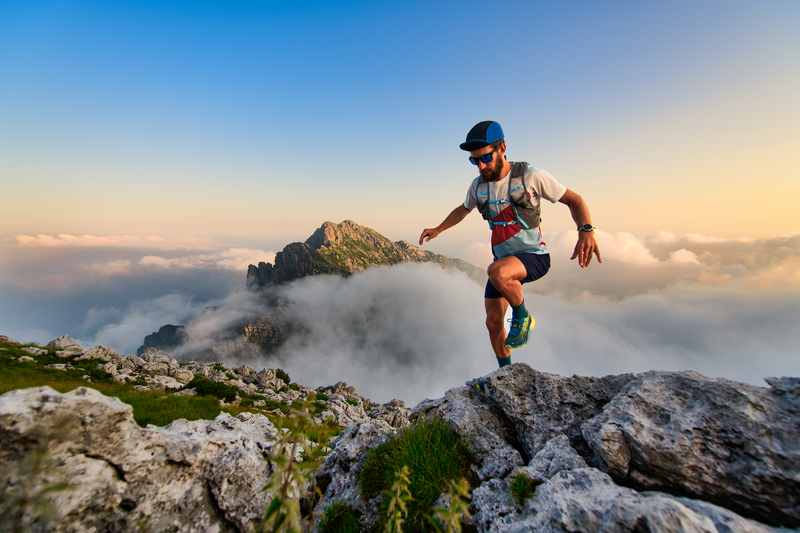
95% of researchers rate our articles as excellent or good
Learn more about the work of our research integrity team to safeguard the quality of each article we publish.
Find out more
ORIGINAL RESEARCH article
Front. Mar. Sci. , 05 July 2021
Sec. Marine Biology
Volume 8 - 2021 | https://doi.org/10.3389/fmars.2021.678848
This article is part of the Research Topic Biological Invasions in the Mediterranean Sea View all 13 articles
Invasive species are one of many anthropogenic challenges to maintaining a healthy marine ecosystem. Two rabbitfish species (Siganus rivulatus and Siganus luridus) are among the more successful migrants from the Red Sea to the Mediterranean, where their intense foraging has caused damage to the algae community, thus reducing primary production and habitat complexity, and impacting nurseries for early life stages. Anecdotal evidence suggests that the impact of rabbitfish on algae is lower in Marine Protected Areas (MPAs) although rabbitfish densities are similar in protected and fished areas. One explanation could be that fear of predators, more often present inside MPAs and an important component of a healthy marine ecosystem, reduces the ecological impacts of rabbitfish. This research aimed to test if such fear effects do occur in rabbitfish. Using controlled mesocosm experiments, we tested S. rivulatus reactions to two chemical predation cues: chemical alarm cues released from a recently killed conspecific fish, and water-borne cues from a tank with a live grouper predator, Epinephelus marginatus. We found that rabbitfish significantly reduce their overall food consumption as well as their bites per minute when exposed to the alarm cue, but not when exposed to the grouper water cue. These results support the idea that MPAs, which effectively increase the density of large piscivores and hence predation, can mitigate the impact of invasive herbivorous species. If the mesocosm results can scale up to natural systems, predation cues may be artificially introduced to other target areas in order to reduce rabbitfish grazing outside reserves. Thus, this study provides information that can be used to manage the ecological impacts caused by invasive rabbitfish, both inside and outside of marine reserves.
The Mediterranean Sea is under an ongoing invasion by hundreds of exotic species, in large part due to the opening of the Suez Canal in 1869 (Belmaker et al., 2009; Katsanevakis et al., 2014; Galil et al., 2015; Castellanos-Galindo et al., 2020; Golani, 2021). Successful alien species exhibit a diversity of ecological traits that may enable them to become established in the Mediterranean Sea (Belmaker et al., 2013; Azzurro et al., 2014; Givan et al., 2017). Two exotic herbivorous fishes, Siganus luridus and Siganus rivulatus (commonly known as rabbitfish), are among the more successful migrants from the Red Sea to the Eastern basin of the Mediterranean (Coll et al., 2010; Golani, 2010; Pickholtz et al., 2018). These species of rabbitfish are among the alien species most threatening to biodiversity in the Mediterranean (Roy et al., 2019). Rabbitfish are efficient herbivores, a trait that plays a key role in supporting the health of coral reefs by controlling harmful algae growth (Fox and Bellwood, 2013; Brandl and Bellwood, 2014). In the Mediterranean, however, their grazing habits have had a detrimental effect, creating barren reefs in areas that were once abundant algal forests (Sala et al., 2011; Verges et al., 2014; Yeruham et al., 2020). Rabbitfish have also been observed to graze on both established algae and on turf which contains macroalgal recruits (Verges et al., 2014), in contrast to native Mediterranean herbivores who feed primarily on adult macrophytes. All of this disturbs the larger ecological community that depends on macroalgae and seagrass for many ecosystem functions, including primary production, nutrient recycling, habitat formation, and nurseries for many marine species (Mineur et al., 2015; Teagle et al., 2017) and leads to a shift in ecosystem functions (Rilov et al., 2020).
Mediterranean coastal ecosystems are often heavily overfished, with top predators disproportionally targeted (Jennings and Kaiser, 1998). MPAs have been established in many places around the Mediterranean basin (Claudet et al., 2020). Marine Protected Area with the highest protection level have had a strong positive impact on the density and biomass of high trophic level species (Giakoumi et al., 2017). For instance, in a series of surveys of marine nature reserves in Israel, higher numbers and larger density of groupers (Epinephelus costae, Epinephelus marginatus, and Mycteroperca rubra) were observed inside marine reserves (Lazarus et al., 2020). Mediterranean groupers are predators of rabbitfish (Aronov and Goren, 2008) and thus can potentially cull the population of prey fish through consumption. But, in the same MPAs surveyed, there were also high numbers of rabbitfish inside reserves, similar to the numbers outside reserves (Rilov et al., 2018; Lazarus et al., 2020).
Despite the high presence of rabbitfish, reef surfaces inside MPAs have a higher cover of marine macrophytes than in unprotected adjacent habitats (Rilov et al., 2018), suggesting reduced grazing pressure. Trophic cascades can be triggered by a reduction in prey density or by changes in prey behavior. Thus, Rilov et al. (2018) proposed that while the higher predator (grouper) density inside MPAs might not significantly affect rabbitfish density, it may reduce rabbitfish grazing activity through fear of predation, i.e., by influencing their behavior. However, this hypothesis has never been experimentally tested.
