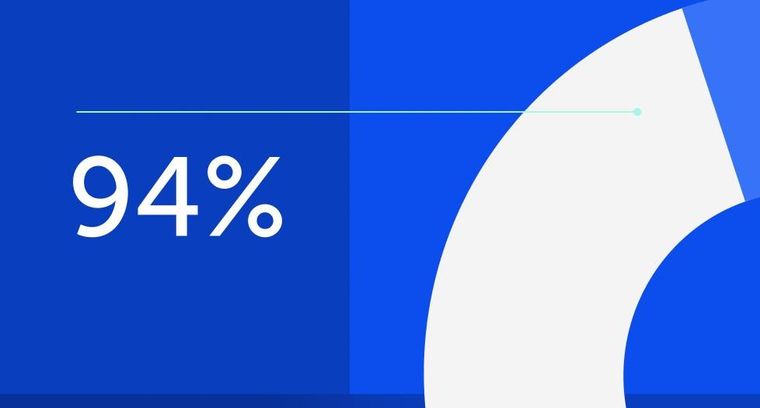
94% of researchers rate our articles as excellent or good
Learn more about the work of our research integrity team to safeguard the quality of each article we publish.
Find out more
ORIGINAL RESEARCH article
Front. Mar. Sci., 15 July 2021
Sec. Marine Biotechnology and Bioproducts
Volume 8 - 2021 | https://doi.org/10.3389/fmars.2021.690749
This article is part of the Research TopicMarine Biotechnology, Revealing an Ocean of OpportunitiesView all 26 articles
The worldwide microplastics pollution is a serious environmental and health problem that is currently not effectively mitigated. In this work we tested jellyfish mucus as a new bioflocculent material capable of sequestration of polystyrene microplastics in aqueous environments. Mucus material was collected from different jellyfish species and was used to trap fluorescently tagged polystyrene microspheres. The efficiency of removal was tested using varying concentrations of microplastics and mucus. The interaction between the microplastics and mucus was determined by viscosity measurements and confocal laser scanning microscopy. Different mucus preparation methods were also tested: freshly prepared, mechanically sheared, freeze-thawed, freeze-dried, and hydrolyzed mucus. The results demonstrate that jellyfish mucus can efficiently sequester polystyrene microplastics particles from the suspension. The fraction of the removed microplastics was highest with freshly prepared mucus and decreased with freeze-thawing and freeze-drying. The mucus ability to sequester microplastics was completely lost in the hydrolyzed mucus. The results imply that the intact jellyfish mucus has the potential to be used as a biopolymer capable of removing microplastics material.
Microplastics are defined as plastic particles ≤5 mm in size (Hartmann et al., 2019). They have predominantly been recognized as marine contaminants with estimated 93 to 236 thousand metric tons floating on the global sea surface (Thompson et al., 2004; Desforges et al., 2014; Ivar do Sul and Costa, 2014; van Sebille et al., 2015). Microplastics also enter freshwater and terrestrial environments, thus endangering the health of these environments as well (Rillig, 2012; Morritt et al., 2014; Faure et al., 2015; Liu M. et al., 2018). They can be found in human settlements, rivers, mountain soils, in the sediments of deepest ocean and even in Arctic snow (Dris et al., 2016; Cai et al., 2017; Wright et al., 2020).
Microplastics have been found in almost every marine habitat and their ingestion has effects on marine and freshwater organisms, either as a consequence of their physical penetration, leaching of chemical additives or infection caused by microbial communities on microplastics (Prinz and Korez, 2020). Moreover, microplastics can be transferred to higher trophic levels in the food web (Lusher, 2015), thus sustaining and multiplying their detrimental effects. The management of microplastics pollution problem, however, is not a solved issue and new mitigation strategies are still emerging (Figure 1).
Figure 1. Currently used microplastics mitigation strategies. The recycling strategies are dependent on the efficient removal and recovery of microplastics, which is indicated with a dashed arrow. Our research proposes to invest into development of novel removal strategies, as for example by using jellyfish mucus (colored in yellow).
At the moment, the mitigation of microplastics pollution is aimed at reducing the use of plastics, reduction of microplastics quantities using microbial consortia, replacement of the conventional plastics with bioplastics, and physico-chemical removal strategies (Oliveira et al., 2020; Wong et al., 2020). Recycling of plastics and microplastics is still not economically feasible in comparison with the low production costs of virgin plastics and low recyclability of microplastics (Prata et al., 2019). Moreover, reduction, replacement and recycling strategies (Figure 1) are all connected with the need for technology development, proper legislative policy, public awareness, consumer waste handling, and incentivized recovery systems (Eriksen et al., 2018).
Wastewater treatment plants have been identified as critical sources of microplastics in the ocean, contributing globally more than 100 billion microplastic particles–MPs (mostly fibers) in the ocean per year (Freeman et al., 2020). At the same time, wastewater treatment plants provide a major sink for microplastics which are retained in the sludge. The microplastics removal rate depends on wastewater treatment technology and varies between 70–99% (Freeman et al., 2020). The current technologies are mostly capable of removing particles larger than 100 μm (Okoffo et al., 2019; Poerio et al., 2019). There are few technologies capable of effectively removing microplastics from the aqueous environments such as membrane biological reactors, rapid sand filters, dissolved air flotation, and micro-screen filtration with disc filters. Other innovative applications are being studied that show potential but also some limitations: dynamic membranes, enhancement in flocculation/coagulation, electrocoagulation, and photocatalytic processes (Talvitie et al., 2017; Hildebrandt et al., 2019; Freeman et al., 2020). The effectiveness of microplastics removal is dependent on microplastics physical properties, in particular size, shape, and the overall particle abundance (Wang et al., 2019; Wong et al., 2020). For example, microparticles can block the microfiltration membranes (pore size >100 nm) or the very costly reverse osmosis membranes (pore size >5 nm). The particular challenge in removing the microplastics is not only their small size but also their inert physicochemical properties against most of the physical and chemical additives for flocculation (Schuhen et al., 2018).
The use of jellyfish mucus is dependent on sustainable availability of the biomaterial and its suitable physical sequestration properties. The life cycles of jellyfish are conducive to bloom events in which huge populations occasionally build up (Goy et al., 1989). There are several reasons why the frequency of jellyfish blooms increased in the last decades such as eutrophication, global overfishing, increased space for polyps, transport of non-indigenous species, and widening of the natural area of non-indigenous species (Boero, 2013; Needleman et al., 2018). Increased jellyfish blooms and consequently increased mucus production can provide an opportunity for their biotechnological exploitation. In the western world, jellyfish are often considered as nuisance especially as undesired by-catch that is categorized as waste biomass. Many recent studies showed that jellyfish biomass can be used as fodder for animals, a source of food for humans, biofuel, biofertilizer, or even as cement additive (Hossain et al., 2013; D’Amico et al., 2016; Bleve et al., 2019; Emadodin et al., 2020). Several bioactive proteins have been isolated from jellyfish with hemolytic, cytotoxic, cardiovascular, and neurotoxic activity (Domenico et al., 2019; Merquiol et al., 2019). In addition, jellyfish have been used to develop new research tools such as Green fluorescence protein (GFP), biocompatible collagen for 2D and 3D cell cultures, or as model systems to study mobility and microgravity (Spangenberg et al., 1994; Purcell, 2009; Najem et al., 2012; Pugliano et al., 2017; Paradiso et al., 2019; Coppola et al., 2020; Mearns-Spragg et al., 2020). Due to their ability to internalize plastic debris, jellyfish have been suggested as a potential invertebrate bioindicators for plastic pollution in pelagic waters (Macali et al., 2018; Iliff et al., 2020; Macali and Bergami, 2020).
