- 1Graduate Program in Evolution, Ecology and Behavior, University at Buffalo, Buffalo, NY, United States
- 2Department of Geology, University at Buffalo, Buffalo, NY, United States
Recruitment is a key demographic process for maintenance of local populations and recovery following disturbance. For marine invertebrates, distribution and abundances of recruits are impacted by spatiotemporal variation in larval supply, settlement rates and post-settlement survival. However, for colonial and modular organisms, differences in survival and growth between settlers and colonial recruits may also affect recruitment patterns. In the Caribbean, shifts in the benthic community structure favoring octocoral’s have been detected, and recruitment has been suggested as key for octocoral’s resilience. Hence, we studied octocoral recruitment dynamics, and evaluated the role of pre-settlement, settlement and post-settlement processes in recruit’s densities. We performed the study at two sites with different octocoral densities, on the south coast of St. John, United States Virgin Islands, and distinguished between processes occurring to recently settled polyps and to colonial recruits. At both sites, we monitored P. homomalla settlers on settlement tiles for 3 months, and colonial recruits of two of the most abundant genera (Eunicea and Pseudoplexaura) for 3 years. In addition, we assessed whether recruits morphological traits affected recruitment and divided recruits of the genus Eunicea based on the presence of large calyces. The major contributor to both, single-polyps and colonial recruit densities was larval supply. Single-polyp densities were not limited by the availability of space, settlement cues, or early post-settlement survival. Height was the only predictor of survival and growth of colonial recruits, with potential growth rates increasing with height. However, large recruits suffered partial mortality often, distorting the relationship between recruit age and size, and causing most recruits to remain in the recruit size class (≤5 cm) longer than a year. Octocorals have been resilient to the conditions that have driven the decline of scleractinian corals throughout the Caribbean, and recruitment has been key to that success. Our results are crucial to understand early life history dynamics of Caribbean octocorals, and highlights the need to standardize the definition of recruit among colonial and modular taxa to facilitate inter-specific comparisons, and to understand future changes in coral reef community assemblages.
Introduction
Recruitment influences population dynamics and the structure of local communities (Keough and Downes, 1982; Roughgarden et al., 1988; Caley et al., 1996). In marine hard bottom ecosystems, algae and invertebrates have complex life cycles starting life with a planktonic stage as larvae or propagules, then transitioning to the substratum as recruits, and developing into juveniles and adults. As a result, most marine hard-bottom communities have open populations, with local settlement dependent on larvae arriving from distant locations (Roughgarden et al., 1985). Complex larval behaviors and an array of biophysical interactions, determine the density of local settlers, after which, early post-settlement survival (i.e., survival until recruits reach the next stage, age, or size) further modifies local recruit densities (Roughgarden et al., 1988; Grosberg and Levitan, 1992; Hurlbut, 1992).
Whether recruitment of benthic marine invertebrates with open populations is limited by larval supply, or by settlement and early post-settlement processes has been the subject of research for decades (Gaines and Roughgarden, 1985; Roughgarden et al., 1988; Raimondi, 1990; Caley et al., 1996; Menge, 2000; Jonsson et al., 2004). Variability in local recruitment has been correlated to differences in larval supply for some species (Gaines and Roughgarden, 1985; Roughgarden et al., 1988; Hughes et al., 2000; Grorud-Colvert and Sponaugle, 2009; Gleason et al., 2018), and to settlement rates and/or early post-settlement mortality in other cases (Keough and Downes, 1982; Hunt and Scheibling, 1997; Vermeij and Sandin, 2008). Nevertheless, identifying the contribution of each stage in their recruitment still remains challenging, since each process is species-specific, context-specific, and scale-dependent (Young et al., 2005; Pineda et al., 2009; Ritson-Williams et al., 2009). For example, larval supply can be affected by adult reproductive strategies (e.g., brooded larvae vs. broadcasted gametes), by larval traits and pelagic larval duration, and by regional and local hydrodynamics (Raimondi and Morse, 2000; Nishikawa et al., 2003; Pineda et al., 2010). Settlement and early post-settlement survival can be impacted by the availability of suitable habitat, larval behavior, and space and/or resource competition within the local community (Mullineaux and Garland, 1993; Hunt and Scheibling, 1997; Arnold and Steneck, 2011). Consequently, the relative importance among larval supply, settlement and post-settlement processes in driving recruitment of many benthic marine invertebrates with open populations is not well understood.
Sessile colonial invertebrates with complex life cycles, and open populations, such as corals, gorgonians, hydroids, bryozoans, and colonial ascidians, often dominate marine benthic communities (Winston, 2010). The astogenetic shift from a solitary settler to a colonial recruit and the different methods used to assess and monitor recruitment add complexity to understand and to compare recruitment dynamics among colonial taxa. Millimeter-scale, solitary settlers, tend to suffer high mortality rates (Deevey, 1947; Babcock and Mundy, 1996; Linares et al., 2008; Trapon et al., 2013; Doropoulos et al., 2016), whereas centimeter-scale colonial recruits, can survive partial damage, and remain in the recruit size-class over longer periods of time. These effects are well known (Hughes and Jackson, 1980; Hughes and Connell, 1987; Vermeij and Sandin, 2008; Edmunds, 2017). However, logistical constraints have led to different methods to assess and monitor recruitment, and even to different definitions of “recruit” (Jenkins et al., 2009; Pineda et al., 2009). For example, many studies of scleractinian corals limit the definition of recruits to single-polyps, and they assess recruitment on settlement tiles (Adjeroud et al., 2017 and references within). Other studies define as recruits all individuals below a certain size, e.g., <5 cm2 for scleractinian corals (Vermeij and Sandin, 2008), and ≤5 cm tall for octocorals (Edmunds and Lasker, 2019), and quantify recruitment directly on the reef. Analyzing recruitment on settlement tiles does not differentiate between settlement and post-settlement processes. Whereas, defining recruits based on a certain size may lead to classifying as recruits older colonies (but small due to partial mortality), or to excluding from the recruit-size class larger recruits (due to inter-specific growth rates). Consequently, recruitment might be over or underestimated depending on the method. Therefore, performing comparisons among taxa and studies is difficult, and the effect of recruitment on changes on benthic invertebrates’ assemblages is poorly understood.
In the Caribbean, coral cover has decreased ∼70% since 1970 (Jackson et al., 2014), and concomitantly species assemblages have changed in composition (Green et al., 2008; Edmunds, 2013). Transitions have favored macroalgae over scleractinian corals (Hughes, 1994; Bruno et al., 2009; Roff and Mumby, 2012). Nevertheless, taxa other than macroalgae have come to dominate the benthos on some reefs (Norström et al., 2009), and several studies have reported increases in octocoral abundances (Norström et al., 2009; Ruzicka et al., 2013; Edmunds and Lasker, 2016; Tsounis et al., 2018) or at least the maintenance of their populations (Lenz et al., 2015). The drivers of coral decline and lack of recovery on Caribbean reefs are complex. Among those drivers, elevated rates of mortality due to acute disturbances (i.e., hurricanes, bleaching events, and disease outbreaks), decline of key herbivores, and recruitment failure have been suggested as key factors impacting corals resilience (Hughes and Tanner, 2000; Crabbe et al., 2008; Mumby et al., 2007; Adam et al., 2015; Hughes et al., 2018). Similar to scleractinian corals, octocoral populations have declined after extreme climatic and bleaching events (Prada et al., 2010; Ruzicka et al., 2013; Tsounis and Edmunds, 2017; Lasker et al., 2020), but in contrast to scleractinian corals, they have persisted (Ruzicka et al., 2013; Tsounis and Edmunds, 2017; Lasker et al., 2020). The demographic processes involved in the recovery and maintenance of octocoral populations are not well understood (Edmunds and Lasker, 2019). However, previous studies have found a correlation between densities of octocoral adults and recruits (Yoshioka, 1996; Privitera-Johnson et al., 2015; Bartlett et al., 2017; Edmunds and Lasker, 2019), which suggests octocoral populations to be recruitment limited, as occurs in other taxa (Connell, 1985; Grigg, 1988). Differences in key demographic processes, such as larval supply, settlement, or post-settlement survival throughout the recruitment phase might greatly influence recruitment success (Doropoulos et al., 2016), but their relative importance in driving octocoral recruitment remains largely unknown.
Accurately characterizing recruitment of Caribbean octocorals is particularly urgent since it may be an important component of octocoral’s resilience and to shifts in the benthic community structure observed in many Caribbean reefs (Edmunds and Lasker, 2016; Lasker et al., 2020). Hence, in this study, we characterized octocoral’s recruitment dynamics and evaluated the contribution of pre-settlement, settlement, and post-settlement processes in determining recruit densities. We distinguished between settlement and post-settlement processes occurring to single-polyps, from post-settlement processes occurring to colonial recruits ≤5 cm tall.
Materials and Methods
We conducted this study at two shallow fringing reefs (6 – 9 m depth) on the south shore of St. John, East Cabritte (18° 18.360′N, 64° 43.140′W) and Europa Bay (18° 19.016′N, 64° 43.798′W) (Figure 1). These reefs are representative of shallow fringing reefs of St. John, and are similar to other reefs throughout the Caribbean (Rogers et al., 2008). The hard substratum at both sites is a mix of igneous rock, carbonate rock generated by scleractinian corals, and sand patches (Riding, 2002). The octocoral community composition at the sites is similar, with 30 species at East Cabritte and 31 at Europa Bay (Tsounis et al., 2018). Those species are common throughout the Caribbean and typically found on fringing reefs and reef flats (Williams et al., 2015). East Cabritte is more exposed to wave action and has lower sedimentation rates than Europa Bay (Tsounis et al., 2018). Octocoral densities are 15.9 ± 0.5 colonies m2 (mean ± standard error) at East Cabritte and 7.6 ± 0.2 colonies m2 at Europa (Lasker et al., 2020). In 2017, the sites were impacted by Hurricanes Irma and Maria, but the hurricanes did not changed abundances at the sites (Lasker et al., 2020).
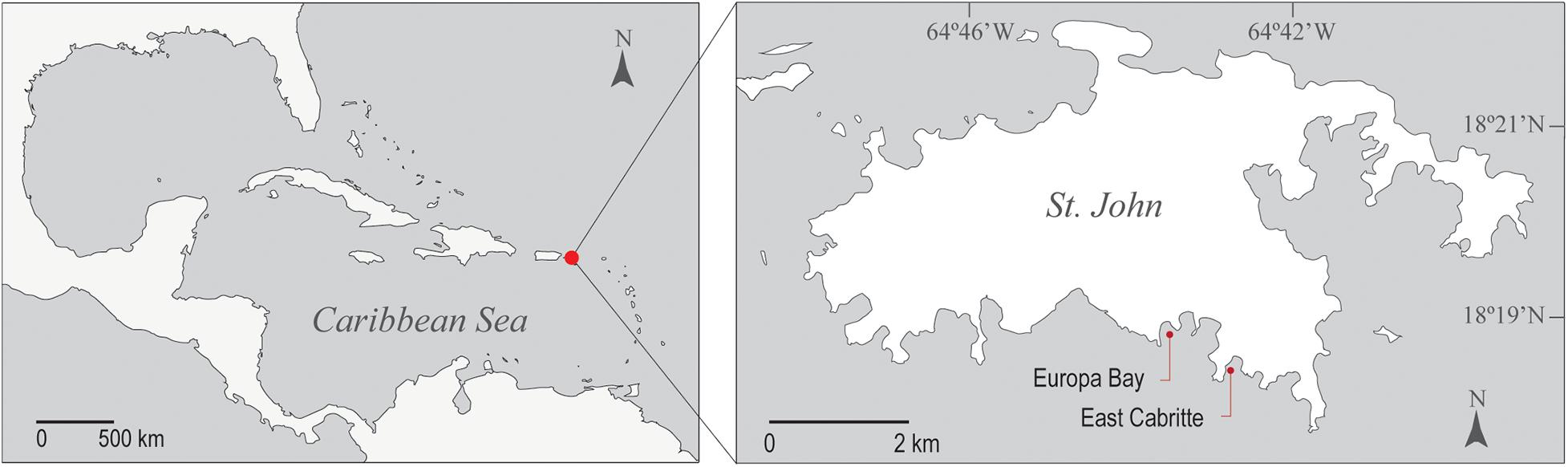
Figure 1. Map of study area in the Caribbean Sea, showing the study sites along the south shore of St John, United States Virgin Islands, Europa Bay (18°19.003N; 064°43.796W), and East Cabritte (18° 18.551N; 064° 43.129W).
To understand the dynamics of single-polyp recruits we made fine-scale temporal observations of settlers at the two sites for 3 years, and combined those data with a settlement experiment, using Plexaura homomalla larvae in the laboratory, and the subsequent survival of those settlers after we transferred them to the reef.
To study recruitment dynamics of colonial recruits, we located, mapped, and monitored their survival and growth on natural substrate at both sites for 3 years. We monitored the survival and growth of colonial recruits of the genera Eunicea and Pseudoplexaura.
