- 1Laboratory for Benthic Ecological Trait Analysis (L-BETA), Biological Oceanography Division, CSIR-National Institute of Oceanography (CSIR-NIO), Regional Centre, Mumbai, India
- 2Chemical Oceanography Division, CSIR-National Institute of Oceanography (CSIR-NIO), Regional Centre, Mumbai, India
The continental margin harbors a variety of habitats that support incredible biodiversity and the function of their oceans' ecosystems. The meiofauna is considered a significant component of the benthic faunal community from the polar to the tropical regions. The meiofaunal community in the deep Indian Ocean, especially along the depth gradient, is poorly investigated. The present study aims to explore the benthic meiofaunal community structure along the depth gradients and its associated environment in the western Indian continental margin (WICM) and abyssal plain in the eastern Arabian Sea. Sediment samples were collected from seven different depths (111–3,918 m) along the WICM including the oxygen minimum zone (OMZ) and abyssal plain. A total of 22 taxa (groups) were encountered along the WICM. The nematodes (85%) were the most dominant taxa in all the depths, followed by copepods (11%), nauplii (5%), and polychaetes (1.36%). Our results suggest that (a) the organic matter has accumulated in OMZ sites; (b) a high amount of total organic carbon did not influence the meiofaunal density or biomass; (c) oxygen and depth gradients were significant drivers of the meiofaunal community, low levels of oxygen contributed to lower taxa diversity and density at 485 and 724 m depths; (d) a significant relationship of meiofaunal density and biomass with chloroplastic pigment equivalent (CPE) values indicates pelagic-benthic coupling. Copepods, nauplii, tanaidaceans, isopods, kinorhynchs, and cumaceans were affected by the low-oxygen conditions at the OMZ sites. Enhanced meiofaunal diversity, density, and biomass at deeper sites (non-OMZ-D) was attributed to increased abundance of copepods, nauplii, tanaidaceans, isopods, kinorhynchs, and cumaceans and were mostly concentrated on the surface sediment (0–4 cm) triggered by enhanced bottom-water oxygen and freshness of available food outside the OMZ except 3,918 m. Therefore, the present study showed the meiofaunal community pattern with respect to depth gradients and their related environmental changes, including the OMZ along the WICM and abyssal plain in the Arabian Sea. Based on this study, the future extent of these underestimated marine ecosystems must be considered a priority to understand its functional consequences.
Introduction
The deep sea is one of the world's most biologically diverse ecosystems. The deep sea starts from about 2,000 m and extends to the Challenger Deep, the deepest ocean trench (10,927 m) in the world. The variations in depth and associated altered environments are a primary factor for the diversity of life. Moreover, this biodiversity has been less explored, and perhaps most of the undiscovered species may still be covered from the oceans (Costello et al., 2012). The continental margin is a geophysical layer covered by the thick continental crust and a thin oceanic crust that extends from the coastal to the deep sea and is comprised of the shelf (0–200 m), slope (200–3,000 m), and rise (3,000–4,000 m). Its surface area covers about 11% of the oceans' total Earth area (Menot et al., 2010). In the past few decades, a variety of habitat scale levels has been identified based on their characteristic such as geomorphological, hydrographical, geochemical and biological along the continental margin (Levin and Sibuet, 2012). Collectively, these features create unique ecological settings such as coral reefs, methane seeps, canyons, oxygen minimum zones (OMZs), seamounts, and hydrothermal vents. These hotspots and the habitat heterogeneity along the continental margin create an environment to host surprisingly high rates of population (Etter et al., 2005), biomass, physiological adaptation, species endemicity (Menot et al., 2010), and some of the most diverse array of benthic life forms on the planet (Grassle and Maciolek, 1992).
Benthic meiofauna represents a group of small-sized animals (between 31 and 500 μm) with a high turnover rate (Zeppilli et al., 2018). They are used as a food source for higher trophic levels, such as several fishes, shrimps, and shellfishes (Danovaro et al., 2007). Apart from their contribution to the food chain, meiofauna also plays a crucial role in sediment energetics and bio-indicators to assess environmental health (Heip et al., 1984; Danovaro et al., 2002; Zeppilli et al., 2015). Meiofauna act as vertical conveyors between the sediments, known as the bioturbation process (Coull, 1999). Further, they contribute to modifying the sediment hydrodynamics and biogeochemical fluxes (Aller and Aller, 1992; Schratzberger and Ingels, 2018). The understanding of the meiofaunal community along the continental margin and the abyssal plain is comparatively lower than that of shallow waters. The quantitative research of the benthic meiofaunal community below the shelf and the continental slope and abyssal depths began in the 1970s (Wigley and McIntyre, 1964; Thiel, 1966). Although, since then, the exploration of meiofauna along the continental margin has increased across all the oceans, this research is relatively limited in the Indian Ocean (Soltwedel, 2000). In the 1970s to the 1990s, the meiofaunal research along the continental margin spread to the marginal slope of East Africa in the northwestern Indian Ocean (Thiel, 1966, 1979; Romano and Dinet, 1981; Duineveld et al., 1997) and western India in the eastern Arabian Sea (Ansari et al., 1980).
The present study demonstrates the meiofaunal community along the Western Indian continental margin (WICM) is impinged upon by the OMZ. The OMZ is one of the most prominent features found in the Arabian Sea, the North Indian Ocean, which hosts about 40% of the global denitrification (Al Azhar et al., 2017). The Arabian Sea is characterized by a very pronounced midwater oxygen minimum zone (OMZ), between 150 and 1,250 m, where, the dissolved oxygen levels were observed below 0.05 mL.L−1 (Reichart et al., 1998) and were frequently changing the OMZ in space and time (Schulz et al., 1996; Lachkar et al., 2018). The western half of the Arabian Sea evidenced cyclonic and anticyclonic quasi-geostrophic mesoscale eddies and fronts with their associated meandering currents (Flagg and Kim, 1998; Shankar et al., 2002; Artamonov, 2006; Resplandy et al., 2011). The highest primary production was observed about 380 g C m−2 year−1 in the eastern part of the Arabian Sea, which was mainly driven by transition phases between SW and NE monsoon (Ivanova et al., 2003). Similarly, high primary productivity was observed off Trivandrum (Malik et al., 2015). The high organic carbon content were mainly associated with a depth of 200–1,200 m (Schulz et al., 1996). A vertical supply of nutrients is driven by eddies (Resplandy et al., 2011) with active nitrate reduction and denitrification (Morrison et al., 1999). Thus, the Arabian Sea is a very dynamic ecosystem—one of many OMZs in the world.
The benthic biodiversity along the WICM, including OMZs and abyssal plain, are poorly understood. Few studies discussed benthic macrofaunal community structure along the WICM (Ingole et al., 2010; Levin et al., 2013), while understanding meiofauna along the continental margin depths below 200 m and extended abyssal plain in the Arabian Sea is sparse. Ansari et al. (1980) studied the meiofaunal community at depths between 20 and 840 m along the continental slope in a 15°N transect. Further, Ansari et al. (2017) showed the meiofaunal community along the depths ranged 500–1,956 m off Ratnagiri region. These studies did not generate any meiofaunal data from the abyssal plain in the Arabian Sea. Further, there is a lack of continuous study from the shelf (non-OMZ), slope (including OMZ) and abyssal plain in the Arabian Sea. Therefore, the present study aims to understand the benthic meiofaunal community structure with their surrounding environment along the WICM (depths between 111 and 2,054 m), including the OMZ and abyssal plain (3,918 m).
Over the past few decades, research and reviews have shown that the abundance of benthic meiofauna generally decreases as the depth increases (Soltwedel, 2000). It has also been observed that the diversity and evenness of meiofauna are highly localized and associated with small-scale geochemical changes (Levin and Mendoza, 2007; Thurber et al., 2010; Van Gaever et al., 2010; Vanreusel et al., 2010). Generally, nematodes are the dominant groups among all meiofaunal communities and tend to be most tolerant than any other groups including polychaetes, to the lower concentration of dissolved oxygen concentration (Levin et al., 1991). Therefore, we are hypothesizing based on the previous studies that (1) the benthic meiofaunal density, biomass, and group diversity will vary due to depth gradients and changes in the concentration of DO; (2) the population will decrease with the increasing depths; and (3) rare taxon groups will be at a minimum in lower DO conditions.
Materials and Methods
Study Area and Sampling Strategy
The sample collection was carried out onboard R/V Sindhu Sadhana maintained by the CSIR-National Institute of Oceanography. The multidisciplinary cruise (SSD068) was conducted from December 7, 2019, to January 6, 2020, along the WICM, eastern Arabian Sea. The hydrographic properties (temperature, salinity, and dissolved oxygen) of the water column were measured with a CTD profiler (SBE 25, Seabird, United States) cast. The study area was categorized based on (i) geophysical features, such as the continental margin (111–2,054 m) and abyssal plain (3,918 m), and (ii) hydrographic properties, i.e., oxygen concentration: Non-OMZ-S (Shelf) (111 m), OMZ (202, 484, and 764 m), and Non-OMZ-D (Deep) (1,204, 2,054, and 3,918 m).
Onboard, sediment samples were collected with a multicore (inner diameter of 10 cm core liners) at seven different water depths (111, 202, 485, 764, 1,204, 2,054, and 3,918 m) along the 15°N transect in the Arabian Sea (Figure 1; Table 1). A single multicore with eight-core liners was deployed at each site, and three replicates (independent undisturbed core liners) were used for the meiofauna study. Another independent undisturbed core liner was used simultaneously from the same multicore deployment for measuring the various environmental conditions in the sediment. The sediment cores were sectioned vertically at every 2 cm till 10 cm depth (0–2, 2–4, 4–6, 6–8, and 8–10 cm) immediately after the multicore came onboard. All vertical sections were preserved in 4% buffered formalin with few drops of Rose Bengal (0.5 g.L−1) for meiofauna analysis (Giere, 2009). For sediment analysis, sections were frozen (−20°C) until analysis at the laboratory.
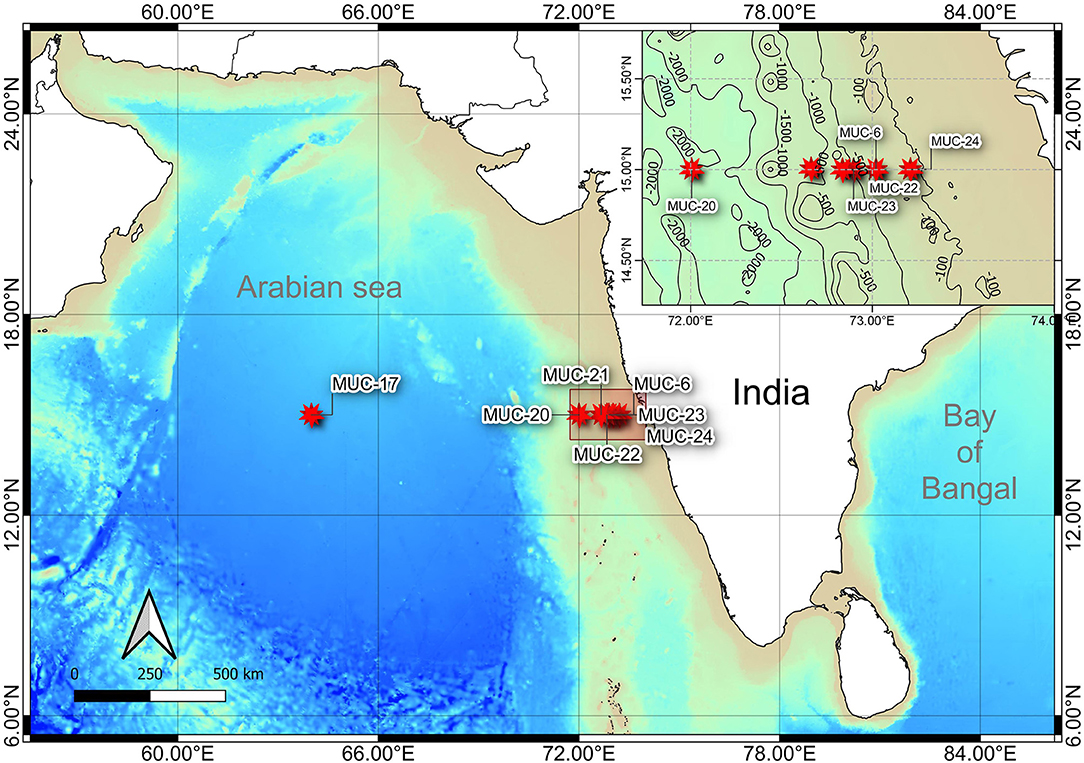
Figure 1. Bathymetric map showing the sampling locations along the Western Indian Continental Margin (WICM) and in abyssal plain, Arabian Sea. Map data: GEBCO 2019 (access date: 21.01.2021).