Jellyfish are known to produce copious amounts of mucus (Ames et al., 2020). The epidermis and gastrodermis of jellyfish contain mucus producing gland cells, which produce thin renewing mucus layers over the external surface of medusa (Heeger and Möller, 1987). Under stress, during reproduction, digestion, and when dying, the amount of released mucus is increased (Patwa et al., 2015) and can reach up to 400 mL/kg of jellyfish per hour (Liu W. et al., 2018). Mucus has been found to have a role in feeding, surface cleaning, and defense against predators. Shanks and Graham (1988) characterized mucus secretion as an important chemical defense mechanism, since it contains toxins and nematocysts. For instance, Cassiopea jellyfish mucus is known to kill certain species of fish as mobile grenades (Ames et al., 2020).
Jellyfish mucus is a hydrogel composed mainly of water (95%), mucins (3%) and other molecules like lipids and nucleic acids (2%) (Bakshani et al., 2018). Mucins are large glycoproteins with molecular weight (MW) between 200 kDa and 200 MDa (Kesimer and Sheehan, 2012). They have O-linked oligosaccharides and are responsible for viscoelastic nature of mucus. Mucins are important for protection of jellyfish epithelium against dehydration, microbial invasion, and physical damage (Pearson et al., 2011; Bakshani et al., 2018). Mucins are produced in secretory cells in the form of compact concentrated granules. This is possible due to high Ca2+ concentrations that screen negative mucin charges. During mucin secretion Ca2+ is replaced with Na+ which is less effective in charge screening, thus allowing the expansion of mucins in seawater. During the expansion mucins adsorb water and their volume may increase 1000–3000-fold which allows the formation of a gel structure (Bakshani et al., 2018), and could be used as an efficient trap for microplastics particles (Patwa et al., 2015).
In this work we tested the potential of jellyfish mucus as a low-cost raw biomaterial to effectively sequester microplastics. The sequestration of microplastics from aqueous environments (e.g., open waters) is technologically not feasible. The accounted floating plastic and microplastic fragments are disproportionately lower than expected from their inputs as microplastics, probably due to ingestion by marine organisms or sinking into the ocean (Corona et al., 2020). Moreover, any removal strategies would be invasive, potentially impacting the marine organisms, and sediments. Finally, removal strategies in open waters would not be cost-effective (Lusher, 2015). Hence, the application of mucus or other removal strategies should be used before the entry of microplastics in the ocean. A lot of methods under development (physical, chemical or biological ones) involve removal strategies in the wastewater treatment plants (Sun et al., 2019; Freeman et al., 2020; Padervand et al., 2020). The aim of the study was to test the ability of differently processed jellyfish mucus to remove polystyrene microplastics material. The mucus was isolated from Cotylorhiza tuberculata, Aurelia aurita, Mnemiopsis leidyi, and Rhizostoma pulmo jellyfish. We have used mucus to sequester fluorescently tagged polystyrene microspheres that were used as model microparticles for our experiments. The efficiency of removal was tested at several polystyrene microparticles and mucus concentrations. The interaction between the microplastics and mucus was determined with viscosity measurements and confocal laser scanning microscopy. Different mucus preparation methods have been tested: freshly prepared, mechanically sheared, freeze-thawed, freeze-dried, and hydrolyzed mucus.
The mucus was collected from three Cnidaria (Class Scyphozoa) C. tuberculata, A. aurita, and R. pulmo jellyfish and the invasive Ctenophore M. leidyi. The medusae were caught with a dip net from the surface in March, April, August, and October 2019 in the Bay of Piran (Northern Adriatic Sea, Slovenia). They were collected in 100 L barrels filled with seawater. The mucus was collected from the individual medusae by two different approaches: through funnel and with a syringe. The mucus from M. leidyi, A. aurita, and C. tuberculata was collected with a 10 mL syringe after gently shaking the individual medusae. Mucus from R. pulmo was collected by transferring individual medusae from the barrel to the large funnel. This species was used for the experiments comparing different mucus storage conditions. The released mucus was accumulated for 5 min. The medusae were further gently agitated (for 5–10 min) to increase the amount of excreted mucus. The translucent mucus was collected in a glass beaker. It was pulled together and was either used fresh, freeze-thawed or freeze-dried prior to the experiments. Fresh mucus was used within 1 h of collection and 10 mL of mucus was used for conducting the experiments. To prepare freeze-dried and freeze-thawed mucus, approximately 300 mL of jellyfish mucus was stored. The mucus was first gently shaken to obtain a more homogenous sample and transferred to acid pre-cleaned combusted (500°C, 4 h) Duran bottles. Next, samples were either freeze-dried for 1 week (due to their high water content) or stored in the freezer at −30°C for 1 week. Prior to conducting the experiments, 30 mg of freeze-dried mucus was used and rehydrated in 1 mL of distilled water to get the same hydration as the original (fresh) mucus (Stabili et al., 2015) by keeping the salt concentration in freeze-dried mucus unchanged. To obtain freeze-thawed samples, the frozen mucus in Duran flasks were placed in a container filled with water at room temperature that was replaced several times over few hours until the mucus was completely thawed. 10 mL of freeze-thawed mucus was used for conducting the experiments.
Polystyrene Divinylbenzene (PS microspheres), dyed with FirefliTM Fluorescent Green with the average particle size of 48 μm, particle density of 1.05 g/cm3, and refractive index 1.59 were purchased from Duke Scientific Corporation. A stock suspension of polystyrene particles (c = 5 mg/mL seawater) was prepared. The appropriate volume of stock suspension (3.13, 6.25, 12.5, 25, 50, and 100 μL) was added to 1 mL of fresh, freeze-dried or freeze-thawed mucus (as prepared in the previous section) to obtain the final concentrations (250, 500, 1000, 2000, 4000, and 8000 microspheres per mL). The samples were incubated for 60 min at room temperature with occasional stirring. The results were calculated based on the average of four replicates of measurements for each sample.
