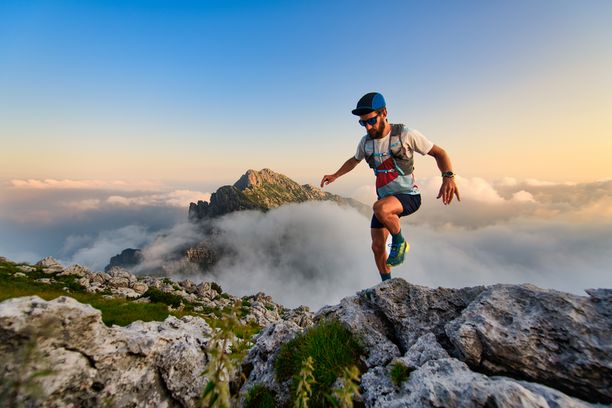
95% of researchers rate our articles as excellent or good
Learn more about the work of our research integrity team to safeguard the quality of each article we publish.
Find out more
ORIGINAL RESEARCH article
Front. Mar. Sci., 22 June 2021
Sec. Marine Biogeochemistry
Volume 8 - 2021 | https://doi.org/10.3389/fmars.2021.684484
The disequilibrium between lead-210 (210Pb) and polonium-210 (210Po) is increasingly used in oceanography to quantify particulate organic carbon (POC) export from the upper ocean. This proxy is based on the deficits of 210Po typically observed in the upper water column due to the preferential removal of 210Po relative to 210Pb by sinking particles. Yet, a number of studies have reported unexpected large 210Po deficits in the deep ocean indicating scavenging of 210Po despite its radioactive mean life of ∼ 200 days. Two precipitation methods, Fe(OH)3 and Co-APDC, are typically used to concentrate Pb and Po from seawater samples, and deep 210Po deficits raise the question whether this feature is biogeochemically consistent or there is a methodological issue. Here, we present a compilation of 210Pb and 210Po studies that suggests that 210Po deficits at depths >300 m are more often observed in studies where Fe(OH)3 is used to precipitate Pb and Po from seawater, than in those using Co-APDC (in 68 versus 33% of the profiles analyzed for each method, respectively). In order to test whether 210Po/210Pb disequilibrium can be partly related to a methodological artifact, we directly compared the total activities of 210Pb and 210Po in four duplicate ocean depth-profiles determined by using Fe(OH)3 and Co-APDC on unfiltered seawater samples. While both methods produced the same 210Pb activities, results from the Co-APDC method showed equilibrium between 210Pb and 210Po below 100 m, whereas the Fe(OH)3 method resulted in activities of 210Po significantly lower than 210Pb throughout the entire water column. These results show that 210Po deficits in deep waters, but also in the upper ocean, may be greater when calculated using a commonly used Fe(OH)3 protocol. This finding has potential implications for the use of the 210Po/210Pb pair as a tracer of particle export in the oceans because 210Po (and thus POC) fluxes calculated using Fe(OH)3 on unfiltered seawater samples may be overestimated. Recommendations for future research are provided based on the possible reasons for the discrepancy in 210Po activities between both analytical methods.
The biological carbon pump is a major mechanism for removing carbon dioxide from the atmosphere principally mediated by the transfer of organic particles from the surface to the deep ocean by different export pathways (Boyd et al., 2019). The flux of particulate organic carbon (POC) varies strongly across regions and time (Buesseler and Boyd, 2009), hindering the estimation of POC export in the global ocean (ranging from 5 to >12 Pg C yr–1; Boyd and Trull, 2007; Henson et al., 2011; Siegel et al., 2014). The naturally occurring radionuclides lead-210 (210Pb, half-life = 22.3 years) and polonium-210 (210Po, half-life = 138 days) have been widely used as particle tracers in the marine environment for decades (e.g., Bacon et al., 1976, 1988; Cochran and Masqué, 2003) and, in the last years, especially, to quantify POC export (e.g., Tang and Stewart, 2019). Indeed, the combination of 210Po/210Pb with other particle export methods, such as sediment traps or the thorium-234/uranium-238 (234Th/238U) pair, has given a broader perspective on downward export fluxes integrating time scales ranging from a few days to several months (Stewart et al., 2007, 2011; Buesseler et al., 2008; Verdeny et al., 2009; Wei et al., 2011; Le Moigne et al., 2013; Ceballos-Romero et al., 2016; Maiti et al., 2016; Roca-Martí et al., 2016; Anand et al., 2018; Hayes et al., 2018).
The use of 210Po/210Pb disequilibrium as a proxy for POC export relies on the assumption that sinking of organic particles generates a deficit of 210Po with respect to 210Pb in the upper water column due to preferential scavenging of Po compared to Pb (Friedrich and Rutgers van der Loeff, 2002; Verdeny et al., 2009). Unlike 210Pb (or 234Th), Po can be assimilated into cells, possibly as an analog of sulfur, and subsequently cycled as the organic matter is regenerated (Stewart et al., 2008). In the particle-poor deep ocean, radioactive equilibrium between 210Pb and 210Po is anticipated because of the long scavenging residence times and short half-life of 210Po (e.g., Bacon et al., 1976; Cochran et al., 1983). Yet, large deficits of 210Po have been observed in the mesopelagic (∼ 100–1000 m) and bathypelagic (>1000 m) zones in different regions of the world ocean, including the North Atlantic (e.g., Kim and Church, 2001; Hong et al., 2013; Rigaud et al., 2015), the North, Equatorial and South Pacific (e.g., Thomson and Turekian, 1976; Nozaki et al., 1990, 1997; Chung and Wu, 2005; Hu et al., 2014), the Arctic Ocean (e.g., Smith et al., 2003; Roca-Martí et al., 2018), and the Southern Ocean (e.g., Friedrich and Rutgers van der Loeff, 2002). Disequilibrium in deep waters has been commonly attributed to the scavenging of 210Po by particles from the local upper water column or from the shelves due to high biological productivity (Hu et al., 2014; Rigaud et al., 2015; Ma et al., 2017). Recently, a model study by De Soto et al. (2018) has argued that the disequilibrium between 210Pb and 210Po at depth in the Porcupine Abyssal Plain, NE Atlantic, could be explained by a significant adsorption of 210Po onto particles as they sink through the water column concurrent with negligible desorption. An alternate explanation is that this 210Po deficit reflects a missing sink of 210Po in deep waters by means, for instance, of 210Po uptake by bacteria and its transfer to higher trophic levels in the oligotrophic ocean (Kim, 2001). Low 210Po/210Pb activity ratios at depth have also been associated with hydrothermal activity (Kadko et al., 1987) and the focusing of atmospherically derived 210Pb (with low 210Po) by isopycnal transport (Nozaki et al., 1990).
One hypothesis that has not been addressed yet is that these 210Po deficits could also be influenced by an analytical bias. Church et al. (2012) pointed out that there is the potential for differential extraction of the Po spike used as a chemical yield tracer (usually 209Po, although 208Po has also been used) versus in situ 210Po, depending on the precipitation method used. Two main methods have been used to pre-concentrate Pb and Po from seawater samples: the iron hydroxide [Fe(OH)3, Thomson and Turekian, 1976] and the cobalt ammonium pyrrolidine dithiocarbamate (Co-APDC, Fleer and Bacon, 1984). The Fe(OH)3 method is the most used analytical procedure for the extraction of 210Pb and 210Po from seawater likely because it is less time-consuming than the Co-APDC procedure. The latter method involves adding APDC to the sample to chelate Pb and Po and subsequent flocculation of the colloidal chelate by adding excess cobalt, followed by sample filtration. To date, studies have shown that both methods are effective in co-precipitating stable Pb and 209Po added to seawater samples as yield monitors, as shown by recoveries of more than 70% of these spikes (Matthews et al., 2007; Rigaud et al., 2013). However, to our knowledge, there has been no systematic evaluation of whether the Fe(OH)3 and Co-APDC methods produce comparable 210Po results.
Here, we present a compilation of 210Pb and 210Po studies classified according to the precipitation method used and whether 210Po deficits at depth were observed. In addition, we directly compare the total activities of 210Pb and 210Po in four duplicate ocean depth-profiles determined by using both precipitation methods, Fe(OH)3 and Co-APDC, on unfiltered seawater samples. We discuss the implications of the results of this comparison for using the 210Po/210Pb pair as a tracer of particle export in the oceans and provide recommendations for future research.
A review of 210Pb and 210Po studies was conducted in order to identify those that reported depth profiles of total (dissolved + particulate) 210Pb and 210Po activities in water depths >300 m, classify them according to the precipitation method used, and determine whether 210Po deficits at depth were found (Figure 1). The compilation includes a total of 213 depth profiles from 41 studies published between 1976 and 2020.
