- 1Marine Science Institute, University of the Philippines, Quezon City, Philippines
- 2Department of Earth Sciences, National History Museum, London, United Kingdom
Tropical coral reefs are threatened by local-scale stressors that are exacerbated by global ocean warming and acidification from the post-industrial increase of atmospheric CO2 levels. Despite their observed decline in the past four decades, little is known on how Philippine coral reefs will respond to ocean warming and acidification. This study explored individual and synergistic effects of present-day (pH 8.0, 28°C) and near-future (pH 7.7, 32°C) scenarios of ocean temperature and pH on the adult Favites colemani, a common massive reef-building coral in Bolinao-Anda, Philippines. Changes in seawater temperature drive the physiological responses of F. colemani, whereas changes in pH create an additive effect on survival, growth, and photosynthetic efficiency. Under near-future scenarios, F. colemani showed sustained photosynthetic competency despite the decline in growth rate and zooxanthellae density. F. colemani exhibited specificity with the Cladocopium clade C3u. This coral experienced lower growth rates but survived projected near-future ocean warming and acidification scenarios. Its pH-thermal stress threshold is possibly a consequence of acclimation and adaptation to local environmental conditions and past bleaching events. This research highlights the importance of examining the susceptibility and resilience of Philippine corals to climate-driven stressors for future conservation and restoration efforts in the changing ocean.
Introduction
Global warming and ocean acidification due to the unprecedented increase in anthropogenic CO2 emissions pose a serious threat to coral reefs worldwide (Hoegh-Guldberg et al., 2007). The ocean’s storage of 90% accumulated excess energy in the climate system over the past century led to a 0.6 ± 0.2°C increase in global sea surface temperatures (IPCC, 2001; Hoegh-Guldberg et al., 2007). The average oceanic uptake of CO2 from anthropogenic emissions is 31%, with uptake rate in 1994–2007 increasing in proportion to the rise in atmospheric CO2 (Gruber et al., 2019). Oceanic uptake may have slowed down the accumulation of CO2 in the atmosphere, but it has resulted in ocean acidification (Sabine et al., 2004; Doney et al., 2009). Under business-as-usual scenarios of atmospheric CO2 emission levels, global sea surface temperatures are projected to increase another 0.3–4.8°C, and sea surface pH to further decrease by 0.06–0.32 pH units by the end of the twenty-first century (IPCC, 2014). With the climate-driven changes in the ocean, coral reefs worldwide are forecast to rapidly degrade over the next 20 years (Hoegh-Guldberg et al., 2017). Record-breaking highs in global surface temperatures (from 2014 to at least 2016) have resulted in the longest global coral bleaching event on record (NOAA, 2015).
Maximum habitat temperatures are close to the upper thermal limits of most tropical corals (Somero, 2010). Changes in habitat pH and temperature may influence the coral’s ability to survive, thrive, and to build and maintain massive complex reef structures (Kleypas and Langdon, 2006; Hoegh-Guldberg et al., 2007). High sea surface temperatures (1°C above average summer value) have been strongly associated with mass coral bleaching (Glynn, 1993; Hoegh-Guldberg et al., 2007; Gattuso et al., 2014). Bleached and/or thermally stressed corals may exhibit decline in growth/calcification rates (Cooper et al., 2008; Tanzil et al., 2009; Cantin et al., 2010), in protein content (Grottoli et al., 2004), and in tissue regeneration capacities (Meesters and Bak, 1993). Inability of corals to recover from bleaching may lead to mortality depending on the severity of the heat stress (Goreau et al., 2000; Kavousi et al., 2015). Further, ocean acidification may affect CaCO3 forming ability of corals through induced calcium carbonate precipitation (Doney et al., 2009) and increased calcium carbonate dissolution rates (Andersson and Gledhill, 2013). Thus, the predicted interaction of ocean warming and acidification may negatively affect coral reef global distribution and their existence in the future. While most studies focused on individual effects, combined ocean warming and acidification effects on corals are scarcely explored (Reynaud et al., 2003; Ogawa et al., 2013; Kavousi et al., 2015).
Coral reef ecosystems are of immense biological, economical, and societal importance (Roth, 2014). Philippine reefs are among the most productive and diverse in the world. The country’s 25,000 km2 of total reef area (Gomez et al., 1994) contribute about US $4.94 billion annually (Cruz-Trinidad et al., 2011) to the nation’s economy in the form of fisheries, tourism, and coastal protection (Conservation International, 2008). A recent nationwide coral reef assessment (2014–2018) estimated that over the past decade, the country lost about a third of its coral reefs (Licuanan et al., 2019). This decline in the local reefs due to both natural and manmade threats (Roberts et al., 2002; Burke et al., 2011) may be amplified by impending global climate driven stressors. Nonetheless, little is known on the effects of low pH and a combination of low pH and high temperature on Philippine corals (Baria, 2016).
The 1997–1998 bleaching event that occurred during a decade of warmest average temperatures saw the highest decline in live coral reef cover (up to 46%) in the Philippines (Arceo et al., 2001; NOAA, 2015). It was during this time that 80% of corals in the Bolinao-Anda Reef Complex (BARC, northwestern Philippines) bleached including the more tolerant massive corals (Arceo et al., 2001; Chou et al., 2002). Further, fish farming of Chanos chanos along the Guiguiwanen channel in Bolinao led to ∼0.2–0.3 decrease in seawater pH in the mariculture area (San Diego-McGlone et al., 2008; Escobar et al., 2013; Lagumen, 2017). Effluent from the mariculture area may adversely affect the adjacent reefs and exacerbate effects of future scenarios of ocean warming and acidification. Interestingly, Favites colemani corals still thrive in the Lucero reef in BARC, which is near Guiguiwanen channel. Could this be a consequence of acclimation and adaptation to local environmental conditions? To address this, we focused our work on this encrusting, submassive to massive reef-building coral F. colemani (Family Faviidae) commonly found in the Philippines (Veron, 2000; Maboloc et al., 2015). This study aims to explore physiological responses of adult F. colemani from Lucero reef to near-future scenarios of ocean warming and acidification.
Materials and Methods
Coral Collection and Acclimatization
Five non-gravid adult Favites colemani colonies (>15 cm), with no pigmented oocytes, were collected in January 2016 at depths of 3–5 m in Lucero, Bolinao (16°24′43.2″ N 119°54′12.2″ E; Garmin Montana 680). Although genotype validation was not conducted, colonies were collected at least 15 m apart horizontally to minimize the chances of incidentally sampling clones. Coral collection was done by chipping the substrate where the colonies were attached. All five colonies were collected during one dive. The coral colonies were placed in aerated seawater tanks in the research boat, and immediately transported to the UP Marine Science Institute Bolinao Laboratory (UPMSI BML) that was a 10–15 min boat ride away from the site.
Fifteen to eighteen explants (6–9 cm2) were fragmented from each colony and attached to plastic screws using cyanoacrylate adhesive (Mighty Bond Xtreme, Pioneer). Explants were tagged and pre-conditioned for 1 month in indoor experimental tanks in a ∼24°C temperature-controlled room with flow-through seawater maintained at 28 ± 0.7°C and under artificial photosynthetic photon flux density of ∼90 μmol m–2s–1 on a 12:12 light-dark cycle. This enabled coral explants to recover from handling stress and acclimate to the conditions in the experiment control tanks. Similar to other studies, coral explants had positive growth, no mortality and no bleaching under relatively lower light intensity than the natural environment (Ohki et al., 2013; Kavousi et al., 2015; Da-Anoy et al., 2019; Manullang et al., 2020).
Experiment Setup
Ocean acidification-thermal stress experiments were conducted in 17 L tanks in a flow through seawater system (Figure 1). Filtered (10 μm) seawater maintained at 28 ± 0.7°C (HC1000A, Hailea) was pumped into each experiment tank from a 45 L mixing tank at a flow rate of ∼2–3 L h–1. Seawater inside each mixing tank was either adjusted to a lower pH value using the mass-flow controlled (Sev-E40, Horibastec) CO2 dosing, or maintained at control pH value through bubbling aeration. Additional mixing in the experiment tanks was provided by a 285 L h–1 submersible water pump (HX800, Hailea). Each experimental tank was placed in 45 L thermal bath with submersible thermostat heater (Eheim, Germany). Four pH-temperature treatments were used: Control (C: pH 8.0, 28°C), ocean acidification (OA: pH 7.7, 28°C), ocean warming (T: pH 8.0, 32°C), and ocean acidification with warming (OAT: pH 7.7, 32°C). pH conditions of 7.7 and 8.0 were chosen as present day (study site pH range ∼8.0–8.1), and IPCC projected seawater pH (based on Representative Concentration Pathway 8.5) by the end of this century (IPCC, 2014) scenarios, respectively. We chose 28°C as control temperature, which represents annual seawater temperature in Bolinao (28.89 ± 0.90°C; Guzman and Conaco, 2016), and 32°C as stress temperature, which represents increases of 4°C from mean annual and 2°C from warmest monthly mean seawater temperatures (30.64 ± 0.79°C; Maboloc et al., 2015) in Bolinao. Seawater temperature inside the experiment tanks were kept stable by using thermal baths with thermostat heaters, and by maintaining the temperatures in the mixing tanks (∼28°C) and the experiment room (∼24°C). Three independent replicate experimental tanks were used for each pH-temperature treatment. No two pH-temperature replicates were dependent on the same treatment water source. Treatment replicates were randomly interspersed in the system (Cornwall and Hurd, 2015). Experimental tanks were illuminated under artificial light with photosynthetic photon flux density of ∼90 μmol m–2s–1 (Onset HOBO) on a 12:12 light-dark cycle to avoid light stress. Temperature and pH in each experimental tank were recorded thrice a day (8:00 a.m., 12:00 p.m., 6:00 p.m.) using pH/conductivity meter (SevenGo, Mettler Toledo) calibrated using NBS buffer solutions. Experimental tank water temperatures were also recorded every 15 min using data logger (UA-002-64, Onset HOBO). The seawater salinity was 32.9 ppt. Seawater samples were collected every week and analyzed using a Total Alkalinity titrator (Kimoto, ATT-05, Japan) calibrated with certified reference material (CRM) for ocean CO2 measurements. The partial pressure of carbon dioxide (pCO2) and aragonite saturation (Ωa) were calculated using the CO2SYS program (Lewis and Wallace, 1998), utilizing K1 and K2 dissociation constants (Mehrbach et al., 1973).
