- 1Institute for the Study of Earth, Oceans, and Space, University of New Hampshire, Durham, NH, United States
- 2Marine Science Institute, University of California, Santa Barbara, Santa Barbara, CA, United States
- 3Lamont-Doherty Earth Observatory, Columbia University, Palisades, NY, United States
- 4Memorial University of Newfoundland, St. John’s, NL, Canada
Sediment resuspension caused by near-bed currents mediates exchange processes between the seafloor and the overlying water column, known as benthic-pelagic coupling. To investigate the effects of sediment resuspension on microbial enzyme activities in bottom waters (<500 m), we conducted onboard erosion experiments using sediment cores taken with a multi-corer from six deep-sea sites in the northern Gulf of Mexico. We then incubated the core-top water with resuspended sediments in roller tanks to simulate bottom water conditions following sediment resuspension. Bacterial cell abundance, particulate organic matter content, and potential rates of three hydrolytic enzymes (leucine aminopeptidases – PEP; β-glucosidases – GLU, lipases – LIP) were monitored during the experimentally-generated erosion events and subsequently in the roller tanks to examine whether resuspension of deep-sea sediments enhances activities of extracellular enzymes in overlying waters. Surficial sediments were resuspended at critical shear stress velocities between 1.4 and 1.7 cm s–1, which parallel bottom water currents of 28 and 34 cm s–1. Only one of our nine cores resisted experimentally generated bottom shear stresses and remained undisturbed, possibly as a result of oil residues from natural hydrocarbon seeps at the investigated site. The most notable enzymatic responses to sediment resuspension were found for LIP activities that increased in overlying waters of all eight of our resuspended cores and remained at high levels during the roller tank incubations. PEP and GLU showed orders of magnitude lower rates and more variable responses to experimentally resuspended sediments compared with LIP. We also found a disconnect between enzyme activities and bacterial cell numbers, indicating a major role of extracellular enzymes physically disconnected from microbial cells in our experiments. Our results demonstrate that sediment resuspension may promote organic matter breakdown in bottom waters by supplying extracellular enzymes without requiring a bacterial growth response. The marked increase in LIP activity suggests that resuspended enzymes may affect the degradation of petroleum hydrocarbons, including those from the natural seeps that are abundant in the investigation area.
Introduction
Benthic-pelagic coupling in aquatic environments involves the exchange of dissolved and particulate matter from the sediment to the overlying water and vice versa. Resuspension of sedimentary matter by near-bed currents plays a key role in these exchanges, affecting ecosystem functions from nutrient fluxes to energy transfer into food webs (Graf, 1992). Due to their shallow water columns, coastal and estuarine systems are particularly prone to sediment resuspension and such transfers (Griffiths et al., 2017), fueling microbial food webs in overlying waters (Ritzrau and Graf, 1992; Ritzrau, 1996; Ritzrau et al., 1997; Boetius et al., 2000; Ziervogel and Arnosti, 2009; Ziervogel et al., 2016). By comparison, information on benthic-pelagic coupling mediated by sediment resuspension in the deep-sea (>500 m depth) is relatively rare and limited to a few oceanographic regions (Turley, 2000), although resuspension events in the deep ocean are widespread and common (Durrieu de Madron et al., 2017).
The few existing studies on deep ocean biogeochemical cycles affected by sediment resuspension demonstrate the importance of upward fluxes for bathypelagic microbial food webs. For instance, Boetius et al. (2000) found close correlations between total particulate matter and heterotrophic microbial activities in near-bed waters in the deep Arabian Sea (2,000–4,500 m water depths), concluding that heterotrophic metabolism in bottom waters was stimulated by particulate and dissolved organic matter resuspended from the seafloor. Our own previous work in the deep (1,600 m) Gulf of Mexico (GOM) showed elevated microbial metabolic activities in turbid bottom waters containing high levels of lithogenic matter as an indicator for resuspended sediments (Ziervogel et al., 2016). Furthermore, budget calculations revealed that the amount of particulate and dissolved organic matter released through resuspended sediments in the deep ocean could close budget gaps between the carbon supplied to the benthos through sinking organic matter and the prokaryotic carbon demand in the deep-sea (Pfannkuche, 1993; Boetius et al., 2000; Baltar et al., 2010).
The above-mentioned studies on deep ocean microbial responses to sediment resuspension based their interpretations of benthic-pelagic coupling on correlation analysis of microbial metabolic rates and total suspended matter in bottom waters. However, these earlier studies could not distinguish between the effects of locally resuspended versus laterally advected sediment suspensions on near-bed microbial metabolic rates at the investigated sites (Ziervogel et al., 2016). This limitation can be resolved by using erosion chambers or flumes, to simulate local resuspension events of autochthonous sedimentary matter. Moreover, defined hydrodynamic conditions within erosion chambers and flumes allow for the determination of bottom shear stresses at which erosion first begins (i.e., the critical shear stress velocity, u∗crit), an important parameter for predicting benthic-pelagic exchange processes in the field.
Past experimental studies with erosion chambers and flumes have focused on the release and vertical transport of organic matter, nutrients, and bulk seabed material during sediment erosion (Wainright, 1987; Thomsen and Gust, 2000; Ziervogel and Bohling, 2003; Kalnejais et al., 2007).
This study focused on the activities of extracellular enzymes in bottom waters following sediment resuspension, which has rarely been investigated in the deep ocean. We conducted onboard erosion chamber experiments on sediment cores followed by roller tank incubations of the core-top water and resuspended material. Experiments were performed at six deep-sea sites in the northern GOM. The sites were located in areas known for their deep-sea coral habitats (AT357, VK826, DC673; White et al., 2012; Fisher et al., 2014); for natural active oil and gas seepage (GC600, MC118; MacDonald et al., 2015; Conti et al., 2016; Martens et al., 2016); and for oil input during the Deepwater Horizon (DWH) oil spill in 2010 (OC26; Atlas and Hazen, 2011; Chanton et al., 2015; Diercks et al., 2021; Romero et al., 2021). Our aim was to examine whether resuspension of deep-sea sediments enhances activities of extracellular enzymes in overlying waters. The focus on enzymatic activities stems from the fact that microbial degradation of complex organic matter is initiated by extracellular enzymes that hydrolyze substrates in the medium outside the cells to sizes sufficiently small for cellular uptake (Arnosti, 2011). We measured potential activities of leucine aminopeptidases, β-glucosidases, and lipases, mediating enzymatic breakdown of peptides, carbohydrates, and lipids, respectively, representing major compound classes within the ocean’s organic matter pool. Lipases are also involved in the biodegradation cascade of petroleum hydrocarbons (Margesin et al., 1999; Kamalanathan et al., 2018; Mislan and Gates, 2019), which are an important carbon source for microbial communities in the investigation area (Orcutt et al., 2010; Ziervogel et al., 2014).
Materials and Methods
Sampling and Site Description
Nine sediment cores (10 cm in diameter) were collected from six different sites in the northern GOM in June/July 2013 during RV Endeavor cruise 527 (Figure 1 and Table 1) using a multi-corer (MC-800, Ocean Instruments). Immediately after recovery, sediment cores with an undisturbed sediment-water interface indicated by visually undisturbed (clear) water overlying the core, were stored in the dark at 5°C until the onset of the onboard experiments (usually within 12 h of sampling). The experimental procedure for each core consisted of an erosion experiment lasting 40–80 min in total, where an erosion chamber was used to resuspend surface sediment, followed by a 10-h roller tank incubation, that simulated conditions in bottom waters containing resuspended sediments. Note that replicate cores for the present study were only available at two of the six sites (Table 1). The remaining sediment cores from each multi-corer cast (8 cores in total) were sectioned and analyzed for different biogeochemical parameters including bulk organic matter (OM) content by loss of ignition, which are reported in Table 1. The complete dataset of sediment parameters is publicly available through the Gulf of Mexico Research Initiative Information & Data Cooperative (GRIIDC) (Joye, 2016). Note that grain-size analysis was not conducted on any of the cores from this expedition. We therefore report published results on sediment properties from other sampling campaigns in the investigation area (Table 1; Montagna et al., 2013).
