- 1Botany Department, Nelson Mandela University, Port Elizabeth, South Africa
- 2DSI/NRF Research Chair in Shallow Water Ecosystems, Institute for Coastal and Marine Research, Nelson Mandela University, Port Elizabeth, South Africa
- 3Department of Forestry, Fisheries and Environment, Cape Town, South Africa
- 4Mossel Bay Municipality, Mossel Bay, South Africa
- 5Zoology Department, Nelson Mandela University, Port Elizabeth, South Africa
- 6Council for Scientific and Industrial Research (CSIR), Stellenbosch, South Africa
Increased nutrient loading associated with rapid population growth is the leading cause of deteriorating water quality in urbanized estuaries globally. Small estuaries are particularly sensitive to changes when connection with the marine environment is restricted, or lost, because of high water retention. The temporarily closed Hartenbos Estuary (South Africa) is an example of how such pressures can culminate in a severely degraded ecosystem. Wastewater treatment work (WWTW) discharges introduce substantial volumes of freshwater (8,000 m3 d–1) and nutrient loads (38 kg DIN d–1 and 22 kg DIP d–1) into this estuary. This constant inflow has necessitated frequent artificial breaching (inducing alternating states) of the estuary mouth to prevent flooding of low-lying developments and, occasionally, to mitigate against extreme events such as fish kills and sewage spills. This study investigated the efficacy of artificial mouth breaching practices in eliciting responses in selected abiotic and biotic parameters. Microalgal (phytoplankton and benthic diatoms), benthic macrofauna and fish community dynamics were assessed in response to mouth state and water quality conditions using a seasonal monitoring programme. The hypereutrophic nature of the Hartenbos Estuary was highlighted by persistent high-biomass phytoplankton accumulations (>100 μg Chl-a l–1), extreme dissolved oxygen conditions (0.4–20.5 mg O2 l–1) and the predominance of harmful algal bloom (HAB) events comprising Nannochloropsis sp. and Heterosigma akashiwo. Artificial breaching of the mouth facilitated limited tidal exchange and occurred approximately bimonthly once water levels exceeded 1.9 m above mean sea level (MSL). Current pressures and management interventions have culminated in an ecosystem void of natural fluctuations and instead characterised by low diversity and shifts between undesirable states. This is highlighted by the near year-round dominance of only a few opportunistic species/groups tolerant of adverse conditions (e.g., Nannochloropsis sp., Halamphora coffeiformis, oligochaetes, estuarine round herring Gilchristella aestuaria, and southern mullet Chelon richardsonii). Therefore, catchment-scale interventions such as the diversion of WWTW discharges and restoration of hydrodynamic variability are management priorities for improving the health and biodiversity of small, closed microtidal systems such as the Hartenbos Estuary.
Introduction
The issue of coastal eutrophication has been researched extensively (Nixon, 1995; Cloern, 2001; Bricker et al., 2008; Le Moal et al., 2019; Lemley and Adams, 2019; Malone and Newton, 2020) due to its worldwide prominence and close linkages with anthropogenic global change pressures (e.g., nutrient enrichment, warming, hydrological modifications). The eutrophication process is driven by an increased rate of supply of nutrients and organic matter that stimulates excessive primary producer growth, which, in turn, causes an array of indirect and undesirable secondary responses, such as oxygen depletion, harmful algal blooms (HABs), habitat loss, reduced biodiversity, and trophic restructuring. The variety of manifestation pathways related to eutrophication is diverse and complex, often culminating in drastic, potentially irreversible, shifts to the structure and functioning of coastal ecosystems, i.e., alternate states (Duarte et al., 2015). Estuaries are particularly susceptible to such perturbations because of their positioning at the nexus between terrestrial and marine exchanges that facilitates inherent spatiotemporal variability regarding physical, chemical, and biotic properties along the salinity gradient (Cloern et al., 2017). Thus, the continued deterioration of coastal and estuarine ecosystems has led to widespread restoration efforts that aim to alleviate the pressures that cause degradation (Duarte and Krause-Jensen, 2018; Boesch, 2019; Le Moal et al., 2019). Such efforts, typically centred around a combination of nutrient reduction strategies and engineering solutions, tend to emanate from legal policies (e.g., Clean Water Act, United States; Water Framework Directive and Marine Strategy Framework Directive, Europe; National Water Act, South Africa) that prompt management interventions when an ecosystem is classified as being in an undesirable condition (Lemley and Adams, 2019). Successful abatement of eutrophication is complicated by shifting ecological baselines and the dissimilar trajectories of ecosystem degradation and recovery (Duarte et al., 2015). Recovery typically requires a greater reduction in pressure compared to that which initiated degradation to reduce or remove negative feedback loops (e.g., internal nutrient loading, altered food webs) that act to maintain the degraded state. This was exemplified by Duarte et al. (2009) that highlighted the convoluted recovery trajectories, and inability to return to past norms, of four European estuaries that underwent significant nutrient load reductions (oligotrophication). This highlights the importance for scientists and managers to consider any environmental changes that have occurred relative to natural conditions (or desired states) when setting restoration targets. Moreover, the recovery of estuarine ecosystems is closely linked to the (1) severity of the disturbance/pressure, and (2) degree of connectivity with adjacent healthy ecosystems.
Microtidal estuaries (tidal range <2 m) that occur in low rainfall climates are prone to periods of prolonged water residency and mouth closure (i.e., ranging from weeks to years) that promotes accumulation of contaminants and, thus, increased susceptibility to anthropogenic pressures (Warwick et al., 2018; Adams et al., 2020). This heightened sensitivity to global change perturbations, compared to well-flushed estuaries with increased marine connectivity, occasionally necessitates the implementation of unique management interventions geared toward mitigating the frequency and severity of deleterious events (e.g., HABs, oxygen depletion, fish kills). Similar to other Mediterranean-like regions (e.g., Australasia, Portugal, California and Texas, United States), small microtidal estuaries with intermittent marine connectivity represent the numerically dominant ecosystem type in South Africa, with >75% of the 290 estuaries classified as either temporarily or predominantly closed (van Niekerk et al., 2020). Of these systems, approximately 30% have been classified as being under high to very high pollution pressure, mainly stemming from wastewater treatment work (WWTW) discharges, agricultural return flows, and urban runoff (van Niekerk et al., 2019; Adams et al., 2020). Unlike developed regions where restoration efforts have been widely adopted and implemented, these anthropogenic nutrient pressures are currently increasing in South Africa. The resultant degradation of estuarine health, together with floodplain development and altered freshwater inflow patterns, has also increased the occurrence of mouth manipulation interventions (van Niekerk et al., 2019).
The potential efficacy of artificial mouth breaching as a management approach to improve water quality in South Africa is justified by enhanced tidal exchange (increased salinity), decreased nutrient concentrations, and dilution of micro- and macroalgal biomass (Human et al., 2016; Lemley et al., 2019). However, premature (low water level) and mistimed (unseasonal) breaching is a concern given that it inhibits the flushing potential and enhances sedimentation. The hypereutrophic Hartenbos Estuary is an example of a small, high-retention South African system that is subject to prolonged periods of mouth closure, significant nutrient pressures, freshwater inflow alterations, and regular mouth manipulation (Lemley et al., 2015). These anthropogenic perturbations have resulted in an ecosystem characterised by excessive primary producer biomass, reduced biodiversity, hypoxic conditions, and intermittent mass fish mortality events. Thus, it provides a suitable case study from which to investigate the management interventions required to improve and/or restore ecosystem functionality in similar heavily degraded microtidal systems. Accordingly, this study aimed to assess whether existing mouth breaching practices are an adequate long-term solution to achieve desirable responses in selected water quality parameters and biotic communities.
Materials and Methods
Study Area
Classified as a large temporarily closed system, the Hartenbos Estuary is located along the warm temperate south coast of South Africa, with the mouth (34°7′2.69″S; 22°7′27.12″E) situated approximately 9 km northeast of Mossel Bay (Figure 1; van Niekerk et al., 2019). The restricted nature of the inlet allows for only periodic marine connectivity via semidiurnal microtidal (≤1 m) exchanges with the adjacent Indian Ocean. With a relatively small catchment (170 km2) and open water (42 ha) area (van Niekerk et al., 2015), the Hartenbos Estuary is approximately 4 km in length and is characterised by shallow water depths (<0.5–2 m). The system has undergone substantial anthropogenic manipulation in recent history, dating back to 1970 when the construction of the Hartebeeskuil Dam, with a total storage capacity of 7.2 × 106 m3, was completed (Bickerton, 1982). Following its construction, the dam water was declared unsuitable for human consumption or irrigation purposes due to the brackish nature of the springs in the Hartenbos catchment. Thus, despite minimal allocations for livestock watering, the Hartebeeskuil Dam serves as a major freshwater obstruction to the downstream estuary, culminating in a poorly flushed ecosystem with little hydrodynamic variability and a loss of natural ecosystem functionality. This lack of natural dynamism was further confounded by the Hartenbos Regional WWTW that became operational in 1986, despite evidence highlighting the potential for deleterious consequences (Bickerton, 1982). At present, the Hartenbos WWTW employs activated sludge, biological nutrient removal, and screw press dewatering technologies to treat sewage (Department of Water Affairs, 2012). Situated within the estuarine functional zone (Figure 1), the Hartenbos WWTW is estimated to discharge 5.3 × 106 m3 of mostly treated effluent to the Hartenbos Estuary annually (Lemley et al., 2014). This volume exceeds both the natural (4.6 × 106 m3) and present-day (2.8 × 106 m3) mean annual runoff estimates for the catchment (van Niekerk et al., 2019). These WWTW discharges contribute disproportionately high dissolved inorganic nitrogen (38.3 kg DIN d–1), and phosphorus (21.5 kg DIP d–1) loads to the estuary, particularly given the small size and predominantly closed nature of the system (Lemley et al., 2014). These WWTW inputs, together with the pressures associated with the surrounding urban (e.g., stormwater drains) and agricultural (e.g., fertilizer application) developments (Figure 1), have resulted in the Hartenbos Estuary being classified as persisting in a predominantly hypereutrophic condition (Lemley et al., 2015). Additionally, the estuary inlet has been artificially breached at lower than natural water levels for decades, resulting in a loss of scouring potential during breaching and potentially contributing to the shallow depths in the lower reaches. The near steady-state inflow of nutrient-rich WWTW effluents has necessitated the formulation and adoption of an expanded mouth management protocol that enables the management authority to breach the mouth when water levels exceed 1.9 m mean sea level (MSL) to prevent flooding of surrounding developments and/or in response to extreme events (e.g., fish kills, sewage spills).
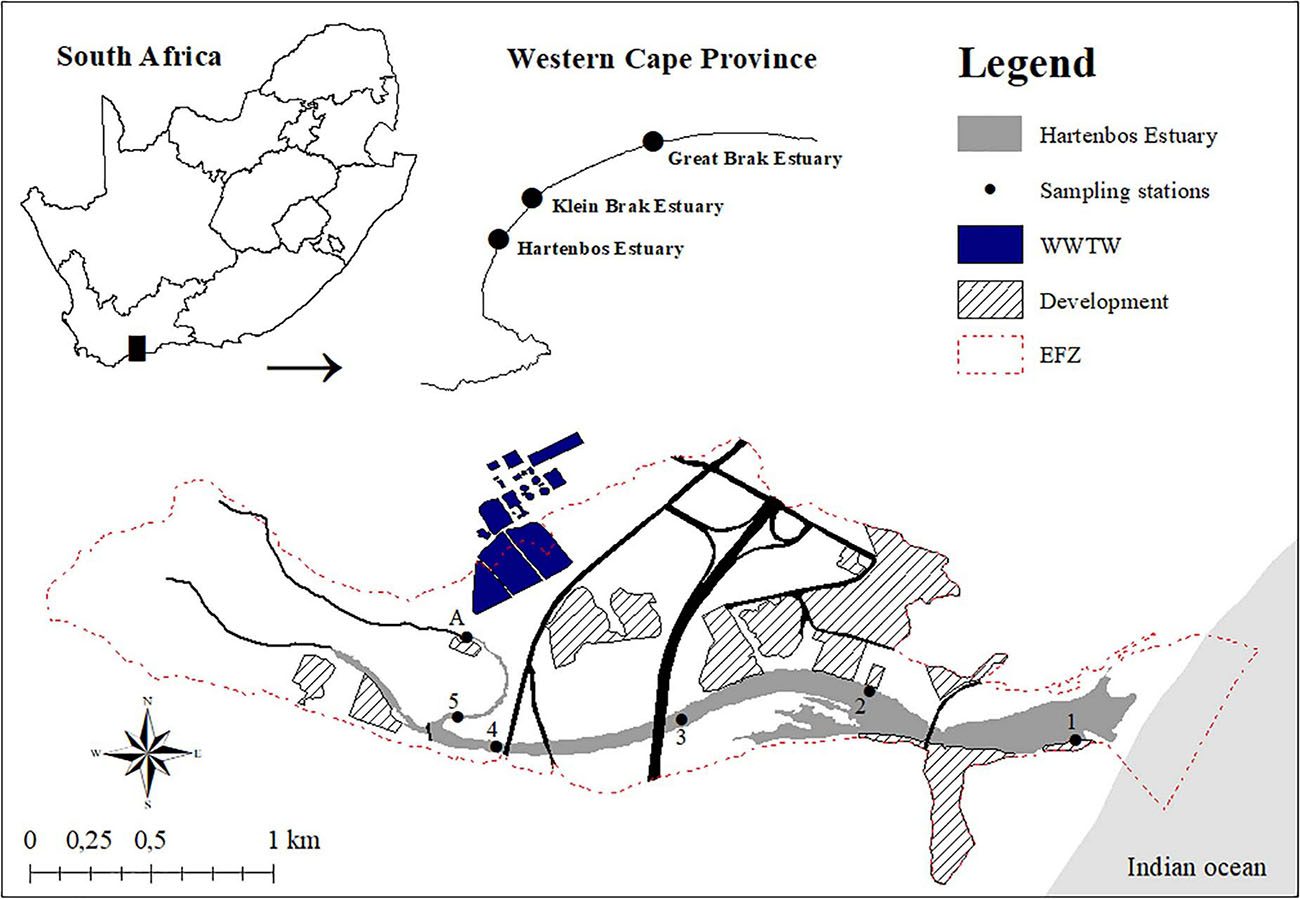
Figure 1. Distribution of the sampling stations, wastewater treatment works (WWTW), and urban developments within the estuarine functional zone (EFZ) of the temporarily closed Hartenbos Estuary, South Africa.