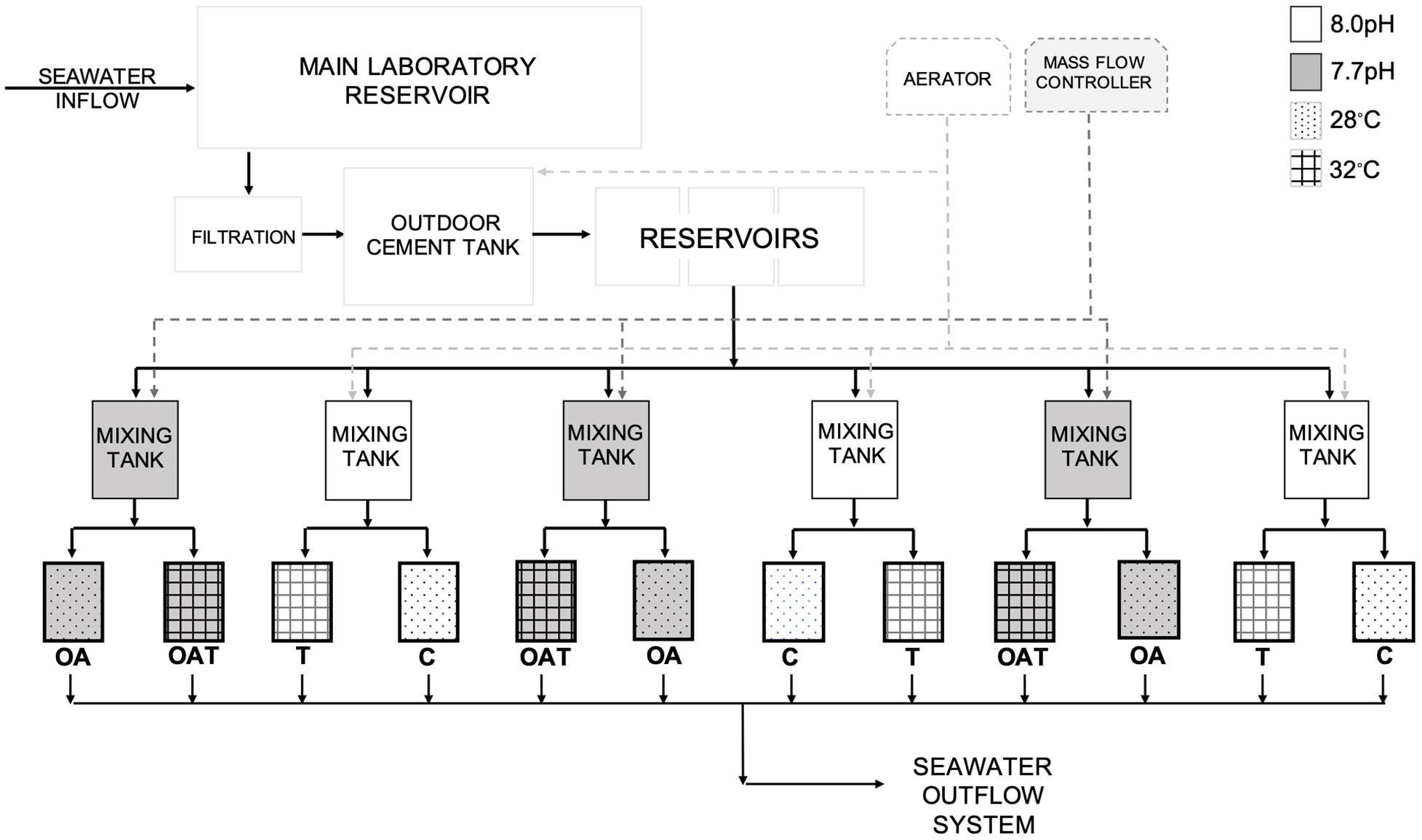
Figure 1. Schematic diagram of ocean acidification-thermal stress system set-up at UPMSI BML. Solid arrows are seawater flow paths as it enters and exits the system. Dashed arrows are inputs of air and CO2 to the system.
Physiological Analyses
Fifteen coral explants were exposed under each pH-temperature treatment (one explant per colony in each experiment tank replicate) for 28 days. The explants were monitored daily for bleaching (Siebeck et al., 2006) and survival (McClanahan, 2004). F. colemani explants that were both bleached and necrosed (no visible living coral tissue) were recorded as “dead” (Figure 2). Quantification of the growth (calcification) rate was done through buoyant weight method. Growth rate (% d–1) was determined using the equation G = (ΔWa/Δt) × 100; where ΔWa is change in final and initial dry coral weight, and Δt is length of experiment (28 days). Dry coral weight (Wa) was calculated using the equation Wa = Ww/(1–(ρw/ρs)); where Ww is buoyant weight of coral explant, ρw (g cm–3) is density of water used for buoyant weight measurement, and ρs (∼2.94 g cm–3) is density of pure aragonite (Jokiel et al., 1978; Davies, 1989; Langdon et al., 2010; Takahashi and Kurihara, 2013). The maximum photosynthetic efficiency of the photosystem II of zooxanthellae, Fv/Fm, was measured weekly (at days 0, 7, 14, 21, 28) through pulse-amplitude modulated (Diving PAM; Walz, Effeltrich, Germany) fluorometry (Schreiber et al., 1986). The explants were dark acclimated for 60 min prior to measurement (Ohki et al., 2013). To quantify zooxanthellae density (× 106 cell cm–2) (Nakamura et al., 2005), coral tissue was obtained from each explant through the water-pik method using 50 mL 0.2 μm filtered seawater (Johannes and Wiebe, 1970). A Neubauer hemocytometer (LeGresley and McDermott, 2010) was used to count zooxanthellae cells under a microscope. Only healthy-looking zooxanthellae were counted, while irregular-shaped and pale-colored cells were excluded. Cell counts were normalized to slurry volume (50 mL), and explant surface area was determined using the paraffin wax method (Stimson and Kinzie, 1991; Veal et al., 2010).
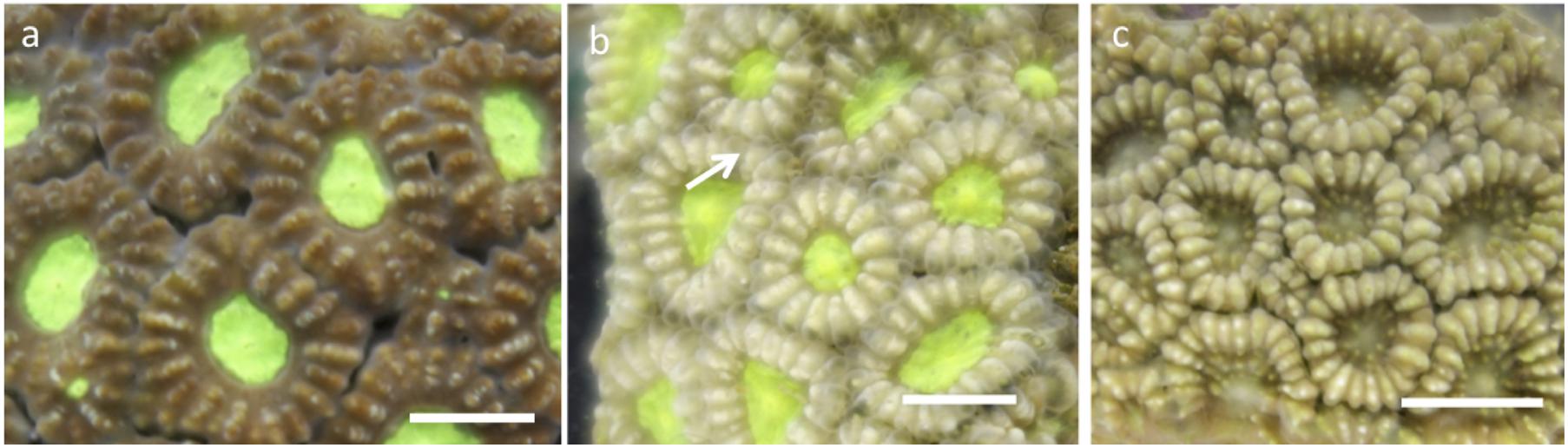
Figure 2. Survival of favites colemani explants: (a) alive: Unbleached with coral tissue, (b) alive: Bleached with coral tissue (c) dead: Bleached and necrosed (no coral tissue, skeleton exposed). Scale = 0.5 cm.
Zooxanthellae Collection and Identification
Prior to the start of the experiment, three explants per F. colemani colony were sampled, preserved in salt saturated DMSO (SSD) buffer (Gaither et al., 2011), and stored at 4°C. At the end of the experiment, explant tissue slurries (1–2 explant per colony per treatment) were pelletized and preserved in SSD. Zooxanthellae diversity was characterized through DGGE fingerprinting. Holobiont DNA was extracted using a modified organic DNA extraction method (Mieog et al., 2009). Following the touchdown thermal cycle protocol of LaJeunesse (2002), the zooxanthella-specific primers ITSintfor2 and the GC-attached ITS2clamp were used to amplify the internal transcribed spacer 2 region (ITS2, ∼350 bp) for downstream DGGE fingerprinting. All ITS2 PCR products (7 μL) were loaded 1:1 with 0.05% bromophenol blue-xylene cyanol solution into an 8% polyacrylamide (37.5:1 acrylamide/bis) denaturing gel with an internal gradient (30–60%) of denaturants (urea and formamide) and were separated by electrophoresis using DCode Universal Mutation Detection System (Bio-Rad) for 18 h at 100 V with a constant tank buffer temperature of 60°C. The acrylamide gels were post-stained with 1 × SYBR Green in 1 × TAE buffer for 30 min and photographed under UV illumination. A subset of prominent bands and likely heteroduplexes from randomly sampled explants were excised and incubated overnight with 500 μL nuclease-free water. Eluates were used as template for re-amplification of the ITS2 using the oligonucleotide primers ITSintfor2 and ITS2reverse following a similar thermal cycling protocol but without the touchdown cycles (LaJeunesse et al., 2010). Bidirectional DNA sequencing was performed (First Base Laboratories, Malaysia) and individual ITS2 sequences were manually trimmed, checked, and aligned using Geneious 6.1.8. Sequences were queried through BLAST (Altschul et al., 1990) in a custom zooxanthellae database from GeoSymbio (Franklin et al., 2012) and additional unique sequences obtained from GenBank (query: ‘‘Symbiodiniaceae’’ ‘‘ITS2’’) via the NCBI portal1.
