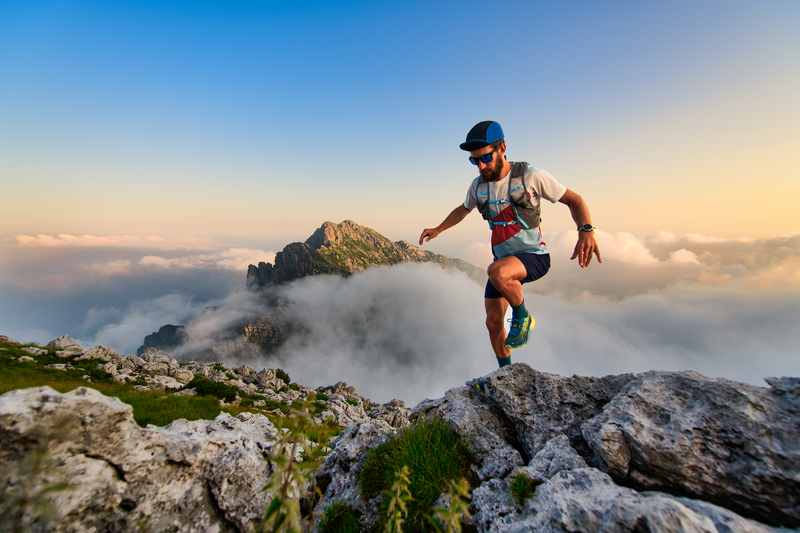
95% of researchers rate our articles as excellent or good
Learn more about the work of our research integrity team to safeguard the quality of each article we publish.
Find out more
ORIGINAL RESEARCH article
Front. Mar. Sci. , 27 July 2021
Sec. Aquatic Physiology
Volume 8 - 2021 | https://doi.org/10.3389/fmars.2021.704907
This article is part of the Research Topic Homeostasis and Physiological Regulation in the Aquatic Animal during Osmotic Stress View all 7 articles
This study compares salinity tolerance between red and black shell Pinctada fucata salinity stress of 20 and 50‰, while 35‰ was used as a control. The hemolymph osmotic pressure, inorganic ion concentration, the activities of Na+-K+ -ATPase, respiratory metabolism related enzymes and liver tissue antioxidant related enzymes were measured at 12 and 24 h after salinity stress. The osmotic pressure and inorganic ion concentration of hemolymph of two strains P. fucata increased significantly with the increase of salinity. The activity of Na+-K+ -ATPase of red P. fucata only decreased under low salinity at 24 h, and was significantly higher than that the control under low salinity at 12 h and high salinity at 12 and 24 h. The succinate dehydrogenase (SDH) activities of the P. fucata treatment groups were significantly higher than those the control at 12 h. The lactate dehydrogenase (LDH) activity increased significantly with salinity at 12 h. and the black P. fucata LDH activity was significantly higher than the control at 24 h, while the LDH activity of red P. fucata was significantly lower than that the control in 50‰ salinity. The superoxide dismutase (SOD) activity of black P. fucata was significantly lower than that the control, while that of red P. fucata was significantly higher than that the control within 24. At 12 h, the catalase (CAT) activity of red P. fucata increased significantly with salinity, but decreased significantly with salinity at 24 h. The CAT activity of black P. fucata was highest at 24 h under low salinity. Glutathione peroxidase (GSH-Px) and alkaline phosphatase (AKP) activities of red P. fucata were significantly higher than those the control under low or high salinity. At high salinity for 24 h, the GSH-Px activity was lowest in black P. fucata, AKP activity was highest. The present study indicates that the physical responses of P. fucata to the salinity stress vary with shell colors. The red P. fucata can quickly respond positively to the change of environmental salinity and reduce the damage caused by the change of environmental salinity.
The Pinctada fucata is an important marine pearl producing molluscan, distributes in tropical and subtropical areas in the coast or offshore seabed (Meng et al., 1996). Since 1949, P. fucata has been cultured in Hainan, Guangdong and Guangxi in China (Ai et al., 2003), and the pearl production peaked in the 1990s, which has brought substantial economic income to the pearl industry (Yang et al., 2017; Yang et al., 2017). In recent years, massive mortality frequently occurs in P. fucata, and has brought considerable economic losses. Evidence has suggested that such massive mortality is often associated with environmental changes (Zhang et al., 2015; Chen et al., 2016). Studies have shown that under the influence of salinity, temperature, pH and dissolved oxygen, physiological indicators, such as osmotic pressure, cellular immunity level, oxygen consumption and ammonia excretion, can be altered, resulting in physiological metabolism imbalance, growth inhibition and even death (Ghiselli et al., 2000; Nan et al., 2004; Liu and Yan, 2006; Ocaño-Higuera et al., 2011).
As one of the vital ecological factors in the marine ecosystem, salinity affects the metabolism, osmotic adjustment and biological rhythm of marine organisms and plays a decisive role in their distribution (Navarro, 1988; Kim et al., 1998). Due to the influence of seasonal rainfall, evaporation and tide, the salinity of seawater usually changes over season. The salinity level of the external water environment directly affects the osmotic pressure of aquatic animals and then regulates their survival, growth and reproduction (Cheng et al., 2002; Garçon et al., 2009; Jasmani et al., 2010). Studies have shown that when the osmotic pressure of aquatic animals’ body fluid is equal to the osmotic pressure of the ambient environment, the adjustment of osmotic pressure consumes the least amount of energy, and the energy conversion efficiency is the highest, thus achieving maximum growth (Chen and Lin, 1995; Masui et al., 2009). Alagarswami and Victor (1976) found that the filtration rate of P. fucata decreased with decreasing salinity. Widdows (1985) found that when salinity was < 20‰, the feeding activity and growth rate of Mytilus edulis decreased. Inorganic ions are the main osmotic factors in the hemolymph of marine shellfish, and K+, Cl–, and Na+ ions are mainly involved in osmotic regulation. In M. edulis and Littorina littorea, Natochin et al. (1979) found that besides the Na+ pump and K+ pump, both species have a Cl– related sodium ion exchange system, which could regulate cell volume and osmotic pressure.
Low-salt or high-salt stress on marine shellfish can reduce shellfish activities and changes physiological status. The hemolymph volume of Haliotis rubra and H. laevigata can increase by 25% in a short time of salinity decrease, while with increasing salinity, their hemolymph volume decreased, and the adhesive force, movement of gill cilia, and heart beat were affected (Edwards, 2003). The metabolic activities of Haliotis cracherodii and Haliotis rufescens under high-salinity stress were swiftly and significantly impacted within 10 min and began to resume in 6–8 h (Berger and Kharazova, 1997). At low salinity, mitochondria in gills of Crassostrea virginica showed a higher ratio of glutamic acid oxidation (Ballantyne and Moyes, 1987). The expression level of α -amylase in P. fucata at salinity 27‰ was significantly higher than that under low and high salt stress (Huang et al., 2016). In American oysters, C. virginica, rapid changes in salinity affected the rate of hemocytes locomotion and extended the spreading time of hemocytes (Fisher and Newell, 1986). Although salinity significantly affects various physiological functions of marine shellfish, physiological responses to salinity stress varied between species.
