- Third Institute of Oceanography, Ministry of Natural Resources, Xiamen, China
To evaluate bioturbation coefficients (DB) and mixing depths (L), 210Pb and 226Ra activity was measured in two sediments cores (from water depths of 5,398 m and 4,428 m), which were collected from seamount areas in the Northwest Pacific. Using a steady-state diffusion mode, we estimated DB values of 16.8 and 24.1 cm2/a, higher than those in abyssal sediments and those predicted by traditional empirical equations. Corresponding L values varied between 19.3 and 23.1 cm. These high values indicate that seamounts are the area of active bioturbation. A one-dimensional model for the transport of total organic carbon (TOC) from the surface layer of sediments to the deep layer was developed using the distribution pattern of the specific activity of excess 210Pb (210Pbex) and its relationship with TOC. The model showed that the TOC flux transmitted downward by bioturbation was 0.09 mmol/(cm2⋅a) and 0.12 mmol/(cm2⋅a).
Introduction
Biological mixing of marine sediments (bioturbation) can substantially modify the physical, chemical, and biological properties of the sediment record (Trauth et al., 1997). These alterations are reflected in any high-resolution sedimentary record as an attenuation of the short-term variability and major phase shifts between various paleoclimatic tracers (Rodríguez-Tovar and Uchman, 2008). Bioturbation by benthic organisms can affect early diagenesis in surface sediments and the sedimentary record. For example, bioturbation can transport newly deposited material to deep sediment. Bioturbation can also affect the structure and evolution of seafloor communities (Aller, 1994). The importance of bioturbation in mediating biogeochemical processes in the upper centimeters of oceanic sediments provides a compelling reason for wanting to quantify in situ rates of bioturbation (Teal et al., 2008). The process of bioturbation, which involves both the dispersal of sediment particles (i.e., sediment reworking) and the transport of interstitial porewater (i.e., bioirrigation) by benthic organisms, occurs in the most oxic sediments and is therefore of global importance.
The bioturbation coefficient is one of the key parameters for the numerical simulation of sediment diagenesis. It is a measure of the transport of organic carbon into the sediment, which places constraints on the redox system of the sediment (Thiel and Tiefsee-Umweltschutz, 2001). Quantification of the bioturbation, therefore, is a prerequisite for the numerical modeling of geochemical processes induced by anthropogenic activities within deep-sea sediment (Suckow et al., 2001).
Seamounts are a unique ecosystem in oceanic environments and are hotspots for pelagic and benthic organisms (Yang et al., 2019). Limited by sampling conditions and other factors, bioturbation in marine sediments around seamounts has been rarely studied until now. Anthropogenic activities, such as the exploitation of polymetallic nodules, can disturb the deep-sea floor and have negative impacts on benthic ecology (Thiel and Tiefsee-Umweltschutz, 2001; Jones et al., 2017). For example, the biogeochemical environment of the seabed along and around seabed mining routes can be altered significantly due to the resuspension of sediments, the release of chemically active substances into the water column, and the subsequent resettlement of the aforementioned materials.
Radioactive disequilibria between radionuclides of the natural uranium and thorium series are used extensively to establish timescales of sedimentation and diagenetic processes within the sediment. 210Pb is a natural radionuclide with a half-life of 22.3 years that has been used extensively to estimate sedimentation rates and biological mixing. 210Pb, produced in the atmosphere and water column by the decay of 226Ra and 222Rn, is exported to the seabed by sinking particles. The 210Pb incorporated into the sediment from this process is called “excess 210Pb” (210Pbex) in that it is of more than 210Pb that is produced in situ from the decay of 226Ra and 222Rn within the sediment column (supported 210Pb). The 210Pbex signal decreases over time and approaches zero after about 100 years. If sediment accumulation alone controls the distribution of 210Pbex, then 210Pbex activity should not be detectable in parts of the sediment column deposited 100 years ago. However, the 210Pbex signal commonly extends downward, below the expected depth for zero activity in deep-sea sediments, owing to sediment mixing by bioturbation (Legeleux et al., 1994; Yang et al., 2019). In this study, we use 210Pb and 226Ra as radiotracers to quantify the bioturbation mixing depth and diffusion coefficient based on a steady-state diffusion model.
Materials and Methods
Study Areas
A large area in the Western Pacific Basin is remarkable for its many large and small seamounts formed during the Cretaceous. Two sediment cores were collected from the MP4 seamount (McDonnell Guyot) of the Marcus-Wake Seamount Cluster in the subtropical Western Pacific Ocean (Figure 1). The heights of these seamounts range from 3,656 to 4,022 m, and the base depth is about 5,500 m.
