- 1The Fredy and Nadine Herrmann Institute of Earth Sciences, Hebrew University of Jerusalem, Jerusalem, Israel
- 2The Interuniversity Institute for Marine Sciences, Eilat, Israel
- 3School of Zoology, George S. Wise Faculty of Life Sciences, Tel Aviv University, Tel Aviv, Israel
Mussels are considered highly efficient marine biomonitors, tracing anthropogenic and natural variations in heavy metals and various organic compounds. While heavy metals depuration processes in biomonitors are of growing interest, less knowledge is available regarding their Pb isotopes and rare earth elements (REEs) accumulation-release dynamics, and their response to short-term anthropogenic and terrigenous perturbations. Here, we report the results of a relocation experiment where a group of mussels (Brachidontes pharaonis) were extracted from a contaminated lagoon in the Gulf of Aqaba, northern Red Sea, and placed in water tanks that were flushed continuously with fresh, uncontaminated seawater. Specimens were removed periodically from the water table over a period of 13 weeks and trace and REEs and Pb isotopic compositions were determined separately for mussel’s shells and soft tissues. The results display a clear decrease over time in the concentrations of various heavy metals and REEs in the soft tissue, in concert with a similar shift in the Pb isotopic compositions toward seawater values. By contrast, the elemental and Pb isotopic composition of the shell presents little change over time. Coupling between the Pb isotopic composition of corresponding soft tissue and shell samples allows back-calculation of the timing and magnitude of abrupt pollution events and presents a novel approach for monitoring short-term pollution events. Nevertheless, given the coastal setting of the studied samples, it is important to consider the effects of terrigenous material on the results. Accordingly, Al-normalized element concentrations, Pb isotopes and calculated Ce anomalies, are used to identify two distinct terrigenous end members controlling the contaminated lagoon and the pristine site. The study demonstrates the potential of using mussels as robust biomonitors of natural and anthropogenic environmental perturbations through the combination between elemental concentrations and the isotopic composition of Pb.
Introduction
Concentrations of heavy metals in the oceans, perturbated by anthropogenic processes, are particularly high in coastal areas (e.g., Steding et al., 2000; Buck et al., 2005; Xu et al., 2014), especially those proximal to big cities, where the discharge of untreated industrial and human waste is most significant (Van Geen et al., 1997; Boyle, 2019). Yet, evaluating the degree of contamination in coastal environments remains challenging because of the complexity in sampling and analyzing water samples and the short residence times of some contaminants in seawater following pollution events. Alternatively, it is possible to use the abundances of contaminants in solid phases within the marine environment as temporal and spatial tracers of pollution. These could be suspended or deposited sediment particles that may adsorb dissolved elements onto their surface from surrounding water, or living organisms that actively circulate seawater and hence incorporate dissolved elements internally, i.e., biomonitors (Rainbow, 2002). An advantage of using marine biomonitors is the ease of processing and analyses (relative to seawater) and their supposedly short response time to local changes in seawater heavy metal contents, whereas solid sediment particles tend to accumulate surrounding elements overtime but do not respond to short term perturbations in the seawater composition. Various species have been recognized to serve as reliable biomonitors, including ascidians, polychaetes, copepods, mussels, clams, oysters, snails and fish (e.g., Hutchinson et al., 1995; Van der Oost et al., 2003; Horiguchi, 2006; Cebrian et al., 2007; Raisuddin et al., 2007; Zhou et al., 2008; Zega et al., 2009; Moloukhia and Sleem, 2011; Carmichael et al., 2012; Tzafriri-Milo et al., 2019). Of these, mussels have been recognized as particularly reliable biomonitors because they have the ability to filter high volumes of water, allowing for the relatively significant accumulation of pollutants in their tissue (Phillips, 1976; Roditi et al., 2000). The use of mussels as biomonitors was introduced in the mid-1970s by Goldberg (1975), who proposed that specimens from coastal and open ocean sites could be beneficial to assess the spatial and temporal trends of various compound concentrations, such as halogenated hydrocarbons, transuranics, heavy metals, and petroleum. This resulted in the establishment of the global program known as “Mussel Watch” (Goldberg et al., 1978; Farrington et al., 1983), which widely uses mussels from the genus Mytilus for marine biomonitoring. The intertidal mussel Brachidontes pharaonis from the family Mytilidae is present in the coasts of the western Pacific Ocean, Indian Ocean, Red Sea, and Mediterranean Sea (Taylor, 1971; Sasekumar, 1974; Barash and Danin, 1986; Morton, 1988). Several studies have demonstrated the metal bio-accumulation capacity of B. pharaonis at polluted and pristine locations along the coasts of the Mediterranean Sea (Göksu et al., 2005; Karayakar et al., 2007; Dar et al., 2018; Hamed et al., 2020). However, to the best of our knowledge, none has presented the depuration dynamics of this species.
Despite numerous studies of the accumulation of heavy metals in mussels (e.g., Chan, 1989; Naimo, 1995; Yap et al., 2003; Fung et al., 2004; Zuykov et al., 2013; Liu and Wang, 2016), their elemental retention and depuration dynamics are still of growing interest. In addition to anthropogenic inputs, terrigenous sources such as atmospheric deposition, rivers and sediments are a prominent source of heavy metals and other elements to the marine environment (Turekian, 1977; Bruland et al., 2013). Similar to anthropogenic pollution such as discrete events of oil pollution and industrial or sewage discharge, terrigenous inputs can also have an abrupt nature, with relatively short episodes of increased fluxes such as dust storms (Mahowald et al., 2009; Ternon et al., 2010), flash floods (Katz et al., 2015), and large-scale sediment resuspension events (Bruland et al., 2008; Torfstein et al., 2020). The terrigenous components may also contribute anthropogenic sourced metals, as these may adsorb heavy metals onto their surfaces during transportation (Nriagu and Pacyna, 1988; Cziczo et al., 2009). Due to the complexity of sampling these and other anthropogenic driven events, examples of the use of mussels as biomonitors for abrupt pulses of marine pollution are scarce.
The Gulf of Aqaba (GoA) is a deep oligotrophic water body connected to the Red Sea across the shallow Straits of Tiran in its southern part (Figure 1). The regional climate is extremely arid (rainfall <30 mm/year), therefore freshwater input from precipitation and fluvial run-off are quantitatively insignificant, with only a few rain events and associated flashfloods each winter, compared to high fluxes of atmospheric dust deposition (Genin et al., 1995; Almogi-Labin et al., 2008; Genin, 2008; Lazar et al., 2008). Two major cities reside in the northern tip of the GoA: Eilat (Israel) and Aqaba (Jordan) (Figure 1). Marine based commerce activity, together with the environmental impacts of big cities, are often in conflict with the well-being of natural resources, making the GoA marine habitats highly sensitive to anthropogenic contamination (Abelson et al., 1999; Wielgus et al., 2004). A man-made inland lagoon (the “Peace Lagoon”, Figure 1) was constructed to support the development of tourism along the seashore. Yet overestimates of the water exchange rates with the open sea had resulted in an almost isolated inland lagoon with very little water exchange with the open waters. Consequently, the lagoon accumulated contaminants and organic material over time, and its interstitial waters became anoxic. At present, the lagoon is considered contaminated and unfit for recreation activities.
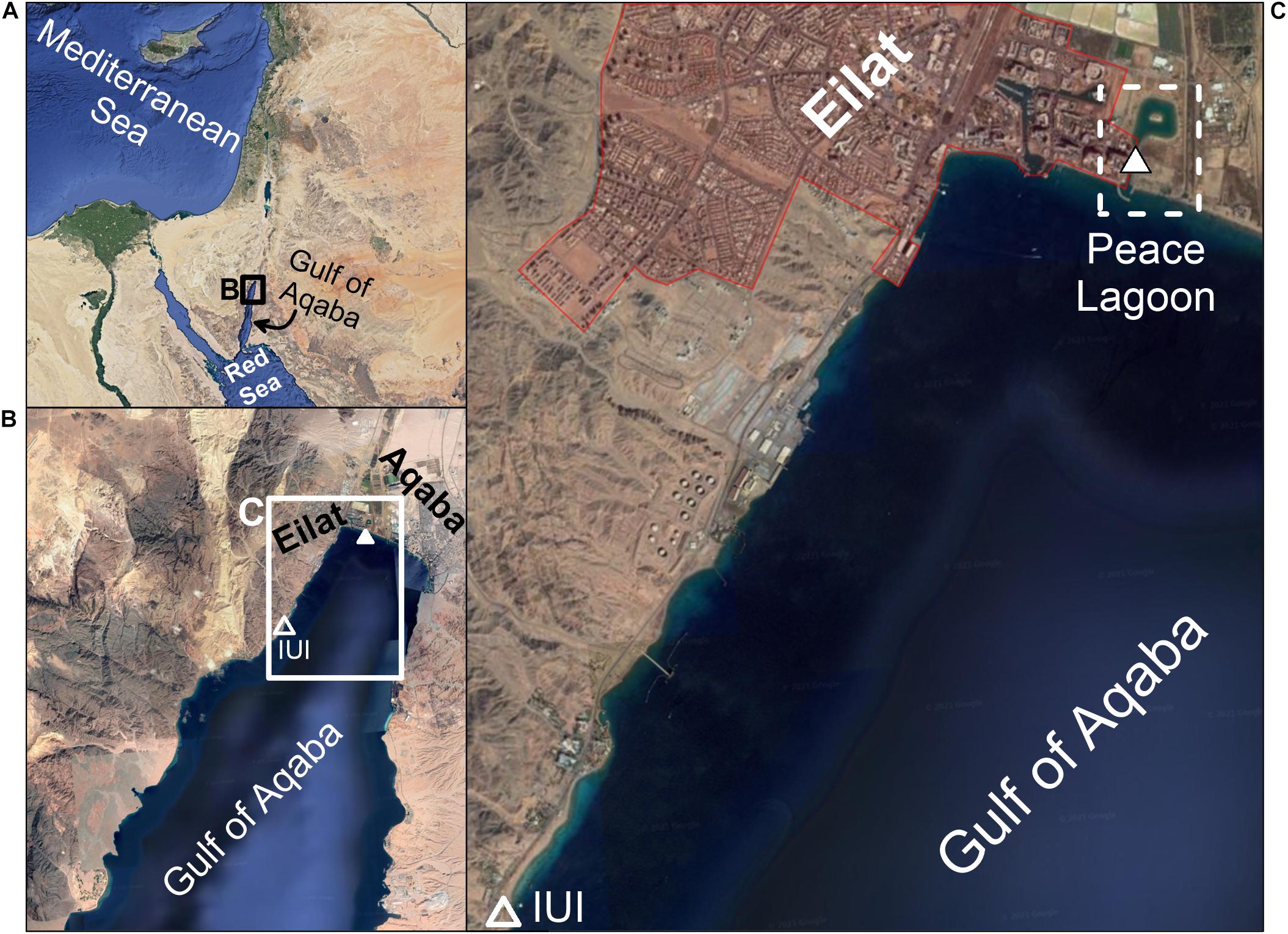
Figure 1. (A) Location map. The location of the Interuniversity Institute (IUI) for Marine Sciences, where the mussels were relocated to, is marked by an empty triangle in panel (B). The location of the Peace Lagoon is presented in panel (C), including the exact site of sampling (white triangle). Map taken from Google Maps.
Several previous studies have provided information about metals in, or near, the GoA, ranging between analyses of seawater (Chase et al., 2011, 2006; Chien et al., 2019; Benaltabet et al., 2020), atmospheric dust (Chen et al., 2008; Torfstein et al., 2017; Chien et al., 2019) and studies of surface sediments (Al-Taani et al., 2014; Barakat et al., 2015). Despite these efforts, we still lack a clear understanding of the sources of contamination, their spread range and their long and short-term impact on the GoA and its marine ecosystem.
In addition to the use of heavy metal distributions as tracers of anthropogenic contamination processes, lead (Pb) isotopes (204Pb, 206Pb, 207Pb, and 208Pb), whose relative abundances often differ between natural and anthropogenic sources, make it possible to infer the source of Pb pollution in the environment (e.g., Boyle et al., 1986; Erel et al., 2002; Komárek et al., 2008). Indeed, the Pb isotopic composition of mussels has previously been used to trace the source of environmental pollution in coastal environments (Labonne et al., 2001, 1998; Richardson et al., 2001; Dang et al., 2015).
The distribution of Rare Earth Elements (REEs) in seawater has been shown to be a useful proxy for quantifying and distinguishing between terrigenous and anthropogenic inputs to the oceans (de Baar et al., 1985; Elderfield, 1988; Hatje et al., 2016). In recent years, the increasing use of REEs in industrial (La, Ce, Pr, Sm, Nd, and Tm) and medical (Gd) fields have resulted in their intrusion into the marine environment and subsequent accumulation in marine biota (Gwenzi et al., 2018; Squadrone et al., 2019). The low concentrations of REEs in seawater hamper their application as in-situ marine-environmental monitors. By contrast, elevated abundances in various marine organisms renders them useful biomonitor for REEs in the marine environment (Bonnail et al., 2017; Ma et al., 2019; Wang et al., 2019).