Statistical Analyses
All data were tested for homogeneity of variances (Levene’s test) and normality (Shapiro-Wilk Test). On each pH-temperature treatment, differences in physico-chemical values between tank replicates were analyzed. The Kruskal-Wallis test was utilized for pH and temperature, and one-way analysis of variance (ANOVA) for pCO2 and aragonite saturation levels (Ωa). Kaplan–Meier model (Lee, 1992) survival analysis was used to investigate survival of F. colemani explants under pH-temperature treatments for 4 weeks. Pairwise comparisons of the survival curves were done using log-rank test (Mantel-Haenszel Test). RStudio (version 0.98.1102) software was used in the analyses. Permutational analysis of variance (PERMANOVA, Anderson, 2001) was used to investigate effects of pH-temperature treatments on growth rate, zooxanthellae density, and maximum photosynthetic efficiency of F. colemani explants. Two-way PERMANOVA was used on growth rates and zooxanthellae densities. The fixed factors in the design were pH (two levels), and temperature (two levels). Three-way PERMANOVA was used on maximum photosynthetic efficiencies. Fixed factors were pH (two levels), temperature (two levels), and time (five levels). Euclidean distances were used for all permutational analyses. Analyses were followed by a posteriori pairwise test when significant values were obtained. This was done to determine differences between levels within a factor. PRIMER 7 and PERMANOVA + 1 version 7.0.11 software were used for these statistical analyses.
Results
Physico-Chemical Parameters
Seawater mean pH and temperature values measured during the experiment are given in Table 1 for the following treatments: C (pH 8.02 ± 0.05, 27.91 ± 0.61°C), OA (pH 7.74 ± 0.05, 27.91 ± 0.60°C), T (pH 8.00 ± 0.05, 32.01 ± 0.43°C), and OAT (pH 7.72 ± 0.05, 32.00 ± 0.45°C). The pCO2 increased, and aragonite saturation levels (Ωa) decreased at low pH in all temperature treatments. pH, temperature, pCO2, and Ωa showed non-significant differences between replicates in each pH-temperature treatment (Supplementary Table 1).

Table 1. Physico-chemical values (mean ± SD) of pH-temperature treatments for 28 days; pH (n = 255), temperature (n = 8064), partial pressure of carbon dioxide (pCO2; n = 15), aragonite saturation levels (Ωa; n = 15).
Survival
The survival of F. colemani explants after 28 days of exposure under both C and OA treatments was 100 ± 0%. Bleaching was only observed under T and OAT treatments. The survival of coral explants under both T and OAT treatments was 66.7 ± 12.17% (Figure 3 and Supplementary Table 2), and mortalities were observed on the 3rd and 4th weeks of exposure. Temperature (χ2 = 11.8; P < 0.001) significantly influenced coral survival. Coral survival was also affected by a decrease in seawater pH but only when this co-occurred with elevated temperature (χ2 = 11.8; P < 0.001) and longer exposure (χ2 = 59.1; P < 0.001) (Table 2).
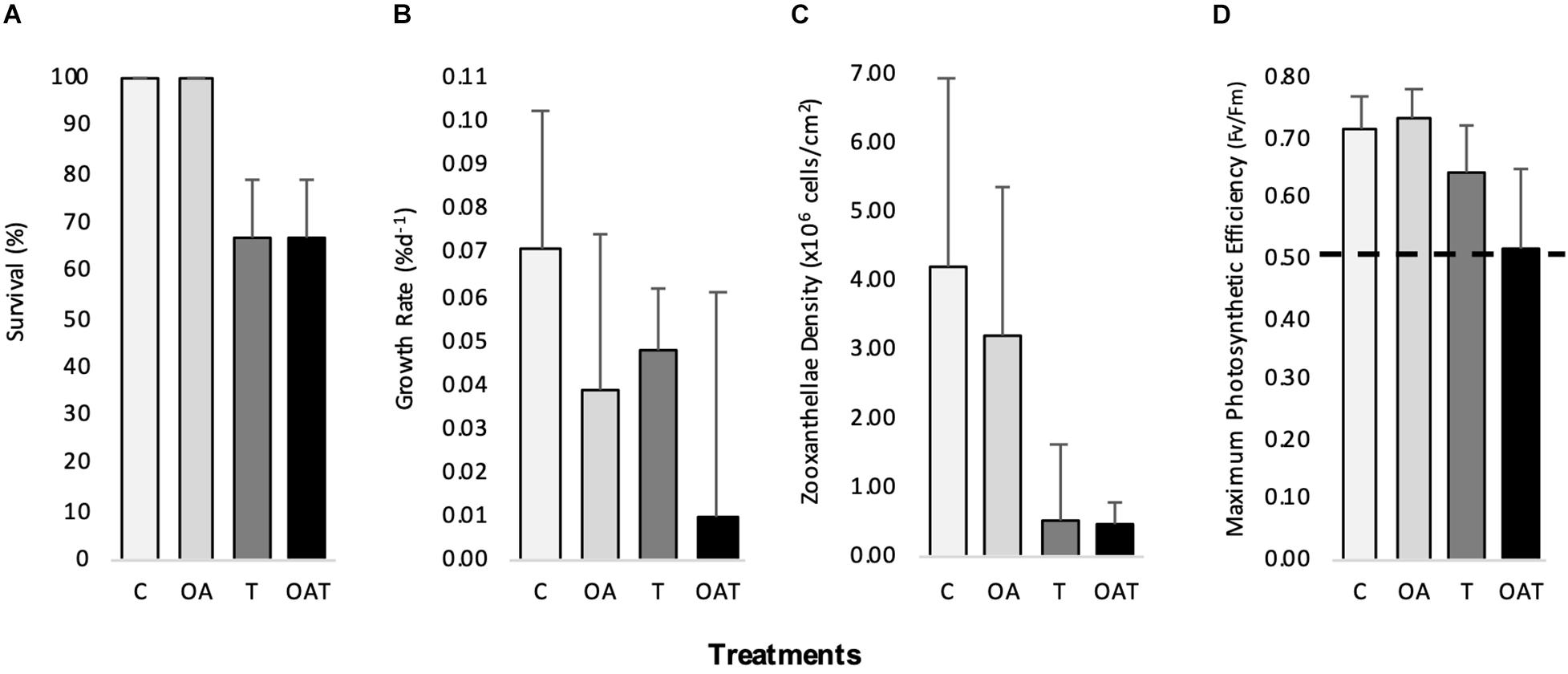
Figure 3. Physiological response (mean ±SD) of Favites colemani after 28 days exposure to pH-temperature stress. (A) survival (B) growth rate (C) zooxanthellae density (D) maximum photosynthetic efficiency (values above horizontal dashed line mean photosynthetically competent). (n = 15 for survival in OA, T, OAT; n = 15 for growth rate, zooanthellae density, maximum photosynthetic efficiency in C, OA, and n = 10 in T, OAT).
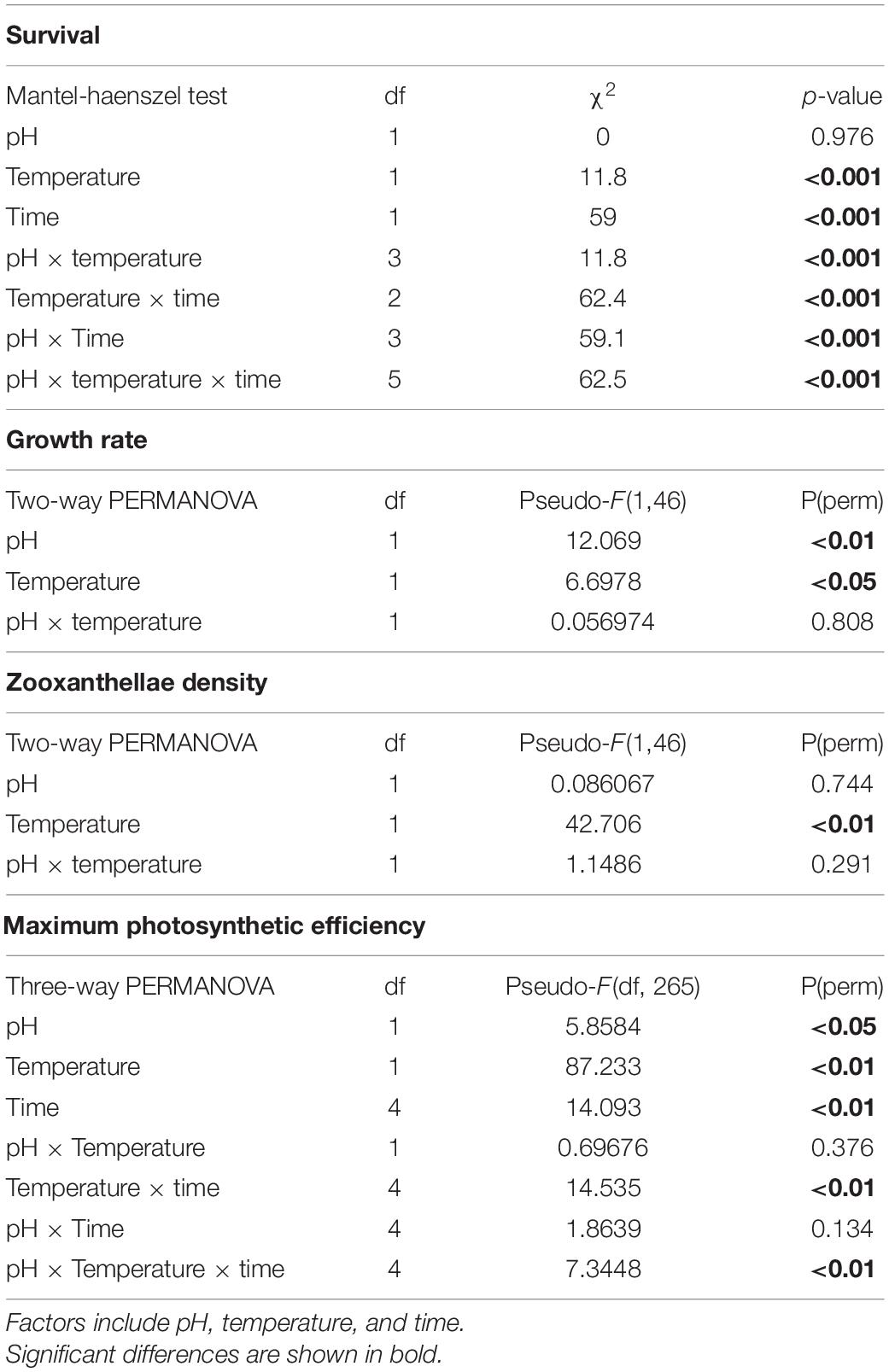
Table 2. Summary of statistical analyses on Favites colemani physiological response under pH-temperature treatments for 28 days.