Core MP4-S05-MC08 is from the seamount and MP4-S01-MC07 is from the foothill of mount MP4 in the Western Pacific Ocean. In the sea basin on the north and south sides of the MP4 seamount, the coverage of polymetallic nodules is more than 90%. The nodules on the north side are mainly medium sized, while those on the south side are mainly small (<3 cm).
Sampling
Two sediment cores were collected from the area around MP4 using multiple corers with an inner diameter of 9.5 cm during the R/V XIANGYANGHONG03 Monitoring and Protection of Ecology and Environment Cruise, which was at sea during July–August 2017 (Table 1). The multiple corers used in our sampling is able to collect sediments without disturbance. Once aboard the ship, multiple-core tubes were extruded and sectioned at 1 cm intervals for the upper 2-cm depth and then at 2-cm intervals to the end. Each subsample was sealed in a clean polyethylene bag and stored frozen at −18°C.

Table 1. Sampling locations, water depth, organic carbon content (Corg), bioturbation (DB), and mixing depth (L).
Assessment of Environmental Background Data
Before analysis, the sediment samples were freeze-dried and homogenized. Water content was determined gravimetrically during freeze-drying.
For the total organic carbon (TOC), 2-g samples were first acidified for 24 h with 4 mol/L HCl and rinsed at least three times with deionized water to remove carbonate carbon. The residues were dried at 50°C, ground, and homogenized. Carbon and nitrogen contents were determined using a Fisons CHN analyzer following the procedure described by Froelich (1980) and modified by Hedges and Stern (1984). Every sample was analyzed in duplicate and the results averaged.
Analysis of 210Pb and 226Ra
The radioactivity of 210Pb was determined using a 46.5 keV gamma-ray with a branching ratio of 4.25% and high-purity germanium (HPGe) detectors (ORTEC 8030, AMETEK, Berwyn, PA, United States). The detection efficiency was calibrated using marine sediment reference standard IAEA-385 (International Atomic Energy Agency, Austria). The specific activity of 210Pb was calculated by the following equation:
where A is the specific activity of 210Pb (Bq/kg), Ni and Nbi are peak areas at 46.5 keV of the sample and the blank respectively, t and tb are counting times for sample and blank respectively, εi is detection efficiency of 210Pb, Y is branch ratio (4.25%), and m is the weight of the sample (kg).
All the values of excess 210Pb were decay corrected (22.3-year half-life) to the date of the core collection. A correction for self-absorption of the low-energy 210Pb gamma rays was made using the method of Cutshall et al. (1983).
The post-homogenized sediments were sealed in a polyethylene box for at least 20 days. The radioactivity of 226Ra was determined by detection of its decay products 214Pb and 214Bi using gamma spectrometry with HPGe detector (ORTEC 8030). The energy transitions are 295.2 keV (18.4%) and 351.9 keV (35.6%) of 214Pb, and 609.3 keV (45.495) and 1,120.3 keV (14.91%) of 214Bi. Our results showed that the specific activities of 226Ra calculated from peak areas under the 295.2 keV were consistent with those under 609.3 keV, but the activities via 351.9 and 1,120.3 keV were inconsistent. The specific activity of 226Ra from 351.9 keV gamma ray was overestimated due to interference of 211Bi, which emits a gamma ray at 351.1 keV (12.91%). The specific activity of 226Ra from the 1,120.3 keV gamma ray fluctuated substantially due to its low branch ratio (14.91%). Thus, only the peak areas under 295.2 and 609.3 keV were used to calculate the specific activity of 226Ra in this study. The detection efficiency of 226Ra was calibrated using marine sediment standard IAEA-385.
The excess 210Pb (210Pbex), i.e., the fraction exceeding parent-isotope activity, was determined by subtracting 226Ra activity from the total 210Pb activity.
Results
Distribution of 210Pb, 226Ra, and 210Pbex in the Sediment Cores
The 210Pb, 226Ra, and 210Pbex profiles are shown in Figure 2. The 226Ra values ranged from 42.75 to 298.87 Bq/kg, with an average of 138.14 ± 67.02 Bq/kg. In the two cores, the highest values were at the subsurface. All profiles show an approximately exponential decrease with depth.
