- 1Scottish Association for Marine Science, Scottish Marine Institute, Oban, United Kingdom
- 2National Institute of Oceanography and Applied Geophysics – OGS, Trieste, Italy
- 3Naval Architecture, Ocean and Marine Engineering, University of Strathclyde, Glasgow, United Kingdom
- 4Master Program-Ecosystem-Based Management of Marine System, University of St Andrews, St Andrews, United Kingdom
- 5European Marine Board, Oostende, Belgium
- 6Marine Science, University of Highlands and Islands, Inverness, United Kingdom
- 7School of Ocean Sciences, Bangor University, Menai Bridge, United Kingdom
Aquaculture and marine renewable energy are two expanding sectors of the Blue Economy in Europe. Assessing the long-term environmental impacts in terms of eutrophication and noise is a priority for both the EU Water Framework Directive and the Marine Strategy Framework Directive, and cumulative impacts will be important for the Maritime Spatial Planning under the Integrated Maritime Policy. With the constant expansion of aquaculture production, it is expected that farms might be established further offshore in more remote areas, as high-energy conditions offer an opportunity to generate more power locally using Marine Renewable Energy (MRE) devices. A proposed solution is the co-location of MRE devices and aquaculture systems using Multi-Purpose Platforms (MPPs) comprising offshore wind turbines (OWTs) that will provide energy for farm operations as well as potentially shelter the farm. Disentangling the impacts, conflicts and synergies of MPP elements on the surrounding marine ecosystem is challenging. Here we created a high-resolution spatiotemporal Ecospace model of the West of Scotland, in order to assess impacts of a simple MPP configuration on the surrounding ecosystem and how these impacts can cascade through the food web. The model evaluated the following specific ecosystem responses: (i) top-down control pathways due to distribution changes among top-predators (harbor porpoise, gadoids and seabirds) driven by attraction to the farming sites and/or repulsion/killing due to OWT operations; (ii) bottom-up control pathways due to salmon farm activity providing increasing benthic enrichment predicated by a fish farm particle dispersal model, and sediment nutrient fluxes to the water column by early diagenesis of organic matter (recycled production). Weak responses of the food-web were found for top-down changes, whilst the results showed high sensitivity to increasing changes of bottom-up drivers that cascaded through the food-web from primary producers and detritus to pelagic and benthic consumers, respectively. We assessed the sensitivity of the model to each of these impacts and the cumulative effects on the ecosystem, discuss the capabilities and limitations of the Ecospace modeling approach as a potential tool for marine spatial planning and the impact that these results could have for the Blue Economy and the EU’s New Green Deal.
Introduction
The concepts of “Blue Growth” and “Blue Economy” refer to sustainable use of ocean resources enhancing economic growth within a system that preserves marine ecosystem health. The energy trilemma on providing secure, sustainable, and affordable energy is a growing challenge, which drives expansion of renewable energy production from the marine environment (World Energy Council, 2019). To mitigate the impact of climate change, the need of expanding the renewable energy installations has also increased and therefore the installation of renewable energy devices at sea (Dannheim et al., 2020). The sustainable use of marine resources is also facing the challenge of providing food and energy for an increasing global human population, which is expected to exceed 9 billion by the middle of the twenty-first century (United Nations, 2019).
The United Nations’ 2030 agenda (United Nations, 2015) sets out Sustainable Development Goals (SDGs) to shift the world toward a sustainable society, with food production (including aquaculture) a key target area (Food and Agriculture Organization [FAO], 2020). Many SDGs directly relate to fisheries and aquaculture, both of which are of critical importance in providing nutrition and employment to millions around the world (Food and Agriculture Organization [FAO], 2020). In 2016 the global production of proteins from marine sources peaked at 171 million tons with aquaculture accounting for 20% of the average per capita intake of animal protein for 3.2 billion people (Food and Agriculture Organization [FAO], 2020). Aquaculture production has seen an annual global increase of ∼6% between 2001 and 2016 and in EU it is expected to increase to 30% by 2030 (Food and Agriculture Organization [FAO], 2020).
Scotland is aiming to double fish farming production by 2050 (Gatward et al., 2017; Weaver et al., 2020). The sector is a major contributor to the Scottish economy, dominated by production of Atlantic Salmon (Salmo salar), and concentrated in sheltered rural sites in western and northern Scotland such as inshore sea lochs (Thompson et al., 1995; Aquaculture Scotland, 2020). Competition for space in near-shore coastal zones can, however, limit expansion of this industry (Jackson et al., 2011). To help alleviate conflicts over spatial management, offshore aquaculture has emerged as a viable alternative for increasing the global seafood production (Gentry et al., 2017; Lester et al., 2018). However, despite the growing interest, in-depth understanding of the ecological implications of offshore farming is necessary in order to develop a consensus of rules to support the management of farm infrastructure vulnerable to storms and wave action of the open seas (Froehlich et al., 2017). Combining the extraction of more than one marine resource has been suggested as a solution for lowering energy production costs by allowing shared use of space and infrastructure through co-located technologies (Stefanakou et al., 2016; Stuiver et al., 2016). Offshore Renewable Energy (ORE) systems such as offshore wind farms, wave device converters, solar energy storage systems, as well as aquaculture, transport and leisure facilities have been proposed as suitable candidates for co-location of Multi-Purpose Platforms (MPPs) (Da Rocha et al., 2010; Casale et al., 2012; Quevedo et al., 2013; H2Ocean, 2018; Abhinav et al., 2020).
MPPs seek to optimize efficient use of marine space to include multiple users, benefit from shared infrastructure and provide opportunities for localized generation of power for off-grid applications (Stuiver et al., 2016; Holm et al., 2017). However, the overall effects of such an aggregation of activities on the surrounding environment may be difficult to predict. By definition, MPPs would contain various elements, each of which can exert different pressures on the surrounding environment (Figure 1).
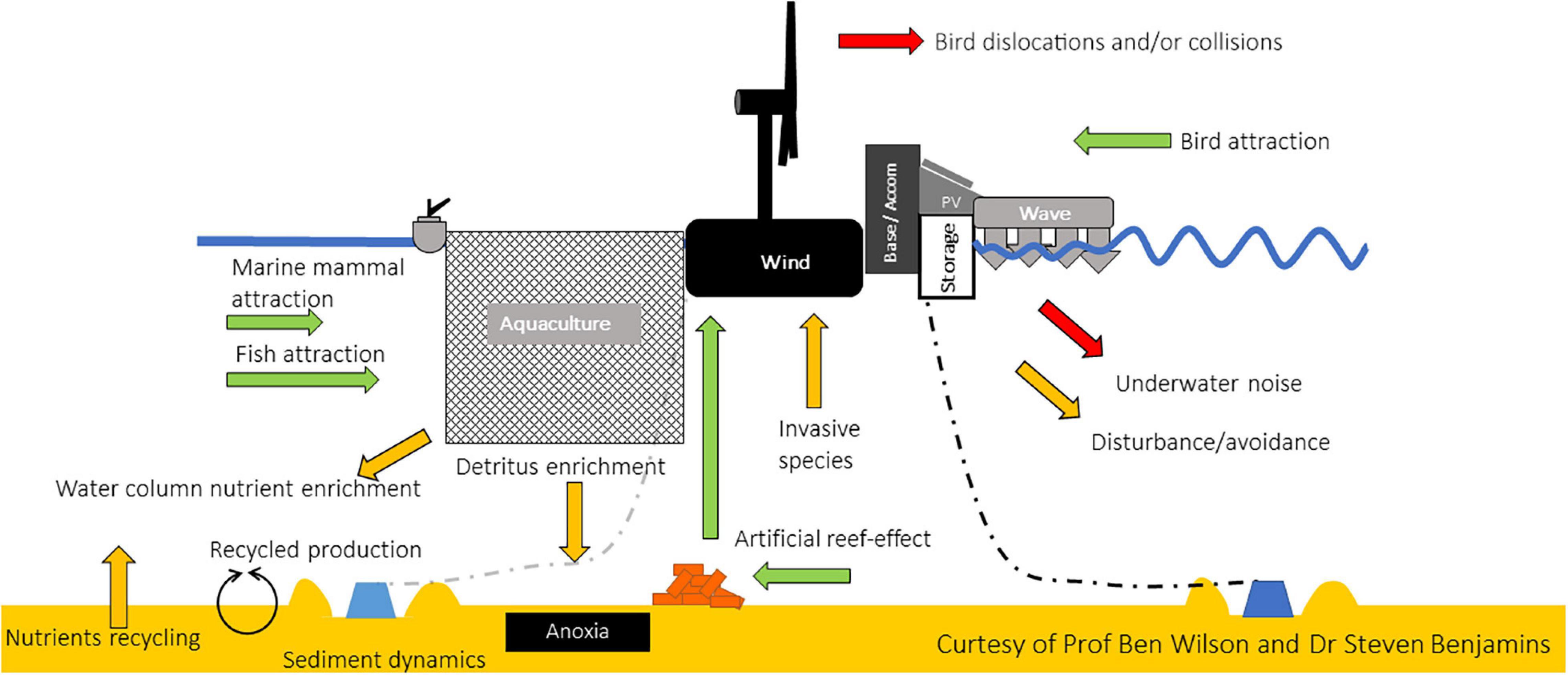
Figure 1. Schematic representation of potential environmental interactions of a hypothetical MPP structure, including aquaculture activity (e.g., fish pens, a barge, and associated mooring structures) co-located with wind turbines, wave device converters, solar PV panels and on board energy storage capacity. Green arrows indicate potential for attraction of species (fish, mammals, birds, epifauna); yellow arrows indicate potential wider ecosystem impacts (substrate availability for invasive species, detritus enrichment, enhanced recycled production, noise); red arrows indicate potential adverse/lethal impacts (dislocations and collisions of birds and bats with wind turbines; underwater noise).
ORE can impact species both indirectly, e.g., by changing habitat properties and directly, e.g., by causing collision risks with moving turbine components (Vanermen et al., 2019; Benjamins et al., 2020) and, for diving species, with static underwater structures (Grecian et al., 2010). ORE site selection is fundamentally important to minimize the direct and indirect impacts on selected species. These impacts could be minimal (Benjamins et al., 2020) or severe when located in key areas, e.g., migration routes, feeding ground (Polaris Wind Power Network, 2018; Vanermen et al., 2019). A main concern, especially when many turbines are placed in close proximity, is the impact that ORE devices can have on acoustically-sensitive species by producing continuous low frequency noise (Madsen et al., 2006; Tougaard et al., 2009, 2020; Bailey et al., 2010; Brandt et al., 2011).
Aquaculture can also have multiple impacts on the surrounding ecosystems (Callier et al., 2018) from increasing productivity around a site due to excess feed spilling into the water column and the accumulation of sedimentary organic matter also enhancing epibenthic communities growth (Tuya et al., 2006; Mente et al., 2010; Bagdonas et al., 2012; Keeley et al., 2014; Skilbrei and Otterå, 2016) as well as the attraction of wild populations of mid- and top-predators (Ross, 1988; Quick et al., 2004; Dempster et al., 2010; Piroddi et al., 2011). These impacts will be affected by moving aquaculture into more exposed, offshore waters even without integration into an MPP; for example, the “footprint” of farm-derived eutrophication of the benthos is expected to be larger, but less intense due to stronger currents and greater water depths in these areas. The co-location of ORE devices with aquaculture site can present synergies and conflicts within the impacts of the single elements. For instance the presence of the fish cages can attract top-predators, which could increase risks of exposure to noise and entanglement (Würsig and Gailey, 2002; Kemper et al., 2003; Benjamins et al., 2014; Dolman and Brakes, 2018).
ORE systems can also have potential benefits: supplying new habitat for colonization of benthic species (Wilson and Elliott, 2009; Krone et al., 2013; Nall et al., 2017; Dannheim et al., 2020), and supplying new nursery areas and feeding grounds (artificial reef effect) (Coolen, 2017; Gill et al., 2018). Similar to existing offshore infrastructure (e.g., oil and gas platforms), an MPP may require establishment of local exclusion zones to other industries (e.g., fishing), thereby inadvertently creating small marine protected areas (Coates et al., 2016; Roach et al., 2018).
Evaluating potential effects of novel or hypothetical infrastructure such as an operational MPP system in a marine ecosystem is challenging and requires an ecosystem-based approach to investigate the cumulative effects of the different MPP elements (Abhinav et al., 2020). Ecosystem models have proven to be a useful tool for modeling natural and anthropogenic variability (Hyder et al., 2015) and, in specific cases have been also used to inform management measures (Fulton et al., 2015; Gascuel et al., 2016). The Ecopath with Ecosim and Ecospace (EwE) modeling approach is considered one of the most suitable tools for evaluating the direct and indirect effects of anthropogenic pressures on ecosystem dynamics (Colleter et al., 2014; Coll et al., 2015; Hyder et al., 2015). EwE models have been successfully used to evaluate how such pressures cascade through the food web. For example, changes to the spatial distribution of top predators (cetaceans, large fish, and seabirds) will affect the entire marine ecosystem through top-down control pathways (Morissette et al., 2010; Piroddi et al., 2011). Similarly, changes in primary productivity can cascade through the food-web by means of bottom-up controls (Coll et al., 2016; Piroddi et al., 2017) as well as environmental drivers (Coll et al., 2016; Serpetti et al., 2017). In recent releases of the EwE software, the options to drive (one-way coupling) Ecospace with external spatial-temporal drivers (Steenbeek et al., 2013, 2016; Christensen et al., 2014) offers the opportunity to apply multiple physical, oceanographic, and environmental drivers that define the cumulative foraging capacity of each functional group and/or species (Christensen et al., 2014). In recent studies this new capability has been explored even further allowing coupling with many other modeling approaches that interact at different levels with the Ecospace framework from hydrodynamic advections models (Tierney et al., 2018), to general additive models predicting top-predators spatial distributions (Harvey, 2018) and habitat preferences (Puts et al., 2020), and to Bayesian hierarchical modeling to explore advantages, limitations and future developments of all coupled modeling techniques (Coll et al., 2019).
In this paper we develop a high-resolution Ecospace model to investigate potential impacts (negative and positive) of a hypothetical Multi-Purpose Platform (MPP) located south west of the Isle of Muck, on the West Coast of Scotland (Figure 2). The hypothetical MPP used consists of an anchored, floating barge with wind turbines (Abhinav et al., 2019; Recalde et al., 2019), co-located with an salmon farm site. The west coast of Scotland is a key region for salmon production, hosting around 200 farming sites located within sheltered fjordic areas (Adams et al., 2020). As the region seeks to achieve sustainable expansion of both aquaculture (Weaver et al., 2020) and of OWT installations, also minimizing the impact on the environment (Tett et al., 2018), this ecosystem represented a perfect opportunity to test the impacts of an MPP site in order to assess conflicts and synergies of its elements.
