- 1Department of Biology, United Arab Emirates University, Al-Ain, United Arab Emirates
- 2Harry Butler Institute, Murdoch University, Murdoch, WA, Australia
- 3Department of Anatomy, College of Medicine and Health Sciences, United Arab Emirates University, Al-Ain, United Arab Emirates
- 4Department of Nutrition and Health, College of Medicine and Health Sciences, United Arab Emirates University, Al-Ain, United Arab Emirates
- 5Department of Biological Sciences, Faculty of Science, Yarmouk University, Irbid, Jordan
Mangrove forests in the Arabian Gulf are under continuous threat. To increase plantations of gray mangrove (Avicennia marina) in the United Arab Emirates (UAE), 27 endophytic actinobacterial isolates obtained from mangrove roots were in vitro pre-screened to detect the polyamines (PAs) [putrescine (Put), spermidine (Spd), and spermine (Spm)]. We also determined the abilities of the endophytic PA-producing actinobacterial isolates in enhancing the growth of A. marina under greenhouse conditions. Although three highly PA-producing isolates were recovered from inside mangrove root tissues, Streptomyces mutabilis UAE1 constantly colonized root and stem inner tissues for 12 weeks, suggesting an endophytic association between this actinobacterial isolate and mangrove seedlings. When roots were inoculated with S. mutabilis, mangrove growth was remarkably enhanced under gnotobiotic and greenhouse conditions. This was evident from the significant (P < 0.05) increases in dry weight and length of root (66.7 and 65.5%, respectively) and shoot (64.8 and 58.0%, respectively), number of branches (64.3%), total leaf area (40.2%), and photosynthetic pigments (54.5% chlorophyll a; 40.0% chlorophyll b; and 53.1% carotenoids) of mangrove compared to the PA-non-producing Streptomyces sp. or control treatment. Growth promotion in plants treated with S. mutabilis was also supported by significant (P < 0.05) elevations in the contents of mangrove in planta PAs, auxins, and cytokinins, accompanied by a decrease in abscisic acid levels. No difference was, however, detected in growth and amounts of PAs or any plant growth regulators (PGRs) in plants treated with or without the PA-non-producing isolate. Our findings indicate that plant growth parameters can be enhanced as a consequence of secretion of Put, Spd, and Spm by S. mutabilis resulting in regulation of endogenous PAs and other PGRs in mangrove tissues. This study is the first record that aims to improve sustainable agricultural management practices using plant growth promoting (PGP) actinobacteria, endophytic in mangrove tissues to promote mangrove growth under greenhouse conditions. Such research may allow this region to be a model to study the synergistic S. mutabilis–mangrove interactions and the future impacts on mangrove reforestation in the Arabian Gulf and elsewhere where mangrove forests are in threat.
Introduction
Mangroves are assemblages of salt-tolerant trees and shrubs that grow in intertidal regions of tropical and subtropical coastlines (Giri et al., 2011; Tregarot et al., 2021). Mangroves are adapted to survive hostile environments because of their specialized roots, called pneumatophores, that enable gaseous exchange and growth in deep, acidic, and anaerobic soils (Howari et al., 2009). There are approximately 84 mangrove species worldwide, of which only two species can grow in the Arabian Gulf (Almahasheer, 2018; Elmahdy et al., 2020). True mangrove (Rhizophora mucronata) and gray mangrove (Avicennia marina) can be found in Iran (Zahed et al., 2010), whereas gray mangrove is the dominant species in the Arabian Gulf countries (Burt, 2014; Elmahdy et al., 2020).
Mangroves play a critical role in supporting human well-being and providing habitat for marine organisms (Tregarot et al., 2021). In addition to acting as intense carbon (C) sinks (Donato et al., 2011), mangrove forests stabilize shorelines, protect lands, and prevent erosions (Ahmed et al., 2021). Despite their importance, mangrove forests are globally under threat (Alongi, 2002); particularly Iran and the Arabian Gulf countries including Kuwait, Bahrain, Qatar, Saudi Arabia, and the United Arab Emirates (UAE; Almahasheer, 2018; Elmahdy et al., 2020). Mangrove conservation has led the UAE to become the largest mangrove-growing country in the Arabian Gulf, covering almost 48% of the overall mangroves in this region. This is the best “fruit” of a successful plantation program undertaken by the Emirate of Abu Dhabi-UAE that was initiated 20–30 years ago.
Endophytes, microorganisms that naturally reside in tissues of living plants, are potential sources of novel natural products for exploitation in agriculture, industry, and medicine (Hallmann et al., 1997; El-Tarabily et al., 2009, 2019). Bacterial endophytes confer profound impacts on their host plants through promoting growth, increasing tolerance to pathogens, and improving phytoremediation (Ma et al., 2016; Christakis et al., 2021; Faria et al., 2021). In addition, they have the ability to produce a plethora of bioactive secondary metabolites to protect plants against environmental stresses (Khare et al., 2018; Kushwaha et al., 2020).
Plant growth promoting (PGP) bacteria (PGPB) are capable to stimulate growth via an array of mechanisms that are broadly classified as direct and indirect mechanisms, known as plant growth promoters and biological control agents (BCAs), respectively (Olanrewaju et al., 2017; Moretti et al., 2020). Direct mechanisms may enhance growth through fixation of nitrogen (N), solubilization of phosphorus (P), sequestration of iron (Fe), and production of plant growth regulators (PGRs), such as auxins, cytokinins (CKs), gibberellins (GA3), ethylene (ET), and abscisic acid (ABA) (Olanrewaju et al., 2017; Gouda et al., 2018). Indirect growth promotion occurs when PGPB reduce the deleterious effects of pathogens on plant growth (Khare et al., 2018; Bektas and Kusek, 2021; Christakis et al., 2021).
In response to environmental stresses, plants produce high levels of ET, which often inhibits plant growth (Glick, 2015; Sham et al., 2019). Many PGPB may stimulate plant growth through the activity of 1-aminocyclopropane-1-carboxylic acid (ACC) deaminase (ACCD) that lowers ACC levels and, hence, ET amounts by breaking ACC to ammonia (NH3) and α-ketobutyrate (Glick, 2015; Olanrewaju et al., 2017; Acuna et al., 2019). Accumulation of polyamines (PAs) can also trigger molecular, biochemical, and physiological responses that promote stress tolerance and increase crop yield (El-Tarabily et al., 2020).
Polyamines are low-molecular-weight aliphatic nitrogenous bases containing two or more amino groups that have potent biological activities (Xu et al., 2009; Alcázar et al., 2020). Chen et al. (2019) have shown the relationship between PAs and flowering time, embryo development, and senescence. Moreover, PAs can mediate the hormonal effects or act independently as signaling molecules in response to environmental stresses (Wu et al., 2018). Exogenous applications of PAs, mainly putrescine (Put), spermidine (Spd), and spermine (Spm), protect plants against damages caused by biotic and abiotic stresses (Handa et al., 2010; Chen et al., 2019).
To conserve mangrove ecosystems in the Arabian Gulf, maintenance and restoration of microbial communities are highly recommended (Allard et al., 2020). In the last few decades, there has been a growing interest in enhancing growth and improving production in crops by using PGPB that produce PAs (Chen et al., 2019; Alcázar et al., 2020). The rhizosphere-competent strains, Actinoplanes deccanensis and Streptomyces euryhalinus, are two marine PA-synthesizing PGP actinobacteria (PGPA) that promote growth and seed yields of the halotolerant plant Salicornia bigelovii in the UAE (El-Tarabily et al., 2020).
In the field of marine agriculture, most research on growth promotion of mangrove has concentrated on PGPB from the rhizosphere (Bashan and Holguin, 2002; Kathiresan and Selvam, 2006; El-Tarabily and Youssef, 2010, 2011). Increasingly, endophytic bacteria have great attributes because they are less exposed to inhospitable environments of the soil and are located in living tissues where relevant activities occur (El-Tarabily et al., 2009, 2019), thus making them ideal for the next generation of PGP agents (Preyanga et al., 2021). Except of one report on endophytic PGPA to promote S. bigelovii growth under greenhouse conditions (El-Tarabily et al., 2019), the effect of applications of endophytic bacteria or fungi to promote growth of mangrove or other marine plants in the greenhouse/nursery/field is mostly negligible.
To the best of our knowledge, no earlier studies have addressed the effect of endophytic PGPA to stimulate growth of mangrove seedlings through production of PAs under greenhouse, naturally competitive nursery, or field environments using seawater irrigation. Here, actinobacteria were targeted because these filamentous bacteria are more adapted to survive harsh desert environments of the UAE (Goodfellow and Williams, 1983; El-Tarabily et al., 2020). The overall objective of the current study was to promote growth of gray mangrove by PA-producing endophytic PGPA. The specific aims of this investigation were to: (i) isolate endophytic actinobacteria from mangrove roots capable of producing PAs; (ii) evaluate the most efficient isolate producing PAs and internally colonizing plant tissues (roots and stems) for mangrove growth promotion under gnotobiotic conditions; and (iii) determine the effects of inoculation of PA-producing endophytic PGPA isolate on mangrove performance in the greenhouse. We also assessed different agronomic parameters, photosynthetic pigment contents, and endogenous PGR levels in tissues that were associated with growth promotion of mangrove.
Materials and Methods
Study Site and Collection of Mangrove Propagules
Viviparous propagules obtained from mother trees or freshly fallen propagules of gray mangrove (A. marina) were collected from the east coast of Abu-Dhabi-UAE (24° 26′ 48.5″ N; 54° 26′ 40.6″ E). Seed capsules (propagules) of almost similar dimensions were washed with water, followed by surface-sterilization using 70% alcohol and then 1.05% Clorox (20% household bleach). Surface-sterilized propagules were then washed 10 times with 0.22 μm membrane filter-sterilized (Millipore Corporation, MA, United States) full-strength seawater (salinity of 40) and air-dried for 30 min in laminar air flow cabinet.
Sediment Characteristics
The dark grayish-black sediment used in the current study was collected from the location described in Section “Study Site and Collection of Mangrove Propagules.” The chemical characteristics of the sediment were: Electrical conductivity = 5.81 dSm– 1; pH = 8.36 (in 0.01 M CaCl2); and organic C = 6.24%. Nutrients (mg kg– 1 sediment) such as available P = 8.83, total P = 85, N as nitrate = 4 and ammonium = 6.4, bicarbonate extractable potassium (K) = 241, oxalate extractable amorphous Fe = 331, and sulfate = 414 were also detected.
Isolation of Endophytic Actinobacteria From Mangrove Roots
All microbiological media in the present study were prepared using Millipore membrane filter-sterilized full-strength seawater. Surface-sterilized mangrove propagules (section “Study Site and Collection of Mangrove Propagules”) were sown in plastic pots (23 cm diameter × 17 cm depth) containing sediment. Pots were maintained in a greenhouse (average daily photosynthetic photon flux density of 700 ± 150 μmol m– 2 s– 1; temperature of 25 ± 2°C; and relative humidity of 60 ± 5%) and watered daily with full strength seawater. Eight pots were prepared with two propagules per pot.
After 4 weeks, seedlings were collected and transferred to the laboratory in coolers for immediate processing, and roots were cut and washed. Fresh root weight was recorded prior to further processing. Roots were soaked in sterile phosphate-buffered saline (PBS) solution, pH 7.0 for 10 min (Rennie et al., 1982). Roots were then surface-disinfested as described by Sardi et al. (1992). Sterility checks were carried out for each sample to monitor the effectiveness of the disinfestation procedures (Hallmann et al., 1997; Sturz et al., 1998).
Roots were macerated as described by Hallmann et al. (1997). After filtering the slurry through sterile cotton cloth, the filtrate was serially diluted (10– 2, 10– 3, and 10– 4) and aliquots (0.2 ml) were spread with a sterile glass rod over the surface of inorganic salt starch agar (ISSA; Küster, 1959) amended with 50 μg ml– 1 of each nystatin and cycloheximide (Sigma-Aldrich Chemie GmbH, Germany). Three replicated plates for each root sample dilution were dried in a laminar flow-cabinet for 15 min before incubation at 28 ± 2°C in dark for 7 days (El-Tarabily et al., 2019). Population density (PD) of endophytic actinobacteria was calculated as log10 colony-forming units (cfu) g– 1 fresh root weight (Hallmann et al., 1997). All colonies were purified on oatmeal agar plates supplemented with 0.1% yeast extract (OMYEA; ISP medium 3; Shirling and Gottlieb, 1966). Both streptomycete and non-streptomycete actinobacteria (SA and NSA, respectively) were identified based on morphological and cultural characteristics according to Cross (1989). Hyphae and spores of all isolates were stored in 20% glycerol at −70°C (Wellington and Williams, 1977).
To determine salt tolerance of isolates, SA and NSA were streaked in triplicates on ISSA medium supplemented with NaCl concentrations up to 80 g l– 1 (8%), and incubated at 28°C in dark for 7 days (Williams et al., 1972). Isolates with strong growth and heavy sporulation on ISSA supplemented with 8% NaCl indicated high salt tolerance, and were further chosen for subsequent experiments.
Qualitative and Quantitative Determination of PAs Produced by Endophytic Isolates
Endophytic actinobacterial isolates were tested for production of Put in Moeller’s decarboxylase agar medium (MDAM) amended with 2 g l– 1 of L-arginine-monohydrochloride (Sigma-Aldrich) and phenol red (Sigma-Aldrich) (Arena and Manca de Nadra, 2001). Plates were incubated at 28°C in dark for 2 days (El-Tarabily et al., 2020) and dark red halo found beneath and around colonies indicated Put production by the decarboxylating isolates.
Positive actinobacterial isolates obtained from the qualitative test were further evaluated for production of Put, Spd, and Spm in Moeller’s decarboxylase broth medium (MDBM) supplemented with 2 g l– 1 L-arginine-monohydrochloride (Arena and Manca de Nadra, 2001) by using reverse-phase high-performance liquid chromatography (HPLC; SpectraLab Scientific Inc., ON, Canada) (Marino et al., 2000). HPLC chromatograms (eight replicates per isolate) were produced by injecting 10 μl aliquot of the sample onto a 10-μm μBondapak C18 column in HPLC (Waters Associates) equipped with a 254-nm UV detector (Smith and Davies, 1985).
In vitro Assessment of PGP Activities by PA-Producing Endophytic Isolates
To detect indole-3-acetic acid (IAA), flasks containing 50 ml inorganic salt starch broth (ISSB; Küster, 1959) supplied with 5 ml of 5% filter-sterilized L-tryptophan (Sigma-Aldrich) (Khalid et al., 2004) were inoculated with 2 ml of each isolate (108 cfu ml– 1). After 7 days of incubation on a 250 rpm orbital shaker incubator in dark (El-Tarabily et al., 2019), suspensions were centrifuged (12,000 × g), and supernatants were collected and 4 ml of Salkowski reagent was added (Gordon and Weber, 1951). IAA equivalents (μg ml– 1) were quantitatively determined at 530 nm using spectrophotometer (UV-2101/3101 PC; Shimadzu Corporation, Analytical Instruments Division, Kyoto, Japan).
Isolates were also grown in glucose peptone broth (di Menna, 1957) supplemented with 5 ml of 5% L-tryptophan to detect IAA and indole-3-pyruvic acid (IPYA), and on medium developed by Strzelczyk and Pokojska-Burdziej (1984) to detect GA3 and CKs [isopentenyl adenine (iPa), isopentenyl adenoside (iPA), and zeatin (Z)] using HPLC (Tien et al., 1979). After 10 days, extraction of PGRs from concentrated filter-sterilized cell-free broth and HPLC parameters were used to determine the concentrations of IAA, IPYA, GA3, iPa, iPA, and Z according to Tien et al. (1979).
To screen for ACCD production, isolates were grown on N-free Dworkin and Foster’s salts minimal agar medium (DF) plates (Dworkin and Foster, 1958) amended with either ACC (3 mM; Sigma-Aldrich) or (NH4)2SO4 (2 g; control). Strains were streaked on media and kept incubated at 28°C for 7 days (El-Tarabily et al., 2019). Growth and heavy sporulation on plates indicated positive for ACCD production. ACCD activity was quantified by monitoring the amount of α keto-butyrate as described by Honma and Shimomura (1978). Protein concentrations were determined as described by Bradford (1976).