Shellfish shell color can be inherited, and there are significant differences in growth, survival and nutritional performance of individuals with different shell colors (Huaiping et al., 2005; Liu et al., 2005; Gu, 2014). The growth environment has an important influence on the choice of shell color, and salinity is one of the important reasons that affect the shell color polymorphism among different populations or within the same population (Guan and He, 2009). The studies on Littorina obtusata and Littorina saxatilis also showed that salinity had a high contribution to the selection of shell color phenotype (Sergievskii and Berger, 1984; Sokolova and Berger, 2000; Phifer-Rixey et al., 2008). Evidence has suggested that the changes of salinity can have regulated the physiology and biochemistry of P. fucata. Liu et al. (2011) found that the oxygen consumption rate and ammonia excretion rate of P. fucata decreased with the increase of salinity in the range of 21–36‰. Under short-term low salinity stress, P. fucata might be vulnerable to diseases due to low activity of lysozyme and catalase (Arisman et al., 2018). Pan et al. (2020) found that the aquaporin expression level of P. fucata returned to the control level (27‰) under high salinity (36‰) stress for 168 h and low salinity (16‰) stress for 72 h. However, there are relatively few studies on the effects of salinity on P. fucata with different shell colors. Therefore, the purpose of this study was to compare and analyze the salinity tolerance of two different shell-colored P. fucata populations by measuring the effects of salinity on osmotic regulation, respiratory metabolism and antioxidant function. Results of the present study can provide a reference for further study of salt tolerance mechanism of P. fucata with different shell colors.
In total, 360 P. fucatas (F11) used in this study were obtained from the Lingshui Station (Hainan, China), Tropical Aquaculture Research and Development Center, South China Sea Fisheries Research Institute, Chinese Academy of Fishery Sciences. Upon transfer to the laboratory, the attached organisms on the shell surface were removed, and healthy individuals with distinct shell color (Figure 1) characteristics and similar size (shell length: 50.75 ± 1.43 mm, body weight: 34.19 ± 1.39 g) were collected. The P. fucata were acclimated in a cement tank (5,000 L) for 7 days. During the acclimation, the water temperature was maintained at 30 ± 1°C; salinity was 35‰; pH was 8.0 ± 0.1; DO was > 6.5 mg/L; light intensity was < 500 Lx and natural photoperiod was used. During the acclimation, 50% water was replaced daily, and the P. fucata was fed with Platymonas subcordiformis at 09:00-09:30 daily with a concentration of 2 × 105 cell/mL. The number of dead P. fucata was recorded and removed daily. Feeding was stopped 1 day before the experiment began.
The experiment was carried out in nine cement tanks (tank volume: 800 L). According to the natural living conditions of P. fucata and the preliminary experimental results, experiment water was adjusted to the desired salinity of 20, 50‰ by mixing natural seawater (35‰), tap water with 24 h aeration and sea salts. Natural double-filtered seawater served as the control. There were three salinity treatments in the experiment, each treatment had three replicates, and each replicate contained 15 P. fucata with red shell and black shell, respectively. Besides the salinity, water quality parameters were maintained at the same level used in the acclimation period. Three shellfish were randomly collected from each parallel at 12 and 24 h, respectively.
The shells were slightly opened by a shell opener, and the adductor muscle was cut off by a scalpel on the left shell, and the pericardial cavity was exposed. Hemolymph was withdrawn from the pericardial cavity of each P. fucata with a 2 mL syringe and was quickly expelled into a 2 mL centrifuging tube. Hemolymph Na+, K+, Cl–, and Ca2+ and osmotic pressure were measured by the PL2000Plus blood gas biochemical analyzer (Nanjing Pulang Medical Equipment Co., Ltd.).
After the hemolymph was collected, the gill and liver tissues were cut off with scissors on an ice tray. Rinsed with cold physiological saline (0.9% NaCl), blotted with filter paper, the tissue samples were placed in a 2 mL centrifugal tube and stored at −80°C. The activities of Na+-K+ -ATPase (Item No. KTB1800) and lactate dehydrogenase (Item No. KTB1110) in gills tissue and the activities of superoxide dismutase (Item No. KTB1030), catalase (Item No. KTB1040), glutathione peroxidase (Item No. KTB1640) and alkaline phosphatase (Item No. KTB1700) in the liver tissue were determined according to the reagent instructions of Abbkine reagent company. The activity of succinic acid dehydrogenase (Item No. A022-1-1) in the gill tissue and total protein (Item No. A045-4-4) was determined according to the instructions of the manufacturer (Nanjing Jiancheng Institute of Biological Engineering).
In the assay, Na+-K+ -ATPase catalyzed ATP hydrolysis to produce ADP and inorganic phosphorus. The content of inorganic phosphorus could reflect the activity of ATPase. The amount of inorganic phosphorus produced by the Na+-K+ -ATPase per mg tissue protein per hour was defined as the enzyme activity unit (U/mg). Lactate activity (U/mL): LDH reduced NAD to NADH, which then interacted with a probe to produce color (λmax = 450 nm). Succinic acid dehydrogenase activity: for determination of SDH activity, one unit of enzyme activity was defined as the reduction of absorbance by 0.01 per minute by 1 mg of tissue protein in the entire chemical reaction medium (U/mgprot). Superoxide dismutase (EC1.15.1.1): superoxide anion (O2–) was provided by xanthine oxidase (XO) catalyzes reaction. O2– reacted with a tetrazolium salt WST-8 dye to form a water-soluble colored formazan product (λmax = 450 nm). When the inhibition percentage in the above-mentioned xanthine oxidase coupling reaction system was 50%, the SOD enzyme activity in the reaction system was defined as an enzyme activity unit (U/mL). Catalase (EC 1.11.1.6) activity: This assay kit was based on the reaction of catalase with methanol, with an optimal concentration of H2O2. The formaldehyde produce could be measured colorimetrically at OD 540 nm. One unit was defined as the amount of enzyme that could cause the formation of 1.0 nmol of formaldehyde per minute at 25°C (nmol/min/ml). Glutathione Peroxidase activity: GSH-Px catalyzed H2O2 to oxidize GSH to produce GSSG, glutathione reductase (GR) catalyzed NADPH to reduce GSSG to regenerate GSH, while NADPH oxidized to produce NADP+, NADPH had a characteristic absorption peak at 340 nm, while NADP+ did not. One unit of enzyme activity was defined at 25°C or 37°C, 1 mol NADPH oxidation per milligram of protein per minute was catalyzed at pH 8.0 (U/mgprot). Alkaline Phosphatase activity: in an alkaline environment, AKP catalyzed phthalate disodium to generate free phenol; phenol reacts with 4-aminoantipyrine and potassium ferricyanide to produce a red quinone derivative, which had characteristic light absorption at 510 nm. One unit of enzyme activity was defined at 37°C, 1 μmol phenol produced per min in 1 mg protein reaction system is defined as a unit of enzyme activity (U/mgprot). Total protein quantification: The content of total protein in gill and liver tissue was determined by BCA method. Under the alkaline condition, protein reduced Cu2+ to Cu+, and Cu+ formed a purple complex with BCA reagent. There was a maximum absorption peak at 562 nm.
All experimental data were expressed as mean ± standard deviation and SPSS 22.0 was used to analyze the hemolymph biochemical indexes and related indexes of gill and liver tissues of P. fucata with the same shell color under different salinity by one-way ANOVA and Duncan multiple comparisons. T-test was used to analyze the differences of hemolymph biochemical indexes gill and liver tissues related indexes with the same salinity and different shell colors, and P < 0.05 was set as the significant difference level.
The response of osmotic pressure in hemolymph to salinity changes was shown in Figure 2. At 12 and 24 h, the hemolymph osmotic pressure increased gradually with the increase of salinity in the range of 20 to 50‰ (P < 0.05). After exposed to 50‰ salinity for 12 h, the hemolymph osmotic pressure of black P. fucata was significantly lower than the red shell (P < 0.05, Figure 2A). Under the same salinity, there was no significant difference in hemolymph osmotic pressure of red shell and black shell P. fucata at 24 h (P < 0.05, Figure 2B).
Figure 2. Effect of salinity on the osmotic pressure of hemolymph of P. fucata with two shell colors (I: 12h; II: 24h). Capital letters indicate the significant difference between salinities of black P. fucata (P < 0.05). Lowercase letters indicate the significant difference between salinities of red P. fucata (P < 0.05). The asterisk “*” indicates significant difference between shell colors under the same salinity (P < 0.05).