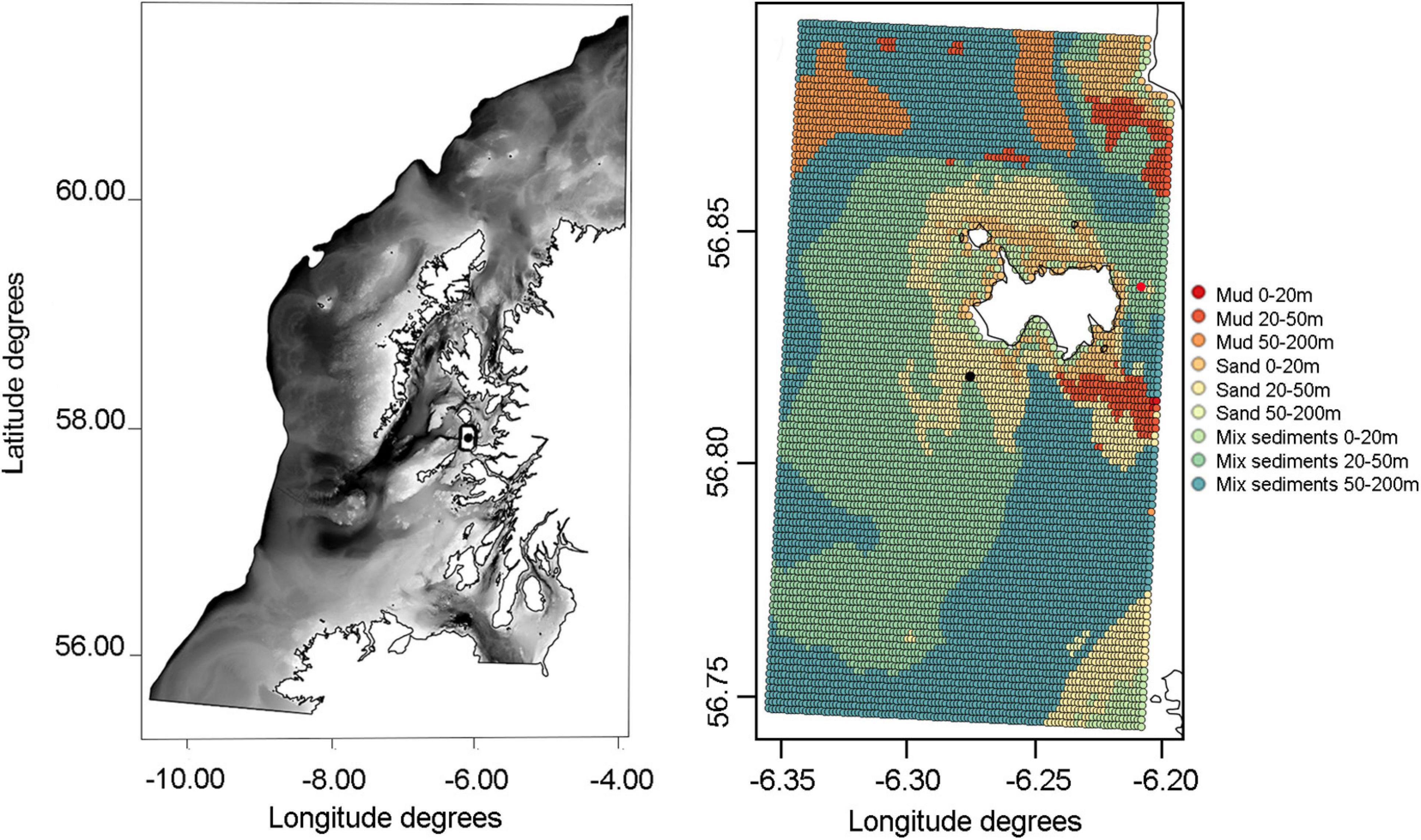
Figure 2. ICES VIa area, showing the continental shelf bathymetry map (up to 200 m depth profile; extracted from https://www.gebco.net/) and the sediment composition around Isle of Muck, the existing operational fish farm (red dot), the Multi-Purpose Platform (MPP) site (black dot) and the Ecospace model domain (black box).
The model’s sensitivity to individual and cumulative impacts of the different pressures produced by the MPP elements on the surrounding ecosystem were investigated. Limitations and capabilities of Ecospace as a potential tool for marine spatial planning were discussed as well as the use of offshore MPP in order to maximize the aquaculture and renewable energy productions within the concepts of “Blue Growth” and “Blue Economy.”
Materials and Methods
The model was built in Ecopath with Ecosim and Ecospace (EwE) version 6.6. This modeling suite has been considered one of the most suitable tools for evaluating the direct and indirect effects of anthropogenic pressures on spatial scale ecosystem dynamics. Ecopath is a mass balance food-web model representing a snapshot of the ecosystem in a given year, and Ecosim that models the temporal dynamics. Ecopath defines the predator/preys species interactions, with species that can also be grouped together in a “functional group” based on trophic and ecological similarities in concurrency with the impact of fishery that is modeled as a top-predator (Christensen and Walters, 2004). Ecosim models use foraging arena theory (Ahrens et al., 2012) where each predator/prey interaction is defined by vulnerability parameters that, assessing the vulnerability of a prey to its predators, affect the strength of the trophic interactions: top-down for vulnerabilities values greater than 2, or bottom-up for vulnerabilities values between 1–2 (for fitted vulnerability please refer to Supplementary Table 4).
The Ecospace domain created in this study is based on the Ecopath, a mass balance model that creates a baseline snapshot of the ecosystem in a given year (1985 in this case), and Ecosim that models the temporal dynamics (1985–2013 in this case). The model includes five fleets (demersal trawl, nephrops trawl, other trawl, potting and diving, and pelagic trawl) and a total of 43 functional groups including marine mammals (5), seabirds (1), fish (23, six of which were composed of adult and juvenile stages for cod, haddock and whiting), invertebrates (5), cephalopods (1), zooplankton (2), benthos (3), primary producers (2), and detritus (1) (Alexander et al., 2015; Serpetti et al., 2017; Harvey, 2018). The Ecopath with Ecosim model was previously fitted to time-series observations (29-year period), cross-validated and used to assess the uncertainty in input data (Serpetti et al., 2017).
Two Ecospace models have previously been developed for this region (Alexander et al., 2016; Harvey, 2018), both covering the same large-scale domains (∼110,000 km2) of the continental shelf (out to ∼200 m depth) encompassing Division Vla of the International Council for Exploration of the Sea (ICES; Figure 2).
In Harvey (2018) the following three cetacean groups were defined: “Harbor porpoises,” “Dolphins” and “Minke whales,” with the distinction based on the ecology and hearing sensitivity ranges of high-, mid- and low-frequency noise for each cetacean group (National Marine Fisheries Service, 2018). For details of Ecopath and Ecosim model input and temporal fitting please refer to Supplementary Material.
Ecospace Model Update
Using the most updated Ecopath with Ecosim model for this ecosystem (Harvey, 2018; Supplementary Material), a high resolution Ecospace model was created for the area south west of the Isle of Muck (Figure 2). The resolution of the model (approximately 100 m × 100 m resolution) was chosen in order to better assess the impact of habitat eutrophication by aquaculture that generally, at these depths, showed a foot-print of a few kilometers (Adams et al., 2020; Supplementary Material NewDEPOMOD outputs.mp4).
The isle of Muck, the smallest of Scotland’s Small Isles, was chosen as a potential study site for this project due to its exposed location and the presence of a nearby operational salmon farm, upon which the present hypothetical case could be modeled as it currently hosts the most offshore salmon farm in Scotland (Figure 2). The current farming site is approximately 500 m from shore off the north-eastern corner of Muck, however we located the hypothetical MPP site off the Southwest at the same distance to simulate more exposed conditions, while still potentially being able to use data output to represent the current farming site. The Ecospace habitat map was created in QGIS (version 3.10.10) by combining extracted georeferenced sediment substrates (from the Mapping European Seabed Habitats project1) and depth (from Seazone/Edina2) properties at each grid node (Figure 2), following the habitat categories (Table 2) adjusted from Alexander et al. (2016).
Please refer to Supplementary Materials for all functional group habitat based foraging, dispersal rates and fishery habitat allocation (Supplementary Table 5).
The baseline Ecopath, Ecosim and Ecospace model was first run without the addition of the MPP site where species distribution changes (1985–2013) were driven by temporal fishing mortalities (from the fitted model in Ecosim) according to different preferences of the functional groups’ habitat foraging usage (Table 2) and their dispersal rates (Supplementary Table 5). The MPP was then virtually located as a new habitat in 2011 and kept operational for 3 years to the end of the temporal model extension (2013) (Supplementary Figure 2). MPP impacts were assessed as an average over the 3 years.
Within the multiple impacts that could be assessed using Ecospace, we focused on top-down ecosystem responses [attraction of top predators (predatory fish, marine mammals, and seabirds) to the MPP site for food vs. incidental mortality of seabirds due to collision with operational OWTs and displacement of marine mammals due to turbine noise] and bottom-up responses (eutrophication by farming activity).
Predator Attractions by Farming Activity (Gadoids, Seabirds, and Seals)
In the West Coast of Scotland, gadoids species such as saithe (Pollachius virens), Atlantic cod (Gadus morhua), whiting (Merlangius merlangus), and haddock (Melanogrammus aeglefinus) have been found to be the most prevalent around the fish farms (Dempster et al., 2009, 2010; Ghanawi and McAdam, 2020). Their attraction is not only directly attributed to excess food, but it also acts as an indirect beckoning via the increase of prey. While smaller cod and whiting consumed excess food pellets (Ghanawi and McAdam, 2020), larger cod, presumably adults, have been found to predate on saithe in the immediate vicinity of salmon farms (Bagdonas et al., 2012).
Multiple reasons arise for the attraction of seabirds and other top predators to the MPP site, from the attraction to the fish farms, either by the fish in the cages and the enhanced productivity around them (Forrest et al., 2007; Vanermen et al., 2015; Dierschke et al., 2016; Callier et al., 2018), and from the attraction to the physical structures that can provide resting and roosting spots (Garthe and Hüppop, 2004; Drewitt and Langston, 2006; Forrest et al., 2007; Dierschke et al., 2016; Callier et al., 2018).
Both gray and harbor seals rely particularly on sandeel and gadid species (Supplementary Table 2), however, as opportunistic feeders, seals prey on available demersal and pelagic fish, including salmonids, as well as cephalopods (Scottish-Government, 2016; Wilson and Hammond, 2019). The high density of fish, both salmonids in the cages and expected concentrations of wild fish, is suspected to attract seals to the vicinity of fish farms. On the West Coast of Scotland, both gray seals (Halichoerus grypus) and harbor seals (Phoca vitulina) are present with overlapping distributions, however, harbor seals showed a more coastal behavior, typically remaining within 50 km from shore (Northridge et al., 2013; Jones et al., 2015).
Seabirds Offshore Wind-Turbine Induced Dislocation
OWTs would potentially cause seabirds attraction to the physical structures for some species and significant avoidance from others (Vanermen et al., 2015, 2019). Mitigating combined impacts might be found associating OWTs and fish farms. Vanermen et al. (2019) summarized the results of a monitoring program designed to identify specie-specific seabirds responses to wind farms: they identified significant avoidance by northern gannet, common guillemot and razorbill of 98, ∼60, and ∼75%, respectively, in contrast, attraction to the wind farm could be demonstrated for herring and great black-backed gulls. In our case of study, the seabirds functional groups is represented by 12 species of seabirds (Waggitt et al., 2020), with Atlantic puffin, common guillemot, northern fulmar and northern gannet showing the highest contributions within the functional group (Table 1). Of the 12 seabird species in the modeled area, the three species showing significant avoidance of the wind farm represented 45% of the biomass group in proportion to their avoidance responses (Table 1; Vanermen et al., 2019). Species showing the attraction of the OWTs represented only a small proportion of the functional group biomass therefore this pressure was not assessed in this study.
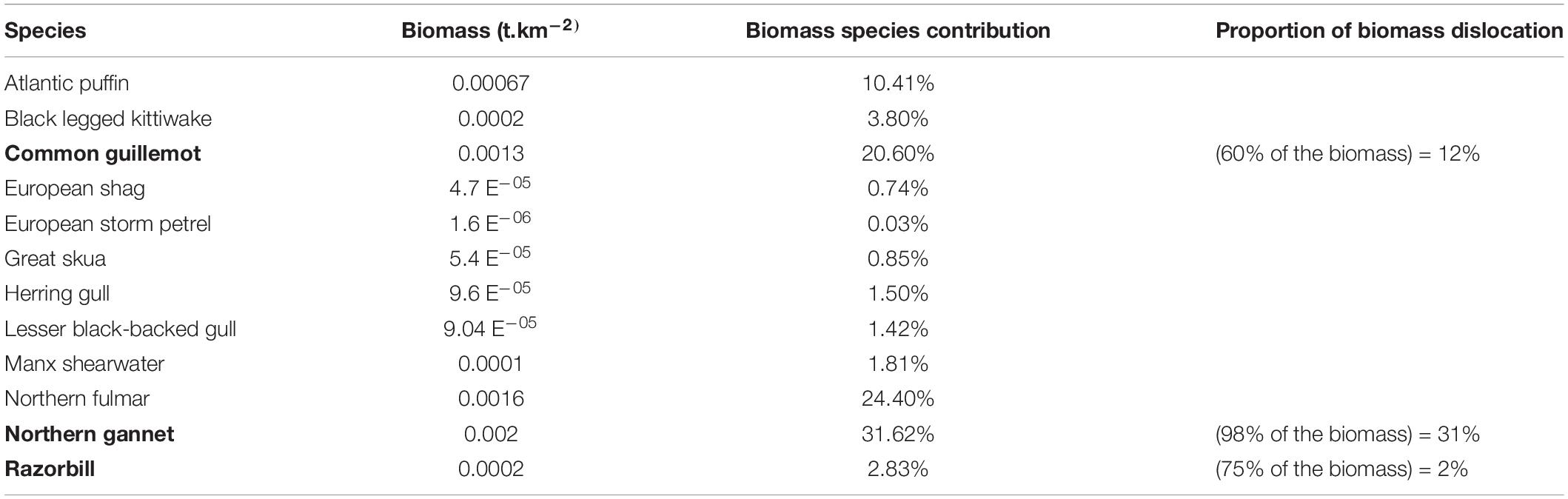
Table 1. Seabirds functional group composition, biomasses, species contributions, and proportion of biomass dislocation for the three species (in bold) showing significant avoidance of wind farms.
Offshore Wind-Turbine Underwater Noise
Operational wind turbines mounted atop a floating barge, such as the MPP proposed here, can be expected to transmit noise into the marine environment. Characteristics and environmental significance (if any) of this underwater noise output depend crucially on technical design features of both turbine(s) and barge, as well as ambient noise levels in the surrounding area. While some information is available on underwater sounds produced by fixed offshore wind turbines (Madsen et al., 2006; Tougaard et al., 2020), similar data on existing floating offshore wind turbines are, as yet, almost non-existent. Turbines proposed here (Abhinav et al., 2019; Recalde et al., 2019) are also much smaller than those used in offshore wind farms, whether fixed or floating, which will affect their sound outputs in terms of both loudness and frequency range. Based on results reported by Madsen et al. (2006) and Tougaard et al. (2020), it can be assumed that sounds produced by floating turbine-bearing structures such as the MPP proposed here will likely be mainly lower-frequency sounds with dominant frequencies of ∼1 kHz or less.
An artificial structure like a barge with wind turbines can transmit low frequency underwater noise that may affect species sensitive to this pressure (Madsen et al., 2006; Tougaard et al., 2020). Marine species differ in terms of their sensitivity to underwater noise of different frequencies (Southall et al., 2007, 2019).