Phosphate-solubilizing ability was analyzed using Pikovskaya’s agar medium (Pikovskaya, 1948) amended with bromophenol blue as an indicator in which tricalcium phosphate was replaced with insoluble rock phosphate. The disappearance of the blue color and production of clear zone beneath culture was an indicator of P-solubilization. The drop of pH and the amount of released soluble P (Murphy and Riley, 1962) in modified National Botanical Research Institute’s phosphate broth (Nautiyal, 1999) were taken as an index for the efficiency of the strains to solubilize P.
To measure nitrogenase activity and NH3 production, acetylene-reduction assays (Dye, 1962) and Nessler’s reagent (Holguin et al., 1992) were used, respectively. For siderophores production, plates of chrome azurol S agar (Schwyn and Neilands, 1987) were inoculated with isolates and incubated for 3 days at 28 ± 2°C in dark. As siderophore producers, actinobacterial isolates developed yellow-orange halo zone around the colony. Eight independent replicates for each strain were used in all these in vitro assays.
Assessment of PGP Parameters Under Gnotobiotic Conditions
To evaluate PGP abilities of isolates under gnotobiotic conditions, surface-sterilized mangrove propagules were sown in plastic pots containing sediment. Pots were maintained in a greenhouse (section “Isolation of Endophytic Actinobacteria From Mangrove Roots”) for 10 days and watered daily with full strength seawater. After 10 days, the pruned-root dip method (Musson et al., 1995) was used to introduce endophytic actinobacterial isolates inside the young seedlings. Root tips (3 mm) were trimmed using a sterilized scalpel to facilitate uptaking the actinobacterial inoculum. Young seedlings were placed in sterile plastic cups for 3 h at 25°C with their roots in direct contact with the inoculum suspension of each isolate (108 cfu ml– 1).
Mangrove seedlings with or without the actinobacterial inoculum were then aseptically planted into glass tubes (300 × 35 mm in diameter) filled with sediment and moistened with seawater. Seedlings, serving as control treatment, were treated with autoclaved ISSB.
Seedlings were daily irrigated with full strength sterilized seawater and maintained in a growth chamber (16/8-h day of 180–200 μmol m– 2 s– 1 fluorescent light/dark; 25/20°C light/dark temperature cycle). Plants were harvested after 6 weeks of transplantation, washed, and separated into roots and shoots. Measurements of dry weight (DW; g) and length (cm) of shoot and root tissues were determined. Each treatment was independently replicated eight times with one seedling in each replicate.
Quantification of Internal Colonization by PA-Producing Actintobacterial Isolates
Mutants resistant to rifampicin of the three promising PA-producing isolates, along with the positive control PA-non-producing isolate, were selected on ISSA medium supplemented with rifampicin (100 μg ml– 1; Sigma-Aldrich) and tested according to Misaghi and Donndelinger (1990). These mutants were also compared to the corresponding wild-type strains for PA production. Morphological features, growth rates, and PA production of mutants were similar to those of parental strains.
For the gnotobiotic (section “Assessment of PGP Parameters Under Gnotobiotic Conditions”) and greenhouse (section “In vivo Assessment of Growth Promotion and Endogenous PGRs in the Greenhouse”) experiments, 4 ml aliquots of 20% glycerol suspension of the four endophytes were individually inoculated into 250-ml ISSB and shaken at 250 rpm on an orbital shaker incubator for 5 days. Cells were centrifuged (12,000 × g) at 20°C for 15 min, and the pellet was suspended in 10 ml PBS and re-centrifuged (El-Tarabily et al., 2019). For each suspension, 0.1 ml of each 10– 3, 10– 4, 10– 5, and 10– 6 dilutions was made in PBS and spread on ISSA. After 5 days of incubation, a final concentration of ∼108 cfu ml– 1 of each isolate was used as an inoculum.
To assess colonization of internal root and stem tissues, the pruned-root dip method (section “Assessment of PGP Parameters Under Gnotobiotic Conditions”) was used to inoculate 10-day-old mangrove seedlings with individual isolates. Free draining pots (36-cm in diameter), filled with 14 kg of sediments collected from the area described in Section “Study Site and Collection of Mangrove Propagules,” were placed in a greenhouse (section “Isolation of Endophytic Actinobacteria From Mangrove Roots”) where seedlings were watered daily with full strength seawater to container capacity. After planting, roots and stems were sampled weekly (for 12 weeks), washed thoroughly with water, and surface-sterilized as described in Section “Study Site and Collection of Mangrove Propagules.” Root and stem samples were homogenized and treated as in Section “Isolation of Endophytic Actinobacteria From Mangrove Roots” in order to determine the PD of the four isolates on ISSA amended with rifampicin. Each treatment was replicated eight times and each replicate was determined by a single pot containing one seedling.
For light and transmission electron microscopy (TEM), root and stem samples of 6-week-old mangrove seedlings inoculated with PA-producing isolates or with the positive control PA-non-producing isolate were fixed with karnovsky’s fixative in 0.17 M phosphate buffer at pH 7.2 containing 2.5 glutaraldehyde (Sigma-Aldrich) and 2% paraformaldehyde (Sigma-Aldrich) for 24 h at 4°C. Tissues were rinsed three times in 0.17 M phosphate buffer (pH 7.2) and post-fixed with 1% aqueous osmium tetroxide for 2 h at 25°C. Tissues were dehydrated with ascending grades of ethanol (30–100%) and dipped into the propylene oxide (Sigma-Aldrich). Finally, tissue samples were infiltrated and embedded in epoxy resin (Epon 812, Agar Scientific, United Kingdom) and polymerized at 60°C in embedding oven for 24 h (Millonig, 1976).
Tissue blocks were trimmed, where semi-thin sections (1.5 μm) and ultra-thin sections (95-nm) were cut with Leica EM7 ultra microtome (Vienna, Austria). Heat-dried semi-thin sections of root and stem specimen were stained with a mixture of 1% toluidine blue and borax (Sigma-Aldrich). For light microscopic (LM) analysis, slides were observed using Olympus BH-2 (Olympus Optical Co. Ltd., Japan) LM equipped with an LM Digital Camera and Software (Jenoptik ProgRes Camera, C12plus, Germany). Ultra-thin sections (90-nm) were then collected on 200 mesh Cu grids and contrasted with 10% uranyl acetate, followed by 3% lead citrate. Finally, the grids were examined under Tecnai Spirit G2 Biotwin TEM operating at 80 kV (FEI Co., Indore, Netherlands).
Identification of the Most Promising Endophytic PA-Producing Actinobacterial Isolate
The 16S rRNA gene sequencing analysis was performed by Deutsche Sammlung von Mikroorganismen und Zellkulturen GmbH (DSMZ)-Germany. Universal 16S rRNA targeting primers: 900R (5′-CCGTCAATTCATTTGAGTTT-3′); 357F (5′-TACGGGAGGCAGCAG-3′); and 800F (5′-ATTAGATACCCTGGTAG-3′) (Rainey et al., 1996; Saeed et al., 2017; Kamil et al., 2018) were used. The 16S rRNA genes sequences of all representatives of this genus were retrieved from the NCBI database1 and aligned with CLUSTAL-X (Thompson et al., 1997) provided in Molecular Evolutionary Genetics Analysis 7.0 (MEGA7; Kumar et al., 2016). The phylogenetic tree was constructed using the maximum-likelihood (ML) method (Felsenstein, 1981), and consistency was investigated by 1,000 resamplings (bootstrap analysis).
Spore chain morphology and spore surface were viewed using scanning electron microscopy (SEM) manufactured by Philips XL-30 SEM (FEI Co., Netherlands).
In vivo Assessment of Growth Promotion and Endogenous PGRs in the Greenhouse
Free draining pots (36 cm in diameter) filled with 14 kg of mangrove sediments were collected as described in Section “Study Site and Collection of Mangrove Propagules.” Inoculated seedlings with the promising PA-producing and the PA-non-producing endophytic isolates were prepared using the pruned-root dip method (section “Assessment of PGP Parameters Under Gnotobiotic Conditions”). A total of three treatments were carried out: (1) Negative control (seedlings inoculated with autoclaved ISSB medium only; no isolate); (2) positive control (seedlings inoculated with the PA-non-producing isolate); and (3) inoculated seedlings with the PA-producing isolate. Each treatment was replicated eight times and each replicate was determined by a single pot containing one seedling in a randomized complete block design (RCBD). Greenhouse trials were conducted in 2018 and were independently repeated in 2019.
Seedlings were placed in the greenhouse (section “Isolation of Endophytic Actinobacteria From Mangrove Roots”) and watered daily with full strength seawater to container capacity. Measurements of DW and length of roots and shoots, the number of branches, and leaf surface area (cm2) were followed monthly and recorded at 9 months post planting (mpp) of propagules. Fluorescence emission of chlorophyll (chl) a and chl b was measured at 645 and 663 nm, respectively (Holden, 1965). Carotenoids were estimated at 470 nm (Davies, 1965).
Put, Spd, and Spm were extracted from tissues of apical parts of root and shoot tissues (Flores and Galston, 1982). Quantitative determination of PAs was performed using benzoyl chloride (Sigma-Aldrich) and normal internal standard of PAs (Redmond and Tseng, 1979). Reverse-phase HPLC chromatograms were produced onto a 10-μm reverse-phase column as previously described (section “Qualitative and Quantitative Determination of PAs Produced by Endophytic Isolates”).
Endogenous auxins and ABA were extracted from mangrove root and shoot as previously described by Guinn et al. (1986), whereas GA3 and CKs were extracted from the same tissues according to Shindy and Smith (1975) and Machàckovà et al. (1993), respectively. HPLC parameters were applied using reverse-phase HPLC (Waters Associates) with a 254-nm UV detector. Concentrations of endogenous PGRs were obtained by comparing peak areas in unknown samples with the corresponding areas obtained in internal standard samples (Sigma-Aldrich) of known concentrations.
The extraction of endogenous ACC was carried out using the method described by Lizada and Yang (1979). Derivatization of the ACC was carried out by adding phenylisothiocyanate (Sigma-Aldrich), and the reverse-phase HPLC chromatograms were produced as described by Lanneluc-Sanson et al. (1986).
All extraction procedures of PAs and other PGRs were conducted on seedlings at 9 mpp of propagules. Eight replicates from independent samples were analyzed for chl a, chl b, carotenoids, PA, IAA, IPYA, ABA, GA3, CKs, and ACC.
Statistical Analyses
All experiments were performed as RCBD. Gnotobiotic and greenhouse experiments were repeated with similar results; and data were combined and analyzed. All data were subjected to analysis of variance (ANOVA) using SAS Software version 9 (SAS Institute Inc., NC, United States). Mean values of treatments were compared using Fisher’s protected least significant difference (LSD) test at P = 0.05 levels. Actinobacterial PD was transformed into log10 cfu g– 1 fresh root or stem weight.
Results
In vitro Evaluation of PAs and Other PGP Activities of Endophytic Actinobacterial Isolates
A total of 27 endophytic actinobacteria were isolated from the surface-sterilized root samples of which 23 (85.2%) isolates grew and heavily sporulated on ISSA plates containing 8% NaCl (Figure 1A), as a relevant indicator of salinity tolerance of certain isolates (Table 1). Four isolates were not chosen for further experiments because they did not grow on ISSA containing 8% NaCl (Figure 1A).
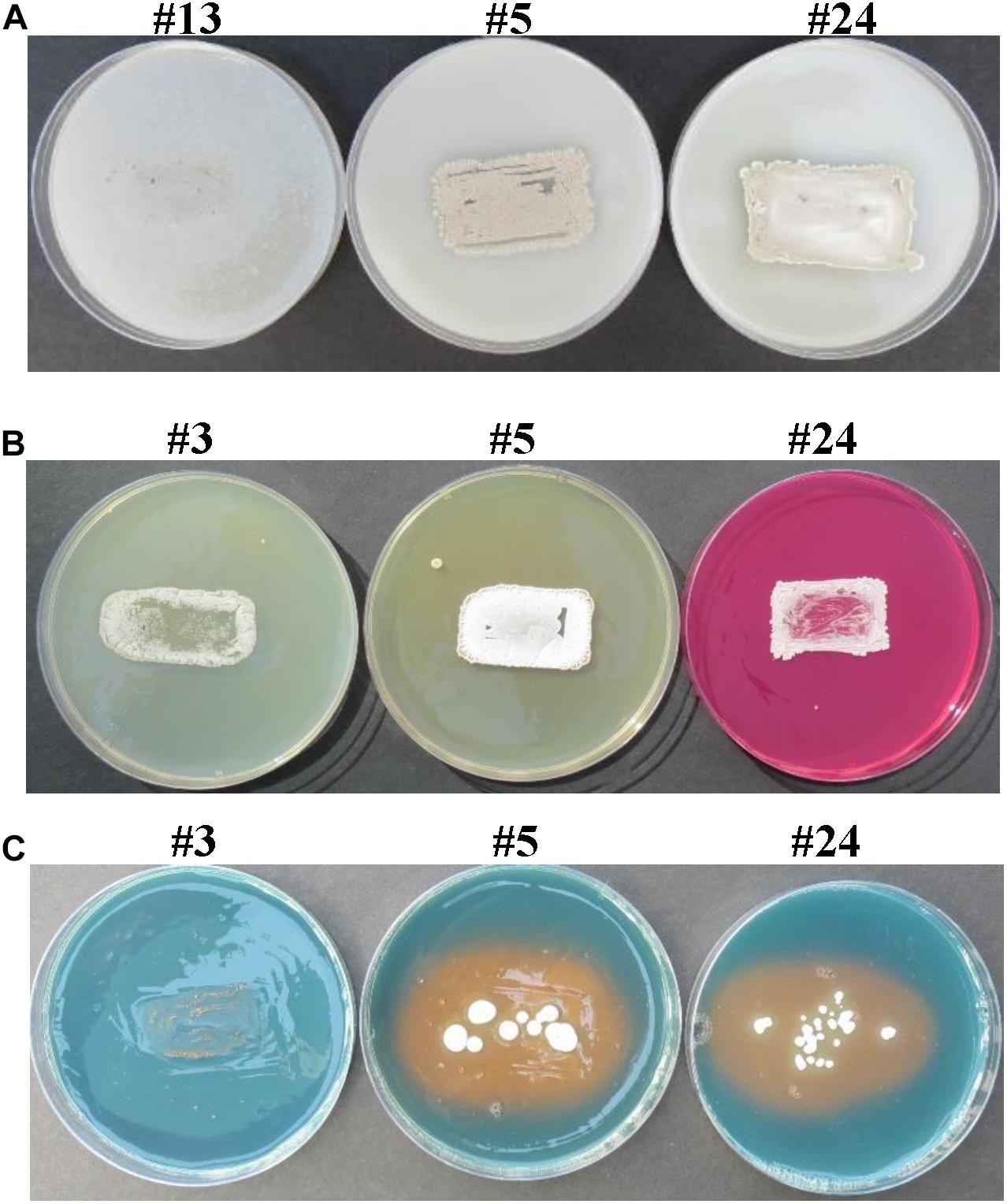
Figure 1. In vitro screening of selected endophytic actinobacteria for salinity tolerance and PGP activities. Actinobacterial isolates possessing (A) salinity tolerance; and production of (B) Put and (C) siderophores. In (A), actinobacteria #5 and #24, but not #13 (non-halotolerant control), were considered salt-tolerant isolates due to growth and heavy sporulation on inorganic salt starch agar supplemented with 8% NaCl. In (B), isolates were tested on Moeller’s decarboxylase agar medium amended with L-arginine-monohydrochloride; and the change from yellow to red color of the phenol-red in isolate #24, but not #3 or #5, indicated production of PA, Put. In (C), isolates were tested on chrome azurol S agar plates; and yellow halo surrounding the colony of #5 and #24, but not #3 (control), indicated the excretion of siderophores. Note that isolate #3 was considered as a halotolerant Streptomyces sp. Isolate #24 represents the endophytic PA-producing Streptomyces mutabilis UAE1, while #5 represents the endophytic PA-non-producing Streptomyces sp. 19-388. PGP, plant growth promoting; PA, polyamine; Put, putrescine.
