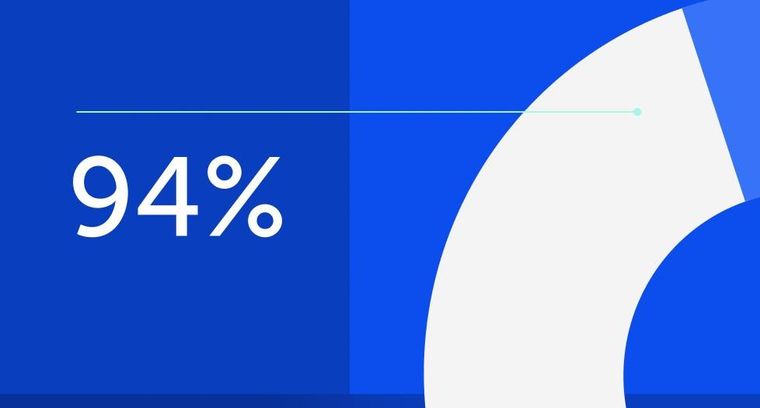
94% of researchers rate our articles as excellent or good
Learn more about the work of our research integrity team to safeguard the quality of each article we publish.
Find out more
ORIGINAL RESEARCH article
Front. Mar. Sci., 26 July 2021
Sec. Marine Megafauna
Volume 8 - 2021 | https://doi.org/10.3389/fmars.2021.707376
Animal-borne video camera systems have long-been used to capture the fine-scale behaviors and unknown aspects of the biology of marine animals. However, their utility to serve as robust scientific tools in the greater bio-logging research community has not been fully realized. Here we provide, for the first time, an application of 360-degree camera technology to a marine organism, using a large tiger shark as a proof-of-concept case study. Leveraging the three-dimensional nature of the imaging technology, we derived 224 seafloor habitat assessments over the course of the nearly 1-h track, whereby the shark was able to survey ∼23,000 square meters of seafloor; over three-times greater than the capacity of non 360-degree cameras. The resulting data provided detailed information on habitat use, diving behavior, and swimming speed, as well seafloor mapping. Our results suggest that 360-degree cameras provide complimentary benefits—and in some cases superior efficiency—than unidirectional video packages, with an enhanced capacity to map seafloor.
The remote monitoring of marine megafauna is made difficult by the logistical challenges of working in the marine environment, as well as the operational limits of the most recent electronic tag technologies. The proliferation of satellite-based telemetry and its application to studying the behavior and physiology of marine megafauna have greatly expanded our ability to describe basic, yet poorly understood elements of these species’ biology (Hart and Hyrenbach, 2009; Hussey et al., 2015; Sequeira et al., 2018), and are now being used to better understand the risks they face (e.g., Queiroz et al., 2019) and to gauge how they will respond to a warming ocean (e.g., Bastien et al., 2020). Satellite-based telemetry is, however, limited in its ability to store and transmit substantial volumes of data, and therefore it cannot fully resolve the relationship between an individual animal’s state and the external environment.
Bio-logging utilizes miniaturized data-loggers which are equipped with sensors that can provide a more integrated and detailed, albeit often shorter-term, understanding of aquatic animal ecology and physiology (Cooke et al., 2004; Rutz and Hays, 2009). These approaches often include tri-axial accelerometers and depth and temperature loggers, which allow for high-resolution evaluations of animal locomotion, foraging, energetics, habitat selection, and physiological performance (Payne et al., 2014; Watanabe et al., 2019, 2020). Cameras have been a central component to bio-logging for some time, and represented, the first types of loggers deployed on wild, marine species (Marshall, 1990, 1998). Cameras are commonly integrated into contemporary bio-logging studies of marine megafauna such as turtles, whales, birds, and sharks (Thiebault et al., 2016; Andrzejaczek et al., 2019; Segre et al., 2019; Smulders et al., 2021), as they provide data that are ultimately needed to explain patterns observed from other sensors (Williams et al., 2020). As animals select those habitats which offer the greatest fitness-related benefits (Cooke et al., 2004), video recordings provide unequivocal information on the habitat chosen by marine animals, which is a necessary data layer to interpret their use of space. Although the benefits of using animal-borne video systems in bio-logging studies to advance hypothesis testing and aspects of behavioral decision making have been well-established (Moll et al., 2007), the limits of previous imaging technology and sensor capabilities have constrained the scientific utility of this tool.