The response of Na+ concentration in hemolymph to salinity was shown in Figure 3. At 12 and 24 h, the Na+ concentration increased gradually with salinity (P < 0.05). Under the same salinity, there was no significant difference in Na+ concentration of red shell and black shell P. fucata at 12 h (Figure 3A). However, the treatment groups of red shell showed a higher Na+ concentration than black shell P. fucata in the salinity 35 and 50‰ at 24 h (P < 0.05, Figure 3B).
Figure 3. Effect of salinity on inorganic ion concentration in the hemolymph of P. fucata with two shell colors (I: 12h, Na+ concentration; II: 24h, Na+ concentration; III: 12h, K+ concentration; IV: 24h, K+ concentration; V: 12h, Cl– concentration; VI: 24h, Cl– concentration; VII: 12h, Ca2+ concentration; VIII: 24 h, Ca2+ concentration).
At 12 and 24 h, the K+ concentration increased gradually with salinity (P < 0.05). Under the same salinity and time (12 or 24 h), the K+ concentration in the hemolymph of red shell was significantly higher than in the black P. fucata (P < 0.05, Figures 3C,D).
The Cl– concentration increased gradually with salinity at 12 and 24 h (P < 0.05), but there was no significant difference between red and black P. fucata at a salinity of 20‰ (P < 0.05). The Cl– concentration in the hemolymph of red shell was significantly higher than in the black P. fucata at 35‰ (P < 0.05). However, the Cl– concentration in the hemolymph of red shell was significantly lower than that in the black shell at 50‰ (P < 0.05, Figures 3E,F).
At 12 and 24 h, the Ca2+ concentration increased gradually with the increase of salinity (P < 0.05). Exposed to 35 and 50‰ salinity, the red shell showed a higher Ca2+ concentration than the black P. fucata at 12 h. At 24 h (P < 0.05), the red shell showed a higher Ca2+ concentration than the black P. fucata at salinity 35‰. However, when salinity is 20 and 50‰, there was no significant difference of Ca2+ concentration between red and black P. fucata (P < 0.05, Figures 3G,H).
At 12 h, the activity of Na+-K+ -ATPase in black P. fucata was significantly lower than that in the control at the salinity of 20 and 50‰ (P < 0.05, Figure 4A). However, the gill Na+-K+ -ATPase activity of red P. fucata was significantly higher than that in the control (P < 0.05). At 12 h within the same salinity, the activity of Na+-K+ -ATPase of red P. fucata was significantly higher than that in the black shell at salinity 20‰ (P < 0.05), and significantly lower than that in the black shell at salinity 35 and 50‰ (P < 0.05). At 24 h, the activity of Na+-K+ -ATPase in black P. fucata was significantly lower than that in the control at the salinity of 20 and 50‰. The Na+-K+ -ATPase activity of red shell increased significantly with the increase of salinity in the range of 20 to 50‰ (P < 0.05). At 24 h, the Na+-K+ -ATPase activity of red P. fucata was significantly lower than that in the black shell at salinity 20 and 35‰ salinity, and significantly higher than that in the black shell at salinity 50‰ (P < 0.05, Figure 4B).
Figure 4. Effects of salinity on the activity of Na+-K+ -ATPase in the gill tissue of P. fucata with two shell colors (I: 12h; II: 24h).
After 12 h, the activity of SDH in the black shell and red shell P. fucata was significantly higher than that in the control at the salinity of 20 and 50‰ (P < 0.05, Figure 5A). At the same salinity, the SDH activity of black was significantly higher than that of the red shell (P < 0.05). After exposure to different salinity for 24 h, the activity of SDH in black P. fucata was significantly lower than that in the control at the salinity of 20 and 50‰ (P < 0.05), but there was no significant difference in red P. fucata. At the same salinity, SDH activity of black shell was significantly higher than that of red shell (P < 0.05, Figure 5B).
Figure 5. Effects of salinity on SDH and LDH activities in the gill tissue of P. fucata with two shell colors (I: 12h, SDH activity; II: 24h, SDH activity; III: 12h, LDH activity; IV: 24h, LDH activity).
After 12 h, the LDH activity of red P. fucata and black P. fucata gradually increased (P < 0.05, Figure 5C). At the same salinity, LDH activity of black shell was significantly lower than that of red shell (P < 0.05). After exposure to different salinity for 24 h, the activity of LDH in black P. fucata was significantly higher than that in the control at the salinity of 20 and 50‰ (P < 0.05). Compared to the control, the LDH activity of red P. fucata decreased significantly at salinity 50‰ (P < 0.05), but there was no significant difference at salinity 20‰ (P < 0.05). At salinity of 20 and 35‰, LDH activity of black shell was significantly lower than that of red shell, and significantly higher than that in red shell at salinity of 50‰ (P < 0.05, Figure 5D).
After exposure to salinity of 20 and 50‰ for 12 or 24 h, the SOD activity of black P. fucata was significantly higher than that in the control (P < 0.05, Figures 6A,B), while that of red shell was significantly lower than that in the control (P < 0.05). At 12 h, the SOD activity of black P. fucata was significantly lower than that of red shell at salinity 20‰, but was significantly higher than that of red shell at salinity 35‰ (P < 0.05, Figure 6A). At 24 h, the SOD activity of black P. fucata was significantly higher than that of red shell at salinity of 35‰, but there was no significant difference in the salinity of 20 and 50‰ (P < 0.05, Figure 6B).
Figure 6. Effects of salinity on SOD and CAT activities in the liver tissue of P. fucata with two shell colors (I: 12h, SOD activity; II: 24h, SOD activity; III: 12h, CAT activity; IV: 24h, CAT activity).
After treated with different salinity for 12 h, the CAT activity of black P. fucata was significantly higher than in the control at salinity 20‰, lower than that of the control at salinity 50‰ but no significant difference (P < 0.05, Figure 6C). The CAT activity of red P. fucata was significantly lower than that in the control at salinity 20‰, but significantly higher than that of the control at the salinity of 50‰ (P < 0.05). The CAT activity of black P. fucata was significantly higher than that of red shell at salinity 20‰ (P < 0.05, Figure 6C). After exposure to the salinity of 20 and 50‰ for 24 h, the CAT activity of black P. fucata was significantly higher than that in the control, the CAT activity of red P. fucata was significantly higher than that in the control at salinity 20‰ (P < 0.05, Figure 6D), but significantly lower than that of the control at a salinity of 50‰ (P < 0.05). The CAT activity of black P. fucata was significantly higher than that of red shell in salinity 20 and 50‰ (P < 0.05, Figure 6D).
After treated with different salinity for 12 h, the GSH-Px activity of red P. fucata was significantly higher than that in the control, while that of black P. fucata was no significant difference from that in the control (P < 0.05, Figure 7A). At the salinity of 35‰, the GSH-Px activity of black P. fucata was significantly higher than that of red shell (P < 0.05, Figure 7A). After exposure to salinity of 20 and 50‰ for 24 h, the GSH-Px activity of black P. fucata was significantly lower than that in the control, while that of red shell was significantly higher than that in the control (P < 0.05, Figure 7B). At the same salinity of 20 and 50‰, the activity of GSH-Px of black P. fucata was significantly higher than that of red P. fucata (P < 0.05, Figure 7B).
Figure 7. Effects of salinity on GSH-Px and AKP activities in the liver tissue of P. fucata with two shell colors (I: 12h, GSH-Px activity; II: 24h, GSH-Px activity; III: 12h, AKP activity; IV: 24h, AKP activity).