Sampling Design
Five sampling surveys were conducted during the period from February 2019 to January 2020 to characterise the variability of selected abiotic and biotic parameters in the Hartenbos Estuary in response to management interventions (e.g., artificial mouth breaching). The seasonal sampling programme encapsulated each of the distinct mouth conditions typical of temporarily closed estuaries in South Africa (sensu Snow and Taljaard, 2007), namely: open (February 26, 2019), semi-closed (i.e., open at high tide, closed at low; May 04, 2019 and January 25, 2020) and closed (July 16, 2019 and September 18, 2019). During each survey, samples were collected at five fixed locations along the length of the estuary (Figure 1) to assess the spatial variability typical of transitional waters. Of the selected parameters, abiotic (physico-chemistry, inorganic nutrients) and microalgal (phytoplankton, benthic microalgae) components were assessed on each sampling date, while fish and macrobenthic fauna data were not available for the first (February 26, 2019; open) and last (January 25, 2020; semi-closed) surveys, respectively. However, despite this, the available data for each component is representative of each season.
Abiotic Variables
Water level data, encompassing the period from January 24, 2019 to January 25, 2020, were obtained from the Department of Water and Sanitation (DWS), South Africa. The monitoring station (K1T010) is located in the mid- to lower reaches (near to Site 2) of the estuary (34°07′02.0″S; 22°06′59.0″E) and the data it records is used by the local management authority as an indicator (water level ≥1.9 m MSL) to initiate the enactment of a mouth management protocol that provides pre-approval for breaching. On each sampling occasion, in-situ depth-interval measurements of abiotic parameters, taken at 0.5 m increments from surface to bottom-waters, were recorded at the fixed sampling localities using a YSI ProDSS multiparameter water quality meter. These included salinity, water temperature (°C), dissolved oxygen (mg l–1), pH, and turbidity (FNU). Water samples for inorganic nutrient analyses were collected at each site using a weighted pop-bottle at specified depths, i.e., surface (0 m), 0.5 m (when water depth ≥1 m), and near-bottom. Samples were filtered onboard through hydrophilic polyvinylidene difluoride (PVDF) 0.47 μm pore-size syringe filters and placed into acid-washed polyethylene bottles before being frozen. Orthophosphate (PO43–), ammonium (NH4+) and total oxidised nitrogen (NOx = NO3– + NO2–) concentrations were determined using standard spectrophotometric methods (Bate and Heelas, 1975; Parsons et al., 1984). Subsequently, inorganic nutrients were categorised as dissolved inorganic nitrogen (DIN = NH4+ + NOx) and phosphorus (DIP = PO43–) and expressed in mg l–1.
Biotic Communities
Multiple biotic communities (i.e., primary producers, consumers) were selected as indicators to provide a robust assessment of estuary health (O’Brien et al., 2016) in response to mouth breaching events. Taxa were identified to the minimum taxonomic resolution required to adequately describe community patterns and responses to changes in water quality and hydrological conditions (see Garmendia et al., 2013; Lemley et al., 2019; Gerwing et al., 2020; Ribeiro et al., 2020).
Water samples for phytoplankton analyses were collected concomitantly with those for inorganic nutrient analyses. Phytoplankton biomass, measured as chlorophyll-a concentration (expressed as μg Chl-a l–1), was determined by filtering replicate samples of a known volume of water (ranging from 100 to 250 ml) through 1.2 μm pore-sized glass-fibre filters (Munktell© MGC). The filters were then placed in aluminium foil and frozen prior to analysis. Once in the laboratory, chlorophyll-a was extracted by placing thawed filters into glass scintillation vials with 10 ml of 95% ethanol (Merck© 4111) for 24 h in a cold (ca. 2°C), dark room. Thereafter, any suspended particles were removed by re-filtering the extracts. Next, the absorbance of the cleared extracts, before and after acidification with 1N HCl, was read using a Thermo ScientificTM GENESYSTM 10S UV–Vis spectrophotometer at a wavelength of 665 nm. Chlorophyll-a concentration was calculated using the equation set out in Snow et al. (2000) that was derived from Nusch (1980). For the purposes of phytoplankton identification and enumeration, depth-interval water samples were fixed with 25% glutaraldehyde solution (Sigma-Aldrich® Chemicals G5882) to a final concentration of 1% (by volume). Next, 10 ml of each fixed sample was placed into 26.5 mm diameter Utermöhl chambers and allowed to settle for 24 h before identification and enumeration (cells ml–1; as per Snow et al., 2000) were conducted using an inverted Leica DMIL phase contrast microscope at a magnification of 630×.
Benthic microalgal communities were sampled along the adjacent shoreline at each of the five sampling stations. Microphytobenthic (MPB) biomass (expressed as mg Chl-a m–2) was determined by collecting four replicate sediment samples (two cores per replicate) from the subtidal zone (0.3–0.5 m depth) using a Perspex twin-corer with an internal diameter of 20 mm. Samples were placed into acid-washed polypropylene specimen containers and frozen prior to analysis. The determination of MPB biomass followed a similar procedure described for phytoplankton biomass, with the only differences being related to the extraction process, i.e., 15 ml of 95% ethanol (Merck 4111) added to the samples and extraction for 6 h (Brito et al., 2009). Samples for benthic diatom community analyses were collected from surface sediments (top few millimetres) in the subtidal zone at each site and living diatoms were harvested using the cover slip method (Bate et al., 2013). The process of diatom preparation for identification was performed according to the method described by Taylor et al. (2005). A minimum of 400 diatom valves were counted using a Zeiss Axioplan light microscope with Differential Interference Contrast (DIC) optics at a magnification of 1000×.
Subtidal macrobenthic fauna were collected at each of the five localities that benthic microalgae samples were collected. A 30 cm2 van Veen stainless steel grab was pushed into the sediment to a maximum depth of 5 cm and triplicate sediment cores were placed in a 20 L bucket (Teske and Wooldridge, 2001). Estuary water was then added, and the sediment homogenised and suspended by swirling (Pillay and Perissinotto, 2008). The suspension was poured through a 500 μm mesh whereafter the process of adding water and resuspension was repeated at least three times until only sediment and large inorganic matter remained in the bucket, which was then rinsed over 2 mm mesh in a box sieve (Pillay and Perissinotto, 2008). The rinsed sample on the 500 μm mesh and any macrofauna (e.g., molluscs) retained in the box sieve were placed into acid-washed plastic bottles with 250 ml of estuary water containing 5% formalin (preservative) and Phloxin-B (pink tissue stain to facilitate invertebrate sorting) (Teske and Wooldridge, 2001; Pillay and Perissinotto, 2008). In the laboratory, each sample was rinsed homogenously onto a 22 cm diameter 500 μm sieve. Random sub-sampling by weight, following the techniques of Glozier et al. (2002), occurred when large amounts of non-invertebrate organic matter were present or if macroinvertebrate counts were large (>1,000 per taxon). The entire or sub-divided sample was sorted in a large tray with a small volume of water added and each specimen was isolated, identified to at least class level, and counted such that abundance could be reported as count per square meter of benthic sediment.
Seine nets, which capture juvenile and smaller species, were deployed to sample the fish community in the Hartenbos Estuary. The seine net was 30 m long and 2 m deep, with a 15 mm bar mesh and a 5 mm bar mesh in the middle 10 m that includes the cod-end. Deployments occurred in the littoral habitats at the five sampling stations during daylight hours. The seine net was deployed from a dinghy in a semi-circle formation and subsequently hauled to shore by four people, i.e., the mean area swept per haul was 160 m2. Fish collected in the seine nets were placed into buckets of estuarine water and then sorted to the lowest taxonomic level. Once the fish were identified, they were returned alive to the system. Fish species were categorised into guilds based on their dependency on estuaries using the international estuarine functional guild classification proposed by Potter et al. (2015), using Whitfield (2019) as a guide for fishes occurring in South African estuaries. For comparison, regular fish surveys of two nearby estuaries the Klein Brak (4 km N) and similarly sized Groot Brak (12 km N) were co-ordinated to overlap with the Hartenbos trips.
Data Analysis
All statistical analyses and graphical illustrations were performed in the R environment (version 3.6.1, R Core Team, 2020). The “vegan” package was used to calculate the effective number of species (ENS) for each of the biotic communities based on species/group abundance. The ENS is the number of equally abundant species required to produce a particular value of a given index; in this case, Shannon’s H′ index, where ENS = exp(H′) (Jost, 2006). The ENS metric is a standardised measure of true species diversity and is more robust against sampling and statistical issues than species richness and other diversity metrics that are related to entropy (uncertainty) (Jost, 2006). This unified approach provides an effective means of interpreting the effect of ecological drivers on biodiversity (Chase and Knight, 2013; Rishworth et al., 2020). Non-metric multidimensional scaling (NMDS) was applied (“vegan” package) to abundance data for phytoplankton, benthic diatom, benthic macrofauna, and fish groups/species to illustrate the variation in community structure between different mouth conditions. A three-dimensional solution (k = 3) was chosen to reduce high stress values. Additionally, selected environmental vectors (salinity, water temperature, dissolved oxygen, pH, turbidity, DIN, DIP, and halocline extent) were fitted (permutations = 999) to the NMDS plots to assess which variables best explain (squared correlation coefficient, r2) community assemblage variance. The Shapiro–Wilk W test was used to evaluate data normality. The interactions between selected abiotic and biotic parameters were evaluated using the non-parametric Spearman’s rank correlation. All analyses were tested at a significance level of α < 0.05.
Results
Abiotic Variables
Water level in the mid- to lower reaches of the Hartenbos Estuary ranged from 0.98 to 2.03 m MSL throughout the study period (Figure 2A). Estuarine water levels display a largely cyclical pattern, with breaching events occurring approximately bimonthly due to the consistent nature of effluent discharges entering the system from the Hartenbos WWTW. A total of 7 authorised breaching events occurred in 2019, including: February 18, April 18, June 14, August 01, September 04, October 10, and December 11. As outlined in the mouth management protocol, the majority of these breaching events were initiated during spring tides (i.e., maximum tidal exchange) once estuarine water levels exceeded 1.9 m MSL. The open mouth conditions and semidiurnal tidal variability observed (Figure 2B1) during the first sampling interval (February 2019) highlight the efficacy of this approach. Mouth manipulations at water levels below the prescribed guideline (≥1.9 m MSL) occurred on September 04 (1.78 m MSL) and December 11 (1.81 m MSL) and were initiated in response to a mass fish mortality event and socio-economic requirements (holiday season), respectively. Notably, the reduced water levels during excavation of the inlet on September 4, 2019 occurred on a neap tide, which prevented tidal exchanges and facilitated rapid mouth closure (Figure 2B4). Similarly, an unauthorised mouth breaching event took place on January 3, 2020 (1.61 m MSL) at very low water levels during a neap tide and subsequently closed 5 days later. The estuarine inlet breached again approximately 2 weeks later (1.72 m MSL), reportedly in response to overwashing from the adjacent coastal environment and rainfall in the hardened lower catchment (stormwater runoff). However, the moderate water levels prior to this natural breaching event culminated in perched, semi-closed mouth conditions prevailing, with seawater only overwashing during high tides (Figure 2B5). High water levels at breaching are required to facilitate scouring of accumulated sediments, thus enabling prolonged periods of open mouth conditions thereafter. The importance of adequate water levels prior to breaching was highlighted by a period of prolonged marine connectivity during October 2019 when an effective breaching event (>2 m MSL; October 10) coincided with spring storm tides (max. 1.96 m MSL; October 27). While semi-closed and closed mouth conditions are not easily distinguished from each other, the water level dataset indicates that periods of marine connectivity (i.e., daily water level amplitude ≥10 cm) only occurred for 12.3% of the study period.
