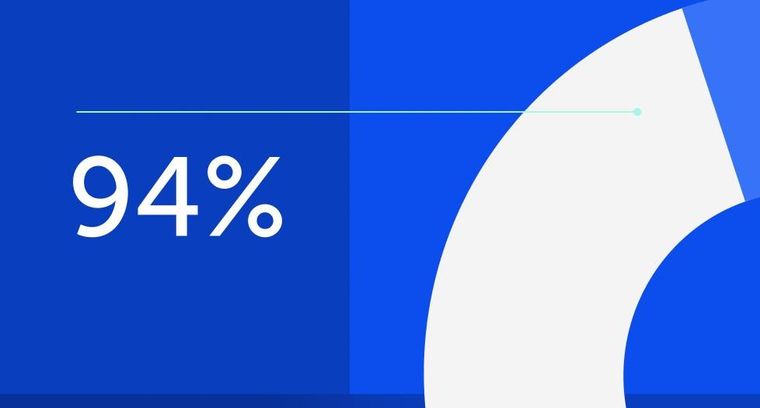
94% of researchers rate our articles as excellent or good
Learn more about the work of our research integrity team to safeguard the quality of each article we publish.
Find out more
ORIGINAL RESEARCH article
Front. Mar. Sci., 22 July 2021
Sec. Marine Megafauna
Volume 8 - 2021 | https://doi.org/10.3389/fmars.2021.683634
Predators must consume enough prey to support costly events, such as reproduction. Meeting high energetic requirements is particularly challenging for migrating baleen whales as their feeding seasons are typically restricted to a limited temporal window and marine prey are notoriously patchy. We assessed the energetic value of the six most common nearshore zooplankton species collected within the Oregon, United States range of the Pacific Coast Feeding Group (PCFG) gray whale (Eschrichtius robustus) feeding grounds, and compared these results to the energetic value of the predominant amphipod species fed on by Eastern North Pacific (ENP) gray whales in the Arctic. Energetic values of Oregon zooplankton differed significantly between species (Kruskal–Wallis χ2 = 123.38, df = 5, p < 0.0001), with Dungeness crab (Cancer magister) megalopae displaying the highest mean caloric content of all tested species (4.21 ± 1.27 kJ g– 1). This value, as well as the mean energetic value of the mysid Neomysis rayii (2.42 ± 1.06 kJ g– 1), are higher than the mean caloric content of Ampelisca macrocephala, the predominant Arctic amphipod. Extrapolations of these results to daily energetic requirements of gray whales indicate that lactating and pregnant gray whales feeding in the PCFG range would require between 0.7–1.03 and 0.22–0.33 metric tons of prey less per day if they fed on Dungeness crab megalopae or N. rayii, respectively, than a whale feeding on A. macrocephala in the Arctic. Yet, these results do not account for differences in availability of these prey species to foraging gray whales. We therefore suggest that other factors, such as prey density, energetic costs of feeding, or natal philopatry and foraging site fidelity play a role in the differences in population sizes between the PCFG and ENP gray whales. Climate change is implicated in causing reduced body condition and increased mortality of both PCFG and ENP gray whales due to decreased prey availability and abundance. Therefore, improved understanding of prey dynamics in response to environmental variability in both regions is critical.
Foraging efficiency is key to predator success. This efficiency must balance energetic cost with energetic reward, forcing predators to make decisions regarding target prey type, size, location, and behavioral effort. To maximize the net energy gained, optimal foraging theory (OFT) posits that an individual should target prey with the most benefit (energy) for the lowest possible cost (MacArthur and Pianka, 1966). Moreover, the marginal value theorem (MVT) illustrates that patch choice requires predators to consider the potential value of future patches relative to a current foraging patch, as well as the time and energy required to search for a new patch (Charnov, 1976). These energetic trade-offs impact predator distribution patterns and population dynamics (Owen-Smith et al., 2010; Louzao et al., 2014), and can be particularly challenging in marine ecosystems where prey is patchy and spatially and temporally dynamic (Hyrenbach et al., 2000).
Foraging decisions by baleen whales cannot afford much error as they are capital breeders with large energetic demands that must be met within a limited temporal window while on feeding grounds. Energy acquired during the foraging period must support the majority of their energetic requirements needed for foraging effort, migration, and reproduction for the subsequent year (Lockyer, 1984). For instance, it is estimated that gray whales (Eschrichtius robustus) must regain 11–29% of their body mass on the feeding grounds (Villegas-Amtmann et al., 2015); hence, finding enough prey is crucial. Prey patch selection by baleen whales is strongly associated with patch density and capture efficiency (Piatt and Methven, 1992; Croll et al., 2005; Goldbogen et al., 2011; Feyrer and Duffus, 2015; Hazen et al., 2015), where whales prioritize the densest patches in the most accessible areas (e.g., shallow to minimize diving costs) to achieve the highest energetic gain for the lowest cost (Croll et al., 2001; Doniol-Valcroze et al., 2011; Goldbogen et al., 2013; Torres et al., 2020).
Additionally, there is increased recognition that prey quality, such as caloric content, is important for the foraging ecology of cetaceans, including baleen whales (Spitz et al., 2012). Variability in the caloric value of zooplankton can occur within and between species, across seasons and between years, and by region (Mauchline, 1980). This variability may be due to differences in lipid composition and storage (Lee et al., 2006), diet (Mauchline, 1980), or reproductive stage (Schaafsma et al., 2018). Foraging decisions by baleen whales can be influenced by prey caloric content. For example, it has been shown that blue whales (Balaenoptera musculus) preferentially feed on a more calorically rich species of krill (Nickels et al., 2018). Caloric decisions may also be an important element in prey patch selection of generalist baleen whales that have multiple prey options. These generalists must maximize both density and caloric intake while minimizing the cost of capture associated with the energetic requirements of behavioral foraging adaptations fit to prey characteristics. For instance, gray whales in the Bering, Chukchi, and Beaufort Seas (hereafter referred to as Arctic) feed predominantly on benthic amphipods (Nerini, 1984; Moore et al., 2003; Coyle et al., 2007a), yet they feed on epibenthic and pelagic zooplankton along the North American west coast (Nerini, 1984; Nelson et al., 2008; Feyrer and Duffus, 2011; Gosho et al., 2011).
Gray whales in the eastern North Pacific Ocean migrate from breeding grounds in Baja California, Mexico to their feeding grounds in the Arctic during the summer (Rice and Wolman, 1971). The benthic amphipod community in this region is dominated by the ampeliscid amphipod, Ampelisca macrocephala, which comprises over 70% of the benthic community (Coyle and Highsmith, 1994) and is the primary prey of the Eastern North Pacific (ENP) gray whale population (Nerini, 1984). ENP individuals feed benthically by diving to the seafloor at mean depths of 40–50 m (Schonberg et al., 2014) and sucking up benthic infauna in the soft sediment (Nerini and Oliver, 1983). A sub-group of this population of gray whales, termed the Pacific Coast Feeding Group (PCFG), does not migrate to the Arctic feeding grounds but rather stops approximately halfway up the North American west coast and utilizes the region from northern California, United States to northern British Columbia, Canada as their foraging ground (Calambokidis et al., 2002). PCFG gray whales are well-known as generalist feeders (Nerini, 1984) based on evidence of feeding on benthic amphipods (Burnham and Duffus, 2016), mysids (Newell and Cowles, 2006; Feyrer and Duffus, 2011), cumaceans (Gosho et al., 2011), crab larvae (Nelson et al., 2008), ghost shrimp (Duffus, 1996; Darling et al., 1998), and herring roe (Darling et al., 1998). The ability to feed on such a wide variety of zooplankton prey requires different feeding behaviors adapted to successfully capture variable prey in a diversity of habitats. Torres et al. (2018) used an unoccupied aerial system (UAS; a.k.a. “drone”) to describe and quantify a variety of foraging tactics employed by PCFG individuals along the Oregon, United States coast, including “headstands,” “bubble blasts,” and “sharking” on reefs, as well as documentation of whales expelling sediment from their mouths.
