- 1British Antarctic Survey, Cambridge, United Kingdom
- 2College of Life and Environmental Sciences, University of Exeter, Exeter, United Kingdom
- 3Plymouth Marine Laboratory, Plymouth, United Kingdom
In aquatic environments, plastic pollution occurs concomitantly with anthropogenic climate stressors such as ocean acidification. Within the Southern Ocean, Antarctic krill (Euphausia Superba) support many marine predators and play a key role in the biogeochemical cycle. Ocean acidification and plastic pollution have been acknowledged to hinder Antarctic krill development and physiology in singularity, however potential multi-stressor effects of plastic particulates coupled with ocean acidification are unexplored. Furthermore, Antarctic krill may be especially vulnerable to plastic pollution due to their close association with sea-ice, a known plastic sink. Here, we investigate the behaviour of nanoplastic [spherical, aminated (NH2), and yellow-green fluorescent polystyrene nanoparticles] in Antarctic seawater and explore the single and combined effects of nanoplastic (160 nm radius, at a concentration of 2.5 μg ml–1) and ocean acidification (pCO2 ∼900, pHT 7.7) on the embryonic development of Antarctic krill. Gravid female krill were collected in the Atlantic sector of the Southern Ocean (North Scotia Sea). Produced eggs were incubated at 0.5 °C in four treatments (control, nanoplastic, ocean acidification and the multi-stressor scenario of nanoplastic presence, and ocean acidification) and their embryonic development after 6 days, at the incubation endpoint, was determined. We observed that negatively charged nanoplastic particles suspended in seawater from the Scotia Sea aggregated to sizes exceeding the nanoscale after 24 h (1054.13 ± 53.49 nm). Further, we found that the proportion of embryos developing through the early stages to reach at least the limb bud stage was highest in the control treatment (21.84%) and lowest in the multi-stressor treatment (13.17%). Since the biological thresholds to any stressors can be altered by the presence of additional stressors, we propose that future nanoplastic ecotoxicology studies should consider the changing global ocean under future climate scenarios for assessments of their impact and highlight that determining the behaviour of nanoplastic particles used in incubation studies is critical to determining their toxicity.
Introduction
In the Southern Ocean, plastic debris has been detected from surface waters (e.g., Jones-Williams et al., 2020; Suaria et al., 2020) to the sea-floor (e.g., Munari et al., 2017; Reed et al., 2018), and across a range of Antarctic biota such as pelagic amphipods (Jones-Williams et al., 2020), pelagic and demersal fishes (Cannon et al., 2016), and benthic invertebrates (Sfriso et al., 2020). Oceanic plastic exists in a continuum of sizes, from the macro to nanoscale (Ter Halle et al., 2017), though size classifications are still evolving. Here, we use the definition provided by Hartmann et al. (2019), referring to a nanoplastic size criterion of <1 micron (μm) to conform to existing definitions for nanomaterials. Whilst the technical challenges of determining exactly how much nanoscale plastic exists in the natural environment have thus far limited the detection of nanoplastic in the Southern Ocean, nanoplastic is predicted to be as pervasive as microplastic (Alimi et al., 2017). Even concerning the better studied microplastic, there is a paucity of data in the Southern Ocean with studies specifically dedicated to microplastic investigation emerging only from 2017 (Tirelli et al., 2020). Consequently, determining the concentration of plastic pollution in the region remains challenging (Cincinelli et al., 2017; Isobe et al., 2017; Lacerda et al., 2019; Jones-Williams et al., 2020; Suaria et al., 2020). Reports of microplastics range from an absence (Kuklinskia et al., 2019) to dense concentrations comparable to concentrations observed in the Northern Hemisphere oceans. For example, Lacerda et al. (2019) estimated a mean microplastic concentration in oceanic surface waters of 1794 km–2 relative to the estimated density range for over 70% of the world’s oceans of 1000–100,000 particles km–2, whilst Isobe et al. (2017) estimated microplastic particles in the order of 100,000 km–2 through the entire water column at sample sites closest to Antarctica. Nevertheless, across studies, the abundance of pelagic microplastics in the Southern Ocean are generally lower than global trends (Tirelli et al., 2020). However, since Antarctica is further from major sources of plastic, transport to the region may take longer and consequently the Southern Ocean may have a larger proportion of plastics within the smaller size classifications than other regions due to fragmentation en route (Obbard, 2018).
As the smallest plastic fraction, nanoplastics are perhaps more hazardous than their micro counterparts (Koelmans et al., 2015). Their greater surface area to volume ratio compared to larger plastics increases their reactivity in marine environments (Tallec et al., 2019). Whilst plastics characteristically have hydrophobic surfaces with no net electrostatic charge, this changes rapidly in seawater as particles accumulate substances from the water column and are subjected to natural processes such as weathering (Galloway et al., 2018). Laboratory studies have tried to assess nanoplastics with differing surface charges (no-charge, carboxylated, and aminated) in simplified conditions of natural seawater, and highlight that surface functionalisation plays a critical role in the behaviours of particles, such as agglomeration (Della Torre et al., 2014; Bergami et al., 2016; Manfra et al., 2017; Tallec et al., 2019). Additionally, in-vivo ecotoxicity studies show that the surface charge of nanoplastics leads to differing exposure routes as well as differing biological responses of marine biota at both cellular and organism level (Corsi et al., 2020). Generally, negatively charged plastic particles (such as carboxylated particles) with a high ionic strength quickly agglomerate within seawater (Lee et al., 2019; Tallec et al., 2019) forming micro-scale aggregates easily ingestible by zooplankton, whilst positively charged particles (including many aminated particles) tend to maintain their nano-specific properties and size (Della Torre et al., 2014; Bergami et al., 2016; Manfra et al., 2017; Pinsino et al., 2017). Aminated particles retaining their smaller size facilitates translocation into cells and triggers physiological disruption such as embryotoxicity (Bergami et al., 2020). Consequently, aminated particles are generally shown to be more toxic to cells and tissues in comparison to carboxylated particles, or those with no surface functionalisation (Corsi et al., 2020).
Nanoplastic may plausibly enter the Antarctic ecosystem via both local and long-range sources (Rowlands et al., 2021). However, research exploring the impacts of nanoplastic on polar species is limited. Polystyrene spheres (40–50 nm, at concentrations of 1 and 5 μg ml–1) were observed to impair immune responses of Antarctic sea urchin (Sterechinus neumayeri) (Bergami et al., 2019), and with the same polymer (50–60 nm, at a concentration of 2.5 μg ml–1) sublethal effects such as reduced swimming behaviour and increased exuviae production were observed in juvenile Antarctic krill (Euphausia superba) (Bergami et al., 2020).
Global trends of ocean acidification, which induce fundamental changes in seawater chemistry including a reduction of seawater pH and carbonate ion concentrations, are exacerbated in the Southern Ocean (Fabry et al., 2011; McBride et al., 2014). Carbonate ions are naturally low in the region and cold temperatures enhance the solubility of CO2, consequently increasing the rate of ocean acidification. Additionally, upwelling of hypercapnic (high CO2 concentration) deep-sea water and freshening of surface waters from glacial and sea-ice melt contribute to further carbonate under-saturation and variations in pH and pCO2 (McNeil and Matear, 2008). Whilst earlier investigations into the impact of ocean acidification focused on calcifying biota (Orr et al., 2005), ocean acidification has been shown to affect a number of physiological and behavioural processes including acid-base regulation abilities and the energy demands to maintain homeostasis (Lannig et al., 2010) and can therefore be critical for non-calcifying organisms such as krill. By the end of the century, models suggest that that in two key Antarctic krill habitats of the Southern Ocean, the Scotia Sea and Weddell Sea, surface water pCO2 levels may reach 584 and 870 μatm, respectively, whilst pCO2 may reach ∼1400 μatm at depth (300–500 m) in the Weddell Sea (Kawaguchi et al., 2011). Antarctic krill spawn negatively buoyant embryos at the ocean surface which sink to depths of 700–1000 m before hatching, and are therefore exposed to enhanced pCO2 concentration at depth (Quetin and Ross, 1984; Kawaguchi et al., 2011, 2013). Investigating the impact of ocean acidification on Antarctic krill embryos, Kawaguchi et al. (2011) observed no significant effects on embryonic development at 1000 μatm pCO2, however at 2000 μatm pCO2, embryonic development was inhibited before the gastrulation stage in 90% of cases and no hatching was observed. Effects were suggested to be associated with hypercapnia and the intracellular acid-base balance hindering protein function, since early embryonic development does not involve calcification.
In a multi-stressor scenario, harmful impacts can be additive, antagonistic or synergistic where the magnitude of the combined impact is equal to, less than or greater than the sum of the singular effects combined, respectively (Norwood et al., 2003). A meta-analysis exploring the effects of multiple abiotic stressors on marine embryos and larvae determined that synergistic interactions (65% of individual tests) were more common than additive (17%) or antagonistic (17%) interactions (Przeslawski et al., 2015). According to Alava et al. (2018) interactions between climate change (e.g., temperature increases, pH decreases, or pCO2 increases) and pollutants may be either climate change dominant, where the climate stressor leads to enhanced susceptibility of biota to chemical toxicity, or contaminant dominant, where chemical exposure leads to an increase in climate stressor susceptibility. Ocean acidification has also been shown to alter bioaccumulation rates of contaminants (Braune et al., 2014; Shi et al., 2016; Alava et al., 2018) and lead to antagonistic, additive or synergistic interactions with other pollutants (Pascal et al., 2010; Benedetti et al., 2016; Lewis et al., 2016; Wilson-McNeal et al., 2020). Another mechanism by which plastic pollution and ocean acidification may interact in their toxicity is via variations in seawater pH modifying the chemical equilibrium of plastics. For example, Piccardo et al. (2020) observed an ultrastructural change of microplastics exposed to acidified conditions at the nanoscale-level, enabling release of chemical additives from the plastic and enhancing ecotoxicity. Despite interaction abilities, plastics are rarely considered in a multi-stressor scenario. However, synergisms between plastic and ocean acidification were observed to negatively impact marine organisms in both the East China Sea (Wang et al., 2020) and in temperate regions (Piccardo et al., 2020), impacting digestion and growth rates, respectively. The potential cumulative impact of these stressors on Antarctic organisms has not yet been explored.