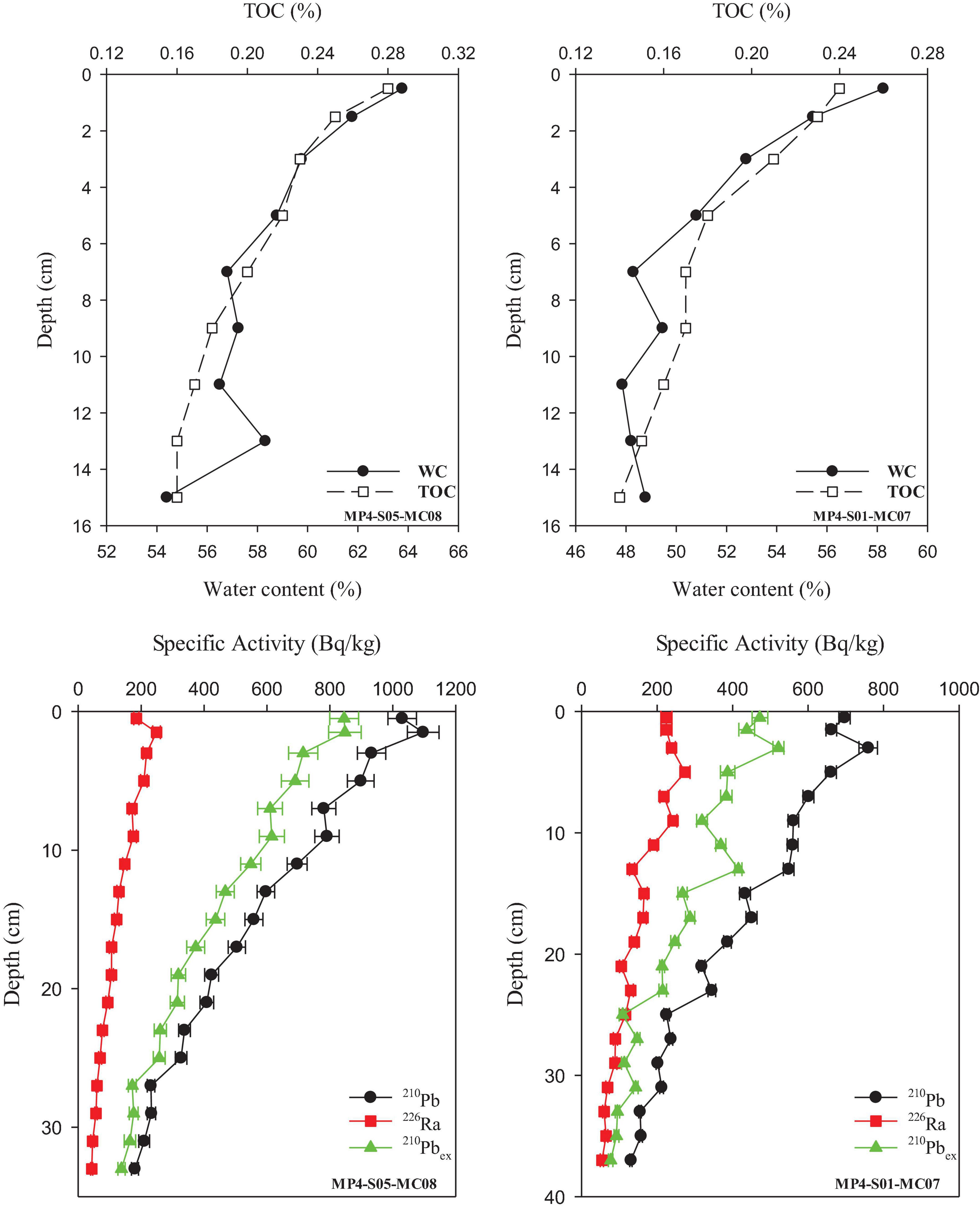
Figure 2. Profiles of total organic carbon, water content, and activity of 210Pb, 226Ra, and 210Pbex in sediment cores.
The 210Pb values ranged from 130.74 to 1659.62 Bq/kg, with an average of 487.73 ± 261.91 Bq/kg. The specific activity of 210Pb in sediments increased with depth within a few centimeters of the surface layer and then decreased exponentially with the depth after reaching the maximum value. The specific activity of 210Pbex decreases exponentially with depth and shows the same tread as 210Pb.
Two stations are located near the Zhanlun seamounts. The highest value (848.02 Bq/kg) at station MP4-S05-MC08 appeared at 1–2 cm in the subsurface layer, while the highest value (759.51 Bq/kg) at station MP4-S01-MC07 appeared at 4–6 cm. These values are comparable to the 210Pb values measured by Suckow et al. (2001) in abyssal sediments in the Peru Basin.
Back mixing, in which benthic animal activity such as feeding carries subsurface sediment to the surface, also occurs in many deep sediments. This activity makes the radionuclides at the surface decline rapidly with depth. However, in the subsurface “wide shoulder” (that is, the nuclide specific activity within a certain depth with the increase of the depth remains unchanged) or a great value.
Examples of this pattern include 210Pbex and 234Thex in abyssal sediments (140°W, 0°−5°N) in the equatorial Pacific (Smith et al., 1996) and 210Pbex and 137Cs in abyssal sediments from the northeastern tropical Atlantic (Pope et al., 1996). A three-dimensional distribution of 210Pb of sediments from the continental slope of Newfoundland showed that the mixing of sediments is uneven and the inclined burrowing movement of benthic animals transports a large amount of surface sediments into the interior. As a result, the maximum value of 210Pb is at a certain depth under the sediment–water interface (Smith and Schafer, 1984).
