- 1Laboratory of Aquatic Nutrition and Feed, College of Fisheries, Guangdong Ocean University, Zhanjiang, China
- 2Aquatic Animals Precision Nutrition and High Efficiency Feed Engineering Research Center of Guangdong Province, Zhanjiang, China
- 3Key Laboratory of Aquatic, Livestock and Poultry Feed Science and Technology in South China, Ministry of Agriculture, Zhanjiang, Guangdong, China
To study the effects of acute ammonia stress on the poisoning reaction of the hybrid grouper (♀ Epinephelus fuscoguttatus × ♂ E. lanceolatu), 300 healthy grouper juveniles with an initial body weight of 51.4 ± 2.57 g were selected for an acute ammonia stress experiment using a half-lethal concentration of ammonia for 24, 48, 72, and 96 h with triplicate. The results show that (1) The half-lethal concentrations of ammonia for a hybrid grouper were 39.5 mg/L for 24 h, 27.3 mg/L for 48 h, 26.5 mg/L for 72 h, and 25.0 mg/L for 96 h, and the safe concentration was 2.50 mg/L. (2) The biochemical indices of the serum and livers of the groupers fluctuated under acute ammonia stress (P < 0.05), and the contents of serum cortisol and lactate reached the maximum value in 72 h (P < 0.05). (3) 24 h ammonia stress increased the activities of alkaline phosphatase, acid phosphatase, catalase, glutathione peroxidase, superoxide dismutase, lysozyme, and the content of immunoglobulin M in the liver (P < 0.05). Meanwhile, all ammonia-stressed fish groups had an increased amount of malondialdehyde in the liver (P < 0.05). (4) The ammonia poisoning caused significantly up-regulation of antioxidant genes, inflammatory cytokines, and apoptosis genes (P < 0.05), and the expression of inflammatory cytokines and apoptosis genes were the highest in 24 h ammonia stress group. (5) The ammonia content in the water changed the abundance and evenness of intestinal microbes in grouper, mainly in that ammonia stress significantly increased the relative abundance of fusobacteria, but significantly decreased the relative abundance of gemmatimonadetes (P < 0.05). It was speculated that apoptosis induced by ammonia poisoning in grouper may be related to oxidative stress and the activation of inflammatory factors, and excessive inflammatory stress may be one of the causes of ammonia poisoning in the hybrid grouper.
Introduction
The hybrid grouper (♀ Epinephelus fuscoguttatus × ♂ E. lanceolatu) is a new cross-species of grouper that is bred from a giant grouper male parent (Epinephelus lanceolatu) and a brown-marbled grouper female parent (Epinephelus fuscoguttatus), belongs to the Perciformes and grouper. In recent years, the hybrid grouper has been widely favored by farmers and consumers due to its excellent growth performance (Ch’ng and Senoo, 2008), delicious meat, high nutritional value, strong disease resistance, and high adaptability to the environment (Liang et al., 2013; Liu et al., 2018c). The grouper’s great development prospects make it a hot topic of scientific research (Wu et al., 2015; Yan et al., 2020).
In an intensive aquatic culture system, ammonia is the most common toxic substance and is considered a common stress factor in the culture environment and an important indicator of water quality. It is formed in the cultivated water as a result of the decomposition of nitrogen-containing organic matter such as animal excretions, secretions, and residues, and animal and plant carcasses (Foss et al., 2004; Liu et al., 2016). When the concentration of ammonia in the water exceeds a certain range, it will cause ammonia poisoning, which may lead to slow growth, tissue erosion, oxidative stress in the body, decreased immunity, and even the death of aquatic animals (Cheng et al., 2015). These physiological changes will differ for different research objects because of their differences in ammonia tolerance (Person-Le Ruyet et al., 1995). Nitrogen compounds in aquaculture water are divided into organic nitrogen and inorganic nitrogen. Organic nitrogen comprises mainly amino acid, protein, nucleic acid, and humic acid, whereas inorganic nitrogen comprises mainly ammonium nitrogen, nitrite nitrogen, and nitrate nitrogen, which are regarded as more toxic than organic nitrogen (Harris et al., 1998). As the main form of inorganic nitrogen in water, ammonia nitrogen exists mainly in the form of non-ionic ammonia and ionic ammonia. In aquaculture systems, ammonia levels can rise rapidly to unsafe levels unless the substance is constantly removed (Hegazi et al., 2010). The increase in ammonia concentration reduces the water quality and aquaculture production (Chen et al., 2019). Therefore, it is important to find ways to reduce the damaging effects of ammonia by elucidating the mechanism of ammonia poisoning in aquatic animals.
Currently, many studies have examined the effects of ammonia stress on aquatic animals (Lu et al., 2018). Originally, researchers thought that the pathogenesis of fish ammonia poisoning was the same as that of mammalian hepatoencephalopathy, because a high content of non-ionic ammonia nitrogen (NH3) in the brain synthesized a large amount of glutamine under the catalysis of glutamine synthetase, making astrocytes swell and causing intracranial hypertension and death (Smart, 1978). For a long time, this hypothesis has been accepted by most scholars. In the early twenty-first century, Der Linden et al. (2001) questioned this view and found that in Cyprinus, after the stress of ammonia the accumulation of glutamine is much greater than constitutes a lethal concentration in mammals. The brain volume expanded by 6.5%, but no animal died. The histological evidence shows that fish skulls have more intracranial space than those of mammals, and they can tolerate more intracranial pressure. Consequently, it was speculated that the increase in intracranial pressure may not be the main cause of the death of fish from ammonia poisoning. However, some physiological stress reactions (or pathological processes) can be induced by the metabolites produced in the process of adaptive regulation, such as oxidative damage and inflammatory hyperactivity, which may be the real cause of fish death (Ip et al., 2005). Similarly, Hegazi et al. (2010) showed that the production of reactive oxygen species is induced by ammonia nitrogen stress, which is the mediator of ammonia poisoning. In recent years, with the continuous innovations in aquatic animal research methods, there is new evidence that fish ammonia poisoning is related to an excessive inflammatory response of the body (Cheng et al., 2015). However, the specific mechanisms of ammonia poisoning in fish must be further explored. Therefore, the purpose of this study was to explore the changes in various antioxidant enzymes, digestive enzymes, and inflammatory factors in hybrid grouper juveniles under acute ammonia nitrogen stress. This would clarify their adaptability to ammonia to provide a reference for the healthy cultivation of groupers and reduce the harm of ammonia.
Materials and Methods
Fish and Pretest
This study was approved by the ethics review board of the Institutional Animal Care and Use Committee at Guangdong Ocean University in Zhanjiang, China. Hybrid groupers (♀ Epinephelus fuscoguttatus × ♂ E. lanceolatu) were purchased from a local farm at Donghai Island (Zhanjiang, China) and were temporarily maintained in an outdoor cement pond (5 m × 4 m × 1.8 m) to test specifications. Water temperature was maintained at 30 ± 1°C, salinity was 28, pH was 7.9 ± 0.1, and the dissolved oxygen concentration was kept at above 5.0 mg/L. The concentrations of total ammonia and nitrite remained at <0.05 mg/L.
A pretest was done before the formal test to calculate the semi-lethal concentration (LC) of ammonia nitrogen for 24 h (LC50,24 h), 48 h (LC50, 48 h), 72 h (LC50, 72 h), and 96 h (LC50, 96 h). A mother liquor of 10 g/L was prepared using analytically pure NH4Cl as an ammonia nitrogen source. The mother liquor was diluted to 7.8, 12.8, 15.8, 19.2, 24.8, 26.4, 28, 29.6, 35.2, 41, and 44.8 mg/L measured values in accordance with the experimental requirements. A total of 330 healthy, active, and uniform groupers were randomly selected and assigned to 33,100 L white plastic buckets (n = 10). No feeding was done during the experiment, and the ammonia nitrogen concentration in each barrel was measured every 6 h using a PTF-001B multiparameter water quality detector (WBD Biotechnology Co., Ltd., China) and 10 g/L NH4Cl mother liquor to adjust concentrations. The toxicity symptoms of the fish were observed, and the number of deaths was recorded at 24, 48, 72, and 96 h. By fitting the broken-line model or the quadratic curve model, the LC50, 24 h; LC50, 48 h; LC50, 72 h; and LC50, 96 h, respectively, were obtained.
Experimental Procedure
After the pretest, 300 healthy and uniformly sized (51.4 g ± 2.57 g) of grouper juveniles were randomly and evenly distributed into 15 100 L plastic buckets. The concentrations of total ammonia nitrogen in each treatment group were LC50, 24 h (39.5 mg/L), LC50, 48 h (27.3 mg/L), LC50, 72 h (26.5 mg/L) and LC50, 96 h (25 mg/L), and the control group was treated without stress. The test was done in triplicate over 96 h, during which no feed was given. There was natural illumination, the temperature of the water was 30°C ± 1°C, salinity was 28, pH was 7.9 ± 0.1, and dissolved oxygen was >5 mg/L. The safe concentration (SC) was calculated as (Sprague, 1971):
Sample Collection
Each treatment was sampled at 0, 24, 48, 72, and 96 h after ammonia stress. At the beginning of the stress, samples of the control group were taken. The blood, liver, and intestines were taken for enzyme activity analysis, mRNA expression determination, and 16S omics determination. Blood was placed in 1.5 mL microcentrifuge tubes and stored at 4°C for 12 h. The blood was later centrifuged (4,000 × g for 15 min at 4°C) and the serum collected and stored at −20°C for biochemical index and immunoenzyme activity analysis. After then, liver and intestine were immediately separated and loaded in 2 mL enzyme-free centrifuge tubes for enzyme activity analysis and 16S omics determination, and part of the liver was placed in an enzyme-free centrifuge tube containing RNA later for mRNA tissue expression determination. All tissue samples were stored at –80°C. Samples of fish surviving at every half-lethal time (24, 48, 72, and 96 h) were collected every 24 h until the end of the test. The above steps were repeated for the sampling method at each time interval.
Enzyme Activity Analysis
Serum and liver biochemical indices were determined by a commercial kit (Nanjing Jiancheng Biotechnology Co., Ltd., Nanjing, China). The activities of superoxide dismutase (SOD), catalase (CAT), glutathione peroxidase (GSH-PX), lysozyme (LYZ), malondialdehyde (MDA), immunoglobulin M (IgM), complement C3 (C3), complement C4 (C4), lactic acid (LD), cortisol (COR) and intestinal lipase (LPS), amylase (AMS), and trypsin (TRYP) were analyzed using commercial ELISA kits (Shanghai Enzyme-linked Biotechnology Co., Ltd., Shanghai, China), and the corresponding operations were done according to their instructions. Each treatment with triplicate, and each sample was tested twice.