Experimental Procedures: Erosion Chamber and Roller Tanks
Sediment cores with overlying water were slowly pushed up into an EROMES laboratory erosion chamber (Kalnejais et al., 2007), yielding a total length of the sediment core in the chamber of 20 cm for each of the experiments. The EROMES consist of a baffled 10 cm inner diameter acrylic core (i.e., same diameter as the sampling cores) with a propeller on top that is connected to a stepper motor. The rotating propeller creates movement of the overlying water, generating defined bottom shear stress velocities (u∗) along the sediment-water interface. The chamber was calibrated prior to the expedition, by determining sediment transport of sieved sand as a function of propeller speed. The shear stress required to resuspend sediment grains of known sizes was determined from the Shield’s threshold curve for sediment erosion (Soulsby and Whitehouse, 1997). The calibration yielded a linear relationship between rotation speed of the propeller and the respective u∗crit values.
The erosion experiments were conducted at room temperature with the chambers kept in an ice bath to maintain close to in situ temperature (5°C) at the sediment-water interface. During experiments, the propeller speed was increased in four to eight steps of 10-min each, yielding a range of u* from 0.4 to 2.4 cm s–1, i.e., the maximum u∗ setting for this instrument. Experiments ended when the water became visibly turbid. The relationship between u∗ near the sediment interface and the overlying freestream velocity (u) is given by the quadratic stress law (e.g., Dyer, 1986):
where τ is the bottom shear stress (Pa), ρ is the fluid density (kg m–3), and CD is the dimensionless drag coefficient of 0.0025 (Ross et al., 2009). Thus, the range of u∗ in our experiments was equivalent to free-stream current velocities above the seafloor (u) of 8 to 48 cm s–1. We defined u∗crit as the velocity when total particulate matter (TPM) values in the overlying core water increased above 100 mg (for the total 1700 ml volume). Initial TPM values before erosion were always ≤50 mg.
In all cases, the sediment-water interface remained visually undisturbed following the lowest u∗ setting (u*INITIAL = 0.4 cm s–1). This low-flow condition represented a more realistic initial condition than stagnant water prior to the onset of the erosion experiment. At the end of the initial and each subsequent 10-min u∗ step, the overlying water was sampled for the biogeochemical parameters described below (Section “Analytical Methods”). The sample volume removed from the overlying water at each sampling (i.e., 150 mL of 1,700 mL total volume) was replaced with GF/F filtered near-bottom water (i.e., ∼5 m above the seafloor) collected from the same site with a CTD rosette. The dilution, which never exceeded 11% for each erosion step, was accounted for when values of measured parameters were calculated.
To simulate bottom water conditions following sediment resuspension, we collected the water overlying the cores after the final erosion step and transferred the water to roller tanks for an additional 10-h incubation. Cylindrical roller tanks incubated on a roller table are widely used to study suspended particle interactions and associated biogeochemical processes under non-turbulent conditions (Shanks and Edmondson, 1989; Unanue et al., 1998; Ploug et al., 2010). Thus, our two-phased experimental approach enabled us to investigate potential effects of sediment resuspension on bottom water heterotrophic activities in the investigation area.
Acrylic roller tanks (total volume: 1.700 mL) were filled without a headspace with the experimental core water after the final erosion step. The core water was carefully siphoned off the sediment core, leaving behind a small volume of water to prevent further disturbance of the sediment-water interface. The tanks were incubated in the dark at in situ temperature (5°C) on a roller table rotating at 2.4 rpm for 10 h, allowing for sufficient time to process the tanks before the start of the next erosion experiment. After the 10-h incubation, the tanks were removed from the roller table and placed upright on a benchtop at room temperature (∼20°C) to allow particles to settle to the bottom of the tanks for 10 min. The upper (visually particle-free) water fraction was carefully siphoned off into a clean beaker. This fraction, hereafter called the surrounding seawater (SSW) fraction, constituted 80% of the tank volume. The second fraction (sediment slurry; SL) contained settled particles in a measured volume of SSW (20% of the tank volume). Both, SSW and SL fractions were separately analyzed for the biogeochemical parameters listed below. As a non-resuspension control, we incubated near-bottom water from a CTD rosette cast at GC600 that did not contain visual particles.
Roller tank incubations were not performed on cores from OC26_1 and DC673. The former core spilled after the conclusion of the erosion experiment, and the latter was taken near the end of the expedition which did not leave enough time for a roller tank experiment. Instead, we decided to extend the erosion experiment with the DC673 core by adding additional shear stress steps (Figure 2F).
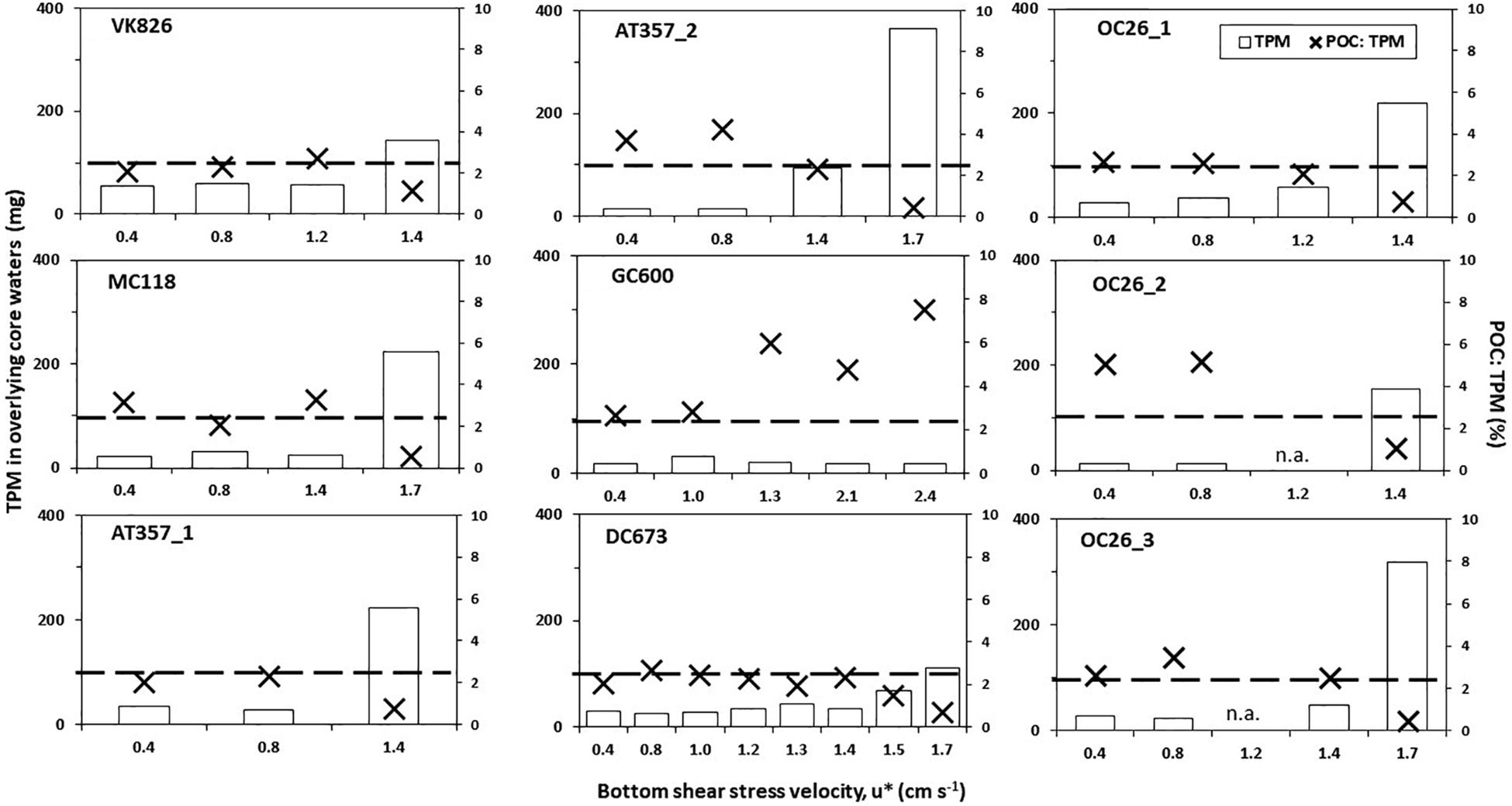
Figure 2. Changes in total particulate matter (TPM; white bars) and organic matter content (POC: TPM; black crosses) in the overlying core waters as a factor of bottom shear stress velocities (u∗) during the erosion chamber experiments. TPM were standardized for the total volume of the core water in each case. Dashed line stands for the TPM threshold for type 2 erosion (u∗crit; see text).