To assess the effectiveness of the mucus to sequester PS microspheres, the gel material (mucus with entrapped PS microspheres) was separated from the surrounding water with spatula and transferred into a new microcentrifuge tube, where it was resuspended in an equal volume of distilled water with vigorous mixing. The 200 μL of resuspended gel samples or the surrounding water were put into microtiter plates and fluorescence was determined with microplate fluorescence reader (Cytation 5, Biotek). In addition, the residual fluorescence, that is the fluorescence of PS microspheres not incorporated in the mucus, was measured. The fluorescence of PS microspheres was excited at 368 nm and detected at 530 nm. The background fluorescence was subtracted from the measured values.
Additionally, to compare the PS sequestration capabilities between different species, we used mucus collected from R. pulmo, M. leidyi, A. aurita, and C. tuberculata. Fresh or freeze-thawed mucus was used, as prepared in the previous section. 1.6 × 106 PS microspheres final concentration was added to 20 mL of 0.45 μm filtered seawater. 10 mL of mucus was then added to PS microspheres suspension in triplicates and mixed by hand. For control, 30 mL solution of 1.6 × 106/mL PS microspheres was used. Vials were incubated at 4°C for 2 h to reduce physical disintegration. One mL of surrounding water was sampled in triplicates after gently shaking the vial and transferred in 2 mL tube (Eppendorf). Fluorescence intensity in residual water was measured on a multiplate reader (SPARK, Tecan) at excitation wavelength 468 nm and emission wavelength 508 nm. The final PS microspheres concentration in the surrounding water (residual fluorescence, see Figure 2) was calculated from the linear regression calibration curve (PS suspension concentration was in the range from 0 to 1.6 × 106/mL, R2 = 0.96).
Figure 2. Residual (water) PS concentration in control (no mucus) and in samples of mucus from four jellyfish species with fresh or thawed mucus. The used PS microspheres concentration was 1.6 × 106/mL. AA – A. aurita; CT – C. tuberculata; MN – M. leidyi; RP – R. pulmo. Each treatment had two biological and three technical replicates.
Finally, we tested the potential leakage of the fluorescent dye from the PS microspheres using blank control (seawater-resuspended PS microspheres from the same batch). Fluorescent dyes are used regularly in microplastics analyses as they absorb onto plastic surfaces, making them fluorescent and easier to detect (Maes et al., 2017). This way, they are suitable for a wide range of applications in medicine, biochemistry, colloid chemistry, and environmental studies (Schür et al., 2019). The blank control was prepared by resuspending 8000 microspheres per mL in seawater as described at the beginning of this section. PS microspheres were observed using confocal laser scanning microscopy (CLSM, see the section Microscopy for more details) at the beginning and after 60 min of incubation. The fluorescence of a minimum of 10 microspheres and the fluorescence of the background, with and without (i.e., after filtration using filters with pore diameter d = 0.20 μm) PS microspheres, were assessed.
A total of 500 μL of 2 M HCl was added to 30 mg of freeze-dried R. pulmo mucus in microcentrifuge tubes. The samples were heated for 60 min at 100°C. After hydrolysis the samples were neutralized with 2 M NaOH. During the hydrolysis the mucus material was occasionally sampled to check the viscosity of the suspension. When the viscosity of the sample was equal to water viscosity, the mucus was considered to be completely hydrolyzed. PS microspheres were added to the hydrolyzed mucus in a concentration of 8000 microspheres/mL. The samples were incubated for 60 min at room temperature. Next, the viscosity of the suspension was measured and compared to the control unhydrolyzed mucus samples.
Different homogenization procedures were tested using fresh mucus to assess the homogenization effects on PS microspheres capture efficiency. The R. pulmo mucus was either untreated (T1), vortexed for 20 s (T2), homogenized with ultrasound homogenizer for 10 s (T3), or homogenized with ultrasound homogenizer for 20 s (T4). Freshly released mucus was collected and distributed into four 50 mL falcon tubes (30 mL in each). After each treatment, 10 mL of mucus was transferred into 40 mL glass vials in triplicates to which 20 mL of 0.45 μm (MCE syringe filter, Millipore) filtered seawater and 1 mL of 3.2 × 107/mL PS microspheres stock suspension were added. In a control sample with no mucus added (C), 1 mL of PS microspheres stock suspension was added to 30 mL of filtered seawater. The vials were shaken manually and incubated at 4°C for 2 h. The surrounding water was subsampled after gentle shaking for fluorescence measurements on a multiplate reader (SPARK, Tecan) at excitation wavelength 468 nm and emission wavelength 508 nm. The difference between treatments and the control were assessed with analysis of variance with post hoc Dunnett tests. Data were analyzed and plotted in R using ggplot2 (Wickham, 2016) and multcomp packages (Hothorn et al., 2008).
For viscosity measurements mucus samples were transferred to the rotational rheometer Physica MCR 302 (Anton Paar, Graz, Austria). All the samples were measured at 25.00°C (± 0.01°C). The samples were placed in the center of the lower rheometer plate using a pipette tip. The PP25 measuring system was used for measurements with approximately 0.07 mL of mucus sample. The gap between the upper and lower measuring plate was reduced to the trim position. The trim gap position was 10 μm higher than the measuring gap position. We have ensured that the gap was completely filled with the sample. A trimming tool was used to remove any excess material along the brim of the plate, so that the sample corresponded to the external diameter of the upper plate. Next, the final measuring gap of 100 μm was approached. During the compression phase of the sample the normal force was constantly monitored on the rheometer and did not exceed 2N. Viscosity was measured at shear rates ranging from 1 to 1000 s–1 in 25 logarithmically spaced steps. All the samples were independently repeated for at least six times to obtain the average values and standard deviations.
For observation of isolated mucus we used LSM 800 Confocal laser scanning microscope based on the inverted Axio Observer Z1 microscope (Zeiss, Göttingen, Germany) operating in wide-field mode. Samples were observed with 20×/NA 0.4 or 63×/NA 0.75 objective with DIC technique. The microscope was operated by ZEN 2.3 (Carl Zeiss Microscopy GmbH) software and images were acquired by AxioCam MRm3 (Zeiss). The samples were prepared by placing 10 μL of mucus on microscopic slides covered by 1.5 # coverslips. To study the interactions between PS microspheres and the mucus the 10 μL mixture of PS microspheres and mucus was stained by 1 μL propidium iodide (final c = 0.1 mg/mL). In confocal laser scanning mode, the optical slicing was performed in 2 μm steps by using Plan-Apochromat 100×/NA 1.40 Oil objective. The image surface area was (85 × 85) μm with the resolution of 1083 × 1083 pixels. Propidium iodide fluorescence was excited at 561 nm diode laser, emission range was between 595 and 700 nm. The fluorescence of PS microspheres was excited at 488 nm diode laser, the emission range was between 600 and 700 nm. The master gain of GaAsP Pmt detectors was set to 650 and 750 V for 488 and 561 nm channel, respectively. The pinhole size was set to 1 AU. The frame time was 7 s and about 30 images in the z direction were taken per sample. The images were visualized, and pseudo colored by ImageJ software (Rueden et al., 2017).