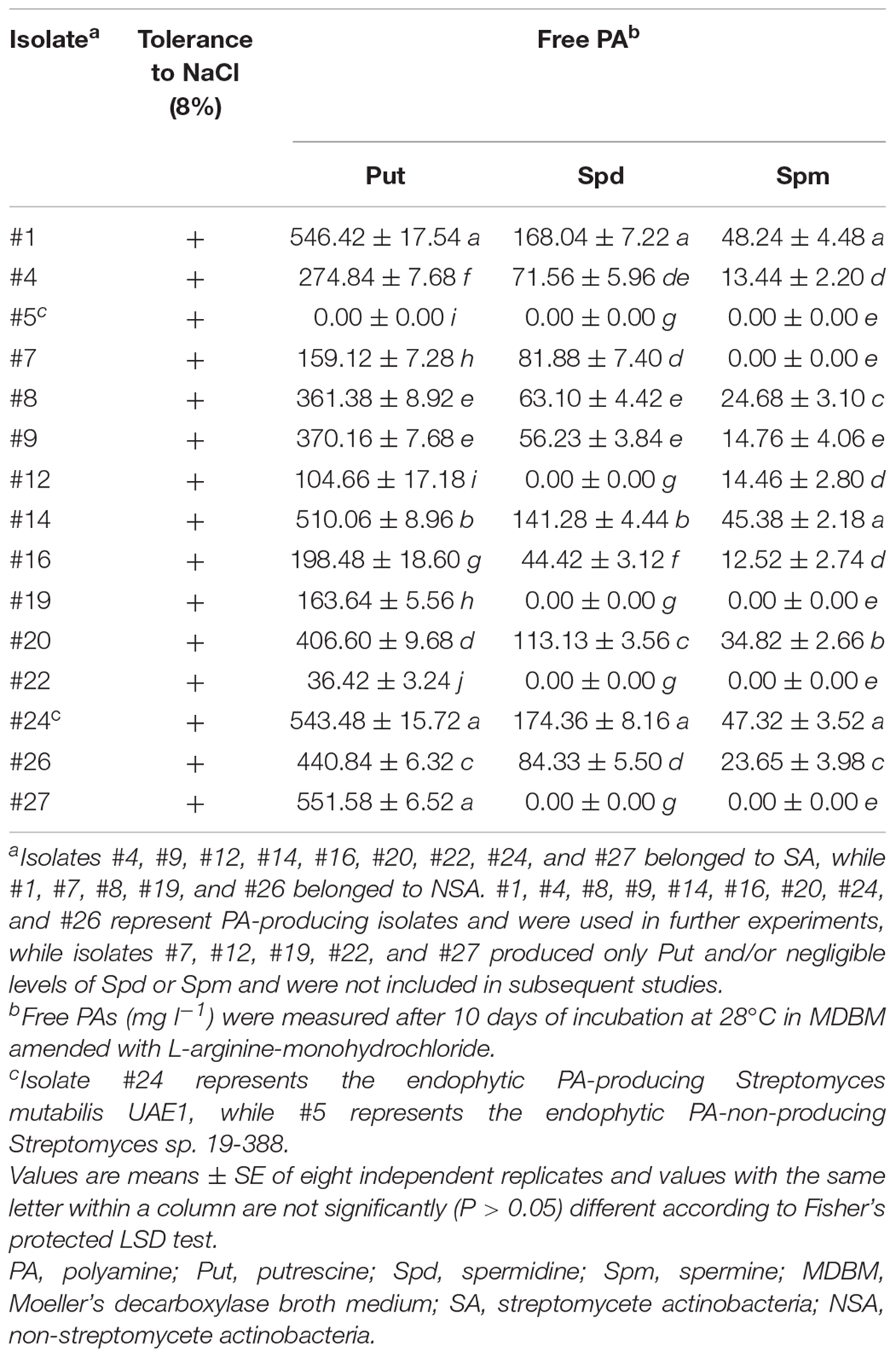
Table 1. In vitro production of the free PAs by selected halotolerant endophytic actinobacterial isolates.
The highly salt-tolerant isolates were tested if they could produce Put in MDAM plates amended with L-arginine (Table 1). Fourteen of them, representing 51.9% of the total actinobacterial endophytes, were able to produce Put in vitro. This was clearly observed when isolates showed relatively moderate to dark red halo surrounding or beneath the colonies (Figure 1B), suggesting that these particular isolates could be considered as potential Put-producing endophytic halotolerant actinobacteria. The other nine isolates showing no or bright red halo in culture media were either non-Put producers or producers of little amount of Put and thus were excluded from subsequent tests.
To determine if they were able to produce other PAs in vitro, quantitative assays were carried out on culture extracts of the 14 isolates. The amounts of Put, Spd, and Spm synthesized by these isolates significantly (P < 0.05) varied (Table 1). According to HPLC analysis, isolates #7 and #12 produced Put and either Spd or Spm, respectively. Three isolates (#19, #22, and #27) produced Put only (Table 1) and were excluded from further studies. The remaining isolates #1, #4, #8, #9, #14, #16, #20, #24, and #26 produced all three PAs and were further tested to synthesize other PGRs in vitro.
Furthermore, three isolates (#4, #16, and #26) produced detectable levels of IAA and/or IPYA; and four (#4, #9, #16, and #26) produced different levels of iPa, iPA, and Z (Table 2). Only isolate #26 produced GA3 in the culture extracts. We also assessed the production of ACCD and nitrogenase by PGPA in vitro, as these enzymes are known for their PGP activities (El-Tarabily et al., 2019). Although isolate #20 produced both enzymes; isolates #9 and #4 produced only ACCD and nitrogenase, respectively (Table 2). Only isolates #1, #8, and #9 were able to solubilize P. Because we ought to find isolates only producing the three PAs regardless of any other PGP activities, all the above-mentioned isolates were not included in the gnotobiotic-related experiments.
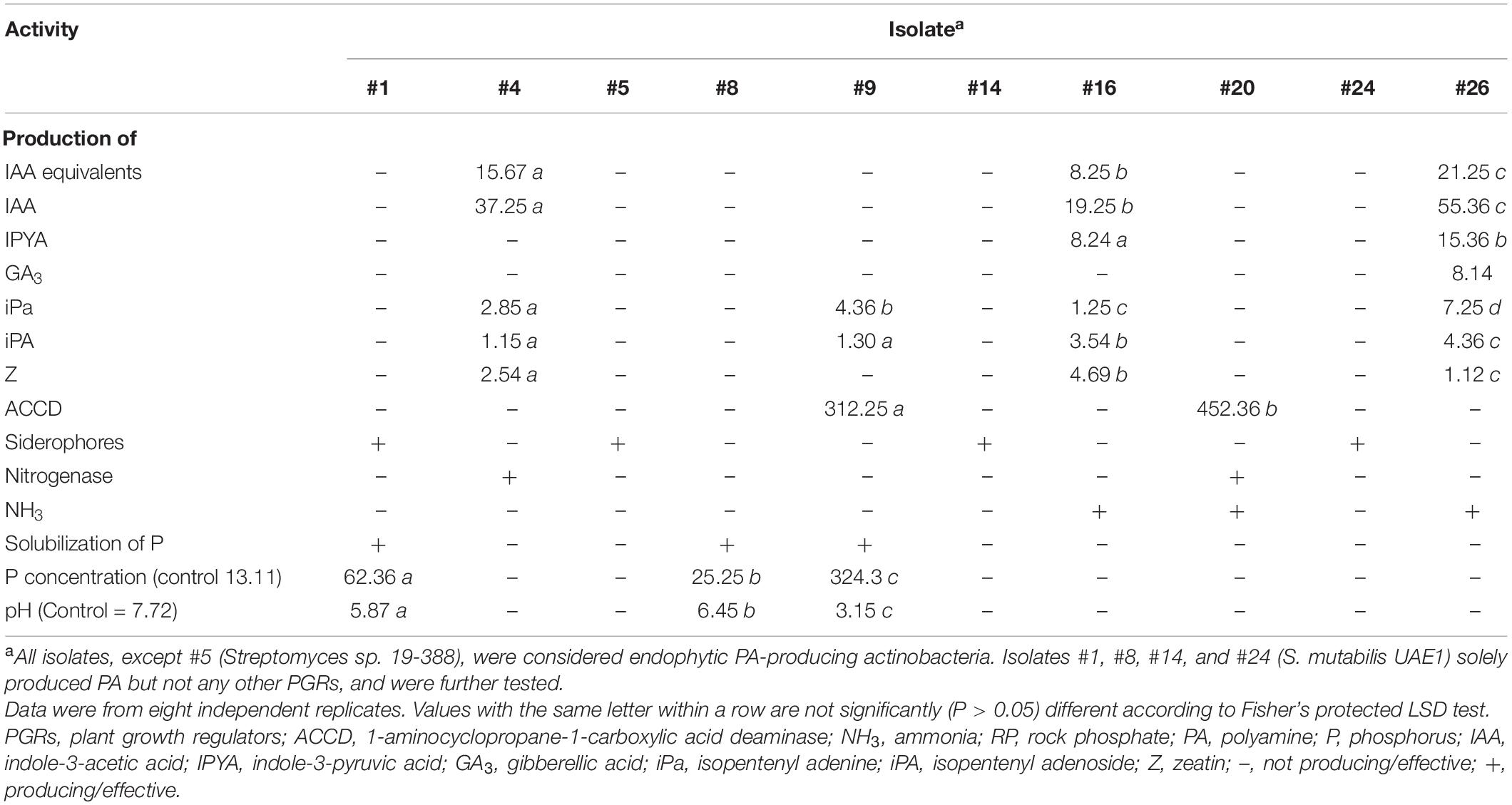
Table 2. In vitro production of PGRs (μg ml–1), ACCD (nanomoles α-keto-butyrate mg–1 protein h–1), siderophores, nitrogenase enzyme, and NH3; and effect of inoculation with RP amended Pikovskaya’s broth by halotolerant PA-producing actinobacteria isolated from mangrove roots on pH levels and concentration of available P (mg l–1).
Our results demonstrated that there were no detectable levels of PGRs, ACCD, and nitrogenase enzymes or P solubilization in the culture extracts of the PA-producing isolates #1, #8, #14, and #24 or the PA-non-producing isolate #5 (Table 2 and Supplementary Figure 1). Except of isolate #8, the other four isolates produced siderophores (Table 2 and Figure 1C), suggesting that these isolates are mainly dependent on PA production.
Evaluation of PGP Features in Mangrove Seedlings Under Gnotobiotic Conditions
In addition to the endophytic actinobacterial isolates producing high levels of PAs (#1, #8, #14, and #24), the endophytic PA-non-producing isolate #5 (positive control) was further screened for growth promotion activities on mangrove under controlled-gnotobiotic conditions. The four endophytic isolates generally showed variable effects on root and shoot growth. First, we noticed that inoculation of mangrove roots with isolates #14 and #24 resulted in maximum significant (P < 0.05) increase in root and stem lengths, which was 60.8–65.7 and 44.5–47.2% higher than non-inoculated plants, respectively (Table 3). To a lesser extent, mangrove seedlings initially inoculated with isolate #1 showed increases by 58.5% in root and 32.2% in stem lengths.
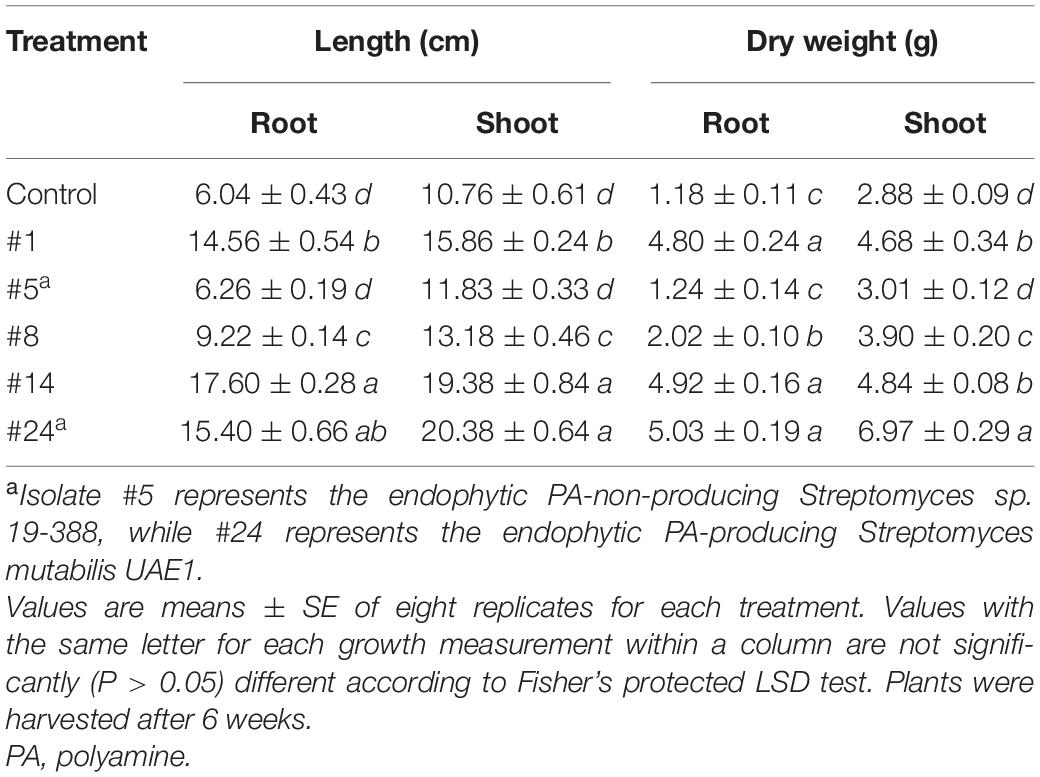
Table 3. Effect of endophytic PA-producing actinobacterial isolates on growth parameters of mangrove (Avicennia marina) under gnotobiotic conditions.
Second, the same endophytic isolates #14 and #24 significantly (P < 0.05) increased DW of roots (76.0 and 76.5%, respectively) and shoots (40.5 and 58.7%, respectively) compared to non-inoculated treatments (Table 3). Isolate #1 also showed approximately similar seedling DW of roots (75.4%) and shoots (62.5%) as those inoculated with the superior isolates.
The measured root and stem lengths and weights of seedlings treated with the endophytic PA-producer (#8) were significantly (P < 0.05) higher than those non-treated or treated with the PA-non-producing isolate #5, but the measurements were significantly (P < 0.05) less than in the other tested PA-producing isolates (Table 3). Accordingly, isolate #8 was not selected for further experiments. Thus, isolates #1, #14, and #24 were tested in the greenhouse. All tested PGP features using isolate #5 were comparable to those of non-inoculated mangrove plants (Table 3).
Endophytic Colonization of Tissues by Selected PA-Producing Actinobacterial Isolates
In general, the potent PA-producing isolates (#1, #14, and #24) recovered at all samplings on a biweekly basis up to 12 weeks, from internal root and stem tissues of mangrove, thus maintaining plant health as well as their endophytic nature. Except of #14, the total population of isolates increased significantly (P < 0.05) in all examined weeks in root and stem tissues (Figure 2). We noticed a significant (P < 0.05) increase until week 6 inside both tissues, followed by a drop in PD of isolate #14 starting week 10 in roots and 8 in stems until the end of the experiment. This indicates that isolate #14 did not sufficiently recover from tissues in week 6 onward. Based on our results, colonization of isolate #1 inside stems was limited. This was evident from the insignificant (P > 0.05) mean of total population in this isolate in mangrove stems between week 2–6 and week 6–12 (Figure 2). This suggests that this isolate does not colonize stem tissues efficiently. Therefore, isolates #1 and #14 were not included in the subsequent greenhouse trials.
