- 1University of Brest- UMR 6539 CNRS/UBO/IRD/Ifremer, LEMAR – IUEM, Plouzané, France
- 2Department of Oceanography, University of Cape Town, Cape Town, South Africa
- 3Marine and Antarctic Research Centre for Innovation and Sustainability (MARIS), University of Cape Town, Cape Town, South Africa
- 4IRD/Laboratoire d’Océanographie Physique et Spatiale (LOPS), IUEM, University of Brest, CNRS/UBO/IRD/Ifremer, Plouzané, France
Omega-3 long-chain polyunsaturated fatty acids (hereafter, omega-3), including eicosapentaenoic-acid (EPA) and docosahexaenoic-acid (DHA), are essential nutritional compounds for humans, providing several benefits related to cardiovascular and neural health. Human intake of omega-3 occurs mostly via seafood, particularly fish. The primary source of omega-3 in aquatic systems is represented by primary producers, from which omega-3 are transferred throughout the food web. Nitrogen is an essential nutrient for primary producers and can be supplied to surface waters as nitrate upwelled from below, or as ammonium and other regenerated nitrogen forms recycled in situ. Eastern Boundary Upwelling Systems (EBUS) are the most productive marine systems on Earth, together covering only 2% of the ocean’s surface area but supporting 25% of the global fish catch, thereby providing food for humans. In EBUS, nitrate and other nutrients are advected to the surface to support the proliferation of a phytoplankton community dominated by known omega-3 producers (i.e., diatoms). Given current climate change-related projections of ocean warming, acidification, deoxygenation, and increased upwelling intensity, phytoplankton community composition in EBUS may change. Additionally, the global production of EPA + DHA is expected to decrease by up to 30%, rendering its supply for human consumption insufficient by 2050. Here we discuss the state of knowledge related to omega-3 transfer from phytoplankton to small pelagic fish in EBUS, including factors that can influence omega-3 production, links to nitrogen cycling, climate change implications for the omega-3 supply to humans, and suggestions for future research directions to improve our understanding of omega-3 in the ocean.
Introduction
Omega-3 long chain polyunsaturated fatty acids (hereafter, omega-3) such as eicosapentaenoic-acid (20:5n-3, EPA) and docosahexaenoic-acid (22:6n-3, DHA) are essential compounds for marine consumers and human health (Ruess and Müller-Navarra, 2019), and are acquired through dietary intake (Arts et al., 2001). The main producers of omega-3 in the ocean are phytoplankton, with the amount of omega-3 contained in consumers being determined by the type of phytoplankton at the base of the food chain, and the transfer efficiency of omega-3 across trophic levels (Dalsgaard et al., 2003). In this context, zooplankton represent a key trophic link between the producers of omega-3 and small pelagic fish (e.g., sardine, anchovy, and herring; Bi and Sommer, 2020), the latter being a major source of omega-3 for human consumption (Hicks et al., 2019), as well as a main constituent of aquaculture feeds (Tacon and Metian, 2009). The supply of omega-3 is expected to change in the near future as a consequence of climate change (e.g., Hixson and Arts, 2016; Colombo et al., 2020), with implications for meeting the omega-3 requirements of a growing human population that is predicted to reach 11 billion by 2100 (Roser, 2013). Major climate-driven factors relevant to omega-3 production include warming (Hixson and Arts, 2016), ocean acidification (Dörner et al., 2020), and variability in upwelling intensity (Puccinelli et al., 2016a).
Coastal upwelling areas are amongst the most productive ocean regions owing to the wind-driven advection of nutrient-rich waters to the surface that promote primary and secondary production (Peterson et al., 1988; Vargas et al., 2007; Flynn et al., 2018). In these regions, nitrate (NO3–) upwelled from depth is the major source of nitrogen (N) for primary production (Waldron and Probyn, 1992; Messié et al., 2009; González-Galisteo et al., 2019), often supporting >>50% of phytoplankton growth (Chavez and Messié, 2009; Messié et al., 2009). At the cellular level, N is required for the synthesis of nucleic acids, amino acids, and chlorophyll (Dagenais-Bellefeuille and Morse, 2013), thus affecting omega-3 production (Yongmanitchai and Ward, 1991; Griffiths et al., 2012). Eastern Boundary Upwelling Systems (EBUS) occupy 2% of ocean surface but support 25% of the global fish catch and 8% of global primary production (Pauly and Christensen, 1995). These systems facilitate broad-scale fertilization of the upper water column and sustain many of the world’s important fisheries (Cury and Roy, 1989; Ward et al., 2006), including those centered around small pelagic fish, which provide up to 50% of annual human protein intake in some regions (e.g., West Africa; FAO, 2014). This is particularly relevant for the EBUS located off the African and South American continents, upon which millions of people rely for economic and food security. For instance, the Peruvian anchovy fishery accounts for 65% of global anchovy production and contributes 90% to the Peruvian fishery sector’s Gross Domestic Product (Aronés et al., 2019).
Here we provide an overview of the current state of knowledge on omega-3 transfer from phytoplankton to small pelagic fish in EBUS, including factors that can influence omega-3 production, links to the N cycle, climate change implications for omega-3 availability to humans, current gaps in our understanding and suggestions for future developments needed to increase our knowledge of omega-3 in the ocean. As an essential compound for human health (Ruess and Müller-Navarra, 2019), omega-3 could aid in ameliorating malnutrition, thus playing a fundamental role in food security. Additionally, understanding the role that upwelling plays in omega-3 production and how this may vary with climate change, is relevant to the following Sustainable Development Goals (SDGs): zero hunger (SDG2), climate action (SDG13), and life under the sea (SDG14).
Omega-3 Production and Role in the Ocean
In the ocean, omega-3 are mostly synthesized by phytoplankton, and phytoplankton community composition is a major factor regulating the amount of omega-3 produced in a particular system (Galloway and Winder, 2015). High food-quality species are characterized by high levels of EPA and/or DHA [e.g., diatoms and dinoflagellates, EPA and/or DHA > 25% of total fatty acids (FA); Hixson and Arts, 2016; Jónasdóttir, 2019] and low food-quality species by lower concentrations of EPA + DHA [e.g., cyanobacteria, which produce mostly short-chain polyunsaturated FA (PUFA), i.e., ≤C18 PUFA; Parrish, 2013]. Specifically, diatoms appear to be a major producer of EPA (Dalsgaard et al., 2003), while dinophytes and haptophytes are the main sources of DHA in the ocean (Figure 1; Remize et al., 2020). FA production can be affected by abiotic factors including temperature, which plays a key role in controlling the level of FA unsaturation, influencing the fluidity of cell membranes (Rousch et al., 2003); light availability, which affects phytoplankton species composition (Harrison et al., 1990); and salinity, which influences membrane permeability (Chen et al., 2008).
