- 1Nutrigenomics and Fish Growth Endocrinology Group, Institute of Aquaculture Torre de la Sal, Consejo Superior de Investigaciones Científicas, Castellón, Spain
- 2Cardiovascular Physiology Research Group, Universidade Federal do Pampa, Rio Grande do Sul, Brazil
- 3Fish Pathology Group, Institute of Aquaculture Torre de la Sal, Consejo Superior de Investigaciones Científicas, Castellón, Spain
- 4Department of Bioactivity and Food Analysis, Institute of Food Science Research, Consejo Superior de Investigaciones Científicas – Universidad Autónoma de Madrid, Madrid, Spain
- 5Department of Food Biotechnology and Microbiology, Institute of Food Science Research, Consejo Superior de Investigaciones Científicas – Universidad Autónoma de Madrid, Madrid, Spain
This study aimed to highlight the relationship between diet, animal performance and mucosal adherent gut microbiota (anterior intestine) in fish fed plant-based diets supplemented with an egg white hydrolysate (EWH) with antioxidant and anti-obesogenic activity in obese rats. The feeding trial with juveniles of gilthead sea bream (Sparus aurata) lasted 8 weeks. Fish were fed near to visual satiety with a fish meal (FM)/fish oil (FO) based diet (CTRL) or a plant-based diet with/without EWH supplementation. Specific growth rate decreased gradually from 2.16% in CTRL fish to 1.88% in EWH fish due to a reduced feed intake, and a slight impairment of feed conversion ratio. Plant-based diets feeding triggered a hyperplasic inflammation of the anterior intestine regardless of EWH supplementation. However, EWH ameliorated the goblet cell depletion, and the hepatic and intestinal lipid accumulation induced by FM/FO replacement. Illumina sequencing of gut mucosal microbiota yielded a mean of 136,252 reads per sample assigned to 2,117 OTUs at 97% identity threshold. The bacterial diversity was similar in all groups, but a significantly lower richness was found in EWH fish. At the phylum level, Proteobacteria reached the highest proportion in CTRL and EWH fish, whereas Firmicutes were decreased and Actinobacteria increased with the FM/FO replacement. The proportion of Actinobacteria was restored by dietary EWH supplementation, which also triggered a highest amount of Bacteroidetes and Spirochaetes. At a closer look, a widespread presence of Lactobacillales among groups was found. Otherwise, polysaccharide hydrolases secretors represented by Corynebacterium and Nocardioides were increased by the FM/FO replacement, whereas the mucin-degrading Streptococcus was only raised in fish fed the plant-based diet without EWH. In addition, in EWH fish, a higher abundance of Propionibacterium was related to an increased concentration of intestinal propionate. The antagonism of gut health-promoting propionate with cholesterol could explain the inferred underrepresentation of primary bile acid biosynthesis and steroid degradation pathways in the EWH fish microbiota. Altogether, these results reinforce the central role of gut microbiota in the regulation of host metabolism and lipid metabolism in particular, suggesting a role of the bioactive EWH peptides as an anti-obesity and/or satiety factor in fish.
Introduction
European aquaculture is still dependent on marine ingredients for feeds, though a high grade of replacement of marine feedstuffs (more than 70%) has been currently accomplished in Norwegian salmon feeds (Ytrestøyl et al., 2015). A high level of fish meal (FM) and fish oil (FO) replacement has also been achieved in a typically carnivorous marine fish, such as European sea bass (Dicentrarchus labrax) (Kousoulaki et al., 2015; Torrecillas et al., 2017). Likewise, plant-based diets with less than 10% of marine ingredients have been proven to support maximum growth from early life stages to completion of sexual maturity in gilthead sea bream (Sparus aurata) (Benedito-Palos et al., 2016; Simó-Mirabet et al., 2018). However, wide-serum metabolomics profiling revealed nutritionally mediated effects in processes of mucosal tissue repair and DNA stability (Gil-Solsona et al., 2019). Furthermore, feeding gilthead sea bream with plant-based diets induced negative effects at gut level, including changes in the expression of mucins, mucosal immunoglobulins (IgT) and other immune-relevant genes (Pérez-Sánchez et al., 2013; Piazzon et al., 2016). This biomarker profile leads to a pro-inflammatory condition, with drawback effects in gut integrity and epithelial barrier functions (Estensoro et al., 2016; Piazzon et al., 2017). However, most of these effects, including changes in sex reversal from male to female, or in mucosal adherent intestinal microbiota composition, were restored by dietary sodium butyrate supplementation, resulting in an improved disease outcome in fish exposed to bacteria or myxozoan parasites (Piazzon et al., 2017; Simó-Mirabet et al., 2018).
Gut microbiota has the capacity to modify and possibly activate food constituents, providing benefits for health (Brown et al., 2015; Davis, 2016; Aoun et al., 2020). Certainly, the persistent imbalance of the gut’s microbial community resulting from exposure to diverse environmental factors, including unhealthy diets, drugs, toxins, and pathogens, has a major impact on health (Chávez-Talavera et al., 2017; Hasan and Yang, 2019; Lee et al., 2020). Among them, diet micro- and macro-nutrients are considered one of the main factors that modulate gut microbiota (Zhang et al., 2018; Leeming et al., 2019). Therefore, understanding the modulation of the gut microbiome by dietary nutrients and vice-versa becomes essential for the development of novel strategies to improve animal and human health. In fact, the targeting of gut microbiota is a promising tool to improve the health and welfare of farmed fish, and gilthead sea bream in particular. Certainly, the intestinal gut microbiota is highly modulated by age and sex reversal in a protandrous hermaphrodite fish such as gilthead sea bream (Piazzon et al., 2019). In addition, the gut microbiota of gilthead sea bream families selected for fast-growth showed a high level of plasticity, which makes them more flexible upon dietary changes, showing at the same time, a better ability to deal with intestinal parasites (Piazzon et al., 2020).
In other studies of this special issue of nutrition and gut microbiota in aquaculture, we also analyzed the effect of probiotics and alternative FM replacers on gilthead sea bream gut microbiota (Moroni et al., 2021; Solé-Jiménez et al., 2021). Meanwhile, bioactive peptides derived from food proteins are considered important modulators of various biological processes, which occur both systemically and locally within the gastrointestinal tract (Moughan et al., 2014). Historically, milk proteins have been considered a rich source of bioactive peptides, but there is now evidence of a wide range of animal and plant protein sources for production of biologically active peptides, comprising meat, bone, eggs, cereals, legumes, yeast, seaweed, and fungi (Brown et al., 2015). In particular, a bioactive egg white hydrolysate (EWH) treated with pepsin showed potent in vitro and in vivo antioxidant and anti-inflammatory properties (Dávalos et al., 2004; Miguel et al., 2004; Garcés-Rimón et al., 2016a), improving oxidative stress and inflammation biomarkers on genetically and diet-induced obese rats (Garcés-Rimón et al., 2016b; Moreno-Fernández et al., 2018a,b). The antioxidant properties of EWH have also been associated with the prevention of metabolic complications arising from the exposure to heavy metals (Rizzetti et al., 2017; Martínez et al., 2019; Gomes Pinheiro et al., 2020). Although earlier studies targeting intestinal microbiota support that EWH has the potential to revert microbial dysbiosis in a rodent model of genetic obesity (Requena et al., 2017), the modulating effects of food-bioactive peptides upon gut microbiota have been much less studied (Wu et al., 2021). Thus, in an attempt to focus on the link between mucosal adherent microbiota and the bioactive properties of EWH in other animal model, we analyzed the potential benefits of dietary EWH supplementation in farmed gilthead sea bream juveniles fed plant-based diets. Special attention was also paid to the evaluation of growth performance, allocation of body fat depots, hepatic and intestinal histopathological scoring, antioxidant status, and intestinal concentration of lactic acid and short chain fatty acids (SCFAs).
Materials and Methods
Ethics Statement
Fish manipulation and tissue collection were carried out according to the Spanish (Royal Decree RD53/2013) and the current EU (2010/63/EU) legislations on the handling of experimental fish. All procedures were approved by the Ethics and Animal Welfare Committee of the Institute of Aquaculture Torre de la Sal (IATS-CSIC, Castellón, Spain), CSIC (permit number 869/2019) and “Generalitat Valenciana” (permit number 2020/VSC/PEA/0010).
Animals
Juveniles of gilthead sea bream (March 2020) were purchased from a Mediterranean hatchery (Piscimar, Burriana, Spain) and adapted for 2 months to the indoor experimental facilities of IATS-CSIC under natural photoperiod and temperature conditions (40°5′N; 0°10′E). Seawater was pumped ashore (open system), oxygen content of water effluents was always above 85% saturation, and unionized ammonia remained below 0.02 mg/L. During the acclimation and experimental period (May–July 2020), water temperature increased from 18°C in May to 25°C in July.
Diets
Extruded isoproteic and isolipidic diets (2, 3 mm pellet size) were formulated by Sparos Lda. (Olhão, Portugal), following current industry practices with plant protein and oil sources as main replacers of fish meal (FM) and fish oil (FO) (Table 1). The inclusion level of FM (including fish protein hydrolysates) and FO in the control diet (CTRL) was 35% and 5%, respectively. In the diet named L-FM/FO, the FM and FO inclusion levels were reduced to 12.5% for FM and fish protein hydrolysates, and to 3% for FO, being this diet conveniently supplemented with monocalcium phosphate, L-tryptophan and DL-methionine. The EWH diet was formulated to be a L-FM/FO diet with EWH added at 7.5% instead of plant proteins and fish protein hydrolysates. The EWH was prepared by pepsin hydrolysis of crude egg white as previously described by Garcés-Rimón et al. (2016a). Briefly, commercial pasteurized egg white was hydrolyzed for 8 h with BC Pepsin 1:3000 (E.C. 3.4.23.1; from pork stomach, E:S:2:100 w:w, pH 2.0, 37°C), purchased from Biocatalysts (Cardiff, United Kingdom). Enzyme inactivation was achieved by increasing the pH to 7.0 with 10 N NaOH. The hydrolysate was centrifuged at 2,500 × g for 15 min and the supernatants were frozen and lyophilized until use as fish feed ingredient.
Feeding Trial
In May 2020, fish of 20–24 g body weight were randomly distributed in nine 90 L tanks to establish triplicate groups of 20 fish each (initial rearing density, 4.8–4.9 kg/m3). All fish were tagged with passive integrated transponders (PIT) (ID-100A 1.25 Nano Transponder, Trovan, Madrid, Spain) into the dorsal skeletal muscle, and were individually weighed and measured at initial, intermediate and final sampling points (every 4 weeks), using a FR-200 Fish Reader W (Trovan) for data capture and pre-processing. The trial lasted 8 weeks, and fish were fed by hand once daily (12 a.m.), 6 days per week, near to visual satiety with CTRL or experimental diets for the entire duration of the trial. Feed intake was registered daily, and normal fish behavior was assessed routinely by camera monitoring. No mortalities were registered through the entire experimental period.
Sample Collection
At the end of the trial and following two fasting days, nine fish per diet (three fish/tank) were anaesthetized with 0.1 g/L of tricaine-methanesulfonate (MS-222, Sigma-Aldrich, St. Louis, MO, United States). Blood was taken from the caudal vessels with heparinized syringes, centrifuged at 3,000 × g for 20 min at 4°C, and plasma samples were stored at −80°C until analyzed. Before tissue collection, fish were sacrificed by cervical section. Liver, intestine (excluding the pyloric caeca) and mesenteric fat were weighed and measured (intestine length) to calculate the hepatosomatic index (HSI), mesenteric fat index (MSI), and intestine weight (IWI) and length (ILI) indexes. Tissue portions (∼0.4 cm) of liver, anterior intestine (AI; immediately after the pyloric caeca) and posterior intestine (PI; immediately before the anal ampoule) were fixed in 10% neutral buffered formalin for subsequent histological analyses. The remaining AI was opened and gently washed with sterile Hanks’s balanced salt solution to remove non-adherent bacteria. Intestinal mucus was scrapped off using the blunt edge of a sterile scalpel and collected into sterile 1.5 mL tubes. The anterior intestine portion was selected due to its importance in fish nutrient absorption and metabolism (Sundell and Rønnestad, 2011). The autochthonous bacteria were selected, because these populations are capable of colonizing the mucosal surface, directly impacting the fish physiology (Hao and Lee, 2004). Mucus samples were kept on ice and DNA extraction was performed immediately after the sampling. Additional fish (10 fish per diet) were sampled 8 h after feeding for the analysis of intestinal SCFA. Briefly, animals were anesthetized and sacrificed by cervical section, intestine was cut out, and the intestinal content was collected by stripping. During the two sampling days corresponding to fasting and postprandial sample collection, all samples were obtained in a short-period lasting 2–3 h, alternating among replicates of each dietary group to avoid biases due to sampling time.
Histological Analysis
Formalin fixed pieces of liver, AI and PI were processed for paraffin embedment, 4 μm-sectioned and stained with Giemsa and periodic acid-Schiff (PAS) following standard procedures. Sections were examined with a Leitz Dialux 22 light microscope connected to an Olympus DP70 camera, and representative microphotographs were taken. The histological alterations observed were scored according to semiquantitative scales. In intestinal sections, cell abundance of differentially stained goblet cells (light- or dark-stained with Giemsa), intraepithelial lymphocytes (IELs) and eosinophilic granular cells (EGCs) were scored ranging from 0 (absence) to 3 (very abundant, meaning 25–30 cells/microscope field at 500× magnification). The degree of lipid vacuolization in enterocytes and the degree of hyperplasia in the lamina propria-submucosa were scored from 0 (absence) to 3 (severe). In liver sections, the degree of lipid and glycogen storage in hepatocytes was scored from 0 (absence) to 3 (pervasive) by Giemsa or PAS staining, respectively. In addition, melanomacrophage centers were quantified in the liver.
