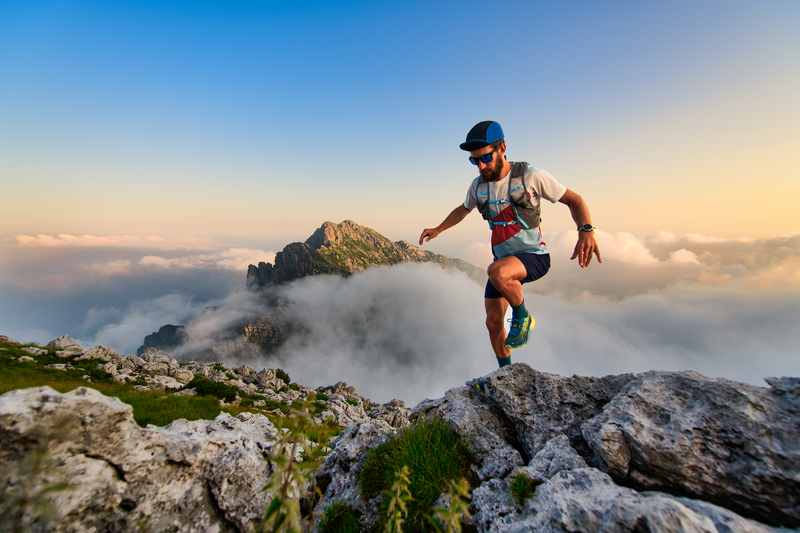
95% of researchers rate our articles as excellent or good
Learn more about the work of our research integrity team to safeguard the quality of each article we publish.
Find out more
ORIGINAL RESEARCH article
Front. Mar. Sci. , 11 May 2021
Sec. Coral Reef Research
Volume 8 - 2021 | https://doi.org/10.3389/fmars.2021.669976
This article is part of the Research Topic Stony Coral Tissue Loss Disease in the Caribbean View all 50 articles
Members of the family Meandrinidae are highly susceptible to stony coral tissue loss disease, resulting in population reductions up to 88% in both Dendrogyra cylindrus and Meandrina meandrites along the Florida Reef Tract. Reductions in abundance on this scale leave these species susceptible to limitations in sexual reproduction and natural recovery without intervention. In response to the ongoing outbreak of the disease across the Caribbean, a variety of genetic rescue projects have been implemented to bring disease susceptible species into ex situ culture and preserve living genetic diversity. In this study, corals being held in a long-term ex situ genetic bank were maintained using artificial lighting and temperature cues programmed to mimic natural cycles in Key Largo, FL, United States. Synchronized broadcast spawning events in both species were documented in aquaria over two annual spawning cycles in 2019 and 2020. Timing of gamete release relative to the perceived date and sunset was highly synchronized with wild observations. Up to 21 unique D. cylindrus genotypes collected from reef locations spanning over 230 km contributed gametes to the larval pool. The majority of these parental colonies are no longer alive in the wild. Repeatable and predictable ex situ spawning events such as these will become an essential tool for managed breeding and assisted fertilization in species suffering from severe population declines. These annual events have the potential to produce thousands of genetically diverse offspring for restoration efforts and offer future hope for the long-term survival of these threatened species.
Global and local anthropogenic impacts have led to severe declines in the abundance of coral species worldwide (Gardner et al., 2003; McLean et al., 2016; Flynn and Forrester, 2019; Hédouin et al., 2020). If stressors such as high water temperatures and increased acidification due to climate change are not improved, declines are predicted to continue (Hoegh-Guldberg et al., 2017). In Florida, United States, coral reefs have undergone a consistent loss of live coral cover over the past four decades (Palandro et al., 2008; Toth et al., 2019) that can be attributed to repeated thermal events (Manzello, 2015), land-based pollution (Staley et al., 2017), sedimentation (Jordan et al., 2010; Miller et al., 2016), and disease outbreaks (Williams and Miller, 2005; Walton et al., 2018). A recent devastating disease outbreak, termed stony coral tissue loss disease (SCTLD) originated in southeast Florida (Precht et al., 2016; Walton et al., 2018) and has spread to the Florida Keys and other areas in the Caribbean (Alvarez-Filip et al., 2019; Weil et al., 2019). SCTLD has affected at least 24 coral species and resulted in very high levels of mortality between initial onset in 2014 and the present (Precht et al., 2016; Walton et al., 2018; Estrada-Saldívar et al., 2020; Heres et al., 2021).
Stony coral tissue loss disease is characterized at the cellular level by a disorder in host-symbiont physiology with lytic necrosis originating in the gastrodermis of the basal body wall and extending into the calicodermis, causing tissue detachment, and sloughing from the skeleton (Landsberg et al., 2020). Emerging data suggest that SCTLD may be linked to certain bacterial taxa (Meyer et al., 2019; Rosales et al., 2020; Ushijima et al., 2020) and positive responses to antibiotic treatment in affected corals indicate that bacteria are involved in disease progression (Aeby et al., 2019; Miller et al., 2020; Neely et al., 2020). Visual signs of corals affected by SCTLD are most like those of the previously described white plague (Bythell et al., 2004), but the disease is distinguished by persistence through multiple years and seasons, the large number of affected species, and the specific order in which it affects species at a given location (SCTLD Case Definition, 2018).
The family Meandrinidae consists of four extant coral genera limited to the Atlantic Ocean and Caribbean. Corals in this family are considered highly susceptible to SCTLD and “are the first to become affected at a site” according to the case definition for the disease (SCTLD Case Definition, 2018) and studies on the spatial progression of the disease (Sharp and Maxwell, 2018). Maze coral Meandrina meandrites and Atlantic pillar coral Dendrogyra cylindrus are both particularly susceptible (Aeby et al., 2019; Miller et al., 2020) and populations of these species have been severely affected (Estrada-Saldívar et al., 2020; Neely and Lewis, 2020). Indeed, D. cylindrus, a naturally rare species on Caribbean reefs prior to the SCTLD outbreak, may already be functionally extinct in Florida (Neely and Lewis, 2020) and demographic projections suggest a high likelihood that the species will become extinct throughout the northern Caribbean in the next few decades (Chan et al., 2019). Multi-year monitoring efforts in Florida have documented a decline in abundance of 88% for M. meandrites, along with a shift to smaller size classes in extant colonies (Walton et al., 2018; Hayes, 2019).
In the face of ongoing threats from climate change, and in direct response to the precipitous declines observed following the SCTLD outbreak, multi-agency, and -institution partnerships in Florida have executed coral “rescue” projects and placed extracted colonies into ex situ holding facilities. The immediate objective of these efforts was to protect susceptible species from exposure to the disease, and in the case of D. cylindrus, to treat and stabilize actively infected individuals (Miller et al., 2020). Coral rescue goals were to preserve genetic diversity in the Florida population before it was lost to SCTLD and to establish land-based gene banks for future repopulation efforts through translocation of fragments or sexually produced offspring from ex situ facilities to in situ coral nurseries or directly onto reefs. Sharp et al. (2020) evaluated the epidemiology of SCTLD as it passed through the middle Florida Keys, identified the existence of intraspecific variability in disease resistance, and suggested that more resilient coral colonies or communities may merit inclusion in future rescue and propagation programs. Thus, future coral rescue efforts from SCTLD endemic areas may continue to occur.
Until recently, broadcast spawning of scleractinian corals in aquaria was a rare and relatively unpredictable occurrence. However, advances in long-distance coral transport (Craggs et al., 2018), aquarium lighting technology, and computer control systems now allow precise replication of the environmental cues necessary to induce gametogenesis and synchronized broadcast spawning in aquaria (Craggs et al., 2017). With this technology, land-based assisted reproduction between colonies previously separated by prohibitively large distances is now possible. Further, Craggs et al. (2020) recently reported the first completed life cycle (i.e., production of an F2 generation) for a broadcast spawning coral in a fully controlled system. Beyond spawning, recent advances in rearing techniques for early post-settlement coral recruits have improved sexually propagated coral survival and growth in land-based systems (Craggs et al., 2019; Henry et al., 2019). Altogether, these advances have made sexual coral propagation in land-based systems a viable strategy for augmenting the genetic diversity that is currently available to restoration programs.
Sexual reproduction is the primary mechanism of evolutionary adaptation in corals and genetic diversity is a critical consideration for interventions aimed at increasing coral population resilience (Baums, 2008). For sessile marine species, a drastic reduction in abundance, such as that caused by SCTLD in D. cylindrus and M. meandrites, may result in limited natural sexual reproductive capacity due to large distances remaining between suitable parents and allee effects (Gascoigne and Lipcius, 2004). Falling below a threshold population density can result in extirpation or extinction of coral reef organisms even when stressors are removed, or conditions improve (Knowlton, 1992). While minimum distances between parents and density thresholds in corals have not been studied in situ due to numerous difficulties, modeling suggests that fertilization is generally limited beyond 30–40 m of separation in broadcast spawning species (Teo and Todd, 2018) due to sperm dilution effects. In addition, reductions in spawning synchrony attributed to environmental change have been observed in both the Red Sea (Shlesinger and Loya, 2019) and the Caribbean (Fogarty and Marhaver, 2019), further decreasing the chance of natural evolutionary adaptation in severely impacted coral populations.