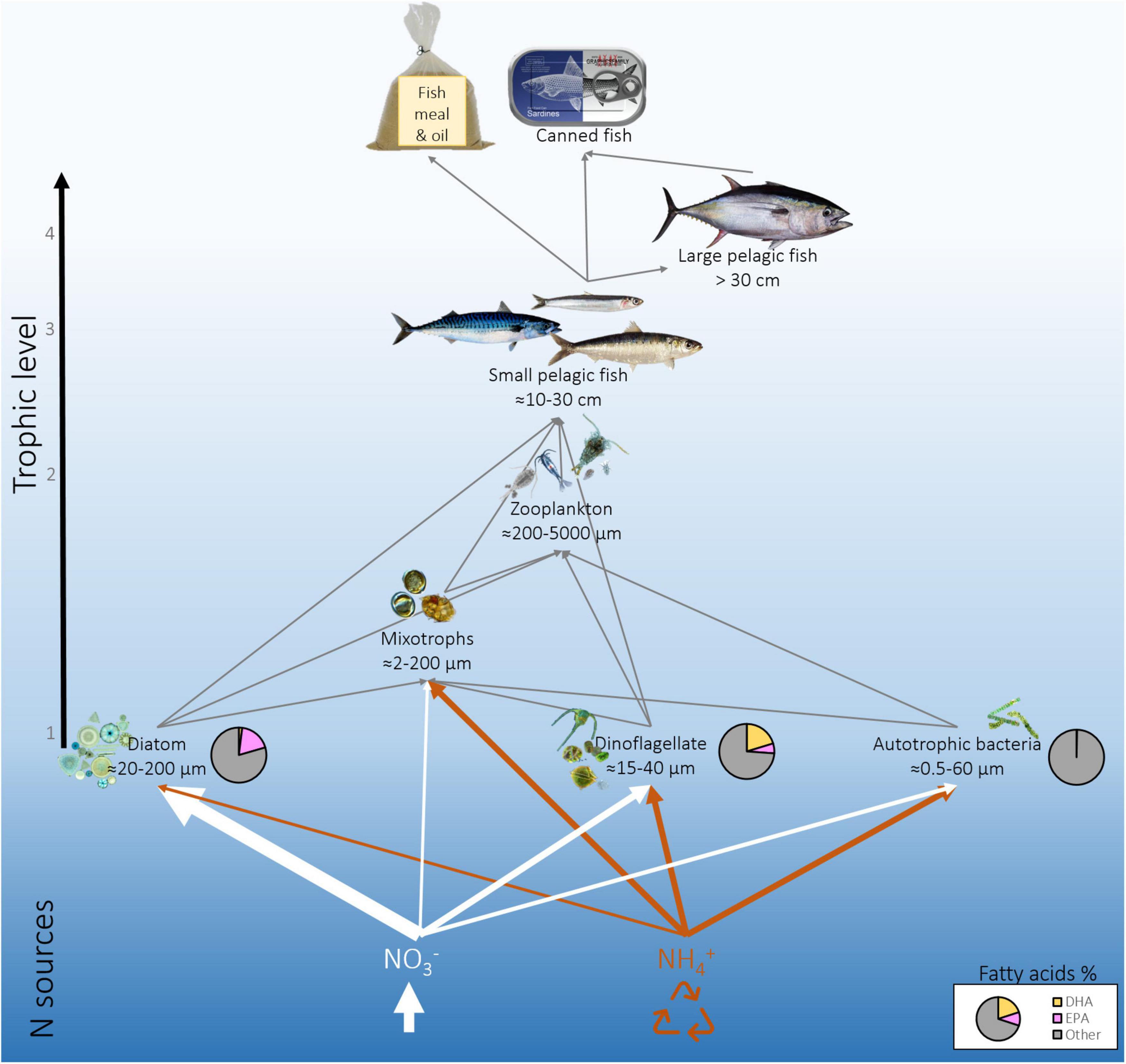
Figure 1. Schematic representation of omega-3 pathways in an upwelling system across trophic levels, focusing on the link between nitrogen (N) sources [newly upwelled N (NO3–, white) and recycled N (NH4+, orange)], select primary producers (diatoms, dinoflagellates, autotrophic bacteria), primary consumers (zooplankton), and higher trophic levels (small and large pelagic fish). The thickness of the white and orange lines indicates the relative use of upwelled NO3– and recycled NH4+ by the different phytoplankton groups. Pie charts show the % of eicosapentaenoic-acid (EPA), docosahexaenoic-acid (DHA), and the sum of the other fatty acids (Other) produced by the three phytoplankton groups. With climate change, upwelling is predicted to intensify, which may increase the upward supply of NO3– and drive the ecosystem towards enhanced diatom growth, higher transfer efficiency, and elevated omega-3 production (i.e., the thick white lines will become thicker). Alternately, warming may drive a net decline in upwelled NO3–, resulting in enhanced NH4+ recycling, a shift toward smaller phytoplankton species, less efficient trophic transfer, and a decline in omega-3 production (i.e., the thick orange arrows will become thicker, and the white arrows will become thinner). We note that these scenarios represent just two possibilities, and that there are likely other potential outcomes. Additionally, it is conceivable that the four EBUS will not respond to climate change in the same way.
Omega-3 are a limiting factor for zooplankton and higher trophic levels production (Bi and Sommer, 2020), as these organisms cannot synthesize these FA in sufficient quantities to support their nutritional requirements and must thus acquire them through their diet (Dalsgaard et al., 2003; Litzow et al., 2006). Omega-3 are precursors to eicosanoid hormones and a major component of cell membranes (Parrish, 2013), playing a fundamental role in maintaining membrane functioning, which can be modified by changing the length and the level of unsaturation of the FA carbon chains (Sinensky, 1974; Guschina and Harwood, 2006). The essential role of omega-3 in marine organism fitness is widely known. For instance, zooplankton growth, development and reproduction output are dependent on the amount of omega-3 available in their food (Müller-Navarra et al., 2000; Brett et al., 2006; Ravet et al., 2010). Similarly, fish brain and optic tectum development and oocyte quality depend on the DHA content of their prey (Bell et al., 1995; Garrido et al., 2007), and fish larvae with a higher EPA + DHA content present enhanced swimming ability, schooling behavior, and higher survival rates after hatching (Ishizaki et al., 2001; Mourente, 2003).
Links Between Marine Nitrogen Cycling and Omega-3
Phytoplankton community composition depends on several factors including nutrient availability. In the euphotic zone, phytoplankton growth can be supported by NO3– supplied from below, for instance via upwelling (yielding “new production”), or by N forms such as ammonium (NH4+) recycled in the upper ocean (“regenerated production”; Dugdale and Goering, 1967). When available, NH4+ is often the preferred N form consumed by phytoplankton because its low oxidation state (-3, the same as organic N) renders its assimilation energetically favorable (Dortch, 1990). However, surface NH4+ concentrations are typically << 1 μM (Paulot et al., 2015), although its flux through the food web can be large (Gruber, 2008; Altieri et al., 2021). High NO3– availability typically leads to growth of large phytoplankton including diatoms (Kudela and Dugdale, 2000; Fawcett and Ward, 2011), while smaller phytoplankton such as cyanobacteria tend toward NH4+ dependence (Probyn and Painting, 1985; Fawcett et al., 2011).
Upwelling events are among the main supply mechanisms of nutrients essential for phytoplankton production (e.g., NO3–, silicate, iron; Dugdale and Wilkerson, 1998; Chavez and Messié, 2009). In upwelling systems, the ratio between new and total (i.e., new + regenerated) production is significantly higher than in the open ocean (0.5–0.8 versus ∼0.1; Eppley and Peterson, 1979; Laws, 2004) due to the near-constant supply of NO3–, which underpins the elevated productivity of these regions (Messié and Chavez, 2015). Similarly, higher-than-average surface-water iron concentrations favor phytoplankton consumption of upwelled NO3– over recycled N (Dugdale and Goering, 1967; Bruland et al., 2001), leading to a phytoplankton community dominated by omega-3-rich diatoms. A change in community from diatoms to dinophytes is commonly observed during an upwelling cycle, especially when silicate, essential for the synthesis of diatom frustules, becomes depleted (Martin-Jézéquel et al., 2000; Tilstone et al., 2000).
Since different phytoplankton contain different amounts of omega-3, changes in community composition can cause variations in omega-3 transfer to consumers, ultimately affecting the omega-3 supply throughout the food web (Bermúdez et al., 2016; Garzke et al., 2016). For instance, it was shown that an increase in pCO2 caused a shift from nanophytoplankton to picoeukaryotes along with a decline in PUFA in both phytoplankton size fractions, which was mirrored by a PUFA reduction in the copepod species feeding on them (Bermúdez et al., 2016). Similarly, interannual FA variations in small pelagic fish in the California Current was attributed to a shift from a dinoflagellate- to a diatom-dominated food source (Litz et al., 2010). Upwelling food webs are made more complex by the presence of mixotrophic nanoflagellates, organisms that are able to directly consume bacteria and picoplankton (Frias-Lopez et al., 2009), but that can also contribute significantly to primary production, especially during periods of upwelling relaxation (Figueiras et al., 2020). Disentangling omega-3 produced by autotrophic versus mixotrophic organisms in the ocean is challenging, and may lead to an over/underestimation of the omega-3 supply from primary producers.
The Eastern Boundary Upwelling Systems
The world’s four major EBUS are associated with the Benguela, California, Canary, and Humboldt Currents. Rates of primary production are comparable among these regions (>900 gCm–2y–1, Table 1), with the exception of the California system where they are two-fold lower (480 gCm–2y–1), probably due to the lower NO3– supply to the euphotic zone (Chavez and Messié, 2009). The availability of regenerated N and the mechanisms of its production (i.e., herbivores, heterotrophic bacteria, higher trophic-level organisms) vary among the systems (Dugdale, 1972). For instance, anchovies are responsible for most N regeneration in the Humboldt system (Kämpf and Chapman, 2016), while in the southern Benguela, up to 48% of the subsurface nutrients are regenerated on-shelf by heterotrophic bacteria (Flynn et al., 2020). EBUS fertilization by dust deposition also influences productivity, especially in the Canary and Benguela systems where the Sahara and Namib Deserts supply nutrients that regularly limit phytoplankton growth, including iron and phosphate (Jickells et al., 2005; Pabortsava et al., 2017).