Antioxidant Capacity
The oxygen radical absorbance capacity (ORAC) assay was used to measure the total plasma antioxidant capacity as previously described (Garcés-Rimón et al., 2016b). ORAC values were quantified by a fluorimeter Polarstar Galaxy plate reader (BMG Labtechnologies GmbH, Germany) with wavelength excitation at 485 nm and wavelength emission measured at 520 nm. Results were expressed as μmol of Trolox (Sigma, United States) equivalent (eq)/mL of plasma.
Lactic Acid and Short Chain Fatty Acid Determinations
Intestine content (200 mg) was homogenized with 0.1% peptone solution with 0.85% NaCl (500 μL) and centrifuged at 10,000 × g for 5 min at 4°C. The supernatant was filtered and 0.2 μL were injected on a HPLC system (Jasco, Tokyo, Japan) equipped with a UV-975 detector. Lactic acid and SCFA were separated using a Rezex ROA Organic Acids column (Phenomenex, Macclesfield, United Kingdom) following the method described by Sanz et al. (2005). The mobile phase was a linear gradient of 0.005 M sulfuric acid in HPLC grade water, and flow rate was 0.6 mL/min. The elution profile was monitored at 210 nm, and peak identification was carried out by comparing the retention times of target peaks with those of standards. Calibration curves of formic acid, acetic acid, propionic acid, butyric acid and lactic acid were prepared in the concentration range of 1 to 100 mM.
DNA Extraction From Mucus Samples
Intestinal mucus samples (200 μl) were treated with 250 μg/mL of lysozyme (Sigma) for 15 min at 37°C. Then, DNA was extracted using the High Pure PCR Template Preparation Kit (Roche) following the manufacturer’s instructions. DNA concentration, quality and purity were measured using a Nanodrop 2000c (Thermo Scientific), and agarose gel electrophoresis (1% w/v in Tris-EDTA buffer). DNA was stored at −20°C until sequencing.
Illumina MiSeq Sequencing and Bioinformatic Analysis
The V3-V4 region of the 16S rRNA gene (reference nucleotide interval 341–805 nt) was sequenced using the Illumina MiSeq system (2 × 300 paired-end run) at the Genomics Unit from the Madrid Science Park Foundation (FPCM). The details on the PCR and sequencing of amplicons were described elsewhere (Piazzon et al., 2019). Raw sequence data were uploaded to the Sequence Read Archive (SRA) under Bioproject accession number PRJNA705868 (BioSample accession numbers: SAMN18105342-68). Raw forward and reverse reads were quality filtered using FastQC1 and pre-processed using Prinseq (Schmieder and Edwards, 2011). Terminal N bases were trimmed in both ends and sequences with >5% of total N bases were discarded. Reads that were <150 bp long, with Phred quality score < 28 in both of the sequence ends and with a Phred average quality score < 26 were excluded. Then, forward and reverse reads were merged using fastq-join (Aronesty, 2013).
Bacteria taxonomy assignment was performed using the Ribosomal Database Project (RDP) release 18 as a reference database (Cole et al., 2014). Reads were aligned with a custom-made pipeline using VSEARCH and BLAST (Altschul et al., 1990; Rognes et al., 2016). Alignment was performed establishing high stringency filters (ł90% sequence identity, ł90% query coverage). Taxonomic assignment results were filtered and data were summarized in an operational taxonomic units (OTUs) table. From the annotation obtained, the discussion and interpretation of the results was based at the level of genus, as taxonomic affiliations with 16S rRNA amplicon sequencing might not be accurate enough at the species level (Winand et al., 2020). Sample depths were normalized by total sum scaling and then made proportional to the total sequencing depth, following the recommendations previously described (McKnight et al., 2019).
Inferred Metagenome and Pathway Analysis
Piphillin was used to normalize the amplicon data by 16S rRNA gene copy number and to infer metagenomic contents (Iwai et al., 2016). This analysis was performed with the OTUs significantly driving the separation by diets in the PLS-DA analysis (described in the “Statistics” section). For the analysis, a sequence identity cut-off of 97% was implemented, and the inferred metagenomic functions were assigned using the Kyoto Encyclopedia of Genes and Genomes database (KEGG, October 2018 Release). Raw KEGG pathway output from Piphillin was analyzed with the R Bioconductor package DESeq2 using default parameters, after flooring fractional counts to the nearest integer (Love et al., 2014; Bledsoe et al., 2016; Piazzon et al., 2020).
Statistics
Data on growth were analyzed by one-way ANOVA using SigmaPlot v14 (Systat Software Inc., San Jose, CA, United States). Normality of the data was verified by Shapiro–Wilk test, and Dunn’s post-test was used for multiple comparisons among groups. Analysis of semiquantitative and quantitative histological data was carried out with the non-parametric Kruskal–Wallis test, followed by Dunn’s post-test for the multiple comparisons. SCFA results were analyzed by one-way ANOVA followed by Holm–Sidak post-test. Rarefaction curves (plotting the number of observed taxonomic assignations against the number of sequences), species richness estimates, and alpha diversity indexes were obtained using the R package phyloseq (McMurdie and Holmes, 2013). Differences in species richness, diversity indexes and phylum abundance were determined by Kruskal–Wallis test using the Dunn’s post-test, with a significance threshold of P < 0.05. Beta diversity across groups was tested with permutational multivariate analysis of variance (PERMANOVA), using the non-parametric method adonis from the R package Vegan with 10,000 random permutations. To study the separation among groups, supervised partial least-squares discriminant analysis (PLS-DA) and hierarchical clustering of samples were sequentially applied, using EZinfo v3.0 (Umetrics, Umeå, Sweden) and the R package ggplot2, respectively. Values of normalized counts of OTUs present in five or more samples were included in the analyses. The contribution of the different genes to the group separation was determined by the minimum Variable Importance in the Projection (VIP) values achieving the complete clustering of the conditions with a VIP value ≥ 1.2. Hotelling’s T2 statistic was calculated by the multivariate software package EZinfo v3.0. All points in the current study were within the 95% confidence limit for T2, thus no outliers were detected and discarded. The quality of the PLS-DA model was evaluated by the parameters R2Y (cum) and Q2 (cum), which indicate the fit and prediction ability, respectively. To assess whether the supervised model was being over-fitted, a validation test consisting on 500 random permutations was performed using SIMCA-P+ v11.0 (Umetrics). The inferred metagenomic pathways were considered differentially represented using a FDR-corrected significance threshold of 0.05.
Results
Growth Performance and Antioxidant Capacity
Data on growth performance are reported in Table 2. Final body weight, feed intake and condition factor were significantly lower (P ≤ 0.004) in EWH fish than in CTRL fish with intermediate values in fish fed the L-FM/FO diet. Specific growth rates (SGR) also varied significantly from 2.16 in CTRL fish to 1.88 in EWH fish, again with intermediate values (2.03) in fish fed the L-FM/FO diet. The opposite trend (not statistically significant, P = 0.06) was found for the feed conversion ratio (FCR) that varied from 1.03 in CTRL fish to 1.10 in EWH fish. HSI, MFI, and IWI were not significantly altered by dietary treatment. However, the intestine length of L-FM/FO fish was larger, and the resulting ILI was significantly higher in this group of fish in comparison to CTRL and EWH groups. Intestine weight of L-FM/FO fish was also larger, although this was not reflected in significant differences in IWI among groups. No statistical differences in plasma antioxidant capacity were observed between different groups, with ORAC values around 6 μmol eq Trolox/mL plasma.
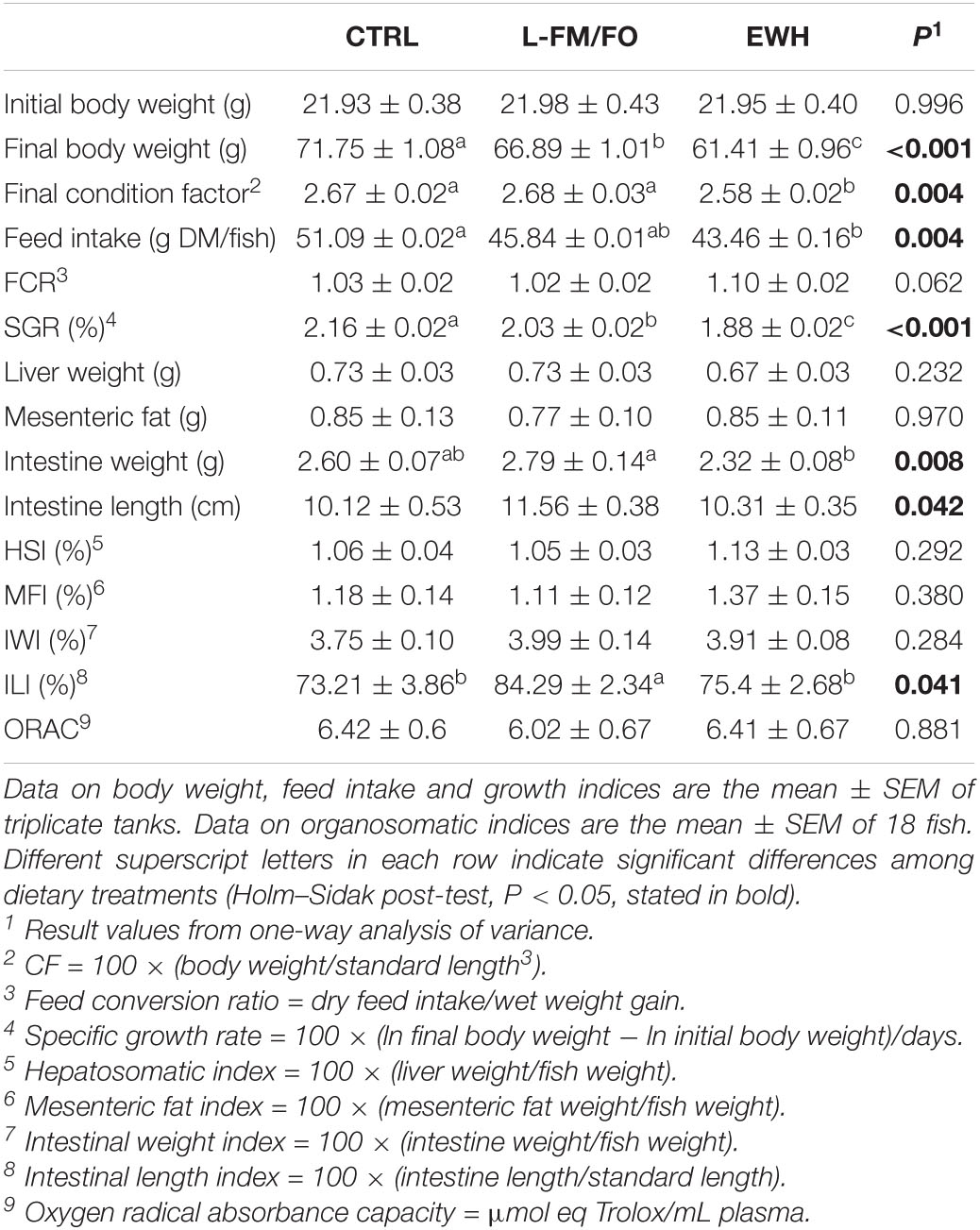
Table 2. Effects of dietary treatment on growth performance and antioxidant capacity of gilthead sea bream juveniles fed to visual satiety from May to July (8 weeks) with control (CTRL), low fish meal/fish oil (L-FM/FO) diet, and EWH diets.
Histological Scoring
The dietary replacement of FM/FO provoked a hyperplasic inflammation in the intestines of both, L-FM/FO and EWH fish, compared to the CTRL fish (Figures 1, 2). Inflammatory cell infiltrates in the epithelium and lamina propria-submucosa consisted mainly of lymphocytes and eosinophilic granular cells. In the AI, a significant submucosal hyperplasia was found in L-FM/FO and EWH fish, though the increase of EGCs and IELs was not significant. In this segment, the abundance of light-stained goblet cells was significantly reduced by the L-FM/FO diet and this effect reverted by the EWH diet. This goblet cell type presented a PAS+ staining pattern indicative of neutral mucins. By contrast, the hyperplasic effect was less severe (not significant) at the PI, where the increase of intraepithelial lymphocytes was the only significant inflammatory sign observed in L-FM/FO and EWH fish. The enterocytes of the CTRL and L-FM/FO fish presented a medium degree of lipid vacuolization, which was significantly reduced by the EWH diet. Remarkably, this lipid depletion in EWH PIs co-occurred with a decrease of lipid depots in the hepatocytes of EWH fish, which were increased with L-FM/FO diets. No differences in glycogen storage were found in the liver of fish fed the different diets, though a significant increase of liver melanomacrophage centers was observed in EWH fish, compared to the other two diets.