This study aimed to address these challenges and build upon novel technologies by inducing gametogenesis and synchronized broadcast spawning in two coral species in the family Meandrinidae, whose populations in Florida and elsewhere have been severely impacted by SCTLD. This work represents the first successful induced gametogenesis and spawning of Atlantic coral species in land-based systems. Corals used were removed from the ocean as part of SCTLD rescue work and thus this research represents an opportunistic augmentation of genetic preservation efforts rather than an extractive exercise conducted solely for the purposes of experimentation. Repeated and predictable sexual reproduction in coral colonies held long-term ex situ under fully controlled conditions represents the first step toward a managed breeding program for threatened species that will be able to produce genetically diverse offspring for future population restoration under improved environmental conditions.
Fragments of D. cylindrus were collected from the Florida Reef Tract in separate efforts between May 2016 and December 2018 in response to the rapid decline of the local population (Neely and Lewis, 2020). Fragments arrived at The Florida Aquarium Center for Conservation (Apollo Beach, FL, United States) between August 2016 and December 2018 and were quarantined, treated with antibiotics when signs of SCTLD were observed (O’Neil et al., 2018), and stabilized in fully recirculating aquaria located in greenhouses receiving natural sunlight. The majority of the fragments were genotyped as described in Chan et al. (2019) at the time of collection, however, if no genotype data was available, each collection site was presumed to represent a single genet.
In March 2019, 41 individual D. cylindrus fragments representing 24 unique genotypes were moved into two identical induced spawning aquaria (n = 24 in Tank A and n = 17 in Tank B) before the onset of gametogenesis, which is reported to begin in May (Szmant, 1986). In February 2020, 19 individual D. cylindrus were removed from the two spawning aquaria and 14 new individuals were added in order to increase the number of unique genotypes represented and as part of a routine redistribution of corals between holding facilities, for a total of 36 corals representing 28 genets in the spawning systems for 2020 (n = 15 in Tank A and n = 21 in Tank B).
Colonies of M. meandrites were collected by the Florida Fish and Wildlife Conservation Commission from the lower Florida Keys in April 2019, prior to the onset of gametogenesis, which begins in June (Pinzón and Weil, 2011). Colonies were transported to The Florida Aquarium on 26 April 2019 and placed directly into the spawning aquarium (n = 13 colonies). Corals were collected prior to any visual observation of SCTLD in the area; however, one colony was treated with topical amoxicillin due to tissue recession along the colony margin and possible SCTLD in July 2019. For 2020, only 9 colonies of M. meandrites were held in the spawning aquarium.
All coral colonies retained a unique identifier tag from collection onward. To determine if there was a minimum reproductive size of coral fragments and to provide information on recommended size for ex situ spawning, the length, width, and height of each D. cylindrus colony in the spawning aquariums was measured in October 2020 using a large ruler and recorded to the nearest 0.5 cm. For M. meandrites, measurements were recorded every 6 months to fulfill permit monitoring requirements.
Induced spawning aquarium design was based on mesocosms described in Craggs et al. (2017), with modifications described here. Each aquarium life support system consisted of an 895 liter (238 cm L × 71 cm W × 53 cm D) main holding aquarium where corals were housed connected to a 345 liter (213 cm L × 58 cm W × 28 cm D) sump. Water flow from the sump back to the holding aquarium was provided by a main recirculation pump (EcoTech Marine Vectra L1) at approximately 10,340 liters per hour, for an operational total system water volume of 1,250 liters. Each holding aquarium also had four additional wave generating pumps (two Maxspect Gyre XF350 and two Reef Octopus Pulse 2) for turbulent water circulation. Water from the holding aquarium overflowed to the sump through two 5 cm diameter standpipes and was filtered through two 150 micron filter socks to remove particulate material.
Each sump contained an area of aquacultured live rock sourced from the Florida Keys and a small fine-grained sand bed to create anaerobic areas for denitrification of nitrate into nitrogen gas. This portion of the sump was illuminated by 96 watts of T5 high output fluorescent lighting (“Wave Point 24” 4-bulb fixture, 2x Sun Wave, and 2x Super Blue 460 lamps) on a 12 h on/off cycle. The non-lighted portion of the sump contained an internal protein skimmer (Reef Octopus Regal 300-INT), a single media reactor (Two Little Fishes PhosBan Reactor 550) with granular activated carbon and granular ferric oxide phosphate remover, and three 500-watt titanium heating elements (BlueLine; two main heaters and one back-up). Water returning to the main aquarium passed through an inline chiller (TECO TK-3000 1/2HP). Activated carbon (800 g) in the media reactor was replaced every 14–21 days and phosphate remover (50–150 g granular ferric oxide) was added as needed to control phosphate levels.
Each aquarium was filled with artificial seawater (Tropic Marin Pro Reef) that was mixed using reverse osmosis filtered and deionized freshwater to a salinity of 35–36 ppt. Salinity was maintained by replacing evaporated water to a marked fill line using additional reverse osmosis deionized water. Water exchanges were performed approximately once a week by removing 20 percent of the total system water, while siphoning detritus and excess algae, and replacing with clean artificial seawater. Major water quality parameters important for the maintenance of coral aquaria were tested weekly (Supplementary Tables 1, 2), including pH and salinity (Hach HQ40D), total alkalinity (LaMotte 4491-DR-01), calcium (Salifert), and orthophosphate (Hach Method 8048). In addition, water samples from each aquarium were sent to a commercial aquarium water quality testing facility (Triton GmbH, Dusseldorf, Germany) for analysis of ionic constituents via inductively coupled plasma-atomic emission spectroscopy testing (ICP-OES) as well as total organic carbon, total nitrogen, and total phosphorous (Supplementary Tables 3, 4).
A variety of supplements were used to maintain seawater components commonly depleted over time in recirculating aquaria. Total alkalinity was maintained through daily manual addition of food-grade sodium bicarbonate. Calcium concentration was maintained through twice weekly additions of granular calcium chloride. Iodide was supplemented using twice weekly additions of Lugol’s solution. Unlike Craggs et al. (2017), no other trace elements were dosed to the aquariums.
Solar, lunar, and temperature cycling was provided by a commercially available microprocessor for aquarium control and monitoring (Neptune Systems Apex Classic). In each spawning aquarium, lighting consisted of eight Radion XR30w G4 Pro lights (EcoTech Marine) connected to the Apex system by a WXM Module (Neptune Systems). Lunar light intensity over the course of each lunar cycle was controlled by the LSM module (Neptune Systems) connected to the Apex, and individual LEDs in the string of lights supplied with the LSM module were replaced with 3.2v 5 mm cool white 5000K bulbs (CREE C513A-WSN-CY0Z0231) to mimic the color temperature of natural moonlight (Sweeney et al., 2011). Moonlights were covered with a spherical diffuser, and the position of the lights over the aquarium was adjusted so that photosynthetically active radiation (PAR) values measured with a LiCor LI-192 underwater quantum sensor on full moon nights were <1 μmol m–2 s–1 in all areas of the aquarium.
Data entered into the “Season Table” of the Apex were programmed to mimic annual fluctuations at Key Largo, Florida, United States (Supplementary Figure 1). Sunrise, sunset, moonrise, moonset, and date of new moon values were acquired from www.timeanddate.com. In the event of two new moons in 1 month, the day changed to the second new moon in the table immediately after the first new moon event occurred. Water temperature for the first day of each month was calculated from the mean of 9 years of sea temperature data acquired from the NOAA National Data Buoy Center Station MLRF1 (Molasses Reef, FL, United States; not all years have complete data due to buoy malfunction). In order to prevent seasonal bleaching stress, the maximum summer temperature in the aquarium was limited to 29.5 C. Daily temperature set points are calculated within the Apex based on temperature values entered for the first day of each month to create a smooth curve over the course of the year, and water temperature in the aquarium was controlled plugging heater and chiller elements into outlets on the EB8 “Energy Bar” for on/off control. Temperature in the aquarium varied by ±0.6°C (programmed as 1°F) from the setpoint.
The “Season Table” of the Apex does not account for annual fluctuations in solar irradiance, only changes in photoperiod due to the shifting time of sunrise and sunset. Changes in theoretical incident irradiance at the ocean surface over the course of the year were estimated from www.clearskycalculator.com, using latitude and longitude values for Molasses Reef, Florida and the time of solar noon to gather the theoretical maximum photosynthetic photon flux density on the first day of each month. An underwater irradiance PAR target of 400 μmol m–2 s–1 at the corals was chosen for the summer maximum, which has been reported at 10 m depth on reefs in Florida at summer mid-day on a clear day (Lesser et al., 2000), and equated to approximately 20% of theoretical incident surface irradiance in the summer months. An LED output of 70% produced an underwater PAR irradiance of 415 μmol m–2 s–1 at the corals (LiCOR LI-192), so 70% output was used as the summer maximum. The maximum daily intensity of the LED lighting for the remainder of the year was adjusted based on values shown in Supplementary Table 5, and values were manually changed in the Apex program approximately once a month.
Each day, the LED lighting was programmed to have a 3 h “ramp-up” time where intensity increased from 0% to the maximum daily intensity. Lighting then stayed at maximum intensity until 3 h prior to sunset, when a “ramp-down” cycle began, reducing intensity from the maximum back to 0% at the programmed sunset time (Supplementary Figure 2). Each color channel of LED bulbs was customized with a varying level of intensity (Supplementary Figure 2).
