- 1Marine and Environmental Biology, University of Southern California, Los Angeles, CA, United States
- 2State Key Laboratory of Marine and Environmental Science, Xiamen University, Xiamen, China
- 3Yellow Sea Fisheries Research Institute, Chinese Academy of Fishery Sciences, Qingdao, China
Understanding the environmental conditions that trigger Pseudo-nitzschia bloom formation and domoic acid (DA) production is critical as the frequency and severity of these toxic blooms increases in the face of anthropogenic change. However, predicting the formation of these harmful blooms in a future ocean remains a challenge. Previous studies have examined the effects of single environmental drivers on Pseudo-nitzschia spp. growth and toxin production, but few have considered the interactions between them. In this multiple driver study with Pseudo-nitzschia multiseries, we used a full factorial matrix experimental design to examine the simultaneous effects of temperature (20 and 25°C), nitrogen source (nitrate and urea), and irradiance (photosynthetically active radiation with and without ultraviolet B radiation; UVB). This strain of P. multiseries was unable to withstand prolonged exposures (>0.5 h) to 0.06 mw⋅cm–2 UVB light, with implications for near-surface bloom formation if future shallower mixed layers increase UVB exposure. Growth rates were inhibited by UVB, but photosynthesis and carbon fixation continued at a reduced capacity. Additionally, DA synthesis continued despite UVB-induced growth inhibition. Warming by 5°C enhanced cellular DA quotas three-fold. Within these warmer treatments, urea-grown cultures exposed to UVB had the highest amount of DA per cell, suggesting that interactive effects between UVB exposure, warming, and urea can synergistically enhance toxin production. However, overall production of toxic biomass was low, as growth-integrated DA production rates were near zero. This indicates that although Pseudo-nitzschia multiseries cell-specific toxicity could worsen in an anthropogenically-altered future ocean, bloom formation may be inhibited by increased exposure to UVB. This multi-variable experimental approach revealed previously unknown interactions that could not have been predicted based on combined effects of single-variable experiments. Although P. multiseries DA production may be enhanced in a future ocean, inherent sensitivity to prolonged UVB exposure may moderate trophic transfer of toxin to coastal food webs.
Introduction
Harmful algal blooms (HABs) threaten human health and are responsible for millions of dollars lost to fisheries and tourism every year (Anderson et al., 2000). In coastal areas around the globe, annual blooms of the diatom Pseudo-nitzschia spp. pose a serious threat to human and ecosystem health. Members of this genus produce domoic acid (DA), an amino acid-like molecule that is a potent neurotoxin that affects humans, marine mammals, and sea birds (Figure 1; Lelong et al., 2012; Trainer et al., 2012; McCabe et al., 2016). DA bioaccumulates in the food web, most notably in commercially harvested species such as shellfish, crustaceans, and finfish (Hallegraeff, 1993), which can lead to strandings and mass mortality of cetaceans, otters, and pinnipeds (McCabe et al., 2016). Human consumption of DA-contaminated seafood causes amnesic shellfish poisoning, a neurological disorder that in severe cases can cause death (Perl et al., 1990). Thus, commercial fisheries must close during toxic bloom events, leading to massive economic losses (Ritzman et al., 2018). The increasing frequency and severity of Pseudo-nitzschia spp. blooms may be linked to climate and anthropogenic change (Fu et al., 2012; Smith et al., 2018; Gobler, 2020; Trainer et al., 2020), but the direct causes of bloom formation and toxin production remain poorly understood.
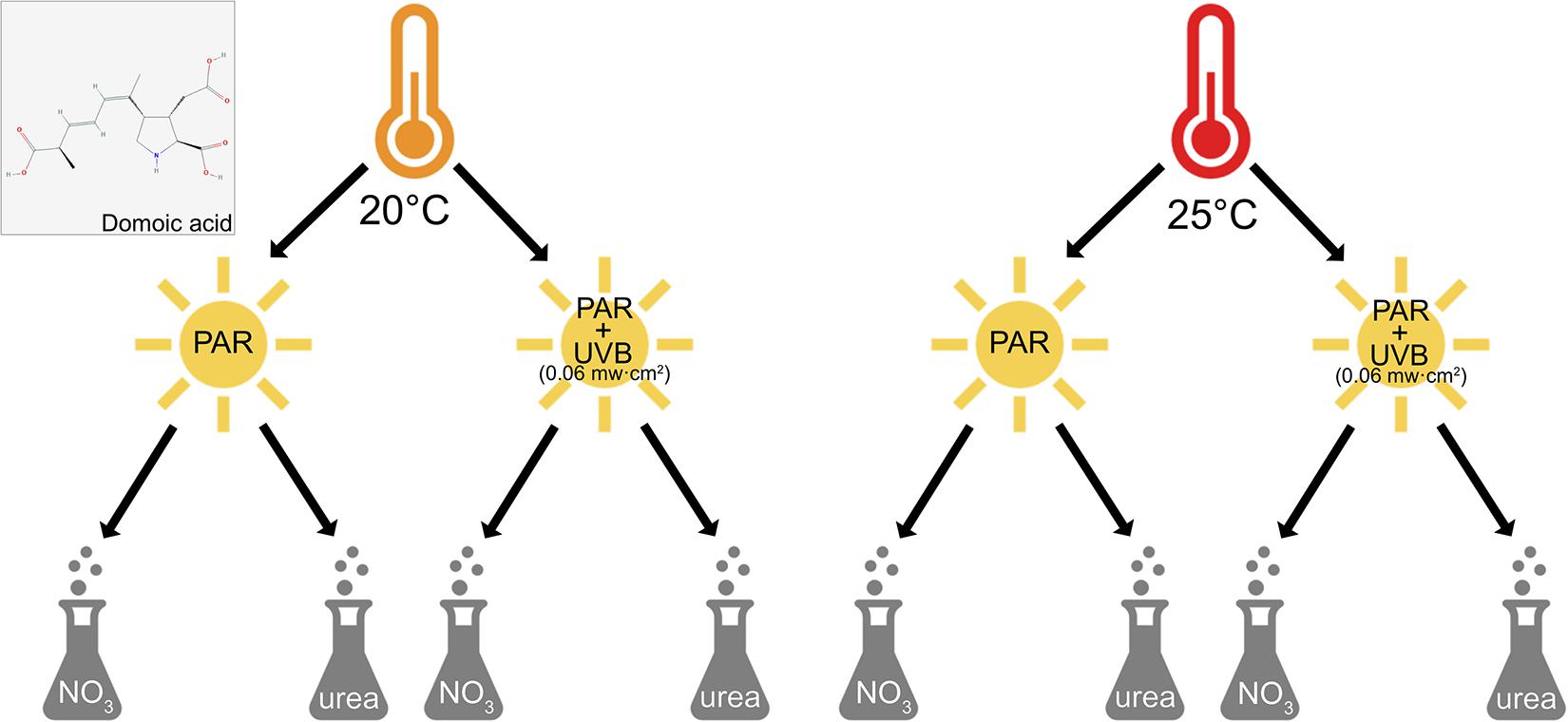
Figure 1. Experimental design and domoic acid molecule. Factorial matrix experimental design used to examine the effects of irradiance (PAR-only vs. +UVB), temperature (20 and 25°C), and nitrogen source (nitrate and urea) on the growth and toxin production of Pseudo-nitzschia multiseries. Also shown is the molecular structure of the nitrogen-rich domoic acid molecule (National Center for Biotechnology Information, 2021). The DA molecule image in this figure was obtained from PubChem.
Climate change and other anthropogenic influences are increasingly altering many aspects of coastal environments, and consequentially, the provenance and occurrence of HABs. Laboratory experiments have demonstrated relationships between DA production and individual environmental drivers. For instance, because warming is an unequivocal consequence of climate change, temperature effects on Pseudo-nitzschia spp. growth and toxicity have been well studied (Lelong et al., 2012; Trainer et al., 2012; Bates et al., 2018). A consistent trend among various Pseudo-nitzschia species is an enhancement of toxin production as temperatures warm above optimal growth temperatures (Bates et al., 1998; Zhu et al., 2017).
However, water temperature is not the only way in which the coastal ocean will be altered in the future. Warming itself modifies other key environmental variables, and in fact induces a cascade of numerous changes. Warming-enhanced stratification of the water column may cause the mixed layer to shoal, and decrease the delivery of nutrients like nitrate to phytoplankton in the surface ocean (Hutchins and Fu, 2017). At the same time, anthropogenic nitrogen sources, such as urea from agricultural runoff and wastewater effluent, are expected to become increasingly important to coastal phytoplankton. It has been suggested that the magnitude of these anthropogenic inputs could rival the natural inputs of nitrate via upwelling or mixing in some places. Additionally, because natural and anthropogenic nitrogen inputs are often separated temporally (i.e., inputs from upwelling and rainwater runoff are seasonal while wastewater effluent is more or less constant), situations may arise whereby urea is the dominant nitrogen source available to phytoplankton (Kudela et al., 2008; Auro and Cochlan, 2013; Howard et al., 2014).
Because nitrogen is required for both growth and DA biosynthesis, the effects of excess nitrogen loading (eutrophication) and chemical speciation on Pseudo-nitzschia spp. have been well documented (Glibert et al., 2005). In fact, both natural and anthropogenic inputs of nitrogen can enhance Pseudo-nitzschia spp. growth and toxicity (Heisler et al., 2008), and blooms have been associated with both upwelling and eutrophication (Lange et al., 1994; Schnetzer et al., 2013). In 2014–2015, a massive, highly toxic Pseudo-nitzschia spp. bloom occurred along the West Coast of the United States that was thought to be the result of high nutrient influxes from upwelling combined with warming (McCabe et al., 2016; McKibben et al., 2017). An accident washing >2,750 tons of urea-dominant fertilizer into Danish waters induced a highly toxic multi-species Pseudo-nitzschia bloom in 2016 (Olesen et al., 2020). Furthermore, Pseudo-nitzschia abundances have been positively correlated with high rainfall, as this runoff can deliver large amounts agricultural nitrogen to the ocean (Rees et al., 2009; Downes-Tettmar et al., 2013). However, both inter- and intra-specific differences in nitrogen preference constrain predictions of how changing nitrogen sources may influence Pseudo-nitzschia bloom dynamics. Examining growth and DA production in the context of nitrogen speciation in a strain-dependent manner thus becomes increasingly important with ongoing anthropogenic change.