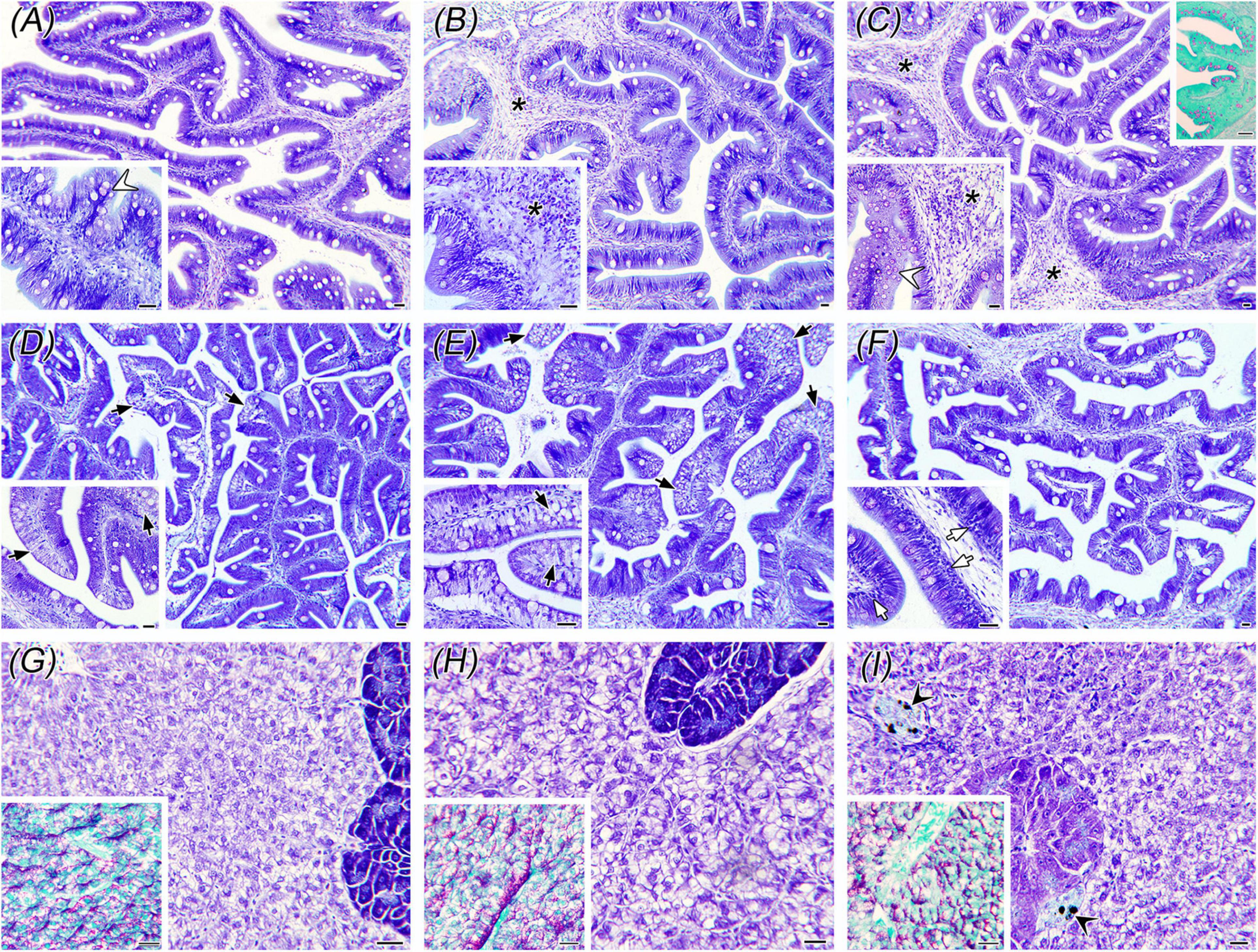
Figure 1. Histological alterations in the AI (A–C), PI (D–F) and liver (G–I) of gilthead sea bream. Panels (A,D,G) correspond to CTRL fish; (B,E,H) to fish fed the L-FM/FO diet; and (C,F,I) to fish fed the EWH diet. In AI, note the high abundance of light-stained goblet cells (white arrowheads) in CTRL (A) and EWH (C) fish, as well as the submucosal hyperplasia (asterisks) in L-FM/FO (B) and EWH (C) fish. The upper insert in panel (C) shows the PAS-stained goblet cells. In the PI, note the presence of lipid vacuolization in enterocytes (black arrows) in CTRL fish (D), which is intensified in L-FM/FO fish (E) and decreased in EWH fish (F). PI of EWH fish presented high abundance of intraepithelial lymphocytes (white arrows). In livers, note the higher lipid storage in L-FM/FO fish (H) and the presence of early melanomacrophage centers (black arrowheads) in EWH fish (I). Glycogen storage did not change among groups (PAS-stained inserts). Scale bars = 20 μm.
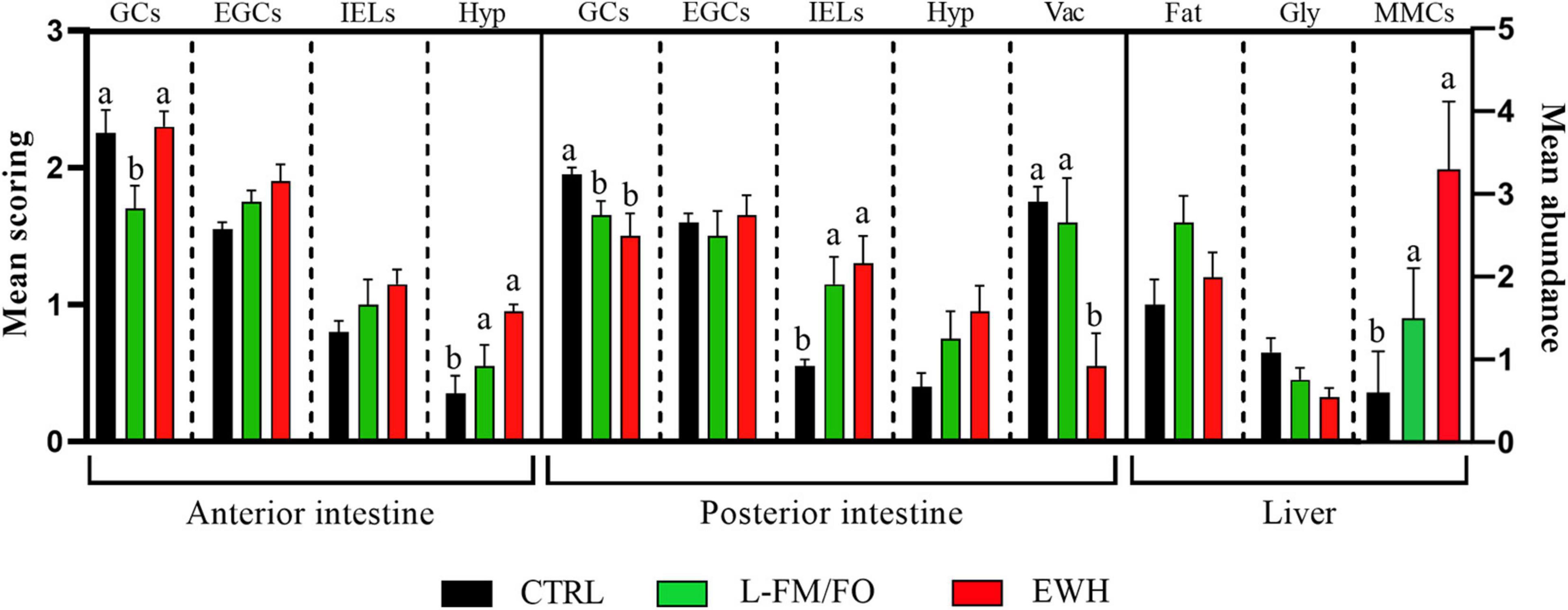
Figure 2. Scoring of histological alterations in fish fed CTRL (black bars), L-FM/FO (green bars) and EWH (red bars) diets. Mean semiquantitative scoring (+SEM) from 0 (absence) to 3 (very abundant) is shown for presence of PAS+ goblet cells (GCs), eosinophilic granular cells (EGCs) and intraepithelial lymphocytes (IELs) in two intestinal segments. Mean semiquantitative scoring (+SEM) from 0 (absence) to 3 (severe) is shown for the degree of hyperplasia in the submucosa (Hyp), vacuolization of enterocytes (Vac) in two intestinal segments, and fat (Fat) and glycogen (Gly) storage in liver. Total melanomacrophage centers (MMCs) were quantified in liver (mean abundance, right y-axis, +SEM). Different letters within each alteration indicate statistically significant differences among diets (P < 0.05).
Intestinal Content in Lactic Acid and Short Chain Fatty Acid
Butyric acid could not be detected in any of the analyzed samples. No statistically significant differences were found among groups for the intestinal concentration of lactic acid, formic acid, acetic acid, or total SCFA (Figure 3 and Supplementary Table 1). The only difference was found in propionic acid, present in significantly higher concentrations in the intestinal content of fish fed EWH (7.40 μmol/g) when compared to the CTRL group (4.14 μmol/g).
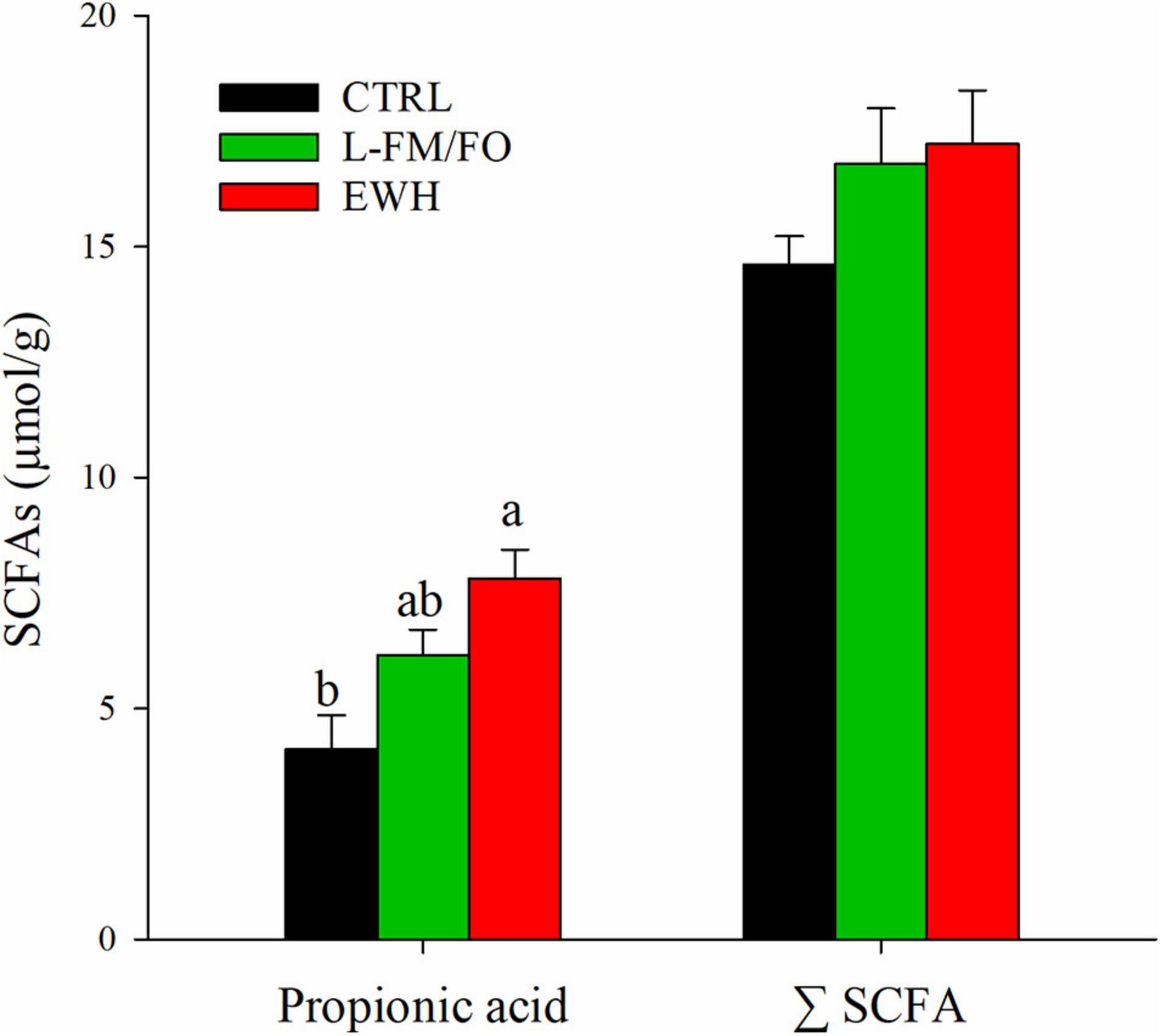
Figure 3. Concentration of intestinal propionic acid and total short chain fatty acids (ΣSCFA) in fish fed CTRL (black bars), L-FM/FO (green bars), and EWH (red bars) diets. Significant differences (one-way ANOVA, Holm–Sidak post-test, P < 0.05) are indicated by different letters, which correspond to pairwise comparisons within each dietary group.
Alpha Diversity and Microbial Composition
Illumina sequencing of the 27 analyzed samples yielded 3,678,804 high quality reads, with a mean of 136,252 reads per sample (Supplementary Table 2). The reads were assigned to 2,117 OTUs at a 97% identity threshold. Rarefaction analysis showed curves that approximated saturation (horizontal asymptote), thus a good coverage of the bacterial community was achieved and the number of sequences for analysis was considered appropriate (Supplementary Figure 1).