Here, we apply and report the addition of 360-degree camera technology to a bio-logging device on a marine predator for the first time. The package was deployed as a case study on a large tiger shark. We use this proof-of-concept pilot study to demonstrate the performance and unique merits of 360-camera technology in bio-logging, specifically deriving robust estimates of swimming speed and seafloor mapping. Our study also provides new insights into the habitat use of tiger sharks in the subtropical Atlantic, underscoring the importance of seagrass to this species at fine spatial and temporal scales. Emerging camera technologies offer researchers exciting opportunities to improve and refine the type and volume of information obtained from animal-borne video surveys.
The data for this paper were collected as part of a multi-year, long-term research program evaluating the interannual behavior and physiology of large sharks throughout the coastal waters of The Commonwealth of The Bahamas (Gallagher et al., 2021). On July 23, 2020, a sub-adult tiger shark (Galeocerdo cuvier) was safely captured using a standardized circle-hook drumline (see Gallagher et al., 2017 for a detailed description of capture methodology) on a shallow carbonate bank of uniform depth (8 m), off Coral Harbor, New Providence Island, The Bahamas (24.93° N, −77.36° W) at approximately 17:30 local time. All animal capture and ethics around tagging were approved by the Carleton University Animal Care Committee. The shark was hooked on the line for approximately 20 min. Tiger sharks have been shown to be extremely robust to capture stress, such that the present hooking duration would have negligible effects on post-release behavior (Gallagher et al., 2014). The individual shark was then secured alongside a 10 m center console research vessel while remaining submerged, where its sex and morphometric measurements were taken. Two mark-recapture identification tags were applied to the shark at the base of the dorsal fin, and a coded acoustic transmitter was surgically implanted ventrally into the peritoneal cavity and then sutured, as part of a long-term study on large shark habitat use and residency within the region (see Gallagher et al., 2021 for more info and tag specifications).
A 360-degree camera (Insta360 ONE X, 115 g weight, 115 mm × 48 mm × 28 mm, D × W × H; Arashi Vision, Inc., Shenzen, China; recording specifications: 360-degree field of view, 2.7 K resolution, 30 frames per second) housed within a waterproof casing (128 g weight, 98 mm × 82 mm × 195 mm; D × W × H, rated to 30 m depth) was secured to frontal and backing plates made of syntactic foam (40 mm × 40 mm × 5 mm), which served as the main payload for a bio-logging device (Figure 1A). Two asset recovery tags were secured to the center of the bio-logger payload using clear silicone: a satellite tag (SPOT-386A, Wildlife Computers, Redmond, WA, United States) and a VHF radio tag (F1840B, Advanced Telemetry Systems, Isanti, MN, United States). Two stainless steel nuts were added to the package to provide forward-facing ballast to reduce the buoyancy from the camera housing, thus allowing the tag to float on the surface in a manner which maintained the vertical orientation of the satellite and radio tag antennae in air. The entire bio-logger package was attached to the left side of the shark’s dorsal fin by drilling two small holes and threading two connected, biodegradable cable ties through and around the package. The heads of the cable ties were then joined together via the eyes of a dissolvable galvanic timed-release swivel (A2 model, Neptune Marine Products, Port Townsend, WA, United States), which would eventually corrode in seawater after an estimated period of ∼6 h (swivel was pre-dissolved to permit a short-term deployment), thereby allowing the positive buoyancy of the package to cause it to naturally release and come off the animal. Once attached, the camera was activated for recording and the shark was released (Figure 1B). The entire time to collect all animal data, apply tags and attach the bio-logger was 12 min. The bio-logger was ultimately recovered by traveling to the most recent ping from the satellite tag while actively using a VHF radio to locate the pings from the VHF transmitter.
Figure 1. (A) Image of 360-degree camera bio-logger unit and (B) attached to tiger shark in present study; (C,D) video footage from camera highlighting varying perspectives for annotation as the shark moves throughout the water column and over seafloor.