Given the expected (hypothetical) underwater noise output of an MPP of the type proposed here, species with greater sensitivity to lower frequencies (e.g., phocid seals and baleen whales, after Southall et al. (2019) might be able to detect the noise from an MPP at distances of several km under quiet ambient noise conditions, with detection ranges for species less sensitive to lower frequencies (e.g., harbor porpoise) being considerably smaller (Madsen et al., 2006). However, this is crucially dependent on the size and type of the turbine (e.g., direct drive or with gear box), potential cumulative effects of multiple turbines, ambient noise from other sources (e.g., shipping), wind speeds and which sound propagation model is used (Tougaard et al., 2020; Stöber and Thomsen, 2021).
Toothed whales such as harbor porpoises utilize echolocation for spatial orientation, foraging and communication (Soto et al., 2006; Gomez et al., 2016; Forney et al., 2017), and have been observed to alter their feeding behaviors when co-occurring with shipping (Dyndo et al., 2015; Wisniewska et al., 2018). However, there is a lack of data with respect to the impacts that continuous noise produced from a static source such as a OWT might have on cetaceans (Tougaard et al., 2020; Stöber and Thomsen, 2021). Porpoises have been observed to reoccupy operational (fixed) offshore wind farms once construction has ceased, suggesting that these turbine noise outputs do not deter porpoises under all circumstances (Scheidat et al., 2011; Teilmann and Carstensen, 2012).
Harbor porpoises have been found to stop feeding for 15 min when closer than 1 km from a source of low frequency noise such as a shipping vessel (Dyndo et al., 2015; Wisniewska et al., 2018). As the low-frequency noise source in our study is continuous and static we assumed the porpoises might cease their feeding behavior at distances from 1 km (Dyndo et al., 2015) up to 7 km at which they no longer are seen to be affected (Wisniewska et al., 2018). It is important to underline that these assumptions are not taking into consideration the species’ capability of acclimation and habituation to background noise.
Like cetaceans, pinnipeds are noise-sensitive species. They are known to behaviorally react to pile driving and shipping noise with avoidance, alteration of diving behaviors (Mikkelsen et al., 2019), interruption of feeding and abandonment of vital habitat (Gomez et al., 2016). However, harbor seals have been shown to not avoid at least some operational offshore wind farm areas (Russell et al., 2016). Harbor seals showed lower sensitivity to low frequency noise compared to porpoises with recorded avoidance up to 500 m from tidal turbine noise (Hastie et al., 2017) and significant decreases up to 2 km (Joe Onoufriou, St Andrews University, EIMR2020 conference, April 21st–23rd, 2020).
Because of lack of information and specific studies assessing the acoustic characteristics of the noise produced by the wind turbines associated with a barge within the MPP, we inferred the responses to this pressure using knowledge gathered assessing the impact of other low-frequency noise sources such as shipping noise for harbor porpoises and preliminary results of tidal turbine noise on harbor seals. Sigmoid functions were used to build the response functions within the avoidance distances and distances from which species no longer are seen to be affected. To simulate the effect of low frequency underwater noise produced by the OWTs, a distance layer from the MPP site was created and response functions related to this distance were applied for harbor porpoise and seals to simulate their responses (Figure 3).
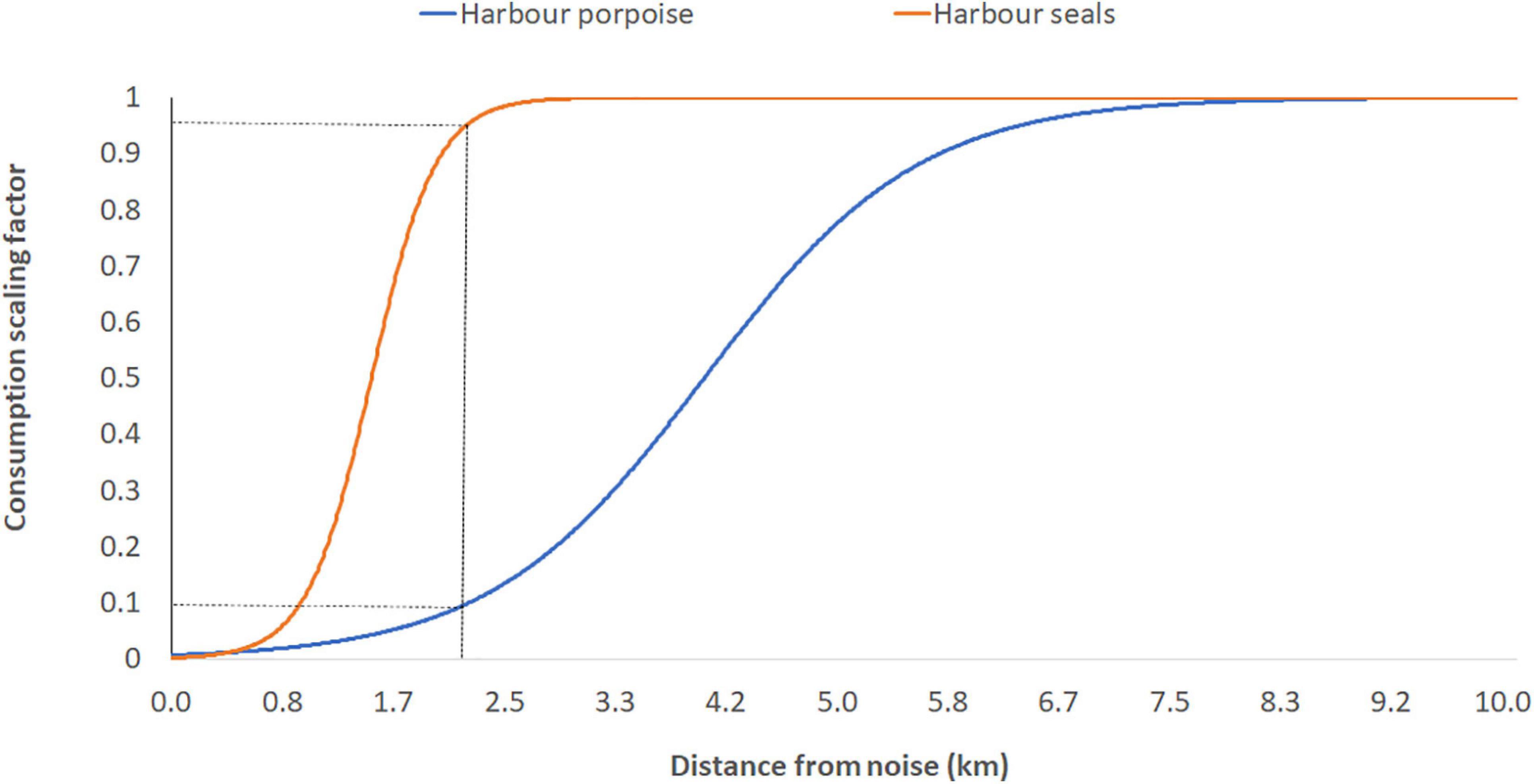
Figure 3. Hypothetical Species distance response functions for harbor porpoise and harbor seals developed for the purposes of this study. The intercept between spatial distance and the species response functions determined the consumption rate scaling factor.
As for the other MPP impact the distance layer was linked to the model with the spatial-temporal framework in 2011, when the hypothetical MPP site was set up. The response functions were applied to the distance layer in order to scale the consumption rates to simulate the cessation of feeding and spatial avoidance of the MPP noise.
Detritus Enrichment: NewDepomod Modeling Approach
In order to assess the organic enrichment caused by the farming cages co-located within MPP site, a particle-tracking modeling software called NewDEPOMOD was used. NewDEPOMOD predicts the depositional footprint of wasted feed and feces on the seabed below and surrounding the fish farm (Adams et al., 2020; Chary et al., 2021). This modeling approach is used widely in Scotland for the licensing of new farms, and expansion of existing farms, and is currently the only model accepted by the Scottish Environment Protection Agency (Scottish Environment Protection Agency, 2020) for aquaculture waste management. The program is coupled with the WeStCOMS hydrodynamic model (Aleynik et al., 2016) to simulated in situ depositional discharges.
A NewDEPOMOD model run was carried out at the MPP site for a configuration of 8 cages (2 × 4 configuration) with a total production of 2,400 tons of salmon per year. A snapshot of the deposition was taken each day, starting from day 10 (to enable some deposition to accumulate), until the end of the run (365 days). The final deposition was calculated as weighted averages interpolated over 100 m cell grid of carbon deposition from the last 90 days of the model run. The deposition covered a total of 91,250 m2, with a highest deposition concentration of 422 gC m–2 yr–1. The annual output deposition was interpolated monthly within the georeferenced model domain (Figure 4; Supplementary Material NewDEPOMOD outputs.mp4) and linked to the Ecospace grid in order to force the relative biomass of detritus in the footprint domain using the temporal-spatial framework plug-in (Steenbeek et al., 2016).
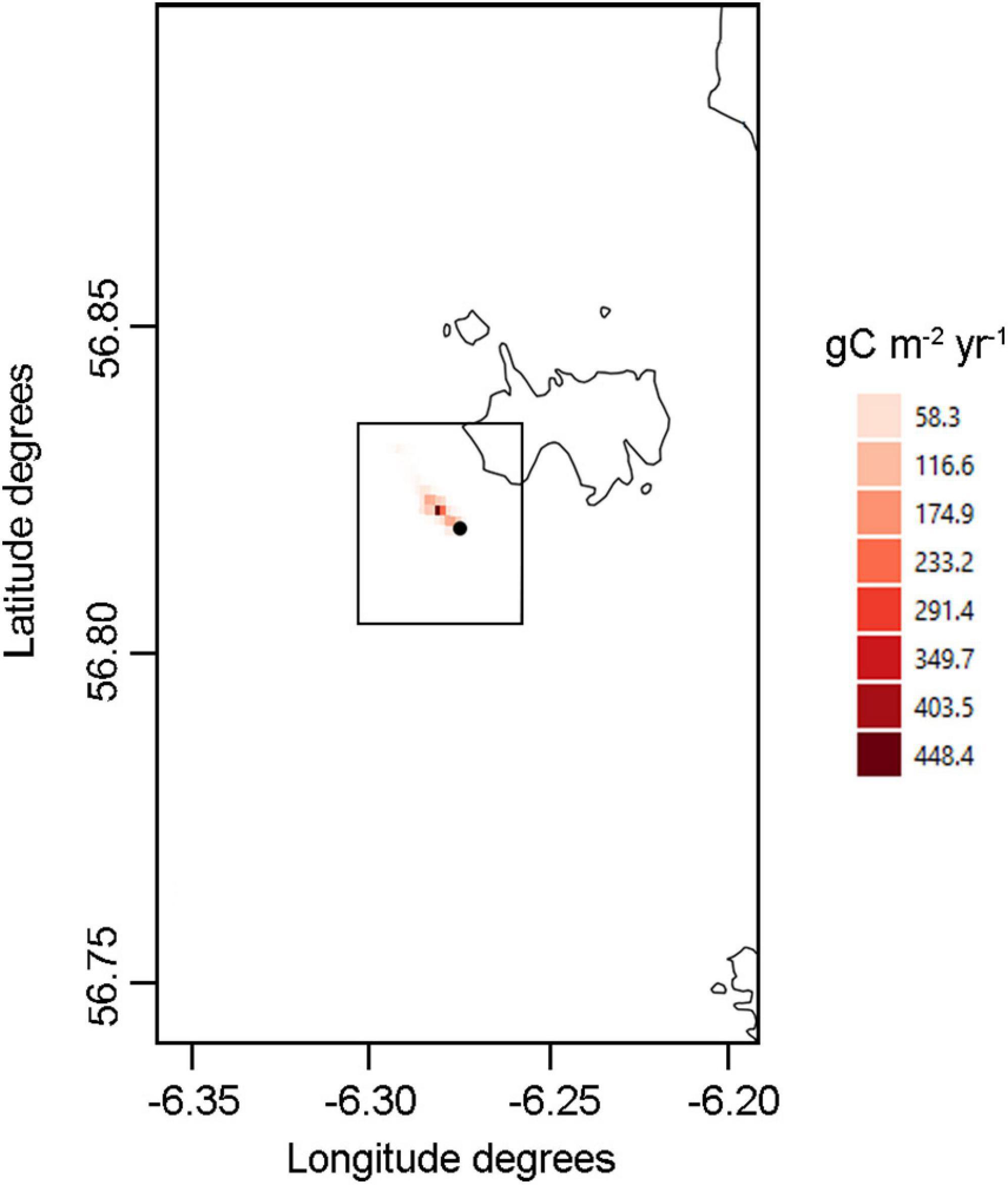
Figure 4. NewDEPOMOD annual carbon deposition output interpolated by the georeferenced Ecospace model domain (100 m × 100 m).
Nutrient Enrichment by Enhanced Secondary Production
The excess organic matter that reach the seabed is mineralized in the sediment, creating a localized increase of recycled nutrients. In shallow shelf seas, nutrients regenerated in the sediment co-regulate primary production events in the water column (Ruardij and Van Raaphorst, 1995; Soetaert and Middelburg, 2009).
Across sediment types, in shallow waters, recycled production can contribute up to 25% of the winter dissolved inorganic nitrogen maximum (Serpetti, 2012). Therefore, assuming that nitrate is often the limiting nutrient of primary productivity (Ryther and Dunstan, 1971; Vitousek and Howarth, 1991) and completely depleted during spring bloom (Serpetti, 2012), we might expect a proportional local increasing of annual primary production driven by nitrogen regenerated from the organic matter enrichment under the fish farm. Therefore we applied a relative annual increase of 25% of phytoplankton biomass proportionally to the intensity of the farming foot-print spatial extent at monthly steps. This percentage is just a crude estimation as we are considering only recycled production by sediment organic matter remineralization processes without considering the remineralization in the pelagic domain neither the local loss of nutrients due to advection and currents. Quantifying the nutrient loadings by fish farms is still controversial, similar nitrogen eutrophication rates were estimated by other case of studies in the Baltic Sea coastal areas (Bonsdorff et al., 1997; Nordvarg, 2001), however, Honkanen and Helminen (2000) also highlighted that local changes of chlorophyll-a is a better indicator for assessing the enhanced productivity caused by fish-farms.
Setting Scenarios and Model Outputs
A region of about 8 km2 was set around the MPP site (box in Figures 2, 4) and model outputs were extracted within the region in order to compare the biomass changes across the scenarios tested. In Ecospace, a fishery exclusion zone was set in this region, therefore the fisheries included this model were not operational close to the MPP elements. A total 6 scenarios were run in Ecospace for the full 29-year period of the model, testing the impact of MPP pressures after the installation of the MPP in 2011:
1. Baseline: no addition of the MPP;
2. Addition of the OWTs, testing the impacts of low frequency noise and induced displacement by OWTs for selected sensitive seabirds species;
3. Addition of the salmon farming habitat, testing the attraction of selected top-predators;
4. Addition of the salmon farming habitat, testing the attraction of selected top-predators and detritus enrichment;
5. Addition of the salmon farming habitat, testing the attraction of selected top-predators, detritus enrichment and phytoplankton increased induced by enhanced recycled production; and
6. Testing cumulative impact of all pressures at the same time.
The MPP multiple impacts were implemented in Ecospace in different ways and activated at a specific time and space using the temporal-spatial framework plug-in (Steenbeek et al., 2016). In Ecospace the salmon farm was added in 2011 as a new habitat (Supplementary Figure 2). Species attraction to the farm was simulated by changing the relative habitat foraging usage for selected species (saithe, Atlantic cod, whiting, haddock, seabirds, and harbor seals) with the highest proportion of preferences at the “farming” habitat (Table 2).