Figure 1. Compilation of studies reporting depth profiles (≥300 m) of total 210Pb and 210Po activities. Legend shows the precipitation method used in each study: Fe(OH)3 TOT siph, Fe(OH)3 TOT filt precip, Fe(OH)3 DISS + PART, Co-APDC TOT, Co-APDC DISS+PART (see section “Compilation of 210Pb and 210Po Studies” for definitions). Many of these studies represent a transect of stations but for clarity the dots represent the location of one of the stations sampled for 210Pb and 210Po per study. The number next to each dot corresponds to the study reference and the numbers in parentheses refer to the number of profiles where deficits of 210Po (total 210Po/210Pb activity ratios <0.8) at depth were found, as shown by at least two data points from ≥300 m, with respect to the total profiles analyzed per study. The inset plot shows the percentage of profiles with deficits of 210Po at depth with respect to the total profiles analyzed per method considering all studies compiled. To avoid duplication, data from the D341 cruise presented in Le Moigne et al. (2013) were excluded from the dataset presented in Ceballos-Romero et al. (2016); data from Roca-Martí et al. (2018) were obtained from a combination of unfiltered and prefiltered samples [Fe(OH)3 TOT siph and Fe(OH)3 DISS + PART].
The precipitation methods were divided into the following categories: (1) Fe(OH)3 TOT siph, where unfiltered seawater samples were precipitated with Fe(OH)3 and the supernatant was siphoned off or decanted to allow further processing of the Fe(OH)3 precipitate; (2) Fe(OH)3 TOT filt precip, where unfiltered seawater samples were precipitated with Fe(OH)3 and the precipitate was filtered; (3) Fe(OH)3 DISS + PART, where prefiltered seawater samples were precipitated with Fe(OH)3 and the particulate fraction was analyzed separately; (4) Co-APDC TOT, where unfiltered seawater samples were precipitated with Co-APDC; (5) Co-APDC DISS+PART, where prefiltered seawater samples were precipitated with Co-APDC and the particulate fraction was analyzed separately.
In addition, we determined whether 210Po deficits at depth were found for those profiles that presented total 210Pb and 210Po activities at least at two depths from ≥300 m. Profiles were considered to show 210Po deficits at depth if they presented total 210Po/210Pb activity ratios <0.8 at least at two depths ≥300 m.
Detailed procedures for the methods compared in this study [Fe(OH)3 TOT siph and Co-APDC TOT in Figure 1] are described below and can be found at the Center for Marine and Environmental Radioactivity website1. Additional details for each set of samples processed using Fe(OH)3 and Co-APDC are provided in Supplementary Table 1 including information on the 209Po and stable Pb tracers, detector background, blank from the stable Pb tracer, and chemical recoveries.
A total of 35 duplicate sample pairs were collected for the determination of total 210Pb and 210Po in seawater (6.5–10.4 L, Supplementary Table 1) using Niskin bottles attached to a conductivity-temperature-depth (CTD) rosette at four locations (Figure 2 and Table 1). Three profiles were collected in the Mediterranean Sea in 2011–2013 (Ionian and Catalano-Balear Seas), while another profile was collected at the Southern Ocean Time Series (SOTS) site in 2018. This allowed to increase the statistical significance and compare results obtained from contrasting oceanographic regimes. Duplicate samples were all collected from the same CTD cast and the same Niskin bottles, except for one profile (Catalano-Balear South, CBS) where duplicate samples were collected from different Niskin bottles.
Figure 2. Study areas where duplicate ocean depth-profiles were collected for total 210Pb and 210Po analyses in the Catalano-Balear (north: CBN; south: CBS) and Ionian Seas (left map), and at the Southern Ocean Time Series (SOTS) site (right map).
Table 1. Total 210Pb and 210Po activities and 210Po/210Pb activity ratios measured using the Fe(OH)3 and Co-APDC precipitation methods [Fe(OH)3 TOT siph and Co-APDC TOT protocols in Figure 1] in four duplicate ocean depth-profiles.
Unfiltered seawater samples were acidified immediately after collection to pH 1–2 using HCl (∼ 1 mL per liter of sample), and spiked with known amounts of 209Po (T1/2 = 125 years, 2–4 dpm, Supplementary Table 1) and stable Pb (4–42 mg, Supplementary Table 1) to monitor the losses of Po and Pb during the radiochemistry procedure. Samples were vigorously shaken after the addition of HCl and each spike. Two solutions of 209Po were used, both in acid media (9 M HCl for the Mediterranean Sea samples; 1 M HNO3 for SOTS) and prepared from standard solutions (Oak Ridge National Laboratory, United States; Eckert & Ziegler, Germany; respectively). The Pb solution (aqueous) was prepared from ancient Pb (>200 years) to minimize 210Pb and 210Po contamination. From each pair of duplicates, one sample was processed using Fe(OH)3 (Thomson and Turekian, 1976; Sarin et al., 1992) and the other using Co-APDC (Boyle and Edmond, 1975; Fleer and Bacon, 1984) as described below. All initial processing, including the precipitation of 210Pb and 210Po, was accomplished at sea.
240 mg of Fe were added to each acidified and spiked sample in the form of FeCl3 solution. After vigorous shaking, samples were allowed to equilibrate for 9–24 h (Supplementary Table 1). Pb and Po isotopes were then precipitated with Fe(OH)3 by adjusting the pH to 8–9 with NH4OH. The precipitate was allowed to settle for a few hours, and then most of the supernatant visibly free of iron hydroxides was carefully siphoned off. The precipitate was transferred into 250 mL plastic bottles and stored for 12–37 days (Supplementary Table 1) until further processing in land-based laboratories: Mediterranean Sea samples were analyzed at Universitat Autònoma de Barcelona and SOTS samples at the Edith Cowan University. There, samples were centrifuged and the excess supernatant removed. Milli-Q water was then added to the precipitates to dissolve salts and remove them by suction after a second centrifugation. Precipitates were transferred into beakers and dissolved using concentrated HCl. After evaporation to near dryness, samples were re-dissolved with ∼ 80 mL of 1 M HCl and ascorbic acid was added to reduce Fe3+ to Fe2+.
After 9–24 h of isotope equilibration, 10 mg of Co and 800 mg of APDC were added to each sample as cobalt nitrate and APDC solutions, shaking the samples vigorously after each reagent addition. Samples were allowed to equilibrate for several hours (6–12 h) and then filtered through 0.2 μm pore-size filters (Whatman membrane filters mixed cellulose ester, WHA10401731, 142 mm diameter). Samples were stored for later processing in the land-based laboratories. The filters with the Co-APDC precipitates were digested at <100°C using concentrated HNO3 in beakers covered with watch glasses. The solutions were then evaporated to near dryness and HNO3 was completely eliminated from the samples by addition of 2 mL of concentrated HCl and subsequent evaporation to near dryness for three consecutive times. The residues were re-dissolved with ∼ 80 mL of 1 M HCl.
Silver disks (0.1 mm thick, 25 mm diameter) were suspended in the 1 M HCl solutions using a nylon string to allow the auto-deposition of Po isotopes (i.e., plating) at ∼ 80°C and constant stirring for at least 6 h (Flynn, 1968). One side of the disks was previously coated with urethane to maximize Po plating on the non-coated side and optimize counting statistics. The time elapsed between sampling and the first Po plating was minimized as much as possible to reduce the uncertainty of 210Po activities (Rigaud et al., 2013). 210Po and 209Po emissions were counted by alpha spectrometry (Fleer and Bacon, 1984) using passivated implanted planar silicon alpha detectors (Canberra, United States) or silicon surface barrier alpha detectors (EG&G Ortec, United States). The disks were counted until a minimum of 400 counts of both isotopes were accumulated or up to a maximum of 5 days. The detector background contributed on average to <1% of the total counts of 209Po and 210Po (Supplementary Table 1). The recoveries of the 209Po tracer averaged 78 ± 8% for the Fe(OH)3 method and 63 ± 16% for Co-APDC (Mann–Whitney rank-sum test, P ≤ 0.001; Supplementary Table 1). Solutions were re-plated and also passed through an anion-exchange resin (AG 1-X8, Sarin et al., 1992) to ensure the complete elimination of Po from samples (Rigaud et al., 2013). Samples were re-spiked with 2–4 dpm of 209Po tracer and stored for at least 6 months in 9 M HCl to allow 210Po ingrowth from 210Pb. After this time, samples were evaporated to near-dryness and re-dissolved with 1 M HCl to determine 210Po ingrowth from 210Pb by re-plating the solutions on silver disks and subsequent measurement of Po isotopes by alpha spectrometry as described above.