The entire annotation of video was performed in 360 degrees, by using playback software to manually adjust viewing position and annotate the video in 1-min intervals for analysis (GoPro Player, GoPro, San Mateo, CA, United States; Figures 1C,D). The animal’s relative position in the water column was characterized qualitatively at each 1-min interval by using the 360-degree camera viewer to classify the shark in the following positions: surface (at or within 1 body length of the surface of the water), mid-water (>1 body length beneath the surface and >1 body length off the bottom), and benthic (at or <1 body length off the bottom). Habitat type was classified at every 1-min interval, by categorizing the benthic environment in each 90-degree pane (moving clockwise; Quadrant 1: North; Quadrant 2: East; Quadrant 3: South; Quadrant 4: West; Figure 2) to a radius of roughly two body lengths of the shark, or 5 m, into the following classifications: sand (no vegetation present), light macroalgae (<25% coverage of turf algae or macrophytes), and light seagrass (1–25% coverage). The nature of this annotation approach qualifies each interval as a discrete measurement, such that the areas visible in forward facing quadrants would not necessarily be identical to those visible in rear facing quadrants. An overall dominant habitat type was determined at each interval in 360 degrees by calculating the habitat type with the greatest frequency at each 1-min interval for all four regions combined, excluding situations where there was even parity or no data were extracted due to an inability to determine habitat type. Swimming speed was estimated by adjusting the 360-degree camera view to focus on the back of the shark, to observe and annotate the movement of the shark’s tail. The total number of tail flicks in both directions was counted every minute (then divided by two and then 60 s) to obtain a calculation of tail-beat frequency (TBF, beats/second). Swimming speed S (m s–1) was then estimated by the following equation:
Figure 2. Seafloor habitat classifications (see legend for colors and habitat types) assessed at every minute interval, in each of the 90-degree quadrants of the video. Derived overall estimates of swim speed at each interval are also provided.
where, SL is average stride length [0.36 body lengths/tail beat; digitized and interpolated from a study on tiger sharks by Nakamura et al. (2011)], TL is total length (230 cm in the present study) and TBF is tail beat frequency, which was calculated at each interval in the present study. Mean speed was estimated in successive minutes over the full recording duration. Differences in shark swimming speed according to animal depth were tested using an ANOVA with Tukey HSD, and we also evaluated differences in speed according to dominant habitat with a non-parametric Kruskal-Wallis (excluding observations where no dominant habitat could be determined). All analyses were performed in RStudio (R version 4.0.4, RStudio version 1.3.1103; R Core Team, 2021; RStudio Team, 2021) and significance was determined at p < 0.05. Data visualization and plotting were performed using the package ggplot2 (Wickham, 2016).
Fifty-eight total minutes of 360-degree footage were obtained from a 230 cm (total length, TL), female tiger shark (Figure 1). The first minute of footage was removed from analysis due to the shark’s proximity to the research vessel, resulting in a range of 54–57 min of footage which were available for each of the analyses. Given the analytical framework of four, 90-degree quadrants, a total of 224 habitat assessments were recorded (Figure 2).
Light seagrass was the dominant habitat used, inferred from the 360-degree analyses, for 29 of the 56 min by the tagged shark at-liberty (Figure 3). The shark appeared to adjust its vertical position when seagrass habitat was more prevalent, whereby surface-oriented behavior stopped and the animal lowered its position to remain in the lower portion of the water column (Figure 3). Swimming speeds ranged from 0.52 to 0.70 m/s, with a mean (±SE) of 0.64 ± 0.005 m/s, suggesting a relatively consistent swim speed along the duration of the track (Figure 2). The total distanced traveled by the shark was estimated to be 2,123 m, or 35.4 meters per minute. Swimming speed varied according to depth, as surface swimming speeds were significantly slower than those from mid-water and benthic (F = 15.72, df = 2, p < 0.001). Swimming speeds were also significantly different according to dominant habitat type (Chi-sq = 12.49, df = 2, p < 0.005), which appeared to be slightly faster when over seagrass habitat.
Figure 3. Qualitative estimates of shark depth over the track, with dominant habitat type represented by color classifications.
Animal-borne video systems have been used widely by ecologists to study the fine-scale interactions between marine animals and their environment for over three decades (Marshall, 1990). Despite their well-established ability to provide detailed, short-term accounts of individual animal activity and their utility in generating and testing fine-scale hypotheses (see review by Moll et al., 2007), their benefits are more commonly skewed toward public engagement and educational value rather than as a monitoring tool.