Laboratory Analysis
Total organic carbon (TOC) in sediment was estimated through wet-oxidation technique using standard potassium dichromate and (K2Cr2O3) and Sulfuric Acid (H2SO4), thereby titration against Ferrous Ammonium Sulfate (NH4)2Fe(SO4)2 (Walkley and Black, 1934). Analytical precision was checked through replicate analyses of selected samples (n = 10) produced precision (percentage standard deviation) of < 5% for TOC. Sediment chlorophyll (Chl a) and phaeopigments (Phaeo) were determined spectrophotometrically after extraction with 90% acetone from the sediment (Danovaro, 2010). The ratio Chl a to phaeopigments was used as the “freshness” of the organic matter (OM) (García and Thomsen, 2008). The sum of Chl a and Phaeo was used to measure an organic matter of phytodetrital origin, also known as Chloroplastic pigment equivalent (CPE). The ratio of Chl a/TOC was used to indicate the bioavailability of the bulk organic matter representing the contribution of phytoplanktonic originated organic carbon (Ramalho et al., 2014). The sediment pore water was extracted by centrifuge technique (Giere, 2009). Inorganic nutrients (NO2−, NO3−, NH4+, PO43−, and SiO4−), total nitrogen (TN), and phosphorous (TP) in all the samples were analyzed in duplicates by colorimetric method (Grasshoff et al., 1999) using a UV-Visible spectrophotometer (UV 3200). Seawater nutrient standards (OSIL, UK) and in-house standards were used to check nutrient measurement reproducibility. Analytical precision was checked by repetitive measurements for NO2−, NO3−, NH4+, PO43−, and SiO4−, obtained at 0.01, 0.07, 0.1, 0.02, and 0.2 μM, respectively. Analytical precisions for TN and TP were found as 10 and 5%, respectively.
For meiofauna, each sediment section was sieved through a stack of 300 and 32 μm mesh size for extraction of metazoan meiofauna from the rest of the fauna. Before sieving, sediments were suspended and poured on the sieve following the suspension-decantation method (Pfannkuche and Thiel, 1988). The procedure was repeated at least five times to increase extraction efficiency. Further, residual sediment was examined for leftover fauna. All individuals were counted and identified up to the group taxon level under a stereo zoom microscope (Leica S8APO, Nikon SMZ18) and images captured using an upright microscope Nikon Ei with camera attachment (Digital Sight 1000). The taxon nauplii were counted separately and then merged with copepods (Itoh et al., 2011; Neira et al., 2018). The nauplii and copepods were used for relative percentage abundance and density comparison. The taxa that contributed <1% of the total meiofaunal abundance of the study area was defined as rare taxa at each depth (Bianchelli et al., 2010).
For biomass estimation, less abundant faunal groups were picked out entirely onto slides; wherever the abundance of the faunal group was high, 100 individuals per group per core were randomly picked out for biomass estimation (Nozais et al., 2005). The sorted organisms' length and width were then computed using camera-based software IS-Capture, pre-calibrated using a standard scale. The length-width measurements were then used to determine the organisms' biomass following the formulae given by Nozais et al. (2005). Biomass of 10 groups (viz., Nematoda, Ostracoda, Kinorhyncha, Polychaeta, Oligochaeta, Arachnida, Nauplii, Tardigrada, and Turbellaria) was computed. The remaining groups were excluded from biomass calculations due to less density per core and lack of conversion factors.
Statistical Analysis
The principal component analysis (PCA) was performed to show the distribution of environmental variables (Euclidean distance) between sampling stations. Before PCA, the environmental data was normalized by transforming with Log(X+1). Pearson correlation was performed to examine multicollinearity among environmental variables and possible inter-relationships between each other. The univariate and multivariate methods have been applied as benthic assessment tools. Univariate indices were calculated for biological data, such as Species richness (S), Margalef index (d) (Margalef, 1968), Pielou's index (J′) (Pielou, 1969), and the Shannon-Wiener index (H′) (Shannon and Weaver, 1949). For multivariate analysis, non-metric multidimensional scaling (n-MDS) was implemented on meiofauna community data. Square root transformation applied on the meiofauna community with Bray–Curtis Similarity was used for n-MDS.
PERMANOVA tests were used to test the significant differences within the factors based on the total meiofauna abundance matrix (square root transformed and Euclidean distance). Similarity percentage (SIMPER) analysis was used to know the contribution of taxa within each group. Redundancy analysis (RDA) was performed to explain the relationship between meiofaunal community composition, biomass and diversity indices, and environmental variables. According to an established protocol, prior to RDA, the gradient lengths were estimated in a detrended correspondence analysis (DCA) (ter Braak and Šmilauer, 2002). No transformation was used for further analysis. The RDA explained the above 95% species-environment relationship, including both the axes. Monte Carlo permutation test used to determine significant relationships between the meiofaunal taxa and environment variables at 499 permutations under the full model. However, the significance was found very low. Therefore, the BIOENV procedure was followed to determine the best explanatory environmental variables (Euclidean distance) shaping the meiofauna community (Bray Curtis similarity) using Spearman's correlation (Clarke et al., 2014).
The univariate and multivariate methods have been applied using the PRIMER v6 software package (Clarke and Gorley, 2006) with the PERMANOVA add-on (Anderson et al., 2008). The Spearman's rank correlations were performed using XLSTAT (Version 2009.6.01, Addinsoft). DCA and RDA were performed in CANOCO v4.53 (ter Braak and Šmilauer, 2002). The study area map was constructed in QGIS v3.10 (QGIS, Development Team, 2020). Mapping data are courtesy of the General Bathymetric Chart of the Oceans Digital Database (Weatherall et al., 2019). Map contours were drawn with 15-arc resolution GEBCO 2019 data downloaded from OpenDEM public domain.
Results
Environmental Parameters
The bottom water temperature and salinity decreased proportionately with increasing depth along the WICM and abyssal plain. Bottom water temperature ranged from 1.7 °C at 3,918 m to 23.6°C at 111 m depths, and similarly, salinity ranged from 34.7 to 35.7 PSU, respectively (Table 1). The bottom water dissolved oxygen varied widely along with the depth due to intense OMZ in the WICM. The OMZ extended from the water depth of 202 m to 764 m in the present study. The depth 485 m has been considered core OMZ due to the least concentration (0.04 mL.L−1) of dissolved oxygen, while the maximum (2.71 mL.L−1) was at the abyssal plain (3,918 m) water depth during the present study. The bottom water dissolved oxygen concentration increased gradually below the OMZ toward deeper depths.
Enrichment and variability of TOC in sediments of WICM were associated with oxygen minima (Table 1). Food availabilities by quality and quantity varied along with the depths. The quantity of TOC and CPE was higher in the OMZ region than in the most profound oxygenated depth sites. The CPE value and the phaeopigment concentration at the core OMZ (485 m) were highest. The quality of available OM (Chl a/TOC) was found lowest at 485 m and 764 m within the OMZ in the WICM and in the abyssal plain (3,918 m) in the non-OMZ-D site. The ratio of Chl a/Phaeo was found to be increased with depth. The highest freshness was recorded at 2,054 m and about zero at the abyssal plain (3,918 m) (Table 1).
The pore water nutrients were found to be influenced due to the OMZ. The nutrients phosphate, silicates, nitrite, nitrate, ammonium, and total phosphorus were maximum at the OMZ site. It tends to decrease toward the non-OMZ-D sites. In contrast, total nitrogen depicted a peak at 1,204 m and its lowest point at 3,918 m.
Several environmental variables were significantly correlated with each other (Supplementary Table 1). TOC showed a significant positive relation with Chl a, phaeophytin, and CPE. Bottom water DO showed a significantly negative relation with Chl a, pheophytin and CPE. Water depth illustrated a significant positive relation with dissolved oxygen while inversely correlated to salinity, bottom water temperature, and OM freshness (Chl a/Pheo) (Supplementary Table 1).
The PCA ordination constructed from the environmental variables related to food, nutrients, and bottom water dissolved oxygen showed that the first two components accounted for about 53.6% (PC1 explained 34.6% and PC2 19%) of the data variability (Figure 2). The OMZ region (6–20) was clustered together as they were characterized by enrichment of CPE and TOC and high contribution of pore water solute, i.e., phosphate, silicate, ammonium, nitrite, nitrate, and total phosphorus, while non-OMZ-S (1–5) was separated due to enrichment of fresh and phytoplanktonic rich OM and total nitrogen. The non-OMZ-D sites were characterized by a higher value of bottom-water dissolved oxygen, CPE, and phaeophytin.
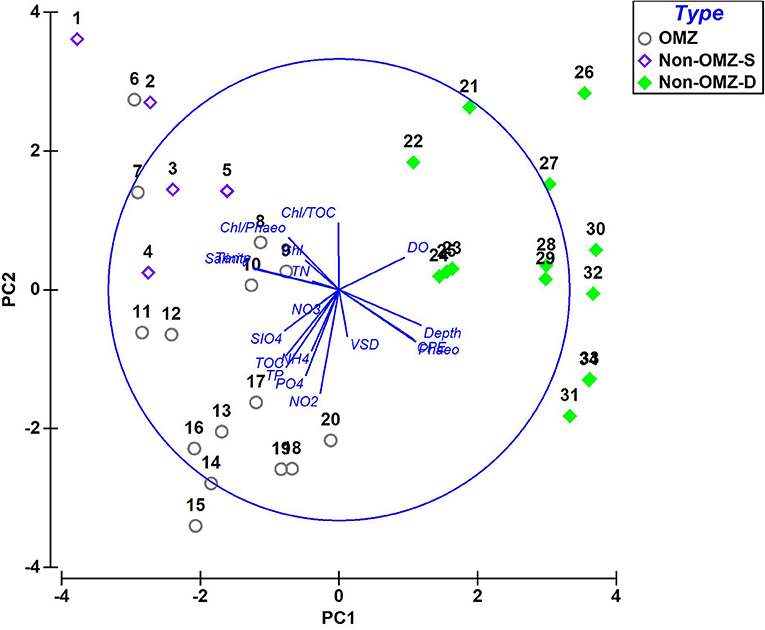
Figure 2. Principal-component analysis (PCA) derived from the contribution of parameters in each benthic zone. PC 1 and 2 accounted for 53.6% of the total variation present. Type: non-OMZ-S – 111 m (1–5); OMZ – 202 m (6–10), 485 m (11–15), 764 m (16–20); non-OMZ-D – 1,204 m (21–25), 2,054 m (26–30), 3,918 m (31–34). Numerical codes represent the sequential number of vertical sections such as 1 = 0–2 cm, 5 = 8–10 cm.