Analytical Methods
Total particulate matter (TPM) was analyzed in the overlying water from the erosion experiments at the end of each shear stress step and in the two fractions from the subsequent roller tank incubations (SL, SSW). TPM was analyzed by filtering experimental water onto pre-combusted and pre-weighed GF/F filters with a nominal pore size of 0.7 μm (Whatman). Filters were rinsed with milli Q water and stored frozen at −20°C until they were re-weighed in the home lab, following a drying period at 60°C for 24 h.
Particulate organic carbon (POC) was analyzed in the overlying water from the erosion experiments at the end of each shear stress step and the two roller tank fractions (SL, SSW). Subsamples were filtered onto pre-combusted GF/F filters that were stored frozen at −20°C until analysis. Filters were acidified with 12 M HCl for 12 h to remove inorganic carbon prior to flash combustion on a Carlo-Erba 1500 Elemental Analyzer using acetanilide as a standard (Knap, 2002).
Potential activities of β-glucosidases (GLU), leucine aminopeptidases (PEP), and lipases (LIP) were measured in the water overlying the sediment cores at the first and final u∗ steps, and in the roller tanks (SL, SSW). Potential enzyme activities were measured using 4-MUF-β-D-glucopyranoside, L-leucine-MCA hydrochloride, and 4-MUF-butyrate as fluorogenic substrate analogs for GLU, PEP, LIP, respectively (Hoppe, 1983). Three mL of experimental water were added to disposable methacrylate cuvettes containing fluorogenic substrate analogs at saturation levels (200 μM for 4-MUF-β-D-glucopyranoside and L-leucine-MCA hydrochloride; 100 μM for 4-MUF-butyrate) as determined at the beginning of the expedition. Duplicate cuvettes per substrate were incubated in the dark, at in situ temperature (5°C). Fluorescence was measured immediately after sample addition and at two additional time points over the course of 24 h under buffered conditions (20 nM borate buffer; pH 9.2) using a Turner Biosystems TBS-380 fluorometer (excitation/emission channels set to “UV”; 365 nm excitation, 440–470 nm emission). Fluorescence levels of standard solutions of methylumbelliferone (MUF) and methylcoumarin (MCA) in seawater were used to calculate potential hydrolysis rates from the assays. Minor changes in fluorescence over time were detected in killed controls (i.e., pasteurized seawater) and used to correct enzymatic hydrolysis rates in live water for abiotic substrate hydrolysis. Enzyme activities were not available from the DC673 erosion experiment.
Enzyme activities reported here represent potential hydrolysis rates, since added substrate proxies compete with naturally occurring substrates for enzyme active sites. Potential hydrolysis rates in this study are expressed as volumetric rates rather than normalized to cell abundance. Volumetric rates consider the total hydrolytic potential of the water including cell-free enzyme activities, thus assessing both, enzymes associated with the cells and those free in the surrounding medium. The latter fraction has been found to be particularly important in the deep ocean (Zhao et al., 2020) and in diffusion-limited environments such as sediments (Vetter et al., 1998).
Bacterial cells were enumerated by flow cytometry in subsamples from the overlying water from the erosion experiments (first and final u∗ steps only) and the two roller tank fractions. One mL subsamples were fixed with 0.1% glutaraldehyde (final concentration) for 10 min at room temperature in the dark and stored frozen at −80°C until analysis (within ∼2 weeks of sampling). Cellular DNA was stained using SYBR Green I (Gasol and Del Giorgio, 2000) and bacterial cells were counted on a BD Accuri C6 Flow Cytometer.
All analytical parameters measured here (TPM, bacterial cell numbers, enzyme activities) are expressed as absolute values standardized for the total volume of the respective experimental device (i.e., 1,700 mL in both cases). This allows for the direct comparison between the parameters measured in the erosion chamber and the roller tanks with the SL and SSW fractions.
Statistical Analysis
Pearson’s correlation coefficients (r) between enzymatic activities and TPM measured at the initial and the final erosion step from the eight eroded cores were calculated in Excel® using the data analysis tool pack. For this analysis we used the differences between the two erosion steps rather than the absolute values to account for variation in baseline enzyme activities among experiments.
Results
Visual examination of the nine cores revealed that none of the cores showed indications of recently sedimented material in the form of fluffy layers. Most notably, the core taken at GC600 had visible oil residues in the uppermost layer. Sediment cores from GC600 had higher organic matter content (OM) in the upper three centimeters (16% OM) than cores from the other sites where OM ranged between 9% (DC673) and 13% (VK826) (Table 1).
Erosion Thresholds
Eight of the nine cores reached TPM levels in the overlying water exceeding the 100 mg threshold, indicating u∗crit values between 1.4 cm s–1 (OC26_1, OC26_2, VK826, AT357_1) and 1.7 cm s–1 (OC26_3, MC118, AT357_2, and DC673). TPM levels in the GC600 core did not reach this threshold even at u∗ of 2.4 cm s–1 (i.e., maximum u∗ setting), ranging between 17.1 and 31.6 mg (Figure 2). The other cores showed TPM levels in the overlying water at u∗crit between 112 mg (DC673) to 366 mg (AT357_2). POC: TPM ratios as a measure of the organic matter content of the resuspended material varied between 0.4% (AT357_2) and 2.5% (OC26_3), and in most cores decreased with increasing shear stress reflecting the initial resuspension of lighter POC relative to mostly inorganic matter within the TPM pool at higher shear stresses.
Enzyme Activities in Response to Sediment Resuspension
Potential activities of the three enzymes assayed in our experiments showed similar levels among the cores and tanks, decreasing in the following order: lipase (LIP) > > peptidase (PEP) > glucosidase (GLU) (Table 2). LIP activities increased between the initial and final shear stress step in all of the resuspended cores by factors 1.5 (OC26_2, OC26_3, MC118), 2 (OC26_1, AT357_1, AT357_2) and 9 (VK826), following the increase in TPM (r = 0.7, p = 0.1, n = 7). The GC600 core that lacked sediment erosion showed a decrease in LIP activities by three orders of magnitude during the erosion chamber experiment. LIP activities remained at high levels at the end of the 10-hour roller tank incubations except for the VK826 tank that showed substantially lower LIP activities compared to the core water at u∗crit. Total LIP activities at the end of the VK826 tank experiment were almost 100% associated with the sediment slurry (SL), which had the highest POC: TPM ratio among the eroded cores (Table 3). LIP activities in the other six tanks were more evenly distributed between SSW and SL (Figure 3).
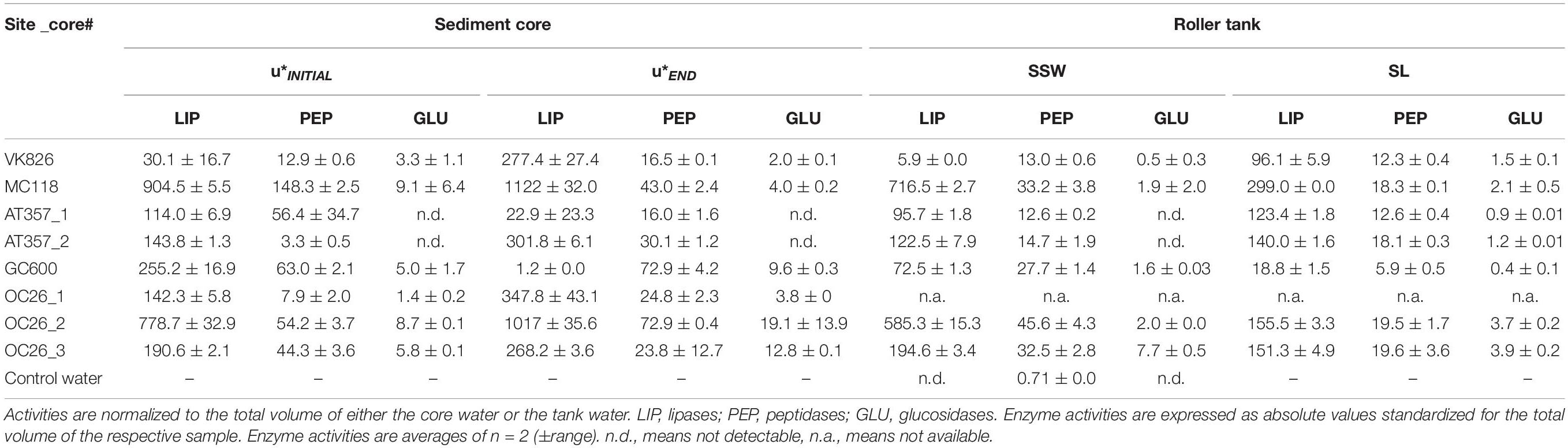
Table 2. Enzyme activities (nmol h−1) in the overlying core waters at the initial (u*INITIAL) and final (u*END) shear stress step during the erosion experiments, and at the end of the roller tank incubations with the partitioning between sediment slurry (SL) and surrounding seawater (SSW).