Another indirect consequence of warming is that phytoplankton cells will be exposed to more intense light regimes in a shallower mixed layer, including both photosynthetically active radiation (PAR; 400–700 nm) and shallow-penetrating ultraviolet B radiation (UVB; 280–315 nm; Beardall et al., 2009). UVB radiation can cause oxidative stress and damage to nucleic acids in diatoms (Mengelt and Prézelin, 2005). Field studies have demonstrated that UV radiation can significantly impact phytoplankton mortality, leading some to suggest that UVB partially inhibits net community production in some areas of the ocean (Llabrés and Agustí, 2006; Agustí and Llabrés, 2007; Llabrés et al., 2010; Godoy et al., 2012). Studies have demonstrated mixed responses of Pseudo-nitzschia spp. to ultraviolet radiation (UVR; 280-400 nm) exposure. One experiment found that UVB caused a loss of fixed carbon in a Pseudo-nitzschia-dominated natural community (Mengelt and Prézelin, 2005); however, DA production was not measured. Another study found that UVR exposure enhanced toxin production in stationary phase batch cultures of P. pungens var. multiseries; growth under these conditions declined for P. fraudulenta and P. pungens var. pungens, yet had no effect on P. pungens var. multiseries, suggesting species-specific responses (Hargraves et al., 1993). Clearly, much remains unresolved regarding how more intense UVR exposures will affect Pseudo-nitzschia spp. growth and toxicity in future oceans.
Despite the multitude of studies identifying particular environmental variables that affect Pseudo-nitzschia growth and toxin production in the lab, the oceanic conditions that fuel blooms in the natural environment remain poorly understood. The majority of these experiments have focused on studying responses to a single factor, yet these conditions do not accurately reflect the natural coastal environment where multiple drivers interactively impact bloom dynamics. Indeed, the few studies that have examined multiple factors have often shown interactive effects between variables (Pan et al., 1998; Sun et al., 2011; Tatters et al., 2012, 2018).
The objective of the present study was to elucidate the potential interactive effects of multiple environmental change drivers on the physiology and toxicity of Pseudo-nitzschia multiseries. We used a full factorial matrix experimental design to examine the combined effects of three environmentally relevant anthropogenic and climate change variables, including temperature (20 vs. 25°C), nitrogen source (nitrate vs. urea), and light (PAR-only vs. UVB-exposed). We hypothesized that experiments examining the complex interactions between these key drivers would better reflect the dynamics occurring in natural coastal ecosystems. Our results suggest that while warming is the main individual driver of increased DA production, there are interactive effects between UVB, warming, and nitrogen source that exacerbate toxicity to a greater extent than could have been predicted by examining each variable in isolation.
Materials and Methods
Culture Conditions
Pseudo-nitzschia multiseries was isolated from Sungo Bay, Yellow Sea, China (37° 02′ N, 122° 33′ E). The ambient sea surface temperature was 19.8°C at the time of isolation. In the laboratory, cells were maintained semi-continuously on modified F/2 medium at 20°C on a 12-h light:12-h dark cycle under 130 μmol m–2 s–1 cool white fluorescent light. Modified F/2 medium (100 μM total nitrogen, 100 μM silicate, 6 μM phosphate; Guillard and Ryther, 1962; Guillard, 1975) was made using natural seawater filtered through an ultrafiltration water purifier (MUF1580-2T, Midea, China), autoclaved, and bubbled with air filtered through a 0.22 μm PVD syringe filter (Membrane Solutions, Nantong) to equilibrate with ambient atmospheric gas concentrations. Quartz culture bottles were used to allow UVB wavelengths to pass through (Zhu et al., 2020).
UVB Intensity and Dose Experiments
Preliminary experiments using in vivo fluorescence (measured with a Trilogy Laboratory Fluorometer, Turner Designs, United States) to calculate growth rates demonstrated that P. multiseries was unable to survive relatively high UVB intensities of 0.18 mw⋅cm–2 for 4 h each day over a 2-day period (Supplementary Table 2). This led us to lower the UVB intensity and analyze the dose duration-dependent responses of P. multiseries photosystem (PS) health.
To determine the duration of ultraviolet B radiation (UVB; 280–315 nm) exposure to use in our full factorial matrix experiments, effective quantum yield of PSII (Fv/Fm) was measured as a proxy for photosynthetic efficiency for P. multiseries exposed to UVB (+UVB; Philips FS20T12-UVB Broadband bulb), concurrent with PAR irradiance (>400 nm; irradiance conditions described in section “Culture Conditions”), for varying durations on a daily basis. Cultures maintained semi-continuously in the exponential growth phase were incubated at 20°C in modified F/2 medium with nitrate (see section “Culture Conditions”) under 130 μmol m–2 s–1 cool white fluorescent light and allowed to acclimate for 2 weeks (∼10 generations) until steady state growth was achieved. New medium was then inoculated at a concentration of ∼20,000 cells per liter, and triplicate quartz bottles were incubated under four different durations of 0.06 mw⋅cm–2 +UVB: 0, 0.5, 1, and 2 h each day, over a 2-day period. This regimen was chosen to simulate transitory mixing of cells to near-surface, high-UVB waters in the natural environment (Denman and Gargett, 1983; Helbling et al., 2003; Tedetti and Sempéré, 2006; Llabrés et al., 2013). Fv/Fm was measured just after inoculation (T0), before and after each UVB exposure (T3, T24, and T27), and at the time of final sampling (T48). P. multiseries was only able to tolerate short term exposures (<0.5 h) to low intensity UVB (0.06 mw⋅cm–2). We found that longer exposure periods caused Fv/Fm to decline to zero by T48, indicating complete inhibition of P. multiseries PSII activity (see section “Determining Sensitivity of Photosynthesis to UVB With Dosage Experiments”). Thus, this dosage was chosen for our full factorial matrix experiments.
Multiple Driver Experimental Design
A three-by-two full factorial matrix experimental design was used to examine all three-way combinations of temperature, nitrogen source, and irradiance, each at two treatment levels (Figure 1). The treatments for each variable were selected based on conditions reflecting contemporary oceans, and those predicted for future oceans altered by climate and anthropogenic change (Duarte, 2014; Hutchins and Fu, 2017). Nitrate treatments used concentrations of 100 μM, whereas concentrations of urea were 50 μM. Total nitrogen concentrations were thus equivalent (100 μM) across nitrogen treatments, as the urea molecule contains two nitrogen atoms.
Cultures were not acclimated to UVB in order to simulate a short-term mixing event, whereby cells residing deeper in the euphotic zone are advected closer to the surface ocean and suddenly exposed to shallow penetrating UVB light (Helbling et al., 2003). Therefore, physiological responses to UVB can be considered consequences of short-term stress rather than acclimated responses.
Cultures were acclimated and grown in their respective temperature-nitrogen source combinations using semi-continuous culturing methods, which allowed the cells to remain in the exponential growth phase. By reducing culture cell density every other day to a concentration in the early exponential growth phase, cells were allowed to grow at rates determined by acclimation to temperature and nitrogen, rather than having a growth rate imposed on them (as in continuous cultures) or growing into nutrient-starved stationary phase (as in batch cultures). Therefore, in our P. multiseries experiments near steady-state exponential growth rates, physiology, and toxicity were measured as they were influenced by temperature and nitrogen source.
Once cultures had achieved acclimated steady-state growth under experimental conditions, fresh medium was inoculated with ∼20,000 cells per liter. All treatments were incubated in 500 mL quartz experimental tubes under their respective conditions continuously for 48-h. Under PAR irradiance, +UVB treatments were exposed to 0.06 mw⋅cm–2 UVB from the UVB bulb for 0.5 h per day at midday (11:45-12:15), twice over the 2-day experimental period. This exposure regimen represents transitory vertical mixing. Incident UVB radiation was measured with a digital ultraviolet radiometer (Solarmeter). PAR-only treatments were grown under the same conditions, but on a different shelf in the same incubator. These PAR-only quartz tubes were wrapped in a UVB-absorbing Ultraphan film 395 (UV Opak, Digefra), which only allows the penetration of PAR wavelengths (400–700 nm; Cai et al., 2017; Zhu et al., 2020).
Analytical Methods
Cell Counts and Growth Rates
Cell count samples were obtained at the beginning of the experiment just after medium inoculation (T0), and again during final sampling (T48). Samples were preserved in a 10% Lugol’s solution, stored at room temperature until counted on a Nikon Eclipse Ts2-FL light microscope (NIKON, Tokyo, Japan) using a Sedgewick Rafter Chamber. Specific growth rates were determined using cell counts (only counting frustules that contained chloroplasts) and the following equation:
where μ is the specific growth rate (per day) and N is the number of cells at T0 and T48.
Elemental Analysis
To measure particulate organic carbon and nitrogen, 20-100 mL of culture was filtered on glass microfiber (GF/F) filters (pre-combusted at 500°C for 2 h), then dried for at least 48 h at 60°C in a drying oven. Dried filters were pelleted for analysis on a Costech 4010 Elemental Analyzer (Fu et al., 2007). For biogenic silica samples, 30–50 mL of culture was filtered on 0.5 μm polycarbonate filters and analyzed using an alkaline digestion and colorimetric assay (Nelson et al., 1995). To measure particulate organic phosphorus, 20–60 mL of culture was filtered on pre-combusted GF/F filters and rinsed with 0.17 M Na2SO4, placed in borosilicate scintillation vials with 2 mL of 0.017 M MgSO4 and dried at 60°C for at least 48 h (Fu et al., 2007). These were then combusted for 4 h at 500°C in order to convert the organic phosphorus to inorganic orthophosphate. Samples were then acidified in 0.2 M hydrochloric acid and analyzed colorimetrically on a spectrophotometer.