After exposure to salinity of 20 and 50‰ for 12 h, the AKP activity of black P. fucata was significantly lower than that in the control, while that of red P. fucata was significantly higher than that in the control (P < 0.05, Figure 7C). After exposure to salinity of 20 and 50‰ for 24 h, the AKP activity of red P. fucata was significantly higher than that in the control, the AKP activity of black P. fucata was significantly lower than that in the control at salinity 20‰ (P < 0.05), but significantly higher than that of the control at salinity of 50‰ (P < 0.05, Figure 7D). After 12 and 24 h, the AKP activity of black P. fucata was significantly higher than that of red P. fucata (P < 0.05, Figures 7C,D).
Salinity is an important ecological variable that impacts the growth and survival of aquatic organisms. Due to the influence of seasonal rainfall and continental runoff, seawater salinity in coastal and estuarine areas tends to change dynamically. When marine bivalves encounter salinity stress, they usually respond to the changes in environmental salinity by closing shells and sealing the mantle cavity, and then by adjusting the hemolymph osmotic pressure to cope with salinity stress (Davenport, 1979; Navarro, 1988; Shui, 2007). For most aquatic animals, osmotic pressure regulation is a basic physiological process, which enables the body to adapt to the difference of internal and external ion concentration. However, osmotic pressure regulation is complicated, because the requirement of living environment varies between organism. The osmotic regulation of marine bivalves has been reported in several species, including common mussel M. edulis (Willmer, 1978; Davenport, 1979), horse mussels Modiolus sp. (Pierce, 1970, 1971), soft clam Mya arenaria (Shumway, 1977; Deaton, 1992), arcid clam Noetia ponderosa (Amende and Pierce, 1980), and Pacific oyster Crassostrea gigas (Shumway, 1977). These studies indicated that the hemolymph osmolality varies directly with seawater density, and is either equal to the ambient osmolality, and or slightly hyper-osmotic (5–50 mOsm/kg) to ambient media over a range of non-lethal salinity. In the present study, the osmotic pressure of hemolymph of P. fucata was significantly lower than that of the control at salinity 20‰, and significantly higher than that of the control at salinity 50‰. The osmotic pressure of P. fucata with two shell colors increased significantly with the increase of environmental salinity at 12 h and 24 h. After 12 h stress at 50‰ salinity, the osmotic pressure of red P. fucata was significantly higher than that of black shell, indicating that the tolerance to high salinity of two shell colors P. fucata is different. The metabolic activities of H. cracherodii and H. rufescens under high-salinity stress were swiftly and significantly impacted within 10 min, and began to resume in 6–8 h (Berger and Kharazova, 1997). Liu et al. (2008) found that the hemolymph osmotic pressure of C. japonica tended to be stable within 0.5 days in each treatment group with a sudden change of salinity. In this study, the osmotic pressure of P. fucata with two shell colors in the experimental group was significantly different from that of the control group at 24 h, which may be due to the large range of salinity changes in the experimental group, leading to a wider range of hemolytic osmotic pressure changes, thus increasing the adjustment and recovery time of P. fucata osmotic pressure. In general, physical responses of shellfish to salinity stress become significantly between 24 and 48 h, and such responses will gradually approach or return to the normal level. The duration of this process is species-specific (Castagna, 1973).
The constant osmotic pressure of shellfish mainly refers to the balance between the main osmotic effector in the hemolymph of shellfish and the content of organic ions and inorganic ions in the external water environment, which will not lead to the expansion or shrinkage of cells and can carry on a normal life. Inorganic ions are the main osmotic effectors in the hemolymph of marine shellfish, among which K+, Na+, Cl–, and other ions mainly participate in osmotic adjustment (Cheng et al., 2002). K+ play an important role in maintaining osmotic pressure in neurons and the normal function of the nervous system (Cooper and Morris, 1997). Intracellular changes of Na+ concentration impinge the osmotic pressure response of the organism, seal the mantle cavity, and preserve cells from extreme salinity (Berger and Kharazova, 1997). Most shellfish keep the concentration of K+ in hemolymph always higher than that in the external environment, but the concentrations of sodium and Cl– are lower than those in the external environment. These three inorganic ions change directly with the external salinity and are not regulated by the nervous system and hormones (Natochin et al., 1979; Hildreth and Stickle, 1980; Deaton, 1992). In the present study, the concentrations of Na+, K+, Cl–, and Ca2+ in the low salinity stress group were significantly lower than those in the control, while those in the high salinity stress group were significantly higher than those in the control. The concentrations of inorganic ions in the hemolymph of P. fucata with two shell colors increased significantly with the increases of salinity, which is consistent with the changing trend of osmotic pressure. The results of this study are similar to the changes of inorganic ions in hemolymph after salinity stress of Haliotis Discus reported by Gao et al. (2017), that the concentration of inorganic ions in hemolymph and the changing trend of osmotic pressure are consistent. However, Ding et al. (2013) found that the inorganic ions concentration of Ruditapes philippinarum in the control (32‰) and the experimental group (15‰) reached the maximum concentration at 48 and 24 h, respectively. The decrease of osmotic pressure is not accompanied by the decrease of inorganic ion concentration. The reason may be that P. fucata’s tolerance to salinity is different from R. philippinarum. During the experiment, P. fucata did not close the shells to form a closed space but maintained the osmotic pressure balance inside and outside the cell through ion exchange with the external environment through ion channels on the cell membrane. In addition, at the same time and under the same salinity, the concentration of inorganic ions in the hemolymph of P. fucata with black shell color and red shell color was significantly different. Chen et al. (2010). found that the shell color purification rates of black shell color and red shell color breeding lines of P. fucata (F3) were 95.83 and 100%, respectively. Chen et al. (2016) found that that there were significant differences in shell height, hinge length, shell width, body mass, shell mass, tissue mass, adductor mass, adductor muscle tension and other major traits between black shell and red shell selected lines of P. fucata (F6). Bauchau (2001) proposed that pigmentation is closely related to the regulation of the shell growth to achieve developmental stability. The results showed that the physiological parameters of P. fucata (F11) with red shell and black shell were changed based on the stable inheritance of shell color. Next, the changes of other physiological indexes in this study also fully reflect this point.
Na+-K+ -ATPase is an ion channel that uses the energy generated by ATP hydrolysis to maintain membrane potential by driving three Na+ outlets and two K+ intents, which is essential to osmotic regulation (Evans and Lambert, 2015; Abdel-Mohsen, 2016). In this study, after 12 h and 24 h of salinity stress, Na+-K+ -ATPase activity of P. fucata with black shell in low-salt and high-salt groups was significantly lower than that in the control, showing an inverted U-shape distribution. After 12 h, P. fucata with red shell was significantly higher in low-salt and high-salt treatment groups than in the control, showing a U-shaped distribution. Zhang et al. (2015) found that the influence of salinity on the activity of Na+-K+ -ATPase in the gill filamentum of Siganus guttatus also showed a U-shaped distribution. The reason for the inverted “U” distribution of Na+-K+ -ATPase activity in black P. fucata may be that it is weak in self-adaptation to the change of salinity, which temporarily inhibits the activity of Na+-K+ -ATPase. After 24 h, the Na+-K+ -ATPase activity in red P. fucata increased significantly with the increase of salinity, which is consistent with the changing trend of osmotic pressure. Liu et al. (2008) found that Na+-K+ -ATPase activity of Eriocheir sinensis is negatively correlated with salinity, which is different from the results of our study, indicating that osmotic regulation of P. fucata is not completely implemented by Na+-K+ -ATPase in the gill tissue. It is possible that in a short time of salinity stress, biogenic amines (dopamine and serotonin) could stimulate phosphorylation of the enzyme in the gills including the Na+-K+ -ATPase, and then ATPases could adjust the ion concentration both in vivo and in vitro and make the osmotic pressure of hemolymph attain a balance. After a long time of adaptation, the penetrability of gill epithelium to water and ions changed and then the activity of gill Na+-K+ -ATPase reached a steady level (Kamemoto, 1991; Mo et al., 1998; Lucu and Flik, 1999; Morris, 2001). Under the same salinity stress for the same time, the Na+-K+ -ATPase in gill tissues of P. fucata with two shell colors were significantly different, which indicated that the ion regulation in P. fucata with two shell colors played different roles.