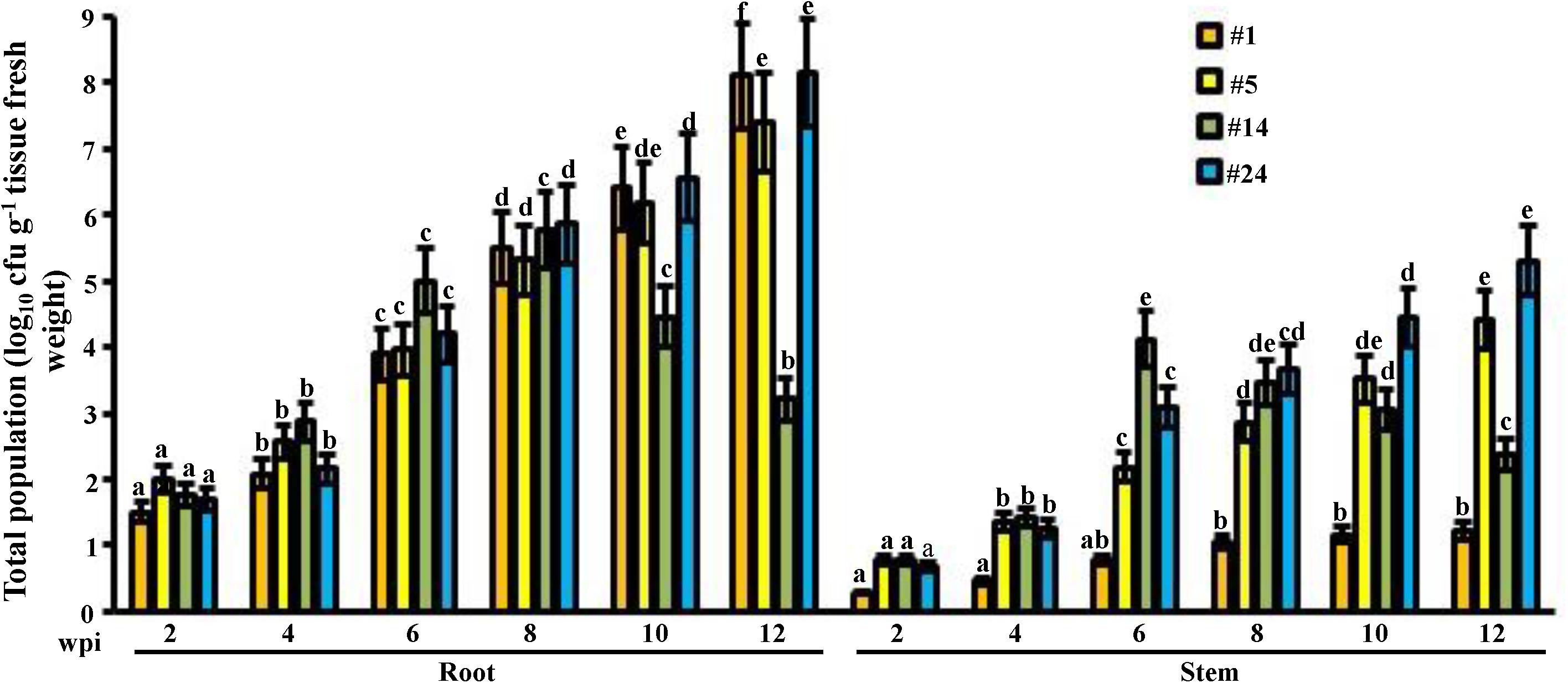
Figure 2. Total population of the promising endophytic actinobacteria in mangrove tissues. Population density of the endophytic PA-non-producing isolate #5 and PA-producing isolates #1, #14, and #24 in the root and stem of gray mangrove grown under greenhouse conditions. Tissues were sampled every 2 wpi. Values are means of eight replicates ± SE for each sampling per treatment. Mean values followed by different letters are significantly (P < 0.05) different from the total populations of each strain in that particular tissue according to Fisher’s protected LSD test. Isolate #24 represents the endophytic PA-producing Streptomyces mutabilis UAE1. PA, polyamine; wpi, weeks post inoculation.
For isolate #24, PD dramatically increased for the tested period of up to 12 weeks of root and stem colonization (Figure 2), thus suggesting a beneficial plant–microbe interaction, i.e., plant growth promotion. From the beginning to the end of the colonization examined weeks, similar increase patterns in tissue PD of the PA-non-producing isolate #5 were observed, making it a perfect positive control for the greenhouse experiments in comparison to the finally selected PA-producing isolate #24.
We also carried out microscopy examination on the tissue-associated actinobacterial isolate #24. At 6 weeks post inoculation (wpi), spores of isolate #24 were abundantly present within parenchyma cells of cortex and xylem of mangrove-inoculated roots (Figure 3). Mycelial growth carrying the spiral spore chain of isolate #24 within cortical cells of roots and stems was also detected. Semi-thin sections revealed that the endophytic actinobacterial isolate #24 could successfully colonize intracellular spaces between root cells and intercellularly within xylem vessels, indicating its abilities to translocate between cortical and xylem conducting tissues (Supplementary Figure 2). We also figured out that many spores belonging to isolate #24 were intracellularly found in the process of germination and formation of germ tubes (Supplementary Figure 2). Not surprisingly, mycelial growth of isolate #5 (PA-non-producer) and straight chain of spores were located within the root and stem of mangrove at 6 wpi (Supplementary Figure 3).
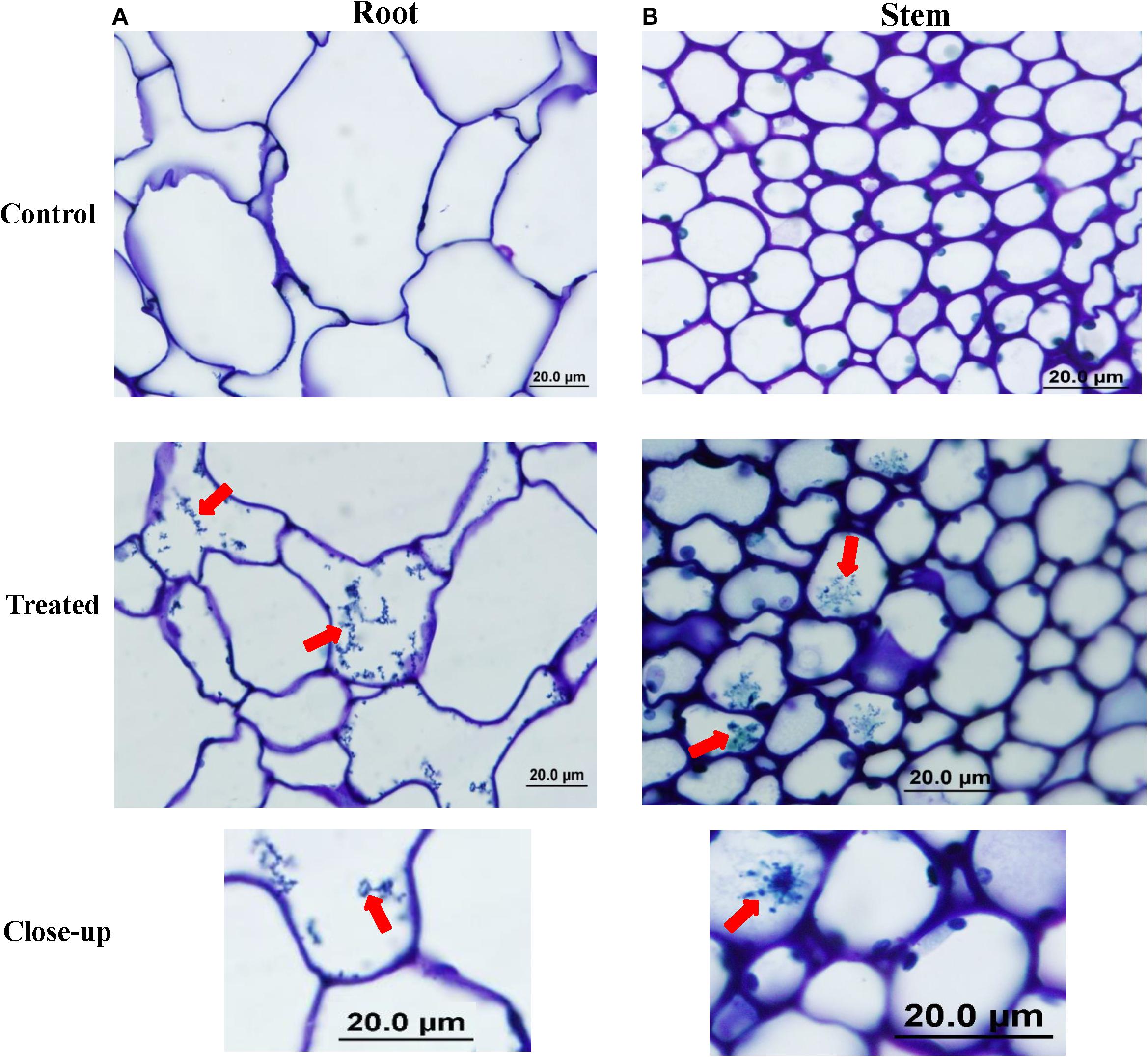
Figure 3. Colonization of mangrove tissues by the endophytic isolate #24. Light micrograph of semi-thin sections of gray mangrove (A) root and (B) stem tissues not inoculated (control; top); or inoculated with isolate #24 (treated; middle); and spiral chain of spores (close-up; bottom) of isolate #24 within treated tissues (1,000×). In (A,B), all sections were stained with 0.1% toluidine blue showing the distribution of mycelium and spiral chain of spores (red arrows) in root and stem tissues within mangrove cells. Isolate #24 represents the PA-producing Streptomyces mutabilis UAE1. PA, polyamine. Bars: 20 μm.
Compared to control treatment (Figure 4A), TEM studies further confirmed the presence of the spiral chain of spores and substrate mycelia of isolate #24 within the cortex of roots (Figure 4B) and stems (Figure 4C). Overall, our data suggest that isolate #24 is an actinobacterial endophyte that inhabits within living root and stem tissues of mangrove.
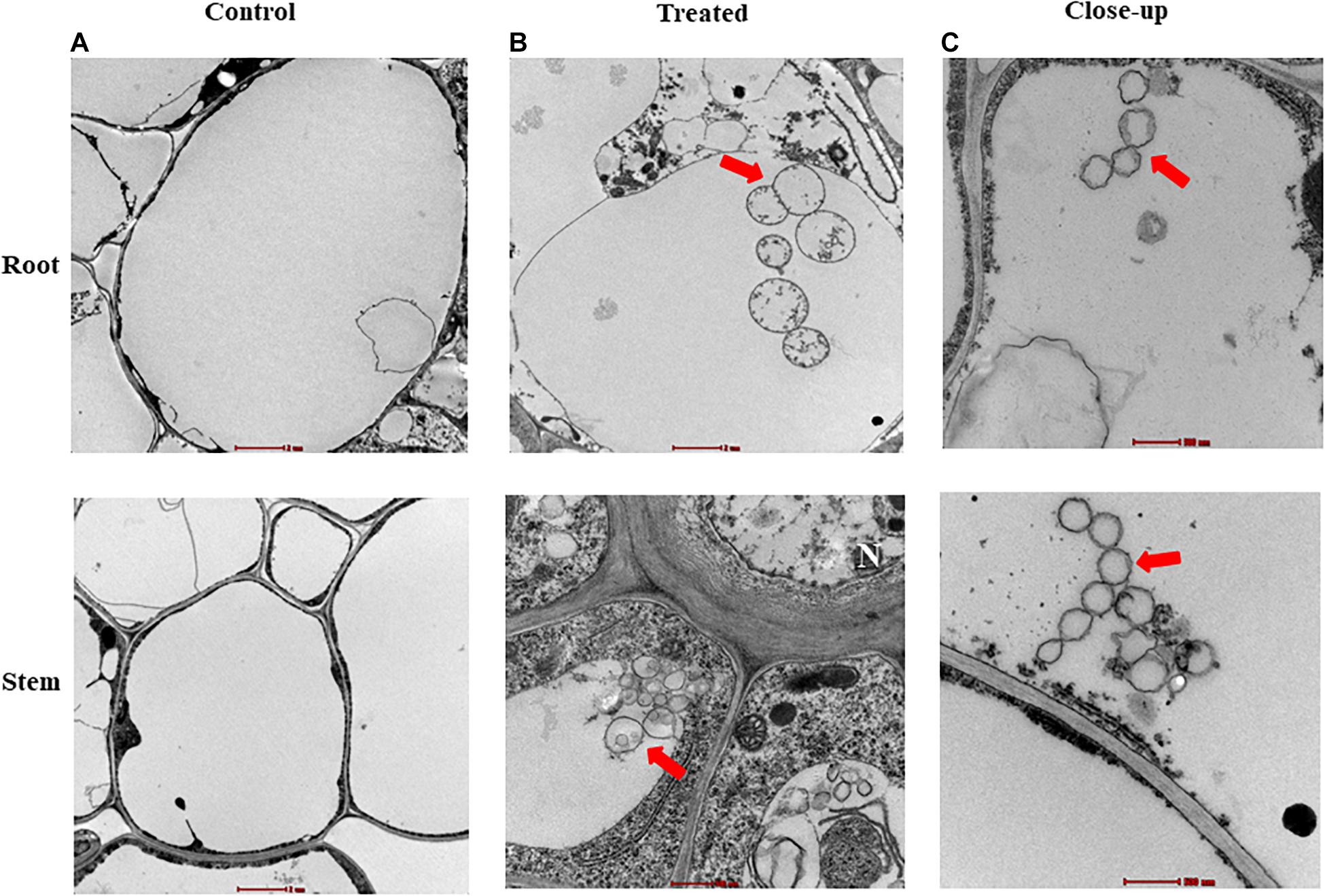
Figure 4. Inter- and intra-cellular colonization of mangrove tissues by the endophytic isolate #24. Transmission electron micrograph of ultra-thin sections of a 6-week-old gray mangrove (A) non-inoculated (control; 6,000×) and (B) inoculated (treated; 6,000×) root (top) and stem (bottom) tissues with Streptomyces mutabilis UAE1. (C) A close-up of spiral chain of spores of S. mutabilis UAE1 within the root (top) and stem (bottom) tissues (20,500×). In (A–C), all sections were stained with uranyl acetate and lead citrate showing the distribution of spiral chain of spores (red arrows). Isolate #24 represents the endophytic PA-producing Streptomyces mutabilis UAE1. PA, polyamine. Bars: (A,B-top) 2 μm; and (B-bottom,C) 500 nm. N, nucleus.
Classification and Identification of the PA-Producing Endophytic Actinobacterial Isolate
The 16S rRNA gene sequence of the promising PA-producing isolate #24 (NCBI Genbank accession number: MT883496) was compared with other species of Streptomyces and a phylogenetic tree was generated. Sequence analysis of 1,520-bp portions of the 16S rRNA gene indicated that this strain was 100% similar to the described species Streptomyces mutabilis NRRL ISP-5169T (NR_044149), but only 98.8% similar to Streptomyces rochei, Streptomyces enissocaeilis, Streptomyces plicatus, and Streptomyces vinaceusdrappus (Figure 5A). The rest of actinobacterial species were less than 99% similar to the isolate-of-interest. In addition, pure cultures of isolate #24 developed light gray aerial mass color and light gray substrate mycelium with no distinctive pigment on the reverse side of cultures on ISP medium 3 (Figure 5B). The isolate formed closed spiral chains consisting of 5–10 mature, smooth-surfaced spores on the aerial hyphae (Figure 5C). Spore chains belonged to section spirales. This suggests that the PA-producing isolate #24 could be considered as S. mutabilis (Preobrazhenskaya and Ryabova in Gauze et al., 1957) Pridham et al. (1958) Strain UAE1.