Meiofaunal Communities and the Distribution Patterns
Overall, 22 taxa (groups) were recorded in the present study. Bathymetric patterns of meiofaunal density appeared to be similar to those for meiofaunal biomass at WICM (Figure 3). The average meiofaunal density was 272.08 ± 48.06 ind. 10 cm−2 in along the transect (including continental margin and abyssal plain). The average meiofaunal density at the continental margin (111–2,054 m) was 278.26 ± 83.97 ind. 10 cm−2 and 234.99 ± 61.60 ind. 10 cm−2 at the abyssal plain (3,918 m). The highest density was recorded at non-OMZ-S (111 m) and declined afterwards in the OMZ sites up to 485 m, but it showed a gradual increase toward non-OMZ-D (2,054 m) depth. Density was generally lower than the sites of comparable depths in other OMZ regions worldwide but higher than the OMZ region of the northeast Arabian Sea. In the OMZ region, an average density ranged from 151 ± 11 (485 m) to 303 ± 16 ind. 10 cm−2 (202 m). The mean lowest abundance of the nematode was observed at 1,204 m (115 ± 111 ind. 10 cm−2) and the relative percentage composition reported maximum (97%; 294 ± 161 ind. 10 cm−2) at 202 m, while copepod and nauplii were absent (Figure 4A; Table 2). The meiofaunal density showed a marked variation between the depths while considering the non-OMZ conditions. The non-OMZ-S (111 m) was recorded with 474 ± 164 ind. 10 cm−2, while density at non-OMZ-D ranged from 166 ± 84 ind. 10 cm−2 (1,204 m) to 409 ± 113 ind. 10 cm−2 (2,054 m). All the depths were dominated by nematodes with an overall mean relative abundance of 85%, followed by copepods (11%), nauplii (5%), and polychaetes (1.36%). Copepods and nauplii were absent at 202 m, and their density increased with increasing depths (Figure 4B). The polychaetes were recorded highest 15 ± 0.9 ind. 10 cm−2 at 111 m (non-OMZ-S) and lowest 0.13 ± 0.18 ind. 10 cm−2 at 202 m within OMZ (Figure 4C). The mean meiofaunal biomass was highest (1198.84 ± 729.11 μg. 10 cm−2) in non-OMZ-D site (2,054 m), followed by (798.01 ± 320.26 μg. 10 cm−2) in the non-OMZ-S (111 m) and the least 104.46 ± 8.02 μg. 10 cm−2 at core OMZ site (485 m) (Figure 3). Copepods were dominant at non-OMZ-D (764–3,918 m) with an overall mean relative biomass of 55.18%. They were absent at the 202 m, where nematodes were found to be dominated. The second highest contributor to biomass were nematodes, followed by polychaetes and nauplii (Figure 3).
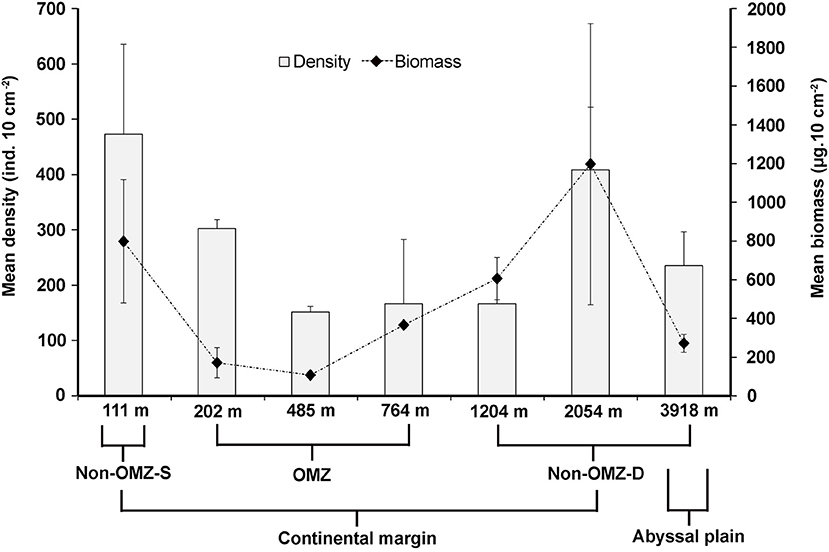
Figure 3. Distribution of total meiofaunal density, and biomass (mean ± SD) in the sediment (0–10 cm) along the depth gradient of WICM.
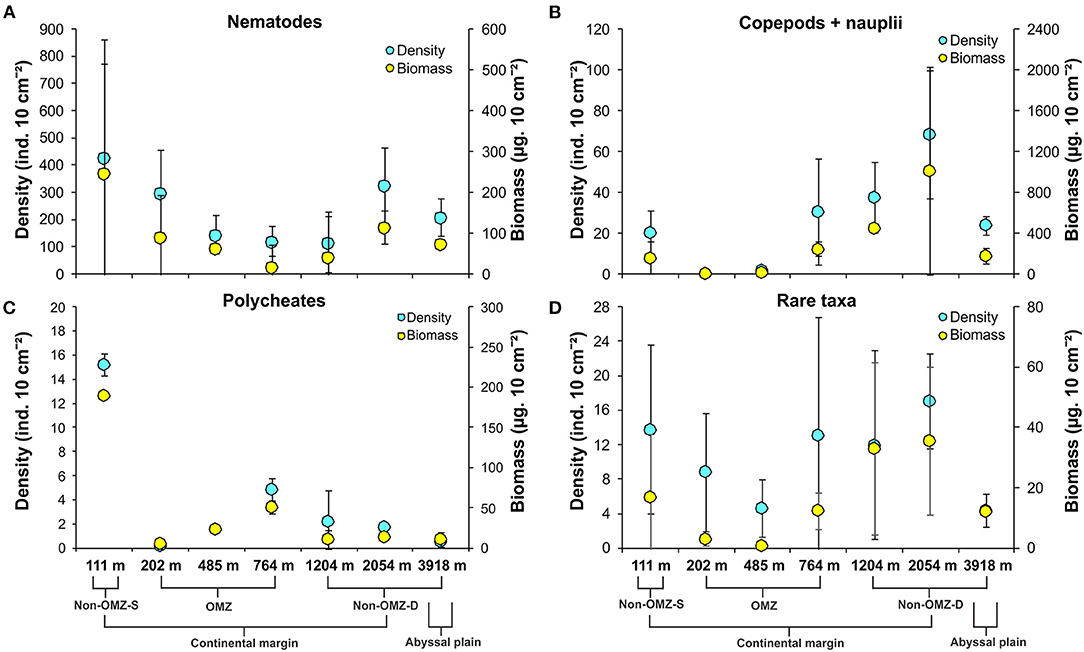
Figure 4. Distribution of density (mean ± SD) and biomass (mean ± SD) of major meiofaunal taxa along the depth gradient of the WICM and abyssal plain. (A) Nematoda; (B) Copepoda + Nauplii; (C) Polychaeta; (D). Rare taxa include nemerteans, ostracods, bivalves, kinorhynchs, gastropods, arachnids, minor phyla, tardigrades, tanaids, turbellarians, insects, oligochaetes, gastrotrichs, sipunculids, cumaceans, isopods, cnidarians, and holothurians.
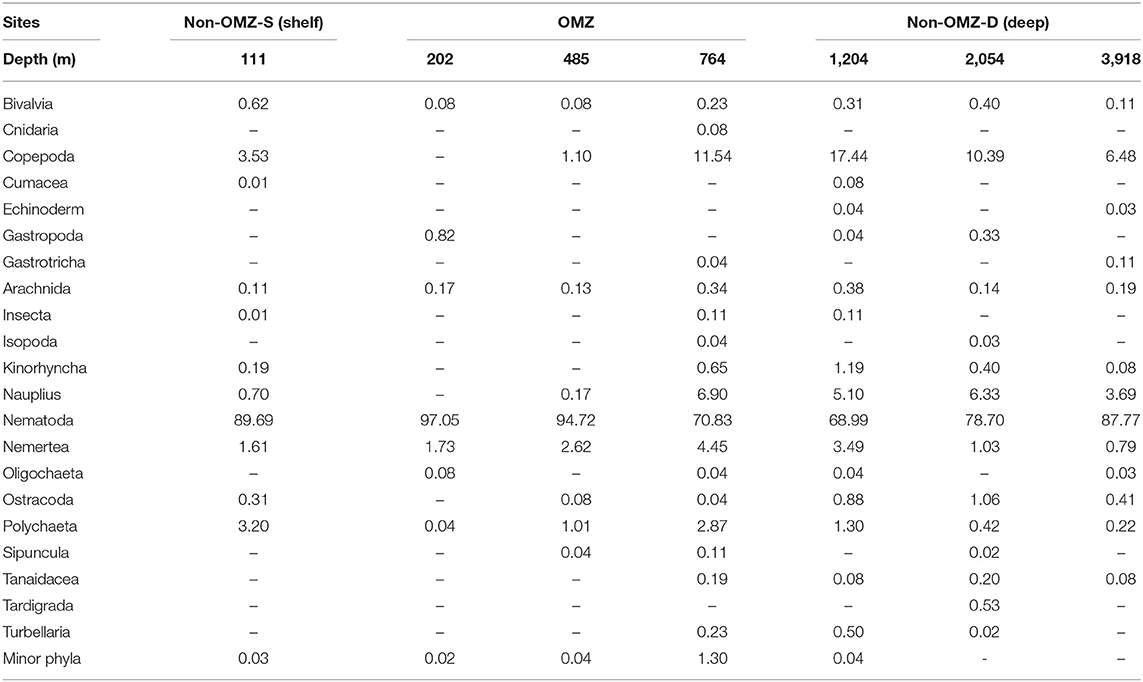
Table 2. Relative percentage abundance (%) of meiofaunal taxa at seven sites sampled along the Western Indian Continental Margin (WICM) and abyssal plain.
The taxa that contributed <1% of the total meiofaunal density are considered as rare taxa in this study (Figure 4D). The relative percentage of rare taxa found in the present study followed in decreasing order include ostracods, bivalves, kinorhynchs, gastropods, arachnids, minor phyla, tardigrades, tanaidaceans, turbellarians, insects, oligochaetes, gastrotrichs, sipunculids, cumaceans, isopods, cnidarians, and holothurians listed. These taxa were represented 3.84% cumulatively of total abundance. Nematodes and copepods were recorded as the dominant taxa in meiofaunal assemblages. Generally, this could increase the negligence in the rare taxa's relative changes and mask the presence of different taxa presence in different habitats. Therefore, taxa contributing <1% were considered rare taxa to understand their distribution pattern along the WICM.
The rare taxa distribution is shown in Figure 5. The number of rare taxa was abundant at 764 cm, falling into the OMZ region. The composition of meiofaunal assemblage inhabiting OMZ differed from the non-OMZ-S and non-OMZ-D sites. Moreover, some taxa were mainly present in a specific habitat. Tardigrades were found only at non-OMZ-D depths (2,054 and 3,918 m), while gastrotrichs and turbellarians were present below 764 m (Figure 6). Kinorhynchs were absent at OMZs depths of 202 and 485 m. The abundance of temporary meiofaunal taxa like isopods and tanaidaceans were found below 764 m. In comparison, cumaceans were founds only at non-OMZ-S (111 m) and non-OMZ-D (1,204 m).
