-scale oceanographic currents will not only affect larval abundance, composition, and levels of connectivity, but may affect rates of infrastructure decay as well, such that the life span of structures in tropical Australian waters is shorter than in temperate systems, and posing the potential for increased contaminant exposure. Corrosion of steel in the marine environment is influenced by many environmental factors, including water velocity and temperature, with accelerated rates of corrosion associated with warmer, faster moving water (Melchers, 2006). As a result, the warm water transported onto Australia’s Northwest shelf by the Leeuwin current may accelerate rates of corrosion and decomposition of infrastructure. At the same time, cyclones are common on the Northwest shelf (1–2 cyclones per year: Drost et al., 2017), producing significant wave heights of greater than 5 m (Cuttler et al., 2018) and maximum wave heights in excess of 17 m (CSIRO-BHP unpublished wave buoy data during cyclone Olwyn, March 12, 2015). Rates of infrastructure decay are therefore unlikely to be linear and may be strongly influenced by the prevailing environmental conditions within the region.
Climate change is modifying the distribution of marine species (e.g., Pecl et al., 2017; Melbourne-Thomas et al., 2021) and decommissioning decisions need to consider the effect of climate change on biodiversity, biomass and recruitment so that changes in the ecosystem due to climate change (such as poleward migration of a species) can be differentiated from those changes brought about by the presence of oil and gas infrastructure. Warming-induced changes in spawning phenology that impacted larval transport and consequently ranges shifts of marine invertebrates were observed in Northwest Atlantic continental shelf areas (Fuchs et al., 2020).
Future decommissioning research in Australia assessing ecological aspects will need to holistically consider habitat value, ecological connectivity of the system, the importance of invasive species, productivity, and the changing environment. Hence, research needs to include modelling based on scenarios and projections for changing conditions into the future.
Contaminant Aspects: Distribution of Contaminants and Decommissioning
There is the potential for contaminants associated with oil and gas infrastructure to influence the value of infrastructure as habitat either by reducing the productivity of fish communities, altering the species composition found at the sites or by reducing the perceived or actual safety of the area for collecting fish or other types of seafood for human consumption. Removing oil and gas infrastructure may resuspend buried contaminants, making them bioavailable to filter feeding organisms and the fish that prey on them. Ongoing exposure to petroleum hydrocarbons and other toxic compounds may alter the health (e.g., diminish reproductive capacity) of fishes that inhabit the area (Codi King et al., 2011; Box 2). It is also possible that the contaminants that were once associated with drill cuttings and PW discharge have either been degraded or dispersed by sediment movement over time. The sources of these contaminants, their fate and transport in the marine environment, and examples from the international literature of how they can be transformed are highlighted in the sections below.
BOX 2. Contaminants from oil and gas infrastructure.
The contaminants that have the highest concern for entering the seafood supply are methylmecury and naturally occurring radiological material (NORM).
Methylmercury bioaccumulation
Methylmercury is a potent developmental neurotoxin and has been associated with cardiovascular disease in adults (Mason et al., 2012; Beckers and Rinklebe, 2017). Seafood consumption, particularly of long lived high trophic level fish or demersal species with strong site-attachment, is the primary route of exposure to methylmercury (Mason et al., 2012). PW can be high in mercury (Neff et al., 2011). As mercury has a high affinity for particles, discharged mercury would be expected to precipitate out of the water column once discharged (Mason et al., 2012). Mercury is methylated by bacteria in low oxygen environments, including marine sediments (Kim et al., 2013). Once formed, methylmercury readily biomagnifies in aquatic food chains, such that its concentrations in upper trophic levels are approximately 10,000 fold higher than in primary producers (Beckers and Rinklebe, 2017). The geochemical processes governing the formation of methylmercury are complex, but the rates of formation are comparatively high in low oxygen environments with abundant sulphides and labile organic material (Bravo et al., 2017), such as would occur in drill cuttings and areas. Indeed, oil contaminated ground water, sediments collected near oil seeps, and sediments collected from oil polluted areas were among those with the highest proportions of mercury methylating bacteria in genomic databases (Podar et al., 2015). Bacteria capable of methylating mercury were also collected from the guts of oligochaete worms (Podar et al., 2015), which are often abundant in oil contaminated sediments. There is also evidence that methylated mercury associated with oil and gas production is entering the food chain. Dab (Limanda limanda), a demersal flatfish, collected from the Ekofisk oil field in the North Sea had the highest concentrations of methylmercury of any fish surveyed in the region, including those from the Baltic Sea (Lang et al., 2017). Studies have not explicitly linked high organic matter input and mercury from PW to rates of mercury methylation in sediments and bioaccumulation in associated seafood resources, however, because of the implications for human health (Sevillano-Morales et al., 2015), this risk should be considered before a decommissioned area is opened to recreational fishing.
Naturally occurring radioactive materials (NORMs)
Petroleum formations contain radionuclides, which may be discharged as a constituent of PW (Neff et al., 2011). Radioactivity of PW depends on the geology—in particular, the distribution of U and Th, the chlorinity and total dissolved solids—in the reservoir (Fisher, 1998). Radium in PW is expected to precipitate with sulphate once it mixes with seawater (Olsvik et al., 2012). The ecotoxicity of these compounds at it relates to the decommissioning context has been recently reviewed, with attention drawn to the paucity of data (Amy et al., 2021). Potentially, radioactive material maybe accumulated by worms, then fish that prey on benthic invertebrates (Olsvik et al., 2012). Modelling studies in the Gulf of Mexico have suggested that discharge of radionuclides do not pose a threat to marine organisms. Some authors have hypothesised that since radioactivity is higher in the Gulf of Mexico than elsewhere, risks to organisms are also low (reviewed in Hosseini et al., 2012). Low risk was also predicted for shrimp and mussels in the North Sea (reviewed in Hosseini et al., 2012). While most studies have found that the risk from exposure to NORMs is negligible, because of the lack of research, the uncertainty around this prediction is high (Hosseini et al., 2012).
Radionuclides can be precipitated as NORM scale on pipelines as the minerals mix with sea water (Hosseini et al., 2012). There is concern that if the pipelines are discarded in situ, that over time, as the pipelines break down, this material would enter marine food chains as discussed above, potentially causing ecological impacts as well as potentially entering the seafood supply. Recent modelling performed on behalf of the Australian Petroleum Production and Exploration Association (APPEA) suggests that while this is a risk, the process would be slow, and material would be diluted into surrounding marine sediments (Advisian, 2017). However, the concentrations of NORMs should be monitored as part of the decommissioning process, so that adverse outcomes can be predicted and mitigated.
Contaminant Distribution
The primary sources of contaminants associated with offshore oil and gas infrastructure include: drilling muds, drill cuttings, PW, and occasionally incidental oil spills or pipeline leaks (Cordes et al., 2016; Lelchat et al., 2020). The impacts of oil spills have been reviewed recently (Barron et al., 2020; Hook, 2020), and would be infrequent, so the remainder of the text focusses on PW, drill cuttings and drilling muds. PW is the water in the formation that is brought to the surface during the production process (Neff et al., 2011). Frequently, this water is discharged into the marine environment (Parkerton et al., 2018). PW contains environmental contaminants at concentrations sufficient to cause toxic impacts in standardised ecotoxicological bioassays (Parkerton et al., 2018), and causes adverse outcomes in fish continuously exposed in the laboratory (reviewed in Bakke et al., 2013; Beyer et al., 2020). However, few impacts from exposure to PW have been measured in the field because of the rapid dilution that occurs in turbulent marine environments (reviewed in Bakke et al., 2013; Beyer et al., 2020). None the less, over the life of an oil platform, it is possible that some of the metals and hydrophobic PAHs could precipitate out and concentrate in the sediment, leading to the accumulation of potential toxic concentrations over time.
Drilling muds are injected into the borehole during drilling, and include lubricants, as well barium and other materials used to decrease the buoyancy of the material and prevent blowouts (Lelchat et al., 2020). Drill cuttings are the materials produced during drilling process, and include drilling muds, as well as the material removed from the borehole. This material may have been in contact with the oil in the formation (Lelchat et al., 2020). For the oldest offshore platforms, an oil based lubricant was used in drilling muds, but these have been phased out since the early 1990s in favour of less toxic synthetic based muds and water based muds (Gagnon and Bakhtyar, 2013).
Contaminant Persistence and Resuspension
Once released into the environment, cuttings piles are resistant to chemical change, as they are frequently anaerobic, slowing the rates of petroleum degradation (Nguyen et al., 2018). Resuspension and dispersion are the primary means of contaminant dispersal (reviewed in Bakke et al., 2013). Drill cuttings can have physical impacts on benthic communities, such as the smothering of marine organisms (Henry et al., 2017). Toxicity from those cuttings piles is primarily driven by the hydrocarbons and depends on the type of lubricant used during drilling (reviewed in Bakke et al., 2013). In the North Sea, when oil-based muds were initially discharged, impacts could be seen at distances greater than 5 km (reviewed in Bakke et al., 2013). Now, the contaminants in oil-based muds have dissipated and been buried, and are impacts rarely seen at distances greater than 500 m (reviewed in Bakke et al., 2013). Physical stressors (such as burial from sedimentation and sediment oxygen levels) may pose a greater risk to organisms than chemical stressors when the less toxic synthetic based muds are used (reviewed in Bakke et al., 2013). Impacts from water-based muds are primarily physical and would be expected to be short-term (Bakke et al., 2013). Even exploratory drilling (which does not result in oil production) can have environmental impacts (Junttila et al., 2018), with altered barite and metal concentrations and changes in sediment grain size measured near exploratory well heads. Some of the metals associated with exploratory drilling were elevated sufficiently to cause toxicity in sediments collected from areas near exploratory drilling (Junttila et al., 2018).
Drill cuttings piles can be dispersed in areas of high current flows (Henry et al., 2017), diluting the contaminants contained within them until they no longer cause adverse impacts. Burial of contaminants via sedimentation also reduces the persistence of their impacts on the marine ecosystem, as they effectively become “out of reach” for biological systems (Henry et al., 2017). How these processes can alter the toxic impact of contaminants contained within drilling muds and cuttings piles is demonstrated in the international literature. Large cuttings piles are present in the northern North Sea, whereas in the south, cuttings piles have been dispersed by storms, currents and tides (Bakke et al., 2013). In the northern regions, impacts were typically measured within 1 km of platforms and persisted for 6–8 years, with longer recovery times for oil based muds (Henry et al., 2017). This recovery is based on the amount of time required for the drill cuttings to be covered by clean sediment. In the southern North Sea, where drill cutting did not accumulate, no impacts are typically measured (Henry et al., 2017). In the Gulf of Mexico, which has a high sedimentation rate, metals from PW were the primary contaminant, not petroleum hydrocarbons from the drilling muds and drill cuttings (Kennicutt et al., 1996b). Detoxification mechanisms for oil related compounds were not elevated in fish collected from platforms in the Gulf of Mexico (McDonald et al., 1996).