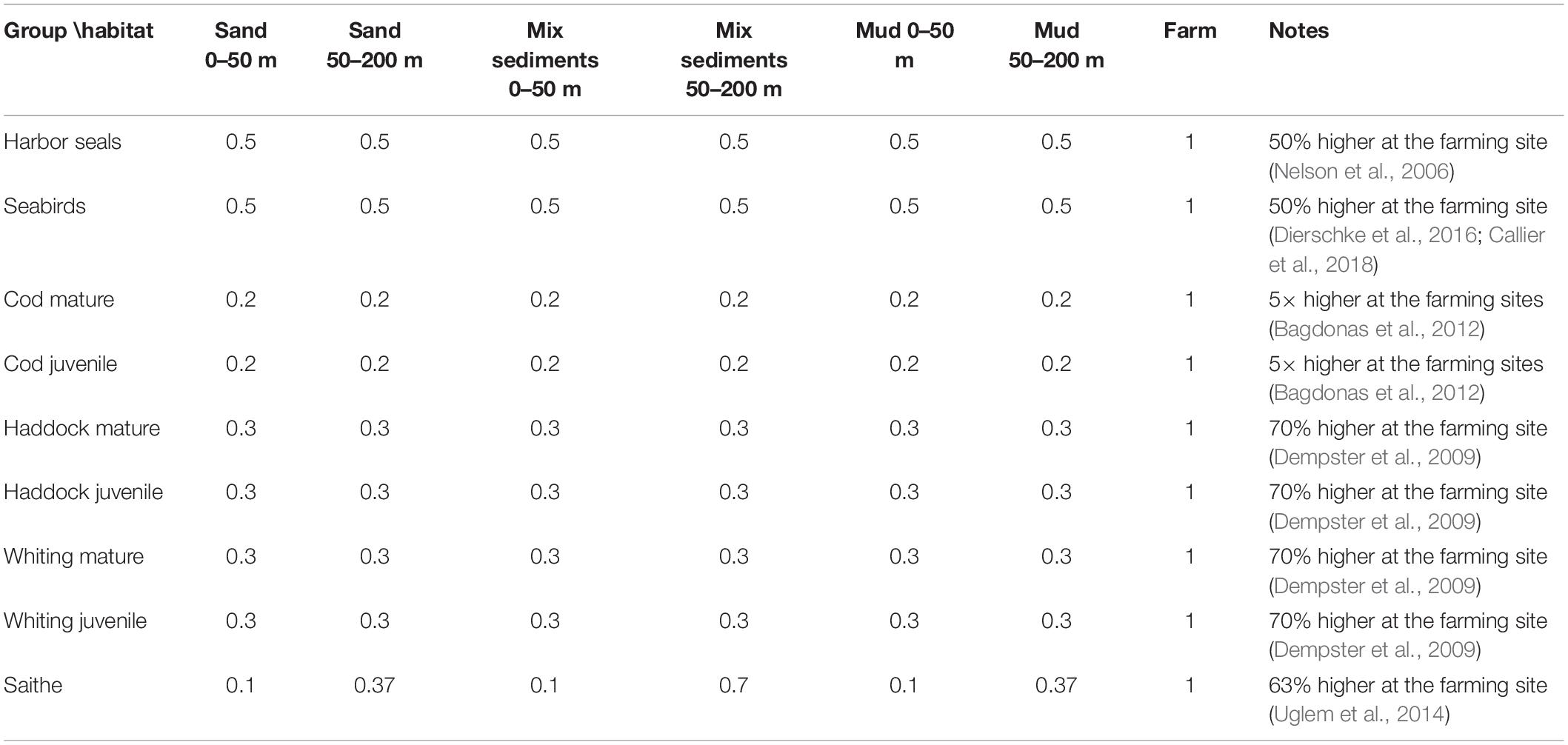
Table 2. Relative changes made for habitat preferences to simulate attraction to the farming site with references used.
Seabird displacement was simulated (2011–2013) forcing the relative temporal-spatial biomass at the “farming habitat” ± 0.5 km.
Salmon farm-induced eutrophication was simulated by proportionally forcing the relative temporal-spatial biomass of detritus in correspondence to the farming foot-print, and the enhanced recycled production directly impacted the relative biomass of phytoplankton for the same spatial foot-print.
Results
Species biomasses were extracted within the region for each scenario and biomass changes for scenarios 2–6 were visualized by standardizing the species biomasses by the baseline in scenario 1 “no addition of the MPP” (Table 3 and Figure 5).
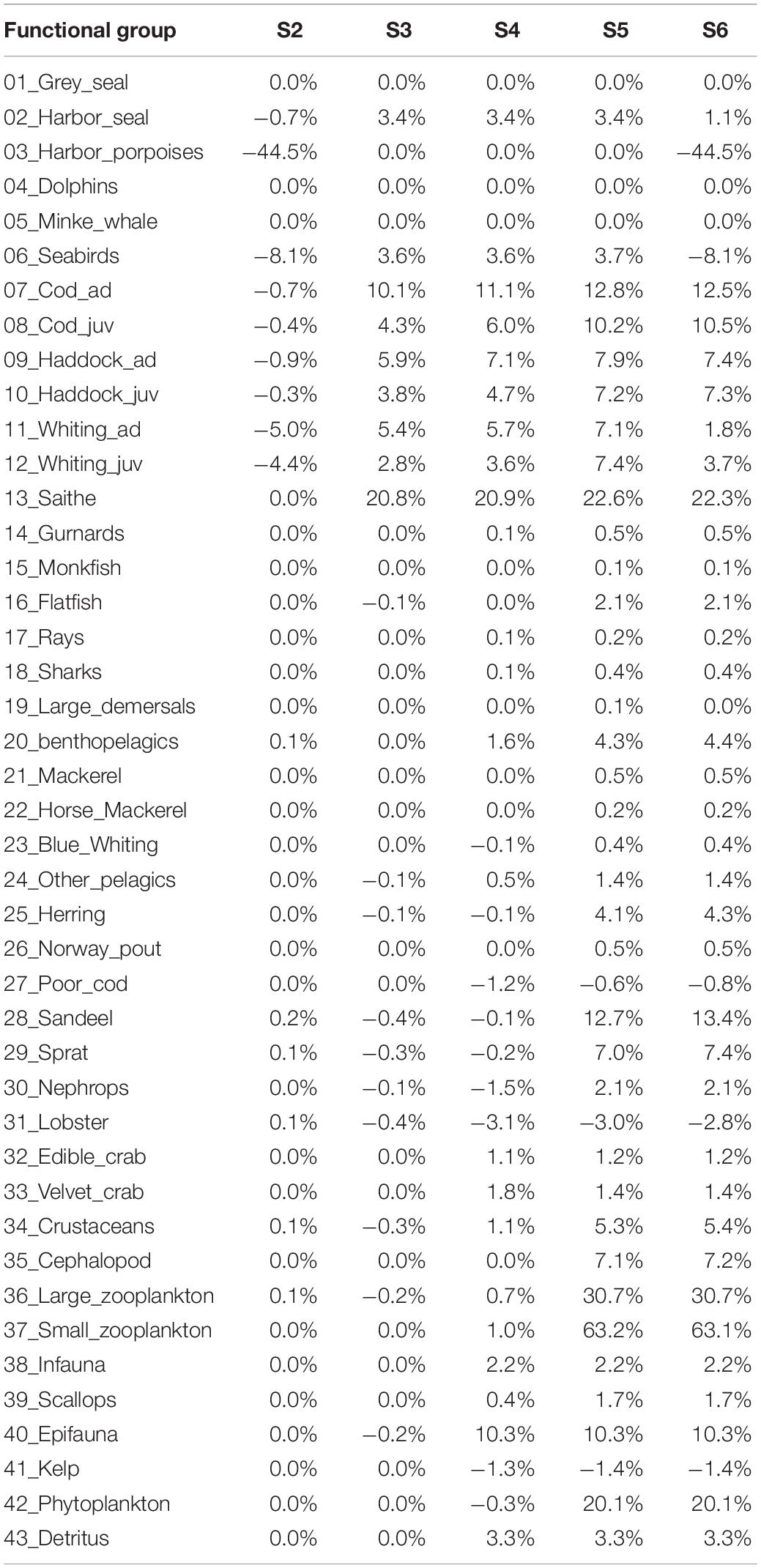
Table 3. Mean relative annual biomasses changes (2011–2013) of functional group across scenario 1–6.
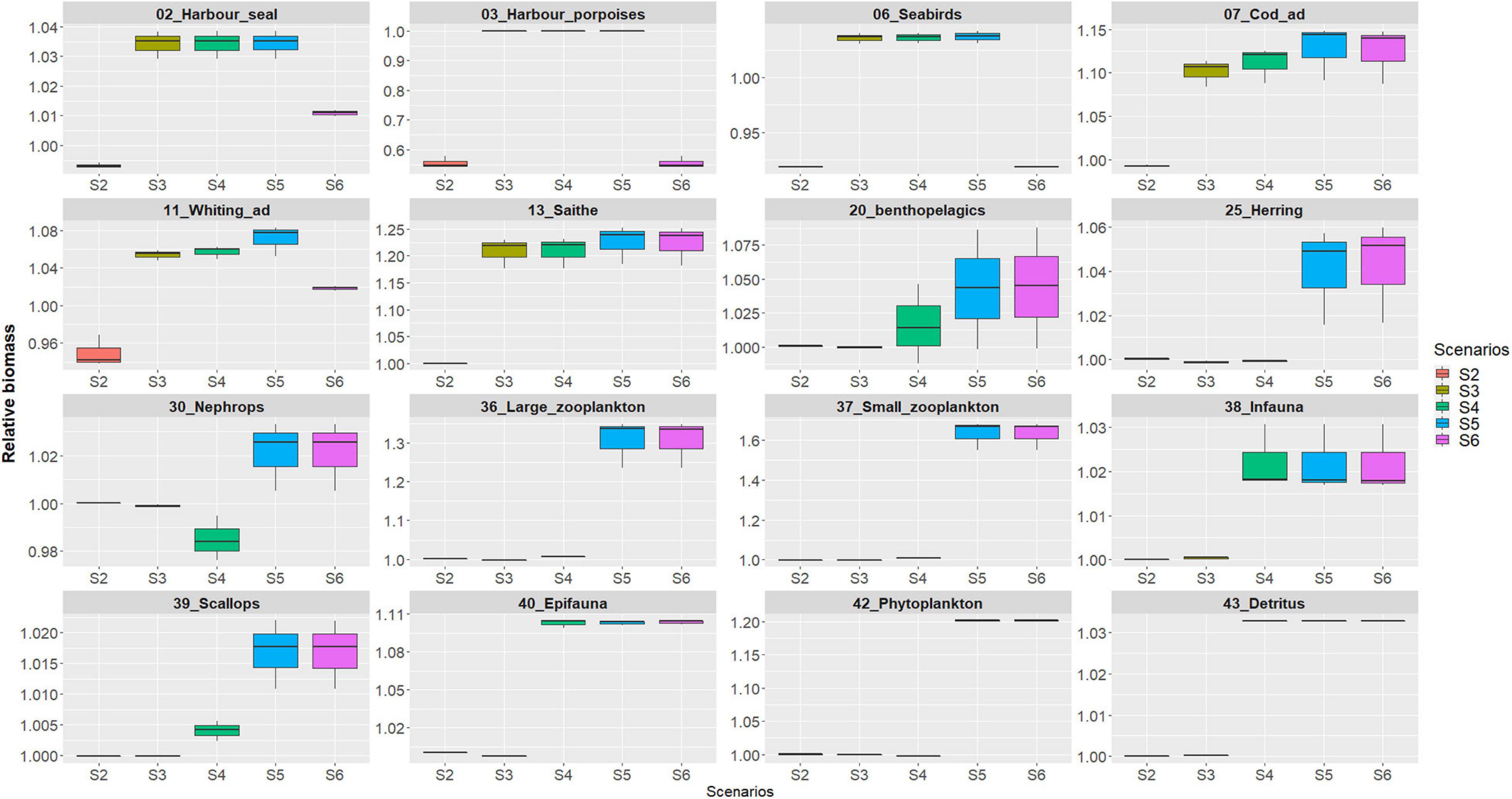
Figure 5. Species relative annual biomasses changes (2011–2013) assessing the impact of MPP pressures within the region for each scenario. Biomass changes for scenarios 2–6 were visualized standardizing the species biomasses by the baseline “no addition of the MPP.”
The results showed between ± 5–25% relative changes for most of the functional groups due to the MPP pressures (Table 3 and Supplementary Figures 3, 4). Higher responses were found for harbor porpoises (down to ∼45%) when testing the assumed impact of OWTs noise, for sandeels (up to ∼20%) and large and small zooplankton (up to ∼30 and 60%, respectively), when testing the impact of detritus enrichment (scenario 5) and primary production (scenario 6).
In Scenario 2 harbor seals and porpoises are the functional groups assumed to be impacted by the low frequency noise produced by OWTs: porpoises showed highest sensitivity (defined by the avoidance of noise up to 7 km from the source; Figure 3), as defined by the response function to this pressure, causing a decrease of their relative biomass within the region of ∼45% (Figure 5), whilst harbor seals showed ∼1% decrease within the region (Figure 5) although localized within 2 km from the MPP source location (Supplementary Figure 3). The decrease of harbor porpoises within the region showed a weak evidence of top-down control through the food-web without significant increases of its major prey species (diet proportion ∼50% juvenile whiting and ∼25% sandeels) (Supplementary Figure 2).
Whiting, and cod and haddock to a lesser extents, also declined under the scenario testing exclusively the impact of noise (scenario 2; Table 3 and Figure 5). Porpoises and gadoids are competing for whiting (adult and juveniles) as a main prey (Supplementary Table 2), and saithe also exhibited a top-down control on whiting (Supplementary Table 4), therefore the decline on whiting is determined by cumulative impact of all his predators changes within the region.
Induced seabirds dislocation by OWTs determined a decrease of seabirds biomass of 8% within the region (Figure 5, scenarios 2 and 6) and the change was significant within a radius of about 0.5 km from the MPP site (Supplementary Figure 5).