In a first attempt to unravel the effects of dietary intervention on gut mucosal microbiota, we analyzed the bacterial diversity of all dietary groups, and no significant differences were found in Shannon and Simpson diversity indexes, but a significantly lower richness (ACE value, P < 0.05) was found in EWH fish (Table 3). At the phylum level (Figure 4), Proteobacteria were the most abundant bacteria, significantly varying from more than 55% in fish fed the L-FM/FO diet to 67.8% in EWH fish. In parallel, a significant decrease in the phylum Firmicutes was found both in L-FM/FO and EWH (16.6–16.8%) groups in comparison to CTRL fish (26.5%). Conversely, the phylum Actinobacteria raised up from ∼6% in CTRL and EWH fish to 18.2% in fish fed the L-FM/FO diet. Finally, in EWH fish, the less abundant Bacteroidetes and Spirochaetes phyla were significantly increased, with values of 2.8% and 2.2%, respectively.
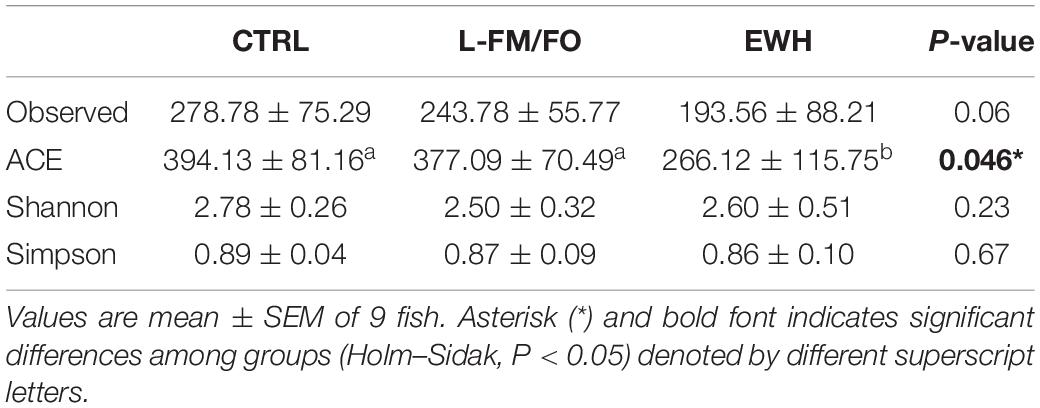
Table 3. Species richness estimators (observed and ACE) and diversity indexes (Shannon and Simpson) of fish fed CTRL, L-FM/FO, and EWH diets.
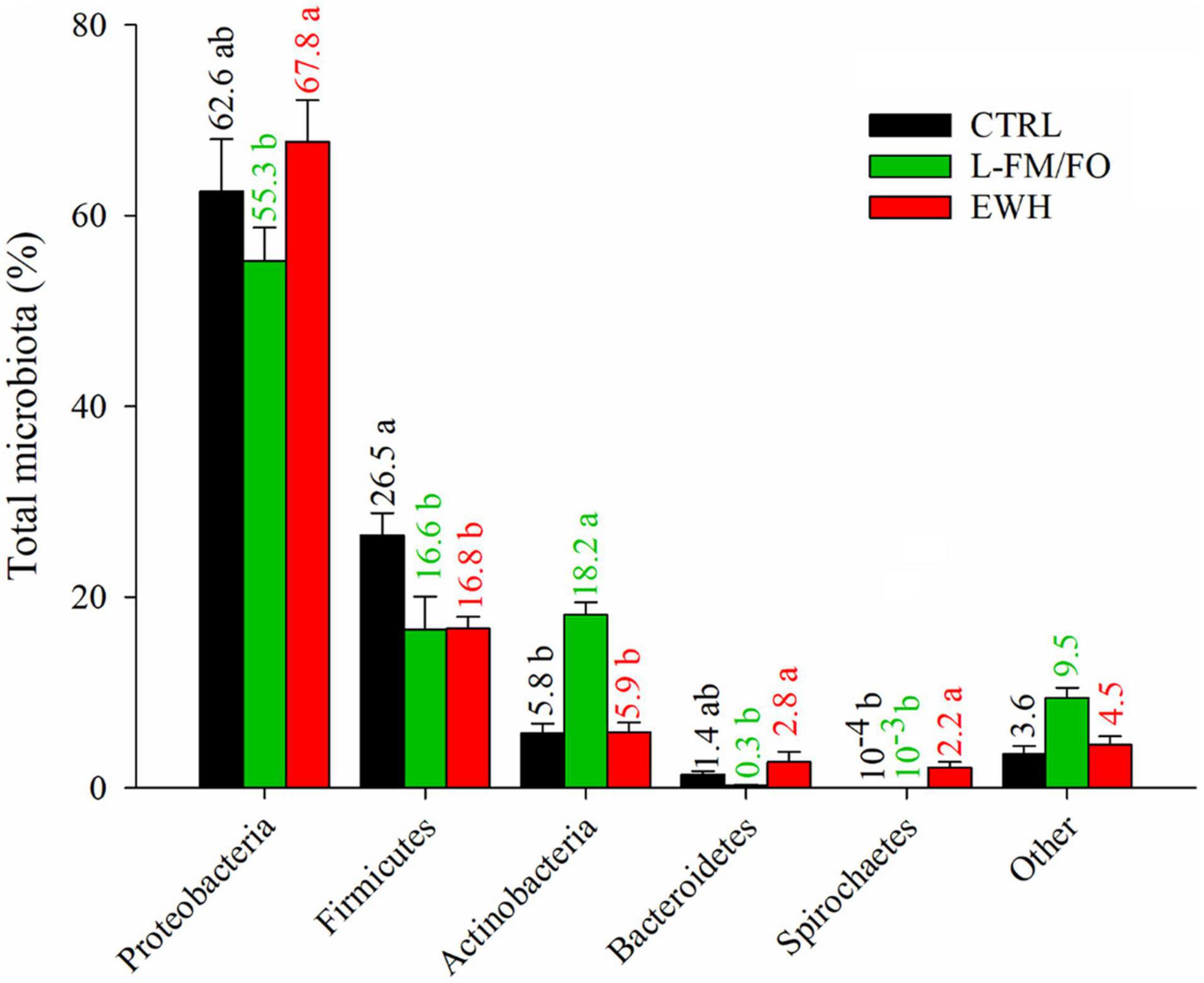
Figure 4. Relative abundance of bacterial phyla in the anterior intestine of fish fed CTRL (black bars), L-FM/FO (green bars), and EWH (red bars) diets. Significant differences (Kruskal–Wallis, Dunn’s post-test, P < 0.05) are indicated by different letters, which correspond to pairwise comparisons within each phylum among dietary groups. The numbers above each bar represent the mean abundance in percentage for each group.
Microbiota Discriminant Analysis
Permutational multivariate analysis of variance test highlighted statistically significant differences in bacterial composition when comparing animals fed different diets (P = 0.048, F = 1.115, R2 = 0.085). Although R2 values detected were quite low, they were in line with what was reported in other microbiota studies (He et al., 2018) due to the complexity and variability of microbiota samples. To validate and study these differences in more detail, a PLS-DA model (R2Y = 99%, Q2 = 70%) with three score components was constructed and statistically validated (Figure 5A and Supplementary Figure 2). The first two components explained more than 80% of total variance, clearly separating CTRL fish from fish fed L-FM/FO diets along x-axis (component 1, 37.4%), whereas component 2 (43.2%) separated the L-FM/FO diets with/without EWH along y-axis. To determine which groups of bacteria were driving these separations at a high level of confidence, the minimum VIP value driving the correct separation of groups in the model was determined throughout a heatmap representation (Figure 5B). Such approach disclosed 165 OTUs (VIP ≥ 1.2), which can be accessed in Supplementary Table 3.
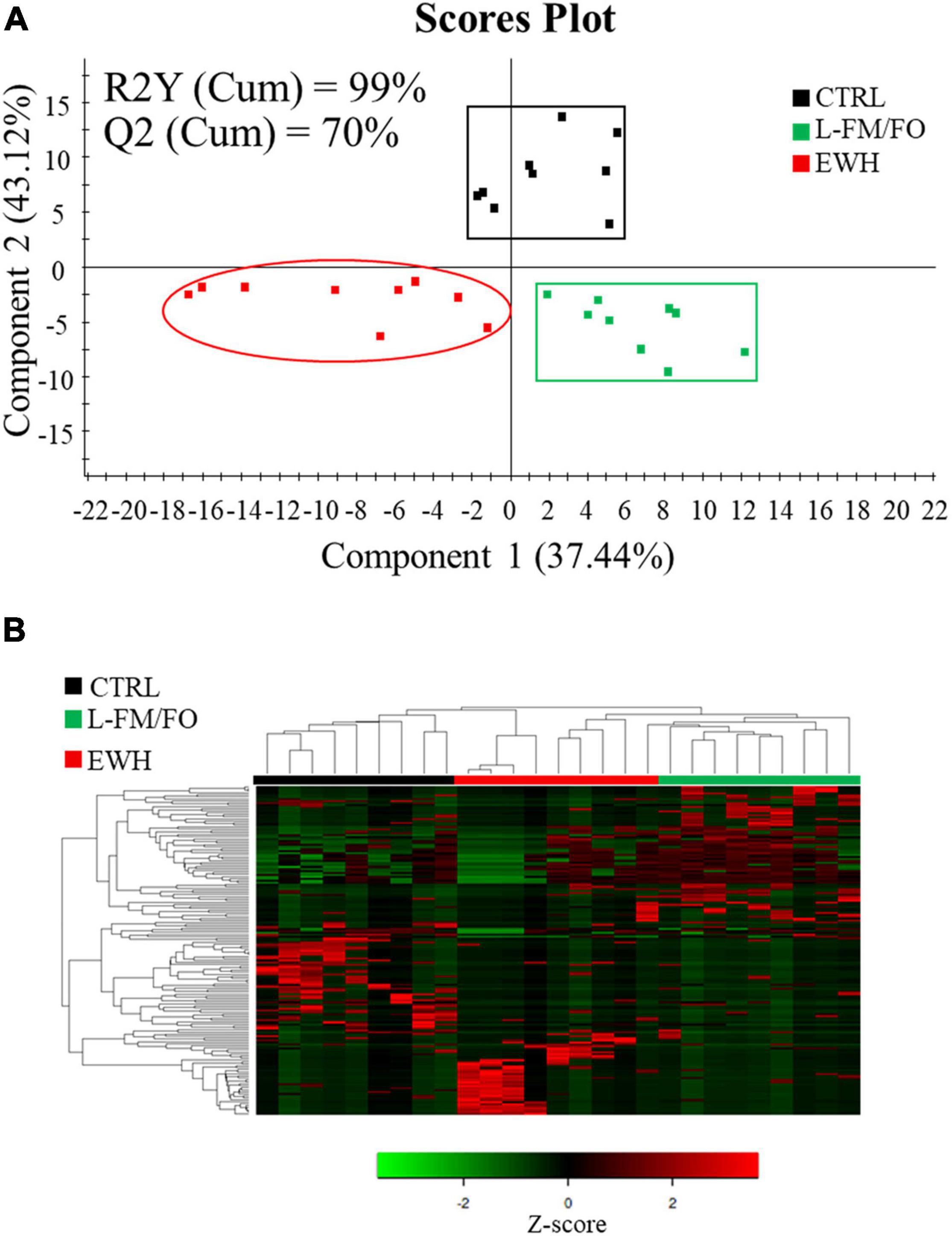
Figure 5. (A) Two-dimensional PLS-DA scores plot constructed using the variable diet. The validation by the permutation test can be found in Supplementary Figure 2. (B) Heatmap representing the abundance distribution (Z-score) of the OTUs identified to be driving the separation by diet among all dietary groups.
Figure 6 shows the list of most abundant bacteria (at least 1% in one of the groups; 46 OTUs out of the 165 with VIP ≥ 1.2) that exclusively drove the separation by dietary groups. For these abundant bacteria, a first type of response was mediated by 16 OTUs that were increasing with the FM/FO replacement and decreasing again in EWH fish. In this group, the presence of Neisseriaceae family and species of Ralstonia, Lactobacillus, Streptococcus, Corynebacterium, and Nocardioides genera was remarkable. A second type of response grouped 15 OTUs present in a significant proportion in the CTRL group, but decreasing in fish fed the two L-FM/FO diets. In this case, dietary plant ingredients produced the decrease of the Comamonadaceae family and Novosphingobium, Mesorhizobium, Klebsiella, Acinetobacter, Brochotrix, Bacillus, Clostridium sensu stricto, and Exiguobacterium genera. The remaining 15 OTUs increased their proportion in fish fed the EWH diet, being in a very low proportion in the other two dietary groups. This response triggered the presence of Bacteroidetes and Spirochaetes phyla, and more specifically of the Flavobacteriaceae family and Cloacibacterium genus. The Rhodospirillales order also increased with the addition of EWH, as well as Granulicatella, Serratia, Bradyrhizobium, Propionibacterium, and Photobacterium genera.