Abundance estimates of the ENP and PCFG indicate the population sizes are two orders of magnitude different, with the ENP estimated at ∼20,000 individuals (Stewart and Weller, 2021), while the PCFG contains only ∼250 individuals (Weller et al., 2013; Calambokidis et al., 2017). Genetic analysis has yet to resolve the degree of reproductive mixing between the ENP and PCFG sub-groups. Mitochondrial genetic data indicates low genetic exchange between sub-groups, yet microsatellite analysis showed no evidence that whales from the two different feeding grounds are reproductively isolated (Frasier et al., 2011; Lang et al., 2014). The relatively large size of the ENP population suggests that migrating all the way to the Arctic feeding grounds is advantageous despite the longer, and therefore more costly, migration. Increased prey quantity, quality, and availability in the Arctic potentially outweigh the trade-off of increased migration costs. Yet, PCFG whales are also able to gain critical energetic mass throughout a foraging season (Soledade Lemos et al., 2020) and successfully recruit calves (Calambokidis and Perez, 2017), all while migrating half as far. Hence, the mystery of the PCFG persists: Why would a gray whale not join the PCFG? Or is life as a PCFG gray whale harder than perceived, perhaps due to reduced prey quality?
To help unravel the mystery of the PCFG, this study aims to (1) assess the quality (caloric content) of different potential zooplankton prey on the Oregon coast, and (2) compare the quality of prey on the Oregon coast (as a proxy for the entire PCFG range) to the quality of prey in the Arctic ENP feeding grounds through extrapolations of gray whale energetic needs using published values in the literature. We hypothesize that Oregon coast prey will vary by species, reproductive stage, and time of year. Furthermore, we anticipate that Arctic prey (ampeliscid amphipods) will be more calorically rich than Oregon prey, contributing to the fact that the majority of ENP gray whales migrate to the Arctic to feed during the summer rather than traveling half as far to feed in the PCFG range. Our aim is to inform the foraging energetics of the PCFG through comparisons to the larger population of ENP gray whales and thus elucidate the existence of this small sub-group and inform population management efforts.
Collection of zooplankton samples was conducted near the coast of Newport (44°38′12″ N, 124°03′08″ W), Oregon, United States, between June and October from 2017 to 2019 as part of a larger study on gray whale ecology (Soledade Lemos et al., 2020). Collection occurred using a simple, low-cost light trap, which was a modified plastic water jug with an LED light placed inside to attract zooplankton (adapted from design in Chan et al., 2016). The light trap was deployed overnight at randomly selected nearshore sites where gray whales were observed foraging previously that day (Figure 1). The bottom habitat of the collection sites was rocky reef, with and without kelp. The trap was deployed overnight with an anchor and float line to keep the trap just above the seafloor. This system is effective at sampling epibenthic species associated with the benthos in rocky coastal habitats where it is difficult to sample using towed nets (Chan et al., 2016). The light trap was collected the subsequent day. In addition, Dungeness (Cancer magister) and porcelain (Porcellanidae sp.) crab larvae were opportunistically sampled in the Newport study area with nets where gray whales were observed feeding on surface swarms (Figure 1).
Figure 1. Locations of prey samples collected with a light trap (open circles) or opportunistic collections of surface swarms of crab larvae (black triangles) in Newport, along the Oregon coast in the Pacific Northwest coast of the United States.
Once collected, all samples were transferred to sterile jars and frozen at −20°C until subsequent sorting and calorimetric analysis. All samples were sorted to species level and separated by reproductive stage. All sorted samples were blotted and wet-weighed prior to being dried in a desiccating oven at 60°C between 48 and 72 h until they reached a constant mass. After reweighing, the dried samples were ground into a homogenous powder and, using a pellet press (Parr Instruments, Moline, IL, United States), were made into 2–100 mg pellets, depending on the amount of sample available each day. Pellet weights were recorded for each sample prior to calorimetry. Where possible, a minimum of three replicate pellets were made per sample. However, the small sample number of certain species or reproductive stages on some sampling days limited replication (Table 1).
Table 1. Energetic values (wet weight) and sample sizes by zooplankton species and reproductive group collected from June to October in 2017 to 2019 in central Oregon.
Pellets were combusted using a semi-micro bomb calorimeter (Model 6725, Parr Instruments, Moline, IL, United States). Calibrations of energetic density estimates were performed using 0.2 g benzoic acid (C6H5COOH) pellets and resultant caloric densities were corrected for uncombusted fuse wire and/or sample (Parr Instruments Company, 2010). Caloric densities with standard deviations (SD) are reported here in kilojoules per gram wet weight (kJ g– 1 WW).
All statistical analyses were conducted using R (version 4.0.2; R Core Team 2020). Data did not meet the assumptions of normality and homogeneity of variance, therefore Kruskal–Wallis rank sum tests were performed to assess differences in energetic value between species, reproductive stage, and time (month and year). If significant differences were detected, pairwise comparisons with the Dunn’s test were carried out. A Bonferroni correction was applied to account for multiple comparisons (Dunn, 1961). A total of eight Kruskal–Wallis tests were performed, resulting in a new Bonferroni-adjusted alpha value of 0.006 (0.05/8). Linear regressions were conducted to test for the effect of reproductive stage on caloric content with day of year (DOY).
Although PCFG gray whales show varying degrees of site fidelity to specific foraging sites within their range (Calambokidis et al., 2017), they also move between foraging sites within and between foraging seasons (Lagerquist et al., 2019). Hence, PCFG whales may gain their annual energetic needs from a variety of locations within the PCFG range. The nearshore bottom habitat along the central Oregon coast is predominantly rocky reef, with interspersed sedimentary areas (Romsos et al., 2007; L. Torres, pers. obs.). The prey associated with this habitat type (Table 1) are similar to those described for British Columbia (Dunham and Duffus, 2002; Feyrer, 2010; Feyrer and Duffus, 2011, 2015), Washington (Scordino et al., 2017), and northern California (Jenkinson, 2001). Therefore, for our extrapolations of PCFG gray whale caloric requirements from prey, we assume that (1) a similar suite of prey species is available to gray whales across the PCFG range as we captured off central Oregon, and (2) caloric estimates by species and reproductive stage documented in our analysis are similar across the PCFG range. However, we recognize that we did not measure the caloric content of all prey types known to be fed on by gray whales in this range, such as herring roe (Darling et al., 1998) and benthic amphipods (Burnham and Duffus, 2016), but these have never been captured in our study’s field site (Oregon). Since it is unlikely that gray whales feeding in the PCFG range exclusively consume one species of prey during the whole foraging season, we created a PCFG composite prey caloric value by calculating the mean caloric value of the six Oregon prey species tested in this study.
Caloric values of A. macrocephala, the predominant amphipod species in the Arctic feeding grounds of ENP gray whales (Nerini, 1984), were compiled from the published literature (Highsmith and Coyle, 1990, 1992; Hondolero et al., 2012; Wilt et al., 2014). These studies report the caloric content of A. macrocephala as dry weight values. In contrast, we have reported wet weight values of caloric content because this unit is relevant to foraging whales, as this is the form in which prey is consumed. Therefore, to facilitate comparison of the number of individual zooplankton by species needed to be consumed by a gray whale to meet their daily energetic needs, we converted the published average dry weight caloric content for A. macrocephala (4.473 kcal g– 1; Hondolero et al., 2012) to average wet weight caloric content (2.021 kJ g– 1) after obtaining the dry and wet weights of the samples used in that study (K. Iken, unpublished data).