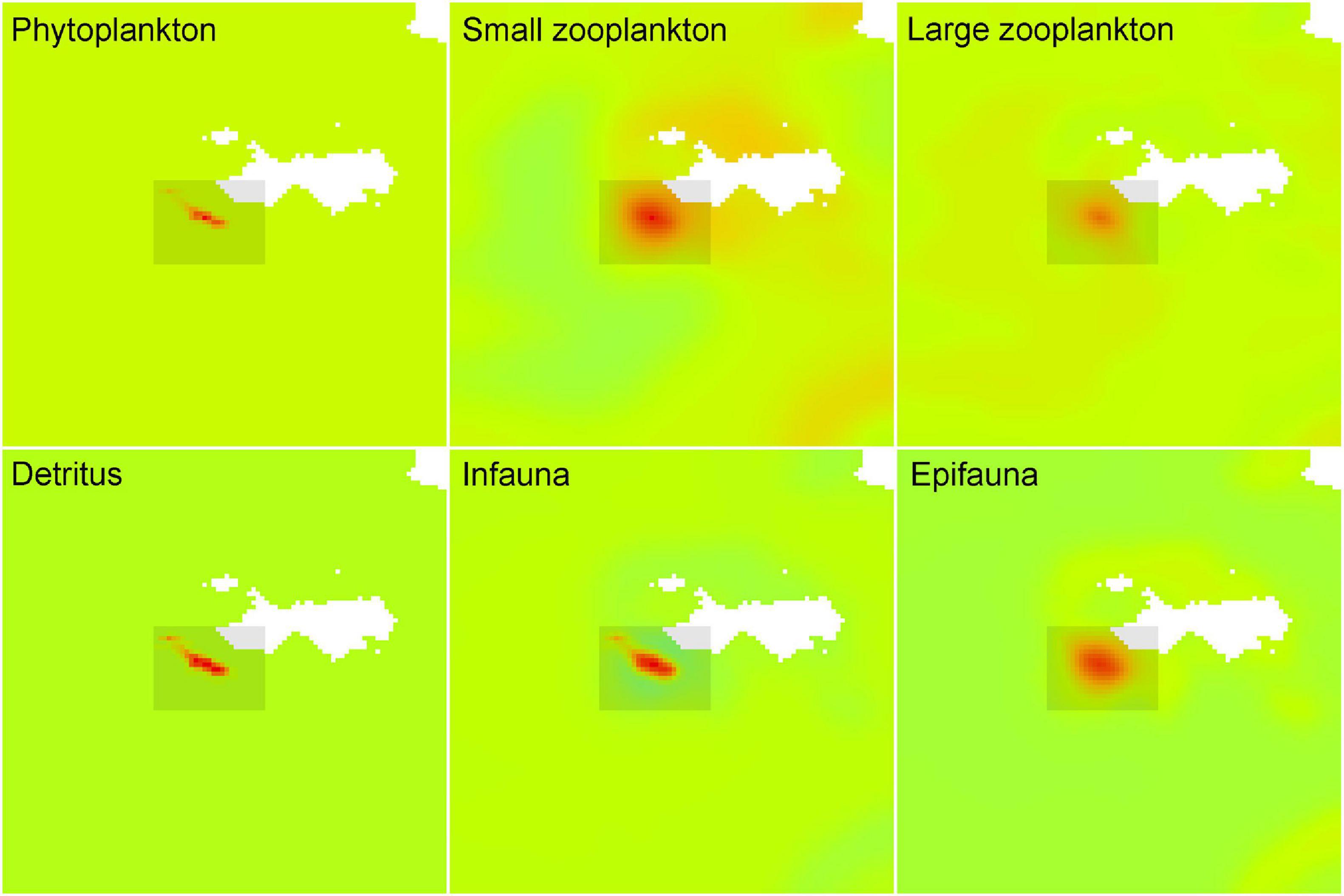
Figure 6. Species relative biomasses extracted at the end of the scenario 6 simulation (2013) showing the relative foot-print increases of phytoplankton, small zooplankton, large zooplankton, and detritus, infauna and epifauna.
In scenarios 3–5 an overall increase of relative biomasses were recorded as expected with the enhancement of region productivity driven by either fish farming attraction of wild stock to salmon cages, detritus enrichment, and enhanced primary production (Supplementary Figures 3, 4). Only a few groups showed decreasing patterns under these scenarios, including poor cod (Trisopterus minutus) with <1% decreases due to predations of many predators (Supplementary Table 2), Nephrops with <2% decreases only in scenario 4 caused by rays’ predation pressure) and lobsters with <4% decreases due to cannibalism). In scenarios 3–5, all the gadoids are attracted to the farming site with saithe being the most responsive to this attraction due to their large increase in biomass up to 2013 (Serpetti et al., 2017) and their top-down controls on other gadoid preys (Supplementary Table 4).
In scenario 4, detritus enrichment rapidly cascaded through the food-web, with an increase in infauna, with a diet consisting of 95% detritus increase, and epifauna, that feed on both detritus and infauna (approximatively 40% each in this model) (Supplementary Table 2). The detritus enrichment caused small increases of biomass for most of the benthic species such as gurnards, rays, benthic sharks, and crabs (Figure 5, Table 3, and Supplementary Figure 4).
Similarly, changes of phytoplankton (scenarios 5 and 6) directly impacted small and large zooplankton with propagation of the increasing production to the pelagic species such as benthopelagic fish, herring and sandeels (Figure 5, Table 3, and Supplementary Figure 4).
In scenario 6 the cumulative impacts overruled the negative effects caused by the noise pressure (Table 3, negative values in scenario 2), and by predator attraction (Table 3, negative values in scenario 3) for most of the species impacted. Only harbor porpoises and seabirds did not show cumulative mitigating impacts (Table 3 and Figure 5).
Under scenario 3, attraction of top-predators, many prey species showed small decreases (Table 3) due to increased predation mortality manly driven by gadoids. This decreases were overruled by increasing benthic productivity (scenario 4) and primary production (scenario 5 and 6) for benthic and pelagic domains, respectively.
Under scenario 4, Nephrops showed a decrease of 2% driven by increasing predation mortality by crustaceans, flatfish and cod, however, the enounced primary production (scenario 5 and 6) overcome this decrease supplying more food for this species (Table 3).
Overall, the results did not showed a cumulative negative impact on any functional group.
Spatially, the biomasses of phytoplankton and detritus are forced on the grid cells proportionally to the carbon deposition output from NEWDepomod (Figure 4): these signals cascaded through the food-web (to small and large zooplankton and to infauna and epifauna from phytoplankton and detritus, respectively) showing larger diffused footprints according to their dispersal rates (∼30 km/year for small-, large zooplankton and epifauna and 3 km/year for infauna) (Figure 6 and Supplementary Table 5).
Discussion
This study focused on a preliminary investigation on the small scale impacts which would occur from a hypothetical Multi-Purpose Platform (MPP) off the West Coast of Scotland. The study quantitatively applies pressures produced by the MPP (aquaculture co-located with offshore wind turbines (OWTs) in order to assess their potential impact on the food-web. The pressures were applied one at time to disentangle the conflicts and synergies of the MPP elements.
The overall changes in biomass calculated within the 8 km2 region set around the MPP site (box in Figures 2, 4) were presented as percentages of changes in relation to the baseline “no addition of the MPP.” The choice of the size of the region was a compromise within potentially small spatial impact of seabirds and harbor seals dislocations as well as attraction to the farm of various top-predators (Supplementary Figure 5), medium spatial impact of salmon farms’ eutrophication, from a few 100 m in shallow waters to 2–3 km in deeper-water (Figure 6) and the uncertain responses of cetaceans to noise that might extend over tens of kilometers (Supplementary Figure 5).
Consequently, the size of the region within which we assessed the MPP’s impacts might have led to overestimation of the impact of top-predators’ noise avoidance whilst underestimating the effects of farm-related eutrophication.
The overall results showed small impacts (both increases and decreases) between ± 5–25% relative changes for most of the functional groups (Supplementary Figure 3). Higher impacts were found for harbor porpoise (with ∼45% decrease) and, small and large zooplankton (with more than 60 and 30% increases, respectively) (Figure 5), but these were localized to a few km from the MPP site (Figure 6 and Supplementary Figure 2).
The ability of species such as harbor seals and porpoises to detect and react to the sound emitted by operational OWTs has been identified as a potential concern, especially when considering cumulative noise from multiple turbines (Koschinski et al., 2003; Tougaard et al., 2020; Stöber and Thomsen, 2021). The model was able to represent estimates of avoidance behavior of sensitive species to underwater noise. When the impact of noise produced by OWTs was tested (Scenario 2), a significant decrease of 45% for harbor porpoise within the region). Like other cetaceans, harbor porpoises rely greatly on their sense of sound for orientation, mating and feeding. Low frequency noise is a known deterrent for cetaceans and they have been documented to avoid high traffic areas (Dyndo et al., 2015; Forney et al., 2017; Wisniewska et al., 2018). Wind turbines might produce low frequency noise: areas with wind turbines could potentially displace cetaceans to areas of suboptimal foraging with reduced prey availability (Forney et al., 2017).
The response function to noise for this species was created using a conservative approach, with a gradual displacement between 0 and 7 km from the source (Supplementary Figure 5), and considering a small impact produced by four wind turbines associated to one barge: if multiple off-shore farm sites are transformed to MPP, the impact on harbor porpoises might be more significant.
While numerous studies exist on the effects of shipping noise on cetaceans and pinnipeds (Dyndo et al., 2015; Mikkelsen et al., 2019), studies on impacts from sustained low frequency noise from operational single or arrays of OWTs are rare. However it has been shown that both species groups to return to OWT farms after initial displacement during construction are not readily available (Scheidat et al., 2011; Teilmann and Carstensen, 2012). Moreover, here we are not considering species habituation to noise.
A recent study demonstrated that seals also responded to low frequency noise from ships by changing their diving behavior (Mikkelsen et al., 2019). Here the potential impact of noise was simulated by a response function using preliminary results from a recent study showing dislocation of harbor seal up to 2 km from a tidal turbine noise source (Joe Onoufriou and Laura Palmer, St Andrews University, EIMR2020 conference presentations, April 21st–23rd 2020) (Figure 3 and Supplementary Figure 5). This assumption was reinforced by recent studies that showed that the low frequency noise can be 5 dB higher than ambient noise at 2.3 km distance from an operational tidal turbine (Risch et al., 2020) that can cause significant avoidance (78%) at 140 m from the source (Palmer et al., in review): however tidal turbines produce considerably louder noise than wind farms. Harbor seals can show significant displacement (up to 25 km) from the wind farm construction site during piling activity, however, not significant displacement during construction as a whole was found (Russell et al., 2016). Spatial and temporal quantification of avoidance of wind farms by harbor seals is therefore still uncertain, accurate environmental assessments are then required in order to quantify the duration of any avoidance (Russell et al., 2016).
Since intermittent low frequency noise alters species behavior for a short duration, a sustained noise from OWTs may alter it for longer, which could have an impact on their food consumption rates. Assessing the impacts of continuous noise is challenging (Mikkelsen et al., 2019) and in this study we are not considering the possibility that species could habituate to the noise to a point at which it no longer interferes with their feeding behaviors. The reason behind habituation which would be recorded as reduced behavioral responses has, however, been misinterpreted and often attributed to other causes (Wright et al., 2007). It is difficult to model animal responses as each individual can behave differently depending on its natural behavior and the historical environmental pressures it has endured. In our approach the impact of noise on the species can only be modeled to show population level responses, therefore we are not considering individual behaviors and ecological adaptation to this pressure. There are different sources of low-frequency noise that can affect top-predators in the West Coast of Scotland, from shipping noise (Harvey, 2018), to tidal turbines (Hastie et al., 2017). Our results in relation to noise impacts are strongly dependent on the source of the noise and species responses to this pressure. The functions that we used have been built inferring these responses from other sources of noise rather than OWTs because of current lack of data. Our results suggested the importance of quantitative measures of noise produced (Risch et al., 2020; Tougaard et al., 2020; Stöber and Thomsen, 2021) in relation with species specific responses (Teilmann and Carstensen, 2012).
Harbor seals are known to be opportunistic feeders and their attraction to fish farms have been controversial. Some studies found them in the vicinity of fish farms (Nelson et al., 2006), while others find no attraction to fish farms (Jacobs and Terhune, 2000). Our model output showed an increase of only ∼3–4% under scenarios testing attraction and increasing production (scenario 3–5). The results for seabirds for the same scenarios mirrored these results with small increases when testing the salmon farms attraction pressure. Seabirds have shown avoidance as well as attraction to OWTs to varying degrees depending on the species (Furness et al., 2013; Marques et al., 2014; Vanermen et al., 2015, 2019; Dierschke et al., 2016; Callier et al., 2018). As the responses are species-specific we used a recent monitoring study that identified sensitive species to attraction and avoidance by offshore wind farms in the Belgian part of the North Sea (Vanermen et al., 2019) and applied proportional dislocations, however, to better assess these impacts the seabirds group should be separated by relevant species. Our results did not show a trade-off with increasing productivity around the salmon farm mitigating the impact of induced dislocation by OWTs on seabird biomass at the MPP site.
Changes of top-predator distributions might have an effect of their prey through top-down controls. In this study only small responses of prey driven by predation were found, with the exception of whiting, which unexpectedly decreased in concurrency to the decrease of its predator, harbor porpoise, when testing the impact of noise (scenario 2). This is due to complex predator/prey and competition interactions: porpoises, cod and saithe are competing for whiting (adult and juveniles) as a main prey, and saithe also exhibited a top-down control on juvenile stages of the other gadoid species. This results suggested that the predation pressure and competition within gadoids have a stronger control on these stocks that other top-predators (Baudron et al., 2019). Ecospace spatial simulations showed that the decrease of whiting biomass is determined by small cumulative impacts among its predators, mainly on its juvenile stages. Temporal scenarios showed that this pressure impacted whiting trophic interactions only at the beginning of the simulation. In fact, the food web reached a new equilibrium by the end of the simulation that suggest that whiting biomass will not be affected by these pressures in the longer run (steady state) (Supplementary Figure 6).
The overall changes caused by MPP pressures are impacting different pathways of the food-web. Scenarios 2 and 3 tested the impact of top-predator distribution changes driven by noise and salmon farming site attraction, respectively: predator top-down controls are weakly cascading thought the food-web as their impacts were distributed across multiple preys reflecting the complexity of their trophic interactions. The attraction to the MPP site was applied to all gadoid top-predators (scenario 3) and model confirmed the higher response (higher relative biomass) of saithe around the farming site being predators of other gadoids and more reliant on excess feed (Dempster et al., 2009, 2010). Saithe is a generalist species, feeding on many organisms including crustaceans, herring, Norway pout, zooplankton and juvenile stages of other gadoid species (Du Buit, 1991; Sarno et al., 2005; Homrum et al., 2012; Supplementary Table 2), upon which saithe exert top-down control (Alexander et al., 2015; Serpetti et al., 2017; Baudron et al., 2019) making the relationships within these groups particularly complex to disentangle. In Norway, mature cod were found feeding on saithe in the vicinity of salmon farms (Bagdonas et al., 2012), suggesting that more data are needed to understand the relationships between these species. Moreover, some mid-trophic level species such as cod and sole (Solea solea) also seems to show behavioral responses to noise (Bailey et al., 2014), which might mitigate this attraction to the MPP site, however, this was not tested in this model.
Scenarios 4–5 tested the impact of two different bottom-up pressures affecting the food-web through two different pathways: detritus enrichment and enhanced primary production. Bottom-up pathways have high energy transfer efficiency (Armengol et al., 2019), where the energy mainly flows to few predators groups, and can strongly affect food web structure and biodiversity (Moore et al., 2004). The detritus pathways did not show an amplification of the signal as the energy produced by the consumption of detritus weakens after each predator- prey interactions (Moore et al., 2004). However, this might be due to the fact that the model does not have a well-defined microbial loop, therefore missing the trophic relationships between infauna and bacterial communities that could amplifying the detritus pathway. Future work should include the microbial loop in the model to highlight the dissipation of energy through these pathways. The primary productivity pathway, however, showed an amplification of the signal through the food-web (Christensen, 2013; Chust et al., 2014), with a large increase of relative biomass of small zooplankton; however this amplification did not cascade to higher trophic level (e.g., large zooplankton and herring) as found for ecosystems with a more planktonic food web structure with high energy transfer efficiency (Heymans and Baird, 2000; Armengol et al., 2019).