Light pollution has been shown to disrupt the timing of gamete release (Boch et al., 2011; Kaniewska et al., 2015). Therefore, in order to phase shift and control the time at which our corals spawned, the mesocosm’s were constructed in a light controlled room, to remove any external light influences other than our daylight and lunar LED’s. The time setting in the Apex controller was manually overridden to shift the local time ahead by 6 h and 30 min, with resulting perceived sunset for the corals occurring at approximately 12:30 pm local time during the month of August. This promotes the ability to monitor corals over many sequential days, as corals can be monitored periodically during normal work hours and negates the need for long nights of work during spawning periods.
Due to the field observed spawn time of M. meandrites occurring shortly after sunset, but during the period of civil and nautical twilight, the lighting on the aquarium holding M. meandrites was further modified to include a 60 min sunset phase prior to sunset and 60 min of twilight post sunset. The sunset phase included a stronger royal blue LED intensity and decreased red intensity. This was followed by two twilight phases occurring from 0 to 30 min after sunset and from 30 to 60 min after sunset. During the twilight phases, overall fixture intensity remained at 1% but the intensity of the individual LED bulbs was reduced (Supplementary Figure 3).
For the 2020 spawning season, one D. cylindrus spawning aquarium (Tank A) was programmed with a sunset and twilight phase identical to the program used for M. meandrites in 2019 (Supplementary Figures 3, 4). The second D. cylindrus spawning aquarium (Tank B) was altered to remove the month of March, essentially shifting all programming data up by 1 month (i.e., the April program was entered for March and similarly for the remainder of 2020; Supplementary Figure 5). Although each of these systems only represents a single replicate, these changes in programming were the first steps to understanding how minor modifications may or may not affect ex situ spawning events.
Each system with brood stock corals received a twice-weekly feed predominantly with a mixture of frozen foods including spirulina enriched brine shrimp, mysis shrimp, copepods and cyclops (Tropical Marine Center Gamma) as well as intermittently offered Golden Pearl Diet 800–1000 micron (Brine Shrimp Direct), Reef-Roids (Polyp Lab), PE Pellets (Piscine Energetics), and R.O.E. (Reef Nutrition). During feeding, recirculation between the coral holding aquarium and the sump was turned off to allow the corals to capture food and two wave generation pumps remained on during feeding to keep food suspended in the aquarium. Food was target fed to each coral, and excess was allowed to circulate in the aquarium for approximately 30 min before filtration was reinitiated. Additional supplemental nitrogen was supplied through the addition of ammonium chloride dosed to 0.02 mg/l total NH3 twice weekly and concentrated amino acids (Polyp Lab Colors) were dosed once weekly (0.25 mL/375 L).
For D. cylindrus, water circulation in the aquariums was turned off after the programmed sunset time and coral colonies were observed with red light beginning on the second night after the programmed full moon in 2019 (15 August 2019). Due to spawning occurring on the second night after the August full moon in 2019, observations in 2020 began on the night of the full moon in July, August and September 2020 and continued until two consecutive days of no spawning had occurred (after an observed spawn) or one full week after the full moon had passed if no spawning was observed. Gamete release time and colony ID was recorded for each colony observed to spawn. As spawning progressed, it became increasingly difficult to determine which individual released gametes as the aquarium became cloudy with sperm and filled with eggs, so data on colony ID was only recorded if the observer had full confidence in the origin of the gametes.
The lack of a clearly defined spawning window from limited field observations resulted in extensive monitoring for spawning in M. meandrites. The spawning tank was checked daily between programmed sunset and 60 min after sunset beginning on day 10 after the full moon of August, September, and October. If no spawn had occurred by day 20 after the full moon, observations were stopped until the following month. Starting on day 14 after the full moon of each month, water pumps and filtration were turned off at sunset and the tank was more intensively monitored for the presence of gametes. In 2020, due to the early spawning times observed in 2019, the tank was turned off 30 min prior to sunset on day 14 after the full moon of September.
In situ spawning observations for the species were compiled from published literature and raw data on D. cylindrus spawning in Florida was provided by Dr. Karen Neely (Nova Southeastern University). Spawning observations for M. meandrites were gathered from the Coral Spawning Research Facebook group and personal communication with Dr. Kristen Marhaver (Caribbean Research and Management of Biodiversity, CARMABI), Dr. Valerie Chamberland (SECORE International), and Dr. Mark Vermeij (CARMABI).
All statistical analyses were performed using R statistical software version 3.6.0 (R Core Team, 2019). Tests were conducted at a significance level of α = 0.05. Means are presented as mean ± SEM. Data were assessed for normality visually with density and q-q plots as well as quantitatively using the Shapiro–Wilk test. When data were normally distributed, Bartlett’s test was used to assess homogeneity of variance. Due to irregular colony morphology, maximum linear dimension was used as the most representative measurement of D. cylindrus colony size. These data were positively skewed and thus analyzed with spawn occurrence as the binomial predictor variable using a Mann–Whitney U test. Spawn timing was quantified as minutes after sunset at first observation of gamete release and compared between in-situ D. cylindrus colonies and those held ex situ using an unpaired t-test for 2019 observations and a Mann–Whitney U test when comparing in situ spawning observations from 2014–2019 to both 2019 and 2020 ex situ data. Aggregate ex situ minutes after sunset spawning data were also positively skewed and thus analyzed among tank/years with a Kruskal–Wallis test and Wilcoxon-Mann–Whitney post hoc with correction for multiple testing.
Gamete release was observed on 17 August through 19 August 2019 in both spawning aquaria, 2–4 nights after the full moon (NAFM; Figures 1, 2). No spawning was observed on 20 or 21 August 2019 (5–6 NAFM). In Tank A, a total of 12 out of 24 colonies spawned over the three days, 6 releasing sperm only and 6 releasing eggs only. In Tank B, a total of 11 out of 17 colonies spawned over the 3 days, 5 releasing sperm only and 6 releasing eggs only. The highest proportion of colonies spawned on 18 August 2019 (3 NAFM) in both spawning tanks, with 41.7% of colonies spawning in Tank A and 52.9% of colonies spawning in Tank B. Although observations were not conducted in September 2019, cloudy tank water after the full moon of September 2019 indicated that spawning had likely also occurred at this time, therefore observations in 2020 were extended to include September.
Figure 1. Daily spawning observations of D. cylindrus colonies in separate controlled systems (Tank A and Tank B) during 2019 (top) and 2020 (bottom). Annual photoperiod, irradiance, lunar, and temperature cycles in the fully enclosed systems were programmed to match those in the Florida Keys and these programmed dates are noted in the top row of x-axis labels, while actual calendar date of spawning observation is noted in the bottom row of x-axis labels. Programmed and actual calendar dates align except for Tank B in 2020, when the month of March was removed from the annual cycle.
Figure 2. Female D. cylindrus colony releasing eggs during the August 2019 ex situ induced spawning event.
Male colonies began releasing sperm between 94 and 122 min after sunset and female colonies began to release eggs between 109 and 139 min after sunset (Figure 3). Gamete release almost always occurred in numerous pulses after the initial recorded release time. Both aquariums, previously isolated from the sump with water circulation shut off, became progressively cloudy due to sperm release and the measured sperm concentration in the water column after spawning was 1 × 105 cells per ml.
Figure 3. Daily spawn timing in D. cylindrus colonies held in controlled systems. Each point represents a single colony. (A) colonies held in Tank A and Tank B during 2019 under the “normal” day/night program. Dashed line represents a period in which 7 additional colonies (6 female and 1 male) spawned without exact times recorded, while adjacent points correspond to the first and last colonies to spawn. (B) colonies held in Tank A during 2020 with an added period of twilight between day and night. (C) colonies held in Tank B during 2020 with the month of March removed from the programmed annual cycle.
A comparison with field observations of D. cylindrus by divers on nights 2 and 3 after the full moon of August 2019 showed that spawning occurred in situ on the same nights after the full moon as it did ex situ (Table 1). However, ex situ corals spawned significantly later after sunset than those in the field (t35 = –5.95, p < 0.001), with ex situ males spawning ∼17 min later on average (89 ± 2 MAS in situ, 106 ± 2 MAS ex situ) and females spawning ∼34 min later on average (90 ± 6 MAS in situ, 124 ± 4 MAS ex situ). This difference persisted when comparing all in situ field observations from 2014 through 2019 (W = 170.5, p < 0.001, in situ male release time of 89 ± 2 MAS and female release time of 95 ± 2 MAS).
Table 1. Previously reported in situ spawning observations for D. cylindrus compared with those observed in controlled systems over 2 years in the present study.
In Tank A (n = 15 colonies), where the lighting program was altered to include a twilight period but retained a normal 2020 solar, lunar, and temperature cycle, only 2 out of 15 colonies (13.3%) spawned in August 2020 (Figure 1). One female colony spawned on 6 August 2020 and another female spawned on 7 August 2020 (3–4 NAFM). A higher proportion of colonies spawned in September 2020, with 8 out of 15 colonies (53.3%) spawning in Tank A over 3 days from 4 through 6 September 2020 (2–4 NAFM).
