- 1Coral Reef Restoration, Assessment and Monitoring Lab, Halmos College of Arts and Sciences, Nova Southeastern University, Dania Beach, FL, United States
- 2Coral REEF Lab, Center for Marine Science, University of North Carolina Wilmington, Wilmington, NC, United States
- 3The Perry Institute for Marine Science, Waitsfield, VT, United States
For decades, coral reef ecosystems have been in decline due to environmental stressors such as rising sea temperatures, increased disease prevalence, and other local anthropogenic sources. Considering this decline, coral restoration efforts in the Caribbean have been implemented to promote reef recovery with a focus on the coral genus Acropora. Current methods target the threatened species Acropora cervicornis and A. palmata, but little is known about the restoration potential of their hybrid taxon, A. prolifera. Using interspecific hybrids with higher fitness than one or both parental species has gained traction as a novel restoration technique. For this study, three in situ coral tree nurseries were established around Great Stirrup Cay, The Bahamas, to compare the growth and survival among acroporid taxa. Three 150 mm fragments from six putative genotypes of each acroporid taxa were collected from reefs around New Providence, The Bahamas, and transported to Great Stirrup Cay in June 2018. One fragment from each genotype was transported to each nursery site, cut into three sections (apical, middle, and basal), and suspended from PVC coral trees. Fragment survival was collected monthly for 13 months, and Total Linear Extension (TLE) values were calculated for each fragment monthly for 12 months. Nursery site significantly affected fragment survival, while taxon and fragment section did not. Total fragment mortality was 29.3% in the first month but ranged from 0 to 5% for the rest of the study period until July 2019 (32.7% of remaining fragments died primarily at N1). Overall, A. prolifera growth was significantly greater than the parental species. Taxon, nursery site, and fragment section were identified as important factors affecting TLE. Apical A. prolifera fragment sections at site N3 had the greatest average linear growth at 12 months and had the greatest average growth rate per month. This study highlights the rapid growth rate of hybrid corals and suggests that fragment sections have equivalent survival and growth. Consequently, these results suggest that restoration managers may capitalize on fast growing hybrids for outplanting to degraded reefs and to increase the scale of nursery projects.
Introduction
In the face of climate change and other environmental threats, conservation and restoration of the world’s natural resources are now at the forefront of scientific research (Harris et al., 2006; Heller and Zavaleta, 2009; Jackson and Hobbs, 2009). Global issues such as deforestation, rising global temperatures, pollution, and the overuse of natural resources are serious threats to marine ecosystems and terrestrial environments (Vitousek, 1994; Derraik, 2002; Shahidul Islam and Tanaka, 2004; Harley et al., 2006; Malhi et al., 2008; Cinner et al., 2015; Hughes et al., 2017b). As such, finding ways of protecting these environments are critical to the continuity of the global biome.
Coral reefs are one of the world’s most important and threatened marine ecosystems (Sebens, 1994; Maragos et al., 1996; Reaka-Kudla, 1997; Hughes et al., 2017a). They host a diversity of ecologically and commercially important marine species (Moberg and Folke, 1999), are essential nursery grounds for numerous fish and invertebrate species (Heck et al., 2008; Holbrook et al., 2015), protect coastlines from storm damage (Cesar et al., 2003; Ferrario et al., 2014; Storlazzi et al., 2019), and support an extensive tourism industry for many island nations and coastal regions (Cesar et al., 2003). Unfortunately, nearly 27% of the world’s coral reefs have been lost due to destructive events and stressors (Cesar et al., 2003). Local anthropogenic threats (e.g., physical damage, overfishing, pollution, sedimentation), and larger global stressors (e.g., temperature increases, increased disease prevalence and storm damage, ocean acidification), are drivers of coral decline (Lirman and Fong, 1997; Hughes and Connell, 1999; Babcock and Smith, 2000; Woodley et al., 2000; Bellwood et al., 2004; Shahidul Islam and Tanaka, 2004; Fox and Caldwell, 2006; Voss and Richardson, 2006; Pandolfi et al., 2011; Smith et al., 2015; Cheal et al., 2017; Hughes et al., 2017b; Hughes et al., 2018). Increased sea temperatures can cause coral bleaching, a stress response during which colony pigmentation may be lost and often the microalgae symbionts found in coral tissue are expelled (Brown, 1997; Baker et al., 2008; Heron et al., 2016), which can lead to colony death if the stress is prolonged (Douglas, 2003; Eakin et al., 2010). Increasing ocean temperatures have also been linked to increases in disease outbreaks, resulting in large-scale coral mortality (Weil, 2004; Harvell et al., 2007; Muller et al., 2007; Eakin et al., 2010). Disturbance to a reef is natural but continued impacts of chronic stressors has changed coral reef community composition from one benthic group to another (Hughes et al., 1985; Hughes, 1994; Lirman, 2001; Bellwood et al., 2004; Knowlton and Jackson, 2008; Jackson et al., 2014; Jones et al., 2020).
In the Caribbean, the increase of such stressors and their compounding effects has led to significant losses in scleractinian coral cover since the late 1970s (Gardner et al., 2003; Eakin et al., 2010; Jackson et al., 2014). Much of this loss is attributed to the severe decline (up to 95%) of the Caribbean acroporid corals, Acropora cervicornis and A. palmata (Aronson and Precht, 2001; Bruckner, 2002). Prior to the 1970s, A. cervicornis and A. palmata were the major contributors to many reef habitats across the Caribbean (Aronson and Precht, 1997; McNeill et al., 1997; Bruckner, 2002; Gardner et al., 2003; Miller and van Oppen, 2003; Bellwood et al., 2004), contributing up to 50% of total stony coral cover above ∼20 m depth (Bellwood et al., 2004). These species provide many ecosystem services, including vital habitats for fish and invertebrates, reef structure through carbonate deposition, and coastal wave protection from storms (Bruckner, 2002). Acroporids primarily rely on asexual reproduction through fragmentation (Rinkevich, 1995; Lirman and Fong, 1997; Smith and Hughes, 1999), but also reproduce sexually through hermaphroditic broadcast spawning (Szmant, 1986; Vargas-Angel and Thomas, 2002; Fogarty et al., 2012). The two parental species are also capable of reproducing with each other to produce an F1 hybrid, Acropora prolifera (van Oppen et al., 2000; Vollmer and Palumbi, 2002; Kitchen et al., 2019). Like the parental species, A. prolifera can reproduce asexually through fragmentation, and the molecular signatures suggest they reproduce sexually with the parental species (Vollmer and Palumbi, 2002; Kitchen et al., 2019; Kitchen et al., 2021). In recent decades, A. cervicornis and A. palmata have declined in abundance primarily from disease, but also bleaching, storm damage, and predation (Aronson and Precht, 2001; Bruckner, 2002; Jackson et al., 2014). In response to their decline, A. cervicornis and A. palmata were listed as threatened under the United States Endangered Species Act as of 2006 (National Marine Fisheries Service, 2006) and as critically endangered by the International Union for the Conservation of Nature (IUCN)’s Red List as of 2008 (Aronson et al., 2008a,b). In recent years, hybrid abundance has increased at some sites in the Caribbean, despite losses in the parental species (Fogarty, 2010, 2012; Japaud et al., 2014; Nylander-Asplin et al., 2021).
To facilitate recovery of A. cervicornis and A. palmata, many organizations through the Caribbean are working to increase Acropora abundance and genetic diversity (Johnson et al., 2011; Young et al., 2012; Baums et al., 2019; Boström-Einarsson et al., 2020). In many cases, these efforts are achieved by the creation and maintenance of coral nurseries, which provides a sheltered area for corals to grow away from the reef and predators. This ‘gardening technique’ proposed by Rinkevich (1995) has been adopted as a general practice for many reef restoration organizations. Based on silviculture practices, coral fragments are collected from different genotypes of the target species, grown in in situ nurseries, and outplanted to local reefs (Rinkevich, 1995; Lirman et al., 2010; Lirman, 2000; Zimmer, 2006). This method of coral gardening has been widely adapted across the globe for large-scale restoration efforts.
Caribbean Acropora restoration research has led to improvements in propagation techniques, as well as the recovery of localized populations of the parental species (Ware et al., 2020), but there is limited information and use of the hybrid in restoration. While the parental species have been in decline, the hybrid has persisted on many reefs in the Caribbean with equal or increased abundance, better survival, and equal or less susceptibility to disease and other environmental pressures (Fogarty, 2012; Irwin et al., 2017; Howe, 2018; Nylander-Asplin et al., 2021; Weil et al., 2020). Furthermore, research on the early life stages of Acropora species in the Pacific suggests hybrid larvae had equal or greater fitness compared to the parental species (Chan et al., 2018, 2019b). Although hybrids are often thought to be sterile (Ortiz-Barrientos et al., 2007), A. prolifera can successfully reproduce with both A. cervicornis (Kitchen et al., 2019) and A. palmata via backcrossing (van Oppen et al., 2000; Vollmer and Palumbi, 2002; Kitchen et al., 2019). Backcrossing is noted among many marine organisms (Arnold and Fogarty, 2009) and provides an avenue for the genetic material from one parent to be exchanged between congenerics, that may have led to reticulate evolution (Veron, 1995; Willis et al., 2006). Backcrossing may enhance the adaptive potential of the threatened parental species in a changing environment by providing increased genetic diversity (Willis et al., 2006), or at the very least, acroporid hybrids may provide needed infrastructure to shallow reefs while the parental species continue to decline.
To identify the potential of using hybrids in restoration, this study investigates factors (nursery site, taxa, fragment section, and genotype) that may influence growth and survival of the threatened Caribbean acroporid coral species and their naturally occurring hybrid at three in situ nurseries in The Bahamas.
Materials and Methods
Study Location
This study was conducted at Great Stirrup Cay (GSC) (25.824 N, −77.91 W), The Bahamas, from June 2018 to July 2019. Great Stirrup Cay is located at the northern end of the Berry Islands in the central Bahamas (Figure 1). Great Stirrup Cay is a private island owned by Norwegian Cruise Line® (NCL), which receives thousands of cruise ship visitors every week. Coral reefs fringe the northern side of the island, and seagrass beds and sand flats are common to the south. The deeper fringing reefs (∼15 m) on the northern side of the island are composed of large mounding corals including Orbicella spp. and Montastraea cavernosa, gorgonians, and sponges. On the eastern side of the island, reefs flats contain scattered acroporid colonies and smaller mounding corals, along with various species of gorgonians.
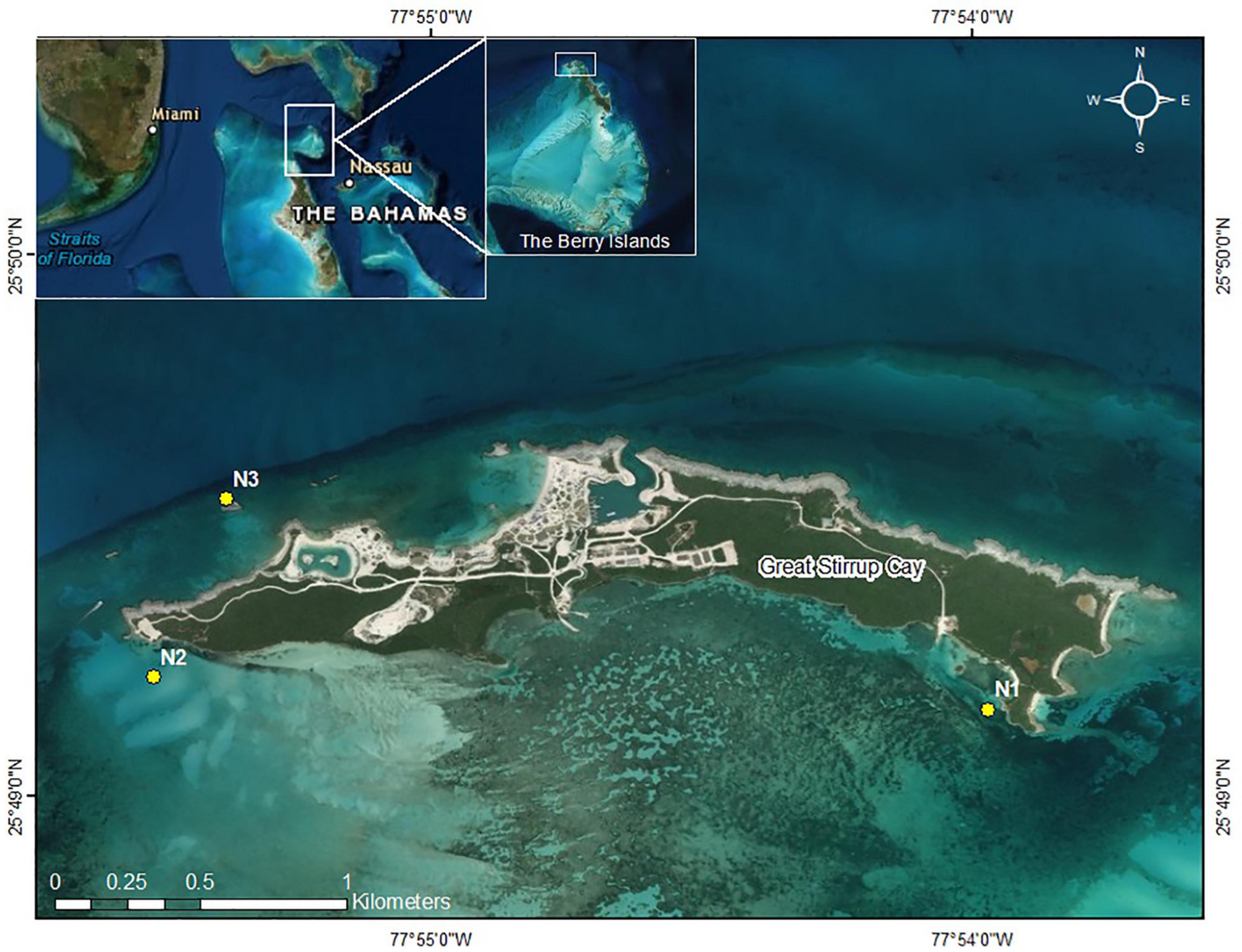
Figure 1. Great Stirrup Cay (GSC). Yellow dotes indicate nursery sites. Inset: The Berry Islands, The Bahamas, in relation to Florida and the greater Bahamas.