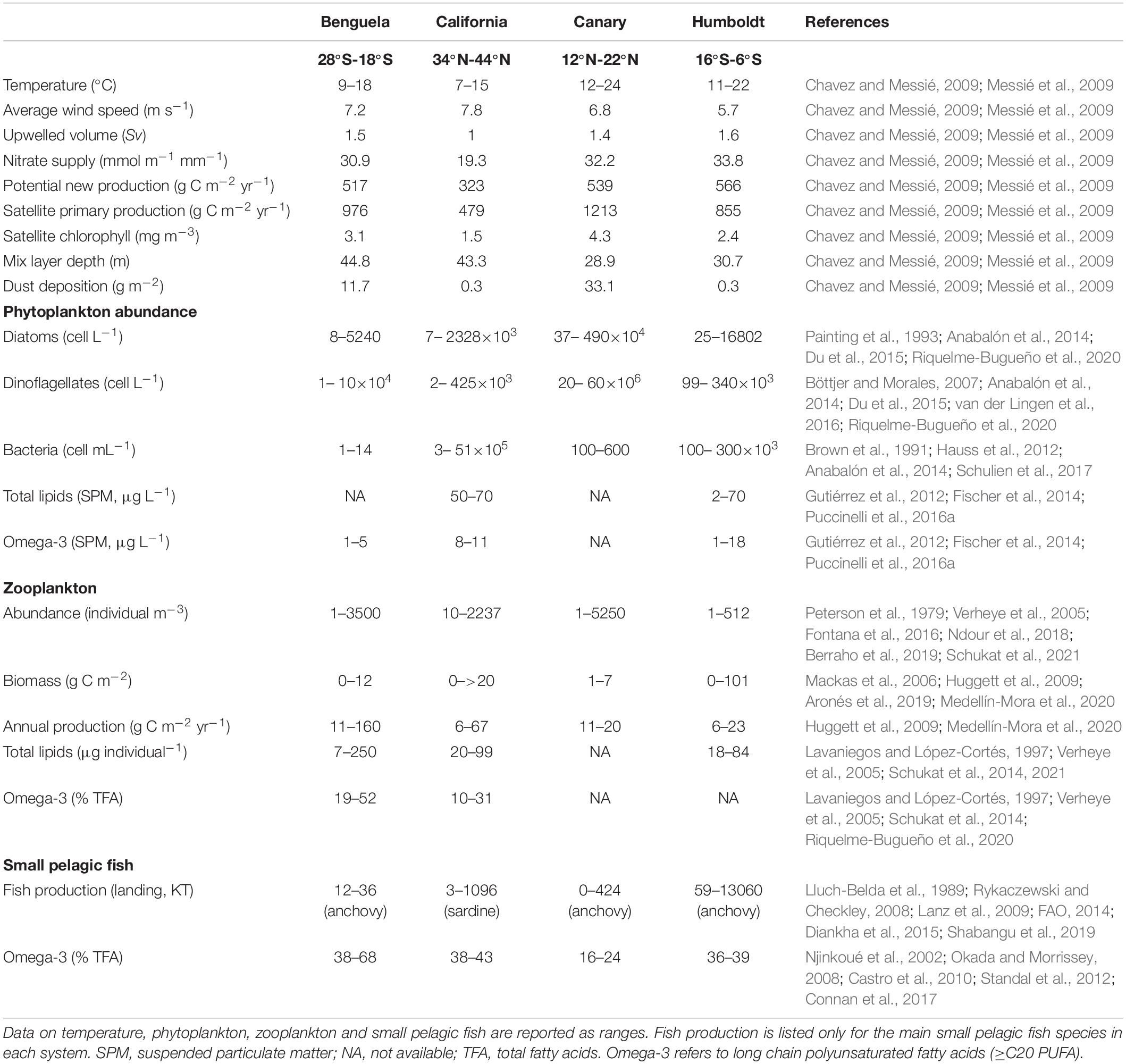
Table 1. Comparison of selected abiotic and biotic parameters in the four Eastern Boundary Upwelling Systems (Benguela, California, Canary, Humboldt) based on published literature.
The high primary productivity of EBUS propagates throughout their ecosystems, with a given concentration of phytoplankton supporting approximately 25- and 50-times as much zooplankton biomass and fish production (Chavez and Messié, 2009; Kämpf, and Chapman, 2016). In the Humboldt system, fish production and zooplankton biomass are an order of magnitude higher than in the other three systems (Table 1), which has been attributed to a high transfer efficiency among trophic levels, resulting in optimal conditions for the proliferation and retention of zooplankton and fish (Chavez and Messié, 2009). The same pattern is not evident in omega-3 content, which varies considerably within and among systems. For instance, Benguela phytoplankton contain the lowest amount of omega-3, while omega-3 in zooplankton and small pelagic fish is higher compared to the other EBUS. We note, however, that limited information is available on omega-3 in EBUS, especially for the Canary system, precluding our ability to generalize while also highlighting the need for further investigations.
Climate Change Effects on Upwelling Systems and N Supply
As a result of climate change, upwelling frequency appears to be increasing in several EBUS (Sydeman et al., 2014) due to increasing wind stress (Narayan et al., 2010). This may increase the nutrient supply to phytoplankton, favoring large diatoms and higher transfer efficiency, yielding enhanced production of omega-3. However, changes to upwelling are likely to be complex (Rykaczewski et al., 2015), and along with warming and/or acidification of surface waters, will modify phytoplankton metabolism and community composition, potentially favoring smaller phytoplankton (e.g., picoeukaryotes) at the expense of the nano- and microphytoplankton (e.g., diatoms, chlorophytes, haptophytes; Pérez et al., 2010; Armbrecht et al., 2014). A decrease in phytoplankton cell size will likely lead to longer food webs and a reduction in trophic transfer efficiency, with a consequent decline in omega-3 transfer to higher trophic levels (Berglund et al., 2007; Bermúdez et al., 2016).
Global warming is predicted to enhance seawater stratification while decreasing ocean mixing, reducing the upward NO3– supply (Falkowski et al., 1998; Sarmiento et al., 1998; Capotondi et al., 2012) and favoring the growth of species such as cyanobacteria and mixotrophic nanoflagellates (Peter and Sommer, 2012; Leles et al., 2018). In general, smaller phytoplankton preferentially consume NH4+ (Fawcett et al., 2011; Glibert et al., 2016), such that their growth should be favored under lower-nutrient conditions, in comparison to that of diatoms, which mainly utilize NO3– (Kudela and Dugdale, 2000; Fawcett and Ward, 2011). In contrast, by increasing the bioavailability of micronutrients such as iron, ocean acidification may promote the growth of phytoplankton reliant on NO3–, the assimilation of which has a high iron requirement (Price et al., 1991; Schoffman et al., 2016). That said, increased iron availability is also predicted to favor the growth of N2 fixers (Wannicke et al., 2018), a group of cyanobacteria (Monteiro et al., 2010) that produce predominantly short-chain PUFA (Parrish, 2013).
While much remains unknown of how different phytoplankton species will respond to climate change, particularly given the number of overlapping drivers and their potentially complex interactions, any change in community composition will have consequences for omega-3 production. These effects will be reflected in the amount of omega-3 transferred to consumers, as well as on their performance and survival strategies. For instance, a decrease in the amount of essential FA available to consumers has been shown to reduce the abundances, growth, and reproduction of zooplankton (Rossoll et al., 2012). At lower pH (i.e., high pCO2), fish larvae tend to stock omega-3 to increase their chances of survival (Díaz-Gil et al., 2015), in contrast to Antarctic krill that appear unaffected by increasing pCO2 (Ericson et al., 2019). Additionally, different consumers have different omega-3 requirements (Litzow et al., 2006), such that their responses to climate change are likely to be variable and difficult to predict.
Knowledge Gaps and Future Directions
There is broad consensus that upwelling shapes the diets of primary and secondary consumers, from coastal (Puccinelli et al., 2016a, b; Docmac et al., 2017) to open-ocean environments (Hauss et al., 2012; Schukat et al., 2021). Globally, the omega-3 supply from fish is projected to soon become insufficient for the growing human population, although the sign and magnitude of future changes in EBUS omega-3 production are unknown. Global estimates indicate that phytoplankton and zooplankton EPA + DHA supplies amount to 80 Mt, yet approximately 90% of EPA + DHA is lost between primary producers and higher predators (Hamilton et al., 2020). Humans require 1.4 Mt of EPA + DHA annually to meet the 500 mg daily recommended intake, yet only 0.8 Mt are available, largely from aquaculture and fisheries (Tocher, 2015; Hamilton et al., 2020). Despite the general understanding that the omega-3 supply to humans is limited, information on omega-3 production and transfer in productive upwelling systems is scarce, and almost absent at the level of primary consumers such as zooplankton and small pelagic fish (Table 1). The available studies are largely laboratory- or mesocosm-based investigations that constrain selected environmental parameters in order to investigate a specific metabolic or physiological pathway (De Troch et al., 2012; Mayor et al., 2015). Meta-analyses have been used as an alternative tool to formulate predictions related to omega-3 supply (e.g., Galloway and Winder, 2015; Hixson and Arts, 2016), but they are usually conducted at a large scale (regional, global) and focused on primary producers. Recently, an algorithm that links satellite chlorophyll-a data and EPA production was developed for the northwestern Atlantic and used for global EPA estimates (Budge et al., 2014). Applying this algorithm to EBUS that collectively support 8% of global primary production yields an EBUS EPA production rate of 19.2 Mt year–1. Assuming a transfer efficiency of 10% among trophic levels and a three-level food chain, we estimate that 0.19 Mt year–1 of EPA is available in the small pelagic fish of EBUS. While this approach is useful for illustrating the role of EBUS in omega-3 production, it has several limitations, such as assuming that diatoms are the only EPA producers, neglecting DHA production, and assuming a 10% transfer efficiency. Additionally, while the studies cited above have advanced our knowledge of omega-3 in the ocean, they do not provide reliable estimates of EPA + DHA or consider future changes in omega-3 production.