Pressures caused by MPP elements impacted different pathways of the food-web with evidence of cumulative mitigating impact. The cumulative application of pressures (scenario 6) that lead to increased productivity of the region e.g., attraction of wild fish (scenario 3), detritus and phytoplankton enrichments (scenario 4 and 5) seems to override the negative impact of noise on harbor seal, adult cod and haddock and whiting adults and juveniles (Table 3).
Harbor porpoises were not attracted to the MPP site: the overall increases in productivity simulated in scenario 2-4- and 5 did not increase their relative biomasses within the region probably due to their high dispersal rate (Supplementary Table 5) and therefore their capability on feeding in other grounds.
Unexpectedly, seabirds did not show cumulative mitigating pressures despite their increases within the region under enhanced productivity scenarios (Table 3): this is probably caused by the relatively strong impact of spatial displacement within the size of the region (Supplementary Figure 5) and highlight, once again the necessity of separated this functional group in relevant species in order to better assess this impacts.
The spatial distributions of infauna and epifauna and small and large zooplankton responded to the farming foot-print enrichment by both detritus and phytoplankton in relation to their dispersal rates, suggesting the importance of carrying out a sensitivity test with different rates and the need for sampling infauna and epifauna on a real farm site in order to validate the model results. Moreover, NewDepomod was run for 1 year and the monthly model output was coupled with Ecospace at monthly timesteps, assuming that the farm was kept operational for 3 continuous years. The 3 years average was chosen for this specific case study as salmon farming production cycle lasts about 2–3 years, often fallowed after harvesting, for 2–6 months, before farming a new generation at the same site (Mowi, 2020). During this time we also assumed that OWTs will not be operational.
The implementation of the MPP pressures perturbated the ecosystem: after 3 years, many functional groups are approaching a new steady state with few exceptions such as cod, benthic fish species and lobster and velvet crab, within invertebrate species (Supplementary Figure 7). Other benthopelagic fish is the only group that could reach significant higher biomass at steady state of the cumulative scenario (Supplementary Figure 7): this group very heterogeneous, constituted by 39 species with pelagic and benthic feeding habits, therefore taking advantage of both productivity increases via detritus and phytoplankton.
This model is a preliminary study of a hypothetical MPP development on a single site. Moving toward renewables as a greener and more sustainable option in the face of climate change, and the necessity of aquaculture production, we propose the use of MPP to maximize the benefits of these expansions and minimize their impacts. In these hypothetical simulations, the proposed MPP site contained a small barge with four wind turbines, however, wind may sometimes be unreliable and not produce sufficient energy to run the farm. An addition of wave energy converter to the MPP would supplement the power produced by the turbines (Langhamer et al., 2010).
As for any model, validation is an important aspect to produce accurate predictions. Assessing uncertainties of EwE spatial outputs associated to the input data is not currently possible in Ecopath with Ecosim. However there are a few examples of validation of Ecospace predictions using spatial time-series of biomass and fishing effort (Romagnoni et al., 2015) and Bayesian belief network approach coupled with Ecospace in order to validate and/or drive the model to assess its performances (Coll et al., 2019). The limited availability of validation material for this study, and uncertainties around the assumptions made regarding noise pressure responses and species habitat preferences were the main limitations to this study. Therefore in future a sensitivity test should be carried out in order to assess the model performances for different proportions of species habitat preferences, for different dispersal rates, and for different response functions to noise. Model validation is also critical through the collection of target data around fish farms and floating OWTs areas.
An MPP site may have difficulties in gaining support from local stakeholders due to conflict of interest for fishers because of the likelihood of imposition of fishery exclusion zones near farms (Bagdonas et al., 2012). To avoid competition for areas between fisheries and aquaculture, a realistic management framework which enforces restrictions would be required if interactions between fisheries and aquaculture become intense (Dempster et al., 2009, 2010). This would also disrupt the aforementioned “ecological service” of the fish feeding on excess waste from the farm as this new food source for wild fish stock may reduce their quality and consequently the value of the catches (Uglem et al., 2020).
The site at which this hypothetical MPP was added in this study was chosen based on the availability of data for an existing farm located in the vicinity of the Isle of Muck. No assessments were carried out to assess whether this would be an economically viable placement for a salmon farm. Future investigations will also need a Marine Environmental Impact Assessment (MEIA). MPPs provide a unique opportunity for more effective usage of marine spaces and economic growth, here presented by a combination of aquaculture and energy. They may also provide more environmentally friendly solutions such as integrated multi-trophic aquaculture systems which are extending aquaculture production while reducing nitrogen release (Troell et al., 2009). These prospects are regarded as possibilities to move into a future with more sustainable economic developments at sea and therefore toward Blue Growth and a Blue economy (Stuiver et al., 2016).
Ecospace can be a useful tool for investigating the potential impact of cumulative pressures on the food-web. The model was able to simulate the impact of several potential pressures produced by the MPP that are impacting the ecosystem food-web from different levels, from top-predator pathways (top-down controls), mid-trophic levels (simulating spatial distribution changes due to predator attractions to salmon farms), to phytoplankton and detritus pathways (bottom-up controls). In some cases the model was also able to identify potential mitigating impact through cumulative effects with detritus and phytoplankton enrichments that seems to mitigate the indirect impact of noise for some species.
Ecospace computations are quite demanding (as they perform the food-web interactions of many species in time and space); therefore it is not possible to run models with high resolution and large spatial domains. A new plug-in, currently under development (Steenbeek et al., 2019), will allow to scale up Ecospace high-resolution models to larger scales. This will allow us to scale-up the results presented in here to a larger West Coast of Scotland Ecospace model domain (Alexander et al., 2016; Harvey, 2018) in order to assess the single and cumulative impacts of multiple MPP installations in the ecosystem.
The impacts of these pressures remain site-specific, depending on the food-web structure, currents, water stratification, potential anoxia events and of course type of aquaculture. Environmental impacts vary considerably depending on the specie farmed, the association of shellfish and kelp farming, through Integrated Multi-Trophic Aquaculture could be an alternative solution to reduce eutrophication by fish farming (Buck et al., 2018).
The major limitation of this approach was based on the lack of current data to develop accurate response function to noise. The responses to this pressure on harbor porpoise and harbor seal were inferred using different sources of noise (from shipping and tidal turbines, respectively) due to lack of quantitative responses of this species in relation to noise generated by OWTs. Consequently these outputs cannot currently be used for spatial management until better specific quantitative data in relation to OWT noise and potential avoidance responses among marine species can be collected and model outputs validated. Similarly, the seabird group used here included a wide range of species with very different ecological needs. Further work is required to adequately separate out these species to allow more accurate evaluation of food web impacts caused by developments such as the MPP in this study.
Data Availability Statement
The original contributions presented in the study are included in the article/Supplementary Material, further inquiries can be directed to the corresponding author/s.
Author Contributions
NS carried out the analysis and wrote the manuscript. SBe and JW contributed to the analysis and writing the manuscript. SBr carried out the NEWDepomod model run. MC, DR, and BW contributed to editing the manuscript. BH carried out the Ecopath with Ecosim update and model fitting. JH contributed to writing the manuscript and in the model analysis. AH contributed to editing the manuscript and support for aquaculture topic. SR contributed on the scenarios runs in Ecospace and writing the manuscript. All authors contributed to the article and approved the submitted version.
Funding
This work was supported by the Engineering and Physical Sciences Research Council UK (EPSRC) and the Natural Environment Research Council UK (NERC), through grant EP/R007497/1 and EP/R007497/2, and the Natural Science Foundation of China (NSFC) through grant 51761135013.
Conflict of Interest
The authors declare that the research was conducted in the absence of any commercial or financial relationships that could be construed as a potential conflict of interest.
Supplementary Material
The Supplementary Material for this article can be found online at: https://www.frontiersin.org/articles/10.3389/fmars.2021.694013/full#supplementary-material
Footnotes
References
Abhinav, K. A., Collu, M., Benjamins, S., Cai, H. W., Hughes, A., Jiang, B., et al. (2020). Offshore multi -purpose platforms for a blue growth: a technological, environmental and socio-economic review. Sci. Total Environ. 734:138256. doi: 10.1016/j.scitotenv.2020.138256
Abhinav, K. A., Collu, M., Ke, S., and Binzhen, Z. (2019). “Frequency domain analysis of a hybrid aquaculture-wind turbine offshore floating system,” in ASME 2019 38th International Conference on Ocean, Offshore and Arctic Engineering, (New York, NY: American Society of Mechanical Engineers).
Adams, T., Black, K., Carpenter, T., Hughes, A., Reinardy, H., and Weeks, R. (2020). Parameterising resuspension in aquaculture waste deposition modelling. Aquac. Environ. Interact. 12, 401–415. doi: 10.3354/aei00372
Ahrens, R. N. M., Walters, C. J., and Christensen, V. (2012). Foraging arena theory. Fish Fish. 13, 41–59. doi: 10.1111/j.1467-2979.2011.00432.x
Alexander, K. A., Heymans, J. J., Magill, S., Tomczak, M. T., Holmes, S. J., and Wilding, T. A. (2015). Investigating the recent decline in gadoid stocks in the west of Scotland shelf ecosystem using a foodweb model. ICES J. Mar. Sci. 72, 436–449. doi: 10.1093/icesjms/fsu149
Alexander, K. A., Meyjes, S. A., and Heymans, J. J. (2016). Spatial ecosystem modelling of marine renewable energy installations: gauging the utility of ecospace. Ecol. Model. 331, 115–128. doi: 10.1016/j.ecolmodel.2016.01.016
Aleynik, D., Dale, A. C., Porter, M., and Davidson, K. (2016). A high resolution hydrodynamic model system suitable for novel harmful algal bloom modelling in areas of complex coastline and topography. Harmful Algae 53, 102–117. doi: 10.1016/j.hal.2015.11.012
Aquaculture Scotland (2020). Interactive Map of Scottish Marine Finfish Aquaculture Sites. Available online at: http://aquaculture.scotland.gov.uk/map/map.aspx (Accessed March 15, 2021)
Armengol, L., Calbet, A., Franchy, G., Rodríguez-Santos, A., and Hernández-León, S. (2019). Planktonic food web structure and trophic transfer efficiency along a productivity gradient in the tropical and subtropical Atlantic Ocean. Sci. Rep. 9:2044. doi: 10.1038/s41598-019-38507-9
Bagdonas, K., Humborstad, O.-B., and Løkkeborg, S. (2012). Capture of wild saithe (Pollachius virens) and cod (Gadus morhua) in the vicinity of salmon farms: three pot types compared. Fish. Res. 134–136, 1–5. doi: 10.1016/j.fishres.2012.06.020
Bailey, H., Brookes, K. L., and Thompson, P. M. (2014). Assessing environmental impacts of offshore wind farms: lessons learned and recommendations for the future. Aquat. Biosyst. 10:8. doi: 10.1186/2046-9063-10-8
Bailey, H., Senior, B., Simmons, D., Rusin, J., Picken, G., and Thompson, P. M. (2010). Assessing underwater noise levels during pile-driving at an offshore windfarm and its potential effects on marine mammals. Mar. Pollut. Bull. 60, 888–897. doi: 10.1016/j.marpolbul.2010.01.003
Baudron, A. R., Serpetti, N., Fallon, N. G., Heymans, J. J., and Fernandes, P. G. (2019). Can the common fisheries policy achieve good environmental status in exploited ecosystems: the west of Scotland demersal fisheries example. Fish. Res. 211, 217–230. doi: 10.1016/j.fishres.2018.10.024
Benjamins, S., Harnois, V., Smith, H. C. M., Johanning, L., Greenhill, L., Carter, C., et al. (2014). Understanding the Potential for Marine Megafauna Entanglement Risk from Marine Renewable Energy Developments (Report No. 791). Inverness: Scottish Natural Heritage.
Benjamins, S., Masden, E., and Coll, M. (2020). Integrating wind turbines and fish farms an evaluation of potential risks to marine and coastal bird species. J. Mar. Sci. Eng. 8:414. doi: 10.3390/jmse8060414
Bonsdorff, E., Blomqvist, E. M., Mattila, J., and Norkko, A. (1997). Coastal eutrophication: causes, consequences and perspectives in the Archipelago areas of the northern Baltic Sea. Estuar. Coast. Shelf Sci. 44, 63–72. doi: 10.1016/S0272-7714(97)80008-X
Brandt, M. J., Diederichs, A., Betke, K., and Nehls, G. (2011). Responses of harbour porpoises to pile driving at the Horns Rev II offshore wind farm in the Danish North Sea. Mar. Ecol. Prog. Ser. 421, 205–216. doi: 10.3354/meps08888
Buck, B. H., Troell, M. F., Krause, G., Angel, D. L., Grote, B., and Chopin, T. (2018). State of the art and challenges for offshore Integrated Multi-Trophic Aquaculture (IMTA). Front. Mar. Sci. 5:165. doi: 10.3389/fmars.2018.00165
Callier, M. D., Byron, C. J., Bengtson, D. A., Cranford, P. J., Cross, S. F., Focken, U., et al. (2018). Attraction and repulsion of mobile wild organisms to finfish and shellfish aquaculture: a review. Rev. Aquac. 10, 924–949. doi: 10.1111/raq.12208
Casale, C., Serri, L., Stolk, N., Yildiz, I., and Cantu’, Y. M. (2012). Synergies, Innovative Designs and Concepts for Multipurpose use of Conversion Platforms. ORECCA Project Report-WP4 (FP7).
Chary, K., Callier, M. D., Covès, D., Aubin, J., Simon, J., and Fiandrino, A. (2021). Scenarios of fish waste deposition at the sub-lagoon scale: a modelling approach for aquaculture zoning and site selection. ICES J. Mar. Sci. fsaa238. doi: 10.1093/icesjms/fsaa238
Christensen, V. (2013). Ecological networks in fisheries: predicting the future? Fisheries 38, 76–81. doi: 10.1080/03632415.2013.757987
Christensen, V., and Walters, C. J. (2004). Ecopath with ecosim: methods, capabilities and limitations. Ecol. Model. 172, 109–139. doi: 10.1016/j.ecolmodel.2003.09.003
Christensen, V., Coll, M., Steenbeek, J., Buszowski, J., Chagaris, D., and Walters, C. J. (2014). Representing variable habitat quality in a spatial food web model. Ecosystems 17, 1397–1412. doi: 10.1007/s10021-014-9803-3
Chust, G., Allen, J. I., Bopp, L., Schrum, C., Holt, J., Tsiaras, K., et al. (2014). Biomass changes and trophic amplification of plankton in a warmer ocean. Glob. Chang. Biol. 20, 2124–2139. doi: 10.1111/gcb.12562
Coates, D. A., Kapasakali, D.-A., Vincx, M., and Vanaverbeke, J. (2016). Short-term effects of fishery exclusion in offshore wind farms on macrofaunal communities in the Belgian part of the North Sea. Fish. Res. 179, 131–138. doi: 10.1016/j.fishres.2016.02.019
Coll, M., Akoglu, E., Arreguin-Sanchez, F., Fulton, E. A., Gascuel, D., Heymans, J. J., et al. (2015). Modelling dynamic ecosystems: venturing beyond boundaries with the Ecopath approach. Rev. Fish Biol. Fish. 25, 413–424. doi: 10.1007/s11160-015-9386-x
Coll, M., Pennino, M. G., Steenbeek, J., Sole, J., and Bellido, J. M. (2019). Predicting marine species distributions: complementarity of food-web andBayesian hierarchical modelling approaches. Ecol. Model. 405, 86–101. doi: 10.1016/j.ecolmodel.2019.05.005
Coll, M., Steenbeek, J., Sole, J., Palomera, I., and Christensen, V. (2016). Modelling the cumulative spatial-temporal effects of environmental drivers and fishing in a NW Mediterranean marine ecosystem. Ecol. Model. 331, 100–114. doi: 10.1016/j.ecolmodel.2016.03.020
Colleter, M., Gascuel, D., Albouy, C., Francour, P., de Morais, L. T., Valls, A., et al. (2014). Fishing inside or outside? A case studies analysis of potential spillover effect from marine protected areas, using food web models. J. Mar. Syst. 139, 383–395. doi: 10.1016/j.jmarsys.2014.07.023
Coolen, J. W. P. (2017). North Sea Reefs: Benthic Biodiversity of Artificial and Rocky Reefs in the Southern North Sea. Ph.D. Thesis. Wageningen: Wageningen University.