Study Species
Acropora cervicornis is typically found on shallow reefs down to 20 m depths; A. palmata is usually found on shallow reef crests to 10 m depths in areas with high wave energy (McNeill et al., 1997; Johnson et al., 2011). Acropora palmata can grow up to ∼10 cm per year (McNeill et al., 1997; Bak et al., 2009), while A. cervicornis has been recorded growing much faster, depending on location and genotype (Gladfelter et al., 1978; Lirman et al., 2014; Schopmeyer et al., 2017). Acropora cervicornis has long, thin branches extending from a central basal attachment, while A. palmata has wide, flattened branches that also extend from a central basal attachment point (Neigel and Avise, 1983). Acropora prolifera is described as having a “bushy” or “palmate” morphology that is intermediate between the parental species and originally attributed, at least in part, to the maternal species (Vollmer and Palumbi, 2002). Recent molecular evidence from hybrid samples across a broader geographic range suggests egg donor is not predictive of hybrid morphology (Kitchen et al., 2021). Hybrids are often found at shallow depths (<2 m) with moderate to high wave energy, but occasionally can be found in deeper, calmer environments (Fogarty, 2012). All three taxa can naturally reproduce asexually via fragmentation, making them ideal candidates for coral restoration (Rinkevich, 1995; Herlan and Lirman, 2008; Griffin et al., 2012; Schopmeyer et al., 2017).
Nursery Sites
Three replicate nursery sites were established at GSC. Nursery locations included two southern sand flat sites (N1 and N2) and one northern reef slope site (N3) with depths ranging from 2.5 to 3.5 m (Figure 1). Site N1 was located near adjacent seagrass beds, while site N2 was established in sand near a boat channel on the western side of the island. Site N3 was the most exposed nursery site in terms of wave action and seasonal wind patterns. Sites were chosen based on depth, accessibility, storm protection, and isolation from human impact. Nursery sites were not placed on the east end of the island near wild A. cervicornis and A. palmata colonies due to limited accessibility from high wave energy and shallow depths. Turbidity was dependent of time of year, with site N1 observationally having greater turbidity than sites N2 and N3 but better protection from wave action due to seasonal weather patterns and storms. Three coral nursery trees© (Nedimyer et al., 2011) were placed 5 m apart in a line at each nursery site. Nursery trees were made from PVC and fiberglass rods with pre-drilled holes along each rod. The trees were tagged and secured to the seabed using sand (helix) anchors or epoxied eyebolts, depending on the substrate type (sand or hard bottom, respectively). Trees were tied to the anchors using polypropylene rope with plastic tubing through a metal shackle, such that the middle branch was at a depth of approximately three meters below the surface. Every tree contained five branches with corals attached to the middle three branches. Each branch was spaced 15 cm apart and installed perpendicular to the one above to avoid abrasion and shading effects between coral fragments. Six corals were attached per branch approximately 10 cm apart using 80 lb. test (0.89 mm) monofilament.
Coral Collection
Acropora cervicornis, A. palmata, and A. prolifera fragments were collected from the reefs around New Providence, The Bahamas, in June 2018 using a hammer and chisel or diagonal cutters. Fragments were collected from colonies between 1 and 3 m depth and ≥10 m apart to increase the confidence of genotypic variation. Collection targeted six putative genotypes for each taxon; a small tissue sample was collected to confirm clonal identity. Three 150 mm branches (fragments) were collected from each donor colony (n = 19 A. cervicornis, n = 18 A. palmata, n = 18 A. prolifera). An extra branch from a separate colony was collected for A. cervicornis due to greater availability and the potential loss of other fragments due to stress of transport. Acropora palmata and A. prolifera were not as widely available, and so only the minimum number of branches were collected. The three branches from each colony were placed in heavy duty plastic zipper bags filled with seawater and transported inside of Bubble Wrap® lined coolers. Ice packs were placed in the coolers for temperature regulation and were flown to GSC. Upon arrival the water was changed and the fragments were transported to nursery site N2. The following day, one 150 mm fragment from each genotype was transported to each nursery site and cut into three smaller 50 mm sections (n = 57 A. cervicornis, n = 51 A. palmata, and n = 49 A. prolifera). The sections were labeled as apical, middle, and basal, as per origin on the donor branch (Figure 2) and were distributed across the three trees at each site (Figure 3). Five A. prolifera and three A. palmata fragments were not included in the nursery due to poor visual condition after transport, and three extra A. cervicornis sections were included from initial collection. Note, this created a slightly uneven sampling design. All taxa, putative genotypes, and fragment sections were replicated at each site in a crossed design (see Supplementary Figure 2). However, later genetic analysis revealed some of the putative genotypes were clone mates and therefore contained more fragments. Tree location, coral section size (length, width, and size/number of branches), and condition data were recorded immediately. Each section was marked by a metal tag attached to the branch of the trees above each coral. An Onset HOBO pendant temperature/light logger was attached to one tree at each site and recorded temperature data every 2 h to capture daily fluctuations and maximize the length of deployment based on available memory.
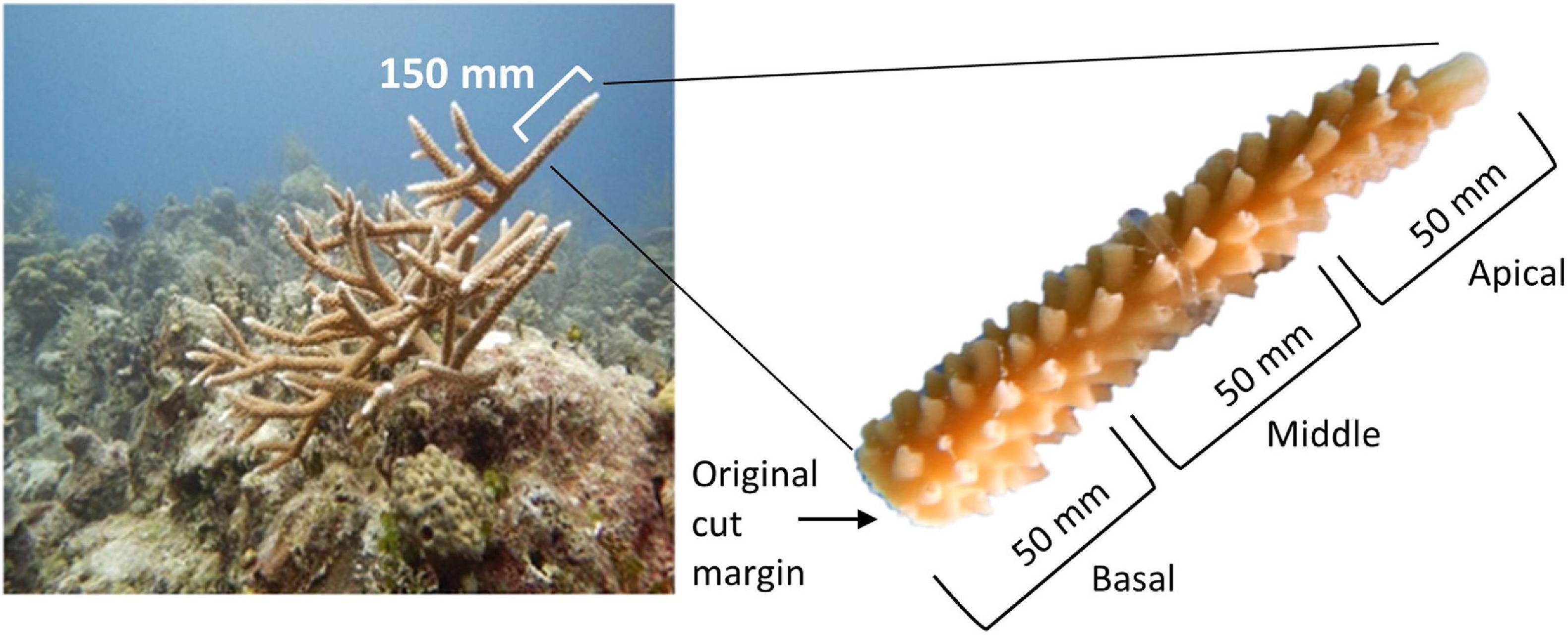
Figure 2. Left: Acropora cervicornis colony showing collection fragment (∼150 mm). Right: Sections of larger 150 mm fragment. Fragment section was designated from the portion of the donor fragment: the first 50 mm (proximal end) were considered the apical fragment section, the next 50 mm were the middle fragment sections, and the interior most 50 mm of the donor fragment were the basal fragment sections.
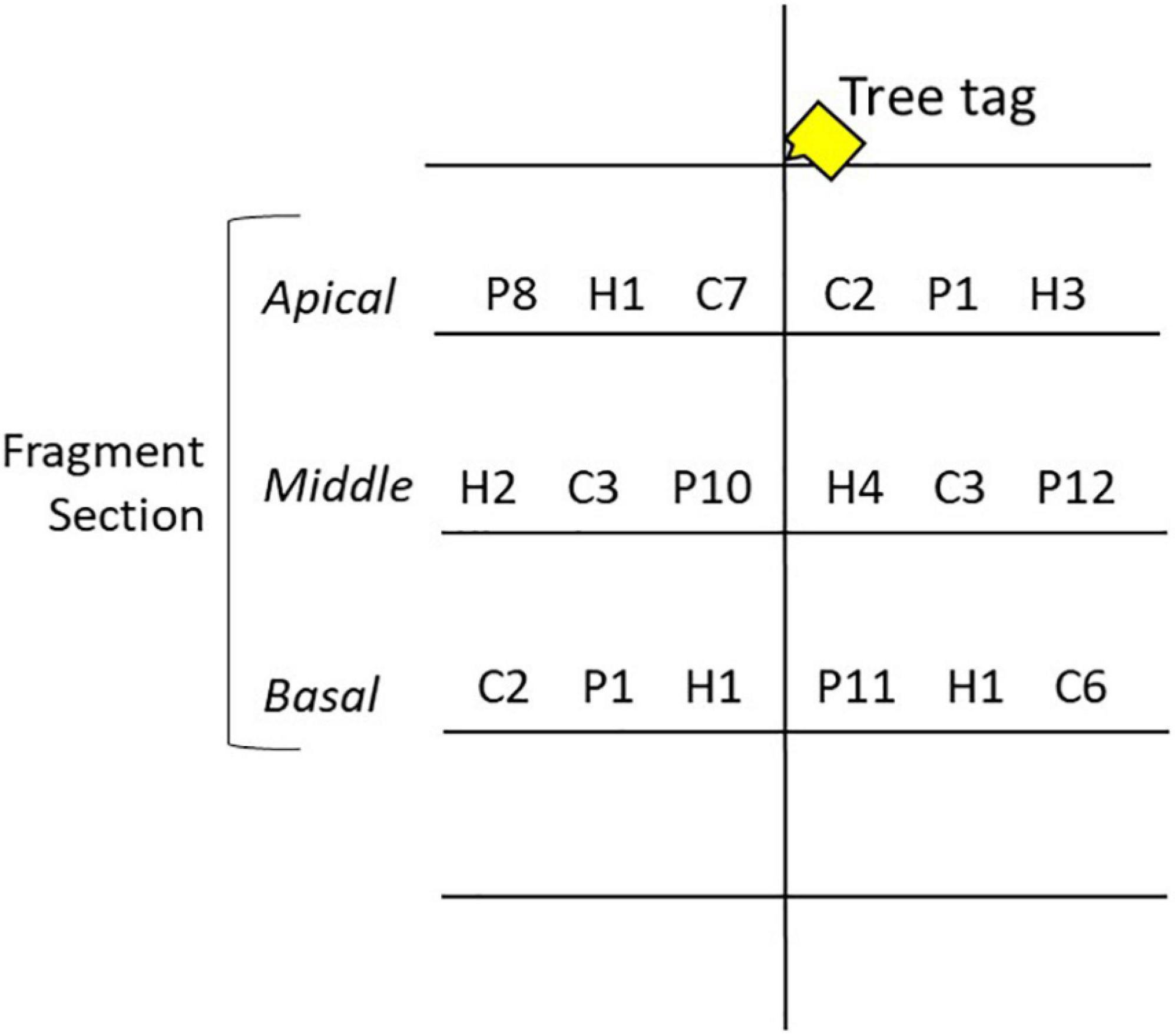
Figure 3. Nursery tree experimental setup. Coral fragments were placed on the middle three tree branches shown here with a letter and number value. Fragment sections were individually tagged. C, P, and H denote taxa [A. cervicornis, A. palmata, and A. prolifera (hybrid), respectively], and numbers denote genotype. The fragment section changed branches between trees at each site. See Supplementary Figure 2 for full experimental design.