Respiratory metabolism is one of the basic physiological activities of animal energy metabolism, reflecting animal metabolic characteristics, physiological conditions and adaptability to external environmental conditions (Marqueze et al., 2006). Aerobic respiration is the main type of respiratory metabolism in aquatic animals, but anaerobic respiration can also provide energy for the body under low salt conditions. SDH not only plays a key role in tricarboxylic acid cycle, but also participates in oxidative phosphorylation. Therefore, the activity of SDH reflects the aerobic metabolism to a certain extent (Gao et al., 2016). LDH can catalyze the anaerobic metabolite lactic acid to pyruvate and release energy, and its activity can reflect the anaerobic metabolism ability to a certain extent. In our study, after salinity stress for 12 h, the SDH activity of P. fucata with two shell colors was significantly higher than that in the control, which is different from the result reported by Nie et al. (2018) that aerobic respiratory metabolism level gradually increased when the clam was close to the isotonic point. The aerobic metabolism of P. fucata was enhanced under salinity stress, which may be related to the large amount of energy required for the transmembrane transport of inorganic ions. After 24 h, the SDH activity of black P. fucata decreased significantly, while that of red P. fucata had no significant difference. It is possible that long-term salinity stress has a certain influence on the aerobic metabolism level of black P. fucata, while red P. fucata gradually adapt to salinity stress. In this study, after 12 h of different salinity stress, LDH activity of P. fucata of both shell colors was significantly increased with the increase of salinity, and anaerobic metabolism was enhanced accordingly. Shi et al. (2017) found in their study on Epinephelus moara that LDH activity first decreased and then increased under salinity stress, which together with aerobic respiration provided energy for resisting environmental stress. Combined with the change of SDH activity for 12 h, it was suggested that the respiratory metabolism of red shell and black shell P. fucata increased under high salt stress to meet the energy consumption of the body. After 24 h stress, LDH activity of black P. fucata was significantly higher than that of the control, while red shell was significantly lower than that of the control when salinity was 50‰. Our results indicate that under salinity stress for 24 h, the anaerobic metabolism of black shell was enhanced while the aerobic metabolism of the body was weakened, which will provide energy for the body. Under the same salinity stress for the same time, the SDH activity and LDH activity in gill tissue of P. fucata with two shell colors showed significant differences, which indicated that the respiratory metabolic intensity of P. fucata with two shell colors changed in different degrees under salinity stress.
Aerobic organisms unceasingly generate ROS by metabolism. Massive accumulation of ROS damages macromolecular substances, such as nucleic acids, carbohydrates, proteins, and lipids (Finkel and Holbrook, 2000; Lushchak, 2011). As a result, equilibrium between the production and removal of ROS will protect oxidative damage and maintain normal physiological capabilities (Martínez-Álvarez et al., 2005; Okoye et al., 2019). In the long process of evolution, aquatic animals have an evolutionarily conserved antioxidant defense system that can eliminate superfluous ROS. In particular, as antioxidant enzymes, SOD and CAT are regarded as the first line of defense against oxygen intoxication (Bhagat et al., 2016; Wang et al., 2020). SOD can catalyze O2– to produce H2O2 and remove O2–, while CAT can catalyze H2O2 to produce water and oxygen. Therefore, the changes in CAT and SOD enzyme activities can reflect changes in the body’s antioxidant system under environmental stress to a certain extent (Burgeot et al., 1996; Peters and Livingstone, 1996). Li et al. (2012) explores the impacts of salinity on the changes of SOD and CAT activities in Cyclina sinensis, and found that the fluctuations of antioxidant enzymes of C. sinensis under salinity stress were basically completed within 24 h after stress. In the present study, after salinity stress for 12 h and 24 h, SOD activity in the liver of black P. fucata was significantly lower than that the control, while SOD activity of red P. fucata was significantly higher than that the control. The reason might be that the salinity stress caused a large number of free radicals in the black P. fucata, and the production rate of free radicals was much faster than the scavenging rate, and the unscavenged free radicals caused oxidative damage to cells so that the SOD activity in the black P. fucata was always lower than that in the control. However, the red P. fucata had adaptability to salinity stress and maintained high SOD activity to compensate for the SOD consumed by the organism to produce free radicals. In this study, the CAT activity of red P. fucata was lowest after 12 h of low salt stress, but after 24 h of low salt stress, the CAT activity recovered and was significantly higher than that of the control, possibly because the SOD activity increased after 12 h of low salt stress produced a lot of H2O2 to inhibit the CAT activity. The study of Zhang et al. (2020) also shows that the increase of SOD expression level leads to excessive accumulation of hydrogen peroxide, thus inhibiting the function of CAT. In addition, as the second line of preserve against oxidative trauma, GSH-Px also has a significant role in cell metabolism and scavenging of free radicals. In cells, GSH-Px can catalyze the reduction of hydroperoxides to hydroxy compounds (Cnubben et al., 2001; Peña-Llopis et al., 2003). In the present study, the variation trend of GSH-Px activity and the changing trend of SOD activity in red-shell P. fucata were consistent. GSH-Px activity increased, and CAT activity decreased under 20‰ salinity for 12 h and salinity 50‰ stress for 24 h, respectively. At this point, the scavenging of H2O2 was mainly dominated by GSH-Px. Regarding enzyme kinetics, GSH-Px in mammals and other vertebrates has a comparatively large affinity for H2O2 compared with CAT (Reddy et al., 1998; Avanzo et al., 2001). GSH-Px is thus largely conscientious for the removal of H2O2 in vertebrates, while CAT and GSH-Px are play complementarily responsible for H2O2 removal (Mourente et al., 2002; Jo et al., 2008). The activity of GSH-Px, in P. fucata with black shell, was significantly lower than that in the control at 24 h, which also has the complementary effect of GSH-Px and CAT. In response to sudden varies in salinity, CAT and GSH-Px had a decisive action in mediating the scavenging of H2O2 to maintain the mobile equilibrium between internal oxidation and reduction. AKP is involved in various metabolic processes such as metabolism, detoxification, and the biosynthesis of macromolecules for diverse fundamental function (Suzuki and Mori, 1990). AKP is vital lysosomal enzymes in marine invertebrates (Rahman and Siddiqui, 2004) and can take part in non-specific immunity, participate in the degradation of foreign proteins, carbohydrates, and lipids or in phagocytosis (Liang et al., 2014). Li et al. (2015) found that the AKP activity of Chlamys Nobilis first decreased and then increased with the increase of salinity. In this study, after 12 or 24 h, the AKP activity of red P. fucata was significantly higher than that of the control, while that of black P. fucata was significantly higher than that of the control after high salt stress for 24 h. The reason may be that P. fucata produces a large number of free radicals under low or high salinity stress, which increases AKP activity and avoids oxidative damage to cells in the body. However, prolonged low salinity stress resulted in excessive energy consumption and many blood cell apoptosis in black P. fucata, which leads to AKP activity decreased, ultimately. Under the same salinity stress for the same time, the activities of SOD, CAT, GSH-Px, and AKP in liver tissue of P. fucata with two shell colors showed different degrees of differences, which indicated that the antioxidant defense mechanism of P. fucata with two shell colors played different roles under salinity stress.
In summary, the osmotic pressure of hemolymph, ion concentration, Na+-K+ -ATPase and enzymes related to respiratory metabolism in gill tissue, enzymes related to an antioxidant in liver tissue of P. fucata with two shell colors under salinity stress were compared and analyzed. We found that salinity changes had different effects on the physiology and biochemistry of the two shell colors P. fucata. However, red P. fucata can quickly respond positively to the change of environmental salinity and reduce the damage caused by the change of environmental salinity. The results can provide a reference for further study of salt tolerance mechanism of P. fucata with different shell colors.