Domoic Acid
Samples for cellular toxin quotas (particulate DA) were collected at the end of the experiment (T48) by filtering 50 mL of culture onto 0.5 μm polycarbonate filters and stored at −20°C until subsequent analysis. Sample extraction and cleanup were carried out according to Wang et al. (2012) with minor modifications to the protocol. For each sample, 8 mL of 10% methanol/water (methanol:water, 1:9, v/v) was added to a 10 mL plastic conical centrifuge tube containing the filter, then homogenized by vortexing at room temperature for 10 min. Cells were disrupted by sonication using a 650 W sonic disrupter at 30% amplitude of power setting for 2 min on an ice slurry to release intracellular DA into solution. The extraction was centrifuged at 10,000 g for 5 min and then filtered with a 0.22 μm MCM syringe filter into an LC vial for liquid chromatography coupled with tandem mass spectrometry (LC-MS/MS) analysis.
Liquid chromatography coupled with tandem mass spectrometry analysis was performed using an U3000 HPLC system (Thermo Scientific, United States) linked to a Thermo TSQ Endura mass spectrometer equipped with an ESI source operated in positive ionization mode. The chromatographic system equipped with a binary pump and an autosampler with a 100 μL sample loop. To obtain maximum abundance of molecular ions and generate higher sensitivity for analyte, optimization of MS/MS parameters was necessary. Using flow injection analysis at 10 μl min–1, the mass spectrometer was tuned using the CRM-DA-f DA standard solution (1 μg mL–1; National Resource Council, Canada) and the final ion source conditions were as follows: spray voltage (kV) of +3.50, sheath gas flow 25 (arb), auxiliary gas flow 15 (arb), sweep gas flow 0 (arb), ion transfer tube temperature of 300°C, and vaporizer temperature of 250°C.
Full scan MS in the positive ion mode gave the predominant peak at [M+H]+m/z 312.0, which was selected as precursor ion. The SRM transitions from the protonated DA ion were m/z 312.0 > 266.0 ([M-HCOOH+H]+) at collision energy (CE) = 15 V used for quantitative analysis, and two confirmatory transitions of m/z 312.0 > 248.0 ([M-HCOOH–H2O+H]+) at CE = 16 V and m/z 312.0 > 161.0 ([M-HCOOH-C2H3O2N-H2O-CH2+H]+) at CE = 22 V, all with a RF lens of 142 V, a dwell time of 250 ms, and a collision induced dissociation gas setting of 1.5 mTorr. Resolution of both quadrupoles Q1 and Q3 were set at 0.7 FWHM. Chromeleon and Xcalibur software were used for the entire MS tune, instrument control, data acquisition, and data analysis. Quantitative analysis was attained by an external standard method with DA calibration solutions at a concentration of 1,000 ng ml–1. DA had a retention time of around 9.49 min. The flow was diverted to waste by a six-way valve for the 0–7.5 min and 11.5–25.0 min of each run to keep the ion source clean.
Photophysiology
The maximum quantum yield of PSII (Fv/Fm), effective quantum yield of PSII (Fv′/Fm′), non-photochemical quenching (NPQ), relative electron transport rate (rETR), and light saturation point (Ik) of live cultures was assessed using a Multi-Color-PAM Chlorophyll Fluorometer (MC-PAM; Heinz Walz GmbH, Germany; Schreiber et al., 2011) at the time of final sampling.
Fv/Fm was measured after 15 min of dark adaptation of the cultures and calculated as
Fv′/Fm′ was measured at an actinic light value close to the experimental PAR irradiance (230 μmol photons m–2 s–1) and calculated using the following the equation:
Non-photochemical quenching was calculated as:
Relative electron transport rate was calculated using the following equation:
where 0.5 is the equal distribution of photons between PSII and PSI, and 0.84, the homogeneous absorption factor, is a widely accepted absorptivity value (Bjrkman and Demmig, 1987; Ralph et al., 2005). Ik was calculated according to Jassby and Platt (1976).
Statistical Methods
Multivariate analyses were conducted in R version 4.0.21 using statistical tools in Rallfun-v382. A percentile bootstrap method for multiple comparisons of 20% trimmed means (t3way) was used to detect significant one-, two-, and three-way interactions between variables (temperature, nitrogen, and irradiance) in the three-by-two factorial matrix experiment. A percentile bootstrap method for comparing 20% trimmed means (t1way) was used to detect significant differences in final (T48) Fv/Fm values in UVB dosage experiments. Pairwise analyses using percentile bootstrapping of 20% trimmed means (trimpb2) were also conducted to test for significant differences between any two matrix treatments for growth rates, DA quotas and production rates, and photophysiology measurements. All tests were performed at the α = 0.05 level. These robust methods were chosen over classical one- and three-way ANOVA tests as they do not assume normality or homoscedasticity (Wilcox, 2003).
Results
Determining Sensitivity of Photosynthesis to UVB With Dosage Experiments
In initial short-term UVB dosage experiments, measurements of the maximum quantum yield of PSII (Fv/Fm) showed that P. multiseries PSII activity was unable to tolerate exposure to 0.06 mw⋅cm–2 UVB for >2 h per day, or any dose of UVB greater than 0.06 mw⋅cm–2 (Supplementary Tables 1, 2). The average Fv/Fm of all treatments at T0 was 0.623 (Figure 2). From T0 to T24 (following one dose of UVB), Fv/Fm declines were similar across all treatments: 44, 47.3, and 42% for 2, 1, and 0.5 h of exposure, respectively (Figure 2). Fv/Fm was significantly different between treatments at T48 (t1way, p = 0.000004), after two UVB doses. In cultures exposed for 2 or 1 h per day, Fv/Fm further decreased to 0.072 and 0.086 (respectively) after a second UVB exposure, declining by a total of 88.7 and 85.8% from T0 to T48. However, Fv/Fm of cultures exposed to UVB for 0.5 h per day only declined to 0.319, representing a 47% decline from T0 to T48 (Figure 2).
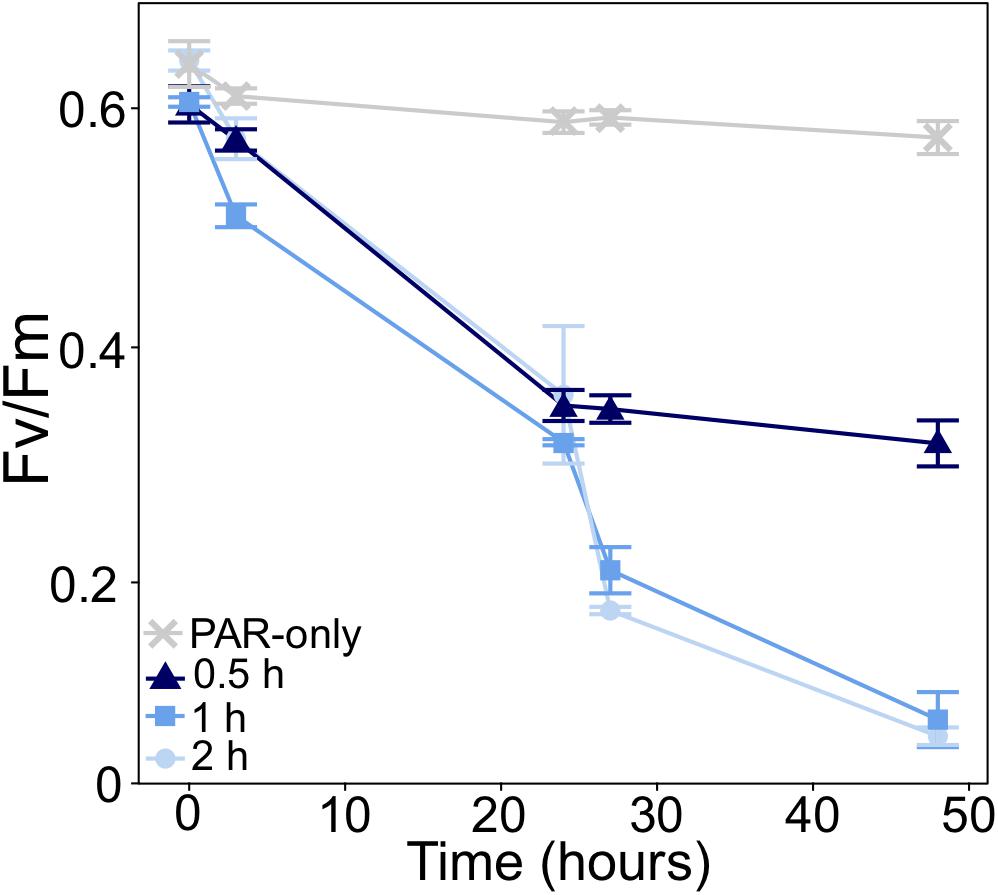
Figure 2. Change in the maximum quantum yield of PSII (Fv/Fm) of P. multiseries in UVB dose experiments. Cultures were exposed to 0.06 mw⋅cm–2 UVB for different lengths of time (0.5, 1, and 2 h). Cultures exposed to photosynthetically active radiation with no UVB (PAR-only) served as a control. UVB exposure occurred once daily (just after T0 and T24). Fv/Fm was measured at the time of inoculation (T0), before and after each UVB exposure, and at the time of final sampling. Error bars represent the standard deviation of the mean (n = 3).