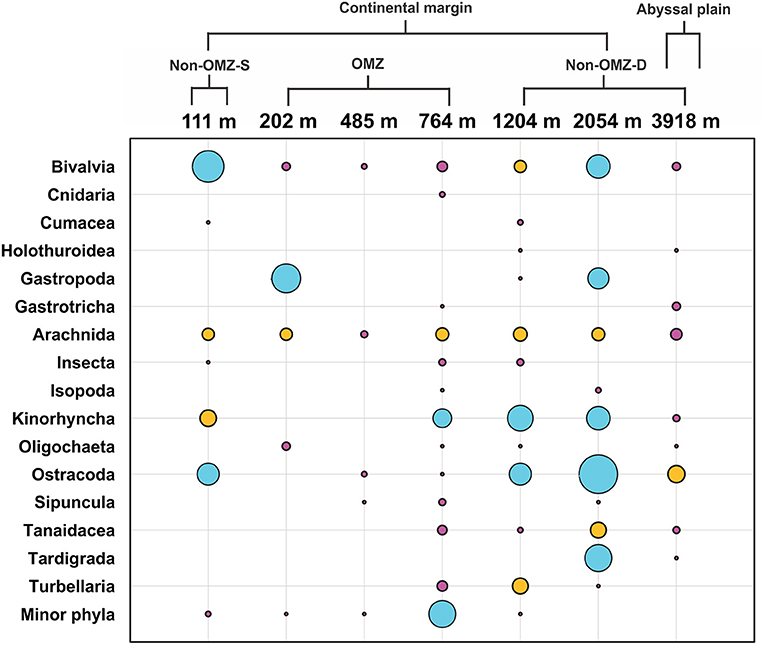
Figure 5. Structure of meiofaunal rare taxa along the depth gradient of WICM and abyssal plain. The bubble size is equivalent to the density of meiofauna in ind. 10 cm−2, the blue bubble signifies value ranges between 1 and 4.5, while the yellow ranges between 0.5 and 1, and pink <0.5.
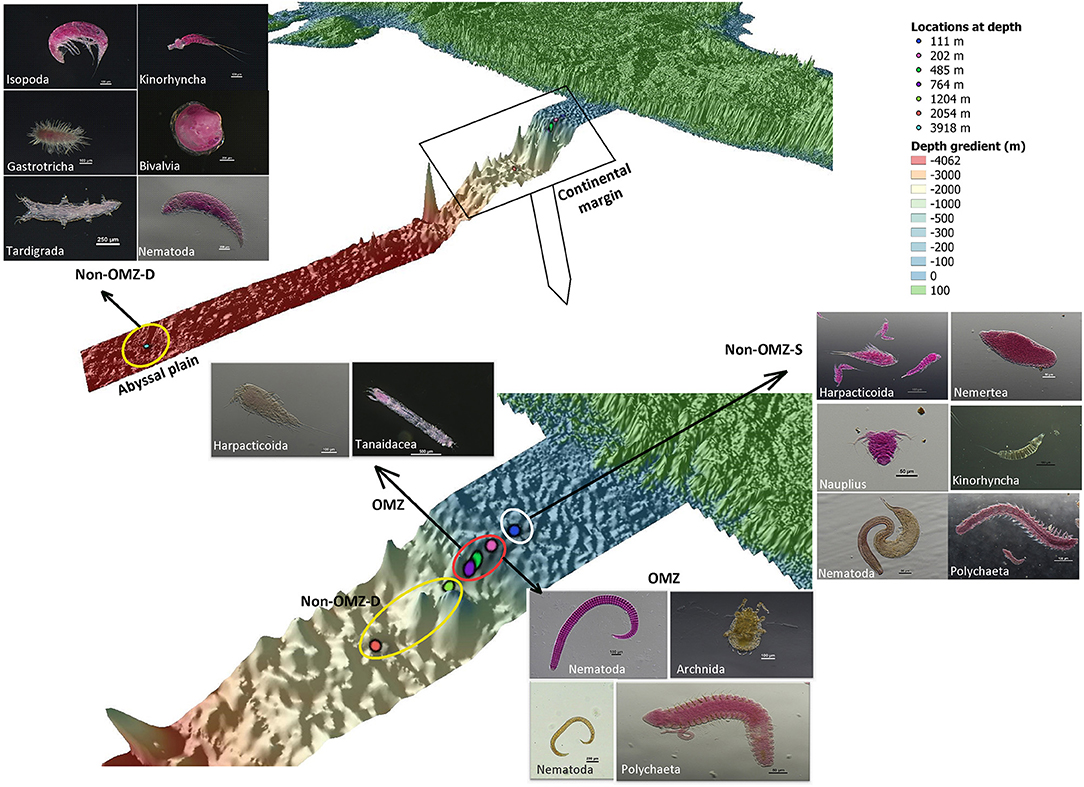
Figure 6. The schematic representation of the distribution of meiofaunal taxa along the depth gradient of WICM and abyssal plain. The stations encircled with colors denotes a particular geomorphological character as White: non-OMZ-S, Red: OMZ and Yellow: non-OMZ-D.
Univariate indices showed marked variation between OMZ and non-OMZ sites. The highest number of taxa (S) was evidenced at 764 m in OMZ and 1,204 in the non-OMZ-D site, while the least S was found within OMZ (202 and 485 m) (Supplementary Figure 1A). Other indices, i.e., d, J′, and H′, showed a similar variation to number of taxa and differed significantly between the depth gradients (Supplementary Figures 1B,C).
The n-MDS ordination of meiofaunal density was similar to meiofaunal biomass (Figure 7), comprising two groups. Group A comprises non-OMZ-S and all non-OMZ-D sites and 764 m from OMZ region, while group B included 202 m and 485 m of OMZ region. PERMONOVA carried out based on meiofaunal density, which illustrated significant effects on the depth gradient (p < 0.05). The SIMPER analysis specified the dissimilarity of 34% between groups A and B due to variation in the density of four main taxa as mentioned earlier, which highlighted copepods, nematodes, nauplii and polychaetes. The kinorhynchs, tanaidaceans, and turbellarians were absent in group B, while ostracods and bivalve showed higher densities in group A compared to group B, where gastropods density was higher at group B compared to group A (Supplementary Table 2).
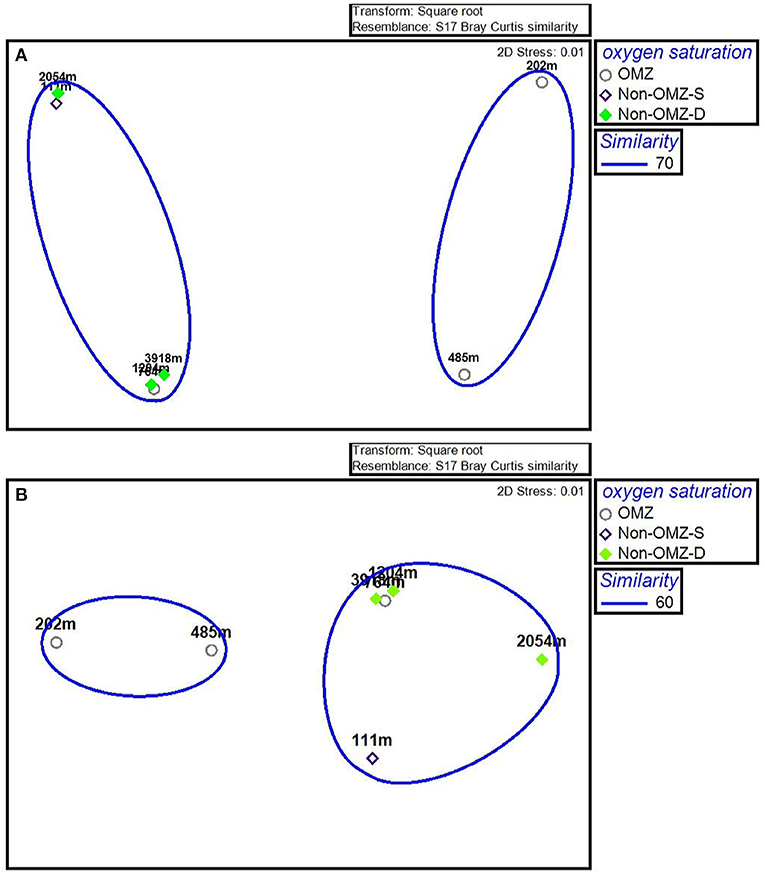
Figure 7. The n-MDS ordination depicting the meiofaunal community on the WICM. (A) Meiofaunal density grouped on 70% similarity and (B) Meiofaunal biomass grouped on 60% similarity.
Vertical Distribution in the Sediments
The vertical profile depicted 80% of the meiofauna was concentrated in the upper 0–2 cm layer followed by the 2–4 cm layer (Figure 8 and Supplementary Figure 2). The core OMZ (485 m) was observed 93% faunal concentration at 0–2 cm layer while other depths of OMZ (202 and 764 m) found density up to 4–6 cm depth in the sediment. Nematode was the dominant taxa recorded throughout the sediment core, from a sediment depth of 0 to 10 cm, but this gradually declined with increasing depth in the sediment. Nematodes contributed 67–98% to the total abundance at all the stations, while the other groups were restricted to the upper few centimeters. Copepods and nauplii were found in the upper 4 cm layer. The relative importance of copepods and nauplii increased in non-OMZ-D sites (Supplementary Figure 3). Meiofaunal biomass was maximum at the upper 0–2 cm layer (42–97%). In the shelf region (111 and 202 m), the meiofauna, was found penetrated up to 6 cm depth below the surface sediment (Figure 8 and Supplementary Figure 2). Below the shelf region, Nematodes were dominant taxa in terms of density, copepods and nauplii dominated here however it contributed higher to biomass at deeper depths (764, 1,204, 2,054, and 3,918 m) and were limited to 0–4 cm of surficial sediment depth.
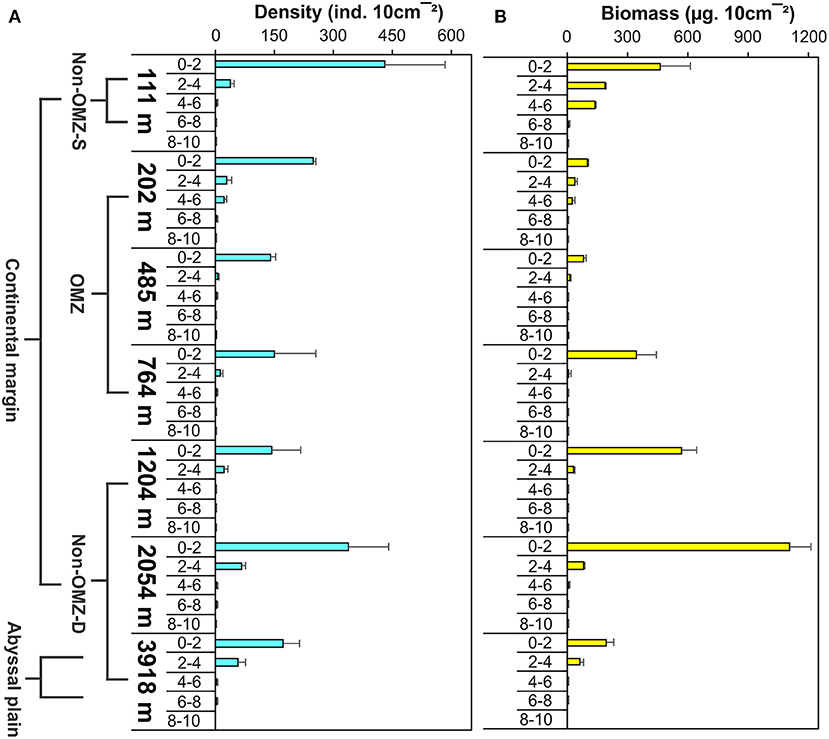
Figure 8. Vertical distribution of meiofauna within the sediment column along WICM and abyssal plain. (A) Meiofaunal density and (B) Meiofaunal biomass.