Distribution of Total Organic Carbon, Water Content, and Total Nitrogen
Total organic carbon varied between 0.14 and 0.28%, with an average of 0.19 ± 0.04%. The pattern shows an exponential decline with depth. The TOC in our study is lower than the central North Pacific (0.21–0.40%) (Müller and Suess, 1979) and the central equatorial Pacific (0.26–0.68%) (Smith et al., 1996).
Water content varied between 47 and 89%. The overall trend is a gradual decrease with depth, which is similar to the change in TOC.
Total nitrogen content at the sediment surface fluctuated between 0.06 and 0.07%, with values at the northern station lower than the southern station. The surface C/N ratios were 4.9 at both stations.
Bioturbation (DB and L)
The relevance of biological mixing in the inventory estimates was inferred from the bioturbation coefficient (DB). DB and bioturbation mixing depth (L) were estimated from the specific activity of the vertical distribution of 210Pbex in the sediment.
Owing to the half-life of 210Pb, the 210Pbex signal is detectable for only about 100 years. Since sedimentation rates in the deep-sea area are less than 1 millimeter per thousand years (Nozaki et al., 1977; Yang et al., 2019), 210Pbex should be detectable only within the top 1 mm of the sediment column. All the earlier studies have found 210Pb in the deep-sea sediments down to a depth of several centimeters, which was interpreted to be a result of bioturbation (Nozaki et al., 1977; Smith and Schafer, 1984; Muñoz et al., 2007).
Goldberg and Koide (1962) and Guinasso and Schink (1975) described the process of bioturbation in a first approximation steady-state diffusion model that included radioactive decay.
This model was improved by Nozaki et al. (1977). When a biological diffusion model is applied, the biological mixing process of tracer material in sediments must meet two conditions: (1) the frequency of biological mixing must be much greater than the disappearance rate of the tracer and (2) the size of the particle exchange must be smaller than the size of the tracer profile and the thickness of the mixing layer.
where z is the depth within the sediment column (cm), A is the tracer-specific activity of 210Pbex (Bq/kg) in the depth of z, ρ is the bulk sediment density (g/cm3), DB is the bioturbation coefficient (cm2/a), S is the sedimentation rate (cm/a), λ is the decay constant of 210Pb (0.031 a–1), and t is the time (a).
In this model, the bioturbation process is considered to be a vortices-like diffusion mixing process. It is assumed that in the mixing layer, the biological disturbance coefficient DB, deposition rate S, and ρ of sediment density are in a constant state, such that .
The solution with the boundary conditions, A = A0 at z = 0 and A→0 at z→∞ (Equation 1) is given by:
Since the deposition rate of oceanic sediments is generally in the order of mm/ka, and the timescale of the action of 210Pb tracer bioturbation is only about 100 a, the deposition of sediments can be ignored within the timescale of the tracer, and the aforementioned equation is simplified to:
An exponential fit to a measured depth profile of 210Pbex, therefore, gives a quantitative measure of the bioturbation. In this model, the mixing depth L is the depth in the sediment at which the fitted tracer concentration has decreased to 1/e and can be computed by:
According to Figure 3, DB and L in MP4-S01-MC07 are 24.1 cm2/a and 23.1 cm, and are 16.8 cm2/a and 19.3 cm in MP4-S05-MC08, respectively.