Prey caloric values were extrapolated by species to the daily energetic requirements (MJ day– 1) for pregnant and lactating female ENP gray whales based on the results of Villegas-Amtmann et al. (2017) to estimate the requirements per day of (1) the biomass of prey, in metric tons, and (2) the number of individual prey items. Villegas-Amtmann et al. (2017) calculated daily energetic requirements for self-maintenance and costs of reproduction over a female’s 2-year reproductive cycle. Biomass (metric tons) of prey required per day was calculated by dividing the daily energetic requirement by the mean caloric value (MJ g– 1) of each prey species, divided by 1 million. Number of individual prey items required per day was calculated by dividing the biomass of prey required per day by the mean wet weight per individual of each prey species.
Light trap deployment depth was 11.2 ± SD 2.98 m. A total of 36 light traps were deployed, however, following recovery, five traps did not contain any zooplankton, likely due to a malfunction of the light used to attract zooplankton to the trap. Therefore, 31 light trap samples and three opportunistic surface samples were analyzed.
In total, nine zooplankton species were identified: the mysids Alienacanthomysis macropsis, Exacanthomysis davisi, Holmesimysis sculpta, and Neomysis rayii, the amphipods Atylus tridens, and Polycheria osborni, Dungeness crab megalopae and porcelain crab larvae, and an unknown species of Caprellidae. A. macropsis, Caprellidae sp., and E. davisi were rare, only being identified in 1, 1, and 4 light trap samples, respectively. These occurrences were represented by a single specimen, which prevented successful calorimetric determination for these three species.
For the mysids, gravid females were identified through the presence of a brood pouch that contained any developmental stage of offspring (from eggs to larvae). Individuals that had a brood pouch without any contents were classed as empty brood pouch females and considered to have already released their young. For A. tridens, gravid females were identified by a clutch of eggs in their pereopods. Reproductive stages could not be discerned for P. osborni due to its small size.
A total of 284 energetic values were determined (Table 1). Caloric content did not vary significantly between years (Kruskal–Wallis χ2 = 3.20, df = 2, p = 0.202). Therefore, samples were pooled across years for all subsequent analyses.
The energetic density between species (pooled across reproductive stage and month) varied significantly (Kruskal–Wallis χ2 = 123.38, df = 5, p ≥ 0.0001; Figure 2A). Dungeness crab megalopae (n = 13) had the highest mean energetic density (4.21 ± 1.27 kJ g– 1) and the amphipod P. osborni (n = 11) had the lowest mean energetic density (0.83 ± 0.21 kJ g– 1). Caloric values of Dungeness crab megalopae and the mysid N. rayii (2.42 ± 1.06 kJ g– 1) were significantly higher than those of the mysid H. sculpta (1.60 ± 0.66 kJ g– 1), porcelain crab larvae (1.17 ± 0.14 kJ g– 1), and the amphipods A. tridens (1.25 ± 0.57 kJ g– 1) and P. osborni (Dunn’s test, p < 0.001). Dungeness crab megalopae and N. rayii caloric contents were also significantly different from one another, with the former having the higher caloric value (Dunn’s test, p = 0.0006). The caloric contents of H. sculpta and P. osborni differed significantly (Dunn’s test, p = 0.0006), with H. sculpta having a higher caloric content. All other pairwise comparisons were not significant (Dunn’s test, p > 0.006). Comparison of the mean caloric values of the prey species tested in this study to the mean caloric value of Arctic amphipod A. macrocephala (2.02 kJ g– 1) showed that two Oregon zooplankton species have higher caloric contents, namely Dungeness crab megalopae and N. rayii (Table 1). The PCFG composite prey caloric value was 1.91 kJ g– 1.
Figure 2. Median caloric content and interquartile ranges (kJ g– 1 wet weight) by (A) species, (B) reproductive stage, and (C) month. Sizes of the zooplankton images are scaled at actual ratios relative to one another.
Since significant differences between species were found, analyses to determine whether energetic values vary by reproductive stage and month were carried out within each species. Sufficient replicate samples for different reproductive stages and months were only obtained for the two mysid species (N. rayii and H. sculpta) and the amphipod A. tridens.
Reproductive stages had significantly different caloric values for H. sculpta (Kruskal–Wallis χ2 = 10.34, df = 2, p = 0.005), however, not for N. rayii (Kruskal–Wallis χ2 = 9.49, p = 0.008) nor A. tridens (Kruskal–Wallis χ2 = 3.35, p = 0.187) (Figure 2B). For H. sculpta, females with empty brood pouches had significantly lower caloric values (1.23 ± 0.49 kJ g– 1) than gravid females (1.74 ± 0.58 kJ g– 1; Dunn’s test, p = 0.0007), but not than individuals with no brood pouch (1.65 ± 0.73 kJ g– 1; Dunn’s test, p = 0.008). No significant differences were identified between H. sculpta gravid females and individuals without a brood pouch (Dunn’s test, p > 0.1).
Significant differences in energetic content between months were only detected for H. sculpta (Kruskal–Wallis χ2 = 15.38, df = 4, p = 0.004; Figure 2C), whereby September was significantly higher than all other months (June, July, August; Dunn’s test, p < 0.002), except October (Dunn’s test, p > 0.4). Linear regressions of caloric content by reproductive stage within each species over time (DOY) revealed that the energetic value of the gravid reproductive stage increased significantly throughout the season for both mysids, H. sculpta (F1,31 = 15.71, p = 0.0004, R2 = 0.32) and N. rayii (F1,35 = 6.138, p = 0.0182, R2 = 0.12) (Figure 3). All other linear regressions conducted were not significant (p > 0.2; Figure 3).
Figure 3. Caloric content (kJ g–1 wet weight) of different reproductive stages (empty brood pouch, gravid, and no brood pouch) as a function of day of year (DOY; ranging from June to October) for the mysids Holmesimysis sculpta and Neomysis rayii, and the amphipod Atylus tridens. A. tridens is only represented on one panel due to small sample size of this species for the empty brood pouch and gravid reproductive stages. Asterisks indicate significant regressions (p < 0.02).
A gray whale feeding exclusively on Dungeness crab megalopae, the highest caloric prey tested in this study (Table 1), would meet its daily energetic requirements by consuming 0.95 or 0.64 (pregnant or lactating, respectively) metric tons day– 1 (Figure 4A). In contrast, a gray whale feeding exclusively on the amphipod P. osborni, the lowest caloric prey tested in this study (Table 1), would meet its daily energetic requirements by consuming 4.82 or 3.25 (pregnant or lactating, respectively) metric tons day– 1 (Figure 4A). A pregnant female would need to consume 1.65, 1.98, 2.50, 3.20, and 3.42 metric tons day– 1, while a lactating female would require 1.12, 1.34, 1.69, 2.16, and 2.31 metric tons day– 1 of N. rayii, A. macrocephala, H. sculpta, A. tridens, and porcelain crab larvae, respectively (Figure 4A). A gray whale feeding on the PCFG composite prey would meet its daily energetic requirements by consuming 2.09 or 1.41 (pregnant or lactating, respectively) metric tons day– 1 (Figure 4A).
Figure 4. Daily prey requirements (A: metric tons; B: number of individuals) needed by pregnant and lactating female gray whales to meet their energetic requirements on the foraging ground. Energetic requirement estimates obtained from Villegas-Amtmann et al. (2017). Note logarithmic scale of y-axis in panel (B).
The number of individual zooplankton a gray whale needs to consume to achieve its daily energetic requirement varies widely by prey species (Figure 4B). The smallest total number of individual prey a gray whale would need daily is 26.8 or 18.1 million individuals of N. rayii, depending on if the gray whale is a pregnant or lactating individual, respectively (Figure 4B). In contrast, if a gray whale fed solely on porcelain crab larvae, the greatest number of individual prey items would be needed, totaling 5,698 or 3,846 million individuals day– 1, depending on the stage of the gray whale (pregnant or lactating, respectively; Figure 4B). A pregnant female would need to consume 26.9, 28.4, 156.9, 222.2, and 2,421 million individuals day– 1, while a lactating female would require 18.2, 19.1, 105.9, 150.0, and 1,634 million individuals day– 1 of Dungeness crab megalopae, A. macrocephala, H. sculpta, A. tridens, and P. osborni, respectively (Figure 4B). A gray whale feeding on the PCFG composite prey would need to capture 96.6 or 65.2 (pregnant or lactating, respectively) million individuals day– 1 (Figure 4B) to meet its daily energetic requirements.