What and where fish graze can be influenced by predation risk since animals must balance the need for food with the need for safety (Hammerschlag et al., 2010). One of the ways in which predators modify prey behavior is by creating a “landscape of fear” that impacts foraging activities (Laundre et al., 2010; Catano et al., 2016). For example, prey may hide in refuges to avoid predation thereby reducing their time spent foraging in risky areas (Orrock et al., 2013). Some species of rabbitfish have developed social strategies to assist in foraging under the threat of predation, such as the formation of adult pair-bonds where one member grazes while the other remains on watch (Woodland, 1990; Brandl and Bellwood, 2015). This strategy decreases their predation risk but also reduces each individual’s foraging time. Changes in foraging behavior may negatively impact the fitness of the prey by lowering their food intake or requiring them to divert resources away from reproduction. However, empirical evidence suggests that there is a high variance in whether and how strongly prey is affected by these changes (Sheriff et al., 2020).
Any change in foraging strategies would also impact the prey’s resources (Peacor and Werner, 1998). These non-consumptive interactions (Abrams, 1995) have been shown to amplify through food chains, especially in the marine environment, and often have a higher impact on the resources of the prey than on the prey themselves (Werner and Peacor, 2003; Preisser et al., 2005; Rasher et al., 2017). One example of a non-consumptive trophic cascade is the impact of a generalist fish predator, the Señorita (Oxyjulis californica), on habitat-forming feather boa kelp. The presence of the Señorita fish has been shown to suppress the grazing of limpets, thereby reducing kelp frond loss (Haggerty et al., 2018). Improving predator stock has been suggested as a successful strategy to aid in the restoration of key algae species by limiting the grazing pressure of herbivores (Gianni et al., 2018). However, changes in grazing patterns under threat of predation have not been shown specifically for rabbitfish.
Behavioral changes only occur when prey can detect predatory cues in their environment (Brown et al., 1999; Luttbeg and Trussell, 2013). Prey responses can be triggered by different stimuli including chemical cues, something particularly common in the marine environment (Chivers and Smith, 1998; Wisenden, 2000). Chemical cues may be released by the predator, or by other prey animals when they are attacked (“chemical alarm cues”). For example, rusty crayfish (Faxonius rusticus) changed their foraging behavior when exposed to water-borne odors of largemouth bass (Wood et al., 2018). In addition, reef urchins (Echinometra viridis) were shown to reduce grazing in response to crushed conspecific cues (Dunn et al., 2018). The ability to quickly and accurately identify predators is key to prey fitness, can be based on many different environmental cues, and may even be learned from experience (Mitchell et al., 2011).
This research aims to experimentally test how rabbitfish food acquisition activities are affected by the presence of a predator, using predator-related stimuli and predation alarm cues. We predict that in the presence of predation cues, rabbitfish will display lower overall consumption, as well as a lower bite rate. Understanding how the presence of predators changes the foraging behavior of these invasive fish can help scientists and conservationists enhance the protection and restoration of healthy ecosystems by reducing the damage caused by overgrazing.
To measure rabbitfish foraging behavior with and without predation cues, we conducted experiments using mesocosms with flow-through seawater to allow near-real sea conditions. Mesocosms have been shown to be useful models for measuring ecological functions (Brown et al., 2011). We conducted the experiments at the Israel Oceanographic and Limnological Research (IOLR) facility in Haifa during the fall of 2019 and the summer of 2020.
To create mesocosms for the experiment, we used four large plastic containers measuring 1.12 × 1.12 × 0.8 m. For each container, we drilled a hole on the bottom of the tank for a drain, and a hole on the side of the tank for water outflow. We connected each tank to a water intake pipe and a water outflow pipe. Water in the tanks was supplied by the IOLR’s constant flow-through system, allowing fresh sea water to circulate in and out of the tanks, thereby mimicking sea conditions. We also connected air inflow tubes with a diffuser in each tank to ensure an oxygen rich environment for the fish.
We collected adult S. rivulatus from the shallow reefs near IOLR and acclimated them to the experimental conditions before the experiment began. The fish were collected at night with hand nets by SCUBA divers and snorkelers. The acclimation period was between six (in 2019) and two (in 2020) months. We considered the fish acclimated when they began to eat regularly, quantified by the fish consuming at least 80% of the food within 5 h of feeding, when fed every other day. Once acclimated, we introduced between 4 and 5 small adult fish (rabbitfish are schooling fish, and placing one individual per tank might add stress), with an average length of 16–17 cm (± 3.4, SD), into each of four mesocosms. In the 2019 experiments, we distributed the fish between tanks in a way that resulted in a similar overall combined foraging rate between tanks. In the 2020 experiments, we measured the total length of each fish prior to introduction into the experiment tanks to ensure a similar overall biomass of fish between tanks.
The aim of the experiments was to determine if, and by how much, rabbitfish foraging behavior changes in the presence of signs of potential predation risk. To analyze this, we tested the reaction of the rabbitfish S. rivulatus to two different chemical cues by (1) introducing pieces of a recently killed conspecific fish into the tanks (hereafter alarm cue) and (2) exposing the fish to water from the holding tank of a live predator (hereafter grouper water). For the alarm cue we sacrificed one of the extra fish from a holding tank, cut the body into three equal pieces, and introduced one piece (sealed in a mesh bag) into each treatment tank. For the grouper water experiments, we simulated the threat of predation by using water from a tank holding a live predator (E. marginatus). We introduced approximately 60 liters of “grouper water” into each treatment tank as the predation risk cue.
For each experiment we used 4 tanks, with predation cues used in 3 tanks and the fourth tank left as a control (Figure 1). Each repetition ran for 7 days, during which we fed the fish every other day to allow time for the fish to become hungry between feedings. For the first six days the fish were allowed to forage without any predation cue. On the last day of each experiment, we added an alarm cue or grouper water cue into the treatment tanks (Figure 1). We measured two variables on days three, five, and seven of each experiment. The first was the amount of food foraged over specific periods of time as a measure of the fish’s overall grazing impact. The second was the effort the rabbitfish spent foraging measured by bite rate per minute. See details below under section “Grazing quantification”. In order to measure grazing behavior without disrupting the fish by the presence of an observer (rabbitfish are highly timid in the presence of humans), we set up online surveillance cameras inside waterproof transparent boxes in each tank.