Meiofaunal Response With the Environment
The results of BIOENV analysis based on the depth gradient indicated that the TOC, Chl a, phaeophytin, CPE, quality of total available OM, depth, dissolved oxygen, phosphate, and ammonia were the primary predictor variable in the best-fit regression model for meiofaunal density that explained 73% of significance according to the permutation test (Supplementary Table 3). The forward stepwise selection method based on Monte Carlo test was not significant for meiofaunal density with environmental variables. Therefore, the best predictor variables influenced meiofaunal densities based on BIOENV procedures were considered. The first two axes of RDA explained 99.8% of the total variance between meiofaunal community structure and environmental variables. The vectors' direction specifies that TOC-related signifiers (Chl a, Phaeo, CPE, phosphate) decreased along the first axis, which was distinctly at the OMZ core site (Figure 9A). Copepods, nauplii, and other crustaceans such as isopods, kinorhynchs and ostracods mainly were reported from the surface sediment layer (0–2 cm), where they are likely associated with increased DO availability, the freshness of OM and depth. Nematodes, polychaetes, gastropods and bivalve were related to the quality of available OM, which was found at non-OMZ-S. Other taxa such as cnidarians, sipunculids, gastrotrichs, turbellarians, minor phyla appear to be associated with Depth, NH4+ and Chl a/TOC (Figure 9A).
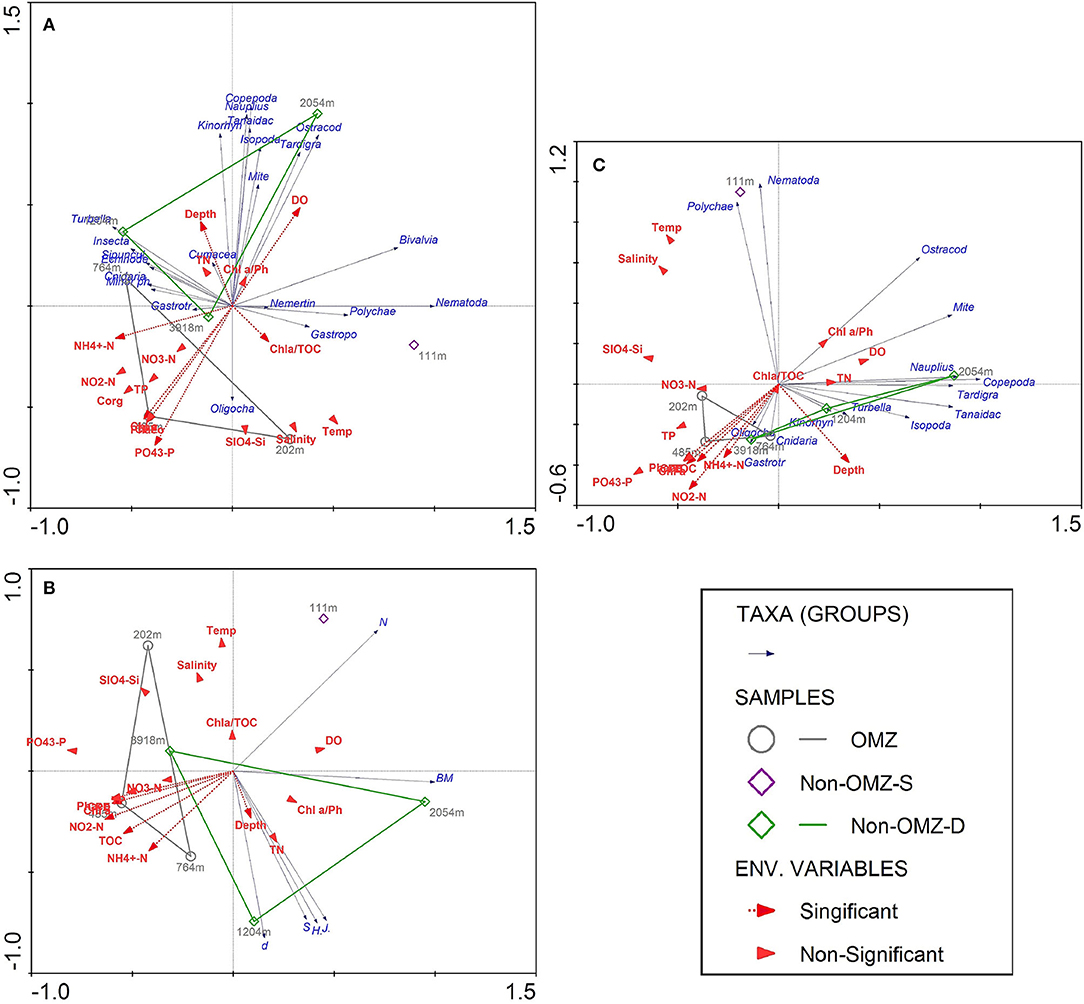
Figure 9. Redundancy analysis (RDA) depicting the meiofauna community of seven sites sampled along the WICM and abyssal plain in relation to environmental variables and depth. Dashed red arrows represent the environmental significant variables (vectors) based on BIOENV procedures. Solid blue arrows are the meiofaunal taxa (responses). Arrows pointing in the same direction are correlated, and longer arrows indicate increasing values. (A) The first two axes explained 99.8% of the total variance of taxon abundance-environment variance; (B) The first two axes explained 100% of the total variance of meiofaunal diversity-environment variance; (C) Biomass-environment variance was explained by 99% of the first 2 axis variance.
For meiofaunal biomass in WICM, Chl a, phaeophytin, quality of total available OM, depth, and phosphate emerged as the variable in the best-fit regression model, explaining 70.8% of significance (Supplementary Table 4). The stepwise forward selection was not significant with meiofaunal biomass and environment variables; thus, the BIOENV best explanatory variables were considered. The RDA analysis illustrated similar variables responsible for distributing the meiofaunal community with meiofaunal biomass (Figure 9C).
Meiofaunal diversity was best explained by TOC, pheophytin, Chl a, CPE, depth and NH4+ (Supplementary Table 5). The RDA analysis illustrated that taxon richness, diversity, and evenness were best explained by depth vector, which were distinctly increased at 1,204 m of non-OMZ-D. The vectors associated with TOC were found maximum toward the OMZ core site, while total meiofaunal density and biomass were inversely related to TOC (Figure 9B).
Discussion
The circulation of dissolved oxygen in the world oceans differs spatially with zones of the low oxygen water centered around the tropics (Stramma et al., 2008). The Arabian Sea, including the WICM, was among the near anoxic condition concentrations at its transitional depths in the oceans. Generally, the West Indian shelf and slope is influenced by the West Indian underwater current flow, which leads to slightly above anoxic condition. However, off Goa region was found anoxic and very static throughout the year (Naqvi et al., 2009). The Arabian Sea OMZ extends between longitudinal 60° and 75° E and latitudinal between 10 and 25° N (Naqvi and Noronha, 1991) and vertically between 150 and 1,200 m depths. In the present study, the OMZ was extended from a depth of 202 to 764 m, where 485 m was considered as the core of OMZ due to the least bottom water DO concentration.
Sediment TOC were significantly higher within the OMZ area than those at the non-OMZ-D, which were oxygenated. The strong inverse correlations between TOC and DO was observed, which was evidenced by earlier studies around the globe, such as Peru (Neira et al., 2001a), Central Chile (Neira et al., 2001b), off Costa Rica (Neira et al., 2018), and the western Indian continental margin (Ingole et al., 2010). The CPE was correlated strongly (r = 0.87), with TOC showing similar depth gradient observations. Therefore, the relationship of TOC, DO, and CPE suggest that the flux of sinking CPE on to the benthic sediment was greater in the oxygen-deficient water columns than in oxygenated waters. Correspondingly, the attenuation coefficient for sinking OM has been reported to be weak beneath OMZs off Peru (Martin et al., 1987), in the Arabian Sea (Haake et al., 1993), and off Mexico (Devol and Hartnett, 2001). This reduced attenuation of sinking OM may be attributed to reduced low oxygen conditions and microbial activity (Fenchel and Finlay, 1995). The high levels of dissolved phosphate in pore water at the OMZ site enhance phosphorite deposition by preserving organic sediments at low oxygen concentrations (Frank et al., 1975). The Chl a/Phaeo ratio was observed similar in OMZ sites (202, 485, and 764 m) with 0.32, which were lower than those of non-OMZ-D and higher than of non-OMZ-S. The higher values of Chl a/Phaeo ratio at two depths of non-OMZ deeper sites (1,204–2,054 m) suggest that the organic matter deposited was relatively fresh. The high TN values at 202 m and non-OMZ-S sites could indicate preferential use of nitrogen during the remineralization of organic matter of phytoplanktonic origin (Sánchez and Carriquiry, 2007).
The role of DO in structuring the benthic meiofauna, especially nematodes from the continental margins, as evidenced earlier in few studies (Cook et al., 2000; Neira et al., 2001a, 2018; Singh and Ingole, 2016). The environmental parameters including DO and meiofaunal taxa other than nematodes and meiofaunal biomass considered in the present study demonstrated new addition to the studies of meiofaunal community patterns along the WICM and abyssal plain in the Arabian Sea. The present study reports the remarkable changes in the meiofaunal taxa's diversity, density, and biomass along the depth gradient. The density was observed to be lower in the present study than OMZ off Chile (Veit-Köhler et al., 2009). The depth gradient as well as OMZ influenced overall density and biomass. Meiofaunal density and biomass usually decreases with increasing water depths (Rex and Etter, 2010). Differing diversity indices were also observed between OMZ v/s non-OMZ sites, where oxygen is the main limiting factor (Sellanes et al., 2010). In the OMZ site, the nematode density was at its maximum while crustacean density and diversity decreased (Table 2; Figure 5), which was also recorded by earlier studies from off Ratnagiri, eastern Arabian Sea (Ansari et al., 2017). Taxa diversity was reduced at 485 and 202 m. In contrast, it was found higher with a gradual increase in oxygen levels at 764 and 1,204 m depths. Similar results were represented by earlier studies (Gooday et al., 2009a; Levin et al., 2009). This resulted from favorable oxygen concentration and abundant food availability, described as the “edge effect” by earlier studies from the Pakistan margin (Levin, 2003; Gooday et al., 2010). Similarly, influence of DO on benthic community structure was reported from off Chile and Peru (Thiel, 1978), Mexico (Levin et al., 1991), and the Black Sea (Rhoads and Morse, 1971).
In the present study, the average density of nematode (114.66–425.39 ind. 10 cm−2) was recorded higher than the previous study (62.9–176.6 ind. 10 cm−2) carried out at 14°N (Singh and Ingole, 2016). The lower density of nematode encountered at 14°N may be variation in sampling gears where spade box corer (50 × 50 × 50 cm) was used for 14°N and multicore has been used in the present study. However, the reported nematode density of Oman margin ranged from 494 to 2495 ind. 10 cm−2 was higher than the present study (Cook et al., 2000). The higher density on Oman margin could be related to the DO ranges (0.13–2.99 mL.L−1), while in present study DO was reported lower (0.04–2.71 mL.L−1) than Oman margin. The reported nematode density of the core OMZ in the present study was comparable to the core OMZ (upper and lower summit of Volcano 7) of the eastern Pacific Ocean (Levin et al., 1991). The relative percentage composition of nematodes was negatively impacted by bottom water oxygen concentration (Supplementary Figure 3). The nematode relative percentage composition at 202 and 485 m was strongly enhanced due to the effect of low oxygen concentration, which led to decrease in the density of other meiofaunal taxa. The absence of harpacticoid copepods, nauplii, and kinorhynchs shows inability to tolerate low oxygen concentrations (Murrell and Fleeger, 1989) at 202 m, and their consistent increase with the increasing DO in non-OMZ-D suggest that oxygen limitations directly control meiofaunal composition at higher taxonomic levels. Similar results were observed from off Peru and Costa Rica (Neira et al., 2018), and the Arabian Sea (Ansari et al., 2017).