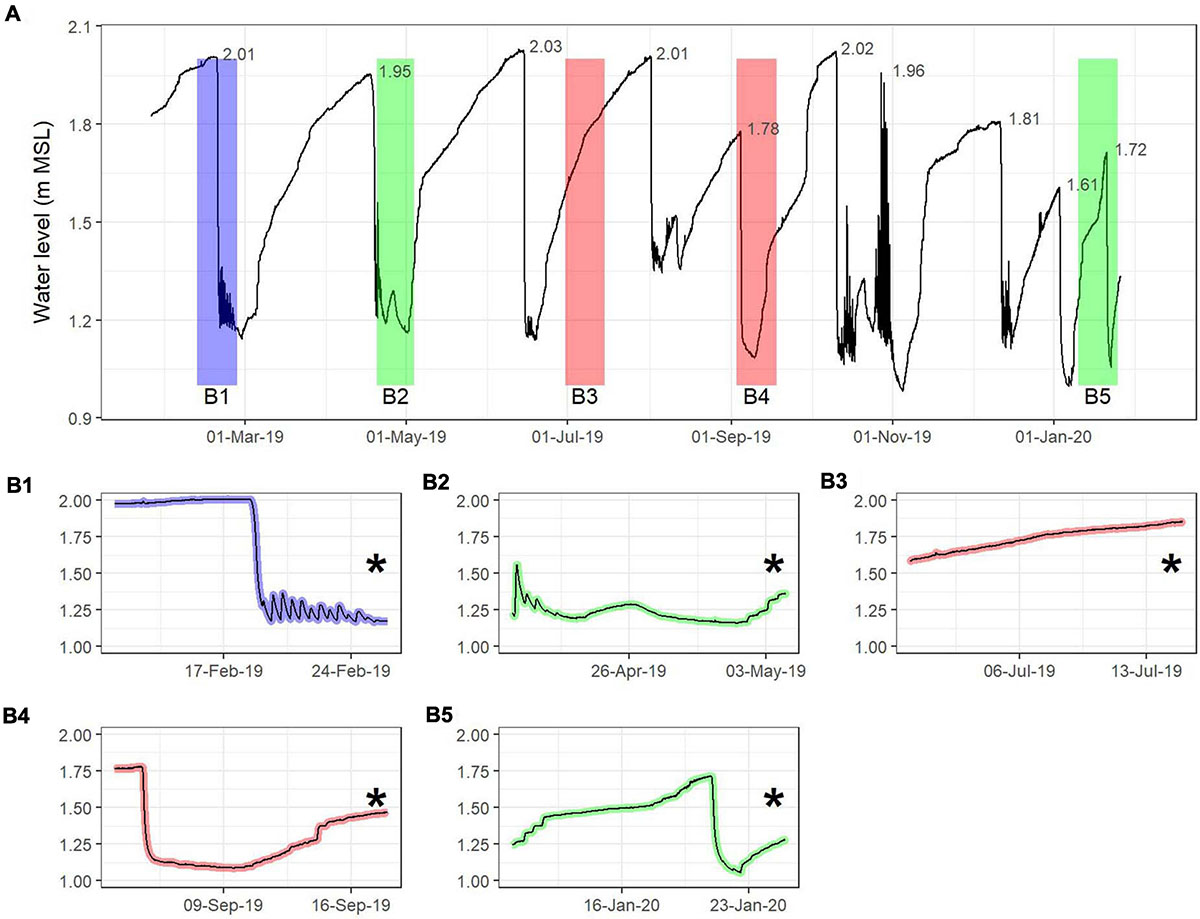
Figure 2. Six-minute interval water level variability (A) recorded throughout the study period (January 24, 2019 to January 25, 2020) at DWS Station K1T010 situated near Site 2 (see Figure 1). Short-term fluctuations are indicated for each of the sampling occasions, i.e., February 2019 (B1), May 2019 (B2), July 2019 (B3), September 2019 (B4), and January 2020 (B5). A 14-day period prior to the sampling date was chosen for this to represent a full tidal cycle that would encapsulate any overwashing events during closed mouth conditions. Sampling intervals (*) are demarcated according to the degree of mouth connectivity with the marine environment on the day of sampling; including open (blue), semi-closed (green), and closed (red) mouth conditions.
The restricted nature of tidal exchange in the Hartenbos Estuary was highlighted by the predominance of meso- (salinity: 5–18) and polyhaline (18–30) conditions throughout the study period (Figure 3). The open mouth phase was characterised by largely homogenous polyhaline conditions (21.1 ± 3.0), while mesohaline conditions (11.5 ± 3.7) were typical of the closed mouth phase (i.e., dominant state under present conditions). Sampling dates classified as exhibiting semi-closed mouth conditions were dissimilar, with polyhaline and mesohaline conditions observed during the May 2019 (19.0 ± 3.9) and January 2020 (8.9 ± 3.1) intervals, respectively. The reduced salinity and limited intrusion of seawater observed during the January 2020 sampling interval can be attributed to the unauthorised breaching event that occurred 3 weeks prior, when estuarine water levels were low (1.61 m MSL) (Figure 2A). Vertical salinity profiles, hereafter referred to as the halocline (i.e., difference between bottom and surface salinity), were observed in the middle reaches (Sites 2–4) during both semi-closed sampling intervals and the closed mouth conditions in September 2019 (Supplementary Table 1). The degree of stratification varied between these dates, i.e., May 2019 (8.0 ± 1.5), September 2019 (9.7 ± 4.5) and January 2020 (3.9 ± 2.6). Notably, continuous freshwater discharges from the Hartenbos WWTW were evidenced by distinct haloclines in the upper reaches (Site 5) on all sampling occasions (Supplementary Table 1).
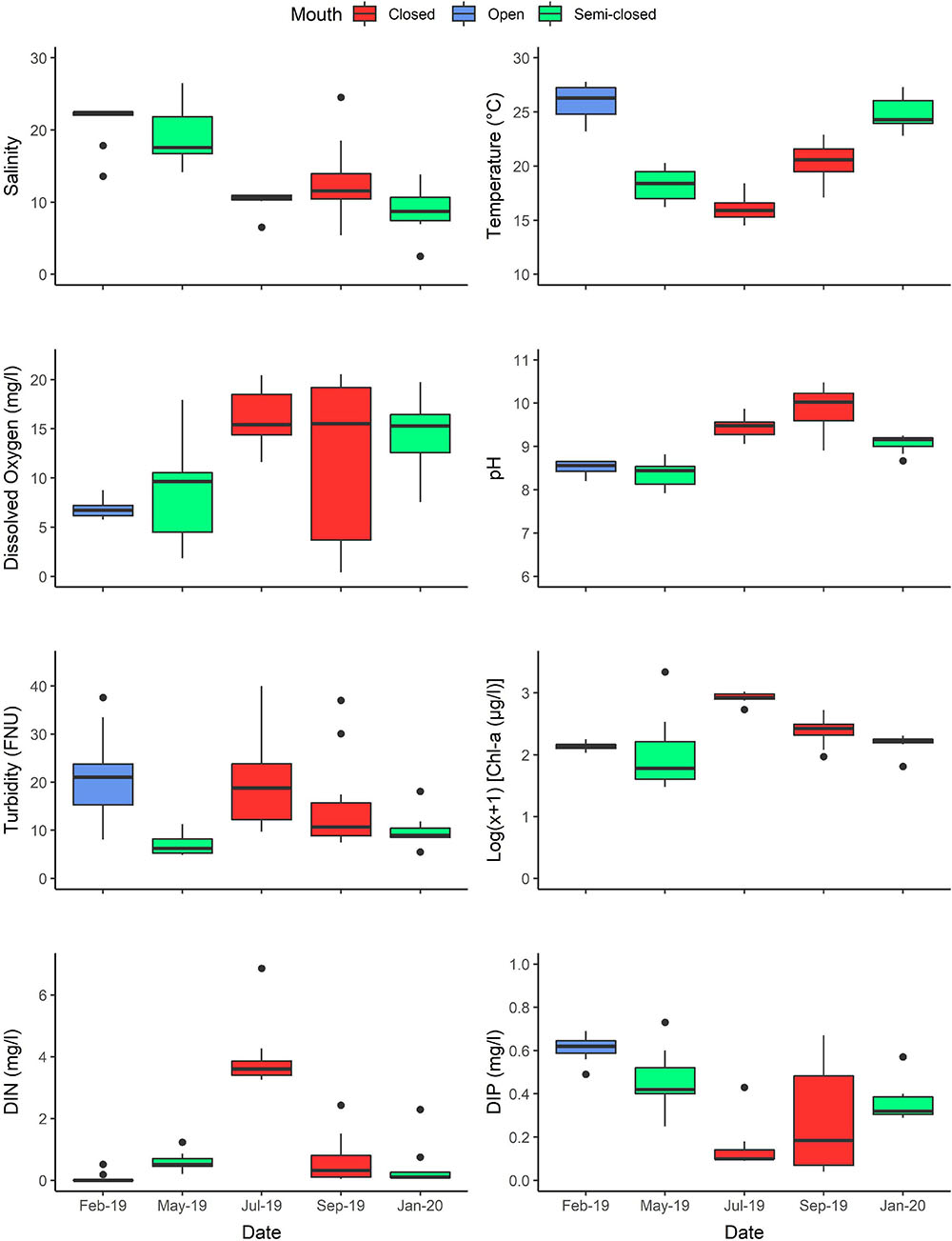
Figure 3. Physico-chemical [salinity, water temperature, dissolved oxygen, pH, and turbidity (FNU)], inorganic nutrients [dissolved inorganic nitrogen (DIN) and phosphorus (DIP)] and phytoplankton biomass (chlorophyll-a) variability recorded on each sampling occasion. The degree of estuary connectivity with the marine environment on each sampling date is indicated, i.e., open (blue), semi-closed (green), and closed (red) mouth conditions. Boxplots represent median values (solid line), interquartile range (IQR = 25th and 75th percentiles; box), ± 1.5 × IQR (whiskers), and outlier values.
Due to the shallow nature of the system, water temperature reflected typical climatic seasonal variations, with the highest temperatures recorded in summer (February 2019: 25.8 ± 1.7°C; January 2020: 25.0 ± 1.5°C) and the lowest in winter (July 2019: 16.1 ± 1.2°C). Additionally, moderate conditions were typical of the autumn (May 2019: 18.2 ± 1.5°C) and spring (September 2019: 20.5 ± 1.6°C) sampling intervals. Surface waters were predominantly warmer than bottom-waters yet typically displayed only marginal fluctuations (thermocline gradient <2°C) due to the shallow-nature of the estuary (Supplementary Table 1). Dissolved oxygen conditions were highly variable throughout the study period, ranging from near-anoxic (0.4 mg l–1; 5.4%) to supersaturated (20.5 mg l–1) levels. This represents a marked deviation from historical records that reported more stable dissolved oxygen conditions prior to 1982, i.e., ranging from 4.2 to 7.7 mg l–1 (Bickerton, 1982). Supersaturated surface waters (>18 mg l–1; >185%) were recorded during all sampling intervals representing closed and semi-closed mouth phases, while the open mouth phase (February 2019) was characterised by homogenous and well-oxygenated conditions (6.9 ± 0.9 mg l–1; 95.0 ± 11.6%). Instances of bottom-water hypoxia (<2 mg l–1) were recorded in the middle reaches in May 2019 (1.8 mg l–1 or 23.1%; semi-closed) and September 2019 (0.4–2.0 mg l–1 or 5.4–25.4%; closed) concomitant with marked haloclines. These fluctuations in dissolved oxygen conditions were primarily correlated with salinity (P < 0.001; r = −0.82) and phytoplankton biomass (P < 0.01; r = 0.44). Similarly, pH (P < 0.001; r = 0.63) and turbidity (P < 0.01; r = 0.37) were positively associated with increased chlorophyll-a concentrations. Severe hypereutrophic conditions (>100 μg Chl-a l–1; log10(x+1) = 2.0) were recorded throughout the study period, with the excessive magnitude thereof evidenced by the phytoplankton biomass maxima observed in February 2019 (178.8 μg l–1), May 2019 (2,209.6 μg l–1), July 2019 (1,039.0 μg l–1), September 2019 (523.9 μg l–1), and January 2020 (205.4 μg l–1). Furthermore, closed mouth conditions (580.1 ± 325.4 μg l–1) supported elevated and more widespread phytoplankton biomass levels compared to the semi-closed (226.8 ± 449.2 μg l–1) and open (136.7 ± 19.1 μg l–1) mouth phases. A similar trend was observed for pH, with more basic conditions recorded during the closed phase (9.7 ± 0.4) compared to periods of inlet connectivity (8.6 ± 0.4). Using a percentile-based approach (sensu Lemley et al., 2015), inorganic nutrients were classified as being in an overall “Poor” state (eutrophic) for dissolved nitrogen (DIN = 3.3 mg l–1) and phosphorus (DIP = 0.6 mg l–1). The autochthonous origin of DIN (i.e., WWTW inputs) was highlighted by an inverse correlation with salinity (P < 0.001; r = −0.47). Yet, despite surface water DIP concentrations typically increasing from the mouth to the upper reaches, indicative of WWTW inputs, an overall positive association (P < 0.001; r = 0.51) with salinity suggests bottom-water remineralisation processes as a key source.