RNA Extraction and Real-Time Quantitative Polymerase Chain Reaction
Transzol UP (TransGen Biotech, Beijing, China) of 1 mL was added to the liver samples, and the total RNA was extracted according to the manufacturer’s protocol. The quantity and quality of isolated RNA were detected at 260 nm and 280 nm using a NanoDrop 2000 spectrophotometer (Gene Company Limited, Guangzhou, China) and by electrophoresis in 1% agarose gel, respectively. The first-strand cDNA was synthesized using PrimeScript RTreagent Kits with cDNA Eraser (Takara, Japan) according to the manufacturer’s instructions. The cDNA was stored at −20°C for real-time quantitative polymerase chain reaction (RT-qPCR). Real-time quantitative polymerase chain reaction (RT-qPCR) was performed in a 384-well plate with a 10 μL reaction volume containing 5 μL of SYBR Green Real-time PCR Master Mix, 0.8 μL of each primer, 1 μL of cDNA sample, and 3.2 μL of RNse Free dH2O. The PCR conditions were set using a thermal programmer at 95°C for 30 s, 40 cycles of 95°C for 5 s, and 60°C for 34 s. Each sample was tested in triplicate. Primers of the reference gene (β-actin) and target gene were designed according to published sequences of groupers (Table 1). Threshold cycle (Ct) values were collected from each sample after finishing the process. The relative expression levels were calculated using the 2–ΔΔCt method (Livak and Schmittgen, 2001).
Intestinal Microbe Analysis
Total genomic microbial DNA was extracted by an MN NucleoSpin 96 SOI kit, then the V3/V4 region of the 16S rDNA gene was amplified by polymerase chain reaction (PCR) at 95°C for 5 min, and then 25 cycles were done at 95°C for 30 s, 50°C for 30 s, and 72°C for 40 s. Finally, primers 338F: ACTCCTACGGGAGGCAGCA and 806R: GGACTACHVGGGTWTCTAAT were used to extend at 72°C for 7 min. PCR products were mixed in accordance with the results of electrophoretic quantification (ImageJ software), and then purified by OMEGA DNA purification columns. After 120 V 40 min electrophoresis, 1.8% agarose gel, the fragments were cut and recycled. The original data were spliced (FLASH, version 1.2.11), and the mosaic (UCHIME algorithm, version 8.1) was removed to obtain a high-quality tag sequence. Intestinal microbe data analysis was done using BMKCloud1.
Statistical Analysis
All data were analyzed using SPSS version 20.0 (SPSS Inc., United States). First, a homogeneity test of variance was done, and then the significance analysis was done (one-way analysis of variance followed by Tukey tests). The results are shown as means ± standard error of the means.
Results
Poisoning Symptoms of Grouper Under Acute Ammonia Toxicity
At the beginning, the reaction of the groupers exposed to the ammonia solution was more intense, and the frequency of the operculum opening and closing was from rapid to slow. The sensitivity of the fishes’ bodies to sound, which showed violent swings, was stronger than that of the control group. Approximately 30 min after the experiment, the body color of the groupers began to lighten. At the same time, their bodies secreted much mucus, which floated in the water in strips. In the LC50, 24 h group, the symptoms of poisoning were obvious after 6 h of stress: the fish did not try to escape when frightened, and they floated in the water, sluggish and spastic. As time passed, some fish lost their balance and eventually died. The specific manifestations were as follows: the dorsal fin was upright, the pectoral fin was stretched forward, the mouth was exaggerated, the gills were bright red or dark red, and the body was rigid and arched.
Serum and Liver Biochemical Indicators
The serum biochemical indices of groupers under acute ammonia stress are shown in Figure 1A. After ammonia stress, the serum biochemical indices of the experimental groups increased relative to that of the control group. Aspartate transaminase (AST) of the 48 and 96 h groups and Alanine transaminase (ALT) of the 48, 72, and 96 h groups were higher than those in the control group (P < 0.05). The glucose (GLU) contents of the 24, 72, and 96 h groups were also higher than those in the control group (P < 0.05), but the GLU content of the 48 h group was not markedly different from that of the control group (P > 0.05). Meanwhile, the contents of serum COR and LD first increased and then decreased during the stress period and reached their maximum values at 72 h, which were higher than those of the control group (P < 0.05).
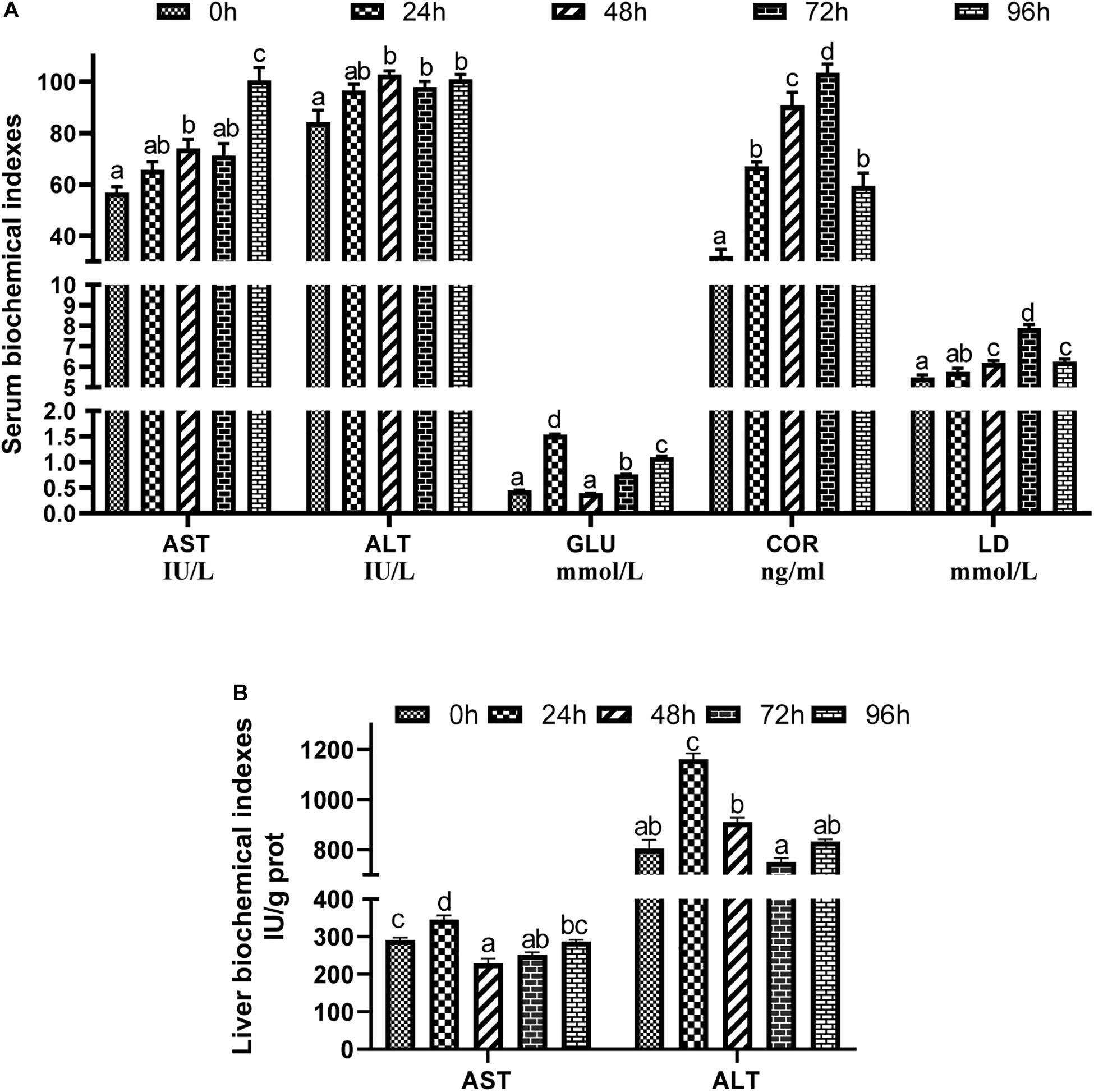
Figure 1. Effects of acute ammonia stress on serum (A) and liver (B) biochecmical indexes of grouper. Values are means ± SEM (n = 3). Different letters assigned to the bars represent significant differences using Tukey’s test (P < 0.05), within the same index and treatment group. AST, aspartate transaminase; ALT, alanine transaminase; GLU, glucose; LD, lactic acid; COR, cortisol.
The liver biochemical indices of the groupers under acute ammonia stress are shown in Figure 1B. The activities of AST and ALT in the liver increased in the 24 h group (P < 0.05). However, there was no significant difference in liver ALT activity between the other groups and the control group (P > 0.05).
Serum Non-specific Immune Indicators
As shown in Figure 2A, the alkaline phosphatase (AKP) activity at each point of time was higher than that of the control group (P < 0.05). Meanwhile, the acid phosphatase (ACP) activity of the 24, 72, and 96 h groups was also higher than that of the control group (P < 0.05), but the ACP activity of the 48 h group was not significantly different from that of the control group (P > 0.05). Relative to the control group, CAT activity increased in the 24 h group and decreased in the 72 and 96 h groups (P < 0.05). After the ammonia stress, SOD and GSH-PX activity decreased first and then increased, reaching the minimum value in the 48 and 72 h groups (P < 0.05). Meanwhile, LYZ activity also decreased after the stress compared with that of the control group (P < 0.05), but the stress duration did not affect LYZ activity (P > 0.05). However, the MDA content increased first and then decreased with stress time, which in the 24, 48, and 72 h groups was higher than that in the control group and in the 96 h group was lower than in the control group (P < 0.05).