Single-Polyp Densities on Settlement Tiles
To test whether single-polyp abundances on settlement tiles were different between sites and among years, we measured recruitment of single-polyps within a month of settlement at our study sites using custom made unglazed stoneware clay tiles (14 × 14 × 1 cm), deployed during the summer spawning period in 2017, 2018, and 2019. At each site, areas with similar densities of octocorals were selected and 30 tiles were installed approximately 1.5 m from each other over an area of 30 × 50 m. Coral larvae tend to settle on the undersides of tiles, and textured surfaces generally enhance settlement (Burt et al., 2009; Nozawa et al., 2011). Hence, we designed the settlement tiles with a flat upper surface, and a complex surface facing the substrate, with 1 cm diameter circular pits and 1 cm wide grooves, both 0.5 cm deep (Supplementary Figure 1A). We attached the tiles to the reef with threaded stainless-steel rods, epoxied into holes previously drilled in the substratum. Then, we positioned the tiles 5-8 cm above the substratum (Supplementary Figure 1D). At least 2 weeks prior to deployment, we seasoned the tiles in seawater under the dock at the Virgin Islands Environmental Resource Station (VIERS). We monitored single-polyp recruitment during the summer, when many of the Caribbean species spawn (Kahng et al., 2011). We placed the tiles on the reef ∼ 2 weeks before the full moon, and we retrieved them ∼ 2 weeks after, i.e., approximately 10 days after each inferred spawning event (see Brazeau and Lasker, 1989, 1990; Coma et al., 1995). The sampling scheme allowed for octocoral settlement before turf algae and other macroinvertebrates overgrew the tiles, minimizing potential competition for space. We sampled in June and July in 2017 and 2018, and at the beginning and end of July in 2019. We also deployed and monitored tiles additional months, which varied between years (Supplementary Table 1). In October 2017, Hurricanes Irma and Maria destroyed 19 tiles at East Cabritte and 2 tiles at Europa Bay. Thus, in November 2017 we analyzed recruitment of single-polyps on 10 tiles at East Cabritte and 28 tiles at Europa Bay.
At the end of each deployment, we transported the tiles to the lab in containers filled with seawater. At the lab, we kept the tiles in individual containers with seawater and counted living octocoral polyps by eye. To test the accuracy of the counts, we randomly selected 15 tiles and inspected them under a dissecting microscope (20× magnification). There were no differences in the number of polyps found. After inspection, we bleached the tiles with a 10% dilution of commercial sodium hypochlorite solution for 24 h, decalcified them with 10% hydrochloric acid, and then conditioned them at 1 m depth under the dock at VIERS for 15 to 17 days before deploying them again. This process allowed the tiles to condition for ∼4 weeks before settlement (2 weeks under VIERS dock and 2 weeks at each study site).
To test whether single-polyp abundances on settlement tiles were different between sites and among years, we built several generalized linear mixed-effects models (GLMM, “lme4” package in R; Bates et al., 2015) with a Poisson distribution and a logarithmic link function. Models included site (2 levels: East Cabritte and Europa Bay), and year (3 levels: 2017, 2018, 2019) as fixed effects, and sampling time (8 levels: June, July, 8-July, 30-July, August, September, October, November) and tile as random effects. To test the hypothesis, we selected the most parsimonious model, with the lowest Akaike Information Criterion (AIC). We could not test differences among all sampling periods and years due to lack of replication, but we tested whether the number of polyps at the peak of spawning each summer differed among years. Thus, we compared recruitment of single-polyps on tiles in July 2017, July 2018, and July 8, 2019 using a Generalized Linear Model (GLM) (R package “MASS;” Venables and Ripley, 2002) with a negative binomial distribution.
The Effect of Tile Conditioning on Larval Settlement
The abundance of single polyps we observed on the settlement tiles used to monitor early recruitment on the sites was the product of larval supply, settlement and post-settlement survival. We always deployed the tiles used to monitor local single-polyp densities (section above) at each site for 2 weeks before spawning. During those 2 weeks, site-specific marine biofilms (bacteria, archaea, fungi, protozoa, and unicellular microalgae) could have developed on the tiles (Shikuma and Hadfield, 2005; Salta et al., 2013; Kegler et al., 2017), impacting larval settlement (c.f., Hadfield, 2011). Therefore, we tested whether conditioning the tiles at each site had an effect on larval settlement by allowing larvae of a common octocoral, Plexaura homomalla, to settle on tiles preconditioned at each site. The collection and spawning of P. homomalla adult colonies, together with the conditions under which larvae were reared at the lab, have been described in detail in Wells et al. (2021).
The tiles we used in this experiment were identical to those used to monitor single-polyp recruitment at the study sites. We conditioned ten tiles at each site for 16 days prior to the start of the experiment. At the start of the experiment, we placed the tiles in 12 L polypropylene containers with 10 μm filtered seawater at 29°C. Two tiles, both conditioned on the same reef, were placed in each container (5 containers per treatment were considered as replicates). We positioned the tiles 5 cm above the bottom with a rigid mesh cylinder, with the rugose surface facing down (Supplementary Figure 2B). A low flow of air from a Pasteur pipette placed along one side of the container circulated water in the containers. At the start of the experiment 300 P. homomalla larvae were added to each container. We changed 40% of the water in each container twice daily during the first 2 days, and then once daily until the completion of the experiment on day 10. During the first 7 days of the experiment, we changed the water with water filtered through a 10-μm wound string cartridge filter, and with water filtered through a 50-μm wound string cartridge filter the following 2 days. After 10 days, larvae were no longer visible in the seawater, and we counted all larvae that settled and metamorphose into polyps (Supplementary Figure 1C). We tested for differences in numbers of polyps that settled on tiles conditioned in the two sites with a Generalized Linear Mixed Effects Model (GLMM) using site as the fixed predictor (factor with 2 levels: East Cabritte and Europa Bay), and each container as a random effect.
Survival of Single-Polyp Recruits on Settlement Tiles
To assess survival of recruits after settlement at each study site, we monitored the survival of the P. homomalla polyps that settled on tiles in the laboratory settlement experiment described above. At the end of the settlement experiment, we carefully deployed the tiles to the reef where the tiles were conditioned. The majority of the polyps (78%) settled on the bottom of the tiles, and we counted them in situ at the time of deployment, and on days 2, 5, 9, 14, 19, and 59-62 after deployment. While on the reef, we observed the natural settlement of unknown octocoral species on the tiles (see Wells et al., 2021). In order to unambiguously follow survival, we mapped the position of each polyp on 5 randomly selected, but previously tagged, tiles at East Cabritte, and 3 tiles at Europa Bay on days 7 and 10, respectively. To map and monitor polyps, we drew a diagram of each settlement tile on which we recorded the position of each polyp. Survival of the mapped polyps was monitored on day 9 at East Cabritte, after which all mapped polyps were monitored on days 12, 14, 17, 19, and 59-62.
To test whether single-polyp survival differed between sites, we built a Mixed Effects Cox proportional hazards model with the coxme R package (Therneau, 2013). Site was a fixed effect and polyps and tiles random effects, with polyps nested within tiles. Cox regression models assume that each covariate has a relative effect in the hazard function that is constant over time (i.e., proportional hazard assumption), and its relative effect is constant over time. We tested whether the data met the proportional hazards assumption prior to the analysis, as well as the effect of outliers. We visualized the probability of survival over time with a Kaplan–Meier (KM) log-rank survival curve using the “survival” R package (Therneau, 2014).
Composition, Abundance, and Size-Structure of Recruits on Reef Substrata
To characterize the density of single-polyp recruits and the density, composition and size-structure of colonial recruits (those larger than 1 polyp and up to 5 cm height) at the study sites, we surveyed, at each site, eight 50 × 50 cm quadrats placed randomly on the substratum along six, fixed 10-m long transects (separated by 10 m), i.e., 48 quadrats per site (see Tsounis et al., 2018 for a description of the transects). The same observer (ÁMQ) conducted the surveys during June and July from 2016 to 2019. Single-polyps (Supplementary Figure 1E) and colonies up to 5 cm height were counted (Supplementary Figures 1F-H). We analyzed the density of single-polyps, which cannot be identified with certainty, separately from colonial recruits, which were identified to genus in most cases. Eunicea spp. recruits, were grouped into two groups based on the presence or absence of large, prominent calyces. Eunicea large calyx recruits had, as the name indicates, large calyces and a stiff surface due to high sclerite density (Bayer, 1961). This group included E. calyculata, E. clavigera, E. tournefortii, E. laciniata, E. succinea, E. mammosa, and E. laxispica recruits. Eunicea spp. with small calyces had greater axial flexibility. This group included E. asperula, E. flexuosa, E. palmeri, E. pinta, and E. pallida. The average number of recruits 0.25 m–2 (±SD) was calculated for each genus/group, at each site and year.
Survival and growth of octocoral recruits has been related to their size (Yoshioka, 1998). Hence, we measured colonial recruit heights with calipers to 1 mm accuracy, and described the relative abundances of different size classes of colonial recruits from the two most abundant genera in our study sites, Eunicea and Pseudoplexaura. Recruits with 2-3 polyps were too small to be measured accurately, and their heights were approximated as being 3 mm. We classified colonial recruits into 4 size classes <0.6 cm, 0.6 – 1.1 cm, 1.2 – 2 cm, 2.1 – 5 cm, based on the 25th, 50th, 75th, and 100th percentiles of the size frequency distribution of recruits in our study sites. We calculated the relative abundance and the average number of recruits of each size class (±SD) within each genus, calyx size, site and year.
We compared single-polyp density on reef substrate between sites and years using GLMs with a negative binomial distribution, with site (2 levels: East Cabritte and Europa Bay) and year (4 levels: 2016, 2017, 2018, and 2019) as the predictors. We tested whether density and composition of colonial recruits between sites were different over the course of the study using Generalized Linear Models (GLM) with a Poisson distribution. We included the density of colonial recruits as the dependent variable and used year, genus (9 levels: Antillogorgia, Eunicea, Gorgonia, Muricea, Muriceopsis, Plexaura, Plexaurella, Pseudoplexaura, Pterogorgia), and site as predictors. In each case, we selected the models with the lowest Akaike Information Criterion (AIC). We performed a post hoc analysis of estimated marginal means (EMMs) (Searle et al., 1980) with the R package “emmeans” (Lenth et al., 2018) to test pairwise differences in the density of colonial recruit of different genera, between sites and among years. To perform the post hoc analyses, we log-transformed the data. We used Generalized Linear Models (GLM) with a negative binomial distribution to test whether recruit size structure of the two most abundant genera (2 levels: Eunicea and Pseudoplexaura) differed between sites and among years, whereas for Eunicea calyx size groups (2 levels: large calyx and small calyx) we used a GLM with a Poisson distribution. In both cases, we included recruit density (0.25 m2) in the models as the dependent variable and genus or calyx size group, size class (4 levels: <0.6 cm, 0.6 -1.1 cm, 1.2 – 2 cm, 2.1 – 5 cm), site and year as predictors. Next, we performed post hoc analyses of EMMs to estimate pairwise differences on the size-frequency distribution of colonial recruits of different genera, and calyx sizes within and between sites and among years using log-transformed data.
Survival and Growth of Colonial Recruits
To assess survival and growth of colonial recruits, we followed the fate of 359 colonial recruits of Eunicea spp. and Pseudoplexaura spp. (Supplementary Figures 1F-H). We mapped recruits along two 10 m transects at East Cabritte (192 recruits), and three 10 m transects at Europa Bay (167 recruits). To compare survival and growth between colonial recruits with different calyx sizes, we divided Eunicea spp. in two groups based on the presence of large calyces. In addition, we mapped and monitored 19 single-polyps of unknown genera. To map the recruits, we installed stainless steel rods at ∼1 m intervals along the transects, and used them to locate the recruit by triangulation, measuring the distances of each recruit from 2 consecutive rods. At East Cabritte, we monitored 25 Eunicea spp. with large calyces, 83 Eunicea spp. with small calyces, 71 Pseudoplexaura spp. and 16 unknown, and at Europa, we monitored 44 Eunicea spp. with large calyces, 40 Eunicea spp. with small calyces, 80 Pseudoplexaura, and 3 unknown.
To test the effect of recruit height on survival and growth, we measured colony heights (cm), from August 2016 to August 2019 or until the recruit died. Initial measurements took place on 4 August 2016, and were repeated 6, 11, 15, 20, 23, 31, and 36 months later. It was impossible to locate and measure all recruits in a single day. Hence, we took the measurements within a maximum of 10 days. We calculated “realized growth” rates based on the average growth of all recruits. We calculated “potential growth” rates excluding those with negative growth and those for which there was evidence of partial mortality. Lastly, we tested whether partial mortality affected subsequent growth rates, and designated all growth measurements that occurred before an observation of negative growth as “pre-damage” and all measurements taken after observed negative growth as “post-damage.”
To test the effect of genus, morphology, and height on the survival of colonial recruits, we built Mixed Effects Cox proportional hazards models. One analysis incorporated genus (2 levels: Eunicea, Pseudoplexaura) and calyx size (2 levels: Eunicea large calyx; Eunicea small calyx) as time-independent covariates, while a separate model incorporated height as a time-dependent covariate. Site, recruit ID (i.e., each colonial recruit), height of the recruit in the time interval prior to dying, and the time-interval of the observation were incorporated as random effects. We selected the most parsimonious models using Akaike Information Criterion (AIC). None of the models incorporated the covariate “damaged” (2 levels: yes/no) because it was positively correlated with recruit height. Prior to analysis, we tested the proportional hazards assumption, the effect of influential observations, and the linearity of the covariate height. We removed colonial recruits from the analysis once they reached juvenile heights (>5 cm). To visualize the risk of death (or the survival rate) due to recruit size we plotted the hazard ratio as a function of recruit height (cm). To visualize differences in survival over time for colonial recruits of different sizes we used Kaplan–Meier (KM) log-rank survival curves of the 0th, 25th, 50th, 75th, and 100th height percentiles. We also used this analysis to obtain non-parametric estimates of the median survival time and standard deviations for colonial recruits in the 4 size classes (<0.6 cm, 0.6 – 1.1 cm, 1.2– 2 cm, 2.1 – 5 cm).