In Australia, sedimentation rates are known to be very low. For example, in the Bass Strait, separating Tasmania from the rest of Australia, sediments accumulate at less than 6–12 cm/1,000 years (Blom and Alsop, 1988). By comparison, rates of sedimentation in the Gulf of Mexico are 0.2 cm per year (Rohal et al., 2020). Sediment deposition on the Northwest shelf of Australia is also expected to be slow (Baker et al., 2008), especially when compared to oil producing regions that have higher biological productivity and higher inputs of riverine sediments. It is plausible that contaminants may be more persistent in Australia, as they are not diluted or buried by sedimentation, and as a consequence, have greater opportunity to exert toxic effects. However, sediments containing drilling muds, and their associated contaminants, may be redistributed by the frequent cyclones that occur offshore of the Northwest shelf (e.g., Dufois et al., 2017) or by the strong currents in the Bass Strait. Contaminant persistence should be quantified on a site specific basis. High contaminant levels may compromise the value of the oil and gas structures as habitat—even if contaminants are buried—since resuspension of sediment “resets” recovery, both by smothering organisms and by making buried contaminants bioavailable (Henry et al., 2017). To minimise impacts on benthic communities, decommissioning options should aim to minimise the resuspension of contaminated sediments if structures and pipelines are removed (Henry et al., 2017), and the potential for contaminant exposure should be estimated.
Bioaccumulation
Contaminants that are associated with sediments can bioaccumulate (Simpson and Batley, 2016). The possible routes of exposure include passive uptake through gills and epidermal layers of burrowing organisms and ingestion of particles by deposit and suspension feeders. Animals that prey on benthic organisms can ingest their contaminant load (Simpson and Batley, 2016). As discussed in Box 2, there is the potential for some contaminants, methylmercury in particular, to be bioaccumulated in filter feedings organisms to an extent that the area around infrastructure is not safe for collection of seafood. This possibility, with consideration of Australia’s cultural diversity, should be excluded before areas are reopened for fishing or repurposed for aquaculture.
Impacts From Continuous Exposure to Compounds in Drilling Muds and PW
Prolonged exposure to compounds in PW and drilling muds disrupts normal endocrine processes in fish and other resident marine organisms, decreasing the value of platforms as habitats. Xenobiotic metabolising enzymes, including CYP1A, are induced by high molecular weight fraction of oil (reviewed in Whyte et al., 2000; Schlenk et al., 2008). These enzymes break down many aromatic compounds, including steroid hormones (reviewed by Whyte et al., 2000) and may interrupt the normal action of steroid receptors (Arukwe et al., 2008). Crude oil and PAH exposure has been shown to interrupt steroidogenic pathways following laboratory exposures (Arukwe et al., 2008; Kennedy and Smyth, 2015), and reduced reproductive output has been noted in fish from chronically oil contaminated areas (Sol et al., 2000; Johnson et al., 2008). Studies of the chronic impacts from PW and drilling muds (in this case defined as adverse outcomes that arise from long term, ongoing exposure to contamination; Kennicutt et al., 1996a), have found that the PAH, alkylphenols, and napthenic acids associated with PW and drilling muds/cuttings are bioaccumulated by marine organisms, and may cause biochemical and reproductive changes (Balk et al., 2011; Bakke et al., 2013).
Surveys of some oil and gas producing areas have shown indications of ongoing exposure of fish to PAH. In the North Sea, elevated PAH metabolites are measured in bile and elevated levels of DNA adducts in haddock (fish which forage in the sediment) collected in areas from around platforms (Balk et al., 2011 reviewed in Beyer et al., 2020). These changes were not measured in fish collected from platforms in the Gulf of Mexico (McDonald et al., 1996), which is unsurprising given that there was little PAH gradient measured in the sediment around the platforms surveyed. Bioindicators of exposure to oil were also not changed in fish collected around platforms offshore of the maritime provinces in Canada, where synthetic drilling muds were utilised (Mathieu et al., 2011). In the Adriatic Sea, published studies have also focussed on the association of fish with oil and gas infrastructure and contaminants impacts. As Italian wells were drilled with synthetic drilling muds, and regulations prohibit the discharge of PW at sea, there have been minimal toxic impacts associated with oil and gas infrastructure (Regoli et al., 2019). While changes in meiofaunal composition in sediment have been observed, the links to toxicity have not been established, and may instead been attributed to alterations in sediment grain size and organic enrichment (Terlizzi et al., 2008; Fraschetti et al., 2016).
As a consequence of the potential for prolonged exposure to PAH and other oil constituents to impact fish reproduction, at platforms where contamination is found to persist, the health of the resident fishes should be determined before concluding that the area is good fish habitat. Uptake and detoxification rates differ between environments and species, and so generalisation between regions is difficult, meaning that research will need to be conducted at a range of Australian sites. Biochemical changes that indicate exposure to oil have been measured in fish collected near platforms on Australia’s Northwest shelf (King et al., 2005; Codi King et al., 2011). If future surveys indicate elevations in the concentrations of oil and other environmental contaminants around platforms, implications for the health of fisheries species and other species of conservation concern may then need to be determined.
Biological Feedbacks: Contaminant-Influenced Changes in Community Structure
Prolonged exposure to contaminants, especially if coupled with enrichment of organic material, may also accelerate the spread of invasive species around infrastructure in the Australian environment. Toxic responses may cause change in benthic species composition, either through outright mortality, or changes to reproduction, feeding, or other physiological parameters that impact an organism’s fitness (Hook et al., 2014). Higher concentrations of environmental contaminants have been associated with increased proportions of invasive species, which frequently have higher tolerance of environmental contaminants (reviewed in Strain et al., 2021). The presence of offshore oil platforms has been shown to change benthic communities in the North Sea and the Gulf of Mexico, both as a result of increased production and enrichment of organic matter, as well as the elevations in environmental contaminants and changes in sediment size around oil platforms (Montagna and Harper, 1996; Henry et al., 2017). In addition, contaminated sediments were found to have decreased larval settlement relative to uncontaminated sediment (Hill et al., 2013). Notably, the abundance of benthic meio/macrofauna can be driven by “top down” processes (such as predation) or “bottom up” processes (increased primary production), and may not reflect contamination at all (Montagna and Harper, 1996). Some of the changes in species composition around oil and gas infrastructure may be associated with the loss of species (suspension feeders, in particular) due to the impacts associated with drilling waste (Lelchat et al., 2020).
In the Gulf of Mexico, a survey of platforms away from the Mississippi River plume found that the concentration of metals, organic matter, and sand (associated with drilling muds) were all increased in abundance near oil and gas platforms (Kennicutt et al., 1996b). Changes in meiofaunal abundance were seen along a metals contamination gradient within 100 m of some platforms (Montagna and Harper, 1996). An increase in the abundance of nematodes and polychaete worms was attributed to increased organic enrichment, while a decrease in harpatacoid copepod and amphipod abundance was associated with toxicity (Montagna and Harper, 1996). When the changes in community structure were examined on the basis of traits, non-selective deposit feeders and organisms that fed on the epifauna increased in abundance near the platforms, but selective feeders decreased in this area (Montagna and Harper, 1996). Studies in the North Sea have also found changes in community structure along a contamination gradient—including an increased abundance of opportunistic or pollution tolerant species (Henry et al., 2017). The potential for contaminant related changes in community structure to influence habitat quality and the potential values of oil and gas infrastructure as either conservation area or for fisheries production should also be evaluated as part of the decision making process around decommissioning in Australia, or any other novel region.
Socioeconomic Considerations
The social and economic values that oil and gas infrastructure contributes to, or alternatively detracts from, need to be determined as part of any assessment of decommissioning options. This includes examining the contribution of the infrastructure, and chosen decommissioning option, to fisheries and other marine sectors, and determining the acceptability of the selected option to stakeholders (Figure 2). Although issues around social acceptability are not new, and industry understanding around gaining social license for their operations has grown, Australia is in the unique position that it has a relatively long history of exploring social license concepts (i.e., how to gain and keep social license). Much of the global literature around the concept of social license arose around the mining industry in Australia (Moffat and Zhang, 2014; Bice et al., 2017). Processes regarding Indigenous rights and perspectives in the context of social license have evolved in recent years (Uffman-Kirsch et al., 2020), and also provide insight for the decommissioning context.
Impacts on Fisheries and Ecosystem Values
Oil and gas infrastructure has the potential to add to fisheries production, meaning a “leave-in-place” decommissioning option has prospective fishery value. In the Gulf of Mexico, numerous platforms have been repurposed as fish habitats through “rigs to reefs” programmes, whereby a platform is deployed as an artificial reef following the end of oil and gas production (Bull and Love, 2019). Since the coastal residents in Louisiana and Texas (US states that border the Gulf of Mexico) include a high proportion of recreational fishers (Bull and Love, 2019), and up to 70% of these fishers utilise these platforms as fishing destinations (Reggio, 1987), these programs have been very popular (reviewed in Bull and Love, 2019).
Video evidence demonstrates that Australian demersal fishes utilise oil platforms as habitat (Pradella et al., 2014; Thomson et al., 2018) as elsewhere in the world (Ajemian et al., 2015; Benfield et al., 2019). This utilisation does not necessarily equate to increased production of fisheries species, in terms of either somatic growth, recruitment to the population, or decreased mortality (Bohnsack, 1989). Increased fishery production would result from platforms if habitat was the limiting factor. Otherwise, artificial habitat from platforms might only serve to attract and aggregate fishes, making them more easily caught, potentially resulting in further declines in overexploited fisheries (but see Smith et al., 2015). As discussed in the section “Ecological Considerations for Decommissioning,” the effect of platforms on fish population dynamics in Australia are likely different from other reported regions such as California and the Gulf of Mexico (Australian waters are more oligotrophic, have different biofouling organisms, and do not have a shell mound; Figure 3). A range of pelagic species are associated with structures in the ocean (Hobday and Campbell, 2009; Champion and Coleman, 2021). While pelagic fisheries world-wide exploit this association via use of fish aggregating devices (FADs), platforms are only likely to enhance catchability and not production of these species.
In Australia there is a large recreational fishing sector—which in some places is larger than the commercial sector in terms of volumes of fish caught (Henry and Lyle, 2003; Lyle et al., 2014; Giri and Hall, 2015; West et al., 2015). There is also high participation in this sector – although this varies by state (Productivity Commission, 2016). It is currently not known how much or intensely the recreational sector might fish around platforms. But with better navigation equipment and larger boats recreational fishers can travel further out and potentially access new areas (including platforms). The above-mentioned contaminant issues should therefore also be considered from a human health and food safety perspective given the active and mobile recreational sector, and the fact that catch is often taken home for consumption (with catch and release being less prevalent than in other parts of the world).
Rigs to reefs where platforms are recycled as artificial reefs may reduce pressure on natural reefs by providing alternative diving and fishing sites and opportunity for tourism. Ningaloo Reef in Western Australia is one of the sites that potentially could benefit from nearby oil and gas infrastructure to relieve growing tourism putting pressure on natural environment (Advisian, 2017). Increased activity on newly constructed reefs can have adverse effect on surrounding habitat and result in overfishing. Therefore, location of the reef needs to be carefully considered and long term monitoring and management plans need to be put in place (Bull and Love, 2019).
If the potential contribution to fisheries is to be used to justify leaving infrastructure in situ, the role of the infrastructure in contributing to fisheries production, rather than just aggregating fishes, need to be determined. The potential for platforms to relieve pressure on natural reefs should also be considered, together with information about local-scale fishing activity, and the implications of different decommissioning options for these activities (and associated stocks). These questions will need to be addressed in a location-specific manner in Australia, given the spatial differences that occur in target species, the nature of both commercial and recreational fisheries and the relative importance of other sectors such as tourism.