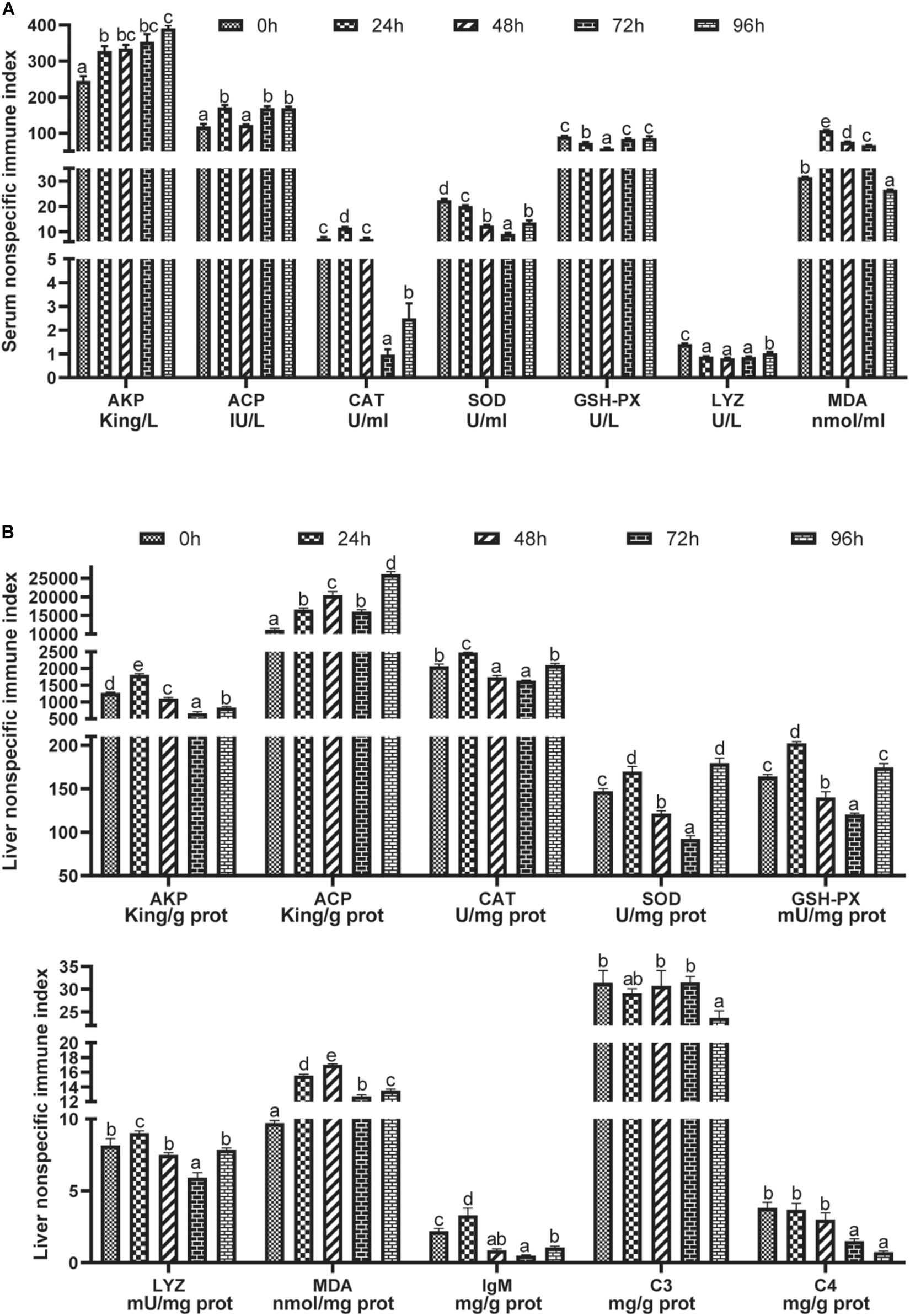
Figure 2. Effects of acute ammonia stress on serum (A) and liver (B) immune responses of grouper. Different letters assigned to the bars represent significant differences using Tukey’s test (P < 0.05). AKP, alkaline phosphatase; ACP, acid phosphatase; CAT, catalase; GSH-PX, glutathione peroxidase; SOD, superoxide dismutase; LYZ, lysozyme; MDA, malondialdehyde; IgM, immunoglobulin M; C3, complement C3; C4, complement C4.
Liver Non-specific Immune Indicators
Liver immune indices are shown in Figure 2B. In the 24 h group, AKP activity was higher than that in the control group, while that of the other groups was lower than that of the control group (P < 0.05). Ammonia stress increased ACP activity in the liver, and the highest value was found in the 96 h group (P < 0.05). Liver CAT, GSH-PX, SOD, and LYZ activities in the 24 h group were significantly higher than other groups (P < 0.05); meanwhile, CAT, SOD and GSH-PX in the 96 h group were significantly higher than those in the 48 and 72 h groups, but CAT and GSH-PX had no significant difference between the 96 h group and the control group (P > 0.05). However, liver SOD activity from ammonia stress in the 96 h group was higher than that in the other groups (except the 24 h group) (P < 0.05). After ammonia stress, the liver MDA content increased relative to that in the control group (P < 0.05) and reached the highest level in the 48 h group, which was higher than that in the other groups (P < 0.05). In the 24 h group under ammonia stress, the content of IgM in the liver had the maximum value, which was markedly higher than in the other groups (P < 0.05). However, the content of IgM decreased after more than 48 h of stress (P < 0.05). At the same time, the contents of C3 in the 96 h group and C4 in the 72 h and 96 h groups were lower than those in the control group (P < 0.05).
Intestinal Digestive Enzyme Activity
After ammonia stress, the activity of the intestinal digestive enzymes decreased (P < 0.05). There was no significant difference in LPS between the control group and the stressed 96 h group (P > 0.05), but the rest had lower levels than those in the control group (P < 0.05). Meanwhile, the minimum values of LPS, AMS, and TRYP appeared in the 48 and 72 h stress groups (Figure 3).
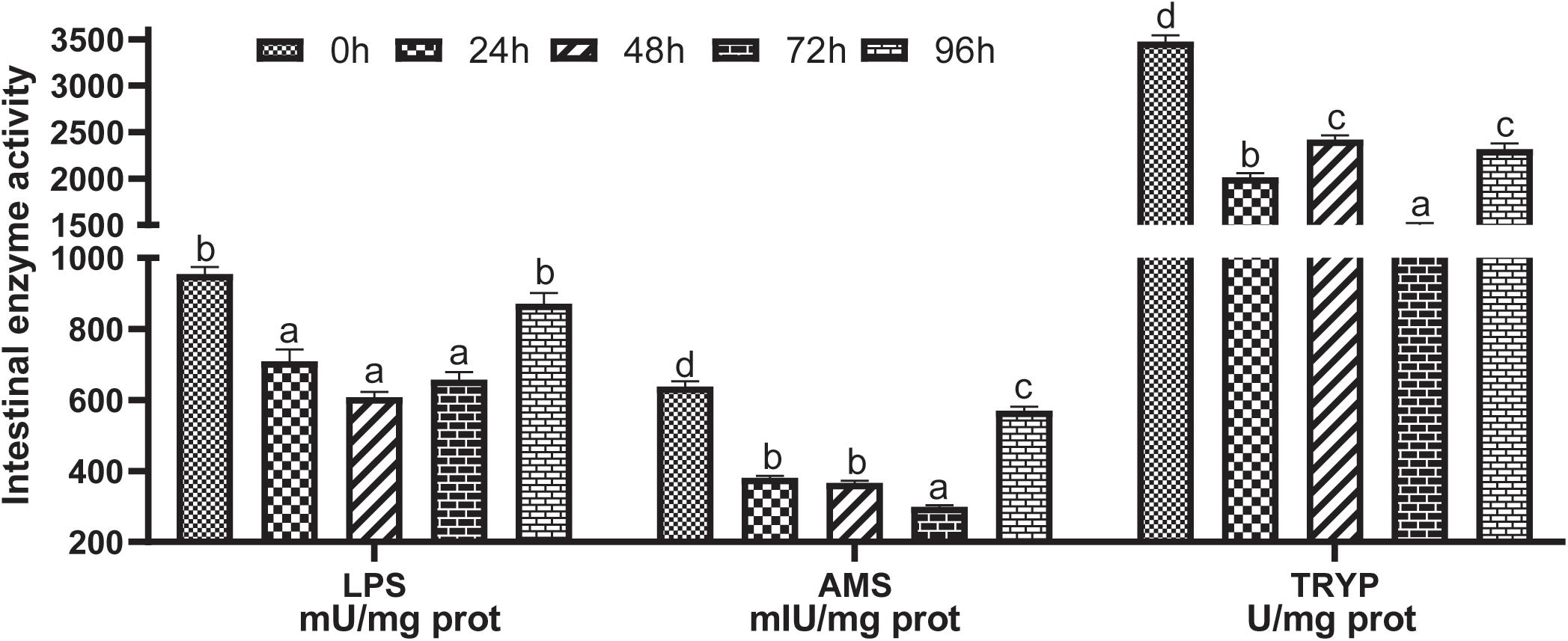
Figure 3. Effects of acute ammonia stress on intestinal digestive enzyme activity of grouper. Different letters assigned to the bars represent significant differences using Tukey’s test (P < 0.05). LPS, intestinal lipase; AMS, amylase; TRYP, trypsin.
Relative Expression of Inflammation-Related Gene mRNA in Liver
As shown in Figure 4A, ammonia stress markedly up-regulated the mRNA expression of tumor necrosis factor α (TNF-α) and interleukin 6 (IL6) (P < 0.05), and the highest expression was found in the 72 and 24 h groups. The expressions of interleukin 1β (IL-1β) and interleukin 8 (IL-8) mRNA were higher in the 24 h group than in the other experimental groups under ammonia stress (P < 0.05). Meanwhile, the expression of IL-8 mRNA in the 72 h group was also higher than in the other three experimental groups (P < 0.05). However, the expression of IL-1β mRNA in the 48 and 96 h groups was lower than that in the other experimental groups (P < 0.05). The expression of IL-8 mRNA in the 24 h group was higher than that of the control group (P < 0.05), but there was no significant difference in the other groups (P > 0.05).