Our application of 360-camera technology provided a fully representative view of the focal animal and its environment (Figure 1), yielding a novel perspective in both vertical and horizontal directions throughout the duration of the track. The ability to manipulate, zoom, and re-position the 360-degree video in post-processing allowed us to approximate the position of the shark in the water column relative to both the surface and the seafloor, therefore providing a qualitative assessment of depth (Figure 3). Video assessment of tail beat frequency often requires researchers to make trade-offs regarding the positioning of unidirectional cameras or whether to include accelerometer sensors in their bio-logger payloads, which can affect drag. These trade-offs were avoided by using a 360-degree camera package. This study served as one of the first published camera tag deployments for tiger sharks in the subtropical Atlantic, which also demonstrated seagrass to be its dominant habitat (Figure 3). Our assessment of dominant habitat, as inferred from the present video analysis, also corroborates recent telemetry work suggesting the importance of seagrass for the species in The Bahamas (Gallagher et al., 2021) and also extends what is known from ocean regions (Heithaus et al., 2002).
Our ability to score a variety of habitat types in 360 degrees as the tiger shark moved over the Great Bahama Bank presented us the unique chance to estimate dominant habitat density over space and time (Figures 2, 4). The resulting translation of 360-degree video data into a two-dimensional bivariate raster demonstrates our tiger shark’s ability to provide autonomous, unbiased, and detailed benthic mapping of its shallow marine ecosystem (Figure 4). Using our assumed field of view of the 360-degree camera as 5 m in all directions, combined with a calculated swim speed of 0.64 m/s and a derived linear distance swum by the individual of 2,123 m, we estimate that our shark could survey ∼22,000 m2 of seafloor in an hour [21,888 m2, a product of the derived swimming speed (0.64 m/s), duration of the track (57.0 min), and assumed 5 m visual radius]. This figure is over three times greater than which would be obtained from the same individual shark if it were using a single front-facing camera (with an assumed field of view of ∼3.0 m wide, an area of 6,566 m2).
Figure 4. Estimates of seagrass density, derived from scoring all 224 habitat assessments in 360 degrees over the course of the entire track, highlighting the ability for marine megafauna tagged with 360-degree cameras to provide detailed information on seafloor habitat.
Whereas electronic tagging has commonly been viewed as a means to monitor the behavior and habitat use of marine megafauna (Costa et al., 2010), our pilot study sheds new light on the benefits of animal-borne video systems for evaluating seafloor habitat in three dimensions. Not discussed here are other merits of using 360-degree cameras which may improve our ability to record predator-prey interactions and cryptic life-history events (e.g., schooling, aggregation, reproductive attempts). The same challenges and disadvantages facing studies using previous imaging technology in studies using animal-borne camera systems apply to our pilot study; namely, that our application was limited by battery life and data storage. We recognize the results provided here were constrained to testing in a constrained area over a short time frame (e.g., a shallow, tropical carbonate bank with good visibility). Nevertheless, the added capacity for detailed information on habitat selection, seafloor structure, and streamlined efficiency to bio-logging payloads suggest that 360-degree cameras should be further explored as they offer complimentary—and in some unique cases—new benefits to the bio-logging of marine megafauna when compared to limited unidirectional camera packages.
The raw data supporting the conclusions of this article will be made available by the authors, without undue reservation, to any qualified researcher.
The animal study was reviewed and approved by the Carleton University Animal Care Committee.
AG and CD conceived this study. AG performed, led, and facilitated all aspects of the long-term study and fieldwork, and wrote the manuscript. NA performed the video annotations. AG, BS, and NP contributed to the data analysis. All authors contributed to revisions of the manuscript and approved the final version.
This work was supported by grants to AG from the Wanderlust Fund, National Geographic, and was also facilitated by Hazmat Productions and Discovery Channel. These funders were not involved in the study design, collection, analysis, interpretation of data, the writing of this article or the decision to submit it for publication.
The authors declare that the research was conducted in the absence of any commercial or financial relationships that could be construed as a potential conflict of interest.
All claims expressed in this article are solely those of the authors and do not necessarily represent those of their affiliated organizations, or those of the publisher, the editors and the reviewers. Any product that may be evaluated in this article, or claim that may be made by its manufacturer, is not guaranteed or endorsed by the publisher.