Figure 1. Graphical workflow summarizing the main steps taken during each weeklong repetition of the two experiments. Fish were introduced on day 1 and fed on days 1, 3, 5, and 7. A predation cue was introduced on day 7. Data was recorded (bites per minute and overall food consumption) on days 3, 5, and 7. There were two types of controls: (1) the tanks to which we did not apply any predation risk cues and (2) the difference in bite rate and food consumption between two consecutive days of the experiment where there was no predation cue (“baseline”) compared to the change in bite rate when we introduced the predation risk cue (“predation”).
We included two types of controls in the experimental design. The first type of control was the tanks to which we did not apply any predation risk cues (Figure 1). These functioned as a global control for any external impacts that might confound the results, such as the effects of surrounding conditions (e.g., weather or water supply). The second type of control was on the individual tank-level, by calculating the difference in bite rate and overall food foraged between two days of the experiment where there was no predation cue (“baseline,” days 3 and 5) compared to the difference between days when we introduced the predation risk cue (“predation cue,” days 5 and 7). This approach controlled for variation in fish behavior between tanks. See Figure 1 for a graphical workflow summarizing the main steps taken within each repetition.
To increase independent replication, we repeated the alarm cue treatment in full three times and the grouper water treatment twice. For each repetition, we repeated the full set of treatments with at least one week in between experiments. We redistributed the fish between the tanks prior to each repetition so that each repetition could be treated as independent. Since we moved fish between tanks, we considered the first day an acclimation period and did not include those measurement in our analysis. We also alternated among the tanks used as controls to reduce potential tank effects. We used the same statistical approach for both experimental treatments: (a) alarm cue (three repetitions, n = 9 treatment tanks) and (b) grouper water (two repetitions, n = 6 treatment tanks). In total we had 5 global controls and 15 paired observations of “baseline” (no predation) and “predation cue.” See Supplementary Table 1 for a summary of experiment dates and repetition types.
To create a depletable food source that can be easily quantified from a visual assay, we used sheets of nori seaweed and ice cube trays. A depletable food source, where the first few bites are more easily grazed then the remaining food, most closely resembles a natural foraging state (Brown and Kotler, 2004). We first tested the food preference of the rabbitfish and found that they were equally likely to eat packaged nori (Porphyra) and fresh Ulva, one of their natural food sources, and therefore we used packaged nori for the experiment due to ease of use and measurement.
We cut each sheet of nori into 10 equal pieces and affixed each piece into an ice cube segment using agarose. Agar is a jelly like substance, obtained from red alga and non-toxic to fish. We diluted the agar in water (2% mixture) and microwaved it for 90 s until the agar was fully dissolved. We rolled each piece of nori around small pebbles, placed each one into an ice cube tray segment, and added some warm agar-water. After the agar cooled and hardened, the top of the nori was loose, and the bottom of the nori was condensed around the pebbles and held in place by the agar. In this way, the initial efforts of the fish to graze were more productive than any continuing effort, which resembles their natural feeding environment.
The food trays were comprised of ten equal pieces of seaweed, one in each ice cube segments. At each interval, we further quantified the portion of seaweed remaining in each ice cube segment on a scale of 0–3. This allowed us to estimate the overall proportion of seaweed remaining in each tank at each measurement time on a scale of 0–30 (10 ice cubes per tank × 3 portions per ice cube). We assigned a value of 0/1 to each algae portion at each observation time (30 per tank, where 0 indicated a portion that was eaten, and 1 indicated a portion of food that remained). As we did not retain data on the fate of each individual ice cube segment, this was done by dividing the total number of algae portions remaining on each tray by the total original number of algae portions under the assumption that grazing was even across ice cube segments.
A single ice cube tray with 10 pieces of nori was placed within each tank. For each day of the experiment when the fish were fed, we cleaned the food trays and replenished the nori before replacing the trays into the tanks. In each repetition, on each measurement day, we recorded the fish bite rate per minute at 15, 45, and 105 min, and the overall consumption of seaweed by the fish at 15, 45, 75, 105, 135, and 165 min after introducing the food tray.
To assess the impact of the predation cue on bite rate, we ran a linear model using the following formula: Δ Bites ∼ Predator + Time + random(Tank). Here Δ Bites refers to the difference in bite rate between two consecutive measurement days in the experiment. Predator indicates whether the measurement is for the baseline (Predator = No; difference between first two measurement days without predation cue, days 3 and 5; see Figure 1) or predation cue (Predator = Yes, difference between measurement days 5 and 7, where day 5 has no predation cue and day 7 has a predation cue), and Tank is the unique tank number for each tank and repetition. We also added the effect of Time on the model where Time indicates the time of observation (15, 45, or 105 min after introduction of the food), and checked for a potential Predator × Time interaction. We ran this model only on the treatment tanks, and separately for the two types of treatment: alarm cue and grouper water.
We ran an additional linear model to confirm that the global controls (the non-treatment tanks) were not significantly different from the treatment tanks on days without predation cues to eliminate the possibility of external confounding effects. We used the formula: Δ Bites ∼ Category + Time + random(Tank). Here Category refers to the status of the Tank, whether it was a treatment tank or a control tank. For alarm cue, treatment tanks n = 9 and control tanks n = 3. For grouper water, treatment tanks n = 6 and control tanks n = 2.
To test whether overall consumption changed under the presence of predation risk cues, we used two statistical models, a linear model and a survival model.
For the linear model, we used the number of food portions remaining at each time in each measurement day as a continuous response variable between 0 and 30 (10 ice cubes per tank, 3 portions per ice cube). Time indicates the time of observation: 15, 45, 75, 105, 135, and 165 min after feeding. We added Predator as a categorical variable, and Time as a continuous variable. We also added Tank as a random effect. As with the bite rate, we compared the change in food remaining between two consecutive measurement days in the experiment without predation cues (days 3 and 5; see Figure 1) versus the change between days with predation cues (days 5 and 7, where day 5 has no predation cue and day 7 has a predation cue). Specifically, we were interested in examining if the amount of food remaining changed over time differently in the tanks with predation cues verses the tanks without predation cues and therefore focused on the interaction between predator and time as in the following formula:
Here “Δ Food remaining” is the difference in the number of portions of food remaining in the tray between days (from 0–30 portions, 1 tray per Tank). We ran the full analysis separately for the two types of treatments: alarm cue and grouper water.