We examined the effects of genus, calyx size, and height, on potential and realized growth rates of colonial recruits using a series of regressions that also included the covariates site, time interval and year in the analyses. To test whether different genera differed on their potential growth rates, an initial set of models included genus, height, time interval (7 levels: From month 6 to 36), and site as predictors. To test whether recruits with different morphological traits differed in their potential growth rates another set of models included calyx size, height, time interval, and site as possible predictors. To test whether recruits of different genus or calyx size differed in partial mortality, we also built models using realized growth rates as the independent variable and as predictors all the covariates stated above, and including also the predictor “damaged” (2 levels: yes/no). To test the effect of calyx size, we used linear mixed effect models (LMMs), evaluated the covariates height, time interval, and each recruit as possible random effects, and selected the model with the lowest AIC. The lack of replication prevented us from using generalized linear mixed-effects models (GLMMs). To test the relevance of the covariates, genus, height, time interval, site, and “damaged” on realized growth we used stepwise algorithms on GLMs, and selected the models with the lowest AICs. We followed the same approach to test genus, height, time interval, and site as predictors of potential growth rates (using only data from recruits that never experienced negative growth).
We tested whether recruits of different genera or calyx size had different growth rates before and after partial damaged, i.e., negative growth. Additionally, we compared differences in growth rates between colonial recruits that never experienced partial mortality and growth rates of those that experienced negative growth (prior to damage). In both cases, we used GLMs with Gamma distributions, adding 1 to all values to eliminate the presence of zeros in the data set. To compare colonial recruits’ growth rates before and after being damaged we evaluated the covariates height and condition (2 levels: pre-damaged/post-damage) used as individual terms and as an interaction. Also, we tested weather growth rates of recruits that never suffered negative growth were different than those experiencing damage (prior to damage), and built GLMs including the covariates calyx size, genus, height, site, and “damage” as individual terms and as an interaction. In both cases, we selected the model with the lowest AIC to test our hypotheses.
Finally, we assessed whether the frequency of growth, zero-growth, or negative growth was different between recruits of different genera, calyx sizes, and size classes. To test the hypothesis that growth, zero-growth and negative growth was size-dependent, we partitioned the colonial recruit cohort into two size classes, above or below 1.1 cm, i.e., the median colonial recruit size. We performed Fisher’s exact tests of independence, and, when necessary, a pairwise Fisher test to test whether the frequency of cases in the three growth categories was affected by the colonial recruit size class, genus, calyx size or site.
Results
The key results of each section below are summarized in Table 1.
(1) Single-polyp densities on settlement tiles:
Octocoral polyps recruited to tiles at East Cabritte in consistently higher numbers than at Europa Bay (Figure 2. GLMM: p < 0.001, Supplementary Table 2.1). At both sites, 99% of the recruits were observed on the underside of tiles, with counts ranging from 0 to 15 polyps tile–1 at East Cabritte, and from 0 to 5 at Europa Bay (Figure 2). At East Cabritte, the greatest number of single-polyp recruits was found in July 2017, with 5.5 ± 4.4 polyps tile–1 (mean ± SD), and a median of 5 polyps tile –1 (Figure 2). Comparing years, there were significantly fewer polyps tile–1 at East Cabritte in July 2018 compared to 2017, with 1.6 ± 2.1 polyps tile–1 (mean ± SD) and a median of 1 polyp tile–1 (Figure 2. GLM: p < 0.05 Supplementary Table 2.2). At Europa Bay, recruitment of single-polyps was zero most of the time, with the exceptions of September and November in 2017, and October in 2019, with the greatest number of single-polyp recruits found in November 2017, with 0.5 ± 1.07 polyps tile–1 (mean ± SD), and a median of 0.5 polyps tile –1 (Figure 2).
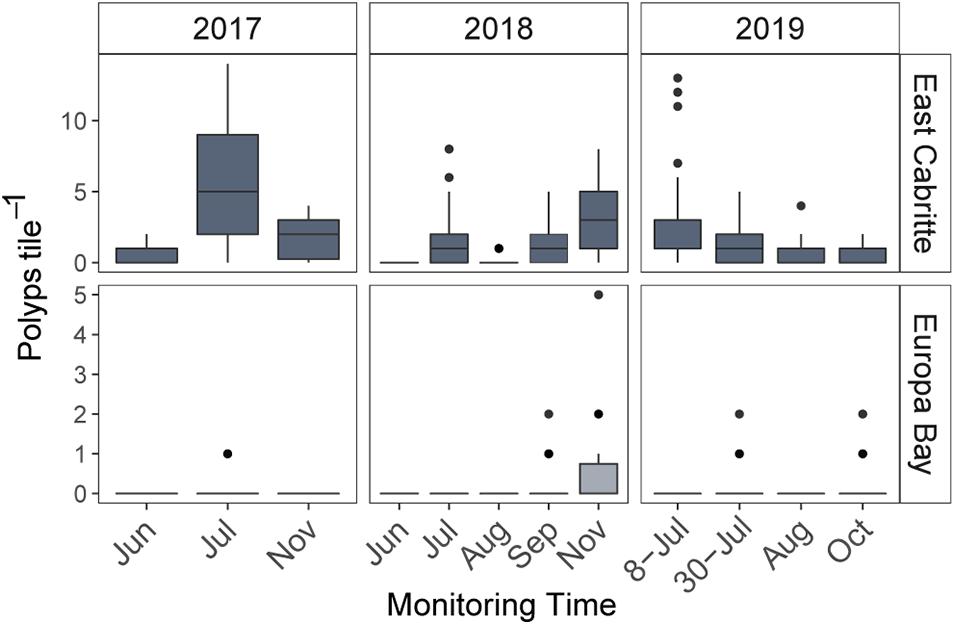
Figure 2. Boxplots comparing the median, 25th and 75th percentiles of polyps tile– 1 among sampling times, and between sites in 2017, 2018, and 2019. Note scales of the y-axes at each site are different, and settlement tiles were monitored different months each year. Recruitment, which was measured within 2 weeks of settlement, was consistently higher at East Cabritte than at Europa Bay each year (p < 0.001). At East Cabritte, the number of polyps on the tiles decreased in July 2018 (p < 0.01) and increased in July 8, 2019.
(2) The effect of tile conditioning on larval settlement:
As on the tiles used to monitor recruitment in the field, the majority of P. homomalla polyps (78%) were found on the bottom of the tiles. Pre-conditioning the settlement tiles on different reefs had an effect on the settlement of P. homomalla larvae under laboratory conditions (GLMM: p < 0.05, Supplementary Table 3.1). We found higher settlement on tiles preconditioned at Europa Bay than at East Cabritte, with an average of 77.8 ± 29.4 polyps tile–1 (mean ± SD) and 61.6 ± 16.3 polyps tile–1 (mean ± SD), respectively.
(3) Survival of single-polyp recruits on settlement tiles:
Two hundred forty four polyps were monitored at East Cabritte, and 78 at Europa Bay on 5 and 3 tiles, respectively. Survival was not significantly different between sites (Coxme: p > 0.05, Supplementary Table 3.2), and the Kaplan-Meier curve showed the probability of surviving up to 20 days was 85 ± 0.02% (Figure 3). Mortality among the 322 monitored polyps ranged from 0 to 2 polyps day–1, with an average of 0.59 polyps day–1. At the end of the experiment, on day 59, only 27 polyps at East Cabritte and 4 polyps at Europa Bay remained on the undersides of the tiles. Tiles were not surveyed between days 20 and 59. Thus, in Figure 3, survivorship between those dates ranged from 72 ± 2% to 7 ± 2% in a single step.
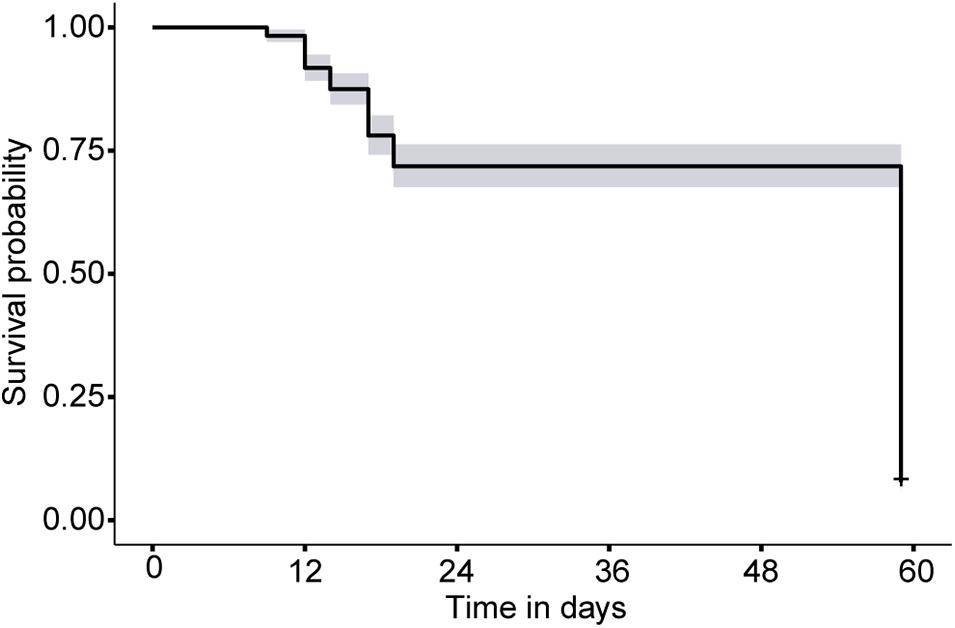
Figure 3. Kaplan-Meier curve depicting survivorship of mapped single polyp recruits on settlement tiles at both sites on St. John United States Virgin Islands. The curve shows survivorship and 95% confidence intervals through 59 days. Time zero corresponds to the point in time when polyps were mapped on the tiles. The large drop in survivorship at 59 days reflects the cumulative loss of individuals between days 20 and 59.
(4) Composition, abundance and size-structure of recruits on reef substrata:
Single-polyp densities differed between sites and among years (most parsimonious GLM model, Supplementary Table 4.1). The most parsimonious GLM to evaluate the abundance and composition of colonial recruits included year, and the genus and site interaction (Supplementary Table 4.2). Recruits were more abundant at East Cabritte than at Europa Bay each year, independently of being single-polyps or colonial (Figure 4. GLMs p < 0.001. Supplementary Table 4).
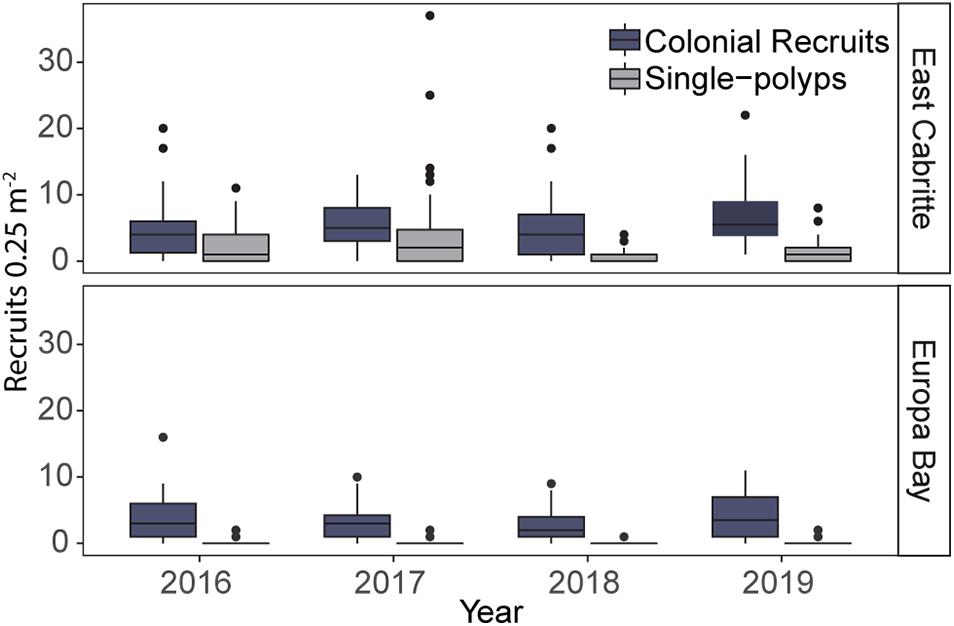
Figure 4. Boxplot comparing the median, 25th and 75th percentiles of single-polyp and colonial recruit abundances on reef substrata from 2016 to 2019. Abundances of single-polyps and colonial recruits were consistently higher at East Cabritte than at Europa Bay (p < 0.001). The abundance of single-polyps and colonial recruits declined in 2018 (p < 0.001), but at East Cabritte, colonial recruit abundances increased in 2019 (p < 0.05).
The maximum density of single-polyps occurred at East Cabritte in 2017, with 3.86 ± 6.69 (mean ± SD) polyps 0.25 m–2, and a median of 2 polyps 0.25 m–2 (Figure 4), whereas, at Europa Bay, the maximum density occurred in 2016, with 0.19 ± 0.5 (mean ± SD) polyps 0.25 m–2 and a median of zero polyps 0.25 m–2 (Figure 4). In 2018, the summer following hurricanes Irma and Maria, the densities of single-polyps markedly declined, especially at East Cabritte, with 0.54 ± 0.96 polyps 0.25 m–2 (mean ± SD) and a median of 0 polyps 0.25 m–2 (Figure 4. GLM: p < 0.001, Supplementary Table 4.1). As for the single-polyp recruits, abundances of colonial recruits decreased in 2018 compared to previous years (Figure 4. GLM: p < 0.001, Supplementary Table 4.2). However, in contrast to single-polyps, they significantly increased in density in 2019 (Figure 4. GLM: p < 0.01, Supplementary Table 4.2). Indeed, the maximum density of colonial recruits occurred that year (2019) at both sites, with 6.9 ± 4.5 recruits 0.25 m–2 (mean ± SD), and a median of 6 recruits 0.25 m–2 at East Cabritte, and with 4.31 ± 3.3 recruits 0.25 m–2 (mean ± SD), and a median of 3 recruits 0.25 m–2 at Europa Bay (Figure 4).