Contrary to our hypothesis, Arctic prey (ampeliscid amphipods) are not the most calorically rich prey species available to gray whales in the eastern North Pacific (of the seven species considered in this study). Rather, two prey species collected in the PCFG range along the Oregon coast, Dungeness crab megalopae and the mysid N. rayii, had higher caloric values than A. macrocephala, which is the predominant ampeliscid amphipod species in the Arctic ENP feeding grounds. Given that the PCFG sub-group of gray whales is considerably smaller (∼250 individuals; Calambokidis et al., 2017) than the ENP (∼20,000; Stewart and Weller, 2021), our comparative results suggest that caloric value of prey may not be a driver in the larger population size of the ENP, but rather prey abundance, depth, habitat, and patch density may influence gray whale foraging ground use.
Baleen whales must hold their breath while diving to search for, locate and consume their prey, making foraging behavior energetically costly for rorqual whales (e.g., blue and humpback whales; Acevedo-Gutiérrez et al., 2002) and likely for gray whales too, which spend only 17% of their time at the surface while feeding (Stelle et al., 2008). Hence, whales must ensure they target prey patches that are dense enough to compensate for these costs (Goldbogen et al., 2012; Torres et al., 2020). Historically, ENP gray whales foraged in high abundances in the northern Bering Sea, particularly in the Chirikov Basin (Johnson and Nelson, 1984), where the benthic amphipod community is considered one of the most productive in the world, with the predominant species, A. macrocephala, reported as being the most productive benthic marine amphipod species (Highsmith and Coyle, 1990). In the 1980s the mean abundance of A. macrocephala was 2,499 ± 1,183 individuals m– 2 (Highsmith and Coyle, 1990), however, recent density estimates demonstrate a large reduction, of up to 50% in some areas (Moore et al., 2003; Coyle et al., 2007b). This reduction in benthic prey populations has occurred simultaneously to increased pelagic fish populations, a reduction of sea ice, and increased air and ocean temperatures, and it is believed that a major ecosystem shift from arctic to subarctic conditions is occurring in the northern Bering Sea (Grebmeier et al., 2006). Coincident with this decline in primary prey and ecosystem change, the foraging habitat of ENP gray whales has shifted further north into the Chukchi Sea to an area where water depths range from 40 to 60 m (Moore et al., 2003; Bluhm et al., 2007; Brower et al., 2017, 2018), which is markedly deeper than where gray whale foraging was previously concentrated in the shelf waters of the northern Bering Sea (20–40 m; Coachman et al., 1975). The increased depth range means that benthically feeding ENP gray whales must dive deeper than previously, which may increase the energetic cost of foraging. In contrast, along the Oregon coast, PCFG gray whales typically forage in water depths that are on average four times shallower (mean = 12.7 m, SD = 3.98 m, n = 345 sightings, L. Torres unpublished data) than feeding habitat in the Chukchi Sea. The shallow depth of PCFG foraging habitat not only reduces dive time and associated energetic costs, but likely also reduces the amount of surface recovery time needed in between dives, thereby increasing the amount of foraging time available to PCFG whales. However, foraging depth does not account for prey availability and therefore is not directly related to consumption rates or energetic costs. Thus, empirical data of foraging gray whale bioenergetics (i.e., kinematics, respiratory patterns) paired with prey availability information are needed to evaluate these hypotheses regarding cost-benefit trade-offs between PCFG and ENP gray whales. Nevertheless, it appears that PCFG whales have three advantages over ENP whales: a considerably shorter migration (Calambokidis et al., 2002), greater or equal prey caloric value, and possible behavioral energetic advantages of shallow foraging habitat. Despite this, the vast majority of gray whales in the North Pacific forage in the Arctic, not the PCFG range. Therefore, we hypothesize that prey within the PCFG range have reduced availability, predictability, and patch density compared to ENP foraging grounds in the Arctic.
Dungeness crab megalopae were the most calorically rich prey tested in this study. A gray whale, regardless of reproductive stage, would need less than half of the required amount of A. macrocephala to reach its daily energetic requirements if it only consumed Dungeness crab megalopae. However, Dungeness crab megalopae occurrence on the Oregon coast is not consistent throughout the summer due to the seasonality of Dungeness crab reproduction (Roegner et al., 2007), with the megalopae larvae only available to nearshore foraging gray whales in the late spring and early summer (Shanks and Roegner, 2007). Therefore, while Dungeness crab megalopae can be considered caloric “gold mines,” they are an ephemeral prey resource for foraging gray whales. Consequently, PCFG gray whales must be flexible foragers with a diverse diet and the ability to switch between prey types as availability dictates.
In contrast to Dungeness crab megalopae, the mysid species N. rayii and H. sculpta are more consistently available throughout the summer along the PCFG range due to their continuous iteroparous reproduction (Mauchline, 1980). While the energetic benefit of feeding on N. rayii is not as large of a benefit as feeding on Dungeness crab megalopae, a diet of the former would result in a gray whale in either reproductive stage requiring approximately one million less individual prey items a day than if it fed on A. macrocephala in the Arctic (Figure 4B). Sighting rates of PCFG whales peak toward the end of the feeding season in August and October (Scordino et al., 2017) and may be explained by the concurrent seasonal increase in caloric content of prey, particularly of gravid mysids (Figure 3). Mysids aggregate by size class and reproductive stage (Kaltenberg and Benoit-Bird, 2013), making patches of gravid individuals with high caloric content even more profitable to foraging gray whales. However, little is known about mysid patch density and distribution due to their preferred shallow, rocky, often kelp-dominated, habitat that makes net-tow and hydroacoustic measurements challenging. The wide range of mysid density estimates within the PCFG range (714–15,541 individuals m– 3) illustrate the high variability and patchiness of this prey resource to gray whales (Kaltenberg and Benoit-Bird, 2013; Feyrer and Duffus, 2015). At small scales, fluctuating oceanographic conditions, such as internal waves, currents, and tides (Prairie et al., 2012), and swarming behaviors, including antipredator responses (Folt and Burns, 1999; Kaltenberg and Benoit-Bird, 2013), drive mysid patch dynamics. These factors make fine-scale mysid density hard to measure since patches may form and disperse faster than they can be quantified (Feyrer and Duffus, 2015).
While caloric content and patch density impact the energetic benefit of foraging decisions, whale foraging behavior can significantly impact the energetic cost side of the equation. Mysid species may be more continuously available throughout the foraging season in the PCFG range, but their patchy distribution may require a gray whale to search harder and/or longer before it finds a suitably dense prey patch to offset foraging costs, as compared to a potentially more stable distribution of A. macrocephala in the Arctic. Two previous studies have associated poor body condition of PCFG gray whales with unfavorable environmental conditions linked with poor prey availability and the costs associated with increased time spent searching (Newell and Cowles, 2006; Soledade Lemos et al., 2020). When mysid patches become less dense and/or abundant, PCFG gray whales may have to work harder to find enough prey to meet their energetic demands. However, the risk of this patchier prey availability in the PCFG range relative to A. macrocephala in the Arctic region may be compensated for by the elevated caloric content of N. rayii and especially Dungeness crab megalopae.