Biotic Communities
A strong inverse correlation (P < 0.001; r = −0.90) was observed between total cell abundance and the ENS for the phytoplankton community (Figure 4). The ENS for phytoplankton was typically low (ca. 1) throughout the study period due to the prevalence of high-biomass and monospecific (>98% relative abundance [RA]; see Table 1) blooms of Nannochloropsis sp. (Class: Eustigmatophyceae), that ranged in abundance from 0.7 × 106 to 2.5 × 106 cells ml–1. During the semi-closed mouth interval in May 2019, a more diverse phytoplankton community was observed (ENS: 3.3 ± 1.1), despite the proliferation of a Heterosigma akashiwo (Class: Raphidophyceae) bloom (max. 7.7 × 105 cells ml–1) in the stratified middle reaches (Table 1). Accordingly, NMDS demonstrated shifts in phytoplankton community structure between the different mouth conditions (Figure 5A). The presence of a halocline was identified as a key variable explaining (P = 0.01; r2 = 0.34) phytoplankton composition during semi-closed mouth periods, while increased turbidity (P < 0.001; r2 = 0.51) and DIN availability (P = 0.04; r2 = 0.22) best explained assemblages during the closed mouth phase.
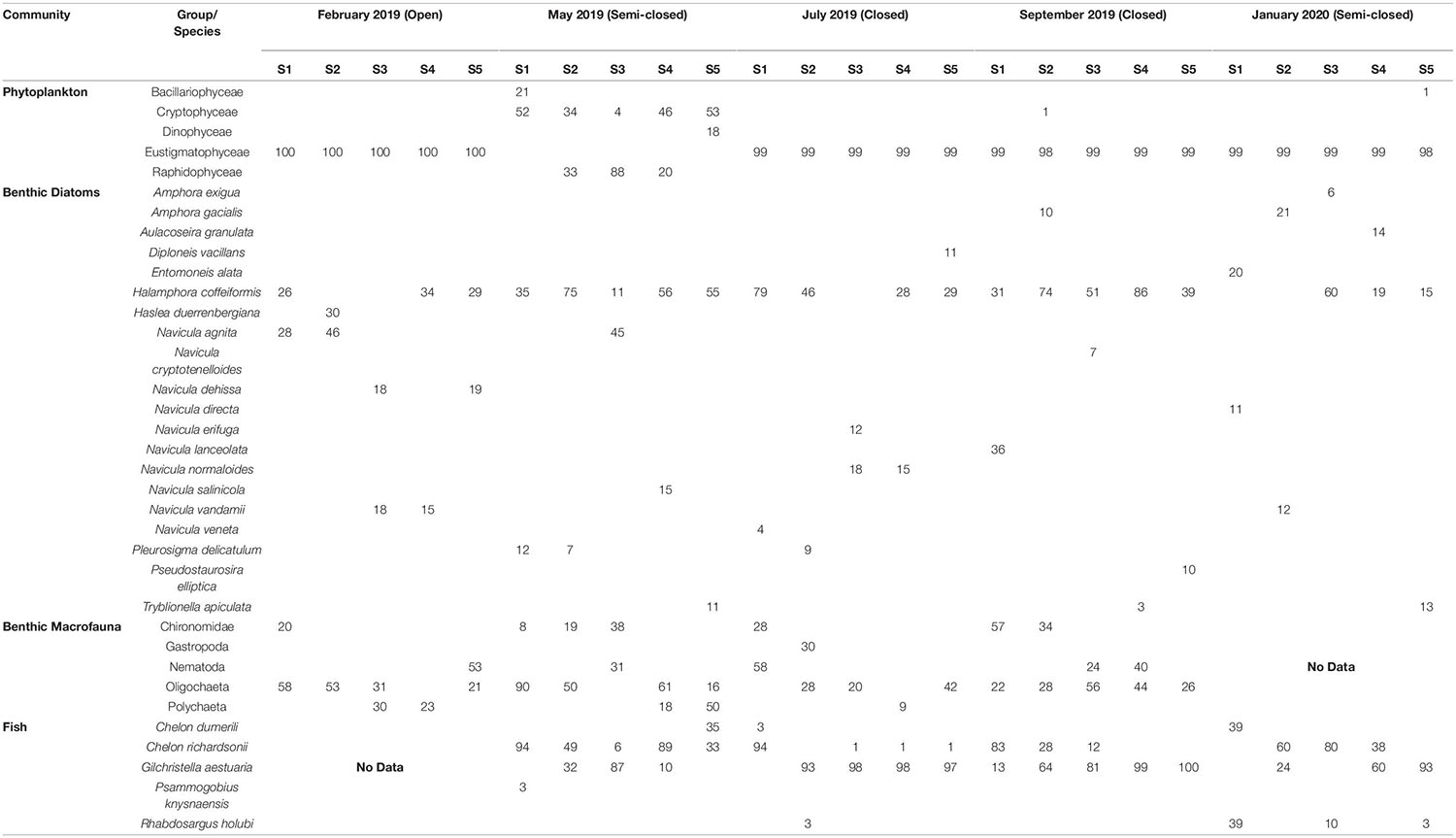
Table 1. Relative abundance (%; RA) of the dominant groups/species (min. ≥ 1%) observed for each biotic community along the length of the Hartenbos Estuary.
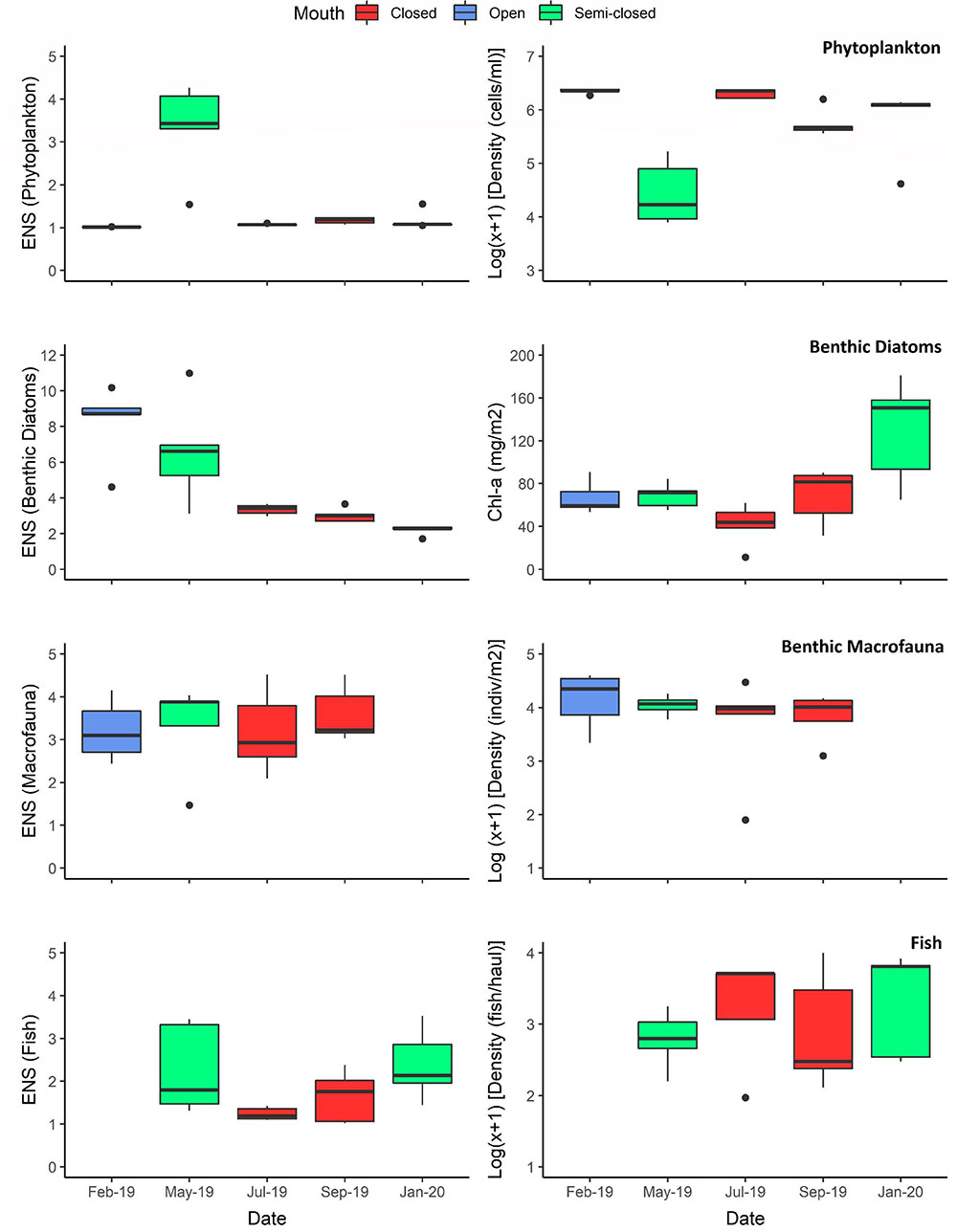
Figure 4. The effective number of species (ENS) for each of the biotic communities–per sampling date–is indicated in the left-hand column, while a quantitative measure for each of these communities is presented in the right-hand column, i.e., phytoplankton (cells per ml), benthic diatoms (microphytobenthic chlorophyll-a), benthic macrofauna (individuals per m2), and ichthyofauna (fish per haul). The degree of estuary connectivity with the marine environment on each sampling date is indicated, i.e., open (blue), semi-closed (green), and closed (red) mouth conditions. Boxplots represent median values (solid line), interquartile range (IQR = 25th and 75th percentiles; box), ± 1.5 × IQR (whiskers), and outlier values.
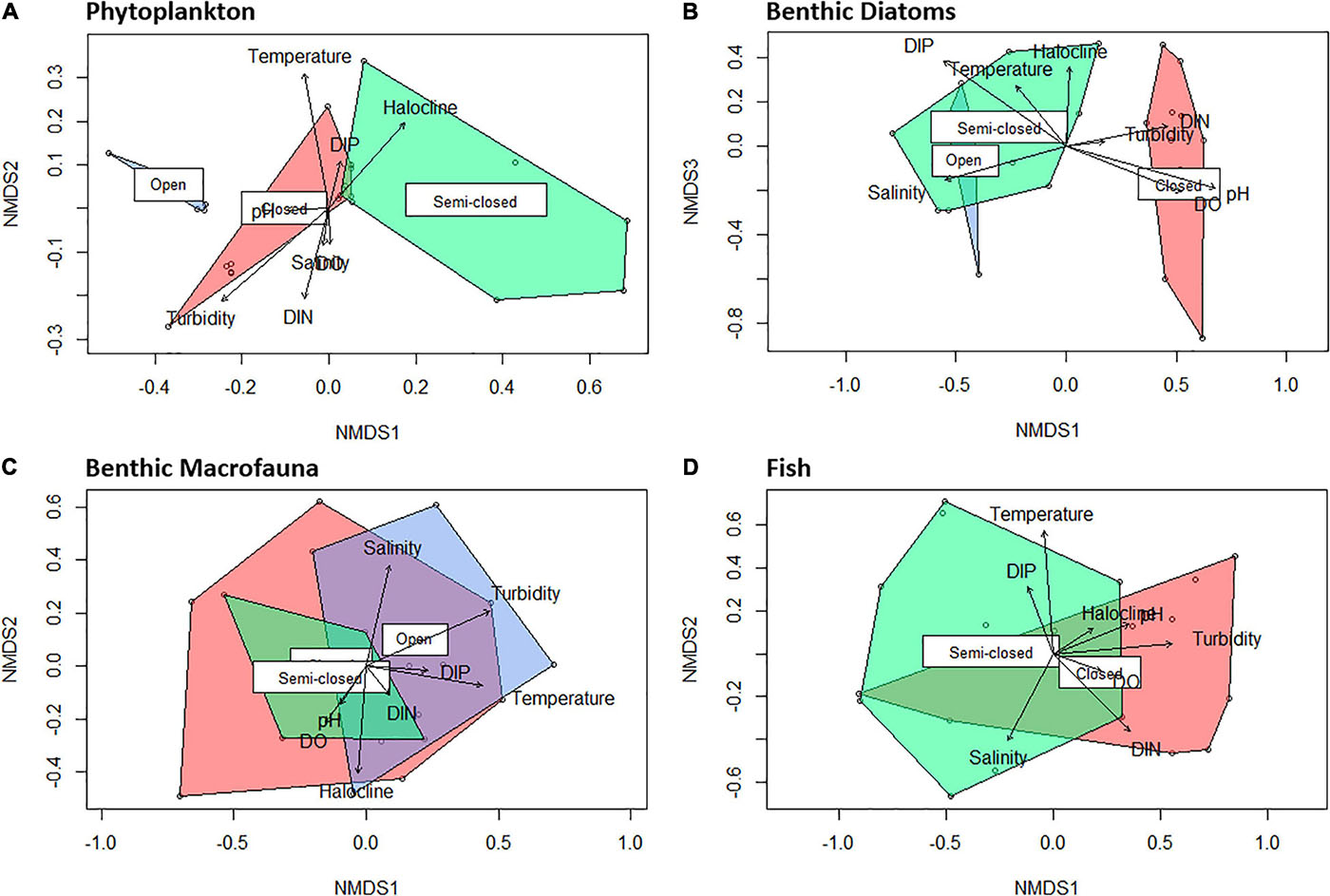
Figure 5. Non-metric multidimensional scaling (NMDS) plots (k = 3) of sampling stations based on phytoplankton [(A); stress = 0.05; non-metric fit, r2 = 0.998], benthic diatoms [(B); stress = 0.12; non-metric fit, r2 = 0.985], benthic macrofauna [(C); stress = 0.08; non-metric fit, r2 = 0.993], and fish [(D); stress = 0.10; non-metric fit, r2 = 0.990] group/species abundance, indicating the distinction between assemblages occurring during open (blue), semi-closed (green), and closed (red) mouth conditions.