The fresh mucus collected from C. tuberculata, A. aurita, M. leidyi, and R. pulmo jellyfish had the ability to remove PS microspheres from the suspension (Figure 2). The data represent residual fluorescence that remained in the surrounding water when mucus was removed. Although fresh A. aurita and M. leidyi mucus had the lowest residual water PS microspheres concentration, indicating the highest PS microspheres removal rates, their mucus was more difficult to obtain and was less stable. As a rule, thawed mucus had a lower microplastics sequestration capability, demonstrated by the increase in residual water PS microspheres concentration compared to fresh samples. The differences between fresh and thawed mucus were not statistically significant for R. pulmo. Since the mucus of R. pulmo was easiest to obtain in large quantities we have used it for further analyses.
The mucus material collected from R. pulmo jellyfish is shown in Figure 3. It contained large oval structures (nematocysts) embedded in a viscous matrix. The nematocysts represent a large fraction of the mucus material and indicate that jellyfish were under stress during the mucus isolation.
Figure 3. The mucus isolated from Rhizostoma pulmo under light microscope (A) and large oval structures called nematocysts (B).
The ability of R. pulmo mucus to sequester fluorescently labeled PS microspheres is shown in Figure 4. The collected mucus had a translucent coloration. After the addition of PS microspheres, mucus became denser and intensely yellow-green colored, suggesting an incorporation of PS microspheres into the mucus structure. To quantify the incorporation of PS microspheres, the fluorescence intensity of the mucus before and after the addition of different concentrations of PS microspheres as well as the residual fluorescence were determined. When 8000 PS microspheres per mL were added to the mucus suspension, approximately 50% were sequestered in the mucus. The fraction of the sequestered PS microspheres did not change significantly with the increasing concentration of microspheres. The validity of the results was confirmed by testing the potential leakage of the fluorescent dyes in our blank control. On average, the fluorescence of the microspheres decreased by only 1.1% after 60 min of suspension in seawater. This decrease could be attributed to bleaching during the light exposure. Importantly, there was no increase of background fluorescence observed that could be attributed to a potential dye leakage, thus validating our experimental approach. This is in line with other similar leakage control experiments, where a negligible dye leakage was observed even after 48 h (Murano et al., 2021).
Figure 4. Fluorescence intensity of the control microspheres suspension (red). The green line shows microspheres sequestered in mucus (30 mg/mL), and the blue line shows residual fluorescence due to microspheres remaining in the surrounding water. The measurements are denoted with dots, while a linear regression fit is represented with a line. In the inset the mucus prior (left) and after the addition of PS microspheres aggregates (right) is shown. The number of replicates for control treatments was three, while for mucus samples between five and seven replicates were used for each treatment, as indicated by colored dots.
The interactions between the mucus and PS microspheres increased the mucus viscosity as provided in Figure 5. The suspension of only PS microspheres had low viscosity that was comparable to water (Figure 5B, dashed lines). The mucus, on the other hand, behaves as a pseudoplastic non-Newtonian fluid where the viscosity of the mucus decreased with the increasing shear rate (Figure 5A, dashed lines). When PS microspheres in the concentration of 8000 particles/mL, representing 0.05% (v/v), were added to the increasing concentrations of mucus the viscosity and pseudoplasticy of the samples increased (Figure 5A). This suggests that the addition of PS microspheres induced structural changes in the mucus important for microspheres sequestration. On the other hand, when the concentration of the mucus was kept constant and the number of PS microspheres was increased, the viscosity response saturated at higher PS to mucus ratios (Figure 5B) as is to be expected due to a finite capacity of mucus to trap microspheres.
Figure 5. Viscosity curves for the mucus – PS microspheres suspensions. (A) The concentration of PS microspheres was kept constant at 8000 particles/mL, the mucus concentration was increased from 1 to 6% (w/v). Two control mucus samples without PS microspheres are shown (c3, c6 with 3 and 6% mucus, respectively). (B) The concentration of mucus was kept constant at 3% whereas the concentration of PS microspheres was increased from 2000 to 9600 particles/mL. Two control samples without added mucus are shown (c8000, c9600 with 8000 and 9600 particles/mL, respectively). The error bars represent standard deviations (n = 5–6 for PS samples and 2–3 for control samples).
To check the microstructure of the microplastics - mucus aggregates we used fluorescence microscopy (Figure 6). The PS microspheres that were embedded in the mucus had a uniform size and fluorescence intensity and were non-homogenously distributed in the mucus network structure. The mucus stained with fluorescent propidium iodide formed a 3D network with flocks of different sizes. Most of the PS microspheres that were trapped in the mucus had point contacts with the mucus. The surface of the PS microspheres was relatively sparsely covered with the mucus. Some of the PS microspheres appeared to interact with each other via depletion interactions.
Figure 6. Fluorescence images of PS microspheres in contact with the mucus observed by 20x objective (A) and 100 x objective (B); PS microspheres (green), mucus stained with propidium iodide (red).
To better characterize the interactions of the individual PS microspheres with mucus network we performed optical slicing of the individual spheres using confocal microscopy (Figure 7). Most of the mucus was either above or below the plane of the PS microsphere and had only limited point contacts with the mucus at different focal planes. This suggests that PS microspheres were trapped in the physical gel polymer structure and that direct chemical interactions were not the main driving forces of microsphere sequestration.
Figure 7. Confocal laser scanning microscopy (CLSM) image of PS microsphere in contact with mucus at different focal plains from bottom to the top (A–F) of the microsphere. The mucus was stained with propidium iodide (red), the PS microsphere is dyed with Firefli™ Fluorescent Green.
To check if polymer higher structures are important for the ability of mucus to trap PS microspheres, we hydrolyzed mucus in 2 M HCl at 100°C. The viscosity of the hydrolyzed sample decreased 21-fold and was comparable to the viscosity of water or water suspension of PS microspheres (Figure 8). The hydrolyzed mucus was not able to sequester the PS microspheres.
Figure 8. Viscosity of mucus, PS microspheres suspension, hydrolyzed mucus and hydrolyzed mucus with PS microspheres is presented with the box and whiskers plots. Three replicates were used for measuring the PS microspheres suspension (control), while n = 5 was used for the rest of the treatments.
In addition to chemical hydrolysis, the mucus structure can also physically disintegrate upon shear stress, which would decrease PS microspheres sequestration. To test this, we mechanically treated mucus with vortex mixing or ultrasonic homogenization (Figure 9). Compared to untreated fresh mucus samples (T1), the mucus removal of PS microspheres decreased for approximately 50 to 80% for vortex treated and long ultrasound homogenized samples, respectively. No significant difference was observed between the short ultrasound homogenization (T3) and untreated PS-mucus samples (T1).