We thank our Bahamian partners and stakeholders who have enabled and supported this work: L. Gittens and the Department of Marine Resources, E. Carey and S. Cant from Bahamas National Trust, as well as A. Phillips and A. Musgrove from Bahamas Dive Guides. We thank J. Halvorsen and the crew of the M/Y Marcato for their logistical support, as well as A. Devine, A. Holm, and B. Anderson for field work support. We also thank P. Matusheski and B. Haslup from Hazmat Productions, as well as H. Swartz, J. Schneier, and C. Allgood from Discovery Channel.
Andrzejaczek, T.K., Gleiss, A.C., Lear, K.O., Pattiaratchi, C.B., Chapple, S., and Meekan, M.G. (2019). Biologging tags reveal links between fine-scale horizontal and vertical movement behaviors in tiger sharks (Galeocerdo cuvier). Front. Mar. Sci. 6:229. doi: 10.3389/fmars.2019.00229
Bastien, G., Barkley, A., Chappus, J., Heath, V., Popov, S., Smith, R., et al. (2020). Inconspicuous, recovering, or northward shift: status and management of the white shark (Carcharodon carcharias) in Atlantic Canada. Can. J. Fish. Aquat. Sci. 77, 1666–1677. doi: 10.1139/cjfas-2020-0055
Cooke, S. J., Hinch, S. G., Wikelski, M., Andrews, R. D., Kuchel, L. J., Wolcott, T. G., et al. (2004). Biotelemetry: a mechanistic approach to ecology. Trends Ecol. Evol. 19, 334–343. doi: 10.1016/j.tree.2004.04.003
R Core Team (2021). R: A Language and Environment for Statistical Computing. R Foundation for Statistical Computing. Vienna: R Core Team.
Costa, D., Block, B., Bograd, S., Fedak, M., and Gunn, J. (2010). “TOPP as a marine life observatory: using electronic tags to monitor the movements, behaviour and habitats of marine vertebrates,” in Proceedings of OceanObs’09: Sustained Ocean Observations and Information for Society, Vol. 2, eds J. Hall, D. E. Harrison, and D. Stammer (Venice: ESA Pub), WPP–306. doi: 10.5270/OceanObs09.cwp.19
Gallagher, A. J., Serafy, J. E., Cooke, S. J., and Hammerschlag, N. (2014). Physiological stress response, reflex impairment, and survival of five sympatric shark species following experimental capture and release. Mar. Ecol. Prog. Ser. 496, 207–218. doi: 10.3354/meps10490
Gallagher, A. J., Shipley, O. N., van Zinnicq Bergmann, M. P., Brownscombe, J. W., Dahlgren, C. P., Frisk, M. G., et al. (2021). Spatial connectivity and drivers of shark habitat use within a large marine protected area in the Caribbean, The Bahamas Shark Sanctuary. Front. Mar. Sci. 7:1223. doi: 10.3389/fmars.2020.608848
Gallagher, A. J., Staaterman, E. R., Cooke, S. J., and Hammerschlag, N. (2017). Behavioural responses to fisheries capture among sharks caught using experimental fishery gear. Can. J. Fish. Aquat. Sci. 74, 1–7. doi: 10.1139/cjfas-2016-0165
Hart, K. M., and Hyrenbach, K. D. (2009). Satellite telemetry of marine megavertebrates: the coming of age of an experimental science. Endang. Species Res. 10, 9–20. doi: 10.3354/esr00238
Heithaus, M., Dill, L., Marshall, G., and Buhleier, B. (2002). Habitat use and foraging behavior of tiger sharks (Galeocerdo cuvier) in a seagrass ecosystem. Mar. Biol. 140, 237–248. doi: 10.1007/s00227-001-0711-7
Hussey, N. E., Kessel, S. T., Aarestrup, K., Cooke, S. J., Cowley, P. D., Fisk, A. T., et al. (2015). Aquatic animal telemetry: a panoramic window into the underwater world. Science 348:6240. doi: 10.1126/science.1255642
Marshall, G. J. (1990). “A Video-Collar to Study Aquatic Fauna: A view from the animal’s back,” in Spirit of Enterprise: The 1990 Rolex Awards, ed. D. Reed (New York, NY: Springer) 57–59.
Marshall, G. J. (1998). Crittercam: an animal-borne imaging and data logging system. Mar. Technol. Soc. J. 32:11.