Typically, two aliquots from each sample were taken before the first and last platings to determine the chemical recovery of stable Pb by inductively coupled plasma-optical emission spectrometry. However, only the second aliquot was taken from CBS samples. Considering all other samples, the recovery of Pb from the first aliquot was on average 89 ± 7% for the Fe(OH)3 method and 78 ± 12% for Co-APDC (Mann–Whitney rank-sum test, P ≤ 0.001; Supplementary Table 1). These results were not significantly different from those determined from the second aliquot [Mann–Whitney rank-sum test, P = 0.276 for Fe(OH)3 and P = 0.401 for Co-APDC; Supplementary Figure 1], indicating that Pb losses occurred during the precipitation with Fe(OH)3 or Co-APDC rather than during the anion-exchange procedure. Therefore, for CBS samples, we assumed that the Pb recoveries at the first plating were the same as those determined at the last plating.
The 210Pb and 210Po blanks measured were equivalent to 0.014–0.016 dpm for the Ionian, Catalano-Balear North (CBN) and SOTS profiles. They increased to 0.270 dpm for the CBS profile due to the higher amount of stable Pb added. The contamination from the Pb solution contributed on average to <4% of the 210Po activity at the first and last platings, except for the CBS profile, where it contributed 25–32% (Supplementary Table 1).
210Pb and 210Po activities at the time of sampling were carefully calculated applying blank, ingrowth, decay and recovery corrections, as detailed by Rigaud et al. (2013). Overall uncertainties in activity accounting for errors in counting, detector background, 209Po activity, and the contamination from the Pb solution were on average 7% for 210Pb (5–10%) and 8% for 210Po (5–23%) for both methods.
Three-way ANOVA tests were run in order to examine whether Fe(OH)3 and Co-APDC resulted in comparable 210Pb and 210Po results and whether potential methodological differences depended on the region or water depth [see section “Direct Comparison of the Fe(OH)3 versus Co-APDC Methods”]. The factors considered were: (i) precipitation method [Fe(OH)3 versus Co-APDC]; (ii) region (Mediterranean Sea versus SOTS); (iii) depth (within the primary production zone versus deeper waters). The base of the primary production zone (PPZ) was defined as the depth where fluorescence declined to 10% of the maximum signal measured in overlying waters (Owens et al., 2015). Statistical analyses were conducted using SigmaPlot 11.0 (Systat Software, Inc., United States) with a significance level set at 0.05.
Two-thirds of the 210Pb and 210Po studies in the literature compilation (Figure 1) used the Fe(OH)3 method, of which 59% analyzed total activities from unfiltered seawater samples [Fe(OH)3 TOT], while the remaining analyzed the dissolved and particulate fractions separately [Fe(OH)3 DISS + PART]. Most of the Fe(OH)3 studies that measured total 210Pb and 210Po siphoned off or decanted the supernatant to allow further processing of the Fe(OH)3 precipitate [Fe(OH)3 TOT siph], while some filtered the precipitate [Fe(OH)3 TOT filt precip]. Almost all the studies that prefiltered the samples siphoned off or decanted the supernatant from the samples as well. The other third of the 210Pb and 210Po studies used the Co-APDC method, of which 71% separated the dissolved and particulate fractions (Co-APDC DISS+PART), while the remaining measured total 210Pb and 210Po from unfiltered samples (Co-APDC TOT). All the Co-APDC studies filtered the precipitate.
In addition to classifying the studies according to the method used, we also determined whether 210Po deficits (total 210Po/210Pb activity ratios <0.8) were found. Interestingly, this compilation shows that 210Po deficits at depths ≥300 m are found in 65–69% of the profiles analyzed using Fe(OH)3, while they are found in only 33% of the profiles analyzed using Co-APDC (Figure 1). This finding is independent of whether 210Pb and 210Po were analyzed on unfiltered or prefiltered seawater samples.
The profiles of 210Pb and 210Po determined using Fe(OH)3 [Fe(OH)3 TOT siph] and Co-APDC (Co-APDC TOT) in duplicate samples collected from the same CTD cast are shown in Figure 3 (see data in Table 1). 210Pb activities were not statistically different between both methods, ranging from 6.0–6.2 to 10.7–10.8 dpm 100 L–1 in the Mediterranean Sea (t-test, P = 0.122), and from 10.4–10.9 to 14.5–16.7 dpm 100 L–1 at SOTS (t-test, P = 0.572). In contrast, 210Po activities were significantly different. In the Mediterranean Sea, 210Po activities ranged from 1.5 to 6.9 dpm 100 L–1 for Fe(OH)3 and from 3.1 to 9.6 dpm 100 L–1 for Co-APDC (t-test, P < 0.001; Ionian, CBN and CBS in Figure 3B and Table 1). In the Southern Ocean, at SOTS, 210Po activities ranged from 5.0 to 13.5 dpm 100 L–1 for Fe(OH)3 and from 7.6 to 16.2 dpm 100 L–1 for Co-APDC (t-test, P < 0.001). The higher activities of both radionuclides at SOTS reflect the higher levels of their grandparent, 226Ra, in the Southern Ocean compared to the Mediterranean Sea (Ku and Lin, 1976; van Beek et al., 2009).
Figure 3. Vertical activity profiles for total 210Po (solid lines) and total 210Pb (dashed lines) measured in duplicate samples using the Fe(OH)3 (yellow, closed symbols) and Co-APDC (green, open symbols) precipitation methods [Fe(OH)3 TOT siph and Co-APDC TOT protocols in Figure 1]. (A) Shows only the upper 500 m of the water column, while (B) shows the entire profiles. Same scale is used for the x- and y-axis to facilitate comparison. The base of the primary production zone (PPZ) is denoted by the horizontal dotted line.
The Fe(OH)3 results showed deficits of 210Po at all sites and throughout the entire profiles. Below the PPZ, activities of 210Po were lower than 210Pb on average by a factor of 1.9 and equilibrium between both radionuclides was only observed at 1600 m at SOTS (Figure 3B). On the contrary, results from Co-APDC showed net removal of 210Po confined in the upper 75–100 m (Figure 3A), and similar 210Pb and 210Po activities at deeper depths. In general, 210Pb and 210Po from Co-APDC reached equilibrium around the PPZ depth (Figure 3A). This is in line with recent studies that found a close overlap between the PPZ depth and the horizon where the 234Th/238U radionuclide pair reaches equilibrium (Puigcorbé et al., 2017; Roca-Martí et al., 2017; Lemaitre et al., 2018; Buesseler et al., 2020a,b). There is, however, one exception to this pattern. At CBS, 210Po activities were lower than 210Pb activities throughout the entire profile for both methods (Figure 3B). Consequently, 210Po/210Pb activity ratios were lower than 1.0 within the PPZ and in deeper waters, averaging 0.37 ± 0.14 for Fe(OH)3 and 0.60 ± 0.10 for Co-APDC (Figure 4 and Table 1). In this area of the Mediterranean Sea, turbidity spikes were observed throughout the entire water column below the surface maximum (Supplementary Figure 2), suggesting a possible net removal of 210Po by particles below the PPZ in such conditions. At the other stations, turbidity spikes below the surface were not as pronounced as at CBS.
Figure 4. 210Po/210Pb activity ratio determined by using the Fe(OH)3 (yellow, closed symbols) and Co-APDC (green, open symbols) methods. Symbols denote different study areas: IONIAN (triangles), CBN (squares), CBS (circles), and SOTS (stars). The vertical solid line indicates 210Po/210Pb activity ratio = 1.0, and the horizontal dotted line is the average depth of the primary production zone in the study areas.
The 210Po/210Pb activity ratios (Figure 4) from both methods were statistically different (t-test, P < 0.001) with mean values from surface waters to the PPZ depth of 0.53 ± 0.10 using Fe(OH)3 and 0.84 ± 0.16 using Co-APDC, and 0.66 ± 0.17 and 0.96 ± 0.10, respectively, below the PPZ excluding the CBS profile.
Figure 5 shows a cross-plot of the 210Pb and 210Po activities obtained from the Fe(OH)3 and Co-APDC methods. 210Pb results from Co-APDC and Fe(OH)3 were statistically similar (ANOVA test, P = 0.418 for the entire dataset, 0.263 for the Mediterranean and 0.849 for SOTS; Figure 5) with differences between mean activities of only 0.2–0.4 dpm 100 L–1 for the entire dataset. There was no significant difference between methods either when comparing samples within the PPZ or below (ANOVA test, P = 0.151 and P = 0.536, respectively). This agrees with the experiments conducted by Chung et al. (1983) in which Fe(OH)3 and Co-APDC produced identical 210Pb results. Chung et al. (1983) also tested the effect of equilibration times between 210Pb and the added stable Pb carrier for times ranging from 1 to 330 days. Their results showed no discernible effect on the measured 210Pb activities.