Figure 9. Residual fluorescence remained in the suspension after mucus samples were shear stressed. Control – PS microspheres added to seawater; SW – seawater without PS microspheres added; T1 – untreated mucus with PS microspheres; T2 – vortex treated for 20 s; T3 – ultrasound homogenized for 10 s; T4 – ultrasound homogenized for 20 s. n = 3 for all treatments. Stars denote a significant difference between treatments and control; * (p < 0.05); ** (p < 0.01); *** (p < 0.001).
For long term storage of mucus freezing could be used. The effects of freeze-thawed and freeze-dried samples on the mucus viscosity and the ability to sequester the PS microspheres are given in Figure 10A. The freshly prepared mucus was the most viscous and pseudoplastic. The viscosity of the freeze-thawed and freeze-dried mucus were significantly lower compared to the fresh mucus. The abilities of different mucus to remove PS microspheres from the water are given in Figure 10B. The freshly prepared mucus sample had the lowest residual fluorescence, indicating the highest removal rate of the PS microspheres. The residual fluorescence was highest with freeze-dried samples which suggests the lowest PS microspheres removal capacity. The ability of the mucus to remove PS microspheres correlated with mucus viscosity and pseudoplasticity. The higher the viscosity and pseudoplasticity of the mucus, the more PS microspheres were removed and the lower the residual fluorescence was observed. This corroborates the mechanical shear and hydrolysis experiments and implies that structure of the mucus is important for PS microspheres entrapment.
Figure 10. The effect of R. pulmo mucus processing on viscosity and ability to sequester PS microspheres. The viscosity curves (A) and the residual fluorescence intensity in the water after addition of mucus (B), n = 4. The concentration of mucus was 3% (w/v). The samples were prepared either freshly, after freeze/thaw, and freeze/dry preprocessing.
In this work we have tested the ability of the mucus material collected from C. tuberculata, A. aurita, M. leidyi, and R. pulmo jellyfish to remove PS microspheres. All tested jellyfish mucus had the capacity to remove PS microspheres. Previously it has been shown that jellyfish mucus could represent a biological matrix for entrapment of dispersed oil droplets (Gemmell et al., 2016). Similarly, Patwa et al., 2015 described gold and quantum dots nanoparticles bio-accumulation in the mucus of A. aurita, P. noctiluca, and M. leidyi. The results of this study report that jellyfish mucus has the ability to sequester PS microplastics. The viscous mucus structure physically entraps microplastics particles from the suspension, which increases the mucus structure. This may be a useful property in applications as a more compact mucus is easier to collect from the liquid. This has been demonstrated independently with three different approaches. The fluorescence of the mucus increased after the addition of fluorescently labeled PS microspheres. Second, the mucus viscosity increased monotonically with the increasing concentration of PS microspheres. Third, the entrapment of PS microspheres in the mucus physical gel network structure was demonstrated with confocal microscopy.
Mucus is a hydrophilic polyelectrolyte material (Verdugo, 2012). On the other hand, PS microspheres are capable of ionic interactions, secondary ion-exchange and hydrophobic interactions (Kazarian et al., 2013). The LogP of PS microspheres is 0.48, suggesting low to medium hydrophobicity of this material. According to the manufacturer information, the inclusion of a fluorescent dye in the PS microspheres should not change the surface properties significantly since PS microspheres are internally dyed to prevent leaching and provide a dye-free surface for coupling (Abraham et al., 1997). The PS microspheres could therefore interact electrostatically with the mucus, however, the contact area between the PS microspheres and mucus was very small and physical entrapment is more likely.
The physical entrapment of PS microspheres in mucus significantly increased its structure (viscosity), suggesting that interactions between mucus and PS microspheres enhanced the gel network structure. The effect of new molecular contacts between mucus and PS microspheres could be significant if the initial connectivity of the system is low. Compared to other polymers which exhibit gel properties, the jellyfish mucus viscosity was relatively low (i.e., 65 mPas at 3% (w/v) at a shear rate of 10 s–1). For example, the viscosity of 2% (w/v) methylcellulose at shear rate of 10 s–1 is 300 mPas (Cerar et al., 2017) whereas 0.25% (w/v) gellan has a viscosity of 100 mPas at 10 s–1 (Haoping et al., 2014). The viscosity of mucus was approximately 30-fold lower than the viscosity of the DNA at the same concentration (Stojković et al., 2015). Given the low initial viscosity of the jellyfish mucus, the addition of PS microspheres and the formation of new contacts with mucus could significantly increase the viscosity of the aggregate as was observed. It is interesting to note that pseudoplasticity of mucus correlated with mucus sequestration ability. The highest PS microspheres sequestration was observed in freshly prepared mucus which had the highest pseudoplasticity. Consistently, a mechanical disintegration by vortex mixing or ultrasonic homogenization decreased the PS microspheres sequestration. The importance of the mucus polymeric network structure for PS microspheres sequestration was most clearly demonstrated with the experiment where we have hydrolyzed the mucus. In the absence of the mucus polymer structure no PS microspheres sequestration was possible.
To use jellyfish mucus for microplastics removal there are several hurdles to overcome. (i) The mucus availability is strongly dependent on seasonal and regional blooms of the jellyfish, which increases the production costs due to the need for rapid and efficient biomaterial processing and storage. (ii) The stability of fresh mucus material is critical and would need to be increased to allow for the long-term storage of mucus. (iii) The effects of microplastics size and shape as well as its composition on sequestration process need to be determined to establish the optimal concentrations and sequestration protocols. (iv) Finally, an in-depth life cycle assessment is crucial to assess the feasibility of using mucus for microplastics sequestration, taking into consideration the sustainable harvesting of jellyfish. In this work we have focused on the removal potential of this material. Should this or similar removal strategies be implemented into microplastics removal processes, the duration and stability of sequestration should be tested as well, to establish the storage conditions of this sequestered material before its potential recycling. As mucus is biodegradable (Bakshani et al., 2018), a potential next step in the processing of removed microplastics is the establishment of biodegradation conditions or the use of degradation enzymes to speed the release of sequestered particles for proper disposal or recycling.
The raw data supporting the conclusions of this article will be made available by the authors, without undue reservation.
ŽL, KK, AR, and DS designed the experiments. ŽL and KK collected the mucus and participated in mucus sequestration, hydrolysis, and homogenization experiments. ŽL, ID, and DS participated in fluorescence and viscosity measurements and microscopy trials. AR analyzed the data. ŽL and DS drafted the manuscript. All authors jointly participated in results interpretation, edited, and approved the manuscript before submission.
The publication is part of a project that has received funding from the European Union Horizon 2020 Research and Innovation Programme under grant agreement no. 774499 – GoJelly project. We would like to acknowledge the financial support from the Slovenian Research Agency (research core funding P4-0116, P1-0237, and P1-0245). The microscopy was supported by University infrastructural center “Microscopy of biological samples” located in Biotechnical faculty, University of Ljubljana. This publication is based upon work from COST Action CA18238 (Ocean4Biotech), supported by COST (European Cooperation in Science and Technology).