In Tank B (n = 21 colonies), where the month of March was removed from the entire program, therefore shifting all following months forward by 1 month, spawning was anticipated to possibly occur in July 2020 as this corresponded to the programmed August 2020. However, no spawning was observed in July 2020 (perceived August). Spawning did occur in actual August and September of 2020, which were the months of September and October as perceived by the corals/programmed in the microprocessor (Figure 1). In August 2020 (perceived September 2020), 12 out of 21 corals spawned (57.1%, 8 females, 3 males, and 1 hermaphrodite). In September 2020 (perceived October 2020), 12 out of 21 corals spawned (57.1% 7 females and 5 males). Some individual colonies spawned in only 1 month and some spawned in both. Between the 2 months, 71.4% of all corals spawned. One individual spawned eggs in August and sperm in September, whereas one individual spawned eggs on 4 August 2020 and then sperm on 5 August 2020, then spawned as a male again on 5 and 6 September.
Initial release of sperm occurred from 104 to 122 min after sunset for males in Tank A (with twilight) and from 92 to 122 min after sunset for males in Tank B (shifted 1 month; Figure 3). Egg release began from 127 to 178 min after sunset in Tank A and 98 to 119 min after sunset in Tank B. Mean minutes after sunset of initial gamete release was different among D. cylindrus colonies from 2019, 2020 Tank A, and 2020 Tank B (Kruskal–Wallis X2 = 18.18, d.f. = 2, and p < 0.001) with 2020 Tank A releasing significantly later than the other two groups.
The maximum dimension of the D. cylindrus fragments in 2020 ranged from 10 to 41 cm, and this measurement generally equated to either the maximum diameter of the base for flat fragments or the height of the pillar on vertical fragments. The fragments in the spawning aquariums had an overall mean maximum dimension of 18.8 ± 1.3 cm. There was no significant difference in mean maximum dimension between the 23 colonies that spawned and the 13 colonies that did not (18.9 ± 1.8 cm and 18.5 ± 1.8 cm, respectively; W = 159.5, p = 0.754). The smallest coral observed spawning had a maximum dimension of 10 cm (10.0 cm L × 9.5 cm W × 3 cm H). This individual was introduced into spawning systems in March 2020 and was observed spawning in September 2020. This fragment was collected from a diseased colony and was housed at two other facilities before being placed into the spawning system. The second smallest reproductive coral measured a maximum dimension of 10.5 cm (10.5 cm L × 9.0 cm W × 8.5 cm H) and spawned successively in 2019 and 2020. A full measurement of all D. cylindrus in the spawning aquariums was not conducted in 2019.
No in situ spawning observations were available for 2020 from Florida waters, as SCTLD had severely affected colonies within close enough distance to the shore for monitoring. When compared to combined in situ gamete release times from 2014 through 2019, mean ex situ gamete release was also later in 2020 (W = 392.5, p < 0.001).
Gamete release was observed in M. meandrites on 29 September through 3 October 2019 (15–19 NAFM; Figures 4, 5). No gamete release was observed after the August or October full moons. A total of 5 out of 13 colonies spawned over the 5 days (2 female, 3 male). The highest proportion of colonies spawned on 1 October 2019 (17 NAFM) with 23.1% of colonies spawning. Egg release was overall very light and at times it was difficult to distinguish from which colony eggs originated. Similar to D. cylindrus, sperm release occurred in multiple pulses separated by several minutes.
Figure 4. Daily spawning observations of M. meandrites colonies in controlled systems during 2019 (top) and 2020 (bottom). Annual photoperiod, irradiance, lunar, and temperature cycles in the fully enclosed systems were programmed to match those in the Florida Keys. Programmed and actual dates align and are noted on the x-axes.
Figure 5. Male M. meandrites colony releasing sperm during the September 2019 ex situ induced spawning event.
In 2020, spawning also occurred on day 15 after the full moon of September; however, it proceeded for 8 days (Figure 4). All 9 colonies that remained in the tank for 2020 were observed spawning in this 8 day period, with 6 colonies releasing sperm and 3 colonies releasing eggs. The volume of gametes produced was generally observed to be much higher than in 2019, with eggs being spawned in obvious pulses versus the slow trickle observed the year prior. Two colonies observed releasing eggs in 2019 released only sperm in 2020, and all male colonies from 2019 remained male in 2020. All three female colonies that spawned in 2020 were not previously observed spawning in 2019.
On the first day that spawning was observed in 2019, eggs were found in the water column at 30 min after sunset but the first release was not observed. The tank had been free of eggs when the tank was checked at 10 min after sunset, so spawning is presumed to have occurred between 10 and 30 min after sunset. No sperm release was observed on this day, but eggs began to cleave and had high fertilization so a minimum of one male is presumed to have spawned also on this day. Over the 5 days of spawning in 2019, sperm release was observed between 19 and 38 min after sunset and egg release was observed between 29 and 64 min after sunset (Figure 6).
Figure 6. Daily spawn timing in M. meandrites colonies held a controlled system in 2019 (left) and 2020 (right). Each point represents a single colony. Dashed lines represent periods in which spawning occured without exact times recorded, yet the event is known to have been within the timeframes depicted. In both cases, at least one male and one female colony spawned.
In 2020, observations were started earlier (30 min before sunset) to capture initial gamete release. Males released sperm from 22 min before sunset to 31 min after sunset and egg release occurred from 27 to 36 min after sunset (Figure 6).
Meandrina meandrites colonies in 2019 ranged in size from 15.5 to 29.0 cm in diameter, and both the smallest colony and largest colony were observed releasing gametes. Colonies in 2020 ranged in size from 17.0 to 30.0 cm in diameter, with a mean maximum diameter of 22.1 ± 4.5 cm. In aggregate all colonies were observed releasing gametes over the 8 spawning days in 2020. The smallest colonies observed spawning in 2020 were 17.0 cm in diameter.
No in situ observations of M. meandrites gamete release could be found in published literature, and observations gathered by the authors are presented in Table 2. In situ spawning has only been recorded in Bonaire and Curacao, and observations occurred from September through December. The majority of observations (with one exception) occur between night 11 and 20 after the full moon, which corresponds with observations in this study. Gamete release before sunset has not been observed in situ as it was in this study, and this may be due to a shift to earlier spawning times ex situ or a lack of in situ observations conducted before sunset.
Table 2. Previously recorded in situ spawning observations for M. meandrites compared with those observed in controlled systems over 2 years in the present study.
This study describes the first fully induced ex situ broadcast spawning of two Atlantic coral species originating from severely impacted wild populations (Estrada-Saldívar et al., 2020; Neely and Lewis, 2020) where natural reproductive success is limited at best. It is possible that the effects of SCTLD may lead to reconsideration of the conservation status for both D. cylindrus [last assessed in 2008 as “Vulnerable” with a stable population trend (Aronson et al., 2008b)] and M. meandrites [last assessed in 2008 as “Least Concern” with a stable population trend (Aronson et al., 2008a)]. In the case of D. cylindrus, natural recovery is highly unlikely. Corals in this study were “rescued” from the ongoing spread of SCTLD, and faced losses of up to 88% of colonies in a single disease outbreak if colonies remained on the reef (Neely and Lewis, 2020). Of the 24 D. cylindrus genets that spawned in controlled systems over 2 years of observation, 20 are no longer alive in the wild and the remaining 4 are actively infected with SCTLD or consist of only small remnant pieces of tissue (Neely pers. comm.). By maintaining threatened genets ex situ, the local diversity of the species is protected, and through the use of techniques outlined in this study, those individuals remain viable to genetically contribute to future generations.
In the wake of SCTLD, the distance between viable reproductive coral colonies on the reef has been greatly increased (Muller et al., 2020), limiting the capacity for natural recovery through sexual recruitment. The original collection location of D. cylindrus fragments that spawned in this study spanned over 230 km of reef, so few of these corals would have had the potential to cross fertilize prior to collection as part of the rescue project. This demonstrates the potential use of this tool for interventions such as assisted gene flow and selective breeding, as valuable broodstock from diverse geographic areas can be maintained in a single location to promote cross-fertilization. Interventions such as these are becoming increasingly common, and necessary, as coral populations around the globe continue to decline (Anthony et al., 2017, 2020; van Oppen et al., 2017).
The ability to predictably spawn corals ex situ not only enables reliable production of offspring from diverse parental stocks, it also facilitates increased understanding of the cues needed to trigger gametogenesis and spawning. Additionally, this practice provides basic life history data for corals that are increasingly exposed to in situ environmental stressors impacting spawning synchrony (Levitan et al., 2014), fecundity (Paxton et al., 2016), and sperm motility (Hagedorn et al., 2016). Although the timing of gamete release in this study was significantly different than wild colonies in Florida, this difference was relatively small (∼17–34 min later on average) and was less than in other studies of ex situ spawning. Neely et al. (2020) showed that D. cylindrus exposed to natural light in ex situ holding spawned ∼50 min later than in situ colonies and gamete release occurred over an extended number of nights after the full moon. Spawning times were also shifted in Craggs et al. (2017), where corals held in mesocosms similar to the current study spawned over an extended number of months and a delayed number of nights after the full moon relative to wild colonies.
Jordan (2018), in a compilation of Caribbean wide spawning observations, identified the peak time of D. cylindrus male gamete release to occur from 93 to 119 min after sunset and the peak time of female gamete release to occur from 102 to 134 min after sunset. With the exception of Tank A in 2020, where twilight was added to the LED lighting program, gamete release times in the current study coincide with published in situ spawning times in other areas of the Caribbean in regards to both the days after full moon and minutes after sunset (Marhaver et al., 2015; Jordan, 2018). Although there was only one replicate tank for the twilight program and we acknowledge that other factors may have been involved, it is worth noting that the spawning in Tank A in 2020, where twilight was added, is the only D. cylindrus spawning event in the present study that occurred outside of the range of in situ spawning times identified by Jordan (2018).