The second analysis on overall consumption was a survival model. We created survival curves showing the probability of each portion of food “surviving” a given amount of time, comparing baseline (no predation cue) and predation cue for each treatment tank. We used the survfit function in the “survivor” package in R (Therneau, 2020) to create the survival curves. The survfit function uses the Kaplan-Meier method to estimate a survival curve which predicts the probability that each patient (here: portion of food) will survive past time “t.” We then used a log rank test to compare the survival curves of the baseline and predation cue to see if they differed significantly. For the log rank test, we included Tank (a unique tank number for each tank and repetition) as a random effect. Finally, we used the Cox regression to estimate an overall effect size using the hazard ratio (“HR”) where HR represents the ratio of the chance a portion of food would be eaten under treatment conditions (predation cue) to the chance a portion of food would be being eaten under control conditions (the baseline period) at any specific point in time. An HR of less than 1 indicates a reduced hazard and an HR over 1 indicates an increased hazard. We ran the full analysis separately for the two types of treatments: alarm cue and grouper water. For both analyses we qualitatively examined the differences between the global control and the treatment tanks, to eliminate the possibility of external confounding effects.
We found that introducing an alarm cue led to a significant decrease in both bite rate per minute and overall food consumption in the treatment tanks. The rabbitfish in the treatment tanks had an overall lower bite rate per minute when exposed to a chemical alarm cue released by a recently killed conspecific fish (Predator effect: p = 0.008; Table 1). There was no significant interaction of observation time on change in bite rate (p = 0.4834) and therefore we report only the basic additive model. In addition, there was no significant difference between change in bite rates per day in the control tanks and in the treatment tanks on days without a predation cue (Category effect: p = 0.981; Figure 2).
Table 1. Summary of model results for the change in bite rates using the formula: Δ Bites ∼ Predator + Time + (1| Tank).
Figure 2. Change in bites per minute when introducing a predator cue into the tanks. (A) Alarm Cue. The left graph shows the results of using a chemical alarm cue released by a recently killed conspecific fish. (B) Grouper Water. The right graph shows the results of using water from a predator’s tank as the predation risk cue. The yellow box plot shows the global control tanks (n = 3 for Alarm Cue; n = 2 for Grouper Water). The blue box plots show the treatment tanks (n = 9 for Alarm Cue; n = 6 for Grouper Water) under two categories – “No predation cue” (difference between measurement days without predation cue) and “Predation cue” (difference between measurement days with and within a predation cue). In the plots, the boxes indicate the inter-quartile range, the horizontal lines mark the medians, the whiskers indicate values within 1.5 times the inter-quartile range beyond either end of the box, and the dots indicate outliers beyond that range.
The reduction in bite rate is supported by findings that overall food consumption by rabbitfish was also lower in the presence of the alarm cue. In the linear model, there was a higher percentage of food portions remaining over time, i.e., lower foraging impact, in treatment tanks with an alarm cue than in tanks with no predation cue (Figure 3), confirmed by the lower change in food consumption over time between consecutive days with no predation cue (Predator × Time: p < 0.001; Table 2). Survival curves produced using the Kaplan-Meier method were also significantly different from each other (log rank test p < 0.0001) and showed a reduction in the percent of food eaten under the alarm cue (Figure 4). The hazard ratio was 0.28, implying that around 28% of food that would have been eaten without a predation cue is eaten in the presence of the alarm cue (Table 3).
Figure 3. Change in number of food portions remaining (out of 30) over time when introducing a predation cue compared to no cue. The dots represent the amount of food remaining at specific times (min) after food was introduced. Note that for illustrative purposes, the data shown here is actual food portions remaining over time, and not the change between days of food portions remaining over time used for analysis. The lines show the linear regression and the shading represents the 95% confidence intervals. Red dots and lines show the baseline food survival. Blue dots and lines show the food survival with treatment. Data shown for (A) Alarm Cue and (B) Grouper Water. Note that a higher percentage of food remaining implies a lower foraging impact. Only the Alarm Cue (“A”) showed an interaction between time and predation cue (p < 0.001).
Table 2. Summary of linear model results for change in food consumption using the formula: Δ Food remaining ∼ Predator × Time + (1| Tank).
Figure 4. Percentage of food eaten at each time (min) after feeding using the Kaplan-Meier method to estimate a survival curve. Gray lines show baseline results for both experiments. Dashed black lines show results of adding predation risk cues. The lines marked by X are the chemical alarm cue treatment and the lines marked by circles (î) are the grouper water treatment. Error lines reflect 95% confidence intervals. Baseline results where similar for both experiments. Only the Alarm Cue showed a significant (log rank test p < 0.0001) reduction in percent of food eaten due to predation cues.
Table 3. Summary of the Cox mixed-effects model fit by maximum likelihood for the impact on food consumption using the formula: Survival ∼ Predator + (1| Tank).
This general effect results from divergent trends between tanks. In five of the nine tanks, fish showed a significant decrease in food survival in days with the predation cue relative to days without it. In the other four tanks there was a small and non-significant effect. Results of food survival by tank can be found in the Supplementary Figure A.
We found no reduction in either bite rate or food consumption when using grouper water from the tank of a live predator to simulate predation. There was no significant change in bite rate (Predator effect: p = 0.438; Table 1 and Figure 2). For food consumption, using a linear model we found no interaction between Predation and Time (Predator × Time: p = 0.209; Table 2) and a small increase in overall food grazed under predation with this predation risk cue using Kaplan-Meier survival curves. Although the difference in survival curves is significant (Grouper Water: p = 0.001; Table 3), the actual difference is negligible (Figure 4).
The results of the experiments indicate that alarm cues significantly impact rabbitfish foraging activities, as measured by changes in the overall amount of food consumed and the bite rate per minute. Interestingly, we found that the rabbitfish reacted differently to different chemical cues of potential predation risk. There was a significant change in foraging activity when the rabbitfish were exposed to chemical alarm cues released by a recently killed conspecific fish. However, there was no noticeable change in foraging behavior when they were exposed to water from a tank holding a live predator (Epinephelus marginatus).