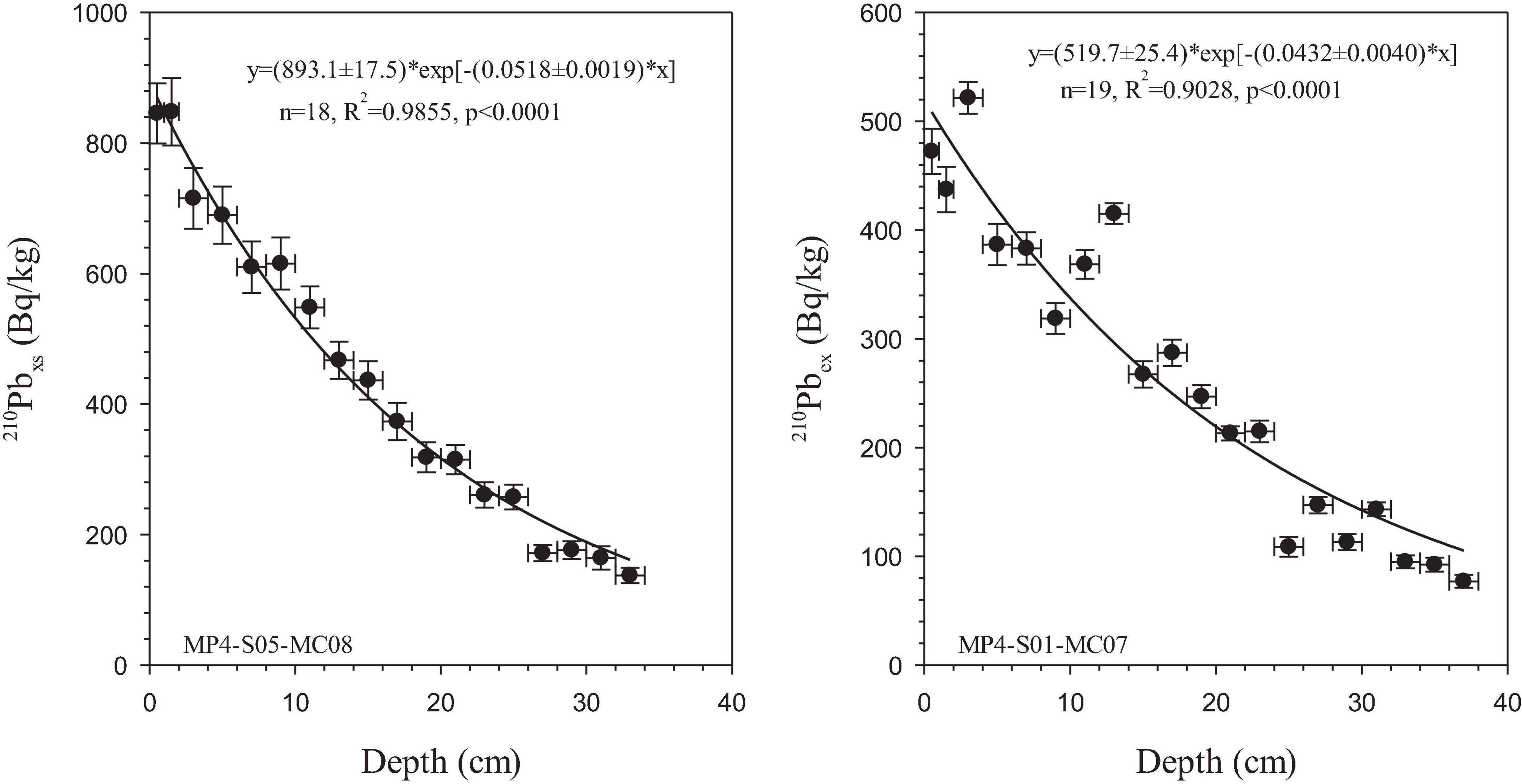
Figure 3. The relationship between specific activity of 210Pbex and depth in sediment cores. The fitting curve and equation are shown in each panel.
The average is 20.5 ± 5.2 cm2/a, which is the same as the global average of 19.98 ± 42.64 cm2/a (Teal et al., 2008) but higher than the bioturbation coefficients of the deep-sea sediments in the Western Pacific and high-productivity areas such as the Peru Basin (Suckow et al., 2001), Washington shelf edge (Yan and Zhou, 2004), Puget Bay (Carpenter et al., 1985), and the southwest polar shelf (Maire et al., 2008). Both stations were sampled in the summer, which, according to the statistics of Teal et al. (2008), is the time when the bioturbation rate is the highest. The aforementioned differences reveal the possible relationship between the intensity of the biological disturbance and sea productivity. Some studies also show that POC output flux in the water column regulates the depth of sediment mixing layer and the intensity of bioturbation (Smith et al., 1997; Boudreau, 1998).
Discussion
There are many factors influencing the bioturbation of sediments, such as deposition rate, organic carbon deposition flux at the sediment–water interface, abundance of benthic organisms, community structure, living habits and disturbance mode, water depth, oxygen solubility in bottom water or penetration of dissolved oxygen in sediments, sediment type, and hydrodynamic conditions. These factors interact with each other to affect biological disturbance, but the relative importance of each factor varies in different marine environments. However, the intensity of biological disturbance in specific sea areas is usually regulated by major influencing factors.
Influence of Water Depth
It can be seen from Table 1 that there is a higher bioturbation coefficient at the station with the shallower water depth. This is consistent with the law discovered by Middelburg et al. (1997) that global marine sediment bioturbation decreases with increasing water depth. An empirical is proposed to describe the relationship between DB and water depth based on data from the Eastern Pacific and the North Atlantic (DB = 5.2×100.762−0.0004×Z). According to this equation, the values of DB (0.51 and 0.21 cm2/a) in our study are two orders of magnitude lower than those derived by 210Pbex.
Although statistics of Middelburg are based on the global sediment bioturbation data, there are only two deep water stations in this study. More data will be needed to confirm and support this theory in the future.