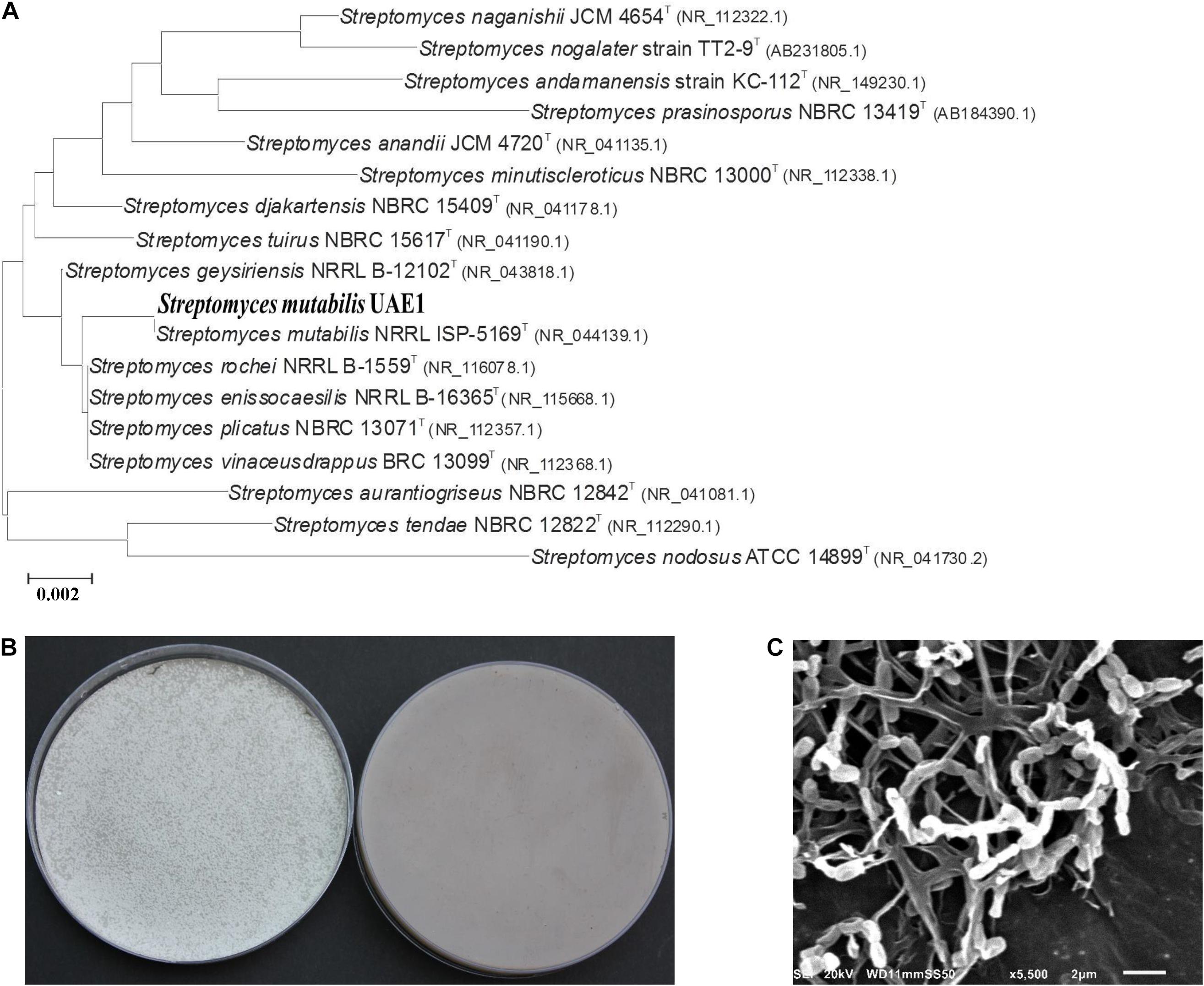
Figure 5. Taxonomic determination of PA-producing Streptomyces mutabilis UAE1, based on phylogenetic, cultural, and morphological characteristics. (A) The tree showing the phylogenetic relationships between S. mutabilis UAE1 (isolate #24; MT883496; 1,520 bp) and other members of Streptomyces spp. on the basis of 16S rRNA sequences. (B) Aerial mycelia (left) and substrate mycelia (right) growing on ISP medium 3 supplemented with yeast extract, and (C) scanning electron micrograph (5,500) of spore chains and structure of S. mutabilis UAE1 which belonged to section spirales. In (A), numbers at nodes indicate percentage levels of bootstrap support based on a maximum-likelihood analysis of 1,000 resampled datasets. PA, polyamine. Bar, 0.002 substitutions per site. GenBank accession numbers are given in parentheses.
The sequence of the 1,521-bp region of 16S rRNA gene (accession number: MT883497) of isolate #5 showed the closest similarity to that of Streptomyces qaidamensis, Streptomyces chartreusis, and Streptomyces osmaniensis, with 98.7, 98.2, and 98.0%, respectively (Supplementary Figure 4). Thus, this suggests that the isolate could be any of these species or a new species that has not been identified previously. Pure cultures of strain ID 19-388 displayed whitish light gray colored aerial spore mass on ISP 3 medium, and produced yellow substrate mycelium with distinctive yellow pigment on the reverse side of cultures (Supplementary Figure 4). Based on the SEM analysis, spores were cylindrical with smooth-textured surface and spore chains were long and straight with >50 spores per chain which belonged to section rectiflexibiles (Supplementary Figure 4). A more reliable identification, such as DNA–DNA hybridization, of this stain is required for proper classifications. Thus, the PA-non-producing endophytic actinobacterial isolate #5, which served as a positive control treatment in gnotobiotic (section “Evaluation of PGP Features in Mangrove Seedlings Under Gnotobiotic Conditions”) and greenhouse (section “Growth Promotion of Mangrove by S. mutabilis UAE1 in the Greenhouse”) experiments, was designated as Streptomyces sp. 19-388.
Growth Promotion of Mangrove by S. mutabilis UAE1 in the Greenhouse
In our greenhouse experiments, we inoculated mangrove seedlings with S. mutabilis UAE1 (isolate #24), Streptomyces sp. 19-388 (#5), or no isolate (control). Although both Streptomyces sp. inoculants did not cause negative impact on growth, inoculations with the PA-producing isolate S. mutabilis undoubtedly promoted growth and development of mangrove (Figure 6). This was clearly observed when S. mutabilis significantly (P < 0.05) increased DW (Figure 6B) and enhanced length (Figure 6C) in roots and shoots, compared to seedlings initially inoculated with Streptomyces sp. 19-388 or non-inoculated plants after 9 mpp of propagules. In comparison to S. mutabilis-inoculated plants, the number of branches decreased significantly by 63.5% in control treatment and 65.0% in the PA-non-producing Streptomyces sp. 19-388 treatment (Figure 6D). The total leaf area in plants treated with S. mutabilis was 43.3 and 37.1% larger than in non-inoculated and Streptomyces sp. 19-388-inoculated plants, respectively (Figure 6E). We did not find significant differences in any of the tested growth-related agronomic characteristics in roots inoculated with Streptomyces sp. 19-388 or control treatment (Figures 6A–E).
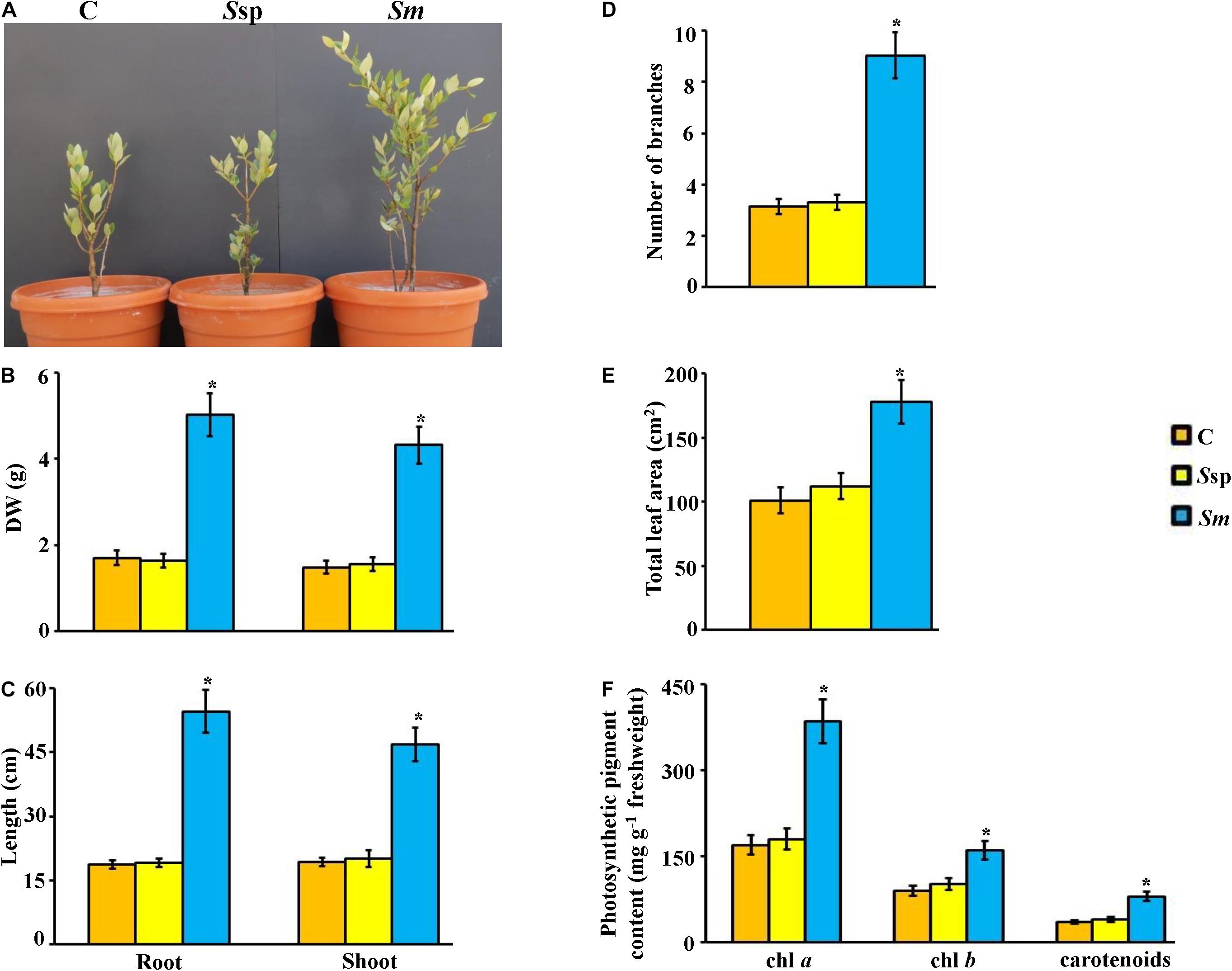
Figure 6. Effect of Streptomyces mutabilis UAE1, on mangrove growth and development. Effect of application of PA-producing S. mutabilis on (A) performance; (B) DW and (C) length of root and shoot tissues; (D) number of branches, (E) total leaf area, and (F) photosynthetic pigment contents of chl a, chl b, and carotenoids on mangrove seedlings. In (A–F), the figure, DW and length of shoot and root tissues, and photosynthetic pigments were measured at 9 months post planting the inoculated mangrove propagules. Values are means ± SE of 16 replicates for each treatment from two different independent experiments. Mean values followed by an asterisk are significantly (P < 0.05) different from each other according to Fisher’s protected LSD test. Bars represent SE. DW, dry weight; control (non-inoculated inorganic salt starch broth); Ssp, PA-non-producing isolate #5 (Streptomyces sp. 19-388); Sm, PA-producing isolate #24 (S. mutabilis UAE1). PA, polyamine; chl, chlorophyll.
Due to the strong association between the photosynthetic pigments and growth rate of crops (El-Tarabily et al., 2019, 2020; Mathew et al., 2020), we measured the contents of chl a, chl b, and carotenoids in planta. Our data showed that amounts of both chlorophyll pigments as well as carotenoids were significantly (P < 0.05) higher in S. mutabilis-inoculated plants than in plants inoculated or not with Streptomyces sp. 19-388 after 9 mpp (Figure 6F). Thus, there was no significant difference between contents of any of the three pigments in control and Streptomyces sp.-inoculated seedlings. In contrast to PA-non-producing Streptomyces sp. 19-388, PA-synthesizing S. mutabilis UAE1 significantly (P < 0.05) stimulated photosynthetic pigment production, and consequently this might result in growth promotion of mangrove.
Effect of S. mutabilis on Endogenous PAs and Phytohormone Contents in Mangrove Tissues
To figure out the mechanism associated with mangrove growth promotion by S. mutabilis UAE1, we analyzed the endogenous levels of free PAs and phytohormones in root and shoot tissues. When S. mutabilis was inoculated in roots, roots and shoots of mangrove had significantly (P < 0.05) higher levels of Put (Figure 7A), Spd (Figure 7B), and Spm (Figure 7C) than those grown with or without amendment of PA-non-producing Streptomyces sp. 19-388. In general, the endogenous three PA amounts in shoots were higher than in roots in all treatments (Figure 7).
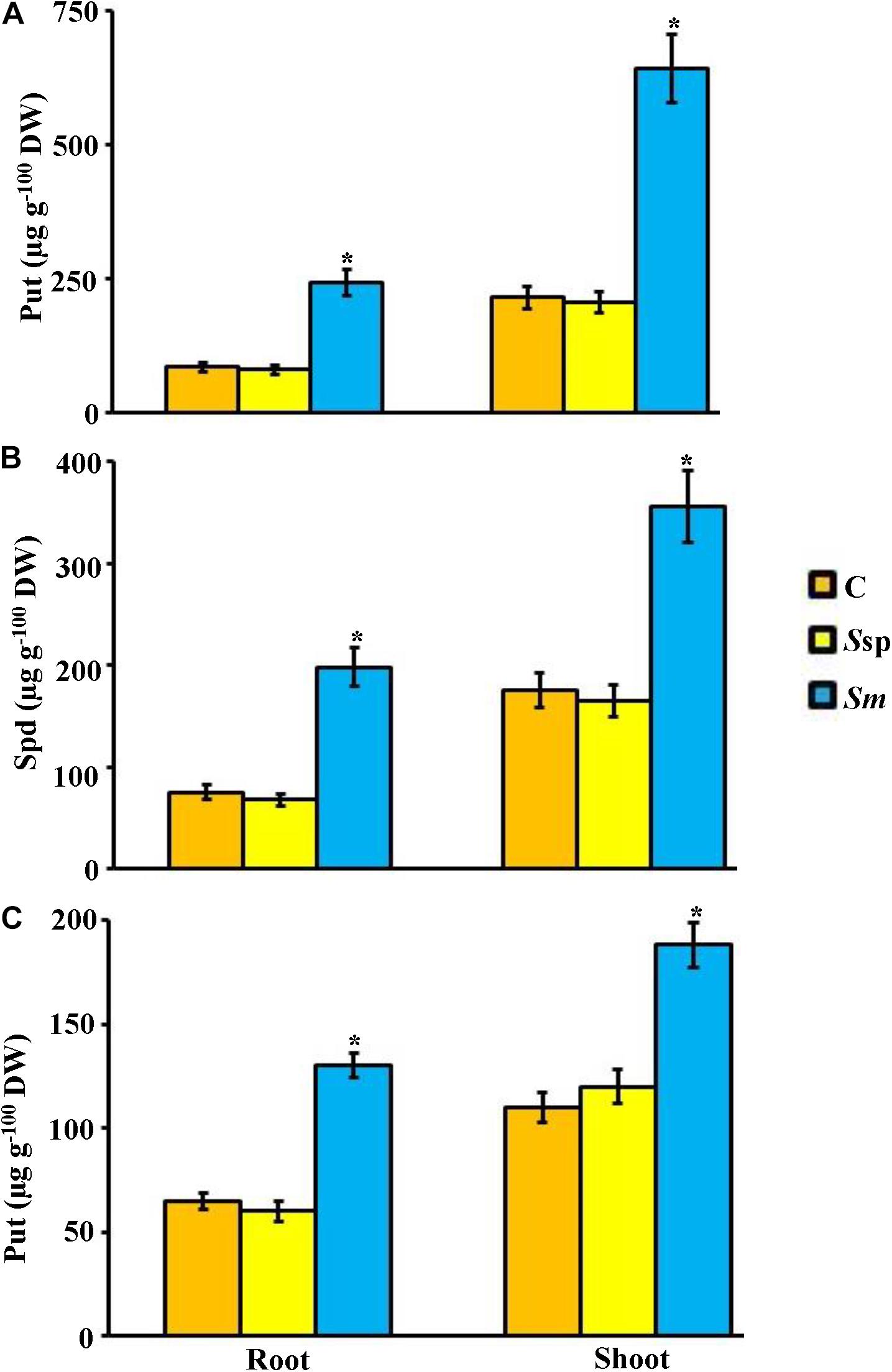
Figure 7. Effect of the endophytic Streptomyces mutabilis UAE1 on endogenous PA contents of mangrove. Endogenous contents of (A) Put, (B) Spd, and (C) Spm in mangrove root and shoot tissues after treatment with C, Ssp, and Sm. Mangrove seedlings were grown in an evaporative-cooled greenhouse and maintained at 30 ± 2°C. Values are means ± SE of 16 replicates for each treatment from two different independent experiments. Mean values followed by an asterisk are significantly (P < 0.05) different from each other according to Fisher’s protected LSD test. Bars represent SE. Endogenous contents of Put, Spd, and Spm were measured at 9 months post planting the inoculated mangrove propagules. DW, dry weight; PA, polyamine; C, control (non-inoculated inorganic salt starch broth); Ssp, PA-non-producing isolate #5 (Streptomyces sp. 19-388); Sm, PA-producing isolate #24 (S. mutabilis UAE1); PA, polyamine, Put, putrescine; Spd, spermidine; Spm, spermine.