Increased salinity (P < 0.001; r2 = 0.51) was highlighted as the environmental vector that best explains benthic diatom community structure during the open and semi-closed mouth phases (Figure 5B). Additionally, the ENS for benthic diatoms showed an inverse association (P = 0.03; r = −0.42) with microphytobenthos (MPB) biomass levels, which ranged from 11.3 to 181.3 mg Chl-a m–2. As such, the ENS for benthic diatoms (Figure 4) declined as tidal exchange became more restricted and MPB biomass increased, i.e., open mouth (8.2 ± 2.1) > semi-closed mouth (4.4 ± 3.0) > closed mouth (3.2 ± 0.4). During closed mouth periods, high pH conditions (P < 0.001; r2 = 0.82) and increased DIN concentrations (P < 0.01; r2 = 0.37) contributed to the reduced diversity of benthic diatom assemblages. The brackish Halamphora coffeiformis species was the most abundant (>50% RA) taxa recorded during all sampling intervals (Table 1). However, the abundance of H. coffeiformis decreased (<35% RA) during the open mouth phase, with other marine (e.g., Navicula agnita, Navicula duerrenbergiana) and brackish (e.g., Navicula dehissa, Navicula vandamii) species presiding in the lower and middle reaches. Diatom species classed as preferring freshwater conditions (e.g., Aulacoseira granulata, Navicula cryptotenelloides, Pseudostaurosira elliptica, Tryblionella apiculata) were confined to the middle and upper reaches during periods of restricted tidal exchange (Table 1), albeit in relatively low abundances (<15% RA).
Benthic macrofauna group diversity demonstrated little variability throughout the sampling period, with similar ENS values recorded during open (3.2 ± 0.7), semi-closed (3.3 ± 1.1), and closed (3.4 ± 0.8) mouth conditions (Figure 4). No correlation (P > 0.1) was observed between ENS and overall density, despite marked increases in benthic macrofauna counts during the open mouth phase (21,236 ± 16,470 indiv. m–2) compared to the closed (10,289 ± 8,318 indiv. m–2) and semi-closed (11,783 ± 4,559 indiv. m–2) periods. Moreover, NMDS highlighted significant overlap (Figure 5C) regarding the composition of macrofaunal assemblages between the designated mouth states, with only turbidity being highlighted as a vector potentially explaining (P = 0.06; r2 = 0.29) community variability. In terms of composition, Oligochaeta were the most abundant and frequently observed group along the length of the estuary on all sampling occasions (Table 1), while Chironomidae were present in the lower to middle reaches throughout. Amphipoda were abundant (>30% RA) in the middle and upper reaches, yet their abundance declined in May 2019 (semi-closed) concomitant with high-biomass accumulations of H. akashiwo in the middle reaches. Polychaeta were abundant (18–50% RA) in the mid- to upper reaches during periods of marine connectivity and declined during the closed mouth phase (<10% RA).
The fish community in the Hartenbos Estuary demonstrated low diversity throughout the study period (Figure 4). That said, marginal increases in the ENS were recorded during semi-closed mouth conditions (2.3 ± 0.9) compared to the closed phase (1.4 ± 0.5). Despite considerable overlap (Figure 5D), fish assemblages were best explained by higher salinity (P = 0.09; r2 = 0.23) and water temperature (P = 0.02; r2 = 0.37) during periods of marine connectivity (semi-closed), while increased turbidity (P = 0.02; r2 = 0.34) was a key factor shaping communities during periods of mouth closure. Mean fish catches (Figure 4) were similar during the closed mouth state (July 2019: 3,320 ± 2,479 fish haul–1; September 2019: 2,743 ± 4,245 fish haul–1) and more variable during the semi-closed sampling dates (May 2019: 818 ± 633 fish haul–1; January 2020: 4,408 ± 3,798 fish haul–1). The total number of fish species recorded on each sampling date ranged from 9 to 15, with a total of 17 throughout the study (Table 2). Of these, taxa categorised as being marine estuarine-dependent were the most frequent (but low in abundance), whilst species belonging to the marine estuarine-opportunist (e.g., southern mullet Chelon richardsonii) and freshwater estuarine-opportunist (Mozambique tilapia Oreochromis mossambicus) guilds were present during each sampling occasion. Two species, the estuarine round herring Gilchristella aestuaria (solely estuarine) and C. richardsonii, were abundant throughout the study period (Table 2). G. aestuaria was dominant (Table 1) in the middle and upper reaches of the estuary, particularly during mesohaline and closed mouth conditions (80–100% RA) (Figure 3). C. richardsonii was prevalent throughout the estuary during periods of marine connectivity, while its distribution was largely confined to the lower reaches during the closed phase. Yet, despite being abundant (33–94% RA) during the semi-closed phase in May 2019, the abundance of C. richardsonii declined (ca. 6% RA) in the middle reaches (Site 3) concomitant with biomass maxima of H. akashiwo. Lastly, the abundance of the Cape stumpnose Rhabdosargus holubi (marine estuarine-dependent) increased in the lower (39% RA) and middle (10% RA) reaches during semi-closed mouth conditions in January 2020.
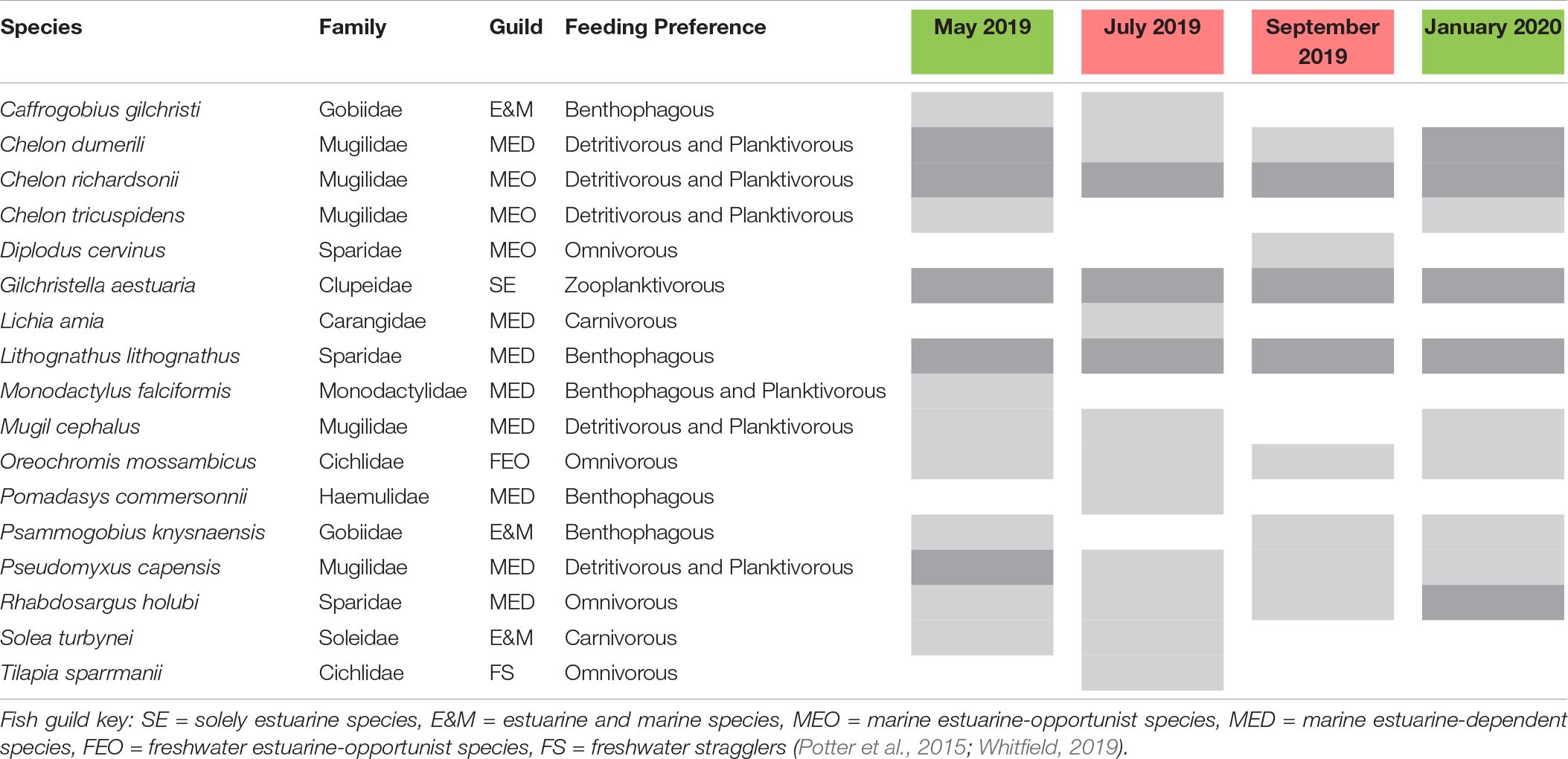
Table 2. List of fish species recorded during each sampling interval and mouth condition (red, closed; green, semi-closed) categorised according to functional guilds, general feeding preferences, and relative abundance (*light grey: >0 but <10% RA; dark grey: ≥10% RA).
Of 37 fish species caught across all three estuaries, 17 were caught in the Hartenbos compared to 36 and 32 in the Klein Brak and Great Brak, respectively (Table 3). One was only caught in the Hartenbos Estuary, the freshwater estuarine-opportunist O. mossambicus, a translocated species. The number of species recorded per haul in the Hartenbos Estuary ranged from 4 to 10 species haul–1, with increased variability observed in the Klein Brak (1–17 species haul–1) and Great Brak (1–15 species haul–1) estuaries. Density ranged from 2,822 fish haul–1 in the Hartenbos to 243 fish haul–1 and 306 fish haul–1 in the Klein Brak and Great Brak, respectively. The large difference in catch between the Hartenbos and the other two estuaries was almost entirely due to the contribution (2,634 fish haul–1) of G. aestuaria and C. richardsonii in that system. This was also the reason that solely estuarine (G. aestuaria) and marine estuarine-opportunist (e.g., C. richardsonii) species comprised 93% of the Hartenbos fish catch compared to 46% in both the Klein Brak and Great Brak. Obligate marine estuarine-dependent and estuarine and marine guilds, in particular benthic species, were poorly represented (<5% of mean abundance) in the Hartenbos Estuary compared to the other two systems.