Effect on Growth Rate
The growth rate of F. colemani explants after 28 days of exposure under C was 0.071 ± 0.031% d–1. Growth rates under both OA (0.038 ± 0.036% d–1) and T (0.047 ± 0.011% d–1) treatments were lower compared to C. The lowest growth rate was seen under OAT (0.010 ± 0.051% d–1) treatment (Figure 3 and Supplementary Table 2). Based on PERMOVA, pH [F(1, 46) = 12.069, P = 0.002] and temperature [F(1, 46) = 6.6978, P = 0.016] significantly affected F. colemani’s growth rate, however, the synergistic effect of pH and temperature [F(1, 46) = 0.0570, P = 0.808] was non-significant (Table 2). Posteriori pairwise test showed a significant decline in growth rate under treatments with either increased temperature alone (P = 0.001) or in combination with decreased pH (P = 0.002) (Supplementary Table 3).
Effect on Maximum Photosynthetic Efficiency (Fv/Fm)
The maximum photosynthetic efficiency of F. colemani explants after 28 days of exposure to OA (0.735 ± 0.045, mean ± SD) was comparable to C (0.713 ± 0.058), but lower values were observed under T (0.642 ± 0.079) and OAT (0.517 ± 0.129) (Figure 3 and Supplementary Table 2). Based on three-way PERMANOVA, the Fv/Fm of the coral explants was significantly affected by pH [F(1, 265) = 5.8584, P = 0017], temperature [F(1, 265) = 87.233, P = 0.001], and duration of exposure to treatments [F(4, 46) = 14.093, P = 0.001; Table 2]. Significant decline in Fv/Fm was observed starting week 2 for T (P = 0.001) and OAT (P = 0.001). This significant decline persisted in week 3 (T: P = 0.001, OAT T: P = 0.001) to week 4 (T: P = 0.002, OAT T: P = 0.001) (Supplementary Table 3). The maximum photosynthetic efficiencies of the coral explants were above 0.5 and remained photosynthetically competent even after exposure to stress treatments (>0.5; Kavousi et al., 2015).
Zooxanthellae Density and Diversity
The zooxanthellae density of F. colemani explants after 28 days of exposure under control conditions was 4.211 ± 2.743 × 106 cells cm–2 (mean ± SD). Decline in zooxanthellae densities was observed under OA (3.208 ± 2.146 × 106 cells cm–2), T (0.492 ± 1.122 × 106 cells cm–2), and OAT (0.476 ± 0.280 × 106 cells cm–2) treatments (Figure 3 and Supplementary Table 2). Based on two-way PERMANOVA, zooxanthellae density was not significantly affected by pH alone [F(1, 46) = 0.0861, P = 0.744], and by interaction of pH and temperature [F(1, 46) = 1.1486, P = 0.291] but by seawater temperature [F(1, 46) = 42.706, P = 0.001; Table 2]. Posteriori pairwise test showed a significant decline in zooxanthellae density under treatments with increased temperature (P = 0.001) (Supplementary Table 3). All pre- and post-experiment explants processed for zooxanthellae clade identification showed the occurrence and possible dominance of Cladocopium sp. clade C3u (formerly a subclade of Symbiodinium) in all F. colemani samples (GenBank Accession Nos. MN031741–MN031752; Supplementary Figure 1).
Discussion
Tropical corals exist in marine environments where natural habitat temperature variabilities are close to their physiological limits. Further increase in temperatures of up to 2°C due to climate change could therefore be intolerable for these organisms and pose “dangerous” climatic and ecological consequences (Somero, 2010; Hennige et al., 2014; Elder and Seibel, 2015). Coral bleaching is highly associated with increase in seawater temperatures (Glynn, 1993; McClanahan, 2004; Hoegh-Guldberg et al., 2007; Gattuso et al., 2014). During 1997–1998 El Niño event, 80% of BARC reefs bleached following a prolonged exposure (7 months) to a major “hotspot” (>1 thermal anomaly above expected max monthly mean SST) in the region, which included submassive and massive corals that are generalized as less susceptible to bleaching (Arceo et al., 2001). Although signaling events that lead to bleaching are partially understood, there is strong evidence that oxidative stress plays a critical role in the host-bleaching cascade (Weis, 2008). However, bleaching may not occur if the coral is able to recover from stress (Goreau et al., 2000; Kavousi et al., 2015). Favites spp. colonies in Kenya (McClanahan, 2004), and F. colemani colonies in BARC (Maboloc et al., 2015) both exhibited post-bleaching survival after the 1997–1998 El Niño event. The survival of F. colemani in this study was also high (66.7 ± 12.3%) under both T and OAT treatments, where the temperature used was 4°C above the annual mean, and 2°C above the maximum monthly mean (30.64 ± 0.79°C; Maboloc et al., 2015) SST in Bolinao. This was also observed during the 2016 thermal bleaching event where no F. colemani colonies in the study site was recorded to have bleached. The 2016 thermal stress began around mid-April to May and peaked during the months of June to July. During this event, sea surface temperatures (SST) in Bolinao rose to 1–2°C above the maximum monthly mean SST (29–30°C). Degree Heating Weeks (DHW) since the onset of warming lasted for about 11–12 weeks. This event caused coral bleaching but with low prevalence (<25%) at the BARC where majority (77%) of the corals comprise five genera (Dipsastrea, Porites, Fungia, Seriatopora, and Montipora) (Quimpo et al., 2020).
Facing the South China Sea, reefs in the BARC experience high temperature variations (Peñaflor et al., 2009; Guzman and Conaco, 2016). Annual mean seawater temperature in Bolinao is 28–29°C (Guzman and Conaco, 2016), while max monthly mean in the Lucero reef can reach 30–31°C (Maboloc et al., 2015). Dry-warm months in the Philippines occur in April–May. Monthly seawater temperatures from December to March (dry-cold months) range from 27 to 28°C. In situ spawning of F. colemani occurs between full moon and last quarter of May, the warmest month of the year (Maboloc et al., 2015). Lucero reef is adjacent to the Guiguiwanen channel, a designated mariculture area where fish farming of Chanos chanos has intensified since it started in the 1990s (San Diego-McGlone et al., 2008). The reef may be exposed to seawater with lower pH levels due to seasonal outflow of mariculture effluent from the channel. Lagumen (2017) observed that expansion of fish farming activities caused a decrease of seawater pH by ∼0.2–0.3 units in the mariculture area. The diurnal pH range in the reef adjacent to the channel (mariculture area) was lower during dry (pH 7.78–8.22, pCO2 235–849) compared to the wet (pH 7.96–8.14, pCO2 305–518) periods. Prior in situ exposure of F. colemani to lowered pH and elevated temperature could be the reason why explants had no mortality after a 28-days exposure to C and OA treatments, which suggests that they may have locally adapted to wide pH and thermal limits.
Corals’ ability to sustain calcification rates that can withstand natural forces of biological and physical erosion is a fundamental aspect in maintenance of reef ecosystems. However, this ability may be compromised by exposure to warmer and acidified waters (Doney et al., 2012; Lough and Cantin, 2014). Our understanding of factors that drive coral calcification is still rudimentary (Tambutte et al., 2011). In this study, F. colemani growth rates significantly declined under OA and T treatments. Some studies suggest that coral growth rates may be adversely affected by seawater pH decrease (Anthony et al., 2008; Doney et al., 2009; Feely et al., 2009; Tanzil et al., 2009), and seawater temperature increase (Leder et al., 1991; Tanzil et al., 2009; Cantin et al., 2010). Scleractinian tropical corals existing near low-pH springs (e.g., Hawaii) or submarine groundwater discharge (Mexico) experience lower tissue density and higher bioerosion rates (Crook et al., 2012; Lubarsky et al., 2018). The decline in growth rate under the OA and T treatments seen in this study may be due to mechanisms that trade off calcification processes in favor of generating more tissue biomass and energy reserves (Strahl et al., 2016). The coral’s capability to retain calcification rates may be affected by stress-induced damages that occur inside coral cell (mitochondria) and zooxanthellae (chloroplast). This further leads to high production of reactive oxygen species (ROS) and reactive nitrogen species (RNS) that may overwhelm oxygen-handling pathways (Venn et al., 2008; Weis, 2008). Consequently, host DNA (Lesser and Farrell, 2004), protein, and membrane (Richier et al., 2005) become damaged. Increased concentrations of the RNS nitric oxide may also initiate an apoptotic cascade leading to coral bleaching (Perez and Weis, 2006). While ocean acidification may be associated to decline in coral calcification, the mechanisms underlying its influence on coral growth remain unclear (Venn et al., 2013). Corals have ectodermis and gastrodermis tissue layers that fold over each other to form the gastrovascular cavity known as coelenteron. Among these extra- and intracellular coral compartments, there is wide carbon chemistry and pH variability (Barott et al., 2017). For instance, the coral ectodermis has pH ranging between 7.9 and 8.3, the gastrodermal cells symbiosome has a pH of around 4, the sub-calicoblastic medium (SCM) has pH ranging between 8.2 and 10, and the coelenteron has pH ranging between 6.6 and 8.5 (Al-Horani et al., 2003; Agostini et al., 2011; McCulloch et al., 2012; Cai et al., 2016). Corals and other coral reef organisms modulate the chemistry of their calcifying fluids (CF) and may override changes in the chemistry of the seawater source to the site of calcification (Cohen and Holcomb, 2009; Cohen et al., 2009). One hypothesis (Kaniewska et al., 2012) suggests that exposure to low pH may overwhelm the coral’s acid-base regulation and cell membrane transporters and may lead to cell acidosis. This pH imbalance then causes damage to both the coral cell and its algal symbiont, leading to increase in ROS and RNS and the disruption of symbiosis. Another consequence of this acidosis is calcium ion (Ca2+) imbalance resulting from an increase in ROS and Ca2+ ion storage disruption in the endoplasmic reticulum. This may then lead to changes in the extracellular matrix, cytoskeletal remodeling, changes in cytoskeletal interactions, disruptions in cell reception and signaling potential, and increased cell death. A study on Stylophora pistillata colonies (Venn et al., 2013) saw reductions in calcification rate only when pH was significantly low in the calcifying cells in addition to the SCM. This highlights the roles of SCM pH regulation and calcifying cell pH homeostasis in the coral’s response to ocean acidification. According to McCulloch et al. (2017), carbonate saturation state in the calcifying fluid (OCF) which is the key driver of calcification, is maintained elevated but at near-constant levels by seasonally varying supply of summer-enhanced metabolic dissolved inorganic carbon (DICCF), and dynamic out-of-phase upregulation of pHCF. In the advent of extreme thermal stress, OCF becomes unstable, and this leads to termination of calcification. As observed in this study, F. colemani exhibited lower growth rates under OA, T, and OAT treatments, and decline in zooxanthellae densities were likewise recorded.