Data Collection
Nursery sites were visited monthly between June 2018 and July 2019, during which the trees were cleaned and data were collected. Data included total length, total width, number of branches >10 mm, branch length(s), % mortality, and condition data (presence/absence of disease, predation, bleaching). Size measurements were taken with calipers to the mm. Images were also taken of each fragment with a scale bar. Linear extension was measured in ImageJ if branch measurements could not be completed in the field (ImageJ Version 1.52n, 2018).
Genetic Analysis
Genetic samples were collected from all donor colonies. A small ∼10 mm sample was cut and placed in a ∼1 mL centrifuge tube and filled with 96% molecular grade ethanol. DNA was extracted using magnetic bead protocol, as described in Fogarty et al., 2012. This was followed by PCR amplification using five microsatellite markers (Fogarty et al., 2012 modified from Baums et al., 2005). After fragment analysis (conducted at Florida State University), peaks for each fragment loci were analyzed using GeneMapper 5TM software. Unique and clonal genotypes were identified using the Excel microsatellite toolkit (Park, 2001). To identify descriptive information (stutter peaks, null alleles, large allele dropout), fragment loci were run through Micro-checker Version 2.2.3 (van Oosterhout et al., 2003).
Statistical Analysis
Fragment survival and TLE data were analyzed using R statistical software (R Core Team, 2017). Various survival and growth plots were created through the package ‘ggplot2’ to examine raw data (Wickham, 2016). A Survival Analysis (Cox model) was run to test if the independent variables of taxa, genotype, fragment section, and nursery site affected total colony mortality in the nursery (Therneau and Grambsch, 2000; Therneau, 2015; Kassambara and Kosinski, 2018). In addition to a survival analysis including all months, a survival analysis was run without the first month to test if there were differences in the factors affecting mortality due to collection, transport, and acclimation to the nursery.
For all growth analyses, data was analyzed up to 12 months, due to the loss of most fragments at site N1 at 13 months. For all fragments, linear extension (mm) was calculated as the total length measurement along the main axis plus the length of all branches >10 mm. This was then multiplied by partial survivorship (%) estimates to get Total Linear Extension (TLE) (mm) of live coral tissue. A Kendall’s tau correlation was done to examine if the number of branches correlated to an increase in TLE. To test differences between changes in linear growth for surviving fragments (Growth = final-initial TLE) at 12 months, a Kruskal Wallis rank sum test was conducted, followed by a post hoc pairwise comparison using the Wilcoxon Rank sum test. An ANOVA was also conducted followed by a Tukey’s HSD post hoc test to confirm factor differences.
To model the response of growth over time as a function of the independent categorical variables of taxa, fragment section, and nursery site, a Generalized Additive Mixed Model (GAMM) was run on the TLE data (Wood, 2011). Genotype, temperature, and light intensity were excluded from the models due to low sample size or the addition of a confounding factor to the model. Once fragments had died, they were excluded from dataset at the time in which they had died. Important terms were identified by backward selection (i.e., each term was sequentially dropped from the full model in turn) using Akaike’s Information Criteria (AIC) scores. The final GAMM was then termed the Minimally Adequate Model (MAM) and was used for resulting analysis and post hoc tests. The MAM was validated by visual examination of the model residuals versus fitted values using plot(gam model) and gam.check(gam model) functions. Model validation did not indicate any problems based on residual plots. Pairwise comparisons on factor levels were then conducted using the “emmeans” package (Lenth, 2019).
As A. palmata has a more planar structure compared to A. cervicornis and A. prolifera, an analysis was conducted to compare average growth rate per month. For each surviving A. palmata fragment at 12 months, the total length∗width measurements were multiplied by two and then by partial survivorship (%) estimates to determine live fragment tissue sizes used in the growth rate equation. Width measurements were taken at the widest central point of the fragment, not including branch extensions. Photographic analysis of initial versus final width was completed for a subset of surviving A. palmata fragments (n = 12, minimum of 3 fragments from each site) to determine if width changed significantly during the full 13-month experimental period. Further description of the growth rate analysis and width comparison can be found in the Supplementary Materials.
When comparing values of new linear growth (mm/12 mo) for each fragment between genotypes, a Kruskal Wallis chi-squared test was used. To test differences between genotypes within a taxon, a One-Way ANOVA (parametric) or Kruskal Wallis chi-squared test (non-parametric) was used. If data met parametric assumptions, a Tukey’s test (Tukey HSD) was used in post hoc analysis and visualized using the “multcompView” package (Graves et al., 2015).
To test differences in prevalence of conditions, a Kruskal-Wallis chi-squared test and Pearson’s chi-squared test were used and data was visualized using the “pgirmess” (Giraudoux, 2018) and “vcd” (Zeileis et al., 2007) packages in R Studio. Conditions examined were bleaching (Blch), paling (Pale), and algal overgrowth interactions (OGA). No other conditions (disease/predation) were reported with enough replicates to be used in analysis.
Average daily temperature and average daily light intensity was calculated in Excel using the HOBO® temperature logger data. Loggers were deployed in March 2018, November 2018, and March 2019. While the study period did not include March 2018, this data was included in the light intensity analysis to increase the sample size and document site variability. Daily temperature data was calculated across the whole study period (June 2018–July 2019). Light intensity was measured in lux (lumen/ft2). Photosynthetically active radiation (PAR) is typically used for light measurements, but to convert from lux to PAR a calibration curve and equation must be generated for each individual logger using a PAR meter (Long et al., 2012), which was not available for comparison. Light intensity was recorded throughout the study period, but due to biofouling of the sensor only the first week of light data after logger deployment was used in statistical analysis. Data was organized in Excel, imported into R, and analyzed with a Kruskal Wallis t-test and resulting post hoc analyses to determine whether the nursery sites had significantly different temperatures over the study period.
Results
Survival and Mortality
Of the initial 157 fragments, 66 (42%) survived to the end of the study period (13 months). Of those fragments surviving at 13 months, 28.8% were A. cervicornis fragments, 31.5% were A. palmata fragments, and 39.7% were A. prolifera fragments. During the first month in the nursery (June 2018), overall total mortality was 29.3% (mortality being defined by fragments with no living tissue, not partial tissue loss). After the first month, monthly mortality ranged from 0 to 5% to July 2019. In July 2019, 32 fragments died, equating to 32.7% of the overall remaining fragments. Mortality was greatest at site N1, where only one fragment (A. palmata, basal fragment) was alive by the end of the study period. Site significantly affected coral fragment survival (Survival Analysis Cox model, z = −5.47, p = 4.5e–08), with site N3 fragments showing the greatest survival throughout the study period (Figure 4). No other factors had a significant effect on survival. Site was also the only significant factor in the survival analysis when the first month was excluded (to account for any mortality due to transport stress) (Survival Analysis Cox model, z = −5.161, p = 2.46e–07).
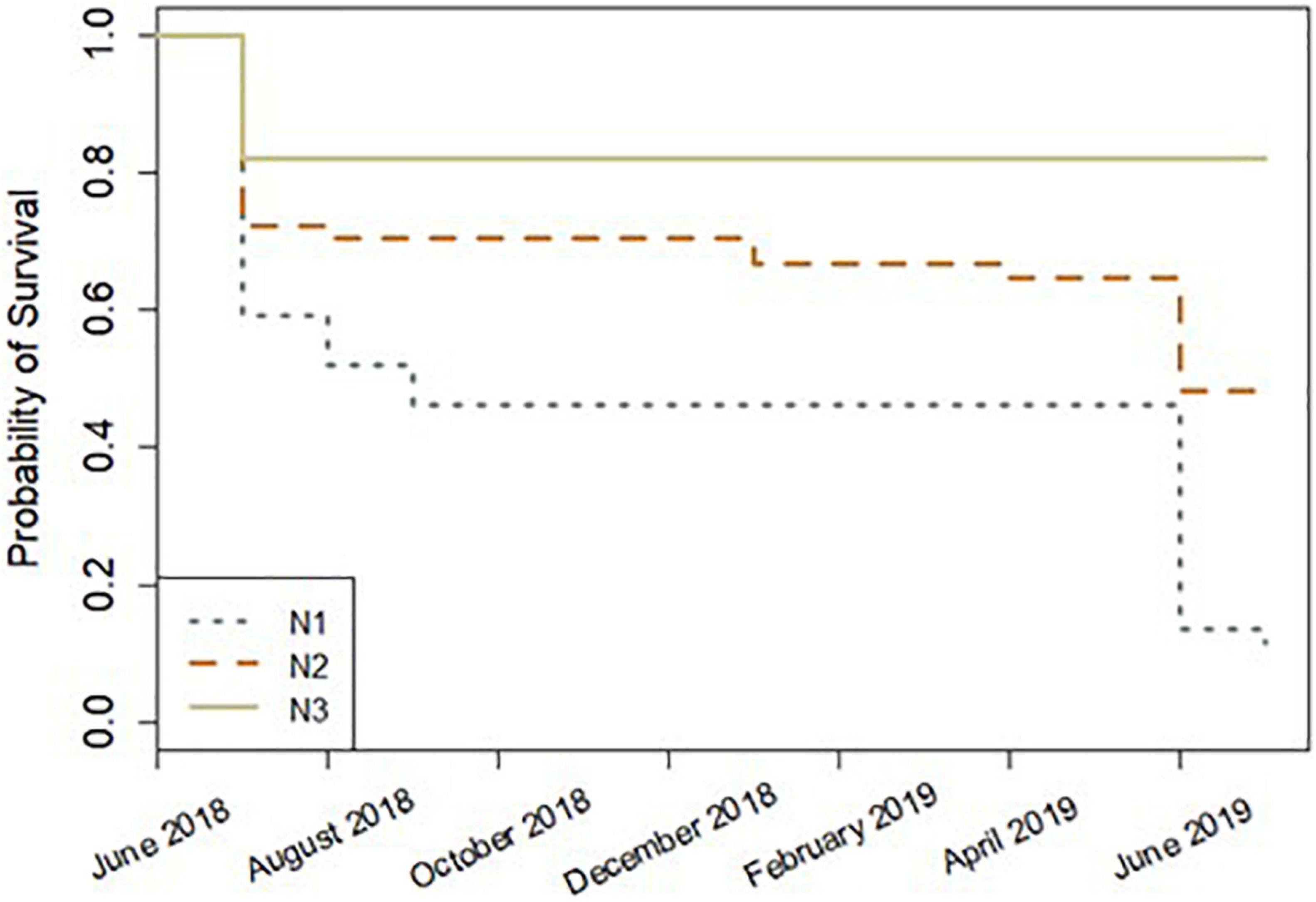
Figure 4. Survival analysis plot by site for 13 months (June 2018–July 2019). Lower survival probability values indicate lower survival, and higher survival probability values indicate greater survival.
Growth
Descriptive Statistics and Linear Growth Analysis
Over 12 months, total TLE (mm) increased by 15.8%. There were no significant differences in the sizes of the ∼50 mm sections at initial nursery setup (Kruskal-Wallis test, p > 0.05). For surviving corals, linear growth (final – initial fragment TLE) and the final number of branches were significantly positively correlated [Kendall’s tau correlation, z(97) = 7.4452, p = 9.678e–14]. The equation that best describes the relationship is: Growth (TLE) = 29.83 + (13.16∗number of branches), where the number of branches changes depending on the individual fragment.