The timing of broadcast spawning in corals is driven by a wide variety of factors and the primary environmental predictor varies among studies. Annual cycles of insolation, which are correlated with seasonal changes in solar irradiance and photoperiod, are strongly related to coral spawning times in both the tropical Pacific (Penland et al., 2004) and tropical western Atlantic (van Woesik et al., 2006). However a rapid increase in sea surface temperature was the dominant cue in other studies (Harrison et al., 1984; Keith et al., 2016). These factors primarily drive the season and month of spawning, whereas the timing of gamete release in relation to lunar and diel cycles is finely tuned by factors such as lunar irradiance (Jokiel et al., 1985; Brady et al., 2016; Oldach et al., 2017), diel photoperiod (Knowlton et al., 1997; Brady et al., 2009), lunar light spectra (Sweeney et al., 2011), the period of darkness after twilight ends (Boch et al., 2011) and local environmental conditions (Sakai et al., 2020). In addition, there is evidence for a genetic component to the timing of gamete release (Levitan et al., 2011).
Although D. cylindrus spawns primarily as a gonochore, with individual colonies producing only eggs or sperm, instances of hermaphroditism do occur (Kabay, 2016; Neely et al., 2018), The observations in this study confirmed this finding, with 4 colonies releasing both eggs and sperm in 2020 (changing from one sex in August to the opposite in September in 3 out of 4 cases, and changing sex on subsequent nights in one instance), and three colonies changing sex between 2019 and 2020. We also observed three colonies of M. meandrites that released sperm in 2019 to release eggs in 2020, representing the first observation of hermaphroditism in this species.
While reproduction in D. cylindrus has been relatively well-studied, there is much less published information on M. meandrites spawning available. This study provides some of the first published information on timing of gamete release for M. meandrites. Gamete release in this study occurred within a consistent timeframe each year, beginning 15 nights after the full moon of September in both years and ranging from 22 min before to 64 min after sunset. The timing of gamete release is consistent with the wild observations gathered by the authors, though spawning ex situ occurred only after the September full moon and not in later months as observed in the southern Caribbean. While inherent differences clearly exist between the in situ and ex situ environment, the close relationship with natural timing in terms of days after full moon and minutes after sunset described here for D. cylindrus may translate to M. meandrites. Thus, we suggest that the ex situ spawning data from controlled systems in this study is an excellent model to inform in situ monitoring and gamete collection efforts for M. meandrites in Florida, where we are unaware of previous spawning observations.
To date, coral restoration activities using sexually-propagated corals have relied on in situ spawning events (Omori, 2005; Omori and Iwao, 2014), transporting corals to ex situ aquaria shortly before spawning (Pollock et al., 2017) or using cryopreserved sperm to cross the barrier of time and distance (Hagedorn et al., 2017). Induced ex situ spawning is a viable tool to produce larvae and sexual recruits on an annual basis to add to existing pipelines for reef restoration. For highly depauperate and at-risk sub-populations such as D. cylindrus in Florida, this may be the only tool currently available to preserve existing genetic diversity and ensure the availability of sexually-derived offspring for future restoration efforts if environmental conditions improve.
Larvae produced ex situ can be used to support reef restoration efforts with varying levels of post-spawn rearing. Coral larvae are easily transported across large distances in high numbers (Petersen et al., 2005), meaning that larvae produced under fully controlled conditions anywhere in the world can be used for direct re-seeding activities (Heyward et al., 2002; dela Cruz and Harrison, 2020). Alternatively, sexually propagated coral recruits and juvenile colonies can be reared in human care for periods of time ranging from a few weeks (Guest et al., 2014; Chamberland et al., 2015), to months (Henry et al., 2019), to a year or more (Craggs et al., 2020).
A major question regarding ex situ coral propagation is the feasibility of operating on scales relevant to enhancement of wild populations. Although the total number of eggs released was not quantified, over 50,000 viable D. cylindrus larvae were produced from only a fraction of the spawn that was collected in 2020. This suggests that by maintaining relatively small coral fragments or colonies (10–30 cm) of diverse genetic origin, it is possible to produce tens or hundreds of thousands of larvae on an annual basis. In addition, this can be achieved in a relatively small footprint, as the two spawning aquaria for D. cylindrus occupy approximately 2.3 m2 of space each and are both located within a room of 16 m2 in size. Achieving high rates of larval settlement and post-settlement survival will be important to the viability of this practice.
This study made numerous modifications to the methods of Craggs et al. (2017), most notably the lack of trace element dosing, less heterotrophic feeding, and a modified means of replicating annual solar irradiance cycles. However, spawning occurred in all aquaria with only relatively minor variations in gamete release time. This indicates that the general method of inducing gametogenesis and spawning is tractable under minor modifications and that these techniques can be successful when all methods and procedures are not replicated with precision. The method was originally developed to induce spawning in Indo-Pacific coral species, but has here proven viable for western Atlantic species when programmed with appropriate local data. In addition to the species in this study, the authors have successfully spawned three additional species of western Atlantic stony corals in the same systems, suggesting that this method can be successful with a wide range of species and life histories.
It is likely that artificial light pollution plays a role in shifting in situ spawning times (Ayalon et al., 2020; Neely et al., 2020), and the observations in this study support that light pollution after sunset results in later gamete release times in D. cylindrus. Spectral LED output during the twilight phases was not precisely measured for this study, but the addition of a twilight phase may have prevented corals from perceiving sunset at the correct time. For species such as M. meandrites that spawn during twilight or even before sunset, spectral changes during these phases may also be important, but light pollution may not be a critical disruption. By modifying and refining the wavelengths of light provided during sunset, twilight, and moonlight, the underlying mechanisms controlling spawning synchrony along with the impacts of light pollution can be further investigated and inform management decisions aimed to preserve spawning synchrony in the face of anthropogenic impacts (Fogarty and Marhaver, 2019). Fully controlled ex-situ systems may offer the only means of isolating and precisely studying these factors (e.g. Slagel et al., 2021).
Development of a new technology or capability inevitably creates questions about how to best manage and employ it. The capability to hold and spawn broodstock colonies as well as rear coral recruits entirely in ex situ systems offers tremendous potential. Important considerations for using this technology include biosecurity, genetics, and integration into the overall system of active coral reef restoration. Corals held and propagated ex situ for genetic preservation may eventually be returned to their native range, creating the potential for unintended introduction of associated organisms. Pettay et al. (2015) document the spread of an Indo-Pacific coral endosymbiont in the Caribbean and LaJeunesse et al. (2005) show that a non-native coral species and associated endosymbionts can survive on Caribbean reefs for decades. While these introductions were not related to ex situ sexual coral reproduction and their ecological implications are not clear, biosecurity has an important role in reducing the risk of unintended consequences. Systems in the present study were maintained with live organisms that only originated in the Florida Keys and corals were only held with organisms native to Florida. Only non-biologically active prepared diets were used for heterotrophic feeding, and no live bacteria additives were used. In addition, ex situ holding comes with the inherent risk of mechanical failure either due to normal wear and tear, power outages, or major storm events. All aquarium systems in the present study are supplied with full back-up generator power, are built above the base flood elevation, are linked to cloud-based monitoring to notify staff when parameters such as temperature or water flow are out of range, and are housed in hurricane force wind-rated building structures.
The use of ex situ breeding to produce sexual recruits for restoration must be guided by sound genetic principles in order to minimize the risk of negative consequences such as founder effects and inbreeding depression (Greenbaum et al., 2014). A minimum number of genets should be included in any sexual propagation program. A minimum of 20–25 genets was suggested to capture >95% of the common alleles present locally within a species (Baums et al., 2019), however, this number may vary depending on the allelic diversity of the population (Shearer et al., 2009; Afiq-Rosli et al., 2019). It is vital to note that, in recognition of these genetic considerations, the Florida Coral Rescue Project aims to collect a minimum of 200 individuals and 50 unique genotypes from each high-priority species impacted by SCTLD. In order to scale up sexual reproduction and managed breeding for restoration, a thorough understanding of genetics of the in situ population must also be available to guide broodstock choice, strategies for transplanting, and how to best manage an influx of sexual recruits into current restoration practices.
Over two consecutive years we documented synchronized ex situ broadcast spawning in two highly SCTLD susceptible coral species in the family Meandrinidae. This work represents an opportunistic study on corals removed from the reef to preserve the diversity of the local population before it was lost to the disease. Here we have shown that at-risk corals can be protected ex situ, gametogenesis and spawning can be maintained using entirely artificial cues, and ex situ spawning occurs at similar times to wild observations. We also present novel information on the spawning time of M. meandrites, and the first documentation of hermaphroditism in this species. Although we do not yet know when it will be safe to return highly disease-susceptible species to the reef post-SCTLD, nor the best methods for incorporating these offspring into the surviving populations, the methods presented here will serve to produce larvae and sexual recruits for further research into these questions for years to come.
The raw data supporting the conclusions of this article will be made available by the authors, without undue reservation.