Furthermore, the energetic input required of a gray whale to capture surface swarms of crab larvae, including both Dungeness crab megalopae and porcelain crab larvae, appears to be very low based on field observations and drone footage (L. Torres, pers obs). When feeding on both species of crab larvae, gray whales appear to exert little energy as they lay at the surface, with minor forward movement, and repeatedly open and close their mouths to capture and filter prey (Supplementary Video). In contrast, PCFG whales are often observed working harder (qualitative assessment based on body dynamics and breathing rates) when foraging on mysids that are commonly found in rock crevices of reef systems and kelp forests (Torres et al., 2018; L. Torres, pers obs). Torres et al. (2018) documented several unique behaviors displayed by PCFG gray whales while foraging in reef habitat, including “bubble blasts” where whales release air while underwater presumably to access or aggregate prey, and “headstands” where whales position themselves head down, flukes up in the water column to push their head/mouth region into the substrate to capture prey. While these qualitative assessments do not account for any variation of internal biomechanics of prey consumption (i.e., gular and tongue pumping), it does suggest that foraging on mysids and benthic amphipods may be more energetically costly than feeding on surface crab larvae. However, quantitative assessments of the energetic requirements of these different foraging behaviors are required to confirm this hypothesis.
It is possible that PCFG whales do not exclusively feed along the West Coast range, but perhaps switch foraging ground use relative to environmental and prey conditions. Classification of a PCFG whale is defined as an individual documented in the range between northern California to northern British Columbia (from 41°N to 52°N) between June 1 and November 30 in at least two different years (International Whaling Commission (IWC), 2012). While this definition requires an individual to show some degree of site fidelity to the PCFG range, it does not preclude PCFG whales from utilizing areas outside of the PCFG range. In fact, satellite tag and photo identification data demonstrate that PCFG individuals utilize areas outside of the PCFG range (i.e., Icy Strait and Kodiak Island, Alaska, United States) in the late spring and early summer, before spending the majority of the summer feeding within the PCFG range (Mate et al., 2010; Gosho et al., 2011; Ford et al., 2013). The PCFG’s small abundance estimate and evidence of matrilineal fidelity (Calambokidis and Perez, 2017) suggests that knowledge of this alternative foraging ground could be culturally transmitted (e.g., Valenzuela et al., 2009; Baker et al., 2013), and, as our study demonstrates, potentially advantageous. Comparative assessments of recruitment rates and population dynamics between the PCFG and ENP relative to oceanographic factors and prey availability may elucidate the relative advantage of this cultural group, and reveal whether feeding in the PCFG range is an exclusive behavior or used as an ecological safety net during unfavorable conditions in the Arctic.
Although our study demonstrates that some prey species found in the PCFG gray whale range have greater caloric value than the predominant prey in ENP feeding grounds in the Arctic, thus enabling PCFG whales to potentially meet their daily energetic requirements faster, the vast majority of gray whales in the North Pacific feed on ampeliscid amphipods in the Arctic. If we assume that gray whales forage optimally (MacArthur and Pianka, 1966; Charnov, 1976), this conundrum indicates that prey patch density and predictability, and behavioral cost of capture likely play a significant role in the trade-offs of foraging ground use. However, reduction of ampeliscid amphipod biomass from the 1980s to the 2000s (Moore et al., 2003; Coyle et al., 2007b) and rapidly changing environmental conditions in the Arctic (Johannessen et al., 2004; Overland and Stabeno, 2004; Wang et al., 2012; Box et al., 2019), raise questions about the stability of this Arctic food resource to gray whales. In fact, ENP gray whales have undergone two unusual mortality events (UME) in the last two decades (1999–2000 and 2019-present), with elevated strandings of emaciated whales likely due to reduced prey availability as a function of the population exceeding carrying capacity (Rugh et al., 2005; Coyle et al., 2007b) and/or environmental change (Le Boeuf et al., 2000; Moore et al., 2001, 2003; Stewart and Weller, 2021). Furthermore, ENP calf production is correlated with spring sea ice conditions in the Arctic, with early access to prey by gestating females resulting in higher calf production in the subsequent year (Perryman et al., 2020). Yet, ongoing climate change is predicted to alter this pattern in the future (Perryman et al., 2020). Even though the abundance estimates for the PCFG have remained relatively stable since 2002 (Calambokidis et al., 2017), environmental changes are also occurring within the PCFG range, with increased occurrence of marine heat waves (Gentemann et al., 2017; Joh and Di Lorenzo, 2017; Frölicher et al., 2018) and resultant changes in prey communities (Peterson et al., 2017; Brodeur et al., 2019). To understand and anticipate gray whale population response to these impacts of climate change, it is important to monitor their prey availability, foraging ecology, and health (i.e., body condition) at both foraging grounds. Addressing these topics will enhance management of both the ENP and PCFG gray whale populations and further our understanding of the factors that limit the carrying capacity of the PCFG region.
The raw data supporting the conclusions of this article will be made available by the authors, without undue reservation.
LH contributed to project development, data collection, data processing, led data analysis, and led manuscript writing. KB provided access to lab and calorimetry instruments, oversaw caloric lab and data analysis, and provided edits to the manuscript. LT conceived the project, acquired funding for fieldwork, oversaw data collection and analysis, and contributed to manuscript preparation. All authors contributed to the article and approved the submitted version.
Funding for this research was provided by the NOAA National Marine Fisheries Service Office of Science and Technology Ocean Acoustics Program, Oregon Sea Grant Program Development Funds, and the Oregon State University (OSU) Marine Mammal Institute and Agricultural Research Foundation.
The authors declare that the research was conducted in the absence of any commercial or financial relationships that could be construed as a potential conflict of interest.
We would like to thank research assistants Robyn Norman and Haley Kent (OSU) for assistance with zooplankton sorting and identification, and Katrin Iken for sharing data on Arctic amphipod dry/wet weight conversions with us. We are very grateful to Dave Weller and Jorge Urbán Ramírez for their insightful comments that improved this manuscript.
The Supplementary Material for this article can be found online at: https://www.frontiersin.org/articles/10.3389/fmars.2021.683634/full#supplementary-material
Acevedo-Gutiérrez, A., Croll, D. A., and Tershy, B. R. (2002). High feeding costs limit dive time in the largest whales. J. Exp. Biol. 205, 1747–1753. doi: 10.1242/jeb.205.12.1747
Baker, C. S., Steel, D., Calambokidis, J., Falcone, E., González-Peral, U., Barlow, J., et al. (2013). Strong maternal fidelity and natal philopatry shape genetic structure in North Pacific humpback whales. Mar. Ecol. Prog. Ser. 494, 291–306. doi: 10.3354/meps10508
Bluhm, B. A., Coyle, K. O., Konar, B., and Highsmith, R. (2007). High gray whale relative abundances associated with an oceanographic front in the south-central Chukchi Sea. Deep. Res. 2 Top. Stud. Oceanogr. 54, 2919–2933. doi: 10.1016/j.dsr2.2007.08.015
Box, J. E., Colgan, W. T., Christensen, T. R., Schmidt, N. M., Lund, M., Parmentier, F. J. W., et al. (2019). Key indicators of Arctic climate change: 1971-2017. Environ. Res. Lett. 14:045010. doi: 10.1088/1748-9326/aafc1b
Brodeur, R. D., Auth, T. D., and Phillips, A. J. (2019). Major shifts in pelagic micronekton and macrozooplankton community structure in an upwelling ecosystem related to an unprecedented marine heatwave. Front. Mar. Sci. 6:1–15. doi: 10.3389/fmars.2019.00212
Brower, A. A., Clarke, J. T., and Ferguson, M. C. (2018). Increased sightings of subArctic cetaceans in the eastern Chukchi Sea, 2008–2016: population recovery, response to climate change, or increased survey effort? Polar Biol. 41, 1033–1039. doi: 10.1007/s00300-018-2257-x
Brower, A. A., Ferguson, M. C., Schonberg, S. V., Jewett, S. C., and Clarke, J. T. (2017). Gray whale distribution relative to benthic invertebrate biomass and abundance: northeastern Chukchi Sea 2009–2012. Deep. Res. 2 Top. Stud. Oceanogr. 144, 156–174. doi: 10.1016/j.dsr2.2016.12.007
Burnham, R. E., and Duffus, D. A. (2016). Gray whale (Eschrichtius robustus) predation and the demise of amphipod prey reserves in Clayoquot Sound. British Columbia. Aquat. Mamm. 42, 123–126. doi: 10.1578/AM.42.2.2016.123
Calambokidis, J., Darling, J. D., Deecke, V., Gearin, P., Gosho, M., Megill, W., et al. (2002). Abundance, range and movements of a feeding aggregation of gray whales (Eschrichtius robustus) from California to southeastern Alaska in 1998. J. Cetacean Res. Manag. 4, 267–276. doi: 10.1016/0022-247X(92)90046-G
Calambokidis, J., Laake, J., and Perez, A. (2017). “Updated analysis of abundance and population structure of seasonal gray whales in the Pacific Northwest, 1996-2015” in International Whaling Commission, SC/A17/GW/05 for the Workshop on the Status of North Pacific Gray Whales. La Jolla: IWC.