Moll, R. J., Millspaugh, J. J., Beringer, J., Sartwell, J., and He, Z. (2007). A new ‘view’of ecology and conservation through animal-borne video systems. Trends Ecol. Evol. 22, 660–668. doi: 10.1016/j.tree.2007.09.007
Nakamura, I., Watanabe, Y. Y., Papastamatiou, Y. P., Sato, K., and Meyer, C. G. (2011). Yo-yo vertical movements suggest a foraging strategy for tiger sharks Galeocerdo cuvier. Mar. Ecol. Prog. Ser. 424, 237–246. doi: 10.3354/meps08980
Payne, N. L., Taylor, M. D., Watanabe, Y. Y., and Semmens, J. M. (2014). From physiology to physics: are we recognizing the flexibility of biologging tools? J. Exp. Biol. 217, 317–322. doi: 10.1242/jeb.093922
Queiroz, N., Humphries, N. E., Couto, A., Vedor, M., da Costa, I., Sequeira, A. M. M., et al. (2019). Global spatial risk assessment of sharks under the footprint of fisheries. Nature 572, 461–466.
RStudio Team (2021). RStudio: Integrated Development Environment for R. RStudio, PBC. Boston, MA: Elsevier.
Rutz, C., and Hays, G. C. (2009). New frontiers in biologging science. Biol. Lett. 5, 289–292. doi: 10.1098/rsbl.2009.0089
Segre, P. S., Cade, D. E., Calambokidis, J., Fish, F. E., Friedlaender, A. S., Potvin, J., et al. (2019). Body flexibility enhances maneuverability in the world’s largest predator. Integr. Comp. Biol. 59, 48–60. doi: 10.1093/icb/icy121
Sequeira, A. M., Rodríguez, J. P., Eguíluz, V. M., Harcourt, R., Hindell, M., Sims, D. W., et al. (2018). Convergence of marine megafauna movement patterns in coastal and open oceans. Proc. Natl. Acad. Sci. U. S. A. 115, 3072–3077.
Smulders, F. O., O’Shea, O. R., and Christianen, M. J. (2021). Animal-borne video reveals atypical behaviour in provisioned green turtles: a global perspective of a widespread tourist activity. Glob. Ecol. Conserv. 25:e01417. doi: 10.1016/j.gecco.2020.e01417
Thiebault, A., Pistorius, P., Mullers, R., and Tremblay, Y. (2016). Seabird acoustic communication at sea: a new perspective using bio-logging devices. Sci. Rep. 6:30972.
Watanabe, Y. Y., Ito, K., Kokubun, N., and Takahashi, A. (2020). Foraging behavior links sea ice to breeding success in Antarctic penguins. Sci. Adv. 6:26. doi: 10.1126/sciadv.aba4828
Watanabe, Y. Y., Payne, N. L., Semmens, J. M., Fox, A., and Huveneers, C. (2019). Swimming strategies and energetics of endothermic white sharks during foraging. J. Exp. Biol. 222:jeb185603. doi: 10.1242/jeb.185603
Keywords: bio-logging, camera, 360, shark, telemetry, monitoring
Citation: Gallagher AJ, Alsudairy NA, Shea BD, Payne NL and Duarte CM (2021) First Application of 360-Degree Camera Technology to Marine Predator Bio-Logging. Front. Mar. Sci. 8:707376. doi: 10.3389/fmars.2021.707376
Received: 09 May 2021; Accepted: 01 July 2021;
Published: 26 July 2021.
Edited by:
Gail Schofield, Queen Mary University of London, United KingdomReviewed by:
Kim Holland, University of Hawaii, United StatesCopyright © 2021 Gallagher, Alsudairy, Shea, Payne and Duarte. This is an open-access article distributed under the terms of the Creative Commons Attribution License (CC BY). The use, distribution or reproduction in other forums is permitted, provided the original author(s) and the copyright owner(s) are credited and that the original publication in this journal is cited, in accordance with accepted academic practice. No use, distribution or reproduction is permitted which does not comply with these terms.
*Correspondence: Austin J. Gallagher, YXVzdGluQGJlbmVhdGh0aGV3YXZlcy5vcmc=
Disclaimer: All claims expressed in this article are solely those of the authors and do not necessarily represent those of their affiliated organizations, or those of the publisher, the editors and the reviewers. Any product that may be evaluated in this article or claim that may be made by its manufacturer is not guaranteed or endorsed by the publisher.
Research integrity at Frontiers
Learn more about the work of our research integrity team to safeguard the quality of each article we publish.