Relationship Between Bioturbation and Organic Carbon and Nitrogen Contents in Sediments
Animals that forage for sediments in the deep ocean far outnumber those that feed on the suspended matter. Because the amount of nutrients in the form of bacteria and other particles is so small, sediment-eating animals have to eat large amounts of sediment to get enough organic carbon to survive. It is estimated that 100 g of sediment may pass through the digestive tract of sea cucumbers every day (Gage and Tyler, 1991). Therefore, the content of organic matter in sediments directly affects the ability of animals to process sediment particles. Boudreau (1998) believed that biological mixing strength increased with the increase of food quantity. They defined the biological diffusion coefficient as a function of the unstable concentration of active organic matter. That is, unstable organic matter degrades as it is mixed downward, and all available unstable organic matter degrades to a certain depth, so the biological diffusion coefficient as a function of organic matter disappears.
Bioturbation in this study area may be controlled by TOC content in sediments. It can be seen from Figure 4. In both cores, there is a good positive correlation between the specific activity of 210Pbex and the content of TOC. This result is consistent with the positive correlation between food sources and bioturbation intensity observed in the northeast Atlantic (Legeleux et al., 1994) and the central equatorial Pacific (Pope et al., 1996; Smith et al., 2000).
Studies have shown that the POC output flux in the water column of the tropical Pacific controls the rate of benthic biological and chemical processes (Pope et al., 1996; Alperin et al., 2002). The research results in the polymetallic nodule area of the Eastern Pacific Ocean also show that the higher the organic carbon content, the stronger the biological disturbance effect (Yan and Zhou, 2004). Unfortunately, in this study, we did not estimate the accumulation flux of organic carbon in the surface sediments.
Transport of Total Organic Carbon by Bioturbation
In bioturbation mixed sediments, organic matter is transported to deep sediments to provide organic carbon for deep organisms. Using the distribution pattern of specific activity of 210Pbex in Figure 4 and the relationship between 210Pbex and TOC, one-dimensional model for the transport of TOC from the surface layer of sediments to the deep layer can be established:
where Fb represents the flux of TOC [mmol/(cm2*a)], dCTOC/dz represents the vertical density gradient of CTOC, and the negative sign indicates the downward direction of the flux. The distribution of TOC content in the sediments of MP4-S05-MC08 and MP4-S01-MC07 stations was fitted, and the following equation was obtained:
It can be seen from the equations aforementioned that there is a good linear correlation in the two stations and the depth, which conforms to the following equation:
Differential of CTOC with respect to depth z, we can get:
The fitted a and the obtained bioturbation coefficient DB were substituted, and the TOC flux transmitted downward by bioturbation at stations MP4-S05-MC08 and MP4-S01-MC07 were calculated to be 0.09 and 0.12 mmol/(cm2⋅a), respectively.
It should be noted that this model is based mainly on the biological disturbance caused by deep 210Pbex and TOC concentration in sediments and the direct relationship between the disturbance process of TOC to calculate the transmission, while getting the coefficient of the DB has been considered in biological disturbance model 210Pb downward mixing in the process of decay. However, this model does not take into account the degradation of downward transmission in the process of TOC; therefore, the results obtained with the model represent the upper limit of the TOC conveying.
Conclusion
Based on the distribution of 210Pbex in sediment cores and one-dimensional steady-state vortex diffusion model, the bioturbation coefficient of marine sediments was found to range from 16.8 to 24.1 cm2/a with an average of 20.5 ± 5.2 cm2/a. The bioturbation mixing depth ranged between 19.3 and 23.1 cm with an average of 21.2 ± 2.7 cm, which is above the global average. This indicates that the region is experiencing a strong bioturbation effect. Bioturbation is influenced mainly by the TOC content in sediments, the composition of controlled biological communities, and water depth. The relationship between 210Pbex and TOC was used to quantify the organic carbon transported from surface sediments to deep layers by core biological activity. We estimated the TOC flux transmitted downward by bioturbation was 0.09 and 0.12 mmol/(cm2⋅a).
Data Availability Statement
The original contributions presented in the study are included in the article/supplementary material, further inquiries can be directed to the corresponding authors.
Author Contributions
FL, CL, and HL contributed to conception and design of the study. LL organized the database. XS performed the statistical analysis. All authors contributed to manuscript revision, read, and approved the submitted version.
Funding
This work was supported by the Scientific Research Foundation of Third Institute of Oceanography, MNR (2020012), the Monitoring and Protection of Ecology and Environment in the eastern Pacific Ocean (No. DY-135-E2-5-02), and Global Change and Air-Sea Interaction II (GASI-01-NPC-STsum).
Conflict of Interest
The authors declare that the research was conducted in the absence of any commercial or financial relationships that could be construed as a potential conflict of interest.