Calambokidis, J., and Perez, A. (2017). “Sightings and follow-up of mothers and calves in the PCFG and implications for internal recruitment” in IWC Report SC/A17/GW/04 for the Workshop on the Status of North Pacific Gray Whales. (La Jolla: IWC).
Chan, B. K. K., Shao, K.-T., Shao, Y.-T., and Chang, Y.-W. (2016). A simplified, economical, and robust light trap for capturing benthic and pelagic zooplankton. J. Exp. Mar. Bio. Ecol. 482, 25–32. doi: 10.1016/j.jembe.2016.04.003
Charnov, E. L. (1976). Optimal foraging, the marginal value theorem. Theor. Popul. Biol. 9, 129–136. doi: 10.1016/0040-5809(76)90040-X
Coachman, L. K., Aagaard, K., and Tripp, R. B. (1975). Bering Strait: the regional physical oceanography. Seattle: University of Washington Press.
Coyle, K. O., Bluhm, B., Konar, B., Blanchard, A., and Highsmith, R. C. (2007a). Amphipod prey of gray whales in the northern Bering Sea: comparison of biomass and distribution between the 1980s and 2002 – 2003. Deep. Res. 2 Top. Stud. Oceanogr. 54, 2906–2918. doi: 10.1016/j.dsr2.2007.08.026
Coyle, K. O., Konar, B., Blanchard, A., Highsmith, R. C., Carroll, J., Carroll, M., et al. (2007b). Potential effects of temperature on the benthic infaunal community on the southeastern Bering Sea shelf: possible impacts of climate change. Deep. Res. 2 Top. Stud. Oceanogr. 54, 2885–2905. doi: 10.1016/j.dsr2.2007.08.025
Coyle, K. O., and Highsmith, R. C. (1994). Benthic amphipod community in the northern Bering Sea - Analysis of potential structuring mechanisms. Mar. Ecol. Prog. Ser. 107, 233–244. doi: 10.3354/meps107233
Croll, D. A., Acevedo-Gutiérrez, A., Tershy, B. R., and Urbán-Ramírez, J. (2001). The diving behavior of blue and fin whales: is dive duration shorter than expected based on oxygen stores? Comp. Biochem. Physiol. A Mol Integr Physiol. 129, 797–809. doi: 10.1016/S1095-6433(01)00348-8
Croll, D. A., Marinovic, B., Benson, S., Chavez, F. P., Black, N., Ternullo, R., et al. (2005). From wind to whales: trophic links in a coastal upwelling system. Mar. Ecol. Prog. Ser. 289, 117–130. doi: 10.3354/meps289117
Darling, J. D., Keogh, K. E., and Steeves, T. E. (1998). Gray whale (Eschrichtius robustus) habitat utilization and prey species off Vancouver Island. B.C. Mar. Mammal Sci. 14, 692–720. doi: 10.1111/j.1748-7692.1998.tb00757.x
Doniol-Valcroze, T., Lesage, V., Giard, J., and Michaud, R. (2011). Optimal foraging theory predicts diving and feeding strategies of the largest marine predator. Behav. Ecol. 22, 880–888. doi: 10.1093/beheco/arr038
Duffus, D. A. (1996). The recreational use of grey whales in southern Clayoquot Sound. Canada. Appl. Geogr. 16, 179–190. doi: 10.1016/0143-6228(96)00002-1
Dunham, J. S., and Duffus, D. A. (2002). Diet of gray whales (Eschrichtius robustus) in Clayoquot Sound. British Columbia, Canada. Mar. Mammal Sci. 18, 419–437. doi: 10.1111/j.1748-7692.2002.tb01046.x
Dunn, O. J. (1961). Multiple Comparisons Among Means. J. Am. Stat. Assoc. 56, 52–64. doi: 10.2307/2282330
Feyrer, L. J. (2010). Differences in embryo production between sympatric species of mysids (family Mysidae) in the shallow coastal waters off Vancouver Island. BC. Mar. Biol. 157, 2461–2465. doi: 10.1007/s00227-010-1510-9
Feyrer, L. J., and Duffus, D. A. (2011). Predatory disturbance and prey species diversity: the case of gray whale (Eschrichtius robustus) foraging on a multi-species mysid (family Mysidae) community. Hydrobiologia 678, 37–47. doi: 10.1007/s10750-011-0816-z
Feyrer, L. J., and Duffus, D. A. (2015). Threshold foraging by gray whales in response to fine scale variations in mysid density. Mar. Mammal Sci. 31, 560–578. doi: 10.1111/mms.12178
Folt, C. L., and Burns, C. W. (1999). Biological drivers of zooplankton patchiness. Trends Ecol. Evol. 14, 300–305. doi: 10.1016/s0169-5347(99)01616-x
Ford, J. K. B., Durban, J. W., Ellis, G. M., Towers, J. R., Pilkington, J. F., Barrett-Lennard, L. G., et al. (2013). New insights into the northward migration route of gray whales between Vancouver Island, British Columbia, and southeastern Alaska. Mar. Mammal Sci. 29, 325–337. doi: 10.1111/j.1748-7692.2012.00572.x
Frasier, T. R., Koroscil, S. M., White, B. N., and Darling, J. D. (2011). Assessment of population substructure in relation to summer feeding ground use in the eastern North Pacific gray whale. Endanger. Species Res. 14, 39–48. doi: 10.3354/esr00340
Frölicher, T. L., Fischer, E. M., and Gruber, N. (2018). Marine heatwaves under global warming. Nature 560, 360–364. doi: 10.1038/s41586-018-0383-9
Gentemann, C. L., Fewings, M. R., and García-Reyes, M. (2017). Satellite sea surface temperatures along the West Coast of the United States during the 2014–2016 northeast Pacific marine heat wave. Geophys. Res. Lett. 44, 312–319. doi: 10.1002/2016GL071039
Goldbogen, J. A., Calambokidis, J., Croll, D. A., Mckenna, M. F., Oleson, E., Potvin, J., et al. (2012). Scaling of lunge-feeding performance in rorqual whales: mass-specific energy expenditure increases with body size and progressively limits diving capacity. Funct. Ecol. 26, 216–226. doi: 10.1111/j.1365-2435.2011.01905.x
Goldbogen, J. A., Calambokidis, J., Oleson, E., Potvin, J., Pyenson, N. D., Schorr, G., et al. (2011). Mechanics, hydrodynamics and energetics of blue whale lunge feeding: efficiency dependence on krill density. J. Exp. Biol. 214, 131–146. doi: 10.1242/jeb.054726
Goldbogen, J. A., Friedlaender, A. S., Calambokidis, J., McKenna, M. F., Simon, M., and Nowacek, D. P. (2013). Integrative approaches to the study of baleen whale diving behavior, feeding performance, and foraging ecology. Bioscience 63, 90–100. doi: 10.1525/bio.2013.63.2.5
Gosho, M., Gearin, P., Jenkinson, R., Laake, J., Mazzuca, L., Kubiak, D., et al. (2011). “Movements and diet of gray whales (Eschrichtius robustus) off Kodiak Island, Alaska, 2002-2005” in Paper SC/M11/AWMP2 presented to the International Whaling Commission AWMP Workshop. (United Kingdom: International Whaling Commission).