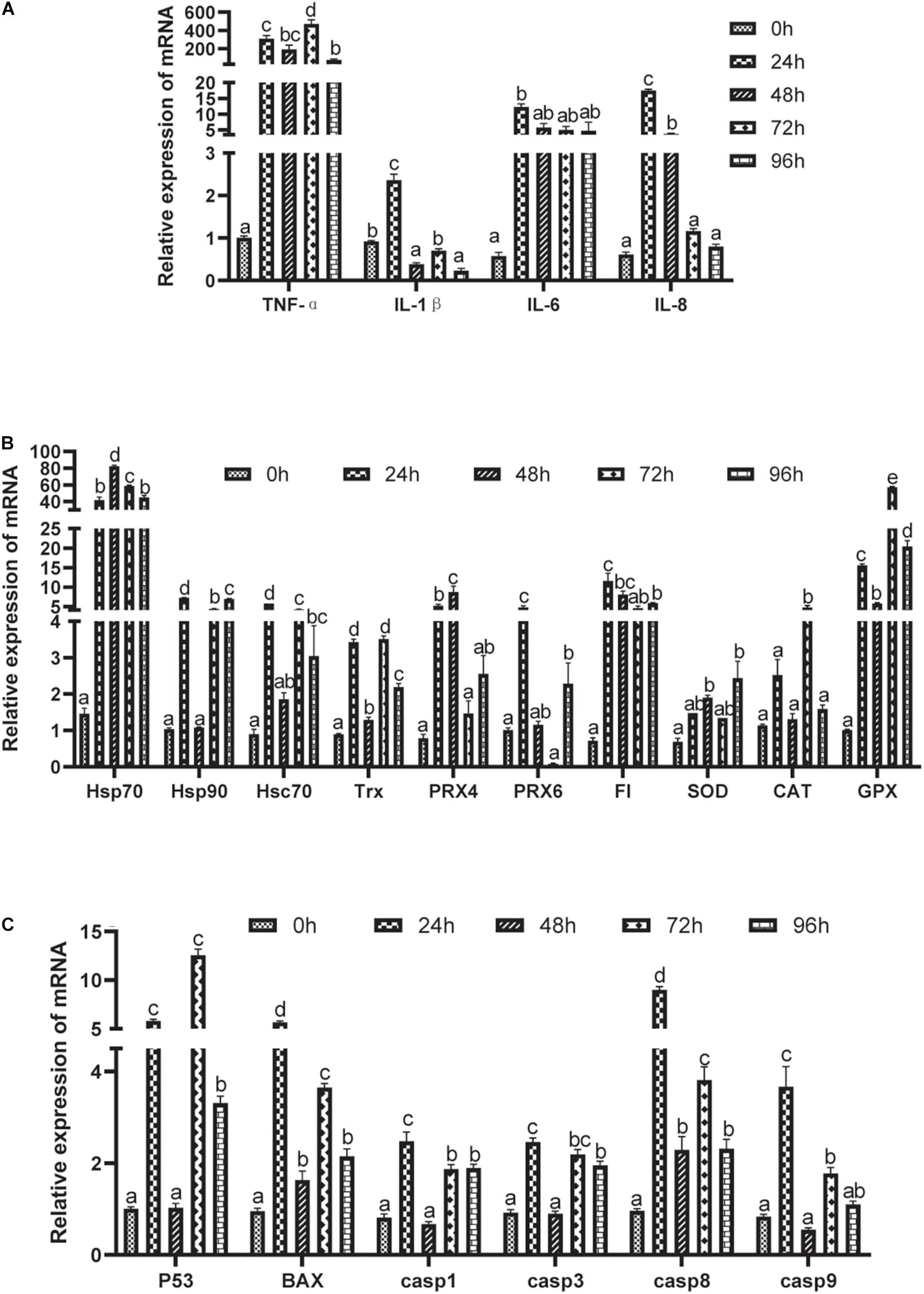
Figure 4. Effects of acute ammonia stress on relative expression of liver mRNA of grouper. (A) Pro-inflammatory factor; (B) antioxidant related gene; (C) apoptosis related genes. Different letters assigned to the bars represent significant differences using Tukey’s test (P < 0.05). TNFα, tumor necrosis factor α; IL-1β, interleukin 1β; IL-6, interleukin6; IL-8, interleukin8; Hsp70, heat shock protein 70; Hsp90, heat shock protein 90; Hsc70, heat shock cognate protein 70; Trx, thioredoxin; Prx-4, peroxiredoxin -4; Prx-6, peroxiredoxin-6; FI, ferritin; SOD, copper/zinc superoxide dismutase; CAT, catalase; GPX, glutathione peroxidase; p53, tumor suppressor protein p53; BAX, bc12-associated X; casp1, caspase 1; casp3, caspase3; casp8, caspase8; casp9, caspase9.
Relative Expression of Antioxidant-Related Gene mRNA in Liver
Acute ammonia stress up-regulated the expression of antioxidant gene mRNA in the liver (P < 0.05) (Figure 4B), but the expressions of heat shock protein 90 (Hsp90), heat shock cognate protein 70 (Hsc70), peroxiredoxin 6 (Prx6), and catalase (CAT) in the 48 h group and peroxiredoxin 4 (Prx4), Prx6, ferritin (FI), and copper/zinc superoxide dismutase (SOD) in the 72 h group were not significantly different from those in the control group (P > 0.05).
Relative Expression of Apoptosis-Related Gene mRNA in Liver
The relative expression of apoptosis-related gene mRNA in the liver is shown in Figure 4C. Acute ammonia stress up-regulated the expression of apoptosis-related genes (P < 0.05). However, 48 h of ammonia stress did not significantly affect the expressions of tumor suppressor protein p53 (p53), caspase 1 (casp1), caspase 3 (casp3), or caspase 9 (casp9) mRNA (P > 0.05). Except for the highest expression of p53 in the 72 h group, the highest expression of the other gene mRNA was found in the 24 h group (P < 0.05).
Intestinal Microbe Composition of Grouper After Stress
16S sequencing was done on the intestines of the groupers before and 24 h after stress; a total of 419,490 pairs of reads were obtained from 6 samples. After splicing and filtering, 403,798 clean tags were generated. Each sample produced at least 47,227 clean tags with an average of 67,300 clean tags. The two groups of operational taxonomic units (OTUs) were analyzed by a Venn diagram. The results showed that there were 320 repeated OTUs in the two groups, and the abundance of OTUs increased after 24 h of stress (Figure 5A). At the phylum level, the dominant intestinal microbes of a hybrid grouper are Firmicutes (35.2%), Proteobacteria (30.4%), and Bacteroidetes (9.94%). The secondary dominant microbes are Actinobacteria (6.51%), Cyanobacteria (5.66%), Acidobacteria (4.21%), and Chloroflexi (2.70%). In our experiment, ammonia stress increased the abundance of Proteobacteria and Bacteroidetes but decreased the abundance of Firmicutes (Figure 5B). At the family level, the dominant intestinal microbes of the hybrid grouper are Bacillaceae (10.4%), Enterobacteriaceae (7.33%), Camellia_oleifera (4.82%), Rhizobiaceae (4.15%), Ruminococcaceae (4.02%), and the secondary dominant microbes are Weeksellaceae (2.91%), Moraxellaceae (2.61%), Lachnospiraceae (2.41%), and Burkholderiaceae (2.10%). Ammonia stress increased the abundance of Enterobacteriaceae, Weeksellaceae, Lachnospiraceae, and other non-dominant bacteria, but decreased the abundance of Bacillaceae, Ruminococcaceae, Camellia_oleifera, and Rhizobiaceae (Figure 5C). Also, the relative abundances of Fusobacteria and Gemmatimonadetes were different before and after ammonia stress at the phylum level (P < 0.05) (Figure 5D).
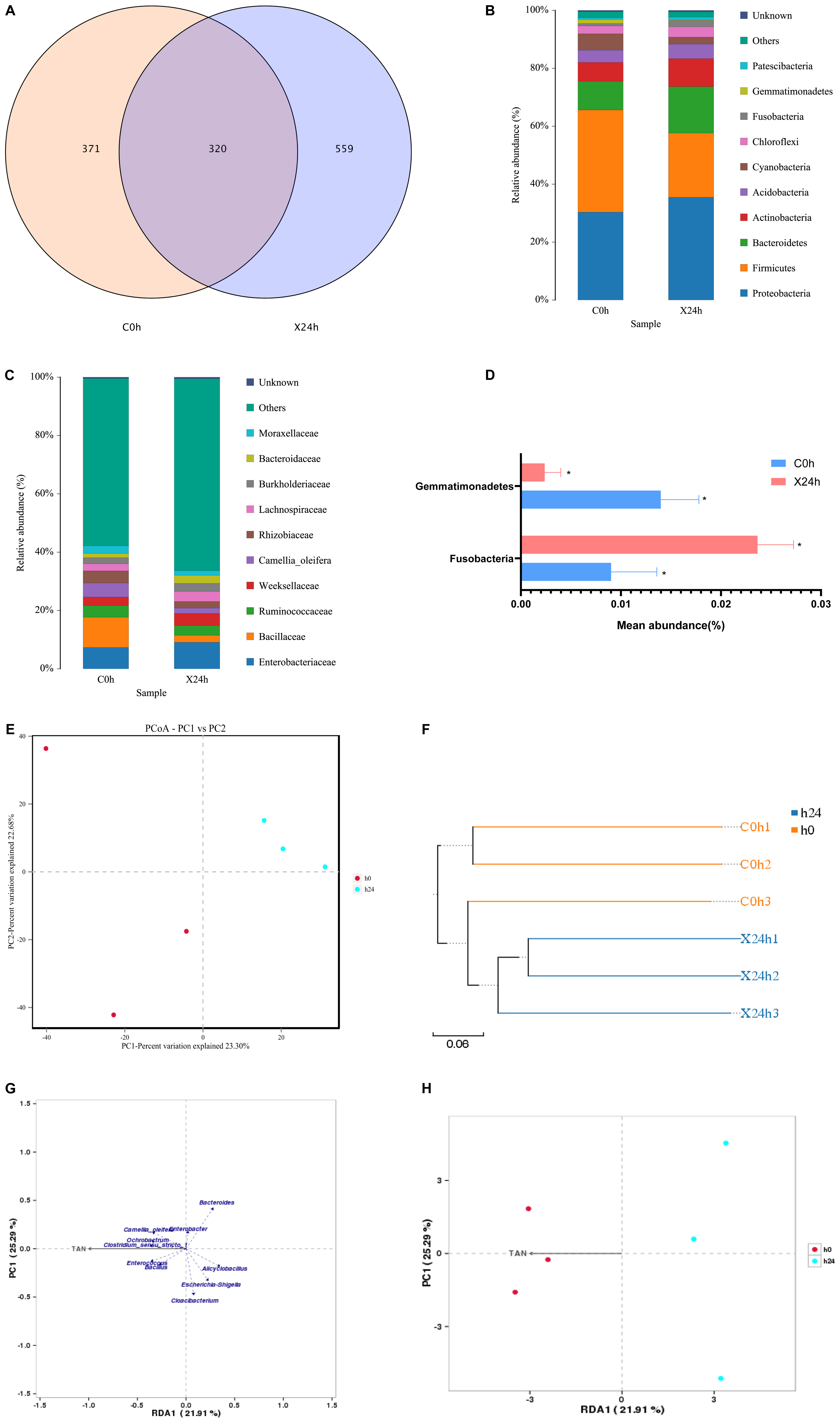
Figure 5. Effects of ammonia stress on intestinal microbial for hybrid grouper. (A) Venn diagram of OTUs comparison. (B) Structure and composition of intestinal microbial at phylum level. (C) Structure and composition of intestinal microbial at family level. (D) Species with significant difference in relative abundance at phylum level (P < 0.05, paired sample T test). (E,F) β-diversity analysis of intestinal microbial. (G,H) RDA/CCA analysis about environmental factors and intestinal microbial.