Growth Rates
Spectral quality of irradiance had the greatest impact on P. multiseries growth (t3way, p = 0.0001). Cell-specific growth rates were <0.05 day–1 for all UVB-exposed (+UVB) treatments (Figure 3A and Supplementary Table 3). In contrast, a temperature-nitrogen source interaction impacting growth rates was observed for the PAR-only treatments (t3way three-way interaction, p = 0.046; Figure 3B, Supplementary Table 4). Growth rates for treatments with nitrate differed between temperatures (20 vs. 25°C), while those of cells grown with urea did not. For nitrate-supported cultures, growth rates declined from 0.58 to 0.39 day–1 (46.3% decrease) with warming from 20 to 25°C (pairwise comparison between two treatments, p < 0.001, and Supplementary Table 5). Conversely, growth rates for urea-supported cells were not significantly different at 0.51 and 0.50 day–1 for 20 and 25°C, respectively.
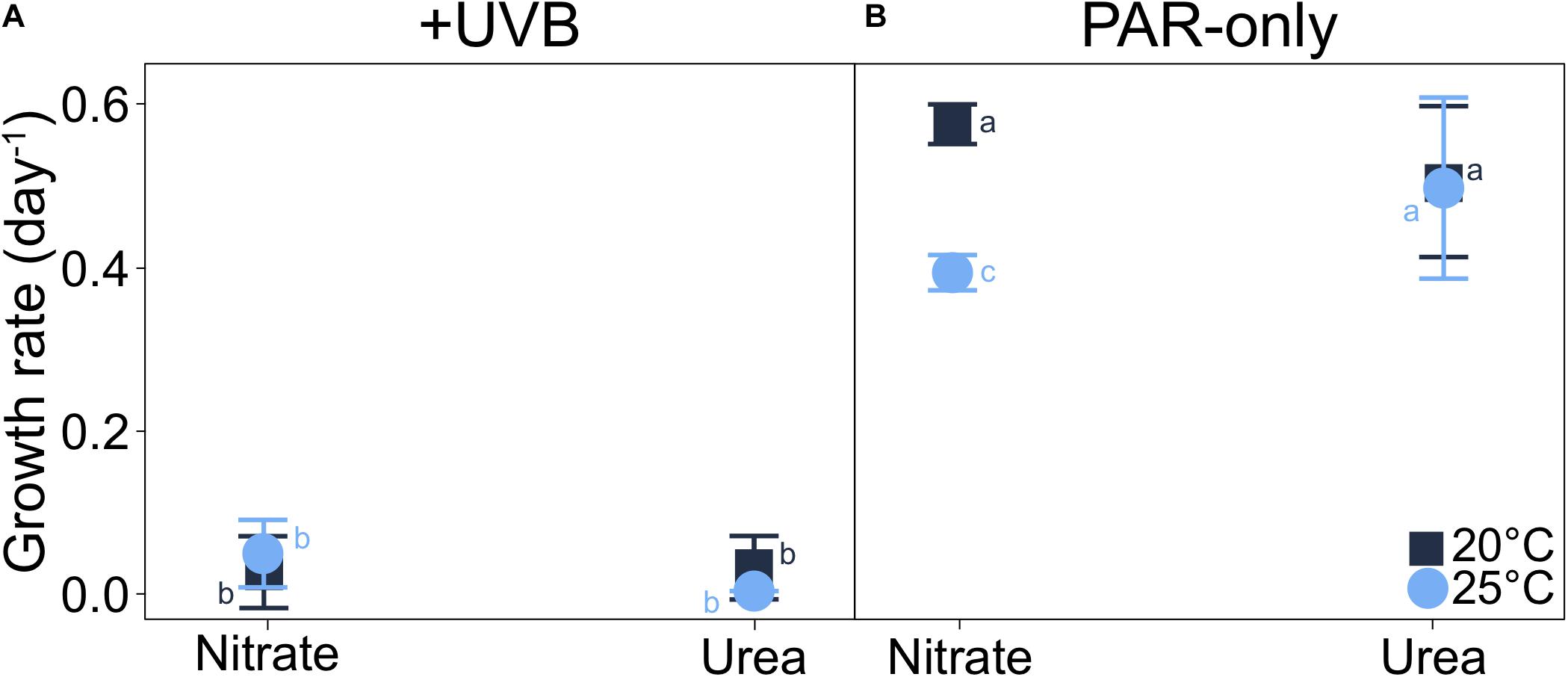
Figure 3. Interaction plots of P. multiseries cell-specific growth rates for each three-way combination of the variables examined (each at two levels of treatment) in the experimental matrix. Data are shown as interactions between temperature and nitrogen source for (A) +UVB and (B) PAR-only cultures. A significant three-way interaction was observed between temperature, light, and nitrogen source (t3way, p = 0.046). Error bars represent the standard deviation of the mean (n = 3). Letters represent statistically significant differences between treatments, based on pairwise analyses (Supplementary Table 5).
Domoic Acid
Cellular DA quotas at 25°C were on average three orders of magnitude greater than DA quotas in the 20°C incubations, regardless of nitrogen source or irradiance (Figure 4A and Supplementary Table 3). Similarly, DA production rates for PAR-only treatments were on average two orders of magnitude greater at 25°C, relative to 20°C (Figure 4B and Supplementary Table 3). Additionally, DA production was greatly reduced by UVB exposure (t3way, p = 0.0001). On average, DA production rates for all +UVB treatments were 8.1 × 10–3 pg cell–1 day–1, while maximum DA production rates, measured for 25°C PAR-only with nitrate or with urea treatments, were two orders of magnitude greater (0.22 and 0.18 pg cell–1 day–1, respectively).
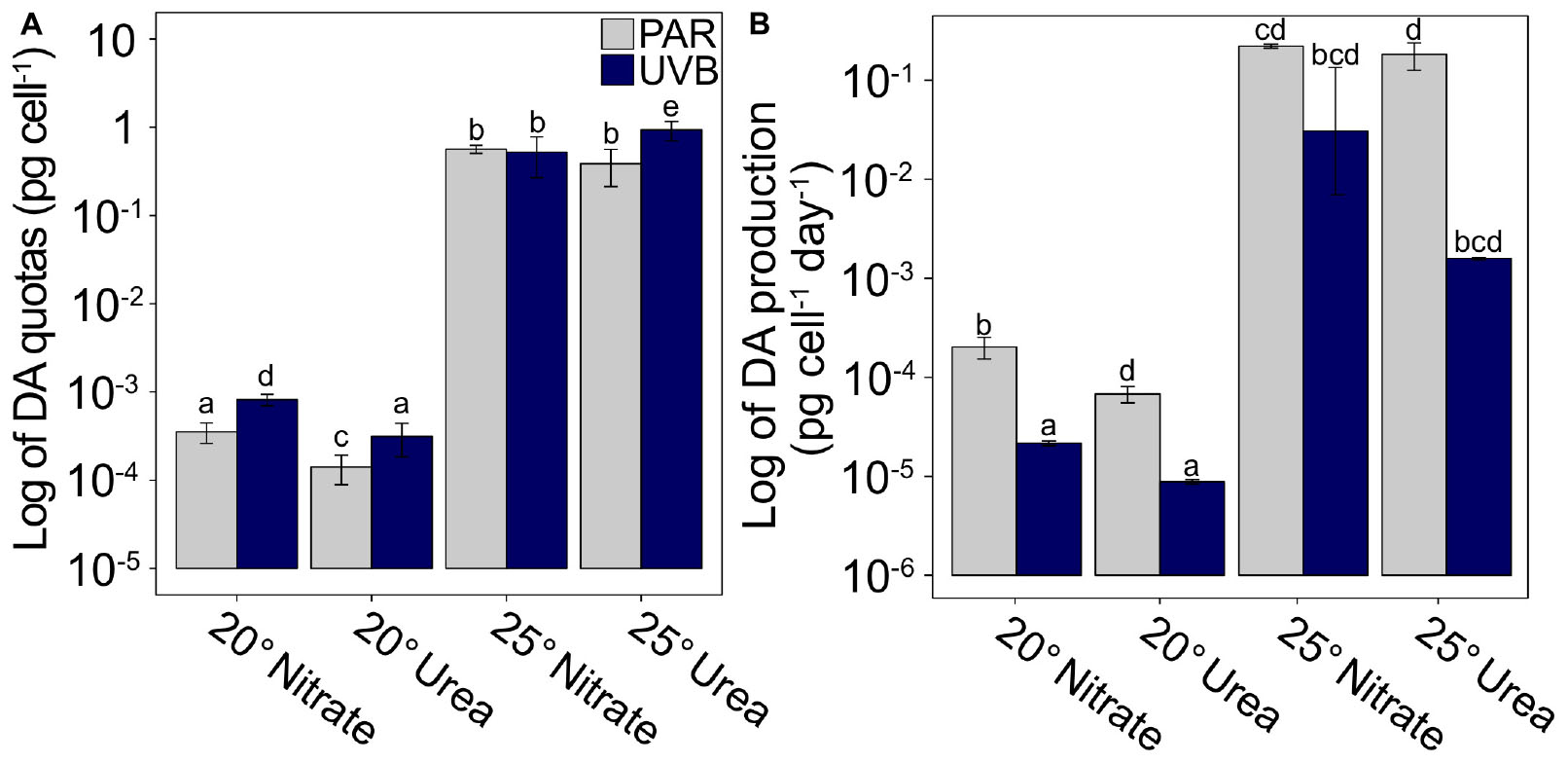
Figure 4. Cellular DA quotas and production rates plotted on a logarithmic scale. (A) DA quotas and (B) DA production rates for each matrix combination of temperature, nitrogen source, and light regime. Scaling demonstrates the large temperature effect on DA quotas and production rates. Error bars represent the standard deviation of the mean (n = 3). Letters represent statistically significant differences between treatments, based on pairwise analyses (Supplementary Table 5).