Antarctic krill (E. superba), referred to hereafter as krill, potentially have heightened vulnerabilities to multi-stressor scenarios due to their physiology, behavioural traits and the rapid environmental changes of their habitats (Rowlands et al., 2021). The stenothermal species has weak genetic differentiation (Zane and Patarnello, 2000) and must contend with a plethora of stressors. As well as the developing embryos being exposed to large fluctuations in carbonate chemistry, krill have potentially elevated exposure risks to plastic debris due to a close association with sea-ice, a known sink for plastic (Peeken et al., 2018; Kelly et al., 2020). Furthermore, beyond a temperature optimum, increasing temperatures are demonstrated to reduce the growth rate of adults (Atkinson et al., 2006) and hatching success of embryos (Perry et al., 2020). Combined stressors are hypothesised to lead to increased energy expenditure for krill embryos to maintain homeostasis (Perry et al., 2020) and may impact a number of physiological, biochemical, and molecular parameters (Kawaguchi et al., 2011). As the keystone species of a highly productive system, the demand on krill is high in this dominant yet simplistic diatom–zooplankton–predator food chain (Murphy et al., 2007; Atkinson et al., 2009) and their role in the biogeochemical cycle is significant (Belcher et al., 2019; Manno et al., 2020). The potential impact of multiple environmental stressors on krill development is therefore critical to explore.
Here, we determine the behaviour of aminated polystyrene nanoplastic in Antarctic seawater in terms of dispersion and stability. We assess the ability of aminated nanoplastic to adhere to and permeate the outermost membrane of the krill embryo (the chorion) and explore the impact this may have on the development of embryos from krill collected in the Scotia Sea, within the Atlantic sector of the Southern Ocean. The sampling location is a particularly high biomass region for krill, with 50% of all Southern Ocean post-larval biomass contained within this sector (Atkinson et al., 2009). We focus our study on krill embryonic development since early life stages are generally considered the most vulnerable to anthropogenic stressors (Kurihara, 2008). We extend existing studies on the sensitivity of krill embryos to future ocean acidification conditions (Kawaguchi et al., 2011, 2013) by exploring the potential cumulative effects of nanoplastic coupled with ocean acidification.
Materials and Methods
Behaviour of Nanoplastic in Antarctic Seawater
To understand the behaviour (surface charge and aggregation state) of polystyrene spheres during the incubation experiment, we tested the stability of 170 nm diameter amino modified polystyrene (PS-NH2) spheres (1% w/v, 2 ml, Spherotech) in open ocean water collected from nearby to the krill collection site (King Edward Point bay, South Georgia, 54.260°S, 36.439°W). We compared the behaviour of nanoplastic in seawater with its behaviour in Milli-Q water, having filtered both media through a polycarbonate membrane (pore size of 100 nm). Nanoplastic stock was vortexed for 30 s and bath sonicated for 60 s prior to dilution. Dynamic Light Scatter (DLS) analyses (Zetasizer Ultra; Malvern Instruments) determined the aggregation state (polydispersity index, PDI), the hydrodynamic diameter of particles/aggregates (Z-average; nm) and the mean surface charge (ζ-potential; mV) of nanoplastic. Measurements were performed in triplicate at 0.5°C in 50 μg ml–1 nanoplastic suspensions at T0 and T24h.
Krill Collection and Embryo Selection
Krill were collected aboard the RRS Discovery in January 2019 (Cruise DY098) using a targeted Rectangular Midwater Trawl with a mouth area of 8 m2 (RMT8) in the northern Scotia Sea (outbound trawl 53.837°S, 39.180°W, inbound trawl 53.808°S, 39.160°W). Active and undamaged gravid females were selected and transferred into individual 500 ml perforated plastic containers within a 320 L holding tank within 30 min of the net recovery. A constant flow of uncontaminated seawater from the ship’s underway pump (∼6 m depth) ensured a turnover of fresh oxygenated seawater and provided the females with a natural food supply (for full protocol, see Perry et al., 2020). As broadcast-spawners, Antarctic krill shed their eggs freely into the water (Gómez-Gutiérrez and Robinson, 2005). As such, mesh with 1 mm apertures were attached ∼25 mm above the base of each container to protect sinking eggs from cannibalism. Krill were kept in darkened conditions and checked every 12 h for spawning.
To control for genetic variability within our incubation experiment, eggs from the brood of a single female krill which was selected at random were utilised. Embryos were selected for incubation from the first spawned egg batch and displayed a layer of fertilisation jelly surrounding the chorion (embryo membrane). The spent female had a wet weight of 1.9 g and length of 60 mm, as measured from the anterior edge of the eye to tip of telson (Morris et al., 1998). Perry et al. (2020), carrying out research during the same cruise, found that embryonic development was not significantly related to wet weight or length of the gravid female krill in the range of 1.25–2.1 g and length of 52–60 mm, respectively; hence we do not expect our results to be affected by the size of the female krill.
Embryo Treatment Conditions
Four separate treatments (detailed below) were carried out during the 6-day experiment on embryo development, with each treatment performed in triplicate. Embryos (n = 673) were split between four treatments, with three replicates (a random subsample of ∼40–60 embryos) per treatment.
Control Treatment
Ambient sea water was collected from the ship’s underway pump in the region that the krill were collected and filtered with a 0.22 μm filter. Non-calibrated salinity measurements were obtained from the ship-fitted system (Surfmet system) output. The pHT was measured directly within incubation wells (Metrohm 826 pH meter, with a LL Primatrode, pH glass electrode and a tris-buffer).
Nanoplastic Treatment
Initial dilutions of fluorescently labelled 160 nm amino (PS-NH2) modified polystyrene nanoparticles (yellow-green, 1% w/v, 2 ml, Spherotech) were centrifuged at 3000 revolutions per minute (RPM) for 2 h at 4°C and washed three times with deionized water to remove the bacteriostatic preservatives. Nanoplastic suspensions were prepared at a final concentration of 2.5 μg ml–1 in filtered seawater. The final solutions were vortexed but not sonicated prior to use. A concentration of 2.5 μg ml–1 was chosen based on acute toxicity thresholds observed with other marine zooplankton incubated with polystyrene nanoplastic (Bergami et al., 2017; Manfra et al., 2017) and to align with previous nanoplastic research on Antarctic krill (Bergami et al., 2020). Aminated particles were chosen since they have been shown to be more toxic to cells and tissues under other exposure scenarios and are generally well dispersed under saline conditions (Della Torre et al., 2014). We utilised fluorescent nanoparticles with the intent of quantifying adhesion to embryo membranes and internalisation of particles via fluorescent microscopy. We later opted for alternative analyses due to difficulties with autofluorescence coupled with new insight that fluorophore alone can adhere to and accumulate within internal tissues of larvae (Catarino et al., 2019).
Ocean Acidification Treatment
Ambient sea water was manipulated to represent a pHT of 7.7 and pCO2 level of ∼900 μatm that reflects future ocean acidification states predicted within the depth range (700–1000 m) of krill embryos (Quetin and Ross, 1984; Kawaguchi et al., 2011) through adding a combination of acid (HCl) and base (Na2CO3). The acid and base additions were calculated using seacarb software (Lavigne and Gattuso, 2010). This calculation relies on knowledge of the total alkalinity (TA), dissolved inorganic carbon concentration (DIC) and pH of the seawater, although one of these three parameters can be estimated if values for the other two are known. In this instance, we based the calculation on values determined for pHT and TA. The pHT was measured as per the control. TA was determined through applying a surface-salinity (S) and temperature (T°C) based algorithm, based on Lee et al. (2006) and refined through spatially intensive carbonate chemistry surveys in the region (M. P. Humphreys, personal communication):
Sub-samples of seawater from the incubation set-up were fixed with 2% mercuric chloride for shore-based carbonate chemistry analysis (Table 1A). The TA and DIC of each sample was measured simultaneously by a potentiometric titration system using a technique based on the method of Edmond (1970), with a closed cell described by Goyet et al. (1991). The accuracy (3 mmol kg–1 for TA and 4 mmol kg–1 for DIC) was determined by analysing Certified Reference Material (CRM) with known TA and DIC concentrations. Carbonate saturation state with respect to aragonite (Ωa) was indirectly calculated from TA and DIC data using the CO2 SYS software (Lewis and Wallace, 1998) with carbonate dissociation constants by Mehrbach et al. (1973) refitted by Dickson and Millero (1987) and sulphate dissociation constants by Dickson (1990).
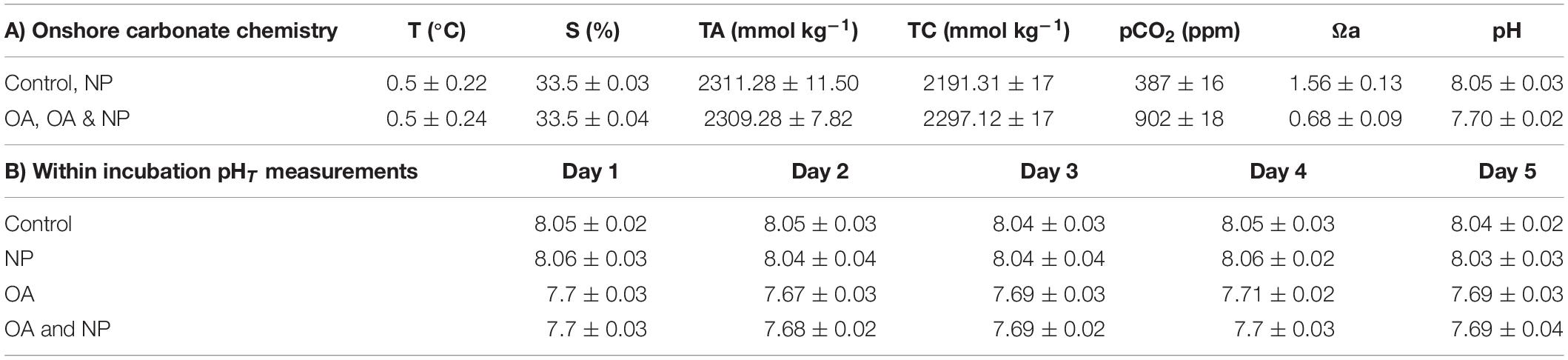
Table 1. Mean ± SD of carbonate system parameters of SW cultures: water temperature (T, °C), total alkalinity (TA, mmol kg–1), total CO2 (TC, mmol kg–1), partial pressure of CO2 (pCO2 ppm), aragonite saturation state (Ωa), salinity (S, %), and pHT.
Nanoplastic and Ocean Acidification Treatment
The multi-stress treatment followed the same method as that of the nanoplastic treatment, with ambient seawater replaced with water manipulated for the ocean acidification treatment, as described above.
Experimental Set-up
Embryos were incubated at 0.5°C in line with Jia et al. (2014) who measured krill development from egg to early juvenile. Due to its antibacterial properties, magnesium chloride (3332 μl L–1) was added to the seawater and ocean acidification solutions prior to them being used to make up each of the four treatments. Treatment solutions were added to 6-well culture plates (10 ml well capacity) to a total volume of 8 ml and sealed with parafilm to minimise CO2 exchange with the atmosphere.