Factors included in the growth analysis based on TLE were taxa, site, and fragment section. When comparing growth (final - initial TLE) for surviving fragments, mean linear growth values did not significantly differ among factor groups (Kruskal Wallis rank sum test, p > 0.05). However, a post hoc comparison indicated A. prolifera had significantly greater average growth (102.5 mm/12 mo ± 14.4 SE), than A. cervicornis (35.6 mm/12 mo ± 7.9 SE) and A. palmata (47.4 mm/12 mo ± 7.2 SE) (Paired Samples Wilcoxon rank sum test, p < 0.05) (Figure 5). Apical fragments had the greatest average growth (78 mm/12 mo ± 4.2 SE), although not significantly different (Paired Samples Wilcoxon rank sum test, p > 0.05) compared to middle fragments (53.3 mm/12 mo ± 4.0 SE) and basal fragments (57.6 mm/12 mo ± 3.6 SE). Site N3 showed greater average growth of fragments (74.1 mm/12 mo ± 4.2 SE) compared to N1 (60.9 mm/12 mo ± 3.0 SE) and N2 (55.2 mm/12 mo ± 4.8 SE), although sites were not significantly different from each other (Paired Samples Wilcoxon rank sum test p < 0.05) (Figure 5). An ANOVA between factor groups suggested a significant effect of taxa on linear growth (Analysis of Variance: Taxa – df = 2, F = 10.344, p = 0.000114). Apical A. prolifera fragments at site N3 had the greatest mean growth based on TLE after 12 months (Figure 5).
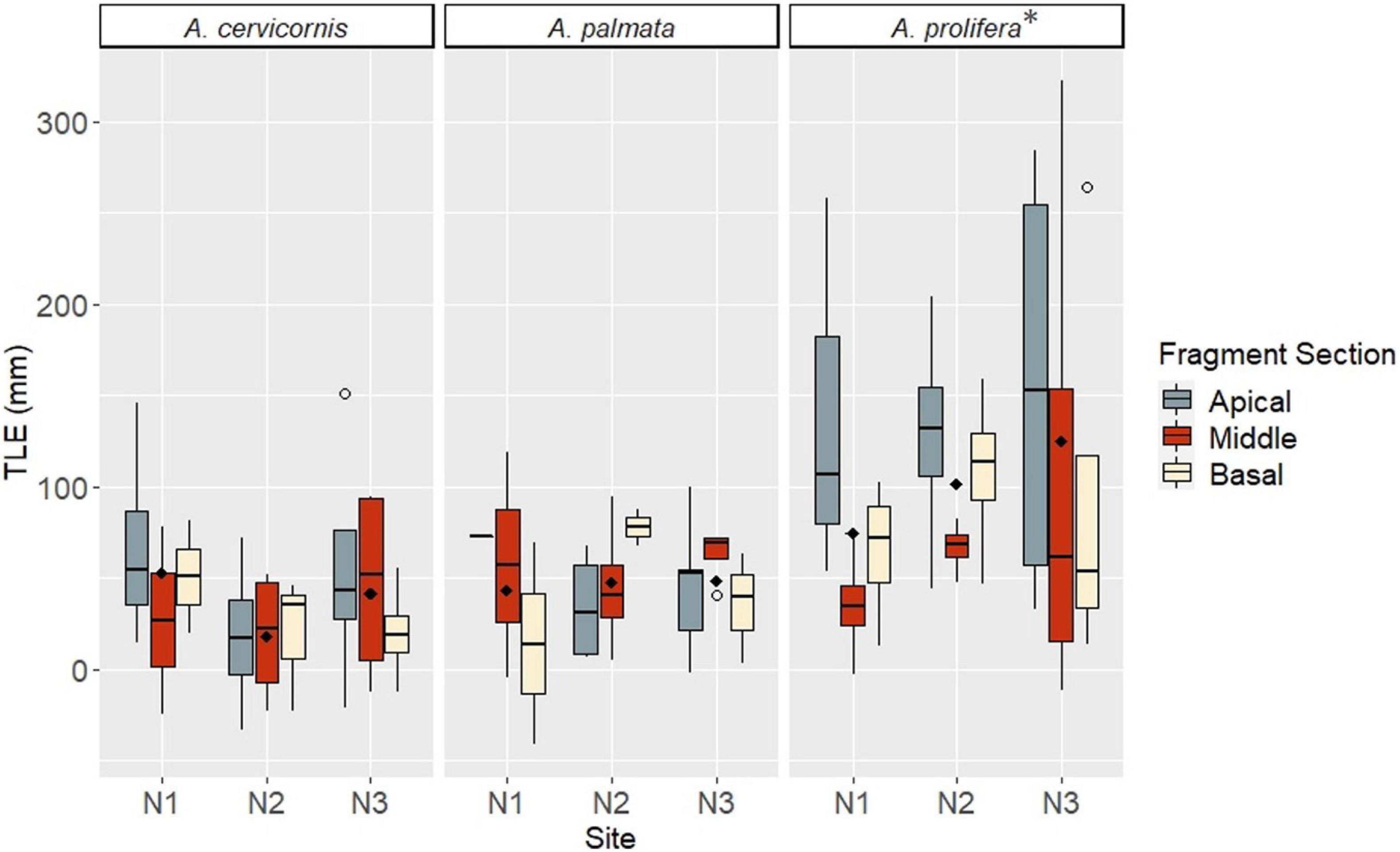
Figure 5. Growth (TLE) by taxa, fragment section, and site at 12 months. Taxa are listed along the top bar of each plot. Site is listed along the x-axis within each taxa group. Fragment sections are differentiated by color in the legend on the right. Open circles indicate outliers; filled diamonds indicate mean values for the site. A * indicates significance.
For the growth rate analysis, apical A. prolifera fragments at site N3 had the greatest average growth rate per month (10.47%) compared to all other factor combinations, and A. prolifera average growth rate per month was significantly greater than the parental species (Paired Samples Wilcoxon rank sum test, p < 0.05). Acropora palmata width measurements found no significant difference between initial and final mean widths (Parametric two-sample t-test, t = 0.154, df = 21.9, p = 0.879). Mean change in width was 3.02 mm and ranged from 0.4 to 9.17 mm, with 90% between 0.4 and 4.58 mm. The Supplementary Materials contain further results of the growth rate and width analyses.
GAMM Analysis
Taxa, site, and fragment section were identified as important factors influencing growth over time (change in TLE, or mm/mo–1) in the MAM (GAMM ANOVA, p < 0.05). The MAM included potential additive and interactive effects of factors that other statistical tests may not account for. To allow for dependency between individual fragments over time, initial size of each fragment was included in the TLE values across all time points.
Acropora prolifera fragments had significantly greater mean TLE values at 12 months compared to A. cervicornis and A. palmata fragments based on the MAM post hoc pairwise comparison (p < 0.05). Acropora prolifera fragments also had the greatest average monthly linear growth across all fragment sections and sites (Figure 6A). Based on the MAM, apical and basal fragment TLE values at 12 months were significantly greater than middle fragments (p < 0.05) (Figure 6B). The MAM indicated apical A. prolifera fragments at site N1 had the greatest TLE (mm) at 12 months (June 2019), before most fragments at N1 died the next month (July 2019). However, 12-month TLE values between sites were not significantly different (p > 0.05) (Figure 6C).
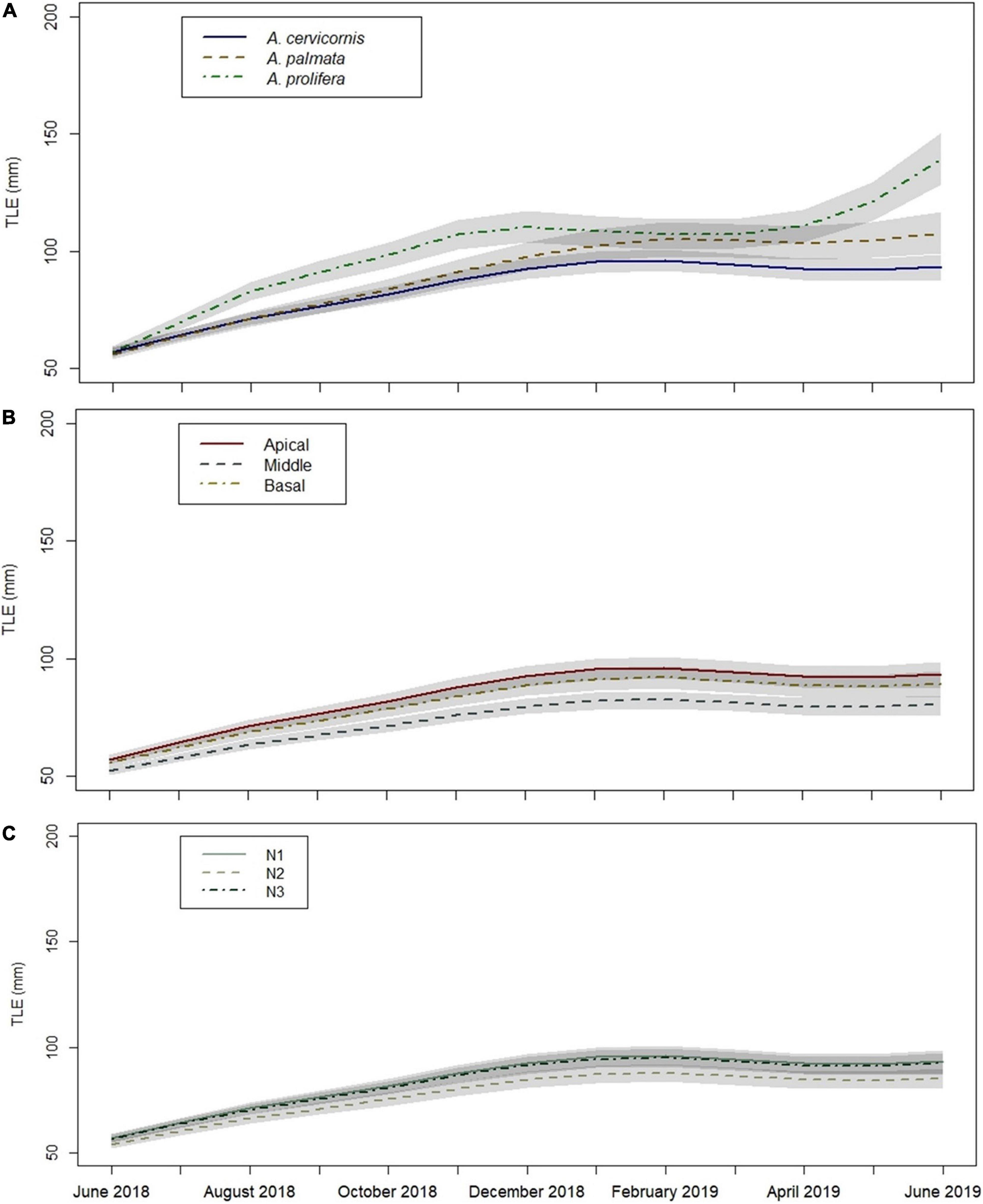
Figure 6. Growth (TLE) over time based on Minimally Adequate Model (MAM) by: (A) taxa, (B) fragment section, and (C) site. Factor variables are given in each figure legend, differentiated by line type and color. Gray shaded areas denote standard error. Site N1 underlies N3 in Figure (C).
Genotype
Genetic analysis confirmed there were five unique A. cervicornis and A. palmata genotypes, and 4 unique A. prolifera genotypes. Micro-checker found no evidence of scoring errors due to stutter peaks, large allele dropout, or null alleles across the five loci for each of the three taxa. Genotype did not have a significant effect on survival (Survival Analysis Cox model, p > 0.05). When analyzing the different genotypes of each taxon individually, genotype had a significant effect on linear growth values between taxa (Kruskal Wallis rank sum test, chi-sq = 33.3, df = 11, p = 0.00048). Genotypes within A. cervicornis were not significantly different from each other (Tukey HSD, p > 0.05). Within A. palmata, the mean linear growth after 12 months in genotype P10 was significantly greater than P1 and P11 (p = 0.021 and p = 0.049, respectively). All other genotypes within A. palmata were not significantly different from each other (Tukey HSD, p > 0.05). Genotype did not have a significant effect on linear growth values within A. prolifera genotypes (chi-sq = 0.72, df = 2, p > 0.05).
Conditions
Disease was not observed during the study period. Condition prevalence did not significantly differ over time (chi-sq = 3.1137, df = 11, p > 0.05). Condition type significantly affected prevalence (chi-sq = 22.28, df = 2, p = 1.453e–5). Prevalence of bleaching was significantly lower than algal overgrowth interactions (OGA) and paling (Multiple comparison test, p < 0.05). There was a significant association between condition and taxa (chi-sq = 16.818, df = 4, p = 0.002097). Prevalence of OGA was less than expected in A. palmata, and prevalence of paling was more than expected in A. palmata; all other combinations of taxa and conditions occurred as expected. There was not a significant association between site and condition (chi-sq = 5.4002, df = 4, p = 0.2486), i.e., all combinations of condition and site occurred as expected.