Coupled physical-biogeochemical models are powerful tools for understanding ocean dynamics and their role in biogeochemical cycles at various spatial (from global to regional) and temporal (event to climate) scales (Brasseur et al., 2009; Bachèlery et al., 2016). Biogeochemical models are often used to represent the cycles of carbon and selected nutrients (e.g., N), but they can also simulate energy flow to higher trophic levels (Koné et al., 2005; Chust et al., 2014). These models encompass a variety of complexities and applications, ranging from Nitrate-Phytoplankton-Zooplankton-Detritus to more complex planktonic food webs (Rose et al., 2007; Aumont et al., 2015; Butenschön et al., 2016). They depend, however, on field observations and experimental data to define parameters and constrain assumptions. 3D-physical-biogeochemical models have been developed for the four EBUS to simulate marine productivity and carbon cycling at low trophic levels (i.e., phytoplankton and zooplankton) (Lachkar and Gruber, 2012; Gutknecht et al., 2013; Auger et al., 2016; Espinoza-Morriberón et al., 2017; Glock et al., 2018; Cheresh and Fiechter, 2020). While the models account for the N source(s) available to phytoplankton and the main nutrients ratios (cell-quota formulation), none of them track omega-3 in either the primary or secondary consumers. The only mathematical model to investigate omega-3 transfer between phytoplankton and zooplankton is the mechanistic approach developed by Perhar et al. (2012, 2013), which accounts for omega-3 availability in its calculation of grazing efficiency. This model has been used to investigate the role of N, phosphorus and omega-3 in zooplankton growth and abundances. A combined physical-biogeochemical model that includes the approach of Perhar et al. (2012, 2013) could be implemented for EBUS to (i) elucidate the mechanisms governing the fate of omega-3 production based on the N source(s) available to phytoplankton, and (ii) quantify the amount of omega-3 available to small pelagic fish, at present and in the future, considering EBUS-specific parameters.
Summary
Omega-3 are essential compounds for all living organisms and their supply is tightly connected to phytoplankton growth and community composition in the ocean, as well as to the efficiency of their transfer through the food web. Human population growth and the likely decline in omega-3 availability linked to global warming underscore the urgency of prioritizing long-term predictions of omega-3 supply in EBUS. In particular, there is a clear need for a predictive model to explore climate-induced changes in omega-3 supply throughout the food web; such a model would be valuable to multiple sectors, including fisheries management and food production.
Author Contributions
EP, LP, SF, EM, and PS conceptualization. EP wrote the first manuscript draft with major contribution from FS, SF, and PS. All authors contributed to the article and approved the submitted version.
Funding
This work is supported by the ISblue project, Interdisciplinary graduate School for the blue planet (ANR-17-EURE-0015) and co-funded by a grant from the French government under the program “Investissements d’Avenir.” This work is a contribution to the SOLAB project (ANR-18-CE32-0009). SF acknowledges the support of a Royal Society/African Academy of Sciences FLAIR fellowship.
Conflict of Interest
The authors declare that the research was conducted in the absence of any commercial or financial relationships that could be construed as a potential conflict of interest.
References
Altieri, K. E., Fawcett, S. E., and Hastings, M. G. (2021). Reactive nitrogen cycling in the atmosphere and ocean. Annu. Rev. Earth Planet. Sci. 49, 513–540. doi: 10.1146/annurev-earth-083120-052147
Anabalón, V., Arístegui, J., Morales, C. E., Andrade, I., Benavides, M., Correa-Ramírez, M. A., et al. (2014). The structure of planktonic communities under variable coastal upwelling conditions off Cape Ghir (31∘N) in the canary current system (NW Africa). Prog. Oceanogr. 120, 320–339. doi: 10.1016/j.pocean.2013.10.015
Armbrecht, L. H., Roughan, M., Rossi, V., Schaeffer, A., Davies, P. L., Waite, A. M., et al. (2014). Phytoplankton composition under contrasting oceanographic conditions: upwelling and downwelling (Eastern Australia). Cont. Shelf Res. 75, 54–67. doi: 10.1016/j.csr.2013.11.024
Aronés, K., Grados, D., Ayón, P., and Bertrand, A. (2019). Spatio-temporal trends in zooplankton biomass in the northern Humboldt current system off Peru from 1961-2012. Deep Sea Res. II Top. Stud. Oceanogr. 169–170, 104656. doi: 10.1016/j.dsr2.2019.104656
Arts, M. T., Ackman, R. G., and Holub, B. J. (2001). “Essential fatty acids” in aquatic ecosystems: a crucial link between diet and human health and evolution. Can. J. Fish. Aquat. Sci. 58, 122–137. doi: 10.1139/f00-224
Auger, P.-A., Gorgues, T., Machu, E., Aumont, O., and Brehmer, P. (2016). What drives the spatial variability of primary productivity and matter fluxes in the North-West African upwelling system? A modelling approach and box analysis. Biogeosci. Discuss. 13, 6419–6440. doi: 10.5194/bg-2016-156
Aumont, O., Ethé, C., Tagliabue, A., Bopp, L., and Gehlen, M. (2015). PISCES-v2: an ocean biogeochemical model for carbon and ecosystem studies. Geosci. Model Dev. 8, 2465–2513. doi: 10.5194/gmd-8-2465-2015
Bachèlery, M.-L., Illig, S., and Dadou, I. (2016). Forcings of nutrient, oxygen, and primary production interannual variability in the southeast Atlantic Ocean. Geophys. Res. Lett. 43, 8617–8625. doi: 10.1002/2016GL070288
Bell, M. V., Batty, R. S., Dick, J. R., Fretwell, K., Navarro, J. C., and Sargent, J. R. (1995). Dietary deficiency of docosahexaenoic acid impairs vision at low light intensities in juvenile herring (Clupea harengus L.). Lipids 30, 443–449. doi: 10.1007/BF02536303
Berglund, J., Müren, U., Båmstedt, U., and Andersson, A. (2007). Efficiency of a phytoplankton-based and a bacterial-based food web in a pelagic marine system. Limnol. Oceanogr. 52, 121–131. doi: 10.4319/lo.2007.52.1.0121
Bermúdez, J. R., Riebesell, U., Larsen, A., and Winder, M. (2016). Ocean acidification reduces transfer of essential biomolecules in a natural plankton community. Sci. Rep. 6:27749. doi: 10.1038/srep27749
Berraho, A., Abdelouahab, H., Baibai, T., Charib, S., Larissi, J., Agouzouk, A., et al. (2019). Short-term variation of zooplankton community in Cintra Bay (Northwest Africa). Oceanologia 61, 368–383. doi: 10.1016/j.oceano.2019.02.001
Bi, R., and Sommer, U. (2020). Food quantity and quality interactions at phytoplankton–zooplankton interface: chemical and reproductive responses in a calanoid copepod. Front. Mar. Sci. 7:274. doi: 10.3389/fmars.2020.00274
Böttjer, D., and Morales, C. E. (2007). Nanoplanktonic assemblages in the upwelling area off concepción (∼36∘S), central chile: abundance, biomass, and grazing potential during the annual cycle. Prog. Oceanogr. 75, 415–434. doi: 10.1016/j.pocean.2007.08.024
Brasseur, P., Gruber, N., Barciela, R., Brander, K., Doron, M., Moussaoui, A. E., et al. (2009). Integrating biogeochemistry and ecology into ocean data assimilation systems. Oceanography 22, 206–215.