Experimental Monitoring
The pHT was monitored throughout the incubation with direct measurements every 24 h (Table 1B). At the midway point (day 3) the incubation water for each treatment was replaced to minimise the impact of embryo respiration on the dissolved oxygen content of the water. Total oxygen consumption of 60 krill embryos for 6 days is predicted to be 15–30 μl (Ikeda, 1984; Yoshida et al., 2004).
Embryos were imaged every 12 h throughout the 6-day incubation (Olympus SZX16 stereomicroscope with a Canon camera appended via a 0.5 magnification lens adaptor). Imaging was carried out within a cold laboratory set at the lowest possible temperature (∼2°C) and imaging time was kept to a minimum to limit temperature impact during observations. Hatched larvae were removed from the experiments to prevent damage to the remaining incubating embryos and preserved on the sixth day at the experiment endpoint along with unhatched embryos.
Embryonic Analysis
Chorion Examination and Nanoplastic Internalisation
To test for adhesiveness of the nanoplastic spheres used in the experiment, embryos from the incubation were preserved in formalin (4%) fixative for onshore analyses. Post-cruise, we investigated the chorions of a sub-sample of embryos from the nanoplastic and multi-stress treatment (n = 10 per treatment) using Scanning Electron Microscope (Zeiss GeminiSEM 500). Embryos were first cleansed of the fixative with Milli-Q water and subjected to an ethanol dehydration gradient. In addition, to quantify any internalised nanoplastic spheres, a further subsample of embryos from the nanoplastic and multi-stress treatment (n = 10 per treatment) were digested (10% KOH, 60°C, 48 h) and filter papers (polycarbonate, diameter 12 mm, pore size 100 nm) containing residual were SEM imaged.
Embryonic Development Scoring
Embryonic development at the end of the incubation period was categorised into five stages comprising of single cell, two cell, multi cell, limb bud, and nauplius using the photographs of Jia et al. (2014) for identification, plus an additional category for entrapped nauplii. Embryos classified as unidentifiable were assumed to be damaged and removed from analyses (n = 12).
Data Analysis
Statistical analyses were conducted in R Studio version 1.4.1103. A binomial regression was used to determine the probability of embryos reaching the limb bud or later stage differing between treatments with assumptions met, i.e., no multicollinearity, linearity in the logit reported and independent error terms. The optimal model was backwards selected from the full model, which contained a three-way interaction between all measured explanatory variables [i.e., treatment (control, nanoplastic, ocean acidification, nanoplastic, and ocean acidification), individual well (to account for variance between replicates), and number of embryos per well (to account for the variance in the number of embryos that were randomly pipetted into each well plate)]. We also included two-way interaction terms for the measured explanatory variables, primarily to determine whether any relationship existed between treatment and the number of embryos per well that may have influenced the probability output of embryos reaching the limb bud or later stage. Additionally, we ran the model with a random downsized equal sample (n = 100 per treatment) which yielded the same results in terms of the significant or non-significant effects, and consequently we present the model utilising all available data.
Results
Behaviour of Polystyrene Nanoplastic in Antarctic Seawater
Results of the nanoplastic characterisation study are shown in Table 2. DLS analyses revealed a consistent dispersion and stability of nanoplastic in Milli-Q over 24 h at 0.5°C as indicated by the unaltered Z-average resembling manufacturer specifications and low PDI values. In contrast, agglomerates of nanoplastic particles were observed in seawater from the outset, with agglomerates surpassing current upper thresholds for particles exhibiting nano-specific behaviours (1000 nm) after 24 h (Z-average, 1054.13 ± 53.49 nm). Measurements of the mean surface charge (ζ-potential) confirmed a negative charge of the plastic spheres, with a value of −28.03 ± 0.02 mV in Milli-Q, and a smaller absolute value of −4.50 ± 2.98 mV observed in seawater.
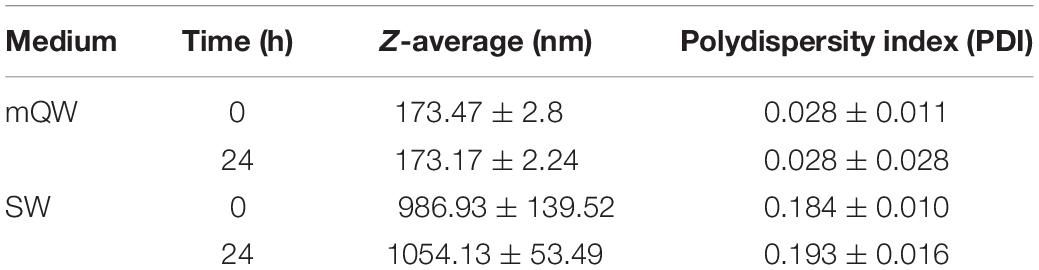
Table 2. Mean ± SD of behavioural parameters of polystyrene nanoplastics (50 μg ml–1) suspended in Milli-Q (mQW) and filtered seawater (FSW) over 24 h.
Chorion Examination and Nanoplastic Internalisation
In the onshore analyses, we firstly confirmed that nanoplastics were easily identifiable via SEM using nanoplastic stock filtered onto a polycarbonate membrane (Figures 1A,B). Examining the chorion of 20 embryos across the nanoplastic and multi-stress treatment with the same SEM method revealed that nanoplastic spheres did not adhere to the chorions (Figures 1C,D). It should be noted that the corrugated surface of krill embryos left some parts of the membrane inaccessible for examination. However, since 20 embryos from nanoplastic treatments were assessed, we would expect to observe some incidences of nanoplastic attachment had the chorion exhibited any adhesive properties. A few nanoplastic particles were observed from the residual of digested embryos from both the nanoplastic and multi-stress incubation (n = 10) rinsed only in Milli-Q (Figures 1E,F), but no nanoplastic was identified in the residual of digested embryos across treatments (n = 10) that underwent additional cleansing steps with both Milli-Q and ethanol. Therefore, the nanoplastic identified in the residual rinsed only with Milli-Q is likely unadhered nanoplastic picked up from the incubation water along with the embryos that withstood the Milli-Q bath rather than nanoplastic that permeated the embryo membrane.
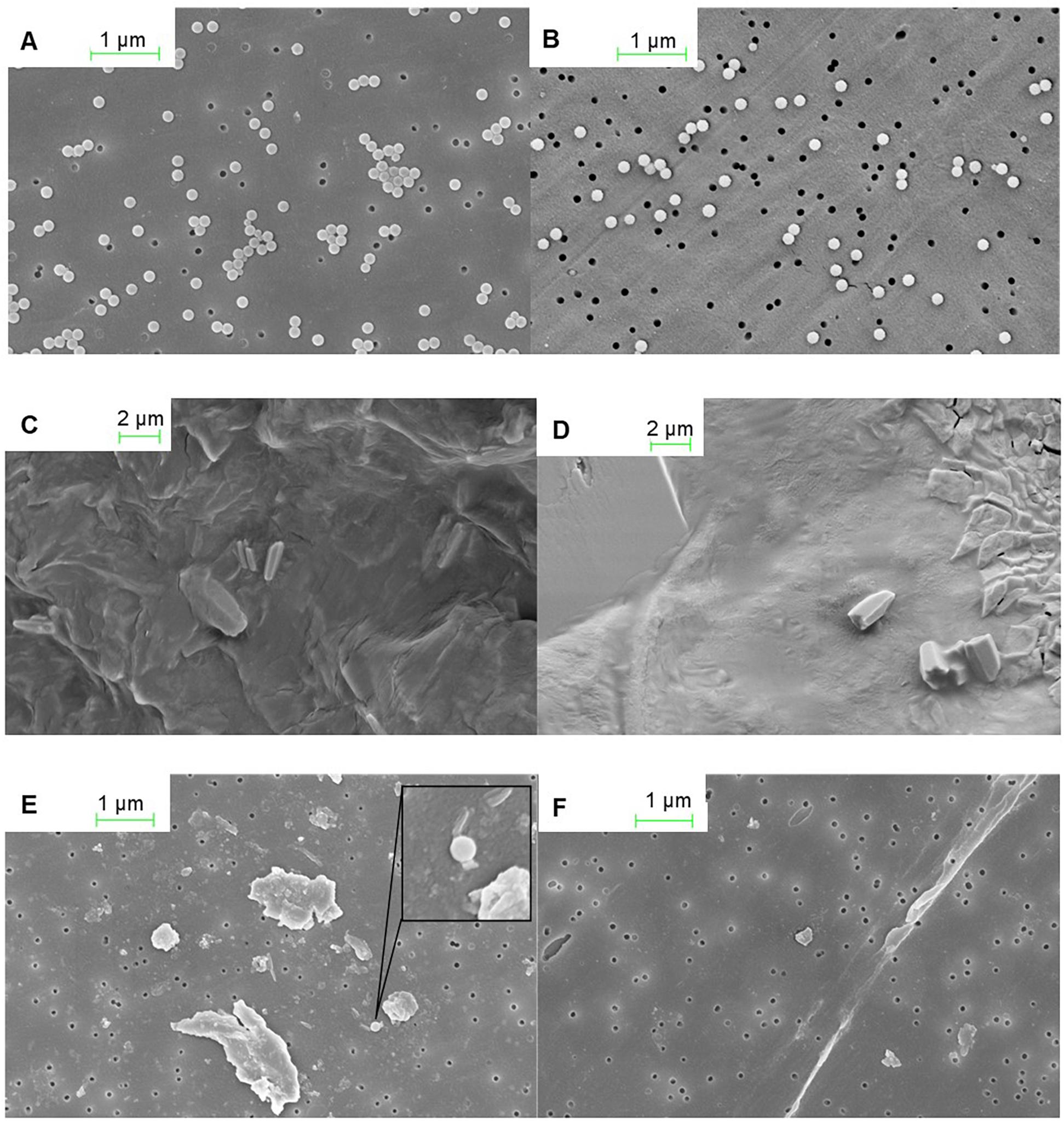
Figure 1. SEM images from nanoplastic investigations of the krill chorion and residual of digested krill embryos. (A) Nanoplastic stock diluted with Milli-Q on a polycarbonate membrane. (B) Nanoplastic stock diluted with Milli-Q on polycarbonate membrane post treatment with10% KOH (60°C, 48 h) to mimic the krill egg digestion. (C) Chorion of a krill embryo from the control treatment. (D) Chorion of a krill embryo from the plastic treatment. (E) Digested (10% KOH, 60°C, 48 h) krill egg residual on a polycarbonate membrane of embryos Milli-Q cleansed. (F) Digested (10% KOH, 60°C, 48 h) krill egg residual on polycarbonate membrane of embryos Milli-Q and ethanol cleansed. SEM images are courtesy of Christian Hacker, University of Exeter.