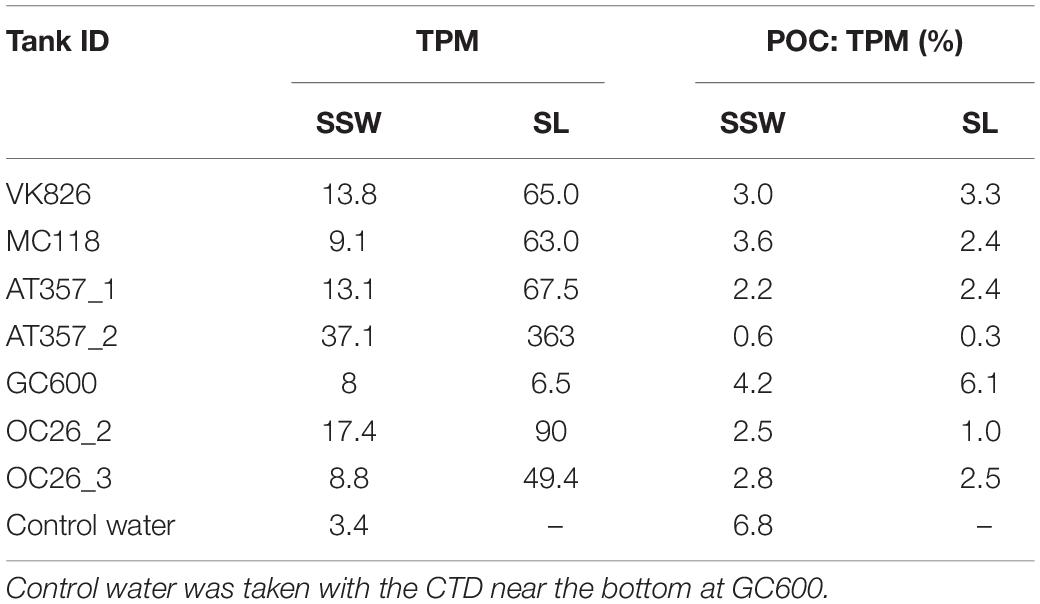
Table 3. Absolute values of total particulate matter (TPM, mg) and POC: TPM ratios at the end of roller tank incubations with the partitioning between sediment slurry (SL) and surrounding seawater (SSW).
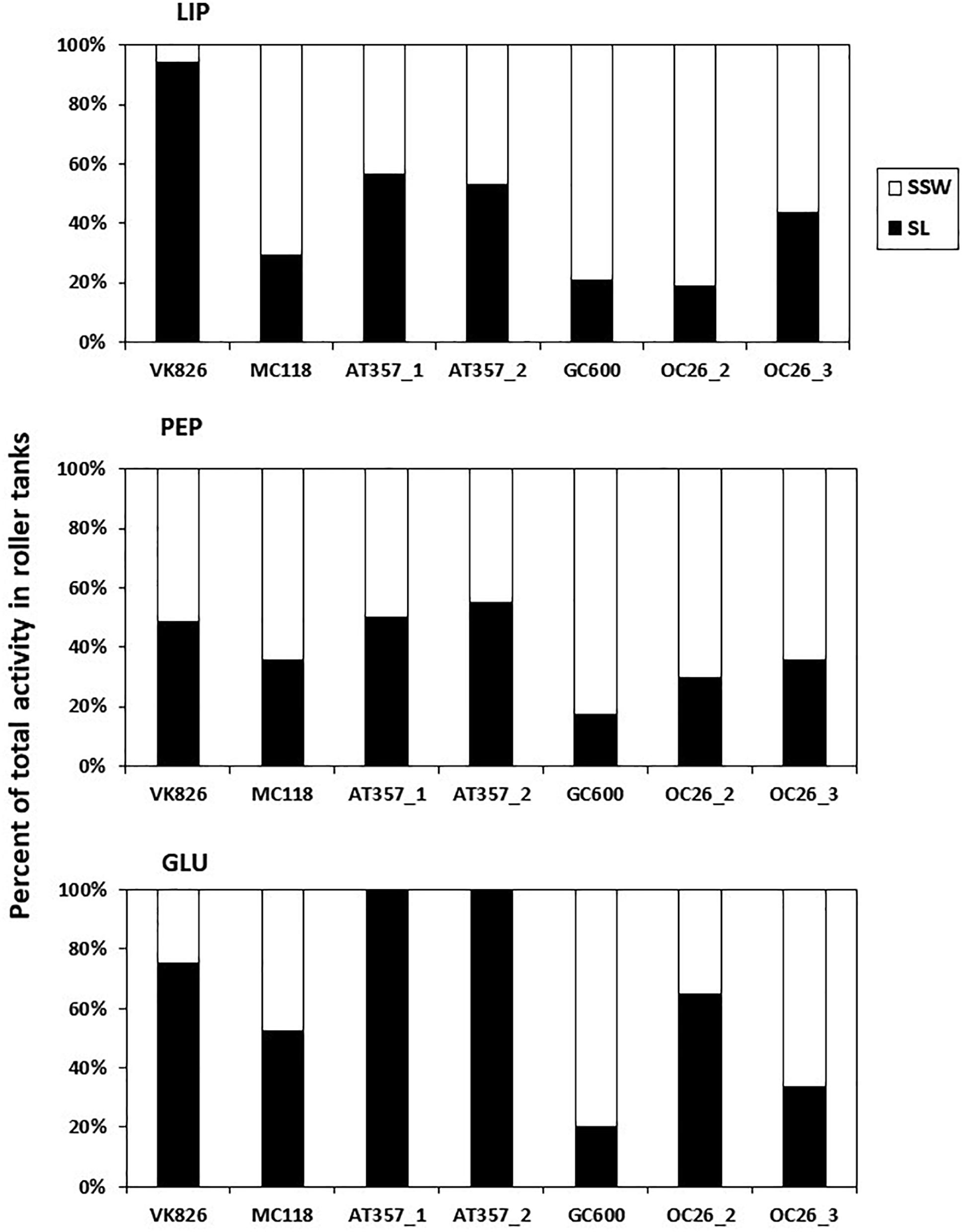
Figure 3. Percent distribution of absolute enzyme activities at the end of roller tank incubations with the partitioning between sediment slurry (SL) and surrounding seawater (SSW). LIP, lipases; PEP, peptidases; GLU, glucosidases.
Responses of PEP and GLU to sediment resuspension were more variable compared with LIP. PEP activities increased in four of the seven resuspended cores by factors 1.3 (OC26_2, VK826, GC600), 3 (OC26_1), and 9 (AT357_2) and decreased in the other three cores by factors 2 (OC26_3) and 3 (MC118, AT357_1) (Table 2). PEP activities at the end of the roller tank incubations were at the same level compared to u∗crit (OC26_2, GC600) or increased by up to a factor 2 (OC26_3, MC118, AT357_1, AT357_2) with 30–50% of activity associated with SL (Figure 3). GLU activities almost doubled between the initial and final shear stress step in the three OC26 cores and the GC600 core that lacked resuspension. At the same time, GLU decreased by a factor 2 in VK826 and MC118 while remaining undetectable throughout the erosion experiments with the two AT357 cores (Table 2). The non-resuspension control tank with bottom water taken with the CTD at GC600 showed only PEP activities among the three enzymes assayed at levels about one order of magnitude lower than those in the experimental water (Table 2).
Bacterial Cell Numbers in Response to Sediment Resuspension
Total bacterial cell numbers in overlying core waters at the beginning of the erosion experiments (after exposure of u∗ = 0.4 cm s–1) ranged between 1.8∗107 (OC26_3) and 13.3∗107 (MC118) (Figure 4). Bacterial cell numbers remained at the same level (± 20%; GC600, OC26_2, VK826, AT357_1, AT357_2) or decreased by a factor 2 (OC26_3, MC118) between the initial shear stress step and u∗crit. Bacterial cell numbers in the roller tank (SL + SSW) increased during four of the six roller tank incubations (OC26_3, VK826. MC118, GC600) or remained at the same levels compared to those at u∗crit (AT357_1, AT357_2). In contrast, cell numbers at the end of the OC26_2 roller tank incubations were a factor 6 lower compared to those at u∗crit. The non-resuspension control tank with bottom water taken with the CTD at GC600 had 1.3∗107 bacterial cells at the end of the incubation (Figure 4), which was similar to the OC26_3 tank.