Social License and Stakeholders
Social license and social acceptance are slightly different concepts (Moffat et al., 2016) but for decommissioning imply that the activity (and the result) meets the expectations of a range of different groups in society and has their ongoing support and/or acceptance. Gaining and keeping social license is not straightforward because it involves approval from different stakeholder groups, including direct users (e.g., fishers, Traditional Owners), groups with indirect interests (e.g., tourists, media) together with political, regulatory and market-based entities (Bice et al., 2017). These stakeholder groups will have different interests and values in offshore infrastructure, the environments in which they occur, and in the decommissioning process itself, which might range from employment, recreational, cultural or environmental values through to the potential for visual disturbance associated with decommissioning activities. Understanding these values can give insight into where social license may be impacted and into processes that may contribute to social acceptance.
The importance of social license in the context of decommissioning is highlighted by the fact that “improved transparency and public engagement in relation to petroleum activities” is a key element of the Australian Government’s enhanced framework for decommissioning (Commonwealth of Australia, 2020). Transparency and knowledge transfer have been key in other locations where oil and gas decommissioning has taken place, such as in the North Sea where Nexstep (National Platform for Re-use & Decommissioning)1 was established to stimulate and facilitate collaboration amongst key stakeholders. Even though it has been 5 years since the establishment of Nextstep, policy renewal is hampered by a divergence between stakeholder alignment and the risk of reputational damage (i.e., social license) (Roos, 2019).
An example of the pertinence of social license in the Australian context is highlighted by Shaw et al. (2018) who indicate that in the case of decommissioning, industry license conditions may stipulate that full removal is required (which may have been supported by stakeholders through consultation), however if industry later considers leaving some infrastructure behind this may not meet the expectation of the stakeholders and could compromise social license for the activity. An improved evidence base regarding social license for efficient policy and practice is required (Shaw et al., 2018). Creating reliable data sources to measure social license competitiveness is (globally) important (National Energy Resources Australia [NERA], 2017) and Australia can be a first mover is this regard. This could also include the consideration that social license is important not only for current industry responsible for paying for the decommissioning, but also for new industry “around” the decommissioning to develop. Further to this, it is not just industry’s social license that needs to be considered, but also that of the relevant regulator (NOPSEMA in the Australian case). Community stakeholders must have confidence that their concerns can be heard, understood and respected, and that the regulator will enforce compliance with regulations (Van Putten et al., 2018). Maintaining that license will require the regulator to consistently demonstrate that it takes community priorities, concerns and issues into account when making decisions and enforcing industry compliance with regulations (noting that government and community expectations of regulators may evolve and mature over time).
An important aspect of social license is comprehensive and transparent approaches to stakeholder engagement and consultation. The introduction of a public comment period for submitted decommissioning environment plans and public reporting of petroleum activities have been suggested as part of a Government initiative to improve accountability for decommissioning activities (Commonwealth of Australia, 2020). But broader than these two suggestions, research into a standardised consultation process that attempts to gain social license should benefit the decommissioning process. For instance, the Free Prior and Informed Consent (FPIC) approach and associated philosophy—currently only required in cases of Indigenous stakeholder consultation—may be applicable in all communities. To undertake good stakeholder identification and consultation that incorporates the FPIC philosophy several approaches can be taken, including systematic stakeholder mapping (Raum, 2018), participatory processes, co-design and knowledge co-production (Newton and Elliott, 2016; Norström et al., 2020). Such approaches are underpinned by transparency and open consultation, together with respect for appropriate cultural protocols. Importantly, risk perspectives of Traditional Owners in the context of offshore activities and connections to sea country are often not well considered but should be incorporated in decommissioning research activities through ethical and culturally appropriate engagement (e.g., see Hedge et al., 2020). For the Australian context, this process will also need to recognise that the connections of Indigenous Peoples in Australia to land and sea Country are spatially complex (e.g., see Horton, 1996), and that multiple Traditional Owner groups may need to be engaged in consultation processes depending on the location of decommissioning activities.
In summary, factors such as the history and context of social license, spatially complex connections to Sea Country for Traditional Owners, and a large recreational fishing sector mean that engagement of stakeholders and consideration of the consequences of different decommissioning scenarios on their interests and values in offshore infrastructure will need to be carefully considered in the Australian context. More generally, to determine whether oil and gas infrastructure has value as habitat for fisheries or other species with conservation value, or conversely, to determine whether undue risk is posed by (for example) resuspending contaminated sediments, site specific assessments of the conditions around the infrastructure will need to be made. This should include an assessment of the species compositions at the infrastructure relative to nearby, natural hard and soft substrate areas, including quantifying the abundance and diversity of introduced species, and how these contribute to the inevitable compositional differences. Since many decommissioning options involve removing the upper portions of platforms (i.e., “topsides”), estimates should be made of how this removal will affect the value and productivity of the system as a whole. The oceanographic connectivity between structures and between structures and other habitats should be determined, to evaluate the potential for oil and gas infrastructure to “seed” larvae for fisheries production and/or to act as a source of introduced pests. Connectivity should also be modelled to determine whether the different pieces of infrastructure (e.g., individual platforms; sections of pipeline) in the area are acting as an interconnected ecosystem or as isolated habitat. The influence of contaminants associated with oil and gas production on the habitat value and the potential for contaminants to enter the food chain needs to be determined, especially if the area could be used for recreational or commercial fishing.
Assessing the Impacts and Risk of Different Decommissioning Options
Decommissioning presents all the hallmarks of a difficult decision-making problem. First, a choice must be made from among several options of what to do with infrastructure at the end of its useful life (as highlighted in Table 1). Each option has different consequences when viewed from the perspective of the various criteria—such as safety, environment, technical, economic, and societal—that influence decision makers and the stakeholders that they are accountable to. The choice of a preferred option therefore entails a trade-off between consequences measured on different scales. These consequences, however, are also shrouded in various degrees of uncertainty, which complicates the trade-off and makes the final decision vulnerable to heuristics and bias (Kahneman, 2011).
Problems of this type are common (e.g., Esmail and Geneletti, 2018) but hard to resolve with unstructured dialogue in committee settings. Consequently, a large variety of decision support tools have been developed to assist. Table 2 summarises six tools that have been used to help identify a preferred decommissioning option and how the methods vary with the scope of the assessment.
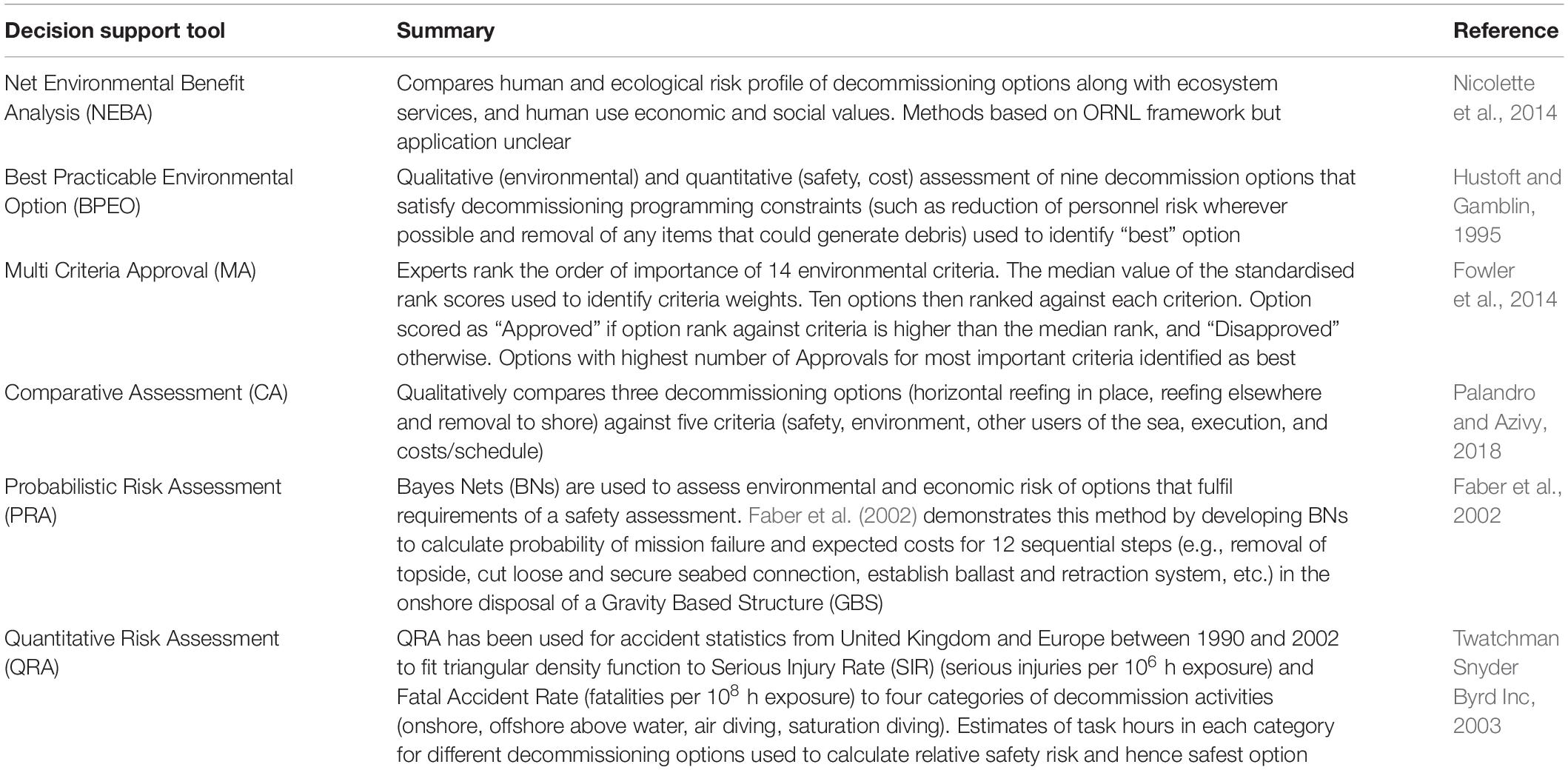
Table 2. Summary of decision support tools that have been used in a decommissioning option evaluation.
One common approach is Multi-Criteria Decision Analysis (MCDA; Table 2)—a term used to describe an array of techniques sharing three common steps: (i) specify the decision context by: (a) identifying a set of alternative choices; and (b) defining the criteria against which these alternatives will judged; (ii) conduct the analysis through some form of (usually weighted) evaluation and scoring of options against criteria; and (iii) identify the preferred option by ranking the aggregated score from highest to lowest (Esmail and Geneletti, 2018). Examples of MCDA include Net Environmental Benefit Analysis, Best Practicable Environmental Option, Multi Criteria Approval and Comparative Assessment (Table 2). MCDA approaches provide an opportunity to bring diverse groups of stakeholders together, increase understanding and empathy by asking them to define their values and formulate decision criteria, and encourages consensus on acceptable outcomes by explicitly incorporating these values into the decision-making process. If carefully planned and conducted, the value of MCDA lies in the shared process as well as the outcome.