The three-way interaction between nitrogen source, temperature, and irradiance was deemed significant for DA quotas (t3way, p = 0.032; Figures 5A,B and Supplementary Table 4). Interactions between light and nitrogen source (t3way, p = 0.032) were observed at each respective temperature treatment (i.e., within 20°C treatments, and within 25°C treatments); therefore, results are presented separately for each temperature treatment in the following sections. DA quotas have been plotted as interaction plots to aid in the comparison of trends both across and within treatments, and to highlight the primary drivers of differences between treatments.
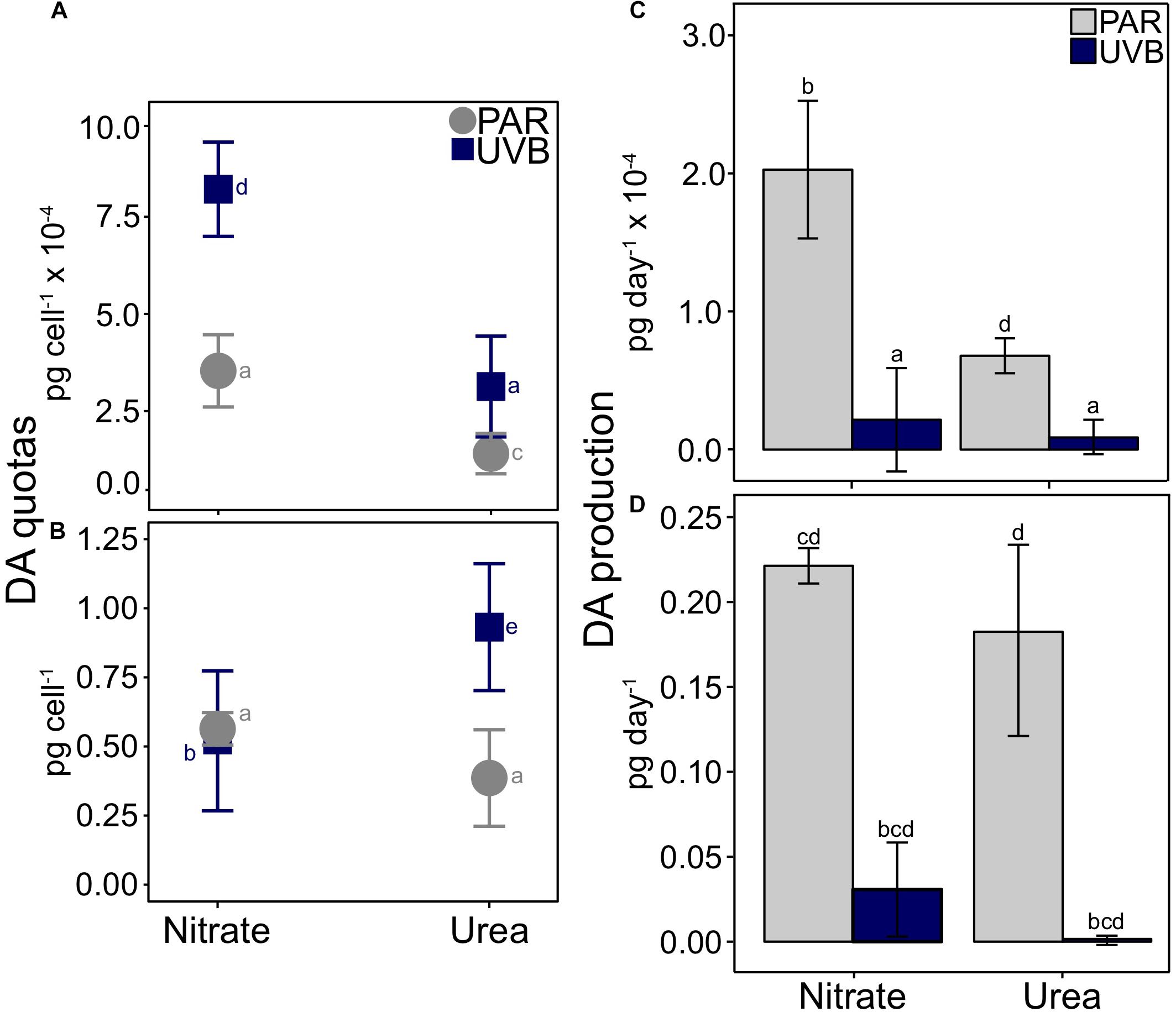
Figure 5. Cellular DA quotas and production rates for P. multiseries. Average DA quotas are shown relative to the interactions between nitrogen source and light for (A) 20°C treatments and (B) 25°C treatments. A significant three-way interaction was observed between temperature, irradiance, and nitrogen (t3way, p = 0.032). Note that scaling for y-axes differs between each plot. DA production rates are plotted with separate scales for 20°C (C) and 25°C treatments (D). Error bars represent the standard deviation of the mean (n = 3). Letters represent statistically significant differences between treatments, based on pairwise analyses (Supplementary Table 5).
Domoic Acid Quotas at 20°C
Within each light treatment, DA quotas were significantly greater with nitrate than with urea (pairwise comparison, PAR p < 0.001; UVB p = 0.001; Figure 5A and Supplementary Tables 3, 5). This difference was greater for +UVB treatments: cultures with nitrate contained 8.21 × 10–4 pg DA cell–1, while cultures with urea contained 3.13 × 10–4 pg DA cell–1. DA quotas also differed between light treatments, especially for nitrate-grown cells; DA quotas significantly increased from 3.54 × 10–4 pg DA cell–1 when grown under PAR-only, to 8.21 × 10–4 pg DA cell–1 with UVB exposure (pairwise comparison, p < 0.001). DA quotas also differed between PAR-only with urea and +UVB with urea treatments (1.41 × 10–4 and 3.13 × 10–4 pg cell–1, respectively; pairwise comparison, p = 0.001), but the magnitude of this difference was smaller compared to nitrate-grown cells (Figure 5A).
Domoic Acid Quotas at 25°C
The greatest DA quotas were observed at 25°C, relative to 20°C treatments (Figures 5A,B and Supplementary Table 3). For the two 25°C treatments with nitrate, cellular DA quotas did not significantly differ between light regimes; PAR-only with nitrate DA quotas were on average 0.56 pg cell–1, similar to those of +UVB with nitrate (0.52 pg cell–1; Figure 5B). Conversely, a large difference in cellular DA quotas was observed between PAR-only with urea and +UVB with urea treatments (pairwise comparison, p < 0.001, and Supplementary Table 5). When 25°C urea treatments were exposed to UVB, cellular DA quotas increased from 0.39 to 0.93 pg cell–1 (Figure 5B). Despite higher cellular DA quotas, DA production rates were much lower in UVB treatments than in PAR treatments at both 20°C (Figure 5C) and 25°C (Figure 5D) for both nitrogen sources, due to the greatly reduced growth rates in all treatments after UVB exposure (Figure 3).
Examining the same four treatments in the context of nitrogen source-effects within light treatments (i.e., 25°C PAR with nitrate vs. with urea, and 25°C +UVB with nitrate vs. with urea) allows for interpretation of the data from another perspective, revealing additional key differences between treatments (Figure 5B). DA quotas differed between nitrogen sources within each light treatment, both in terms of magnitudes and direction of change. Under a PAR-only light regime, DA production was 1.46 times higher with nitrate (0.56 pg cell–1) than urea (0.39 pg cell–1), but the difference was marginally not significant at p = 0.058 (pairwise comparison). In contrast, +UVB treatments produced significantly more DA with urea (0.93 pg cell–1) compared to treatments with nitrate (0.52 pg cell–1; p < 0.001).
Photophysiology
Large differences were observed between PAR-only and +UVB treatments for Fv/Fm and effective quantum yield (Fv′/Fm′; Figures 6A,B and Supplementary Table 6). At 20°C, Fv/Fm decreased with UVB exposure by 58.7% for nitrate and 52.5% for urea. For 25°C treatments with nitrate and with urea, Fv/Fm declined in +UVB treatments by 42.2 and 45.2%, respectively (Figure 6A). The magnitude of decline was greater for Fv′/Fm′: 78.8 and 71.9% for 20°C treatments with nitrate and with urea, and 74.5 and 74.5% for 25°C treatments with nitrate and with urea (Figure 6B). Furthermore, a significant temperature-nitrogen interaction was observed for both Fv/Fm and Fv′/Fm′ (t3way, p = 0.007 and p = 0.001, Supplementary Table 4). For PAR-only treatments, Fv/Fm did not significantly differ between 20 and 25°C treatments, with urea averaging 0.592 and 0.579, respectively (Figure 6A). A similar trend was observed for Fv′/Fm′ values, which were 0.496 and 0.508, respectively (Figure 6B). However, Fv/Fm and Fv′/Fm′ for PAR-only with nitrate treatments did differ between temperatures; with an increase in temperature, Fv/Fm decreased by 14.8% from 0.610 to 0.520 (Figure 6A), while Fv′/Fm′ decreased by 21.1% from 0.536 to 0.432 (Figure 6B). These temperature-dependent nitrogen source effects were not observed for +UVB treatments.