Figure 5. Comparison of 210Po (gray) and 210Pb (black) determined by using the Co-APDC (y-axis) versus the Fe(OH)3 (x-axis) methods. Symbols denote different study areas: IONIAN (triangles), CBN (squares), CBS (circles), and SOTS (stars). Open symbols show samples from the primary production zone, while closed symbols correspond to deeper samples. Solid line indicates 1:1 relationship.
In contrast, 210Po activities from samples processed using Co-APDC were significantly higher than those obtained by using Fe(OH)3 (ANOVA test, P < 0.001; Figure 5). The difference in the mean 210Po activities observed between the two precipitation methods was 3.0 dpm 100 L–1 for the entire dataset [Co-APDC: 8.6 ± 3.6 dpm 100 L–1; Fe(OH)3: 5.6 ± 2.3 dpm 100 L–1], which corresponds to 35% of the 210Po activity obtained with the Co-APDC method. The same statistical analysis was applied separately for the Mediterranean Sea profiles and SOTS (ANOVA test, P = 0.003 and P < 0.001, respectively), showing that the difference in absolute and relative terms between the two methods was larger in the Southern Ocean. At SOTS the difference was 5.1 dpm 100 L–1, equivalent to 41% of the 210Po activity obtained with the Co-APDC method, while in the Mediterranean Sea these values were 1.8 dpm 100 L–1 and 28%, respectively. Further, the use of Co-APDC resulted in higher 210Po activities both within the PPZ and in deeper waters (ANOVA test, P < 0.001 for both; Figure 5), obtaining a difference between methods equal to 32% and 36% of the 210Po activity obtained with the Co-APDC method, respectively.
Our results from four duplicate 210Pb and 210Po profiles support that either scavenging method can be used for reliably extracting 210Pb from seawater, but the Fe(OH)3 TOT siph method underestimates 210Po activities.
The compilation of 210Pb and 210Po studies summarized in Figure 1 suggests that the Fe(OH)3 and Co-APDC methods may yield disparate results for 210Po. As these studies are from different oceanographic regimes and were conducted at different times, a direct comparison of methods is difficult. However, the total 210Pb and 210Po activity results presented here from four duplicate profiles indicate that the Fe(OH)3 TOT siph method underestimated 210Po activities throughout the entire water column. 210Po activities are calculated from the ratio between the count rate of 210Po to 209Po multiplied by the known activity of 209Po added to the samples, and applying appropriate ingrowth and decay corrections (Rigaud et al., 2013). Therefore, while similarly high 209Po recoveries were obtained for both methods [78 ± 8% for Fe(OH)3 and 63 ± 16% for Co-APDC], 210Po was scavenged differently. Below we discuss two possible hypotheses that could explain how the Fe(OH)3 method may have resulted in a higher extraction of 209Po than 210Po from unfiltered samples and, in turn, led to the calculation of lower 210Po activities compared with the Co-APDC method: (1) the Fe(OH)3 protocol did not quantitatively extract all of the dissolved 210Po from seawater due to organic complexation; (2) siphoning of the supernatant from unfiltered samples precipitated with Fe(OH)3 resulted in a loss of particles and associated 210Po activity.
First, different chemical speciation between the natural 210Po present in seawater and the artificial 209Po added to the samples (in acid media) may prevent a complete equilibration between the isotopes over 9–24 h (Supplementary Table 1) and result in a differential extraction when precipitating with Fe(OH)3. Little is known about the speciation of Po in seawater, but it may behave similarly to other group 16 metalloids, such as selenium (Stewart et al., 2008), which is predominantly found in organic form in surface seawater (Cutter and Cutter, 2001). Organic speciation of Po in seawater may arise through its distinctive biogeochemistry in which it is assimilated into organic matter and recycled with it. Previous studies have shown that 210Po penetrates into the cytoplasm of bacteria and phytoplankton and associates with proteins and sulfur containing compounds in bacteria, phytoplankton and zooplankton (Fisher et al., 1983; Cherrier et al., 1995; Stewart and Fisher, 2003a,b). Therefore, at least some of the dissolved 210Po atoms present in seawater, especially in the upper water column, would have likely been recycled and perhaps present as organic species. Further, Chuang et al. (2013) showed that Po is particularly prone to chelation by organic ligands like hydroxamate siderophores. Such organic speciation of 210Po in seawater may reduce its adsorption onto iron hydroxides, whereas 210Po would be effectively co-precipitated as a dithiocarbamate chelate with the Co-APDC method (Boyle and Edmond, 1975). If that was the case, regional differences in seawater chemistry and Po speciation would result in site-specific discrepancies between the Fe(OH)3 and Co-APDC methods, in line with the results obtained in this study. In contrast, the 209Po spike added to the acidified samples would not be speciated in the same way as natural 210Po. After acidification and tracer addition, samples in the present study were allowed to equilibrate for 9–24 h before precipitation. This equilibration time, although typical of many studies using the Fe(OH)3 method, may have been too short to destroy organic ligands. As a consequence, the 209Po spike may not have completely equilibrated with natural 210Po, leading to less scavenging of 210Po than of 209Po on iron hydroxides.
Another possible explanation of the difference between the methods may be related to the fact that total (dissolved + particulate) 210Pb and 210Po activities were measured. Total seawater samples in the present study were acidified to pH 1–2 immediately after collection (see section “Duplicate Profiles of 210Pb and 210Po”). Marine biogenic particles typically have 210Po/210Pb activity ratios >1 (Cochran and Masqué, 2003), due both to adsorption of 210Po onto particle surfaces and its incorporation into the particles. In contrast, 210Pb is only adsorbed onto particle surfaces. As a consequence, 210Po may not solubilize in an acidified sample over the 9–24 h allowed before precipitation. Samples processed with the Co-APDC method were filtered through 0.2 μm filters after precipitation and were subsequently digested with concentrated HNO3, which would have effectively dissolved any particles in the sample along with the Co-APDC precipitate. For the samples precipitated with the Fe(OH)3 method, most of the supernatant water was siphoned off at sea and the precipitate was returned to the laboratory with residual supernatant. Despite Fe(OH)3 TOT siph being a common procedure for the determination of total 210Pb and 210Po (see Figure 1), we suggest that this method may result in a loss of particles and associated 210Po activity from the samples. If that was the case, the methodological offset between the total Fe(OH)3 and Co-APDC protocols would depend on the particulate 210Pb and 210Po activities in seawater and vary as a function, for example, of place, time of year and phytoplankton biomass. Indeed, previous studies in the Mediterranean Sea and the Southern Ocean have reported that a significant fraction of the total 210Po activity is associated with the particulate phase. For example, in the NW Mediterranean Sea, particulate 210Po (>0.2 μm) activities in surface waters were reported to amount to 21% of the total activities (Masqué et al., 2002b). This agrees with an average of 19% of particulate 210Po (>0.7 μm) measured at the DYFAMED site (NW Mediterranean Sea) from surface waters to >2400 m (unpublished results, P. Masqué). Similarly, in the Antarctic Circumpolar Current, the relative importance of particulate 210Po (>1 μm) to total activities was on average 14% in the upper 600 m of the water column, including the sampling of phytoplankton blooms (Friedrich and Rutgers van der Loeff, 2002). These levels of particulate 210Po comprise a significant fraction of the difference observed here between the Fe(OH)3 and Co-APDC methods [28% for the Mediterranean Sea and 41% for SOTS, see section “Direct Comparison of the Fe(OH)3 versus Co-APDC Methods”]. It is important to emphasize that this potential bias would probably be unnoticeable for 210Pb because particulate 210Pb activities only amounted to 3–8% of the total activity in these studies (Friedrich and Rutgers van der Loeff, 2002; Masqué et al., 2002b), which are within the uncertainties associated with the 210Pb measurements. However, the results from Friedrich and Rutgers van der Loeff (2002) and the DYFAMED site obtained by using the Fe(OH)3 DISS + PART method showed a significant disequilibrium between total 210Pb and 210Po at depths ≥300 m (average total 210Po/210Pb activity ratio = 0.63 ± 0.05 and 0.79 ± 0.13, respectively). This suggests that a possible loss of particles when siphoning the supernatant from unfiltered samples precipitated with Fe(OH)3 may not fully explain the differences observed between the two precipitation methods.