Risk assessment is the second major class of tools with a large array of qualitative, semi-quantitative and quantitative techniques that are typically applied within environmental, financial and safety domains (e.g., see Table 2). Methods for merging risk estimates across domains, for example by converting deaths, illnesses, and environmental outcomes into monetary units or utility units, are available. National Research Council (1996) discusses the strengths and weakness of such an approach in a risk-based decision-making processes, but caution against it in favour of a less technical “deliberative analytical” process that involves analytical experts, public officials and interested and affected parties. Both qualitative and quantitative approaches have been used for decommissioning around the world (Table 2) and for fisheries in Australia (e.g., Hobday et al., 2011), however, techniques for merging risk estimates across different domains are rarely employed by Australia’s state and federal environmental agencies.
Current Australian Government policy states that any proposed deviation from complete removal of offshore oil and gas infrastructure must deliver equal or better environmental, safety and well integrity outcomes, compared to complete removal (Australian Government Department of Industry, Science, Energy and Resources [DISER], 2018). The Environmental Plan (EP) for any such proposal must also evaluate the environmental impacts and risks of all feasible options, including partial and complete removal (National Offshore Petroleum Safety and Environmental Management Authority [NOPSEMA], 2020b). NOPSEMA warns, however, that while MCDA tools can support consultations with stakeholders on alternative decommissioning options, they do not directly relate to the acceptance criteria for an EP (as described in section 10(A) of the Offshore Petroleum and Greenhouse Gas Storage (Environment) Regulations 2009) which must demonstrate that the proposed option can be undertaken in such a way that the environmental impacts and risks, including potentially cumulative risks, can be managed to a level that is acceptable and ALARP (National Offshore Petroleum Safety and Environmental Management Authority [NOPSEMA], 2020a; Box 3).
BOX 3. Environmental risk assessment for Australian decommissioning.
There is no single-accepted framework for environmental risk assessment but it is often characterised as a three-phase process that begins with problem formulation, followed by risk calculations, and culminating in a risk management or evaluation phase (National Research Council, 1996; United States Environmental Protection Agency [USEPA], 1998; Renn, 2008). These phases align quite well with the describe, detail and evaluate content requirements of an EP.
The problem formulation phase includes a description of the environmental values that are potentially threatened by a substance or activity (the assessment endpoints) and a hazard analysis. The hazard analysis tries to identify all the ways that the assessment endpoints interact with, and might be harmed by, a substance or activity—i.e., identify the pathways to harm or equivalently the adverse outcome pathways (Ankley et al., 2010).
In this initial stage the EP needs to demonstrate a suitable understanding of the environment(s) in which the proposed option will take place (National Offshore Petroleum Safety and Environmental Management Authority [NOPSEMA], 2019b). This understanding should address ecological, socio-economic and cultural features to ensure that the assessment endpoints adequately capture the diversity of environmental values that may be impacted, but also to allow the hazard analysis to expose the possibility of cumulative impacts through pathways that involve interactions with other industries and users.
Risk calculations usually distinguish between the likelihood of an adverse effect and the magnitude or consequence of that effects (although the nomenclature varies widely between disciplines). Methods for calculating likelihood and consequence can be broadly classified into qualitative or quantitative according to whether likelihood and consequence are expressed in a relative way using an ordinal scale (e.g., high, medium, low), or probability is used to express likelihood and a ratio scale is used to describe consequences (e.g., fish biomass, sediment concentration of a contaminant).
NOPSEMA guidance is agnostic as to which risk calculation method should be used, although any emphasis on cumulative risks effectively precludes qualitative techniques. The guidance does, however, distinguish between “higher order” and “lower order” environmental risks and impacts. The latter is defined as situations wherein the environmental value is not formally managed, is less vulnerable to the impact or risk, is widely distributed, is not protected and/or threatened, and there is confidence in the effectiveness of control measures (National Offshore Petroleum Safety and Environmental Management Authority [NOPSEMA], 2019b).
For higher order risks the EP needs to be more comprehensive which suggests that the environmental risk assessment could adopt a staged-approach, whereby an initial, relatively rapid calculation technique is used to separate and screen out low order risks from higher order ones, and then a more detailed resource intensive approach is applied to any environmental values that are identified as high order. This type of approach would be consistent with other environmental risk assessments (for example Hobday et al., 2011) and is useful when the number of potentially affected environmental values is large.
A large proportion of NOPSEMA guidance is directed towards the risk evaluation phase, perhaps because this phase can be contentious as it entails judgement about the acceptability of risks. Here the EP must demonstrate that all risks have been reduced to ALARP, and for higher-order risks must show separately that the risks are acceptable. For low-order risks, adherence to control methods specified by industry standards would be considered ALARP and thereby acceptable, but this presumes that standards for low-order interactions identified in the problem formulation or risk calculation phase exist and are relevant to Australian conditions. For higher-order risks and impacts, the ALARP commitment requires the titleholder to implement all control measures where the cost is not grossly disproportionate to the environmental benefit, and subsequently demonstrate that any residual (or unmanaged) risks or impacts are acceptable. In practise this requires environmental standards and measurable acceptance criteria to be specified for any higher-order risks or impacts identified in the problem formulation and/or risk calculation phases, and precludes qualitative risk calculations because ordinal risk or impact estimates cannot be used to measure the magnitude of the deviation between a prediction, an observation or a measurable standard.
In Australian waters, any proposed deviation from the decommissioning base case of complete infrastructure removal must address several information requirements and risk-related challenges:
• Sufficient understanding of the distribution and sensitivity of environmental values to decommission-related threats to distinguish low -order from high order environmental risks.
• Sufficient understanding of the distribution and magnitude of other socioeconomic activities in the vicinity of decommissioned infrastructure to identify the possibility of cumulative impacts.
• A risk assessment methodology that can screen-out low order risks in a relatively rapid but defensible fashion.
• Industry standards for low order impact control/risk mitigation methods and measurable acceptance criteria for any high-order environmental values.
• A quantitative risk assessment methodology that can be used to predict (possibly cumulative) risks to any high order environmental values, and demonstrate ALARP and acceptable levels of impact on these values.
Recommendations for Decommissioning Research in Australia
Repurposing Australian offshore oil and gas infrastructure, either as habitat for commercially or ecologically important species, for carbon capture and storage (e.g., see Hastings and Smith, 2020), or for other purposes, may, provide environmental benefit with minimal environmental risk. These risks may differ from those identified in research elsewhere in the world because of differences in biodiversity, biological productivity, ocean/climate effects (e.g., low sedimentation rates, rising temperatures, cyclonic activity, and shallow water depth), as well as sociodemographic and cultural characteristics. A thorough assessment of the ecological value of the infrastructure as habitat in the location of interest, and an environmental risk assessment, must therefore be performed, perhaps quantitatively for high order environmental values and/or cumulative risks (Box 3). The risk assessment should characterise the biological production at infrastructure, including relative to nearby natural habitat (such as natural reefs); the risk to both seafood contamination and ecological health posed by legacy contamination at the site (Box 2); and the prospective environmental risks posed by introduced species and other marine pests.
Knowledge gaps that currently preclude a comprehensive risk assessment for decommissioning in Australia, as well as other understudied regions of the world, include uncertainty about the impacts of long-term exposure to Naturally Occurring Radioactive Material (NORMs) and the unresolved potential for oil and gas infrastructure to be active areas for mercury methylation (Box 3). Questions about socioeconomic impacts may also be a potential obstacle to successful decommissioning. Values that are held by Australia’s diverse Aboriginal and Torres Straight Island communities, must be incorporated into the decision-making process and seafood safety assessments must be culturally appropriate (Wilson et al., 2015).
We suggest that decommissioning research activities in Australia should address three key elements and interdependencies—ecological considerations, contaminants and socioeconomics (section on “Status of Decommissioning Research Internationally and in Australia,” Figure 5), in a manner that integrates decision support tools (section on “Assessing the Impacts and Risk of Different Decommissioning Options”). The particular science questions relating to these elements are likely to be location specific, given the variability of marine environments in Australia (Figure 1), the different ages and types of offshore infrastructure, and potential differences related to social acceptance and consultation between different regions of Australia. Science underpinning the assessment of environmental risk of decommissioning options should be connected to research relating to options for repurposing offshore infrastructure, such as carbon capture and storage or offshore aquaculture (Sommer et al., 2019). For each decommissioning region, reviewing what is known about each of the elements in Figure 5, and then investing in research to address gaps, is one way to demonstrate awareness of the range of issues, and can also underpin the selection of decommissioning options for assets.
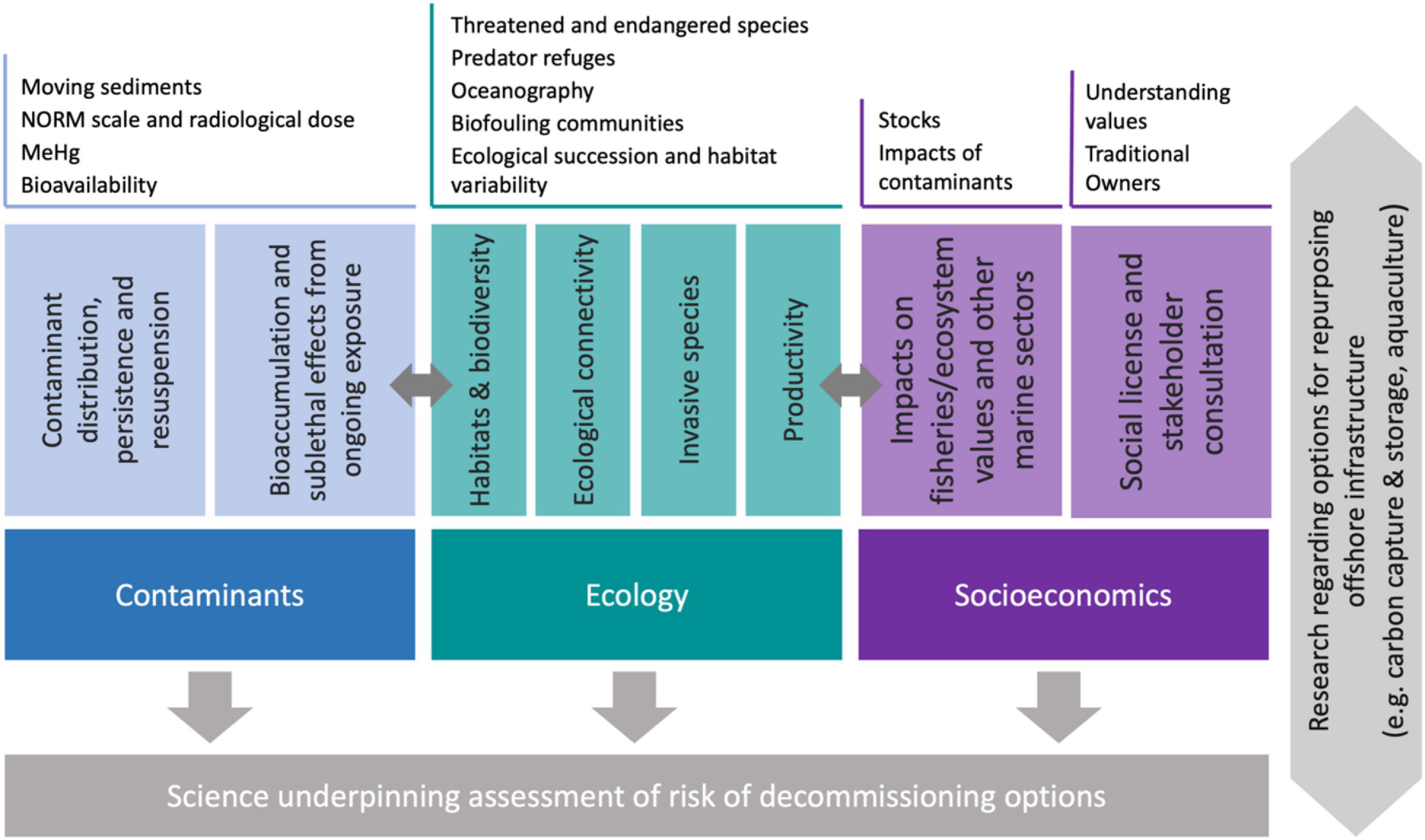
Figure 5. A general framework for integrated research to assess the risk of decommissioning options for oil and gas infrastructure in Australia.