In this study, we explore the depuration process of heavy metals, REEs and Pb isotopes in mussels (B. pharaonis). To this end, a native population living in the Peace Lagoon (Figure 1) were relocated to pristine seawater tanks in the Interuniversity Institute (IUI) for Marine Sciences (Figure 1) where heavy metals concentrations were shown to be lower compared with the north shore, in the vicinity of the lagoon (Herut et al., 1999; Chase et al., 2011, 2006; Chien et al., 2019). The shell and soft tissues were analyzed for their heavy metal and REEs concentrations and Pb isotopic composition over a period of 13 weeks in order to demonstrate the depuration trends from highly contaminated values to the low and natural baseline.
Materials and Methods
Experimental Setup and Sample Processing
A batch of 18 adult (∼3 cm in length) Red Sea mussels B. pharaonis were collected from the Peace Lagoon in the north beach of Eilat (32°54′84.4″N 34°96′82.3″E) and translocated to a running seawater tank in the IUI (Figure 1) for the rest of the depuration experiment. The water tank volume was 120 liters with a flowing water rate of 8.5 liters per minute. A pair of mussels was removed every week during the first 6 weeks (Tables 1–3). The two last samples were removed after 12 and 13 weeks. A pair of mussels was sampled immediately after extraction from the Peace Lagoon (i.e., at “day 0”, without being exposed to the water tank).
After removal from the water tank, the samples were carefully transferred to a clean lab where they were processed in a class 100 environment. Samples were first rinsed in ultrapure MQ water (18.2 MΩ cm) and sonicated over several cycles to remove external debris and particles. The shell and soft tissue were then separated and freeze-dried. After weighing the dry samples, the shell was gently leached with 0.05 N HNO3, to remove the outer rim that could have potentially been contaminated during the sampling, or contain external residue. We are aware that this might also be the part of the shell that could have formed during the experiment, but in terms of mass balance, this would be negligible and probably not observed when measuring the untreated bulk shell sample.
The soft tissue was digested in acid cleaned Teflon (PFA) beakers (Savillex, United States) through several cycles of heated H2O2–HNO3 mixtures, while the shells were digested using 1 N HNO3. All reagents used in this study were ultrapure solutions (commercial or in-house double distilled) and their concentrations were adjusted with ultrapure MQ water. Generally, two biological replicates were processed and analyzed for soft tissue elemental abundances while one individual was used for soft tissue and shell Pb isotopic composition. The number of replicates used for elemental abundances and Pb isotopic composition at each sampling date is given in Tables 1–3.
Analyses of Trace and Rare Earth Elements Concentrations
The digested samples were dried on a hotplate, re-dissolved in 3% HNO3, and analyzed for their trace (Al, V, Cr, Mn, Fe, Co, Ni, Cu, Zn, Cd, Pb, and Th) and REE (La, Ce, Pr, Nd, Sm, Eu, Gd, Tb, Dy, Ho, Er, Tm, Yb, and Lu) abundances on an Agilent 7,500cx ICP-MS at the Institute of Earth Sciences, Hebrew University of Jerusalem. A multi-elemental standard solution (3% HNO3 matrix) was used for instrumental signal calibration. Each sample was spiked online with internal standards (Sc, Re, and Rh) to follow and correct for instrumental drifts. Additional monitoring of the intra- and inter- session drift was achieved through the analyses of an in-house standard solution every 10–15 samples, yielding a long term precision of <2% (2σ). The results were corrected for procedural blank values.
Analyses of Pb Isotopic Composition
The remaining solution after ICP-MS analysis was dried, re-dissolved in 1 N HBr, and then Pb purified using standard ion chromatography (e.g., Torfstein et al., 2018) and the details are summarized hereafter. The HBr solution was taken through a series of purification steps to separate a purified Pb fraction. First, 100 μl of AG1X-8® 100–200 mesh anion resin were loaded onto Teflon micro-columns and cleaned over repeated cycles of MQ water and 6 N HCl. The samples were then loaded to the columns and the matrix was removed using 1 N HBr and 2 N HCl. Pb was eluted into Teflon beakers using 6 N HCl. The samples were then dried and re-dissolved in 3% HNO3 doped to 50 ppb Tl to account for instrument mass fractionation. These aliquots were analyzed for their Pb isotopic composition on a Neptune Plus multi collector ICP-MS, together with repeated measurements of the NIST SRM-981 standard which was used for accuracy and instrumental drifts corrections.
Data Treatment
The uncertainty for elemental concentrations is based on the standard deviation of duplicate samples (i.e., two biological replicates), except when duplicates were not available, in which case the relative uncertainty average of the rest of the samples was applied (Tables 1, 2 and Figure 2). The detection limits were defined as three-fold the standard deviation of full procedural blanks processed and analyzed with the samples (n = 3). Samples below blank or detection limit values, the higher of the two, are marked by “nd” in Tables 1, 2.
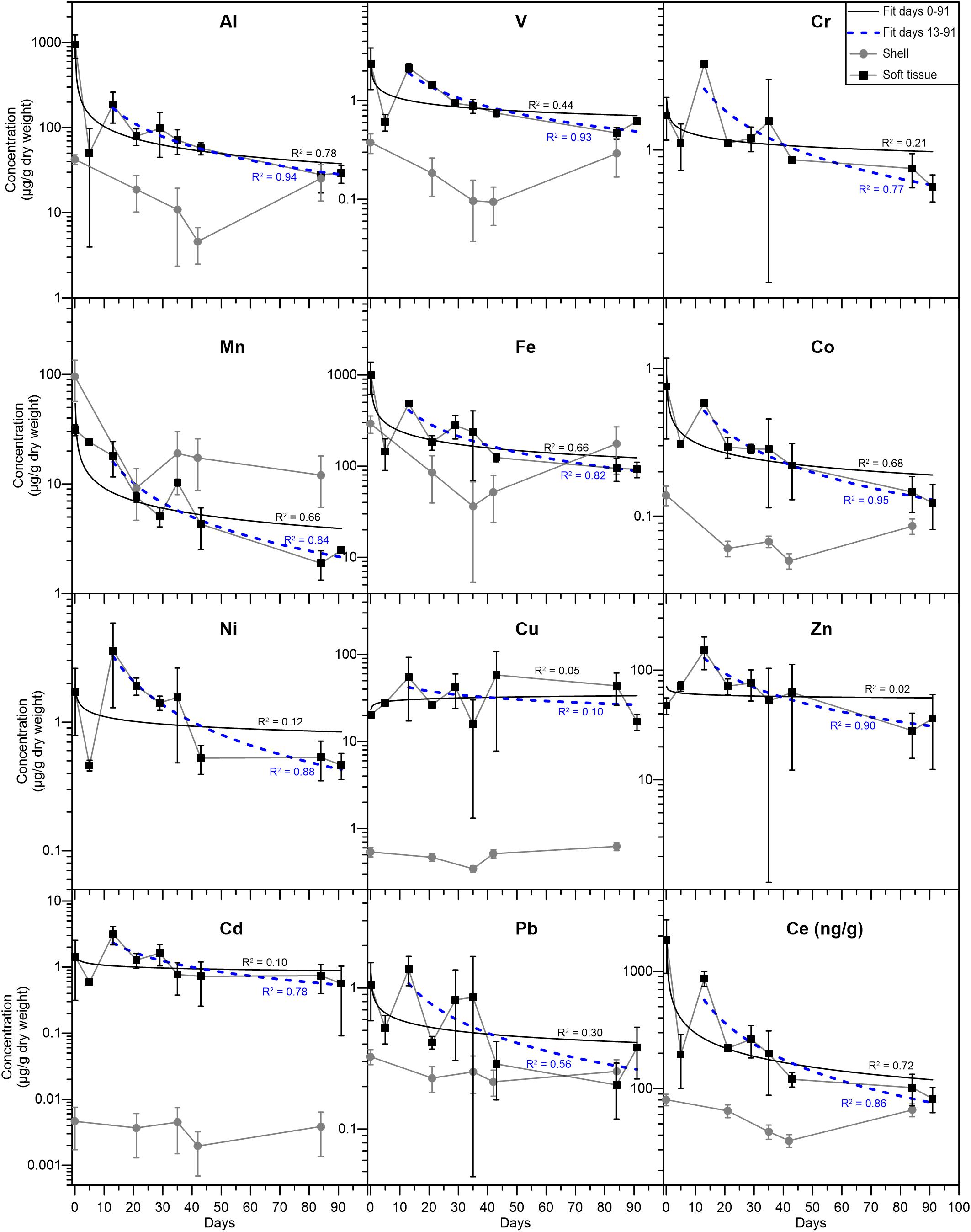
Figure 2. Elemental concentrations (μg/g dry weight) in the soft tissue (black squares) and shell (gray circles). The solid (black) and dashed (blue) curves represent power law fits for the elemental abundances from days 0–91 and 13–91, respectively, with the corresponding R2 values. Error bars mark the standard deviation of duplicate analyses (see section “Data treatment” for details). Ce is presented as ng/g (dry weight) and its temporal evolution is similar to that of the other REEs (Table 2).
The uncertainty for Pb isotopic ratios was evaluated on the basis of a biological duplicate processing and analyses (sample from day 0 for the soft tissue and sample from day 35 for the shell). The standard deviation from the average of the duplicates was applied to the rest of the samples (Table 3 and Figure 3).
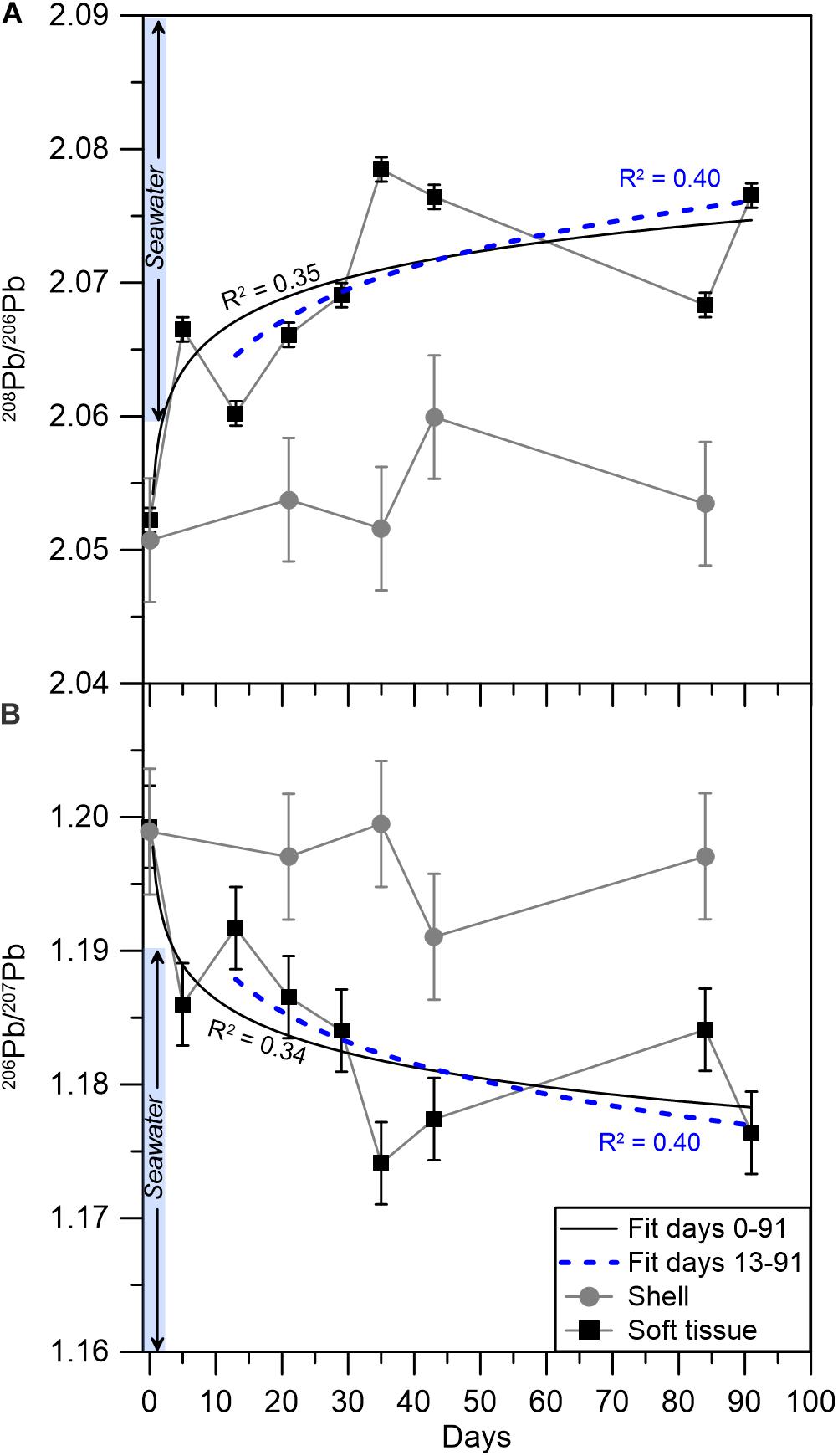
Figure 3. Pb isotopic compositions in the soft tissue (black squares) and shell (gray circles) for (A) 208Pb/206Pb and (B) 206Pb/207Pb. The solid (black) and dashed (blue) curves represent power law fits for the elemental abundances from days 0–91 and 13–91, respectively, with the corresponding R2 values. Error bars mark the standard deviation of one duplicate analyses, which was applied to the rest of the samples (see section “Data treatment” for details). The pale blue bar represents the range of seawater Pb isotopic compositions in the GoA (Benaltabet et al., 2020).