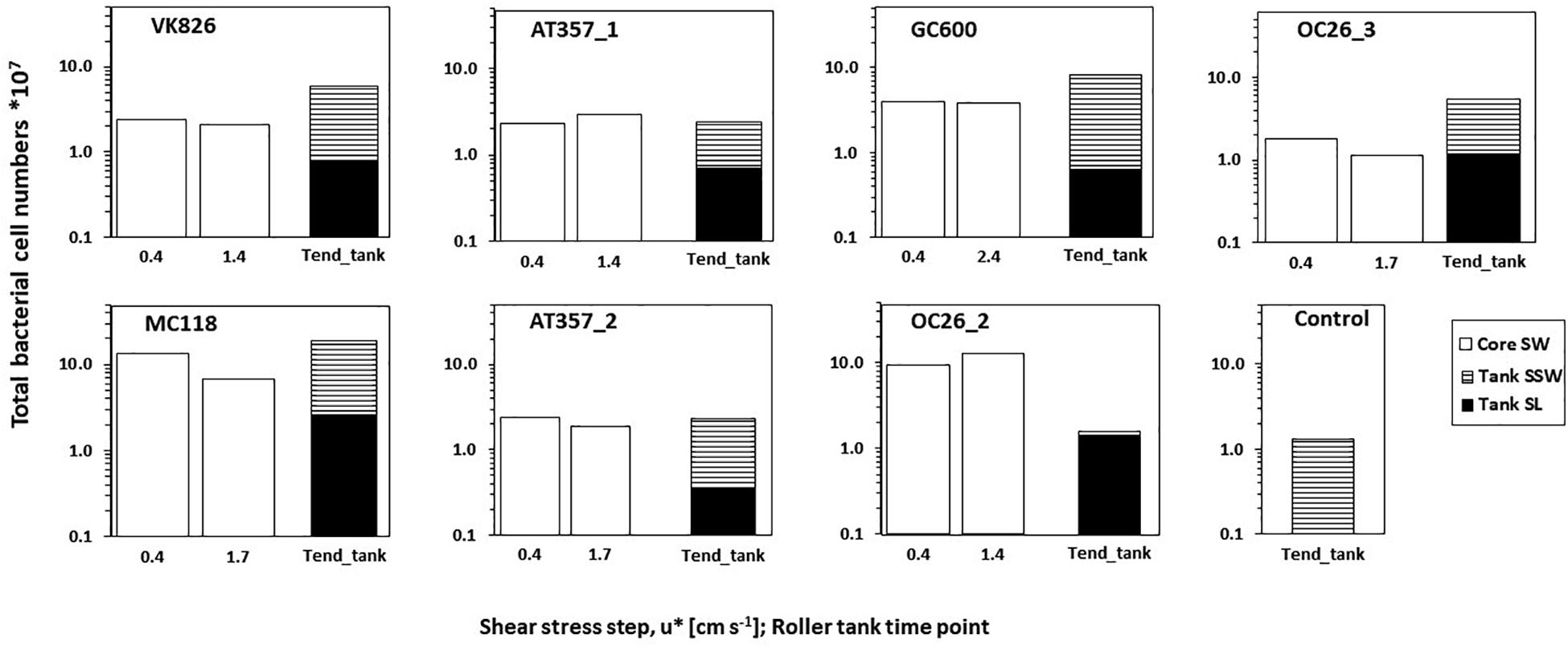
Figure 4. Bacterial cell numbers in the overlying core waters at the initial and final (= u*crit) shear stress step during the erosion experiments (Core SW), and at the end of roller tank incubations (Tend_tank) with the partitioning between sediment slurry (Tank SL) and surrounding seawater (Tank SSW). Cell numbers are standardized for the total volume of either the core water or the tank water.
Discussion
Sediment resuspension and subsequent fluxes of sedimentary organic matter into the overlying water play an important role in bottom water elemental cycling. Our two-phased experimental approach was designed to investigate the erosion thresholds and microbial enzymatic responses to experimentally resuspended sediments in deep GOM waters. In addition to providing new insights into benthic-pelagic coupling processes at the investigated sites, our results provide important data for predicting sediment transport in the deep GOM.
The u∗crit values reported here of 1.4 to 1.7 cm s–1 correspond to near-bottom flow velocities of 28 to 34 cm s–1, which are in the range of bottom currents reported in the investigation area. Martens et al. (2016) reported peak near-bed currents at MC118 of 20 cm s–1, while near-bed currents at VK826 were found to reach approximately 30 cm s–1 (Davies et al., 2010). Shallower nearby sites with water depths around 300 m regularly experience near-bed current velocities of up to 50 cm s–1 (Ross et al., 2009). We therefore conclude that bottom water flow velocities in our investigation area regularly exceed critical values for sediment resuspension.
Our u∗crit values for deep GOM sediments are in the same range as those reported for type 2 erosion from other deep-sea environments, characterized by mass or bulk erosion of the seabed (Amos et al., 1992). Using a laboratory flume, Thomsen and van Weering (1998) reported a u∗crit value of 1.60 cm s–1 for sediment cores from the deep NE Atlantic Ocean (Goban Spur). Similarly, erosion chamber experiments with deep slope sediments, also from Gobun Spur, revealed u∗crit values up to 2 cm s–1 (Thomsen and Gust, 2000). Earlier studies on sediment resuspension in the area of OC26 reported lower u∗crit values than those reported here (0.25–0.8 cm s–1; Diercks et al., 2021, 2018). This lower u∗crit is comparable to values measured for type 1 erosion, which describes resuspension of unconsolidated surficial layers often of biogenic origin (known as fluffy layers) without an appreciable increase in TPM in the water above the sediment (Amos et al., 1992). Resuspension of fluffy layers have been reported at other deep-sea (Beaulieu and Baldwin, 1998; de Jesus Mendes et al., 2007) and coastal water sites (Ziervogel and Bohling, 2003) but not in any of the cores examined in the present study.
The erodibility of marine sediments depends on a combination of sedimentological (e.g., grain size, mineralogy) and biological properties of the benthos (Amos et al., 1992). Surficial sediments from our investigated sites have previously been classified as terrigenous clay (Balsam and Beeson, 2003) with a mud content ranging between 93% (GC600) and 99% (MC118) (Montagna et al., 2013; Table 1). Thus, minor variations in sediment grain sizes and mud contents cannot explain the observed differences in erosion behavior between GC600 and our other investigated sites. The GC600 sediment core had visible oil residues in the uppermost layer, presumably from natural seeps in the area. Oil residues stemming from natural seeps episodically reach the seafloor by a variety of pathways at this station (Giering et al., 2018). Moreover, oil and gas seepage in this area have been shown to fuel elevated benthic microbial metabolism (Orcutt et al., 2010). At the time of our sampling, microbial methane oxidation and sulfate reduction rates in surficial sediments at GC600 were orders of magnitude higher compared to sediments from the other investigated sites (Joye, 2016). Enhanced microbial metabolic activities are often coupled with the release of exopolymeric substances (EPS) and the formation of biofilms. EPS interact with sediment particles acting as glue and thus increasing the cohesiveness and stability of the sediment (Tolhurst et al., 2002). In addition, crude oil residues in the GC600 sediment core possibly added to the stability of the sediment, enhancing its physical strength and resistance to erosion.
Additional biological properties that affect sediment stability include macrofaunal burrows and structures such as polychaete tubes that act as biological roughness elements on the near-bed flow (Friedrichs et al., 2000). Polychaete tubes were present in our sediment cores at varying abundance and distribution based on visual observation. Heterogeneity of macrofaunal abundance and their structures, which has been observed in soft sediments at scales of centimeters to meters (Morrisey et al., 1992), may result in small scale variations of erosion thresholds. The latter may in part explain the range of u∗crit among replicate cores observed at OC26 and AT357 (Figure 2).