Experience in the Gulf of Mexico illustrates how an integrated research framework (Figure 5) can succeed. Repurposing offshore oil and gas infrastructure in a “rigs to reefs” program in this region was ultimately successful because: (i) the operators’ stakeholder engagement and consultation process identified recreational fishing as a community value (reviewed in Bull and Love, 2019); (ii) surveys of contaminant levels showed that they were below thresholds of concern (Kennicutt et al., 1996a), and unlikely to be affecting benthic biodiversity (Montagna et al., 2002) or fisheries productivity (McDonald et al., 1996); and (iii) the platforms were known to provide habitat for Red Snapper, a commercially important species (Ajemian et al., 2015). These studies, when integrated, provided the scientific underpinning to maintain the social license required to support the “leave in place” decommissioning option. While there are multiple factors that distinguish the Australian decommissioning context from the Gulf of Mexico and elsewhere, the framework in Figure 5 can be used by operators to help identify similar science needs to underpin decommissioning decisions in Australian waters. Armed with this knowledge, operators, regulators, marine stakeholders and the general public can be confident that sound decisions are being made to manage the transition of oil and gas infrastructure in an environmentally and socially acceptable way.
Author Contributions
JM-T and SH conceived the study. All authors contributed to the writing of the manuscript.
Conflict of Interest
The authors declare that the research was conducted in the absence of any commercial or financial relationships that could be construed as a potential conflict of interest.
Publisher’s Note
All claims expressed in this article are solely those of the authors and do not necessarily represent those of their affiliated organizations, or those of the publisher, the editors and the reviewers. Any product that may be evaluated in this article, or claim that may be made by its manufacturer, is not guaranteed or endorsed by the publisher.
Acknowledgments
We thank John Keesing and Ken Fitzpatrick for valuable discussion and suggestions, and Rowan Trebilco and Derek Fulton for assistance with the figures. Thank you to Christine Lamont and David Christensen from NOPSEMA for assistance with data. Thank you to Andrew Taylor and NERA for advice on Figure 1. We also thank Alan Williams, Andrew Ross, and the two reviewers for constructive comments that improved previous versions of this paper.
Footnotes
References
Advisian (2017). Scientific Literature Review Environmental Impacts of Decommissioning Options. North Sydney, NSW: Advisian.
Advisian (2020). Offshore Oil and Gas Decommissioning Liability (Australia): Executive Summary. Australia: NERA.
Ajemian, M. J., Wetz, J. J., Shipley-Lozano, B., Shively, J. D., and Stunz, G. W. (2015). An analysis of artificial reef fish community structure along the northwestern gulf of mexico shelf: potential impacts of “Rigs-to-Reefs” Programs. PLoS One 10:e0126354. doi: 10.1371/journal.pone.0126354
Amy, M., Dafforn, K., Penrose, B., Chariton, A., and Cresswell, T. (2021). Ecotoxicological effects of decommissioning offshore petroleum infrastructure: a systematic review. Crit. Rev. Environ. Sci. Technol. doi: 10.1080/10643389.2021.1917949
Ankley, G. T., Bennett, R. S., Erickson, R. J., Hoff, D. J., Hornung, M. W., Johnson, R. D., et al. (2010). Adverse outcome pathways: a conceptual framework to support ecotoxicology research and risk assessment. Environ. Toxicol. Chem. 29, 730–741. doi: 10.1002/etc.34
Arukwe, A., Nordtug, T., Kortner, T. M., Mortensen, A. S., and Brakstad, O. G. (2008). Modulation of steroidogenesis and xenobiotic biotransformation responses in zebrafish (Danio rerio) exposed to water-soluble fraction of crude oil. Environ. Res. 107, 362–370. doi: 10.1016/j.envres.2008.02.009
Australian Government Department of Industry, Science, Energy and Resources [DISER] (2018). Offshore Petroleum Decommissioning Guideline. Canberra: DISER.
Baker, C., Potter, A., Tran, M., and Heap, A. D. (2008). Geomorphology and Sedimentology of the Northwest Marine Region of Australia. Geoscience Australia, Record 2008/07. Canberra: Geoscience Australia, 220.
Bakke, T., Klungsoyr, J., and Sanni, S. (2013). Environmental impacts of produced water and drilling waste discharges from the Norwegian offshore petroleum industry. Mar. Environ. Res. 92, 154–169. doi: 10.1016/j.marenvres.2013.09.012
Balk, L., Hylland, K., Hansson, T., Berntssen, M. H. G., Beyer, J., Jonsson, G., et al. (2011). Biomarkers in natural fish populations indicate adverse biological effects of offshore oil production. PLoS One 6:e19735. doi: 10.1371/journal.pone.0019735
Barron, M. G., Vivian, D. N., Heintz, R. A., and Yim, U. H. (2020). Long-Term ecological impacts from oil spills: comparison of exxon valdez, hebei spirit, and deepwater horizon. Environ. Sci. Technol. 54, 6456–6467. doi: 10.1021/acs.est.9b05020
Beckers, F., and Rinklebe, J. (2017). Cycling of mercury in the environment: sources, fate, and human health implications: a review. Crit. Rev. Environ. Sci. Technol. 47, 693–794. doi: 10.1080/10643389.2017.1326277
Beckley, L. E., Muhling, B. A., and Gaughan, D. J. (2009). Larval fishes off Western Australia: influence of the Leeuwin current North-West shelf. J. R. Soc. West. Aust. 92, 101–109.
Benfield, M. C., Kupchik, M. J., Palandro, D. A., Dupont, J. M., Blake, J. A., and Winchell, P. (2019). Documenting deepwater habitat utilization by fishes and invertebrates associated with Lophelia pertusa on a petroleum platform on the outer continental shelf of the Gulf of Mexico using a remotely operated vehicle. Deep Sea Res. I Oceanogr. Res. Pap. 149:103045. doi: 10.1016/j.dsr.2019.05.005
Bernstein, B. B. (2015). Evaluating alternatives for decommissioning California’s offshore oil and gas platforms. Integr. Environ. Assess. Manag. 11, 537–541. doi: 10.1002/ieam.1657
Beyer, J., Goksoyr, A., Hjermann, D. O., and Klungsoyr, J. (2020). Environmental effects of offshore produced water discharges: a review focused on the Norwegian continental shelf. Mar. Environ. Res. 162:105155. doi: 10.1016/j.marenvres.2020.105155
Bice, S., Brueckner, M., and Pforr, C. (2017). Putting social licence to operate on the map: a social, actuarial and political risk and licensing model (SAP Model). Resourc. Policy 53, 46–55. doi: 10.1016/j.resourpol.2017.05.011
Blom, W. M., and Alsop, D. B. (1988). Carbonate mud sedimentation on a temperate shelf: bass basin, southeastern Australia. Sediment. Geol. 60, 269–280. doi: 10.1016/0037-0738(88)90124-8
Bohnsack, J. A. (1989). Are high densities of fishes at artificial reefs the result of habitat limitation or behavioral preference? Bull. Mar. Sci. 44, 631–645.
Bond, T., Prince, J., McLean, D. L., and Partridge, J. C. (2020). Comparing the utility of industry ROV and hybrid-AUV imagery for surveys of fish along a subsea pipeline. Mar. Technol. Soc. J. 54, 33–42. doi: 10.4031/mtsj.54.3.5
Braga, M. D. A., Pavia, S. V., de Gurjao, L. M., Teixeira, C. E. P., Gurgel, A. L. A. R., Periera, P. H. C., et al. (2021). Retirement risks: invasive coral on old oil platform on the Brazilian equatorial continental shelf. Mar. Pollut. Bull. 165:112156. doi: 10.1016/j.marpolbul.2021.112156
Bravo, A. G., Bouchet, S., Tolu, J., Bjorn, E., Mateos-Rivera, A., and Bertilsson, S. (2017). Molecular composition of organic matter controls methylmercury formation in boreal lakes. Nat. Commun. 8:14255.
Bull, A. S., and Love, M. S. (2019). Worldwide oil and gas platform decommissioning: a review of practices and reefing options. Ocean Coast. Manag. 168, 274–306. doi: 10.1016/j.ocecoaman.2018.10.024
Carvalho, S., Moura, A., Curdia, J., da Fonseca, L. C., and Santos, M. N. (2013). How complementary are epibenthic assemblages in artificial and nearby natural rocky reefs? Mar. Environ. Res. 92, 170–177.
Champion, C., and Coleman, M. A. (2021). Seascape topography slows predicted range shifts in fish under climate change. Limnol. Oceanogr. 6, 143–153. doi: 10.1002/lol2.10185
Claisse, J. T., Love, M. S., Meyer-Gutbrod, E. L., Williams, C. M., and Pondella, D. J. II (2019). Fishes with high reproductive output potential on California offshore oil and gas platforms. Bull. Mar. Sci. 95, 515–534. doi: 10.5343/bms.2019.0016
Claisse, J. T., Pondella, D. J., Love, M., Zahn, L. A., Williams, C. M., Williams, J. P., et al. (2014). Oil platforms off California are among the most productive marine fish habitats globallyle. Proc. Natl. Acad. Sci. U.S.A. 111, 15462–15467. doi: 10.1073/pnas.1411477111
Claisse, J. T., Pondella, D. J., Love, M., Zahn, L. A., Williams, C. M., and Bull, A. S. (2015). Impacts from partial removal of decommissioned oil and gas platforms on fish biomass and production on the remaining platform structure and surrounding shell mounds. PLoS One 10:e0135812. doi: 10.1371/journal.pone.0135812
Codi King, S., Conwell, C., Haasch, M., Mondon, J., Mueller, J., Zhu, S., et al. (2011). “Field evaluation of a suite of biomarkers in an australian tropical reef species, stripey seaperch (Lutjanus carponotatus): assessment of produced water from the harriet a platform,” in Produced Water, eds K. Lee and J. Neff (New York, NY: Springer), 608.
Commonwealth of Australia (2006). A Guide to the Integrated Marine and Coastal Regionalisation of Australia Version 4.0. Canberra: Department of the Environment and Heritage.
Commonwealth of Australia (2020). Enhancing Australia’s Decommissioning Framework for Oil and Gas Activities, Consultation Paper, Australian Government Department of Industry, Science, Energy and Resources. Canberra: Australian Government.