Alpha diversity indices as shown in Table 2: the Chao1, ACE, Shannon, Simpson, and Coverage index of each sample at 97% similarity level were calculated. The results of paired sample t-tests showed that there was a difference between OTU and Shannon indices before and after ammonia stress (P < 0.05). However, there were no significant differences in ACE, Chao1, Simpson, and Coverage indices (P > 0.05). Based on the binary Jaccard PCoA analysis shown in Figure 5E, the ammonia stress of the 24 h group reduced the intestinal microbial diversity of the hybrid grouper. In accordance with the different distance matrix information, the samples were classified for the unweighted pair group method with arithmetic mean (UPGMA) clustering analysis; the more similar the samples were, the shorter the common branches were. The UPGMA clustering result of a weighted UniFrac is shown in Figure 5F, in which the samples before and after ammonia stress were clustered in the same branch. Both UPGMA and PCoA showed that the samples were strongly aggregated under environmental influence, indicating that ammonia stress markedly affected the composition of intestinal microbes in groupers. The results of RDA–CCA analysis showed that there was a negative correlation between Cloacibacterium, Escherichia_Shigella, ANcylostoma, Bacteroides, and ammonia. Bacillus, Enterococcus, Clostridium_sensu_stricto_1, Ochrobactrum, and Camellia_oleifera were positively correlated with ammonia (Figure 5G). Meanwhile, Figure 5H shows that ammonia in water affected the intestinal microbes of the tested groupers (P < 0.05).
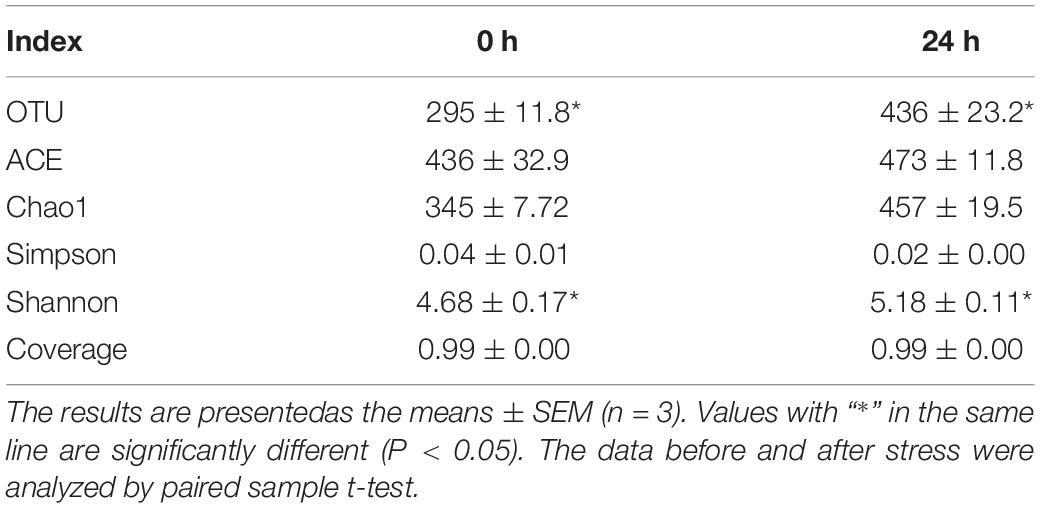
Table 2. Sequencing read analysis and Alpha diversity indices of intestinal microbial in hybrid grouper before and after ammonia stress.
Discussion
Poisoning Symptoms of Grouper After Ammonia Toxicity
Toxic reaction is the most obvious characteristic of ammonia stress in grouper. Ammonia refers to nitrogen existing in the form of free ammonia (NH3) and the ammonium ion (NH4+) in water, among which free ammonia (non-ionic ammonium) is the main hazard to fishes. Most bony fishes are sensitive to ammonia (Cheng et al., 2015; Ren et al., 2016; Xing et al., 2016), and there are many reports on how the toxic concentration and mechanism of free ammonia affects fishes (Zhang et al., 2018; Kr et al., 2019). Exposure of fishes to high ammonia can lead to ammonia poisoning; typical symptoms include decreased growth performance (Elshafai et al., 2004), liver tissue lesions, immunosuppression, hyperactivity, coma, and even death (Benli et al., 2008). In this study, we found that under acute ammonia stress, hybrid groupers appeared to have local hyperemia, and some stress behaviors appeared in the early stage of poisoning, including manic restlessness, frequent collision or rollover, shortness of breath, and increased frequency of gill flap agitation. As the ammonia stress continued and the ammonia concentration rose, the activity of the fish body decreased, respiration was blocked, activity was slow, the secretion of body surface mucus increased, and spasms occurred at the verge of death. This was consistent with ammonia poisoning in other fishes (Rodrigues et al., 2007). At the early stage of ammonia stress, the groupers’ rapid respiration and increased exercise were due to the rapid increase in blood ammonia concentration caused by the high concentration of environmental ammonia (Zhang and Wood, 2009), which affected the blood–oxygen transport capacity of the groupers, so it is necessary to improve the air exchange rate of the body to a certain extent (Cheng and Chen, 1998). The results of this study showed that the acute toxicity of ammonia to the groupers was linear with ammonia concentration and stress duration. With the increase in ammonia concentration, mortality increased sharply and the time to death shortened. Similarly, when the stress time was prolonged, the toxic effects of ammonia increased and the LC50 decreased. This suggests that a high concentration of ammonia stress has a significant effect on the immunity of aquatic organisms; in particular, long-term high-concentration stress will lead to a decline in immunity.
Effects of Ammonia Stress on Biochemical Indexes
Acute ammonia stress can not only cause ammonia poisoning in fish, but also change biochemical indexes of fish. AST and ALT are important transaminases widely found in animals; they play an important role in amino acid synthesis and catabolism. The liver is the main gland of nutrient digestion in animal bodies and also the main site of urea synthesis, with a detoxification function. Under normal conditions, there is only a small amount of AST and ALT in serum. When the membranes of hepatocytes and mitochondria are damaged, the membrane permeability increases, then AST and ALT are released into the blood (Feng et al., 2016). In this study, the activity of serum AST and ALT increased after ammonia stress, and the activity of liver AST and ALT in the 24 h stress group was markedly higher than those in the other experimental groups, which suggested that the two enzymes were induced by stress, and their activities increased, which enhanced the excretion of ammonia. When the experimental groupers were ammonia-stressed for 48–96 h, AST and ALT in their serum were markedly higher than those in the control group, but ALT in the liver was not markedly different from that in the control group. However, in the 48 and 72 h groups, ALT was markedly lower than that in the control group. The reason may be that with the prolongation of ammonia stress duration, the hepatocytes were damaged.
COR is a kind of hormone produced by the adrenal gland and was produced in a large amount during the stress reaction. Generally, COR enters the blood directly and is not stored; therefore, the content of COR in the blood can be used as an index to measure the stress intensity of fish (Hsieh et al., 2003). The results showed that the content of COR in the serum of each experimental group was markedly higher than that before the stress, which may indicate that the free ammonia in the water entered the organism during the stress process of ammonia, making the fish produce a stress response.
GLU is an important energy substance for many kinds of tissue metabolism, and stable blood GLU concentration plays an important role in maintaining the normal life activities of fishes. The secretion of COR can release GLU from the liver, which is transported to the blood for energy use in a stress response. Studies have shown that stress can lead to a marked increase in blood GLU in fishes (Yang et al., 2019); this is consistent with the findings on the 24, 72, and 96 h groups.
LD is a kind of fatigue substance produced by glycolysis of muscles when there is an insufficient oxygen supply. With the extension of stress time, the LD content increased gradually, similar to that of Atlantic salmon (Salmo salar L.) (Iversen et al., 2005) and chinook salmon (Oncorhynchus tshawytscha) (Schreck et al., 1995) after environmental stress. Sulikowski et al. (2006) showed that the presence of GLU, LD, and COR in blood can indicate the degree of stress, thus it can be concluded that the stress on the groupers was the strongest after 72 h.
Effects of Ammonia Stress on Non-specific Immune
Meanwhile, acute ammonia stress can stimulate non-specific immunity of fish. The immune system is divided into the specific immune system and the non-specific immune system. The non-specific immune system of fishes plays a leading role in coping with adverse environmental stress; in particular, ACP, AKP, SOD, CAT, GSH-PX, LYZ, and other enzymes play an important role in immune regulation (Ming et al., 2015). AKP is an important index of fish disease diagnosis and physiological activities (Giannini et al., 2005). ACP is an important part of lysosome, which can ensure that the fish is not invaded by pathogens (Yan et al., 2019). SOD and CAT are the enzymes of oxidative metabolism in the body; they work together to make the production and elimination of free radicals reach a dynamic balance and reduce oxidative damage (Li et al., 2007). They are important protective enzyme systems in the body (Martinezalvarez et al., 2005). GSH-PX is a ubiquitous enzyme that catalyzes the decomposition of H2O2, thus protecting the integrity of cell membrane structures and functions. LYZ is involved in a variety of metabolic processes and is an indicator of the health and immune status of aquatic animals (Liu et al., 2011). MDA is the end product of lipid oxidation; the MDA content in the body shows the degree of oxidative damage of lipid by reactive oxygen species and free radicals (Peng et al., 2009). The results of this study show that AKP in all stress groups and ACP in the 24, 72, and 96 h groups were higher than that in the control group. This may be related to the marked increase in MDA content after 24–72 h of stress. Meanwhile, serum SOD and LYZ markedly decreased after stress, and GSH-PX markedly decreased under high concentrations of acute stress. This shows that the activity of antioxidant enzymes was inhibited under the stress of a high concentration of ammonia or a low concentration of ammonia for a long time, so that the antioxidant capacity of the groupers’ bodies was reduced, and the bodies could not effectively remove the oxygen free radicals produced over time. This led to oxygen free radicals accelerating lipid peroxidation and increasing MDA. As time passed, the ammonia accumulation in the groupers became more toxic, the defense system of free radicals was destroyed, and the concentration of hydrogen peroxide and singlet oxygen increased, resulting in a rapid decline in enzyme activity and the imbalance of the immune system. Therefore, the activity of SOD and CAT was markedly lower than that of the 24 h stress group. Meanwhile, the levels of liver CAT, SOD, and GSH-PX were markedly higher in the 24 h group than those in other groups; this was consistent with the results of other stress studies (Burmester et al., 2012). Those levels were markedly lower at 48 and 72 h of ammonia stress than those in other groups. The reason may be that under this condition, the level of active oxygen free radicals and the content of MDA increased, and the physiological regulation of external stress to maintain the balance of the antioxidant level of the body tissue led to a compensatory increase in the activity of antioxidant enzymes (Liu et al., 2018a). In an LC, the extension of stress duration may have caused some damage to the antioxidant system, which showed that relative to the control group, MDA content increased and antioxidant enzyme activity decreased in the 48 and 72 h groups. When fishes are exposed to high concentrations of environmental ammonia, many immunosuppressive factors are produced in the lymphoid tissues (Barton and Iwama, 1991). These factors usually lead to a reduction about immune response of lymphocytes and phagocytes (Mock and Peters, 1990). This is consistent with the increase in IgM content in this study after 24 h of stress. However, ammonia stress for a long time may have an irreversible toxic effect on a grouper, damaging its normal physiological function. Its body’s ability to regulate ammonia stress becomes weaker and weaker. The accumulated toxicity of ammonia in the body increases and the defense system of free radicals is damaged, resulting in an immune system imbalance (Liu et al., 2018a). These groupers also show a decrease in IgM and C4 in the liver. This is consistent with the immune suppression of rainbow trout (Oncorhynchus mykiss) (Vedel et al., 1998), Atlantic halibut (Hippoglossus hippoglossus) (Paust et al., 2011), and darkbarbel catfish (Pelteobagrus fulvidraco) (Li et al., 2014) after acute or periodic ammonia stimulation.