Results
Heavy Metals and REEs
Elemental abundances were measured in a total of 17 soft tissue and seven shell samples (Tables 1, 2). A large suite of elements displays a general ongoing decrease in soft tissue concentrations with time from day 0 to 91. These include Al, V, Cr, Mn, Fe, Co, Ni (Figure 2), and the REEs (Table 2). Other elements show a more moderate decrease (Pb, Cd, and Zn) or a negligible change with time (Cu) (Figure 2). Regardless of the general trend, all elements (besides Mn and Cu) displayed a sharp increase in soft tissue concentrations at day 13, after which concentrations continued to gradually decrease. Compared to the soft tissue, the shell fraction concentration presented little change over time in all elements apart from Mn, which shows a coeval decrease in both fractions.
Pb Isotopes
The isotopic composition of Pb was measured on a subset of 10 soft tissue and 6 shell samples (Table 3 and Figure 3). The soft tissue samples display a gradual shift in 206Pb/207Pb and 208Pb/206Pb compositions from 1.199 to 1.176 and 2.052 to 2.077, respectively. At day 13, similar to the elemental abundance patterns, the 206Pb/207Pb and 208Pb/206Pb soft tissue compositions shift sharply toward higher and lower ratios, respectively. Thereafter, the isotopic ratios continue their initial trend and shift toward lower 206Pb/207Pb ratios and higher 208Pb/206Pb ratios. By contrast, and similar to elemental abundance patterns, the shell compositions display little variation regardless of the time the sample was exposed to pristine seawater. Moreover, this unchanging composition corresponds, within uncertainty, to the composition measured in the soft tissue at the start of the experiment, which represents the lagoon environment.
Discussion
Long-Term Depuration Trends in Elemental Abundances
To a first order, all metals (except for Cu) and REEs studied here display a gradual decrease in the soft tissue concentrations (Tables 1, 2 and Figure 2), from the moment they were moved from the lagoon environment to the water tanks. Although not measured directly in this study, seawater elemental concentrations in the vicinity of the IUI and the lagoon can be estimated based on previous studies (Chase et al., 2011, 2006; Chien et al., 2019). Previously reported surface dissolved Al, Mn, Fe, Co, Cu, Zn, Cd, and Pb concentrations near the IUI coast are generally lower than surface concentrations at the north shore near the lagoon and are more similar to surface concentrations measured at the open sea (Figure 1, Station A), further away from shore (Supplementary Table 1). Moreover, Herut et al. (1999) have shown that native gastropods (Cellana rota) in the vicinity of the IUI presented lower levels of soft tissue Zn, Fe, Cu, and Mn when compared with specimens from the GoA north shore. These observations suggest that the decrease in soft tissue concentrations over time represents the elemental depuration as a result of the relocation from the lagoon to the pristine IUI environment.
The decrease in elemental abundances is observed 5 days after the relocation to the water tanks, as soft tissue concentrations decreased by 51 to 86% between days 0 and 5. These rates are similar to the rapid depuration rates demonstrated by the mussel Perna virdis (Yap et al., 2003) and the bivalve Paphia undulata (El-Gamal, 2011). No significant decrease trend is observed in the mussel’s shells because the fraction of the shell that formed during the experiment is negligible relative to its bulk weight. Hence, it appears that while the soft tissue can biomonitor short-term changes in the organism’s environment, the shell represents long-term chronic conditions. It is recognized that the elemental evolution trends are noisy, most likely reflecting the combined natural variability between different specimens, their initial heterogeneity in the lagoon and their individual response to the relocation which could be influenced by their different dry weights (ranging between 10–63 mg) and size, and possibly even their position in the water tank (Phillips, 1980).
To better understand the dynamics of metal concentrations in the soft tissue, the Pearson correlation coefficient (r) was examined between all studied metals in the samples collected after the relocation at days 5–91 (Table 4), as any deviation from a linear relationship between metals might suggest a different depuration dynamic modulating soft tissue metal concentrations. Soft tissue Al, V, Cr, Fe, Co, Ni, Zn, Cd, and Pb concentrations between days 5 and 91 present positive correlations with one another (r = 0.76–0.98, p < 0.03). When the day 0 samples, which represent the lagoon compositions, are also considered in the calculation, the correlation yields significantly lower values (Supplementary Table 2). Regardless of the calculation time span, it appears that the soft tissue passively records the ambient seawater composition of these metals, without an apparent preference. Inversely, poor correlations are presented for Mn and Cu when compared with the other metals suggesting that there might be other controls on their soft tissue abundances.
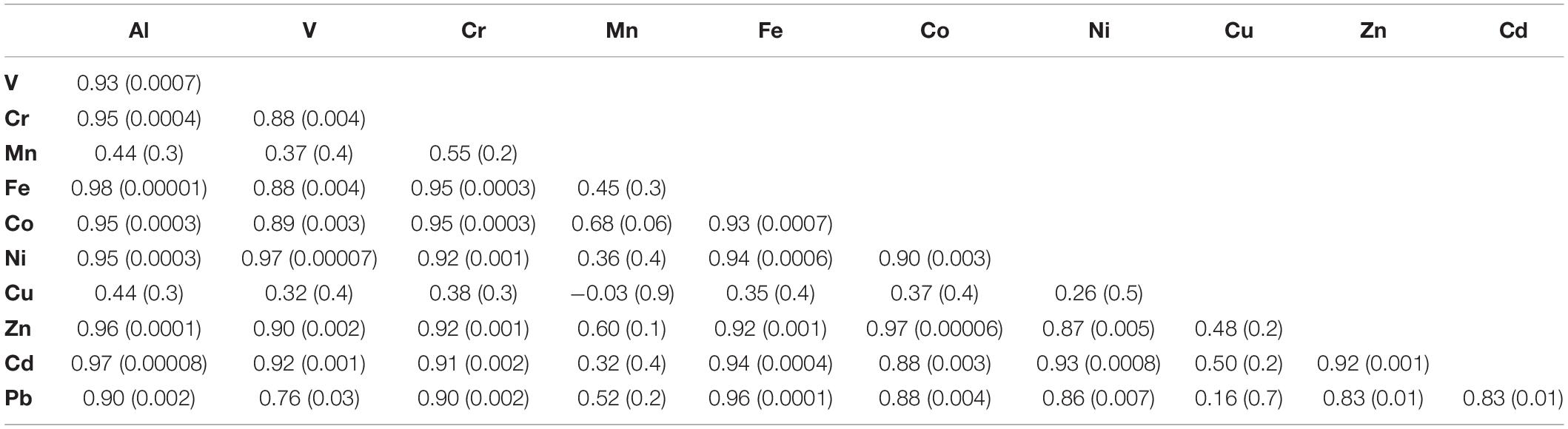
Table 4. Pearson correlation coefficient values (r) for soft tissue trace elements concentrations for samples collected between days 5 and 91 with corresponding p-values in brackets.
Soft tissue Cu concentrations do not present the same depuration trend similar to the rest of the metals, as they remain relatively constant over the course of the relocation experiment (Figure 2). This could be the result of chronically high dissolved Cu concentrations at the GoA western coast, which may be similar to those at the lagoon. However, as established before (Supplementary Table 1; Chase et al., 2011) high dissolved Cu concentrations are less plausible and a biological mechanism through which the soft tissue retains Cu might explain the constant Cu concentrations throughout the experiment. Similar observations were made by Lorenzo et al. (2003), who transferred mussels (Mytilus edulis) from a Cu enriched environment to clean seawater and reported low Cu depuration rates relative to the model expected rates, owing to biological regulation of Cu. It is worth noting that despite the difference in species, the final soft tissue Cu concentrations reported by Lorenzo et al. (2003) were similar to those presented here (Figure 2). Furthermore, in a metal depuration experiment performed on the Mediterranean mussel Mytilus galloprovincialis, relatively low Cu depuration rates were observed (Anacleto et al., 2015). A possible explanation is that mollusks might actively retain high levels of Cu (and Zn) through metallothioneins, as these are biologically essential metals (Amiard et al., 2006). Similarly, soft tissue Zn, Cd, Fe, and Mn concentrations at day 91 are within the same order of magnitude as the values reported for the gastropod C. rota (Herut et al., 1999) near the IUI coast, while Cu concentrations are ∼15–40 times higher. Moreover, while Cr, Mn, Ni, Cd, and Pb soft tissue concentrations at day 91 are similar to the average natural values reported for in-situ B. pharaonis by Hamed et al. (2020) in the Mediterranean Sea, Fe, Cu, and Zn are higher by a factor of ∼12, 15, and 10, respectively. It is conceivable that when exposed to high ambient levels of bio-essential metals, B. pharaonis will actively retain optimal high soft tissue concentrations (White and Rainbow, 1982; Amiard et al., 2006).
Out of the entire suite of metals studied, Mn is the only metal that presents higher or similar concentrations in the shell compared to the soft tissue (besides Al and Fe, which show similar concentrations at day 84). In addition, only Mn shell and soft tissue concentrations are significantly correlated (r = 0.97, p < 0.01), implying that they are both modulated by the same mechanism. In their study of the mussel M. eduils, Freitas et al. (2016) have shown that shell Mn contents are not directly controlled by ambient dissolved and particulate Mn concentrations nor by kinetic effects, but are mediated by a physiological mechanism associated with the extra-pallial fluid (EPF). It is possible that the decrease in shell Mn after the relocation to the water tanks is coupled to the decrease in soft tissue Mn and related to the connections between the soft tissue and the shell through the EPF (Crenshaw, 1972; Freitas et al., 2016). This might also explain the lack of correlation between soft tissue Mn and other metals, given the high affinity of the EPF to Mn2+ (Yin et al., 2005).
Al-Normalized Ratios, REE, and Pb Isotopes as Proxies for Terrigenous Inputs
Terrigenous inputs such as rivers, atmospheric aerosols and terrestrial and marine sediments are the main sources of metals to the oceans (Turekian, 1977; Bruland et al., 2013) with Al being a prominent proxy used to evaluate terrigenous fluxes to the marine environment (Baker et al., 2016; Jickells et al., 2016). To better characterize the controls of terrigenous components over the elemental compositions discussed here, we present the mussel’s soft tissue metal concentrations normalized to Al (Supplementary Figure 1). The Al-normalized metal ratios allows a better estimation of relative elemental depletion/enrichment in various organic (Bekteshi et al., 2015) and inorganic phases (Shelley et al., 2015; Jickells et al., 2016). Following the relocation of the mussels from the lagoon to the water tanks, all metal/Al ratios between days 0 and 5 displayed a significant increase and remained relatively constant thereafter. This shift clearly depicts the transition from the terrigenous dominated and Al rich shallow lagoon, where exchange with open seawater is limited, to the relatively Al-depleted seawater environment. Moreover, while most metal/Al ratios shifted by up to two order of magnitude, the increase in Fe/Al ratios was smaller (Supplementary Figure 1), reflecting the joint association of both Fe and Al with terrigenous material. For comparison, the Al-normalized Th abundances remains relatively stable throughout the entire duration of the experiment, reflecting the strong association of Th with terrigenous inputs.
The transition to a pristine environment is further illustrated in the mussel’s soft tissue Pb isotopic composition, which is considered in the context of previously reported GoA seawater Pb compositions (Figure 4) and its respective end members (Lee et al., 2015; Chien et al., 2019; Benaltabet et al., 2020). These include seafloor sediments, open Red Sea waters and aerosols, the latter being the most significant source of anthropogenic Pb to the GoA (Chien et al., 2019; Benaltabet et al., 2020). The initial Pb isotopic composition of both the shell and the soft tissue at day 0 represents the lagoon end member, which presents a mixture between sediments and GoA open seawater. Five days after the translocation, the soft tissue presents open seawater compositions, portraying the organism’s rapid response to its ambient seawater Pb composition. However, this trend stops at day 13, when the soft tissue isotopic composition shifts toward the lagoon end member. The sample collected at the following week at day 21 and all subsequent samples, presents Pb compositions similar to open seawater, portraying the organism’s prolonged response to ambient pristine waters. The composition of the shell did not overlap with the GoA seawater field throughout the entire experiment, depicting the limited response of the shells to the translocation.