The authors declare that the research was conducted in the absence of any commercial or financial relationships that could be construed as a potential conflict of interest.
Abraham, M. H., Chadha, H. S., Leitao, R. A. E., Mitchell, R. C., Lampert, W. J., Kaliszan, R., et al. (1997). Determintion of solute lipophilicity, as log P(octanol) and log P(alcane) using poly(styrene-divinlybenzene) and immobilized artificial membrane stationary phase in reversed-phase high-performance liquid chromatography. J. Chromatogr. A 766, 35–47. doi: 10.1016/s0021-9673(96)00977-6
Ames, C. L., Klompen, A. M. L., Badhiwala, K., Muffett, K., Reft, A. J., Kumar, M., et al. (2020). Cassiosomes are stinging-cell structures in the mucus of the upside-down jellyfish Cassiopea xamachana. Commun. Biol. 3:67. doi: 10.1038/s42003-020-0777-8
Bakshani, C. R., Morales-Garcia, A. L., Althaus, M., Wilcox, M. D., Pearson, J. P., Bythell, J. C., et al. (2018). Evolutionary conservation of the antimicrobial function of mucus: a first defence against infection. NPJ Biofilms Microbiomes 4:14. doi: 10.1038/s41522-018-0057-2
Bleve, G., Ramires, F. A., Gallo, A., and Leone, A. (2019). Identification of safety and quality parameters for preparation of jellyfish based novel food products. Foods. 8:263. doi: 10.3390/foods8070263
Boero, F. (2013). Review of jellyfish blooms in the Mediterranean and Black Sea. GFCM Stud. Rev. 92, 1–53. doi: 10.1007/978-94-007-5316-7_1
Cai, L., Wang, J., Peng, J., Tan, Z., Zhan, Z., Tan, X., et al. (2017). Characteristic of microplastics in the atmospheric fallout from Dongguan city, China: preliminary research and first evidence. Environ. Sci. Pollut. Res. 24, 24928–24935. doi: 10.1007/s11356-017-0116-x
Cerar, J., Dogsa, I., Jamnik, A., and Tomšič, M. (2017). Physicochemical data on aqueous polymeric systems of methyl cellulose and lambda- and kappa-carrageenan: SAXS, rheological, densitometry, and sound velocity measurements. Data in Brief 15, 427–438. doi: 10.1016/j.dib.2017.09.025
Coppola, D., Oliviero, M., Vitale, G. A., Lauritano, C., D’Ambra, I., Iannace, S., et al. (2020). Marine collagen from alternative and sustainable sources: extraction, processing and applications. Mar. Drugs 18:214. doi: 10.3390/md18040214
Corona, E., Martin, C., Marasco, R., and Duarte, C. M. (2020). Passive and active removal of marine microplastics by a mushroom coral (Danafungia scruposa). Front. Mar. Sci. 7:128. doi: 10.3389/fmars.2020.00128
D’Amico, P., Leone, A., Giusti, A., and Armani, A. (2016). “Jellyfish and humans: not just negative interactions,” in Jellyfish: Ecology, Distribution Patterns and Human Interactions, ed. G. L. Mariottini (Hauppauge, NY: Nova Science Publisher), 331–351.
Desforges, J. P., Galbraith, M., Dangerfield, N., and Ross, P. S. (2014). Widespread distribution of microplastics in subsurface seawater in the NE Pacific Ocean. Mar. Pollut. Bull. 79, 94–99. doi: 10.1016/j.marpolbul.2013.12.035
Domenico, S., Rinaldis, G., Paulmery, M., Piraino, S., and Leone, A. (2019). Barrel jellyfish (Rhizostoma pulmo) as source of antioxidant peptides. Mar. Drugs 17:134. doi: 10.3390/md17020134
Dris, R., Gasperi, J., Saad, M., Mirande, C., and Tassin, B. (2016). Synthetic fibers in atmospheric fallout: a source of microplastics in the environment? Mar. Pollut. Bull. 104, 290–293. doi: 10.1016/j.marpolbul.2016.01.006
Emadodin, I., Reinsch, T., Rotter, A., Orlando-Bonaca, M., Taube, F., and Javidpour, J. (2020). A perspective on the potential of using marine organic fertilizers for the sustainable management of coastal ecosystem services. J. Environ. Sustain. 3, 105–115. doi: 10.1007/s42398-020-00097-y
Eriksen, M., Thiel, M., Prindiville, M., and Kiessling, T. (2018). “Microplastic: what are the solutions?,” in Freshwater Microplastics: The Handbook of Environmental Chemistry, Vol. 58, eds M. Wagner and S. Lambert (Chambridge: Springer), 273–298. doi: 10.1007/978-3-319-61615-5_13
Faure, F., Saini, C., and Potter, G. (2015). An evaluation of surface micro- and mesoplastic pollution in pelagic ecosystems of the Western Mediterranean Sea. Environ. Sci. Pollut. Res. 22, 12190–12197. doi: 10.1007/s11356-015-4453-3
Freeman, S., Booth, M. A., Sabbah, I., Tiller, R., Dierking, J., Klun, K., et al. (2020). Between source and sea: the role of wastewater treatment in reducing marine microplastics. J. Environ. Manage. 266:110642. doi: 10.1016/j.jenvman.2020.110642
Gemmell, B. J., Bacosa, H. P., Liu, Z., and Buskeya, E. J. (2016). Can gelatinous zooplankton influence the fate of crude oil in marine environments? Mar. Pollut. Bull. 113, 483–487. doi: 10.1016/j.marpolbul.2016.08.065
Goy, J., Morand, P., and Etienne, M. (1989). Long-term fluctuations of Pelagia noctiluca (Cnidaria, Scyphomedusa) in the western Mediterranean Sea. Prediction by climatic variables. Deep-Sea Res. 36, 269–279. doi: 10.1016/0198-0149(89)90138-6
Haoping, X., Min, S., Ying, L., Jinling, J., and Tao, M. (2014). A novel in situ gel formulation of ranitidine for oral sustained delivery. Biomol. Ther. 22, 161–165. doi: 10.4062/biomolther.2013.109
Hartmann, N., Hüffer, T., Thompson, R., Hassellöv, M., Verschoor, A., Daugaard, A., et al. (2019). Are we speaking the same language? Recommendations for a definition and categorization framework for plastic debris. Environ. Sci. Tech. 53, 1039–1047. doi: 10.1021/acs.est.8b05297
Heeger, T., and Möller, H. (1987). Ultrastructural observations on prey capture and digestion in the scyphomedusa Aurelia aurita. Mar. Biol. 96, 391–400. doi: 10.1007/bf00412523
Hildebrandt, L., Voigt, N., Zimmermann, T., Reese, A., and Proefrock, D. (2019). Evaluation of continuous flow centrifugation as an alternative technique to sample microplastic from water bodies. Mar. Pollut. Bull. 62, 1596–1605.