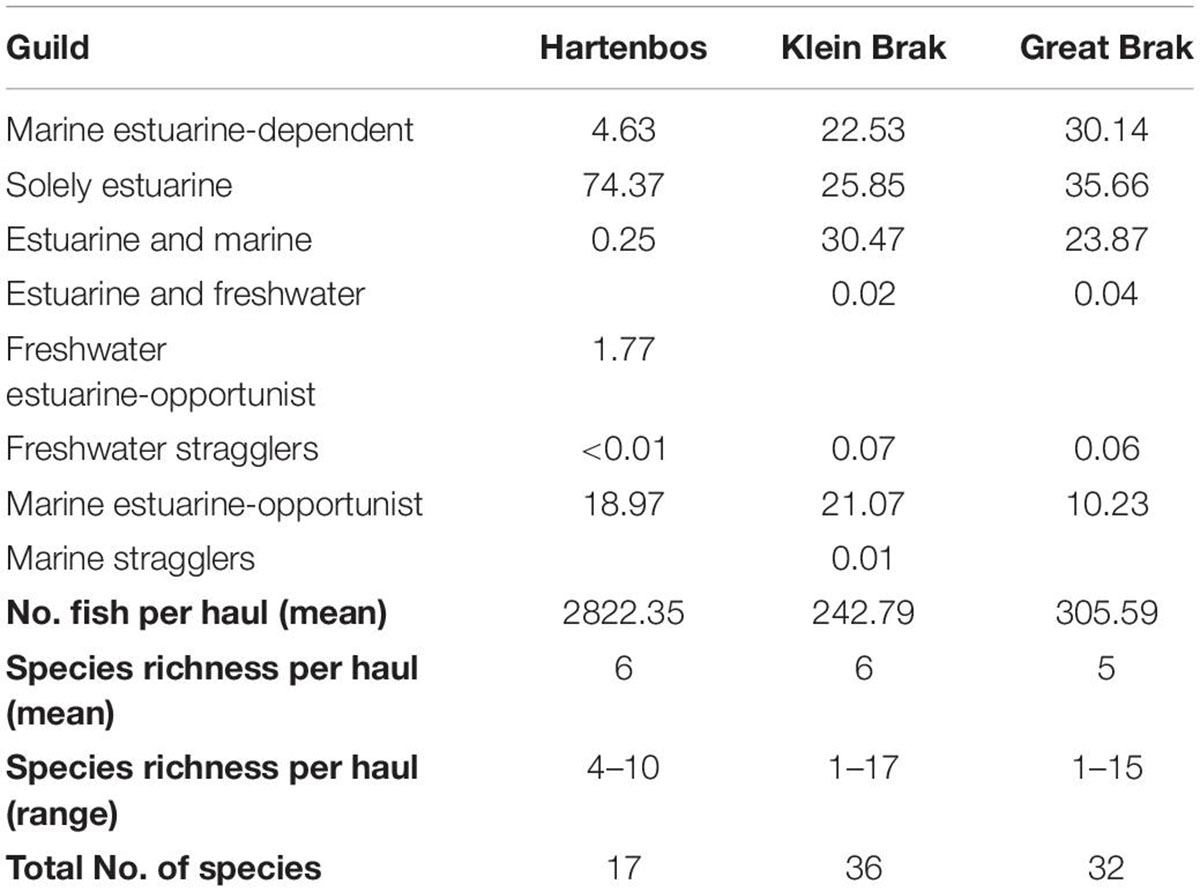
Table 3. Fish guild composition and abundance (%) in the Hartenbos and nearby Klein Brak and Great Brak estuaries sampled over the same study-period.
Discussion
In South Africa, national legislation recognises the inherent socio-economic and ecological importance of estuaries and necessitates the formulation of continually revised estuarine management plans (EMP) that set management objectives and guide effective implementation strategies (Adams et al., 2020). The considerable socio-economic value and long history of anthropogenic perturbations in the Hartenbos Estuary prompted the development of such an EMP, with the second generation thereof completed in 2018 (Mossel Bay Local Municipality, 2018). Some of the key management objectives identified in the Hartenbos EMP were to (1) restore a degree of estuarine functionality and health (e.g., re-establish natural flow regime, enhance tidal exchange), (2) improve water quality (e.g., WWTW discharges), (3) effectively manage mouth interventions, and (4) promote research and monitoring. Understanding baseline conditions and being cognisant of the fact that these shift with concurrent global change pressures is critical to establishing the efficacy of management measures in achieving these objectives (Duarte et al., 2009, 2015).
Artificial breaching of the Hartenbos Estuary mouth is the most frequently implemented practice geared toward addressing the EMP management objectives. The near bimonthly frequency of mechanical breaching highlighted in this study is necessitated by the continuous supply of nutrient-rich effluent discharges from the Hartenbos WWTW (Lemley et al., 2014). These WWTW inflows have facilitated the predominance of mesohaline and polyhaline salinity conditions (5–30) during all mouth states and represent a loss of the marine and hypersaline states that characterised the estuary prior to the WWTW becoming operational in 1986 (Bickerton, 1982). Additionally, augmented flow regimes have resulted in aseasonal mouth breaching at low water levels (i.e., to protect low-lying developments) that has led to increased sedimentation, restricted tidal exchanges, and premature mouth closure. This reduced flushing potential is further confounded by the loss of natural hydrodynamic variability that ensued after the construction of the upstream dam in 1970 (i.e., dam capacity exceeds mean annual runoff). The loss of freshwater inflow, together with steady-state WWTW inputs and premature breaching, have also impacted the seasonal recruitment signals to the marine environment. Such conditions culminate in an ecosystem with high accumulation potential and increased vulnerability to anthropogenic pressures (Warwick et al., 2018). Accordingly, the Hartenbos Estuary was shown to preside in a hypereutrophic state throughout this study, as evidenced by persistent and excessive (1) in-situ inorganic nutrient concentrations, (2) dissolved oxygen and pH fluctuations, (3) phytoplankton blooms, and (4) low trophic biodiversity. Despite partial restoration of the salinity gradient during periods of marine connectivity, increased tidal exchange only elicited responses in primary producer communities (increased diversity, community shifts), while consumer communities were less responsive. The severity of this state shift is highlighted by previous studies (James and Harrison, 2008; Lemley et al., 2014, 2015) that reported similar trajectories of ecological degradation in the system. This heavily utilised and managed estuary serves as a useful case-study for other comparable estuaries globally facing similar pressures.
High-biomass phytoplankton blooms (>100 μg Chl-a l–1) are at the forefront of eutrophication-induced symptoms in the Hartenbos Estuary. During this study, dense and largely monospecific (ENS ∼ 1) HABs of Nannochloropsis sp. dominated during well-mixed and/or prolonged water residency conditions, with peak biomass maxima (>1,000 μg Chl-a l–1) observed during the closed phase. Similar blooms of Nannochloropsis sp., albeit to a lesser extent, have been recorded in an intermittently open Australian estuary during the closed phase characterised by meso- to polyhaline conditions and significant internal nutrient loading (Scanes et al., 2020). Notably, despite enhanced tidal exchanges promoting increased phytoplankton diversity (ENS > 3) in the Hartenbos Estuary, a shift to a temporary alternate state was evidenced by an exceptional HAB of H. akashiwo (ca. 2,200 μg Chl-a l–1) in the polyhaline and stratified (i.e., well-defined halocline) middle reaches. This species has been shown to cause extensive, and potentially recurrent, HABs in brackish (meso- to polyhaline) and stratified waters of numerous predominantly open estuaries along the south coast of South Africa (Lemley et al., 2017). Nannochloropsis sp. and H. akashiwo are both considered non-toxic species, instead imparting harmful effects on the ecosystem indirectly through oversynthesis of growth phase by-products (e.g., reactive oxygen species, mucus) and facilitation of unfavourable abiotic conditions. During this study, the accumulation and subsequent collapse of an extensive Nannochloropsis sp. bloom caused a drastic shift in dissolved oxygen (supersaturated to near-anoxic) and pH (>10) levels that culminated in a mass fish mortality event on September 2, 2019. This prompted a breaching event at low water levels (1.78 m MSL) 2 days later (Figure 2), followed by rapid mouth closure and recolonisation of dense Nannochloropsis sp. blooms. The effects of the mucus-forming H. akashiwo bloom observed during this study were evidenced by supersaturated surface waters (>17 mg l–1), hypoxic bottom-waters (<2 mg l–1), reduced amphipod abundance, and HAB avoidance by the otherwise abundant marine estuarine-opportunist, C. richardsonii. Thus, although artificial breaching facilitates shifts in phytoplankton community composition, the continuous supply of nutrient-rich WWTW discharges maintains undesirable conditions irrespective of estuarine mouth condition.
Benthic diatom assemblages in the Hartenbos Estuary were primarily structured by salinity and water residency fluctuations. Periods of enhanced tidal exchange were characterised by increased species diversity, presence of marine taxa (lower reaches), and microphytobenthic (MPB) biomass dilution. Contrastingly, the onset of mouth closure elicited a reduction in diversity, MPB biomass accumulation, and the increased prevalence of freshwater species (upper reaches). Yet despite these responses, the overall degraded state of the benthic diatom community in the Hartenbos Estuary was exemplified by the persistently low diversity (ENS < 8, which equates to Shannon H′ < 2) and predominance of the brackish H. coffeiformis species throughout the study period. Moreover, high pH levels (>9) and increased internal nutrient loading (i.e., driven by extreme oxygen fluctuations; Bartoli et al., 2021) stemming from high-biomass HABs of Nannochloropsis sp. are likely responsible for contributing to the low benthic diatom diversity (ENS < 4) observed during the closed mouth phase. The hypereutrophic nature of the system is highlighted by the elevated benthic diatom diversity recorded in two less-impacted neighbouring temporarily closed estuaries, namely the Klein Brak (H′ > 3; oligotrophic) and Great Brak (H′ > 2.5; mesotrophic) estuaries (Lemley et al., 2015). Current pressures on the Hartenbos Estuary, such as WWTW inputs and premature mouth breaching, limit the extent of tidal exchanges and promote the dominance of opportunistic nutrient-tolerant taxa, thus, constraining benthic diatom diversity and colonisation by marine/freshwater taxa. These conditions may alter trophic relationships between benthic microalgae and primary consumers, with deposit-feeding benthic macrofauna and ichthyological taxa being limited by the dominance of less palatable and potentially harmful (Lassus et al., 2016) diatom species (e.g., H. coffeiformis).
Benthic macrofauna displayed consistently high density (mean > 10,000 indiv. m–2) and low group diversity (ENS < 3.5) throughout the study period. These observations support the hypothesis that small microtidal and intermittently closed estuaries are dominated by many individuals of few species (Teske and Wooldridge, 2001, 2003; Coelho et al., 2015) that are broadly euryhaline tolerant (Wooldridge and Bezuidenhout, 2016). Yet, similar to microalgal communities, the relatively stable community composition observed here represents opportunistic taxa with a high level of tolerance to adverse environmental conditions (e.g., extreme oxygen fluctuations, HABs). The ubiquitous presence of oligochaetes, chironomids, and polychaetes in this study is supported by the expected shift toward deposit feeding taxa that are tolerant of unstable eutrophic conditions characterised by high organic loads and instances of oxygen depletion. Their persistence and the near absence of burrowing crustaceans, such as Kraussillichirus kraussi (common sandprawn) that were previously abundant (Bickerton, 1982), suggests that recovery periods (tidal intrusion) are too short, reproduction is inhibited by low salinity (mesohaline), and that compensatory recruitment from the sea is limited. Similar observations from temporarily closed systems have been reported in the hypereutrophic Salgados Lagoon (Portugal; Coelho et al., 2015) and eutrophic Broke Inlet (Australia; Tweedley et al., 2012). Amphipods are considered sensitive to anthropogenic perturbations and are generally responsive to state changes, with increased abundance expected in healthier ecosystems (Dauvin, 2018). Thus, the constrained abundance of amphipods observed in this study–particularly during the HAB of H. akashiwo–is further suggestion of the degraded state of the Hartenbos Estuary. Mouth management practices that prompt salinity and tidal variability were shown to have little effect on benthic macrofauna assemblages, suggesting that other factors (e.g., sediment properties) need to be considered to explain fine-scale variability (Teske and Wooldridge, 2003). At the broad-scale, however, the relatively consistent community composition does not seem to mirror abiotic state shifts in the short term but instead differs when compared to similar South African ecosystems. For example, amphipods constitute the most abundant group in the oligotrophic East Kleinemonde Estuary during open and closed mouth conditions, while oligochaetes are largely absent (Wooldridge and Bezuidenhout, 2016). This reflects an overall state shift in the Hartenbos Estuary rather than a response at the level of management interventions.