While the general pattern of predation risk impacts on foraging is well known (Brown and Kotler, 2004; Laundre et al., 2010), it had not been tested previously for rabbitfish. Rabbitfish are invasive in the Mediterranean and their intensive grazing has been shown to damage the local algae communities (Sala et al., 2011; Verges et al., 2014). The stress of alien species on marine ecosystems may also decrease resilience to sea warming (Corrales et al., 2018). The results presented in this study show that this detrimental overgrazing might be partially mitigated by the presence of predators whose predation activities produce an alarm cue which may reduce the foraging activity of the surviving fish. Another way of looking at this is that the Mediterranean Sea currently suffers from a synergy between having fewer predators and new grazers with high abundance.
There has been little research on how fishing protection inside MPAs affects the overall ecological impact of invasive species (Iacarella et al., 2019). The results of this study support the potential effectiveness of MPAs to indirectly mitigate the impact of invasive herbivorous species through trophic cascades, showing yet another benefit of MPAs as an effective tool to protect and restore healthy ecosystems. By limiting fishing, MPAs specifically protect commercially valuable, often larger predatory fish, the same fish that prey on rabbitfish. The higher density of predators inside MPAs (Giakoumi et al., 2017; Rilov et al., 2018; Lazarus et al., 2020) can change the foraging behavior of rabbitfish and through that mitigate their negative impact, thereby preserving the algae and seagrass that rabbitfish eat.
Our results were obtained from mesocosms experiments and hence are only the first step in understanding the ability of predators to reduce the impact of rabbitfish under natural settings. For example, the limited volume of water within mesocosms may have increased the signal of the alarm cue on rabbitfish foraging compared to that observed in nature. Indeed, there have been mixed results about the impact of MPAs on trophic cascades (Shears and Babcock, 2002, 2003; Byrnes et al., 2006; Malakhoff and Miller, 2021). It is likely we will only understand the full potential of MPAs to control invasive species once more MPAs become better enforced and more mature (Giakoumi et al., 2019a). Performing field studies to compare the foraging in situ and over longer temporal duration in the presence of predation cues could show the cascading impact of predation on foraging activity in the context of a more complex and realistic environment.
There are a number of possible reasons for why the fish did not react to the presence of the grouper water as opposed to the alarm cue. It is possible that the chemical cues released by the predator into the water were simply not concentrated enough to elicit a response. Alternatively, the rabbitfish might react to the presence of other predators, but not specifically to E. marginatus, or to the presence of this predator when it is alone. A study of trophic cascades in kelp forests showed that predator diversity was key to controlling the effects of herbivores on community structure (Byrnes et al., 2006). In the Mediterranean, rabbitfish predators include fish from the grouper family as well as other native predator species such as the greater amberjack (Seriola dumerili), bluefish (Pomatomus saltatrix), Mediterranean moray (Muraena helena), and the Common dentex (Dentex dentex) (Giakoumi et al., 2019b). Other combinations of chemical cues from these predators may have elicited a behavioral response in the rabbitfish.
An additional possibility is that rabbitfish would not react to the presence of any specific predator or combination of predators, but only to a chemical alarm cue released from conspecific individuals during a predator attack. The ability of fish to associate a specific predator’s odor with predation is likely a learned behavior associated with the proximately of predator odor to chemical alarm cues (Brown, 2003; Mitchell et al., 2015). Since these fish were collected from areas with few large groupers that posed a risk, it is likely the rabbitfish did not learn to be wary of groupers. In addition, a recent experiment showed that changes in crayfish behavior as a result of predator odors were dependent on both the prey familiarity with the predator, based on previous exposure, and the actual diet of the predator (Beattie and Moore, 2018), supporting the idea that the presence of a potential predator alone may not be enough to trigger an effect. One possible implication is that if only certain predators are present, or if the predator’s main diet is composed of other prey species, their presence alone may not be sufficient to impact rabbitfish foraging behavior. Finally, it is possible that the rabbitfish would respond more strongly to visual cues than to odor.
We also found significant variation in the reaction of each tank’s fish to the presence of predation cues, where some tanks did not show any reduction in food consumption under treatment conditions (Supplementary Figure 1). This may be partially explained by variation in signal strength. Although efforts were made to ensure that all fish tanks received the same magnitude of alarm cue, for example by dividing fish pieces equally and including some fish guts in each tank, it is possible that some tanks received a stronger signal. Alternatively, this variation among tanks may indicate that individuals react differently to predation risk. Variation in vigilance and other anti-predator behaviors have been shown to directly translate into variations in individual survival and are thus traits that can be selected for (Steinhoff et al., 2020).
These differences among individual fish and between different predation cues support previous findings that prey reactions to predator risk can vary based on many variables, including individual fitness, resource availability, and predator type (Sheriff et al., 2020). In addition, these experiments were only run on S. rivulatus, one of the two invasive rabbitfish species in the Mediterranean. Since S. rivulatus and S. luridus both show adaptation to different food sources (Lundberg and Golani, 1995), it is possible that the different species would react differently to predation risk.
Prey use multiple strategies to mitigate their predation risk (Sansom et al., 2009; Kotler et al., 2010). In this experiment it is difficult to tease apart the strategies of avoidance and vigilance as the fish were not able to choose between multiple foraging spots, and therefore were less able to avoid the predation cue. In addition, since there was a strong signal in both overall consumption and bite rate, there is no way to conclusively say if the fish spend less time foraging (avoidance) or they foraged less efficiently (vigilance). If instead, for example, the bite rate had remained unchanged, but there was lower overall food consumption under predation, it would suggest vigilance rather than avoidance.
The strong reaction of rabbitfish to chemical alarm cues suggests that the higher density of predators within MPAs may in fact mitigate the impact of these invasive species. Outside of MPAs, predator presence can also be encouraged through the use of artificial habitats and specific fishing bans. In addition, predation cues might be used directly as a management tool, that is, be artificially introduced to specific environments, even when few predators are present, in order to reduce rabbitfish grazing. Further research into the differences between types of predation cues and prey mitigation strategies could help determine how best to use these results to manage the ecological impacts caused by invasive rabbitfish under natural settings, especially in hotspots of highly impacted areas (Katsanevakis et al., 2016). Ultimately, this information can be used to manage the ecological impacts caused by invasive rabbitfish, both inside and outside of marine reserves.
The raw data supporting the conclusions of this article will be made available by the authors, without undue reservation.
The animal study was reviewed and approved by the Israel Oceanographic and Limnological Research Ethics Committee. Permit# F1933.