Sediment resuspension in our experimental chamber affected extracellular enzyme activities in overlying waters. Most notable enzymatic responses were found for lipase activities (LIP) that followed increasing TPM in the overlying water in all of the experimentally resuspended cores. The other two enzyme activities assayed here (i.e., peptidases [PEP] and glucosidases [GLU]) showed orders of magnitude lower rates and more variable responses to experimentally resuspended sediments compared with LIP.
The order of enzyme activities observed here, with maximum LIP activities, differs from the order of PEP > GLU > LIP frequently observed in surface seawaters (Hoppe and Arnosti, 2002). Somewhat higher LIP activities at rates similar to PEP have been reported for deep ocean sediments (Poremba and Hoppe, 1995). Enhanced LIP activities were also found in the benthic-boundary layer of the deep Arabian Sea (Boetius et al., 2000), supporting the notion that deep-sea sediments may be an important source of lipid hydrolyzing enzymes in near-bed waters.
Refractory organic matter from surface water productivity, which is usually enriched in lipid complexes following sedimentation (Rullkötter, 2006) may stimulate LIP activities in marine sediments. Petroleum hydrocarbons from natural seeps and the DWH fallout that accumulated in surficial sediments in our investigation area following the spill (Romero et al., 2021) represent additional substrates for LIP in the investigated sediments (Kamalanathan et al., 2018). A connection between petroleum hydrocarbons and LIP activities were also found in one of our earlier studies at OC26 where rapid LIP activities reflected degradation of sedimented oily particulate matter (known as marine oil snow) following the DWH oil spill (Ziervogel et al., 2016).
Our results show that sediment resuspension in the northern GOM has the potential to enhance bottom water enzymatic activities by up to one order of magnitude relative to calm conditions. The extent of enzyme activity enhancement observed here is comparable to those reported from earlier field observations at OC26 and other experimental studies conducted in shallow coastal waters (Table 4). Increasing enzyme activities following sediment resuspension as in the case of LIP, were not always accompanied by increasing bacterial cell numbers between the beginning and the end of the erosion experiments. This pattern was observed in four of the experimentally resuspended cores for which cell counts were available (Figure 4). The disconnect between LIP and bacterial cell numbers in our experiments point to the importance of extracellular enzyme activities dissociated from the cells that produced the enzymes. Cell-free enzymes play an important role in marine elemental cycles (Baltar, 2018), particularly in diffusion-limited environments such as sediments (Vetter et al., 1998). Cell-free enzymes in sediments attach to particles forming sediment-enzyme complexes, which protect the enzymes from degradation and thus enhance their hydrolytic lifetimes relative to dissolved enzymes (Ziervogel et al., 2007). Higher sorption capacities of LIP relative to PEP and GLU with mineral surfaces could preserve the former in sediments, representing an alternative explanation for the elevated LIP activities reported here.

Table 4. Maximum enhancement as the fold increase of potential enzyme activities in bottom waters affected by resuspension.
Conclusion
Our results from onboard erosion experiments coupled with roller tank incubations reveal the following scenario for benthic-pelagic coupling in our investigation area: Following a resuspension event, hydrolytic enzymes produced by benthic microbes and sorbed to sediment particles get entrained into the overlying water where they remain active over extended periods of time as demonstrated by the high levels of enzyme activities at the end of the roller tank incubations. The magnitude of enzyme activities in roller tanks associated with resuspended sediments, which in most cases was between 40% and 100% of total tank water activities (Figure 4), are particularly important considering the fate of sediment-associated hydrolytic activities in near-bed waters. However, resuspended sediments often coagulate and aggregate, resulting in rapid particle redeposition on the seafloor (Thomsen and McCave, 2000). Resuspended particles that remain dispersed in the water, as observed in our 10-hour roller tank experiments, likely experience extended residence times in bottom waters. Field observations from other deep-sea environments reported residence times of bottom water turbidity layers of weeks to months (Puig et al., 2013; Durrieu de Madron et al., 2017; Jia et al., 2019). Lingering ‘clouds’ of resuspended sediments may stimulate enzymatic breakdown and microbial activity in deep ocean waters that generally exhibit lower microbial metabolic rates compared with those in the upper ocean (Aristegui et al., 2009). Enhanced enzymatic breakdown of resuspended organic matter (e.g., LIP) potentially increases degradation of more refractory sedimentary material, including petroleum hydrocarbons that are abundant in the northern GOM.
Data Availability Statement
The data presented in this study are publicly available through the Gulf of Mexico Research Initiative Information & Data Cooperative (GRIIDC) at https://data.gulfresearchinitiative.org/data/R4.x268.000:0101.
Author Contributions
KZ, JS, and UP planned the research. KZ and JS carried out the shipboard experiments with AJ who conducted the flowcytometry measurements. KZ wrote the manuscript with input from all co-authors. All authors contributed to the article and approved the submitted version.
Funding
Funding was provided by a grant from BP/the Gulf of Mexico Research Initiative to support the ECOGIG research consortium administered by the University of Georgia. This is ECOGIG contribution # 572.
Conflict of Interest
The authors declare that the research was conducted in the absence of any commercial or financial relationships that could be construed as a potential conflict of interest.
Publisher’s Note
All claims expressed in this article are solely those of the authors and do not necessarily represent those of their affiliated organizations, or those of the publisher, the editors and the reviewers. Any product that may be evaluated in this article, or claim that may be made by its manufacturer, is not guaranteed or endorsed by the publisher.
Acknowledgments
We thank the captain and crew of the RV Endeavor cruise 527 as well as chief scientist Joe Montoya (Georgia Tech) for assistance during sampling. We also thank Carol Arnosti (UNC) who gave invaluable advice during planning of the experiments, Tracy Villareal (UTex) who let us use his flow cytometer at sea, and Linda Kalnejais (UNH) who provided the EROMES chamber.
References
Amos, C. L., Daborn, G. R., Christian, H. A., Atkinson, A., and Robertson, A. (1992). In situ erosion measurements on fine-grained sediments from the Bay of Fundy. Mar. Geol. 108, 175–196. doi: 10.1016/0025-3227(92)90171-D
Aristegui, J., Gasol, J. M., Duarte, C. M., and Herndl, G. J. (2009). Microbial oceanography of the dark ocean’s pelagic realm. Limnol. Oceanogr. 54, 1501–1529. doi: 10.4319/lo.2009.54.5.1501
Arnosti, C. (2011). Microbial extracellular enzymes and the marine carbon cycle. Annu. Rev. Mar. Sci. 3, 401–425. doi: 10.1146/annurev-marine-120709-142731
Atlas, R. M., and Hazen, T. C. (2011). Oil biodegradation and bioremediation: a tale of the two worst spills in US history. Environ. Sci. Technol. 45, 6709–6715. doi: 10.1021/es2013227
Balsam, W. L., and Beeson, J. P. (2003). Sea-floor sediment distribution in the Gulf of Mexico. Deep Sea Res. Part Oceanogr. Res. Pap. 50, 1421–1444. doi: 10.1016/j.dsr.2003.06.001
Baltar, F. (2018). Watch out for the “living dead”: cell-free enzymes and their fate. Front. Microbiol. 8:2438. doi: 10.3389/fmicb.2017.02438
Baltar, F., Arístegui, J., Sintes, E., Gasol, J. M., Reinthaler, T., and Herndl, G. J. (2010). Significance of non-sinking particulate organic carbon and dark CO2 fixation to heterotrophic carbon demand in the mesopelagic northeast Atlantic. Geophys. Res. Lett. 37, 1–6. doi: 10.1029/2010GL043105
Beaulieu, S., and Baldwin, R. (1998). Temporal variability in currents and the benthic boundary layer at an abyssal station off central California. Deep Sea Res. Part II Top. Stud. Oceanogr. 45, 587–615. doi: 10.1016/S0967-0645(97)00095-7
Boetius, A., Springer, B., and Petry, C. (2000). Microbial activity and particulate matter in the benthic nepheloid layer (BNL) of the deep Arabian Sea. Deep-Sea Res. Part Ii-Top. Stud. Oceanogr. 47, 2687–2706. doi: 10.1016/S0967-0645(00)00045-X
Chanton, J., Zhao, T., Rosenheim, B. E., Joye, S., Bosman, S., Brunner, C., et al. (2015). Using natural abundance radiocarbon to trace the flux of petrocarbon to the seafloor following the deepwater horizon oil spill. Environ. Sci. Technol. 49, 847–854. doi: 10.1021/es5046524
Chrost, R. J., and Riemann, B. (1994). Storm-stimulated enzymatic decomposition of organicmatter in benthic/pelagic coastal mesocosms. Mar. Ecol.-Prog. Ser. 108, 185–192. doi: 10.3354/meps108185
Conti, A., D’Emidio, M., Macelloni, L., Lutken, C., Asper, V., Woolsey, M., et al. (2016). Morpho-acoustic characterization of natural seepage features near the Macondo Wellhead (ECOGIG site OC26, Gulf of Mexico). Deep Sea Res. Part II Top. Stud. Oceanogr. 129, 53–65. doi: 10.1016/j.dsr2.2015.11.011
Davies, A. J., Duineveld, G. C. A., van Weering, T. C. E., Mienis, F., Quattrini, A. M., Seim, H. E., et al. (2010). Short-term environmental variability in cold-water coral habitat at Viosca Knoll, Gulf of Mexico. Deep Sea Res. Part Oceanogr. Res. Pap. 57, 199–212. doi: 10.1016/j.dsr.2009.10.012
de Jesus Mendes, P. A., Thomsen, L., Holscher, B., de Stigter, H. C., and Gust, G. (2007). Pressure effects on the biological degradation of organo-mineral aggregates in submarine canyons. Mar. Geol. 246, 165–175. doi: 10.1016/j.margeo.2007.05.012
Diercks, A.-R., Dike, C., Asper, V. L., DiMarco, S. F., Chanton, J. P., and Passow, U. (2018). Scales of seafloor sediment resuspension in the northern Gulf of Mexico. Elem. Sci. Anthr. 6:32. doi: 10.1525/elementa.285
Diercks, A. R., Romero, I. C., Larson, R. A., Schwing, P., Harris, A., Bosman, S., et al. (2021). Resuspension, redistribution, and deposition of oil-residues to offshore depocenters after the deepwater horizon oil spill. Front. Mar. Sci. 8:630183. doi: 10.3389/fmars.2021.630183
Durrieu de Madron, X., Ramondenc, S., Berline, L., Houpert, L., Bosse, A., et al. (2017). Deep sediment resuspension and thick nepheloid layer generation by open-ocean convection. J. Geophys. Res. Oceans 122, 2291–2318. doi: 10.1002/2016JC012062
Dyer, K. R. (1986). Coastal and Estuarine Sediment Dynamics. New York, NY: Wiley Interscience Publication.