Brett, M. T., Müller-Navarra, D. C., Ballantyne, A. P., Ravet, J. L., and Goldman, C. R. (2006). Daphnia fatty acid composition reflects that of their diet. Limnol. Oceanogr. 51, 2428–2437. doi: 10.4319/lo.2006.51.5.2428
Brown, P. C., Painting, S. J., and Cochrane, K. L. (1991). Estimates of phytoplankton and bacterial biomass and production in the northern and southern Benguela ecosystems. S. Afr. J. Mar. Sci. 11, 537–564. doi: 10.2989/025776191784287673
Bruland, K. W., Rue, E. L., and Smith, G. J. (2001). Iron and macronutrients in California coastal upwelling regimes: implications for diatom blooms. Limnol. Oceanogr. 46, 1661–1674. doi: 10.4319/lo.2001.46.7.1661
Budge, S. M., Devred, E., Forget, M.-H., Stuart, V., Trzcinski, M. K., Sathyendranath, S., et al. (2014). Estimating concentrations of essential omega-3 fatty acids in the ocean: supply and demand. ICES J. Mar. Sci. 71, 1885–1893. doi: 10.1093/icesjms/fsu003
Butenschön, M., Clark, J., Aldridge, J. N., Allen, J. I., Artioli, Y., Blackford, J., et al. (2016). ERSEM 15.06: a generic model for marine biogeochemistry and the ecosystem dynamics of the lower trophic levels. Geosci. Model Dev. 9, 1293–1339. doi: 10.5194/gmd-9-1293-2016
Capotondi, A., Alexander, M. A., Bond, N. A., Curchitser, E. N., and Scott, J. D. (2012). Enhanced upper ocean stratification with climate change in the CMIP3 models. J. Geophys. Res. 117:C04031. doi: 10.1029/2011JC007409
Castro, L. R., Claramunt, G., González, H. E., Krautz, M. C., Llanos-Rivera, A., Méndez, J., et al. (2010). Fatty acids in eggs of anchoveta Engraulis ringens during two contrasting winter spawning seasons. Mar. Ecol. Prog. Ser. 420, 193–205. doi: 10.3354/meps08819
Chavez, F. P., and Messié, M. (2009). A comparison of eastern boundary upwelling ecosystems. Prog. Oceanogr. 83, 80–96. doi: 10.1016/j.pocean.2009.07.032
Chen, G.-Q., Jiang, Y., and Chen, F. (2008). Salt-induced alterations in lipid composition of diatom nitzschia laevis (bacillariophyceae) under heterotrophic culture condition1. J. Phycol. 44, 1309–1314. doi: 10.1111/j.1529-8817.2008.00565.x
Cheresh, J., and Fiechter, J. (2020). Physical and biogeochemical drivers of alongshore pH and oxygen variability in the California current system. Geophys. Res. Lett. 47:e2020GL089553. doi: 10.1029/2020GL089553
Chust, G., Allen, J. I., Bopp, L., Schrum, C., Holt, J., Tsiaras, K., et al. (2014). Biomass changes and trophic amplification of plankton in a warmer ocean. Glob. Chang. Biol. 20, 2124–2139. doi: 10.1111/gcb.12562
Colombo, S. M., Rodgers, T. F. M., Diamond, M. L., Bazinet, R. P., and Arts, M. T. (2020). Projected declines in global DHA availability for human consumption as a result of global warming. Ambio 49, 865–880. doi: 10.1007/s13280-019-01234-6
Connan, M., Bonnevie, B. T., Hagen, C., van der Lingen, C. D., and McQuaid, C. (2017). Diet specialization in a colonial seabird studied using three complementary dietary techniques: effects of intrinsic and extrinsic factors. Mar. Biol. 164:171. doi: 10.1007/s00227-017-3201-2
Cury, P., and Roy, C. (1989). Optimal environmental window and pelagic fish recruitment success in upwelling areas. Can. J. Fish. Aquat. Sci. 46, 670–680. doi: 10.1139/f89-086
Dagenais-Bellefeuille, S., and Morse, D. (2013). Putting the N in dinoflagellates. Front. Microbiol. 4:4369. doi: 10.3389/fmicb.2013.00369
Dalsgaard, J., St. John, M., Kattner, G., Müller-Navarra, D., and Hagen, W. (2003). Fatty acid trophic markers in the pelagic marine environment. Adv. Mar. Biol. 46, 225–340.
De Troch, M., Boeckx, P., Cnudde, C., Van Gansbeke, D., Vanreusel, A., Vincx, M., et al. (2012). Bioconversion of fatty acids at the basis of marine food webs: insights from a compound-specific stable isotope analysis. Mar. Ecol. Prog. Ser. 465, 53–67. doi: 10.3354/meps09920
Diankha, O., Thiaw, M., Sow, B. A., Brochier, T., Gaye, A. T., and Brehmer, P. (2015). Round sardinella (Sardinella aurita) and anchovy (Engraulis encrasicolus) abundance as related to temperature in the senegalese waters. Thalassas 31, 9–17.
Díaz-Gil, C., Catalán, I. A., Palmer, M., Faulk, C. K., and Fuiman, L. A. (2015). Ocean acidification increases fatty acids levels of larval fish. Biol. Lett. 11:20150331. doi: 10.1098/rsbl.2015.0331
Docmac, F., Araya, M., Hinojosa, I. A., Dorador, C., and Harrod, C. (2017). Habitat coupling writ large: pelagic-derived materials fuel benthivorous macroalgal reef fishes in an upwelling zone. Ecology 98, 2267–2272. doi: 10.1002/ecy.1936
Dörner, I., Hauss, H., Aberle, N., Lohbeck, K., Spisla, C., Riebesell, U., et al. (2020). Ocean acidification impacts on biomass and fatty acid composition of a post-bloom marine plankton community. Mar. Ecol. Prog. Ser. 647, 49–64. doi: 10.3354/meps13390
Dortch, Q. (1990). The interaction between ammonium and nitrate uptake in phytoplankton. Mar. Ecol. Prog. Ser. 61, 183–201. doi: 10.3354/meps061183
Du, X., Peterson, W., and O’Higgins, L. (2015). Interannual variations in phytoplankton community structure in the northern California current during the upwelling seasons of 2001-2010. Mar. Ecol. Prog. Ser. 519, 75–87. doi: 10.3354/meps11097
Dugdale, R. C. (1972). Chemical oceanography and primary productivity in upwelling regions. Geoforum 3, 47–61. doi: 10.1016/0016-7185(72)90085-1
Dugdale, R. C., and Goering, J. J. (1967). Uptake of new and regenerated forms of nitrogen in primary productivity. Limnol. Oceanogr. 12, 196–206. doi: 10.4319/lo.1967.12.2.0196
Dugdale, R. C., and Wilkerson, F. P. (1998). Silicate regulation of new production in the equatorial Pacific upwelling. Nature 391, 270–273. doi: 10.1038/34630
Eppley, R. W., and Peterson, B. J. (1979). Particulate organic matter flux and planktonic new production in the deep ocean. Nature 282, 677–680. doi: 10.1038/282677a0
Ericson, J. A., Hellessey, N., Kawaguchi, S., Nichols, P. D., Nicol, S., Hoem, N., et al. (2019). Near-future ocean acidification does not alter the lipid content and fatty acid composition of adult Antarctic krill. Sci. Rep. 9:12375. doi: 10.1038/s41598-019-48665-5
Espinoza-Morriberón, D., Echevin, V., Colas, F., Tam, J., Ledesma, J., Vásquez, L., et al. (2017). Impacts of El Niño events on the Peruvian upwelling system productivity. J. Geophys. Res. 122, 5423–5444. doi: 10.1002/2016JC012439
Falkowski, P. G., Barber, R. T., and Smetacek, V. (1998). Biogeochemical controls and feedbacks on ocean primary production. Science 281, 200–206. doi: 10.1126/science.281.5374.200
FAO (2014). State of the World Fisheries and Aquaculture 2014. Rome: Food and agriculture organization.