Effects of Ammonia Stress on Digestive Enzymes
In addition, the digestive enzyme activity of intestine was also affected by water environment. The activity of digestive enzymes can reflect the basic characteristics of the digestive physiology of aquatic animals (Lundstedt et al., 2004). The digestive systems of fishes are highly sensitive to reactive oxygen species, and an increase in their digestive enzyme activity is related to their synthesis and secretion of enzymes (Gisbert et al., 2004). In the present study, stress in the 24–72 h groups markedly decreased the activity of three digestive enzymes, but after 96 h of stress, LPS and AMS increased markedly compared with that of the other experimental groups, which suggests that a low concentration of ammonia stress can increase the activity of digestive enzymes, and a high concentration of ammonia stress can inhibit that activity (Liu et al., 2018b). It was speculated that this may be because a grouper adapts better to a low concentration of ammonia over a long time than to a high concentration for a short time.
Effects of Ammonia Stress on Immune Factors and Apoptosis
Apoptosis induced by ammonia poisoning in hybrid groupers may be related to oxidative stress and activation of inflammatory factors. Previous studies have found that hyperglycemia caused brain astrocytes and microglia to swell and produce metabolites by activating N-Methyl-D-aspartic acid receptors and Ca2+. Depending on the pathway of nitric oxide synthetase, hyperglycemia can produce many free radicals, accelerate protein oxidation and lipid peroxidation, accumulate aldehydes and ketones, and cause oxidative damage to the body (Rao and Norenberg, 2012). It also releases many pro-inflammatory factors into the blood, causing an inflammatory reaction and apoptosis (Mckenna et al., 2006). A study of Litopenaeus vannamei showed that Microcystis aeruginosa and microcystin-LR exposure can induce damage from exposure and increased oxidative stress and inflammatory reactions (Duan et al., 2020). However, there have been few studies on oxidative damage, inflammatory response, and apoptosis caused by ammonia poisoning in fishes.
Ammonia-poisoned fishes can incur apoptosis in many ways, and p53 is an important regulator of apoptosis (Soengas et al., 1999). In this study, it was found that the mRNA expression of the p53 gene was markedly up-regulated in the 24, 72, and 96 h stress groups. This was consistent with the fact that free radical production induced by ammonia stress can up-regulate the expression of p53 (Zhang et al., 2018), which suggests that p53 could be involved in ammonium-induced apoptosis. Also, p53 can induce apoptosis by up-regulating the transcription of bcl2-associated X (Bax) (Zeng et al., 2014). Based on the results of this study, it was speculated that apoptosis also might be induced by a p53–Bax pathway in groupers after ammonia poisoning (Zhang et al., 2018). Bax is an important cell death promoting gene in Mammalia and fish that can induce the release of cytochrome c, leading to casp activation (Wei et al., 2001), which is an effective indicator of stress-induced apoptosis in aquatic animals (Fulda and Debatin, 2006). In the present study, casp mRNA expression was markedly up-regulated after stress, except in the 48 h stress group, which further suggested that casp dependent apoptosis may be related to ammonia-poisoning-induced apoptosis in grouper liver cells (Chen et al., 2006). It has been reported that free radicals and inflammation may also participate in apoptosis (Chen et al., 2006). Ammonia stress markedly up-regulated the expressions of Hsp70, Hsp90, Hsc70, thioredoxin (Trx), Prx-4, Prx-6, FI, SOD, CAT, and glutathione peroxidase (GPX) mRNA, which occurred to cope with oxidative stress. An increase in oxidative stress is one of the effects of environmental pollution on aquatic animals (Min et al., 2018). Oxidative stress leads to not only apoptosis but also inflammation (Kim et al., 2011). An inflammatory response is the normal defense response of the body to tissue damage and is an indispensable part of tissue repair (Seyan et al., 2010). In this study, it was found that ammonia stress markedly up-regulated the expressions of TNF-α, IL-1β, IL-6, and IL-8 mRNA, which were consistent with studies of rainbow trout (Vedel et al., 1998), Atlantic halibut (Paust et al., 2011), and darkbarbel catfish (Li et al., 2014). The activation of inflammatory cytokines can activate an NF-κB channel and apoptosis (Lam et al., 2011). NF-κB acts as a messenger in enhancing an environmental stress response and activates a series of genes related to inflammation, including IL and TNF (Jia et al., 2014). These results suggested that the apoptosis of groupers under ammonia stress may be related to oxidative stress and an inflammatory response.
Effects of Ammonia Stress on Intestinal Microbes
Food intake (Jiang et al., 2020) and the environment (Duan et al., 2020) have a great influence on the composition of intestinal microbes. Intestinal microbes are one of the main factors affecting the metabolism and growth performance of a host (Cani and Delzenne, 2009). Studies have shown that oxidative stress and an inflammatory response are often associated with intestinal microbial imbalance (Ganz et al., 2011). In the present study, acute ammonia stress markedly changed the intestinal microbial diversity of the groupers. Intestinal microbes are closely related to host health (Xiong et al., 2015). In the present study, the intestinal microbes of the hybrid groupers were Firmicutes, Proteobacteria, and Bacteroidetes; the relative abundance of Proteobacteria and Bacteroidetes was higher after acute ammonia stress. Proteobacteria participate in the degradation of the carbon complex in an aquatic ecosystem (Klase et al., 2019), which is an indication of intestinal microbial imbalance and inflammation (Mirpuri et al., 2014). Bacteroidetes are considered as polymeric organic matter degraders (Liu et al., 2019). Therefore, groupers may alleviate oxidative stress and inflammatory reactions by increasing the abundance of intestinal dominant bacteria. After ammonia stress, the abundance of Fusobacteria increased markedly. Fusobacteria can activate the host’s inflammatory response, thus protecting the host from pathogens that promote tumor growth (Kelly et al., 2018). This finding is consistent with that in the present study. RDA–CCA analysis of environmental factors and intestinal microbes showed that Cloacibacterium, Escherichia_Shigella, Ancylostoma, and Bacteroides were negatively correlated with ammonia and that Bacillus, Enterococcus, Clostridium sensu stricto 1, Ochrobactrum, and Camellia_oleifera were positively correlated with ammonia, which also suggests that environmental factors played an important role in the stabilization of intestinal microorganisms.
Conclusion
In conclusion, acute ammonia stress can lead to an increase in MDA content and the disorder in the immune system of hybrid groupers. Apoptosis induced by ammonia poisoning in hybrid groupers may be related to oxidative stress and activation of inflammatory factors. Furthermore, the increase in Fusobacteria can be used as a potential indicator of ammonia stress in hybrid groupers.
Data Availability Statement
The original contributions presented in the study are included in the article.
Ethics Statement
The animal study was reviewed and approved by the Institutional Animal Care and Use Committee at Guangdong Ocean University.
Author Contributions
XD and BT: designed study and manuscript revision. XY, YC, HaL, SZ, SC, QY, HoL, and YY: experimental operation and sampling and data analysis. XY: drafted manuscript. All authors contributed to the article and approved the submitted version.
Funding
This study was supported financially by the National Key R&D Program of China (Grant No. 2019YFD0900200), National Natural Science Foundation of China (Grant No. NSFC31972808), Postgraduate Education Innovation Project of Guangdong Ocean University (Grant No. 202028), Science and Technology Bureau of Zhanjiang (Grant Nos. 2020A03010 and 2020A05003), Agriculture Research System of China (Grant No. CARS-47), and “Chong Yi Liu” project of Guangdong Ocean University (Grant No. 231419011).
Conflict of Interest
The authors declare that the research was conducted in the absence of any commercial or financial relationships that could be construed as a potential conflict of interest.
Footnotes
References
Barton, B. A., and Iwama, G. K. (1991). Physiological changes in fish from stress in aquaculture with emphasis on the response and effects of corticosteroids. Annu. Rev. Fish Dis. 1, 3–26. doi: 10.1016/0959-8030(91)90019-g
Benli, A. C. K., Köksal, G., and Özkul, A. (2008). Sublethal ammonia exposure of Nile tilapia (Oreochromis niloticus L.): effects on gill, liver and kidney histology. Chemosphere 72, 1355–1358. doi: 10.1016/j.chemosphere.2008.04.037
Burmester, V., Nimptsch, J., and Wiegand, C. (2012). Adaptation of freshwater mussels to cyanobacterial toxins: response of the biotransformation and antioxidant enzymes. Ecotoxicol. Environ. Saf. 78, 296–309. doi: 10.1016/j.ecoenv.2011.11.037
Cani, P. D., and Delzenne, N. M. (2009). The role of the gut microbiota in energy metabolism and metabolic disease. Curr. Pharm. Des. 15, 1546–1558. doi: 10.2174/138161209788168164
Chen, S., Yu, Y., Gao, Y., Yin, P., and Tian, L. (2019). Exposure to acute ammonia stress influences survival, immune response and antioxidant status of pacific white shrimp (Litopenaeus vannamei) pretreated with diverse levels of inositol. Fish Shellfish Immunol. 89, 248–256. doi: 10.1016/j.fsi.2019.03.072
Chen, X. Y., Shao, J. Z., Xiang, L. X., and Liu, X. M. (2006). Involvement of apoptosis in malathion-induced cytotoxicity in a grass carp (Ctenopharyngodon idellus) cell line. Comp. Biochem. Physiol. Part C Toxicol. Pharmacol. 142, 36–45. doi: 10.1016/j.cbpc.2005.10.010
Cheng, C. H., Yang, F., Ling, R., Liao, S., Miao, Y., Ye, C., et al. (2015). Effects of ammonia exposure on apoptosis, oxidative stress and immune response in pufferfish (Takifugu obscurus). Aquat. Toxicol. 164, 61–71. doi: 10.1016/j.aquatox.2015.04.004
Cheng, S., and Chen, J. (1998). Effects of nitrite exposure on the hemolymph electrolyte, respiratory protein and free amino acid levels and water content of Penaeus japonicus. Aquat. Toxicol. 44, 129–139. doi: 10.1016/s0166-445x(98)00064-2
Ch’ng, C. L., and Senoo, S. (2008). Egg and larval development of a new hybrid grouper, tiger grouper Epinephelus fuscoguttatus × giant grouper E. lanceolatus. Aquac. Sci. 56, 505–512.