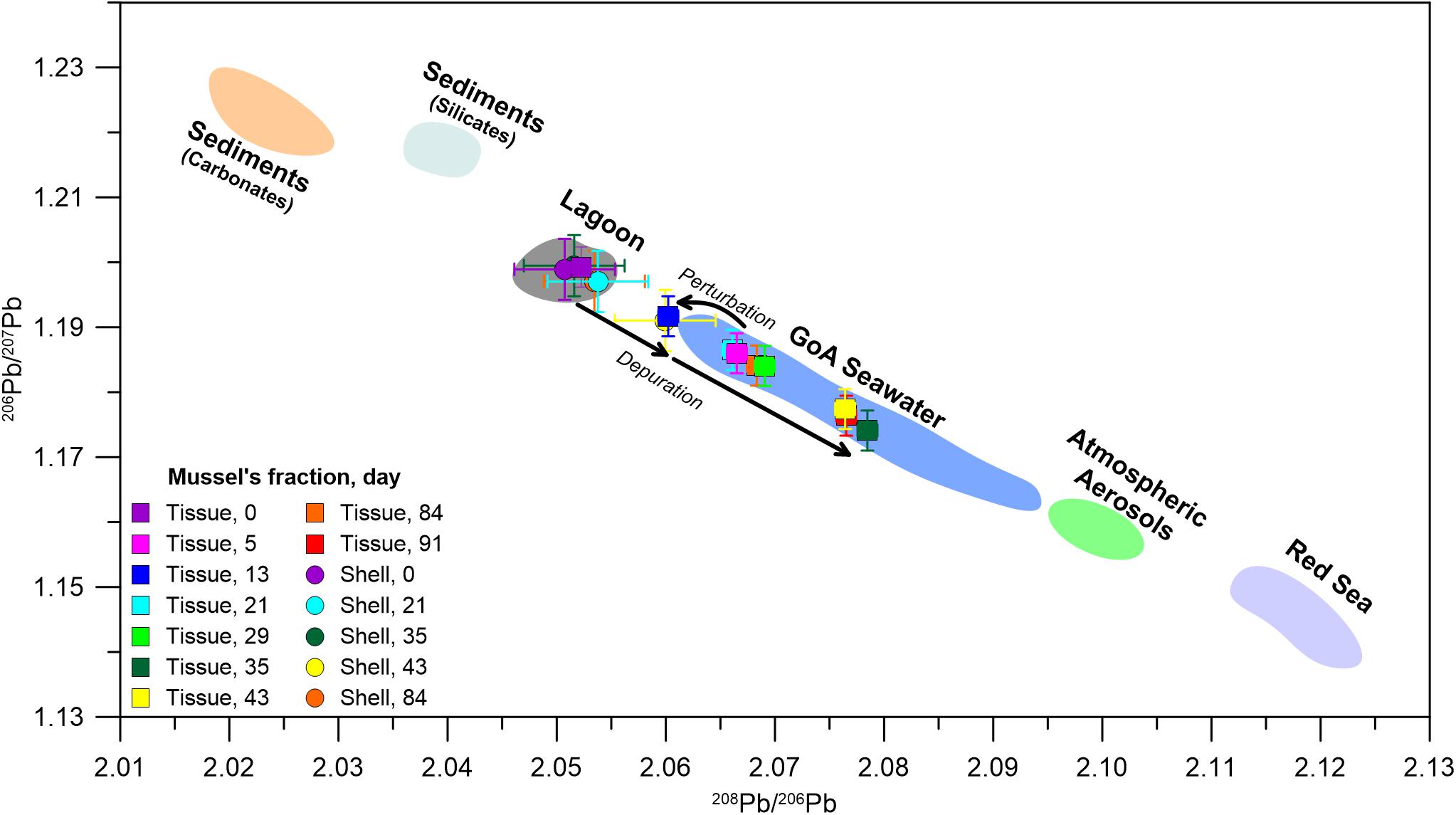
Figure 4. The isotopic composition of 208Pb/206Pb versus 206Pb/207Pb in soft tissue (squares) and shells (circles). Colored markers represent the different samples through the length of the experiment. Shaded areas represent the regional end members: GoA seawater (blue field; Benaltabet et al., 2020), atmospheric aerosols (Chien et al., 2019), GoA leached and residual sediment fractions (Benaltabet et al., 2020), which represent the carbonate (orange field) and silicate (light blue field) fractions of bottom sediments, respectively. Red Sea seawater compositions (purple field) are assumed to correspond with Arabian Sea compositions (after Lee et al., 2015). For more information regarding the suggested end members the reader is referred to Chien et al. (2019) and Benaltabet et al. (2020). The day 0 shell and soft tissue composition represents the lagoon end member (gray field).
The REE concentrations are normalized to a well-established reference composition, such as the Post Archean Australian Shale (PAAS, Taylor and McLennan, 1985), which helps reveal their relative enrichment and certain REE anomalies (e.g., Ce, Eu, and Gd), providing information regarding sources or sinks of certain elements (Elderfield and Greaves, 1982; de Baar et al., 1983; Hatje et al., 2016). For example, dissolved Ce may be oxidized from Ce3+ to Ce4+, resulting in a decrease in solubility and a negative Ce anomaly in seawater (de Baar, 1983). By contrast, anoxic conditions may result in a positive Ce anomaly in sediment’s pore-waters (Elderfield and Sholkovitz, 1987). External sources of anthropogenic Gd, associated with medical and industrial wastewaters inputs due to its use in magnetic resonance imaging, may result in a positive Gd anomaly in seawater (Kümmerer and Helmers, 2000; Nozaki et al., 2000; Hatje et al., 2014). The Ce and Gd anomalies are defined as their deviation from an expected, PAAS-normalized ratio, as defined by Eqs 1 (Mclennan, 1989) and 2 (de Baar et al., 1985):
where the PAAS-normalized concentrations are indicated by subscript N and ∗ denotes the theoretical interpolated concentration based on neighboring elements. Accordingly, positive and negative Ce and Gd anomalies will feature Ce/Ce∗ and Gd/Gd∗ ratios higher and lower than 1, respectively.
Figure 5 presents the distribution of soft tissue REEs relative to the composition of PAAS, displaying an enrichment of the light REEs (LREE, La-Eu) relative to the heavy REEs (HREE, Gd-Lu). Similar observations were made for clams (Bonnail et al., 2017), fish, crustaceans, and mollusks (Li et al., 2016; Wang et al., 2019) and were suggested to stem from biological fractionation that favors LREE over HREE (Wang et al., 2019). The samples collected at day 0 display high REE/PAAS ratios (an order of magnitude higher than the rest of the samples) with no anomalies (Figure 5A), reflecting the domination of terrigenous sources in the lagoon environment. Five days after the translocation, REE concentrations decrease significantly, but shift back to higher values on day 13 while also displaying a positive Ce anomaly (Figure 5B). Afterward, the REE concentrations as well as the Ce anomaly at the samples collected at days 21–91, return to values that are similar to day 5. Moreover, following the relocation to the water tanks, the PAAS normalized pattern reveals an increasing positive Gd anomaly (Figures 5A,C), which suggests an anthropogenic source of Gd to the GoA waters.
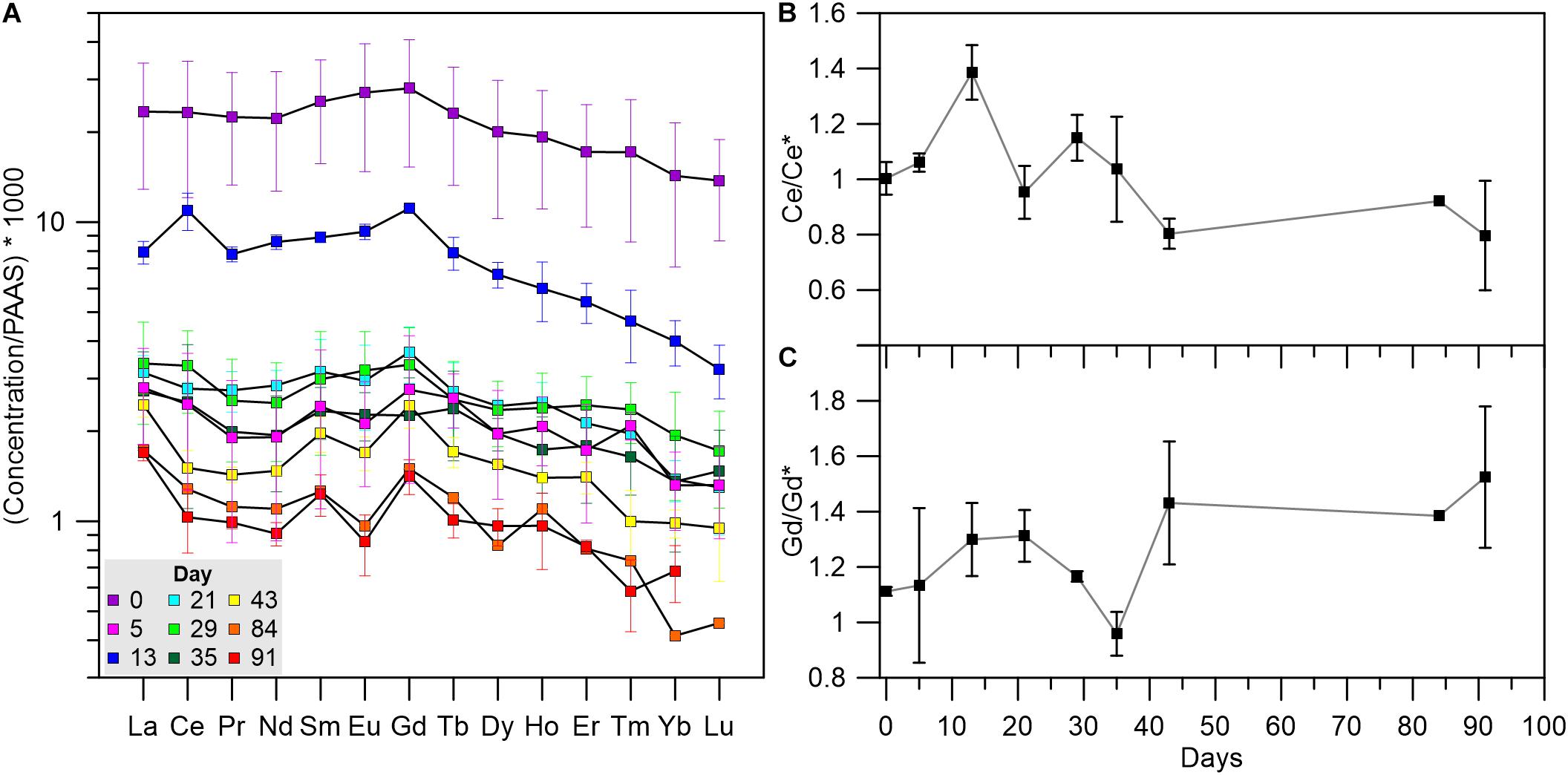
Figure 5. (A) Soft tissue REE concentrations relative to PAAS (Taylor and McLennan, 1985). Colored symbols represent the different sampling days through the length of the experiment. Error bars mark the standard deviation of duplicate analyses (see section “Data treatment” for details). (B) Ce/Ce* ratios representing the Ce anomaly throughout the experiment. (C) Gd/Gd* ratios representing the Gd anomaly throughout the experiment.
Short-Term Perturbation
During the depuration experiment, the soft tissue samples presented a 2–8-fold increase in elemental concentrations between days 5 and 13 (Figure 2), as well as a shift in Pb isotopic composition toward the lagoon and sedimentary end members (Figures 3, 4) and an increase in the PAAS- normalized REE concentrations (Figure 5). Moreover, when examining the subsequent samples, soft tissue Al, V, Cr, Mn, Fe, Co, Cd, Zn, Ni, and Pb concentrations gradually decrease from day 13 to 91, following a power law curve, with high R2 values of 0.56 for Pb to R2 = 0.95 for Co (Figure 2). When compared to the R2 values for the period between 0 and 91 days, all of the above mentioned R2 values for the period between 13 and 91 days are significantly higher. The fact that the decrease in soft tissue concentrations after day 13 closely follows a well-defined curve, suggests that the increase in concentrations in day 13 is associated with a compositional perturbation in the water tank’s seawater composition (rather than being associated with analytical noise) that effectively reset the experiment at day 13. Moreover, 7 days after the day 13 perturbation, metal levels decreased by 33 to 70%, at rates similar to the initial decrease in concentrations following the relocation to the water tanks (between days 0 to 5) and to previously reported depuration rates (Yap et al., 2003; El-Gamal, 2011). The rate of increase in soft tissue metal abundances in response to the perturbation is comparable with the increases in Cd and Zn reported by Yap et al. (2003), who exposed mussels (P. virdis) to high levels of seawater Cd and Zn in a controlled laboratory experiment and followed the change of accumulated metals over time. By contrast, the study carried out by Liu and Wang (2016), who translocated two oyster species from a natural to a contaminated environment and followed metal accumulation with time reported limited increases in the soft tissue metals concentrations after 5 days of exposure. However, the difference in metal accumulation rates compared to our results can probably be attributed to different environmental conditions (Mubiana and Blust, 2007; Casas et al., 2008) and species types (Rainbow, 2002).