Under greenhouse conditions, the concentration of phytohormones in tissues of plant inoculated with S. mutabilis UAE1 and in those inoculated with Streptomyces sp. 19-388 or not was significantly (P < 0.05) different (Figure 8). Inoculated seedlings with S. mutabilis were characterized by almost 41–44 and 30–33% higher concentration of IAA in roots and shoots, respectively, than those in positive (Streptomyces sp. 19-388) and negative (no isolate) control treatments (Figure 8A). Likewise, the tested IPYA showed similar patterns of increase in both tissues of seedlings treated with the PA-producing isolate (Figure 8B). There was 19–21, 38–49, and 49–55% greater contents of iPA, iPa, and Z, respectively, in tissues of seedlings originally treated with S. mutabilis than those in other treatments (Figures 8C–E). We noticed, however, that ABA concentration was decreased by approximately 2.5-fold in both tissues of S. mutabilis-inoculated compared to Streptomyces sp. 19-388-inoculated and control plants (Figure 8F).
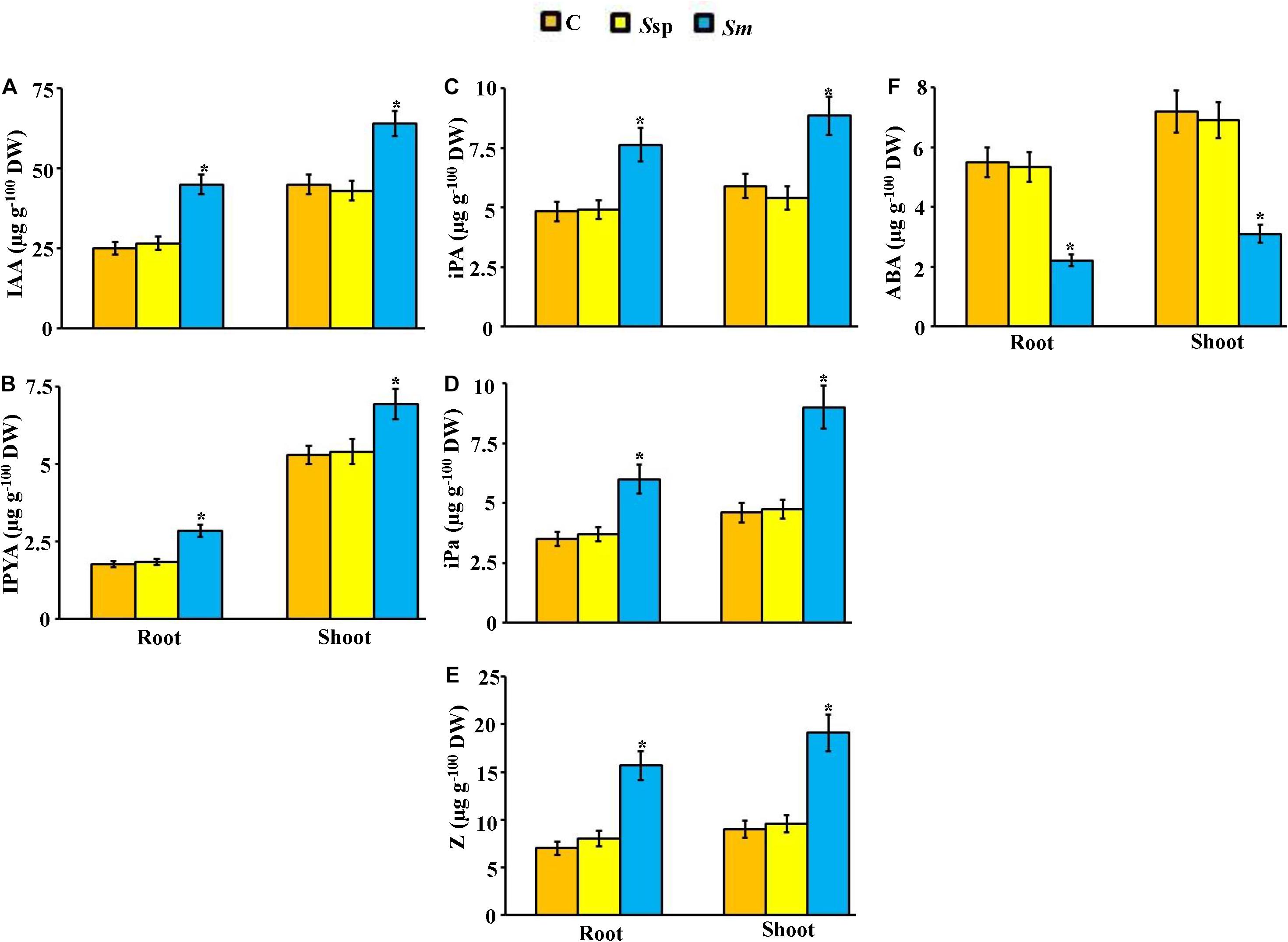
Figure 8. Effect of the endophytic Streptomyces mutabilis UAE1 on endogenous PGR contents of mangrove. Endogenous contents of auxins (A) IAA and (B) IPYA; cytokinins (C) iPA, (D) iPa, and (E) Z; and (F) ABA in mangrove root and shoot tissues after treatment with C, Ssp, and Sm. Mangrove seedlings were grown in an evaporative-cooled greenhouse and maintained at 30 ± 2°C. Values are means ± SE of 16 replicates for each treatment from two different independent experiments. Mean values followed by an asterisk are significantly (P < 0.05) different from each other according to Fisher’s protected LSD test. Bars represent SE. Endogenous contents of auxins, cytokinins, and ABA were measured at 9 months post planting the inoculated mangrove propagules. DW, dry weight; C, control (non-inoculated inorganic salt starch broth); Ssp, PA-non-producing isolate #5 (Streptomyces sp. 19-388); Sm, PA-producing isolate #24 (S. mutabilis UAE1); PA, polyamine; IAA, indole-3-acetic acid; IPYA, indole-3-pyruvic acid; iPA, isopentenyl adenine; iPa, isopentenyl adenoside; Z, zeatin; ABA, abscisic acid.
In our controlled-greenhouse experiments, there was no significant difference in the endogenous levels of GA3 and ACC in mangrove tissues in the three treatments (Supplementary Figure 5). The levels of all phytohormones in shoot tissues were relatively higher than in root tissues. Taken together, our data suggest that S. mutabilis UAE1 is a PA-producing endophytic isolate that increases PA production in planta, thus regulating endogenous hormonal levels to enhance growth of mangrove.
Discussion
The present study reports—for the first time—increased growth of mangrove planted in sediments and irrigated with seawater using PA-producing endophytic PGPA, as an important application in marine agricultural activities. Our current project integrates biological diversity, ecological significance, and socio-economic impact on coastal communities through restoration of mangrove forests in the Arabian Gulf. Here, we identified and characterized S. mutabilis UAE1 isolated from mangrove roots, which stimulated growth of seawater-irrigated mangrove seedlings under controlled greenhouse conditions. Consistent with previous studies, Streptomyces is the most dominant genus among endophytic actinobacterial communities of mangrove plants (Xu et al., 2016; Li et al., 2017). Toumatia et al. (2016) have found that S. mutabilis IA1 is an endophyte colonizing wheat tissues possessing biocontrol and PGP properties. In another study, the performance of S. mutabilis showing antitubercular and antimicrobial activities against bacterial pathogens has also been reported (Mahmoud et al., 2015).
In the present study, PA production by S. mutabilis UAE1 was determined according to the following procedure: (i) Halotolerant actinobacterial isolates identified as endophytes were in vitro screened based on high production of the three PAs (Put, Spd, and Spm); (ii) isolates secreting PGRs, other than PAs, were eliminated; (iii) all PA-producing isolates were tested for their PGP abilities under gnotobiotic conditions; (iv) the sole strain colonizing both root and stem tissues of mangrove was chosen; and (v) assessing S. mutabilis UAE1 as a PGPA isolate on agronomic characteristics and endogenous PA and other PGR contents in mangrove seedlings irrigated with seawater. In these trials, Streptomyces sp. ID 19-388, the PA-non-producing isolate #5, was used as a positive control.
In general, the implementation of PGPB that can fix N or solubilize P has a positive impact on growth of marine plants including mangrove (El-Tarabily and Youssef, 2010). Seedlings of S. bigelovii irrigated with seawater have shown high performance in growth and seed yields after the application of N-fixing and/or P-solubilizing bacteria originally obtained from mangrove rhizosphere (Bashan et al., 2000; Rueda-Puente et al., 2003). The P-solubilizing Oceanobacillus picturae, isolated from mangrove soils promoted growth of mangrove seedlings under saline conditions in the greenhouse (El-Tarabily and Youssef, 2010). Our results demonstrated that the endophyte S. mutabilis did not fix N, solubilize P, or produce other PGRs. Thus, production of high amount of the three PAs by this strain contributed to growth promotion of mangrove in the gnotobiotic and greenhouse experiments. This current report is the first to reveal the potential of endophytic actinobacteria isolated from root tissues to stimulate mangrove growth under greenhouse conditions by possibly stimulating PA and regulating phytohormone levels in planta.
Killiny and Nehela (2020) have proposed that enhanced growth can possibly be due to the function of PAs as secondary messengers for phytohormones (e.g., auxins) within plant cells. Growth promotion by S. mutabilis, in the present study, was associated with the increase of endogenous PA levels, enhancement of photosynthetic pigments, and increases of IAA and IPYA in roots and shoots. Following the application of PA-producing rhizosphere-competent actinobacteria (El-Tarabily et al., 2020) or Bacillus amyloliquefaciens (Idris et al., 2007), increases in endogenous auxins in treated plants have been detected. We also found that growth promotion by the PA-producing isolate was associated with increases in iPA, iPa, and Z levels. PAs might also play a key regulatory role in plant growth and development through regulating auxin/CKs ratio (Ikeuchi et al., 2016). Similar observations of high PAs contributing to plant growth promotion through regulation of endogenous CK levels have also been reported (Ortíz-Castro et al., 2008). Our study sheds light on the role of PAs as a plant growth promoter in marine agriculture. The effects of PAs in inducing mangrove growth can be explained as the augmentation of Put, Spd, and Spm concentrations and the balance of auxin and CKs in plant tissues.
In general, plant responses to unfavorable environment can be modulated by various plant hormones and PGRs. Synergistic and antagonistic interactions between PAs and other phytohormones, such as ABA and ET, may occur to regulate cellular processes in plants (Killiny and Nehela, 2020). Other studies of endogenous ABA deficiency have shown that ABA can limit ET production, consequently leading to shoot and root growth under water stress (Sharp and LeNoble, 2002). In S. bigelovii, elevated PAs in roots and shoots and increased productivity of seeds have been linked to decreasing ABA levels in both tissues when plants were inoculated with the PA-producing actinobacterial isolate (El-Tarabily et al., 2020). Thus, the endogenous levels of ACC (the natural precursor of ET) remained unaffected. ACC contents in tissues of S. bigelovii treated with another PA-producing rhizosphere competent isolate of Streptomyces tritolerans isolated from mangrove sediments were comparable to control treatments, albeit increases in endogenous PAs and auxins (Mathew et al., 2020). Similarly, treating mangrove propagules with the PA-producing isolate S. mutabilis not only increased PAs in plant tissues and enhanced growth of mangrove seedlings, but also lowered ABA contents and maintained normal levels of ACC in planta. Our data support previous findings that the regulatory role of PAs in plant growth can modulate ABA both under normal and stressful conditions (Hatmi et al., 2018; Alcázar et al., 2020). Thus, this modulation is mostly dependent on plant species and degree of tolerance to stress (Rangan et al., 2014).
Except Put, Spd, and Spm, no other PGRs were produced in culture filtrates of S. mutabilis UAE1. Thus, enhanced growth of mangrove due to high levels of PAs produced by this strain would result in increase of PA levels, regulation of endogenous auxins/CKs, and suppression of ABA in plant tissues. Accordingly, we proposed a model showing the possible mechanism of S. mutabilis UAE1 to stimulate the growth of gray mangrove under seawater irrigation and greenhouse conditions (Figure 9). We could not eliminate the possibility that the PGP activity of S. mutabilis UAE1 might be due to other mechanisms/metabolites that were not examined in this study. Elucidating regulatory mechanisms responsible for the PGP ability of this endophytic actinobacterium is on top of our priorities. Although the endophytic nature of S. mutabilis exerted beneficial effects on plant development, mobilization of this actinobacterial strain within tissues and translocation of PAs/PGRs from roots to stems could maximize the efficiency of PGP properties in plant tissues. Among other strategies, bioaccumulation (from soil to root or inside colonizing tissues) or translocation (from root to shoot tissues), PGPB promote growth and enhance phytoremediation (Ma et al., 2016; Liu et al., 2017). In our study, PAs produced by S. mutabilis UAE1 to promote growth of mangrove could be attributed to the expression of genes mediating auxins, CKs and by reducing ABA contents in cells.