Ichthyofaunal diversity and abundance are governed by mouth dynamics in temporarily closed estuaries and typically display lower diversity and increased abundance of estuarine resident species compared to permanently open systems (James et al., 2007). The fish community in the Hartenbos Estuary was characterised by low overall diversity, with marginal increases observed during semi-closed (ENS: 2.3 ± 0.9) compared to closed (ENS: 1.4 ± 0.5) mouth phases. A total of 17 species representing nine families were recorded during this study, with Mugilidae (5 spp.) and marine estuarine-dependant (8 spp.) taxa being the most numerically dominant (Table 2). G. aestuaria (solely estuarine) and C. richardsonii (marine-estuarine opportunist) dominated the catches in this study. Overall species richness was typically low throughout the study period (n ≤ 10), with the exception of the first closed mouth sampling interval in July 2019 (n = 15). This is similar to historical records for the Hartenbos Estuary reported in 1981 (n = 12; open mouth) and 1994 (n = 9; closed mouth) (Bickerton, 1982; James and Harrison, 2008). Despite little change to the core community (i.e., G. aestuaria, C. richardsonii, Chelon dumerili, Lithognathus lithognathus, Mugil cephalus, Psammogobius knysnaensis), notable shifts in the composition of ichthyofaunal assemblages are evident when comparing these historical surveys with the current study. For example, the speckled sandgoby P. knysnaensis (estuarine and marine) and white steenbras L. lithognathus (marine estuarine-dependent) are now in very low numbers (Table 2). Both are benthic feeders but P. knysnaensis is also commensal in K. kraussi burrows, which have disappeared from most of the system. Overall, the abundance and occurrence of benthic species were much lower in the Hartenbos compared to the nearby Klein Brak and Great Brak estuaries, most likely due to the physiological stress induced by recurrent instances of bottom-water hypoxia. Recruitment failure of benthic species was highlighted by the absence of Solea turbynei (blackhand sole) juveniles, with only limited numbers of adults caught in the more oxygenated shallows (<20 cm depth).
The shift toward meso- and polyhaline conditions in recent times due to frequent/premature mouth breaching and continuous WWTW inflows has resulted in a loss of marine estuarine-opportunist species (e.g., Diplodus capensis, Galeichthys feliceps) that were recorded prior to the enactment of these pressures (Bickerton, 1982). That said, marine estuarine-opportunist Mugilidae, notably C. richardsonii, are now dominant. This species and the freshwater estuarine-opportunist O. mossambicus typically capitalize on the competitive advantage provided by eutrophication and other environmental perturbations (Lamberth et al., 2010; Viskich et al., 2016). Perhaps of more concern is the complete absence of the estuarine resident Atherina breviceps (Cape silverside) species that previously dominated catches during closed mouth conditions in the 1990s, i.e., subsequent to the WWTW becoming operational (James and Harrison, 2008). This represents a potentially significant alteration to the trophic structure of the Hartenbos Estuary given the importance of A. breviceps as a fodder fish for higher trophic levels. The growth and spawning success of A. breviceps adults is largely dependent on the availability of suitable habitat (clear waters, submerged macrophytes) and prey (amphipods) (Whitfield, 2019). Thus, the increased frequency and magnitude of HABs are likely responsible for shaping an environment that is unconducive (e.g., highly turbid, organic rich, hypoxic) to the survival of this species, instead encouraging it to complete its life cycle in the nearshore environment. The absence of A. breviceps is also likely responsible, in part, for the high abundance of G. aestuaria which no longer has to compete for resources. The latter is a fast growing, short-lived species that has a greater chance of completing its life cycle during the brief periods of improved water quality observed within the system. Furthermore, small-bodied and phytophyllic species, such as G. aestuaria, are more resilient to poor water quality (Van Treeck et al., 2020). Current catchment management practices have dampened the flushing potential in the Hartenbos Estuary, resulting in excessive sedimentation that reduces the nursery value of the system by limiting suitable shallow littoral zones (Whitfield et al., 2012). For example, the marine estuarine-dependent R. holubi typically inhabits shallow littoral habitats, thus, its limited residency in the Hartenbos Estuary is indicative of sedimentation and marine connectivity issues. The degraded state of the estuary is highlighted by the increased overall species richness (n = 36 and 32, respectively) and abundance of R. holubi in the nearby Klein Brak and Great Brak estuaries. Concurrent sampling yielded 50–70 fish haul–1 and 60–65% occurrence of R. holubi in these systems. Overall, marine estuarine-dependent, estuarine and marine, estuarine and freshwater, and marine straggler species were functionally absent (<5% RA) in the Hartenbos Estuary compared to the Klein Brak and Great Brak estuaries (>50% RA).
The loss of natural hydrodynamic variability (e.g., dam impoundment) and the continuous supply of excessive WWTW inputs (flow and nutrients) has necessitated frequent and premature mouth breaching interventions to meet socio-ecological requirements. From a broad ecological perspective, these practices have culminated in an ecosystem void of typical abiotic and biotic fluctuations associated with small microtidal and intermittently closed estuaries. Despite distinct seasonal water temperature fluctuations (14–28°C), the continuous input of nutrient-rich WWTW discharges has resulted in aseasonal freshwater inflow, nutrient loading, and phytoplankton community patterns that, in turn, act synergistically to hinder the efficacy of faunal recruitment periods (e.g., fish in spring/summer). The loss of natural ecosystem functioning in the Hartenbos Estuary is further evidenced by the loss of the marine-dominated, and occasionally hypersaline (salinity > 38), conditions that characterised the system prior to the construction of the WWTW (Bickerton, 1982). The low diversity and shift to undesirable alternate states observed for each of the biotic communities in the Hartenbos Estuary demonstrates the magnitude and severity of current pressures on the system. For example, hypereutrophic conditions and restricted tidal exchanges were shown to favour the near year-round dominance of only a few opportunistic species/groups tolerant to adverse conditions (e.g., Nannochloropsis sp., H. coffeiformis, oligochaetes, G. aestuaria, and C. richardsonii). The general unresponsiveness of selected indicator parameters to current mitigation efforts (mouth manipulation) suggests that management objectives (both ecological and socio-economic) set out in the Hartenbos EMP are not being met. In the short-term, the mouth management protocol could be improved by ensuring that breaching events only occur at sufficient water levels (>2 m MSL) and in conjunction with spring tides to ensure maximum flushing and prolonged periods of tidal exchange. Additionally, nutrient levels observed in this study (Figure 3) reflect concentrations already subject to significant abiotic (e.g., tidal dilution) and biological (e.g., phytoplankton uptake) transformations. Thus, continuous monitoring of WWTW discharges (i.e., volumes, nutrient concentrations) is required to better understand the nutrient dynamics and stoichiometry of inflows to the Hartenbos Estuary and, subsequently, facilitate informed decision-making. However, a catchment-scale approach is needed to improve, and potentially restore, ecosystem functionality (e.g., water quality, flow regimes). A reduction in the volume and nutrient loads entering the estuary directly from the Hartenbos WWTW should serve as the core focus. This can be achieved through (1) effluent diversion through artificial wetlands before entering the estuary, (2) reuse of outflows for non-consumptive purposes (e.g., irrigation, industrial), (3) treatment upgrades, and (4) diversion of wastewater offshore (Adams et al., 2020; Taylor et al., 2020). At present, a quarter (2,000 m3 d–1) of the daily WWTW outflow is allocated for reuse as irrigation water on agricultural lands. This represents a positive step toward reducing the eutrophication pressure, although, management authorities should endeavour to divert all WWTW inputs away from the estuary to facilitate its recovery from potentially long-lasting consequences (e.g., legacy nutrients stored in the sediments) associated with surplus nutrient loading. Finally, the removal of the upstream Hartebeeskuil Dam (i.e., unpotable) should be considered a long-term management goal given that it would restore an element of natural hydrological variability and reduce sedimentation rates in the downstream estuary. These insights provide an important platform in terms of management plans and interventions of estuaries facing eutrophication and increased prevalence of connectivity loss to the ocean through global change pressures (e.g., drought, nutrient enrichment, water abstraction). It is therefore imperative that a long-term ecosystem-level framework is contextualised rather than focusing on short-term interventions (artificial breaching) if effective ecosystem health from a socio-ecological perspective is to be realised.
Data Availability Statement
The original contributions presented in the study are included in the article/Supplementary Material, further inquiries can be directed to the corresponding author/s.
Ethics Statement
The animal study was reviewed and approved by Department of Forestry, Fisheries and Environment (DFFE), South Africa.
Author Contributions
DL conceptualized the study, wrote the original draft, performed the statistical analyses, created the figures, and interpreted the results. SL, MN, and GR were responsible for components of data collection and analyses, contributed to the interpretation of results, and reviewed the manuscript. LN, WM, and JA contributed to the article by providing comments and edits to the manuscript. JA was also responsible for providing the necessary funding. All authors contributed to the article and approved the submitted version.
Funding
The National Research Foundation (NRF) of South Africa is thanked for providing postdoctoral fellowship funding to DL (Grant Number: 120709) and MN (Grant Number: 132717). This work is based on the research supported by the DSI/NRF Research Chair in Shallow Water Ecosystems (UID: 84375) that provided the necessary funding to undertake field surveys and laboratory analyses. LN was funded through the Coastal Systems CSIR DSI Parliamentary Grant (PG). Opinions expressed, and conclusions arrived at are those of the authors and do not necessarily represent the official views of the funding agency.
Conflict of Interest
The authors declare that the research was conducted in the absence of any commercial or financial relationships that could be construed as a potential conflict of interest.
Acknowledgments
The authors would like to thank all the field and laboratory assistants, as well as Neil van Wyk from the Department of Water and Sanitation (DWS) for providing the water level data.
Supplementary Material
The Supplementary Material for this article can be found online at: https://www.frontiersin.org/articles/10.3389/fmars.2021.688933/full#supplementary-material
Supplementary Table 1 | Spatial variability of selected abiotic parameters along the length of the Hartenbos Estuary on each sampling occasion. The degree of vertical salinity (halocline) and temperature (thermocline) stratification at each station is calculated by subtracting bottom-water values from surface values (∗positive values indicate elevated bottom-water values compared to surface waters, and vice versa).
References
Adams, J. B., Taljaard, S., van Niekerk, L., and Lemley, D. A. (2020). Nutrient enrichment as a threat to the ecological resilience and health of South African microtidal estuaries. Afr. J. Aquat. Sci. 45, 23–40. doi: 10.2989/16085914.2019.1677212
Bartoli, M., Benelli, S., Lauro, M., Magri, M., Vybemaite-Lubiene, I., and Petkuviene, J. (2021). Variable oxygen levels lead to variable stoichiometry of benthic nutrient fluxes in a hypertrophic estuary. Estuar. Coast. 44, 689–703. doi: 10.1007/s12237-020-00786-1
Bate, G. C., and Heelas, B. V. (1975). Studies on the nitrate nutrition of two indigenous Rhodesian grasses. J. Appl. Ecol. 12, 941–952.
Bate, G. C., Smailes, P. A., and Adams, J. B. (2013). Epipelic diatoms in the estuaries of South Africa. Water SA 39, 105–118. doi: 10.4314/wsa.v39i1.11
Bickerton, I. B. (1982). Estuaries of the Cape Part II: Synopses of Available Information on Individual Systems. Report No. 11 Hartenbos (CMS1). eds A. E. F. Heydorn and J. R. Grindley (Stellenbosch: CSIR Research Report 410).
Boesch, D. F. (2019). Barriers and bridges in abating coastal eutrophication. Front. Mar. Sci. 6:123. doi: 10.3389/fmars.2019.00123
Bricker, S. B., Longstaff, B., Dennison, W., Jones, A., Boicourt, K., Wicks, C., et al. (2008). Effects of nutrient enrichment in the nation’s estuaries: a decade of change. Harmful Algae 8, 21–32. doi: 10.1016/j.hal.2008.08.028
Brito, A., Newton, A., Tett, P., and Fernandes, T. F. (2009). Development of an optimal methodology for the extraction of microphytobenthic chlorophyll. J. Int. Environ. Appl. Sci. 4, 42–54.
Chase, J. M., and Knight, T. M. (2013). Scale-dependent effect sizes of ecological drivers on biodiversity: why standardised sampling is not enough. Ecol. Lett. 16, 17–26. doi: 10.1111/ele.12112
Cloern, J. E. (2001). Our evolving conceptual model of the coastal eutrophication problem. Mar. Ecol. Prog. Ser. 210, 223–253. doi: 10.3354/meps210223
Cloern, J. E., Jassby, A. D., Schraga, T. S., Nejad, E., and Martin, C. (2017). Ecosystem variability along the estuarine salinity gradient: examples from long-term study of San Francisco Bay. Limnol. Oceanogr. 62, S272–S291. doi: 10.1002/lno.10537
Coelho, S., Pérez-Ruzafa, A., and Gamito, S. (2015). Effects of organic pollution and physical stress on benthic macroinvertebrate communities from two intermittently closed and open coastal lagoons (ICOLLs). Est. Coast. Shelf Sci. 167, 276–285. doi: 10.1016/j.ecss.2015.08.013
Dauvin, J.-C. (2018). Twenty years of application of Polychaete/Amphipod ratios to assess diverse human pressures in estuarine and coastal marine environments: a review. Ecol. Indic. 95, 427–435. doi: 10.1016/j.ecolind.2018.07.049
Department of Water Affairs (2012). Green Drop Progress Report, Chap. 13. Western Cape Province: Department of Water Affairs, 398–450.