Embryonic Development
The proportion of embryos reaching at least the limb bud development stage after 6 days of incubation was highest in the control treatment (21.84%) and lowest in the multi-stress treatment (13.17%) (Figure 2). The binomial regression model reflects the data with the probability of embryos developing to at least the limb bud stage significantly lower for the multi-stress treatment compared with the control (B = −1.09 ± 0.39 SE, p = 0.006). No significant difference in embryo development was observed for the singular nanoplastic (B = −0.13 ± 0.34 SE, p = 0.689) or ocean acidification (B = −0.04 ± 0.37 SE, p = 0.920) treatments compared to the control. The probability of reaching the limb bud stage was positively correlated with number of embryos per incubation well (B = 0.04 ± 0.01 SE, p = 0.001) but no interaction effect was observed between treatment and number of eggs per well which was consequently removed from the statistical model.
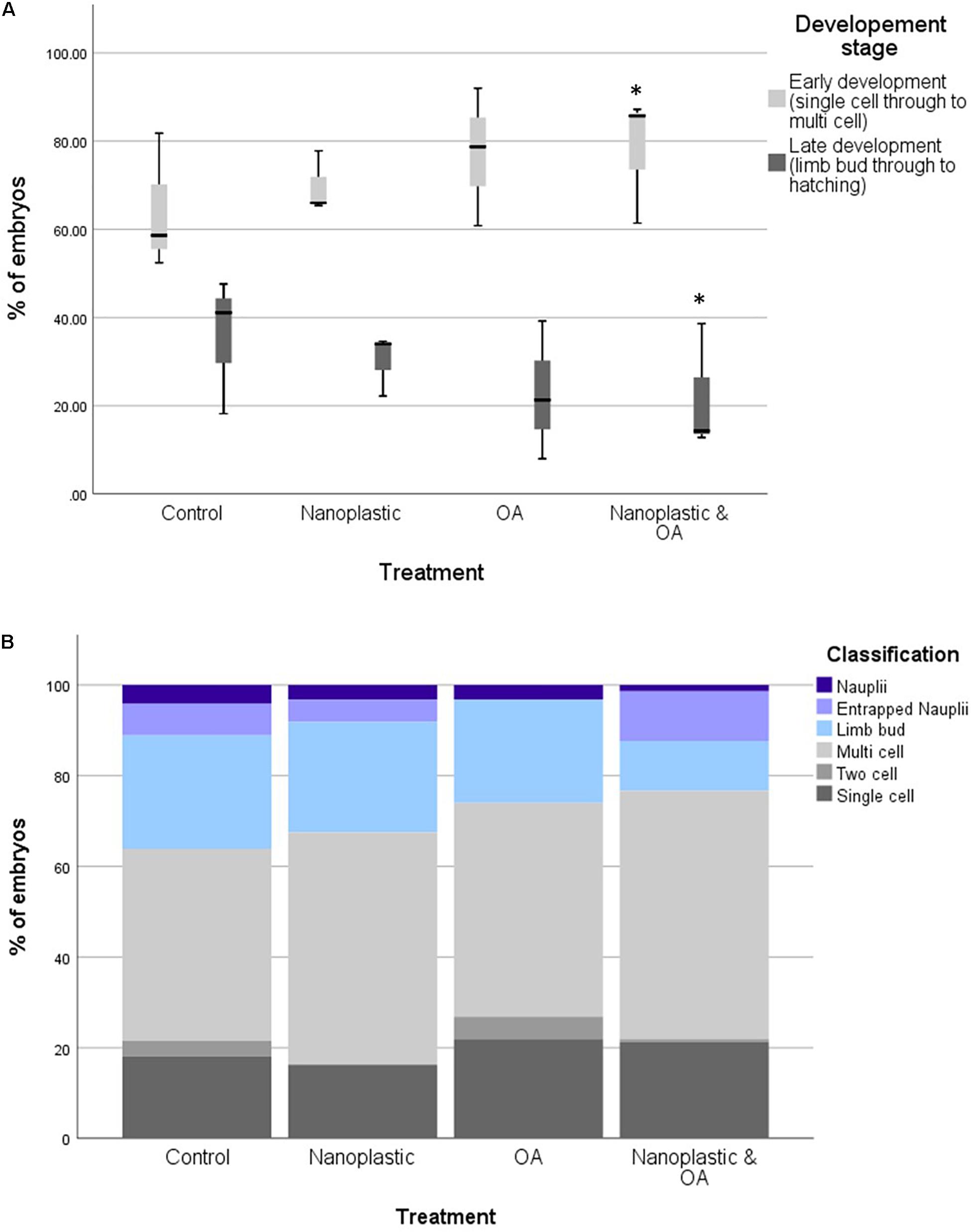
Figure 2. (A) Percentages of krill embryos at the early (single cell to multi cell) and late (limb bud to hatching) developmental stage within each triplicate after 6 days of incubation in treatment conditions. Treatment conditions include control, nanoplastic (NP), ocean acidification (OA), nanoplastic and ocean acidification (NP and OA). Asterisk marks statistically significant difference from the control *<0.05 with results from a binomial GLM. (B) The proportion of krill embryos at the early (single cell to multi cell) and late (limb bud to hatching) developmental stage after 6 days of incubation in treatment conditions. Treatment conditions include control, nanoplastic (NP), ocean acidification (OA), nanoplastic and ocean acidification (NP and OA). Total embryos analysed across the three replicates per treatment were 119 (control), 103 (NP), 107 (OA), and 129 (NP and OA).
Total hatch success rate in the experiment was low at <2% across all treatments (1.66% control, 1.30% nanoplastic, 1.44% ocean acidification, and 0.5% nanoplastic and ocean acidification: Figure 2). These results reflect the low hatching success (mean = 5.7 ± SD 2%) of embryos collected within the same location of South Georgia during the same research cruise also incubated at 0.5°C (Perry et al., 2020). The limited number of nauplii that did hatch did so from 4 days post-fertilisation in line with Perry et al. (2020) who observed hatching from day 4 at 0.5°C. Some developed nauplii became entrapped in the membrane and failed to hatch (Figure 3).
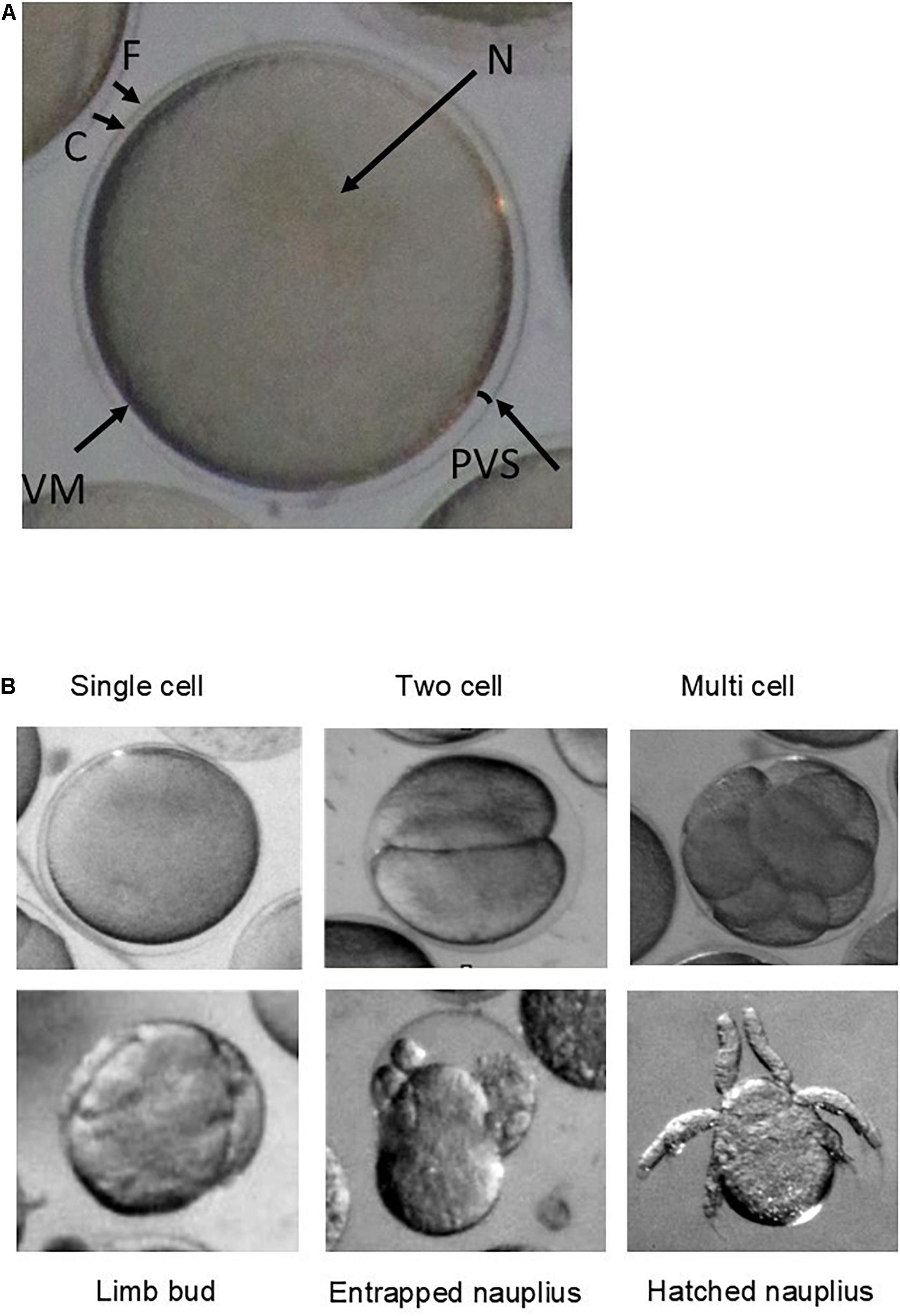
Figure 3. (A) Microscope image (Olympus SZX16 stereomicroscope with a Canon camera appended via a 0.5 magnification lens adaptor) of a newly spawned krill egg from outer to inner embryo: (F) fertilisation jelly, (C) chorion (egg membrane), (PVS) perivitelline space, (VM) vitelline membrane, and (N) nucleus. (B) Microscope images (Olympus SZX16 stereomicroscope with a Canon camera appended via a 0.5 magnification lens adaptor) of each development stage categorised as: single cell, two cell, multiple cell, limb bud, entrapped nauplius, and nauplius.