Grebmeier, J. M., Overland, J. E., Moore, S. E., Farley, E. V., Carmack, E. C., Cooper, L. W., et al. (2006). A major ecosystem shift in the northern Bering Sea. Science 311, 1461–1464. doi: 10.1126/science/1121365
Hazen, E. L., Friedlaender, A. S., and Goldbogen, J. A. (2015). Blue whales (Balaenoptera musculus) optimize foraging efficiency by balancing oxygen use and energy gain as a function of prey density. Sci. Adv. 1:e1500469. doi: 10.1126/sciadv.1500469
Highsmith, R. C., and Coyle, K. O. (1990). High productivity of northern Bering Sea benthic amphipods. Lett. Nat. 344, 862–864. doi: 10.1038/344862a0
Highsmith, R. C., and Coyle, K. O. (1992). Productivity of arctic amphipods relative to gray whale energy requirements. Mar. Ecol. Prog. Ser. 83, 141–150. doi: 10.3354/meps083141
Hondolero, D., Bluhm, B. A., and Iken, K. (2012). Caloric content of dominant benthic species from the northern Bering and Chukchi Seas : historical comparisons and the effects of preservation. Polar Biol. 35, 637–644. doi: 10.1007/s00300-011-1107-x
Hyrenbach, K. D., Forney, K. A., and Dayton, P. K. (2000). Marine protected areas and ocean basin management. Aquat. Conserv. Mar. Freshw. Ecosyst. 10, 437–458.
International Whaling Commission (IWC). (2012). Report of the Scientific Committee. J. Cetacean Res. Manag. 1, 1–52.
Jenkinson, R. S. (2001). Gray Whale (Eschrichtius robustus) Prey Availability and Feeding Ecology in Northern California, 1999-2000. Arcata: Humboldt State University. Master’s thesis.
Joh, Y., and Di Lorenzo, E. (2017). Increasing Coupling Between NPGO and PDO Leads to Prolonged Marine Heatwaves in the Northeast Pacific. Geophys. Res. Lett. 44, 11,663–11,671. doi: 10.1002/2017GL075930
Johannessen, O. M., Bengtsson, L., Miles, M. W., Kuzmina, S. I., Semenov, V. A., Alekseev, G. V., et al. (2004). Arctic climate change: observed and modelled temperature and sea-ice variability. Tellus 56A, 328–341. doi: 10.1111/j.1600-0870.2004.00092.x
Johnson, K. R., and Nelson, C. H. (1984). Side-scan sonar assessment of gray whale feeding in the Bering Sea. Science 225, 1150–1151. doi: 10.1126/science.225.4667.1150
Kaltenberg, A. M., and Benoit-Bird, K. J. (2013). Intra-patch clustering in mysid swarms revealed through multifrequency acoustics. ICES J. Mar. Sci. 70, 883–891. doi: 10.1093/icesjms/fst176
Lagerquist, B. A., Palacios, D. M., Winsor, M. H., Irvine, L. M., Follett, T. M., and Mate, B. R. (2019). Feeding home ranges of pacific coast feeding group gray whales. J. Wildl. Manag. 83, 925–937. doi: 10.1002/jwmg.21642
Lang, A. R., Calambokidis, J., Scordino, J., Pease, V. L., Klimek, A., Burkanov, V. N., et al. (2014). Assessment of genetic structure among eastern North Pacific gray whales on their feeding grounds. Mar. Mammal Sci. 30, 1473–1493. doi: 10.1111/mms.12129
Le Boeuf, B. J., Perez-Cortes, M. H., Urbán, R. J., Mate, B. R., and Ollervides, U. F. (2000). High gray whale mortality and low recruitment in 1999: potential causes and implications. (Eschrichtius robustus). J. Cetacean Res. Manag 2, 85–99.
Lee, R. F., Hagen, W., and Kattner, G. (2006). Lipid storage in marine zooplankton. Mar. Ecol. Prog. Ser. 307, 273–306. doi: 10.3354/meps307273
Lockyer, C. (1984). Review of baleen whale (Mysticeti) reproduction and implications for management. Rep. Int. Whal. Comm. 6, 27–50.
Louzao, M., Wiegand, T., Bartumeus, F., and Weimerskirch, H. (2014). Coupling instantaneous energy-budget models and behavioural mode analysis to estimate optimal foraging strategy: an example with wandering albatrosses. Mov. Ecol. 2:8. doi: 10.1186/2051-3933-2-8
MacArthur, R. H., and Pianka, E. R. (1966). On Optimal Use of a Patchy Environment. Am. Nat. 100, 603–609. doi: 10.1086/282454
Mate, B. R., Lagerquist, B., and Irvine, L. (2010). “Feeding habitats, migrations and winter reproductive range movements derived from satellite-monitored radio tags on eastern North Pacific gray whales” in SC/62/BRG21 Presented to the Scientific Committee of the International Whaling Commission. (Agadir: IWC).
Mauchline, J. (1980). “The biology of mysids and euphausiids” in Advances in Marine Biology. eds J. H. S. Blaxter, F. S. Russell, and M. Yonge (London: Academic Press). 3–369.
Moore, S. E., Grebmeier, J. M., and Davies, J. R. (2003). Gray whale distribution relative to forage habitat in the northern Bering Sea: current conditions and retrospective summary. Can. J. Zool. 81, 734–742. doi: 10.1139/z03-043
Moore, S. E., Jorge Urbán, R., Perryman, W. L., Gulland, F., Hector Perez-Cortes, M., Wade, P. R., et al. (2001). Are gray whales hitting “K” hard? Mar. Mammal Sci. 17, 954–958. doi: 10.1111/j.1748-7692.2001.tb01310.x
Nelson, T. A., Duffus, D. A., Robertson, C., and Feyrer, L. J. (2008). Spatial-temporal patterns in intra-annual gray whale foraging: characterizing interactions between predators and prey in Clayquot Sound, British Columbia, Canada. Mar. Mammal Sci. 24, 356–370. doi: 10.1111/j.1748-7692.2008.00190.x
Nerini, M. K. (1984). “A review of gray whale feeding ecology” in The Gray Whale, Eschrichtius Robustus. eds M. Jones, S. L. Swartz, and S. Leatherwood (Cambridge: Academic Press). 423–448. doi: 10.1016/b978-0-08-092372-7.50024-8
Nerini, M. K., and Oliver, J. S. (1983). Gray Whales and the Structure of the Bering Sea Benthos. Oecologia 59, 224–225. doi: 10.1007/bf00378840
Newell, C. L., and Cowles, T. J. (2006). Unusual gray whale Eschrichtius robustus feeding in the summer of 2005 off the central Oregon coast. Geophys. Res. Lett. 33, L22S11. doi: 10.1029/2006GL027189
Nickels, C. F., Sala, L. M., and Ohman, M. D. (2018). The morphology of euphausiid mandibles used to assess selective predation by blue whales in the southern sector of the California Current System. J. Crustac. Biol. 38, 563–573. doi: 10.1093/jcbiol/ruy062
Overland, J. E., and Stabeno, P. J. (2004). Is the climate of the Bering Sea warming and affecting the ecosystem? EOS 85, 309–316. doi: 10.1029/2004EO330001
Owen-Smith, N., Fryxell, J. M., and Merrill, E. H. (2010). Foraging theory upscaled: the behavioural ecology of herbivore movement. Philos. Trans. R. Soc. B Biol. Sci. 365, 2267–2278. doi: 10.1098/rstb.2010.0095
Parr Instruments Company. (2010). Parr Operating Instruction Manual No. 457M, 6725 Semimicro Calorimeter. USA: Parr Instruments Company.