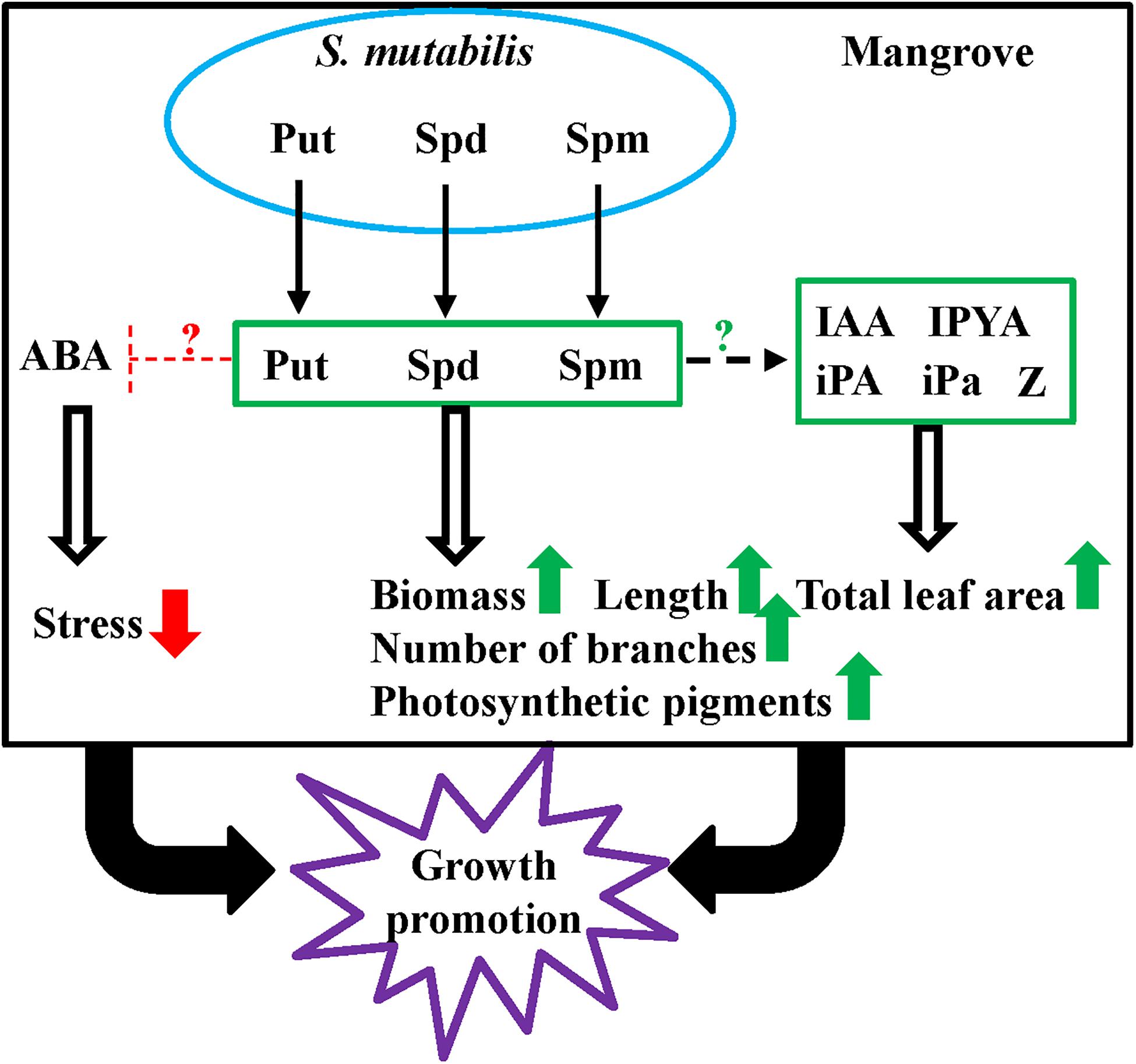
Figure 9. Model of the endophytic Streptomyces mutabilis UAE1 promoting growth of mangrove. The increased PA (Put, Spd, and Spm) levels produced by the endophytic S. mutabilis UAE1 induce endogenous contents of Put, Spd, and Spm in tissues of mangrove, resulting in increasing other PGRs in plant tissues. In planta ABA was reduced, resulting in stress relief in plant tissues. This ultimately leads to increase biomass and length of roots and stems, number of branches of stems, and photosynthetic pigments in leaves. Taken together, the PA-producing isolate S. mutabilis UAE1 has the ability to promote growth of mangrove seedlings. Green and red arrows represent increase/stimulation and decrease/inhibition, respectively. ?, unknown induction/suppression mechanism. PA, polyamine; Put, putrescine; Spd, spermidine, Spm, spermine; IAA, indole-3-acetic acid; IPYA, indole-3-pyruvic acid; iPA, isopentenyl adenine; iPa, isopentenyl adenoside; Z, zeatin; GA3, gibberellic acid; ABA, abscisic acid.
This is the first study to utilize PA-producing microorganisms to enhance growth of mangrove under greenhouse conditions. These findings would in the future be adapted to natural competitive nursery and/or field environments. The endophytic actinobacterial isolate S. mutabilis UAE1, displaying all these PGP characteristics and boosting plant productivity in such saline conditions similar to those found in the UAE, can potentially be up-scaled to the field level. In the long run, this beneficial approach can be used in coastal areas of the Arabian Gulf to propagate marine plant species (e.g., mangrove) for reforestation purposes.
Data Availability Statement
The datasets presented in this study can be found in online repositories. The names of the repository/repositories and accession number(s) can be found below: The 16S rRNA gene sequences of Streptomyces mutabilis UAE1 and Streptomyces sp. 19-388 were deposited in Genbank accession numbers MT883496 and MT883497, respectively.
Author Contributions
KE-T and SA conceived and designed the research, supervised the study, analyzed the data, and wrote the manuscript. AE, AH, and EG performed the greenhouse experiments. SA developed the phylogenetic analyses. KE-T, GR, and ST performed the microscopic experiments. AE, AH, EG, and MA assisted with the experiments and/or data evaluation. All authors critically revised the manuscript and approved the final version.
Funding
This project was funded by the Abu Dhabi Research Award (AARE2019) for Research Excellence-Department of Education and Knowledge (ADEK-007; Grant No. 21S105) to KE-T and the Khalifa Center for Biotechnology and Genetic Engineering-UAEU (Grant No. 12R028) to SA.
Conflict of Interest
The authors declare that the research was conducted in the absence of any commercial or financial relationships that could be construed as a potential conflict of interest.
Publisher’s Note
All claims expressed in this article are solely those of the authors and do not necessarily represent those of their affiliated organizations, or those of the publisher, the editors and the reviewers. Any product that may be evaluated in this article, or claim that may be made by its manufacturer, is not guaranteed or endorsed by the publisher.
Acknowledgments
KE-T would like to thank the library at the Murdoch University, Australia for the valuable online resources and comprehensive databases.
Supplementary Material
The Supplementary Material for this article can be found online at: https://www.frontiersin.org/articles/10.3389/fmars.2021.710200/full#supplementary-material
Footnotes
References
Acuna, J. J., Campos, M., Mora, M. L., Jaisi, D. P., and Jorquera, M. A. (2019). ACCD-producing rhizobacteria from an Andean Altiplano native plant (Parastrephia quadrangularis) and their potential to alleviate salt stress in wheat seedlings. Appl. Soil Ecol. 136, 184–190. doi: 10.1016/j.apsoil.2019.01.005
Ahmed, S., Kamruzzaman, M., Azad, M. S., and Khan, M. N. I. (2021). Fine root biomass and its contribution to the mangrove communities in three saline zones of Sundarbans, Bangladesh. Rhizosphere 17:100294. doi: 10.1016/j.rhisph.2020.100294
Alcázar, R., Bueno, M., and Tiburcio, A. F. (2020). Polyamines: small amines with large effects on plant abiotic stress tolerance. Cells 9:2373. doi: 10.3390/cells9112373
Allard, S. M., Costa, M. T., Bulseco, A. N., Helfer, V., Wilkins, L. G. E., Hassenrück, C., et al. (2020). Introducing the mangrove microbiome initiative: identifying microbial research priorities and approaches to better understand, protect, and rehabilitate mangrove ecosystems. mSystems 5:e00658-20.
Almahasheer, H. (2018). Spatial coverage of mangrove communities in the Arabian Gulf. Environ. Monit. Assess. 190:85.
Alongi, D. (2002). Present state and future of the world’s mangrove forests. Environ. Conserv. 29, 331–349. doi: 10.1017/s0376892902000231
Arena, M. E., and Manca de Nadra, M. C. (2001). Biogenic amine production by Lactobacillus. J. Appl. Microbiol. 90, 158–162. doi: 10.1046/j.1365-2672.2001.01223.x
Bashan, Y., and Holguin, G. (2002). Plant growth-promoting bacteria: a potential tool for arid mangrove reforestation. Trees 16, 159–166. doi: 10.1007/s00468-001-0152-4
Bashan, Y., Moreno, M., and Troyo, E. (2000). Growth promotion of the seawater-irrigated oilseed halophyte Salicornia bigelovii inoculated with mangrove rhizosphere bacteria and halotolerant Azospirillum spp. Biol. Fertil. Soils 32, 265–272. doi: 10.1007/s003740000246
Bektas, I., and Kusek, M. (2021). Biological control of onion basal rot disease using phosphate solubilising rhizobacteria. Biocontrol Sci. Technol. 31, 190–205. doi: 10.1080/09583157.2020.1839381
Bradford, M. (1976). A rapid and sensitive method for the quantitation of microgram quantities of protein utilizing the principle of protein-dye binding. Anal. Biochem. 72, 248–258. doi: 10.1016/0003-2697(76)90527-3
Burt, J. A. (2014). The environmental costs of coastal urbanization in the Arabian Gulf. City 18, 760–770. doi: 10.1080/13604813.2014.962889
Chen, D., Shao, Q., Yin, L., Younis, A., and Zheng, B. (2019). Polyamine function in plants: metabolism, regulation on development, and roles in abiotic stress responses. Front. Plant Sci. 9:1945. doi: 10.3389/fpls.2018.01945
Christakis, C. A., Daskalogiannis, G., Chatzaki, A., Markakis, E. A., Mermigka, G., Sagia, A., et al. (2021). Endophytic bacterial isolates from halophytes demonstrate phytopathogen biocontrol and plant growth promotion under high salinity. Front. Microbiol. 12:681567. doi: 10.3389/fmicb.2021.681567
Cross, T. (1989). “Growth and examination of actinomycete-some guidelines,” in Bergey’s Manual of Systematic Bacteriology, Vol. 4, eds S. T. Williams, M. E. Sharpe, and J. G. Holt (Baltimore, MD: Williams and Wilkins), 2340–2343.
Davies, B. H. (1965). “Analysis of carotenoid pigments,” in Chemistry and Biochemistry of Plant Pigments, ed. T. W. Goodwin (London: Academic Press), 489–532.
di Menna, M. E. (1957). The isolation of yeasts from soil. J. Gen. Microbiol. 17, 678–688. doi: 10.1099/00221287-17-3-678
Donato, D. C., Kauffman, J. B., Murdiyarso, D., Kurnianto, S., Stidham, M., and Kanninen, M. (2011). Mangroves among the most carbon-rich forests in the tropics. Nat. Geosci. 4, 293–297. doi: 10.1038/ngeo1123
Dworkin, M., and Foster, J. (1958). Experiments with some microorganisms which utilize ethane and hydrogen. J. Bacteriol. 75, 592–601. doi: 10.1128/jb.75.5.592-603.1958
Dye, D. W. (1962). The inadequacy of the usual determinative tests for identification of Xanthomonas spp. N. Z. J. Sci. 5, 393–416.
Elmahdy, S. I., Ali, T. A., Mohamed, M. M., Howari, F. M., Abouleish, M., and Simonet, D. (2020). Spatiotemporal mapping and monitoring of mangrove forests changes from 1990 to 2019 in the Northern Emirates, UAE using random forest, kernel logistic regression and naive bayes tree models. Front. Environ. Sci. 8:102. doi: 10.3389/fenvs.2020.00102
El-Tarabily, K., and Youssef, T. (2010). Enhancement of morphological, anatomical and physiological characteristics of seedlings of the mangrove Avicennia marina inoculated with a native phosphate-solubilizing isolate of Oceanobacillus picturae under greenhouse conditions. Plant Soil 332, 147–162. doi: 10.1007/s11104-010-0280-y
El-Tarabily, K., and Youssef, T. (2011). Improved growth performance of the mangrove Avicennia marina seedlings using a 1-aminocyclopropane-1-carboxylic acid deaminase-producing isolate of Pseudoalteromonas maricaloris. Plant Growth Regul. 65, 473–483. doi: 10.1007/s10725-011-9618-6
El-Tarabily, K. A., AlKhajeh, A. S., Ayyash, M. M., Alnuaimi, L. H., Sham, A., ElBaghdady, K. Z., et al. (2019). Growth promotion of Salicornia bigelovii by Micromonospora chalcea UAE1, an endophytic 1-aminocyclopropane-1-carboxylic acid deaminase-producing actinobacterial isolate. Front. Microbiol. 10:1694. doi: 10.3389/fmicb.2019.01694
El-Tarabily, K. A., ElBaghdady, K. Z., AlKhajeh, A. S., Ayyash, M. M., Aljneibi, R. S., El-Keblawy, A., et al. (2020). Polyamine-producing actinobacteria enhance biomass production and seed yield in Salicornia bigelovii. Biol. Fertil. Soils 56, 499–519. doi: 10.1007/s00374-020-01450-3
El-Tarabily, K. A., Nassar, A. H., Hardy, G. E., and Sivasithamparam, K. (2009). Plant growth promotion and biological control of Pythium aphanidermatum, a pathogen of cucumber, by endophytic actinomycetes. J. Appl. Microbiol. 106, 13–26. doi: 10.1111/j.1365-2672.2008.03926.x
Faria, P. S. A., Marques, V. O., Selari, P. J. R. G., Martins, P. F., Silva, F. G., and Sales, J. F. (2021). Multifunctional potential of endophytic bacteria from Anacardium othonianum Rizzini in promoting in vitro and ex vitro plant growth. Microbiol. Res. 242:126600. doi: 10.1016/j.micres.2020.126600
Felsenstein, J. (1981). Evolutionary trees from DNA sequences: a maximum likelihood approach. J. Mol. Evol. 17, 368–376. doi: 10.1007/bf01734359
Flores, H. E., and Galston, A. W. (1982). Analysis of polyamines in higher plants by high performance liquid chromatography. Plant Physiol. 69, 701–706. doi: 10.1104/pp.69.3.701
Gauze, G. F., Preobrazhenskaya, T. P., Kudrina, E. S., Blinov, N. O., Ryabova, I. D., and Sveshnikova, M. A. (1957). Problems of Classification of Actinomycetes-Antagonists. Medgiz: Government Publishing House of Medical Literature, 1–398.
Giri, C., Ochieng, E., Tieszen, L. L., Zhu, Z., Singh, A., Loveland, T., et al. (2011). Status and distribution of mangrove forests of the world using earth observation satellite data. Glob. Ecol. Biogeogr. 20, 154–159. doi: 10.1111/j.1466-8238.2010.00584.x
Glick, B. R. (2015). “Introduction to plant growth promoting bacteria,” in Beneficial Plant-Bacterial Interactions, ed. B. R. Glick (Cham: Springer International Publishing), 1–28. doi: 10.1007/978-3-030-44368-9_1
Goodfellow, M., and Williams, S. (1983). Ecology of actinomycetes. Annu. Rev. Microbiol. 37, 189–216.
Gordon, S. A., and Weber, R. P. (1951). Colorimetric estimation of indoleacetic acid. Plant Physiol. 26, 192–195. doi: 10.1104/pp.26.1.192
Gouda, S., Kerry, R. G., Das, G., Paramithiotis, S., Shin, H. S., and Patra, J. K. (2018). Revitalization of plant growth promoting rhizobacteria for sustainable development in agriculture. Microbiol. Res. 206, 131–140. doi: 10.1016/j.micres.2017.08.016
Guinn, G., Brummett, D. L., and Beier, R. C. (1986). Purification and measurement of abscisic acid and indole-acetic acid by high performance liquid chromatography. Plant Physiol. 81, 997–1002. doi: 10.1104/pp.81.4.997
Hallmann, J., Quadt-Hallmann, A., Mahaffee, W. F., and Kloepper, J. W. (1997). Bacterial endophytes in agricultural crops. Can. J. Microbiol. 43, 895–914. doi: 10.1139/m97-131
Handa, A. K., Nambeesan, S., Mengiste, T., Laluk, L., AbuQamar, S., and Mattoo, A. K. (2010). Polyamine spermidine is an upstream negator of ethylene-regulated pathogenesis of Botrytis cinerea in tomato leaf. Int. Symp. Tomato Dis. 914, 109–112. doi: 10.17660/actahortic.2011.914.18
Hatmi, S., Villaume, S., Trotel-Aziz, P., Barka, E. A., Clément, C., and Aziz, A. (2018). Osmotic stress and ABA affect immune response and susceptibility of grapevine berries to gray mold by priming polyamine accumulation. Front. Plant Sci. 9:1010. doi: 10.3389/fpls.2018.01010
Holden, M. (1965). “Chlorophylls,” in Chemistry and Biochemistry of Plant Pigments, ed. T. W. Goodwin (London: Academic Press), 462–488.