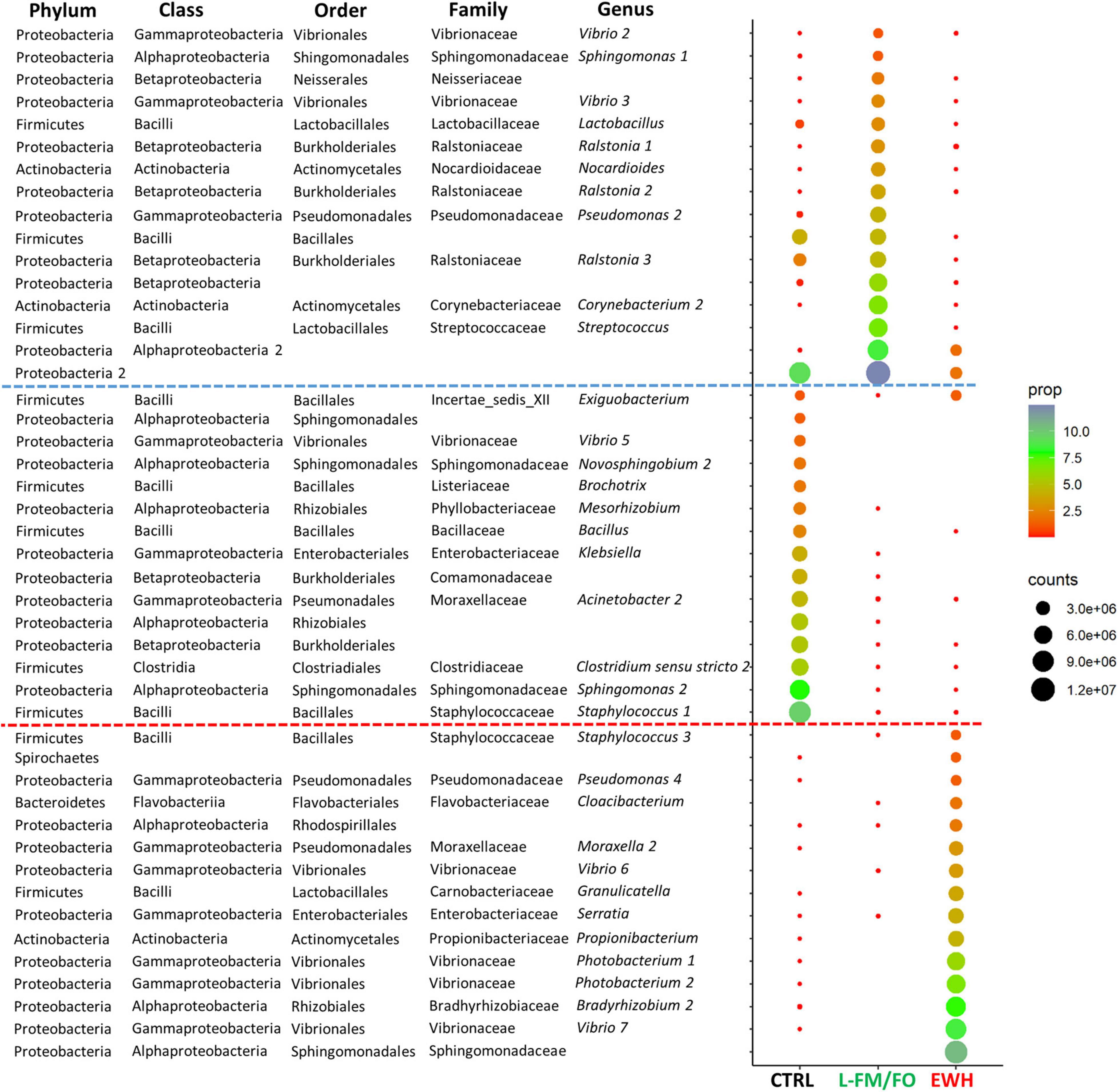
Figure 6. Dotplot map depicting the most abundant genera (more than 1% of the total microbiota in at least one dietary group) from the 165 significant OTUs identified in Figure 5B. The size of the dots represents the normalized counts in each dietary group (CTRL, L-FM/FO, and EWH). The color scale represents the mean abundance, in percentage, of each genus within each group. OTUs above the blue dotted line showed an increased abundance in L-FM/FO groups; OTUs between the dotted lines showed an increased abundance in the CTRL group; OTUs below the red dotted line showed an increased abundance in the EWH group. The numbers after the genus names correspond to different OTUs assigned to the same genus that probably belong to different species.
Inferred Metagenome and Pathway Analysis
With the aim of assessing the biological significance of the diet-induced differences in the microbiota of the different groups, a pathway analysis was conducted with the inferred metagenomes of the 165 OTUs that drove the separation by diet (Table 4). The results showed that 15 pathways could be significantly changing in the comparison between fish fed CTRL and the L-FM/FO diet without EWH, whereas the comparison between EWH and CTRL groups rendered 28 pathways. In both comparisons, pathways related to signaling pathways of rat sarcoma (RAS), sphingolipids, GnRH, cAMP, and Fc gamma R-mediated phagocytosis were strongly overrepresented in the two groups of fish fed L-FM/FO diets, whereas Staurosporine biosynthesis, neuroactive ligand–receptor interaction and cholesterol metabolism were underrepresented. By contrast, only two pathways corresponding to primary bile acid biosynthesis and steroid degradation were consistently underrepresented in the microbiota of EWH fish when comparisons are made with the other two groups. This was coupled to an overrepresentation of the longevity regulating pathway.
Discussion
Enzymatic hydrolysis of animal and plant proteins has been used as a basic method for the conversion of underused protein products into highly digestible peptides (Benjakul et al., 2014; Egerton et al., 2018a). Additionally, protein hydrolysates containing antioxidant peptides possess a high therapeutic potential for the management of chronic diseases, but also as safe additives to halt lipid peroxidation, improving the quality and consumer satisfaction of several food products (Auwal et al., 2017; Cicero et al., 2017). Thus, the antioxidants and anti-inflammatory properties of EWH have shown beneficial effects in different experimental rat models (Requena et al., 2017), and we discussed herein the potential benefits of dietary EWH supplementation in fish fed experimental diets with a high replacement of marine feedstuffs by alternative plant ingredients.
From our results, it is conclusive that dietary EWH supplementation triggered a reduced feed intake and a slight impairment of feed conversion ratio in gilthead sea bream. In rodents, EWH administration reduced body weight gain in obese animals, and this decrease was related to a reduced deposit of fat in different tissues, especially white adipose tissue, but no effects on growth or food intake were observed in obese or control rats fed EWH (Miguel et al., 2006; Moreno-Fernández et al., 2018a). It should be also noted that proteins in their natural state do not contribute to the flavor of food, but hydrolyzed derived peptides can modify the sensory quality of proteins causing food rejection (Iwaniak et al., 2019). Therefore, we cannot exclude a taste effect on the apparent satiety effect of the EWH in our experimental gilthead sea bream model. Moreover, fish protein hydrolysates, absent in EWH diet, are known feed attractants in aquaculture, which could enhance the feed palatability of CTRL and L-FM/FO diets (Kasumyan and Døving, 2003; Aguila et al., 2007). Alternatively, the modulation of the intestinal microbiota, particularly with respect to production of SCFA, might also contribute to explain the observed effects on growth and feed intake. SCFA, such as butyrate, propionate and acetate, are end products of microbial fermentation implicated in a multitude of physiological functions (Morrison and Preston, 2016), but similar to fiber, protein fermentation also produces SCFA (Macfarlane, 1992). However, while propionate production remains relatively stable, the rate of acetate and butyrate production is lowered when they are generated from protein fermentation (Aguirre et al., 2016). In agreement with this, the intestinal concentration of propionate was much higher than for other SCFA in our model of a carnivorous fish fed hyperproteic diets. It is difficult to categorize individual SCFA into purely obesogenic or anti-obesogenic, though acetate seems to be predominantly obesogenic, whereas butyrate and propionate are broadly anti-obesogenic (Chakraborti, 2015). The beneficial effects of butyrate have been reported in a large extent in gilthead sea bream as a highly promising additive to counteract undesired effects of plant-based diets at the local and systemic level (Robles et al., 2013; Benedito-Palos et al., 2016; Piazzon et al., 2017; Simó-Mirabet et al., 2018). However, we found than intestinal butyrate was below the detection limit in all the studied groups, whereas the highest concentration of propionate was achieved in EWH fish, and its relevance is further discussed later on.
Gut microbiota studies are emerging as effective approaches for promoting farmed fish health, contributing to improve the productivity of the aquaculture sector (Brugman et al., 2018; Egerton et al., 2018b; Egan et al., 2020). In this regard, it is noteworthy that measurements of gut microbiota diversity are considered a good indicator of animal health, becoming dietary factors one of the main regulators of intestinal microbial diversity (Moschen et al., 2012). Thus, the ability of replacement diets and/or feed additives to retain a gut microbiome composition close to that of fish fed diets with high contents of FM and FO is envisaged in gilthead sea bream (Fontinha et al., 2021), as well as in other fish species of interest in aquaculture (Egerton et al., 2020; Niu et al., 2020). Though it exists a high variability of response to protein supplements across different animal models (Clarke et al., 2014; Liu et al., 2014; Butteiger et al., 2016; Beaumont et al., 2017), we did not detect changes in gut microbiota diversity of gilthead sea bream fed EWH diet. Regarding gut microbiota richness, a negative correlation with obesity has been largely reported in humans (Turnbaugh et al., 2008; Le Chatelier et al., 2013; Sze and Schloss, 2016; Peters et al., 2018). However, recent evidence suggests that this association cannot be considered as widespread among the population (Stanislawski et al., 2019). Similarly, the lower microbiota richness of our EWH fish with a reduced feed intake was mainly driven by three animals with extreme low richness values (Supplementary Figure 1).
Despite all the above findings, changes in the composition of mucosal adherent bacterial communities are already found at the phylum level (Figure 4). Proteobacteria, Firmicutes, Actinobacteria, and Bacteroidetes Phyla dominated the autochthonous microbiota of the intestine of fish, as it has been also observed in previous studies in this species (Kormas et al., 2014; Estruch et al., 2015; Piazzon et al., 2019), with a fifth phylum, Spirochaetes, increasing in EWH fish. Furthermore, discriminant analysis (Figure 5) helped to disclose 46 dominant bacteria (VIP ≥ 1.2, >1% in abundance) (Figure 6) with a significantly higher presence associated to a particular diet, and a decrease in the other groups. This fact revealed a specific organization of the gut microbiota in response to each dietary treatment, which allowed to discover differences at all taxonomic levels. Firstly, Proteobacteria (facultative anaerobic organisms) were presented in all analyzed fish, as it is considered one of the most abundant symbionts in marine fish because of their highly flexible metabolic properties (Tarnecki et al., 2017; Ikeda-Ohtsubo et al., 2018). Within this phylum, high abundance values were found for the Vibrionaceae family in all dietary groups. Species of this family help to digest organic substances due to the production of lipases, amylases and proteases, but some of those species can also produce harmful enzymes like neuraminidases and act as causative agents of fish pathologies (Egerton et al., 2018b).
Firmicutes are also some common intestinal symbionts in fish and mammals (Lozupone et al., 2012; Ghanbari et al., 2015). In our farmed fish, Firmicutes ranged from 26.5% in the CTRL group to ∼17% in the L-FM/FO and EWH groups. Both plant-based diets shared important proportions of Lactobacillales, known to inhibit fish pathogens due to the natural production of bacteriocins (Balcázar et al., 2007; Sugita et al., 2007; Shahid et al., 2017; Ringø et al., 2018). This abundance of Lactobacillales in plant-based diets is caused by their ability to use indigestible fiber and fermentable polysaccharides for their metabolism and growth (Gajardo et al., 2017; Theilmann et al., 2017). In the last decade, Lactobacillales have been extensively studied due to their potential use as probiotics (Gillor et al., 2008; Heo et al., 2012), with particular importance to aquaculture, where the avoidance of the use of antibacterial drugs for facing fish pathogens is one of the main challenges (Sahoo et al., 2016). Bacteria of the family Carnobacteriaceae and of the genera Lactobacillus and Streptococcus, all of them present in our plant-based dietary groups, are prone to produce these antimicrobial agents (Elayaraja et al., 2014). However, the remarkable proportion of Streptococcus in L-FM/FO can also display negative effects as this genus has been described among the reduced group of bacteria capable of producing all the enzymes needed for complete mucin degradation (Derrien et al., 2010). Hence, the goblet cell depletion observed on the L-FM/FO fish could be partly explained by the higher abundance of this genus, which is practically not present in the CTRL and EWH groups. Protective mucus at the intestinal mucosa consists of a gel overlying the epithelium based on the production and secretion of mucins, mostly by goblet cells but also by enterocytes (Pelaseyed et al., 2014). The amount of goblet cells of the AI was altered by FM/FO replacement, but interestingly the profile of CTRL fish was restored by EWH supplementation, suggesting that EWH could be re-stimulating mucus secretion in the AI. In a similar manner, milk-derived peptides have already demonstrated to stimulate rat intestinal mucus secretion and improve intestinal barrier (Giromini et al., 2019). Here we hypothesize that these effects could be, at least in part, induced by the modulation of mucin degrading bacterial populations.
The phylum Bacteroidetes increased in EWH fish, with a predominance of bacteria assigned as Cloacibacterium. In gilthead sea bream, fermentation produced by species of this genus is a major process for the metabolism of glucose in SCFA that might be used later in other chemoautotrophic processes (Kormas et al., 2014). The Firmicutes/Bacteroidetes (F/B) ratio is a widely documented factor correlated with obesity in mammals. Changes in these phyla proportions are regarded as dysbiosis (Stojanov et al., 2020), and an increased F/B ratio has been related with obesity in humans (Ley et al., 2006) and rats (Requena et al., 2017). However, the correlation between obesity and F/B ratio in mammals can be controversial (Magne et al., 2020) and has not been demonstrated in fish. In any case, in our fish model, Firmicutes suffered a decrease from 26.5% in CTRL group to ∼17% in the plant-based diets, whereas Bacteroidetes were only increased in the EWH group. Thus EWH fish showed the lowest F/B ratio (6 in EWH fish vs. 18.9 and 55.3 in CTRL and L-FM/FO fish, respectively). Firmicutes and Bacteroidetes represent more than 90% of the total bacterial communities in mammals (Magne et al., 2020), whereas, in fish, Proteobacteria are among the most abundant. Thus, although our results are in agreement with previous studies in mammals, further works are needed to determine the validity of this ratio in fish, and the possible implication of Proteobacteria in these correlations.