The mean meiofaunal biomass was highest in non-OMZ-D (2,054 m) followed by 111 m (non-OMZ-S) and was lowest at the core OMZ (485 m), which was affected by the low DO. These findings may have attributed to a process, where low oxygen concentrations may influence the body size of individuals across the whole community related changes or within specific taxa (Rex and Etter, 2010). The effect on body size at the deficient oxygen levels was documented by earlier studies in the Arabian Sea (Levin et al., 1991; Gooday et al., 2009b; Rohal et al., 2014). The biomass of copepods was more dominant at non-OMZ-D than any other taxa from metazoan meiofauna. The experimental studies have shown that crustaceans were the most susceptible to changes in oxygen levels. The LC50 (in mg O2 L−1) of crustaceans were higher than for other organisms studied (Vaquer-Sunyer and Duarte, 2008). The predictions were made that the fishes should be the first to be affected among benthic fauna, followed by crustaceans, worms, echinoderms, and molluscs as oxygen lowers (Vaquer-Sunyer and Duarte, 2008). A similar effect was also documented through experimental studies, where crustaceans such as shore crabs Carcinus maenas (L.) and shrimp Crangon crangon (L.) in the Danish fjords (Jørgensen and Jorgensen, 1980) and amphipod Monoporeia affinis, harpacticoids Microarthridion littorale, and Pseudobradya sp. in the Baltic Sea (Modig and Ólafsson, 1998) were wiped out due to low oxygen levels.
Results showed marked variations in the assemblages of rare taxa between OMZ and non-OMZ sites. The masking of the relative importance and the distribution of rare taxa in different habitats was caused by the dominance of nematodes and copepods (Bianchelli et al., 2010). Moreover, some taxa were exclusively observed in specific depths and their associated habitat. The isopods and tanaidaceans were found below 764 m at non-OMZ-D depths. However, cumaceans were recorded at 111 and 1,204 m, which were subjected to lower food quality and freshness (Cartes and Sorbe, 1996). Therefore, it seems like oxygen was the limiting factor for distribution of isopods, tanaidaceans and cumaceans, was supported by study in the OMZ off Chile (Veit-Köhler et al., 2009). However, tardigrades, gastrotrichs, turbellarians, and kinorhynchs were utterly absent in OMZ sites. Gastrotrichs and kinorhynchs were also absent in the low oxygen concentration sites Ant-7104 and Con-7161 off Chile (Veit-Köhler et al., 2009).
The sediment vertical distribution of meiofaunal density and biomass at WICM ranged similar to OMZ off Costa Rica (Neira et al., 2018), due to the approx. homologous environmental conditions and geographical location (latitude) among both regions. The maximum fauna was found in the upper 4 cm of the sediment depth, where the OM flux is low (Lambshead and Hodda, 1994). The community size structure was smaller, evidenced by the decrease in biomass wherever nematodes were dominant at the OMZ site. The annelids and their function (bioturbation) were reported in a few studies from OMZ and mainly contributed via tolerant annelids to hypoxic conditions (Levin et al., 2000; Smith et al., 2000). This may enhance the sediment layers' reworking and induce mixing downward (Levin et al., 1991), which supports nematode survival rates in the subsurface and deeper sediments (Braeckman et al., 2010). The enhanced relative proportion of copepods at non-OMZ-D sites influences the total meiofaunal biomass because of their comparably high average individual body weight (Jensen, 1988).
The meiofaunal density and biomass were known to influence by several aspects, for example, habitat heterogeneity (Gooday et al., 2010), bathymetric patterns (Gambi et al., 2010), food availability (Cook et al., 2000), and oxygen concentration (Levin, 2003; Neira et al., 2018). In the present study, the RDA plot signifies the role of dissolved oxygen (Figure 9), where a positive correlation of meiofaunal density, biomass and a number of crustacean's taxa was shown. The meiofaunal taxon richness (S and d), diversity (H′) and taxonomic evenness (J′) was found to be distinctly lower at 202 and 485 m of OMZ stations (Supplementary Figure 1), while depth also showed significant positive relation (Figure 9C). Furthermore, the meiofaunal density and biomass were observed to be positively related to food input and quality. A strong positive relation of TOC and its signifiers Chl a, phaeophytin, CPE, at OMZ (Figure 9) affect meiofaunal density, diversity and biomass which is represented by BIOENV results (Supplementary Tables 3–5). The influence of organic matter perturbation in the sediment on meiofaunal biomass has been recorded in earlier studies (Soltwedel, 2000).
OM quality and bioavailability generally decrease with depth due to degradation in the water column process. Due to this, an increase in meiofaunal density was observed at the shelf area due to OM's freshness and bioavailable OM quality (Figure 3). Nematodes and polychaetes density and biomass showed a positive relationship with OM's freshness and quality, which were illustrated in RDA plot (Figure 9). Therefore, meiofaunal density and biomass decrease with increasing depths. The CPE values were comparatively high, and meiofaunal density and abundance were found to be low at the core OMZ. Similar results were recorded at NW African margin (Soltwedel, 1997), and it has been suggested that phytodetritus deposits in that area had been transported over long distances and, therefore, were more degraded. Several investigations in OMZs from other parts of the world have noted a correlation between food quality and the lack of oxygen (Danovaro et al., 2000; Neira et al., 2001a). Therefore, food availability is a regulating factor along with the vertical sediment profile (Ingels et al., 2009). The importance of food quantity and availability on nematode abundance and diversity has been emphasized by Cook et al. (2000) from the Arabian Sea.
Conclusion
The present study reveals several characteristics of benthic meiofaunal communities and their response to changing depths and associated environmental factors primarily DO along the WICM. The study area was classified and summarized below with corresponding meiofaunal community characteristics based on the geophysical and hydrographic characters. The depth ranges and their related changes in environmental characteristics recorded along the WICM. The continental margin was observed with different DO concentrations, including intense OMZ with higher TOC and CPE. The density and biomass of the meiofaunal community were low within the OMZ region, where phytodetritus (CPE), TOC, and pore water nutrient were accumulated in higher amounts. The meiofaunal density showed a higher value in non-OMZ-S, while the lowest recorded at the intense OMZ (485 m) region. The diversity was observed moderate at non-OMZ-S and D, while the highest recorded at lower OMZ (764 m). The rare taxon showed the minimum richness at the lowest DO concentration along the OMZ while increasing in developing DO values. However, the result of the present study did not support our third hypothesis, as the population density did not show a trend of decreasing with increasing depths along the WICM, which could be due to several factors like OMZ and different geophysical structure in the study area. OMZs represent a principal barrier for benthic species between abyssal plain (food limitation) and continental shelf (plenty of food). These ecosystems may hold an answer to unaddressed questions such as diversified genetic speciation through evolutionary adaptations to ever reducing oxygenation of the world ocean due to global warming and eutrophication. Moreover, this study suggests that more exploration of the meiofaunal community will allow us to understand how the community patterns influence the ecosystem functioning along the heterogenous WICM and abyssal floor in the Arabian Sea.
Data Availability Statement
The original contributions presented in the study are included in the article/Supplementary Material; further inquiries can be directed to the corresponding author/s.
Author Contributions
SS designed this study. SS and SG carried out the field sampling. SK, SA, AC, and SC analyzed the meiofauna. UP, BS, SG, and SC estimated environmental variables. SS, SG, and SK performed statistical analysis, plotted map, figures, and wrote the manuscript. All authors contributed to the article and approved the submitted version.
Funding
This study received logistic and financial support for a cruise (SSD-068) from the institutional fund only.
Conflict of Interest
The authors declare that the research was conducted in the absence of any commercial or financial relationships that could be construed as a potential conflict of interest.
Acknowledgments
We are thankful to the Director, CSIR-NIO, India, and the Scientist-in-charge, CSIR–NIO, Regional Centre, Mumbai, for providing facilities. Authors wish to acknowledge Dr. Haimanti Biswas, Principal Scientist, CSIR-NIO, and Chief Scientist, Cruise SSD068, and Late Dr. Veronica Fernandes, Scientist, and Ship cell people, for their logistical and technical support during the cruise. We also thank the Captain and all support staff for their help during the cruise SSD068. SG would like to acknowledge the Council of Industrial and Scientific Research (CSIR), India, for awarding and granting the Senior Research Fellowship (CSIR-SRF). This is CSIR-NIO contribution No. 6748.
Supplementary Material
The Supplementary Material for this article can be found online at: https://www.frontiersin.org/articles/10.3389/fmars.2021.671444/full#supplementary-material
References
Al Azhar, M., Lachkar, Z., Lévy, M., and Smith, S. (2017). Oxygen minimum zone contrasts between the Arabian Sea and the Bay of Bengal implied by differences in remineralization depth. Geophys. Res. Lett. 44:114. doi: 10.1002/2017GL075157
Aller, R. C., and Aller, J. Y. (1992). Meiofauna and solute transport in marine muds. Limnol. Oceanogr. 37, 1018–1033. doi: 10.4319/lo.1992.37.5.1018
Anderson, M. J., Gorley, R. N., and Clarke, K. R. (2008). PERMANOVA+ for PRIMER: Guide to Software and Statistical Methods. Plymouth: PRIMER-E Ltd.
Ansari, Z. A., Badesab, S., Singh, R., and Kitazato, H. (2017). Meiofaunal distribution across the oxygen minimum zone of continental margin, North East Arabian Sea. Int. J. Mar. Sci. 7, 59–66. doi: 10.5376/ijms.2017.07.0007
Ansari, Z. A., Parulekar, A. H., and Jagtap, T. G. (1980). Distribution of sub-littoral meiobenthos off Goa coast, India. Hydrobiologia 74, 209–214. doi: 10.1007/BF00008754
Artamonov, Y. V. (2006). “The circulation and structure of water masses ofthe Arabian Sea based on synoptic surveys,” in The Mesoscale Structure ofthe Epipelagic Ecosystem of the Open Northern Arabian Sea, eds K. Banse and S. A. Piontkovski (Hyderabad: Universities Press), 12–46.
Bianchelli, S., Gambi, C., Zeppilli, D., and Danovaro, R. (2010). Metazoan meiofauna in deep-sea canyons and adjacent open slopes: a large-scale comparison with focus on the rare taxa. Deep. Res. Part I Oceanogr. Res. Pap. 57, 420–433. doi: 10.1016/j.dsr.2009.12.001
Braeckman, U., Provoost, P., Gribsholt, B., Van Gansbeke, D., Middelburg, J. J., Soetaert, K., et al. (2010). Role of macrofauna functional traits and density in biogeochemical fluxes and bioturbation. Mar. Ecol. Prog. Ser. 399, 173–186. doi: 10.3354/meps08336
Cartes, J. E., and Sorbe, J. C. (1996). Temporal population structure of deep-water cumaceans from the western Mediterranean slope. Deep. Res. Part I Oceanogr. Res. Pap. 43, 1423–1438. doi: 10.1016/S0967-0637(96)00067-2
Clarke, K. R., Gorley, R., Somerfield, P., and Warwick, R. (2014). Change in Marine Communities: An Approach to Statistical Analysis and Interpretation, 3rd edition. Plymouth: Prim.