Discussion
We aimed to determine the vulnerability of Antarctic krill embryos under singular and multi-stressor nanoplastic and ocean acidification exposure scenarios. We found that exposure of embryos to the multi-stressor treatment negatively impacted development, significantly reducing the probability of reaching the limb bud stage compared to the control (B = −1.09 ± 0.39 SE, p = 0.006), with a reduction in reaching the limb bud or later stage of 8.67%. These findings support our hypothesis that a multi-stressor nanoplastic and ocean acidification scenario is detrimental to krill embryogenesis, and impacts are not necessarily addressed when approaching the stressors in singularity.
We observed that whilst nanoplastic spheres remained stable as individual unaggregated particles in Milli-Q water, within seawater the behaviour of nanoplastic spheres changes quickly with the formation of microscale aggregates. This aggregation of nanospheres in seawater contradicts the typical properties of amino modified particles which often have high stability (Della Torre et al., 2014), strong positive charges and remain dispersed due to a sufficiently high ζ-potential ensuring repulsive mechanisms (Lin et al., 2010). Nonetheless, a negative charge of aminated particles has been reported elsewhere when tested in a seawater solution (González-Fernández et al., 2018). A shift towards a smaller absolute value in the ζ-potential of nanoplastic in seawater compared to Milli-Q as we observed (−4.50 ± 2.98 mV in SW, −28.03 ± 0.02 mV in mQW) has also previously been documented (Della Torre et al., 2014; Lee et al., 2019). This reduction in the negative charge of nanoplastics in sea water may be attributed to cations such as Na+, K+, Ca2+, and Mg2+ being attracted to the surface of the nanoplastic as well as due to proteins and other compounds (Lee et al., 2019). The alteration in surface charge can consequently lead to instability in the dispersal of nanoplastic and fast agglomeration since reduced ζ-potential leads to attractive forces between colloids outweighing the repulsive mechanisms, and the particles can then adhere when they collide (Lin et al., 2010). This instability of nanoplastic in seawater is an important consideration since particles lose their nano-specific properties, such as their ability to permeate cells, when the size of aggregates exceeds the nano scale, as observed in our experiment.
When considering internalisation of nanoplastic within our incubated embryos, we have inconclusive evidence that permeation of the krill chorion occurred. Whilst some nanoplastic particles were observed in the residual of the digested embryos rinsed only in Milli-Q water, we believe this to be unadhered nanoplastic picked up from the incubation water along with the embryos that withstood the Milli-Q bath since embryos cleansed more rigorously prior to digestion, i.e., through additional cleansing steps with both Milli-Q water and ethanol, showed no nanoplastic in digested residual. In opposition to our findings, Lee et al. (2019) observed polystyrene nanoplastic internalisation in zebrafish embryos, with 50 nm particles internalised to a much larger extent than 200 and 500 nm particles. They also observed the hydrodynamic diameter of the negatively charged particles to remain stable over 48 h, which is likely attributable to the freshwater environment in which they were tested since the behaviour of nanoplastic in freshwater compared to seawater is known to differ (Koelmans et al., 2015). In our incubation experiment, the lack of internalisation of nanoplastic particles may be explained by using 160 nm nanoplastics, which readily aggregated in Antarctic seawater to the micro-scale.
We also found that the polystyrene nanoplastic spheres did not attach to the krill chorion. Whilst nanoplastic adherence to euphausiid chorions has not previously been explored, our observation differs to that of Kashiwada (2006) who found polystyrene nanoplastic (39.4 nm) adsorbed on the surface of Japanese medaka (Oryzias latipes) embryos, and Lee et al. (2019) who observed polystyrene nanoplastic (50–500 nm) attached to the chorions of zebrafish embryos. Chorion physicality is observed to vary across aquatic organisms. Eggs of some fish species, for example, are observed to have an adhesive chorion whilst others are harder and less adhesive (Goto et al., 2019). Chorions are also known to differ between euphausiid species, for example, Gómez-Gutiérrez et al. (2010) determined that the chorions of Euphausia pacifica eggs were firm, smooth and elastic whilst Thysanoessa spinifera chorions were soft and sticky with particles attached. We observed Antarctic krill chorions to be firm, smooth and non-sticky which may offer an explanation for the lack of nanoplastic adhered. These differences in chorion characteristics, likely impacting nanoplastic adherence, in turn may affect the bioavailability and toxicity of plastic particulates, for example by membrane breakages initiating damage (Nel et al., 2009). Therefore, we suggest that chorion features of krill embryos might offer an additional protection to the exposure of nanoplastic compared to marine organisms (including other euphausiid species) showing a chorion adhesive characteristic.
Regarding embryonic development, hatch success rates were low (<2%) across treatments. A low hatch success rate is not uncommon in Antarctic krill studies with hatch success varying from 0 to 100% (Yoshida et al., 2004; Perry et al., 2020) and often reduced in field based observations, e.g., 0–54% (Perry et al., 2020) and 0–48% (Yoshida et al., 2004) compared to laboratory based observations. The causes of such variability remain somewhat unclear. Yoshida et al. (2004) postulate the high variation in krill hatching success observed in the field observed can be explained by the condition of the female krill when caught, further finding in a laboratory setting that hatch success is influenced by fatty acid composition of embryos, which is influenced by the diet of maternal krill. Yet neither weight nor length of the gravid krill were observed to impact hatch success in observations of Perry et al. (2020). Whilst variability in hatch success of krill embryos is likely influenced by an array of environmental factors, as well as individual krill variability and requires further investigation, we focus on the likelihood of handling effects driving the low hatch success within our incubation. Our low hatch success rate resembled the mean hatching success that Perry et al. (2020) observed in field experiments with embryos collected on the same research cruise, within the same location of South Georgia and incubated at the same temperature of 0.5°C (mean hatching success = 5.7 ± SD 2%). However, Perry et al. (2020) also observed high variability in hatch success across multiple krill from different schools (15% ± SD 17%) and therefore exclude handling effects and experimental set-up as a driver of hatch success. We adopt the same assumption since the gravid female that produced the embryos used in our incubation experiment was kept in the same conditions as other gravid females that produced embryos with high hatch success (Perry et al., 2020). Moreover, Antarctic krill embryos are generally observed to be robust towards handling compared to embryos of other krill species (Harrington and Ikeda, 1986). Consequently, it is our opinion that the limited development to the later embryo stages which occurred even in the control (<22%) and low hatch success rate <2% is representative of Antarctic krill’s reproductive outputs. Nonetheless, our experiment was limited by the availability of gravid females during the research expedition and further incubation experiments would be beneficial to understand whether observed responses to treatments are reflective of the wider krill population.
We observe no significant difference in development between the singular nanoplastic treatment and control for Antarctic krill embryos (B = −0.13 ± 0.34 SE, p = 0.689). Previous studies of other species suggest the effect of nanoplastic on embryogenesis is influenced dramatically by size and charge of the particles. As examples, Tallec et al. (2018) assessed the impact of polystyrene nanoplastic of varying sizes and functionality on Pacific oyster (Crassostrea gigas) embryos, determining that positively charged particles of the smallest size (50 nm) had the strongest toxicity, though uptake of nanoplastic was not quantified. Further, Della Torre et al. (2014) observed positively charged polystyrene nanoplastic spheres (50 nm) caused severe developmental defects in Mediterranean sea urchin (Paracentrotus lividus) embryos while no embryotoxicity was observed for negatively charged nanoplastic (40 nm). The reduced uptake and consequent lower toxicity of negatively charged particles was attributed to the formation of large aggregates in seawater, unlike positive particles which remained dispersed. We expect the same influences regarding surface charge and aggregation (ζ-potential −4.50 ± 2.98 mV with particle aggregation Z-average 1054.13 ± 53.49 nm) for our findings.
We also observe no significant differences between the ocean acidification (pCO2 ∼900 μatm) treatment and ambient control (∼390 μatm) in agreement with Kawaguchi et al. (2011) who observed no significant effect on krill embryonic development at a similar pCO2 (1000 μatm). Further, Kawaguchi et al. (2013) determined that at pCO2 levels above 1000 μatm a sharp decline in hatch success is observed, reaching ∼20% of control levels at 1500 μatm pCO2. Building on findings of Kawaguchi et al. (2011, 2013), we show that when introducing another environmental stressor (in this instance nanoplastic), ocean acidification becomes an important consideration for embryogenesis at a lower pCO2 of 900 μatm. An understanding as to how the pCO2 thresholds alter in a multi-stressor nanoplastic and ocean acidification scenario is therefore required.
Whilst multi-stressor plastic and ocean acidification ecotoxicology studies are limited, Wang et al. (2020) determined that ocean acidification conditions under pH 7.7 enhanced the toxicity of 2 μm polystyrene microplastic to Korean mussels (Mytilus coruscus) by significantly inhibiting digestive enzymes and impacting oxidative responses. However, since the uptake of plastic particulates was not quantified, the underlying cause of the enhanced toxicity is unknown. In our experiment, since nanoplastic was not observed to adhere to or penetrate the chorion, we suggest an indirect effect on the developmental impairment of krill embryos in a multi-stressor scenario, perhaps associated with leachates from the polystyrene spheres. Martínez-Gómez et al. (2017) concluded leachates from fluorescent polystyrene microspheres (6 μm) caused a higher toxicity to sea urchin (P. lividus) embryos than virgin and aged polystyrene themselves, attributing toxicity to styrene/ethylene monomers, as well as unknown toxic chemicals and additives (such as antioxidant) from the polystyrene microsphere production. With the same species, Oliviero et al. (2019) determined microplastic flakes from PVC toys hindered larval development at the highest dose used in their experiments (30 mg L–1) with the main effect being attributed to leached chemicals such as phthalates.
One possible explanation for embryo development being less successful under the multi-stressor scenario (8.67% less than the control) in our study is that the ocean acidification treatment altered the speciation, bioavailability and therefore toxicity of chemicals within the nanoplastic spheres under the reduced pH condition, as previously observed for ionisable chemicals (Millero et al., 2009). Among the different variables that may influence leaching behaviour, Quina et al. (2009) determined pH of the leachant solution to be the most important. Piccardo et al. (2020) observed that sea urchin (P. lividus) larvae, exposed to treatments of polyethylene terephthalate (PET) 0.1 mg ml–1 at various sizes (small: 5–60 μm, medium: 61–499 μm, and large: 500–3000 μm) and PET leachates, present a reduction of limb length under more acidic conditions (pH 7.5) compared to the control (pH 8.0). Adverse effects from the microplastic treatments were also attributed to leaching of chemicals such as nonyl-and octyl-phenols and bisphenol-A, common additives in plastic packaging, since plastic flake sizes were not compatible with the feeding apparatus of the animal. The assumption was further supported by spectral analysis which identified that under a lower pH condition, intensity peaks of PET were reduced, which were attributed to the release of chemical additives and structural modifiers. In the case of our study, the low pH may have altered the leaching of chemicals and/or fluorophores from the fluorescent nanoparticles. Quantifying leachates was beyond the scope of our study, however for broadening our knowledge of nanoplastic toxicity, nanoplastic leachate treatments should be considered in future ecotoxicology experiments and chemical analyses conducted to validate leachate assumptions.