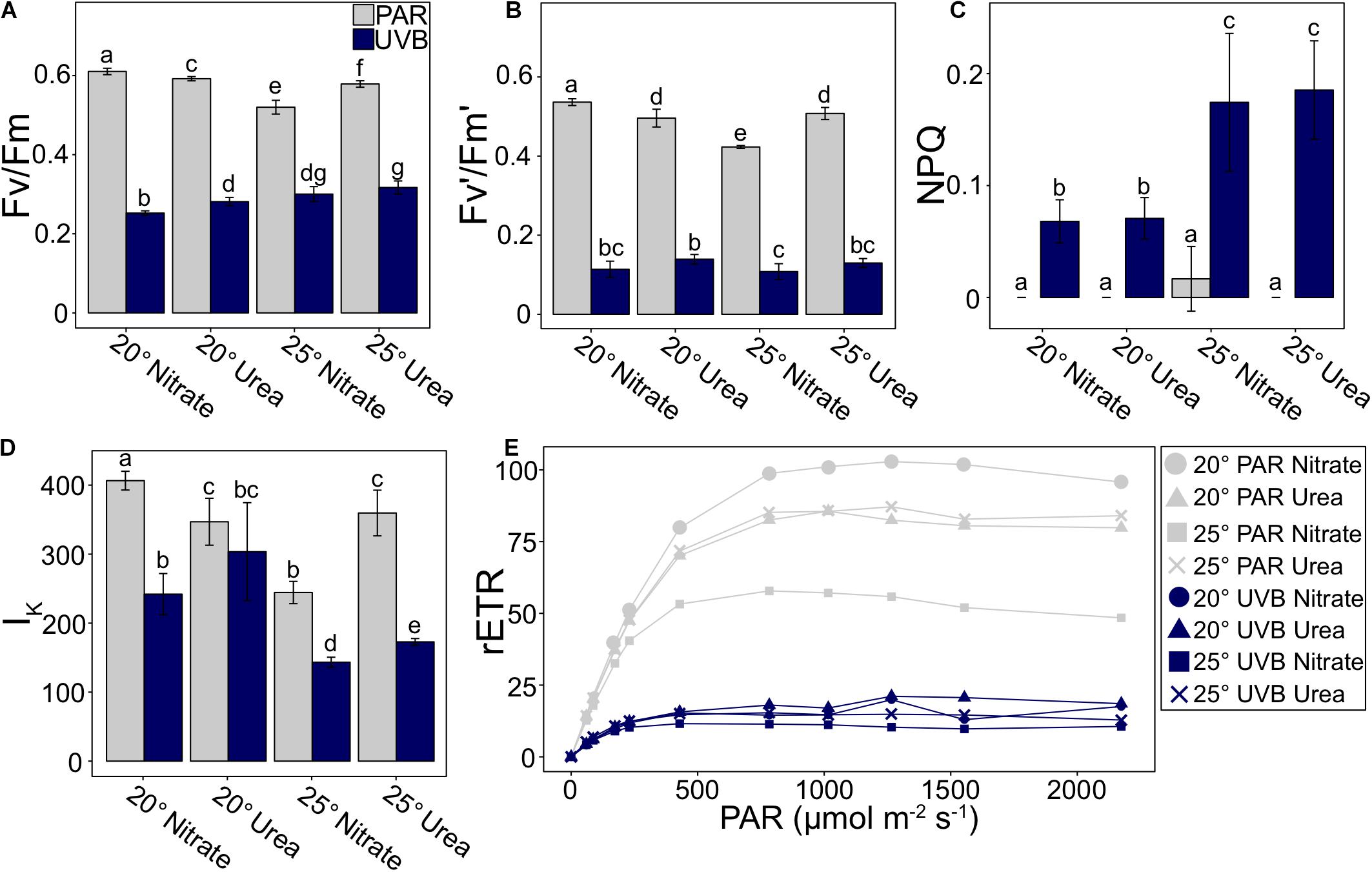
Figure 6. P. multiseries photophysiological parameters measured at the time of final sampling, including (A) maximum quantum yield of photosystem II (Fv/Fm), (B) effective quantum yield of PSII (Fv′/Fm′), (C) non-photochemical quenching (NPQ), (D) light saturation point (IK), and (E) relative electron transport rate (rETR) vs. PAR irradiance curve. Error bars represent the standard deviation of the mean (n = 3). Letters represent statistically significant differences between treatments, based on pairwise analyses (Supplementary Table 5).
Non-photochemical quenching was 0 for all PAR-only treatments, except for a very low value of 0.017 at 25°C with nitrate (Figure 6C and Supplementary Table 6). The temperature-light interaction was deemed significant at the p = 0.003 level (t3way). For +UVB treatments, NPQ values were higher at 25°C with both nitrate and with urea (0.174 and 0.185, respectively) relative to 20°C treatments (0.068 and 0.071; Figure 6C). Differences between nitrogen treatments were not significant within each temperature.
Measured differences in the irradiance at the light saturation point (Ik) were mainly driven by light (t3way, p = 0.0001; Figure 6D, Supplementary Tables 4, 6). Decreases were observed from PAR-only to +UVB treatments at 20°C with nitrate (40.5%), 25°C with nitrate (41.4%), and 25°C with urea (51.9%), but 20°C with urea only decreased by 12.5%. Furthermore, trends within PAR-only treatments were under different controls than UVB-exposed treatments. For PAR-only, urea treatments did not vary between temperatures, while the Ik for nitrate treatments decreased with an increase in temperature. In contrast, within +UVB treatments, measured Ik values were greater at 20°C relative to 25°C within each nitrogen source treatment. Additionally, at each respective temperature, Ik was greater for treatments with urea compared to treatments with nitrate (Figure 6D).
Furthermore, maximum relative electron transport rates (rETRmax) for +UVB treatments were on average 5.37x lower than PAR-only treatments (Figure 6E and Supplementary Table 6). Differences between UVB treatments were not large in magnitude. In contrast, rETRmax for PAR-only treatments were dependent on interactions between nitrogen source and temperature (t3way, p = 0.001, Supplementary Table 4); urea treatments were similar between temperatures, yet there was an inverse relationship between temperature and rETRmax for nitrate treatments (Figure 6E).
Elemental Ratios and Quotas
The amount of carbon per cell (cellular carbon quota) significantly increased with UVB exposure (t3way, p = 0.0003; Table 1 and Supplementary Tables 4, 7). This increase was observed to a greater extent for 25°C treatments; relative to PAR-only, +UVB increased carbon quotas by 30 and 50% at 25°C with nitrate and 25°C with urea treatments, respectively. This +UVB effect was not observed for 20°C treatments. Similar light effects were observed for cellular nitrogen quotas (Table 1 and Supplementary Table 7). Smaller cellular nitrogen pools were measured at 20°C and were little influenced by UVB-exposure, yet at 25°C UVB-exposure increased nitrogen quotas relative to PAR-only treatments by 27.5 and 46.7% for both nitrate and urea treatments, respectively (Table 1 and Supplementary Table 7). However, while temperature alone did not significantly influence cellular carbon pools, it did influence nitrogen pools, demonstrated by increased nitrogen per cell for 25°C treatments (t3way, p = 0.012).
Cellular phosphorus quotas were most influenced by irradiance (t3way, p = 0.002, and Supplementary Table 4), as each paired treatment showed a percent increase of at least 30% with UVB-exposure (Table 1 and Supplementary Table 7). Warming also influenced differences in measured cellular phosphorus quotas (t3way, p = 0.007). The smallest cellular phosphorus pools were measured for 20°C PAR-only with nitrate (5.17 × 10–8 μmol P cell–1), while the largest pools were an order of magnitude greater for 25°C +UVB with urea treatments (1.18 × 10–7 μmol P cell–1). Change in nitrogen source did not result in significant differences in phosphorus quotas between treatments (Table 1).
Temperature significantly increased biogenic silica (BSi) quotas (t3way, p = 0.014, Supplementary Table 4) for all treatments except 25°C PAR-only with nitrate (Table 1 and Supplementary Table 7). However, irradiance effects were marginally insignificant at the p = 0.054 level, and interactive effects between nitrogen and temperature were not significant. Cellular BSi quotas at 20°C were not significantly impacted by nitrogen source (Table 1).
C:N and C:P ratios were significantly impacted by temperature (t3way, p = 0.016 and p = 0.012, Supplementary Table 4). The largest C:N ratio was measured in the 25°C PAR with nitrate treatment, while the smallest was observed for 20°C UVB with urea (Table 1 and Supplementary Table 7). All C:P ratios measured for 20°C treatments were significantly above the Redfield Ratio, while 25°C treatments were below (with the exception 25°C +UVB with nitrate). Nitrogen, irradiance, and interactive effects on C:P were not deemed statistically significant. Temperature effects on BSi:C ratios were marginally insignificant at the p = 0.056 level, with irradiance being a more significant driver of differences between treatments (t3way, p = 0.026). PAR-only treatments had larger BSi:C ratios than their paired UVB-exposed treatments, except for 25°C with nitrate. No significant differences in N:P ratios were detected between treatments (Table 1 and Supplementary Table 7).
Discussion
Dependency of Growth Under PAR-Only Conditions on Temperature-Nitrogen Interactions
Our multiple-driver experimental design revealed previously unknown interactions between temperature, nitrogen, and UVB in this HAB species. P. multiseries growth rates under PAR-only conditions were moderately affected by nitrogen source in a temperature-dependent manner. Growth rates for nitrate treatments declined with warming, while growth rates for cultures with urea did not differ between temperatures. Previous studies examining the effects of nitrogen source on Pseudo-nitzschia spp. growth rates have demonstrated a great degree of variability between both species and strains. Growth rates measured for two strains of P. multiseries were slower for urea-supported cultures relative to nitrate, while growth rates for another strain did not differ between nitrogen treatments. For some strains of P. calliantha and P. fraudulenta, growth rates decreased in urea-supported cultures compared to nitrate and ammonium. In contrast, no difference between nitrogen substrates was observed for P. australis (Thessen et al., 2009; Martin-Jézéquel et al., 2015). Based on a metanalysis of nitrogen source-effects on growth rates for several species of Pseudo-nitzschia, Radan and Cochlan (2018) hypothesized that differences in culture conditions (PAR irradiance, temperature, and nitrogen concentration) partially contributed to differences in growth rates. This demonstrates that interactive effects between variables are important to consider. Nevertheless, there is a great deal of both intra- and inter-specific variability in terms of Pseudo-nitzschia nitrogen source preference (Thessen et al., 2009; Auro and Cochlan, 2013). The mechanisms driving P. multiseries nitrogen source preference and utilization remain unclear, yet it is evident that this interactive effect on nitrogen utilization has implications for bloom formation and photosynthesis in future oceans with altered nitrogen source availability.