KO and JC designed the experiment and programming. RS and KO conducted animal care and collected data. JP and KO drafted the manuscript and conducted analysis. RS compiled data and all Supplementary Material. All authors edited the manuscript and prepared for publication.
Funding for this project was provided by NOAA Office of Protected Resources (CRCP Award #NA18NOS4820206) and the Paul G. Allen Family Foundation. In-kind funding for coral collection was provided by Nova Southeastern University, Keys Marine Laboratory, and Florida Fish and Wildlife Conservation Commission. Significant additional funding for coral care and all publishing fees were provided by The Florida Aquarium.
The authors declare that the research was conducted in the absence of any commercial or financial relationships that could be construed as a potential conflict of interest.
This work could not have been completed without our partners in coral rescue, including Nova Southeastern University, Keys Marine Laboratory, the Association of Zoos and Aquariums, Florida Fish and Wildlife Conservation Commission, Florida Department of Environmental Protection, NOAA Office of Protected Resources and Coral Health and Disease Program, Frost Museum of Science, Coral Restoration Foundation, Mote Marine Laboratory and Aquarium, and the Florida Keys National Marine Sanctuary. We specifically thank the Florida Coral Rescue Team leads, Lisa Gregg and Jennifer Moore, for their endless support, and Karen Neely and Cindy Lewis for spearheading the pillar coral rescue and providing in situ spawning data. We also thank the team at CARMABI and SECORE for sharing M. meandrites in situ spawning data. Finally, we thank Florida Aquarium staff (Emily Williams, Austyn Bushman, Jenny Lee, Rob Gray, and Jacob Harpring) and University of Florida students (Joseph Henry, Aaron Pilnick) who helped with coral observations, coral care, and system construction. Collections were conducted and corals held under Florida Keys National Marine Sanctuary permits FKNMS-2015-156, FKNMS-2016-121, FKNMS-2017-100 and by FWC SAL-19-2142-SCRP.
The Supplementary Material for this article can be found online at: https://www.frontiersin.org/articles/10.3389/fmars.2021.669976/full#supplementary-material
Aeby, G., Ushijima, B., Campbell, J. E., Jones, S., Williams, G., Meyer, J. L., et al. (2019). Pathogenesis of a tissue loss disease affecting multiple species of corals along the Florida reef tract. Front. Mar. Sci. 6:678. doi: 10.3389/fmars.2019.00678
Afiq-Rosli, L., Huang, D., Toh, T. C., Taira, D., Ng, C. S. L., Song, T., et al. (2019). Maximising genetic diversity during coral transplantation from a highly impacted source reef. Conserv.Genet. 20, 629–637. doi: 10.1007/s10592-019-01164-6
Alvarez-Filip, L., Estrada-Saldívar, N., Pérez-Cervantes, E., Molina-Hernández, A., and González-Barrios, F. J. (2019). A rapid spread of the stony coral tissue loss disease outbreak in the Mexican Caribbean. PeerJ 7:e8069. doi: 10.7717/peerj.8069
Anthony, K., Bay, L. K., Costanza, R., Firn, J., Gunn, J., Harrison, P., et al. (2017). New interventions are needed to save coral reefs. Nat. Ecol. Evol. 1, 1420–1422. doi: 10.1038/s41559-017-0313-5
Anthony, K. R., Helmstedt, K. J., Bay, L. K., Fidelman, P., Hussey, K. E., Lundgren, P., et al. (2020). Interventions to help coral reefs under global change—a complex decision challenge. PLoS One 15:e0236399. doi: 10.1371/journal.pone.0236399
Aronson, R., Bruckner, A., Moore, J., Precht, B., and Weil, E. (2008a). Dendrogyra cylindrus. The IUCN Red List of Threatened Species 2008: e.T133124A3582471. doi: 10.2305/IUCN.UK.2008.RLTS.T133124A3582471.en
Aronson, R., Bruckner, A., Moore, J., Precht, B., and Weil, E. (2008b). Meandrina meandrites. The IUCN Red List of Threatened Species 2008: e.T133224A3640365. doi: 10.2305/IUCN.UK.2008.RLTS.T133224A3640365.en
Ayalon, I., Rosenberg, Y., Benichou, J. I., Campos, C. L. D., Sayco, S. L. G., Nada, M. A. L., et al. (2020). Coral gametogenesis collapse under artificial light pollution. Curr. Biol. 31, 413–419.e3. doi: 10.1016/j.cub.2020.10.039
Baums, I. B. (2008). A restoration genetics guide for coral reef conservation. Mol. Ecol. 17, 2796–2811. doi: 10.1111/j.1365-294X.2008.03787.x
Baums, I. B., Baker, A. C., Davies, S. W., Grottoli, A. G., Kenkel, C. D., Kitchen, S. A., et al. (2019). Considerations for maximizing the adaptive potential of restored coral populations in the western Atlantic. Ecol. Appl. 29:e01978. doi: 10.1002/eap.1978
Boch, C., Ananthasubramaniam, B., Sweeney, A. M., Doyle, F. J., and Morse, D. E. (2011). Effects of light dynamics on coral spawning synchrony. Biol. Bull. 220, 161–173. doi: 10.1086/BBLv220n3p161
Brady, A. K., Hilton, J. D., and Vize, P. D. (2009). Coral spawn timing is a direct response to solar light cycles and is not an entrained circadian response. Coral Reefs 28, 677–680. doi: 10.1007/s00338-010-0589-2
Brady, A. K., Willis, B. L., Harder, L. D., and Vize, P. D. (2016). Lunar phase modulates circadian gene expression cycles in the broadcast spawning coral Acropora millepora. Biol. Bull. 230, 130–142. doi: 10.1086/BBLv230n2p130
Bythell, J., Pantos, O., and Richardson, L. (2004). “White plague, white band, and other ‘white’ diseases,” in Coral Health and Disease, eds E. Rosenberg, and Y. Loya (Berlin: Springer), 351–365.
Chamberland, V., Vermeij, M., Brittsan, M., Carl, M., Schick, M., Snowden, S., et al. (2015). Restoration of critically endangered elkhorn coral (Acropora palmata) populations using larvae reared from wild-caught gametes. Glob. Ecol. Conserv. 4, 526–537. doi: 10.1016/j.gecco.2015.10.005
Chan, A. N., Lewis, C. L., Neely, K. L., and Baums, I. B. (2019). Fallen pillars: the past, present, and future population dynamics of a rare, specialist coral–algal symbiosis. Front. Mar. Sci. 6:218. doi: 10.3389/fmars.2019.00218
Craggs, J., Guest, J., Bulling, M., and Sweet, M. (2019). Ex situ co culturing of the sea urchin, Mespilia globulus and the coral Acropora millepora enhances early post-settlement survivorship. Sci. Rep. 9:12984. doi: 10.1038/s41598-019-49447-9
Craggs, J., Guest, J., Davis, M., and Sweet, M. (2020). Completing the life cycle of a broadcast spawning coral in a closed mesocosm. Invertebr. Reprod. Dev. 64, 244–247. doi: 10.1080/07924259.2020.1759704
Craggs, J., Guest, J. R., Brett, A., Davis, M., and Sweet, M. (2018). Maintaining natural spawning timing in Acropora corals following long distance inter-continental transportation. J. Zoo Aquar. Res. 6, 30–36. doi: 10.19227/jzar.v6i2.317
Craggs, J., Guest, J. R., Davis, M., Simmons, J., Dashti, E., and Sweet, M. (2017). Inducing broadcast coral spawning ex situ: closed system mesocosm design and husbandry protocol. Ecol. Evol. 7, 11066–11078. doi: 10.1002/ece3.3538
dela Cruz, D. W., and Harrison, P. L. (2020). Enhancing coral recruitment through assisted mass settlement of cultured coral larvae. PLoS One 15:e0242847. doi: 10.1371/journal.pone.0242847
Estrada-Saldívar, N., Molina-Hernández, A., Pérez-Cervantes, E., Medellın-Maldonado, F., González-Barrios, F. J., and Alvarez-Filip, L. (2020). Reef-scale impacts of the stony coral tissue loss disease outbreak. Coral Reefs 39, 861–866. doi: 10.1007/s00338-020-01949-z
Flynn, R. L., and Forrester, G. E. (2019). Boat anchoring contributes substantially to coral reef degradation in the British Virgin Islands. PeerJ 7:e7010. doi: 10.7717/peerj.7010
Fogarty, N. D., and Marhaver, K. L. (2019). Coral spawning, unsynchronized. Science 365, 987–988. doi: 10.1126/science.aay7457
Gardner, T. A., Côté, I. M., Gill, J. A., Grant, A., and Watkinson, A. R. (2003). Long-term region-wide declines in Caribbean corals. Science 301, 958–960. doi: 10.1126/science.1086050
Gascoigne, J., and Lipcius, R. N. (2004). Allee effects in marine systems. Mar. Ecol. Prog. Ser. 269, 49–59. doi: 10.3354/meps269049
Greenbaum, G., Templeton, A. R., Zarmi, Y., and Bar-David, S. (2014). Allelic richness following population founding events–a stochastic modeling framework incorporating gene flow and genetic drift. PLoS One 9:e115203. doi: 10.1371/journal.pone.0115203
Guest, J. R., Baria, M. V., Gomez, E. D., Heyward, A. J., and Edwards, A. J. (2014). Closing the circle: is it feasible to rehabilitate reefs with sexually propagated corals? Coral Reefs 33, 45–55. doi: 10.1007/s00338-013-1114-1
Hagedorn, M., Carter, V. L., Lager, C., Ciani, J. F. C., Dygert, A. N., Schleiger, R. D., et al. (2016). Potential bleaching effects on coral reproduction. Reprod. Fertil. Dev. 28, 1061–1071. doi: 10.1038/s41598-017-14644-x
Hagedorn, M., Carter, V. L., Henley, E. M., Van Oppen, M. J., Hobbs, R., and Spindler, R. E. (2017). Producing coral offspring with cryopreserved sperm: a tool for coral reef restoration. Sci. Rep. 7:14432. doi: 10.1038/s41598-017-14644-x
Harrison, P. L., Babcock, R. C., Bull, G. D., Oliver, J. K., Wallace, C. C., and Willis, B. L. (1984). Mass spawning in tropical reef corals. Science 223, 1186–1189. doi: 10.1126/science.223.4641.1186
Hayes, N. K. (2019). A Characterization of a Southeast Florida Stony Coral Assemblage After a Disease Event. Master’s thesis. Fort Lauderdale, FL: Nova Southeastern University, 37.