Cook, A. A., John, P., Hawkins, L. E., Mitchell, N., and Levin, L. A. (2000). Nematode abundance at the oxygen minimum zone in the Arabian Sea. Deep. Res. Part II Top. Stud. Oceanogr. 47, 75–85. doi: 10.1016/S0967-0645(99)00097-1
Costello, M. J., Wilson, S., and Houlding, B. (2012). Predicting total global species richness using rates of species description and estimates of taxonomic effort. Syst. Biol. 61, 871–883. doi: 10.1093/sysbio/syr080
Coull, B. C. (1999). Role of meiofauna in estuarine soft-bottom habitats. Aust. J. Ecol. 24, 327–343. doi: 10.1046/j.1442-9993.1999.00979.x
Danovaro, R. (2010). Methods for the study of Deep-sea Sediments, Thier Functioning and Biodiversity. Boca Raton, MA: CRC Press Taylor & Francis Group. doi: 10.1201/9781439811382
Danovaro, R., Gambi, C., and Mirto, S. (2002). Meiofaunal production and energy transfer efficiency in a seagrass Posidonia oceanica bed in the western Mediterranean. Mar. Ecol. Prog. Ser. 234, 95–104. doi: 10.3354/meps234095
Danovaro, R., Scopa, M., Gambi, C., and Fraschetti, S. (2007). Trophic importance of subtidal metazoan meiofauna: evidence from in situ exclusion experiments on soft and rocky substrates. Mar. Biol. 152, 339–350. doi: 10.1007/s00227-007-0696-y
Danovaro, R., Tselepides, A., Otegui, A., and Della Croce, N. (2000). Dynamics of meiofaunal assemblages on the continental shelf and deep-sea sediments of the Cretan Sea (NE Mediterranean): relationships with seasonal changes in food supply. Prog. Oceanogr. 46, 367–400. doi: 10.1016/S0079-6611(00)00026-4
Devol, A. H., and Hartnett, H. E. (2001). Role of the oxygen-deficient zone in transfer of organic carbon to the deep ocean. Limnol. Oceanogr. 46, 1684–1690. doi: 10.4319/lo.2001.46.7.1684
Duineveld, G. C. A., De Wilde, P. A. W. J., Berghuis, E. M., Kok, A., Tahey, T., and Kromkamp, J. (1997). Benthic respiration and standing stock on two contrasting continental margins in the western Indian Ocean: The Yemen-Somali upwelling region and the margin off Kenya. Deep. Res. Part II Top. Stud. Oceanogr. 44, 1293–1317. doi: 10.1016/S0967-0645(97)00006-4
Etter, R. J., Rex, M. A., Chase, M. R., and Quattro, J. M. (2005). Population differentiation decreases with depth in deep-sea bivalves. Evolution. 59, 1479–1491. doi: 10.1111/j.0014-3820.2005.tb01797.x
Fenchel, T., and Finlay, B. J. (1995). Ecology and evolution in anoxic worlds. Ecol. Evol. Anoxic Worlds. 9, 259–260.
Flagg, C. N., and Kim, H. S. (1998). Upper ocean currents in the northern Arabian Sea from shipboard ADCP measurements collected during the 1994-1996 U.S. JGOFS and ONR programs. Deep. Res. Part II Top. Stud. Oceanogr. 45, 1917–1959. doi: 10.1016/S0967-0645(98)00059-9
Frank, M., Rowe, G. T., and Jipa, D. (1975). Marine phosphorite formation off Peru. SEPM J. Sediment. Res. 45, 243–251. doi: 10.1306/212F6D20-2B24-11D7-8648000102C1865D
Gambi, C., Lampadariou, N., and Danovaro, R. (2010). Latitudinal, longitudinal and bathymetric patterns of abundance, biomass of metazoan meiofauna: Importance of the rare taxa and anomalies in the deep Mediterranean Sea. Adv. Oceanogr. Limnol. 1, 167–197. doi: 10.4081/aiol.2010.5299
García, R., and Thomsen, L. (2008). Bioavailable organic matter in surface sediments of the Nazaré canyon and adjacent slope (Western Iberian Margin). J. Mar. Syst. 74, 44–59. doi: 10.1016/j.jmarsys.2007.11.004
Giere, O. (2009). Meiobenthology: The Microscopic Motile Fauna of Aquatic Sediments. Berlin; Heidelberg: Springer Science & Business Media.
Gooday, A. J., Bett, B. J., Escobar, E., Ingole, B., Levin, L. A., Neira, C., et al. (2010). Habitat heterogeneity and its influence on benthic biodiversity in oxygen minimum zones. Mar. Ecol. 31, 125–147. doi: 10.1111/j.1439-0485.2009.00348.x
Gooday, A. J., Jorissen, F., Levin, L. A., Middelburg, J. J., Naqvi, S. W. A., Rabalais, N. N., et al. (2009a). Historical records of coastal eutrophication-induced hypoxia. Biogeosciences 6, 1707–1745. doi: 10.5194/bg-6-1707-2009
Gooday, A. J., Levin, L. A., Aranda da Silva, A., Bett, B. J., Cowie, G. L., Dissard, D., et al. (2009b). Faunal responses to oxygen gradients on the Pakistan margin: A comparison of foraminiferans, macrofauna and megafauna. Deep. Res. Part II Top. Stud. Oceanogr. 56, 488–502. doi: 10.1016/j.dsr2.2008.10.003
Grasshoff, K., Kremling, K., and Ehrhardt, M. (1999). Methods of Seawater Analysis. New York, NY: Wiley-VCH. doi: 10.1002/9783527613984
Grassle, J. F., and Maciolek, N. J. (1992). Deep-sea species richness: regional and local diversity estimates from quantitative bottom samples. Am. Nat. 139, 313–341. doi: 10.1086/285329
Haake, B., Ittekkot, V., Rixen, T., Ramaswamy, V., Nair, R. R., and Curry, W. B. (1993). Seasonality and interannual variability of particle fluxes to the deep Arabian sea. Deep. Res. Part I 40, 1323–1344. doi: 10.1016/0967-0637(93)90114-I
Heip, C., Herman, R., and Vincx, M. (1984). Variability and productivity of meiobenthos in the Southern Bight of the North Sea. Cons. Perm. Int. pour l'exploration la mer 183, 51–56.
Ingels, J., Kiriakoulakis, K., Wolff, G. A., and Vanreusel, A. (2009). Nematode diversity and its relation to the quantity and quality of sedimentary organic matter in the deep Nazaré Canyon, Western Iberian Margin. Deep. Res. Part I Oceanogr. Res. Pap. 56, 1521–1539. doi: 10.1016/j.dsr.2009.04.010
Ingole, B. S., Sautya, S., Sivadas, S., Singh, R., and Nanajkar, M. (2010). Macrofaunal community structure in the western Indian continental margin including the oxygen minimum zone. Mar. Ecol. 31, 148–166. doi: 10.1111/j.1439-0485.2009.00356.x
Itoh, M., Kawamura, K., Kitahashi, T., Kojima, S., Katagiri, H., and Shimanaga, M. (2011). Bathymetric patterns of meiofaunal abundance and biomass associated with the Kuril and Ryukyu trenches, western North Pacific Ocean. Deep. Res. Part I Oceanogr. Res. Pap. 58, 86–97. doi: 10.1016/j.dsr.2010.12.004
Ivanova, E., Schiebel, R., Deo Singh, A., Schmiedl, G., Niebler, H.-S., and Hemleben, C. (2003). Primary production in the Arabian Sea during the last 135 000 years. Palaeogeogr. Palaeoclimatol. Palaeoecol. 197, 61–82. doi: 10.1016/S0031-0182(03)00386-9
Jensen, P. (1988). Nematode assemblages in the deep-sea benthos of the Norwegian Sea. Deep Sea Res. Part A, Oceanogr. Res. Pap. 35, 1173–1184. doi: 10.1016/0198-0149(88)90008-8
Jørgensen, B. B., and Jorgensen, B. B. (1980). Seasonal oxygen depletion in the bottom waters of a danish fjord and its effect on the benthic community. Oikos 34, 68. doi: 10.2307/3544551
Lachkar, Z., Lévy, M., and Smith, S. (2018). Intensification and deepening of the Arabian Sea oxygen minimum zone in response to increase in Indian monsoon wind intensity. Biogeosciences 15, 159–186. doi: 10.5194/bg-15-159-2018
Lambshead, P. J. D., and Hodda, M. (1994). The impact of disturbance on measurements of variability in marine nematode populations. Vie Milieu 44, 21–27.
Levin, L. (2003). Oxygen minimum zone benthos: adaptation and community response to hypoxia. Oceanogr. Mar. Biol. an Annu. Rev. 41, 1–45.
Levin, L. A., Ekau, W., Gooday, A. J., Jorissen, F., Middelburg, J. J., Naqvi, S. W. A., et al. (2009). Effects of natural and human-induced hypoxia on coastal benthos. Biogeosciences 6, 2063–2098. doi: 10.5194/bg-6-2063-2009
Levin, L. A., Gage, J. D., Martin, C., and Lamont, P. A. (2000). Macrobenthic community structure within and beneath the oxygen minimum zone, NW Arabian Sea. Deep. Res. Part II Top. Stud. Oceanogr. 47, 189–226. doi: 10.1016/S0967-0645(99)00103-4
Levin, L. A., Huggett, C. L., and Wishner, K. F. (1991). Control of deep-sea benthic community structure by oxygen and organic-matter gradients in the eastern Pacific Ocean. J. Mar. Res. 49, 763–800. doi: 10.1357/002224091784995756
Levin, L. A., McGregor, A. L., Mendoza, G. F., Woulds, C., Cross, P., Witte, U., et al. (2013). Macrofaunal colonization across the Indian margin oxygen minimum zone. Biogeosciences 10, 7161–7177. doi: 10.5194/bg-10-7161-2013
Levin, L. A., and Mendoza, G. F. (2007). Community structure and nutrition of deep methane-seep macrobenthos from the North Pacific (Aleutian) Margin and the Gulf of Mexico (Florida Escarpment). Mar. Ecol. 28, 131–151. doi: 10.1111/j.1439-0485.2006.00131.x
Levin, L. A., and Sibuet, M. (2012). Understanding continental margin biodiversity: A new imperative. Ann. Rev. Mar. Sci. 4, 79–112. doi: 10.1146/annurev-marine-120709-142714
Malik, A., Fernandes, C. E. G., Gonsalves, M. J. B. D., Subina, N. S., Mamatha, S. S., Krishna, K., et al. (2015). Interactions between trophic levels in upwelling and non-upwelling regions during summer monsoon. J. Sea Res. 95, 56–69. doi: 10.1016/j.seares.2014.10.012
Martin, J. H., Knauer, G. A., Karl, D. M., and Broenkow, W. W. (1987). VERTEX: carbon cycling in the northeast Pacific. Deep Sea Res. Part A, Oceanogr. Res. Pap. 34, 267–258. doi: 10.1016/0198-0149(87)90086-0
Menot, L., Sibuet, M., Carney, R. S., Levin, L. A., Rowe, G. T., Billett, D. S. M., et al. (2010). “New perceptions of continental margin biodiversity,” in Life in the World's Oceans: Diversity, Distribution, and Abundance, ed A. D. McIntyre (Wiley-Blackwell Publishing Ltd.). doi: 10.1002/9781444325508.ch5
Modig, H., and Ólafsson, E. (1998). Responses of Baltic benthic invertebrates to hypoxic events. J. Exp. Mar. Bio. Ecol. 229, 133–148. doi: 10.1016/S0022-0981(98)00043-4
Morrison, J. M., Codispoti, L. A., Smith, S. L., Wishner, K., Flagg, C., Gardner, W. D., et al. (1999). The oxygen minimum zone in the Arabian Sea during 1995. Deep. Res. Part Ii-Topical Stud. Oceanogr. 46, 1903–1931. doi: 10.1016/S0967-0645(99)00048-X
Murrell, M. C., and Fleeger, J. W. (1989). Meiofauna abundance on the Gulf of Mexico continental shelf affected by hypoxia. Cont. Shelf Res. 9, 1049–1062. doi: 10.1016/0278-4343(89)90057-5
Naqvi, S. W. A., Naik, H., Jayakumar, A., Pratihary, A. K., Narvenkar, G., Kurian, S., et al. (2009). “Seasonal anoxia over the Western Indian continental shelf,” in Geophysical Monograph Series, Geographic Information System eds J. D. Wiggert, R. R. Hood, S. W. A. Naqvi, K. H. Brink, and S. L. Smith (Washington, DC: American Geophysical Union).