The reduction in embryo development we observed under the multi-stressor condition of nanoplastic and ocean acidification may also be associated with additional energy requirements to maintain homeostasis. Acid/base regulations bear a substantial energetic cost; for example, sea urchins (P. lividus) were observed to have up to a 20-fold increase in gene upregulation, and consequently an increased energy consumption to reach the same developmental stage at a lower pH (7.5–7.0) compared to ambient conditions (Martin et al., 2011). In the aforementioned Piccardo et al. (2020) study, authors suggested that reduced growth of sea urchin within in the ocean acidification/plastic treatments could have been due to increased energy consumption induced by pH stress which consequently enhanced the ecotoxicological responses of the embryos to leachates, i.e., there was a synergistic effect of an increased energy consumption and gene transcription associated with chemical damages. To fully understand the mechanisms driving developmental hindrance in this multi-stressor Southern Ocean scenario, additional in-depth physiological, biochemical, and molecular analyses are required such as correlation with reactive oxygen species (ROS) (e.g., Lee et al., 2019; Wang et al., 2020) and investigation of the impacts on gene expression, cellular morphology and phagocytic activity (e.g., Browne et al., 2008; Bergami et al., 2019).
Conclusion and Future Direction
Ocean acidification is expected to increase in the future, with Southern Ocean pCO2 predicted to rise to 1400 μatm in the depth range of Antarctic krill by the year 2100 (Kawaguchi et al., 2011). Cumulative global plastic production is expected to reach 34 billion metric tons by 2050 (Garside, 2019) with an estimated 4.8–12.7 Mt entering the oceans as macroscopic litter and microplastic particles each year (Geyer et al., 2017; Worm et al., 2017). Regardless of increased plastic input into oceanic systems, the degradation of existing oceanic plastic will facilitate an increase in micro- and nano-scale plastic debris. Here, our results show that the development of Antarctic krill embryos is lowest under a multi-stress nanoplastic and ocean acidification scenario, with a reduction of ∼9% in the probability of embryos reaching later development stages in the observed time period compared to the control. Consequently, results are indicative of the need to consider plastic pollution within a multi-stressor scenario when addressing early development of Antarctic krill. However, results should be interpreted with the understanding that more robust experiments are required to determine whether findings are representative of the wider Antarctic krill population. Additionally, further work is required to quantify nanoplastic doses, sizes, and surface charges and ocean acidification pCO2 thresholds within a multi-stress scenario. Moreover, nanoplastic multi-stress investigations should not be confined to predicted future ocean acidification conditions. Perry et al. (2020) found that water temperatures above 3°C markedly decreased krill hatching success. Ocean warming is evidenced to lead to synergistic reactions with other contaminants in Antarctic species such as Antarctic scallop (Adamussium colbecki) (Benedetti et al., 2016) and stenothermal Antarctic species, such as krill, may be more sensitive to warming-chemical synergisms (Rowlands et al., 2021). Since the biological thresholds to stressors can be lower in combination compared to in singularity, future nanoplastic ecotoxicity studies should begin to acknowledge the changing climate in terms of both ocean acidification and ocean warming for a more realistic representation of existing marine habitats.
Data Availability Statement
The raw data supporting the conclusions of this article will be made available by the authors, without undue reservation.
Author Contributions
ER and CM undertook the conceptualisation, conducted the experimental design and field experiments, and carried out the original drafting of the manuscript. ER did the analysis of krill eggs in the laboratory and data analysis. TG, MC, CL, VP, and ST assisted with data interpretation and provided a general overview. All authors contributed significantly to the redrafting, reviewing, and editing of the manuscript.
Funding
ER was funded by a NERC GW4+ scholarship NE/L002434/1. TG was supported by grants NE/N006178 and NE/S003975/1. CM, VP, and ST were supported by NERC funding to the British Antarctic Survey “Ecosystems” and “Paleo Environments, Ice Sheets and Climate Change” science programmes.
Conflict of Interest
The authors declare that the research was conducted in the absence of any commercial or financial relationships that could be construed as a potential conflict of interest.
Publisher’s Note
All claims expressed in this article are solely those of the authors and do not necessarily represent those of their affiliated organizations, or those of the publisher, the editors and the reviewers. Any product that may be evaluated in this article, or claim that may be made by its manufacturer, is not guaranteed or endorsed by the publisher.
Acknowledgments
We would like to acknowledge the British Antarctic Survey Polar Ocean Ecosystem Time Series (POETS) project, and all involved in krill collection. We thank Franki Perry, Kirstie-Jones Williams, and Bjorg Apeland for assistance with fieldwork as well as RRS Discovery officers and crew (DY098). For bio-imaging support we thank Christian Hacker and for useful discussion on DLS analyses, Diogo Fernandes. For support with onshore carbonate chemistry analyses we also thank S. Sandrini.
References
Alava, J. J., Cisneros-Montemayor, A. M., Sumaila, U. R., and Cheung, W. W. L. (2018). Projected amplification of food web bioaccumulation of MeHg and PCBs under climate change in the Northeastern Pacific. Sci. Rep. 8, 1–12. doi: 10.1038/s41598-018-31824-5
Alimi, O. S., Farner Budarz, J., Hernandez, L. M., and Tufenkji, N. (2017). Microplastics and nanoplastics in aquatic environments: aggregation deposition, and enhanced contaminant transport. Environ. Sci. Technol. 52, 1704–1724. doi: 10.1021/acs.est.7b05559
Atkinson, A., Shreeve, R. S., Hirst, A. G., Rothery, P., Tarling, G. A., Pond, D. W., et al. (2006). Natural growth rates in Antarctic krill (Euphausia superba): II. Predictive models based on food, temperature, body length, sex, and maturity stage. Limnol. Oceanogr. 51, 973–987. doi: 10.4319/lo.2006.51.2.0973
Atkinson, A., Siegel, V., Pakhomov, E. A., Jessopp, M. J., and Loeb, V. (2009). A re-appraisal of the total biomass and annual production of Antarctic krill. Deep. Res. Part I Oceanogr. Res. Pap. 56, 727–740. doi: 10.1016/j.dsr.2008.12.007
Belcher, A., Henson, S. A., Manno, C., Hill, S. L., Atkinson, A., Thorpe, S E., et al. (2019). Krill faecal pellets drive hidden pulses of particulate organic carbon in the marginal ice zone. Nat. Commun. 10:889. doi: 10.1038/s41467-019-08847-1
Benedetti, M., Lanzoni, I., Nardi, A., d’Errico, G., Di Carlo, M., Fattorini, D., et al. (2016). Oxidative responsiveness to multiple stressors in the key Antarctic species, Adamussium colbecki: interactions between temperature, acidification and cadmium exposure. Mar. Environ. Res. 121, 20–30. doi: 10.1016/J.MARENVRES.2016.03.011
Bergami, E., Bocci, E., Vannuccini, M. L., Monopoli, M., Salvati, A., Dawson, K. A., et al. (2016). Nano-sized polystyrene affects feeding, behavior and physiology of brine shrimp Artemia franciscana larvae. Ecotoxicol. Environ. Saf. 123, 18–25. doi: 10.1016/J.ECOENV.2015.09.021
Bergami, E., Krupinski Emerenciano, A., González-Aravena, M., Cárdenas, C. A., Hernández, P., Silva, J. R. M. C., et al. (2019). Polystyrene nanoparticles affect the innate immune system of the Antarctic sea urchin Sterechinus neumayeri. Polar Biol. 42, 743–757. doi: 10.1007/s00300-019-02468-6
Bergami, E., Manno, C., Cappello, S., Vannuccini, M. L., and Corsi, I. (2020). Nanoplastics affect moulting and faecal pellet sinking in Antarctic krill (Euphausia superba) juveniles. Environ. Int. 143:105999. doi: 10.1016/j.envint.2020.105999
Bergami, E., Pugnalini, S., Vannuccini, M. L., Manfra, L., Faleri, C., Savorelli, F., et al. (2017). Long-term toxicity of surface-charged polystyrene nanoplastics to marine planktonic species Dunaliella tertiolecta and Artemia franciscana. Aquat. Toxicol. 189, 159–169. doi: 10.1016/j.aquatox.2017.06.008
Braune, B. M., Gaston, A. J., Hobson, K. A., Gilchrist, H. G., and Mallory, M. L. (2014). Changes in food web structure alter trends of mercury uptake at two seabird colonies in the Canadian Arctic. Environ. Sci. Technol. 48, 13246–13252. doi: 10.1021/es5036249
Browne, M. A., Dissanayake, A., Galloway, T. S., Lowe, D. M., and Thompson, R. C. (2008). Ingested microscopic plastic translocates to the circulatory system of the mussel, Mytilus edulis (L.). Environ. Sci. Technol. 42, 5026–5031. doi: 10.1021/es800249a
Cannon, S. M. E., Lavers, J. L., and Figueiredo, B. (2016). Plastic ingestion by fish in the Southern Hemisphere: a baseline study and review of methods. Mar. Pollut. Bull. 107, 286–291. doi: 10.1016/j.marpolbul.2016.03.057
Catarino, A. I., Frutos, A., and Henry, T. B. (2019). Use of fluorescent-labelled nanoplastics (NPs) to demonstrate NP absorption is inconclusive without adequate controls. Sci. Total Environ. 670, 915–920. doi: 10.1016/j.scitotenv.2019.03.194
Cincinelli, A., Scopetani, C., Chelazzi, D., Lombardini, E., Martellini, T., Katsoyiannis, A., et al. (2017). Microplastic in the surface waters of the Ross Sea (Antarctica): occurrence, distribution and characterization by FTIR. Chemosphere 175, 391–400. doi: 10.1016/J.CHEMOSPHERE.2017.02.024
Corsi, I., Bergami, E., and Grassi, G. (2020). Behavior and bio-interactions of anthropogenic particles in marine environment for a more realistic ecological risk assessment. Front. Environ. Sci. 8:60. doi: 10.3389/fenvs.2020.00060
Della Torre, C., Bergami, E., Salvati, A., Faleri, C. ∥, Cirino, P., Dawson, K. A., et al. (2014). Accumulation and embryotoxicity of polystyrene nanoparticles at early stage of development of sea urchin embryos Paracentrotus lividus. Environ. Sci. Technol. 48, 12302–12311. doi: 10.1021/es502569w
Dickson, A. G. (1990). Standard potential of the (AgCl(s) + 1/2H2(g) = Ag(s) + HCl(aq) cell and the dissociation constant of bisulfate ion in synthetic sea water from 273.15 to 318.15. K. J. Chem. Therm. 22, 113–127.