Fawcett, S. E., and Ward, B. B. (2011). Phytoplankton succession and nitrogen utilization during the development of an upwelling bloom. Mar. Ecol. Prog. Ser. 428, 13–31. doi: 10.3354/meps09070
Fawcett, S. E., Lomas, M. W., Casey, J. R., Ward, B. B., and Sigman, D. M. (2011). Assimilation of upwelled nitrate by small eukaryotes in the Sargasso Sea. Nat. Geosci. 4, 717–722. doi: 10.1038/ngeo1265
Figueiras, F. G., Arbones, B., Castro, C. G., Froján, M., and Teixeira, I. G. (2020). About pigmented nanoflagellates and the importance of mixotrophy in a coastal upwelling system. Front. Mar. Sci. 7:144. doi: 10.3389/fmars.2020.00144
Fischer, A. M., Ryan, J. P., Levesque, C., and Welschmeyer, N. (2014). Characterizing estuarine plume discharge into the coastal ocean using fatty acid biomarkers and pigment analysis. Mar. Environ. Res. 99, 106–116. doi: 10.1016/j.marenvres.2014.04.006
Flynn, R. F., Burger, J. M., Pillay, K., and Fawcett, S. E. (2018). Wintertime rates of net primary production and nitrate and ammonium uptake in the southern Benguela upwelling system. Afr. J. Mar. Sci. 40, 253–266. doi: 10.2989/1814232X.2018.1502095
Flynn, R. F., Granger, J., Veitch, J. A., Siedlecki, S., Burger, J. M., Pillay, K., et al. (2020). On-shelf nutrient trapping enhances the fertility of the Southern Benguela Upwelling System. J. Geophys. Res. 125:e2019JC015948. doi: 10.1029/2019JC015948
Fontana, R. E., Elliott, M. L., Largier, J. L., and Jahncke, J. (2016). Temporal variation in copepod abundance and composition in a strong, persistent coastal upwelling zone. Prog. Oceanogr. 142, 1–16. doi: 10.1016/j.pocean.2016.01.004
Frias-Lopez, J., Thompson, A., Waldbauer, J., and Chisholm, S. W. (2009). Use of stable isotope-labelled cells to identify active grazers of picocyanobacteria in ocean surface waters. Environ. Microbiol. 11, 512–525. doi: 10.1111/j.1462-2920.2008.01793.x
Galloway, A. W. E., and Winder, M. (2015). Partitioning the relative importance of phylogeny and environmental conditions on phytoplankton fatty acids. PLoS One 10:e0130053. doi: 10.1371/journal.pone.0130053
Garrido, S., Rosa, R., Ben-Hamadou, R., Cunha, M. E., Chícharo, M. A., and van der Lingen, C. D. (2007). Effect of maternal fat reserves on the fatty acid composition of sardine (Sardina pilchardus) oocytes. Comp. Biochem. Physiol. B Biochem. Mol. Biol. 148, 398–409. doi: 10.1016/j.cbpb.2007.07.008
Garzke, J., Hansen, T., Ismar, S. M. H., and Sommer, U. (2016). Combined effects of ocean warming and acidification on copepod abundance, body size and fatty acid content. PLoS One 11:e0155952. doi: 10.1371/journal.pone.0155952
Glibert, P. M., Wilkerson, F. P., Dugdale, R. C., Raven, J. A., Dupont, C. L., Leavitt, P. R., et al. (2016). Pluses and minuses of ammonium and nitrate uptake and assimilation by phytoplankton and implications for productivity and community composition, with emphasis on nitrogen-enriched conditions. Limnol. Oceanogr. 61, 165–197. doi: 10.1002/lno.10203
Glock, N., Erdem, Z., Wallmann, K., Somes, C. J., Liebetrau, V., Schönfeld, J., et al. (2018). Coupling of oceanic carbon and nitrogen facilitates spatially resolved quantitative reconstruction of nitrate inventories. Nat. Commun. 9:1217. doi: 10.1038/s41467-018-03647-5
González-Galisteo, S., Packard, T. T., Gómez, M., Herrera, A., Dugdale, R. C., Wilkerson, F. P., et al. (2019). Calculating new production from nitrate reductase activity and light in the Peru current upwelling. Prog. Oceanogr. 173, 78–85. doi: 10.1016/j.pocean.2019.02.009
Griffiths, M. J., van Hille, R. P., and Harrison, S. T. L. (2012). Lipid productivity, settling potential and fatty acid profile of 11 microalgal species grown under nitrogen replete and limited conditions. J. Appl. Phycol. 24, 989–1001. doi: 10.1007/s10811-011-9723-y
Gruber, N. (2008). “The marine nitrogen cycle: overview and challenges,” in Nitrogen in the Marine Environment, ed. N. Gruber, D. G. Capone, D. A. Bronk, M. R. Mulholland, and E. J. Carpenterer (Amsterdam: Elsevier), 1–50. doi: 10.1016/B978-0-12-372522-6.00001-3
Guschina, I. A., and Harwood, J. L. (2006). Mechanisms of temperature adaptation in poikilotherms. FEBS Lett. 580, 5477–5483. doi: 10.1016/j.febslet.2006.06.066
Gutiérrez, M. H., Pantoja, S., and Lange, C. B. (2012). Biogeochemical significance of fatty acid distribution in the coastal upwelling ecosystem off Concepción (36∘S), Chile. Org. Geochem. 49, 56–67. doi: 10.1016/j.orggeochem.2012.05.010
Gutknecht, E., Dadou, I., Le Vu, B., Cambon, G., Sudre, J., Garçon, V., et al. (2013). Coupled physical/biogeochemical modeling including O2-dependent processes in the eastern boundary upwelling systems: application in the Benguela. Biogeosciences 10, 3559–3591. doi: 10.5194/bg-10-3559-2013
Hamilton, H. A., Newton, R., Auchterlonie, N. A., and Müller, D. B. (2020). Systems approach to quantify the global omega-3 fatty acid cycle. Nat. Food 1, 59–62. doi: 10.1038/s43016-019-0006-0
Harrison, P. J., Thompson, P. A., and Calderwood, G. S. (1990). Effects of nutrient and light limitation on the biochemical composition of phytoplankton. J. Appl. Phycol. 2, 45–56. doi: 10.1007/BF02179768
Hauss, H., Franz, J. M. S., and Sommer, U. (2012). Changes in N:P stoichiometry influence taxonomic composition and nutritional quality of phytoplankton in the Peruvian upwelling. J. Sea Res. 73, 74–85. doi: 10.1016/j.seares.2012.06.010
Hicks, C. C., Cohen, P. J., Graham, N. A. J., Nash, K. L., Allison, E. H., D’Lima, C., et al. (2019). Harnessing global fisheries to tackle micronutrient deficiencies. Nature 574, 95–98. doi: 10.1038/s41586-019-1592-6
Hixson, S. M., and Arts, M. T. (2016). Climate warming is predicted to reduce omega-3, long-chain, polyunsaturated fatty acid production in phytoplankton. Glob. Chang. Biol. 22, 2744–2755. doi: 10.1111/gcb.13295
Huggett, J., Verheye, H., Escribano, R., and Fairweather, T. (2009). Copepod biomass, size composition and production in the Southern Benguela: spatio–temporal patterns of variation, and comparison with other eastern boundary upwelling systems. Prog. Oceanogr. 83, 197–207. doi: 10.1016/j.pocean.2009.07.048
Ishizaki, Y., Masuda, R., Uematsu, K., Shimizu, K., Arimoto, M., and Takeuchi, T. (2001). The effect of dietary docosahexaenoic acid on schooling behaviour and brain development in larval yellowtail. J. Fish Biol. 58, 1691–1703. doi: 10.1111/j.1095-8649.2001.tb02323.x
Jickells, T. D., An, Z. S., Andersen, K. K., Baker, A. R., Bergametti, G., Brooks, N., et al. (2005). Global iron connections between desert dust, ocean biogeochemistry, and climate. Science 308, 67–71. doi: 10.1126/science.1105959
Jónasdóttir, S. H. (2019). Fatty acid profiles and production in marine phytoplankton. Mar. Drugs 17:151. doi: 10.3390/md17030151
Kämpf, J., and Chapman, P. (2016). Upwelling Systems of the World. Cham: Springer International Publishing.
Koné, V., Machu, E., Penven, P., Andersen, V., Garçon, V., Fréon, P., et al. (2005). Modelling the primary and secondary productions of the southern Benguela upwelling system: a comparative study through two biogeochemical models. Glob. Biogeochem. Cycles 19:GB4021. doi: 10.1029/2004GB002427
Kudela, R. M., and Dugdale, R. C. (2000). Nutrient regulation of phytoplankton productivity in Monterey Bay, California. Deep Sea Res. II Top. Stud. Oceanogr. 47, 1023–1053. doi: 10.1016/S0967-0645(99)00135-6
Lachkar, Z., and Gruber, N. (2012). A comparative study of biological production in eastern boundary upwelling systems using an artificial neural network. Biogeosciences 9, 293–308. doi: 10.5194/bg-9-293-2012
Lanz, E., Nevarez-Martinez, M., López-Martinez, J., and Dworak, J. A. (2009). Small pelagic fish catches in the Gulf of California associated with sea surface temperature and chlorophyll. Calif. Coop. cean Fish. Investig.Rep. 50, 134–146.