Coral growth rates after bleaching events may decline, experience setbacks, or cease altogether (Cooper et al., 2008; Carilli et al., 2009), but the surviving corals may still recover to pre-bleaching growth rates after some years (Lough and Cantin, 2014). Based on the study by Ogawa et al. (2013), corals exhibit differential expression of candidate calcification genes after prolonged exposure to combined pH-temperature stress. In this study, decreased pH and increased seawater temperature have additive adverse effect on F. colemani’s growth rates, with lowest values observed under OAT. This suggests that while F. colemani exhibited post-bleaching recovery in the past (McClanahan, 2004; Maboloc et al., 2015), adverse effects on its growth, distribution, and post-bleaching recovery may be aggravated in a future scenario of co-occurring ocean warming and acidification.
The divergent responses of corals under stressed scenarios are likely reflective of the holobiont’s stress response machinery, as well as the variable interactions between the host and its symbionts. It is probable that the coral’s stress response is influenced by its zooxanthellae and their photosynthetic capacity. The disruption of the coral-zooxanthellae relationship and reduction in its photochemical efficiencies are potentially driven by decline in seawater pH and are highly correlated with elevated temperatures (McClanahan, 2004; Hoegh-Guldberg et al., 2007; Anthony et al., 2008; Weis, 2008; Gattuso et al., 2014; Davies et al., 2018). In this study, seawater temperature dominantly influenced F. colemani’s zooxanthellae density. Cell damage and death may occur due to increase in ROS and RNS during thermal stress and cell acidosis (Richier et al., 2005; Weis, 2008; Kaniewska et al., 2012). High temperatures may cause damage to zooxanthellae’s photosynthetic apparatus at photosystem II, the thylakoid membranes, and the Calvin cycle (Lesser, 1996; Weis, 2008). As a stress defense mechanism, the observed decline in zooxanthellae densities under T, and OAT treatments may have resulted from the coral’s active digestion and selective expulsion of damaged or incompetent symbiont cells (Lesser, 1996, 1997; Downs et al., 2002; Smith et al., 2005; Venn et al., 2008; Weis, 2008). Similarly, seawater pH and temperature additively affected Fv/Fm of F. colemani explants. Despite the recorded decline in zooxanthellae density, F. colemani explants remained photosynthetically competent (>0.5; Kavousi et al., 2015) under T, and OAT treatments. Corals expel and digest damaged cells also as a regulatory means to maintain constant symbiont density and stable carbon concentration within their tissues (Downs et al., 2009; Fujise et al., 2014). In some cases, corals under stressed treatments may also expel morphologically normal but photosynthetically incompetent/damaged symbiont cells as a stress coping mechanism (Lesser, 1997; Fujise et al., 2014). One study revealed that under elevated temperatures, two species of scleractinian corals (Acroporidae) sustained high maximum photosynthetic efficiency (Fv/Fm) values and expelled zooxanthellae cells that are morphologically normal with low Fv/Fm values (Fujise et al., 2014). Massive corals such as F. colemani may likely be provided with photoprotection by its thicker tissues and high levels of stored energy (Thornhill et al., 2011; Grottoli et al., 2014; Strahl et al., 2016).
Coral-zooxanthellae associations can influence the adaptation of corals and the maintenance of coral reefs. For instance, bleaching presents an opportunity for some coral taxa to undergo “shuffling” of dominant zooxanthellae types to more tolerant species as an adaptation to current environmental conditions (Gates and Edmunds, 1999; Kinzie, 1999). Some corals can improve their thermal tolerance through switching their dominant symbiont type from Cladocopium sp. to the generally more tolerant Durusdinium sp. (Fabricius et al., 2004; LaJeunesse et al., 2004; Berkelmans and van Oppen, 2006; Stat and Gates, 2011). In this study, however, no “shuffling” of dominant symbionts was observed, as F. colemani colonies maintained their hosting Cladocopium clade C3u before and after a 28-day exposure to pH-temperature treatments. While it is possible that the DGGE may have not detected rare symbionts (Da-Anoy et al., 2019), one likely reason for this response is its probable adaptation to environmental changes via other mechanisms. For one, host genotype may play a key role on varying physiological responses (Parkinson et al., 2015). In the same way, different zooxanthellae species or clades, as they are sometimes physiologically adapted to certain environments, may likewise vary in response to a stressor (Fabricius et al., 2004; Jones et al., 2008; Brading et al., 2011). Highly stable coral-zooxanthellae symbiotic associations could be observed in some coral species throughout bleaching events, after transplantation, and over wide geographical and temperature ranges (LaJeunesse et al., 2004; Stat et al., 2009). For instance, the Cladocopium clade C15 was hosted by Porites lobata colonies found on both fore reefs and warmer more variable back reefs in American Samoa (Barshis et al., 2010). Cladocopium thermophilum (C3-Gulf) dominated corals found in Persian/Arabian Gulf, one of the hottest environments in the world (Hume et al., 2013). Clade C1 also exhibits local adaptation to high temperatures (Howells et al., 2011) and thermal stress resistance due to acclimation of the coral holobiont (Bellantuono et al., 2012; Palumbi et al., 2014). Another study has also observed F. colemani hosting C3u symbionts but showed less apparent reduction in Fv/Fm and symbiont density after a 72-h exposure to thermal stress (Da-Anoy et al., 2019). In this current study, that F. colemani harbors Cladocopium clade C3u through the month-long experiment could either indicate the coral host’s sufficient physiological adaptation to local environmental temperature and pH variations (Berkelmans and van Oppen, 2006; Noonan et al., 2013) as a result of recurring exposure to local stress (Thornhill et al., 2011; Grottoli et al., 2014), or the zooxanthellae clade’s resistance to the stressors being investigated. Either way, more genetic and physiological investigations are still needed to unveil host and host-symbiont responses as well as other adaptive mechanisms to ocean warming and acidification.
Conclusion
The physiological responses of F. colemani in this study indicate that this coral may have lower growth rates and yet still survive projected near-future ocean warming and acidification scenarios. This supports the hypothesis that F. colemani’s pH-thermal stress tolerance is a result of possible acclimation and adaptation to local environmental conditions and past bleaching events. This study adds to the limited knowledge on how Philippine corals would respond to independent and interactive effects of pH and temperature stress. The study highlights the significance of exploring individual and integrative effects of climate-driven stressors on Philippine reef building corals as a guide for future conservation and restoration efforts in the changing ocean. Studies on coral physiological responses at earlier life stages and finer genetic investigations on the host and its symbionts are warranted to better understand local acclimation, adaptation, and stress tolerance to ocean warming and acidification.
Data Availability Statement
The sequence data that support the findings of this study have been deposited in GenBank with the accession numbers: MN031741–MN031752.
Author Contributions
MT, RV, RR-G, and MS-M contributed to conception and design of the study. MT and AT performed the experiments. MT and MS-M analyzed the data. RV, RR-G, and MS-M provided supervision. MT wrote the original draft of the manuscript. MT, AT, RR-G, and MS-M reviewed and edited the manuscript. All authors contributed to the article and approved the submitted version.
Funding
This study was supported by the Department of Science and Technology—Philippine Council for Agriculture, Aquatic, and Natural Resource Research and Development (DOST-PCAARRD) through the project “Identifying resilient coral species” under the program on Molecular genetic and genomic studies of coral resilience in support of coral restoration and rehabilitation efforts (Project Code: QSR-MR-COR.02.01).
Conflict of Interest
The authors declare that the research was conducted in the absence of any commercial or financial relationships that could be construed as a potential conflict of interest.
Publisher’s Note
All claims expressed in this article are solely those of the authors and do not necessarily represent those of their affiliated organizations, or those of the publisher, the editors and the reviewers. Any product that may be evaluated in this article, or claim that may be made by its manufacturer, is not guaranteed or endorsed by the publisher.
Acknowledgments
We are grateful to the Marine Biogeochemistry Laboratory and Bolinao Marine Laboratory of the Marine Science Institute, University of the Philippines for the valuable logistical and laboratory support provided. We would also like to thank Drs. Haruko Kurihara, Atsushi Watanabe, and Toshihiro Miyajima for the design of the mass flow controller used in the experiments. This is UP-MSI contribution number 484.
Supplementary Material
The Supplementary Material for this article can be found online at: https://www.frontiersin.org/articles/10.3389/fmars.2021.704487/full#supplementary-material
Footnotes
References
Agostini, S., Suzuki, Y., Higuchi, T., Casareto, B. E., Yoshinaga, K., Nakano, Y., et al. (2011). Biological and chemical characteristics of the coral gastric cavity. Coral Reefs 31, 147–156. doi: 10.1007/s00338-011-0831-6
Al-Horani, F. A., Al-Moghrabi, S. M., and De Beer, D. (2003). The mechanism of calcification and its relation to photosynthesis and respiration in the scleractinian coral Galaxea fascicularis. Mar. Biol. 142, 419–426. doi: 10.1007/s00227-002-0981-8
Altschul, S. F., Gish, W., Miller, W., Myers, E. W., and Lipman, D. J. (1990). Basic local alignment search tool. J. Mol. Biol. 215, 403–410.
Anderson, M. J. (2001). A new method for non-parametric multivariate analysis of variance. Austral. Ecol. 26, 32–46. doi: 10.1111/j.1442-9993.2001.01070.pp.x
Andersson, A. J., and Gledhill, D. (2013). Ocean acidification and coral reefs: effects on breakdown, dissolution, and net ecosystem calcification. Annu. Rev. Mar. Sci. 5, 321–348. doi: 10.1146/annurev-marine-121211-172241
Anthony, K. R. N., Kline, D. I., Díaz-Pulido, G., Dove, S., and Hoegh-Guldberg, O. (2008). Ocean acidification causes bleaching and productivity loss in coral reef builders. Proc. Natl. Acad. Sci. 105, 17442–17446. doi: 10.1073/pnas.0804478105
Arceo, H. O., Quibilan, M. C., Aliño, P. M., Lim, G., and Licuanan, W. Y. (2001). Coral bleaching in Philippine reefs: Coincident evidences with meso-scale thermal anomalies. B. Mar. Sci. 69, 579–593.