Dickson, A. G., and Millero, F. J. A. (1987). Comparison of the equilibrium constants for the dissociation of carbonic acid in seawater media. Deep Sea Res Part I. Pergamon J. 34, 1733–1743.
Edmond, J. M. (1970). High precision determination of titration alkalinity and total carbon dioxide content of sea water by potentiometric titration. Deep Sea Research and Oceanogr. Abstracts 17, 737–750.
Fabry, V., McClintock, J., Mathis, J., and Grebmeier, J. (2011). Ocean acidification at high latitudes: the bellwether. Oceanography 22, 160–171. doi: 10.5670/oceanog.2009.105
Galloway, T., Cole, M., and Lewis, C. (2018). Interactions of microplastic debris throughout the marine ecosystem. Nat. Ecol. Evol. 1:0116. doi: 10.1038/s41559-017-0116
Garside, M. (2019). Global Cumulative Production of Plastic 1950–2050 [WWW Document]. Statista. Available online at: https://www.statista.com/statistics/1019758/plastics-production-volume-worldwide/ (2019) (accessed May 13, 21).
Geyer, R., Jambeck, J. R., and Law, K. L. (2017). Production, use, and fate of all plastics ever made. Sci. Adv. 3, 25–29. doi: 10.1126/sciadv.1700782
Gómez-Gutiérrez, J., Peterson, W. T., and Miller, C. B. (2010). Embryo biometry of three broadcast spawning euphausiid species applied to identify cross-shelf and seasonal spawning patterns along the Oregon coast. J. Plankton Res. 32, 739–760. doi: 10.1093/plankt/fbq028
Gómez-Gutiérrez, J., and Robinson, C. J. (2005). Embryonic, early larval development time, hatching mechanism and interbrood period of the sac-spawning euphausiid Nyctiphanes simplex Hansen. J. Plankton Res. 27, 279–295. doi: 10.1093/plankt/fbi003
González-Fernández, C., Tallec, K., Le Goïc, N., Lambert, C., Soudant, P., Huvet, A., et al. (2018). Cellular responses of Pacific oyster (Crassostrea gigas) gametes exposed in vitro to polystyrene nanoparticles. Chemosphere 208, 764–772. doi: 10.1016/j.chemosphere.2018.06.039
Goto, R., Saito, T., Matsubara, T., and Yamaha, E. (2019). “Microinjection of marine fish eggs,” in Methods in Molecular Biology, eds C. Liu and Y. Du (New York, NY: Humana Press Inc), 475–487. doi: 10.1007/978-1-4939-8831-0_27
Goyet, C., Beauverger, C., Brunet, C., and Poisson, A. (1991). Distribution of carbon dioxide partial pressure in surface waters of the Southwest Indian Ocean. Tellus B Chem. Phys. Meteorol. 43, 1–11. doi: 10.3402/tellusb.v43i1.15242
Harrington, S. A., and Ikeda, T. (1986). Laboratory observations on spawning, brood size and egg hatchability of the Antarctic krill Euphausia superba from Prydz Bay. Antarctica. Mar. Biol. Int. J. Life Ocean. Coast. Waters 92, 231–235. doi: 10.1007/BF00392840
Hartmann, N. B., Hüffer, T., Thompson, R. C., Hassellöv, M., Verschoor, A., Daugaard, A. E., et al. (2019). Are we speaking the same language? Recommendations for a definition and categorization framework for plastic debris. Environ. Sci. Technol. 53, 1039–1047. doi: 10.1021/acs.est.8b05297
Ikeda, T. (1984). Development of the larvae of the Antarctic krill (Euphausia superba) observed in the laboratory. J. Exp. Mar. Biol. Ecol. 75, 107–117. doi: 10.1016/0022-0981(84)90175-8
Isobe, A., Uchiyama-Matsumoto, K., Uchida, K., and Tokai, T. (2017). Microplastics in the Southern Ocean. Mar. Pollut. Bull. 114, 623–626. doi: 10.1016/j.marpolbul.2016.09.037
Jia, Z., Virtue, P., Swadling, K. M., and Kawaguchi, S. (2014). A photographic documentation of the development of Antarctic krill (Euphausia superba) from egg to early juvenile. Polar Biol. 37, 165–179. doi: 10.1007/s00300-013-1420-7
Jones-Williams, K., Galloway, T., Cole, M., Stowasser, G., Waluda, C., and Manno, C. (2020). Close encounters - microplastic availability to pelagic amphipods in sub-antarctic and antarctic surface waters. Environ. Int. 140, 105792. doi: 10.1016/j.envint.2020.105792
Kashiwada, S. (2006). Distribution of nanoparticles in the see-through medaka (Oryzias latipes). Environ. Health Perspect. 114, 1697–1702. doi: 10.1289/ehp.9209
Kawaguchi, S., Ishida, A., King, R., Raymond, B., Waller, N., Constable, A., et al. (2013). Risk maps for Antarctic krill under projected Southern Ocean acidification. Nat. Clim. Chang. 3, 843–847. doi: 10.1038/nclimate1937
Kawaguchi, S. S., Kurihara, H., King, R., Hale, L., Berli, T., Robinson, J. P., et al. (2011). Will krill fare well under Southern Ocean acidification? Biol. Lett. 7, 288–291. doi: 10.1098/rsbl.2010.077
Kelly, A., Lannuzel, D., Rodemann, T., Meiners, K. M., and Auman, H. J. (2020). Microplastic contamination in east Antarctic sea ice. Mar. Pollut. Bull. 154:111130. doi: 10.1016/j.marpolbul.2020.111130
Koelmans, A. A., Besseling, E., and Shim, W. J. (2015). “Nanoplastics in the Aquatic Environment. Critical Review,” in Marine Anthropogenic Litter, eds M. Bergmann and L. K. M. Gutow (Cham: Springer International Publishing), 325–340. doi: 10.1007/978-3-319-16510-3_12
Kuklinskia, P., Leszek, W., Mariusz, K., Tomasz, G., Hanna, L.-K., Michał, B., et al. (2019). Offshore surface waters of Antarctica are free of microplastics, as revealed by a circum-Antarctic study | Elsevier Enhanced Reader. Mar. Pollut. Bull. 149:110573. doi: 10.1016/j.marpolbul.2019.110573
Kurihara, H. (2008). Effects of CO2-driven ocean acidification on the early developmental stages of invertebrates. Mar. Ecol. Prog. Ser. 373, 275–284. doi: 10.2307/24872932
Lacerda, A. L. D. F., Rodrigues, L., dos, S., van Sebille, E., Rodrigues, F. L., Ribeiro, L., et al. (2019). Plastics in sea surface waters around the Antarctic Peninsula. Sci. Rep. 9:3977. doi: 10.1038/s41598-019-40311-4
Lannig, G., Eilers, S., Pörtner, H. O., Sokolova, I. M., and Bock, C. (2010). Impact of ocean acidification on energy metabolism of oyster, Crassostrea gigas — changes in metabolic pathways and thermal response. Mar. Drugs 8, 2318–2339. doi: 10.3390/md8082318
Lavigne, H., and Gattuso, J. P. (2010). Seacarb: Seawater Carbonates Chemistry With R.R Package Version 2.3.3. Available online at: http://CRAN.Rproject.org/ package-seacarb
Lee, K., Tong, L. T., Millero, F. J., Sabine, C. L., Dickson, A. G., Goyet, C., et al. (2006). Global relationships of total alkalinity with salinity and temperature in surface waters of the world’s oceans. Geophys. Res. Lett. 33:L19605. doi: 10.1029/2006GL027207
Lee, W. S., Cho, H.-J. J., Kim, E., Huh, Y. H., Kim, H.-J. J., Kim, B., et al. (2019). Bioaccumulation of polystyrene nanoplastics and their effect on the toxicity of Au ions in zebrafish embryos. Nanoscale 11, 3200–3207. doi: 10.1039/c8nr09321k
Lewis, C., Ellis, R. P., Vernon, E., Elliot, K., Newbatt, S., and Wilson, R. W. (2016). Ocean acidification increases copper toxicity differentially in two key marine invertebrates with distinct acid-base responses. Sci. Rep. 6:21554. doi: 10.1038/srep21554
Lewis, E., and Wallace, D. W. R. (1998). Program Developed for CO2SYS System Calculations. ORNL/CDIAC-105. Oak Ridge, TN: Carbon Dioxide Information Analysis Center, Oak Ridge National Laboratory, U.S. Department of Energy.
Lin, D., Tian, X., Wu, F., and Xing, B. (2010). Fate and transport of engineered nanomaterials in the environment. J. Environ. Qual. 39, 1896–1908. doi: 10.2134/jeq2009.0423
Manfra, L., Rotini, A., Bergami, E., Grassi, G., Faleri, C., and Corsi, I. (2017). Comparative ecotoxicity of polystyrene nanoparticles in natural seawater and reconstituted seawater using the rotifer Brachionus plicatilis. Ecotoxicol. Environ. Saf. 145, 557–563. doi: 10.1016/J.ECOENV.2017.07.068
Manno, C., Fielding, S., Stowasser, G., Murphy, E. J., Thorpe, S. E., and Tarling, G. A. (2020). Continuous moulting by Antarctic krill drives major pulses of carbon export in the north Scotia Sea, Southern Ocean. Nat. Commun. 11. doi: 10.1038/s41467-020-19956-7
Martin, S., Richier, S., Pedrotti, M., Dupont, S., Castejon, C., Gerakis, Y., et al. (2011). Early development and molecular plasticity in the Mediterranean sea urchin Paracentrotus lividus exposed to CO 2 -driven acidification. J. Exp. Biol. 214(Pt 8), 1357–1368. doi: 10.1242/jeb.051169
Martínez-Gómez, C., León, V. M., Calles, S., Gomáriz-Olcina, M., and Vethaak, A. D. (2017). The adverse effects of virgin microplastics on the fertilization and larval development of sea urchins. Mar Env. Res. 130, 69–76. doi: 10.1016/j.marenvres.2017.06.016
McBride, M. M., Dalpadado, P., Drinkwater, K. F., Godø, O. R., Hobday, A. J., Hollowed, A. B., et al. (2014). Krill, climate, and contrasting future scenarios for Arctic and Antarctic fisheries. ICES J. Mar. Sci. 71, 1934–1955. doi: 10.1093/icesjms/fsu002
McNeil, B. I., and Matear, R. J. (2008). Southern Ocean Acidification: a tipping point at 450 ppm atmospheric CO2. Proc. Natl. Acad. Sci.U.S.A. 105, 18860–18864. doi: 10.1073/pnas.0806318105
Mehrbach, C., Culberson, C. H., Hawley, J. E., and Pytkowicx, R. M. (1973). Measurement of the apparent dissociation constants of carbonic acid in seawater at atmospheric pressure1. Limnol. Oceangr. 18, 897–907.