Temperature and Light Results
During the study period, water temperatures ranged from 21.2°C to 33.5°C across all sites. Water temperatures at N1 ranged from 21.2°C to 33.5°C, at site N2 from 22.5°C to 32.3°C, and at N3 from 21.8°C to 32.9°C. Water temperature did not significantly differ between sites (Kruskal-Wallis test, p > 0.05), although site N1 had the greatest number of days (134) above a published bleaching threshold (29.8°C, Manzello et al., 2007) compared to sites N2 and N3 (123 and 126 days, respectively). Summer months (June–September 2018, June–July 2019) were compared specifically to determine if there were significant temperature differences in times of potentially greater heat stress. In the summer months, water temperatures ranged from 29.01°C to 31.72°C in 2018 and from 27.98°C to 33.45°C in 2019. When comparing just the summer months, there were no significant differences in mean temperatures between sites in 2018 and 2019 (One-way ANOVA/Kruskal-Wallis test, p > 0.05). There were six periods in the summer months where seven or more consecutive days were >29.8°C. Temperature stress based on Degree Heating Weeks (DHW) was calculated, which considers the number of days the sea surface temperature is ≥1°C above the approximate mean summer maximum temperature over a 12-week period (Wellington et al., 2001; Liu et al., 2006; Kayanne, 2017). For this study one DHW is equal to seven days ≥30.8°C. At all sites in July 2018 there were 8 to 9 consecutive days above this threshold, corresponding to 1 DHW. At sites N2 and N3 in June 2019, 7 consecutive days were above the threshold, also corresponding to 1 DHW. In July 2019, all but one coral fragment had died at site N1, likely attributed to bleaching.
Daily light intensity was variable between sites, although the variability was not consistent across logger deployments. At one week after initial logger deployment (March 2018), daily average light intensity ranged from 438.65 to 1434.47 lumen/ft2 across all sites but was not significantly different between sites (Kruskal-Wallis, p > 0.05). In November 2018, daily average light intensity ranged from 145.83 to 459.75 lumen/ft2 across all sites one week post-deployment and was significantly greater at site N2 than N1 and N3 (Kruskal-Wallis rank sum test, chi-sq = 9.719, df = 2, p = 0.00775; Paired Samples Wilcoxon rank sum test, p < 0.05). Sites N1 and N3 did not significantly differ from each other after the second logger deployment (Paired Samples Wilcoxon rank sum test, p > 0.05). At one week after the third logger deployment (March 2019), daily average light intensity ranged from 387.5 to 8987.47 lumen/ft2 across all sites. Daily average light intensity one week after the third deployment was significantly different between sites (Kruskal-Wallis rank sum test, chi-sq = 12.445, df = 2, p = 0.00198), where light intensity was significantly greater at site N3 than sites N1 and N2, and light intensity at site N1 was significantly lower than N2 (Paired Samples Wilcoxon rank sum test, p < 0.05). Site N1 had lower light intensity than N2 and N3 in March 2018 and 2019 and had lower intensity than site N2 in November of 2018.
Discussion
This study is the first to examine differences in survivorship and growth between the parental and hybrid taxa of Caribbean acroporid corals in a nursery setting, along with differences among fragment sections and nursery locations. While there are reservations about using hybrids in coral restoration due to genetic swamping concerns, the benefits of including fast-growing hybrid coral to quickly increase reef structure likely outweighs the potential long-term drawbacks. This study identified three main findings that will be beneficial to restoration management: (1) the hybrid taxon, A. prolifera, demonstrated greater growth in a shallow water nursery setting than the parental species, (2) using non-apical fragments did not compromise survival or growth, and (3) nursery site selection plays an important role in coral fragment survival.
There is growing evidence to suggest the A. prolifera hybrid has similar, if not higher, fitness than the parental species and may be a faster growing taxon overall (Gladfelter et al., 1978; Fogarty, 2012; Howe, 2018; Weil et al., 2020; Nylander-Asplin et al., 2021). This study provides further evidence that A. prolifera grows faster than the parental species. Our results found that the number of branches correlates with increased TLE over time, which is consistent with Lirman et al. (2014) and may explain why the prolifically branching hybrid had greater growth. As seen in other studies (Gladfelter et al., 1978; Crossland, 1981; Scheufen et al., 2017), growth of all taxa fluctuated seasonally and was greater in warmer months than in the cooler winter/spring months. Prior research has investigated the growth of wild acroporid coral colonies, where growth rates were higher in certain A. prolifera genotypes compared to A. cervicornis (Bowden-Kerby, 2008). In contrast, linear growth rates in A. cervicornis were higher than in A. prolifera in Puerto Rico (Weil et al., 2020), suggesting colony growth may be highly dependent on site location, environmental conditions, and genotype. With its rapid growth and prolific branching morphology, the hybrid is likely to reach outplanting goals by quickly increasing coral biomass and reef structure, albeit the fused branches of the hybrid taxon may provide a different ecological service than the parental species. For example, the structure of A. palmata serves as a place for larger fish and invertebrates to live and hide. In contrast, the hybrid’s fused branches are more compact, and may be more beneficial to the smaller fish and invertebrates.
Wild hybrid colonies have been found in greater abundance and in better health than their parental counterparts at some Caribbean sites (Fogarty, 2012; Hernández-Fernández et al., 2019; Nylander-Asplin et al., 2021). In surveys across the Caribbean, hybrid disease prevalence was equivalent to A. palmata and less than A. cervicornis (Fogarty, 2012). Despite hybrids often inhabiting shallower habitats (∼1 m) than the parental species, hybrids had comparable prevalence of paling or bleaching, which was low overall at surveyed sites (Fogarty, 2012). These characteristics, combined with the equal survivorship and rapid growth seen in our study, make the hybrid an ideal candidate to scale-up restoration. Addition of the hybrid could increase reef structure while also increasing the probability of genetic diversity within taxa (Willis et al., 2006; Richards and Hobbs, 2015; Nylander-Asplin et al., 2021). Hybrids could provide shallow water habitat with limited bleaching and paling, fast growth, and potentially less susceptibility to disease compared to one or both parental species, as our work and previous research indicates.
There is concern that the hybrid may outcompete the parental species or reduce genetic diversity if included in restoration practices, as seen in other research in forestry practices (Merkle et al., 2006; Richards and Hobbs, 2015; Kovach et al., 2016). However, concerns about genetic swamping of the parental species on evolutionary scales must not outweigh the immediate ecological need for shallow coral reefs, particularly when the state of coral reefs is dire. Genetics also play an important role in a coral’s resistance to climate change and disease (Baums, 2008; Vollmer and Kline, 2008; Drury et al., 2016, 2017; O’Donnell et al., 2017; Baums et al., 2019; Chan et al., 2018, 2019a,b). With the inclusion of the hybrid, there is potential for greater sharing of genetic material across the three acroporid taxa via backcrossing, which may improve the adaptive potential of coral populations (Baums et al., 2019). Chan et al. (2019a) details a decision tree to address if/when a hybrid should be used in conservation efforts overall. To specifically address A. prolifera concerns, pilot studies could investigate differences in growth and survival of nursery grown coral by outplanting fragments in the same area in separate clusters, with enough separation between colonies to reduce competition between coral taxa. While there is overlap in habitat range among Caribbean acroporid taxa (Fogarty, 2012), further separation by habitat type/depth could help address competition concerns. This could include outplanting A. prolifera to shallow back reef areas, A. palmata along reef crests, and A. cervicornis to deeper reef slopes.
Apical fragments displayed the greatest TLE increase compared to middle and basal fragments, with the apical hybrid fragments having the greatest growth overall. Because these fragments were at the tips of the donor colony and contained the apical polyp, they were the primary location of growth on the original colony (Gladfelter et al., 1989; Rinkevich, 2000; Bowden-Kerby, 2001). This supports the idea that collecting from the tips of donor colonies may lead to a faster rate of growth, while also reducing impact to the donor colonies (Rinkevich, 2000; Bowden-Kerby, 2001; Herlan and Lirman, 2008). Previous studies have demonstrated gradients along A. cervicornis branches, where carbon compound transport was allocated toward the tips of colonies (Taylor, 1977) and respiration was higher in the terminal tips of A. palmata colonies (Gladfelter et al., 1989). The results of these studies indicate that the tips of acroporid colonies are areas of increased growth, where metabolic rates may be greater compared to the rest of the colony (Taylor, 1977; Gladfelter et al., 1989). In this study, there were no significant differences in linear growth by fragment section, which could be accounted for by branching on both cut margins of the middle and basal sections. Some studies have found that pruning of larger colonies of branching corals in a nursery leads to increased productivity after one year (Lirman et al., 2014), and similar exposure in massive corals by microfragmentation has led to a greater increase in tissue compared to singular colony (Page et al., 2018). In contrast, excessive pruning may lead to an increased risk of disease/overgrowth or reduce reproductive capability in the long-term (Epstein et al., 2001; Muller and van Woesik, 2012). Here, middle and basal fragments had two areas of recent exposed tissue from initial fragmentation, which can lead to increased risk of disease and other deleterious stress responses (Muller and van Woesik, 2012). Lesion colonization by opportunistic settlers, like algae, may affect the long-term growth of nursery fragments. In this study, initial algal settlement on the exposed coral skeleton was observed across all sites in the first month of nursery placement before the coral had an opportunity to heal. While no disease was observed on nursery fragments in this study, open or overgrown lesions may have contributed to partial mortality, leading to differences in growth between fragment sections. Further investigation of metabolic and chemical differences within a colony are needed to understand the role fragment section may play in nursery expansion and outplanting.
Site selection has proven to be an important factor in the success of coral nurseries, with temperature, water quality, and depth affecting survival (Shafir et al., 2006; Johnson et al., 2011; Young et al., 2012). The high mortality observed during the first month was likely due to transportation and acclimation stress. Transportation stress is difficult to avoid but can be reduced by multiple water changes and temperature control, if available. Storm and severe weather conditions may increase the risk of impact on nursery sites (Bowden-Kerby, 2001; Young et al., 2012), though previous research indicates that establishing nursery sites in areas with increased water flow may allow for higher survival (Edwards, 2010). Here, the site with the greatest survival and average growth, N3, was located on the unprotected northern side of the island. While site N1 fragment growth did not significantly differ from the other sites, it did have the lowest overall survival. Although we were unable to measure hydrodynamics at the study sites, we did observe stagnant conditions at site N1, likely contributing to the bleaching and subsequent mortality at this site in July 2019. Survival at site N2 was lower than N3 but greater than N1, possibly due to increased water flow from the channel near this nursery location. Temperature and depth were consistent across all sites; therefore, it is likely that other environmental conditions, while not directly measured in this study, influenced survival and growth. Research by Nakamura and van Woesik (2001) demonstrated that branches in Acropora digitifera survived better in increased water flow conditions, even when exposed to higher water temperatures. Similarly, natural colonies and outplants of A. cervicornis had greater survival and abundance in areas with moderate to high water flow (D’Antonio et al., 2016; van Woesik et al., 2020). In this study, the negative impacts of stagnant water and increased water temperatures at all sites during the summer months likely outweighed the benefits of a more protected location. As suggested by Schopmeyer et al. (2017), survivorship < 80% over 12 months after collection may be due to poor nursery locations or genotypic differences. While Schopmeyer et al. (2017) compared A. cervicornis fragments, the same benchmarks could be applied for all taxa used in this study, and as such it is likely that site differences contributed to the less-than-ideal survival.
The light intensity data recorded after the third deployment (March 2019) shows differences between sites, with site N1 having the lowest intensity. Site N1 was located nearest to seagrass beds but in fine sediment compared to the other two nursery locations. It was the site that observationally had the greatest turbidity across the study period, which aligns with the low lux (lumens/ft2) values from the light logger data. However, it is difficult to extrapolate this information further into the summer months. PAR is a useful metric to determine the ideal light intensity for photosynthesizing organisms, such as coral symbionts. Unfortunately, the lux data collected in this study requires direct calibration with a PAR meter, which was not available for comparison. As such, the light intensity data was used only as a secondary indicator of environmental conditions after temperature in this study. Turbid conditions may reduce the impact of irradiance on coral health (Wagner et al., 2010; van Woesik et al., 2012; Morgan et al., 2017; Sully and van Woesik, 2020; van Woesik et al., 2020). Other research has shown connectivity between adjacent seagrass beds and coral reefs via fish species and particulate matter (Dorenbosch et al., 2005; Heck et al., 2008), which could lead to increased food/nutrient supply (and therefore growth) for nursery fragments. However, high sediment input and long-term turbidity can increase prevalence of disease and other stressors to corals, likely impacting long-term growth and survival (Pollock et al., 2014; Ng et al., 2016).
Overall, growth is only a secondary measure of success if nursery fragments do not survive. As such, site selection based on survival alone should be a priority before considering growth. Site selection criteria should consider depth, water temperature, site accessibility, hydrodynamics, and nutrient flux in the area, which could be evaluated using smaller pilot studies. Locations with optimal depth, moderate water flow, adequate light attenuation, and a limited range of temperatures will likely lead to the most successful coral fragment survival and growth (Edwards, 2010; Johnson et al., 2011).