The compilation of 210Pb and 210Po studies shows that deficits of 210Po at depth are more often observed when using Fe(OH)3 versus Co-APDC, regardless of whether 210Pb and 210Po were analyzed on unfiltered or prefiltered seawater samples (Figure 1). This observation suggests that the organic complexation hypothesis may be the major explanation for the difference between methods. We acknowledge, however, that further experiments are needed in order to test other Fe(OH)3 protocols and elucidate the underlying reasons behind the mismatch observed in this study. In particular, samples should be processed identically with respect to the treatment of the precipitate [filtered for both the Fe(OH)3 and Co-APDC methods]. Thus, at this point, our findings cannot be extrapolated to other Fe(OH)3 protocols, such as that used in the GEOTRACES program for the determination of 210Pb and 210Po in filtered seawater samples (Cutter et al., 2017).
The comparison of methods presented in this study reveals that while Fe(OH)3 and Co-APDC yield comparable results for 210Pb, up to 40% lower 210Po activities can be measured when using the Fe(OH)3 method on unfiltered seawater samples. Moreover, unlike the iron hydroxide method, samples processed using Co-APDC showed radioactive equilibrium between 210Pb and 210Po at depth (see section “210Pb and 210Po Activities”), consistent with the long scavenging residence times in the deep ocean compared with the mean life of 210Po.
The lower 210Po/210Pb activity ratios measured by using the Fe(OH)3 method are apparent not only in deep samples, where large 210Po deficits have been reported in multiple studies [most of them using Fe(OH)3, Figure 1], but are also evident in samples from the euphotic zone and the upper twilight zone (Figure 4). This observation has important implications with respect to calculations of the export flux of POC (or other elements of interest) associated with sinking particles in the upper ocean. To evaluate these implications, we present the 210Po-derived fluxes from a 1-D steady-state model (Equation 1), integrating the 210Po deficits observed down to the PPZ depth for the duplicate samples processed by using Fe(OH)3 and Co-APDC (Figure 6):
Figure 6. 210Po fluxes calculated from integrating the 210Po deficit down to the base of the primary production zone using the Fe(OH)3 (yellow) and Co-APDC (green) precipitation methods. Labels indicate the ratio between the Fe(OH)3-derived to the Co-APDC-derived fluxes.
where (210Pb – 210Po) is the integrated 210Po deficit with respect to 210Pb down to the depth of the PPZ (dpm m–2) and λ210Po is the decay constant of 210Po (0.0050 d–1). In addition to the PPZ depth, a relative light depth of 0.1% photosynthetically available radiation (PAR) could also be chosen as a reference depth to compare particle flux estimates from different sites (Buesseler et al., 2020b), but we only used the PPZ depth because we lack PAR data for some of the profiles.
The 210Po fluxes obtained from our four duplicate profiles are shown in Figure 6. At CBS, the fluxes derived from Fe(OH)3 and Co-APDC are similar within uncertainties (34 ± 4 and 26 ± 4 dpm m–2 d–1, respectively). In contrast, for the Ionian Sea, CBN and SOTS profiles, export estimates from the Fe(OH)3 method are a factor of 2 to 8 higher compared with Co-APDC estimates. This comparison clearly reveals how different conclusions can be drawn solely depending on the method used, where the Fe(OH)3 method as applied here can lead to overestimated 210Po fluxes. This suggests that 210Po fluxes estimated from a commonly used Fe(OH)3 protocol may be compromised to different degrees depending on the study area. The resulting exaggerated 210Po flux would cause a proportional overestimation of the POC fluxes when multiplying the 210Po flux by the POC/210Po ratio associated with sinking particles.
This study highlights that two commonly used methods for extracting 210Pb and 210Po from seawater can produce different activities for 210Po. On unfiltered seawater samples, precipitating 210Pb and 210Po with Fe(OH)3 and siphoning off the supernatant shows total 210Po activities up to 40% lower than those obtained with the Co-APDC method in which the precipitate is filtered. Deficits of 210Po can be used to quantify POC fluxes and, therefore, the Fe(OH)3 method may lead to artificially high 210Po-derived POC fluxes. This finding has also important implications for understanding the behavior of Po in marine systems and defining possible new applications of this element to study biogeochemical cycles (e.g., sulfur).
Possible explanations for the lower 210Po activities observed with the Fe(OH)3 method include complexation of dissolved 210Po in seawater preventing complete equilibration with the 209Po tracer added to the samples, or the loss of particles when siphoning the supernatant from the samples. The compilation of 210Pb and 210Po studies presented here suggests that the former may be the major explanation for the difference between methods. Future research is needed to investigate whether longer sample storage after acidification and spiking allows more complete equilibration between natural 210Po and the 209Po tracer and gives results more comparable to those from the Co-APDC method. For these tests, we recommend using filtered seawater samples so that only the dissolved fraction is involved, and unfiltered seawater samples with filtration of the Fe(OH)3 and Co-APDC precipitates. Processing a series of identical samples with increasing times for isotope equilibration before Fe(OH)3 co-precipitation would allow determination of how quickly equilibration between 210Po and 209Po is reached. Laboratory experiments using natural seawater and seawater treated with ultra-violet irradiation (as done for trace metals), together with dissolved organic matter measurements on the samples, may also be useful to test the hypothesis that organic complexation of Po leads to differential extraction of the 209Po spike and the in situ 210Po when precipitating with Fe(OH)3.
The original contributions presented in the study are included in the article/Supplementary Material, further inquiries can be directed to the corresponding author.
MR-M, VP, MC, NC, JG-O, and PM: contributed to conception and design. MR-M, VP, MC, and NC: contributed to the sampling and sample processing. MR-M, VP, MC, NC, JG-O, JKC, and PM: contributed to analysis and interpretation of data and drafted and revised the article. All authors contributed to the article and approved the submitted version.
MR-M was supported by an Endeavour Research Fellowship (6054) from the Australian Government, the Woods Hole Oceanographic Institution’s Ocean Twilight Zone study, and the Ocean Frontier Institute. VP received funding from the Edith Cowan University under the Early Career Researcher Grant Scheme (G1003456) and the Collaboration Enhancement Scheme (G1003362). MC is currently funded by an ETH Zurich Postdoctoral Fellowship Program (17-2 FEL-30), co-funded by the Marie Curie Actions for People COFUND Program. Support to JKC was provided by the National Science Foundation grant OCE-1736591. The authors acknowledge the financial support from the Spanish Ministry of Science, Innovation and Universities through the “María de Maeztu” program for Units of Excellence (CEX2019-000940-M), the Australian Research Council LIEF Project (LE170100219), and the Generalitat de Catalunya (MERS; 2017 SGR-1588).
The authors declare that the research was conducted in the absence of any commercial or financial relationships that could be construed as a potential conflict of interest.
The reviewer WG declared a past co-authorship with the author NC to the handling editor.
The reviewer MRL declared a past co-authorship with several of the authors MR-M, NC, PM, VP to the handling editor.
We acknowledge the crew and scientists on board the B/O García del Cid, B/O Ángeles Alvariño, and R/V Investigator during the COSTEM (Ref. CMT2009-07806), MedSeA and IN2018_V02 expeditions, respectively. We are grateful to Patricia Cámara-Mor and Flavia Tarquinio for collecting and processing samples at sea at CBS and SOTS, respectively. We would like to thank Joan Manuel Bruach for his help over the years in the Environmental Radioactivity Laboratory (LRA, UAB). We also acknowledge discussions with Claudia Benitez-Nelson, Thomas Church, and Edward A. Boyle. We are also grateful to David Glover for his valuable advice on statistical analysis and to the reviewers and Ken Buesseler for their comments on earlier drafts. Finally, we thank the many authors who have shared their results with us to make the 210Po/210Pb compilation possible. The IAEA is grateful for the support provided to its Environment Laboratories by the Government of the Principality of Monaco.
The Supplementary Material for this article can be found online at: https://www.frontiersin.org/articles/10.3389/fmars.2021.684484/full#supplementary-material
Anand, S. S., Rengarajan, R., Shenoy, D., Gauns, M., and Naqvi, S. W. A. (2018). POC export fluxes in the Arabian Sea and the Bay of Bengal: a simultaneous 234Th/238U and 210Po/210Pb study. Mar. Chem. 198, 70–87. doi: 10.1016/J.MARCHEM.2017.11.005
Bacon, M. P., Belastock, R. A., Tecotzky, M., Turekian, K. K., and Spencer, D. W. (1988). Lead-210 and polonium-210 in ocean water profiles of the continental shelf and slope south of New England. Cont. Shelf Res. 8, 841–853. doi: 10.1016/0278-4343(88)90079-9
Bacon, M. P., Brewer, P. G., Spencer, D. W., Murray, J. W., and Goddard, J. (1980a). Lead-210, polonium-210, manganese and iron in the Cariaco Trench. Deep Sea Res. A Oceanogr. Res. Pap. 27, 119–135. doi: 10.1016/0198-0149(80)90091-6
Bacon, M. P., Spencer, D. W., and Brewer, P. G. (1976). 210Pb/226Ra and 210Po/210Pb disequilibria in seawater and suspended particulate matter. Earth Planet. Sci. Lett. 32, 277–296. doi: 10.1016/0012-821X(76)90068-6
Bacon, M. P., Spencer, D. W., and Brewer, P. G. (1980b). “Lead-210 and polonium-210 as marine geochemical tracers: review and discussion of results from the Laborador Sea,” in Natural Radiation Environment III, Vol. 1, eds T. F. Gesell and W. M. Lowder (Houston, TX: Technical Information Center/U.S. Department of Energy), 473–501.