SV and JB conceived of the framework project of which these experiments were one part. DSG, JB, and GR conceived of and planned the experiments. DSG carried out the experiments, analyzed the data with support from JB, and took the lead in writing the manuscript. GR helped supervise the project. All authors provided critical feedback and helped shape the research, analysis, and manuscript.
This research was part of the EXOFISHMED project funded by the ANR (grant ANR- 17-CE32-0003), a project-based funding agency for research in France.
The authors declare that the research was conducted in the absence of any commercial or financial relationships that could be construed as a potential conflict of interest.
We would like to thank Dar Golomb, Erez Yeruham, and Shahar Malamud for their help in setting up the mesocosm and catching the fish. We would also like to thank all the members of the Belmaker Lab for their generous help, and particularly to Sarah Ohayon, Tal Gavriel, and Nitzan Yitzchak for help feeding the fish; Renanel Pickholtz for help with the experimental design, and Itai Granot for help with the analysis.
The Supplementary Material for this article can be found online at: https://www.frontiersin.org/articles/10.3389/fmars.2021.678848/full#supplementary-material
Abrams, P. A. (1995). Implications of dynamically variable traits for identifying, classifying, and measuring direct and indirect effects in ecological communities. Am. Nat. 146, 112–134. doi: 10.1086/285789
Aronov, A., and Goren, M. (2008). Ecology of the mottled grouper (Mycterropeca rubra) in the Eastern Mediterranean. Electron. J. Ichthyol. 2, 43–55.
Azzurro, E., Tuset, V. M., Lombarte, A., Maynou, F., Simberloff, D., Rodríguez-Pérez, A., et al. (2014). External morphology explains the success of biological invasions. Ecol. Lett. 17, 1455–1463. doi: 10.1111/ele.12351
Beattie, M. C., and Moore, P. A. (2018). Predator recognition of chemical cues in crayfish: diet and experience influence the ability to detect predation threats. Behaviour 155, 505–530. doi: 10.1163/1568539x-00003501
Belmaker, J., Brokovich, E., China, V., Golani, D., and Kiflawi, M. (2009). Estimating the rate of biological introductions: Lessepsian fishes in the Mediterranean. Ecology 90, 1134–1141. doi: 10.1890/07-1904.1
Belmaker, J., Parravicini, V., and Kulbicki, M. (2013). Ecological traits and environmental affinity explain Red Sea fish introduction into the Mediterranean. Glob. Chang. Biol. 19, 1373–1382. doi: 10.1111/gcb.12132
Brandl, S. J., and Bellwood, D. R. (2014). Individual-based analyses reveal limited functional overlap in a coral reef fish community. J. Anim. Ecol. 83, 661–670. doi: 10.1111/1365-2656.12171
Brandl, S. J., and Bellwood, D. R. (2015). Coordinated vigilance provides evidence for direct reciprocity in coral reef fishes. Sci. Rep. 5, 1–13.
Brown, G. E. (2003). Learning about danger: chemical alarm cues and local risk assessment in prey fishes. Fish and Fisheries 4, 227–234. doi: 10.1046/j.1467-2979.2003.00132.x
Brown, J. S., and Kotler, B. P. (2004). Hazardous duty pay and the foraging cost of predation. Ecol. Lett. 7, 999–1014. doi: 10.1111/j.1461-0248.2004.00661.x
Brown, J. S., Laundre, J. W., and Gurung, M. (1999). The ecology of fear: optimal foraging, game theory, and trophic interactions. J. Mammal. 80, 385–399. doi: 10.2307/1383287
Brown, L. E., Edwards, F. K., Milner, A. M., Woodward, G., and Ledger, M. E. (2011). Food web complexity and allometric scaling relationships in stream mesocosms: implications for experimentation. J. Anim. Ecol. 80, 884–895. doi: 10.1111/j.1365-2656.2011.01814.x
Byrnes, J., Stachowicz, J. J., Hultgren, K. M., Randall Hughes, A., Olyarnik, S. V., and Thornber, C. S. (2006). Predator diversity strengthens trophic cascades in kelp forests by modifying herbivore behaviour. Ecol. Lett. 9, 61–71.
Castellanos-Galindo, G. A., Robertson, D. R., and Torchin, M. E. (2020). A new wave of marine fish invasions through the Panama and Suez canals. Nat. Ecol. Evol. 4, 1444–1446. doi: 10.1038/s41559-020-01301-2
Catano, L. B., Rojas, M. C., Malossi, R. J., Peters, J. R., Heithaus, M. R., Fourqurean, J. W., et al. (2016). Reefscapes of fear: predation risk and reef hetero-geneity interact to shape herbivore foraging behaviour. J. Anim. Ecol. 85, 146–156. doi: 10.1111/1365-2656.12440
Chivers, D. P., and Smith, R. J. F. (1998). Chemical alarm signalling in aquatic predator-prey systems: a review and prospectus. Écoscience 5, 338–352. doi: 10.1080/11956860.1998.11682471
Claudet, J., Loiseau, C., Sostres, M., and Zupan, M. (2020). Underprotected marine Protected areas in a global biodiversity hotspot. One Earth 2, 380–384. doi: 10.1016/j.oneear.2020.03.008
Coll, M., Piroddi, C., Steenbeek, J., Kaschner, K., Ben Rais Lasram, F., Aguzzi, J., et al. (2010). The biodiversity of the Mediterranean Sea: estimates, patterns, and threats. PLoS One 5:e11842. doi: 10.1371/journal.pone.0011842
Corrales, X., Coll, M., Ofir, E., Heymans, J. J., Steenbeek, J., Goren, M., et al. (2018). Future scenarios of marine resources and ecosystem conditions in the Eastern Mediterranean under the impacts of fishing, alien species and sea warming. Sci. Rep. 8, 1–16. doi: 10.1079/9781786390981.0001
Dunn, R. P., Altieri, A. H., Miller, K., Yeager, M. E., and Hovel, K. A. (2018). Contrasting behavioral responses to predatory risk cues reflect different foraging strategies in two Caribbean sea urchins. Mar. Ecol. Prog. Ser. 604, 187–198. doi: 10.3354/meps12733
Fox, R. J., and Bellwood, D. R. (2013). Niche partitioning of feeding microhabitats produces a unique function for herbivorous rabbitfishes (Perciformes, Siganidae) on coral reefs. Coral Reefs 32, 13–23. doi: 10.1007/s00338-012-0945-5
Galil, B. S., Boero, F., Campbell, M. L., Carlton, J. T., Cook, E., Fraschetti, S., et al. (2015). ‘Double trouble’: the expansion of the Suez Canal and marine bioinvasions in the Mediterranean Sea. Biological Invasions 17, 973–976. doi: 10.1007/s10530-014-0778-y
Giakoumi, S., Pey, A., Di Franco, A., Francour, P., Kizilkaya, Z., Arda, Y., et al. (2019a). Exploring the relationships between marine protected areas and invasive fish in the world’s most invaded sea. Ecol. Appl. 29, e01809.