Hédouin, L., Rouzé, H., Berthe, C., Perez-Rosales, G., Martinez, E., Chancerelle, Y., et al. (2020). Contrasting patterns of mortality in Polynesian coral reefs following the third global coral bleaching event in 2016. Coral Reefs 39, 939–952. doi: 10.1007/s00338-020-01914-w
Henry, J. A., O’Neil, K. L., and Patterson, J. T. (2019). Native herbivores improve sexual propagation of threatened staghorn coral Acropora cervicornis. Front. Mar. Sci. 6:713. doi: 10.3389/fmars.2019.00713
Heres, M. M., Farmer, B. H., Elmer, F., and Hertler, H. (2021). Ecological consequences of Stony coral tissue loss disease in the Turks and Caicos Islands. Coral Reefs 40, 609–624. doi: 10.1007/s00338-021-02071-4
Heyward, A. J., Smith, L. D., Rees, M., and Field, S. N. (2002). Enhancement of coral recruitment by in situ mass culture of coral larvae. Mar. Ecol. Prog. Ser. 230, 113–118. doi: 10.3354/meps230113
Hoegh-Guldberg, O., Poloczanska, E. S., Skirving, W., and Dove, S. (2017). Coral reef ecosystems under climate change and ocean acidification. Front. Mar. Sci. 4:158. doi: 10.3389/fmars.2017.00158
Jokiel, P. L., Ito, R. Y., and Liu, P. M. (1985). Night irradiance and synchronization of lunar release of planula larvae in the reef coral Pocillopora damicornis. Mar. Biol. 88, 167–174. doi: 10.1007/BF00397164
Jordan, A. C. (2018). Patterns in Caribbean Coral Spawning. Master’s thesis. Fort Lauderdale, FL: Nova Southeastern University.
Jordan, L. K. B., Banks, K. W., Fisher, L. E., Walker, B. K., and Gilliam, D. S. (2010). Elevated sedimentation on coral reefs adjacent to a beach nourishment project. Mar. Pollut. Bull. 60, 261–271. doi: 10.1016/j.marpolbul.2009.08.032
Kabay, L. (2016). Population Demographics and Sexual Reproduction Potential of the Pillar Coral, Dendrogyra cylindrus, on the Florida Reef Tract. Master’s thesis. Fort Lauderdale, FL: Nova Southeastern University.
Kaniewska, P., Alon, S., Karako-Lampert, S., Hoegh-Guldberg, O., and Levy, O. (2015). Signaling cascades and the importance of moonlight in coral broadcast mass spawning. eLife 4:e09991. doi: 10.7554/eLife.09991
Keith, S. A., Maynard, J. A., Edwards, A. J., Guest, J. R., Bauman, A. G., van Hooidonk, R., et al. (2016). Coral mass spawning predicted by rapid seasonal rise in ocean temperature. Proc. R. Soc. B Biol. Sci. 283:20160011. doi: 10.1098/rspb.2016.0011
Knowlton, N. (1992). Thresholds and multiple stable states in coral reef community dynamics. Am. Zool. 32, 674–682. doi: 10.1093/icb/32.6.674
Knowlton, N., Mate, J. L., Guzman, H. M., Rowan, R., and Jara, J. (1997). Direct evidence for reproductive isolation among the three species of the Montastraea annularis complex in Central America (Panama and Honduras). Mar. Biol. 127, 705–711. doi: 10.1007/s002270050061
LaJeunesse, T. C., Lee, S., Bush, S., and Bruno, J. F. (2005). Persistence of non-caribbean algal symbionts in Indo-Pacific mushroom corals released to Jamaica 35 years ago. Coral Reefs 24, 157–159. doi: 10.1007/s00338-004-0436-4
Landsberg, J. H., Kiryu, Y., Peters, E. C., Wilson, P. W., Perry, N., Waters, Y., et al. (2020). Stony coral tissue loss disease in Florida is associated with disruption of host–zooxanthellae physiology. Front. Mar. Sci. 7:576013. doi: 10.3389/fmars.2020.576013
Lesser, M. P. L., Mazel, C., Phinney, D., and Yentsch, C. S. (2000). Light absorption and utilization by colonies of the congeneric hermatypic corals Montastraea faveolata and Montastraea cavernosa. Limnol. Oceanogr. 45, 76–86. doi: 10.4319/lo.2000.45.1.0076
Levitan, D. R., Boudreau, W., Jara, J., and Knowlton, N. (2014). Long-term reduced spawning in Orbicella coral species due to temperature stress. Mar. Ecol. Prog. Ser. 515, 1–10. doi: 10.3354/meps11063
Levitan, D. R., Fogarty, N. D., Jara, J., Lotterhos, K. E., and Knowlton, N. (2011). Genetic, spatial, and temporal components of precise spawning synchrony in reef building corals of the Montastraea annularis species complex. Evolution 65, 1254–1270. doi: 10.1111/j.1558-5646.2011.01235.x
Manzello, D. P. (2015). Rapid recent warming of coral reefs in the Florida Keys. Sci. Rep. 5:16762. doi: 10.1038/srep16762
Marhaver, K. L., Vermeij, M. J., and Medina, M. M. (2015). Reproductive natural history and successful juvenile propagation of the threatened Caribbean pillar coral Dendrogyra cylindrus. BMC Ecol. 15:9. doi: 10.1186/s12898-015-0039-7
McLean, M., Cuetos-Bueno, J., Nedlic, O., Luckymiss, M., and Houk, P. (2016). Local stressors, resilience, and shifting baselines on coral reefs. PLoS One 11:e0166319. doi: 10.1371/journal.pone.0166319
Meyer, J. L., Castellanos-Gell, J., Aeby, G. S., Häse, C. C., Ushijima, B., and Paul, V. J. (2019). Microbial community shifts associated with the ongoing Stony coral tissue loss disease outbreak on the Florida reef tract. Front. Microbiol. 10:2244. doi: 10.3389/fmicb.2019.02244
Miller, C. V., May, L. A., Moffitt, Z. J., and Woodley, C. M. (2020). Exploratory Treatments for Stony Coral Tissue Loss Disease: Pillar Coral (Dendrogyra cylindrus). Charleston, SC: NOAA Technical Memorandum NOS NCCOS 245, 78.
Miller, M. W., Karazsia, J., Groves, C. E., Griffin, S., Moore, T., Wilber, P., et al. (2016). Detecting sedimentation impacts to coral reefs resulting from dredging the Port of Miami, Florida USA. PeerJ 4:e2711. doi: 10.7717/peerj.2711
Muller, E. M., Sartor, C., Alcaraz, N. I., and van Woesik, R. (2020). Spatial epidemiology of the stony-coral-tissue-loss disease in Florida. Front. Mar. Sci. 7:163. doi: 10.3389/fmars.2020.00163
Neely, K. L., and Lewis, C. (2020). Rapid population decline of the pillar coral Dendrogyra cylindrus along the Florida reef tract. bioRxiv [Preprint]. doi: 10.1101/2020.05.09.085886
Neely, K. L., Lewis, C., Chan, A. N., and Baums, I. B. (2018). Hermaphroditic spawning by the gonochoric pillar coral Dendrogyra cylindrus. Coral Reefs 37, 1087–1092. doi: 10.1007/s00338-018-1730-x
Neely, K. L., Macaulay, K. A., Hower, E. K., and Dobler, M. A. (2020). Effectiveness of topical antibiotics in treating corals affected by Stony coral tissue loss disease. PeerJ 8:e9289. doi: 10.7717/peerj.9289
O’Neil, K., Neely, K. L., and Patterson, J. (2018). Nursery Management and Treatment of Disease-Ravaged Pillar Coral (Dendrogyra cylindrus) on the Florida Reef Tract. Miami, FL: Florida DEP, 1–13.
Oldach, M. J., Workentine, M., Matz, M. V., Fan, T. Y., and Vize, P. D. (2017). Transcriptome dynamics over a lunar month in a broadcast spawning acroporid coral. Mol. Ecol. 26, 2514–2526. doi: 10.1111/mec.14043
Omori, M. (2005). Success of mass culture of Acropora corals from egg to colony in open water. Coral Reefs 24:563. doi: 10.1007/s00338-005-0030-4
Omori, M., and Iwao, K. (2014). Methods of Farming Sexually Propagated Corals and Outplanting for Coral Reef Rehabilitation; With List of References for Coral Reef Rehabilitation Through Active Restoration Measure. Okinawa: Akalima Marine Science Laboratory, 1–22.