Conclusion
The hybrid coral utilized in this study showed greater fitness than the parental species. Coral restoration managers should consider the fast-growing hybrid A. prolifera as an option for restoration. The hybrid survives as well as and grows faster than the parental species, and as such is a potential option to increase shallow reef infrastructure through restoration. Including the hybrid taxa and increasing the number of unique parental genotypes in a nursery will increase genetic diversity among all three taxa in future restoration activities. As shown in this study, evaluating appropriate nursery sites before setup is crucial to project success. Although apical tips of colonies prove to be a source for fast growing tissue, further research is needed to confirm there are no tradeoffs between growth and survival. Finally, our study took place over the course of one year at a remote island in the Bahamas. Incorporating the hybrid in different aspects of active restoration at sites throughout the Caribbean or at larger scales would help determine how this taxon fits into the larger picture of coral restoration.
Data Availability Statement
The datasets presented in this article are not readily available due to authorization required by the program sponsor. Upon approval, data will be provided by the corresponding author. Requests to access the datasets should be directed to CV, Y2Fzc2llLnZhbnd5bmVuQGdtYWlsLmNvbQ==.
Author Contributions
CV, MH, and NF designed the study. CD obtained permitting for the project. CV, MH, NF, and CD scouted and set up nursery sites. CV, MH, and CD collected corals for the nurseries. MH and CV were responsible for project logistics, data collection, and nursery maintenance, with assistance from NF and DG, and conducted genetic processing and analysis. NF and DG were responsible for project budgeting. CV organized and analyzed the data and wrote the manuscript drafts. CV, MH, NF, and DG contributed to interpretation of results. All authors contributed to final draft edits before submission.
Funding
Financial support was provided by Norwegian Cruise Line® (NF, DG, and CD grant) and the South Florida Chapter of the Explorers Club (CV grant). This research was supported in part by the US National Science Foundation Project OCE-1538469/1929979 (NF grant). Permits were issued to the Perry Institute of Marine Science from the Bahamas Environment, Science, and Technology Division. Norwegian Cruise Line® had initial input on the proposal for this study and various dive staff assisted with nursery maintenance. The funding bodies did not participate in the data analysis, interpretation, or in the writing of this manuscript.
Conflict of Interest
The authors declare that the research was conducted in the absence of any commercial or financial relationships that could be construed as a potential conflict of interest.
Publisher’s Note
All claims expressed in this article are solely those of the authors and do not necessarily represent those of their affiliated organizations, or those of the publisher, the editors and the reviewers. Any product that may be evaluated in this article, or claim that may be made by its manufacturer, is not guaranteed or endorsed by the publisher.
Acknowledgments
We would like to thank all the staff at the NCL Miami office for their support in logistics and approval for this project, including Morgan McCall, the GSC dive team, and the GSC island staff for their assistance with travel, accommodations, and logistics on the island. Thanks also go to Hayley Jo Carr and the staff of the Perry Institute of Marine Science located on New Providence for their assistance with site scouting, coral collections, and transportation assistance. Finally, we thank Rosanna Milligan for assistance with data analysis and Nova Southeastern University’s past and present members of the CRRAM and REEF labs for their assistance with data collection and monitoring on this project, including Megan Bock, Kelly Pitts, Elizabeth McDonald, Hannah Nylander-Asplin, Leah Harper, Alexandra Hiley, Grace Hanson, Nicholas Jones, Nicole Hayes, Alanna Waldman, Ellen Goldenberg, and Shane Wever. Findings from this manuscript were also reported in the final thesis presentation from VanWynen (2020).
Supplementary Material
The Supplementary Material for this article can be found online at: https://www.frontiersin.org/articles/10.3389/fmars.2021.669966/full#supplementary-material
Supplementary Figure 1 | Coral collection locations around New Providence, shown by the yellow points. Inset: New Providence (in box) in relation to Florida and the Berry Islands.
Supplementary Figure 2 | Nursery site experimental setup. Coral fragments were placed on the middle three tree branches shown here with a letter and number value. Fragment sections were individually tagged. C, P, and H denote taxa (A. cervicornis, A. palmata, and A. prolifera (hybrid), respectively), and numbers denote genotype. Not all putative genotypes were unique, and so some genotypes had a higher number of fragments after analysis. The fragment section changed branches between trees at each site. Dashes denote no fragment attached.
Supplementary Figure 3 | Growth (TLE) over time by genotype. TLE (mm) is along the y-axis, and time is along the x-axis. C, P, and H denote A. cervicornis, A. palmata, and A. prolifera (hybrid), respectively.
Supplementary Figure 4 | Frequency analysis of conditions present across the study period by taxa. Conditions are listed along the top x-axis, with taxon listed along the y-axis. Algal overgrowth, bleaching, and paling are denoted by OGA, Blch, and Pale, respectively. Blue boxes indicate that the condition occurred more than expected for a specific taxon, while red indicates the condition occurred less than expected. Gray boxes indicate that a condition occurred as expected for that taxon.
Supplementary Figure 5 | Mean daily temperature by site from June 2018 to July 2019. Purple line denotes published approximate bleaching threshold at 29.8°C (Manzello et al., 2007). Sites are differentiated by color, shown in the legend.
Supplementary Table 1 | Linear growth (TLE) at 12 months by genotype. C, P, and H denote A. cervicornis, A. palmata, and A. prolifera (hybrid), respectively. Genotype with ∗ indicates only 3 fragments left at 12 months.
Supplementary Table 2 | Monthly temperature ranges (°C) from June 2018 to July 2019.
Supplementary Table 3 | GPS coordinates for nursery locations.
References
Arnold, M. L., and Fogarty, N. D. (2009). Reticulate evolution and marine organisms: the final frontier? Int. J. Mol. Sci. 10, 3836–3860. doi: 10.3390/ijms10093836
Aronson, R. B., and Precht, W. F. (1997). Stasis, biological disturbance, and community structure of a Holocene coral reef. Paleobiology 23, 326–346. doi: 10.1017/s0094837300019710
Aronson, R. B., and Precht, W. F. (2001). White-band disease and the changing face of Caribbean coral reefs. Hydrobiologia 460, 25–38. doi: 10.1007/978-94-017-3284-0_2
Aronson, R., Bruckner, A., Moore, J., Precht, B., and Weil, E. (2008a). Acropora cervicornis, Staghorn coral. UK: IUCN Red List of Threatened Species.
Aronson, R., Bruckner, A., Moore, J., Precht, B., and Weil, E. (2008b). Acropora palmata, Elkhorn coral. UK: The IUCN Red List of Threatened Species.
Babcock, R., and Smith, L. (2000). “Effects of sedimentation on coral settlement and survivorship,” in Proceedings of the 9th International Coral Reef Symposium, Bali.
Bak, R. P. M., Neuwland, G., and Meesters, E. H. (2009). Coral growth rates revisited after 31 years: what is causing lower extension rates in Acropora palmata? Bull. Mar. Sci. 84, 287–294.
Baker, A. C., Glynn, P. W., and Riegl, B. (2008). Climate change and coral reef bleaching: an ecological assessment of long-term impacts, recovery trends and future outlook. Estuar. Coast. Shelf Sci. 80, 435–471.
Baums, I. B. (2008). A restoration genetics guide for coral reef conservation. Mol. Ecol. 17, 2796–2811. doi: 10.1111/j.1365-294x.2008.03787.x
Baums, I. B., Baker, A. C., Davies, S. W., Grottoli, A. G., Kenkel, C. D., Kitchen, S. A., et al. (2019). Considerations for maximizing the adaptive potential of restored coral populations in the western Atlantic. Ecol. Appl. 29: e01978.
Baums, I. B., Hughes, C. R., and Hellberg, M. E. (2005). Mendelian microsatellite loci for the Caribbean coral Acropora palmata. Mar. Ecol. Prog. Ser. 288, 115–127. doi: 10.3354/meps288115
Bellwood, D. R., Hughes, T. P., Folke, C., and Nystrom, M. Y. (2004). Confronting the coral reef crisis. Nature 429, 827–833. doi: 10.1038/nature02691
Boström-Einarsson, L., Babcock, R. C., Bayraktarov, E., Ceccarelli, D., Cook, N., Ferse, S. C. A., et al. (2020). Coral restoration – A systematic review of current methods, successes, failures and future directions. PLoS One 15:e0226631. doi: 10.1371/journal.pone.0226631
Bowden-Kerby, A. (2001). Low-tech coral reef restoration methods modeled after fragmentation process. Bull. Mar. Sci. 69, 915–931.
Bowden-Kerby, A. (2008). “Restoration of threatened Acropora cervicornis corals: intraspecific variation as a factor in mortality, growth, and self-attachment,” in Proceedings of the 11th International Coral Reef Symposium, Fort Lauderdale, FL.
Bruckner, A. W. (2002). Proceedings of the Caribbean Acropora Workshop: Potential Application of the U.S. Endangerd Species Act as a Conservation Strategy. Silver Spring, MD: NOAA Technical Memorandum.
Cesar, H., Burke, L., and Pet-Soede, L. (2003). The Economics of Worldwide Coral Reef Degradation. Arnhem: CEEC
Chan, W. Y., Hoffman, A. A., and van Oppen, M. J. H. (2019a). Hybridization as a conservation management tool. Conserv. Lett. 12:e12652.
Chan, W. Y., Peplow, L. M., and van Oppen, M. J. H. (2019b). Interspecific gamete compatibility and hybrid larval fitness in reef-building corals: implications for coral reef restoration. Sci. Rep. 9:4757.
Chan, W. Y., Peplow, L. M., Menéndez, P., Hoffman, A. A., and van Oppen, M. J. H. (2018). Interspecific hybridization may provide novel opportunities for coral reef restoration. Front. Mar. Sci. 5:160.
Cheal, A. J., MacNeil, M. A., Emslie, M. J., and Sweatman, H. (2017). The threat to coral reefs from more intense cyclones under climate change. Glob. Chang. Biol. 23, 1511–1524. doi: 10.1111/gcb.13593
Cinner, J. E., Pratchett, M. S., Graham, N. A. J., Messmer, V., Fuentes, M. M. P. B., and Ainsworth, T. (2015). A framework for understanding climate change impacts on coral reef social–ecological systems. Reg. Environ. Change 16, 1133–1146.
Crossland, C. J. (1981). “Seasonal growth of Acropora cf. Formosa and Pocillopora damicornis on a high latitude reef (Houtman Abrolhos, Western Australia),” in Proceedings of the Fourth International Coral Reef Symposium, Philippines.
D’Antonio, N. L., Gilliam, D. S., and Walker, B. K. (2016). Investigating the spatial distribution and effects of nearshore topography on Acropora cervicornis abundance in Southeast Florida. PeerJ 4:e2473. doi: 10.7717/peerj.2473
Derraik, J. G. B. (2002). The pollution of the marine environment by plastic debris: a review. Mar. Pollut. Bull. 44, 842–852. doi: 10.1016/s0025-326x(02)00220-5
Dorenbosch, M., Grol, M. G. G., Christianen, M. J. A., Nagelkerken, I., and van der Velde, G. (2005). Indo-Pacific seagrass beds and mangroves contribute to fish density and diversity on adjacent coral reefs. Mar. Ecol. Prog. Ser. 302, 63–76. doi: 10.3354/meps302063
Douglas, A. E. (2003). Coral bleaching—-how and why? Mar. Pollut. Bull. 46, 385–392. doi: 10.1016/s0025-326x(03)00037-7
Drury, C., Dale, K. E., Panlilio, J. M., Miller, S. V., Lirman, D., Larson, E. A., et al. (2016). Genomic variation among populations of threatened coral: Acropora cervicornis. BMC Genom. 17:286.
Drury, C., Manzello, D., and Lirman, D. (2017). Genotype and local environment dynamically influence growth, disturbance response and survivorship in the threatened coral, Acropora cervicornis. PLoS One 12:e0174000. doi: 10.1371/journal.pone.0174000
Eakin, C. M., Morgan, J. A., Heron, S. F., Smith, T. B., Liu, G., Alvarez-Filip, L., et al. (2010). Caribbean corals in crisis: record thermal stress, bleaching, and mortality in 2005. PLoS One 5:e13969.
Edwards, A. J. (2010). Reef Rehabilitation Manual. Australia: Coral Reef Targeted Research and Capacity Building for Management Program.
Epstein, N., Bak, R. P. M., and Rinkevich, B. (2001). Strategies for gardening denuded coral reef areas: the applicability of using different types of coral material for reef restoration. Restor. Ecol. 9, 432–442. doi: 10.1046/j.1526-100X.2001.94012.x
Ferrario, F., Beck, M. W., Storlazzi, C. D., Micheli, F., Shepard, C. C., and Airoldi, L. (2014). The effectiveness of coral reefs for coastal hazard risk reduction and adaptation. Nat. Commun. 5:3794.
Fogarty, N. D. (2010). Reproductive Isolation and Hybridization Dynamics in Threatened Caribbean Acroporid Corals. Ph.D. Thesis, Florida State University: College of Arts and Sciences.