Cordes, E. E., Jones, D. O. B., Schlacher, T. A., Amon, D. J., Bernardino, A. F., Brooke, S., et al. (2016). Environmental impacts of the deep-water oil and gas industry: a review to guide management strategies. Front. Environ. Sci. 4:58. doi: 10.3389/fenvs.2016.00058
Cresswell, G., and Peterson, J. (1993). The leeuwin current south of Western Australia. Mar. Freshw. Res. 44, 285–303. doi: 10.1071/mf9930285c
Cuttler, M., Hansen, J., Lowe, R., and Drost, E. J. F. (2018). Response of a fringing reef coastline to the direct impact of a tropical cyclone. Limnol. Oceanogr. Lett. 3, 31–38. doi: 10.1002/lol2.10067
Drost, E. J. F., Lowe, R. J., Ivey, G. N., Jones, N. L., and Pequignet, C. A. (2017). The effects of tropical cyclone characteristics on the surface wave fields in Australia’s North West region. Cont. Shelf Res. 139, 35–53. doi: 10.1016/j.csr.2017.03.006
Dufois, F., Lowe, R. J., Branson, P., and Fearns, P. (2017). Tropical cyclone-driven sediment dynamics over the australian north west shelf. J. Geophys. Res. 122, 10225–10244. doi: 10.1002/2017jc013518
Duarte, C. M., Pitt, K. A., Lucas, C. H., Purcell, J. E., Uye, S.-i., Robinson, K., et al. (2013). Is global ocean sprawl a cause of jellyfish blooms? Front. Ecol. Environ. 11:91–97. doi: 10.1890/110246
Emery, B. M., Washburn, L., Love, M. S., Hishimoto, M. M., and Ohlmann, J. C. (2006). Do oil and gas platforms off California reduce recruitment of bocaccio (Sebastes paucispinis) to natural habitat? An analysis based on trajectories derived from high-frequency radar. Fish. Bull. 104, 391–400. doi: 10.1093/jhered/ess002
Esmail, B. A., and Geneletti, D. (2018). Multi-criteria decision analysis for nature conservation: a review of 20 years of applications. Methods Ecol. Evol. 9, 42–53. doi: 10.1111/2041-210X.12899
Faber, M. H., Kroon, I. B., Kragh, E., and Decosemaeker, D. B. P. (2002). Risk assessment of decommissioning options using bayesian networks. J. Offshore Mechanics Arctic Eng. 124, 231–238. doi: 10.1115/1.1491974
Fisher, R. S. (1998). Geologic and geochemical controls on Naturally Occurring Radioactive Materials (NORM) in produced water from oil, gas, and geothermal operations. Environ. Geosci. 5, 139–150. doi: 10.1046/j.1526-0984.1998.08018.x
Fowler, A. M., Jørgensen, A. M., Svendsen, J. C., Macreadie, P. I., Jones, D. O., Boon, A. R., et al. (2018). Environmental benefits of leaving offshore infrastructure in the ocean. Front. Ecol. Environ. 16:571–578. doi: 10.1002/fee.1827
Fowler, A. M., Macreadie, P. I., and Booth, D. J. (2015). Should we “reef” obsolete oil platforms? Proc. Natl. Acad. Sci. U.S.A. 112:E102. doi: 10.1073/pnas.1422274112
Fowler, A. M., Macreadie, P. I., Jones, D. O. B., and Booth, D. J. (2014). A multi-criteria decision approach to decommissioning of offshore oil and gas infrastructure. Ocean Coast. Manag. 87, 20–29. doi: 10.1016/j.ocecoaman.2013.10.019
Fraschetti, S., Guarnieri, G., Gambi, C., Bevilacqua, S., Terlizzi, A., and Danovaro, R. (2016). Impact of offshore gas platforms on the structural and functional biodiversity of nematodes. Mar. Environ. Res. 115, 56–64. doi: 10.1016/j.marenvres.2016.02.001
Fuchs, H. L., Chant, R. J., Hunter, E. J., Curchitser, E. N., Gerbi, G. P., and Chen, E. Y. (2020). Wrong-way migrations of benthic species driven by ocean warming and larval transport. Nat. Clim. Chang. 10, 1052–1056. doi: 10.1038/s41558-020-0894-x
Gagnon, M. M., and Bakhtyar, S. (2013). Induction of fish biomarkers by synthetic-based drilling muds. PLoS One 8:e69489. doi: 10.1371/journal.pone.0069489
Giri, K., and Hall, K. (2015). South Australian Recreational Fishing Survey 2013/14, Fisheries Victoria Internal Report Series No. 62. Queenscliff, VIC: Fisheries Victoria.
Goddard, J. H. R., and Love, M. S. (2010). Megabenthic invertebrates on shell mounds associated with oil and gas platforms off California. Bull. Mar. Sci. 86, 533–554.
Hastings, A., and Smith, P. (2020). Achieving net zero emissions requires the knowledge and skills of the oil and gas industry. Front. Clim. 2:601778. doi: 10.3389/fclim.2020.601778
Hedge, P., van Putten, E. I., Hunter, C., and Fischer, M. (2020). Perceptions, motivations and practices for indigenous engagement in marine science in Australia. Front. Mar. Sci. 7:522. doi: 10.3389/fmars.2020.00522
Henry, G. W., and Lyle, J. M. (2003). The National Recreational and Indigenous Fishing Survey July 2003. FRDC Project No. 99/158. Canberra, ACT: Australian Government Department of Agriculture.
Henry, L. A., Harries, D., Kingston, P., and Roberts, J. M. (2017). Historic scale and persistence of drill cuttings impacts on North Sea benthos. Mar. Environ. Res. 129, 219–228. doi: 10.1016/j.marenvres.2017.05.008
Hill, N. A., Simpson, S. L., and Johnston, E. L. (2013). Beyond the bed: effects of metal contamination on recruitment to bedded sediments and overlying substrata. Environ. Pollut. 173, 182–191. doi: 10.1016/j.envpol.2012.09.029
Hobday, A. J., and Campbell, G. W. (2009). Topographic preferences and habitat partitioning by pelagic fishes off southern Western Australia. Fish. Res. 95, 332–340. doi: 10.1016/j.fishres.2008.10.004
Hobday, A. J., Smith, A. D. M., Stobutzki, I. C., Bulman, C., Daley, R., Dambacher, J. M., et al. (2011). Ecological risk assessment for the effects of fishing. Fish. Res. 108, 372–384.
Holst, S., and Jarms, G. (2007). Substrate choice ad settlement preferences of planula larvae of five Scyphozoa (Cnidaria) from German Bight, North Sea. Mar. Biol. 151, 863–871. doi: 10.1007/s00227-006-0530-y
Hook, S. E. (2020). Beyond thresholds: a holistic approach to impact assessment is needed to enable accurate predictions of environmental risk from oil spills. Integr. Environ. Assess. Manag. 16, 813–830. doi: 10.1002/ieam.4321
Hook, S. E., Gallagher, E. P., and Batley, G. E. (2014). The role of biomarkers in the assessment of aquatic ecosystem health. Integr. Environ. Assess. Manag. 10, 327–341. doi: 10.1002/ieam.1530
Horton, D. R. (1996). The AIATSIS Map of Indigenous Australia. Australian Institute of Aborigi- nal and Torres Strait Island Studies. Available online at: https://aiatsis.gov.au/explore/map-indigenous-australia (Accessed April 14, 2021).
Hosseini, A., Brown, J. E., Gwynn, J. P., and Dowdall, M. (2012). Review of research on impacts to biota of discharges of naturally occurring radionuclides in produced water to the marine environment. Sci. Total Environ. 438, 325–333. doi: 10.1016/j.scitotenv.2012.08.047
Hustoft, R., and Gamblin, R. (1995). “Preparing for the decommissioning of the Heather Field,” in Proceedings of the Offshore Europe Conference, September 1995. Society of Petroleum Engineers 30372, (Aberdeen).
Johnson, L. L., Arkoosh, M. R., Bravo, C. F., Collier, T. K., Krahn, M. M., Meador, J. P., et al. (2008). “The effects of polycyclic aromatic hydrocarbons in fish from Puget Sound, Washington,” in The Toxicology of Fishes, eds R. T. Di Giulio and D. E. Hinton (Boca Raton, FL: CRC Press).
Junttila, J., Dijkstra, N., and Aagaard-Sorensen, S. (2018). Spreading of drill cuttings and sediment recovery of three exploration wells of different ages, SW Barents Sea, Norway. Mar. Pollut. Bull. 135, 224–238. doi: 10.1016/j.marpolbul.2018.06.064
Kennedy, C. J., and Smyth, K. R. (2015). Disruption of the rainbow trout reproductive endocrine axis by the polycyclic aromatic hydrocarbon benzo a pyrene. Gen. Comp. Endocrinol. 219, 102–111. doi: 10.1016/j.ygcen.2015.03.013
Kennicutt, M. C., Boothe, P. N., Wade, T. L., Sweet, S. T., Rezak, R., Kelly, F. J., et al. (1996a). Geochemical patterns in sediments near offshore production platforms. Can. J. Fish. Aquat. Sci. 53, 2554–2566. doi: 10.1139/f96-214
Kennicutt, M. C., Green, R. H., Montagna, P., and Roscigno, P. F. (1996b). Gulf of Mexico offshore operations monitoring experiment (GOOMEX), phase I: sublethal responses to contaminant exposure - introduction and overview. Can. J. Fish. Aquat. Sci. 53, 2540–2553. doi: 10.1139/f96-213
Kim, H. H., Kucharzyk, K. H., Zhang, T., and Deshusses, M. A. (2013). Mechanisms regulating mercury bioavailability for methylating microorganisms in the aquatic environment: a critical review. Environ. Sci. Technol. 47, 2441–2456. doi: 10.1021/es304370g
King, S. C., Johnson, J. E., Haasch, M. L., Ryan, D. A. J., Ahokas, J. T., and Burns, K. A. (2005). Summary results from a pilot study conducted around an oil production platform on the Northwest Shelf of Australia. Mar. Pollut. Bull. 50, 1163–1172. doi: 10.1016/j.marpolbul.2005.04.027
Lang, T., Kruse, R., Haarich, M., and Wosniok, W. (2017). Mercury species in dab (Limanda limanda) from the North Sea, Baltic Sea and Icelandic waters in relation to host-specific variables. Mar. Environ. Res. 124, 32–40. doi: 10.1016/j.marenvres.2016.03.001
Lelchat, F., Dussauze, M., Lemaire, P., Theron, M., Toffin, L., and Le Floch, S. (2020). Measuring the biological impact of drilling waste on the deep seafloor: an experimental challenge. J. Hazard. Mater. 389:122132. doi: 10.1016/j.jhazmat.2020.122132
Love, M. S., and York, A. (2005). A comparison of the fish assemblages associated with an oil/gas pipeline and adjacent seafloor in the Santa Barbara Channel, southern California bight. Bull. Mar. Sci. 77, 101–117.
Love, M. S., Caselle, J. E., and Snook, L. (2000). Fish assemblages around seven oil platforms in the Santa Barbara Channel area. Fish. Bull. 98, 96–117.
Love, M. S., Schroeder, D. M., and Lenarz, W. H. (2005). Distribution of bocaccio (Sebastes paucispinis) and cowcod (Sebastes levis) around oil platforms and natural outcrops off California with implications for larval production. Bull. Mar. Sci. 77, 397–408.
Love, M. S., Caselle, J., and Snook, L. (1999). Fish assemblages on mussel mounds surrounding seven oil platforms in the Santa Barbara Channel and Santa Maria Basin. Bull. Mar. Sci. 65, 497–513.