Holguin, G., Guzman, M. A., and Bashan, Y. (1992). Two new nitrogen-fixing bacteria from the rhizosphere of mangrove trees: their isolation, identification and in vitro interaction with rhizosphere Staphylococcus sp. FEMS Microbiol. Ecol. 101, 207–216. doi: 10.1111/j.1574-6941.1992.tb01657.x
Honma, M., and Shimomura, T. (1978). Metabolism of 1-aminocyclopropane-1-carboxylic acid. Agric. Biol. Chem. 42, 1825–1831. doi: 10.1080/00021369.1978.10863261
Howari, F. M., Jordan, B. R., Bouhouche, N., and WyllieEcheverria, S. (2009). Field and remote-sensing assessment of mangrove forests and seagrass beds in the northwestern part of the United Arab Emirates. J. Coast. Res. 251, 48–56. doi: 10.2112/07-0867.1
Idris, E. E., Iglesias, D. J., Talon, M., and Borriss, R. (2007). Tryptophan-dependent production of indole-3-acetic acid (IAA) affects level of plant growth promotion by Bacillus amyloliquefaciens FZB42. Mol. Plant Microbe Interact. 20, 619–626. doi: 10.1094/mpmi-20-6-0619
Ikeuchi, M., Ogawa, Y., Iwase, A., and Sugimoto, K. (2016). Plant regeneration: cellular origins and molecular mechanisms. Development 143, 1442–1451. doi: 10.1242/dev.134668
Kamil, F. H., Saeed, E. E., El-Tarabily, K. A., and AbuQamar, S. F. (2018). Biological control of mango dieback disease caused by Lasiodiplodia theobromae using streptomycete and non-streptomycete actinobacteria in the United Arab Emirates. Front. Microbiol. 9:829. doi: 10.3389/fmicb.2018.00829
Kathiresan, K., and Selvam, M. M. (2006). Evaluation of beneficial bacteria from mangrove soil. Bot. Mar. 49, 86–88.
Khalid, A., Arshad, M., and Zahir, Z. A. (2004). Screening plant growth-promoting rhizobacteria for improving growth and yield of wheat. J. Appl. Microbiol. 96, 473–480. doi: 10.1046/j.1365-2672.2003.02161.x
Khare, E., Mishra, J., and Arora, N. K. (2018). Multifaceted interactions between endophytes and plant: developments and prospects. Front. Microbiol. 9:2732. doi: 10.3389/fmicb.2018.02732
Killiny, N., and Nehela, Y. (2020). Citrus polyamines: structure, biosynthesis, and physiological functions. Plants 9:426. doi: 10.3390/plants9040426
Kumar, S., Stecher, G., and Tamura, K. (2016). MEGA7: molecular evolutionary genetics analysis version 7.0 for bigger datasets. Mol. Biol. Evol. 33, 1870–1874. doi: 10.1093/molbev/msw054
Kushwaha, P., Kashyap, P. L., Bhardwaj, A. K., Kuppusamy, P., Srivastava, A. K., and Tiwari, R. K. (2020). Bacterial endophyte mediated plant tolerance to salinity: growth responses and mechanisms of action. World J. Microbiol. Biotechnol. 36:26.
Küster, E. (1959). Outline of a comparative study of criteria used in characterization of the actinomycetes. Int. Bull. Bacteriol. Nomencl. Taxon. 9, 97–104. doi: 10.1099/0096266x-9-2-97
Lanneluc-Sanson, D., Phan, C. T., and Granger, R. L. (1986). Analysis by reverse-phase high-pressure liquid chromatography of phenylisothiocyanate-deriderivatized 1-aminocyclopropane-1-carboxylic acid in apple extracts. Anal. Biochem. 155, 322–327. doi: 10.1016/0003-2697(86)90441-0
Li, F. N., Pan, Z., Tuo, L., Liu, S. W., Zuo, X., and Chen, L. (2017). Studies on the diversity and novelty of endophytic actinobacteria isolated from mangrove plants collected in Macao. Chin. J. Antibiot. 42, 26–34.
Liu, H., Carvalhais, L. C., Crawford, M., Singh, E., Dennis, P. G., Pieterse, C. M. J., et al. (2017). Inner plant values: diversity, colonization and benefits from endophytic bacteria. Front. Microbiol. 8:2552. doi: 10.3389/fmicb.2017.02552
Lizada, M. C., and Yang, S. F. (1979). A simple and sensitive assay for 1-aminocyclopropane-1-carboxylic acid. Anal. Biochem. 100, 140–145. doi: 10.1016/0003-2697(79)90123-4
Ma, Y., Oliveira, R. S., Freitas, H., and Zhang, C. (2016). Biochemical and molecular mechanisms of plant-microbe-metal interactions: relevance for phytoremediation. Front. Plant Sci. 7:918. doi: 10.3389/fpls.2016.00918
Machàckovà, I., Krekule, J., Eder, J., Seidlovà, F., and Strnad, M. (1993). Cytokinins in photoperiodic induction of flowering Chenopodium species. Physiol. Plant. 87, 160–166. doi: 10.1111/j.1399-3054.1993.tb00138.x
Mahmoud, A. Y., Abdallah, H. M., El-Halawani, M. A., and Jiman-Fatani, A. A. M. (2015). Anti-tuberculous activity of treponemycin produced by a Streptomyces strain MS-6-6 isolated from Saudi Arabia. Molecules 20, 2576–2590. doi: 10.3390/molecules20022576
Marino, M., Maifreni, M., Moret, S., and Rondinini, G. (2000). The capacity of Enterobacteriaceae species to produce biogenic amines in cheese. Lett. Appl. Microbiol. 31, 169–173. doi: 10.1046/j.1365-2672.2000.00783.x
Mathew, B. T., Torky, Y., Amin, A., Mourad, A. I., Ayyash, M. M., El-Keblawy, A., et al. (2020). Halotolerant marine rhizosphere-competent actinobacteria promote Salicornia bigelovii growth and seed production using seawater irrigation. Front. Microbiol. 11:552. doi: 10.3389/fmicb.2020.00552
Millonig, G. (1976). Laboratory Manual of Biological Electron Microscopy, ed. M. Saviolo, Vercelli. 1–67.
Misaghi, I. J., and Donndelinger, I. J. (1990). Endophytic bacteria in symptom-free cotton plants. Phytopathology 80, 808–811. doi: 10.1094/phyto-80-808
Moretti, L. G., Crusciol, C. A., Kuramae, E. E., Bossolani, J. W., Moreira, A., Costa, N. R., et al. (2020). Effects of growth-promoting bacteria on soybean root activity, plant development, and yield. Agron. J. 112, 418–428. doi: 10.1002/agj2.20010
Murphy, J., and Riley, J. P. (1962). A modified single solution method for the determination of phosphate in natural waters. Anal. Chim. Acta 27, 31–36. doi: 10.1016/s0003-2670(00)88444-5
Musson, G., McInroy, J. A., and Kloepper, J. W. (1995). Development of delivery systems for introducing endophytic bacteria into cotton. Biocontrol Sci. Technol. 5, 407–416. doi: 10.1080/09583159550039602
Nautiyal, C. S. (1999). An efficient microbiological growth medium for screening phosphate solubilizing microorganisms. FEMS Microbiol. Lett. 170, 265–270. doi: 10.1111/j.1574-6968.1999.tb13383.x
Olanrewaju, O. S., Glick, B. R., and Babalola, O. O. (2017). Mechanisms of action of plant growth promoting bacteria. World J. Microbiol. Biotechnol. 33:197.
Ortíz-Castro, R., Valencia-Cantero, E., and López-Bucio, J. (2008). Plant growth promotion by Bacillus megaterium involves cytokinin signaling. Plant Signal. Behav. 3, 263–265. doi: 10.4161/psb.3.4.5204
Pikovskaya, R. I. (1948). Mobilization of phosphorus in soil in connection with the vital activity of some microbial species. Microbiology 17, 362–370.
Preyanga, R., Anandham, R., Krishnamoorthy, R., Senthilkumar, M., Gopal, N. O., Vellaikumar, A., et al. (2021). Groundnut (Arachis hypogaea) nodule Rhizobium and passenger endophytic bacterial cultivable diversity and their impact on plant growth promotion. Rhizosphere 17:100309. doi: 10.1016/j.rhisph.2021.100309
Pridham, R. G., Hesseltine, C. W., and Benedict, R. G. (1958). A guide for the classification of streptomycetes according to selected groups: placement of strains in morphological sections. Appl. Microbiol. 6, 52–79. doi: 10.1128/am.6.1.52-79.1958
Rainey, F. A., Ward-Rainey, N., Kroppenstedt, R. M., and Stackebrandt, E. (1996). The genus Nocardiopsis represents a phylogenetically coherent taxon and a distinct actinomycete lineage: proposal of Nocardiopsaceae fam. nov. Int. J. Syst. Bacteriol. 46, 1088–1092. doi: 10.1099/00207713-46-4-1088
Rangan, P., Subramani, R., Kumar, R., Singh, A. K., and Singh, R. (2014). Recent advances in polyamine metabolism and abiotic stress tolerance. Biomed Res. Int. 2014:239621.
Redmond, J. W., and Tseng, A. (1979). High-pressure liquid chromatographic determination of putrescine, spermidine and spermine. J. Chromatogr. 170, 479–481. doi: 10.1016/s0021-9673(00)95481-5
Rennie, R. J., De Freitas, J. R., Ruschel, A. P., and Vose, P. B. (1982). Isolation and identification of N2-fixing bacteria associated with sugar cane (Saccharum sp.). Can. J. Microbiol. 28, 462–467. doi: 10.1139/m82-070
Rueda-Puente, E., Castellanos, T., Troyo-Diéguez, E., León-Alvarez, J. L., and Murillo-Amador, B. (2003). Effects of a nitrogen-fixing indigenous bacterium (Klebsiella pneumoniae) on the growth and development of the halophyte Salicornia bigelovii as a new crop for saline environments. J. Agron. Crop Sci. 189, 323–332. doi: 10.1046/j.1439-037x.2003.00051.x
Saeed, E. E., Sham, A., Salmin, Z., Abdelmowla, Y., Iratni, R., El-Tarabily, K. A., et al. (2017). Streptomyces globosus UAE1, a potential effective biocontrol agent for black scorch disease in date palm plantations. Front. Microbiol. 8:1455. doi: 10.3389/fmicb.2017.01455
Sardi, P., Saracchi, M., Quaroni, S., Petrolini, B., Borgonovi, G. E., and Merli, S. (1992). Isolation of endophytic Streptomyces strains from surface-sterilized roots. Appl. Environ. Microbiol. 58, 2691–2693. doi: 10.1128/aem.58.8.2691-2693.1992
Schwyn, B., and Neilands, J. B. (1987). Universal chemical assay for the detection and determination of siderophores. Anal. Biochem. 160, 47–56. doi: 10.1016/0003-2697(87)90612-9
Sham, A., Al-Ashram, H., Whitley, K., Iratni, R., El-Tarabily, K. A., and AbuQamar, S. F. (2019). Metatranscriptomic analysis of multiple environmental stresses identifies RAP2.4 gene associated with Arabidopsis immunity to Botrytis cinerea. Sci. Rep. 9:17010.
Sharp, R. E., and LeNoble, M. E. (2002). ABA, ethylene and the control of shoot and root growth under water stress. J. Exp. Bot. 53, 33–37. doi: 10.1093/jxb/53.366.33
Shindy, W. W., and Smith, O. E. (1975). Identification of plant hormones from cotton ovules. Plant Physiol. 55, 550–554. doi: 10.1104/pp.55.3.550
Shirling, E. B., and Gottlieb, D. (1966). Methods for characterization of Streptomyces species. Int. J. Syst. Bacteriol. 16, 313–340. doi: 10.1099/00207713-16-3-313
Smith, M. A., and Davies, P. J. (1985). Separation and quantitation of polyamines in plant tissue by high performance liquid chromatography of their dansyl derivatives. Plant Physiol. 78, 89–91. doi: 10.1104/pp.78.1.89
Strzelczyk, E., and Pokojska-Burdziej, A. (1984). Production of auxins and gibberellin-like substances by mycorrhizal fungi, bacteria and actinomycetes isolated from the soil and the mycorrhizosphere of pine (Pinus silvestris L.). Plant Soil 81, 185–194. doi: 10.1007/bf02197150
Sturz, A. V., Christie, B. R., and Matheson, B. G. (1998). Associations of bacterial endophyte populations from red clover and potato crops with potential for beneficial allelopathy. Can. J. Microbiol. 44, 162–167. doi: 10.1139/w97-146
Thompson, J. D., Gibson, T. J., Plewniak, F., Jeanmougin, F., and Higgins, D. G. (1997). The CLUSTAL_X windows interface: flexible strategies for multiple sequence alignment aided by quality analysis tools. Nucleic Acids Res. 25, 4876–4882. doi: 10.1093/nar/25.24.4876
Tien, T. M., Gaskings, M. H., and Hubbell, D. H. (1979). Plant growth substances produced by Azospirillum brasilense and their effect on the growth of pearl millet (Pennisetum americanum L.). Appl. Environ. Microbiol. 37, 1016–1024. doi: 10.1128/aem.37.5.1016-1024.1979
Toumatia, O., Compant, S., Yekkour, A., Goudjal, Y., Sabaou, N., Mathieu, F., et al. (2016). Biocontrol and plant growth promoting properties of Streptomyces mutabilis strain IA1 isolated from a Saharan soil on wheat seedlings and visualization of its niches of colonization. S. Afr. J. Bot. 105, 234–239. doi: 10.1016/j.sajb.2016.03.020
Tregarot, E., Caillaud, A., Cornet, C. C., Taureau, F., Catry, T., Cragg, S. M., et al. (2021). Mangrove ecological services at the forefront of coastal change in the French overseas territories. Sci. Total Environ. 763:14004.
Wellington, E. M. H., and Williams, S. T. (1977). Preservation of actinomycete inoculum in frozen glycerol. Microbios Lett. 6, 151–157.
Williams, S. T., Shameemullah, M., Watson, E. T., and Mayfield, C. I. (1972). Studies on the ecology of actinomycetes in soil. VI. The influence of moisture tension on growth and survival. Soil Biol. Biochem. 4, 215–225. doi: 10.1016/0038-0717(72)90014-4
Wu, J., Shu, S., Li, C., Sun, J., and Guo, S. (2018). Spermidine-mediated hydrogen peroxide signaling enhances the antioxidant capacity of salt-stressed cucumber roots. Plant Physiol. Biochem. 128, 152–162. doi: 10.1016/j.plaphy.2018.05.002
Xu, C., Wu, X., and Zhang, H. (2009). Impact of D-Arg on drought resistance and endogenous polyamines in mycorrhizal Pinus massoniana. J. Nanjing For. Univ. 33, 019–023.
Xu, M., Li, J., Dai, S. J., Gao, C. Y., Liu, J. M., and Tuo, L. (2016). Study on diversity and bioactivity of actinobacteria isolated from mangrove plants collected from Zhanjiang in Guangdong Province. Chin. J. Antibiot. 41, 26–34.
Keywords: endophytic actinobacteria, halophyte, mangrove, plant growth promotion, polyamines
Citation: El-Tarabily KA, Ramadan GA, Elbadawi AA, Hassan AH, Tariq S, Ghazal EW, Abo Gamar MI and AbuQamar SF (2021) The Marine Endophytic Polyamine-Producing Streptomyces mutabilis UAE1 Isolated From Extreme Niches in the Arabian Gulf Promotes the Performance of Mangrove (Avicennia marina) Seedlings Under Greenhouse Conditions. Front. Mar. Sci. 8:710200. doi: 10.3389/fmars.2021.710200
Received: 15 May 2021; Accepted: 24 June 2021;
Published: 23 July 2021.
Edited by:
Huda Mahmoud, Kuwait University, KuwaitReviewed by:
Christopher Milton Mathew Franco, Flinders University, AustraliaSven E. Beer, Tel Aviv University, Israel
Copyright © 2021 El-Tarabily, Ramadan, Elbadawi, Hassan, Tariq, Ghazal, Abo Gamar and AbuQamar. This is an open-access article distributed under the terms of the Creative Commons Attribution License (CC BY). The use, distribution or reproduction in other forums is permitted, provided the original author(s) and the copyright owner(s) are credited and that the original publication in this journal is cited, in accordance with accepted academic practice. No use, distribution or reproduction is permitted which does not comply with these terms.
*Correspondence: Khaled A. El-Tarabily, a3RhcmFiaWx5QHVhZXUuYWMuYWU=; Synan F. AbuQamar, c2FidXFhbWFyQHVhZXUuYWMuYWU=