Hossain, S. T., Sugimoto, H., Asagi, N., Araki, T., Ueno, H., Morokuma, M., et al. (2013). The use of desalinated-dried jellyfish and rice bran for controlling weeds and rice yield. J. Org. Syst. 8, 28–37.
Hothorn, T., Bretz, F., and Westfall, P. (2008). Simultaneous inference in general parametric models. Biom. J. 50, 346–363. doi: 10.1002/bimj.200810425
Iliff, S. M., Wilczek, E. R., Harris, R. J., Bouldin, R., and Stoner, E. W. (2020). Evidence of microplastics from benthic jellyfish (Cassiopea xamachana) in Florida estuaries. Mar. Pollut. Bull. 159:111521. doi: 10.1016/j.marpolbul.2020.111521
Ivar do Sul, J., and Costa, M. F. (2014). The present and future of microplastic pollution in the marine environment. Environ. Pollut. 185, 352–364. doi: 10.1016/j.envpol.2013.10.036
Kazarian, A. A., Taylor, M. R., Haddad, P. R., Nesterenko, P. N., and Paull, B. (2013). Ion-exchange and hydrophobic interactions affecting selectivity for neutral and charged solutes on three structurally similar agglomerated ion-exchange and mixed-mode stationary phases. Anal. Chim. Acta. 25:803, 143–53. doi: 10.1016/j.aca.2013.03.063
Kesimer, M., and Sheehan, J. K. (2012). Mass spectrometric analysis of mucin core proteins. Methods Mol. Biol. 842, 67–79. doi: 10.1007/978-1-61779-513-8_4
Liu, M., Lu, S., Song, Y., Lei, L., Hu, J., Lv, W., et al. (2018). Microplastic and mesoplastic pollution in farmland soils in suburbs of Shanghai. China Environ. Pollut. 242, 855–862. doi: 10.1016/j.envpol.2018.07.051
Liu, W., Mo, F., Jiang, G., Liang, H., Ma, C., Li, T., et al. (2018). Stress-induced mucus secretion and its composition by a combination of proteomics and metabolomics of the jellyfish Aurelia coerulea. Mar. Drugs 16:341. doi: 10.3390/md16090341
Lusher, A. (2015). “Microplastics in the marine environment: distribution, interactions and effects,” in Marine Anthropogenic Litter, eds M. Bergmann, L. Gutow, and M. Klages (Cham: Springer International Publishing), 245–307. doi: 10.1007/978-3-319-16510-3_10
Macali, A., Semenov, A., Venuti, V., Crupi, V., D’Amico, F., Rossi, B., et al. (2018). Episodic records of jellyfish ingestion of plastic items reveal a novel pathway for trophic transference of marine litter. Sci. Rep. 8:6105. doi: 10.1038/s41598-018-24427-7
Macali, A., and Bergami, E. (2020). Jellyfish as innovative bioindicator for plastic pollution. Ecol. Indic. 115:106375. doi: 10.1016/j.ecolind.2020.106375
Maes, T., Jessop, R., Wellner, N., Haupt, K., and Mayes, A. G. (2017). A rapid-screening approach to detect and quantify microplastics based on fluorescent tagging with Nile Red. Sci. Rep. 7:44501.
Mearns-Spragg, A., Tilman, J., Tams, D., and Barnes, A. (2020). The biological evaluation of jellyfish collagen as a new research tool for the growth and culture of iPSC derived microglia. Front. Mar. Sci. 7:689. doi: 10.3389/fmars.2020.00689
Merquiol, L., Romano, G., Ianora, A., and D’Ambra, I. (2019). Biotechnological applications of scyphomedusae. Mar. Drugs 17:604. doi: 10.3390/md17110604
Morritt, D., Stefanoudis, P., Pearce, D., Crimmen, O., and Clark, P. (2014). Plastic in the Thames: a river runs through it. Mar. Pollut. Bull. 78, 196–200. doi: 10.1016/j.marpolbul.2013.10.035
Murano, C., Donnarumma, V., Corsi, I., Casotti, R., and Palumbo, A. (2021). Impact of microbial colonization of polystyrene microbeads on the toxicological responses in the sea urchin Paracentrotus lividus. Environ. Sci. Technol. 55, 7990–8000. doi: 10.1021/acs.est.1c00618
Najem, J., Sarles, S. A., Akle, B., and Leo, J. D. (2012). Biomimetic jellyfish-inspired underwater vehicle actuated by ionic polymer metal composite actuators. Smart Mater. Struc. 21:11. doi: 10.1088/0964-1726/21/9/094026
Needleman, K. R., Neylan, I. P., and Erickson, B. T. (2018). Environmental and ecological effects of climate change on venomous marine and amphibious species in the wilderness. Wilderness Environ. Med. 29, 343–356. doi: 10.1016/j.wem.2018.04.003
Okoffo, E. D., O’Brien, S., O’Brien, J. W., Tscharke, B., and Thomas, K. V. (2019). Wastewater treatment plants as a source of plastics in the environment: a review of occurrence, methods for identification, quantification and fate. Environ. Sci. Water Res. Technol. 5, 1908–1931. doi: 10.1039/C9EW00428A
Oliveira, J., Belchior, A., Silva, V. D., Rotter, A., Petrovski, Z., Almeida, P. L., et al. (2020). Marine environmental plastic pollution: mitigation by microorganism degradation and recycling valorization. Front. Mar. Sci. 7:567126. doi: 10.3389/fmars.2020.567126
Padervand, M., Lichtfouse, E., Robert, D., and Wang, C. (2020). Removal of microplastics from the environment. A review. Environ. Chem. Lett. 18, 807–828. doi: 10.1007/s10311-020-00983-1
Paradiso, F., Fitzgerald, J., Yao, S., Barry, F., Taraballi, F., Gonzalez, D., et al. (2019). Marine collagen substrates for 2D and 3D ovarian cancer cell systems. Front. Bioeng. Biotechnol. 7:343. doi: 10.3389/fbioe.2019.00343
Patwa, A., Thiéry, A., and Lombard, F. (2015). Accumulation of nanoparticles in “jellyfish” mucus: a bio-inspired route to decontamination of nano-waste. Sci. Rep. 5:11387. doi: 10.1038/srep11387
Pearson, R., Tellam, R., Xu, B., Zhao, Z., Willcox, M., and Kongsuwan, K. (2011). Isolation, biochemical characterization and anti-adhesion property of mucin from the blue blubber jellyfish (Catostylus mosaicus). Bio. Meth. 2, 21–30. doi: 10.5376/bm.2011.02.0004
Poerio, T., Piacentini, E., and Mazzei, R. (2019). Membrane processes for microplastic removal. Molecules 24:4148. doi: 10.3390/molecules24224148
Pugliano, M., Vanbellinghen, X., Schwinté, P., Benkirane-Jessel, N., and Keller, L. (2017). Combined jellyfish collagen type II, human stem cells and Tgf-β3 as a therapeutic implant for cartilage repair. J. Stem Cell. Res. Ther. 7:4. doi: 10.4172/2157-7633.1000382