Naqvi, S. W. A., and Noronha, R. J. (1991). Nitrous oxide in the Arabian Sea. Deep Sea Res. Part A, Oceanogr. Res. Pap. 38, 871–890. doi: 10.1016/0198-0149(91)90023-9
Neira, C., Ingels, J., Mendoza, G., Hernandez-Lopez, E., and Levin, L. A. (2018). Distribution of meiofauna in bathyal sediments influenced by the oxygen minimum zone off Costa Rica. Front. Mar. Sci. 5, 1–17. doi: 10.3389/fmars.2018.00448
Neira, C., Sellanes, J., Levin, L. A., and Arntz, W. E. (2001a). Meiofaunal distributions on the Peru margin: Relationship to oxygen and organic matter availability. Deep Sea Res. Part I Oceanogr. Res. Pap. 48, 2453–2472. doi: 10.1016/S0967-0637(01)00018-8
Neira, C., Sellanes, J., Soto, A., Gutiérrez, D., and Gallardo, V. A. (2001b). Meiofauna and sedimentary organic matter off Central Chile: response to changes caused by the 1997-1998 El Niño. Oceanol. Acta 24, 313–328. doi: 10.1016/S0399-1784(01)01149-5
Nozais, C., Perissinotto, R., and Tita, G. (2005). Seasonal dynamics of meiofauna in a South African temporarily open/closed estuary (Mdloti Estuary, Indian Ocean). Estuar. Coast. Shelf Sci. 62, 325–338. doi: 10.1016/j.ecss.2004.09.020
Pfannkuche, O., and Thiel, H. (1988). “Sample Processing,” in Introduction to the Study of Meiofauna, eds R. P. Higgins and H. Thiel (Washington DC: Smithsonian Institute Press), 134–145.
QGIS, Development Team (2020). QGIS Geographic Information System. Open Source Geospatial Foundation Project. Available online at: http://www.qgis.org/ (accessed January 20, 2021).
Ramalho, S. P., Adão, H., Kiriakoulakis, K., Wolff, G. A., Vanreusel, A., and Ingels, J. (2014). Temporal and spatial variation in the Nazaré Canyon (Western Iberian margin): Inter-annual and canyon heterogeneity effects on meiofauna biomass and diversity. Deep. Res. Part I Oceanogr. Res. Pap. 83, 102–114. doi: 10.1016/j.dsr.2013.09.010
Reichart, G.-J. J., Lourens, L. J., and Zachariasse, W. J. (1998). Temporal variability in the northern Arabian Sea Oxygen Minimum Zone (OMZ) during the last 225,000 year. Paleoceanography 13, 607–621. doi: 10.1029/98PA02203
Resplandy, L., Lévy, M., Madec, G., Pous, S., Aumont, O., and Kumar, D. (2011). Contribution of mesoscale processes to nutrient budgets in the Arabian Sea. J. Geophys. Res. Ocean. 116:C11007. doi: 10.1029/2011JC007006
Rex, M., and Etter, R. (2010). Deep-Sea Biodiversity: Pattern and Scale. Cambridge: Harvard University Press.
Rhoads, D. C., and Morse, J. W. (1971). Evolutionary and ecologic significance of oxygen-deficient marine basins. Lethaia 4, 413–428. doi: 10.1111/j.1502-3931.1971.tb01864.x
Rohal, M., Thistle, D., and Easton, E. E. (2014). Meiofaunal abundances and faunal similarity on the continental rise off the coast of California. Deep. Res. Part I Oceanogr. Res. Pap. 93, 131–144. doi: 10.1016/j.dsr.2014.07.004
Romano, J. C., and Dinet, A. (1981). “Rélation entre l'abondance du méiobenthos et la biomasse des sédimento superficiels estimée par la mesure des adénosines 5'phosphate (ATP, ADP, AMP),” in Géochimie organique des sédiments marins profonds, ORGON IV, Golfe d'Aden, Mer d'Oman, eds R. Pelet, C. Caratini, M. Arnould, S. Garlenc, and P. Laborde (Revue de l'Institut Français du Pétrole).
Sánchez, A., and Carriquiry, J. D. (2007). Acumulación de Corg, Norg, Porg y BSi en la margen de Magdalena, BCS (México), durante los últimos 26 ka. Ciencias Mar. 33:2007. doi: 10.7773/cm.v33i1.1036
Schratzberger, M., and Ingels, J. (2018). Meiofauna matters: The roles of meiofauna in benthic ecosystems. J. Exp. Mar. Bio. Ecol. 502, 12–25. doi: 10.1016/j.jembe.2017.01.007
Schulz, H., Von Rad, U., and Von Stackelberg, U. (1996). Laminated sediments from the oxygen-minimum zone of the northeastern Arabian Sea. Geol. Soc. London Spec. Publ. 116, 185–207. doi: 10.1144/GSL.SP.1996.116.01.16
Sellanes, J., Neira, C., Quiroga, E., and Teixido, N. (2010). Diversity patterns along and across the Chilean margin: A continental slope encompassing oxygen gradients and methane seep benthic habitats. Mar. Ecol. 31, 111–124. doi: 10.1111/j.1439-0485.2009.00332.x
Shankar, D., Vinayachandran, P. N., and Unnikrishnan, A. S. (2002). The monsoon currents in the north Indian Ocean. Prog. Oceanogr. 52, 63–120. doi: 10.1016/S0079-6611(02)00024-1
Shannon, C. E., and Weaver, W. (1949). The Mathematical Theory of Communication. Univ. Illinois Press. doi: 10.1145/584091.584093
Singh, R., and Ingole, B. S. (2016). Structural and functional study of the nematode community from the Indian western continental margin with reference to habitat heterogeneity and oxygen minimum zone. Biogeosciences 12, 11537–11585. doi: 10.5194/bgd-12-11537-2015
Smith, C. R., Levin, L. A., Hoover, D. J., McMurtry, G., and Gage, J. D. (2000). Variations in bioturbation across the oxygen minimum zone in the northwest Arabian Sea. Deep Sea Res. Part II: Top. Stud. Oceanography. 47, 227–257. doi: 10.1016/S0967-0645(99)00108-3
Soltwedel, T. (1997). Meiobenthos distribution pattern in the tropical East Atlantic: Indication for fractionated sedimentation of organic matter to the sea floor? Mar. Biol. 129, 747–756. doi: 10.1007/s002270050217
Soltwedel, T. (2000). Metazoan meiobenthos along continental margins: A review. Prog. Oceanogr. 46, 59–84. doi: 10.1016/S0079-6611(00)00030-6
Stramma, L., Johnson, G. C., Sprintall, J., and Mohrholz, V. (2008). Expanding oxygen-minimum zones in the tropical oceans. Science 320, 655–658. doi: 10.1126/science.1153847
ter Braak, C. J. F., and Šmilauer, P. (2002). CANOCO Reference Manual and CanoDraw for Windows User's Guide: Software for Canonical Community Ordination (Version 4.5). New York, NY: Sect. Permut. Methods. Microcomput. Power.
Thiel, H. (1966). Quantitative untersuchungen über die meiofauna des tiefseebodens. Veröffentlichungen des Instituts für Meeresforsch. Bremerhaven 2, 131–148.
Thiel, H. (1978). “Benthos in upwelling regions,” in Upwelling Ecosystems, eds R. Boje and M. Tomczak (Berlin; Heidelberg: Springer).
Thiel, H. (1979). First quantitative data on red sea deep benthos. Mar. Ecol. Prog. Ser. 1, 347–350. doi: 10.3354/meps001347
Thurber, A. R., Kröger, K., Neira, C., Wiklund, H., and Levin, L. A. (2010). Stable isotope signatures and methane use by New Zealand cold seep benthos. Mar. Geol. 272, 260–269. doi: 10.1016/j.margeo.2009.06.001
Van Gaever, S., Raes, M., Pasotti, F., and Vanreusel, A. (2010). Spatial scale and habitat-dependent diversity patterns in nematode communities in three seepage related sites along the Norwegian Sea margin. Mar. Ecol. 31, 66–77. doi: 10.1111/j.1439-0485.2009.00314.x
Vanreusel, A., Fonseca, G., Danovaro, R., Da Silva, M. C., Esteves, A. M., Ferrero, T., et al. (2010). The contribution of deep-sea macrohabitat heterogeneity to global nematode diversity. Mar. Ecol. 31, 6–20. doi: 10.1111/j.1439-0485.2009.00352.x
Vaquer-Sunyer, R., and Duarte, C. M. (2008). Thresholds of hypoxia for marine biodiversity. Proc. Natl. Acad. Sci. U.S.A. 105, 15452–15457. doi: 10.1073/pnas.0803833105
Veit-Köhler, G., Gerdes, D., Quiroga, E., Hebbeln, D., and Sellanes, J. (2009). Metazoan meiofauna within the oxygen-minimum zone off Chile: results of the 2001-PUCK expedition. Deep. Res. Part II Top. Stud. Oceanogr. 56, 1105–1111. doi: 10.1016/j.dsr2.2008.09.013
Walkley, A., and Black, I. A. (1934). An examination of the degtjareff method for determining soil organic matter, and a proposed modification of the chromic acid titration method. Soil Sci. 37, 29–38. doi: 10.1097/00010694-193401000-00003
Weatherall, P. A., Sandwell, D. T., Arndt, J. E., Bringensparr, C., Dorschel, B., Ferrini, V., et al. (2019). The GEBCO_2019 Grid - a continuous terrain model of the global oceans and land. Publ. Data Libr. doi: 10.5285/836f016a-33be-6ddc-e053-6c86abc0788e
Wigley, R. L., and McIntyre, A. D. (1964). Some quantitative comparisons of offshore meiobenthos and macrobenthos south of Martha's Vineyard. Limnol. Oceanogr. 9, 485–493. doi: 10.4319/lo.1964.9.4.0485
Zeppilli, D., Leduc, D., Fontanier, C., Fontaneto, D., Fuchs, S., Gooday, A. J., et al. (2018). Characteristics of meiofauna in extreme marine ecosystems: a review. Mar. Biodivers. 48, 35–71. doi: 10.1007/s12526-017-0815-z
Keywords: meiofauna, rare taxa, benthic diversity, continental margin, oxygen minimum zone, Arabian Sea, Indian Ocean
Citation: Sautya S, Gaikwad S, Khokher S, Pradhan UK, Chatterjee S, Choudhury A, Sahu B and Attri S (2021) Distribution Pattern of the Benthic Meiofaunal Community Along the Depth Gradient of the Western Indian Continental Margin, Including the OMZ and Abyssal Plain. Front. Mar. Sci. 8:671444. doi: 10.3389/fmars.2021.671444
Received: 23 February 2021; Accepted: 18 May 2021;
Published: 28 June 2021.
Edited by:
Neloy Khare, Ministry of Earth Sciences, IndiaReviewed by:
Indranil Mukherjee, Academy of Sciences of the Czech Republic (ASCR), CzechiaM. Belal Hossain, Noakhali Science and Technology University, Bangladesh
Copyright © 2021 Sautya, Gaikwad, Khokher, Pradhan, Chatterjee, Choudhury, Sahu and Attri. This is an open-access article distributed under the terms of the Creative Commons Attribution License (CC BY). The use, distribution or reproduction in other forums is permitted, provided the original author(s) and the copyright owner(s) are credited and that the original publication in this journal is cited, in accordance with accepted academic practice. No use, distribution or reproduction is permitted which does not comply with these terms.
*Correspondence: Sabyasachi Sautya, c2F1dHlhJiN4MDAwNDA7bmlvLm9yZw==
†ORCID: Sabyasachi Sautya orcid.org/0000-0003-2229-5834
Santosh Gaikwad orcid.org/0000-0002-8624-9725
Sanofar Khokher orcid.org/0000-0002-3508-940X
Umesh Kumar Pradhan orcid.org/0000-0002-7732-9985
Amita Choudhury orcid.org/0000-0001-9331-3628