Duarte, C. M., Borja, A., Carstensen, J., Elliott, M., Krause-Jensen, D., and Marbà, N. (2015). Paradigms in the recovery of estuarine and coastal ecosystems. Estuar. Coast. 38, 1202–1212. doi: 10.1007/s12237-013-9750-9
Duarte, C. M., Conley, D. J., Carstensen, J., and Sánchez-Camacho, M. (2009). Return to Neverland: shifting baselines affect eutrophication restoration targets. Estuar. Coast. 32, 29–36. doi: 10.1007/s12237-008-9111-2
Duarte, C. M., and Krause-Jensen, D. (2018). Intervention options to accelerate ecosystem recovery from coastal eutrophication. Front. Mar. Sci. 5:470. doi: 10.3389/fmars.2018.00470
Garmendia, M., Borja, Á, Franco, J., and Revilla, M. (2013). Phytoplankton composition indicators for the assessment of eutrophication in marine waters: present state and challenges within the European directives. Mar. Pollut. Bull. 66, 7–16. doi: 10.1016/j.marpolbul.2012.10.005
Gerwing, T. G., Cox, K., Gerwing, A. M. A., Campbell, L., Macdonald, T., Dudas, S. E., et al. (2020). Varying intertidal invertebrate taxonomic resolution does not influence ecological findings. Est. Coast. Shelf Sci. 232:106516. doi: 10.1016/j.ecss.2019.106516
Glozier, N., Culp, J., and Halliwell, D. (2002). Revised Guidance for Sample Sorting and Sub-Sampling Protocols for EEM Benthic Invertebrate Community Surveys. Saskatoon: National Water Research Institute, Environment Canada.
Human, L. R. D., Snow, G. C., and Adams, J. B. (2016). Responses in a temporarily open/closed estuary to natural and artificial mouth breaching. S. Afr. J. Bot. 10, 39–48. doi: 10.1016/j.sajb.2015.12.002
James, N. C., Cowley, P. D., Whitfield, A. K., and Lamberth, S. J. (2007). Fish communities in temporarily open/closed estuaries from the warm- and cool-temperate regions of South Africa: a review. Rev. Fish. Biol. Fisheries 17, 565–580. doi: 10.1007/s11160-007-9057-7
James, N. C., and Harrison, T. D. (2008). A preliminary survey of the estuaries on the south coast of South Africa, Cape St Blaize, Mossel Bay—Robberg Peninsula, Plettenberg Bay, with particular reference to the fish fauna. Trans. Roy. Soc. S. Afr. 63, 111–127. doi: 10.1080/00359190809519216
Lamberth, S. J., Branch, G. M., and Clark, B. M. (2010). Estuarine refugia and fish responses to a large anoxic, hydrogen sulphide, ‘black tide’ event in the adjacent marine environment. Est. Coast Shelf. Sci. 86, 203–215. doi: 10.1016/j.ecss.2009.11.016
Lassus, P., Chomérat, N., Hess, P., and Nézan, E. (2016). Toxic and harmful microalgae of the World Ocean. Denmark, International Society for the Study of Harmful Algae / Intergovernmental Oceanographic Commission of UNESCO. IOC Manuals and Guides, 68 (Bilingual English/French). Harmful Algae. 63:203.
Le Moal, M., Gascuel-Odoux, C., Ménesguen, A., Souchon, Y., Étrillard, C., Levain, A., et al. (2019). Eutrophication: a new wine in an old bottle? Sci. Total Environ. 651, 1–11. doi: 10.1016/j.scitotenv.2018.09.139
Lemley, D. A., and Adams, J. B. (2019). “Eutrophication,” in Encyclopedia of Ecology, Second Edn, ed. B. Fath (Oxford: Elsevier), 86–90. doi: 10.1016/B978-0-12-409548-9.10957-1
Lemley, D. A., Adams, J. B., Rishworth, G. M., and Bouland, C. (2019). Phytoplankton responses to adaptive management interventions in eutrophic urban estuaries. Sci. Total Environ. 6693:133601. doi: 10.1016/j.scitotenv.2019.133601
Lemley, D. A., Adams, J. B., and Taljaard, S. (2017). Comparative assessment of two agriculturally-influenced estuaries: similar pressure, different response. Mar. Pollut. Bull. 117, 136–147. doi: 10.1016/j.marpolbul.2017.01.059
Lemley, D. A., Adams, J. B., Taljaard, S., and Strydom, N. A. (2015). Towards the classification of eutrophic condition in estuaries. Est. Coast. Shelf Sci. 164, 221–232. doi: 10.1016/j.ecss.2015.07.033
Lemley, D. A., Taljaard, S., Adams, J. B., and Strydom, N. A. (2014). Nutrient characterisation of river inflow into the estuaries of the Gouritz Water Management Area. South Africa. Water SA 40, 687–698. doi: 10.4314/wsa.v40i4.14
Malone, T. C., and Newton, A. (2020). The globalization of cultural eutrophication in the coastal ocean: causes and consequences. Front. Mar. Sci. 7:670. doi: 10.3389/fmars.2020.00670
Mossel Bay Local Municipality (2018). Hartenbos Estuarine Management Plan: Second Generation. East London: EOH Coastal & Environmental Services.
Nixon, S. W. (1995). Coastal marine eutrophication: a definition, social causes, and future concerns. Ophelia 4, 199–219. doi: 10.1080/00785236.1995.10422044
Nusch, E. A. (1980). Comparison of different methods for chlorophyll and phaeopigment determination. Arch. Hydrobiol. Beih. Ergeb. Limnol. 14, 14–36.
O’Brien, A., Townsend, K., Hale, R., Sharley, D., and Pettigrove, V. (2016). How is ecosystem health defined and measured? A critical review of freshwater and estuarine studies. Ecol. Indic. 69, 722–729. doi: 10.1016/j.ecolind.2016.05.004
Parsons, T. R., Maita, Y., and Lalli, C. M. (1984). A manual of Chemical and Biological Methods for Seawater Analysis. New York, NY: Pergamon Press, 173.
Pillay, D., and Perissinotto, R. (2008). The benthic macrofauna of the St. Lucia Estuary during the 2005 drought year. Est. Coast. Shelf Sci. 77, 35–46. doi: 10.1016/j.ecss.2007.09.004
Potter, I. C., Tweedley, J. R., Elliott, M., and Whitfield, A. K. (2015). The ways in which fish use estuaries: a refinement and expansion of the guild approach. Fish Fish. 16, 230–239. doi: 10.1111/faf.12050
R Core Team (2020). R: A Language and Environment for Statistical Computing. Vienna: R Foundation for Statistical Computing.
Ribeiro, L., Brotas, V., Hernández-Fariñas, T., Jesus, B., and Barillé, L. (2020). Assessing alternative microscopy-based approaches to species abundance description of intertidal diatom communities. Front. Mar. Sci 7:36. doi: 10.3389/fmars.2020.00036
Rishworth, G. M., Adams, J. B., Bird, M. S., Carrasco, N. K., Dänhardt, A., Dannheim, J., et al. (2020). Cross-continental analysis of coastal biodiversity change. Phil. Trans. R. Soc. B 375:20190452. doi: 10.1098/rstb.2019.0452
Scanes, P. R., Ferguson, A., and Potts, J. (2020). Catastrophic events and estuarine connectivity influence presence of aquatic macrophytes and trophic status of intermittently-open coastal lagoons in eastern Australia. Est. Coast. Shelf Sci. 238:106732. doi: 10.1016/j.ecss.2020.106732
Snow, G. C., Bate, G. C., and Adams, J. B. (2000). The effects of a single freshwater release into the Kromme Estuary. 2: microalgal response. Water SA 26, 301–310.
Snow, G. C., and Taljaard, S. (2007). Water quality in South African temporarily open/closed estuaries: a conceptual model. Afr. J. Aquat. Sci. 32, 99–111. doi: 10.2989/AJAS.2007.32.2.1.198
Taylor, D. I., Oviatt, C. A., Giblin, A. E., Tucker, J., Diaz, R. J., and Keay, K. (2020). Wastewater input reductions reverse historic hypereutrophication of Boston Harbor. USA. Ambio. 49, 187–196. doi: 10.1007/s13280-019-01174-1
Taylor, G. C., de la Rey, P. A., and van Rensburg, L. (2005). Recommendations for the collection, preparation and enumeration of diatoms from riverine habitats for water quality monitoring in South Africa. Afr. J. Aquat. Sci. 30, 65–75. doi: 10.2989/16085910509503836
Teske, P. R., and Wooldridge, T. H. (2001). A comparison of the macrobenthic faunas of permanently open and temporarily open/closed South African estuaries. Hydrobiologia 464, 227–243. doi: 10.1023/A:1013995302300
Teske, P. R., and Wooldridge, T. H. (2003). What limits the distribution of subtidal macrobenthos in permanently open and temporarily open/closed South African estuaries? Salinity vs. sediment particle size. Est. Coast. Shelf Sci. 57, 225–238.
Tweedley, J. R., Warwick, R. M., Valesini, F. J., Platell, M. E., and Potter, I. C. (2012). The use of benthic macroinvertebrates to establish a benchmark for evaluating the environmental quality of microtidal, temperate southern hemisphere estuaries. Mar. Pollut. Bull. 64, 1210–1221. doi: 10.1016/j.marpolbul.2012.03.006
van Niekerk, L., Adams, J. B., James, N. C., Lamberth, S. J., MacKay, C. F., Turpie, J. K., et al. (2020). An estuary ecosystem classification encompassing biogeography, size and types of diversity that supports estuarine protection, conservation and management. Afr. J. Aquat. Sci. 45, 199–216. doi: 10.2989/16085914.2019.1685934
van Niekerk, L., Adams, J. B., Lamberth, S. J., MacKay, C. F., Taljaard, S., Turpie, J. K., et al. (2019). South African National Biodiversity Assessment 2018: Technical Report. Volume 3: Estuarine Realm. CSIR report number CSIR/SPLA/EM/EXP/2019/0062/A. Pretoria: South African National Biodiversity Institute.
van Niekerk, L., Taljaard, S., Adams, J. B., Fundisi, D., Huizinga, P., Lamberth, S. J., et al. (2015). Desktop Provisional Eco-Classification of The Temperate Estuaries in South Africa. WRC Report No.2187/1/15. Pretoria: Water Research Commission.
Van Treeck, R., Van Wichelen, J., and Wolter, C. (2020). Fish species sensitivity classification for environmental impact assessment, conservation and restoration planning. Sci. Total Environ. 708:135173. doi: 10.1016/j.scitotenv.2019.135173
Viskich, M., Griffiths, C. L., Erasmus, C., and Lamberth, S. (2016). Long-term physical, chemical and biological changes in a small, urban estuary. Afr. J. Mar. Sci. 38, 23–37. doi: 10.2989/1814232X.2015.1116466
Warwick, R. M., Tweedley, J. R., and Potter, I. C. (2018). Microtidal estuaries warrant special management measures that recognize their critical vulnerability to pollution and climate change. Mar. Pollut. Bull. 135, 41–46. doi: 10.1016/j.marpolbul.2018.06.062
Whitfield, A. K. (2019). Fishes of southern African estuaries: from species to systems. Afr. J. Aqu. Sci. 45, 229–230.
Whitfield, A. K., Bate, G. C., Adams, J. B., Cowley, P. D., Froneman, P. W., Gama, P. T., et al. (2012). A review of the ecology and management of temporarily open/closed estuaries in South Africa, with particular emphasis on river flow and mouth state as primary drivers of these systems. Afr. J. Mar. Sci. 34, 163–180. doi: 10.2989/1814232X.2012.675041
Keywords: eutrophication, harmful algal blooms, hypoxia, mouth management, nutrient loading
Citation: Lemley DA, Lamberth SJ, Manuel W, Nunes M, Rishworth GM, van Niekerk L and Adams JB (2021) Effective Management of Closed Hypereutrophic Estuaries Requires Catchment-Scale Interventions. Front. Mar. Sci. 8:688933. doi: 10.3389/fmars.2021.688933
Received: 31 March 2021; Accepted: 10 May 2021;
Published: 14 June 2021.
Edited by:
Ulisses Miranda Azeiteiro, University of Aveiro, PortugalReviewed by:
Akihide Kasai, Hokkaido University, JapanMichael S. Wetz, Texas A&M University Corpus Christi, United States
Copyright © 2021 Lemley, Lamberth, Manuel, Nunes, Rishworth, van Niekerk and Adams. This is an open-access article distributed under the terms of the Creative Commons Attribution License (CC BY). The use, distribution or reproduction in other forums is permitted, provided the original author(s) and the copyright owner(s) are credited and that the original publication in this journal is cited, in accordance with accepted academic practice. No use, distribution or reproduction is permitted which does not comply with these terms.
*Correspondence: Daniel A. Lemley, lemleydaniel7@gmail.com; DanielAlan.Lemley@mandela.ac.za