Purcell, J. E. (2009). Extension of methods for jellyfish and ctenophore trophic ecology to large-scale research. Hydrobiologia 616, 23–50. doi: 10.1007/s10750-008-9585-8
Prata, J. C., Silva, A., Costa, J. P., Mouneyrac, C., Walker, T. R., Duarte, A. C., et al. (2019). Solutions and integrated strategies for the control and mitigation of plastic and microplastic pollution. Int. J. Environ. Res. Public Health 16:2411. doi: 10.3390/ijerph16132411
Prinz, N., and Korez, Š (2020). “Understanding how microplastics affect marine biota on the cellular level is important for assessing ecosystem function: a review,” in YOUMARES 9 - The Oceans: Our Research, eds S. Jungblut, V. Liebich, and M. Bode-Dalby (Cham: Springer), 101–120. doi: 10.1007/978-3-030-20389-4_6
Rillig, M. C. (2012). Microplastic in terrestrial ecosystems and the soil? Environ. Sci. Technol. 46, 6453–6645. doi: 10.1021/es302011r
Rueden, C. T., Schindelin, J., Hiner, M. C., DeZonia, B. E., Walter, A. E., Arena, E. T., et al. (2017). ImageJ2: imageJ for the next generation of scientific image data. BMC Bioinformatics 18:529. doi: 10.1186/s12859-017-1934-z
Schuhen, K., Sturm, M. T., and Herbort, A. F. (2018). “Technological approaches for the reduction of microplastic pollution in seawater desalination plants and for sea salt extraction,” in Plastics in the Environment, ed. A. Gomiero, (IntechOpen), doi: 10.5772/intechopen.81180
Schür, C., Rist, S., Baun, A., Mayer, P., Hartmann, N. B., and Wagner, M. (2019). When fluorescence is not a particle: the tissue translocation of microplastics in Daphnia magna seems an artifact. Environ. Toxicol. Chem. 38, 1495–1503. doi: 10.1002/etc.4436
Shanks, A. L., and Graham, W. M. (1988). Chemical defense in a scyphomedusa. Mar. Ecol. Prog. Ser. 45, 81–86. doi: 10.3354/meps045081
Spangenberg, D. B., Jernigan, T., Philput, C., and Lowe, B. (1994). Graviceptor development in jellyfish ephyrae in space and on Earth. Adv. Space Res. 14, 317–325. doi: 10.1016/0273-1177(94)90418-9
Stabili, L., Schirosi, R., Parisi, M. G., Piraino, S., and Cammarata, M. (2015). The mucus of Actinia equina (Anthozoa, Cnidaria): an unexplored resource for potential applicative purposes. Mar. Drugs 3, 5276–5296. doi: 10.3390/md13085276
Stojković, B., Sretenovic, S., Dogsa, I., Poberaj, I., and Stopar, D. (2015). Viscoelastic properties of levan-DNA mixtures important in microbial biofilm formation as determined by micro- and macrorheology. Biophys. J. 108, 758–765. doi: 10.1016/j.bpj.2014.10.072
Sun, J., Dai, X., Wang, Q., van Loosdrecht, M. C. M., and Ni, B. J. (2019). Microplastics in wastewater treatment plants: detection, occurrence and removal. Water Res. 152, 21–37. doi: 10.1016/j.watres.2018.12.050
Talvitie, J., Mikola, A., Koistinen, A., and Setala, O. (2017). Solutions to microplastic pollution – removal of microplastics from wastewater effluent with advanced wastewater treatment technologies. Water Res. 123, 401–407. doi: 10.1016/j.watres.2017.07.005
Thompson, R. C., Olsen, Y., Mitchell, R. P., Davis, A., Rowland, S. J., John, A. W. G., et al. (2004). Lost at sea: where is all the plastic? Science 304:838. doi: 10.1126/science.1094559
van Sebille, E., Wilcox, C., Lebreton, L., Maximenko, N., Hardesty, B. D., et al. (2015). A global inventory of small floating plastic debris. Environ. Res. Lett. 10:124006. doi: 10.1088/1748-9326/10/12/124006
Verdugo, P. (2012). Supramolecular dynamics of mucus. Cold Spring Harb. Perspect Med. 2:a009597. doi: 10.1101/cshperspect.a009597
Wang, Z., Lin, T., and Chen, W. (2019). Occurrence and removal of microplastics in an advanced drinking water treatment plant (ADWTP). Sci. Total Environ. 700:134520. doi: 10.1016/j.scitotenv.2019.134520
Wong, J. K. H., Lee, K. K., Tang, K. H. D., and Yap, P. S. (2020). Microplastics in the freshwater and terrestrial environments: prevalence, fates, impacts and sustainable solutions. Sci. Total Environ. 719:137512. doi: 10.1016/j.scitotenv.2020.137512
Keywords: jellyfish, mucus, microplastics, polystyrene, viscosity, microscopy
Citation: Lengar Ž, Klun K, Dogsa I, Rotter A and Stopar D (2021) Sequestration of Polystyrene Microplastics by Jellyfish Mucus. Front. Mar. Sci. 8:690749. doi: 10.3389/fmars.2021.690749
Received: 04 April 2021; Accepted: 11 June 2021;
Published: 15 July 2021.
Edited by:
Giovanna Romano, Anton Dohrn Zoological Station, ItalyReviewed by:
Anna Palumbo, Stazione Zoologica Anton Dohrn, ItalyCopyright © 2021 Lengar, Klun, Dogsa, Rotter and Stopar. This is an open-access article distributed under the terms of the Creative Commons Attribution License (CC BY). The use, distribution or reproduction in other forums is permitted, provided the original author(s) and the copyright owner(s) are credited and that the original publication in this journal is cited, in accordance with accepted academic practice. No use, distribution or reproduction is permitted which does not comply with these terms.
*Correspondence: David Stopar, ZGF2aWQuc3RvcGFyQGJmLnVuaS1sai5zaQ==; Ana Rotter, YW5hLnJvdHRlckBuaWIuc2k=
†These authors share senior authorship
Disclaimer: All claims expressed in this article are solely those of the authors and do not necessarily represent those of their affiliated organizations, or those of the publisher, the editors and the reviewers. Any product that may be evaluated in this article or claim that may be made by its manufacturer is not guaranteed or endorsed by the publisher.
Research integrity at Frontiers
Learn more about the work of our research integrity team to safeguard the quality of each article we publish.