The same 9 genera were present at both sites (Figure 5A), and recruits of Eunicea spp. And Pseudoplexaura spp. Were the most abundant at both sites, each year (Figure 5A. EMMs p < 0.001, Supplementary Figure 2). However, Antillogorgia spp., and Plexaura spp. recruits were more abundant at East Cabritte (Figures 5A,B. EMMs: p < 0.001, Supplementary Figure 2), whereas Gorgonia spp., Muricea spp., Muriceopsis spp., and Plexaurella spp. were more abundant at Europa Bay (Figure 5A. EMMs: p < 0.001, Supplementary Figure 2). At East Cabritte, Eunicea spp. recruits were more abundant than Pseudoplexaura spp., with maximum densities of 4.08 ± 3.09 recruits 0.25 m–2 in 2019, and 1.36 ± 1.56 recruits 0.25 m–2 (Mean ± SD) in 2017, respectively (Figure 5A. EMMs p < 0.001, Supplementary Figure 2). At Europa Bay, densities of Eunicea spp. And Pseudoplexaura spp. recruits were similar, reaching densities of 2.6 ± 2.25 recruits 0.25 m–2 in 2019 (Figure 5A. EMMs p > 0.05, Supplementary Figure 2).
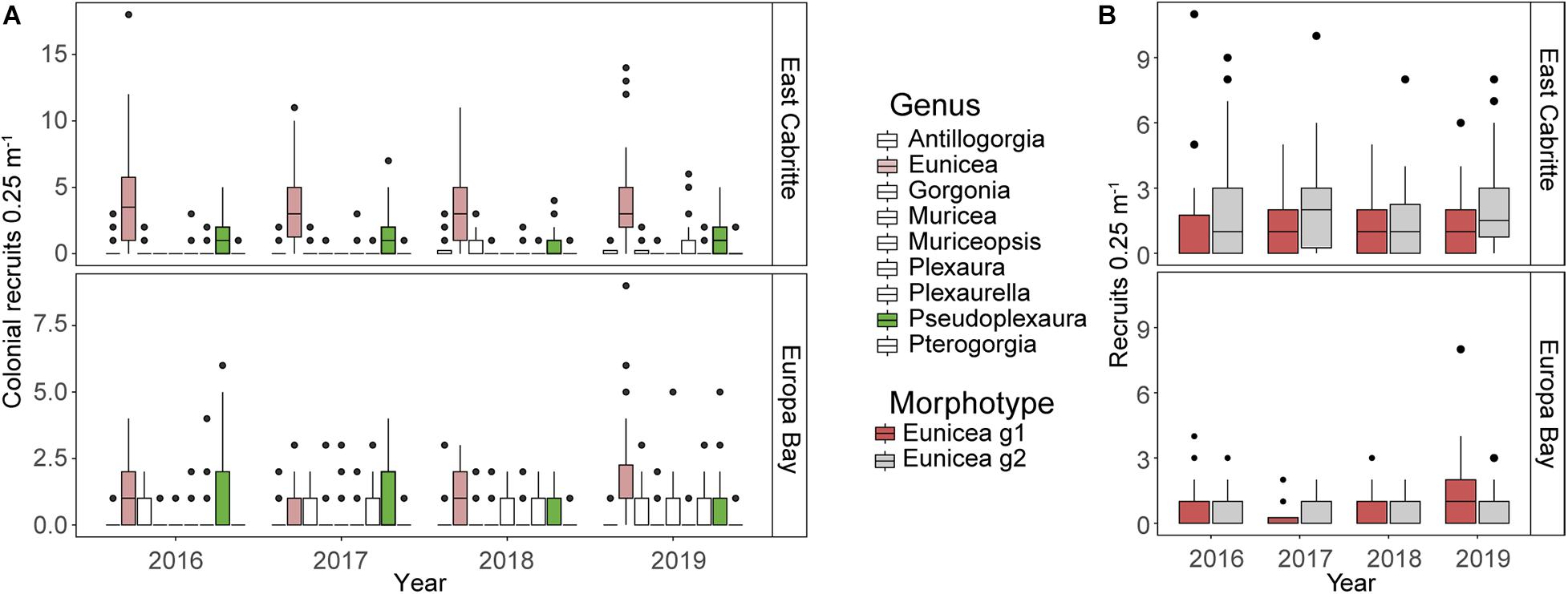
Figure 5. Boxplots comparing the median, 25th and 75th percentiles abundances of colonial recruits 0.25 m–2 at East Cabritte and Europa Bay from 2016 to 2019. (A) compares abundances among the nine genera present at the study sites. Eunicea and Pseudoplexaura, highlighted in color, were the two most abundant genera at both sites, but they were more abundant at East Cabritte than at Europa Bay (p < 0.001). Genera are alphabetically ordered in the x-axis. (B) depicts the abundances of Eunicea spp. recruits with two different morphological traits (Eunicea g1: recruits with prominent calyces and Eunicea g2: recruits without prominent calyces), with Eunicea g2 more abundant at East Cabritte, whereas Eunicea g1 were more abundant at Europa Bay (p < 0.001).
The most parsimonious model describing the abundances of Eunicea spp. recruits with different calyx size included year, and the interaction between site and calyx size as predictors (Supplementary Table 5). At Europa Bay, the relative abundances of recruits with different calyx sizes were similar, with 0.41 ± 0.69 recruits 0.25 m–2 (mean ± SD) (EEMs p > 0.05. Supplementary Figure 3). However, at East Cabritte Eunicea group 2 recruits (small calyces) were more abundant compared to Eunicea group 1 (large calyces) (Figure 5B, EEMs p < 0.05, Supplementary Figure 3). In both sites, abundances of recruits of different calyx sizes did not change between 2016 and 2018. However, in 2019, abundances of recruits with both calyx sizes significantly increased to 0.9 ± 1.27 recruits 0.25 m–2 (mean ± SD) at Europa Bay, whereas only Eunicea group 2 increased to 1.96 ± 1.97 recruits 0.25 m–2 (mean ± SD) at East Cabritte (Figure 5B, EEMs p < 0.05, Supplementary Figure 3).
Size class structure of the colonial recruits varied between years, and genera, and there also was an interaction between site and size class (Supplementary Table 6.1). Similarly, the analysis of size class structure with respect to calyx morphology identified calyx size, and the interaction between sites and size class as the best predictors (Supplementary Table 6.2). Independent of genera and calyx morphology, recruits within the 0.6 – 1.2 cm size-class were the most abundant at East Cabritte (Figures 6A,B. EMMs p < 0.001. Supplementary Figures 4A,B). Whereas at Europa Bay, recruits > 2.1 cm were the most abundant (EMMs p < 0.05, Supplementary Figure 4.1).
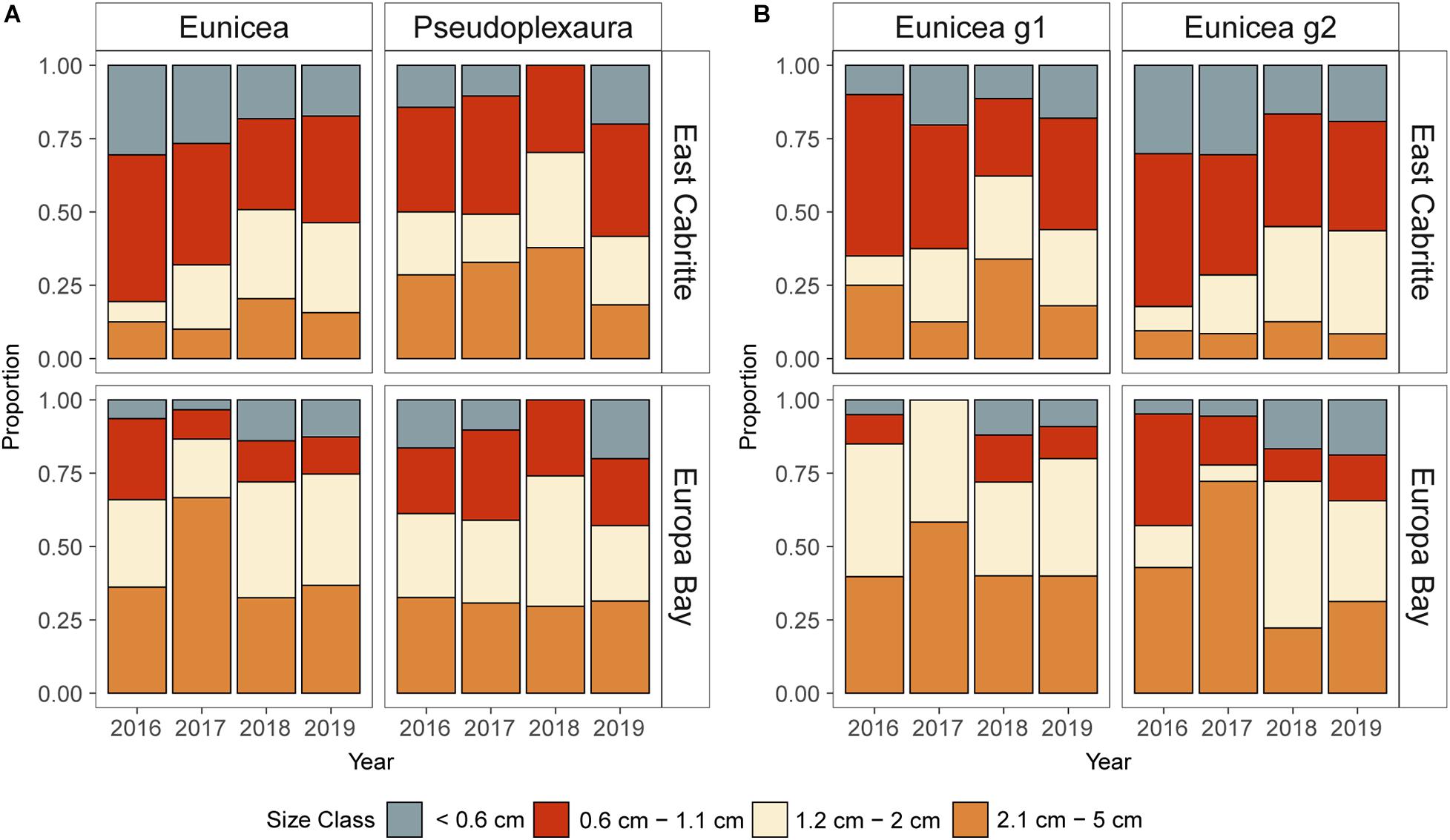
Figure 6. Stacked bar graphs depicting the size frequency distributions of colonial recruits at East Cabritte and Europa Bay from 2016 to 2019. (A) Eunicea spp. and Pseudoplexaura spp. recruits. (B) Eunicea calyx sizes Eunicea g1 (With prominent calyces) and Eunicea g2 (without prominent calyces). Independently of genus or calyx size, small recruits (≤1.1 cm tall) were proportionately more common at East Cabritte, while larger recruits (>1.2 cm tall) were more common at Europa Bay (p < 0.001).
(5) Survival and growth of colonial recruits:
The positions of 359 colonial recruits present on the reefs at East Cabritte and Europa Bay were mapped in August 2016. In September 2017, Hurricanes Irma and Maria displaced some of the rods used to find the recruits, and 63 of them could not be located after summer 2017. After 36 months, only ∼ 6% (n = 17) of the initially mapped recruits were still alive.
There were no significant differences in survivorship between Eunicea spp., and Pseudoplexaura spp. (Coxme: p > 0.05, Supplementary Table 7.1), or between colonial recruits with different calyx morphology (Coxme: p > 0.05, Supplementary Table 7.2), and only their height influenced their survival (Figure 7. Coxme: p < 0.001, Supplementary Table 7.3). The hazard ratio of 0.75 indicates the risk of dying decreased by 25% with each additional cm of height (Figure 7 and Supplementary Table 7.3). The median survival time for recruits ≤ 1.1 cm tall was 11 months (Figure 8), while the median survivorship of 2 and 5 cm tall recruits was 15 and 31 months, respectively. The probability of surviving 12 months ranged from 40% for the smallest size class (<0.6 cm) to 76% for recruits ≤ 5 cm (Figure 8).
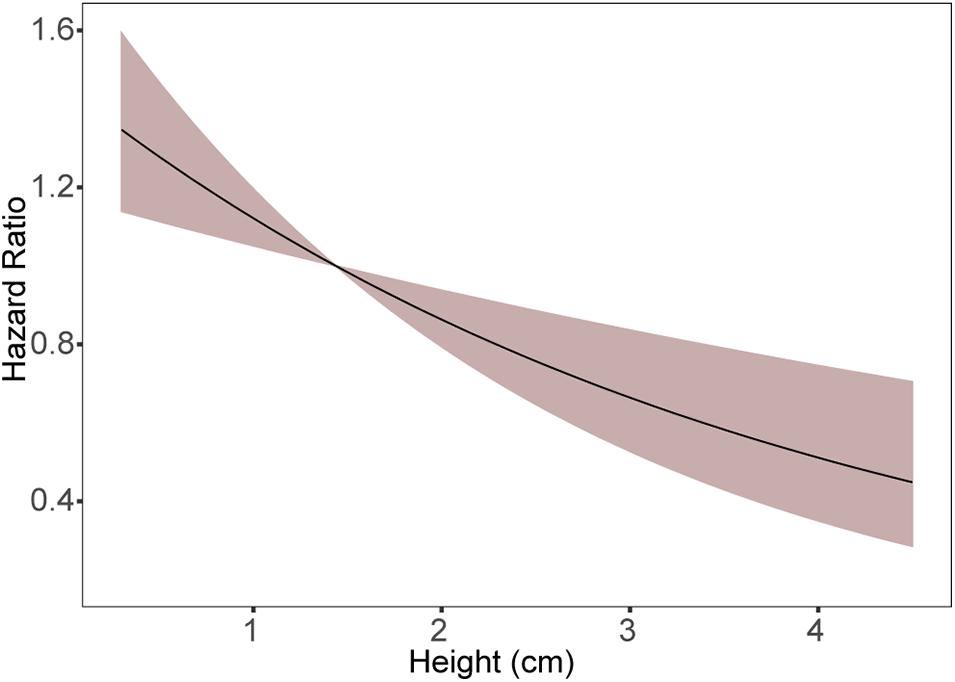
Figure 7. Proportional Hazard Ratio as a function of colonial recruit height. The Hazard ratio = 1 (equal hazard) when recruits height equals the mean height (1.1 cm).