A wide range of Spirochaetes is found in aquatic habitats, but this phylum usually comprises a low proportion (<1%) of fish intestinal microbiota (Givens et al., 2015; Le and Wang, 2020). In mammals, the presence or increase of Spirochaetes has been associated to lean individuals in fecal microbiomes of captive cynomolgus monkeys (Koo et al., 2019) and in oral microbiomes of diabetes mellitus type 2 patients (Tam et al., 2018). Concordantly, in this study, the addition of EWH in the diet significantly increased the abundance of this phylum up to a 2.2%, pointing to a potential role of these bacteria in the reduced feed intake and decreased weight gain of this group of fish.
The phylum Actinobacteria significantly increased in the L-FM/FO group, mainly due to the increase in Corynebacterium and Nocardioides, which represented ∼10% of the overall bacterial population in this dietary group. These bacteria have been described to produce polysaccharide hydrolases (Anandan et al., 2016), which is compatible with the higher fiber content of our plant-based diets. Lastly, 73% of the total Actinobacteria found in the EWH group belongs to the genus Propionibacterium, present in significantly lower proportions in the other two groups. Since Propionibacterium is the best natural producer of propionate (Zárate, 2012; González-Garcia et al., 2017), this observation supported the higher concentrations of intestinal propionic acid in fish fed the EWH diet. Microbial production of propionate has been related to a healthier gut state (Hosseini et al., 2011; Louis and Flint, 2017), lowering lipogenesis (Weitkunat et al., 2016) and triggering the secretion of satiety peptides, such as glucagon-like peptide-1 and peptide YY (Chambers et al., 2015). Moreover, propionate acts as an inhibitory factor of food intake via its antagonism with the cholesterol synthesis (Harris et al., 2012; Chakraborti, 2015). In agreement with this, we found herein that primary bile acid biosynthesis and steroid degradation were consistently underrepresented in the inferred metagenome of EWH fish in comparison to the other two groups (Table 4). To clarify, these results do not imply that bacteria are expressing primary bile acid biosynthesis genes, but that some bacteria within the detected populations might be expressing molecules that could affect such pathway. Indeed, bile acids represent a significant host factor that modulates the microbiome of obese mice and the digestion and absorption of dietary lipids (Zheng et al., 2017). Likewise, in the present study, the down-regulation of bile acids biosynthesis, together with the decreased F/B ratio and the increased Spirochaetes phylum in the EWH group could be describing the link between the bioactive egg white hydrolysate and an anti-obesogenic response. This assumption is supported by the reduced lipid vacuolization in intestines, and by the restoration of normal liver fat deposition in association with an increase in the number of hepatic melanomacrophage centers, as already found during feed restriction in lesser guitarfish (Zapteryx brevirostris) (Neyrão et al., 2019). Studies addressing the gut metatranscriptome in close association to host changes of metabolism and intestinal transcriptome should be conducted to validate this hypothesis and unravel the molecular interactions behind the effects.
In summary, altogether, these results reinforce the central role of gut microbiota in the regulation of host metabolism and lipid metabolism in particular, which might suggest a role of the EWH derived bioactive peptides as an anti-obesity and/or satiety factor in fish, although the ultimate mechanisms of action still remains to be established. From a practical point of view, the potential use of this functional food ingredient in finishing diets, and the role of gut microbiota in tuning filet fatty acid composition of marketable fish merits further research.
Data Availability Statement
The datasets presented in this study can be found in online repositories. The names of the repository/repositories and accession number(s) can be found below: https://www.ncbi.nlm.nih.gov/, SAMN18105342-68 and PRJNA705868.
Ethics Statement
The animal study was reviewed and approved by Ethics and Animal Welfare Committee of the Institute of Aquaculture Torre de la Sal (IATS-CSIC, Castellón, Spain), CSIC (Permit number 869/2019) and “Generalitat Valenciana” (permit number 2020/VSC/PEA/0010).
Author Contributions
FN-C, MCP, IE, MM, and JP-S contributed to the formal analysis. FN-C, MM, JP-S, and MCP contributed to the writing-original manuscript. IE, AS-B, GW, ML-M, JC-G, MCP, and TR contributed to the writing-review and editing. GW, MM, AS-B, and JP-S contributed to the conceptualization. All authors read and approved the final manuscript and contributed to the experimental investigation.
Funding
This work was supported by the EU H2020 Research Innovation Program under the TNA Program (project AE150009) at IATS-CSIC Research Infrastructure within AQUAEXCEL2020 Project (652831). This output reflects only the author’s view and the European Union cannot be held responsible for any use that may be made of the information contained herein. Additional funding was obtained by a Spanish MICINN project (Bream-AquaINTECH and RTI2018–094128-B-I00). MCP was funded by a Ramón y Cajal Postdoctoral Research Fellowship [RYC2018-024049-I/AEI/10.13039/501100011033 co-funded by the European Social Fund (ESF) and ACOND/2020 Generalitat Valenciana].
Conflict of Interest
The authors declare that the research was conducted in the absence of any commercial or financial relationships that could be construed as a potential conflict of interest.
Publisher’s Note
All claims expressed in this article are solely those of the authors and do not necessarily represent those of their affiliated organizations, or those of the publisher, the editors and the reviewers. Any product that may be evaluated in this article, or claim that may be made by its manufacturer, is not guaranteed or endorsed by the publisher.
Acknowledgments
We thank to Paula Simó-Mirabet and Enrique Rosell-Moll for their assistance in the fish handling and feeding during the trial, and J. Monfort and L. Rodríguez from the histology Service of the IATS-CSIC for histological processing.
Supplementary Material
The Supplementary Material for this article can be found online at: https://www.frontiersin.org/articles/10.3389/fmars.2021.698484/full#supplementary-material
Footnotes
References
Aguila, J., Cuzon, G., Pascual, C., Domingues, P. M., Gaxiola, G., Sánchez, A., et al. (2007). The effects of fish hydrolysate (CPSP) level on Octopus maya (Voss and Solis) diet: digestive enzyme activity, blood metabolites, and energy balance. Aquaculture 273, 641–655. doi: 10.1016/j.aquaculture.2007.07.010
Aguirre, M., Eck, A., Koenen, M. E., Savelkoul, P. H. M., Budding, A. E., and Venema, K. (2016). Diet drives quick changes in the metabolic activity and composition of human gut microbiota in a validated in vitro gut model. Res. Microbiol. 167, 114–125.
Altschul, S. F., Gish, W., Miller, W., Myers, E. W., and Lipman, D. J. (1990). Basic local alignment search tool. J. Mol. Biol. 215, 403–410. doi: 10.1016/S0022-2836(05)80360-2
Anandan, R., Dharumadurai, D., and Manogaran, G. P. (2016). “An introduction to Actinobacteria,” in Actinobacteria: Basics and Biotechnological Applications, eds D. Dhanasekaran and Y. Jiang (Rijeka: InTechOpen), 3–38. doi: 10.5772/62329
Aoun, A., Darwish, F., and Hamod, N. (2020). The Influence of the Gut Microbiome on Obesity in Adults and the Role of Probiotics, Prebiotics, and Synbiotics for Weight Loss. Prev. Nutr. Food Sci. 25, 113–123. doi: 10.3746/pnf.2020.25.2.113
Aronesty, E. (2013). Comparison of sequencing utility programs. Open Bioinform. J. 7, 1–8. doi: 10.2174/1875036201307010001
Auwal, S. M., Zarei, M., Abdul-Hamid, A., and Saari, N. (2017). Response surface optimisation for the production of antioxidant Hydrolysates from stone fish protein using Bromelain. Evid. Based Complement. Alternat. Med. 2017:4765463. doi: 10.1155/2017/4765463
Balcázar, J. L., Vendrell, D., de Blas, I., Ruiz-Zarzuela, I., Gironés, O., and Múzquiz, J. L. (2007). In vitro competitive adhesion and production of antagonistic compounds by lactic acid bacteria against fish pathogens. Vet. Microbiol. 122, 373–380. doi: 10.1016/j.vetmic.2007.01.023
Beaumont, M., Portune, K. J., Steuer, N., Lan, A., Cerrudo, V., Audebert, M., et al. (2017). Quantity and source of dietary protein influence metabolite production by gut microbiota and rectal mucosa gene expression: a randomized, parallel, double-blind trial in overweight humans. Am. J. Clin. Nutr. 106, 1005–1019. doi: 10.3945/ajcn.117.158816
Benedito-Palos, L., Ballester-Lozano, G. F., Simó, P., Karalazos, V., Ortiz, Á, Calduch-Giner, J., et al. (2016). Lasting effects of butyrate and low FM/FO diets on growth performance, blood haematology/biochemistry and molecular growth-related markers in gilthead sea bream (Sparus aurata). Aquaculture 454, 8–18. doi: 10.1016/j.aquaculture.2015.12.008
Benjakul, S., Yarnpakdee, S., Senphan, T., Halldorsdottir, S. M., and Kristinsson, H. G. (2014). “Fish protein hydrolysates: production, bioactivities and applications,” in Antioxidants and functional components in aquatic foods, ed. H. G. Kristinsson (Chichester: John Wiley & Sons), 237–281. doi: 10.1002/9781118855102.ch9
Bledsoe, J. W., Peterson, B. C., Swanson, K. S., and Small, B. C. (2016). Ontogenetic characterization of the intestinal microbiota of channel catfish through 16S rRNA gene sequencing reveals insights on temporal shifts and the influence of environmental microbes. PLoS One 11:e0166379. doi: 10.1371/journal.pone.0166379
Brown, L., Poudyal, H., and Panchal, S. K. (2015). Functional foods as potential therapeutic options for metabolic syndrome. Obes. Rev. 16, 914–941. doi: 10.1111/obr.12313
Brugman, S., Ikeda-Ohtsubo, W., Braber, S., Folkerts, G., Pieterse, C., and Bakker, P. (2018). A comparative review on microbiota manipulation: lessons from fish, plants, livestock, and human research. Front. Nutr. 5:80. doi: 10.3389/fnut.2018.00080
Butteiger, D. N., Hibberd, A. A., McGraw, N. J., Napawan, N., Hall-Porter, J. M., and Krul, E. S. (2016). Soy protein compared with milk protein in a western diet increases gut microbial diversity and reduces serum lipids in golden Syrian hamsters. J. Nutr. 146, 697–705. doi: 10.3945/jn.115.224196
Chakraborti, C. K. (2015). New-found link between microbiota and obesity. World J. Gastrointest. Pathophysiol. 6, 110–119. doi: 10.4291/wjgp.v6.i4.110
Chambers, E. S., Viardot, A., Psichas, A., Morrison, D. J., Murphy, K. G., Zac-Varghese, S. E., et al. (2015). Effects of targeted delivery of propionate to the human colon on appetite regulation, body weight maintenance and adiposity in overweight adults. Gut 64, 1744–1754. doi: 10.1136/gutjnl-2014-307913
Chávez-Talavera, O., Tailleux, A., Lefebvre, P., and Staels, B. (2017). Bile acid control of metabolism and inflammation in obesity, type 2 diabetes, dyslipemia, and non-alcoholic fatty liver disease. Gastroenterology 7, 1679–1694. doi: 10.1053/j.gastro.2017.01.055
Cicero, A. F., Fogacci, F., and Colletti, A. (2017). Potential role of bioactive peptides in prevention and treatment of chronic diseases: a narrative review. Br. J. Pharmacol. 174, 1378–1394. doi: 10.1111/bph.13608
Clarke, S. F., Murphy, E. F., O’sullivan, O., Lucey, A. J., Humphreys, M., Hogan, A., et al. (2014). Exercise and associated dietary extremes impact on gut microbial diversity. Gut 63, 1913–1920. doi: 10.1136/gutjnl-2013-306541
Cole, J. R., Wang, Q., Fish, J. A., Chai, B., McGarrell, D. M., Sun, Y., et al. (2014). Ribosomal database project: data and tools for high throughput rRNA analysis. Nucleic Acids Res. 42, D633–D642. doi: 10.1093/nar/gkt1244