Bam, W., Maiti, K., Baskaran, M., Krupp, K., Lam, P. J., and Xiang, Y. (2020). Variability in 210Pb and 210Po partition coefficients (Kd) along the US GEOTRACES Arctic transect. Mar. Chem. 219:103749. doi: 10.1016/J.MARCHEM.2020.103749
Boyd, P. W., Claustre, H., Levy, M., Siegel, D. A., and Weber, T. (2019). Multi-faceted particle pumps drive carbon sequestration in the ocean. Nature 568, 327–335. doi: 10.1038/s41586-019-1098-2
Boyd, P. W., and Trull, T. W. (2007). Understanding the export of biogenic particles in oceanic waters: is there consensus? Prog. Oceanogr. 72, 276–312. doi: 10.1016/j.pocean.2006.10.007
Boyle, E. A., and Edmond, J. M. (1975). “Determination of Trace Metals in Aqueous Solution by APDC Chelate Co-precipitation,” in Analytical Methods in Oceanography, ed. T. R. P. Gibb (Washington, DC: American Chemical Society), 44–55. doi: 10.1021/ba-1975-0147.ch006
Buesseler, K. O., Benitez-Nelson, C. R., Roca-Martí, M., Wyatt, A. M., Resplandy, L., Clevenger, S. J., et al. (2020a). High-resolution spatial and temporal measurements of particulate organic carbon flux using thorium-234 in the northeast Pacific Ocean during the EXport Processes in the Ocean from RemoTe Sensing field campaign. Elem. Sci. Anthr. 8:030. doi: 10.1525/elementa.030
Buesseler, K. O., and Boyd, P. (2009). Shedding light on processes that control particle export and flux attenuation in the twilight zone of the open ocean. Limnol. Oceanogr. 54, 1210–1232. doi: 10.4319/lo.2009.54.4.1210
Buesseler, K. O., Boyd, P. W., Black, E. E., and Siegel, D. A. (2020b). Metrics that matter for assessing the ocean biological carbon pump. Proc. Natl. Acad. Sci. U.S.A. 117, 9679–9687. doi: 10.1073/PNAS.1918114117
Buesseler, K. O., Lamborg, C., Cai, P., Escoube, R., Johnson, R., Pike, S., et al. (2008). Particle fluxes associated with mesoscale eddies in the Sargasso Sea. Deep Sea Res. 2 Top. Stud. Oceanogr. 55, 1426–1444. doi: 10.1016/j.dsr2.2008.02.007
Ceballos-Romero, E., Le Moigne, F. A. C., Henson, S., Marsay, C. M., Sanders, R. J., García-Tenorio, R., et al. (2016). Influence of bloom dynamics on particle export efficiency in the North Atlantic: a comparative study of radioanalytical techniques and sediment traps. Mar. Chem. 186, 198–210. doi: 10.1016/j.marchem.2016.10.001
Cherrier, J., Burnett, W. C., and LaRock, P. A. (1995). Uptake of polonium and sulfur by bacteria. Geomicrobiol. J. 13, 103–115. doi: 10.1080/01490459509378009
Choi, H. Y., Stewart, G., Lomas, M. W., Kelly, R. P., and Moran, S. B. (2014). Linking the distribution of 210Po and 210Pb with plankton community along Line P, Northeast Subarctic Pacific. J. Environ. Radioact. 138, 390–401. doi: 10.1016/j.jenvrad.2014.02.009
Chuang, C.-Y., Santschi, P. H., Ho, Y.-F., Conte, M. H., Guo, L., Schumann, D., et al. (2013). Role of biopolymers as major carrier phases of Th, Pa, Pb, Po, and Be radionuclides in settling particles from the Atlantic Ocean. Mar. Chem. 157, 131–143. doi: 10.1016/j.marchem.2013.10.002
Chung, Y., and Finkel, R. (1988). 210Po in the western Indian Ocean: distributions, disequilibria and partitioning between the dissolved and particulate phases. Earth Planet. Sci. Lett. 88, 232–240. doi: 10.1016/0012-821X(88)90080-5
Chung, Y., Finkel, R., Bacon, M. P., Cochran, J. K., and Krishnaswami, S. (1983). Intercomparison of 210Pb measurements at GEOSECS station 500 in the northeast Pacific. Earth Planet. Sci. Lett. 65, 393–405. doi: 10.1016/0012-821X(83)90178-4
Chung, Y., and Wu, T. (2005). Large 210Po deficiency in the northern South China Sea. Cont. Shelf Res. 25, 1209–1224. doi: 10.1016/j.csr.2004.12.016
Church, T., Rigaud, S., Baskaran, M., Kumar, A., Friedrich, J., Masqué, P., et al. (2012). Intercalibration studies of 210Po and 210Pb in dissolved and particulate seawater samples. Limnol. Oceanogr. Methods 10, 776–789. doi: 10.4319/lom.2012.10.776
Cochran, J. K., Bacon, M. P., Krishnaswami, S., and Turekian, K. K. (1983). 210Po and 210Pb distributions in the central and eastern Indian Ocean. Earth Planet. Sci. Lett. 65, 433–452. doi: 10.1016/0012-821X(83)90180-2
Cochran, J. K., and Masqué, P. (2003). Short-lived U/Th series radionuclides in the ocean: tracers for scavenging rates, export fluxes and particle dynamics. Rev. Mineral. Geochemistry 52, 461–492. doi: 10.2113/0520461
Cutter, G., Casciotti, K., Croot, P., Geibert, W., Heimbürger, L.-E., Lohan, M., et al. (2017). Sampling and Sample-handling Protocols for GEOTRACES Cruises. Version 3.0. Bremerhaven: GEOTRACES Standards and Intercalibration Committee, 139.
Cutter, G. A., and Cutter, L. S. (2001). Sources and cycling of selenium in the western and equatorial Atlantic Ocean. Deep Sea Res. 2 Top. Stud. Oceanogr. 48, 2917–2931. doi: 10.1016/S0967-0645(01)00024-8
De Soto, F., Ceballos-Romero, E., and Villa-Alfageme, M. (2018). A microscopic simulation of particle flux in ocean waters: application to radioactive pair disequilibrium. Geochim. Cosmochim. Acta 239, 136–158. doi: 10.1016/J.GCA.2018.07.031
Fisher, N. S., Burns, K. A., Cherry, R. D., and Heyraud, M. (1983). Accumulation and cellular distribution of 241Am, 210Po and 210Pb in two marine algae. Mar. Ecol. Prog. Ser. 11, 233–237.