Fogarty, N. D. (2012). Caribbean acroporid coral hybrids are viable across life history stages. Mar. Ecol. Prog. Ser. 446, 145–159. doi: 10.3354/meps09469
Fogarty, N. D., Vollmer, S. V., and Levitan, D. R. (2012). Weak prezygotic isolating mechanisms in threatened Caribbean Acropora corals. PLoS One 7:e30486. doi: 10.1371/journal.pone.0030486
Fox, H., and Caldwell, R. (2006). Recovery from blast fishing on coral reefs: a tale of two scales. Ecol. Appl. 16, 1631–1635. doi: 10.1890/1051-0761(2006)016[1631:rfbfoc]2.0.co;2
Gardner, T. A., Côté, I. M., Gill, J. A., Grant, A., and Watkinson, A. R. (2003). Long-term region-wide declines in caribbean corals. Science 301, 958–960. doi: 10.1126/science.1086050
Giraudoux, P. (2018). pgirmess: Spatial Analysis and Data Mining for Field Ecologists. R package. version 1.6.9.
Gladfelter, E. H., Michel, G., and Sanfelici, A. (1989). Metabolic gradients along a branch of the reef coral Acropora palmata. Bull. Mar. Sci. 44, 1166–1173.
Gladfelter, E. H., Monahan, R. K., and Gladfelter, W. B. (1978). Growth rates of five reef-building corals in the northeastern Caribbean. Bull. Mar. Sci. 28, 728–734.
Graves, S., Piepho, H. P., Selzer, L., and Dorai-Raj, S. (2015). multcompView: Visualizations of Paired Comparisons. R package version 0.1-7.
Griffin, S., Spathias, H., Moore, T. D., Baums, I., and Griffin, B. A. (2012). “Scaling up Acropora nurseries in the Caribbean and improving techniques,” in Proceedings of the 12th International Coral Reef Symposium, Australia.
Harley, C. D. G., Hughes, A. R., Hultgren, K. M., Miner, B. G., Sorte, C. J. B., Thornber, C. S., et al. (2006). The impacts of climate change in coastal marine systems. Ecol. Lett. 9, 228–241. doi: 10.1111/j.1461-0248.2005.00871.x
Harris, J. A., Hobbs, R. J., Higgs, E., and Aronson, J. (2006). Ecological restoration and global climate change. Restor. Ecol. 14, 170–176.
Harvell, D., Jordan-Dahlgren, D., Merkel, S., Rosenberg, D., Raymundo, L., Smith, G., et al. (2007). Coral disease, environmental drivers, and the balance between coral and microbial associates. Oceanography 20, 172–195. doi: 10.5670/oceanog.2007.91
Heck, K. L., Carruthers, T. J. B., Duarte, C. M., Hughes, A. R., Kendrick, G., Orth, R. J., et al. (2008). Trophic transfers from seagrass meadows subsidize diverse marine and terrestrial consumers. Ecosystem 11, 1198–1210. doi: 10.1007/s10021-008-9155-y
Heller, N. E., and Zavaleta, E. S. (2009). Biodiversity management in the face of climate change: a review of 22 years of recommendations. Biol. Conserv. 142, 14–32. doi: 10.1016/j.biocon.2008.10.006
Herlan, J., and Lirman, D. (2008). “Development of a coral nursery program for the threatened coral Acropora cervicornis in Florida,” in Proceedings of the 11th International Coral Reef Symposium, Ft. Lauderdale, FL.
Hernández-Fernández, L., González, de Zayas, R., Olivera, Y. M., Pina Amargós, F., Bustamante López, C., et al. (2019). Distribution and status of living colonies of Acropora spp. in the reef crests of a protected marine area of the Caribbean (Jardines de la Reina National Park, Cuba). PeerJ 7:e6470. doi: 10.7717/peerj.6470
Heron, S. F., Maynard, J. A., van Hooidonk, R., and Eakin, C. M. (2016). Warming trends and bleaching stress of the world’s coral reefs 1985-2012. Sci. Rep. 6:38402.
Holbrook, S. J., Schmitt, R. J., Messmer, V., Brooks, A. J., Srinivasan, M., Munday, P. L., et al. (2015). Reef fishes in biodiversity hotspots are at greatest risk from loss of coral species. PLoS One 10:e0124054. doi: 10.1371/journal.pone.0124054
Howe, C. N. (2018). The Acclimatization of the Caribbean Fused Staghorn Coral Acropora Prolifera to Non-natal Locations. master’s thesis. Virgin Islands: University of the Virgin Islands.
Hughes, T. P. (1994). Catastrophes, phase shifts, and large-scale degradation of a Caribbean coral reef. Science 265, 1547–1551. doi: 10.1126/science.265.5178.1547
Hughes, T. P., and Connell, J. H. (1999). Multiple stressors on coral reefs: a long-term perspective. Limnol. Oceanogr. 44, 932–940. doi: 10.4319/lo.1999.44.3_part_2.0932
Hughes, T. P., Anderson, K. D., Connolly, S. R., Heron, S. F., Kerry, J. T., Lough, J. M., et al. (2018). Spatial and temporal patterns of mass bleaching of corals in the Anthropocene. Science 359, 80–83. doi: 10.1126/science.aan8048
Hughes, T. P., Barnes, M. L., Bellwood, D. R., Cinner, J. E., Cumming, G. S., Jackson, J. B. C., et al. (2017a). Coral reefs in the Anthropocene. Nature 546, 82–90.
Hughes, T. P., Keller, B. D., Jackson, J. B. C., and Boyle, M. J. (1985). Mass mortality of the echinoid Diadema antillarium Phillipi in Jamaica. Bull. Mar. Sci. 36, 377–384.
Hughes, T. P., Kerry, J. T., Alvarez-Noriega, M., Alvarez-Romero, J. G., Anderson, K. D., Baird, A. H., et al. (2017b). Global warming and recurrent mass bleaching of corals. Nature 543, 373–377.
Irwin, A., Greer, L., Humson, R., Devlin-Durante, M., Cabe, P., Lescinsky, H., et al. (2017). Age and intraspecific diversity of resilient Acropora communities in Belize. Coral Reefs 36, 1111–1120. doi: 10.1007/s00338-017-1602-9
Jackson, J., Donovan, M., Cramer, K., and Lam, V. (2014). Status and Trends of Caribbean Coral Reefs 1970-2012. Switzerland: Global Coral Reef Monitoring Network, IUCN.
Jackson, S. T., and Hobbs, R. J. (2009). Ecological restoration in the light of ecological history. Science 325, 567–569. doi: 10.1126/science.1172977
Japaud, A., Fauvelot, C., and Bouchon, C. (2014). Unexpected high densities of the hybrid coral Acropora prolifera (Lamarck 1816) in Guadeloupe Island, Lesser Antilles. Coral Reefs Springer Verlag. 33, 593–593. doi: 10.1007/s00338-014-1169-7
Johnson, M., Lustic, C., Bartels, E., Baums, I., Gilliam, D., Larson, L., et al. (2011). Caribbean Acropora Restoration Guide: Best Practices for Propagation and Population Enhancement. Arlington, VA: The Nature Conservancy.
Jones, N. P., Figueiredo, J., and Gilliam, D. S. (2020). Thermal stress-related spatiotemporal variations in high-latitude coral reef benthic communities. Coral Reefs 39, 1661–1673. doi: 10.1007/s00338-020-01994-8
Kassambara, A., and Kosinski, M. (2018). survminer: Drawing Survival Curves using ‘ggplot2. Available Online at: https://CRAN.R-project.org/package=survminer.
Kayanne, H. (2017). Validation of degree heating weeks as a coral bleaching index in the northwestern Pacific. Coral Reefs 36, 63–70. doi: 10.1007/s00338-016-1524-y
Kitchen, S. A., Osborne, C. C., Fogarty, N. D., and Baums, I. B. (2021). Morphotype is not linked to mitochondrial haplogroups of Caribbean acroporid hybrids. Coral Reefs 1–8. doi: 10.1007/s00338-021-02135-5
Kitchen, S. A., Ratan, A., Bedoya-Reina, O. C., Burhans, R., Fogarty, N. D., Miller, W., et al. (2019). Genomic variants among threatened acropora corals. G3 9, 1633–1646. doi: 10.1534/g3.119.400125
Knowlton, N., and Jackson, J. B. C. (2008). Shifting baselines, local impacts, and global change on coral reefs. PLoS Biol. 6:e54. doi: 10.1371/journal.pbio.0060054
Kovach, R. P., Luikart, G., Lowe, W. H., Boyer, M. C., and Muhlfeld, C. C. (2016). Risk and efficacy of human-enabled interspecific hybridization for climate-change adaptation: response to Hamilton and Miller. Conserv. Biol. 30, 428–430. doi: 10.1111/cobi.12678
Lenth, R. (2019). emmeans: Estimated Marginal Means, Aka Least-Squares Means. R package version 1.4.
Lirman, D. (2000). Fragmentation in the branching coral Acropora palmata (Lamarck): growth, survivorship, and reproduction of colonies and fragments. J. Exp. Mar. Biol. Ecol. 251, 41–57. doi: 10.1016/s0022-0981(00)00205-7
Lirman, D. (2001). Competition between macroalgae and corals: effects of herbivore exclusion and increased algal biomass on coral survivorship and growth. Coral Reefs 19, 392–399. doi: 10.1007/s003380000125
Lirman, D., and Fong, P. (1997). Patterns of damage to the branching coral Acropora palmata following Hurricane Andrew: damage and survivorship of hurricane-generated asexual recruits. J. Coast. Res. 13, 67–72.
Lirman, D., Schopmeyer, S., Galvan, V., Drury, C., Baker, A. C., and Baums, I. B. (2014). Growth dynamics of the threatened Caribbean staghorn coral Acropora cervicornis: influence of host genotype, symbiont identity, colony size, and environmental setting. PLoS One 9:e107253. doi: 10.1371/journal.pone.0107253
Lirman, D., Thyberg, T., Herlan, J., Hill, C., Young-Lahiff, C., Schopmeyer, S., et al. (2010). Propagation of the threatened staghorn coral Acropora cervicornis: methods to minimize the impacts of fragment collection and maximize production. Coral Reefs 29, 729–735. doi: 10.1007/s00338-010-0621-6
Liu, G., Strong, A. E., Skirving, W., and Arzayus, L. F. (2006). “Overview of NOAA coral reef watch program’s near-real time satellite global coral bleaching monitoring activities,” in Proceedings of the 10th International Coral Reef Symposium, Gurugram.
Long, M. H., Rheuban, J. E., Berg, P., and Zieman, J. C. (2012). A comparison and correction of light intensity loggers to photosynthetically active radiation sensors. Limnol. Oceanogr. Methods 10, 416–424. doi: 10.4319/lom.2012.10.416
Malhi, Y., Roberts, J. T., Betts, R. A., Killeen, T. J., Li, W., and Nobre, C. A. (2008). Climate change, deforestation, and the state of the Amazon. Science 319, 169–172.
Manzello, D. P., Berkelmans, R., and Hendee, J. C. (2007). Coral bleaching indices and thresholds for the Florida reef tract, Bahamas, and St. Croix, US Virgin Islands. Mar. Pollut. Bull. 54, 1923–1931. doi: 10.1016/j.marpolbul.2007.08.009
Maragos, J. E., Crosby, M. P., and McManus, J. W. (1996). Coral reefs and biodiversity: a critical and threatened relationships. Oceanography 9, 93–99.
McNeill, D. F., Budd, A. F., and Borne, F. P. (1997). Earlier (late Pliocene) first appearance of the Caribbean reef-building coral Acropora palmata: stratigraphic and evolutionary implications. Geology 25, 891–894. doi: 10.1130/0091-7613(1997)025<0891:elpfao>2.3.co;2
Merkle, S. A., Andrade, G. M., Nairn, C. J., Powell, W. A., and Maynard, C. A. (2006). Restoration of threatened species: a noble cause for transgenic trees. Tree Genet. Genomes 3, 111–118. doi: 10.1007/s11295-006-0050-4
Miller, D. J., and van Oppen, M. J. H. (2003). A ‘fair go’ for coral hybridization. Mol. Ecol. 12, 805–807. doi: 10.1046/j.1365-294x.2003.01808.x
Moberg, F., and Folke, C. (1999). Ecological goods and services of coral reef ecosystems. Ecol. Econ. 29, 215–233. doi: 10.1016/s0921-8009(99)00009-9
Morgan, K. M., Perry, C. T., Johnson, J. A., and Smithers, S. G. (2017). Nearshore turbid-zone corals exhibit high bleaching tolerance on the great barrier reef following the 2016 ocean warming event. Front. Mar. Sci. 4:224.