Dávalos, A., Miguel, M., Bartolomé, B., and López-Fandiño, R. (2004). Antioxidant Activity of peptides derived from egg white proteins by enzymatic hydrolysis. J. Food Protect. 67, 1939–1944. doi: 10.4315/0362-028x-67.9.1939
Davis, C. D. (2016). The gut microbiome and its role in obesity. Nutr. Today 51, 167–174. doi: 10.1097/NT.0000000000000167
Derrien, M., van Passel, M. W., van de Bovenkamp, J. H., Schipper, R. G., de Vos, W. M., and Dekker, J. (2010). Mucin-bacterial interactions in the human oral cavity and digestive tract. Gut Microbes 1, 254–268. doi: 10.4161/gmic.1.4.12778
Egan, S., Fukatsu, T., and Francino, M. P. (2020). Opportunities and challenges to microbial symbiosis research in the microbiome era. Front. Microbiol. 11:1150. doi: 10.3389/fmicb.2020.01150
Egerton, S., Culloty, S., Whooley, J., Stanton, C., and Ross, R. P. (2018a). Characterization of protein hydrolysates from blue whiting (Micromesistius poutassou) and their application in beverage fortifcation. Food Chem. 245, 698–706. doi: 10.1016/j.foodchem.2017.10.107
Egerton, S., Culloty, S., Whooley, J., Stanton, C., and Ross, R. P. (2018b). The gut microbiota of marine fish. Front. Microbiol. 9:873. doi: 10.3389/fmicb.2018.00873
Egerton, S., Wan, A., Murphy, K., Collins, F., Ahern, G., Sugrue, I., et al. (2020). Replacing fishmeal with plant protein in Atlantic salmon (Salmo salar) diets by supplementation with fish protein hydrolysate. Sci. Rep. 10:4194. doi: 10.1038/s41598-020-60325-7
Elayaraja, S., Annamalai, N., Mayavu, P., and Balasubramanian, T. (2014). Production, purification and characterization of bacteriocin from Lactobacillus murinus AU06 and its broad antibacterial spectrum. Asian Pac. J. Trop. Biomed. 4, S305–S311. doi: 10.12980/APJTB.4.2014C537
Estensoro, I., Ballester-Lozano, G. F., Benedito-Palos, L., Grammes, F., Martos-Sitcha, J. A., Mydland, L. T., et al. (2016). Dietary butyrate helps to restore the intestinal status of a marine teleost (Sparus aurata) fed extreme diets low in fish meal and fish oil. PLoS One 11:e0166564. doi: 10.1371/journal.pone.0166564
Estruch, G., Collado, M. C., Penaranda, D. S., Tomas Vidal, A., Jover Cerda, M., Perez Martinez, G., et al. (2015). Impact of fishmeal replacement in diets for gilthead sea bream (Sparus aurata) on the gastrointestinal microbiota determined by pyrosequencing the 16S rRNA gene. PLoS One 10:e0136389. doi: 10.1371/journal.pone.0136389
Fontinha, F., Magalhães, R., Moutinho, S., Santos, R., Campos, P., Serra, C. R., et al. (2021). Effect of dietary poultry meal and oil on growth, digestive capacity, and gut microbiota of gilthead seabream (Sparus aurata) juveniles. Aquaculture 530:735879. doi: 10.1016/j.aquaculture.2020.735879
Gajardo, K., Jaramillo-Torres, A., Kortner, T. M., Merrifield, D. L., Tinsley, J., Bakke, A. M., et al. (2017). Alternative protein sources in the diet modulate microbiota and functionality in the distal intestine of Atlantic Salmon (Salmo salar). Appl. Environ. Microbiol. 83:e02615-16. doi: 10.1128/AEM.02615-16
Garcés-Rimón, M., González, C., Uranga, J., López-Miranda, V., López-Fandiño, R., and Miguel, M. (2016a). Pepsin egg white hydrolysate ameliorates obesity-related oxidative stress, inflammation and steatosis in Zucker fatty rats. PLoS One 11:e0151193. doi: 10.1371/journal.pone.0151193
Garcés-Rimón, M., López-Expósito, I., López-Fandiño, R., and Miguel, M. (2016b). Egg white hydrolysates with in vitro biological multi-activity to control complications associated to metabolic syndrome. Eur. Food Res. Technol. 242, 61–64. doi: 10.1007/s00217-015-2518-7
Ghanbari, M., Kneifel, W., and Domig, K. J. (2015). A new view of the fish gut microbiome: advances from next-generation sequencing. Aquaculture 448, 464–475. doi: 10.1016/j.aquaculture.2015.06.033
Gillor, O., Etzion, A., and Riley, M. A. (2008). The dual role of bacteriocins as anti- and probiotics. Appl. Microbiol. Biotechnol. 81, 591–606. doi: 10.1007/s00253-008-1726-5
Gil-Solsona, R., Calduch-Giner, J., Nácher-Mestre, J., Lacalle-Bergeron, L., Sancho, J. V., Hernández, F., et al. (2019). Contributions of MS metabolomics to gilthead sea bream (Sparus aurata) nutrition. Serum fingerprinting of fish fed low fish meal and fish oil diets. Aquaculture 498, 503–512. doi: 10.1016/j.aquaculture.2018.08.080
Giromini, F. C., Rebucci, R., and Baldi, A. (2019). Dairy proteins and bioactive peptides: modeling digestion and the intestinal barrier. J. Dairy Sci. 102, 929–942. doi: 10.3168/jds.2018-15163
Givens, C. E., Ransom, B., Bano, N., and Hollibaugh, J. T. (2015). Comparison of the gut microbiomes of 12 bony fish and 3 shark species. Mar. Ecol. Prog. Ser. 518, 209–223. doi: 10.3354/meps11034
Gomes Pinheiro, J. E. J., Martinez, C. S., Zambelli Moraes, P., Stasiaki, J. E., Trost, M. E., Vassallo, D. V., et al. (2020). Bioactive peptides derived from egg white reduce cadmium-induced damage in the reproductive system. J. Funct. Foods 67:103823. doi: 10.1016/j.jff.2020.103823
González-Garcia, R. A., McCubbin, T., Navone, L., Stowers, C., Nielsen, L. K., and Marcellin, E. (2017). Microbial propionic acid production. Fermentation 3:21. doi: 10.3390/fermentation3020021
Hao, W. L., and Lee, Y. K. (2004). Microflora of the gastrointestinal tract: a review. Methods Mol. Biol. 268, 491–502.
Harris, K., Kassis, A., Major, G., and Chou, C. J. (2012). Is the gut microbiota a new factor contributing to obesity and its metabolic disorders? J. Obes. 2012:879151. doi: 10.1155/2012/879151
Hasan, N., and Yang, H. (2019). Factors affecting the composition of the gut microbiota, and its modulation. PeerJ 7:e7502. doi: 10.7717/peerj.7502
He, Y., Wu, W., Zheng, H. M., Li, P., McDonald, D., Sheng, H. F., et al. (2018). Regional variation limits applications of healthy gut microbiome reference ranges and disease models. Nat. Med. 24, 1532–1535. doi: 10.1038/s41591-018-0164-x
Heo, W. S., Kim, E. Y., Kim, Y. R., Hossain, M. T., and Kong, I. S. (2012). Salt effect of nisin Z isolated from a marine fish on the growth inhibition of Streptococcus iniae, a pathogen of streptococcosis. Biotechnol. Lett. 34, 315–320. doi: 10.1007/s10529-011-0766-6
Hosseini, E., Grootaert, C., Verstraete, W., and Van de Wiele, T. (2011). Propionate as a health-promoting microbial metabolite in the human gut. Nutr. Rev. 69, 245–258. doi: 10.1111/j.1753-4887.2011.00388.x
Ikeda-Ohtsubo, W., Brugman, S., Warden, C. H., Rebel, J. M. J., Folkerts, G., and Pieterse, C. M. J. (2018). How can we define “optimal microbiota?”: a comparative review of structure and functions of microbiota of animals, fish, and plants in agriculture. Front. Nutr. 5:90. doi: 10.3389/fnut.2018.00090
Iwai, S., Weinmaier, T., Schmidt, B. L., Albertson, D. G., Poloso, N. J., Dabbagh, K., et al. (2016). Piphillin: improved prediction of metagenomic content by direct inference from human microbiomes. PLoS One 11:e0166104. doi: 10.1371/journal.pone.0166104
Iwaniak, A., Hrynkiewicz, M., Bucholska, J., Minkiewicz, P., and Darewicz, M. (2019). Understanding the nature ofbitter-taste di- and tripeptides derived from food proteins based on chemometric analysis. J. Food Biochem. 43:e12500. doi: 10.1111/jfbc.12500
Kasumyan, A. O., and Døving, K. B. (2003). Taste preferences in fishes. Fish Fish. 4, 289–347. doi: 10.1046/j.1467-2979.2003.00121.x
Koo, B. S., Hwang, E. H., Kim, G., Oh, H., Son, Y., Lee, D., et al. (2019). Evaluation of fecal microbiomes associated with obesity in captive cynomolgus monkeys (Macaca fascicularis). J. Vet. Sci. 20:e19. doi: 10.4142/jvs.2019.20.e19
Kormas, K. A., Meziti, A., Mente, E., and Frentzos, A. (2014). Dietary differences are reflected on the gut prokaryotic community structure of wild and commercially reared sea bream (Sparus aurata). Microbiol. Open 3, 718–728. doi: 10.1002/mbo3.202
Kousoulaki, K., Sæther, B. S., Albrektsen, S., and Noble, C. (2015). Review on European sea bass (Dicentrarchus labrax, Linnaeus, 1758) nutrition and feed management: a practical guide for optimizing feed formulation and farming protocols. Aquac. Nutr. 21, 129–151. doi: 10.1111/anu.12233
Le Chatelier, E., Nielsen, T., Qin, J., Prifti, E., Hildebrand, F., Falony, G., et al. (2013). Richness of human gut microbiome correlates with metabolic markers. Nature 500, 541–546. doi: 10.1038/nature12506
Le, M. H., and Wang, D. (2020). Structure and membership of gut microbial communities in multiple fish cryptic species under potential migratory effects. Sci. Rep. 10:7547. doi: 10.1038/s41598-020-64570-8
Lee, K. H., Song, Y., Wu, W., Yu, K., and Zhang, G. (2020). The gut microbiota, environmental factors, and links to the development of food allergy. Clin. Mol. Allergy 18:5. doi: 10.1186/s12948-020-00120-x
Leeming, E. R., Johnson, A. J., Spector, T. D., and Le Roy, C. I. (2019). Effect of diet on the gut microbiota: rethinking intervention duration. Nutrients 11:2862. doi: 10.3390/nu11122862
Ley, R., Turnbaugh, P., Klein, S., and Gordin, J. I. (2006). Human gut microbes associated with obesity. Nature 444, 1022–1023. doi: 10.1038/4441022a
Liu, X., Blouin, J. M., Santacruz, A., Lan, A., Andriamihaja, M., Wilkanowicz, S., et al. (2014). High-protein diet modifies colonic microbiota and luminal environment but notcolonocyte metabolism in the rat model: the increased luminal bulk connection. Am. J. Physiol. Gastrointest. Liver Physiol. 307, G459–G470. doi: 10.1152/ajpgi.00400.2013
Louis, P., and Flint, H. J. (2017). Formation of propionate and butyrate by the human colonic microbiota. Environ. Microbiol. 19, 29–41. doi: 10.1111/1462-2920.13589
Love, M. I., Huber, W., and Anders, S. (2014). Moderated estimation of fold change and dispersion for RNA-seq data with DESeq2. Genome Biol. 15:550. doi: 10.1186/s13059-014-0550-8
Lozupone, C. A., Stombaugh, J. I., Gordon, J. I., Jansson, J. K., and Knight, R. (2012). Diversity, stability and resilience of the human gut microbiota. Nature 489, 220–230. doi: 10.1038/nature11550
Macfarlane, G. T. (1992). Estimation of shot-chain fatty acid production from protein by human intestinal bacteria based on branched-chain fatty acid measurements. FEMS Microbiol. Lett. 101, 81–88. doi: 10.1111/j.1574-6968.1992.tb05764.x
Magne, F., Gotteland, M., Gauthier, L., Zazueta, A., Pesoa, S., Navarrete, P., et al. (2020). The Firmicutes/Bacteroidetes ratio: a relevant marker of gut dysbiosis in obese patients? Nutrients 12:1474. doi: 10.3390/nu12051474
Martínez, C. S., Alterman, C. D., Vera, G., Gallego, A. M., Uranga, J. A., Peçanha, F. M., et al. (2019). Egg White Hydrolysate as a functional food ingredient to prevent the toxic cognitive effects achieved after a long-term aluminum exposure in rats. Sci. Rep. 9:1868. doi: 10.1038/s41598-018-38226-7
McKnight, D. T., Huerlimann, R., Bower, D. S., Schwarzkopf, L., Alford, R. A., and Zenger, K. R. (2019). Methods for normalizing microbiome data: an ecological perspective. Methods Ecol. Evol. 10, 389–400. doi: 10.1111/2041-210X.13115
McMurdie, P. J., and Holmes, S. (2013). Phyloseq: an R package for reproducible interactive analysis and graphics of microbiome census data. PLoS One 8:e61217. doi: 10.1371/journal.pone.0061217
Miguel, M., López-Fandiño, R., Ramos, M., and Aleixandre, M. A. (2006). Long-term intake of egg white hydrolysate attenuates the development of hypertension in spontaneously hypertensive rats. Life Sci. 78, 2960–2966. doi: 10.1016/j.lfs.2005.11.025
Miguel, M., Recio, I., Gómez-Ruiz, J. A., Ramos, M., and López-Fandiño, R. (2004). Angiotensin I-converting enzyme inhibitory activity of peptides derived from egg white proteins by enzymatic hydrolysis. J. Food Protect. 67, 1914–1920. doi: 10.4315/0362-028x-67.9.1914