Publisher’s Note
All claims expressed in this article are solely those of the authors and do not necessarily represent those of their affiliated organizations, or those of the publisher, the editors and the reviewers. Any product that may be evaluated in this article, or claim that may be made by its manufacturer, is not guaranteed or endorsed by the publisher.
Acknowledgments
We sincerely appreciate the Monitoring and Protection of Ecology and Environment Cruise in 2017 and the crew of the R/V XIANGYANGHONG03 for their assistance in sample collection.
References
Aller, R. C. (1994). Bioturbation and remineralization of sedimentary organic matter: effects of redox oscillation. Chem. Geol. 114, 331–345. doi: 10.1016/0009-2541(94)90062-0
Alperin, M. J., Suayah, I. B., Benninger, L. K., and Martens, C. S. (2002). Modern organic carbon burial fluxes, recent sedimentation rates, and particle mixing rates from the upper continental slope near Cape Hatteras, North Carolina (USA). Deep Sea Res. II Top. Stud. Oceanogr. 49, 4645–4665. doi: 10.1016/S0967-0645(02)00133-9
Boudreau, B. P. (1998). Mean mixed depth of sediments: the wherefore and the why. Limnol. Oceanogr. 43, 524–526. doi: 10.4319/lo.1998.43.3.0524
Carpenter, R., Peterson, M., and Bennett, J. (1985). 210Pb-derived sediment accumulation and mixing rates for the greater Puget Sound region. Mar. Geol. 64, 291–312. doi: 10.1016/0025-3227(85)90109-4
Cutshall, N. H., Larsen, I. L., and Olsen, C. R. (1983). Direct analysis of 210Pb in sediment samples: self-absorption corrections. Nucl. Instrum. Methods Phys. Res. 206, 309–312. doi: 10.1016/0167-5087(83)91273-5
Gage, J. D., and Tyler, P. A. (1991). Deep-Sea Biology: A Natural History of Organisms at the Deep-Sea Floor. Cambridge: Cambridge University Press.
Goldberg, E. D., and Koide, M. (1962). Geochronological studies of deep sea sediments by the ionium/thorium method. Geochim. Cosmochim. Acta. 26, 417–450. doi: 10.1016/0016-7037(62)90112-6
Guinasso, N. Jr., and Schink, D. (1975). Quantitative estimates of biological mixing rates in abyssal sediments. J. Geophys. Res. 80, 3032–3043. doi: 10.1029/JC080i021p03032
Hedges, J. I., and Stern, J. H. (1984). Carbon and nitrogen determinations of carbonate-containing solids1. Limnol. Oceanogr. 29, 657–663. doi: 10.4319/lo.1984.29.3.0657
Jones, D. O., Kaiser, S., Sweetman, A. K., Smith, C. R., Menot, L., Vink, A., et al. (2017). Biological responses to disturbance from simulated deep-sea polymetallic nodule mining. PLoS One 12:e0171750. doi: 10.1371/journal.pone.0171750
Legeleux, F., Reyss, J.-L., and Schmidt, S. (1994). Particle mixing rates in sediments of the northeast tropical Atlantic: evidence from 210Pbxs, 137Cs, 228Thxs and 234Thxs downcore distributions. Earth Planet. Sci. Lett. 128, 545–562. doi: 10.1016/0012-821X(94)90169-4
Maire, O., Lecroart, P., Meysman, F., Rosenberg, R., Duchêne, J.-C., and Grémare, A. (2008). Quantification of sediment reworking rates in bioturbation research: a review. Aquat. Biol. 2, 219–238. doi: 10.3354/ab00053
Middelburg, J. J., Soetaert, K., and Herman, P. M. (1997). Empirical relationships for use in global diagenetic models. Deep Sea Res. I Oceanogr. Res. Pap. 44, 327–344. doi: 10.1016/S0967-0637(96)00101-X
Müller, P. J., and Suess, E. (1979). Productivity, sedimentation rate, and sedimentary organic matter in the oceans—I. Organic carbon preservation. Deep Sea Res. Part A Oceanogr. Res. Pap. 26, 1347–1362. doi: 10.1016/0198-0149(79)90003-7
Muñoz, P., Sellanes, J., Lange, C., Palma, M., and Salamanca, M. A. (2007). Temporal variability of 210Pb fluxes and bioturbation in shelf sediments beneath the high primary production area off concepción, central-southern Chile (36°S). Prog. Oceanogr. 75, 586–602. doi: 10.1016/j.pocean.2007.08.015
Nozaki, Y., Cochran, J. K., Turekian, K. K., and Keller, G. (1977). Radiocarbon and 210Pb distribution in submersible-taken deep-sea cores from Project FAMOUS. Earth Planet. Sci. Lett. 34, 167–173. doi: 10.1016/0012-821X(77)90001-2