Lavaniegos, B. E., and López-Cortés, D. (1997). Fatty acid composition and community structure of plankton from the san lorenzo channel, gulf of California. Estuar. Coast. Shelf Sci. 45, 845–854. doi: 10.1006/ecss.1997.0245
Laws, E. A. (2004). New production in the equatorial Pacific: a comparison of field data with estimates derived from empirical and theoretical models. Deep Sea Res. I Oceanogr. Res. Pap. 51, 205–211. doi: 10.1016/j.dsr.2003.10.003
Leles, S. G., Polimene, L., Bruggeman, J., Blackford, J., Ciavatta, S., Mitra, A., et al. (2018). Modelling mixotrophic functional diversity and implications for ecosystem function. J. Plankton Res. 40, 627–642. doi: 10.1093/plankt/fby044
Litz, M. N. C., Brodeur, R. D., Emmett, R. L., Heppell, S. S., Rasmussen, R. S., O’Higgins, L., et al. (2010). Effects of variable oceanographic conditions on forage fish lipid content and fatty acid composition in the northern California Current. Mar. Ecol. Prog. Ser. 405, 71–85. doi: 10.3354/meps08479
Litzow, M. A., Bailey, K. M., Prahl, F. G., and Heintz, R. (2006). Climate regime shifts and reorganization of fish communities: the essential fatty acid limitation hypothesis. Mar. Ecol. Prog. Ser. 315, 1–11. doi: 10.3354/meps315001
Lluch-Belda, D., Crawford, R. J. M., Kawasaki, T., MacCall, A. D., Parrish, R. H., Schwartzlose, R. A., et al. (1989). World-wide fluctuations of sardine and anchovy stocks: the regime problem. S. Afr. J. Mar. Sci. 8, 195–205. doi: 10.2989/02577618909504561
Mackas, D. L., Peterson, W. T., Ohman, M. D., and Lavaniegos, B. E. (2006). Zooplankton anomalies in the California current system before and during the warm ocean conditions of 2005. Geophys. Res. Lett. 33:22. doi: 10.1029/2006GL027930
Martin-Jézéquel, V., Hildebrand, M., and Brzezinski, M. A. (2000). Silicon metabolism in diatoms: implications for growth. J. Phycol. 36, 821–840. doi: 10.1046/j.1529-8817.2000.00019.x
Mayor, D. J., Sommer, U., Cook, K. B., and Viant, M. R. (2015). The metabolic response of marine copepods to environmental warming and ocean acidification in the absence of food. Sci. Rep. 5:13690. doi: 10.1038/srep13690
Medellín-Mora, J., Atkinson, A., and Escribano, R. (2020). Community structured production of zooplankton in the eastern boundary upwelling system off central/southern Chile (2003–2012). ICES J. Mar. Sci. 77, 419–435. doi: 10.1093/icesjms/fsz193
Messié, M., and Chavez, F. P. (2015). Seasonal regulation of primary production in eastern boundary upwelling systems. Prog. Oceanogr. 134, 1–18. doi: 10.1016/j.pocean.2014.10.011
Messié, M., Ledesma, J., Kolber, D. D., Michisaki, R. P., Foley, D. G., and Chavez, F. P. (2009). Potential new production estimates in four eastern boundary upwelling ecosystems. Prog. Oceanogr. 83, 151–158. doi: 10.1016/j.pocean.2009.07.018
Monteiro, F. M., Follows, M. J., and Dutkiewicz, S. (2010). Distribution of diverse nitrogen fixers in the global ocean. Glob. Biogeochem. Cycles 24:GB3017. doi: 10.1029/2009GB003731
Mourente, G. (2003). “Accumulation of DHA (docosahexaenoic acid; 22:6n-3) in larval and juvenile fish brain,” in The Big Fish Bang, eds H. Browman and A. B. Skiftesvik (Bergen: Institute of Marine Research), 239–248.
Müller-Navarra, D. C., Brett, M. T., Liston, A. M., and Goldman, C. R. (2000). A highly unsaturated fatty acid predicts carbon transfer between primary producers and consumers. Nature 403, 74–77. doi: 10.1038/47469
Narayan, N., Paul, A., Mulitza, S., and Schulz, M. (2010). Trends in coastal upwelling intensity during the late 20th century. Ocean Sci. 6, 815–823. doi: 10.5194/os-6-815-2010
Ndour, I., Berraho, A., Fall, M., Ettahiri, O., and Sambe, B. (2018). Composition, distribution and abundance of zooplankton and ichthyoplankton along the Senegal-Guinea maritime zone (West Africa). Egypt. J. Aquat. Res. 44, 109–124. doi: 10.1016/j.ejar.2018.04.001
Njinkoué, J.-M., Barnathan, G., Miralles, J., Gaydou, E.-M., and Samb, A. (2002). Lipids and fatty acids in muscle, liver and skin of three edible fish from the Senegalese coast: Sardinella maderensis, Sardinella aurita and Cephalopholis taeniops. Comp. Biochem. Physiol. B Biochem. Mol. Biol. 131, 395–402. doi: 10.1016/S1096-4959(01)00506-1
Okada, T., and Morrissey, M. T. (2008). Seasonal changes in intrinsic characteristics of pacific sardine (Sardinops sagax). J. Aquat. Food Prod. Technol. 16, 51–71. doi: 10.1300/J030v16n01_05
Pabortsava, K., Lampitt, R. S., Benson, J., Crowe, C., McLachlan, R., Le Moigne, F. A. C., et al. (2017). Carbon sequestration in the deep Atlantic enhanced by Saharan dust. Nat. Geosci. 10, 189–194. doi: 10.1038/ngeo2899
Painting, S. J., Lucas, M. I., Peterson, W. T., Brown, P. C., Hutchings, L., and Mitchell-Innes, B. A. (1993). Dynamics of bacterioplankton, phytoplankton and mesozooplankton communities during the development of an upwelling plume in the southern Benguela. Mar. Ecol. Prog. Ser. 100, 35–53.
Parrish, C. C. (2013). Lipids in marine ecosystems. ISRN Oceanogr. 2013:e604045. doi: 10.5402/2013/604045
Paulot, F., Jacob, D. J., Johnson, M. T., Bell, T. G., Baker, A. R., Keene, W. C., et al. (2015). Global oceanic emission of ammonia: constraints from seawater and atmospheric observations. Glob. Biogeochem. Cycles 29, 1165–1178. doi: 10.1002/2015GB005106
Pauly, D., and Christensen, V. (1995). Primary production required to sustain global fisheries. Nature 374, 255–257. doi: 10.1038/374255a0
Pérez, F. F., Padín, X. A., Pazos, Y., Gilcoto, M., Cabanas, M., Pardo, P. C., et al. (2010). Plankton response to weakening of the Iberian coastal upwelling. Glob. Chang. Biol. 16, 1258–1267. doi: 10.1111/j.1365-2486.2009.02125.x
Perhar, G., Arhonditsis, G. B., and Brett, M. T. (2012). Modelling the role of highly unsaturated fatty acids in planktonic food web processes: a mechanistic approach. Environ. Rev. 20, 155–172. doi: 10.1139/a2012-007
Perhar, G., Arhonditsis, G. B., and Brett, M. T. (2013). Modelling the role of highly unsaturated fatty acids in planktonic food web processes: sensitivity analysis and examination of contemporary hypotheses. Ecol. Inform. 13, 77–98. doi: 10.1016/j.ecoinf.2012.10.005
Peter, K. H., and Sommer, U. (2012). Phytoplankton Cell size: intra- and interspecific effects of warming and grazing. PLoS One 7:e49632. doi: 10.1371/journal.pone.0049632
Peterson, W. T., Arcos, D. F., McManus, G. B., Dam, H., Bellantoni, D., Johnson, T., et al. (1988). The nearshore zone during coastal upwelling: daily variability and coupling between primary and secondary production off central Chile. Prog. Oceanogr. 20, 1–40. doi: 10.1016/0079-6611(88)90052-3
Peterson, W. T., Miller, C. B., and Hutchinson, A. (1979). Zonation and maintenance of copepod populations in the Oregon upwelling zone. Deep. Sea. Res. part A. Oceanographic Research Papers 26, 467–494. doi: 10.1016/0198-0149(79)90091-8
Price, N. M., Andersen, L. F., and Morel, F. M. M. (1991). Iron and nitrogen nutrition of equatorial Pacific plankton. Deep Sea Res. A. Oceanogr. Res. Pap. 38, 1361–1378. doi: 10.1016/0198-0149(91)90011-4
Probyn, T. A., and Painting, S. J. (1985). Nitrogen uptake by size-fractionated phytoplankton populations in Antarctic surface waters. Limnol. Oceanogr. 30, 1327–1332. doi: 10.4319/lo.1985.30.6.1327
Puccinelli, E., McQuaid, C. D., and Noyon, M. (2016a). Spatio-temporal variation in effects of upwelling on the fatty acid composition of benthic filter feeders in the Southern Benguela Ecosystem: not all upwelling is equal. PLoS One 11:e0161919. doi: 10.1371/journal.pone.0161919
Puccinelli, E., Noyon, M., and McQuaid, C. D. (2016b). Hierarchical effects of biogeography and upwelling shape the dietary signatures of benthic filter feeders. Mar. Ecol. Prog. Ser. 543, 37–54. doi: 10.3354/meps11567
Ravet, J. L., Brett, M. T., and Arhonditsis, G. B. (2010). The effects of seston lipids on zooplankton fatty acid composition in Lake Washington, Washington, USA. Ecology 91, 180–190.