Perryman, W. L., Joyce, T., Weller, D. W., and Durban, J. W. (2020). Environmental factors influencing eastern North Pacific gray whale calf production 1994-2016. Mar. Mammal Sci. 37, 448–462. doi: 10.1111/mms.12755
Peterson, W. T., Fisher, J. L., Strub, P. T., Du, X., Risien, C., Peterson, J., et al. (2017). The pelagic ecosystem in the Northern California Current off Oregon during the 2014–2016 warm anomalies within the context of the past 20 years. J. Geophys. Res. Ocean. 122, 7267–7290. doi: 10.1002/2017JC012952
Piatt, J. F., and Methven, D. A. (1992). Threshold foraging behavior of baleen whales. Mar. Ecol. Prog. Ser. 84, 205–210. doi: 10.3354/meps084205
Prairie, J. C., Sutherland, K. R., Nickols, K. J., and Kaltenberg, A. M. (2012). Biophysical interactions in the plankton: a cross-scale review. Limnol. Oceanogr. Fluids Environ. 2, 121–145. doi: 10.1215/21573689-1964713
Rice, D. W., and Wolman, A. A. (1971). Life History and Ecology of the Gray Whales (Eschrichtius Robustus). Oklahoma: American Society of Mammalogists.
Roegner, G. C., Armstrong, D. A., and Shanks, A. L. (2007). Wind and tidal influences on larval crab recruitment to an Oregon estuary. Mar. Ecol. Prog. Ser. 351, 177–188. doi: 10.3354/meps07130
Romsos, C. G., Goldfinger, C., Robison, R., Milstein, R. L., Chaytor, J. D., and Wakefield, W. W. (2007). Development of a regional seafloor surficial geologic habitat map for the continental margins of Oregon and Washington, USA. Mapping the Seafloor for Habitat Characterization: geological Association of Canada, Special Paper. (Canada: Geological Association of Canada). 219–243.
Rugh, D. J., Hobbs, R. C., Lerczak, J. A., and Breiwick, J. M. (2005). Estimates of abundance of the eastern North Pacific stock of gray whales (Eschrichtius robustus) 1997-2002. J. Cetacean Res. Manag. 7, 1–12. doi: 10.1578/am.44.1.2018.1
Schaafsma, F. L., Cherel, Y., Flores, H., van Franeker, J. A., Lea, M.-A., Raymond, B., et al. (2018). Review: the energetic value of zooplankton and nekton species of the Southern Ocean. Mar. Biol. 165:129. doi: 10.1007/s00227-018-3386-z
Schonberg, S. V., Clarke, J. T., and Dunton, K. H. (2014). Distribution, abundance, biomass and diversity of benthic infauna in the Northeast Chukchi Sea, Alaska: relation to environmental variables and marine mammals. Deep. Res. 2 Top. Stud. Oceanogr. 102, 144–163. doi: 10.1016/j.dsr2.2013.11.004
Scordino, J. J., Gosho, M., Gearin, P. J., Akmajian, A., Calambokidis, J. C., and Wright, N. (2017). Individual gray whale use of coastal waters off northwest Washington during the feeding season 1984 – 2011: implications for management. J. Cetacean Res. Manag. 16, 57–69.
Shanks, A. L., and Roegner, G. C. (2007). Recruitment Limitation in Dungeness Crab Populations Is Driven by Variation in Atmospheric Forcing. Ecology 88, 1726–1737. doi: 10.1890/06-1003.1
Soledade Lemos, L., Burnett, J. D., Chandler, T. E., Sumich, J. L., and Torres, L. G. (2020). Intra- and inter-annual variation in gray whale body condition on a foraging ground. Ecosphere 11:e03094. doi: 10.1002/ecs2.3094
Spitz, J., Trites, A. W., Becquet, V., Brind’Amour, A., Cherel, Y., Galois, R., et al. (2012). Cost of Living Dictates what Whales, Dolphins and Porpoises Eat: the Importance of Prey Quality on Predator Foraging Strategies. PLoS One 7:e50096. doi: 10.1371/journal.pone.0050096
Stelle, L. L., Megill, W. M., and Kinzel, M. R. (2008). Activity budget and diving behavior of gray whales (Eschrichtius robustus) in feeding grounds off British Columbia. Mar. Mammal Sci. 24, 462–478. doi: 10.1111/j.1748-7692.2008.00205.x
Stewart, J. D., and Weller, D. W. (2021). “Abundance of eastern North Pacific gray whales 2019/2020. U.S” in Department of Commerce, NOAA Technical Memorandum NMFS-SWFSC-639. United States: NOAA. doi: 10.25923/bmam-pe91
Torres, L. G., Barlow, D. R., Chandler, T. E., and Burnett, J. D. (2020). Insight into the kinematics of blue whale surface foraging through drone observations and prey data. PeerJ. 8:e8906. doi: 10.7717/peerj.8906
Torres, L. G., Nieukirk, S. L., Lemos, L., and Chandler, T. E. (2018). Drone up! Quantifying whale behavior from a new perspective improves observational capacity. Front. Mar. Sci. 5:319. doi: 10.3389/fmars.2018.00319
Valenzuela, L. O., Sironi, M., Rowntree, V. J., and Seger, J. (2009). Isotopic and genetic evidence for culturally inherited site fidelity to feeding grounds in southern right whales (Eubalaena australis). Mol. Ecol. 18, 782–791. doi: 10.1111/j.1365-294X.2008.04069.x
Villegas-Amtmann, S., Schwarz, L. K., Gailey, G., Sychenko, O., and Costa, D. P. (2017). East or west: the energetic cost of being a gray whale and the consequence of losing energy to disturbance. Endanger. Species Res. 34, 167–183. doi: 10.3354/esr00843
Villegas-Amtmann, S., Schwarz, L. K., Sumich, J. L., Costa, D. P., and Costa, D. P. (2015). A bioenergetics model to evaluate demographic consequences of disturbance in marine mammals applied to gray whales. Ecosphere 6:183. doi: 10.1890/ES15-00146.1
Wang, M., Overland, J. E., and Stabeno, P. (2012). Future climate of the Bering and Chukchi Seas projected by global climate models. Deep. Res. 2 Top. Stud. Oceanogr. 6, 46–57. doi: 10.1016/j.dsr2.2012.02.022
Weller, D. W., Brownell, R. L., Laake, J. L., Moore, J. E., Rosel, P. E., Taylor, B. L., et al. (2013). “Report of the National Marine Fisheries Service Gray Whale Stock Identification Workshop” in NOAA Technical Memorandum NOAA-TM-NMFS-SWFSC-507. (USA: NAOO).
Keywords: gray whales, eastern North Pacific, caloric content, Oregon, energetic trade-off, zooplankton
Citation: Hildebrand L, Bernard KS and Torres LG (2021) Do Gray Whales Count Calories? Comparing Energetic Values of Gray Whale Prey Across Two Different Feeding Grounds in the Eastern North Pacific. Front. Mar. Sci. 8:683634. doi: 10.3389/fmars.2021.683634
Received: 21 March 2021; Accepted: 05 July 2021;
Published: 22 July 2021.
Edited by:
Lars Bejder, University of Hawai‘i at Mānoa, United StatesReviewed by:
Jorge Urbán Ramírez, Universidad Autónoma de Baja California Sur, MexicoCopyright © 2021 Hildebrand, Bernard and Torres. This is an open-access article distributed under the terms of the Creative Commons Attribution License (CC BY). The use, distribution or reproduction in other forums is permitted, provided the original author(s) and the copyright owner(s) are credited and that the original publication in this journal is cited, in accordance with accepted academic practice. No use, distribution or reproduction is permitted which does not comply with these terms.
*Correspondence: Lisa Hildebrand, bGlzYS5oaWxkZWJyYW5kQG9yZWdvbnN0YXRlLmVkdQ==
Disclaimer: All claims expressed in this article are solely those of the authors and do not necessarily represent those of their affiliated organizations, or those of the publisher, the editors and the reviewers. Any product that may be evaluated in this article or claim that may be made by its manufacturer is not guaranteed or endorsed by the publisher.
Research integrity at Frontiers
Learn more about the work of our research integrity team to safeguard the quality of each article we publish.