Fisher, C. R., Hsing, P.-Y., Kaiser, C. L., Yoerger, D. R., Roberts, H. H., Shedd, W. W., et al. (2014). Footprint of Deepwater Horizon blowout impact to deep-water coral communities. Proc. Natl. Acad. Sci. U S A. 111, 11744–11749. doi: 10.1073/pnas.1403492111
Friedrichs, M., Graf, G., and Springer, B. (2000). Skimming flow induced over a simulated polychaete tube lawn at low population densities. Mar. Ecol. Prog. Ser. 192, 219–228. doi: 10.3354/meps192219
Gasol, J. M., and Del Giorgio, P. A. (2000). Using flow cytometry for counting natural planktonic bacteria and understanding the structure of planktonic bacterial communities. Sci. Mar. 64, 197–224. doi: 10.3989/scimar.2000.64n2197
Giering, S. L. C., Yan, B., Sweet, J., Asper, V., Diercks, A., Chanton, J. P., et al. (2018). The ecosystem baseline for particle flux in the Northern Gulf of Mexico. Elem. Sci. Anthr. 6:6. doi: 10.1525/elementa.264
Graf, G. (1992). Benthic-pelagic coupling - a benthic view. Oceanogr. Mar. Biol. 30, 149–190. doi: 10.1007/s11356-015-4997-2
Griffiths, J. R., Kadin, M., Nascimento, F. J. A., Tamelander, T., Törnroos, A., Bonaglia, S., et al. (2017). The importance of benthic–pelagic coupling for marine ecosystem functioning in a changing world. Glob. Change Biol. 23, 2179–2196. doi: 10.1111/gcb.13642
Hoppe, H.-G. (1983). Significance of exoenzymatic activities in the ecology of brackish water: measurements by means of methylumbelliferyl-substrates. Mar. Ecol. Prog. Ser. 11, 299–308. doi: 10.3354/meps011299
Hoppe, H.-G., and Arnosti, C. (2002). “Ecological significance of bacterial enzymes in the marine environment,” in Enzymes in the Environment, eds R. G. Burns and R. P. Dick (New York, NY: Marcel Dekker), 73–97.
Jia, Y., Tian, Z., Shi, X., Liu, J. P., Chen, J., Liu, X., et al. (2019). Deep-sea sediment resuspension by internal solitary waves in the Northern South China sea. Sci. Rep. 9:12137. doi: 10.1038/s41598-019-47886-y
Joye, S. (2016). R/V Endeavor: EN527 Water and Sediment Chemistry, Gulf of Mexico, June 20 – July 2, 2013. Gulf of Mexico Research Initiative Information and Data Cooperative (GRIIDC). Available online at: https://data.gulfresearchinitiative.org/data/R1.x132.134:0026
Kalnejais, L. H., Martin, W. R., Signell, R. P., and Bothner, M. H. (2007). Role of sediment resuspension in the remobilization of particulate-phase metals from coastal sediments. Environ. Sci. Technol. 41, 2282–2288. doi: 10.1021/es061770z
Kamalanathan, M., Xu, C., Schwehr, K., Bretherton, L., Beaver, M., Doyle, S. M., et al. (2018). Extracellular enzyme activity profile in a chemically enhanced water accommodated fraction of surrogate oil: toward understanding microbial activities after the deepwater horizon oil spill. Front. Microbiol. 9:798. doi: 10.3389/fmicb.2018.00798
MacDonald, I. R., Garcia-Pineda, O., Beet, A., Asl, S. D., Feng, L., Graettinger, G., et al. (2015). Natural and unnatural oil slicks in the Gulf of Mexico. J. Geophys. Res. Oceans 120, 8364–8380. doi: 10.1002/2015JC011062
Mallet, C., Agogué, H., Bonnemoy, F., Guizien, K., Orvain, F., and Dupuy, C. (2014). Structures of benthic prokaryotic communities and their hydrolytic enzyme activities resuspended from samples of intertidal mudflats: an experimental approach. Trophic Significance Microb. Biofilm Tidal Flats 92, 158–169. doi: 10.1016/j.seares.2014.01.005
Margesin, R., Zimmerbauer, A., and Schinner, F. (1999). Soil lipase activity – a useful indicator of oil biodegradation. Biotechnol. Tech. 13, 859–863. doi: 10.1023/A:1008928308695
Martens, C. S., Mendlovitz, H. P., Seim, H., Lapham, L., and D’Emidio, M. (2016). Sustained in situ measurements of dissolved oxygen, methane and water transport processes in the benthic boundary layer at MC118, northern Gulf of Mexico. Gulf Mex. Ecosyst. - Macondo Blowout 129, 41–52. doi: 10.1016/j.dsr2.2015.11.012
Mislan, M., and Gates, I. D. (2019). Release of sugars and fatty acids from heavy oil biodegradation by common hydrolytic enzymes. Sci. Rep. 9:15584. doi: 10.1038/s41598-019-51796-4
Montagna, P. A., Baguley, J. G., Cooksey, C., and Hyland, J. L. (2013). Deepwater Horizon Oil Spill: Assessment of Potential Impacts on the Deep Soft-Bottom Benthos. Interim Data Summary Report. NOAA Technical Memorandum NOS NCCOS 166. Charleston, SC: NOAA, National Centers for Coastal Ocean Science.