Muller, E. M., and van Woesik, R. (2012). Caribbean coral diseases: primary transmission or secondary infection? Glob. Change Biol. 18, 3529–3535. doi: 10.1111/gcb.12019
Muller, E. M., Rogers, C. S., Spitzack, A. S., and van Woesik, R. (2007). Bleaching increases likelihood of disease on Acropora palmata (Lamarck) in Hawksnest Bay, St John, US Virgin Islands. Coral Reefs 27, 191–195. doi: 10.1007/s00338-007-0310-2
Nakamura, T. V., and van Woesik, R. (2001). Water-flow rates and passive diffusion partially explain differential survival of corals during the 1998 bleaching event. Mar. Ecol. Prog. Ser. 212, 301–304. doi: 10.3354/meps212301
National Marine Fisheries Service (2006). Endangered and Threatened Species: Final Listing Determinations for Elkhorn Coral and Staghorn Coral. Seattle, WA: Amazon Digital Services LLC.
Nedimyer, K., Gaines, K., and Roach, S. (2011). Coral Tree Nursery© : an innovative approach to growing corals in an ocean-based field nursery. Int. J. Bioflux Soc. 4, 442–446.
Neigel, J. E., and Avise, J. C. (1983). Clonal diversity and population structure in a reef-building coral, Acropora cervicornis: self-recognition analysis and demographic interpretation. Int. J. Organic Evol. 37, 437–453. doi: 10.2307/2408259
Ng, C. S. L., Toh, T. C., and Chou, L. M. (2016). Coral restoration in Singapore’s sediment-challenged sea. Reg. Stud. Mar. Sci. 8, 422–429. doi: 10.1016/j.rsma.2016.05.005
Nylander-Asplin, H. F., Hill, R. L., Doerr, J. C., Greer, L., and Fogarty, N. D. (2021). Population dynamics and genotypic richness of the threatened Acropora spp. and their hybrid in the U.S. Virgin Islands. Coral Reefs 40, 965–971. doi: 10.1007/s00338-021-02093-y
O’Donnell, K. E., Lohr, K. E., Bartels, E., and Patterson, J. T. (2017). Evaluation of staghorn coral (Acropora cervicornis, Lamarck 1816) production techniques in an ocean-based nursery with consideration of coral genotype. J. Exp. Mar. Biol. Ecol. 487, 53–58. doi: 10.1016/j.jembe.2016.11.013
Ortiz-Barrientos, D., Counterman, B. A., and Noor, M. A. (2007). Gene expression divergence and the origin of hybrid dysfunctions. Genetica 129, 71–81. doi: 10.1007/s10709-006-0034-1
Page, C. A., Muller, E. M., and Vaughan, D. E. (2018). Microfragmenting for the successful restoration of slow growing massive corals. Ecol. Eng. 123, 86–94. doi: 10.1016/j.ecoleng.2018.08.017
Pandolfi, J. M., Connolly, S. R., Marshall, D. J., and Cohen, A. L. (2011). Projecting coral reef futures under global warming and ocean acidification. Science 333, 418–422. doi: 10.1126/science.1204794
Pollock, F. J., Lamb, J. B., Field, S. N., Heron, S. F., Schaffelke, B., Shedrawi, G., et al. (2014). Sediment and turbidity associated with offshore dredging increase coral disease prevalence on nearby reefs. PLoS One 9:e102498. doi: 10.1371/journal.pone.0102498
R Core Team (2017). R: a Language and Environment for Statistical Computing. Vienna: R Foundation for Statistical Computing.
Reaka-Kudla, M. L. (1997). The global biodiversity of coral reefs: a comparison with rain forests. in Biodiversity II: Understanding and Protecting our Biological Resources, eds E. O. Wilson, D. E. Wilson, and M. L. Reaka-kudla. Washington, DC: Joseph Henry Press
Richards, Z. T., and Hobbs, J. A. (2015). Hybridisation on coral reefs and the conservation of evolutionary novelty. Curr. Zool. 61, 132–145. doi: 10.1093/czoolo/61.1.132
Rinkevich, B. (1995). Restoration strategies for coral reefs damaged by recreational activities: the use of sexual and asexual recruits. Restor. Ecol. 3, 241–251. doi: 10.1111/j.1526-100x.1995.tb00091.x
Rinkevich, B. (2000). Steps towards the evaluation of coral reef restoration by using small branch fragments. Mar. Biol. 136, 807–812. doi: 10.1007/s002270000293
Scheufen, T., Krämer, W. E., Iglesias-Prieto, R., and Enríquez, S. (2017). Seasonal variation modulates coral sensibility to heat-stress and explains annual changes in coral productivity. Sci. Rep. 7:4937.
Schopmeyer, S. A., Lirman, D., Bartels, E., Gilliam, D. S., Goergen, E. A., Griffin, S. P., et al. (2017). Regional restoration benchmarks for Acropora cervicornis. Coral Reefs 36, 1047–1057. doi: 10.1007/s00338-017-1596-3
Sebens, K. P. (1994). Biodiversity of coral reefs: What are we losing and why? Am. Zool. 34, 115–133. doi: 10.1093/icb/34.1.115
Shafir, S., Van Rijn, J., and Rinkevich, B. (2006). Steps in the construction of underwater coral nursery, an essential component in reef restoration acts. Mar. Biol. 149, 679–687. doi: 10.1007/s00227-005-0236-6
Shahidul Islam, M., and Tanaka, M. (2004). Impacts of pollution on coastal and marine ecosystems including coastal and marine fisheries and approach for management: a review and synthesis. Mar. Pollut. Bull. 48, 624–649. doi: 10.1016/j.marpolbul.2003.12.004
Smith, L. D., and Hughes, T. P. (1999). An experimental assessment of survival, re-attachment and fecundity of coral fragments. J. Exp. Mar Biol. Ecol. 235, 147–164. doi: 10.1016/s0022-0981(98)00178-6
Smith, S. J., Edmonds, J., Hartin, C. A., Mundra, A., and Calvin, K. (2015). Near-term acceleration in the rate of temperature change. Nat. Clim. Chang. 5, 333–336. doi: 10.1038/nclimate2552
Storlazzi, C. D., Reguero, B. G., Cole, A. D., Lowe, E., Shope, J. B., Gibbs, A. E., et al. (2019). Rigorously Valuing the Role of U.S. Coral Reefs in Coastal Hazard Risk Reduction: Open File Report. 2019-1027. Virginia: USGS
Sully, S., and van Woesik, R. (2020). Turbid reefs moderate coral bleaching under climate-related temperature stress. Glob. Change Biol. 26, 1367–1373. doi: 10.1111/gcb.14948
Szmant, A. M. (1986). Reproductive ecology of Caribbean reef corals. Coral Reefs 5, 43–54. doi: 10.1007/bf00302170
Taylor, D. L. (1977). “Intra-colonial transport of organic compounds and calcium in some Atlantic reef corals,” in Proceedings of the Third Internation Coral Reef Symposium, Miami, FL.
Therneau, T. M., and Grambsch, P. M. (2000). _Modeling Survival Data: Extending the Cox Model. New York: Springer.
van Oosterhout, C., Hutchinson, W. F., Wills, D. P. M., and Shipley, P. F. (2003). Micro-Checker Version 2.2.3. Hull, UK: University of Hull.
van Oppen, M. J. H., Willis, B. L., Vugt, H. V., and Miller, D. J. (2000). Examination of species boundaries in the Acropora cervicornis group (Scleractinia, Cnidaria) using nuclear DNA sequence analyses. Mol. Ecol. 9, 1363–1373. doi: 10.1046/j.1365-294x.2000.01010.x
van Woesik, R., Houk, P., Isechal, A. L., Idechong, J. W., Victor, S., and Golbuu, Y. (2012). Climate-change refugia in the sheltered bays of Palau: analogs of future reefs. Ecol. Evol. 2, 2474–2484. doi: 10.1002/ece3.363
van Woesik, R., Roth, L. M., Brown, E. J., McCaffrey, K. R., and Roth, J. R. (2020). Niche space of corals along the Florida reef tract. PLoS One 15:e0231104. doi: 10.1371/journal.pone.0231104
VanWynen, C. M. (2020). An Investigation into the Factors Influencing Growth and Survival of Caribbean Acroporid Corals in a Floating Nursery. master’s thesis. Dania, FL: Nova Southeastern University.
Vargas-Angel, B., and Thomas, J. D. (2002). Sexual reproduction of Acropora cervicornis in nearshore waters off Fort Lauderdale, Florida, USA. Coral Reefs 21, 25–26. doi: 10.1007/s00338-001-0208-3
Veron, J. E. N. (1995). Corals in Space and Time: the Biogeography and Evolution of the Scleractinia. Ithaca, NY: Cornell University Press.
Vitousek, P. M. (1994). Beyond global warming: ecology and global change. Ecology 75, 1861–1876. doi: 10.2307/1941591
Vollmer, S. V., and Kline, D. I. (2008). Natural disease resistance in threatened staghorn corals. PLoS One 3:e3718. doi: 10.1371/journal.pone.0003718
Vollmer, S. V., and Palumbi, S. R. (2002). Hybridization and the evolution of reef coral diversity. Science 296, 2023–2025. doi: 10.1126/science.1069524
Voss, J. D., and Richardson, L. L. (2006). Coral diseases near lee stocking Island, Bahamas: patterns and potential driver. Dis. Aquat. Organ. 69, 33–40. doi: 10.3354/dao069033
Wagner, D. E., Kramer, P., and van Woesik, R. (2010). Species composition, habitat, and water quality influence coral bleaching in southern Florida. Mar. Ecol. Prog. Ser. 408, 65–78. doi: 10.3354/meps08584
Ware, M., Garfield, E. N., Nedimyer, K., Levy, J., Kaufman, L., Precht, W., et al. (2020). Survivorship and growth in staghorn coral (Acropora cervicornis) outplanting projects in the Florida Keys National Marine Sanctuary. PLoS One 15:e0231817. doi: 10.1371/journal.pone.0231817
Weil, E. (2004). “Coral reef diseases in the wider Caribbean,” in Coral Health and Disease, eds E. Rosenberg and Y. Loya (Berlin: Springer).
Weil, E., Hammerman, N. M., Becicka, R. L., and Cruz-Motta, J. J. (2020). Growth dynamics in Acropora cervicornis and a. prolifera in southwest Puerto Rico. PeerJ 8:e8435. doi: 10.7717/peerj.8435
Wellington, G. M., Glynn, P. W., Strong, A. E., Navarrete, S. A., Wieters, E., and Hubbard, D. (2001). Crisis on coral reefs linked to climate change. Adv. Earth Space Sci. 82, 1–5. doi: 10.1029/01eo00001
Willis, B. L., van Oppen, M. J. H., Miller, D. J., Vollmer, S. V., and Ayre, D. J. (2006). The role of hybridization in the evolution of reef corals. Annu. Rev. Ecol. Evol. Syst. 37, 489–517. doi: 10.1146/annurev.ecolsys.37.091305.110136
Wood, S. N. (2011). Fast stable restricted maximum likelihood and marginal likelihood estimation of semiparametric generalized linear models. J. R. Stat. Soc. 73, 3–36. doi: 10.1111/j.1467-9868.2010.00749.x
Woodley, J., Alcolada, P., Austin, T., Barnes, J., Claro-Madruga, R., Ebaks-Petrie, G., et al. (2000). Status of Coral Reefs of the World: 2000. Western Australia: Australian Institute of Marine Science.
Young, C. N., Schopmeyer, S. A., and Lirman, D. (2012). A review of reef restoration and coral propagation using the threatened genus Acropora in the Caribbean and Western Atlantic. Bull. Mar. Sci. 88, 1075–1098. doi: 10.5343/bms.2011.1143
Zeileis, A., Meyer, D., and Hornik, K. (2007). Residual-based shadings for visualizing (conditional). independence. J. Comput. Graph. Stat. 16, 507–525. doi: 10.1198/106186007x237856
Keywords: Acropora, hybrid, coral restoration, coral nursery, Caribbean
Citation: VanWynen CM, Hightshoe MV, Fogarty ND, Dahlgren CP and Gilliam DS (2021) Should Hybrids Be Used in Coral Nurseries? A Case Study Comparing Caribbean Acropora spp. and Their Hybrid in the Bahamas. Front. Mar. Sci. 8:669966. doi: 10.3389/fmars.2021.669966
Received: 19 February 2021; Accepted: 15 July 2021;
Published: 13 August 2021.
Edited by:
Carlos Prada, University of Rhode Island, United StatesReviewed by:
Claudia Patricia Ruiz-Diaz, Sociedad Ambiente Marino, Puerto RicoSusana Enríquez, National Autonomous University of Mexico, Mexico
Joshua Patterson, University of Florida, United States
Copyright © 2021 VanWynen, Hightshoe, Fogarty, Dahlgren and Gilliam. This is an open-access article distributed under the terms of the Creative Commons Attribution License (CC BY). The use, distribution or reproduction in other forums is permitted, provided the original author(s) and the copyright owner(s) are credited and that the original publication in this journal is cited, in accordance with accepted academic practice. No use, distribution or reproduction is permitted which does not comply with these terms.
*Correspondence: Cassie M. VanWynen, Y2Fzc2llLnZhbnd5bmVuQGdtYWlsLmNvbQ==