The cause of the perturbation between days 5 and 13 is unknown, and could be related to a natural change in the influxing seawater composition, possibly due to sediment resuspension along the coast, or to contamination of the water tank. The relatively high Ce anomaly at day 13 (Figure 5) may be indicative of a sedimentary source, as positive Ce anomalies are a common feature in some marine sediments (de Baar, 1983; Toyoda et al., 1990; Pattan et al., 2005). Moreover, several studies suggested that mollusks effectively accumulate LREEs from sediments and suspended particles (Bonnail et al., 2017; Ma et al., 2019; Wang et al., 2019). Hence, a perturbation associated with a terrigenous source will be promptly recorded in the mussel’s soft tissue. This, coupled with the shift of the Pb isotopic composition toward sedimentary compositions (Figure 4) may suggest that the cause of the perturbation on day 13 is linked to a terrigenous source. Interestingly, the Al normalized ratios of several elements (e.g., Ni, Co, Fe, Cr, V, Pb Cd, Zn, and Cu) display a relatively large shift between day 0 and day 5 but remain stable thereafter (Supplementary Figure 1), even after the day 13 perturbation. This implies that the terrigenous end member responsible for the perturbation has a different composition, characterized by a higher metal/Al ratio, compared to the lagoon end member. In other words, one terrigenous end member dominates the lagoon area and a second dominates the IUI coastal waters.
To better characterize the two terrigenous end members, the progress of the soft tissue depuration of various proxies versus Al is outlined in Figure 6. Zn and Pb were chosen to represent examples of anthropogenic metals in coastal waters (e.g., John et al., 2007; Boyle, 2019), while the shifts in the Ce anomaly value (Ce/Ce∗) and the isotopic composition of Pb (206Pb/207Pb) represent the competing influences of terrigenous and seawater end member values. The lagoon environment is dominated by mixing of a terrigenous end member (“Terr1,” Figure 6) with the seawater end member (“SW,” Figure 6). While Terr1 features high metal concentrations and high 206Pb/207Pb ratios with no Ce anomaly (i.e., Ce/Ce∗ ≈ 1), the seawater end member is characterized by low metal concentrations (Chase et al., 2011; Chien et al., 2019; Benaltabet et al., 2020), a negative Ce anomaly, a common feature in oxygenated waters (Elderfield and Greaves, 1982; Alibo and Nozaki, 1999) and low 206Pb/207Pb ratios (Benaltabet et al., 2020). After the relocation to the water tanks, the mussel’s soft tissue composition shifted rapidly to a separate mixing curve, with a different terrigenous end member (“Terr2,” Figure 6), defined by high metal abundances and lower Al contents relative to Terr1, a positive Ce anomaly and high 206Pb/207Pb ratios. Following the day 13 perturbation, the soft tissue compositions shifted toward Terr2, and thereafter subsided toward the composition of the seawater end member, as the mussels gradually depurated the accumulated metals from the perturbation.
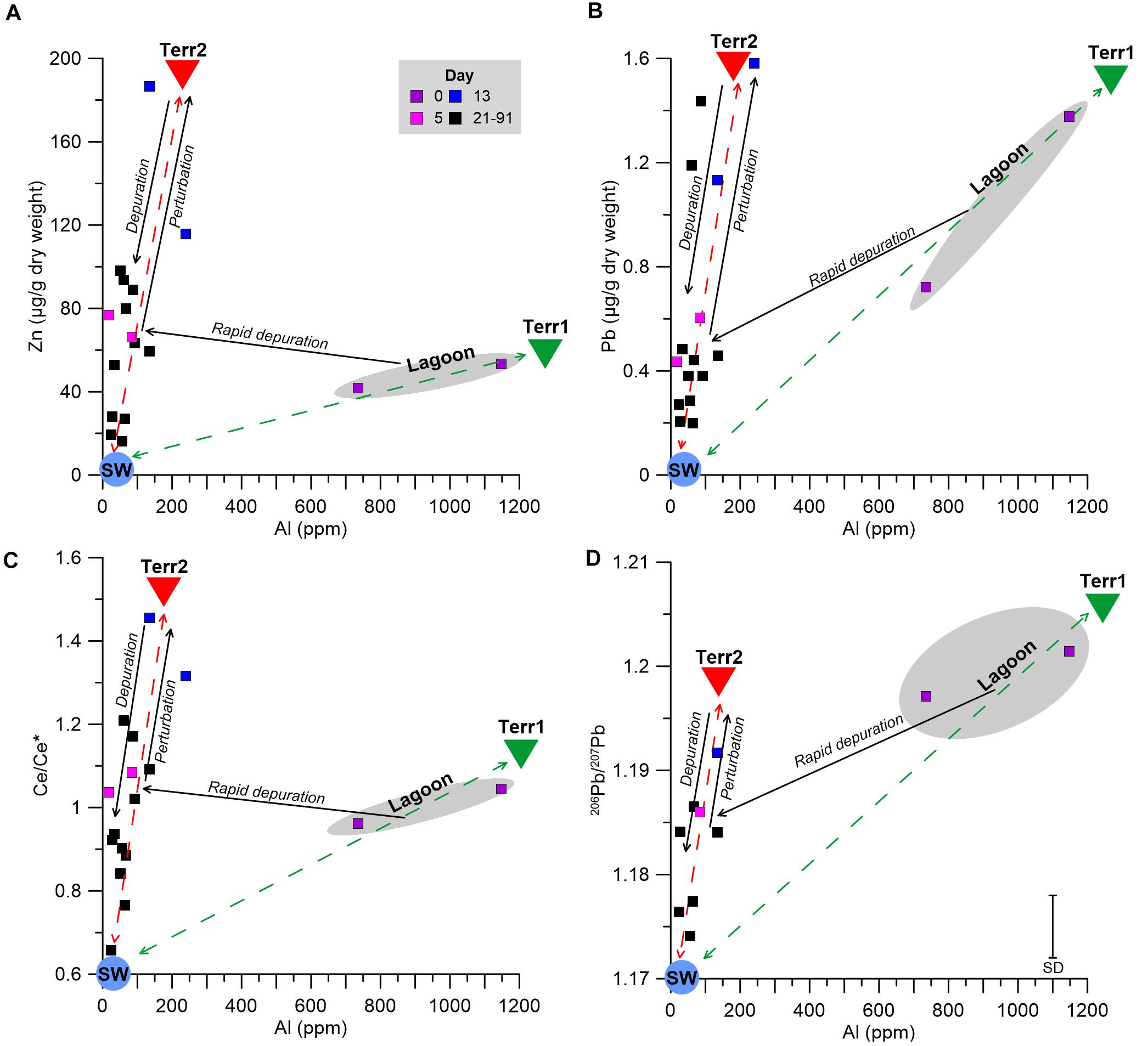
Figure 6. Soft tissue Zn (A) and Pb (B) concentrations, Ce/Ce* (C), and 206Pb/207Pb compositions (D) versus soft tissue Al concentrations. The dashed lines represent the mixing curves between the two terrigenous (Terr1 and Terr2; green and red triangles, respectively) and seawater (SW; pale blue circle) end members. Solid arrows represent the temporal trend throughout the experiment: the rapid depuration after the relocation from the lagoon environment (gray field, purple squares) to the IUI (pink squares) between days 0 and 5, the brief perturbation toward Terr2 between days 5 and 13 (blue squares), and the long term depuration between days 21 and 91 (black squares).
Long-Term Biomonitoring
Due to biological controls and the variability of elemental partitioning coefficients between seawater, soft tissue and shell (White and Rainbow, 1982; Chong and Wang, 2001; Amiard et al., 2006), metal abundances in the soft tissue and shell are markedly different (Figure 2). By contrast, the isotopic composition of the shell and soft tissue at day 0 overlap (Figures 3, 4) because Pb isotopic fractionation during assimilation is negligible (Russell Flegal and Stukas, 1987) and both phases acquired the long term local lagoon composition (Figure 4). The ratio between the Pb isotopic composition in the soft tissue and shell is expressed in Eq. 3:
where Rtissue and Rshell are the 206Pb/207Pb ratios (or other Pb isotopic ratios) in the soft tissue and shell, respectively. In the lagoon samples (day 0), the Rtissue/Rshell ratio presents an approximate value of one and continuously decreases as the soft tissue registers lower 206Pb/207Pb seawater compositions (Figure 3B).
Thus, mature mussels grown in stable conditions should display an identical Pb isotopic composition in both the shell and soft tissue, i.e., Rtissue/Rshell = 1. Accordingly, the coupled Pb isotopic composition of mussels soft tissue and shell can be used for long-term biomonitoring in coastal environments, even if the initial isotopic composition is unknown, as any deviation from Rtissue/Rshell = 1 will represent a shift toward a new compositional end member (Figure 7). If the perturbation is of chronic nature, the Rtissue/Rshell ratio will remain constant, representing the current long-term composition of surrounding seawater, as is the case here, where the seawater composition changed after their relocation.
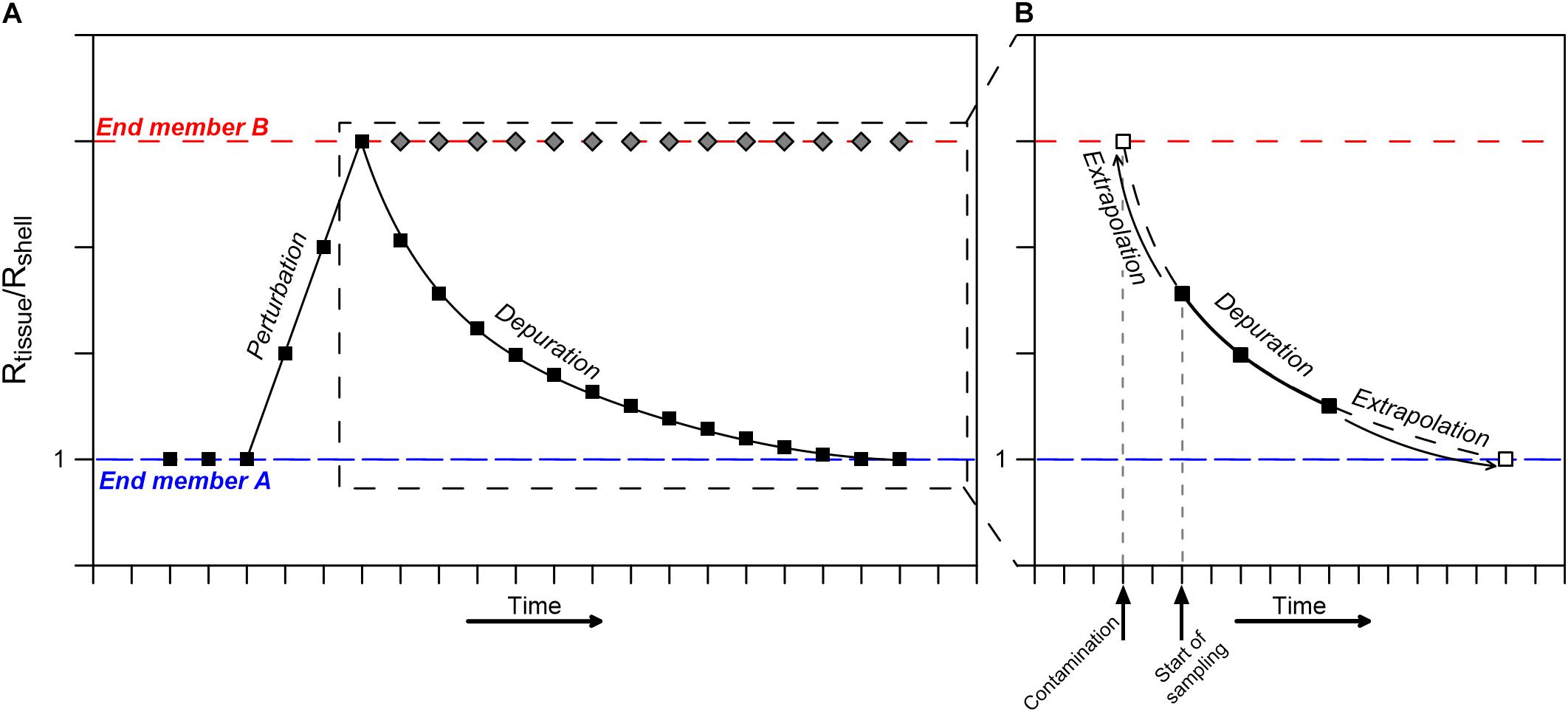
Figure 7. A conceptual representation of a perturbation/pollution event reflected by the ratio between soft tissue (Rtissue) and shell (Rshell) Pb isotopic compositions. (A) A perturbation represented by an exposure to end member B (dashed red line) will result in an increase in the Rtissue/Rshell ratio. Subsequently, if the contamination persists (chronic contamination), the Rtissue/Rshell ratio will remain high (until eventually the end member B signal starts dominating the bulk shell composition after a significant period of time not considered here). If the perturbation is brief, the Rtissue/Rshell ratio will gradually return to a value of 1 (end member A, dashed blue line) following a power law curve. (B) Rtissue/Rshell ratio shortly after a contamination event, depicting how a partial set of observations (e.g., black squares) can be used to extract the timing of the suspected perturbation/contamination event through back extrapolation of the calculated power law depuration curve to the contaminating end member composition (empty square).