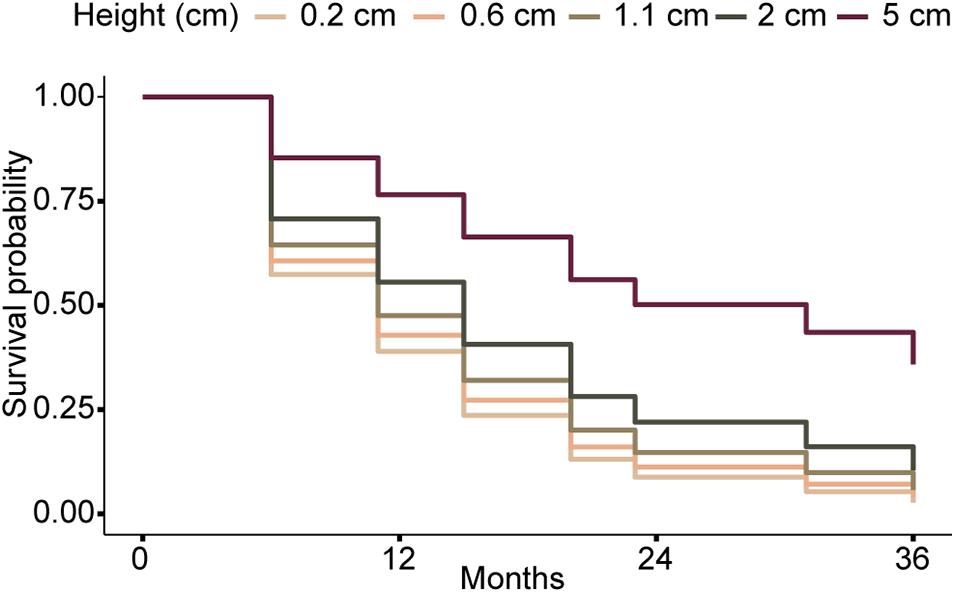
Figure 8. Kaplan-Meier curves depicting survivorship of colonial recruits of different sizes over time (assuming recruits do not change in size). Sizes correspond to the 25th, 50th, 75th, and 100th percentiles of colonial recruit heights in our areas of study. Confidence intervals are not depicted for visual clarity.
We measured growth rates of colonial recruits for which there were multiple observations (N = 189). Fifty-seven recruits (∼ 30%) displayed net negative growth, 16 (∼ 8.5%) did not (Figure 9) grow, and eleven (∼ 6%) made it to the juvenile stage, i.e., grew above 5 cm. Four of those recruits (∼ 2%) were alive until the end of the experiment, and the maximum height that we observed after 3 years was reached by a Pseudoplexaura sp. (18 cm). Growth rates ranged from –1.3 cm/month to 0.7 cm month–1 (Figure 9). The most parsimonious LMM model of growth included height as a random effect, nested within time interval (Supplementary Table 8.1). Genus, time interval, site and calyx size among Eunicea spp. were not informative in explaining variation in realized growth rates of colonial recruits.
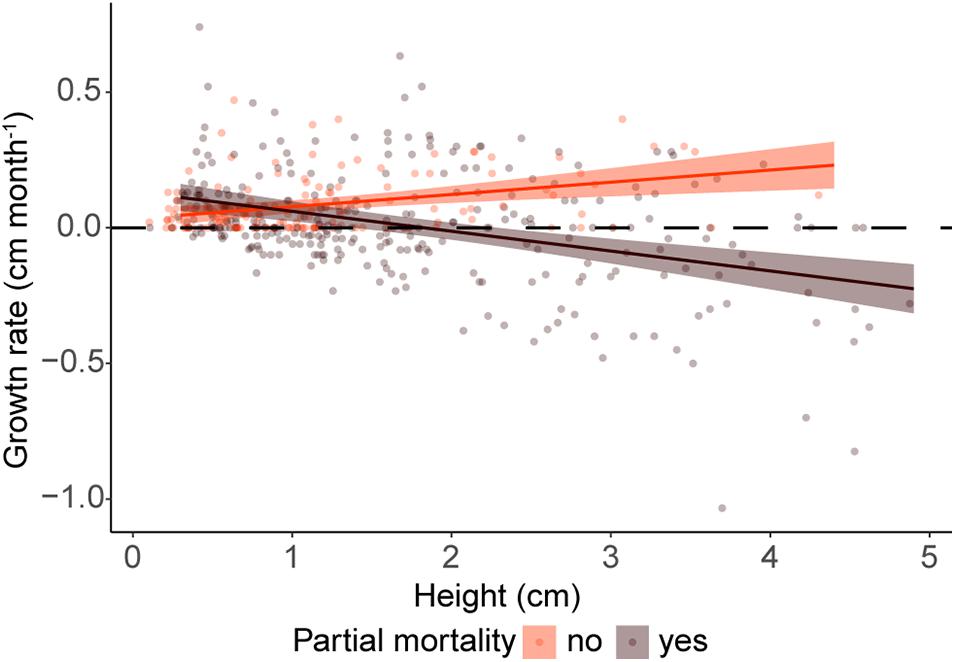
Figure 9. Realized growth rates as a function of height for colonial recruits that suffered (brown) and never suffered (red) partial mortality. The horizontal dashed lined represent no-growth.
The model with the lowest AIC included height and time interval as predictors of potential growth (Supplementary Table 9.1). When the presence of damage was added to the analysis, the best model of realized growth rates included the covariate “damage” as both, an individual and an interaction term (Supplementary Table 9.2). Colonial recruits that never suffered partial mortality increased their growth rates by 0.04 cm/month with each additional cm in height (Figure 9. GLM: p < 0.001, Supplementary Table 9.2). Conversely, the growth rates of recruits that experienced partial mortality, decreased by 0.07 cm/month with each cm in height (Figure 9. GLM: p < 0.05, Supplementary Table 9.3). Additionally, potential growth rates decreased significantly during the time interval right after Hurricanes Irma and Maria in 2017 (GLM: p < 0.05, Supplementary Table 9.1), but we did not detect differences among other time intervals (GLM: p < 0.05, Supplementary Table 9.1).
The final GLMs testing whether different genera or calyx morphologies had different growth rates before and after being damaged, included as predictors the terms calyx size, genus and “condition” (i.e., pre-damage vs. post-damage; Supplementary Table 10). In both cases, growth rates were similar before and after partial mortality (GLMs: p > 0.05, Supplementary Table 10).
The most parsimonious GLM testing the effect of height and partial mortality on recruit growth rate included the interaction term height and partial mortality (Figure 9 and Supplementary Table 11.1), while, the best GLM testing the effect of genus and calyx size included two interaction terms, calyx size by partial mortality and genus by partial mortality (Supplementary Tables 11.2, 11.3).
Cases of zero net-growth were more common at East Cabritte than at Europa Bay (p < 0.001), and we observed an absence of growth in the 28% of our surveys at the former site compared to 13% at Europa Bay (Figure 10). This absence of growth at East Cabritte, impacted in particular recruits < 1.2 cm tall (Figure 10, p < 0.05), whereas recruits > 1.2 cm tall experienced negative growth more frequently (25-32% of the sampling times; p < 0.05), independently of site, genera or calyx size.
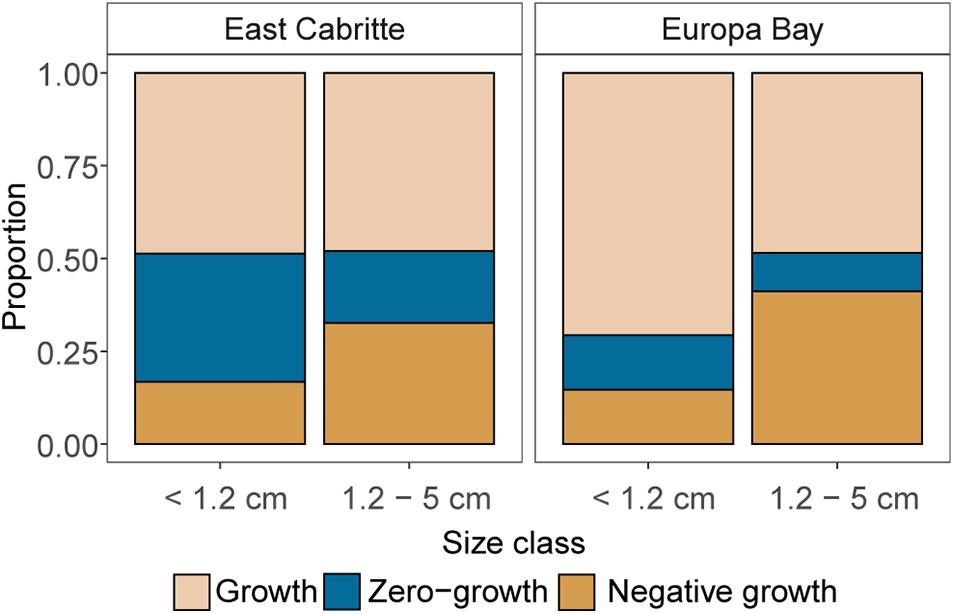
Figure 10. Proportions of events in which growth, zero-growth and negative growth were observed for colonial recruits smaller and larger than median recruit height (1.1 cm) at each study site. Negative growth, i.e., partial mortality, occurred more often to larger recruits (>1.2 cm tall), independently of site, whereas we observed no-growth more frequently on smaller recruits at East Cabritte.
Discussion
Among benthic colonial taxa, the shift from a solitary to a colonial form adds a layer of complexity to their life histories and introduces trajectories in growth and size, such as partial mortality that are not available to unitary organisms. That complexity impacts the measurement and our understanding of recruitment. Definitions of recruitment generally have been operational (Hunt and Scheibling, 1997; Vermeij and Sandin, 2008), and studies of colonial taxa, have either followed single-polyps or used size to define the recruit life-history stage. By distinguishing single-polyps from the colonial recruits they develop into, we have identified larval supply as a major driver of octocoral densities in the earliest stages of recruitment, and partial mortality as a post-settlement process that uncouples the relationship between recruit age and size. By providing a comprehensive analysis of the recruitment phase for one of the most abundant taxa on Caribbean coral reefs, the present study facilitates future comparisons among benthic colonial taxa, and contributes to our understanding of their population dynamics.
Single-Polyp Recruit Dynamics
We expected the supply of larvae to the sites would be similar, and local recruit densities would be driven mainly by settlement and post-settlement processes, as occurs for other marine invertebrate taxa (Jenkins, 2005; Bohn et al., 2013; Harper, 2017). Most octocorals at the study sites are broadcast spawners that release gametes that are fertilized and develop while being dispersed in the water column (Kahng et al., 2011). Developing embryos require days to develop and may spend several weeks in the water column (Graham et al., 2013; Coelho, 2018). The south coast of St. John is exposed to a generally westward flow of water. The study sites were <2 km apart, both exposed to the swell that comes out of the southeast. In contrast to our expectations, we found larval supply was the primary predictor of single-polyp densities at both sites. Recruitment of single-polyps on the reef substratum at East Cabritte was two-fold higher than at Europa Bay. Those differences on single-polyp densities could have been driven by the absence of suitable habitat to settle, i.e., free space and settlement cues to metamorphose (Hadfield and Paul, 2001; Arnold and Steneck, 2011), or by different post-settlement survival at each site. However, we found a similar pattern on the settlement tiles we deployed each year. Furthermore, P. homomalla larvae settled successfully on tiles conditioned at each site, and settled in larger numbers on tiles preconditioned at Europa Bay, indicating differences in biofilms were not driving the patterns. Survival of the newly settled P. homomalla polyps was similar between sites. These results suggest the lower recruitment of single-polyps to tiles at Europa Bay than at East Cabritte was not limited by settlement and post-settlement processes, leaving supply as the most plausible explanation for the large difference in single-polyp densities between sites.
Factors that could account for the differences in supply to the sites include self-recruitment and differences in currents operating on scales of kilometers and less. Although self-recruitment can occur, even for species with potential for long-distance dispersal (Cowen and Sponaugle, 2009), the prevalent westward flow and the short distance between sites (<2 km) suggests gametes from broadcast spawners would not develop quickly enough to self-recruit. Nonetheless, environmental factors such as wave exposure and coastal topography can lead to highly variable larval supply at small-scales (sites separated by 100 s meters) (Gaines and Roughgarden, 1985; Hunt and Scheibling, 1997; Pineda et al., 2009), and local eddies may have generated differences in the delivery of larvae between bays (Monismith, 2007).
We did not detect differences in the post-settlement survival of P. homomalla between sites and ∼ 95% of the polyps in the present study survived 15 days. The similarity in survival at the two sites, as well as the initially high survival, likely reflects the lack of competitors and perhaps resident predators on the tiles. Heterotrophic invertebrates can affect the survival of scleractinian coral settlers on artificial substratum (Vermeij, 2006; Arnold and Steneck, 2011), but those competitors were not present on our tiles, which had only been on the reef for 2 weeks. Survival shortly after settlement reported in other studies tends to be much lower than we observed (Keough and Downes, 1982; Hunt and Scheibling, 1997), and Lasker et al. (1998) reported 40% survival for polyps of Plexaura kuna in Panama (Lasker et al., 1998). In that study, polyps were subject to competition with heterotrophic invertebrates that were present on the settlement tiles and to predation, since the settlement plates were attached to the reef facing upwards. Similarly, Evans et al. (2013) observed high mortality of polyps on plates that were exposed to predators. In our experiment polyps settled mostly on the undersides of tiles that also had a variety of structural features, which were likely to provide protection against predation (Doropoulos et al., 2016). Our observations are important in characterizing the similarity of processes at the two sites, but over longer time scales, heterotrophic invertebrates become common on cryptic surfaces (Jackson, 1977), and competition under those conditions may be substantially lower.