Climate and anthropogenic change may modify the inorganic nitrogen species available to phytoplankton in future oceans. In contemporary coastal oceans, primary productivity is primarily supported by naturally-supplied nitrate delivered to the surface ocean via mixing and upwelling (Dugdale and Goering, 1967). However, future warming-induced stratification of the water column may reduce the intensity of upwelling and mixing, thereby decreasing the delivery of nitrate to the surface ocean (Hutchins and Fu, 2017). Additionally, the supply of nitrogen from anthropogenic sources (e.g., urea from agricultural runoff and wastewater effluent) is expected to increase as global fertilizer use and human populations in coastal areas expand (Glibert et al., 2006). Anthropogenic inputs of alternative nitrogen species may eventually rival or exceed natural inputs of nitrate, becoming the dominant species of nitrogen available to phytoplankton in coastal areas (Howard et al., 2014). Because of the importance of nitrogen to growth, photosynthesis, and toxin biosynthesis, and the previously documented impacts of nitrogen species on Pseudo-nitzschia toxin biosynthesis (Howard et al., 2007; Thessen et al., 2009; Auro and Cochlan, 2013; Martin-Jézéquel et al., 2015; Radan and Cochlan, 2018), the effects of this predicted eutrophication and changing nitrogen speciation on Pseudo-nitzschia spp. may have important implications for HAB modeling and forecasting (Glibert et al., 2005).
Despite potential changes to nitrogen speciation and availability in coastal zones, our data suggest that P. multiseries grows at the same rate on both urea and nitrate at temperatures representative of a future, warmer ocean (25°C). Temperature and nitrogen also interacted to impact photosynthetic parameters like Fv/Fm, Fv′/Fm′, Ik, and rETRmax in a similar manner; measurements for nitrate-supported treatments decreased with warming, while temperature had negligible effects on the photophysiology of urea-supported cells. Therefore, at warmer temperatures (under PAR-only conditions), P. multiseries blooms and primary productivity may not be strongly influenced by shifting nitrogen sources. This aligns with previous evidence demonstrating that diatom nitrogen metabolism is highly flexible, enabling cells to alter uptake and assimilation mechanisms quickly and efficiently when other nitrogen sources become available (Smith et al., 2019). However, in the present study nitrate-supported growth rates at 25°C were slightly lower than maximum observed rates at 20°C for PAR-only treatments. This suggests that future warming may incrementally reduce P. multiseries growth, with implications for bloom development and primary productivity relative to contemporary nitrate-dominated systems. However, the trends just described are only applicable to PAR-only treatments. Therefore, these results are most relevant to populations of cells residing deeper in the water column where UVB is largely attenuated (see below).
P. multiseries Growth Under UVB Exposure
Despite the differential effects alternative nitrogen sources and warming may have on P. multiseries growth rates, increased UVB exposure in a future surface ocean may have an overpowering influence on bloom formation. Inhibition of cellular division across all treatments exposed to 0.06 mw⋅cm–2 UVB demonstrates that the growth of this strain of P. multiseries is strikingly sensitive to UVB. This dose of UVB is similar to near-surface measurements in the natural environment. For example, summertime UVB radiation measured just below the surface in the coastal Red Sea was 0.084 mw⋅cm–2 (Al-Aidaroos et al., 2014). Other studies examining the impact of UVR on diatoms have also used UVB doses similar to or lower than those measured at the air-sea interface, reasoning that these lower irradiances are more reflective of the light regimes present at the depths to which phytoplankton are mixed (Nilawati et al., 1997; Liang et al., 2006; Helbling et al., 2011).
Many studies have demonstrated the sensitivity of phytoplankton to UVB, causing declines in both growth and photosynthesis (Cullen and Lesser, 1991; Helbling et al., 1992; Smith et al., 1992; Neale et al., 1994; Nilawati et al., 1997; Gao et al., 2007a, b; Guan and Gao, 2010; Li Y. et al., 2012; Cai et al., 2017; Zhang et al., 2019). However, only a handful of studies have specifically examined Pseudo-nitzschia sp. in this regard. For instance, UVB exposure of polar P. seriata caused growth rates to decline by 50% and Fv/Fm to decline by 27% (Nilawati et al., 1997). UVR contributed to inhibition of both growth and Fv′/Fm′ in P. pungens, with warming playing a role in alleviating the severity of inhibition (Chen et al., 2018). Hargraves et al. (1993) reported declines in P. fraudulenta and P. pungens var. pungens growth rates with exposure to either artificial or natural UV light, respectively, yet growth was not fully inhibited. On the other hand, P. pungens var. multiseries growth was unaffected by either type of UVR exposure. Furthermore, field studies found that in a mixed diatom community dominated by P. australis, loss of fixed carbon was greater in treatments exposed to UVB (Mengelt and Prézelin, 2005). Therefore, it is clear that UVB alone can impose controls on phytoplankton growth and photosynthesis, with implications for Pseudo-nitzschia blooms in the natural environment.
We propose that the co-occurrence of UVB inhibition of growth and increased toxicity for some treatments is a situation similar to growth limitation by low silicate or phosphate availability. Growth rates are typically inversely correlated with DA production, since as cellular division decreases due to nutrient limitation or other growth-limiting stressors, DA production increases (Bates et al., 1991; Zhu et al., 2017). Because DA is a secondary metabolite, only produced when there is enough energy, this inverse correlation is hypothesized to be a function of re-allocation of energy resources within the cell (Pan et al., 1998). This has previously been studied in the context of growth limitation by silicate and phosphate. These studies have shown that growth inhibition by macronutrient limitation can enhance cellular toxin quotas (Bates et al., 1991, 1996; Pan et al., 1996; Fehling et al., 2004; Sun et al., 2011; Tatters et al., 2012). In a chemostat culture experiment using silicate limitation to control P. multiseries growth rates, DA quotas were inversely correlated with growth rates (Bates et al., 1996). The authors concluded that DA was “produced during conditions of stress” as part of secondary metabolism occurring when cellular division is not the main energy sink, or when there is sufficient energy for growth. Therefore, when cellular division is slowed or inhibited by macronutrient limitation, unused energy is reallocated toward toxin production, increasing cellular DA quotas. This is similar to our growth-inhibited UVB exposed cultures. UVB-exposure stressed cellular growth rates and, in some treatments, enhanced cell-specific toxicity. We hypothesize that these observed increases in toxicity could therefore be a stress response to UVB-limited growth, whereby DA production is a sink for energy not being used for growth.
Other research suggests that ultraviolet A radiation (UVA; 320–400 nm) wavelengths can help to mitigate cellular UVB damage (Quesada et al., 1995) by stimulating photo-repair mechanisms (DNA repair and antioxidant mechanisms; Mitchell and Karentz, 1993), the synthesis of photoprotective pigments (mycosporine-like amino acids; Lesser, 1996; Xu and Gao, 2010), and increased photosynthetic carbon fixation (Mengelt and Prézelin, 2005; Gao et al., 2007a; Li et al., 2011). Our study did not incorporate UVA wavelengths, but we hypothesize that the inclusion of this portion of the light spectrum in our experimental design may have decreased the sensitivity of P. multiseries to damaging UVB rays. Therefore, our measurements may represent a maximum estimate of damaging UVB-related effects.
Furthermore, in the natural environment, self-shading can occur in dense phytoplankton blooms, reducing effective light exposure and decreasing oxidative stress within the population (Barros et al., 2003). Self-shading thus acts as a photoprotection mechanism for in situ blooms. However, our use of semi-continuous culture techniques maintained cultures at low cell abundances, such that photoprotection from harmful UVB irradiance as a result of self-shading was minimal. Accordingly, our estimates of UVB-impacts on P. multiseries physiology and toxicity represent an upper estimate. Our results nevertheless suggest that UVB could be an important control on P. multiseries growth in the natural environment. Future studies using full spectrum natural sunlight at a range of intensities are needed to validate our findings and examine the potential synergistic or antagonistic effects of both UVA and UVB.
The extent to which UVB impacts P. multiseries in the natural environment will depend on changing physical oceanography. Projected ocean warming will intensify stratification of surface waters and cause the mixed layer to shoal, increasing the residence time of cells in the near-surface ocean. Consequentially, light exposure integrated over depth will increase, exposing cells to more intense PAR and especially to additional shallow penetrating UVB rays (Beardall et al., 2009; Gonçalves et al., 2010; Steinacher et al., 2010; Gao et al., 2019). Therefore, the inhibition of growth by UVB exposure observed in the present study suggests that the ability of P. multiseries to form near-surface blooms in a future ocean may be inhibited by increases in UVB exposure and intensity.
Cells Continued to Function Despite Inhibition of Growth and Damage to Photosystems
Pseudo-nitzschia multiseries photosystem health was also impaired by UVB but was less sensitive compared to cellular division. Fv/Fm, Fv′/Fm′, Ik, and rETRmax decreased with UVB exposure relative to PAR-only treatments, indicating stress, damage to photosynthetic proteins, and a diminished capacity of photosystems to absorb and process light energy. In another study, the marine cyanobacterium Trichodesmium exhibited sharp declines in Fv′/Fm′ just 10 min after initial exposure to UVB, concurrent with measured reductions in carbon fixation rates (Cai et al., 2017). Similarly, sharp declines in Fv/Fm soon after UVB exposure were observed in the UVB-dosage experiments of the present study (section “Determining Sensitivity of Photosynthesis to UVB With Dosage Experiments”) in addition to the decreases in Fv/Fm and Fv′/Fm′ for all UVB treatments relative to PAR-only in the matrix experiments. This suggests that UVB damage to P. multiseries photosystems may have also reduced, but not fully inhibited, carbon fixation rates. Furthermore, NPQ increased with UVB exposure, suggesting diversion of a greater proportion of energy away from damaged reaction centers that are unable to handle the amount of incoming light energy. NPQ increased to a greater degree for 25°C treatments, indicating that cells increased photosynthetic stress-responses as a result of warming. Although +UVB impaired photosynthetic processes, photosystem function was not fully inhibited. Therefore, despite the suppression of cellular division, cells were able to survive and continue photosynthesis under UVB stress. This allows P. multiseries to obtain energy for cellular functions such as carbon fixation, nutrient uptake, and DA synthesis, as well as stress mitigation and repair.