Pope, R., DeMaster, D., Smith, C., and Seltmann, H. Jr. (1996). Rapid bioturbation in equatorial Pacific sediments: evidence from excess 234Th measurements. Deep Sea Res. II Top. Stud. Oceanogr. 43, 1339–1364. doi: 10.1016/0967-0645(96)00009-4
Rodríguez-Tovar, F. J., and Uchman, A. (2008). Bioturbational disturbance of the cretaceous-palaeogene (K-Pg) boundary layer: implications for the interpretation of the K-Pg boundary impact event. Geobios 41, 661–667. doi: 10.1016/j.geobios.2008.01.003
Smith, C. R., Berelson, W., Demaster, D. J., Dobbs, F. C., Hammond, D., Hoover, D. J., et al. (1997). Latitudinal variations in benthic processes in the abyssal equatorial Pacific: control by biogenic particle flux. Deep Sea Res. II Top. Stud. Oceanogr. 44, 2295–2317. doi: 10.1016/S0967-0645(97)00022-2
Smith, C. R., Hoover, D. J., Doan, S. E., Pope, R. H., Demaster, D. J., Dobbs, F. C., et al. (1996). Phytodetritus at the abyssal seafloor across 10° of latitude in the central equatorial Pacific. Deep Sea Res. II Top. Stud. Oceanogr. 43, 1309–1338. doi: 10.1016/0967-0645(96)00015-X
Smith, C. R., Levin, L. A., Hoover, D. J., McMurtry, G., and Gage, J. D. (2000). Variations in bioturbation across the oxygen minimum zone in the northwest Arabian Sea. Deep Sea Res. Part II Top. Stud. Oceanogr. 47, 227–257. doi: 10.1016/S0967-0645(99)00108-3
Smith, J., and Schafer, C. (1984). Bioturbation processes in continental slope and rise sediments delineated by Pb–210, microfossil and textural indicators. J. Mar. Res. 42, 1117–1145. doi: 10.1357/002224084788520738
Suckow, A., Treppke, U., Wiedicke, M. H., and Weber, M. E. (2001). Bioturbation coefficients of deep-sea sediments from the Peru Basin determined by gamma spectrometry of 210Pbexc. Deep Sea Res. II Top. Stud. Oceanogr. 48, 3569–3592. doi: 10.1016/S0967-0645(01)00057-1
Teal, L., Bulling, M. T., Parker, E., and Solan, M. (2008). Global patterns of bioturbation intensity and mixed depth of marine soft sediments. Aquat. Biol. 2, 207–218. doi: 10.3354/ab00052
Thiel, H., and Tiefsee-Umweltschutz, F. (2001). Evaluation of the environmental consequences of polymetallic nodule mining based on the results of the TUSCH Research Association. Deep Sea Res. II Top. Stud. Oceanogr. 48, 3433–3452. doi: 10.1016/S0967-0645(01)00051-0
Trauth, M. H., Sarnthein, M., and Arnold, M. (1997). Bioturbational mixing depth and carbon flux at the seafloor. Paleoceanography 12, 517–526. doi: 10.1029/97PA00722
Yan, Q., and Zhou, H. (2004). Bioturbation in near-surface sediments from the COMRA polymetallic nodule area: evidence from excess 210Pb measurements. Chinese Sci. Bull. 49, 2538–2542.
Keywords: seamount, 210Pb, 226Ra, bioturbation, Northwest Pacific, TOC
Citation: Lin F, Lin C, Lin H, Sun X and Lin L (2021) 210Pb-Derived Bioturbation Rates in Sediments Around Seamounts in the Tropical Northwest Pacific. Front. Mar. Sci. 8:701897. doi: 10.3389/fmars.2021.701897
Received: 28 April 2021; Accepted: 29 November 2021;
Published: 22 December 2021.
Edited by:
Weifeng Yang, Xiamen University, ChinaReviewed by:
Leonardo Langone, Institute of Polar Sciences, National Research Council (CNR), ItalyJunwen Wu, Shantou University, China
Copyright © 2021 Lin, Lin, Lin, Sun and Lin. This is an open-access article distributed under the terms of the Creative Commons Attribution License (CC BY). The use, distribution or reproduction in other forums is permitted, provided the original author(s) and the copyright owner(s) are credited and that the original publication in this journal is cited, in accordance with accepted academic practice. No use, distribution or reproduction is permitted which does not comply with these terms.
*Correspondence: Feng Lin, bGluZmVuZ0B0aW8ub3JnLmNu; Cai Lin, bGluY2FpQHRpby5vcmcuY24=; Hui Lin, bGluaHVpQHRpby5vcmcuY24=; Xiuwu Sun, c3VueGl1d3VAdGlvLm9yZy5jbg==; Li Lin, bGlubGlAdGlvLm9yZy5jbg==