Morrisey, D. J., Howitt, L., Underwood, A. J., and Stark, J. S. (1992). Spatial variation in soft-sediment benthos. Mar. Ecol. Prog. Ser. 81, 197–204. doi: 10.3354/meps081197
Orcutt, B. N., Joye, S. B., Kleindienst, S., Knittel, K., Ramette, A., Reitz, A., et al. (2010). Impact of natural oil and higher hydrocarbons on microbial diversity, distribution, and activity in Gulf of Mexico cold-seep sediments. Gulf Mex. Cold Seeps 57, 2008–2021. doi: 10.1016/j.dsr2.2010.05.014
Pfannkuche, O. (1993). Benthic response to the sedimentation of particulate organic matter at the BIOTRANS station, 47°N, 20°W. Deep Sea Res. Part II Top. Stud. Oceanogr. 40, 135–149. doi: 10.1016/0967-0645(93)90010-K
Ploug, H., Terbrüggen, A., Kaufmann, A., Wolf-Gladrow, D., and Passow, U. (2010). A novel method to measure particle sinking velocity in vitro, and its comparison to three other in vitro methods. Limnol. Oceanogr. Methods 8, 386–393. doi: 10.4319/lom.2010.8.386
Poremba, K., and Hoppe, H.-G. (1995). Spatial variation of benthic microbial production and hydrolytic enzymatic activity down the continental slope of the Celtic Sea. Mar. Ecol. Prog. Ser. 118, 237–245. doi: 10.3354/meps118237
Puig, P., de Madron, X. D., Salat, J., Schroeder, K., Martin, J., Karageorgis, A. P., et al. (2013). Thick bottom nepheloid layers in the western Mediterranean generated by deep dense shelf water cascading. Prog. Oceanogr. 111, 1–23. doi: 10.1016/j.pocean.2012.10.003
Pusceddu, A., Fiordelmondo, C., and Danovaro, R. (2005). Sediment resuspension effects on the benthic microbial loop in experimental microcosms. Microb. Ecol. 50, 602–613. doi: 10.1007/s00248-005-5051-6
Ritzrau, W. (1996). Microbial activity in the benthic boundary layer: small-scale distribution and its relationship to the hydrodynamic regime. J. Sea Res. 36, 171–180.
Ritzrau, W., and Graf, G. (1992). Increase of microbial biomass in the benthic turbidity zone of kiel bight after resuspension by a storm event. Limnol. Oceanogr. 37, 1081–1086. doi: 10.4319/lo.1992.37.5.1081
Ritzrau, W., Thomsen, L., Lara, R. J., and Graf, G. (1997). Enhanced microbial utilisation of dissolved amino acids indicates rapid modification of organic matter in the benthic boundary layer. Mar. Ecol. Prog. Ser. 156, 43–50. doi: 10.3354/meps156043
Romero, I. C., Chanton, J. P., Brooks, G. R., Bosman, S., Larson, R. A., Harris, A., et al. (2021). Molecular markers of biogenic and oil-derived hydrocarbons in deep-sea sediments following the deepwater horizon spill. Front. Mar. Sci. 8:637970. doi: 10.3389/fmars.2021.637970
Ross, C. B., Gardner, W. D., Richardson, M. J., and Asper, V. L. (2009). Currents and sediment transport in the mississippi canyon and effects of hurricane georges. Cont. Shelf Res. 29, 1384–1396. doi: 10.1016/j.csr.2009.03.002
Rullkötter, J. (2006). “Organic matter: the driving force of early diagenesis,” in Marine Geochemistry, 2 Edn. eds H. D. Schulz and M. Zabel (Berlin: Springer-Verlag), 125–186.
Shanks, A. L., and Edmondson, E. W. (1989). Laboratory-made artificial marine snow: a biological model of the real thing. Mar. Biol. 101, 463–470. doi: 10.1007/bf00541648
Soulsby, R. L., and Whitehouse, R. (1997). “Threshold of sediment motion in coastal environments,” in Proceedings of the Pacific Coasts and Ports ‘97 Conference, Vol. 1, (Christchurch: University of Canterbury), 149–154.
Thomsen, L., and Gust, G. (2000). Sediment erosion thresholds and characteristics of resuspended aggregates on the western European continental margin. Deep Sea Res. Part Oceanogr. Res. Pap. 47, 1881–1897. doi: 10.1016/S0967-0637(00)00003-0
Thomsen, L., and van Weering, T. (1998). Spatial and temporal variability of particulate matter in the benthic boundary layer at the N.W. European Continental Margin (Goban Spur). Prog. Oceanogr. 42, 61–76. doi: 10.1016/S0079-6611(98)00028-7
Thomsen, L. A., and McCave, I. N. (2000). Aggregation processes in the benthic boundary layer at the Celtic Sea continental margin. Deep-Sea Res. Part -Oceanogr. Res. Pap. 47, 1389–1404. doi: 10.1016/S0967-0637(99)00110-7
Tolhurst, T., Gust, G., and Paterson, D. M. (2002). “The influence of an extracellular polymeric substance (EPS) on cohesive sediment stability,” in Fine Sediment Dynamics in the Marine Environment, Proceedings in Marine Science Series, eds J. Winterwerp and C. Kranenburg (Pergamon: Elsevier inc), 409–425. doi: 10.1016/s1568-2692(02)80030-4
Turley, C. (2000). Bacteria in the cold deep-sea benthic boundary layer and sediment-water interface of the NE Atlantic. FEMS Microbiol. Ecol. 33, 89–99. doi: 10.1016/S0168-6496(00)00058-1
Unanue, M. A., Azua, I., Arrieta, J. M., Herndl, G. J., and Iriberri, J. (1998). Laboratory-made particles as a useful approach to analyse microbial processes in marine macroaggregates. FEMS Microbiol. Ecol. 26, 325–334. doi: 10.1111/j.1574-6941.1998.tb00517.x
Vetter, Y. A., Deming, J. W., Jumars, P. A., and Kreiger-Brockett, B. B. (1998). A predictive model of bacterial foraging by means of freely released extracellular enzymes. Microb. Ecol. 36, 75–92. doi: 10.1007/s002489900095
Wainright, S. C. (1987). Stimulation of heterotrophic microplankton production by resuspended marine sediments. Science 238, 1710–1712. doi: 10.1126/science.238.4834.1710
White, H. K., Hsing, P.-Y., Cho, W., Shank, T. M., Cordes, E. E., Quattrini, A. M., et al. (2012). Impact of the deepwater horizon oil spill on a deep-water coral community in the Gulf of Mexico. Proc. Natl. Acad. Sci. U S A. 109:20303. doi: 10.1073/pnas.1118029109
Zhao, Z., Baltar, F., and Herndl, G. J. (2020). Linking extracellular enzymes to phylogeny indicates a predominantly particle-associated lifestyle of deep-sea prokaryotes. Sci. Adv. 6:eaaz4354. doi: 10.1126/sciadv.aaz4354
Ziervogel, K., and Arnosti, C. (2009). Enzyme activities in the Delaware Estuary affected by elevated suspended sediment load. Estuar. Coast. Shelf Sci. 84, 253–258. doi: 10.1016/j.ecss.2009.06.022
Ziervogel, K., and Bohling, B. (2003). Sedimentological parameters and erosion behaviour of submarine coastal sediments in the south-western Baltic Sea. Geo-Mar. Lett. 23, 43–52. doi: 10.1007/s00367-003-0123-4
Ziervogel, K., Dike, C., Asper, V., Montoya, J., Battles, J., D×Souza, N., et al. (2016). Enhanced particle fluxes and heterotrophic bacterial activities in Gulf of Mexico bottom waters following storm-induced sediment resuspension. Deep Sea Res. Part II Top. Stud. Oceanogr 129, 77–88. doi: 10.1016/j.dsr2.2015.06.017
Ziervogel, K., D’souza, N., Sweet, J., Yan, B., and Passow, U. (2014). Natural oil slicks fuel surface water microbial activities in the northern Gulf of Mexico. Front. Microbiol. 5:188. doi: 10.3389/fmicb.2014.00188
Keywords: sediment resuspension, extracellular enzymes, lipase, benthic-pelagic coupling, deep-sea, northern Gulf of Mexico
Citation: Ziervogel K, Sweet J, Juhl AR and Passow U (2021) Sediment Resuspension and Associated Extracellular Enzyme Activities Measured ex situ: A Mechanism for Benthic-Pelagic Coupling in the Deep Gulf of Mexico. Front. Mar. Sci. 8:668621. doi: 10.3389/fmars.2021.668621
Received: 16 February 2021; Accepted: 18 October 2021;
Published: 16 November 2021.
Edited by:
Andrew James Manning, HR Wallingford, United KingdomReviewed by:
Fay Couceiro, University of Portsmouth, United KingdomKatrin Linse, British Antarctic Survey (BAS), United Kingdom
Copyright © 2021 Ziervogel, Sweet, Juhl and Passow. This is an open-access article distributed under the terms of the Creative Commons Attribution License (CC BY). The use, distribution or reproduction in other forums is permitted, provided the original author(s) and the copyright owner(s) are credited and that the original publication in this journal is cited, in accordance with accepted academic practice. No use, distribution or reproduction is permitted which does not comply with these terms.
*Correspondence: Kai Ziervogel, a2FpLnppZXJ2b2dlbEB1bmguZWR1