Colonial Recruit Dynamics
Larger recruits survived longer and grew faster than smaller recruits. Previous studies of octocorals, scleractinians, and sponges have shown the relationship between survival and size is maintained across different life history stages, with adults having higher probability of survival than juveniles and recruits (Babcock, 1991; Yoshioka and Yoshioka, 1991; De Caralt et al., 2008; Borgstein et al., 2020, among others). Nevertheless, only a few studies have addressed the relationship between survival and size among colonies that can be considered recruits. We found the probability of surviving 1 year ranged between 32% (recruits < 0.6 cm), and 74% (≤5 cm height). This is consistent with the general observation that size mediates the ability of benthic invertebrates to survive competition for space, escape predation, and avoid being smothered by sediment (Jackson, 1977; Hughes and Jackson, 1985; Lasker, 1990). Our result highlights the importance of size to survive the earliest stages of development.
Even though, the relationship between size and survival is common, the relationship between growth rate and size is not as obvious. We found an increase in potential growth rate by 4 mm y–1 with each cm in height. Goffredo and Lasker (2008) also found a positive relationship between size and growth in colonies of A. elisabethae, with colonies following a Von Bertalanffy growth curve (Von Bertalanffy, 1938). In contrast, Yoshioka (1994) did not find growth rate of octocorals to be dependent on size, and similarly, other studies have found intra and inter-specific growth rates of scleractinian recruits to be highly variable and independent of size (Vermeij, 2006; Edmunds, 2017). Monitoring the colonies only once a year (Vermeij, 2006; Edmunds, 2017), and calculating net growth instead of potential (Yoshioka, 1994; Vermeij, 2006), and in the case of scleractinians disparities between measuring growth as surface areas vs. linear extension, may had masked the relationship between growth rate and size. Further assessments of the effects of size on recruit growth should be designed at shorter temporal scales, should differentiate between potential and net growth rates (but see Edmunds, 2017), and use measures of colony size that closely track biomass.
We did not specifically test whether growth rate affected survival, but we found that larger recruits grow faster and survive longer than smaller recruits. In contrast, Edmunds (2017) did not find growth rate to be a strong predictor of survival for small scleractinian coral colonies (≤4 cm wide). Since growth rate affects the time required for a recruit to “escape in size” (Hughes and Jackson, 1980; Babcock, 1991; Meesters et al., 1997), our results, as well as previous studies, indicate processes such as growth rate and survival are not independent, but synergistic, and in the case of octocoral recruits, the synergy is a fundamental component of their life history strategy.
While reaching larger sizes is generally beneficial for survival, larger size in colonial organisms is also associated with a greater risk of partial mortality (Hughes and Jackson, 1980; Hughes and Connell, 1987; Stocker, 1991; Turon et al., 1998; Vermeij, 2006), which also may affect survival. In our study, larger recruits grew faster and survived longer, but they also had a greater risk of negative growth (i.e., partial mortality) than smaller recruits. For instance, 87% of the colonial recruits that survived the first year persisted in the “recruit” size class for more than a year. Partial mortality was presumably caused by grazers, but in most cases, we were not able to identify the source of the damage. Larger recruits were often able to survive partial mortality, as we observed scar tissue on several recruits. Regeneration after injury has been positively correlated to colony size among octocorals, scleractinian corals, and sponges (Connell, 1973; Bak, 1977; Wahle, 1983; Plucer-Rosario and Randall, 1987; Harriott and Fisk, 1988; Bavestrello et al., 1997). Larger colonies can allocate greater amounts of energy to the injured part of the colony, increasing regeneration rates after losing tissue (Oren et al., 2001). Furthermore, regeneration after tissue loss may be fast among octocorals. For example, regeneration rates of 6.0 - 7.8 mm d–1, 6.8-8.6 mm d–1, and even 10.6 mm d–1, have been measured experimentally for large colonies of the octocorals Eunicea mammosa, Plexaura homomalla (Wahle, 1983), and Eunicea flexuosa (da Silveira and Van’t Hof, 1977). However, surviving partial mortality may impact survival by keeping recruits in a vulnerable size class for longer periods of time, and in our study, uncoupled the relationship between recruit age and height.
Our use of 5 cm as the height separating recruits from “juvenile” colonies is a compromise that inevitably includes colonies that are more than a year old and excludes colonies that grow over 5 cm in their first year. We only observed 3 recruits that grew 5 cm in their first year, indicating the 5 cm cut-off did not exclude many 1-year old colonies among the species that we observed recruiting. The combination of slow growth and partial mortality indicates that many colonies that are older than a year will be identified as recruits using the 5 cm upper height limit. Six of the 17 recruits that survived the entire 3-year span of the study never reached 5 cm height. Reducing the upper size limit of recruits to 3 cm would exclude many but not all older colonies as 13% of the colonies exhibited growth rates of ≤3 cm in a year. The magnitude of this effect is undoubtedly site specific as different communities are likely to have different mixes of fast and slow growing species and there is no reason to expect that the incidence of partial mortality will be similar on all reefs. Thus, defining octocoral recruits based on a maximum height yields a recruitment rate that is not an instantaneous measure, but is rather a running average of the abundance of “recruits” that incorporates the processes affecting the initial settlement of individuals and their subsequent survival and growth over several years. Our results are similar to those reported by Edmunds (2017) for small scleractinian coral colonies, where small colonies, that on the basis of size could be characterized as recruits, were instead up to 7 years old. Changes in the abundance of individuals in these “recruit” classes are indicative of changes in the input of colonies to the population. Although not a true measure of a recruitment rate, it is important to differentiate this size class of octocorals due to the different rates of survival and growth associated with these small colonies. True measures of recruitment require methodologies that can identify which individuals are new to a site and surveys that sample at high enough frequency to quantify the effects of each spawning event. For octocorals, which spawn during multiple months throughout the year, and whose single-polyp recruits do not leave a skeleton after dying, this will require monthly sampling of recruitment on tiles, or natural substrata surveys in which all new individuals are either marked or removed (Lasker and Porto-Hannes, 2020).
Evidence for Octocoral Recruitment Success on Contemporary Caribbean Reefs
The environmental stressors that have contributed to the dramatic decline of scleractinian coral populations, also affect octocorals, but unlike scleractinian corals, octocoral communities have proven to be resilient to those stressors, and successfully recruit. Recruitment was high in our study, and we only observed a decrease in recruit densities in the year following hurricanes Irma and Maria. Although methodological discrepancies among studies make comparisons difficult, our results suggest octocoral recruits may be better suited for the current conditions on Caribbean reefs than scleractinian coral recruits. As for scleractinian corals, survival of single-polyps was low, with 91% mortality over 2 months. Nonetheless, survival of colonial recruits ranged between 40 and 74%, whereas Edmunds reported survival rates of 23-50% for juvenile corals on reefs close to our areas of study (2000). Moreover, the potential growth rates we found for octocoral recruits (≤2.1 cm y–1) were markedly higher than those reported by Edmunds (≤8 mm y–1) in 2000, and comparable to the 1.0 to 3.4 cm y–1 growth rates reported for small scleractinian coral colonies in Curaçao and Bonaire in the late 1970s and early 1980s (Bak and Engel, 1979; Van Moorsel, 1985, 1988). Macroalgae such as Lobophora variegata and Dictyota pulchella, which are detrimental to scleractinian coral survival and growth (Box and Mumby, 2007), are dominant components of contemporary coral reefs across the Caribbean. The rapid vertical growth of octocorals may be advantageous to minimize competition against macroalgae or other benthic invertebrates (Hughes and Jackson, 1985; Yoshioka and Yoshioka, 1991; Sanchez, 2004; Box and Mumby, 2007).
Studies of scleractinian corals suggest that environmental stresses are leading to the replacement of previously competitive dominant species by stress-tolerant, fast-growing, weedy, generalist taxa (Darling et al., 2012, 2013). While octocorals exhibit depth and habitat differentiation (Kinzie, 1973; Lasker and Coffroth, 1983), many of the most abundant species at our study sites are found in a variety of environments, indicating this community may be more resistant to environmental change. Moreover, the similar survival of recruits that we found at sites with different environmental conditions, highlights the ability of octocorals to colonize and persist in diverse environments.
Conclusion and Caveats
Our observations and experiments suggest larval supply was the main driver of local densities of octocoral single-polyps and colonial recruits at our study sites. Survival of both single-polyps and colonial recruits did not differ between sites. Among colonial recruits only size was important in predicting their survival, growth rate and partial mortality. As the pattern of larval supply also matches adult abundance at the two sites, it suggests the populations are recruitment limited.
The detailed characterization of the fates of single polyp and colonial recruits highlights the complexity of assessing recruitment when sampling intervals are longer than the time required for a single polyp to develop into a colony. Such sampling schemes require the inclusion of colonial recruits, but differences in growth rates as colonies grow, coupled with partial mortality results in a “recruit” class that mixes colonies of different ages. The recruit class, defined in this manner, may be more analogous to a seed or seedling bank where there are individuals of varying ages all capable of growing into a larger size class if given the opportunity.
How future environmental change due to global warming will impact octocoral assemblages is poorly understood. Predicting those effects requires a clearer understanding of any advantages that octocoral recruits may have relative to other taxa. Further studies that identify the interactions between octocoral recruits and other benthic invertebrates should include how octocoral morphology and/or vertical growth influences the outcome of those interactions. Understanding the resilience of octocoral communities to environmental change also requires the study of processes affecting adults as well as those affecting recruitment. For instance, large scale mortality of adult octocorals could jeopardize larval supply. Further research that links the different life-history stages (e.g., recruits vs. juveniles vs. adults) is needed to identify the drivers of population growth of octocorals. Demographic approaches such as matrix models and integral projection models coupled with biophysical models of connectivity offer the potential to address these issues. Moreover, to understand shifts of community assemblages on Caribbean reefs, future research needs to identify the drivers of population growth not only on octocorals and scleractinian corals but also on other dominant taxa (i.e., sponges and macroalgae).
Data Availability Statement
The raw data supporting the conclusions of this article will be made available by the authors, without undue reservation.
Author Contributions
ÁM-Q collected the data and analyzed the results. Both authors conceptualized and conducted the study, and wrote and approved the submitted version of the manuscript.
Funding
This research has been funded by the National Science Foundation grants OCE1756381 and OCE1801475, and by funding awarded to ÁM-Q by the Mark Diamond Research Fund (Graduate Student Association at the University at Buffalo).
Conflict of Interest
The authors declare that the research was conducted in the absence of any commercial or financial relationships that could be construed as a potential conflict of interest.
Publisher’s Note
All claims expressed in this article are solely those of the authors and do not necessarily represent those of their affiliated organizations, or those of the publisher, the editors and the reviewers. Any product that may be evaluated in this article, or claim that may be made by its manufacturer, is not guaranteed or endorsed by the publisher.
Acknowledgments
We thank the staff of the University of the Virgin Islands, Virgin Islands Environmental Resource Station, logistical support in the field and Aran Mooney (Woods Hole Oceanographic Institute) for the use of Scalywag. We also thank the many students and volunteers from the University at Buffalo, who contributed to manufacturing the settlement tiles. Special thanks to Jacqueline Krawiecki, Christopher Wells and Kaitlyn Tonra for providing help and support during the field work and the data collection. Thanks to Peter Edmunds (California State University Northridge) not only for the logistics in the field, but also for providing insightful and helpful comments throughout the project and for reviewing the last versions of the manuscript. In addition, we also thank Mary Alice Coffroth, Adam Wilson (University at Buffalo), and Lorenzo Bramanti (CNRS-LECOB Observatoire Oceanologique Banyuls sur Mer) for their comments and reviews on the later stages of the manuscript.
Supplementary Material
The Supplementary Material for this article can be found online at: https://www.frontiersin.org/articles/10.3389/fmars.2021.705563/full#supplementary-material
References
Adam, T. C., Burkepile, D. E., Ruttenberg, B. I., and Paddack, M. J. (2015). Herbivory and the resilience of Caribbean coral reefs: knowledge gaps and implications for management. Mar. Ecol. Prog. Ser. 520, 1–20. doi: 10.3354/meps11170
Adjeroud, M., Kayal, M., and Penin, L. (2017). “Importance of recruitment processes in the dynamics and resilience of coral reef assemblages,” in Marine Animal Forests, eds S. Rossi, L. Bramanti, A. Gori, and C. Orejas (Berlin: Springer). doi: 10.1007/978-3-319-21012-4_12
Arnold, S. N., and Steneck, R. S. (2011). Settling into an Increasingly Hostile World: the Rapidly Closing “Recruitment Window” for Corals. PLoS One 6:e28681. doi: 10.1371/journal.pone.0028681
Babcock, R., and Mundy, C. (1996). Coral recruitment: consequences of settlement choice for early growth and survivorship in two scleractinians. J. Exp. Mar. Biol. Ecol. 206, 179–201. doi: 10.1016/s0022-0981(96)02622-6
Babcock, R. C. (1991). Comparative demography of three species of scleractinian corals using age-and size-dependent classifications. Ecol. Monogr. 61, 225–244. doi: 10.2307/2937107
Bak, R. P. M. (1977). Coral Reefs and Their Zonation in Netherlands Antilles. Am. Assoc. Pet. Geol. Stud. Geol. 45, 3–16. doi: 10.1306/st4393c1
Bak, R. P. M., and Engel, M. S. (1979). Distribution, abundance and survival of juvenile hermatypic corals (Scleractinia) and the importance of life history strategies in the parent coral community. Mar. Biol. 54, 341–352. doi: 10.1007/bf00395440
Bartlett, L. A., Brinkhuis, V. I. P., Ruzicka, R. R., Colella, M. A., Lunz, K. S., Leone, E. H., et al. (2017). “Dynamics of stony coral and octocoral juvenile assemblages following disturbance on patch reefs of the Florida reef tract,” in Corals in a changing world, eds C. Duque and E. D. Camacho (London, UK: Intech Open), 99–120.