Fleer, A. P., and Bacon, M. P. (1984). Determination of 210Pb and 210Po in seawater and marine particulate matter. Nucl. Instrum. Methods Phys. Res. 223, 243–249. doi: 10.1016/0167-5087(84)90655-0
Flynn, W. W. (1968). The determination of low levels of polonium-210 in environmental materials. Anal. Chim. Acta 43, 221–227. doi: 10.1016/S0003-2670(00)89210-7
Friedrich, J., and Rutgers van der Loeff, M. M. (2002). A two-tracer (210Po–234Th) approach to distinguish organic carbon and biogenic silica export flux in the Antarctic Circumpolar Current. Deep Sea Res. 1 Oceanogr. Res. Pap. 49, 101–120. doi: 10.1016/S0967-0637(01)00045-0
Gascó, C., Antón, M. P., Delfanti, R., González, A. M., Meral, J., and Papucci, C. (2002). Variation of the activity concentrations and fluxes of natural (210Po,210Pb) and anthropogenic (239,240Pu, 137Cs) radionuclides in the Strait of Gibraltar (Spain). J. Environ. Radioact. 62, 241–262. doi: 10.1016/S0265-931X(01)00167-9
Hayes, C. T., Black, E. E., Anderson, R. F., Baskaran, M., Buesseler, K. O., Charette, M. A., et al. (2018). Flux of particulate elements in the North Atlantic Ocean constrained by multiple radionuclides. Global Biogeochem. Cycles 32, 1738–1758. doi: 10.1029/2018GB005994
Henson, S. A., Sanders, R., Madsen, E., Morris, P. J., Le Moigne, F., and Quartly, G. D. (2011). A reduced estimate of the strength of the ocean’s biological carbon pump. Geophys. Res. Lett. 38:L04606. doi: 10.1029/2011GL046735
Hong, G. H., Baskaran, M., Church, T. M., and Conte, M. (2013). Scavenging, cycling and removal fluxes of 210Po and 210Pb at the Bermuda time-series study site. Deep Sea Res. 2 Top. Stud. Oceanogr. 93, 108–118. doi: 10.1016/j.dsr2.2013.01.005
Horowitz, E. J., Cochran, J. K., Bacon, M. P., and Hirschberg, D. J. (2020). 210Po and 210Pb distributions during a phytoplankton bloom in the North Atlantic: implications for POC export. Deep Sea Res. 1 Oceanogr. Res. Pap. 164, 103339. doi: 10.1016/j.dsr.2020.103339
Hu, W., Chen, M., Yang, W., Zhang, R., Qiu, Y., and Zheng, M. (2014). Enhanced particle scavenging in deep water of the Aleutian Basin revealed by 210Po- 210Pb disequilibria. J. Geophys. Res. Oceans 119, 3235–3248. doi: 10.1002/2014JC009819
Kadko, D., Bacon, M. P., and Hudson, A. (1987). Enhanced scavenging of 210Pb and 210Po by processes associated with the East Pacific Rise near 8°45’N. Earth Planet. Sci. Lett. 81, 349–357. doi: 10.1016/0012-821X(87)90122-1
Kim, G. (2001). Large deficiency of polonium in the oligotrophic ocean’s interior. Earth Planet. Sci. Lett. 192, 15–21. doi: 10.1016/S0012-821X(01)00431-9
Kim, G., and Church, T. M. (2001). Seasonal biogeochemical fluxes of 234Th and 210Po in the Upper Sargasso Sea: influence from atmospheric iron deposition. Global Biogeochem. Cycles 15, 651–661. doi: 10.1029/2000GB001313
Ku, T.-L., and Lin, M.-C. (1976). 226Ra distribution in the Antarctic Ocean. Earth Planet. Sci. Lett. 32, 236–248. doi: 10.1016/0012-821X(76)90064-9
Le Moigne, F. A. C., Villa-Alfageme, M., Sanders, R. J., Marsay, C., Henson, S., and García-Tenorio, R. (2013). Export of organic carbon and biominerals derived from 234Th and 210Po at the Porcupine Abyssal Plain. Deep Sea Res. 1 Oceanogr. Res. Pap. 72, 88–101. doi: 10.1016/j.dsr.2012.10.010
Lemaitre, N., Planchon, F., Planquette, H., Dehairs, F., Fonseca-Batista, D., Roukaerts, A., et al. (2018). High variability of particulate organic carbon export along the North Atlantic GEOTRACES section GA01 as deduced from 234Th fluxes. Biogeosciences 15, 6417–6437. doi: 10.5194/bg-15-6417-2018
Ma, H., Yang, W., Zhang, L., Zhang, R., Chen, M., Qiu, Y., et al. (2017). Utilizing 210Po deficit to constrain particle dynamics in mesopelagic water, western South China Sea. Geochem. Geophys. Geosyst. 18, 1594–1607. doi: 10.1002/2017GC006899
Maiti, K., Bosu, S., D’Sa, E. J., Adhikari, P. L., Sutor, M., and Longnecker, K. (2016). Export fluxes in northern Gulf of Mexico–comparative evaluation of direct, indirect and satellite-based estimates. Mar. Chem. 184, 60–77. doi: 10.1016/j.marchem.2016.06.001
Masqué, P., Isla, E., Sanchez-Cabeza, J. A., Palanques, A., Bruach, J. M., Puig, P., et al. (2002a). Sediment accumulation rates and carbon fluxes to bottom sediments at the western Bransfield Strait (Antarctica). Deep. Res. 2 Top. Stud. Oceanogr. 49, 921–933. doi: 10.1016/S0967-0645(01)00131-X
Masqué, P., Sanchez-Cabeza, J. A., Bruach, J. M., Palacios, E., and Canals, M. (2002b). Balance and residence times of 210Pb and 210Po in surface waters of the northwestern Mediterranean Sea. Cont. Shelf Res. 22, 2127–2146. doi: 10.1016/S0278-4343(02)00074-2
Matthews, K. M., Kim, C.-K., and Martin, P. (2007). Determination of 210Po in environmental materials: a review of analytical methodology. Appl. Radiat. Isot. 65, 267–279. doi: 10.1016/J.APRADISO.2006.09.005
Moore, R. M., and Smith, J. N. (1986). Disequilibria between 226Ra, 210Pb and 210Po in the Arctic Ocean and the implications for chemical modification of the Pacific water inflow. Earth Planet. Sci. Lett. 77, 285–292. doi: 10.1016/0012-821X(86)90140-8
Niedermiller, J., and Baskaran, M. (2019). Comparison of the scavenging intensity, remineralization and residence time of 210Po and 210Pb at key zones (biotic, sediment-water and hydrothermal) along the East Pacific GEOTRACES transect. J. Environ. Radioact. 198, 165–188. doi: 10.1016/J.JENVRAD.2018.12.016
Nozaki, Y., Ikuta, N., and Yashima, M. (1990). Unusually large 210Po deficiencies relative to 210Pb in the Kuroshio Current of the East China and Philippine seas. J. Geophys. Res. 95:5321. doi: 10.1029/JC095iC04p05321
Nozaki, Y., Zhang, J., and Takeda, A. (1997). 210Pb and 210Po in the equatorial Pacific and the Bering Sea: the effects of biological productivity and boundary scavenging. Deep Sea Res. 2 Top. Stud. Oceanogr. 44, 2203–2220. doi: 10.1016/S0967-0645(97)00024-6
Obata, H., Nozaki, Y., Alibo, D. S., and Yamamoto, Y. (2004). Dissolved Al, In, and Ce in the eastern Indian Ocean and the Southeast Asian Seas in comparison with the radionuclides 210Pb and 210Po. Geochim. Cosmochim. Acta 68, 1035–1048. doi: 10.1016/j.gca.2003.07.021
Owens, S. A., Pike, S., and Buesseler, K. O. (2015). Thorium-234 as a tracer of particle dynamics and upper ocean export in the Atlantic Ocean. Deep Sea Res. 2 Top. Stud. Oceanogr. 116, 42–59. doi: 10.1016/j.dsr2.2014.11.010
Puigcorbé, V., Roca-Martí, M., Masqué, P., Benitez-Nelson, C. R., Rutgers van der Loeff, M. M., Laglera, L. M., et al. (2017). Particulate organic carbon export across the Antarctic circumpolar current at 10°E: differences between north and south of the Antarctic Polar Front. Deep Sea Res. 2 Top. Stud. Oceanogr. 138, 86–101. doi: 10.1016/j.dsr2.2016.05.016
Rigaud, S., Puigcorbé, V., Cámara-Mor, P., Casacuberta, N., Roca-Martí, M., Garcia-Orellana, J., et al. (2013). A methods assessment and recommendations for improving calculations and reducing uncertainties in the determination of 210Po and 210Pb activities in seawater. Limnol. Oceanogr. Methods 11, 561–571. doi: 10.4319/lom.2013.11.561
Rigaud, S., Stewart, G., Baskaran, M., Marsan, D., and Church, T. (2015). 210Po and 210Pb distribution, dissolved-particulate exchange rates, and particulate export along the North Atlantic US GEOTRACES GA03 section. Deep. Res. 2 Top. Stud. Oceanogr. 116, 60–78. doi: 10.1016/j.dsr2.2014.11.003
Ritchie, G. D., and Shimmield, G. B. (1991). “The Use of 210Po/210Pb Disequilibria in the Study of the Fate of Marine Particulate Matter,” in Radionuclides in the Study of Marine Processes, eds P. J. Kershaw and D. S. Woodhead (Dordrecht: Springer), 142–153. doi: 10.1007/978-94-011-3686-0_15
Roca-Martí, M., Puigcorbé, V., Friedrich, J., Rutgers van der Loeff, M. M., Rabe, B., Korhonen, M., et al. (2018). Distribution of 210Pb and 210Po in the Arctic water column during the 2007 sea-ice minimum: particle export in the ice-covered basins. Deep Sea Res. 1 Oceanogr. Res. Pap. 142, 94–106. doi: 10.1016/J.DSR.2018.09.011
Roca-Martí, M., Puigcorbé, V., Iversen, M. H., Rutgers van der Loeff, M. M., Klaas, C., Cheah, W., et al. (2017). High particulate organic carbon export during the decline of a vast diatom bloom in the Atlantic sector of the Southern Ocean. Deep Sea Res. 2 Top. Stud. Oceanogr. 138, 102–115. doi: 10.1016/j.dsr2.2015.12.007
Roca-Martí, M., Puigcorbé, V., Rutgers van der Loeff, M. M., Katlein, C., Fernández-Méndez, M., Peeken, I., et al. (2016). Carbon export fluxes and export efficiency in the central Arctic during the record sea-ice minimum in 2012: a joint 234Th/238U and 210Po/210Pb study. J. Geophys. Res. Ocean. 121, 5030–5049. doi: 10.1002/2016JC011816
Sarin, M. M., Bhushan, R., Rengarajan, R., and Yadav, D. N. (1992). Simultaneous determination of 238U series nuclides in waters of Arabian Sea and Bay of Bengal. Indian J. Mar. Sci. 21, 121–127.