Da Rocha, A. B., Lino, F. J., Correia, N., Matos, J. C., Marques, M., and Morais, T. (2010). Offshore Renewable Energy Development of Ocean Technology Projects at INEGI. Available online at: https://web.fe.up.pt/˜falves/offshore.pdf (accessed April 7, 2021).
Dannheim, J., Bergström, L., Birchenough, S. N. R., Brzana, R., Boon, A. R., Coolen, J. W. P., et al. (2020). Benthic effects of offshore renewables: identification of knowledge gaps and urgently needed research. ICES J. Mar. Sci. 77, 1092–1108. doi: 10.1093/icesjms/fsz018
Dempster, T., Sanchez-Jerez, P., Uglem, I., and Bjorn, P. A. (2010). Species-specific patterns of aggregation of wild fish around fish farms. Estuar. Coast. Shelf Sci. 86, 271–275. doi: 10.1016/j.ecss.2009.11.007
Dempster, T., Uglem, I., Sanchez-Jerez, P., Fernandez-Jover, D., Bayle-Sempere, J., Nilsen, R., et al. (2009). Coastal salmon farms attract large and persistent aggregations of wild fish: an ecosystem effect. Mar. Ecol. Prog. Ser. 385, 1–14. doi: 10.3354/meps08050
Dierschke, V., Furness, R. W., and Garthe, S. (2016). Seabirds and offshore wind farms in European waters: avoidance and attraction. Biol. Conserv. 202, 59–68. doi: 10.1016/j.biocon.2016.08.016
Dolman, S. J., and Brakes, P. (2018). Sustainable Fisheries management and the welfare of bycaught and entangled cetaceans. Front. Vet. Sci. 5:287. doi: 10.3389/fvets.2018.00287
Drewitt, A. L., and Langston, R. H. W. (2006). Assessing the impacts of wind farms on birds. IBIS 148, 29–42. doi: 10.1111/j.1474-919x.2006.00516.x
Du Buit, M. H. (1991). Food and feeding of saithe (Pollachius virens L.) off Scotland. Fish. Res. 12, 307–323. doi: 10.1016/0165-7836(91)90015-8
Dyndo, M., Wisniewska, D., Rojano-Doñate, L., Ryan, P., and Madsen, T. (2015). Harbour porpoises react to low levels of high frequency vessel noise. Sci. Rep. 5:11083. doi: 10.1038/srep11083
Food and Agriculture Organization [FAO] (2020). The State of World Fisheries and Aquaculture 2020. Sustainability in Action. Rome: FAO.
Forney, K. A., Southall, B. L., Slooten, E., Dawson, S., Read, A. J., Baird, R. W., et al. (2017). Nowhere to go: noise impact assessments for marine mammal populations with high site fidelity. Endanger. Species Res. 32, 391–413. doi: 10.3354/esr00820
Forrest, B., Keeley, N., Gillespie, P., Hopkins, G., Knight, B., Govier, D., et al. (2007). Review of the Ecological Effects of Marine Finfish Aquaculture: Final Report. Cawthron Report 1285. Nelson: Cawthron Institute.
Froehlich, H. E., Smith, A., Gentry, R. R., and Halpern, B. S. (2017). Offshore aquaculture: i know it when i see it. Front. Mar. Sci. 4:154. doi: 10.3389/fmars.2017.00154
Fulton, E. A., Bax, N. J., Bustamante, R. H., Dambacher, J. M., Dichmont, C., Dunstan, P. K., et al. (2015). Modelling marine protected areas: insights and hurdles. Philos. Trans. R. Soc. B Biol. Sci. 370:20140278.
Furness, R. W., Wade, H. M., and Masden, E. A. (2013). Assessing vulnerability of marine bird populations to offshore wind farms. J. Environ. Manag. 119, 56–66. doi: 10.1016/j.jenvman.2013.01.025
Garthe, S., and Hüppop, O. (2004). Scaling possible adverse effects of marine wind farms on seabirds: developing and applying a vulnerability index. J. Appl. Ecol. 41, 724–734. doi: 10.1111/j.0021-8901.2004.00918.x
Gascuel, D., Coll, M., Fox, C., Guenette, S., Guitton, J., Kenny, A., et al. (2016). Fishing impact and environmental status in European seas: a diagnosis from stock assessments and ecosystem indicators. Fish Fish. 17, 31–55. doi: 10.1111/faf.12090
Gatward, I., Parker, A., Billing, S., and Black, K. D. (2017). Scottish Aquaculture: A View Towards 2030. Scotland: Scottish Aquaculture Innovation Centre.
Gentry, R. R., Lester, S. E., Kappel, C. V., White, C., Bell, T. W., Stevens, J., et al. (2017). Offshore aquaculture: spatial planning principles for sustainable development. Ecol. Evol. 7, 733–743. doi: 10.1002/ece3.2637
Ghanawi, J., and McAdam, B. J. (2020). Using fatty acid markers to distinguish between effects of salmon (Salmo salar) and halibut (Hippoglossus hippoglossus) farming on mackerel (Scomber scombrus) and whiting (Merlangius merlangus). Aquac. Res. 51, 2229–2242. doi: 10.1111/are.14568
Gill, A. B., Birchenough, S., Jones, A., Judd, A., Jude, S., Payo-Payo, A., et al. (2018). Offshore Energy and Marine Spatial Planning. London: Routledge.
Gomez, C., Lawson, J., Wright, A., Buren, A., Tollit, D., and Lesage, V. (2016). A systematic review on the behavioural responses of wild marine mammals to noise: the disparity between science and policy. Can. J. Zool. 94, 801–819. doi: 10.1139/cjz-2016-0098
Grecian, W. J., Inger, R., Attrill, M. J., Bearhop, S., Godley, B. J., Witt, M. J., et al. (2010). Potential impacts of wave-powered marine renewable energy installations on marine birds. IBIS 152, 683–697. doi: 10.1111/j.1474-919x.2010.01048.x
H2Ocean (2018). Development of a Wind-Wave Power Open-Sea Platform Equipped for Hydrogen Generation with Support for Multiple Users of Energy. Stuart, FL: H2Ocean.
Harvey, B. J. (2018). Exploring Impacts of Noise From Shipping and Acoustic Deterrent Devices on Cetaceans on the West Coast of Scotland using an Ecosystem Modelling Approach. Ecosyst.-Based Manag. Mar. Syst. MSc.
Hastie, G. D., Russell, D. J. F., Lepper, P., Elliott, J., Wilson, B., Benjamins, S., et al. (2017). Harbour seals avoid tidal turbine noise: implications for collision risk. J. Appl. Ecol. 55, 684–693. doi: 10.1111/1365-2664.12981
Heymans, J. J., and Baird, D. (2000). A carbon flow model and network analysis of the northern Benguela upwelling system. Namibia. Ecol. Model. 126, 9–32. doi: 10.1016/S0304-3800(99)00192-1
Holm, P., Buck, B. H., and Langan, R. (2017). “Introduction: new approaches to sustainable offshore food production and the development of offshore platforms,” in Aquaculture Perspective of Multi-Use Sites in the Open Ocean, eds B. Buck and R. Langan (Cham: Springer).
Homrum, E., Hansen, B., Steingrund, P., and Hátún, H. (2012). Growth, maturation, diet and distribution of saithe (Pollachius virens) in Faroese waters (NE Atlantic). Mar. Biol. Res. 8, 246–254. doi: 10.1080/17451000.2011.627921
Honkanen, T., and Helminen, H. (2000). Impacts of fish farming on eutrophication: comparisons among different characteristics of ecosystem. Int. Rev. Hydrobiol. 85, 673–686. doi: 10.1002/1522-2632(200011)85:5/6<673::AID-IROH673<3.0.CO;2-O
Hyder, K., Rossberg, A. G., Allen, J. I., Austen, M. C., Barciela, R. M., Bannister, H. J., et al. (2015). Making modelling count - increasing the contribution of shelf-seas community and ecosystem models to policy development and management. Mar. Policy 61, 291–302. doi: 10.1016/j.marpol.2015.07.015
Jackson, D., Drumm, A., Fredheim, A., Lader, P., Fernáandez Otero, R., Institute, M., et al. (2011). OATP (Evaluation of the promotion of Offshore Aquaculture Through a Technology Platform). Galway: Marine Institute.
Jacobs, S. R., and Terhune, J. M. (2000). Harbor seal (Phoca vitulina) numbers along the new brunswick coast of the bay of fundy in autumn in relation to aquaculture. Northeast. Nat. 7, 289–296. doi: 10.2307/3858361
Jones, E. L., McConnell, B. J., Smout, S., Hammond, P. S., Duck, C. D., Morris, C. D., et al. (2015). Patterns of space use in sympatric marine colonial predators reveal scales of spatial partitioning. Mar. Ecol. Prog. Ser. 534, 235–249. doi: 10.3354/meps11370
Keeley, N. B., Macleod, C. K., Hopkins, G. A., and Forrest, B. M. (2014). Spatial and temporal dynamics in macrobenthos during recovery from salmon farm induced organic enrichment: when is recovery complete? Mar. Pollut. Bull. 80, 250–262. doi: 10.1016/j.marpolbul.2013.12.008
Kemper, C. M., Pemberton, D., Cawthorn, M., Heinrich, S., Mann, J., Würsig, B., et al. (2003). Aquaculture and marine mammals: co-existence or conflict? CSIRO Publishing. Available online at: https://risweb.st-andrews.ac.uk/portal/en/researchoutput/aquaculture-and-marine-mammals-coexistence-or-conflict(e4ce9e02-e800-4fe1-a99c-71a6c2ecf469).html (Accessed April 7, 2021).
Koschinski, S., Culik, B. M., Henriksen, O. D., Tregenza, N., Ellis, G., Jansen, C., et al. (2003). Behavioural reactions of free-ranging porpoises and seals to the noise of a simulated 2 MW windpower generator. Mar. Ecol. Prog. Ser. 265, 263–273. doi: 10.3354/meps265263
Krone, R., Gutow, L., Joschko, T. J., and Schröder, A. (2013). Epifauna dynamics at an offshore foundation – Implications of future wind power farming in the North Sea. Mar. Environ. Res. 85, 1–12. doi: 10.1016/j.marenvres.2012.12.004
Langhamer, O., Haikonen, K., and Sundberg, J. (2010). Wave power—Sustainable energy or environmentally costly? A review with special emphasis on linear wave energy converters. Renew. Sustain. Energy Rev. 14, 1329–1335. doi: 10.1016/j.rser.2009.11.016
Lester, S. E., Stevens, J. M., Gentry, R. R., Kappel, C. V., Bell, T. W., Costello, C. J., et al. (2018). Marine spatial planning makes room for offshore aquaculture in crowded coastal waters. Nat. Commun. 9:945. doi: 10.1038/s41467-018-03249-1
Madsen, P. T., Wahlberg, M., Tougaard, J., Lucke, K., and Tyack, P. (2006). Wind turbine underwater noise and marine mammals: implications of current knowledge and data needs. Mar. Ecol. Prog. Ser. 309, 279–295. doi: 10.3354/meps309279
Marques, A. T., Batalha, H., Rodrigues, S., Costa, H., Pereira, M. J. R., Fonseca, C., et al. (2014). Understanding bird collisions at wind farms: an updated review on the causes and possible mitigation strategies. Biol. Conserv. 179, 40–52. doi: 10.1016/j.biocon.2014.08.017
Mente, E., Martin, J. C., Tuck, I., Kormas, K. A., Santos, M. B., Bailey, N., et al. (2010). Mesoscale effects of aquaculture installations on benthic and epibenthic communities in four Scottish sea lochs. Aquat. Living Resour. 23, 267–276. doi: 10.1051/alr/2010030
Mikkelsen, L., Johnson, M., Wisniewska, D. M., Neer, A., van Siebert, U., Madsen, P. T., et al. (2019). Long-term sound and movement recording tags to study natural behavior and reaction to ship noise of seals. Ecol. Evol. 9, 2588–2601. doi: 10.1002/ece3.4923
Moore, J. C., Berlow, E. L., Coleman, D. C., Ruiter, P. C., de Dong, Q., Hastings, A., et al. (2004). Detritus, trophic dynamics and biodiversity. Ecol. Lett. 7, 584–600. doi: 10.1111/j.1461-0248.2004.00606.x
Morissette, L., Kaschner, K., and Gerber, L. R. (2010). Ecosystem models clarify the trophic role of whales off Northwest Africa. Mar. Ecol. Prog. Ser. 404, 289–302. doi: 10.3354/meps08443
Nall, C. R., Schläppy, M.-L., and Guerin, A. J. (2017). Characterisation of the biofouling community on a floating wave energy device. Biofouling 33, 379–396. doi: 10.1080/08927014.2017.1317755
National Marine Fisheries Service (2018). 2018 Revisions to: Technical Guidance for Assessing the Effects of Anthropogenic Sound on Marine Mammal Hearing (Version 2.0): Underwater Thresholds for Onset of Permanent and Temporary Threshold Shifts (Report No. NMFS-OPR-59). Washington, DC: NOAA.
Nelson, M., Gilbert, J., and Boyle, K. (2006). The influence of siting and deterrence methods on seal predation at Atlantic salmon (Salmo salar) farms in Maine, 2001–2003. Can. J. Fish. Aquat. Sci. 63, 1710–1721. doi: 10.1139/f06-067
Nordvarg, L. (2001). Predictive models and eutrophication effects of fish farms. Acta Universitatis Upsaliensis. Comprehensive Summaries of Uppsala Dissertations from the Faculty of Science and Technology 602. Available online at: https://www.diva-portal.org/smash/get/diva2:166747/FULLTEXT01.pdf (Accessed April 29, 2021)
Northridge, S., Coram, A., and Gordon, J. (2013). Investigations on Seal Depredation at Scottish Fish Farms. Report to Marine Scotland. Edinburgh: Scottish Government.
Piroddi, C., Bearzi, G., and Christensen, V. (2011). Marine open cage aquaculture in the eastern Mediterranean Sea: a new trophic resource for bottlenose dolphins. Mar. Ecol. Prog. Ser. 440, 255–266. doi: 10.3354/meps09319
Piroddi, C., Coll, M., Liquete, C., Macias, D., Greer, K., Buszowski, J., et al. (2017). Historical changes of the Mediterranean Sea ecosystem: modelling the role and impact of primary productivity and fisheries changes over time. Sci. Rep. 7, 1–18.