The raw data supporting the conclusions of this article will be made available by the authors, without undue reservation.
JS and MC conceived and designed the project, and wrote the manuscript. ZF and JY measured and collected the data. SZ collected the samples and carried out the analysis. ZM, WZ, and GY supervisioned, wrote—reviewed and edited the manuscript. All authors contributed to the article and approved the submitted version.
This research was financially supported by Central Public-interest Scientific Institution Basal Research Fund, CAFS (No. 2020TD55), Guangxi Key Research and Development Program (Guike AB18221090), Natural Science Foundation of Hainan Province (319QN338), and Beihai Science and Technology Planning Project (Beikehe 201995002).
The authors declare that the research was conducted in the absence of any commercial or financial relationships that could be construed as a potential conflict of interest.
All claims expressed in this article are solely those of the authors and do not necessarily represent those of their affiliated organizations, or those of the publisher, the editors and the reviewers. Any product that may be evaluated in this article, or claim that may be made by its manufacturer, is not guaranteed or endorsed by the publisher.
Abdel-Mohsen, H. (2016). Assessment of respiratory and ion transport potential of Penaeus japonicus gills in response to environmental pollution. Mediter. Mar. Sci. 10, 5–18. doi: 10.12681/mms.118
Ai, H., Li, Y., and Ding, Y. (2003). A review of study on diseases in pearl oyster. Shanghai Fish. Univ. 12, 61–64.
Alagarswami, K., and Victor, A. (1976). Salinity tolerance and rate of filtration of the pearl oyster Pinctada fucata. J. Mar. Biol. Assoc. India 18, 149–158.
Amende, L. M., and Pierce, S. K. (1980). Cellular volume regulation in salinity stressed molluscs: the response of Noetia ponderosa (Arcidae) red blood cells to osmotic variation. J. Compar. Physiol. 138, 283–289. doi: 10.1007/bf00691562
Arisman, N., Istiqomad, N., and Yoshimatsu, T. (2018). Impact of short-term hyposalinity stress on Akoya pearl oyster, Pinctada fucata (Gould 1850). Asian Fish. Sci. 31, 265–275.
Avanzo, J. L., de Mendonça, C. X. Jr., Pugine, S. M. P., and de Cerqueira Cesar, M. (2001). Effect of vitamin E and selenium on resistance to oxidative stress in chicken superficial pectoralis muscle. Compar. Biochem. Physiol. Part C Toxicol. Pharmacol. 129, 163–173. doi: 10.1016/s1532-0456(01)00197-1
Ballantyne, J. S., and Moyes, C. D. (1987). Osmotic effects on fatty acid, pyruvate, and ketone body oxidation in oyster gill mitochondria. Physiol. Zool. 60, 713–721. doi: 10.1086/physzool.60.6.30159987
Bauchau, V. (2001). Developmental stability as the primary function of the pigmentation patterns in bivalve shells? Belgian J. Zool. 131, 23–28.
Berger, V. J., and Kharazova, A. (1997). “Mechanisms of salinity adaptations in marine molluscs,” in Interactions and adaptation strategies of marine organisms. Cham: Springer, 115–126.
Bhagat, J., Ingole, B., and Singh, N. (2016). Glutathione S-transferase, catalase, superoxide dismutase, glutathione peroxidase, and lipid peroxidation as biomarkers of oxidative stress in snails: A review. Invertebrate Surv. J. 13, 336–349.
Burgeot, T., Bocquéné, G., Porte, C., Dimeet, J., Santella, R., Pfhol-Leszkowicz, A., et al. (1996). Bioindicators of pollutant exposure in the northwestern Mediterranean Sea. Mar. Ecol. Prog. Ser. 131, 125–141. doi: 10.3354/meps131125
Castagna, M. (1973). Salinity tolerance of some marine bivalves from inshore and estuarine environments in Virginia waters on the western mid-Atlantic coast. Malacologia 12, 47–96.
Chen, J., Liu, Z., Sun, X., Wang, H., and Du, X. (2010). Analysis on growth characteristic and genetic diversity in the selected lines (F3) of four shell colors of Pincatada martensii. J. Shanghai Ocean Univ. 19, 588–595.
Chen, J.-C., and Lin, C.-Y. (1995). Responses of oxygen consumption, ammonia-N excretion and urea-N excretion of Penaeus chinensis exposed to ambient ammonia at different salinity and pH levels. Aquaculture 136, 243–255. doi: 10.1016/0044-8486(95)01060-2
Chen, M., Liu, B., Yan, J., Guo, H., Wu, K., and Li, Y. (2016). Comparison analysis of major traits among four shell color selective lines of pearl oyster (Pinctada fucata). South China Fish. Sci. 12, 118–122.
Cheng, W., Yeh, S.-P., Wang, C.-S., and Chen, J.-C. (2002). Osmotic and ionic changes in Taiwan abalone Haliotis diversicolor supertexta at different salinity levels. Aquaculture 203, 349–357. doi: 10.1016/s0044-8486(01)00606-8
Cnubben, N. H., Rietjens, I. M., Wortelboer, H., van Zanden, J., and van Bladeren, P. J. (2001). The interplay of glutathione-related processes in antioxidant defense. Environ. Toxicol. Pharmacol. 10, 141–152. doi: 10.1016/s1382-6689(01)00077-1
Cooper, A. R., and Morris, S. (1997). Osmotic and ionic regulation by Leptograpsus variegatus during hyposaline exposure and in response to emersion. J. Exper. Mar. Biol. Ecol. 214, 263–282. doi: 10.1016/s0022-0981(96)02778-5
Davenport, J. (1979). Is Mytilus edulis a short term osmoregulator? Compar. Biochem. Physiol. Part A Mol. Integr. Physiol. 64, 91–95. doi: 10.1016/0300-9629(79)90436-5
Deaton, L. E. (1992). Osmoregulation and epithelial permeability in two euryhaline bivalve molluscs: Mya arenaria and Geukensia demissa. J. Exper. Mar. Biol. Ecol. 158, 167–177. doi: 10.1016/0022-0981(92)90224-x
Ding, J., Wang, R., Yan, X., Zhao, L.-Q., Yang, F., and Wang, L.-S. (2013). Comparative tolerance to low salinity stress in Manila clam Ruditapes philippinarum with three shell colors. J. Dalian Ocean Univ. 28, 264–268.
Edwards, S. (2003). Assessment of the physiological effect of altered salinity on greenlip (Haliotis laevigata) and blacklip (Haliotis rubra) abalone using respirometry. Aquacult. Res. 34, 1361–1365. doi: 10.1046/j.1365-2109.2003.00943.x
Evans, A. N., and Lambert, F. N. (2015). Na+/K+-ATPase α1 mRNA expression in the gill and rectal gland of the Atlantic stingray, Dasyatis sabina, following acclimation to increased salinity. BMC Res. Notes 8:219. doi: 10.1186/s13104-015-1216-7
Finkel, T., and Holbrook, N. J. (2000). Oxidants, oxidative stress and the biology of ageing. Nature 408, 239–247. doi: 10.1038/35041687
Fisher, W. S., and Newell, R. I. (1986). Salinity effects on the activity of granular hemocytes of American oysters, Crassostrea virginica. Biol. Bull. 170, 122–134. doi: 10.2307/1541385
Gao, X., Li, Y., Li, X., Wu, F., Song, C., and Liu, Y. (2017). The response and osmotic pressure regulation mechanism of Haliotis discus hannai (Mollusca, Gastropoda) to sudden salinity changes. Hydrobiologia 795, 181–198. doi: 10.1007/s10750-017-3129-z
Gao, X., Zhang, M., Li, X., Shi, C., Song, C., and Liu, Y. (2016). Effects of LED light quality on the growth, metabolism, and energy budgets of Haliotis discus discus. Aquaculture 453, 31–39. doi: 10.1016/j.aquaculture.2015.11.033
Garçon, D. P., Masui, D. C., Mantelatto, F. L., Furriel, R. P., McNamara, J. C., and Leone, F. A. (2009). Hemolymph ionic regulation and adjustments in gill (Na+, K+)-ATPase activity during salinity acclimation in the swimming crab Callinectes ornatus (Decapoda, Brachyura). Compar. Biochem. Physiol. Part A Mol. Integr. Physiol. 154, 44–55. doi: 10.1016/j.cbpa.2009.04.624
Ghiselli, A., Serafini, M., Natella, F., and Scaccini, C. (2000). Total antioxidant capacity as a tool to assess redox status: critical view and experimental data. Free Rad. Biol. Med. 29, 1106–1114. doi: 10.1016/s0891-5849(00)00394-4
Gu, X. (2014). The variation analysis of nutrient composition and the growth trait of clams with different shell color and decorative pattern. China: Ninbo University.