Sarin, M. M., Kim, G., and Church, T. M. (1999). 210Po and 210Pb in the South-equatorial Atlantic: distribution and disequilibrium in the upper 500 m. Deep. Res. 2 Top. Stud. Oceanogr. 46, 907–917. doi: 10.1016/S0967-0645(99)00008-9
Shimmield, G. B., Ritchie, G. D., and Fileman, T. W. (1995). The impact of marginal ice zone processes on the distribution of 210Pb, 210Po and 234Th and implications for new production in the Bellingshausen Sea, Antarctica. Deep Sea Res. 2 Top. Stud. Oceanogr. 42, 1313–1335. doi: 10.1016/0967-0645(95)00071-W
Siegel, D. A., Buesseler, K. O., Doney, S. C., Sailley, S. F., Behrenfeld, M. J., and Boyd, P. W. (2014). Global assessment of ocean carbon export by combining satellite observations and food-web models. Global Biogeochem. Cycles 28, 181–196. doi: 10.1002/2013GB004743
Smith, J. N., Moran, S. B., and Macdonald, R. W. (2003). Shelf–basin interactions in the Arctic Ocean based on 210Pb and Ra isotope tracer distributions. Deep Sea Res. 1 Oceanogr. Res. Pap. 50, 397–416. doi: 10.1016/S0967-0637(02)00166-8
Stewart, G., Cochran, J. K., Miquel, J. C., Masqué, P., Szlosek, J., Rodriguez y Baena, A. M., et al. (2007). Comparing POC export from 234Th/238U and 210Po/210Pb disequilibria with estimates from sediment traps in the northwest Mediterranean. Deep Sea Res. 1 Oceanogr. Res. Pap. 54, 1549–1570. doi: 10.1016/j.dsr.2007.06.005
Stewart, G., and Fisher, N. S. (2003a). Bioaccumulation of polonium-210 in marine copepods. Limnol. Oceanogr. 48, 2011–2019. doi: 10.4319/lo.2003.48.5.2011
Stewart, G., and Fisher, N. S. (2003b). Experimental studies on the accumulation of polonium-210 by marine phytoplankton. Limnol. Oceanogr. 48, 1193–1201. doi: 10.4319/lo.2003.48.3.1193
Stewart, G., Fowler, S. W., and Fisher, N. S. (2008). “The Bioaccumulation of U- and Th-Series Radionuclides in Marine Organisms,” in U-Th series nuclides in aquatic systems, eds S. Krishnaswami and J. K. Cochran (Amsterdam: Elsevier Science), 269–305. doi: 10.1016/S1569-4860(07)00008-3
Stewart, G., Moran, S. B., and Lomas, M. W. (2010). Seasonal POC fluxes at BATS estimated from 210Po deficits. Deep Sea Res. 1 Oceanogr. Res. Pap. 57, 113–124. doi: 10.1016/j.dsr.2009.09.007
Stewart, G., Moran, S. B., Lomas, M. W., and Kelly, R. P. (2011). Direct comparison of 210Po, 234Th and POC particle-size distributions and export fluxes at the Bermuda Atlantic Time-series Study (BATS) site. J. Environ. Radioact. 102, 479–489. doi: 10.1016/j.jenvrad.2010.09.011
Tang, Y., Castrillejo, M., Roca-Martí, M., Masqué, P., Lemaitre, N., and Stewart, G. (2018). Distributions of total and size-fractionated particulate 210Po and 210Pb activities along the North Atlantic GEOTRACES GA01 transect: GEOVIDE cruise. Biogeosciences 15, 5437–5453. doi: 10.5194/bg-15-5437-2018
Tang, Y., and Stewart, G. (2019). The 210Po/210Pb method to calculate particle export: lessons learned from the results of three GEOTRACES transects. Mar. Chem. 217:103692. doi: 10.1016/j.marchem.2019.103692
Thomson, J., and Turekian, K. K. (1976). 210Po and 210Pb distributions in ocean water profiles from the Eastern South Pacific. Earth Planet. Sci. Lett. 32, 297–303. doi: 10.1016/0012-821X(76)90069-8
van Beek, P., Sternberg, E., Reyss, J.-L., Souhaut, M., Robin, E., and Jeandel, C. (2009). 228Ra/226Ra and 226Ra/Ba ratios in the Western Mediterranean Sea: barite formation and transport in the water column. Geochim. Cosmochim. Acta 73, 4720–4737. doi: 10.1016/J.GCA.2009.05.063
Verdeny, E., Masqué, P., Garcia-Orellana, J., Hanfland, C., Cochran, J. K., and Stewart, G. (2009). POC export from ocean surface waters by means of 234Th/238U and 210Po/210Pb disequilibria: a review of the use of two radiotracer pairs. Deep Sea Res. 2 Top. Stud. Oceanogr. 56, 1502–1518. doi: 10.1016/j.dsr2.2008.12.018
Verdeny, E., Masqué, P., Maiti, K., Garcia-Orellana, J., Bruach, J. M., Mahaffey, C., et al. (2008). Particle export within cyclonic Hawaiian lee eddies derived from 210Pb–210Po disequilibrium. Deep Sea Res. 2 Top. Stud. Oceanogr. 55, 1461–1472. doi: 10.1016/j.dsr2.2008.02.009
Wei, C.-L., Lin, S.-Y., Sheu, D. D.-D., Chou, W.-C., Yi, M.-C., Santschi, P. H., et al. (2011). Particle-reactive radionuclides (234Th, 210Pb, 210Po) as tracers for the estimation of export production in the South China Sea. Biogeosciences 8, 3793–3808. doi: 10.5194/bg-8-3793-2011
Wei, C.-L., and Murray, J. W. (1994). The behavior of scavenged isotopes in marine anoxic environments: 210Pb and 210Po in the water column of the Black Sea. Geochim. Cosmochim. Acta 58, 1795–1811. doi: 10.1016/0016-7037(94)90537-1
Keywords: marine chemistry, radiochemistry, polonium isotopes, precipitation methods, Co-APDC, Fe(OH)3, 210Po/210Pb disequilibrium, particle export
Citation: Roca-Martí M, Puigcorbé V, Castrillejo M, Casacuberta N, Garcia-Orellana J, Cochran JK and Masqué P (2021) Quantifying 210Po/210Pb Disequilibrium in Seawater: A Comparison of Two Precipitation Methods With Differing Results. Front. Mar. Sci. 8:684484. doi: 10.3389/fmars.2021.684484
Received: 23 March 2021; Accepted: 11 May 2021;
Published: 22 June 2021.
Edited by:
Eric ’Pieter Achterberg, GEOMAR Helmholtz Centre for Ocean Research Kiel, GermanyReviewed by:
Michiel Rutgers van der Loeff, Alfred Wegener Institute, Helmholtz Centre for Polar and Marine Research (AWI), GermanyCopyright © 2021 Roca-Martí, Puigcorbé, Castrillejo, Casacuberta, Garcia-Orellana, Cochran and Masqué. This is an open-access article distributed under the terms of the Creative Commons Attribution License (CC BY). The use, distribution or reproduction in other forums is permitted, provided the original author(s) and the copyright owner(s) are credited and that the original publication in this journal is cited, in accordance with accepted academic practice. No use, distribution or reproduction is permitted which does not comply with these terms.
*Correspondence: Montserrat Roca-Martí, bXJvY2FtYXJ0aUB3aG9pLmVkdQ==; bXJvY2FtYXJ0aUBkYWwuY2E=
Disclaimer: All claims expressed in this article are solely those of the authors and do not necessarily represent those of their affiliated organizations, or those of the publisher, the editors and the reviewers. Any product that may be evaluated in this article or claim that may be made by its manufacturer is not guaranteed or endorsed by the publisher.
Research integrity at Frontiers
Learn more about the work of our research integrity team to safeguard the quality of each article we publish.