Der Linden, A. V., Verhoye, M., and Nilsson, G. E. (2001). Does Anoxia Induce Cell Swelling in Carp Brains? In Vivo MRI Measurements in Crucian Carp and Common Carp. J. Neurophysiol. 85, 125–133. doi: 10.1152/jn.2001.85.1.125
Duan, Y., Xiong, D., Wang, Y., Dong, H., and Zhang, J. (2020). Effects of Microcystis aeruginosa and microcystin-LR on intestinal histology, immune response, and microbial community in Litopenaeus vannamei. Environ. Pollut. 265:114774. doi: 10.1016/j.envpol.2020.114774
Elshafai, S. A., Elgohary, F. A., Nasr, F. A., Der Steen, N. P. V., and Gijzen, H. J. (2004). Chronic ammonia toxicity to duckweed-fed tilapia (Oreochromis niloticus). Aquaculture 232, 117–127. doi: 10.1016/s0044-8486(03)00516-7
Feng, J., wang, P., He, J., Lou, Y., Dang, H., and Deng, R. (2016). Effects of replacement of fish meal by fermented soybean meal on growth performance, body composition, serum biochemical indices and liver tissue morphology of juvenile large yellow croaker (Larimichthys crocea). Chin. J. Anim. Nutr. 28, 3493–3502.
Foss, A., Sæther, B.-S., and Evensen, T. H. (2004). Effect of chronic ammonia exposure on growth in juvenile Atlantic cod. Aquaculture 237, 179–189. doi: 10.1016/j.aquaculture.2004.03.013
Fulda, S., and Debatin, K. (2006). Extrinsic versus intrinsic apoptosis pathways in anticancer chemotherapy. Oncogene 25, 4798–4811. doi: 10.1038/sj.onc.1209608
Ganz, M., Csak, T., Nath, B., Szabo, G., and Medicine, D. O. (2011). Lipopolysaccharide induces and activates the Nalp3 inflammasome in the liver. World J. Gastroenterol. 17, 4772–4778. doi: 10.3748/wjg.v17.i43.4772
Giannini, E. G., Testa, R., and Savarino, V. (2005). Liver enzyme alteration: a guide for clinicians. Can. Med. Assoc. J. 172, 367–379. doi: 10.1503/cmaj.1040752
Gisbert, E., Piedrahita, R. H., and Conklin, D. E. (2004). Ontogenetic development of the digestive system in California halibut (Paralichthys californicus) with notes on feeding practices. Aquaculture 232, 455–470. doi: 10.1016/s0044-8486(03)00457-5
Harris, J. O., Maguire, G. B., Edwards, S. J., and Hindrum, S. M. (1998). Effect of ammonia on the growth rate and oxygen consumption of juvenile greenlip abalone, Haliotis laevigata Donovan. Aquaculture 160, 259–272. doi: 10.1016/s0044-8486(97)00249-4
Hegazi, M. M., Attia, Z. I., and Ashour, O. A. (2010). Oxidative stress and antioxidant enzymes in liver and white muscle of Nile tilapia juveniles in chronic ammonia exposure. Aquat. Toxicol. 99, 118–125. doi: 10.1016/j.aquatox.2010.04.007
Hsieh, S. L., Chen, Y. N., and Kuo, C. (2003). Physiological responses, desaturase activity, and fatty acid composition in milkfish (Chanos chanos) under cold acclimation. Aquaculture 220, 903–918. doi: 10.1016/s0044-8486(02)00579-3
Ip, Y. K., Leong, M. W., Sim, M. Y., Goh, G. S., Wong, W. P., and Chew, S. F. (2005). Chronic and acute ammonia toxicity in mudskippers, Periophthalmodon schlosseri and Boleophthalmus boddaerti: brain ammonia and glutamine contents, and effects of methionine sulfoximine and MK801. J. Exp. Biol. 208:1993. doi: 10.1242/jeb.01586
Iversen, M., Finstad, B., Mckinley, R. S., Eliassen, R. A., Carlsen, K. T., and Evjen, T. (2005). Stress responses in Atlantic salmon (Salmo salar L.) smolts during commercial well boat transports, and effects on survival after transfer to sea. Aquaculture 243, 0–382.
Jia, R., Cao, L., Du, J., Wang, J., and Liu, Y. (2014). Effects of carbon tetrachloride on oxidative stress, inflammatory response and hepatocyte apoptosis in common carp (Cyprinus carpio). Aquat. Toxicol. 152, 11–19. doi: 10.1016/j.aquatox.2014.02.014
Jiang, J., Chen, L., Wu, S., Lv, L., and Zhao, X. (2020). Effects of difenoconazole on hepatotoxicity, lipid metabolism and gut microbiota in zebrafish (Danio rerio). Environ. Pollut. 265:114844. doi: 10.1016/j.envpol.2020.114844
Kelly, D., Yang, L., and Pei, Z. (2018). Gut Microbiota, Fusobacteria, and Colorectal Cancer. Diseases 6:109. doi: 10.3390/diseases6040109
Kim, H. Y., Park, J., Lee, K. H., Lee, D. U., and Lee, S. M. (2011). Ferulic acid protects against carbon tetrachloride-induced liver injury in mice. Toxicology 282, 104–111. doi: 10.1016/j.tox.2011.01.017
Klase, G., Lee, S., Liang, S., Kim, J., Zo, Y. G., and Lee, J. (2019). The microbiome and antibiotic resistance in integrated fishfarm water: implications of environmental public health. Sci. Total Environ. 649, 1491–1501. doi: 10.1016/j.scitotenv.2018.08.288
Kr, M., Sunar, M. C., and Gk, M. G. (2019). Acute ammonia toxicity and the interactive effects of ammonia and salinity on the standard metabolism of European sea bass (Dicentrarchus labrax). Aquaculture 511:734273. doi: 10.1016/j.aquaculture.2019.734273
Lam, F. W. S., Wu, S. Y., Lin, S. J., Lin, C. C., Chen, Y., Wang, H., et al. (2011). The expression of two novel orange-spotted grouper (Epinephelus coioides) TNF genes in peripheral blood leukocytes, various organs, and fish larvae. Fish Shellfish Immunol. 30, 618–629. doi: 10.1016/j.fsi.2010.12.011
Li, K., Zheng, T., Tian, Y., Xi, F., Yuan, J., Zhang, G., et al. (2007). Beneficial effects of Bacillus licheniformis on the intestinal microflora and immunity of the white shrimp, Litopenaeus vannamei. Biotechnol. Lett. 29, 525–530. doi: 10.1007/s10529-006-9291-4
Li, M., Yu, N., Qin, J. G., Li, E., Du, Z., and Chen, L. (2014). Effects of ammonia stress, dietary linseed oil and Edwardsiella ictaluri challenge on juvenile darkbarbel catfish Pelteobagrus vachelli. Fish Shellfish Immunol. 38, 158–165. doi: 10.1016/j.fsi.2014.03.015
Liang, H., Huang, D., Wu, Y., Wang, C., and Zhong, W. (2013). Effects of Temperature and Salinity on Survival and Food Intake of Grouper Hybrid (Epinephelus lanceolatus♀×E. fuscoguttatus♂). J. Guang. Ocean Univ. 33, 22–26.
Liu, H., Guo, W., Tan, Z., Yang, S., and Sun, C. (2016). Effects of pH, Ammonia and Nitrite on Oxygen Consumption and Ammonia Excretion of Perinereis aibuhitensis. J. Guang. Ocean Univ. 36, 52–56.
Liu, J., Xue, C., Sun, H., Zheng, Y., Meng, Z., and Zhang, X. (2019). Carbohydrate catabolic capability of a Flavobacteriia bacterium isolated from hadal water. Syst. Appl. Microbiol. 42, 263–274. doi: 10.1016/j.syapm.2019.01.002
Liu, X. L., Xi, Q. Y., Yang, L., Li, H. Y., Jiang, Q. Y., Shu, G., et al. (2011). The effect of dietary Panax ginseng polysaccharide extract on the immune responses in white shrimp, Litopenaeus vannamei. Fish Shellfish Immunol. 30, 495–500. doi: 10.1016/j.fsi.2010.11.018
Liu, Y., Hu, J., Zhou, S., Peng, X., and Ma, Z. (2018a). Effects of acute ammonia nitrogen stress on antioxidant enzymes activity and digestive enzymes activity in larval Lates calcarifer. J. South. Agric. 49, 2087–2095.
Liu, Y., Hu, J., Zhou, S., Yang, R., Qin, J., Ma, Z., et al. (2018b). Effect of Acute Ammonia Stress on Antioxidant Enzymes and Digestive Enzymes in Barramundi Lates calcarifer Larvae. Isr. J. Aquac. 70, 1058–1069.