Love, M. S., Kui, L., and Claisse, J. T. (2019a). The role of jacket complexity in structuring fish assemblages in the midwaters of two California oil and gas platforms. Bull. Mar. Sci. 95, 597–615. doi: 10.5343/bms.2017.1131
Love, M. S., Nishimoto, M. M., Snook, L., and Kui, L. (2019b). An analysis of the sessile, structure-forming invertebrates living on California oil and gas platforms. Bull. Mar. Sci. 95, 583–596. doi: 10.5343/bms.2017.1042
Love, M. S., Nishimoto, M., Clark, S., and Schroeder, D. M. (2012). Recruitment of young-of-the-year fishes to natural and artificial offshore structures within central and southern California waters, 2008-2010. Bull. Mar. Sci. 88, 863–882. doi: 10.5343/bms.2011.1101
Lyle, J. M., Stark, K. E., and Tracey, S. R. (2014). 2012-13 Survey of Recreational Fishing in Tasmania. Hobart, TAS: The Institute for Marine and Antarctic Studies.
Macreadie, P. I., Fowler, A. M., and Booth, D. J. (2011). Rigs-to-reefs: will the deep sea benefit from artificial habitat? Front. Ecol. Environ. 9:455–461. doi: 10.1890/100112
Macreadie, P. I., Fowler, A. M., and Booth, D. J. (2012). Rigs-to-reefs policy: can science trump public sentiment? Front. Ecol. Environ. 10:179–180. doi: 10.1890/12.WB.013
Mason, R. P., Choi, A. L., Fitzgerald, W. F., Hammerschmidt, C. R., Lamborg, C. H., Soerensen, A. L., et al. (2012). Mercury biogeochemical cycling in the ocean and policy implications. Environ. Res. 119, 101–117. doi: 10.1016/j.envres.2012.03.013
Mathieu, A., Hanlon, J., Myers, M., Melvin, W., French, B., DeBlois, E., et al. (2011). “Studies on fish health around the Terra Nova oil development site on the Grand Banks before and after release of produced water,” in Produced Water: Environmental Risks and Advances in Mitigation Technologies, eds K. Lee and J. Neff (New York, NY: Springer), 375–399. doi: 10.1007/978-1-4614-0046-2_20
McDonald, S. J., Willett, K. L., Thomsen, J., Beatty, K. B., Connor, K., Narasimhan, T. R., et al. (1996). Sublethal detoxification responses to contaminant exposure associated with offshore production platforms. Can. J. Fish. Aquat. Sci. 53, 2606–2617. doi: 10.1139/f96-217
McLean, D. L., Partridge, J. C., Bond, T., Birt, M. J., Bornt, K. R., and Langlois, T. J. (2017). Using industry ROV videos to assess fish associations with subsea pipelines. Cont. Shelf Res. 141, 76–97. doi: 10.1016/j.csr.2017.05.006
McLean, D. L., Taylor, M. D., Partridge, J. C., Gibbons, B., Langlois, T. J., Malseed, B. E., et al. (2018). Fish and habitats on wellhead infrastructure on the north west shelf of Western Australia. Cont. Shelf Res. 164, 10–27. doi: 10.1016/j.csr.2018.05.007
McLean, D., Cure, K., Abdul Wahab, M. A., Galaiduk, R., Birt, M., Vaughan, B., et al. (2021). A comparison of marine communities along a subsea pipeline with those in surrounding seabed areas. Cont. Shelf Res. 219:104394. doi: 10.1016/j.csr.2021.104394
Melbourne-Thomas, J., Audzijonyte, A., Brasier, M. J., Cresswell, K. A., Fogarty, H. E., Haward, M., et al. (2021). Poleward bound: adapting to climate-driven species redistribution. Rev. Fish Biol. Fish. doi: 10.1007/s11160-021-09641-3 Online ahead of print,
Melchers, R. E. (2006). Examples of mathematical modelling of long term general corrosion of structural steels in sea water. Corros. Eng. Sci. Technol. 41, 38–44. doi: 10.1179/174327806x93992
Meyer-Gutbrod, E. L., Kui, L., Nishimoto, M. M., Love, M. S., Schroeder, D. M., and Miller, R. J. (2019a). Fish densities associated with structural elements of oil and gas platforms in southern California. Bull. Mar. Sci. 95, 639–656. doi: 10.5343/bms.2018.0078
Meyer-Gutbrod, E. L., Love, M. S., Claisse, J. T., Page, H. M., Schroeder, D. M., and Miller, R. J. (2019b). Decommissioning impacts on biotic assemblages associated with shell mounds beneath southern California offshore oil and gas platforms. Bull. Mar. Sci. 95, 683–701. doi: 10.5343/bms.2018.0077
Meyer-Gutbrod, E. L., Love, M. S., Schroeder, D. M., Claisse, J. T., Kui, L., and Miller, R. J. (2020). Forecasting the legacy of offshore oil and gas platforms on fish community structure and productivity. Ecol. Appl. 30:e02185.
Moffat, K., and Zhang, A. (2014). The paths to social licence to operate: an integrative model explaining community acceptance of mining. Resour. Policy 39, 61–70. doi: 10.1016/j.resourpol.2013.11.003
Moffat, K., Lacey, J., Zhang, A., and Leipold, S. (2016). The social licence to operate: a critical review. Forestry 89, 477–488. doi: 10.1093/forestry/cpv044
Montagna, P. A., and Harper, D. E. (1996). Benthic infaunal long term response to offshore production platforms in the Gulf of Mexico. Can. J. Fish. Aquat. Sci. 53, 2567–2588. doi: 10.1139/f96-215
Montagna, P., Jarvis, S. C., and Kennicutt, M. C. (2002). Distinguishing between contaminant and reef effects on meiofauna near offshore hydrocarbon platforms in the Gulf of Mexico. Can. J. Fish. Aquat. Sci. 59, 1584–1592. doi: 10.1139/f02-131
National Energy Resources Australia [NERA] (2017). Sector Competitiveness Plan 2017. Australia: NERA.
National Energy Resources Australia [NERA] (2019). National Decommissioning Research Initiative (NDRI) Background. Australia: NERA.
National Offshore Petroleum Safety and Environmental Management Authority [NOPSEMA] (2019a). Annual Report 2018-19. Perth, WA: NOPSEMA.
National Offshore Petroleum Safety and Environmental Management Authority [NOPSEMA] (2019b). Environmental Plan Decision Making. Document Number N-04750-GL1721 Revision 6. Perth, WA: NOPSEMA.
National Offshore Petroleum Safety and Environmental Management Authority [NOPSEMA] (2019c). Introducing NOPSEMA. Perth, WA: NOPSEMA.
National Offshore Petroleum Safety and Environmental Management Authority [NOPSEMA] (2020a). Environmental Plan Content Requirement. Document Number N-04750-GN1344 A339814. Perth, WA: NOPSEMA.
National Offshore Petroleum Safety and Environmental Management Authority [NOPSEMA] (2020b). Section 572 Maintenance and Removal of Property. Document Number: N-00500-PL1903 A720369. Perth, WA: NOPSEMA.
National Offshore Petroleum Safety and Environmental Management Authority [NOPSEMA] (2021). Decommissioning Compliance Strategy 2021-2025. Perth, WA: NOPSEMA.
National Research Council (1996). Understanding Risk: Informing Decisions in a Democratic Society. Washington, DC: The National Academies Press. doi: 10.17226/5138
Neff, J., Lee, K., and DeBlois, E. M. (2011). “Produced water: overview of composition, fates, and effects,” in Produced Water: Environmental Risks and Advances in Mitigation Technologies, eds K. Lee and J. Neff (New York, NY: Springer), 3–54. doi: 10.1007/978-1-4614-0046-2_1
Newton, A., and Elliott, M. (2016). A Typology of stakeholders and guidelines for engagement in transdisciplinary, participatory processes. Front. Mar. Sci. 3:230. doi: 10.3389/fmars.2016.00230
Nguyen, T. T., Cochrane, S. K. J., and Landfald, B. (2018). Perturbation of seafloor bacterial community structure by drilling waste discharge. Mar. Pollut. Bull. 129, 615–622. doi: 10.1016/j.marpolbul.2017.10.039
Nicolette, J., Travers, M., and Price, D. (2014). “Utilizing a net environmental benefit analysis approach to support the selection of offshore decommissioning alternatives,” in Proceedings of the 21st International Petroleum Environmental Conference, (Houston, TX).
Nishimoto, M. M., Simons, R. D., and Love, M. S. (2019). Offshore oil production platforms as potential sources of larvae to coastal shelf regions off southern California. Bull. Mar. Sci. 95, 535–558. doi: 10.5343/bms.2019.0033
Norström, A. V., Cvitanovic, C., Löf, M. F., West, S., Wyborn, C., Balvanera, P., et al. (2020). Principles for knowledge co-production in sustainability research. Nat. Sustain. 3, 182–190.
Olsvik, P. A., Berntssen, M. H. G., Hylland, K., Eriksen, D. O., and Nolen, E. (2012). Low impact of exposure to environmentally relevant doses of Ra-226 in Atlantic cod (Gadus morhua) embryonic cells. J. Environ. Radioact. 109, 84–93. doi: 10.1016/j.jenvrad.2012.02.003
Page, H. (2019). Distribution and potential larval connectivity of the non-native Watersipora (Bryozoa) among harbors, offshore oil platforms, and natural reefs. Aquat. Invasions 14, 615–637. doi: 10.3391/ai.2019.14.4.04
Page, H. M., Dugan, J. E., Culver, C. S., and Hoesterey, J. C. (2006). Exotic invertebrate species on offshore oil platforms. Mar. Ecol. Prog. Ser. 325, 101–107. doi: 10.3354/meps325101
Page, H. M., Dugan, J., and Childress, J. (2005). Role of Food Subsidies and Habitat Structure in Influencing Benthic Communities of Shell Mounds at Sites of Existing and Former Offshore oil Platforms. US Department of the Interior, Minerals Management Service, Pacific OCS Study 2005- 001. Coastal Research Center, Marine Science Institute, University of California, Santa Barbara, California. MMS Cooperative Agreement Number 14-35-0001-31063. Santa Barbara, CA: Marine Science Institute, 32.
Palandro, D., and Azivy, A. (2018). Overview of decommissioning option assessment: a case for comparative assessment. Paper Presented at the SPE Symposium: Decommissioning and Abandonment, Kuala Lumpur, Malaysia, December 2018, Kuala Lumpur. doi: 10.2118/193991-MS
Palumbi, S. R. (2003). Population genetics, demographic connectivity, and the design of marine reserves. Ecol. Appl. 13, S146–S158.
Parkerton, T. F., Bok, M., Ireland, A. W., and Prosser, C. M. (2018). An evaluation of cumulative risks from offshore produced water discharges in the Bass Strait. Mar. Pollut. Bull. 126, 610–621. doi: 10.1016/j.marpolbul.2017.10.003
Pecl, G. T., Araújo, M. B., Bell, J. D., Blanchard, J., Bonebrake, T. C., Chen, I.-C., et al. (2017). The universal impacts of species on the move. Science 355:eaa19214.