Baria, M. V. B. (2016). The impacts of elevated temperature and ocean acidification on spawning and early life stages of corals. PhD Ryukyus: University of Ryukyus.
Barott, K. L., Barron, M. E., and Tresguerres, M. (2017). Identification of a molecular pH sensor in coral. P. Roy. Soc. B. 284:1866. doi: 10.1098/rspb.2017.1769
Barshis, D. J., Stillman, J. H., Toonen, R. J., Smith, L. W., and Birkeland, C. (2010). Protein expression and genetic structure of the coral Porites lobata in an environmentally extreme Samoan back reef: does host genotype limit phenotypic plasticity? Mol. Ecol. 19, 1705–1720. doi: 10.1111/j.1365-294X.2010.04574.x
Bellantuono, A. J., Hoegh-Guldberg, O., and Rodriguez-Lanetty, M. (2012). Resistance to thermal stress in corals without changes in symbiont composition. Proc. R. Soc. B 279, 1100–1107. doi: 10.1098/rspb.2011.1780
Berkelmans, R., and van Oppen, M. J. H. (2006). The role of zooxanthellae in the thermal tolerance of corals: a “nugget of hope” for coral reefs in an era of climate change. Proc. Royal Soc. B. 273, 2305–2312. doi: 10.1098/rspb.2006.3567
Brading, P., Warner, M. E., Davey, P., Smith, D. J., Achterberg, E. P., and Suggett, D. J. (2011). Differential effects of ocean acidification on growth and photosynthesis among phylotypes of Symbiodinium (Dinophyceae). Limnol. Oceanogr. 56, 927–938. doi: 10.4319/lo.2012.57.4.1255
Burke, L., Reytar, K., Spalding, M., and Perry, A. (2011). Reefs at risk revisited. Washington, DC: World Resources Institute, 114.
Cai, W. J., Ma, Y., Hopkinson, B. M., Grottoli, A. G., Warner, M. E., Ding, Q., et al. (2016). Microelectrode characterization of coral daytime interior pH and carbonate chemistry. Nat. Commun. 7:11144. doi: 10.1038/ncomms11144
Cantin, N. E., Cohen, A. L., Karnauskas, K. B., Tarrant, A. M., and McCorkle, D. C. (2010). Ocean warming slows coral growth in the central Red Sea. Science 329, 322–325. doi: 10.1126/science.1190182
Carilli, J. E., Norris, R. D., Black, B. A., Walsh, S. M., and McField, M. (2009). Local stressors reduce coral resilience to bleaching. PLoS One 4:e6324. doi: 10.1371/journal.pone.0006324
Chou, L. M., Tuan, V. S., Philreefs, Yeemin, T., Cabanban, A., Suharsono, et al. (2002). “Status of Southeast Asia coral reefs,” in Status of Coral Reefs of the World. GCRMN Report, Vol. 7, ed. C. R. Wilkinson (Townsville: Australian Institute of Marine Science), 123–152.
Cohen, A. L., and Holcomb, M. (2009). Why corals care about ocean acidification: uncovering the mechanism. Oceanography 22, 118–127. doi: 10.5670/oceanog.2009.102
Cohen, A. L., McCorkle, D. C., de Putron, S., Gaetani, G. A., and Rose, K. A. (2009). Morphological and compositional changes in the skeletons of new coral recruits reared in acidified seawater: insights into the biomineralization response to ocean acidification. Geochem. Geophy. Geosy. 10:Q07005. doi: 10.1029/2009GC002411
Conservation International. (2008). Economic values of coral reefs, mangroves, and seagrasses: a global compilation. Arlington, VA: Center for Applied Biodiversity Science, Conservation International.
Cooper, T. F., De’Ath, G., Fabricius, K. E., and Lough, J. M. (2008). Declining coral calcification in massive Porites in two nearshore regions of the northern Great Barrier Reef. Glob. Change. Biol. 14, 529–538. doi: 10.1111/j.1365-2486.2007.01520.x
Cornwall, E. C., and Hurd, C. L. (2015). Experimental design in ocean acidification research: problems and solutions. ICES J. Mar. Sci. 73, 572–581. doi: 10.1093/icesjms/fsv118
Cruz-Trinidad, A., Geronimo, R. C., Cabral, R. B., and Aliño, P. M. (2011). How much are the Bolinao-Anda coral reefs worth? Ocean. Coast. Manag. 54, 696–705. doi: 10.1016/j.ocecoaman.2011.07.002
Crook, E. D., Potts, D., Rebolledo-Vieyra, M., Hernandez, L., and Paytan, A. (2012). Calcifying coral abundance near low-pH springs: implications for future ocean acidification. Coral Reefs 31, 239–245. doi: 10.1007/s00338-011-0839-y
Da-Anoy, J. P., Cabaitan, P. C., and Conaco, C. (2019). Species variability in the response to elevated temperature of select corals in north-western Philippines. J. Mar. Biol. Assoc. 2019, 1–7. doi: 10.1017/S0025315419000158
Davies, P. S. (1989). Short-term growth measurements of corals using an accurate buoyant weighing technique. Mar. Biol. 101, 389–395. doi: 10.1007/BF00428135
Davies, S. W., Ries, J. B., Marchetti, A., and Castillo, K. D. (2018). Symbiodinium functional diversity in the coral Siderastrea siderea is influenced by thermal stress and reef environment, but not ocean acidification. Front. Mar. Sci. 5:150. doi: 10.3389/fmars.2018.00150
Doney, S. C., Fabry, V. J., Feely, R. A., and Kleypas, J. A. (2009). Ocean acidification: the other CO2 problem. Annu. Rev. Mar. Sci. 1, 169–192. doi: 10.1146/annurev.marine.010908.163834
Doney, S. C., Ruckelshaus, M., Duffy, J. E., Barry, J. P., Chan, F., English, C. A., et al. (2012). Climate change impacts on marine ecosystems. Annu. Rev. Mar. Sci. 4, 11–37. doi: 10.1146/annurev-marine-041911-111611
Downs, C. A., Fauth, J. E., Halas, J. C., Dustan, P., Bemiss, J., and Woodley, C. M. (2002). Oxidative stress and seasonal coral bleaching. Free. Radical. Biol. Med. 33, 533–543. doi: 10.1016/S0891-5849(02)00907-3
Downs, C. A., Kramarsky-Winter, E., Martinez, J., Kushmaro, A., Woodley, C. M., Loya, Y., et al. (2009). Symbiophagy as a cellular mechanism for coral bleaching. Autophagy 5, 211–216. doi: 10.4161/auto.5.2.7405
Elder, L. E., and Seibel, B. A. (2015). The thermal stress response to diel vertical migration in the hyperiid amphipod Phronimase dentaria. Comp. Biochem. Physiol., Part A Mol. Integr. Physiol. 187, 20–26. doi: 10.1016/j.cbpa.2015.04.008
Escobar, M. T. L., Sotto, L. P. A., Jacinto, G. S., Benico, G. A., San Diego-McGlone, M. L., and Azanza, R. V. (2013). Eutrophic conditions during the 2010 fish kill in Bolinao and Anda, Pangasinan, Philippines. J. Environ. Sci. Manag. Spec. Issue. 1, 29–35.
Fabricius, K. E., Mieog, J. C., Colin, P. L., Idip, D., and van Oppen, M. J. H. (2004). Identity and diversity of coral endosymbionts (zooxanthellae) from three Palauan reefs with contrasting bleaching, temperature and shading histories. Mol. Ecol. 13, 2445–2458. doi: 10.1111/j.1365-294X.2004.02230.x
Feely, R. A., Doney, S. C., and Cooley, S. R. (2009). Ocean acidification: present conditions and future changes in a high-CO2 world. Oceanography. 22:4. doi: 10.5670/oceanog.2009.95
Franklin, E. C., Stat, M., Pochon, X., Putnam, H. M., and Gates, R. D. (2012). GeoSymbio: a hybrid, cloud−based web application of global geospatial bioinformatics and ecoinformatics for Symbiodinium–host symbioses. Mol. Ecol. Resour. 12, 369–373. doi: 10.1111/j.1755-0998.2011.03081.x
Fujise, L., Yamashita, H., Suzuki, G., Sasaki, K., Liao, L. M., and Koike, K. (2014). Moderate thermal stress causes active and immediate expulsion of photosynthetically damaged zooxanthellae (Symbiodinium) from corals. PLoS One 9:e114321. doi: 10.1371/journal.pone.0114321
Gaither, M. R., Szabó, Z., Crepeau, M. W., Bird, C. E., and Toonen, R. J. (2011). Preservation of corals in salt-saturated DMSO buffer is superior to ethanol for PCR experiments. Coral Reefs 30, 329–333. doi: 10.1007/s00338-010-0687-1
Gates, R. D., and Edmunds, P. J. (1999). The physiological mechanisms of acclimatization in tropical reef corals. Am. Zool. 39, 30–43. doi: 10.1093/icb/39.1.30
Gattuso, J. P., Hoegh-Guldberg, O., and Pörtner, H. O. (2014). “Cross-chapter box on coral reefs,” in Climate Change 2014: Impacts, Adaptation, and Vulnerability Part A: Global and Sectoral Aspects Contribution of Working Group II to the Fifth Assessment Report of the Intergovernmental Panel of Climate Change, eds C. B. Field, V. R. Barros, D. J. Dokken, K. J. Mach, M. D. Mastrandrea, and T. E. Bilir (New York, NY: Cambridge University Press), 97–100.