This approach can also be applied to other heavy isotopic systems where no isotopic fractionation is associated with the assimilation into the shell and soft tissue (e.g., Nd, U, and Th). However, the isotopic composition of Pb is especially useful for monitoring marine pollution given its sensitivity to anthropogenic inputs and ample reports of natural and anthropogenic end member compositions (e.g., Bollhöfer and Rosman, 2001, 2000; Labonne et al., 2001; Erel et al., 2006; Boyle et al., 2014; Dang et al., 2015).
Biomonitoring of Short-Term Pollution Events
Although serendipitously demonstrated here (i.e., by the perturbation between day 5 and 13), biomonitoring of short-term (daily) events is often challenged by the rapid compositional change in soft tissue compositions in the days following the event, hampering the use of mussels and other marine filter-feeding organisms as biomonitors of abrupt and short pollution events. Nevertheless, our results provide the possibility to overcome this shortcoming by extrapolating observations backward toward the onset of the event.
Consider a pollution event that is identified along a coastline, but evidence (e.g., visual observations, smell, direct seawater analyses) only starts accumulating several days after the actual event. Thus, the exact timing and location of the contamination remain largely unknown. Yet, following alerts of a potential pollution event, it is possible to initiate continuous sampling of in-situ living mussels at a daily resolution over a period of 1–2 weeks and analyze the isotopic compositions of their soft tissue and shell. The results will yield a partial segment of the longer-term depuration pattern during which the Rtissue/Rshell ratio gradually returns to a value of 1 following a power law curve (Figure 7B). A backward extrapolation of this curve, allows determining the timing of contamination, as long as the initial contamination value can be estimated, even if only roughly. The latter can be reasonably evaluated in coastal environments where recurring pollution events take place, or by assuming a regional anthropogenic Pb isotope compositional end member. Accordingly, the time of contamination (t) can be determined using Eq. 4 solved for t:
Where Rc represents the composition of the contaminant, Rshell is the measured composition in the shell and m is the power constant given by the depuration curve. Alternatively, if the timing (t) of the pollution event is well known, Eq. 4 can be solved for Rc and cross-referenced with literature report to learn about the source of the contaminant end member (e.g., anthropogenic or natural/terrigenous). This approach assumes that the soft tissue is a sensitive recorder of ambient seawater compositions, as seen here and by others (e.g., Yap et al., 2003; El-Gamal, 2011; Anacleto et al., 2015). Moreover, if coupled with a spatial survey, this approach can also provide information regarding the geographic sources of the contaminants, and how these progress spatially and temporally.
In summary, mussels (B. pharaonis) growing in a contaminated lagoon were relocated to a pristine environment for depuration and their soft tissue and shells were analyzed for heavy metal and REE concentrations, as well as the isotopic composition of Pb.
The shell retained its original composition and did not present significant change in metal abundances (apart from Mn) during the experiment. On the other hand, most of the soft tissue metal concentrations (Al, V, Cr, Mn, Fe, Co, Ni, Zn, Cd, and Pb) and the REEs presented a gradual decrease in concentrations in the 91 days following the relocation from the lagoon to the water tanks, with the bulk majority of the decrease (51–86%) restricted to the first 5 days. The decrease trend was disrupted by an abrupt increase in metal and REE concentrations at day 13, after which concentrations decreased gradually following a power law trend (R2 of up to 0.95).
Pb isotope ratios of both the shells and soft tissue at the lagoon represent a mixture of previously reported seafloor sediment and open seawater compositions. While the composition of the shells remains rather constant throughout the experiment (206Pb/207Pb = 1.191 – 1.199, 208Pb/206Pb = 2.051 – 2.060), the soft tissue compositions (206Pb/207Pb = 1.174 – 1.199, 208Pb/206Pb = 2.052 – 2.078) shift gradually toward open seawater values from days 5 to 91, while briefly shifting toward the sedimentary end member at day 13. This shift is also illustrated by the REEs pattern relative to PAAS, which features a positive Ce anomaly at day 13. Hence, we conclude that this compositional shift was driven by an environmental perturbation linked to a terrigenous source. By comparing soft tissue metal concentrations, Ce anomaly ratios, Pb isotopic compositions and Al concentrations, it is shown that following the relocation to the water tanks, the mussels were controlled by a separate mixing curve between GoA seawater and a local terrigenous end member (possibly marine sediments), which is different than the terrigenous end member dominating the lagoon environment.
The soft tissue of B. pharaonis may be used to biomonitor short-term environmental perturbations or pollution events. The ratio between the Pb isotopic composition of the soft tissue and shell sampled following an event may be used to determine the exact timing and possibly geographic location of the pollution, when both the latter are a-priori unknown.
By implementing geochemical tools such as Pb isotopic compositions, Al-normalization and REE patterns, we demonstrated the potential of mussels as biomonitors of short-term fluctuations or alternatively, of long-term seawater compositions. In both cases, the combined suite of geochemical proxies can be used to provide robust quantitative constraints on the sources and magnitude of pollution events, providing important tools for implementing and developing environmental management policies.
Data Availability Statement
The original contributions presented in the study are included in the article/Supplementary Material, further inquiries can be directed to the corresponding author/s.
Author Contributions
TB, EG-H, and AT wrote the manuscript. EG-H and AT conceived the project and conducted fieldwork and analyses. All authors contributed to the article and approved the submitted version.
Funding
Funding was provided by the Israel Science Foundation grants 927/15 and 834/19 and the Hebrew University Ring Foundation to AT, and the Bester and Pfeifer scholarships to TB.
Conflict of Interest
The authors declare that the research was conducted in the absence of any commercial or financial relationships that could be construed as a potential conflict of interest.
Acknowledgments
We thank Barak Yarden for his assistance in sample preparation and Ofir Tirosh for his assistance with the instrumental analyses.
Supplementary Material
The Supplementary Material for this article can be found online at: https://www.frontiersin.org/articles/10.3389/fmars.2021.669329/full#supplementary-material
References
Abelson, A., Shteinman, B., Fine, M., and Kaganovsky, S. (1999). Mass transport from pollution sources to remote coral reefs in Eilat (Gulf of Aqaba, Red Sea). Mar. Pollut. Bull. 38, 25–29. doi: 10.1016/S0025-326X(98)00081-2
Alibo, D. S., and Nozaki, Y. (1999). Rare earth elements in seawater: particle association, shale-normalization, and ce oxidation. Geochim. Cosmochim. Acta 63, 363–372. doi: 10.1016/S0016-7037(98)00279-8
Almogi-Labin, A., Edelman-Furstenberg, Y., and Hemleben, C. (2008). “Variations in the biodiversity of thecosomatous pteropods during the Late Quaternary as a response to environmental changes in the Gulf of Aden–Red Sea–Gulf of Aqaba ecosystem,” in Aqaba-Eilat, the Improbable Gulff: Environment, Biodiversity and Preservation, ed. F. D. Por (Jerusalem: The Hebrew University Magnes Press), 31–48.
Al-Taani, A. A., Batayneh, A., Nazzal, Y., Ghrefat, H., Elawadi, E., and Zaman, H. (2014). Status of trace metals in surface seawater of the Gulf of Aqaba, Saudi Arabia. Mar. Pollut. Bull. 86, 582–590. doi: 10.1016/j.marpolbul.2014.05.060
Amiard, J. C., Amiard-Triquet, C., Barka, S., Pellerin, J., and Rainbow, P. S. (2006). Metallothioneins in aquatic invertebrates: their role in metal detoxification and their use as biomarkers. Aquat. Toxicol. 76, 160–202. doi: 10.1016/j.aquatox.2005.08.015
Anacleto, P., Luísa, A., and Leonor, M. (2015). Effects of depuration on metal levels and health status of bivalve molluscs. Food Control 47, 493–501. doi: 10.1016/j.foodcont.2014.07.055
Baker, A. R., Landing, W. M., Bucciarelli, E., Cheize, M., Fietz, S., Hayes, C. T., et al. (2016). Trace element and isotope deposition across the air–sea interface: progress and research needs. Philos. Trans. R. Soc. A Math. Phys. Eng. Sci. 374:20160190. doi: 10.1098/rsta.2016.0190
Barakat, S. A., Al-Rousan, S., and Al-Trabeen, M. S. (2015). Use of scleractinian corals to indicate marine pollution in the northern Gulf of Aqaba, Jordan. Environ. Monit. Assess. 187:42. doi: 10.1007/s10661-015-4275-2
Barash, A., and Danin, Z. (1986). Further additions to the knowledge of Indo-Pacific Mollusca in the Mediterranean Sea. Spixiana 9, 117–141.