Bates, D., Mächler, M., Bolker, B., and Walker, S. (2015). Fitting Linear Mixed-Effects Models Using lme4. J. Stat. Softw. 67, 1–48.
Bavestrello, G., Cerrano, C., Zanzi, D., and Cattaneo-Viettri, R. (1997). Damage by fishing activities to the Gorgonian coral Paramuricea clavata in the Ligurian Sea. Aquat. Conserv. 7, 253–262. doi: 10.1002/(sici)1099-0755(199709)7:3<253::aid-aqc243>3.0.co;2-1
Bayer, F. M. (1961). The shallow-water Octocorallia of the West Indian region. Studies on the Fauna of Curaçao and other Caribbean islands. The Hague: Martinus Nijhoff. 1–373.
Bohn, K., Richardson, C. A., and Jenkins, S. R. (2013). Larval microhabitat associations of the non-native gastropod Crepidula fornicata and effects on recruitment success in the intertidal zone. J. Exp. Mar. Biol. Ecol. 448, 289–297. doi: 10.1016/j.jembe.2013.07.020
Borgstein, N., Beltrán, D. M., and Prada, C. (2020). Variable growth across species and life stages in Caribbean reef octocorals. Front. Mar. Sci. 7:483. doi: 10.3389/fmars.2020.00483
Box, S. J., and Mumby, P. J. (2007). Effect of macroalgal competition on growth and survival of juvenile Caribbean corals. Mar. Ecol. Prog. Ser. 342, 139–149. doi: 10.3354/meps342139
Brazeau, D. A., and Lasker, H. R. (1989). The reproductive cycle and spawning in a Caribbean gorgonian. Biol. Bull. 176, 1–7. doi: 10.2307/1541882
Brazeau, D. A., and Lasker, H. R. (1990). Sexual reproduction and external brooding by the Caribbean gorgonian Briareum asbestinum. Mar. Biol. 104, 465–474. doi: 10.1007/bf01314351
Bruno, J. F., Sweatman, H., Precht, W. F., Selig, E. R., and Schutte, V. G. W. (2009). Assessing evidence of phase shifts from coral to macroalgal dominance on coral reefs. Ecology 90, 1478–1484. doi: 10.1890/08-1781.1
Burt, J., Bartholomew, A., Bauman, A., Saif, A., and Sale, P. F. (2009). Coral recruitment and early benthic community development on several materials used in the construction of artificial reefs and breakwaters. J. Exp. Mar. Biol. Ecol. 373, 72–78. doi: 10.1016/j.jembe.2009.03.009
Caley, M. J., Carr, M. H., Hixon, M. A., Hughes, T. P., Jones, G. P., and Menge, B. A. (1996). Recruitment and the local dynamics of open marine populations. Annu. Rev. Ecol. Syst. 2, 477–500. doi: 10.1146/annurev.ecolsys.27.1.477
Coelho, M. A. (2018). Life history traits of Caribbean octocorals of the genus Antillogorgia and their implications for larval dispersal. Ph.D. thesis. Buffalo: State University of New York.
Coma, R., Ribes, M., Zabala, M., and Gili, J. M. (1995). Reproduction and cycle of gonadal development in the Mediterranean gorgonian Paramuricea clavata. Mar. Ecol. Prog. Ser. 117, 173–183. doi: 10.3354/meps117173
Connell, J. H. (1973). Population ecology of reef-building corals. Biol. Geol. Coral Reefs 2, 205–245. doi: 10.1016/b978-0-12-395526-5.50015-8
Connell, J. H. (1985). The consequences of variation in initial settlement vs. post-settlement mortality in rocky intertidal communities. J. Exp. Mar. Biol. Ecol. 93, 11–45. doi: 10.1016/0022-0981(85)90146-7
Cowen, R. K., and Sponaugle, S. (2009). Larval dispersal and marine population connectivity. Annu. Rev. Mar. Sci. 1, 443–466. doi: 10.1146/annurev.marine.010908.163757
Crabbe, M. J., Martinez, E., Garcia, C., Chub, J., Castro, L., and Guy, J. (2008). Growth modelling indicates hurricanes and severe storms are linked to low coral recruitment in the Caribbean. Mar. Environ. Res. 65, 364–368. doi: 10.1016/j.marenvres.2007.11.006
da Silveira, F. L., and Van’t Hof, T. (1977). Regeneration in the gorgonian Plexura flexuosa (Cnidaria, Octocorallia). Bijdragen tot de Dierkunde 47, 98–108. doi: 10.1163/26660644-04701002
Darling, E. S., Alvarez-Filip, L., Oliver, T. A., and McClanahan, T. R. (2012). Evaluating life-history strategies of reef corals from species traits. Ecol. Lett. 15, 1378–1386. doi: 10.1111/j.1461-0248.2012.01861.x
Darling, E. S., McClanahan, T. R., and Côté, I. M. (2013). Life histories predict coral community disassembly under multiple stressors. Glob. Chang. Biol. 19, 1930–1940. doi: 10.1111/gcb.12191
De Caralt, S., Uriz, M. J., and Wijffels, R. H. (2008). Grazing, differential size-class dynamics and survival of the Mediterranean sponge Corticium candelabrum. Mar. Ecol. Prog. Ser. 360, 97–106. doi: 10.3354/meps07365
Deevey, E. S. (1947). Life tables for natural populations of animals. Q. Rev. Biol. 22, 283–314. doi: 10.1086/395888
Doropoulos, C., Roff, G., Bozec, Y.-M., Zupan, M., Werminghausen, J., and Mumby, P. J. (2016). Characterizing the ecological trade-offs throughout the early ontogeny of coral recruitment. Ecol. Monogr. 86, 20–44. doi: 10.1890/15-0668.1
Edmunds, P. (2013). Decadal-scale changes in the community structure of coral reefs of St. John, US Virgin Islands. Mar. Ecol. Prog. Ser. 489, 107–123. doi: 10.3354/meps10424
Edmunds, P., and Lasker, H. (2016). Cryptic regime shift in benthic community structure on shallow reefs in St. John, US Virgin Islands. Mar. Ecol. Prog. Ser. 559, 1–12. doi: 10.3354/meps11900
Edmunds, P., and Lasker, H. (2019). Regulation of population size of arborescent octocorals on shallow Caribbean reefs. Mar. Ecol. Prog. Ser. 615, 1–14. doi: 10.3354/meps12907
Edmunds, P. J. (2017). Intraspecific variation in growth rate is a poor predictor of fitness for reef corals. Ecology 98, 2191–2200. doi: 10.1002/ecy.1912
Evans, M. J., Coffroth, M. A., and Lasker, H. R. (2013). Effects of predator exclusion on recruit survivorship in an octocoral (Briareum asbestinum) and a scleractinian coral (Porites astreoides). Coral Reefs 32, 597–601. doi: 10.1007/s00338-012-1001-1
Gaines, S., and Roughgarden, J. (1985). Larval settlement rate: a leading determinant of structure in an ecological community of the marine intertidal zone. Proc. Natl. Acad. Sci. U. S. A. 82, 3707–3711. doi: 10.1073/pnas.82.11.3707
Gleason, D. F., Harbin, L. R., Divine, L. M., and Matterson, K. O. (2018). The role of larval supply and competition in controlling recruitment of the temperate coral Oculina arbuscula. J. Exp. Mar. Biol. Ecol. 506, 107–114. doi: 10.1016/j.jembe.2018.06.006
Goffredo, S., and Lasker, H. R. (2008). An adaptive management approach to an octocoral fishery based on the Beverton-Holt model. Coral Reefs 27, 751–761. doi: 10.1007/s00338-008-0391-6
Graham, E. M., Baird, A. H., Connolly, S. R., Sewell, M. A., and Willis, B. L. (2013). Rapid declines in metabolism explain extended coral larval longevity. Coral Reefs 32, 539–549. doi: 10.1007/s00338-012-0999-4
Green, D., Edmunds, P., and Carpenter, R. (2008). Increasing relative abundance of Porites astreoides on Caribbean reefs mediated by an overall decline in coral cover. Mar. Ecol. Prog. Ser. 359, 1–10. doi: 10.3354/meps07454
Grigg, R. (1988). Recruitment limitation of a deep benthic hard-bottom octocoral population in the Hawaiian Islands. Mar. Ecol. Prog. Ser. 45, 121–126. doi: 10.3354/meps045121
Grorud-Colvert, K., and Sponaugle, S. (2009). Larval supply and juvenile recruitment of coral reef fishes to marine reserves and non-reserves of the upper Florida Keys. USA. Mar. Biol. 156, 277–288. doi: 10.1007/s00227-008-1082-0
Grosberg, R. K., and Levitan, D. R. (1992). For adults only? Supply-side ecology and the history of larval biology. Trends Ecol. Evol. 7, 130–133. doi: 10.1016/0169-5347(92)90148-5
Hadfield, M., and Paul, V. (2001). “Natural chemical cues for settlement and metamorphosis of marine-invertebrate larvae,” in Marine Chemical Ecology, eds J. McClintock and B. Baker (Boca Raton, Florida, USA: CRC Press), 431–461. doi: 10.1201/9781420036602.ch13
Hadfield, M. G. (2011). Biofilms and marine invertebrate larvae: what bacteria produce that larvae use to choose settlement sites. Annu. Rev. Mar. Sci. 3, 453–470. doi: 10.1146/annurev-marine-120709-142753
Harper, L. M. (2017). Variation In Coral Recruitment And Juvenile Distribution Along The Southeast Florida Reef Tract. Ph.D. thesis. United States: NOVA Southeastern University.
Harriott, V. J., and Fisk, D. A. (1988). Coral transplantation as a reef management option. Proc. 6th Int. Coral Reef Symp. 2, 375–379.
Hughes, T. P. (1994). Catastrophes, phase shifts, and large-scale degradation of a Caribbean coral reef. Science 265, 1547–1551. doi: 10.1126/science.265.5178.1547
Hughes, T. P., Anderson, K. D., Connolly, S. R., Heron, S. F., Kerry, J. T., Lough, J. M., et al. (2018). Spatial and temporal patterns of mass bleaching of corals in the Anthropocene. Science 359, 80–83. doi: 10.1126/science.aan8048
Hughes, T. P., Baird, A. H., Dinsdale, E. A., Moltschaniwskyj, N. A., Pratchett, M. S., Tanner, J. E., et al. (2000). Supply-side ecology works both ways: the link between benthic adults, fecundity, and larval recruits. Ecology 81, 2241–2249. doi: 10.1890/0012-9658(2000)081[2241:ssewbw]2.0.co;2
Hughes, T. P., and Connell, J. H. (1987). Population dynamics based on size or age? a reef-coral analysis. Am. Nat. 129, 818–829. doi: 10.1086/284677
Hughes, T. P., and Jackson, J. B. C. (1980). Do corals lie about their age? some demographic consequences of partial mortality, fission, and fusion. Science 209, 713–715. doi: 10.1126/science.209.4457.713
Hughes, T. P., and Jackson, J. B. C. (1985). Population dynamics and life histories of foliaceous corals. Ecol. Monogr. 55, 142–166.
Hughes, T. P., and Tanner, J. E. (2000). Recruitment failure, life histories, and long-term decline of Caribbean corals. Ecology 81, 2250–2263. doi: 10.1890/0012-9658(2000)081[2250:rflhal]2.0.co;2
Hunt, H., and Scheibling, R. (1997). Role of early post-settlement mortality in recruitment of benthic marine invertebrates. Mar. Ecol. Prog. Ser. 155, 269–301. doi: 10.3354/meps155269
Hurlbut, C. (1992). Larval release and supply predict temporal variation in settlement of a colonial ascidian. Mar. Ecol. Prog. Ser. 80, 215–219. doi: 10.3354/meps080215
Jackson, E. J., Donovan, M., Cramer, K., and Lam, V. (2014). Status and trends of Caribbean coral reefs: 1970-2012. Global Coral Reef Monitoring Network. International union for the conservation of nature global marine and polar program. Gland, Switzerland: IUCN
Jackson, J. B. C. (1977). Competition on Marine Hard Substrata: the Adaptive Significance of Solitary and Colonial Strategies. Am. Nat. 111, 743–767. doi: 10.1086/283203
Jenkins, S. R. (2005). Larval habitat selection, not larval supply, determines settlement patterns and adult distribution in two chthamalid barnacles. J. Anim. Ecol. 74, 893–904. doi: 10.1111/j.1365-2656.2005.00985.x
Jenkins, S. R., Marshall, D., and Fraschetti, S. (2009). “Settlement and recruitment,” in Marine Hard Bottom Communities: Patterns, Dynamics, Diversity, And Change, ed. M. Wahl (Berlin, Heidelberg: Springer), 177–190.