Giakoumi, S., Pey, A., Thiriet, P., Francour, P., and Guidetti, P. (2019b). Patterns of predation on native and invasive alien fish in Mediterranean protected and unprotected areas. Mar. Environ. Res. 150:104792. doi: 10.1016/j.marenvres.2019.104792
Giakoumi, S., Scianna, C., Plass-Johnson, J., Micheli, F., Grorud-Colvert, K., Thiriet, P., et al. (2017). Ecological effects of full and partial protection in the crowded Mediterranean Sea: a regional meta-analysis. Sci. Rep. 7, 1–12.
Gianni, F., Bartolini, F., Airoldi, L., and Mangialajo, L. (2018). Reduction of herbivorous fish pressure can facilitate focal algal species forestation on artificial structures. Mar. Environ. Res. 138, 102–109.
Givan, O., Parravicini, V., Kulbicki, M., and Belmaker, J. (2017). Trait structure reveals the processes underlying fish establishment in the Mediterranean. Glob. Ecol. Biogeogr. 26, 142–153. doi: 10.1111/geb.12523
Golani, D. (2010). “Colonization of the Mediterranean by Red Sea fishes via the Suez Canal - Lessepsian migration,” in Fish Invasions of the Mediterranean Sea:Change and Renewal, Vol. 145, eds D. Golani and B. Appelbaum-Golani (Sofia-Moscow: Pensoft Publishers), 188.
Golani, D. (2021). An updated checklist of the Mediterranean fishes of Israel, with illustrations of recently recorded species and delineation of Lessepsian migrants. Zootaxa 4956:zootaxa.4956.1.1.
Haggerty, M. B., Anderson, T. W., and Long, J. D. (2018). Fish predators reduce kelp frond loss via a trait-mediated trophic cascade. Ecology 99, 1574–1583. doi: 10.1002/ecy.2380
Hammerschlag, N., Heithaus, M. R., and Serafy, J. E. (2010). Influence of predation risk and food supply on nocturnal fish foraging distributions along a mangrove–seagrass ecotone. Mar. Ecol. Prog. Ser. 414, 223–235. doi: 10.3354/meps08731
Iacarella, J. C., Saheed, D., Dunham, A., and Ban, N. C. (2019). Non-native species are a global issue for marine protected areas. Front. Ecol. Environ. 17:495–501.
Jennings, S., and Kaiser, M. (1998). The Effects of fishing on marine ecosystems. Adv. Mar. Biol. 34, 201–352. doi: 10.1016/s0065-2881(08)60212-6
Katsanevakis, S., Coll, M., Piroddi, C., Steenbeek, J., Ben Rais Lasram, F., Zenetos, A., et al. (2014). Invading the Mediterranean Sea: biodiversity patterns shaped by human activities. Front. Mar. Sci. 1:32. doi: 10.3389/fmars.2014.00032
Katsanevakis, S., Tempera, F., and Teixeira, H. (2016). Mapping the impact of alien species on marine ecosystems: the Mediterranean Sea case study. Divers. Distrib. 22, 694–707. doi: 10.1111/ddi.12429
Kotler, B. P., Brown, J., Mukherjee, S., Berger-Tal, O., and Bouskila, A. (2010). Moonlight avoidance in gerbils reveals a sophisticated interplay among time allocation, vigilance and state-dependent foraging. Proc. R. Soc. B Biol. Sci. 277, 1469–1474. doi: 10.1098/rspb.2009.2036
Laundre, J. W., Hernandez, L., and Ripple, W. J. (2010). The landscape of fear: ecological implications of being afraid. Open Ecol. J. 3, 1–7. doi: 10.2174/1874213001003030001
Lazarus, M., Frid, O., and Yahel, R. (2020). Survey of Marine Reserves in the Israeli Mediterranean, s.l. Tel Aviv: Israel Nature and Parks Authority.
Lundberg, B., and Golani, D. (1995). Diet Adaptations of Lessepsian Migrant rabbitfishes, Siganus luridus and S. rivulatus, to the algal resources of the Mediterranean coast of Israel. Mar. Ecol. 16, 73–89. doi: 10.1111/j.1439-0485.1995.tb00395.x
Luttbeg, B., and Trussell, G. C. (2013). How the informational environment shapes how prey estimate predation risk and the resulting indirect effects of predators. Am. Nat. 181, 182–194. doi: 10.1086/668823
Malakhoff, K. D., and Miller, R. J. (2021). After 15 years, no evidence for trophic cascades in marine protected areas. Proc. R. Soc. B Biol. Sci. 288:20203061. doi: 10.1098/rspb.2020.3061
Mineur, F., Arenas, F., Assis, J., Davies, A. J., Engelen, A. H., Fernandes, F., et al. (2015). European seaweeds under pressure: consequences for communities and ecosystem functioning. J. Sea Res. 98, 91–108. doi: 10.1016/j.seares.2014.11.004
Mitchell, M. D., Chivers, D. P., McCormick, M. I., and Ferrari, M. C. (2015). Learning to distinguish between predators and non-predators: understanding the critical role of diet cues and predator odours in generalisation. Sci. Rep. 5, 1–10. doi: 10.1201/9781420009125.ch1
Mitchell, M. D., McCormick, M. I., Ferrari, M. C. O., and Chivers, D. P. (2011). Coral reef fish rapidly learn to identify multiple unknown predators upon recruitment to the reef. PLoS One 6:e15764.