Glynn, P. W. (1993). Coral reef bleaching: ecological perspectives. Coral Reefs 12, 1–17. doi: 10.1007/BF00303779
Gomez, E. D., Aliño, P. M., Yap, H. T., and Licuanan, W. Y. (1994). A review of the status of Philippine reefs. Mar. Pollut. Bull. 29, 62–68. doi: 10.1016/0025-326X(94)90427-8
Goreau, T., McClanahan, T., Hayes, R., and Strong, A. L. (2000). Conservation of coral reefs after the 1998 global bleaching event. Conserv. Biol. 14, 5–15. doi: 10.1046/j.1523-1739.2000.00011.x
Grottoli, A. G., Rodrigues, L. J., and Juarez, C. (2004). Lipids and stable carbon isotopes in two species of Hawaiian corals, Porites compressa and Montipora verrucosa, following a bleaching event. Mar. Biol. 145, 621–631. doi: 10.1007/s00227-004-1337-3
Grottoli, A. G., Warner, M. E., Levas, S. J., Aschaffenburg, M. D., Schoepf, V., Baumann, J., et al. (2014). The cumulative impact of annual coral bleaching can turn some coral species winners into losers. Glob. Chang. Biol. 20, 3823–3833. doi: 10.1111/gcb.12658
Gruber, N., Clement, D., Carter, B. R., Feely, R. A., van Heuven, S., Hoppema, M., et al. (2019). The oceanic sink for anthropogenic CO2 from 1994 to 2007. Science 363, 1193–1199. doi: 10.1126/science.aau5153
Guzman, C., and Conaco, C. (2016). Gene expression dynamics accompanying the sponge thermal stress response. PLoS One 11:e0165368. doi: 10.1371/journal.pone.0165368
Hennige, S., Roberts, J. M., and Williamson, P. (eds) (2014). An updated synthesis of the impacts of ocean acidification on marine biodiversity. Technical Series No. 75, Montreal: Secretariat of the Convention on Biological Diversity, 99.
Hoegh-Guldberg, O., Mumby, P. J., Hooten, A. J., Steneck, R. S., Greenfield, P., Gomez, E., et al. (2007). Coral reefs under rapid climate change and ocean acidification. Science 318, 1737–1742. doi: 10.1126/science.1152509
Hoegh-Guldberg, O., Poloczanska, E. S., Skirving, W., and Dove, S. (2017). Coral reef ecosystems under climate change and ocean acidification. Front. Mar. Sci. 4:158. doi: 10.3389/fmars.2017.00158
Howells, J. E., Beltran, V. H., Larsen, N. W., Bay, L. K., Willis, B. L., and van Oppen, M. J. H. (2011). Coral thermal tolerance shaped by local adaptation of photosymbionts. Nat. Clim. Change. 2, 116–120. doi: 10.1038/nclimate1330
Hume, B., D’Angelo, C., Burt, J., Baker, A. C., Riegl, B., and Wiedenmann, J. (2013). Corals from the Persian/Arabian Gulf as models for thermotolerant reef-builders: Prevalence of clade C3 Symbiodinium, host fluorescence and ex situ temperature tolerance. Mar. Poll. Bull. 72, 313–322. doi: 10.1016/j.marpolbul.2012.11.032
IPCC (2001). Climate Change 2001: Synthesis Report. A Contribution of Working Groups I, II, and III to the Third Assessment Report of the Intergovernmental Panel on Climate Change, eds R. T. Watson and Core Writing Team (Cambridge: Cambridge University Press), 398.
IPCC (2014). “Climate change 2014: synthesis report,” in part B: regional aspects. Contribution of working groups I, II and III to the fifth assessment report of the Intergovernmental Panel on Climate Change, eds R. K. Pachauri and L. A. Meyer (Geneva: IPCC), 151.
Johannes, R. E., and Wiebe, W. J. (1970). Method for determination of coral tissue biomass Composition. Limnol. Oceanogr. 15, 822–824. doi: 10.4319/lo.1970.15.5.0822
Jokiel, P. L., Maragos, J. E., and Franzisket, L. (1978). “Coral growth: buoyant weight technique,” in Coral Reefs: Research Methods, eds D. R. Stoddart and R. E. Johannes (Paris: UNESCO monographs on oceanographic methodology), 529–542.
Jones, A. M., Berkelmans, R., van Oppen, M. J. H., Mieog, J. C., and Sinclair, W. (2008). A community change in the algal endosymbionts of a scleractinian coral following a natural bleaching event: field evidence of acclimatization. Proc. Royal Soc. B. 275, 1359–1365. doi: 10.1098/rspb.2008.0069
Kaniewska, P., Campbell, P. R., Kline, D. I., Rodriguez-Lanetty, M., Miller, D. J., Dove, S., et al. (2012). Major cellular and physiological impacts of ocean acidification on a reef building coral. PLoS One 7:e34659. doi: 10.1371/journal.pone.0034659
Kavousi, J., Reimer, J. D., Tanaka, Y., and Nakamura, T. (2015). Colony-specific investigations reveal highly variable responses among individual corals to ocean acidification and warming. Mar. Environ. Res. 109, 9–20. doi: 10.1016/j.marenvres.2015.05.004
Kinzie, R. A. (1999). Sex, symbiosis and coral communities. Am. Zool. 39, 80–91. doi: 10.1093/ICB/39.1.80
Kleypas, J. A., and Langdon, C. (2006). Coral reefs and changing seawater chemistry in Coral reefs and climate change: science and management. AGU monograph series, coastal and estuarine studies. Am. Geophys. 61, 73–110. doi: 10.1029/61CE06
Langdon, C., Gattuso, J. P., and Andersson, A. (2010). “Measurements of calcification and dissolution of benthic organisms and communities,” in Guide to Best Practices for Ocean Acidification Research and Data Reporting, eds U. Riebesell, V. J. Fabry, L. Hansson, and J.-P. Gattuso (Luxembourg: Publications Office of the European Union), 213–232. doi: 10.7717/peerj.411
Lubarsky, K. A., Silbiger, N. J., and Donahue, M. J. (2018). Effects of submarine groundwater discharge on coral accretion and bioerosion on two shallow reef flats. Limnol. Oceanogr. 63, 1660–1676. doi: 10.1002/lno.10799
Lagumen, M. C. T. (2017). Spatial and Temporal Variability of Carbonate Parameters in Bolinao-Anda, Pangasinan. MSc, Philippines: University of the Philippines.
LaJeunesse, T. C. (2002). Diversity and community structure of symbiotic dinoflagellates from Caribbean coral reefs. Mar. Biol. 141, 387–400. doi: 10.1007/s00227-002-0829-2
LaJeunesse, T. C., Bhagooli, R., Hidaka, M., DeVantier, L., Done, T., Schmidt, G. W., et al. (2004). Closely related Symbiodinium spp. differ in relative dominance in coral reef host communities across environmental, latitudinal and biogeographic gradients. Mar. Ecol. Progr. Ser. 284, 147–161. doi: 10.3354/meps284147
LaJeunesse, T. C., Pettay, D. T., Sampayo, E. M., Phongsuwan, N., Brown, B., Obura, D. O., et al. (2010). Long-standing environmental conditions, geographic isolation and hostsymbiont specificity influence the relative ecological dominace and genetic diversification of coral endosymbionts in the genus Symbiodinium. J. Biogeogr. 37, 785–800. doi: 10.1111/j.1365-2699.2010.02273.x
Leder, J. J., Szmant, A. M., and Swart, P. K. (1991). The effect of prolonged ‘bleaching’ on skeletal banding and stable isotopic composition in Montastrea annularis. Coral Reefs 10, 19–27. doi: 10.1007/BF00301902
Lee, E. T. (1992). Statistical Methods for Survival Data Analysis, 2nd Edn. New York, NY: John Wiley & Sons.
LeGresley, M., and McDermott, G. (2010). “Counting chamber methods for quantitative phytoplankton analysis – haemocytometer, Palmer-Maloney cell and Sedgewick-Rafter cell,” in Microscopic and Molecular Methods for Quantitative Phytoplankton Analysis, eds B. Karlson, C. Cusack, and E. Bresnan (Paris: UNESCO), 25–30.