Piola, R. F., Dafforn, K. A., and Johnston, E. L. (2009). The influence of antifouling practices on marine invasions. Biofouling 25, 633–644. doi: 10.1080/08927010903063065
Podar, M., Gilmour, C. C., Brandt, C. C., Soren, A., Brown, S. D., Crable, B. R., et al. (2015). Global prevalence and distribution of genes and microorganisms involved in mercury methylation. Sci. Adv. 1:e1500675. doi: 10.1126/sciadv.1500675
Pondella, D. J., Zahn, L. A., Love, M. S., Siegel, D., and Bernstein, B. B. (2015). Modeling fish production for Southern California’s petroleum platforms. Integr. Environ. Assess. Manag. 11, 584–593. doi: 10.1002/ieam.1689
Pradella, N., Fowler, A. M., Booth, D. J., and Macreadie, P. I. (2014). Fish assemblages associated with oil industry structures on the continental shelf of north-western Australia. J. Fish Biol. 84, 247–255. doi: 10.1111/jfb.12274
Productivity Commission (2016). Marine Fisheries and Aquaculture, Final Report. Canberra: Productivity Commission.
Purcell, J. E. (2005). Climate effects on formation of jellyfish and ctenophore blooms: a review. J. Mar. Biol. Ass. U.K. 85, 461–476. doi: 10.1017/s0025315405011409
Raitt, P., Selman, A., and Lanoëlle, C. (2019). Engineering and environmental studies for decommissioning of subsea infrastructure. APPEA J. 59, 277–288.
Raum, S. (2018). A framework for integrating systematic stakeholder analysis in ecosystem services research: stakeholder mapping for forest ecosystem services in the UK. Ecosyst. Serv. 29, 170–184. doi: 10.1016/j.ecoser.2018.01.001
Reggio, V. C. Jr. (1987). Rigs to Reefs: The Use of Obsolete Petroleum Structures as Artificial Reefs. OCSReport/MMS 87-0015. Metairie, LA: Minerals Management Service, 17.
Regoli, F., d’Errico, G., Nardi, A., Mezzelani, M., Fattorini, D., Benedetti, M., et al. (2019). Application of a weight of evidence approach for monitoring complex environmental scenarios: the case-study of off-shore platforms. Front. Mar. Sci. 6:377. doi: 10.3389/fmars.2019.00377
Renn, O. (2008). Risk Governance: Coping with Uncertainty in a Complex World. London: Routledge, 368.
Ridgway, K. R., and Godfrey, J. S. (2015). The source of the leeuwin current seasonality. J. Geophys. Res. 120, 6843–6864.
Rohal, M., Barrera, N., Escobar-Briones, E., Brooks, G., Hollander, D., Larson, R., et al. (2020). How quickly will the offshore ecosystem recover from the 2010 Deepwater Horizon oil spill? Lessons learned from the 1979 Ixtoc-1 oil well blowout. Ecol. Indic. 117:106593. doi: 10.1016/j.ecolind.2020.106593
Roos, P. (2019). Policy Change in Offshore Decommissioning Governance: Dealing with Environmental Politics and Coping with Ecological Uncertainty. Masters Thesis. Wageningen: Wageningen University.
Russell, D. J. F., Brasseur, S. M. J. M., Thompson, D., Hastie, G. D., Janik, V. M., Aarts, G., et al. (2014). Marie mammals trace anthropogenic structures at sea. Curr. Biol. 24, R638–R639.
Schläppy, M.-L., Robinson, L., Camilieri-Asc, V., and Miller, K. (2021). Trash or treasure? Considerations for future research on oil and gas decommissioning research. Front. Mar. Sci. 8:642539. doi: 10.3389/fmars.2021.642539
Schlenk, D., Celander, M., Gallagher, E. P., George, S., James, M., Kullman, S. W., et al. (2008). “Biotransformation in fishes,” in The Toxicology of Fishes, eds R. T. Di Giulio and D. E. Hinton (Boca Raton, FL: CRC Press).
Schramm, K. D., Marne, M. J., Elsdon, T. S., Jones, C. M., Saunders, B. J., Newman, S. J., et al. (2021). Fish associations with shallow water subsea pipelines compared to surrounding reefs and soft sediment habitats. Sci. Rep. 11:6238. doi: 10.1038/s41598-021-85396-y
Schroeder, D. M., and Love, M. S. (2004). Ecological and political issues surrounding decommissioning of offshore oil facilities in the Southern California Bight. Ocean Coastal Manag. 47, 21–48. doi: 10.1016/j.ocecoaman.2004.03.002
Schulze, A., Erdner, D. L., Grimes, C. J., Holstein, D. M., and Miglietta, M. P. (2020). Artificial reefs in the Northern Gulf of Mexico: community ecology amid the “Ocean Sprawl”. Front. Mar. Sci. 7:447. doi: 10.3389/fmars.2020.00447
Sevillano-Morales, J. S., Cejudo-Gomez, M., Ramirez-Ojeda, A. M., Martos, F. C., and Moreno-Rojas, R. (2015). Risk profile of methylmercury in seafood. Curr. Opin. Food Sci. 6, 53–60. doi: 10.1016/j.cofs.2016.01.003
Shaw, J. L., Seares, P., and Newman, S. J. (2018). Decommissioning Offshore Infrastructure: A Review of Stakeholder Views and Science Priorities. Perth, WA: WAMSI, 74.
Simpson, S. L., and Batley, G. E. (2016). Sediment Quality Assessment: A Practical Guide. Melbourne: CSIRO Publishing, 359.
Smith, J. A., Lowry, M. B., and Suthers, I. M. (2015). Fish attraction to artificial reefs not always harmful: a simulation study. Ecol. Evol. 5, 4590–4602. doi: 10.1002/ece3.1730
Sol, S. Y., Johnson, L. L., Horness, B. H., and Collier, T. K. (2000). Relationship between oil exposure and reproductive parameters in fish collected following the Exxon Valdez oil spill. Mar. Pollut. Bull. 40, 1139–1147. doi: 10.1016/s0025-326x(00)00074-6
Sommer, B., Fowler, A. M., Macreadie, P. I., Palandro, D. A., Aziz, A. C., and Booth, D. J. (2019). Decommissioning of offshore oil and gas structures - environmental opportunities and challenges. Sci. Total Environ. 658, 973–981. doi: 10.1016/j.scitotenv.2018.12.193
Strain, E. M. A., Steinberg, P. D., Vozzo, M., Johnston, E. L., Abbiati, M., Aguilera, M. A., et al. (2021). A global analysis of complexity-biodiversity relationships on marine artificial structures. Glob. Ecol. Biogeogr. 30, 140–153.
Terlizzi, A., Bevilacqua, S., Scuderi, D., Fiorentino, D., Guarnieri, G., Giangrande, A., et al. (2008). Effects of offshore platforms on soft-bottom macro-benthic assemblages: a case study in a Mediterranean gas field. Mar. Pollut. Bull. 56, 1303–1309. doi: 10.1016/j.marpolbul.2008.04.024
Thomson, P. G., Fowler, A. M., Davis, A. R., Pattiaratchi, C., and Booth, D. J. (2018). Some old movies become classics - a case study determining the scientific value of ROV inspection footage on a platform on Australia’s North West Shelf. Front. Mar. Sci. 5:471.
Twatchman Snyder Byrd Inc (2003). Comparative Health and Safety Risk Assessment of Decommissioning Large Offshore Platforms. Study for the US Department of Interior, Minerals Management Service. TSB Project 23021. Houston, TX: Twatchman Snyder and Byrd Inc.
Uffman-Kirsch, L. B., Richardson, B. J., and van Putten, E. I. (2020). A New paradigm for social licenceas a path to marine sustainability. Front. Mar. Sci. 7:571373. doi: 10.3389/fmars.2020.571373
United States Environmental Protection Agency [USEPA] (1998). Guidelines for Environmental Risk Assessment. EPA/630/R-95/002F. Washington, DC: USEPA, 188.
Van Putten, I. E., Cvitanovic, C., Fulton, E., Lacey, J., and Kelly, R. (2018). The emergence of social licence necessitates reforms in environmental regulation. Ecol. Soc. 23:24. doi: 10.5751/ES-10397-230324
Villareal, T. A., Hanson, S., Qualia, S., Jester, E. L. E., Granade, H. R., and Dickey, R. W. (2007). Petroleum prouction platforms as sites for expansion of ciguatera in the northwestern Gulf of Mexico. Harmful Algae 6, 253–259. doi: 10.1016/j.hal.2006.08.008
Vodopivec, M., Peliz, ÁJ., and Malej, A. (2017). Offshore marine constructions as propagators of moon jellyfish dispersal. Environ. Res. Lett. 12:084003. doi: 10.1088/1748-9326/aa75d9
Wells, F. E. (2018). A low number of invasive marine species in the tropics: a case study from Pilbara (Western Australia). Manag. Biol. Invasions 9, 227–237. doi: 10.3391/mbi.2018.9.3.05
Wells, F. E., Tan, K. S., Todd, P. A., Jaafar, Z., and Yeo, D. C. J. (2019). A low number of introduced marine species in the tropics: a case study from Singapore. Manag. Biol. Invasions 10, 23–45. doi: 10.3391/mbi.2019.10.1.03
West, L. D., Stark, K. E., Murphy, J. J., Lyle, J. M., and Ochwada-Doyle, F. A. (2015). Survey of Recreational Fishing in New South Wales and the ACT 2013/14, Fisheries Final Report Series No. 149. Wollongong, NSW: NSW Department of Primary Industries.
Whyte, J. J., Jung, R. E., Schmitt, C. J., and Tillitt, D. E. (2000). Ethoxyresorufin-O-deethylase (EROD) activity in fish as a biomarker of chemical exposure. Crit. Rev. Toxicol. 30, 347–570. doi: 10.1080/10408440091159239
Wilson, M. J., Frickel, S., Nguyen, D., Bui, T., Echsner, S., Simon, B. R., et al. (2015). A targeted health risk assessment following the Deepwater Horizon Oil Spill: polycyclic aromatic hydrocarbon exposure in Vietnamese-American shrimp consumers. Environ. Health Perspect. 123, 152–159. doi: 10.1289/ehp.1408684
Keywords: oil and gas decommissioning, Australia, decision-making, productivity, connectivity, contaminants, social license
Citation: Melbourne-Thomas J, Hayes KR, Hobday AJ, Little LR, Strzelecki J, Thomson DP, van Putten I and Hook SE (2021) Decommissioning Research Needs for Offshore Oil and Gas Infrastructure in Australia. Front. Mar. Sci. 8:711151. doi: 10.3389/fmars.2021.711151
Received: 18 May 2021; Accepted: 05 July 2021;
Published: 29 July 2021.
Edited by:
Allyson O’Brien, The University of Melbourne, AustraliaReviewed by:
Erin L. Meyer-Gutbrod, University of South Carolina, United StatesTerry Whitledge, University of Alaska Fairbanks, United States
Copyright © 2021 Melbourne-Thomas, Hayes, Hobday, Little, Strzelecki, Thomson, van Putten and Hook. This is an open-access article distributed under the terms of the Creative Commons Attribution License (CC BY). The use, distribution or reproduction in other forums is permitted, provided the original author(s) and the copyright owner(s) are credited and that the original publication in this journal is cited, in accordance with accepted academic practice. No use, distribution or reproduction is permitted which does not comply with these terms.
*Correspondence: Jess Melbourne-Thomas, amVzcy5tZWxib3VybmUtdGhvbWFzQGNzaXJvLmF1