Millero, F., Woosley, R., DiTrolio, B., and Waters, J. (2009). Effect of ocean acidification on the speciation of metals in Seawater. Oceanography 22, 72–85. doi: 10.5670/oceanog.2009.98
Morris, D., Watkins, J., Ricketts, C., Buchholz, F., and Priddle, J. (1998). An assessment of the merits of length and weight measurements of Antarctic krill Euphausia superba. Br. Antarc. Surv. Bull. 79, 29–50.
Munari, C., Infantini, V., Scoponi, M., Rastelli, E., Corinaldesi, C., and Mistri, M. (2017). Microplastics in the sediments of Terra Nova Bay (Ross Sea, Antarctica). Mar. Pollut. Bull. 122, 161–165. doi: 10.1016/J.MARPOLBUL.2017.06.039
Murphy, E. J., Watkins, J. L., Trathan, P. N., Reid, K., Meredith, M. P., Thorpe, S. E., et al. (2007). Spatial and temporal operation of the Scotia Sea ecosystem: a review of large-scale links in a krill centred food web. Philos. Trans. R. Soc. B Biol. Sci. 362, 113–148. doi: 10.1098/rstb.2006.1957
Nel, A. E., Mädler, L., Velegol, D., Xia, T., Hoek, E. M. V., Somasundaran, P., et al. (2009). Understanding biophysicochemical interactions at the nano-bio interface. Nat. Mater. 8, 543–557. doi: 10.1038/nmat2442
Norwood, W. P., Borgmann, U., Dixon, D. G., and Wallace, A. (2003). Effects of metal mixtures on aquatic biota: a review of observations and methods. Hum. Ecol. Risk Assess. An Int. J. 9, 795–811. doi: 10.1080/713610010
Obbard, R. W. (2018). Microplastics in polar regions: the role of long range transport. Curr. Opin. Environ. Sci. Heal. 1, 24–29. doi: 10.1016/j.coesh.2017.10.004
Oliviero, M., Tato, T., Schiavo, S., Manzo, S., and Beiras, R. (2019). Leachates of micronized plastic toys provoke embryotoxic effects upon sea urchin Paracentrotus lividus ∗. Environ. Pollut. 247, 706–715. doi: 10.1016/j.envpol.2019.01.098
Orr, J. C., Fabry, V. J., Aumont, O., Bopp, L., Doney, S. C., Feely, R. A., et al. (2005). Anthropogenic ocean acidification over the twenty-first century and its impact on calcifying organisms. Nature 437, 681–686. doi: 10.1038/nature04095
Pascal, P.-Y., Fleeger, J. W., Galvez, F., and Carman, K. R. (2010). The toxicological interaction between ocean acidity and metals in coastal meiobenthic copepods. Mar. Pollut. Bull. 60, 2201–2208. doi: 10.1016/J.MARPOLBUL.2010.08.018
Peeken, I., Primpke, S., Beyer, B., Gütermann, J., Katlein, C., Krumpen, T., et al. (2018). Arctic sea ice is an important temporal sink and means of transport for microplastic. Nat. Commun. 9, 1505. doi: 10.1038/s41467-018-03825-5
Perry, F. A., Kawaguchi, S., Atkinson, A., Sailley, S. F., Tarling, G. A., Mayor, D. J., et al. (2020). Temperature–induced hatch failure and nauplii malformation in Antarctic Krill. Front. Mar. Sci. 7:501. doi: 10.3389/fmars.2020.00501
Piccardo, M., Provenza, F., Grazioli, E., Cavallo, A., Terlizzi, A., and Renzi, M. (2020). PET microplastics toxicity on marine key species is influenced by pH, particle size and food variations. Sci. Total Environ. 715, 136947. doi: 10.1016/j.scitotenv.2020.136947
Pinsino, A., Bergami, E., Della, Torre, C, Vannuccini, M. L., Addis, P., Secci, M., et al. (2017). Amino-modified polystyrene nanoparticles affect signalling pathways of the sea urchin (Paracentrotus lividus) embryos. Nanotoxicology 11, 201–209. doi: 10.1080/17435390.2017.1279360
Przeslawski, R., Byrne, M., and Mellin, C. (2015). A review and meta-analysis of the effects of multiple abiotic stressors on marine embryos and larvae. Glob. Chang. Biol. 21, 2122–2140. doi: 10.1111/gcb.12833
Quetin, L. B., and Ross, R. M. (1984). Depth distribution of developing Euphausia superba embryos, predicted from sinking rates. Mar. Biol. 79, 47–53. doi: 10.1007/BF00404984
Quina, M. J., Bordado, J. C. M., and Quinta-ferreira, R. M. (2009). The influence of pH on the leaching behaviour of inorganic components from municipal solid waste APC residues. Waste Manag. 29, 2483–2493. doi: 10.1016/j.wasman.2009.05.012
Reed, S., Clark, M., Thompson, R., and Hughes, K. A. (2018). Microplastics in marine sediments near Rothera Research Station. Antarctica. Mar. Pollut. Bull. 133, 460–463. doi: 10.1016/j.marpolbul.2018.05.068
Rowlands, E., Galloway, T., and Manno, C. (2021). A Polar outlook: potential interactions of micro- and nano-plastic with other anthropogenic stressors. Sci. Total Environ. 754, 142379. doi: 10.1016/j.scitotenv.2020.142379
Sfriso, A. A., Tomio, Y., Rosso, B., Gambaro, A., Sfriso, A., Corami, F., et al. (2020). Microplastic accumulation in benthic invertebrates in Terra Nova Bay (Ross Sea. Antarctica). Environ. Int. 137:105587. doi: 10.1016/j.envint.2020.105587
Shi, W., Zhao, X., Han, Y., Che, Z., Chai, X., and Liu, G. (2016). Ocean acidification increases cadmium accumulation in marine bivalves: a potential threat to seafood safety. Sci. Rep. 6:20197. doi: 10.1038/srep20197
Suaria, G., Perold, V., Lee, J. R., Lebouard, F., Aliani, S., and Ryan, P. G. (2020). Floating macro- and microplastics around the Southern Ocean: results from the Antarctic Circumnavigation Expedition. Environ. Int. 136, 105494. doi: 10.1016/J.ENVINT.2020.105494
Tallec, K., Blard, O., González-Fernández, C., Brotons, G., Berchel, M., Soudant, P., et al. (2019). Surface functionalization determines behavior of nanoplastic solutions in model aquatic environments. Chemosphere 225, 639–646. doi: 10.1016/j.chemosphere.2019.03.077
Tallec, K., Huvet, A., Di Poi, C., González-Fernández, C., Lambert, C., Petton, B., et al. (2018). Nanoplastics impaired oyster free living stages, gametes and embryos. Environ. Pollut. 242, 1226–1235. doi: 10.1016/j.envpol.2018.08.020
Ter Halle, A., Jeanneau, L., Martignac, M., Jardé, E., Pedrono, B., Brach, L., et al. (2017). Nanoplastic in the North Atlantic Subtropical Gyre. Environ. Sci. Technol. 51, 13689–13697. doi: 10.1021/acs.est.7b03667
Tirelli, V., Suaria, G., and Lusher, A. L. (2020). “Microplastics in polar samples,” in Handbook of Microplastics in the Environment, eds T. Rocha-Santos, M. Costa, and C. Mouneyrac (Cham: Springer International Publishing), 1–42. doi: 10.1007/978-3-030-10618-8_4-1
Wang, X., Huang, W., Wei, S., Shang, Y., Gu, H., Wu, F., et al. (2020). Microplastics impair digestive performance but show little effects on antioxidant activity in mussels under low pH conditions. Environ. Pollut. 258:113691. doi: 10.1016/j.envpol.2019.113691
Wilson-McNeal, A., Hird, C., Hobbs, C., Nielson, C., Smith, K. E., Wilson, R. W., et al. (2020). Fluctuating seawater pCO2/pH induces opposing interactions with copper toxicity for two intertidal invertebrates. Sci. Total Environ. 748:141370. doi: 10.1016/j.scitotenv.2020.141370
Worm, B., Lotze, H. K., Jubinville, I., Wilcox, C., and Jambeck, J. (2017). Plastic as a persistent marine pollutant. Annu. Rev. Environ. Resour. 42, 1–26. doi: 10.1146/annurev-environ-102016-060700
Yoshida, T., Toda, T., Hirano, Y., Matsuda, T., and Kawaguchi, S. (2004). Effect of temperature on embryo development time and hatching success of the Antarctic krill Euphausia superba Dana in the laboratory. Mar. Freshw. Behav. Physiol. 37, 137–145. doi: 10.1080/10236240410001705789
Keywords: nanoparticle, plastic pollution, multi-stressor, Antarctic krill, Scotia Sea, embryonic development, egg abnormality
Citation: Rowlands E, Galloway T, Cole M, Lewis C, Peck V, Thorpe S and Manno C (2021) The Effects of Combined Ocean Acidification and Nanoplastic Exposures on the Embryonic Development of Antarctic Krill. Front. Mar. Sci. 8:709763. doi: 10.3389/fmars.2021.709763
Received: 14 May 2021; Accepted: 16 July 2021;
Published: 03 August 2021.
Edited by:
Stefania Ancora, University of Siena, ItalyReviewed by:
Chiara Gambardella, National Research Council (CNR), ItalyArnaud Huvet, Institut Français de Recherche pour l’Exploitation de la Mer (IFREMER), France
Copyright © 2021 Rowlands, Galloway, Cole, Lewis, Peck, Thorpe and Manno. This is an open-access article distributed under the terms of the Creative Commons Attribution License (CC BY). The use, distribution or reproduction in other forums is permitted, provided the original author(s) and the copyright owner(s) are credited and that the original publication in this journal is cited, in accordance with accepted academic practice. No use, distribution or reproduction is permitted which does not comply with these terms.
*Correspondence: Emily Rowlands, ZW1pcm93QGJhcy5hYy51aw==; Clara Manno, Y2xhbm5vQGJhcy5hYy51aw==