Lesser, M. P. (1996). Elevated temperatures and ultraviolet radiation cause oxidative stress and inhibit photosynthesis in symbiotic dinoflagellates. Limnol. Oceanogr. 41, 271–283. doi: 10.4319/LO.1996.41.2.0271
Lesser, M. P. (1997). Oxidative stress causes coral bleaching during exposure to elevated temperatures. Coral Reefs 16, 187–192. doi: 10.1007/s003380050073
Lesser, M. P., and Farrell, J. H. (2004). Exposure to solar radiation increases damage to both host tissues and algal symbionts of corals during thermal stress. Coral Reefs 23, 367–377. doi: 10.1007/s00338-004-0392-z
Lewis, E., and Wallace, D. W. R. (1998). Program developed for CO2 system calculations. Carbon Dioxide Information Analysis Center, Oak Ridge National Laboratory. Oak Ridge, TN: U.S. Dept. of Energy, doi: 10.15485/1464255
Licuanan, W. Y., Robles, R., and Reyes, M. (2019). Status and recent trends in coral reefs of the Philippines. Mar. Pollut. Bull. 142, 544–550. doi: 10.1016/j.marpolbul.2019.04.013
Lough, J. M., and Cantin, N. E. (2014). Perspectives on massive coral growth rates in a changing ocean. Biol. Bull. 226, 187–202. doi: 10.1086/BBLv226n3p187
Maboloc, E. A., Jamodiong, E. A., and Villanueva, R. D. (2015). Reproductive biology and larval development of the scleractinian corals F. colemani and F. abdita (Faviidae) in northwestern Philippines. Invertebr. Reprod. Dev. 60, 1–11. doi: 10.1080/07924259.2015.1086829
Manullang, C., Millyaningrum, I. H., Iguchi, A., Miyagi, A., Tanaka, Y., Nojiri, Y., et al. (2020). Responses of branching reef corals Acropora digitifera and Montipora digitata to elevated temperature and pCO2. Peer J. 8:e10562. doi: 10.7717/peerj.10562
McClanahan, T. R. (2004). The relationship between bleaching and mortality of common corals. Mar. Biol. 144, 1239–1245. doi: 10.1007/s00227-003-1271-9
McCulloch, M., D’Olivo, J., Falter, J., Holcomb, M., and Trotter, J. A. (2017). Coral calcification in a changing World and the interactive dynamics of pH and DIC upregulation. Nat. Commun. 8:15686. doi: 10.1038/ncomms15686
McCulloch, M., Falter, J., Trotter, J., and Montagna, P. (2012). Coral resilience to ocean acidification and global warming through pH up-regulation. Nat. Clim. Change 2, 623–627. doi: 10.1038/nclimate1473
Meesters, E. H., and Bak, R. P. M. (1993). Effects of coral bleaching on tissue regeneration potential and colony survival. Mar. Ecol. Progr. Ser. 96, 189–198. doi: 10.3354/meps096189
Mehrbach, C., Culberson, C. H., Hawley, J. E., and Pytkowicz, R. N. (1973). Measurement of the apparent dissociation constants of carbonic acid in seawater at atmospheric pressure. Limnol. Oceanogr. 18, 897–907. doi: 10.4319/lo.1973.18.6.0897
Mieog, J. C., van Oppen, M. J. H., Berkelmans, R., Stam, W. T., and Olsen, J. L. (2009). Quantification of algal endosymbionts (Symbiodinium) in coral tissue using real-time PCR. Mol. Ecol. Res. 9, 74–82. doi: 10.1111/j.1755-0998.2008.02222.x
Nakamura, T., Van Woesik, R., and Yamasaki, H. (2005). Photoinhibition of photosynthesis is reduced by water flow in the reef-building coral Acropora digitifera. Mar. Ecol. Prog. Ser. 301, 109–118. doi: 10.3354/meps301109
NOAA (2015). National Centers for Environmental Information, State of the Climate: Global Analysis for Annual 2015. Available online at: http://www.ncdc.noaa.gov/sotc/global/201513
Noonan, S. H. C., Fabricius, K. E., and Humphrey, C. (2013). Symbiodinium community composition in scleractinian corals is not affected by life-long exposure to elevated carbon dioxide. PLoS One 8:e63985. doi: 10.1371/journal.pone.0063985
Ogawa, D., Bobeszko, T., Ainsworth, T., and Leggat, W. (2013). The combined effects of temperature and CO2 lead to altered gene expression in Acropora aspera. Coral Reefs 32, 895–907. doi: 10.1007/s00338-013-1046-9
Ohki, S., Irie, T., Inoue, M., Shinmen, K., Kawahata, H., Nakamura, T., et al. (2013). Symbiosis increases coral tolerance to ocean acidification. Biogeosci. Discuss. 10, 7013–7030. doi: 10.5194/bgd-10-7013-2013
Palumbi, S. R., Barshis, D. J., Traylor-Knowles, N., and Bay, R. A. (2014). Mechanisms of reef coral resistance to future climate change. Science 344, 895–898. doi: 10.1126/science.1251336
Parkinson, J. E., Banaszak, A. T., Altman, N. S., LaJeunesse, T. C., and Baums, I. B. (2015). Intraspecific diversity among partners drives functional variation in coral symbioses. Sci. Rep. 5:15667. doi: 10.1038/srep15667
Peñaflor, E. L., Skirving, W. J., Strong, A. E., Heron, S. F., and David, L. T. (2009). Sea-surface temperature and thermal stress in the Coral Triangle over the past two decades. Coral Reefs 28:841. doi: 10.1007/s00338-009-0522-8
Perez, S., and Weis, V. M. (2006). Nitric oxide and cnidarian bleaching: an eviction notice mediates the breakdown of symbiosis. J. Exp. Biol. 209, 2804–2810. doi: 10.1242/jeb.02309
Quimpo, T. J. R., Requilme, J. N. C., Gomez, E. J., Sayco, S. L. G., Tolentino, M. P. S., and Cabaitan, P. C. (2020). Low coral bleaching prevalence at the Bolinao-Anda Reef Complex, northwestern Philippines during the 2016 thermal stress event. Mar. Pollut. Bull. 160:111567. doi: 10.1016/j.marpolbul.2020.111567
Reynaud, S., Leclercq, N., Romaine-Lioud, S., Ferrier-Pagès, C., Jaubert, J., and Gattuso, J. P. (2003). Interacting effects of CO2 partial pressure and temperature on photosynthesis and calcification in a scleractinian coral. Glob. Change Biol. 9, 1660–1668. doi: 10.1046/j.1365-2486.2003.00678.x
Richier, S., Furla, P., Plantivaux, A., Merle, P.-L., and Allemand, D. (2005). Symbiosis-induced adaptation to oxidative stress. J. Exp. Biol. 208, 277–285. doi: 10.1242/jeb.01368
Roberts, C. M., McClean, C. J., Veron, J. E. N., Hawkins, J. P., Allen, G. R., McAllister, D. E., et al. (2002). Marine biodiversity hotspots and conservation priorities for tropical reefs. Science 295, 1280–1284. doi: 10.1126/science.1067728
Roth, M. S. (2014). The engine of the reef: photobiology of the coral-algal symbiosis. Front. Microbiol. 5:422. doi: 10.3389/fmicb.2014.00422
Sabine, L., Feely, R. A., Gruber, N., Key, R. M., Lee, K., Bullister, J. L., et al. (2004). The oceanic sink for anthropogenic CO2. Science 305:367. doi: 10.1126/science.1097403
San Diego-McGlone, M. L., Azanza, R. V., Villanoy, C. L., and Jacinto, G. S. (2008). Eutrophic waters, algal bloom and fish kill in fish farming areas in Bolinao, Pangasinan, Philippines. Mar. Poll. Bull. 57, 295–301. doi: 10.1016/j.marpolbul.2008.03.028
Schreiber, U., Schliwa, U., and Bilger, W. (1986). Continuous recording of photochemical and non-photochemical chlorophyll fluorescence quenching with a new type of modulation fluorometer. Photosyn. Res. 10, 51–62. doi: 10.1007/BF00024185
Siebeck, U. E., Marshall, N. J., Klüter, A., and Hoegh-Guldberg, O. (2006). Monitoring coral bleaching using a colour reference card. Coral Reefs 25, 453–460. doi: 10.1007/s00338-006-0123-8
Smith, D. J., Suggett, D. J., and Baker, N. R. (2005). Is photoinhibition of zooxanthellae photosynthesis the primary cause of thermal bleaching in corals? Glob. Chang. Biol. 11, 1–11. doi: 10.1111/j.1529-8817.2003.00895.x
Somero, G. N. (2010). The physiology of climate change: how potentials for acclimatization and genetic adaptation will determine “winners” and “losers”. J. Exp. Biol. 213, 912–920. doi: 10.1242/jeb.037473
Stat, M., and Gates, R. D. (2011). Clade D Symbiodinium in scleractinian corals: a “nugget” of hope, a selfish opportunist, an ominous sign, or all of the above. J. Mar. Biol. 730, 1–9. doi: 10.1155/2011/730715
Stat, M., Loh, W. K. W., LaJeunesse, T. C., Hoegh-Guldberg, O., and Carter, D. A. (2009). Stability of coral–endosymbiont associations during and after a thermal stress event in the southern Great Barrier Reef. Coral Reefs 28, 709–713. doi: 10.1007/s00338-009-0509-5
Stimson, J., and Kinzie, R. A. I. I. I. (1991). The temporal pattern and rate of release of zooxanthellae from the reef coral Pocillopora damicornis (Linnaeus) under nitrogen enrichment and control conditions. J. Exp. Mar. Biol. Ecol. 153, 63–74. doi: 10.1016/S0022-0981(05)80006-1
Strahl, J., Francis, D., Doyle, J., Humphrey, C., and Fabricius, K. (2016). Biochemical responses to ocean acidification contrast between tropical corals with high and low abundances at volcanic carbon dioxide seeps. ICES. J. Mar. Sci. 73, 897–909. doi: 10.1093/icesjms/fsv194
Takahashi, A., and Kurihara, H. (2013). Ocean acidification does not affect the physiology of the tropical coral Acropora digitifera during a 5-week experiment. Coral Reefs 32, 305–314. doi: 10.1007/s00338-012-0979-8
Tambutte, S., Holcomb, M., Ferrier-Pages, C., Reynaud, S., Tambutte, E., Zoccola, D., et al. (2011). Coral biomineralization: from the gene to the environment. J. Exp. Mar. Biol. Ecol. 408, 58–78. doi: 10.1016/j.jembe.2011.07.026
Tanzil, J. T. I., Brown, B. E., Tudhope, A. W., and Dunne, R. P. (2009). Decline in skeletal growth of the coral Porites lutea from the Andaman Sea, South Thailand between 1984 and 2005. Coral Reefs 28, 519–528. doi: 10.1007/s00338-008-0457-5
Thornhill, D. J., Rotjan, R. D., Todd, B. D., Chilcoat, G. C., Iglesias-Prieto, R., Kemp, D. W., et al. (2011). A connection between colony biomass and death in Caribbean reef-building corals. PLoS One 6:e29535. doi: 10.1371/journal.pone.0029535
Veal, C. J., Carmi, M., Fine, M., and Hoegh-Guldberg, O. (2010). Increasing the accuracy of surface area estimation using single wax dipping of coral fragments. Coral Reefs 29, 893–897. doi: 10.1007/s00338-010-0647-9
Venn, A. A., Loram, J. E., and Douglas, A. E. (2008). Photosynthetic symbioses in animals. J. Exp. Bot. 59, 1069–1080. doi: 10.1093/jxb/erm328
Venn, A. A., Tambutté, E., Holcomb, M., Laurent, J., Allemand, D., and Tambutte, S. (2013). Impact of seawater acidification on pH at the tissue-skeleton interface and calcification in reef corals. Proc. Nat. Acad. Sci. 110, 1634–1639. doi: 10.1073/pnas.1216153110
Veron, J. E. N. (2000). Corals of the world. Townsville: Australian Institute of Marine Science Australia.
Keywords: ocean warming, ocean acidification, tropical coral, growth, zooxanthellae
Citation: Tañedo MCS, Villanueva RD, Torres AF, Ravago-Gotanco R and San Diego-McGlone ML (2021) Individual and Interactive Effects of Ocean Warming and Acidification on Adult Favites colemani. Front. Mar. Sci. 8:704487. doi: 10.3389/fmars.2021.704487
Received: 03 May 2021; Accepted: 10 August 2021;
Published: 09 September 2021.
Edited by:
Yehuda Benayahu, Tel Aviv University, IsraelReviewed by:
Christopher Edward Cornwall, Victoria University of Wellington, New ZealandChristopher Paul Jury, University of Hawai‘i at Mānoa, United States
Copyright © 2021 Tañedo, Villanueva, Torres, Ravago-Gotanco and San Diego-McGlone. This is an open-access article distributed under the terms of the Creative Commons Attribution License (CC BY). The use, distribution or reproduction in other forums is permitted, provided the original author(s) and the copyright owner(s) are credited and that the original publication in this journal is cited, in accordance with accepted academic practice. No use, distribution or reproduction is permitted which does not comply with these terms.
*Correspondence: Mikhael Clotilde S. Tañedo, bWN0YW5lZG9AZ21haWwuY29t
†Deceased