Palandro, D. A., Andréfouët, S., Hu, C., Hallock, P., Müller-Karger, F. E., Dustan, P., et al. (2008). Quantification of two decades of shallow-water coral reef habitat decline in the Florida Keys National Marine Sanctuary using Landsat data (1984–2002). Remote Sens. Environ. 112, 3388–3399. doi: 10.1016/j.rse.2008.02.015
Paxton, C. W., Baria, M. V. B., Weis, V. M., and Harii, S. (2016). Effect of elevated temperature on fecundity and reproductive timing in the coral Acropora digitifera. Zygote 24, 511–516. doi: 10.1017/S0967199415000477
Penland, L., Kloulechad, J., Idip, D., and Van Woesik, R. (2004). Coral spawning in the western Pacific Ocean is related to solar insolation: evidence of multiple spawning events in Palau. Coral Reefs 23, 133–140.
Petersen, D., Hatta, M., Laterveer, M., and van Bergen, D. (2005). Ex situ transportation of coral larvae for research, conservation, and aquaculture. Coral Reefs 24, 510–513. doi: 10.1007/s00338-005-0498-y
Pettay, D. T., Wham, D. C., Smith, R. T., Iglesias-Prieto, R., and LaJeunesse, T. C. (2015). Microbial invasion of the Caribbean by an Indo-Pacific coral zooxanthella. Proc. Natl. Acad. Sci. U.S.A. 112, 7513–7518. doi: 10.1073/pnas.1502283112
Pinzón, J. H., and Weil, E. (2011). Cryptic species within the Atlantic-Caribbean genus Meandrina (Scleractinia): a multidisciplinary approach and description of the new species Meandrina jacksoni. Bull. Mar. Sci. 87, 823–853. doi: 10.5343/bms.2010.1085
Pollock, F. J., Katz, S. M., Davies, S., Hein, M., Torda, G., Matz, M. V., et al. (2017). Coral larvae for restoration and research: a large-scale method for rearing Acropora millepora larvae, inducing settlement, and establishing symbiosis. PeerJ 5:e3732. doi: 10.7717/peerj.3732
Precht, W. F., Gintert, B. E., Robbart, M. L., Fura, R., and Van Woesik, R. (2016). Unprecedented disease-related coral mortality in Southeastern Florida. Sci. Rep. 6:31374. doi: 10.1038/srep31374
R Core Team (2019). R: A Language and Environment for Statistical Computing. Vienna: R Foundation for Statistical Computing.
Rosales, S. M., Clark, A. S., Huebner, L. K., Ruzicka, R. R., and Muller, E. M. (2020). Rhodobacterales and Rhizobiales are associated with Stony coral tissue loss disease and its suspected sources of transmission. Front. Microbiol. 11:681. doi: 10.3389/fmicb.2020.00681
Sakai, Y., Hatta, M., Furukawa, S., Kawata, M., Ueno, N., and Maruyama, S. (2020). Environmental factors explain spawning day deviation from full moon in the scleractinian coral Acropora. Biol. Lett. 16:20190760. doi: 10.1098/rsbl.2019.0760
SCTLD Case Definition (2018). Florida Coral Disease Response Research & Epidemiology Team. Available online at: https://floridadep.gov/sites/default/files/Copy%20of%20StonyCoralTissueLossDisease_CaseDefinition%20final%2010022018.pdf (accessed April 20, 2021).
Sharp, W. C., and Maxwell, K. E. (2018). Investigating the Ongoing Coral Disease Outbreak in the Florida Keys: Collecting Corals to Diagnose the Etiological Agent (s) and Establishing Sentinel Sites to Monitor Transmission Rates and the Spatial Progression of the Disease. Final Report to the Florida Department of Environmental Protection. St. Petersburg, FL: Fish and Wildlife Research Institute, 1–31.
Sharp, W. C., Shea, C. P., Maxwell, K. E., Muller, E. M., and Hunt, J. H. (2020). Evaluating the small-scale epidemiology of the stony-coral-tissue-loss-disease in the middle Florida Keys. PLoS One 15:e0241871. doi: 10.1371/journal.pone.0241871
Shearer, T. L., Porto, I., and Zubillaga, A. L. (2009). Restoration of coral populations in light of genetic diversity estimates. Coral Reefs 28, 727–733. doi: 10.1007/s00338-009-0520-x
Shlesinger, T., and Loya, Y. (2019). Breakdown in spawning synchrony: a silent threat to coral persistence. Science 365, 1002–1007. doi: 10.1126/science.aax0110
Slagel, S., Lohr, K., O’Neil, K., and Patterson, J. (2021). Growth, calcification, and photobiology of the threatened coral Acropora cervicornis in natural versus artificial light. Zoo Biology 1–7. doi: 10.1002/zoo.21589
Staley, C., Kaiser, T., Gidley, M. L., Enochs, I. C., Jones, P. R., Goodwin, K. D., et al. (2017). Differential impacts of land-based sources of pollution on the microbiota of Southeast Florida coral reefs. Appl. Environ. Microbiol. 83:e03378-16. doi: 10.1128/AEM.03378-16
Sweeney, A. M., Boch, C. A., Johnsen, S., and Morse, D. E. (2011). Twilight spectral dynamics and the coral reef invertebrate spawning response. J. Exp. Biol. 214, 770–777. doi: 10.1242/jeb.043406
Szmant, A. M. (1986). Reproductive ecology of Caribbean reef corals. Coral Reefs 5, 43–53. doi: 10.1007/BF00302170
Teo, A., and Todd, P. A. (2018). Simulating the effects of colony density and intercolonial distance on fertilisation success in broadcast spawning scleractinian corals. Coral Reefs 37, 891–900. doi: 10.1007/s00338-018-1715-9
Toth, L. T., Stathakopoulos, A., Kuffner, I. B., Ruzicka, R. R., Colella, M. A., and Shinn, E. A. (2019). The unprecedented loss of Florida’s reef-building corals and the emergence of a novel coral-reef assemblage. Ecology 100:e02781. doi: 10.1002/ecy.2781
Ushijima, B., Meyer, J. L., Thompson, S., Pitts, K., Marusich, M. F., Tittl, J., et al. (2020). Disease diagnostics and potential coinfections by Vibrio coralliilyticus during an ongoing coral disease outbreak in Florida. Front. Microbiol. 11:569354. doi: 10.3389/fmicb.2020.569354
van Oppen, M. J., Gates, R. D., Blackall, L. L., Cantin, N., Chakravarti, L. J., Chan, W. Y., et al. (2017). Shifting paradigms in restoration of the world’s coral reefs. Glob. Change Biol. 23, 3437–3448. doi: 10.1111/gcb.13647
van Woesik, R., Lacharmoise, F., and Köksal, S. (2006). Annual cycles of solar insolation predict spawning times of Caribbean corals. Ecol. Lett. 9, 390–398. doi: 10.1111/j.1461-0248.2006.00886.x
Walton, C. J., Hayes, N. K., and Gilliam, D. S. (2018). Impacts of a regional, multi-year, multi-species coral disease outbreak in Southeast Florida. Front. Mar. Sci. 5:323. doi: 10.3389/fmars.2018.00323
Weil, E., Hernández-Delgado, E., Gonzalez, M., Williams, S., Suleimán-Ramos, S., Figuerola, M., et al. (2019). Spread of the new coral disease “SCTLD” into the Caribbean: implications for Puerto Rico. Reef Encounter 34, 38–43.
Keywords: SCTLD, coral reproduction, coral conservation, spawning, Florida Reef Tract, aquarium, Dendrogyra, Meandrina
Citation: O’Neil KL, Serafin RM, Patterson JT and Craggs JRK (2021) Repeated ex situ Spawning in Two Highly Disease Susceptible Corals in the Family Meandrinidae. Front. Mar. Sci. 8:669976. doi: 10.3389/fmars.2021.669976
Received: 19 February 2021; Accepted: 07 April 2021;
Published: 11 May 2021.
Edited by:
William F. Precht, Dial Cordy and Associates, Inc., United StatesReviewed by:
Lyndon Mark DeVantier, Coral Reef Research, AustraliaCopyright © 2021 O’Neil, Serafin, Patterson and Craggs. This is an open-access article distributed under the terms of the Creative Commons Attribution License (CC BY). The use, distribution or reproduction in other forums is permitted, provided the original author(s) and the copyright owner(s) are credited and that the original publication in this journal is cited, in accordance with accepted academic practice. No use, distribution or reproduction is permitted which does not comply with these terms.
*Correspondence: Keri L. O’Neil, a29uZWlsQGZsYXF1YXJpdW0ub3Jn
Disclaimer: All claims expressed in this article are solely those of the authors and do not necessarily represent those of their affiliated organizations, or those of the publisher, the editors and the reviewers. Any product that may be evaluated in this article or claim that may be made by its manufacturer is not guaranteed or endorsed by the publisher.
Research integrity at Frontiers
Learn more about the work of our research integrity team to safeguard the quality of each article we publish.