Guan, Y., and He, M. (2009). Research progress on shell color polymorphism of marine economic shellfish. Mar. Sci. Bull. 28, 108–114.
Hildreth, J. E., and Stickle, W. B. (1980). The effects of temperature and salinity on the osmotic composition of the southern oyster drill, Thais haemastoma. Biol. Bull. 159, 148–161. doi: 10.2307/1541015
Huaiping, Z., Guofan, Z., and Xiao, L. (2005). Comparison of growth and survival of larvae among different shell color stocks of bay scallop Argopecten irradians irradians (Lamarck 1819). Chinese J. Oceanol. Limnol. 23:183. doi: 10.1007/bf02894236
Huang, G., Guo, Y., Li, L., Fan, S., Yu, Z., and Yu, D. (2016). Genomic structure of the α-amylase gene in the pearl oyster Pinctada fucata and its expression in response to salinity and food concentration. Gene 587, 98–105. doi: 10.1016/j.gene.2016.04.044
Jasmani, S., Jayasankar, V., and Wilder, M. (2010). Na/K-ATPase activity and osmo-ionic regulation in adult whiteleg shrimp Litopenaeus vannamei exposed to low salinities. Aquaculture 304, 88–94. doi: 10.1016/j.aquaculture.2010.03.025
Jo, P. G., Choi, Y. K., and Choi, C. Y. (2008). Cloning and mRNA expression of antioxidant enzymes in the Pacific oyster, Crassostrea gigas in response to cadmium exposure. Compar. Biochem. Physiol. Part C Toxicol. Pharmacol. 147, 460–469. doi: 10.1016/j.cbpc.2008.02.001
Kim, W., Kim, J., Kim, M., Park, C., and Huh, H. (1998). Effects of sudden changes in salinity on endogenous rhythms of the spotted sea bass Lateolabrax sp. Mar. Biol. 131, 219–225. doi: 10.1007/s002270050314
Li, Z., Qian, J., Lao, C., and Liu, Z. (2015). Synergistic effects of temperature and salinity on the activities of immune-related enzymes of Chlamys nobilis (Reeve). Adv. Mar. Sci. 32, 227–237.
Li, Z.-N., Lin, T., Yao, Z., Lai, Q.-F., Lu, J.-X., Wang, H., et al. (2012). Effects of water salinity on the antioxidant enzyme activities and growth of clam Cyclina sinensis. Chinese J. Ecol. 31, 2625–2630.
Liang, S., Luo, X., You, W., Luo, L., and Ke, C. (2014). The role of hybridization in improving the immune response and thermal tolerance of abalone. Fish Shellfish Immunol. 39, 69–77. doi: 10.1016/j.fsi.2014.04.014
Liu, H., Pan, L., and Fu, L. (2008). Effect of salinity on hemolymph osmotic pressure, sodium concentration and Na+-K+-ATPase activity of gill of Chinese crab, Eriocheir sinensis. J. Ocean Univ. China 7, 77–82. doi: 10.1007/s11802-008-0077-2
Liu, J., Yu, D., and Li, J. (2011). Effects of salinity and pH on oxygen consumption and ammonia excretion rates in pinctada fucata. Oceanol. Limnol. Sinica 4, 603–607.
Liu, X., Deng, Y., and Zhang, G. (2005). Growth of eight Pacific abalone families at three temperatures. Acta Oceanol. Sinica 24, 148–153.
Liu, X., and Yan, A. (2006). Recovery and adaptation process of Pelteobagrus fulvidraco in the experimental circulatory system after transportation stress. J. Fisheries China 4, 495–501.
Lucu, C. E., and Flik, G. (1999). Na+-K+-ATPase and Na+/Ca2+ exchange activities in gills of hyperregulating Carcinus maenas. Am. J. Physiol. 276, R490–R499.
Lushchak, V. I. (2011). Environmentally induced oxidative stress in aquatic animals. Aquatic Toxicol. 101, 13–30. doi: 10.1016/j.aquatox.2010.10.006
Marqueze, A., Kucharski, L. C., and Da Silva, R. S. M. (2006). Effects of anoxia and post-anoxia recovery on carbohydrate metabolism in the jaw muscle of the crab Chasmagnathus granulatus maintained on carbohydrate-rich or high-protein diets. J. Exper. Mar. Biol. Ecol. 332, 198–205. doi: 10.1016/j.jembe.2005.11.009
Martínez-Álvarez, R. M., Morales, A. E., and Sanz, A. (2005). Antioxidant defenses in fish: biotic and abiotic factors. Rev. Fish Biol. Fisheries 15, 75–88. doi: 10.1007/s11160-005-7846-4
Masui, D. C., Mantelatto, F. L., McNamara, J. C., Furriel, R. P., and Leone, F. A. (2009). Na+, K+-ATPase activity in gill microsomes from the blue crab, Callinectes danae, acclimated to low salinity: novel perspectives on ammonia excretion. Compar. Biochem. Physiol. Part A Mol. Integr. Physiol. 153, 141–148. doi: 10.1016/j.cbpa.2009.01.020
Mo, J. L., Devos, P., and Trausch, G. (1998). Dopamine as a modulator of ionic transport and Na+/K+-ATPase activity in the gills of the Chinese crab Eriocheir sinensis. J. Crustacean Biol. 18, 442–448. doi: 10.1163/193724098x00278
Morris, S. (2001). Neuroendocrine regulation of osmoregulation and the evolution of air-breathing in decapod crustaceans. J. Exper. Biol. 204, 979–989. doi: 10.1242/jeb.204.5.979
Mourente, G., Dıaz-Salvago, E., Bell, J. G., and Tocher, D. R. (2002). Increased activities of hepatic antioxidant defence enzymes in juvenile gilthead sea bream (Sparus aurata L.) fed dietary oxidised oil: attenuation by dietary vitamin E. Aquaculture 214, 343–361. doi: 10.1016/s0044-8486(02)00064-9
Nan, X., Xi-Chun, W., You-Fang, D., Ren, L., and Kun-Lun, T. (2004). Effect of initial fluid resuscitation on subsequent treatment in uncontrolled hemorrhagic shock in rats. Shock 21, 276–280. doi: 10.1097/01.shk.0000110622.42625.cb
Natochin, Y. V., Berger, V. Y., Khlebovich, V., Lavrova, E., and Michailova, O. Y. (1979). The participation of electrolytes in adaptation mechanisms of intertidal molluscs’ cells to altered salinity. Compar. Biochem. Physiol. Part A Mol. Integr. Physiol. 63, 115–119. doi: 10.1016/0300-9629(79)90636-4
Navarro, J. M. (1988). The effects of salinity on the physiological ecology of Choromytilus chorus (Molina, 1782)(Bivalvia: Mytilidae). J. Exper. Mar. Biol. Ecol. 122, 19–33. doi: 10.1016/0022-0981(88)90209-2
Nie, H., Wenhao, L., Dongdong, L., Donglin, L., and Xiwu, Y. (2018). Effects of temperature and salinity on respiratory metabolic enzyme activities of Clinochardium California. Acta Eco. Zool. 22, 85–89. doi: 10.1002/9781119155423.ch9
Ocaño-Higuera, V. M., Maeda-Martínez, A. N., Lugo-Sánchez, M. E., García-Sánchez, G., Márquez-Ríos, E., Gómez-Jimenez, S., et al. (2011). Effect of emerged shipment on the physiological condition of the adductor muscle in adult giant lion’s paw scallop Nodipecten subnodosus (Sowerby 1835). Aquacult. Res. 42, 1087–1095. doi: 10.1111/j.1365-2109.2010.02693.x
Okoye, C. N., MacDonald-Jay, N., and Kamunde, C. (2019). Effects of bioenergetics, temperature and cadmium on liver mitochondria reactive oxygen species production and consumption. Aquatic Toxicol. 214:105264. doi: 10.1016/j.aquatox.2019.105264
Pan, X., Liu, H., Xu, M., Xu, H., Zhang, H., and He, M. (2020). Cloning and expression analysis of aquaporin gene AQP4 cDNA from Pinctada fucata martensii. J. Trop. Oceanogr. 39, 66–75.