Remize, M., Planchon, F., Loh, A. N., Grand, F. L., Bideau, A., Goic, N. L., et al. (2020). Study of synthesis pathways of the essential polyunsaturated fatty acid 20:5n-3 in the diatom Chaetoceros muelleri using 13C-isotope labelling. Biomolecules 10:797. doi: 10.3390/biom10050797
Riquelme-Bugueño, R., Pantoja, S., Jorquera, E., Anabalón, V., Srain, B., and Schneider, W. (2020). Fatty acid composition in the endemic Humboldt Current krill, Euphausia mucronata (Crustacea, Euphausiacea) in relation to the phytoplankton community and oceanographic variability off Dichato coast in central Chile. Prog. Oceanogr. 188:102425. doi: 10.1016/j.pocean.2020.102425
Rose, K. A., Werner, F. E., Megrey, B. A., Aita, M. N., Yamanaka, Y., Hay, D. E., et al. (2007). Simulated herring growth responses in the Northeastern Pacific to historic temperature and zooplankton conditions generated by the 3-dimensional NEMURO nutrient–phytoplankton–zooplankton model. Ecol. Model. 202, 184–195. doi: 10.1016/j.ecolmodel.2006.06.020
Roser, M. (2013). Future Population Growth. Available online at: https://ourworldindata.org/future-population-growth [Accessed November 6, 2020].
Rossoll, D., Bermúdez, R., Hauss, H., Schulz, K. G., Riebesell, U., Sommer, U., et al. (2012). Ocean acidification-induced food quality deterioration constrains trophic transfer. PLoS One 7:e34737. doi: 10.1371/journal.pone.0034737
Rousch, J. M., Bingham, S. E., and Sommerfeld, M. R. (2003). Changes in fatty acid profiles of thermo-intolerant and thermo-tolerant marine diatoms during temperature stress. J. Exp. Mar. Biol. Ecol. 295, 145–156. doi: 10.1016/S0022-0981(03)00293-4
Ruess, L., and Müller-Navarra, D. C. (2019). Essential biomolecules in food webs. Front. Ecol. Evol. 7:269. doi: 10.3389/fevo.2019.00269
Rykaczewski, R. R., and Checkley, D. M. (2008). Influence of ocean winds on the pelagic ecosystem in upwelling regions. Proc. Natl. Acad. Sci. U.S.A. 105, 1965–1970. doi: 10.1073/pnas.0711777105
Rykaczewski, R. R., Dunne, J. P., Sydeman, W. J., García-Reyes, M., Black, B. A., and Bograd, S. J. (2015). Poleward displacement of coastal upwelling-favorable winds in the ocean’s eastern boundary currents through the 21st century. Geophys. Res. Lett. 42, 6424–6431. doi: 10.1002/2015GL064694
Sarmiento, J. L., Hughes, T. M. C., Stouffer, R. J., and Manabe, S. (1998). Simulated response of the ocean carbon cycle to anthropogenic climate warming. Nature 393, 245–249. doi: 10.1038/30455
Schoffman, H., Lis, H., Shaked, Y., and Keren, N. (2016). Iron–nutrient interactions within phytoplankton. Front. Plant Sci. 7:1223. doi: 10.3389/fpls.2016.01223
Schukat, A., Auel, H., Teuber, L., Lahajnar, N., and Hagen, W. (2014). Complex trophic interactions of calanoid copepods in the Benguela upwelling system. J. Sea Res. 85, 186–196. doi: 10.1016/j.seares.2013.04.018
Schukat, A., Hagen, W., Dorschner, S., Correa Acosta, J., Pinedo Arteaga, E. L., Ayón, P., et al. (2021). Zooplankton ecological traits maximize the trophic transfer efficiency of the Humboldt current upwelling system. Prog. Oceanogr. 193:102551. doi: 10.1016/j.pocean.2021.102551
Schulien, J. A., Peacock, M. B., Hayashi, K., Raimondi, P., and Kudela, R. M. (2017). Phytoplankton and microbial abundance and bloom dynamics in the upwelling shadow of Monterey Bay, California, from 2006 to 2013. Mar. Ecol. Prog. Ser. 572, 43–56. doi: 10.3354/meps12142
Shabangu, F., Geja, Y., Phillips, M., Merkle, D., and Coetzee, C. (2019). Pelagic Recruitment Survey. Cape Town: Department of agriculture forestry and fisheris.
Sinensky, M. (1974). Homeoviscous adaptation—a homeostatic process that regulates the viscosity of membrane lipids in Escherichia coli. Proc. Natl. Acad. Sci. U.S.A. 71, 522–525.
Standal, I. B., Rainuzzo, J., Axelson, D. E., Valdersnes, S., Julshamn, K., and Aursand, M. (2012). Classification of geographical origin by PNN analysis of fatty acid data and level of contaminants in oils from Peruvian Anchovy. J. Am. Oil Chem. Soc. 89, 1173–1182. doi: 10.1007/s11746-012-2031-0
Sydeman, W. J., García-Reyes, M., Schoeman, D. S., Rykaczewski, R. R., Thompson, S. A., Black, B. A., et al. (2014). Climate change and wind intensification in coastal upwelling ecosystems. Science 345, 77–80. doi: 10.1126/science.1251635
Tacon, A. G. J., and Metian, M. (2009). Fishing for feed or fishing for food: increasing global competition for small pelagic forage fish. Ambio 38, 294–302. doi: 10.2307/40390239
Tilstone, G., Míguez, B., Figueiras, F., and Fermín, E. (2000). Diatom dynamics in a coastal ecosystem affected by upwelling: coupling between species succession, circulation and biogeochemical processes. Mar. Ecol Prog. Ser. 205, 23–41. doi: 10.3354/meps205023
Tocher, D. R. (2015). Omega-3 long-chain polyunsaturated fatty acids and aquaculture in perspective. Aquaculture 449, 94–107. doi: 10.1016/j.aquaculture.2015.01.010
van der Lingen, C. D., Hutchings, L., Lamont, T., and Pitcher, G. C. (2016). Climate change, dinoflagellate blooms and sardine in the southern Benguela current large marine ecosystem. Environ. Dev. 17, 230–243. doi: 10.1016/j.envdev.2015.09.004
Vargas, C. A., Martínez, R. A., Cuevas, L. A., Pavez, M. A., Cartes, C., GonzÁlez, H. E., et al. (2007). The relative importance of microbial and classical food webs in a highly productive coastal upwelling area. Limnol. Oceanogr. 52, 1495–1510. doi: 10.4319/lo.2007.52.4.1495
Verheye, H. M., Hagen, W., Auel, H., Ekau, W., Loick, N., Rheenen, I., et al. (2005). Life strategies, energetics and growth characteristics of Calanoides carinatus (Copepoda) in the Angola-Benguela frontal region. Afr. J. Mar. Sci. 27, 641–651. doi: 10.2989/18142320509504124
Waldron, H. N., and Probyn, T. A. (1992). Nitrate supply and potential new production in the Benguela upwelling system. S. Afr. J. Mar. Sci. 12, 29–39. doi: 10.2989/02577619209504688
Wannicke, N., Frey, C., Law, C. S., and Voss, M. (2018). The response of the marine nitrogen cycle to ocean acidification. Glob. Chang. Biol. 24, 5031–5043. doi: 10.1111/gcb.14424
Ward, T. M., Mcleay, L. J., Dimmlich, W. F., Rogers, P. J., Mcclatchie, S., Matthews, R., et al. (2006). Pelagic ecology of a northern boundary current system: effects of upwelling on the production and distribution of sardine (Sardinops sagax), anchovy (Engraulis australis) and southern bluefin tuna (Thunnus maccoyii) in the Great Australian Bight. Fish. Oceanogr. 15, 191–207. doi: 10.1111/j.1365-2419.2006.00353.x
Keywords: omega-3, food web, small pelagic fish, nitrogen supply, coastal upwelling, plankton, climate change, biogeochemical model
Citation: Puccinelli E, Sardenne F, Pecquerie L, Fawcett SE, Machu E and Soudant P (2021) Omega-3 Pathways in Upwelling Systems: The Link to Nitrogen Supply. Front. Mar. Sci. 8:664601. doi: 10.3389/fmars.2021.664601
Received: 05 February 2021; Accepted: 04 May 2021;
Published: 04 June 2021.
Edited by:
Susana Agusti, King Abdullah University of Science and Technology, Saudi ArabiaReviewed by:
Heidi Pethybridge, Oceans and Atmosphere (CSIRO), AustraliaGleyci A. O. Moser, Rio de Janeiro State University, Brazil
Jose Juan Barrera-Alba, Federal University of São Paulo, Brazil
Copyright © 2021 Puccinelli, Sardenne, Pecquerie, Fawcett, Machu and Soudant. This is an open-access article distributed under the terms of the Creative Commons Attribution License (CC BY). The use, distribution or reproduction in other forums is permitted, provided the original author(s) and the copyright owner(s) are credited and that the original publication in this journal is cited, in accordance with accepted academic practice. No use, distribution or reproduction is permitted which does not comply with these terms.
*Correspondence: Eleonora Puccinelli, ZWxlb25vcmEucHVjY2luZWxsaUB1bml2LWJyZXN0LmZy; ZWxlb25vcmFwdWNjaW5lbGxpQGdtYWlsLmNvbQ==