Polaris Wind Power Network (2018). The Demolition of all 48 Taiwan Wind Turbines in Long Island, Shandong Province, in Chinese. Available online at: http://news.bjx.com.cn/html/20170821/844599.shtml (accessed May 29, 2018).
Puts, M., Taylor, M., Núñez-Riboni, I., Steenbeek, J., Stabler, M., Mollmann, C., et al. (2020). Insights on integrating habitat preferences in process-oriented ecological models – a case study of the southern North Sea. Ecol. Model. 431:109189. doi: 10.1016/j.ecolmodel.2020.109189
Quevedo, E., Cartón, M., Delory, E., Castro, A., Hernández, J., Llinás, O., et al. (2013). “Multi-use offshore platform configurations in the scope of the FP7 TROPOS Project,” in Proceedings of the 2013 MTS/IEEE OCEANS - Bergen, (Bergen: IEEE), 1–7. doi: 10.1109/OCEANS-Bergen.2013.6608061
Quick, N. J., Middlemas, S. J., and Armstrong, J. D. (2004). A survey of antipredator controls at marine salmon farms in Scotland. Aquaculture 230, 169–180. doi: 10.1016/S0044-8486(03)00428-9
Recalde, L., Yue, H., Leithead, W., Anaya-Lara, O., Liu, H. D., and You, J. (2019). “Hybrid renewable energy systems sizing for offshore multi-purpose platforms,” in International Conference on Offshore Mechanics and Arctic Engineering-American Society of Mechanical Engineers, 7. doi: 10.1115/OMAE2019-96017
Risch, D., van Geel, N., Gillespie, D., and Wilson, B. (2020). Characterisation of underwater operational sound of a tidal stream turbine. J. Acoust. Soc. Am. 147, 2547–2555. doi: 10.1121/10.0001124
Roach, M., Cohen, M., Forster, R., Revill, A. S., and Johnson, M. (2018). The effects of temporary exclusion of activity due to wind farm construction on a lobster (Homarus gammarus) fishery suggests a potential management approach. ICES J. Mar. Sci. 75, 1416–1426. doi: 10.1093/icesjms/fsy006
Romagnoni, G., Mackinson, S., Hong, J., and Eikeset, A. M. (2015). The Ecospace model applied to the North Sea: evaluating spatial predictions with fish biomass and fishing effort data. Ecol. Model. 300, 50–60. doi: 10.1016/j.ecolmodel.2014.12.016
Ross, A. (1988). Controlling Nature’s Predators on Fish Farms: A Report. United Kingdom: Marine Conservation Society.
Ruardij, P., and Van Raaphorst, W. (1995). Benthic nutrient regeneration in the ERSEM ecosystem model of the North Sea. Neth. J. Sea Res. 33, 453–483. doi: 10.1016/0077-7579(95)90057-8
Russell, D. J. F., Hastie, G. D., Thompson, D., Janik, V. M., Hammond, P. S., Scott-Hayward, L. A. S., et al. (2016). Avoidance of wind farms by harbour seals is limited to pile driving activities. J. Appl. Ecol. 53, 1642–1652. doi: 10.1111/1365-2664.12678
Ryther, J. H., and Dunstan, W. M. (1971). Nitrogen, phosphorus, and eutrophication in the coastal marine environment. Science 171, 1008–1013. doi: 10.1126/science.171.3975.1008
Sarno, B., Glass, C., and Smith, G. (2005). Differences in diet and behaviour of sympatric saithe and pollack in a Scottish Sea Loch. J. Fish Biol. 45, 1–11. doi: 10.1111/j.1095-8649.1994.tb01080.x
Scheidat, M., Tougaard, J., Brasseur, S., Carstensen, J., Petel, T., van, P., et al. (2011). Harbour porpoises (Phocoena phocoena) and wind farms: a case study in the Dutch North Sea. Environ. Res. Lett. 6:025102. doi: 10.1088/1748-9326/6/2/025102
Scottish Environment Protection Agency (2020). Aquaculture Modelling Regulatory Modelling Guidance for the Aquaculture Sector July 2019 – Version 1.1. Available online at: https://www.sepa.org.uk/media/450279/regulatory-modelling-guidance-for-the-aquaculture-sector.pdf (Accessed November 27, 2020)
Scottish-Government (2016). Grey and Harbour Seal Diet Composition and Prey Consumption in the West of Scotland 2010/11 Supporting Paper. Calton Hill: Scottish-Government.
Serpetti, N. (2012). Modelling and Mapping the Physical and Biogeochemical Properties of Sediments on the North Sea Coastal Waters. Available online at: https://ethos.bl.uk/OrderDetails.do?uin=uk.bl.ethos.553875 (Accessed December 1, 2020).
Serpetti, N., Baudron, A. R., Burrows, M. T., Payne, B. L., Helaouët, P., Fernandes, P. G., et al. (2017). Impact of ocean warming on sustainable fisheries management informs the ecosystem approach to fisheries. Sci. Rep. 7:13438. doi: 10.1038/s41598-017-13220-7
Skilbrei, O. T., and Otterå, H. (2016). Vertical distribution of saithe (Pollachius virens) aggregating around fish farms. ICES J. Mar. Sci. 73, 1186–1195. doi: 10.1093/icesjms/fsv261
Soetaert, K., and Middelburg, J. J. (2009). Modeling eutrophication and oligotrophication of shallow-water marine systems: the importance of sediments under stratified and well-mixed conditions. Hydrobiologia 629, 239–254. doi: 10.1007/s10750-009-9777-x
Soto, N. A., Johnson, M., Madsen, P. T., Tyack, P. L., Bocconcelli, A., and Borsani, J. F. (2006). Does intense ship noise disrupt foraging in deep-diving cuvier’s beaked whales (ziphius Cavirostris)? Mar. Mamm. Sci. 22, 690–699. doi: 10.1111/j.1748-7692.2006.00044.x
Southall, B. L., Finneran, J. J., Reichmuth, C., Nachtigall, P. E., Ketten, D. R., Bowles, A. E., et al. (2019). Marine mammal noise exposure criteria: updated scientific recommendations for residual hearing effects. Aquat. Mamm. 45, 125–232. doi: 10.1578/am.45.2.2019.125
Southall, B. L., Bowles, A. E., Ellison, W. T., Finneran, J. J., Gentry, R. L., Greene, C. R., et al. (2007). Marine mammal noise exposure criteria: initial scientific recommendations. Aquat. Mamm. 33, 411–414. doi: 10.1578/AM.33.4.2007.411
Steenbeek, J., Buszowski, J., Christensen, V., Akoglu, E., Aydin, K., Ellis, N., et al. (2016). Ecopath with Ecosim as a model-building toolbox: source code capabilities, extensions, and variations. Ecol. Model. 319, 178–189. doi: 10.1016/j.ecolmodel.2015.06.031
Steenbeek, J., Claudet, J., and Coll, M. (2019). “Assessing the ecological and fisheries benefits of MPA networks in the Western Mediterranean Sea using a geographically nested ecosystem modelling approach,” in Ecopath 35 Years Conference: Making Ecosystem-Based Management Operational, (Florida, USA: Ecopath Research and Development Consortium).
Steenbeek, J., Coll, M., Gurney, L., Melin, F., Hoepffner, N., Buszowski, J., et al. (2013). Bridging the gap between ecosystem modeling tools and geographic information systems: driving a food web model with external spatial-temporal data. Ecol. Model. 263, 139–151. doi: 10.1016/j.ecolmodel.2013.04.027
Stefanakou, A., Dagkinis, I., Lilas, T., Maglara, A., and Vatistas, A. (2016). “Development of a floating wind-desalination multi-use platform (MUP) in the context of optimal use of maritime space,” in Proceedings of the Offshore Energy and Storage Symposiyum (OSES) and Industry Connector Event, (Valletta: University of Malta).
Stöber, U., and Thomsen, F. (2021). How could operational underwater sound from future offshore wind turbines impact marine life? J. Acoust. Soc. Am. 149:1791. doi: 10.1121/10.0003760
Stuiver, M., Soma, K., Koundouri, P., van den Burg, S., Gerritsen, A., Harkamp, T., et al. (2016). The governance of multi-use platforms at sea for energy production and aquaculture: challenges for policy makers in European seas. Sustainability 8:333. doi: 10.3390/su8040333
Teilmann, J., and Carstensen, J. (2012). Negative long term effects on harbour porpoises from a large scale offshore wind farm in the Baltic—evidence of slow recovery. Environ. Res. Lett. 7:045101. doi: 10.1088/1748-9326/7/4/045101
Tett, P., Verspoor, E., Hunter, D.-C., Coulson, M., Hicks, N., Davidson, K., et al. (2018). Review of the Environmental Impacts of Salmon Farming in Scotland. Report for the Environment, Climate Change and Land Reform (ECCLR) Committee. The Scottish Parliament. Oban: Scottish Association for Marine Science, 196.
Thompson, S., Treweek, J. R., and Thurling, D. J. (1995). The potential application of strategic environmental assessment (sea) to the farming of Atlantic Salmon (Salmo-Salar L) in Mainland. J. Environ. Manag. 45, 219–229. doi: 10.1006/jema.1995.0070
Tierney, K. M., Heymans, J. J., Muir, G. K. P., Cook, G. T., Buszowski, J., Steenbeek, J., et al. (2018). Modelling marine trophic transfer of radiocarbon (C-14) from a nuclear facility. Environ. Model. Softw. 102, 138–154. doi: 10.1016/j.envsoft.2018.01.013
Tougaard, J., Henriksen, O. D., and Miller, L. A. (2009). Underwater noise from three types of offshore wind turbines: estimation of impact zones for harbor porpoises and harbor seals. J. Acoust. Soc. Am. 125, 3766–3773. doi: 10.1121/1.3117444
Tougaard, J., Hermannsen, L., and Madsen, P. T. (2020). How loud is the underwater noise from operating offshore wind turbines? J. Acoust. Soc. Am. 148, 2885–2893. doi: 10.1121/10.0002453
Troell, M., Joyce, A., Chopin, T., Neori, A., Buschmann, A. H., and Fang, J.-G. (2009). Ecological engineering in aquaculture — potential for integrated multi-trophic aquaculture (IMTA) in marine offshore systems. Aquaculture 297, 1–9. doi: 10.1016/j.aquaculture.2009.09.010
Tuya, F., Sanchez-Jerez, P., Dempster, T., Boyra, A., and Haroun, R. J. (2006). Changes in demersal wild fish aggregations beneath a sea-cage fish farm after the cessation of farming. J. Fish Biol. 69, 682–697. doi: 10.1111/j.1095-8649.2006.01139.x
Uglem, I., Karlsen, O., Sanchez-Jerez, P., and Saether, B. S. (2014). Impacts of wild fishes attracted to open-cage salmonid farms in Norway. Aquac. Environ. Interact. 6, 91–103. doi: 10.3354/aei00112
Uglem, I., Toledo-Guedes, K., Sanchez-Jerez, P., Ulvan, E. M., Evensen, T., and Sæther, B. S. (2020). Does waste feed from salmon farming affect the quality of saithe (Pollachius virens L.) attracted to fish farms? Aquac. Res. 51, 1720–1730. doi: 10.1111/are.14519
United Nations (2015). Transforming our World: the 2030 Agenda for Sustainable Development. Available online at: https://www.un.org/sustainabledevelopment/development-agenda/ (Accessed March 15, 2021)
United Nations (2019). World Population Prospects 2019: Highlights. Available online at: https://population.un.org/wpp/Publications/Files/WPP2019_Highlights.pdf (Accessed March 15, 2021).
Vanermen, N., Courtens, W., Walle, M., Verstraete, H., and Stienen, E. (2019). Seabird Monitoring at the Thornton Bank Offshore Wind Farm-Final Displacement Results After 6 Years of Post-Construction Monitoring & an Explorative Bayesian Analysis of Common Guillemot Displacement Using INLA. 85–116.
Vanermen, N., Onkelinx, T., Courtens, W., Van de walle, M., Verstraete, H., and Stienen, E. W. M. (2015). Seabird avoidance and attraction at an offshore wind farm in the Belgian part of the North Sea. Hydrobiologia 756, 51–61. doi: 10.1007/s10750-014-2088-x
Vitousek, P. M., and Howarth, R. W. (1991). Nitrogen limitation on land and in the sea: how can it occur? Biogeochemistry 13, 87–115. doi: 10.1007/BF00002772
Waggitt, J. J., Evans, P. G. H., Andrade, J., Banks, A. N., Boisseau, O., Bolton, M., et al. (2020). Quantifying seabird and cetacean distributions at annual and monthly scales in the North-East Atlantic. J. Appl. Ecol. 57, 253–269. doi: 10.1111/1365-2664.13525
Weaver, R., Hanks, J., Low, J., Flint, J., Nixon, C., and Ferguson, A. (2020). Supporting the Economic, Social and Environmental Sustainability of the UK’sMarine Sectors. Victoria Quay: Marine Scotland.
Wilson, J. C., and Elliott, M. (2009). The habitat-creation potential of offshore wind farms. Wind Energy 12, 203–212. doi: 10.1002/we.324
Wilson, L. J., and Hammond, P. S. (2019). The diet of harbour and grey seals around Britain: examining the role of prey as a potential cause of harbour seal declines. Aquat. Conserv. Mar. Freshw. Ecosyst. 29, 71–85. doi: 10.1002/aqc.3131
Wisniewska, D., Johnson, M., Teilmann, J., Siebert, U., Galatius, A., Dietz, R., et al. (2018). High rates of vessel noise disrupt foraging in wild harbour porpoises (Phocoena phocoena). Proc. R. Soc. B Biol. Sci. 285:20172314. doi: 10.1098/rspb.2017.2314
Wright, A., Aguilar de Soto, N., Baldwin, A., Bateson, M., Beale, C., Clark, C., et al. (2007). Are Marine Mammals Stressed By Anthropogenic Noise? J. Comp. Psychol. 20, 274–316.
Keywords: Ecopath with Ecosim, Ecospace, Marine Strategy Framework Directive, marine spatial planning, multi-purpose platform, offshore wind, West Coast of Scotland, aquaculture
Citation: Serpetti N, Benjamins S, Brain S, Collu M, Harvey BJ, Heymans JJ, Hughes AD, Risch D, Rosinski S, Waggitt JJ and Wilson B (2021) Modeling Small Scale Impacts of Multi-Purpose Platforms: An Ecosystem Approach. Front. Mar. Sci. 8:694013. doi: 10.3389/fmars.2021.694013
Received: 12 April 2021; Accepted: 31 May 2021;
Published: 08 July 2021.
Edited by:
Iñigo Muxika, Technological Center Expert in Marine and Food Innovation (AZTI), SpainReviewed by:
Jan Vanaverbeke, Royal Belgian Institute of Natural Sciences, BelgiumRobin Kundis Craig, The University of Utah, United States
Copyright © 2021 Serpetti, Benjamins, Brain, Collu, Harvey, Heymans, Hughes, Risch, Rosinski, Waggitt and Wilson. This is an open-access article distributed under the terms of the Creative Commons Attribution License (CC BY). The use, distribution or reproduction in other forums is permitted, provided the original author(s) and the copyright owner(s) are credited and that the original publication in this journal is cited, in accordance with accepted academic practice. No use, distribution or reproduction is permitted which does not comply with these terms.
*Correspondence: Natalia Serpetti, c2VycGV0dGluQGdtYWlsLmNvbQ==