Bekteshi, L., Lazo, P., Qarri, F., and Stafilov, T. (2015). Application of the normalization process in the survey of atmospheric deposition of heavy metals in Albania through moss biomonitoring. Ecol. Indic. 56, 50–59. doi: 10.1016/j.ecolind.2015.03.001
Benaltabet, T., Lapid, G., and Torfstein, A. (2020). Seawater Pb concentration and isotopic composition response to daily time scale dust storms in the Gulf of Aqaba, Red Sea. Mar. Chem. 227:103895. doi: 10.1016/j.marchem.2020.103895
Bollhöfer, A., and Rosman, K. J. R. (2000). Isotopic source signatures for atmospheric lead: the southern hemisphere. Geochim. Cosmochim. Acta 64, 3251–3262. doi: 10.1016/S0016-7037(00)00436-1
Bollhöfer, A., and Rosman, K. J. R. (2001). Isotopic source signatures for atmospheric lead: the northern hemisphere. Geochim. Cosmochim. Acta 65, 1727–1740. doi: 10.1016/S0016-7037(00)00630-X
Bonnail, E., Pérez-López, R., Sarmiento, A. M., Nieto, J. M., and DelValls, T. Á (2017). A novel approach for acid mine drainage pollution biomonitoring using rare earth elements bioaccumulated in the freshwater clam Corbicula fluminea. J. Hazard. Mater. 338, 466–471. doi: 10.1016/j.jhazmat.2017.05.052
Boyle, E. A. (2019). “Anthropogenic trace elements in the ocean,” in Encyclopedia of Ocean Sciences, Amsterdam: Elsevier Ltd, 195–202. doi: 10.1016/B978-0-12-409548-9.11592-1
Boyle, E. A., Chapnick, S. D., Shen, G. T., and Bacon, M. P. (1986). Temporal variability of lead in the western North Atlantic. J. Geophys. Res. 91, 8573–8593. doi: 10.1029/JC091iC07p08573
Boyle, E. A., Lee, J.-M., Echegoyen, Y., Noble, A., Moos, S., Carrasco, G., et al. (2014). Anthropogenic lead emissions in the ocean: the evolving global experiment. Oceanography 27, 69–75. doi: 10.5670/oceanog.2014.10
Bruland, K., Lohan, M. C., Aguilar-Islas, A. M., Smith, G. J., Sohst, B., and Baptista, A. (2008). Factors influencing the chemistry of the near-field Columbia River plume: nitrate, silicic acid, dissolved Fe, and dissolved Mn. J. Geophys. Res. 113, 1–23. doi: 10.1029/2007JC004702
Bruland, K., Middag, R., and Lohan, M. C. (2013). “Controls of Trace Metals in Seawater,” in Treatise on Geochemistry: Second Edition, eds Mottl, J. Michael, Elderfield, and Henry (Philadelphia, PA: Elsevier Ltd), doi: 10.1016/B978-0-08-095975-7.00602-1
Buck, N. J., Gobler, C. J., and Sañudo-Wilhelmy, S. A. (2005). Dissolved trace element concentrations in the East River–Long Island Sound system: relative importance of autochthonous versus allochthonous sources. Environ. Sci. Technol. 39, 3528–3537. doi: 10.1021/es048860t
Carmichael, R. H., Jones, A. L., Patterson, H. K., Walton, W. C., Pérez-Huerta, A., Overton, E. B., et al. (2012). Assimilation of oil-derived elements by oysters due to the deepwater horizon oil spill. Environ. Sci. Technol. 46, 12787–12795. doi: 10.1021/es302369h
Casas, S., Gonzalez, J.-L., Andral, B., and Cossa, D. (2008). Relation between metal concentration in water and metal content of marine mussels (Mytilus galloprovincialis): impact of physiology. Environ. Toxicol. Chem. 27, 1543–1552. doi: 10.1897/07-418
Cebrian, E., Uriz, M. J., and Turon, X. (2007). Sponges as biomonitors of heavy metals in spatial and temporal surveys in northwestern Mediterranean: multispecies comparison. Environ. Toxicol. Chem. 26, 2430–2439. doi: 10.1897/07-292.1
Chan, H. M. (1989). Temporal and spatial fluctuations in trace metal concentrations in transplanted mussels in Hong Kong. Mar. Pollut. Bull. 20, 82–86. doi: 10.1016/0025-326X(89)90231-2
Chase, Z., Paytan, A., Beck, A., Biller, D., Bruland, K. W., Measures, C., et al. (2011). Evaluating the impact of atmospheric deposition on dissolved trace-metals in the Gulf of Aqaba, Red Sea. Mar. Chem. 126, 256–268. doi: 10.1016/j.marchem.2011.06.005
Chase, Z., Paytan, A., Johnson, K. S., Street, J., and Chen, Y. (2006). Input and cycling of iron in the Gulf of Aqaba, Red Sea. Global Biogeochem. Cycles 20, 1–11. doi: 10.1029/2005GB002646
Chen, Y., Paytan, A., Chase, Z., Measures, C., Beck, A., Sañudo-Wilhelmy, S. A., et al. (2008). Sources and fluxes of atmospheric trace elements to the Gulf of Aqaba, Red Sea. J. Geophys. Res. Atmos. 113, 1–13. doi: 10.1029/2007JD009110
Chien, C.-T., Benaltabet, T., Torfstein, A., and Paytan, A. (2019). Contributions of atmospheric deposition to Pb concentration and isotopic composition in seawater and particulate matters in the Gulf of Aqaba, Red Sea. Environ. Sci. Technol. 53, 6162–6170. doi: 10.1021/acs.est.9b00505
Chong, K., and Wang, W. X. (2001). Comparative studies on the biokinetics of Cd, Cr, and Zn in the green mussel Perna viridis and the Manila clam Ruditapes philippinarum. Environ. Pollut. 115, 107–121. doi: 10.1016/S0269-7491(01)00087-2
Crenshaw, M. A. (1972). The inorganic composition of molluscan extrapallial fluid. Biol. Bull. 143, 506–512. doi: 10.2307/1540180
Cziczo, D. J., Stetzer, O., Worringen, A., Ebert, M., Weinbruch, S., Kamphus, M., et al. (2009). Inadvertent climate modification due to anthropogenic lead. Nat. Geosci. 2, 333–336. doi: 10.1038/ngeo499
Dang, D. H., Schäfer, J., Brach-Papa, C., Lenoble, V., Durrieu, G., Dutruch, L., et al. (2015). Evidencing the impact of coastal contaminated sediments on mussels through Pb stable isotopes composition. Environ. Sci. Technol. 49, 11438–11448. doi: 10.1021/acs.est.5b01893
Dar, M. A., Belal, A. A., and Madkour, A. G. (2018). The differential abilities of some molluscs to accumulate heavy metals within their shells in the Timsah and the Great Bitter lakes, Suez Canal, Egypt. Egypt. J. Aquat. Res. 44, 291–298. doi: 10.1016/j.ejar.2018.11.008
de Baar, H. J. W. (1983). The Marine Geochemistry of the Rare Earth Elements. Falmouth, MA: Woods Hole Oceanographic Institution.
de Baar, H. J. W., Bacon, M. P., and Brewer, P. G. (1983). Rare-earth distributions with a positive Ce anomaly in the Western North Atlantic Ocean. Nature 301, 324–327. doi: 10.1038/301324a0
de Baar, H. J. W., Brewer, P. G., and Bacon, M. P. (1985). Anomalies in rare earth distributions in seawater: Gd and Tb. Geochim. Cosmochim. Acta 49, 1961–1969. doi: 10.1016/0016-7037(85)90090-0
Elderfield, H. (1988). The oceanic chemistry of the rare-earth elements. Philos. Trans. R. Soc. Lond. Ser. A Math. Phys. Sci. 325, 105–126.
Elderfield, H., and Greaves, M. J. (1982). The rare earth elements in seawater. Nature 296, 214–219. doi: 10.1038/296214a0
Elderfield, H., and Sholkovitz, E.R. t (1987). Rare earth elements in the pore waters of reducing nearshore sediments. Earth Planet. Sci. Lett. 82, 280–288. doi: 10.1016/0012-821x(87)90202-0
El-Gamal, M. M. (2011). The effect of depuration on heavy metals, petroleum hydrocarbons, and microbial contamination levels in Paphia undulata (Bivalvia: Veneridae). Czech J. Anim. Sci. 56, 345–354. doi: 10.17221/2395-cjas
Erel, Y., Axelrod, T., Veron, A., Mahrer, Y., Katsafados, P., and Dayan, U. (2002). Transboundary atmospheric lead pollution. Environ. Sci. Technol. 36, 3230–3233. doi: 10.1021/es020530q
Erel, Y., Dayan, U., Rabi, R., Rudich, Y., and Stein, M. (2006). Trans boundary transport of pollutants by atmospheric mineral dust. Environ. Sci. Technol. 40, 2996–3005. doi: 10.1021/es051502l
Farrington, J. W., Bowen, V. T., Goldberg, E. D., Risebrough, R. W., and Martin, J. H. (1983). U.S. ‘Mussel Watch’ 1976-1978: an overview of the trace-metal, DDE, PCB, hydrocarbon, and artificial radionuclide data. Environ. Sci. Technol. 17, 490–496. doi: 10.1021/es00114a010
Freitas, P. S., Clarke, L. J., Kennedy, H., and Richardson, C. A. (2016). Manganese in the shell of the bivalve Mytilus edulis: seawater Mn or physiological control? Geochim. Cosmochim. Acta 194, 266–278. doi: 10.1016/j.gca.2016.09.006
Fung, C. N., Lam, J. C. W., Zheng, G. J., Connell, D. W., Monirith, I., Tanabe, S., et al. (2004). Mussel-based monitoring of trace metal and organic contaminants along the east coast of China using Perna viridis and Mytilus edulis. Environ. Pollut. 127, 203–216. doi: 10.1016/j.envpol.2003.08.007
Genin, A. (2008). “The physical setting of the Gulf of Aqaba: an explanation for a unique occurrence of tropical communities in the subtropics,” in Aqaba-Eilat, the Improbable Gulf: Environment, Biodiversity and Preservation, ed. F. D. Por (Jerusalem: The Hebrew University Magnes Press), 15–20.
Genin, A., Lazar, B., and Brenner, S. (1995). Vertical mixing and coral death in the Red Sea following the eruption of Mount Pinatubo. Nature 377, 507–510. doi: 10.1038/377507a0
Göksu, M. Z. L., Akar, M., Çevik, F., and Findik, Ö (2005). Bioaccumulation of some heavy metals (Cd, Fe, Zn, Cu) in two bivalvia species (Pinctada radiata Leach, 1814 and Brachidontes pharaonis Fischer, 1870). Turk. J. Vet. Anim. Sci. 29, 89–93.
Goldberg, E. D. (1975). The mussel watch–a first step in global marine monitoring. Mar. Pollut. Bull. 6:111. doi: 10.1016/0025-326X(75)90271-4
Goldberg, E. D., Bowen, V. T., Farrington, J. W., Harvey, G., Martin, J. H., Parker, P. L., et al. (1978). The mussel watch. Environ. Conserv. 5, 101–125. doi: 10.1017/S0376892900005555
Gwenzi, W., Mangori, L., Danha, C., Chaukura, N., Dunjana, N., and Sanganyado, E. (2018). Sources, behaviour, and environmental and human health risks of high-technology rare earth elements as emerging contaminants. Sci. Total Environ. 636, 299–313. doi: 10.1016/j.scitotenv.2018.04.235
Hamed, E. S. A. E., Khaled, A., Ahdy, H., Omar Ahmed, H., and Aly Abdelrazek, F. (2020). Health risk assessment of heavy metals in three invertebrate species collected along Alexandria Coast, Egypt. Egypt. J. Aquat. Res. 46, 389–395. doi: 10.1016/j.ejar.2020.11.001
Hatje, V., Bruland, K., and Flegal, A. R. (2014). Determination of rare earth elements after pre-concentration using NOBIAS-chelate PA-1® resin: method development and application in the San Francisco Bay plume. Mar. Chem. 160, 34–41. doi: 10.1016/j.marchem.2014.01.006
Hatje, V., Bruland, K. W., and Flegal, A. R. (2016). Increases in anthropogenic gadolinium anomalies and rare earth element concentrations in San Francisco Bay over a 20 year record. Environ. Sci. Technol. 50, 4159–4168. doi: 10.1021/acs.est.5b04322
Herut, B., Kress, N., Shefer, E., and Hornung, H. (1999). Trace element levels in mollusks from clean and polluted coastal marine sites in the Mediterranean, Red and North Seas. Helgol. Mar. Res. 53, 154–162. doi: 10.1007/s101520050021
Horiguchi, T. (2006). Masculinization of female gastropod mollusks induced by organotin compounds, focusing on mechanism of actions of tributyltin and triphenyltin for development of imposex. Environ. Sci. 13, 77–87.
Hutchinson, T. H., Jha, A. N., and Dixon, D. R. (1995). The polychaete Platynereis dumerilii (audouin and milne-edwards): a new species for assessing the hazardous potential of chemicals in the marine environment. Ecotoxicol. Environ. Saf. 31, 271–281. doi: 10.1006/eesa.1995.1074
Jickells, T. D., Baker, A. R., and Chance, R. (2016). Atmospheric transport of trace elements and nutrients to the oceans. Philos. Trans. R. Soc. A Math. Phys. Eng. Sci. 374:20150286. doi: 10.1098/rsta.2015.0286
John, S. G., Park, J. G., Zhang, Z., and Boyle, E. A. (2007). The isotopic composition of some common forms of anthropogenic zinc. Chem. Geol. 245, 61–69. doi: 10.1016/j.chemgeo.2007.07.024
Karayakar, F., Erdem, C., and Cicik, B. (2007). Seasonal variation in copper, zinc, chromium, lead and cadmium levels in hepatopancreas, gill and muscle tissues of the mussel Brachidontes pharaonis Fischer, collected along the Mersin coast, Turkey. Bull. Environ. Contam. Toxicol. 79, 350–355. doi: 10.1007/s00128-007-9246-z
Katz, T., Ginat, H., Eyal, G., Steiner, Z., Braun, Y., Shalev, S., et al. (2015). Desert flash floods form hyperpycnal flows in the coral-rich Gulf of Aqaba, Red Sea. Earth Planet. Sci. Lett. 417, 87–98. doi: 10.1016/j.epsl.2015.02.025
Komárek, M., Ettler, V., Chrastný, V., and Mihaljevič, M. (2008). Lead isotopes in environmental sciences: a review. Environ. Int. 34, 562–577. doi: 10.1016/j.envint.2007.10.005
Kümmerer, K., and Helmers, E. (2000). Hospital effluents as a source of gadolinium in the aquatic environment. Environ. Sci. Technol. 34, 573–577. doi: 10.1021/es990633h
Labonne, M., Ben Othman, D., and Luck, J. M. (1998). Recent and past anthropogenic impact on a mediterranean lagoon: lead isotope constraints from mussel shells. Appl. Geochem. 13, 885–892. doi: 10.1016/S0883-2927(98)00016-X
Labonne, M., Ben Othman, D., and Luck, J. M. (2001). Pb isotopes in mussels as tracers of metal sources and water movements in a lagoon (Thau Basin, S. France). Chem. Geol. 181, 181–191. doi: 10.1016/S0009-2541(01)00281-9
Lazar, B., Erez, J., Silverman, J., Rivlin, T., Rivlin, A., Dray, M., et al. (2008). “Recent environmental changes in the chemical-biological oceanography of the Gulf of Aqaba (Eilat),” in Aqaba-Eilat, Improbable Gulf. Environment, Biodiversity and Preservation, ed. F. D. Por (Jerusalem: Magnes Press), 49–61.