Orrock, J. L., Preisser, E. L., Grabowski, J. H., and Trussell, G. C. (2013). The cost of safety: refuges increase the impact of predation risk in aquatic systems. Ecology 94, 573–579. doi: 10.1890/12-0502.1
Peacor, S. D., and Werner, E. E. (1998). Predator effects on an assemblage of consumers through induced changes in consumer foraging behavior. Ecology 81, 1998–2010. doi: 10.1890/0012-9658(2000)081[1998:peoaao]2.0.co;2
Pickholtz, R. S. M., Kiflawi, M., Friedlander, A. M., and Belmaker, J. (2018). Habitat utilization by an invasive herbivorous fish (Siganus rivulatus) in its native and invaded range. Biol. Invasions 20, 3499–3512. doi: 10.1007/s10530-018-1790-4
Preisser, E. L., Bolnick, D. I., and Benard, M. E. (2005). Scared to death? The effects of intimidation and consumption in predator-prey. Ecology 86, 501–509. doi: 10.1890/04-0719
Rasher, D. B., Hoey, A. S., and Hay, M. E. (2017). Cascading predator effects in a Fijian coral reef ecosystem. Sci. Rep. 7, 1–10. doi: 10.1201/9781420003796.ch1
Rilov, G., Peleg, O., Guy-Haim, T., and Yeruham, E. (2020). Community dynamics and ecological shifts on Mediterranean vermetid reefs. Mar. Environ. Res. 160:105045. doi: 10.1016/j.marenvres.2020.105045
Rilov, G., Peleg, O., Yeruham, E., Garval, T., Vichik, A., and Raveh, O. (2018). Alien turf: overfishing, overgrazing and invader domination in south-eastern Levant reef ecosystems. Aquat. Conserv: Mar. Freshw. Ecosyst. 28, 351–369. doi: 10.1002/aqc.2862
Roy, H. E., Bacher, S., Essl, F., Adriaens, T., Aldridge, D. C., Bishop, J. D. D., et al. (2019). Developing a list of invasive alien species likely to threaten biodiversity and ecosystems in the European Union. Glob. Chang. Biol. 25, 1032–1048.
Sala, E., Kizilkaya, Z., Yildirim, D., and Ballesteros, E. (2011). Alien marine fishes deplete algal biomass in the Eastern Mediterranean. PLoS One 6:e17356. doi: 10.1371/journal.pone.0017356.g005
Sansom, A., Lind, J., and Cresswell, W. (2009). Individual behavior and survival: the roles of predator avoidance, foraging success, and vigilance. Behav. Ecol. 20, 1168–1174. doi: 10.1093/beheco/arp110
Shears, N. T., and Babcock, R. C. (2002). Marine reserves demonstrate top-down control of community structure on temperate reefs. Oecologia 132, 131–142. doi: 10.1007/s00442-002-0920-x
Shears, N. T., and Babcock, R. C. (2003). Continuing trophic cascade effects after 25 years of no-take marine reserve protection. Mar. Ecol. Prog. Ser. 246, 1–16. doi: 10.3354/meps246001
Sheriff, M. J., Peacor, S. D., Hawlena, D., and Thaker, M. (2020). Non-consumptive predator effects on prey population size: a dearth of evidence. J. Anim. Ecol. 89, 1302–1316. doi: 10.1111/1365-2656.13213
Steinhoff, P. O. M., Warfen, B., Voigt, S., Uhl, G., and Dammhahn, M. (2020). Individual differences in risk-taking affect foraging across different landscapes of fear. Oikos 129, 1891–1902. doi: 10.1111/oik.07508
Teagle, H., Hawkins, S. J., Moore, P. J., and Smale, D. A. (2017). The role of kelp species as biogenic habitat formers in coastal marine ecosystems. J. Exp. Mar. Biol. Ecol. 492, 81–98. doi: 10.1016/j.jembe.2017.01.017
Therneau, T. (2020). A Package for Survival Analysis in R. R package version 3.2-7. Available online at: https://CRAN.R-project.org/package=survival (accessed April 26, 2021).
Verges, A., Tomas, F., Cebrian, E., Ballesteros, E., Kizilkaya, Z., Dendrinos, P., et al. (2014). Tropical rabbitfish and the deforestation of a warming temperate sea. J. Ecol. 102, 1518–1527. doi: 10.1111/1365-2745.12324
Werner, E. E., and Peacor, S. D. (2003). A review of trait-mediated indirect interactions in ecological communities. Ecology 84, 1083–1100. doi: 10.1890/0012-9658(2003)084[1083:arotii]2.0.co;2
Wisenden, B. D. (2000). Olfactory assessment of predation risk in the aquatic environment. Philos. Trans. R. Soc. London Ser. B: Biol. Sci. 355, 1205–1208. doi: 10.1098/rstb.2000.0668
Wood, T. C., Kelley, R. E., and Moore, P. A. (2018). Feeding in fear: indirect effects of predatory fish on macrophyte communities mediated by altered crayfish foraging behaviour. Freshw. Biol. 63, 1523–1533. doi: 10.1111/fwb.13181
Woodland, D. (1990). Revision of the fish family siganidae with descriptions of two new species and comments on distribution and biology. Indo-Pacific Fishes 19, 121–136.
Keywords: invasive species, predator effects, foraging, rabbitfish, MPA, siganus
Citation: Shapiro Goldberg D, Rilov G, Villéger S and Belmaker J (2021) Predation Cues Lead to Reduced Foraging of Invasive Siganus rivulatus in the Mediterranean. Front. Mar. Sci. 8:678848. doi: 10.3389/fmars.2021.678848
Received: 10 March 2021; Accepted: 14 June 2021;
Published: 05 July 2021.
Edited by:
Jason Michael Hall-Spencer, University of Plymouth, United KingdomReviewed by:
Daniele Ventura, Sapienza University of Rome, ItalyCopyright © 2021 Shapiro Goldberg, Rilov, Villéger and Belmaker. This is an open-access article distributed under the terms of the Creative Commons Attribution License (CC BY). The use, distribution or reproduction in other forums is permitted, provided the original author(s) and the copyright owner(s) are credited and that the original publication in this journal is cited, in accordance with accepted academic practice. No use, distribution or reproduction is permitted which does not comply with these terms.
*Correspondence: Daphna Shapiro Goldberg, RGFwaG5hMkBtYWlsLnRhdS5hYy5pbA==
Disclaimer: All claims expressed in this article are solely those of the authors and do not necessarily represent those of their affiliated organizations, or those of the publisher, the editors and the reviewers. Any product that may be evaluated in this article or claim that may be made by its manufacturer is not guaranteed or endorsed by the publisher.
Research integrity at Frontiers
Learn more about the work of our research integrity team to safeguard the quality of each article we publish.