Liu, Y., Wang, J., Li, B., Qiao, H., Liu, X., Hao, T., et al. (2018c). Dietary manganese requirement of juvenile hybrid grouper, Epinephelus lanceolatus × E. fuscoguttatus. Aquac. Nutr. 24, 215–223. doi: 10.1111/anu.12549
Livak, K. J., and Schmittgen, T. D. (2001). Analysis of relative gene expression data using real-time quantitative PCR and the 2– Δ Δ CT method. Methods 25, 402–408. doi: 10.1006/meth.2001.1262
Lu, X., Luan, S., Dai, P., Meng, X., Cao, B., Luo, K., et al. (2018). iTRAQ-based comparative proteome analysis for molecular mechanism of defense against acute ammonia toxicity in Pacific White shrimp Litopenaeus vannamei. Fish Shellfish Immunol. 74, 52–61. doi: 10.1016/j.fsi.2017.12.030
Lundstedt, L., Melo, J. F. B., and Moraes, G. (2004). Digestive enzymes and metabolic profile of Pseudoplatystoma corruscans (Teleostei: Siluriformes) in response to diet composition. Comp. Biochem. Physiol. Part B: Biochem. Mol. Biol. 137, 331–339. doi: 10.1016/j.cbpc.2003.12.003
Martinezalvarez, R. M., Morales, A. E., and Sanz, A. (2005). Antioxidant Defenses in Fish: biotic and Abiotic Factors. Rev. Fish Biol. Fish. 15, 75–88. doi: 10.1007/s11160-005-7846-4
Mckenna, M. C., Waagepetersen, H. S., Schousboe, A., and Sonnewald, U. (2006). Neuronal and astrocytic shuttle mechanisms for cytosolic-mitochondrial transfer of reducing equivalents: current evidence and pharmacological tools. Biochem. Pharmacol. 71, 399–407. doi: 10.1016/j.bcp.2005.10.011
Min, B.-H., Ravikumar, Y., Lee, D.-H., Choi, K. S., and Kim, B.-M. (2018). Age-dependent antioxidant responses to the bioconcentration of microcystin-LR in the mysid crustacean, Neomysis awatschensis. Environ. Pollut. 232, 284–292. doi: 10.1016/j.envpol.2017.09.050
Ming, J. H., Ye, J. Y., Zhang, Y. X., Xu, P., and Xie, J. (2015). Effects of dietary reduced glutathione on growth performance, non-specific immunity, antioxidant capacity and expression levels of IGF-I and HSP70 mRNA of grass carp (Ctenopharyngodon idella). Aquaculture 438, 39–46. doi: 10.1016/j.aquaculture.2014.12.038
Mirpuri, J., Raetz, M., Sturge, C. R., Wilhelm, C. L., Benson, A., Savani, R. C., et al. (2014). Proteobacteria-specific IgA regulates maturation of the intestinal microbiota. Gut Microbes 5, 28–39. doi: 10.4161/gmic.26489
Mock, A., and Peters, G. (1990). Lysozyme activity in rainbow trout, Oncorhynchus mykiss (Walbaum), stressed by handling, transport and water pollution. J. Fish Biol. 37, 873–885. doi: 10.1111/j.1095-8649.1990.tb03591.x
Paust, L. O., Foss, A., and Imsland, A. K. (2011). Effects of chronic and periodic exposure to ammonia on growth, food conversion efficiency and blood physiology in juvenile Atlantic halibut (Hippoglossus hippoglossus L.). Aquaculture 315, 400–406. doi: 10.1016/j.aquaculture.2011.03.008
Peng, S., Chen, L., Qin, J., Hou, J., Yu, N., Long, Z., et al. (2009). Effects of dietary vitamin E supplementation on growth performance, lipid peroxidation and tissue fatty acid composition of black sea bream (Acanthopagrus schlegeli) fed oxidized fish oil. Aquac. Nutr. 15, 329–337. doi: 10.1111/j.1365-2095.2009.00657.x
Person-Le Ruyet, J., Chartois, H., and Quemener, L. (1995). Comparative acute ammonia toxicity in marine fish and plasma ammonia response. Aquaculture 136, 181–194. doi: 10.1016/0044-8486(95)01026-2
Rao, K. V. R., and Norenberg, M. D. (2012). Brain energy metabolism and mitochondrial dysfunction in acute and chronic hepatic encephalopathy. Neurochem. Int. 60, 697–706. doi: 10.1016/j.neuint.2011.09.007
Ren, Q., Li, M., Yuan, L., Song, M., Xing, X., Shi, G., et al. (2016). Acute ammonia toxicity in crucian carp Carassius auratus and effects of taurine on hyperammonemia. Comp. Biochem. Physiol. C Toxicol. Pharmacol. 190, 9–14. doi: 10.1016/j.cbpc.2016.08.001
Rodrigues, R. V., Schwarz, M. H., Delbos, B., and Sampaio, L. S. A. (2007). Acute toxicity and sublethal effects of ammonia and nitrite for juvenile cobia Rachycentron canadum. Aquaculture 271, 553–557. doi: 10.1016/j.aquaculture.2007.06.009
Schreck, C. B., Jonsson, L., Feist, G. W., and Reno, P. W. (1995). Conditioning improves performance of juvenile chinook salmon, Oncorhynchus tshawytscha, to transportation stress✩. Aquaculture 135, 99–110. doi: 10.1016/0044-8486(95)01018-1
Seyan, A. S., Hughes, R. D., and Shawcross, D. L. (2010). Changing face of hepatic encephalopathy:Role of inflammation and oxidative stress. World J. Gastroenterol. 16, 3347–3357. doi: 10.3748/wjg.v16.i27.3347
Smart, G. R. (1978). Investigations of the toxic mechanisms of ammonia to fish–gas exchange in rainbow trout (Salmo gairdneri) exposed to acutely lethal concentrations. J. Fish Biol. 12, 93–104. doi: 10.1111/j.1095-8649.1978.tb04155.x
Soengas, M. S., Alarcon, R. M., Yoshida, H., Giaccia, A. J., Hakem, R., Mak, T. W., et al. (1999). Apaf-1 and caspase-9 in p53-dependent apoptosis and tumor inhibition. Science 284, 156–159. doi: 10.1126/science.284.5411.156
Sprague, J. B. (1971). Measurement of pollutant toxicity to fish—III: sublethal effects and “safe” concentrations. Water Res. 5, 245–266. doi: 10.1016/0043-1354(71)90171-0
Sulikowski, J. A., Fairchild, E. A., Rennels, N., Howell, W. H., and Tsang, P. C. W. (2006). The Effects of Transport Density on Cortisol Levels in Juvenile Winter Flounder, Pseudopleuronectes americanus. J.World Aquac. Soc. 37, 107–112. doi: 10.1111/j.1749-7345.2006.00013.x
Vedel, N. E., Korsgaard, B., and Jensen, F. B. (1998). Isolated and combined exposure to ammonia and nitrite in rainbow trout (Oncorhynchus mykiss): effects on electrolyte status, blood respiratory properties and brain glutamine/glutamate concentrations. Aquat. Toxicol. 41, 325–342. doi: 10.1016/s0166-445x(97)00071-4
Wei, M. C., Zong, W., Cheng, E. H., Lindsten, T., Panoutsakopoulou, V., Ross, A. J., et al. (2001). Proapoptotic BAX and BAK: a Requisite Gateway to Mitochondrial Dysfunction and Death. Science 292, 727–730. doi: 10.1126/science.1059108
Wu, Y., Wang, C., Liang, H., and Luo, H. (2015). Effects of Different Cyclic Starvation and Refeeding Regimes on Growth of Hybrid Grouper (Epinephelus fuscoguttatus ♀× E. lanceolatus ♂). J. Guangd. Ocean Univ. 35, 21–26.
Xing, X., Li, M., Yuan, L., Song, M., Ren, Q., Shi, G., et al. (2016). The protective effects of taurine on acute ammonia toxicity in grass carp Ctenopharynodon idellus. Fish Shellfish Immunol. 56, 517–522. doi: 10.1016/j.fsi.2016.08.005
Xiong, J., Wang, K., Wu, J., Qiuqian, L., Yang, K., Qian, Y., et al. (2015). Changes in intestinal bacterial communities are closely associated with shrimp disease severity. Appl. Microbiol. Biotechnol. 99, 6911–6919. doi: 10.1007/s00253-015-6632-z
Yan, X., Dong, X., Tan, B., Zhang, S., Chi, S., Liu, H., et al. (2020). Influence of different oil sources on growth, disease resistance, immune response and immune-related gene expression on the hybrid grouper (♀ Epinephelus fuscoguttatus×♂ E. lanceolatu), to Vibrio parahaemolyticus challenge. Fish Shellfish Immunol. 99, 310–321. doi: 10.1016/j.fsi.2020.02.025
Yan, X., Yang, J., Dong, X., Tan, B., Zhang, S., Chi, S., et al. (2019). The optimal dietary protein level of large−size grouper Epinephelus coioides. Aquac. Nutr. 26, 705–714. doi: 10.1111/anu.13030
Yang, S., Wu, H., He, K., Yan, T., Zhou, J., Zhao, L. L., et al. (2019). Response of AMP-activated protein kinase and lactate metabolism of largemouth bass (Micropterus salmoides) under acute hypoxic stress. Sci.Total Environ. 666, 1071–1079. doi: 10.1016/j.scitotenv.2019.02.236
Ye, H., Xu, M., Chen, L., Tan, X., and Wang, A. (2019). Effects of dietary plant protein sources influencing hepatic lipid metabolism and hepatocyte apoptosis in hybrid grouper (Epinephelus lanceolatus♂× Epinephelus fuscoguttatus♀). Aquaculture 506, 437–444.
Zeng, C., Sun, H., Xie, P., Wang, J., Zhang, G., Chen, N., et al. (2014). The role of apoptosis in MCLR-induced developmental toxicity in zebrafish embryos. Gig. Sanit. 149, 25–32. doi: 10.1016/j.aquatox.2014.01.021
Zhang, L., and Wood, C. M. (2009). Ammonia as a stimulant to ventilation in rainbow trout Oncorhynchus mykiss. Respir. Physiol. Neurobiol. 168, 261–271. doi: 10.1016/j.resp.2009.07.011
Keywords: ammonia poisoning, serum biochemistry, non-specific immunity, genes, intestinal microbial, half-lethal concentrations
Citation: Yan X, Chen Y, Dong X, Tan B, Liu H, Zhang S, Chi S, Yang Q, Liu H and Yang Y (2021) Ammonia Toxicity Induces Oxidative Stress, Inflammatory Response and Apoptosis in Hybrid Grouper (♀ Epinephelus fuscoguttatus × ♂ E. lanceolatu). Front. Mar. Sci. 8:667432. doi: 10.3389/fmars.2021.667432
Received: 13 February 2021; Accepted: 24 March 2021;
Published: 21 April 2021.
Edited by:
Sanjay K. Gupta, Indian Institute of Agricultural Biotechnology (ICAR), IndiaReviewed by:
Morteza Yousefi, Peoples’ Friendship University of Russia, RussiaMahmoud A. O. Dawood, Kafrelsheikh University, Egypt
Seyed Hossein Hoseinifar, Gorgan University of Agricultural Sciences and Natural Resources, Iran
Ahmed Esmat Abdel Moneim, Helwan University, Egypt
Copyright © 2021 Yan, Chen, Dong, Tan, Liu, Zhang, Chi, Yang, Liu and Yang. This is an open-access article distributed under the terms of the Creative Commons Attribution License (CC BY). The use, distribution or reproduction in other forums is permitted, provided the original author(s) and the copyright owner(s) are credited and that the original publication in this journal is cited, in accordance with accepted academic practice. No use, distribution or reproduction is permitted which does not comply with these terms.
*Correspondence: Xiaohui Dong, ZG9uZ3hpYW9odWkyMDAzQDE2My5jb20=; Beiping Tan, YnB0YW5AMTI2LmNvbQ==