Peña-Llopis, S., Ferrando, M. D., and Peña, J. B. (2003). Fish tolerance to organophosphate-induced oxidative stress is dependent on the glutathione metabolism and enhanced by N-acetylcysteine. Aquatic Toxicol. 65, 337–360. doi: 10.1016/s0166-445x(03)00148-6
Peters, L., and Livingstone, D. (1996). Antioxidant enzyme activities in embryologic and early larval stages of turbot. J. Fish. Biol. 49, 986–997. doi: 10.1111/j.1095-8649.1996.tb00095.x
Phifer-Rixey, M., Heckman, M., Trussell, G., and Schmidt, P. (2008). Maintenance of clinal variation for shell colour phenotype in the flat periwinkle Littorina obtusata. J. Evol. Biol. 21, 966–978. doi: 10.1111/j.1420-9101.2008.01549.x
Pierce, S. K. Jr. (1970). The water balance of modiolus (Mollusca: Bivalvia: Mytilidae): osmotic concentrations in changing salinities. Compar. Biochem. Physiol. 36, 521–533. doi: 10.1016/0010-406x(70)91028-5
Pierce, S. K. Jr. (1971). Volume regulation and valve movements by marine mussels. Compar. Biochem. Physiol. Part A Physiol. 39, 103–117. doi: 10.1016/0300-9629(71)90350-1
Rahman, M., and Siddiqui, M. (2004). Biochemical effects of vepacide (from Azadirachta indica) on Wistar rats during subchronic exposure. Ecotoxicol. Environ. Safety 59, 332–339. doi: 10.1016/j.ecoenv.2003.07.013
Reddy, K. V., Kumar, T. C., Prasad, M., and Reddanna, P. (1998). Pulmonary lipid peroxidation and antioxidant defenses during exhaustive physical exercise: the role of vitamin E and selenium. Nutrition 14, 448–451. doi: 10.1016/s0899-9007(98)00016-1
Sergievskii, S., and Berger, V. J. (1984). Physiological differences of principal shell-colour phenotypes of the gastropod mollusc Littorina obtusata. Biol. Moria 2, 36–44.
Shi, Z., Liao, Y., Wang, X., Zhang, C., Peng, S., and Gao, Q. (2017). Impact of the abrupt salinity decrease on ion-regulation enzyme activity in the gill and serum osmolality from Epinehelus moara. J. Safety Environ. 17, 1210–1214.
Shui, B. (2007). A study on effect of several environmental factors on quick purification of Sinonovacula constricta (Lamarck). Modern Fish. Infor. 22, 6–10.
Shumway, S. (1977). Effect of salinity fluctuation on the osmotic pressure and Na+, Ca 2+ and Mg2+ ion concentrations in the hemolymph of bivalve molluscs. Mar. Biol. 41, 153–177. doi: 10.1007/bf00394023
Sokolova, I., and Berger, V. J. (2000). Physiological variation related to shell colour polymorphism in White Sea Littorina saxatilis. J. Exper. Mar. Biol. Ecol. 245, 1–23. doi: 10.1016/s0022-0981(99)00132-x
Suzuki, T., and Mori, K. (1990). Hemolymph lectin of the pearl oyster, Pinctada fucata martensii: a possible non-self recognition system. Dev. Compar. Immunol. 14, 161–173. doi: 10.1016/0145-305x(90)90088-v
Wang, N., Yang, J., Zhang, H., Soon, T. K., Liu, H., Li, S., et al. (2020). Differential responses to low salinity on gene expression, physiological and biochemical indexes between the golden and brown noble scallops Chlamys nobilis. Aquacult. Res. 51, 316–325. doi: 10.1111/are.14377
Widdows, J. (1985). “The effects of fluctuating and abrupt changes in salinity on the performance of Mytilus edulis”, in: Marine biology of polar regions effects of stress on marine organisms. Chichester: John Wiley.
Willmer, P. (1978). Volume regulation and solute balance in the nervous tissue of an osmoconforming bivalve (Mytilus edulis). J. Exper. Biol. 77, 157–179. doi: 10.1242/jeb.77.1.157
Yang, C., Hao, R., Deng, Y., Liao, Y., Wang, Q., Sun, R., et al. (2017). Effects of protein sources on growth, immunity and antioxidant capacity of juvenile pearl oyster Pinctada fucata martensii. Fish Shellfish Immunol. 67, 411–418. doi: 10.1016/j.fsi.2017.06.037
Zhang, J., Luo, Y., Huang, Y., Zhong, Y., Liu, Q., Li, J., et al. (2015). Genetic structure of inbred and hybrid families of pearl oyster Pinctada martensii. Genom. Appl. Biol. 34, 723–730.
Keywords: Pinctada fucata, salinity, osmotic regulation, respiratory metabolism, antioxidant
Citation: Sun J, Chen M, Fu Z, Yang J, Zhou S, Yu G, Zhou W and Ma Z (2021) A Comparative Study on Low and High Salinity Tolerance of Two Strains of Pinctada fucata. Front. Mar. Sci. 8:704907. doi: 10.3389/fmars.2021.704907
Received: 04 May 2021; Accepted: 08 July 2021;
Published: 27 July 2021.
Edited by:
Yan Shi, Hohai University, ChinaReviewed by:
You-Ting Zhu, Shanghai Ocean University, ChinaCopyright © 2021 Sun, Chen, Fu, Yang, Zhou, Yu, Zhou and Ma. This is an open-access article distributed under the terms of the Creative Commons Attribution License (CC BY). The use, distribution or reproduction in other forums is permitted, provided the original author(s) and the copyright owner(s) are credited and that the original publication in this journal is cited, in accordance with accepted academic practice. No use, distribution or reproduction is permitted which does not comply with these terms.
*Correspondence: Wenli Zhou, d2x6aG91NjlAMTI2LmNvbQ==; Zhenhua Ma, emhlbmh1YS5tYUBob3RtYWlsLmNvbQ==
†These authors have contributed equally to this work
Disclaimer: All claims expressed in this article are solely those of the authors and do not necessarily represent those of their affiliated organizations, or those of the publisher, the editors and the reviewers. Any product that may be evaluated in this article or claim that may be made by its manufacturer is not guaranteed or endorsed by the publisher.
Research integrity at Frontiers
Learn more about the work of our research integrity team to safeguard the quality of each article we publish.