Jonsson, P. R., Berntsson, K. M., and Larsson, A. I. (2004). Linking larval supply to recruitment: flow-mediated control of initial adhesion of barnacle larvae. Ecology 85, 2850–2859. doi: 10.1890/03-0565
Kahng, S., Benayahu, Y., and Lasker, H. (2011). Sexual reproduction in octocorals. Mar. Ecol. Prog. Ser. 443, 265–283. doi: 10.3354/meps09414
Kegler, P., Kegler, H. F., Gärdes, A., Ferse, S. C. A., Lukman, M., Alfiansah, Y. R., et al. (2017). Bacterial biofilm communities and coral larvae settlement at different levels of anthropogenic impact in the Spermonde archipelago, Indonesia. Front. Mar. Sci. 4:270. doi: 10.3389/fmars.2017.00270
Keough, M. J., and Downes, B. J. (1982). Recruitment of marine invertebrates: the role of active larval choices and early mortality. Oecologia 54, 348–352. doi: 10.1007/bf00380003
Kinzie, A. (1973). The zonation of West Indian gorgonians. Bull. Mar. Sci. 23, 93–155. doi: 10.7228/manchester/9780719064746.003.0005
Lasker, H., Kim, K., and Coffroth, M. (1998). Production, settlement, and survival of plexaurid gorgonian recruits. Mar. Ecol. Prog. Ser. 162, 111–123. doi: 10.3354/meps162111
Lasker, H. R. (1990). Clonal propagation and population dynamics of a gorgonian coral. Ecology 71, 1578–1589. doi: 10.2307/1938293
Lasker, H. R., and Coffroth, M. A. (1983). Octocoral Distributions at Carrie Bow Cay, Belize. Mar. Ecol. Progr. Ser. 13, 21–28. doi: 10.3354/meps013021
Lasker, H. R., Martínez-Quintana, Á, Bramanti, L., and Edmunds, P. J. (2020). Resilience of octocoral forests to catastrophic storms. Sci. Rep. 10:4286.
Lasker, H. R., and Porto-Hannes, I. (2020). Species level identification of Antillogorgia spp. recruits identifies multiple pathways of octocoral success on Caribbean reefs. Coral Reefs 40, 41–51. doi: 10.1007/s00338-020-02014-5
Lenth, R., Buerkner, P., Herve, M., Love, J., Riebl, H., and Singmann, H. (2018). emmeans: Estimated Marginal Means, aka Least-Squares Means. R package version 1.1.
Lenz, E. A., Bramanti, L., Lasker, H. R., and Edmunds, P. J. (2015). Long-term variation of octocoral populations in St. John, US Virgin Islands. Coral Reefs 34, 1099–1109. doi: 10.1007/s00338-015-1315-x
Linares, C., Coma, R., Mariani, S., Díaz, D., Hereu, B., and Zabala, M. (2008). Early life history of the Mediterranean gorgonian Paramuricea clavata: implications for population dynamics. Invertebrate Biol. 127, 1–11. doi: 10.1111/j.1744-7410.2007.00109.x
Meesters, E. H., Wesseling, I., and Bak, R. P. M. (1997). Coral colony tissue damage in six species of reef-building corals: partial mortality in relation with depth and surface area. J. Sea Res. 37, 131–144. doi: 10.1016/s1385-1101(96)00004-4
Menge, B. A. (2000). Recruitment vs. postrecruitment processes as determinants of barnacle population abundance. Ecol. Monogr. 70, 265–288. doi: 10.1890/0012-9615(2000)070[0265:rvppad]2.0.co;2
Mullineaux, L. S., and Garland, E. D. (1993). Larval recruitment in response to manipulated field flows. Mar. Biol. 116, 667–683. doi: 10.1007/bf00355484
Mumby, P. J., Hastings, A., and Edwards, H. J. (2007). Thresholds and the resilience of Caribbean coral reefs. Nature 450, 98–101. doi: 10.1038/nature06252
Nishikawa, A., Katoh, M., and Sakai, K. (2003). Larval settlement rates and gene flow of broadcast-spawning (Acropora tenuis) and planula-brooding (Stylophora pistillata) corals. Mar. Ecol. Prog. Ser. 256, 87–97. doi: 10.3354/meps256087
Norström, A., Nyström, M., Lokrantz, J., and Folke, C. (2009). Alternative states on coral reefs: beyond coral–macroalgal phase shifts. Mar. Ecol. Prog. Ser. 376, 295–306. doi: 10.3354/meps07815
Nozawa, Y., Tanaka, K., and Reimer, J. D. (2011). Reconsideration of the surface structure of settlement plates used in coral recruitment studies. Zool. Stud. 50, 53–60.
Oren, U., Benayahu, Y., Lubinevsky, H., and Loya, Y. (2001). Colony integration during regeneration in the stony coral Favia favus. Ecology 82, 802–813. doi: 10.2307/2680199
Pineda, J., Porri, F., Starczak, V., and Blythe, J. (2010). Causes of decoupling between larval supply and settlement and consequences for understanding recruitment and population connectivity. J. Exp. Mar. Biol. Ecol. 392, 9–21. doi: 10.1016/j.jembe.2010.04.008
Pineda, J., Reyns, N. B., and Starczak, V. R. (2009). Complexity and simplification in understanding recruitment in benthic populations. Popul. Ecol. 51, 17–32. doi: 10.1007/s10144-008-0118-0
Plucer-Rosario, G., and Randall, R. H. (1987). Preservation of rare coral species by transplantation and examination of their recruitment and growth. Bull. Mar. Sci. 41, 585–593.
Prada, C., Weil, E., and Yoshioka, P. M. (2010). Octocoral bleaching during unusual thermal stress. Coral Reefs 29, 41–45. doi: 10.1007/s00338-009-0547-z
Privitera-Johnson, K., Lenz, E. A., and Edmunds, P. J. (2015). Density-associated recruitment in octocoral communities in St. John, US Virgin Islands. J. Exp. Mar. Biol. Ecol. 473, 103–109. doi: 10.1016/j.jembe.2015.08.006
Raimondi, P. T. (1990). Patterns, mechanisms, consequences of variability in settlement and recruitment of an intertidal barnacle. Ecol. Monogr. 60, 283–309. doi: 10.2307/1943059
Raimondi, P. T., and Morse, A. N. C. (2000). The consequences of complex larval behavior in a coral. Ecology 81, 3193–3211. doi: 10.1890/0012-9658(2000)081[3193:tcoclb]2.0.co;2
Riding, R. (2002). Structure and composition of organic reefs and carbonate mud mounds: concepts and categories. Earth Sci. Rev. 58, 163–231. doi: 10.1016/s0012-8252(01)00089-7
Ritson-Williams, R., Arnold, S., Fogarty, N., Steneck, R. S., Vermeij, M., and Paul, V. J. (2009). New perspectives on ecological mechanisms affecting coral recruitment on reefs. Smithson. Contrib. Mar. Sci. 38, 437–457. doi: 10.5479/si.01960768.38.437
Roff, G., and Mumby, P. J. (2012). Global disparity in the resilience of coral reefs. Trends Ecol. Evol. 27, 404–413. doi: 10.1016/j.tree.2012.04.007
Rogers, C. S., Miller, J., Muller, E. M., Edmunds, P., Nemeth, R. S., Beets, J. P., et al. (2008). “Ecology of coral reefs in the US Virgin Islands,” in Coral reefs of the USA, eds A. B. Riegl and R. E. Dodge (Dordrecht, the Netherlands.: Springer), 303–337.
Roughgarden, J., Gaines, S., and Possingham, H. (1988). Recruitment dynamics in complex life cycles. Science 241, 1460–1466. doi: 10.1126/science.11538249
Roughgarden, J., Iwasa, Y., and Baxter, C. (1985). Demographic Theory for an Open Marine Population with Space-Limited Recruitment. Ecology 66, 54–67. doi: 10.2307/1941306
Ruzicka, R., Colella, M., Porter, J., Morrison, J., Kidney, J., Brinkhuis, V., et al. (2013). Temporal changes in benthic assemblages on Florida Keys reefs 11 years after the 1997/1998 El Niño. Mar. Ecol. Prog. Ser. 489, 125–141.
Salta, M., Wharton, J. A., Blache, Y., Stokes, K. R., and Briand, J.-F. (2013). Marine biofilms on artificial surfaces: structure and dynamics. Environ. Microbiol. 15, 2879–2893.
Sanchez, J. A. (2004). Evolution and dynamics of branching colonial form in marine modular cnidarians: gorgonian octocorals. Hydrobiologia 530, 283–290. doi: 10.1007/978-1-4020-2762-8_33
Searle, S. R., Speed, F. M., and Milliken, G. A. (1980). Population marginal means in the linear model: an alternative to least squares means. Am. Stat. 34, 216–221. doi: 10.2307/2684063
Shikuma, N. J., and Hadfield, M. G. (2005). Temporal variation of an initial marine biofilm community and its effects on larval settlement and metamorphosis of the tubeworm Hydroides elegans. Biofilms 2, 231–238. doi: 10.1017/s1479050506002018
Stocker, L. J. (1991). Effects of size and shape of colony on rates of fission, fusion, growth and mortality in a subtidal invertebrate. J. Exp. Mar. Biol. Ecol. 149, 161–175. doi: 10.1016/0022-0981(91)90043-v
Trapon, M., Pratchett, M., Adjeroud, M., Hoey, A., and Baird, A. (2013). Post-settlement growth and mortality rates of juvenile scleractinian corals in Moorea, French Polynesia versus Trunk Reef, Australia. Mar. Ecol. Prog. Ser. 488, 157–170. doi: 10.3354/meps10389
Tsounis, G., and Edmunds, P. J. (2017). Three decades of coral reef community dynamics in St. John, USVI: a contrast of scleractinians and octocorals. Ecosphere 8:e01646.
Tsounis, G., Edmunds, P. J., Bramanti, L., Gambrel, B., and Lasker, H. R. (2018). Variability of size structure and species composition in Caribbean octocoral communities under contrasting environmental conditions. Mar. Biol. 165:29.
Turon, X., Tarjuelo, I., and Uriz, M. J. (1998). Growth dynamics and mortality of the encrusting sponge Crambe crambe (Poecilosclerida) in contrasting habitats: correlation with population structure and investment in defense. Funct. Ecol. 12, 631–639. doi: 10.1046/j.1365-2435.1998.00225.x
Van Moorsel, G. W. N. M. (1985). Disturbance and growth of juvenile corals (Agaricia humilis and Agaricia agaricites, Scleractinia) in natural habitats on the reef of Curacao. Mar. Ecol. Prog. Ser. 24, 99–112. doi: 10.3354/meps024099
Van Moorsel, G. W. N. M. (1988). Early maximum growth of stony corals (Scleractinia) after settlement on artificial substrata on a Caribbean reef. Mar. Ecol. Prog. Ser. 50, 127–135. doi: 10.3354/meps050127
Venables, W. N., and Ripley, B. D. (2002). “Random and mixed effects,” in Modern applied statistics with S: statistics and computing, eds J. Chambers, W. Eddy, W. Hardle, S. Sheather, and L. Tierney (New York, USA: Springer-Verlag), 271–299.
Vermeij, M. J. A. (2006). Early life-history dynamics of Caribbean coral species on artificial substratum: the importance of competition, growth and variation in life-history strategy. Coral Reefs 25, 59–71. doi: 10.1007/s00338-005-0056-7
Vermeij, M. J. A., and Sandin, S. A. (2008). density-dependent settlement and mortality structure the earliest life phases of a coral population. Ecology 89, 1994–2004. doi: 10.1890/07-1296.1
Von Bertalanffy, L. (1938). A quantitative theory of organic growth (inquiries on growth laws. II). Hum. Biol. 10, 181–213.
Wahle, C. M. (1983). Regeneration of injuries among Jamaican gorgonians: the roles of colony physiology and environment. Biol. Bull. 165, 778–790. doi: 10.2307/1541478
Wells, C. D., Martínez-Quintana, Á, Tonra, K. J., and Lasker, H. R. (2021). Algal turf negatively affects recruitment of a Caribbean octocoral. Coral Reefs 27, 1–9.
Williams, S. M., Chollett, I., Roff, G., Cortés, J., Dryden, C. S., and Mumby, P. J. (2015). Hierarchical spatial patterns in Caribbean reef benthic assemblages. J. Biogeogr. 42, 1327–1335. doi: 10.1111/jbi.12509
Winston, J. E. (2010). Life in the colonies: learning the alien ways of colonial organisms. Integr. Comp. Biol. 50, 919–933. doi: 10.1093/icb/icq146
Yoshioka, P., and Yoshioka, B. (1991). A comparison of the survivorship and growth of shallow-water gorgonian species of Puerto Rico. Mar. Ecol. Prog. Ser. 69, 253–260. doi: 10.3354/meps069253
Yoshioka, P. M. (1994). Size-specific life history pattern of a shallow-water gorgonian. J. Exp. Mar. Biol. Ecol. 184, 111–122. doi: 10.1016/0022-0981(94)90169-4
Yoshioka, P. M. (1996). Variable recruitment and its effects on the population and community structure of shallow-water gorgonians. Bull. Mar. Sci. 59, 433–443.
Yoshioka, P. M. (1998). Are large colonies a “key factor” in the dynamics of gorgonian populations? Rev. Biol. Trop. 98, 137–143.
Keywords: recruitment, larval supply, colonial, settlement, post-settlement survival, octocoral, resilience, partial mortality
Citation: Martínez-Quintana Á and Lasker HR (2021) Early Life-History Dynamics of Caribbean Octocorals: The Critical Role of Larval Supply and Partial Mortality. Front. Mar. Sci. 8:705563. doi: 10.3389/fmars.2021.705563
Received: 05 May 2021; Accepted: 18 August 2021;
Published: 07 September 2021.
Edited by:
Yehuda Benayahu, Tel Aviv University, IsraelReviewed by:
Francoise Cavada-Blanco, EDGE of Existence Programme, Conservation and Policy, Zoological Society of London, United KingdomRafael A. Cabral-Tena, Ensenada Center for Scientific Research and Higher Education, Mexico
Copyright © 2021 Martínez-Quintana and Lasker. This is an open-access article distributed under the terms of the Creative Commons Attribution License (CC BY). The use, distribution or reproduction in other forums is permitted, provided the original author(s) and the copyright owner(s) are credited and that the original publication in this journal is cited, in accordance with accepted academic practice. No use, distribution or reproduction is permitted which does not comply with these terms.
*Correspondence: Ángela Martínez-Quintana, YW0yOThAYnVmZmFsby5lZHU=