Lee, J. M., Boyle, E. A., Gamo, T., Obata, H., Norisuye, K., and Echegoyen, Y. (2015). Impact of anthropogenic Pb and ocean circulation on the recent distribution of Pb isotopes in the Indian Ocean. Geochim. Cosmochim. Acta 170, 126–144. doi: 10.1016/j.gca.2015.08.013
Li, J. X., Zheng, L., Sun, C. J., Jiang, F. H., Yin, X. F., Chen, J. H., et al. (2016). Study on ecological and chemical properties of rare earth elements in tropical marine organisms. Chin. J. Anal. Chem. 44, 1539–1546. doi: 10.1016/S1872-2040(16)60963-5
Liu, X., and Wang, W.-X. (2016). Time changes in biomarker responses in two species of oyster transplanted into a metal contaminated estuary. Sci. Total Environ. 544, 281–290. doi: 10.1016/j.scitotenv.2015.11.120
Lorenzo, J. I., Aierbe, E., Mubiana, V. K., Blust, R., and Beiras, R. (2003). “Indications of regulation on copper accumulation in the blue mussel Mytilus edulis,” in Molluscan Shellfish Safety, eds A. Villalba, B. Reguera, J. L. Romalde, and R. Beiras (London: UNESCO), 533–544.
Ma, L., Dang, D. H., Wang, W., Evans, R. D., and Wang, W.-X. (2019). Rare earth elements in the Pearl River Delta of China: potential impacts of the REE industry on water, suspended particles and oysters. Environ. Pollut. 244, 190–201. doi: 10.1016/j.envpol.2018.10.015
Mahowald, N. M., Engelstaedter, S., Luo, C., Sealy, A., Artaxo, P., Benitez-Nelson, C., et al. (2009). Atmospheric iron deposition: global distribution, variability, and human perturbations. Ann. Rev. Mar. Sci. 1, 245–278. doi: 10.1146/annurev.marine.010908.163727
Mclennan, S. M. (1989). Rare earth elements in sedimentary rocks: influence of provenance and sedimentary processes. Geochem. Mineral. Rare Earth Elem. Rev. Mineral. 21, 169–200. doi: 10.1515/9781501509032-010
Moloukhia, H., and Sleem, S. (2011). Bioaccumulation, fate and toxicity of two heavy metals common in industrial wastes in two aquatic molluscs. J. Am. Sci. 7, 459–464.
Morton, B. (1988). The population dynamics and reproductive cycle of Brachidontes variabilis (Bivalvia: Mytilidae) in a Hong Kong mangrove. Malacol. Rev. 21, 109–117.
Mubiana, V. K., and Blust, R. (2007). Effects of temperature on scope for growth and accumulation of Cd, Co, Cu and Pb by the marine bivalve Mytilus edulis. Mar. Environ. Res. 63, 219–235. doi: 10.1016/j.marenvres.2006.08.005
Naimo, T. J. (1995). A review of the effects of heavy metals on freshwater mussels. Ecotoxicology 4, 341–362. doi: 10.1007/BF00118870
Nozaki, Y., Lerche, D., Alibo, D. S., and Tsutsumi, M. (2000). Dissolved indium and rare earth elements in three Japanese rivers and Tokyo Bay: evidence for anthropogenic Gd and In. Geochim. Cosmochim. Acta 64, 3975–3982. doi: 10.1016/S0016-7037(00)00472-5
Nriagu, J. O., and Pacyna, J. M. (1988). Quantitative assessment of worldwide contamination of air, water and soils by trace metals. Nature 333, 134–139. doi: 10.1038/333134a0
Pattan, J. N., Pearce, N. J. G., and Mislankar, P. G. (2005). Constraints in using Cerium-anomaly of bulk sediments as an indicator of paleo bottom water redox environment: a case study from the Central Indian Ocean Basin. Chem. Geol. 221, 260–278. doi: 10.1016/j.chemgeo.2005.06.009
Phillips, D. J. H. (1976). The common mussel Mytilus edulis as an indicator of pollution by zinc, cadmium, lead and copper. II. Relationship of metals in the mussel to those discharged by industry. Mar. Biol. 38, 71–80. doi: 10.1007/BF00391487
Phillips, D. J. H. (1980). Quantitative Aquatic Biological Indicators: Their Use to Monitor Trace metal and Organochlorine Pollution. London: Applied Science Publishers.
Rainbow, P. S. (2002). Trace metal concentrations in aquatic invertebrates: why and so what? Environ. Pollut. 120, 497–507. doi: 10.1016/S0269-7491(02)00238-5
Raisuddin, S., Kwok, K. W. H., Leung, K. M. Y., Schlenk, D., and Lee, J. S. (2007). The copepod Tigriopus: a promising marine model organism for ecotoxicology and environmental genomics. Aquat. Toxicol. 83, 161–173. doi: 10.1016/j.aquatox.2007.04.005
Richardson, C., Chenery, S., and Cook, J. (2001). Assessing the history of trace metal (Cu, Zn, Pb) contamination in the North Sea through laser ablation-ICP-MS of horse mussel Modiolus modiolus shells. Mar. Ecol. Prog. Ser. 211, 157–167. doi: 10.3354/meps211157
Roditi, H. A., Fisher, N. S., and Sañudo-Wilhelmy, S. A. (2000). Field testing a metal bioaccumulation model for zebra mussels. Environ. Sci. Technol. 34, 2817–2825. doi: 10.1021/es991442h
Russell Flegal, A., and Stukas, V. J. (1987). Accuracy and precision of lead isotopic composition measurements in seawater. Mar. Chem. 22, 163–177. doi: 10.1016/0304-4203(87)90006-5
Sasekumar, A. (1974). Distribution of macrofauna on a Malayan mangrove shore. J. Anim. Ecol. 43, 51–69. doi: 10.2307/3157
Shelley, R. U., Morton, P. L., and Landing, W. M. (2015). Elemental ratios and enrichment factors in aerosols from the US-GEOTRACES North Atlantic transects. Deep. Res. 2 Top. Stud. Oceanogr. 116, 262–272. doi: 10.1016/j.dsr2.2014.12.005
Squadrone, S., Brizio, P., Stella, C., Mantia, M., Battuello, M., Nurra, N., et al. (2019). Rare earth elements in marine and terrestrial matrices of Northwestern Italy: implications for food safety and human health. Sci. Total Environ. 660, 1383–1391. doi: 10.1016/j.scitotenv.2019.01.112
Steding, D. J., Dunlap, C. E., and Flegal, A. R. (2000). New isotopic evidence for chronic lead contamination in the San Francisco Bay estuary system: implications for the persistence of past industrial lead emissions in the biosphere. Proc. Natl. Acad. Sci. U.S.A. 97, 11181–11186. doi: 10.1073/pnas.180125697
Taylor, J. D. (1971). Reef associated molluscan assemblages in the western Indian Ocean. Symp. Zool. Soc. Lond. 28, 501–534.
Taylor, S. R., and McLennan, S. M. (1985). The Continental Crust: Its Composition and Evolution. Oxford: Blackwell Scientific Publications.
Ternon, E., Guieu, C., Löye-Pilot, M. D., Leblond, N., Bosc, E., Gasser, B., et al. (2010). The impact of Saharan dust on the particulate export in the water column of the North Western Mediterranean Sea. Biogeosciences 7, 809–826. doi: 10.5194/bg-7-809-2010
Torfstein, A., Goldstein, S. L., and Stein, M. (2018). Enhanced Saharan dust input to the levant during Heinrich stadials. Quat. Sci. Rev. 186, 142–155. doi: 10.1016/j.quascirev.2018.01.018
Torfstein, A., Kienast, S. S., Yarden, B., Rivlin, A., Isaacs, S., and Shaked, Y. (2020). Bulk and export production fluxes in the Gulf of Aqaba, Northern Red Sea. ACS Earth Space. Chem. 4, 1461–1479. doi: 10.1021/acsearthspacechem.0c00079
Torfstein, A., Teutsch, N., Tirosh, O., Shaked, Y., Rivlin, T., Zipori, A., et al. (2017). Chemical characterization of atmospheric dust from a weekly time series in the north Red Sea between 2006 and 2010. Geochim. Cosmochim. Acta 211, 373–393. doi: 10.1016/j.gca.2017.06.007
Toyoda, K., Nakamura, Y., and Masuda, A. (1990). Rare earth elements of Pacific pelagic sediments. Geochim. Cosmochim. Acta 54, 1093–1103. doi: 10.1016/0016-7037(90)90441-M
Turekian, K. K. (1977). The fate of metals in the oceans. Geochim. Cosmochim. Acta 41, 1139–1144. doi: 10.1016/0016-7037(77)90109-0
Tzafriri-Milo, R., Benaltabet, T., Torfstein, A., and Shenkar, N. (2019). The potential use of invasive ascidians for biomonitoring heavy metal pollution. Front. Mar. Sci. 6:611. doi: 10.3389/fmars.2019.00611
Van der Oost, R., Beyer, J., and Vermeulen, N. P. E. (2003). Fish bioaccumulation and biomarkers in environmental risk assessment: a review. Environ. Toxicol. Pharmacol. 13, 57–149. doi: 10.1016/S1382-6689(02)00126-6
Van Geen, A., Adkins, J. F., Boyle, E. A., Nelson, C. H., and Palanques, A. (1997). A120 yr record of widespread contamination from mining of the Iberian pyrite belt. Geology 25, 291–294. doi: 10.1130/0091-76131997025<0291:AYROWC<2.3.CO;2
Wang, Z., Yin, L., Xiang, H., Qin, X., and Wang, S. (2019). Accumulation patterns and species-specific characteristics of yttrium and rare earth elements (YREEs) in biological matrices from Maluan Bay, China: implications for biomonitoring. Environ. Res. 179:108804. doi: 10.1016/j.envres.2019.108804
White, S. L., and Rainbow, P. S. (1982). Regulation and accumulation of copper, zinc and cadmium by the shrimp Palaemon elegans. Mar. Ecol. Prog. Ser. 8, 95–101. doi: 10.3354/meps008095
Wielgus, J., Chadwick-Furman, N. E., and Dubinsky, Z. (2004). Coral cover and partial mortality on anthropogenically impacted coral reefs at Eilat, northern Red Sea. Mar. Pollut. Bull. 48, 248–253. doi: 10.1016/j.marpolbul.2003.08.008
Xu, Y., Sun, Q., Yi, L., Yin, X., Wang, A., Li, Y., et al. (2014). The source of natural and anthropogenic heavy metals in the sediments of the Minjiang River Estuary (SE China): implications for historical pollution. Sci. Total Environ. 493, 729–736. doi: 10.1016/j.scitotenv.2014.06.046
Yap, C. K., Ismail, A., Tan, S. G., and Omar, H. (2003). Accumulation, depuration and distribution of cadmium and zinc in the green- lipped mussel Perna viridis (Linnaeus) under laboratory conditions. Hydrobiologia 498, 151–160.
Yin, Y., Huang, J., Paine, M. L., Reinhold, V. N., and Chasteen, N. D. (2005). Structural characterization of the major extrapallial fluid protein of the mollusc Mytilus edulis: implications for function. Biochemistry 44, 10720–10731. doi: 10.1021/bi0505565
Zega, G., Pennati, R., Candiani, S., Pestarino, M., and De Bernardi, F. (2009). Solitary ascidians embryos (Chordata, Tunicata) as model organisms for testing coastal pollutant toxicity. Isj 6, 29–34.
Zhou, Q., Zhang, J., Fu, J., Shi, J., and Jiang, G. (2008). Biomonitoring: an appealing tool for assessment of metal pollution in the aquatic ecosystem. Anal. Chim. Acta 606, 135–150. doi: 10.1016/j.aca.2007.11.018
Keywords: biomonitoring, mussels, depuration, heavy metals, Pb isotopes, rare earth elements, red sea, pollution
Citation: Benaltabet T, Gutner-Hoch E and Torfstein A (2021) Heavy Metal, Rare Earth Element and Pb Isotope Dynamics in Mussels During a Depuration Experiment in the Gulf of Aqaba, Northern Red Sea. Front. Mar. Sci. 8:669329. doi: 10.3389/fmars.2021.669329
Received: 18 February 2021; Accepted: 11 May 2021;
Published: 28 June 2021.
Edited by:
Kenneth Mei Yee Leung, City University of Hong Kong, Hong Kong, SAR ChinaReviewed by:
Chee Kong Yap, Putra Malaysia University, MalaysiaPaula Sánchez Marín, Spanish Institute of Oceanography, Spain
Copyright © 2021 Benaltabet, Gutner-Hoch and Torfstein. This is an open-access article distributed under the terms of the Creative Commons Attribution License (CC BY). The use, distribution or reproduction in other forums is permitted, provided the original author(s) and the copyright owner(s) are credited and that the original publication in this journal is cited, in accordance with accepted academic practice. No use, distribution or reproduction is permitted which does not comply with these terms.
*Correspondence: Tal Benaltabet, dGFsLmJlbmFsdGFiZXRAbWFpbC5odWppLmFjLmls