Moreno-Fernández, S., Garcés-Rimón, M., González, C., Uranga, J. A., López-Miranda, V., Vera, G., et al. (2018a). Pepsin egg white hydrolysate ameliorates metabolic syndrome in high-fat-high-dextrose fed rats. Food Funct. 9, 78–86. doi: 10.1039/c7fo01280b
Moreno-Fernández, S., Garcés-Rimón, M., Uranga, J. A., Astier, J., Landrier, J. F., and Miguel, M. (2018b). Expression enhancement in brown adipose tissue of genes related to thermogenesis and mitochondrial dynamics after administration of pepsin egg white hydrolysate. Food Funct. 9, 6600–6608. doi: 10.1039/c8fo01754a
Moroni, F., Naya-Català, F., Piazzon, M. C., Rimoldi, S., Calduch-Giner, J. À, Giardini, A., et al. (2021). The effects of nisin-producing Lactococcus lactis strain used as probiotic on gilthead sea bream (Sparus aurata) growth, gut microbiota, and transcriptional response. Front. Mar. Sci. 8:659519. doi: 10.3389/fmars.2021.659519
Morrison, D. J., and Preston, T. (2016). Formation of short chain fatty acids by the gut microbiota and their impact on human metabolism. Gut Microbes 7, 189–200. doi: 10.1080/19490976.2015.1134082
Moschen, A. R., Wieser, V., and Tilg, H. (2012). Dietary factors: major regulators of the gut’s microbiota. Gut Liver 6, 411–416. doi: 10.5009/gnl.2012.6.4.411
Moughan, P. J., Rutherfurd, S. M., Montoya, C. A., and Dave, L. A. (2014). Food-derived bioactive peptides–a new paradigm. Nutr. Res. Rev. 27, 16–20. doi: 10.1017/S0954422413000206
Neyrão, I. M., Conrado, A. L. V., Takatsuka, V., Malavasi Bruno, C. E., and Guedes de Azevedo, V. (2019). Quantification of liver lipid deposition and melano-macrophages in lesser guitarfish Zapteryx brevirostris submitted to different feeding cycles. Comp. Clin. Pathol. 28, 805–810. doi: 10.1007/s00580-019-02953-8
Niu, K. M., Lee, B. J., Kothari, D., Lee, W. D., Hur, S. W., Lim, S. G., et al. (2020). Dietary effect of low fish meal aquafeed on gut microbiota in olive flounder (Paralichthys olivaceus) at different growth stages. Microbiol. Open 9:e992. doi: 10.1002/mbo3.992
Pelaseyed, T., Bergström, J. H., Gustafsson, J. K., Ermund, A., Birchenough, G. M., Schütte, A., et al. (2014). The mucus and mucins of the goblet cells and enterocytes provide the first defense line of the gastrointestinal tract and interact with the immune system. Immunol. Rev. 260, 8–20. doi: 10.1111/imr.12182
Pérez-Sánchez, J., Estensoro, I., Redondo, M. J., Calduch-Giner, J. A., Kaushik, S., and Sitjà-Bobadilla, A. (2013). Mucins as diagnostic and prognostic biomarkers in a fish-parasite model: transcriptional and functional analysis. PLoS One 8:e65457. doi: 10.1371/journal.pone.0065457
Peters, B. A., Shapiro, J. A., Church, T. R., Miller, G., Trinh-Shrevin, C., Yuen, E., et al. (2018). A taxonomic signature of obesity in a large study of American adults. Sci. Rep. 8:9749. doi: 10.1038/s41598-018-28126-1
Piazzon, C., Galindo-Villegas, J., Pereiro, P., Estensoro, I., Calduch-Giner, J. A., Gómez-Casado, E., et al. (2016). Differential Modulation of IgT and IgM upon parasitic, bacterial, viral and dietary challenges in a perciform fish. Front. Immunol. 7:637. doi: 10.3389/fimmu.2016.00637
Piazzon, M. C., Calduch-Giner, J. A., Fouz, B., Estensoro, I., Simó-Mirabet, P., Puyalto, M., et al. (2017). Under control: how a dietary additive can restore the gut microbiome and proteomic profile, and improve disease resilience in a marine teleostean fish fed vegetable diets. Microbiome 5:164. doi: 10.1186/s40168-017-0390-3
Piazzon, M. C., Naya-Català, F., Perera, E., Palenzuela, O., Sitjà-Bibadilla, A., and Pérez-Sánchez, J. (2020). Genetic selection for growth drives differences in intestinal microbiota composition and parasite disease resistance in gilthead sea bream. Microbiome 8:168. doi: 10.1186/s40168-020-00922-w
Piazzon, M. C., Naya-Català, F., Simó-Mirabet, P., Picard-Sánchez, A., Roig, F. J., Calduch-Giner, J. A., et al. (2019). Sex, Age, and Bacteria: how the intestinal microbiota is modulated in a protandrous hermaphrodite fish. Front. Microbiol. 10:2512. doi: 10.3389/fmicb.2019.02512
Requena, T., Miguel, M., Garcés-Rimón, M., Martínez-Cuesta, M. C., Lopez-Fandiño, R., and Peláez, C. (2017). Pepsin egg white hydrolysate modulates gut microbiota in Zucker obese rats. Food Funct. 8, 437–443. doi: 10.1039/c6fo01571a
Ringø, E., Hoseinifar, S. H., Ghosh, K., Doan, H. V., Beck, B. R., and Song, S. K. (2018). Lactic acid bacteria in finfish—an update. Front. Microbiol. 9:1818. doi: 10.3389/fmicb.2018.01818
Rizzetti, D. A., Martín, A., Corrales-Cordon, P., Fernandez, F., Simões, M. R., Peçanha, F. M., et al. (2017). Egg White Hydrolysate prevents cardiovascular disorders induced by mercury: role of the Angiotensin converting enzyme (ACE) and NADPH oxidase. Toxicol. Lett. 281, 158–174. doi: 10.1016/j.toxlet.2017.10.001
Robles, R., Lozano, A. B., Sevilla, A., Márquez, L., Nuez-Ortín, W., and Moyano, F. (2013). Effect of partially protected butyrate used as feed additive on growth and intestinal metabolism in sea bream (Sparus aurata). Fish Physiol. Biochem. 39, 1567–1580. doi: 10.1007/s10695-013-9809-3
Rognes, T., Flouri, T., Nichols, B., Quince, C., and Mahe, F. (2016). VSEARCH: a versatile open source tool for metagenomics. PeerJ 4:e2584. doi: 10.7717/peerj.2584
Sahoo, T. K., Jena, P. K., Patel, A. K., and Seshadri, S. (2016). Bacteriocins and their applications for the treatment of bacterial diseases in aquaculture: a review. Aquac. Res. 47, 1013–1027. doi: 10.1111/are.12556
Sanz, M. L., Polemis, N., Morales, V., Corzo, N., Drakoularakou, A., Gibson, G. R., et al. (2005). In vitro investigation into the potential prebiotic activity of honey oligosaccharides. J. Agric. Food Chem. 53, 2914–2921. doi: 10.1021/jf0500684
Schmieder, R., and Edwards, R. (2011). Quality control and preprocessing of metagenomic datasets. Bioinformatics 27, 863–864. doi: 10.1093/bioinformatics/btr026
Shahid, M., Hussain, B., Riaz, D., Khurshid, M., Ismail, M., and Tariq, M. (2017). Identification and partial characterization of potential probiotic lactic acid bacteria in freshwater Labeo rohita and Cirrhinus mrigala. Aquac. Res. 48, 1688–1698. doi: 10.1111/are.13006
Simó-Mirabet, P., Felip, A., Estensoro, I., Martos-Sitcha, J. A., de las Heras, V., Calduch-Giner, J., et al. (2018). Impact of low fish meal and fish oil diets on the performance, sex steroid profile and male-female sex reversal of gilthead sea bream (Sparus aurata) over a three-year production cycle. Aquaculture 490, 64–74. doi: 10.1016/j.aquaculture.2018.02.025
Solé-Jiménez, P., Naya-Català, F., Piazzon, M. C., Estensoro, I., Calduch-Giner, J. À, Sitjà-Bobadilla, A., et al. (2021). Reshaping of gut microbiota in gilthead sea bream fed microbial and processed animal proteins as the main dietary protein source. Front. Mar. Sci. 8:705041. doi: 10.3389/fmars.2021.705041
Stanislawski, M. A., Dabelea, D., Lange, L. A., Wagner, B. D., and Lozupone, C. A. (2019). Gut microbiota phenotypes of obesity. NPJ Biofilms Microbiomes 5:18. doi: 10.1038/s41522-019-0091-8
Stojanov, S., Berlec, A., and Štrukelj, B. (2020). The Influence of Probiotics on the Firmicutes/Bacteroidetes Ratio in the treatment of obesity and inflammatory bowel disease. Microorganisms 8:1715. doi: 10.3390/microorganisms8111715
Sugita, H., Ohta, K., Kuruma, A., and Sagesaka, T. (2007). An antibacterial effect of Lactococcus lactis isolated from the intestinal tract of the Amur catfish, Silurus asotus Linnaeus. Aquac. Res. 38, 1002–1004.
Sundell, K. S., and Rønnestad, I. (2011). “Intestinal absorption,” in Encyclopedia of Fish Physiology, ed. P. F. Anthony (San Diego, CA: Academic Press), 1311–1321.
Sze, M. A., and Schloss, P. D. (2016). Looking for a signal in the noise: revisiting obesity and the microbiome. mBio 7:e01018-16. doi: 10.1128/mBio.01018-16
Tam, J., Hoffmann, T., Fischer, S., Bornstein, S., Gräßler, J., and Noack, B. (2018). Obesity alters composition and diversity of the oral microbiota in patients with type 2 diabetes mellitus independently of glycemic control. PLoS one 13:e0204724. doi: 10.1371/journal.pone.0204724
Tarnecki, A. M., Burgos, F. A., Ray, C. L., and Arias, C. R. (2017). Fish intestinal microbiome: diversity and symbiosis unraveled by metagenomics. J. Appl. Microbiol. 123, 2–17. doi: 10.1111/jam.13415
Theilmann, M. C., Goh, Y. J., Nielsen, K. F., Klaenhammer, T. R., Barrangou, R., and Abou Hachem, M. (2017). Lactobacillus acidophilus metabolizes dietary plant glucosides and externalizes their bioactive phytochemicals. mBio 8:e01421-17. doi: 10.1128/mBio.01421-17
Torrecillas, S., Robaina, L., Caballero, M. J., Montero, D., Calandra, G., Mompel, D., et al. (2017). Combined replacement of fishmeal and fish oil in European sea bass (Dicentrarchus labrax): production performance, tissue composition and liver morphology. Aquaculture 474, 101–112. doi: 10.1016/j.aquaculture.2017.03.031
Turnbaugh, P. J., Hamady, M., Yatsunenko, T., Cantarel, B. L., Duncan, A., Ley, R. E., et al. (2008). A core gut microbiome in obese and lean twins. Nature 457, 480–484. doi: 10.1038/nature07540
Weitkunat, K., Schumann, S., Nickel, D., Kappo, K. A., Petzke, K. J., Kipp, A. P., et al. (2016). Importance of propionate for the repression of hepatic lipogenesis and improvement of insulin sensitivity in high-fat diet-induced obesity. Mol. Nutr. Food Res. 60, 2611–2621. doi: 10.1002/mnfr.201600305
Winand, R., Bogaerts, B., Hoffman, S., Lefevre, L., Delvoye, M., Van Braekel, J., et al. (2020). Targeting the 16s rRNA gene for bacterial identification in complex mixed samples: comparative evaluation of second (Illumina) and third (Oxford Nanopore Technologies) generation sequencing technologies. Int. J. Mol. Sci. 21:298. doi: 10.3390/ijms21010298
Wu, S., Bekhit, A. E., Wu, Q., Chen, M., Liao, X., Wang, J., et al. (2021). Bioactive peptides and gut microbiota: candidates for a novel strategy for reduction and control of neurodegenerative diseases. Trends food Sci. Technol. 108, 164–176. doi: 10.1016/j.tifs.2020.12.019
Ytrestøyl, T., Synnøve Aas, T., and Åsgård, T. (2015). Utilisation of feed resources in production of Atlantic salmon (Salmo salar) in Norway. Aquaculture 448, 365–374. doi: 10.1016/j.aquaculture.2015.06.023
Zárate, G. (2012). “Dairy propionibacteria: less conventional probiotics to improve the human and animal health,” in Probiotic in Animals, ed. E. Cid (Rijeka: InTechOpen), 153–202. doi: 10.5772/50320
Zhang, N., Ju, Z., and Zuo, T. (2018). Time for food: the impact of diet on gut microbiota and human health. Nutrition 51–52, 80–85. doi: 10.1016/j.nut.2017.12.005
Keywords: bioactive peptide, egg white hydrolysate, gut microbiota, bile salts, lipid metabolism, Sparus aurata
Citation: Naya-Català F, Wiggers GA, Piazzon MC, López-Martínez MI, Estensoro I, Calduch-Giner JA, Martínez-Cuesta MC, Requena T, Sitjà-Bobadilla A, Miguel M and Pérez-Sánchez J (2021) Modulation of Gilthead Sea Bream Gut Microbiota by a Bioactive Egg White Hydrolysate: Interactions Between Bacteria and Host Lipid Metabolism. Front. Mar. Sci. 8:698484. doi: 10.3389/fmars.2021.698484
Received: 21 April 2021; Accepted: 03 November 2021;
Published: 26 November 2021.
Edited by:
Vikas Kumar, University of Idaho, United StatesCopyright © 2021 Naya-Català, Wiggers, Piazzon, López-Martínez, Estensoro, Calduch-Giner, Martínez-Cuesta, Requena, Sitjà-Bobadilla, Miguel and Pérez-Sánchez. This is an open-access article distributed under the terms of the Creative Commons Attribution License (CC BY). The use, distribution or reproduction in other forums is permitted, provided the original author(s) and the copyright owner(s) are credited and that the original publication in this journal is cited, in accordance with accepted academic practice. No use, distribution or reproduction is permitted which does not comply with these terms.
*Correspondence: Jaume Pérez-Sánchez, amFpbWUucGVyZXouc2FuY2hlekBjc2ljLmVz