Increases in cellular elemental quotas with UVB exposure and warming provide additional evidence for continued carbon fixation and nutrient uptake in stressed cells. Carbon per cell increased with UVB exposure for 25°C treatments. This effect was not observed at 20°C, indicating an interactive effect whereby UVB exposure magnifies the response of cells previously stressed by warming. Similarly, nitrogen and silica cell quotas were higher for 25°C UVB-exposed treatments, relative to the respective PAR-only treatments, though silica differences were marginal for the 25°C urea-supported treatments. Phosphorus quotas increased with UVB exposure at both temperatures, but the increase at 25°C was greater. These increased carbon, nitrogen, silica, and phosphorus pools suggest that both photosynthesis and nutrient uptake mechanisms continued to function in stressed cells. Because cellular division was inhibited by UVB, the fixed carbon and nutrients taken up by cells could not be used for growth related to cellular division. In other diatoms, cellular nutrient storage pools have increased when growth was inhibited by other stressors such as phosphate, silicate, nitrogen, and iron limitation (Kilham et al., 1977; Hutchins and Bruland, 1998; Tantanasarit et al., 2013; Liefer et al., 2019). This nutrient storage capacity has implications for growth resumption and potential bloom formation upon relief and recovery from stress.
The continued survival and functioning of cells under stress raises questions about post-stress recovery. Depending on the severity and duration of exposure to UVB, it is possible that P. multiseries may be able to recover and resume normal functioning. Studies have shown that cells can recover from UVB-caused photosynthetic inhibition once the stressor has been removed (Villafañe et al., 2007; Roncarati et al., 2008; Halac et al., 2009; Helbling et al., 2011; Cai et al., 2017). Chen et al. (2018) demonstrated that recovery rates of P. pungens exposed to UVR were enhanced with warming. We hypothesize that the kinetics of recovery may play an important role in bloom formation for P. multiseries residing in environments with dynamic light regimes. Damage from short-term vertical mixing of P. multiseries to the surface ocean may be mitigated by subsequent mixing back down to depth, which provides stressed cells respite from shallow penetrating UVB rays and time to repair damage and recover. Reduced PAR-irradiances are also beneficial to recovery from photoinhibition (Helbling et al., 2011). Previous research suggests that shorter mixing events are not as inhibitory to phytoplankton community carbon fixation, as these short periods of exposure are not terribly damaging to photosystems (Helbling et al., 2003). However, future shoaling of the mixed layer may prevent mixing to depths at which UVB wavelengths are completely attenuated, reducing the ability of cells to recover. This cellular recovery aspect was not investigated in the present study, and thus further research with long-term tracking of post-stress growth and photophysiology is needed to draw further conclusions.
Multiple Stressor Effects on P. multiseries Toxicity
Temperature was the main driver of differences in cellular DA toxin quotas between treatments, regardless of nitrogen source or light spectrum. Although there were significant differences between 20°C nutrient and irradiance treatments, they were small. Therefore, any interactive effects occurring at 20°C are unlikely to have a great influence on the severity of a toxic bloom. On average, DA quotas were three orders of magnitude greater for 25°C treatments compared to 20°C incubations. This suggests that during current heatwave events or in a future consistently warmer ocean, P. multiseries toxicity per cell will increase. Previous laboratory studies have also demonstrated that warming above optimal growth temperatures can enhance toxin production in several Pseudo-nitzschia species (Bates et al., 1998; Zhu et al., 2017). Field-based observational studies have also positively correlated temperature and DA (Downes-Tettmar et al., 2013; McKibben et al., 2015). In contrast, there has been one documented occurrence of a negative correlation between DA quotas and temperature, whereby greater DA quotas were measured in P. seriata incubated at 4°C compared to 15°C (Lundholm et al., 1994). This demonstrates heterogeneity in warming responses of the Pseudo-nitzschia genus, indicating that more species should be studied with regard to temperature effects. This becomes problematic as global ocean mean temperatures rise, and the frequency of marine heat waves increases (Oliver et al., 2018). The effects of warm anomalies are already being felt in coastal ecosystems; the extensive and highly toxic 2014–2015 “Blob” bloom occurred when an anomalously warm water mass developed off the West Coast of North America (McCabe et al., 2016; McKibben et al., 2017). More frequent events such as these could have devastating impacts for coastal ecosystems. Adding another layer of complexity, within the 25°C treatments, DA quotas appear to be related to nitrogen source and irradiance. This implies that although temperature was the main driver of increased toxicity, it likely cannot be the sole parameter used to draw accurate conclusions about the threat of toxic bloom events in warmer coastal oceans.
Nitrogen source and UVB interacted with warming to influence DA quotas. The most DA was measured in the 25°C +UVB treatment with urea. In addition to warming stress, UVB also caused an increase in DA quotas for most treatments, relative to respective PAR-only treatments. However, the magnitude of this increase between PAR-only and +UVB treatments was temperature- and nitrogen source-dependent. DA biosynthesis and cellular division, both energetically demanding processes, tend to be decoupled: as cells become thermally-stressed, growth rates decline and DA production increases (Pan et al., 1998). This is hypothesized to be caused by a reallocation of cellular energy and resources within the cell away from growth and toward DA biosynthesis (Lelong et al., 2012; Trainer et al., 2012; Bates et al., 2018). Previous studies have attributed this increased DA to growth suppression by nutrient limitation (Si, P, and Fe) or warming stress (Maldonado et al., 2002; Sun et al., 2011; Tatters et al., 2012; Zhu et al., 2017). Our study suggests that UVB, which also suppresses P. multiseries growth, triggers increased DA production. Few studies have examined the impacts of UVB on Pseudo-nitzschia spp., and to our knowledge only one has also measured DA. Hargraves et al. (1993) found that P. pungens var. multiseries growth was unaffected by UV exposure using natural sunlight (representative of a 1.5 m depth in a coastal area), but DA quotas were greater in treatments without UVB exposure measured in stationary phase batch cultures. Differences between results from Hargraves et al. and our own study may lie in the severity of UVB exposure and effects on growth. While UVB did not affect growth rates in the other study, we hypothesize that the marked UVB inhibition of growth contributed to greater DA yields in our study.
Light spectral quality strongly influenced calculated DA production rates. The calculation for DA production integrates growth rates and DA quotas, assuming DA production is proportional to growth. This provides us with an estimate of how much DA could be produced by a bloom, based on both the toxicity per cell and the rate at which this toxin-containing cellular biomass accumulates. P. multiseries was not able to grow with UVB exposure, so under this assumption the calculated DA production was also zero. Although this was true for two of the temperature-nitrogen pairings (20°C with urea and 25°C with nitrate), as DA quotas were equal between PAR and +UVB light treatments, DA quotas were greater with UVB relative to PAR-only for the other two temperature-nitrogen combinations (20°C with nitrate and 25°C with urea). This contradicts the assumption of the DA production rate calculation, whereby cells must be growing in order to produce DA. Therefore, calculated production rates can be somewhat deceptive because of this growth rate effect. In the present study, P. multiseries cells were able to continue photosynthesis and the uptake of nutrients. This excess energy and nitrogen not being used for growth may instead be used in other secondary metabolic processes, such as DA production. Some studies have found an inverse relationship between Pseudo-nitzschia DA production and growth rates. As cells become stressed for growth, DA production increases (Lelong et al., 2012; Trainer et al., 2012; Bates et al., 2018).
Our results suggest that although UVB treatments had near-zero growth rates, it is likely that the surviving cells were producing DA. In spite of this increased cell-specific toxicity, the amount of DA produced over time is what determines the ultimate accumulation of toxic biomass in a bloom. Although cells in UVB-exposed 20°C treatments with nitrate and 25°C treatments with urea were more toxic relative to their PAR counterparts, the inhibition of growth due to UVB prevents rapid biomass accumulation. Therefore, a toxic bloom is not likely to manifest under these conditions.
Because we did not measure dissolved DA (i.e., the fraction released into the medium), it is important to consider that our estimates of DA production may be low. Godinho et al. (2018) found that in P. multiseries batch cultures, 63-98% of total measured DA was released into culture medium during exponential growth, and 99% was released during stationary phase. They hypothesized that cell death and lysis beginning in late exponential phase, and increasing during stationary phase, was the cause of increased dissolved DA. In the present semi-continuous experiment, final sampling occurred during steady state exponential growth phase for PAR-only cultures; dissolved DA can account for as little as 10% of the total under these growth conditions (Sun et al., 2011). However, growth was arrested in UVB-exposed cultures, and it is likely that many cells died due to exposure to this stressor, as indicated by empty (yet intact) frustules observed under the microscope. It is therefore possible that our estimates including only particulate DA may represent a minimum estimate of total DA produced, especially in UVB treatments. Most published studies have focused on particulate DA, because this is the form usually assumed to be involved in trophic transfer and biomagnification of the toxin. Nonetheless, future studies should measure both fractions, as extracellular DA could be important to consider in UVB-induced cell mortality.
Interactions Between Irradiance and Nitrogen Source May Impact Cellular Energetics
Our study also showed that UVB reduces photosystem function, which may limit light harvesting capabilities. Light energy plays an important role in nitrogen uptake; when PAR irradiance is limiting, the uptake of urea, a reduced form of nitrogen, is less energetically expensive relative to nitrate (Legrand et al., 1998; Li J. et al., 2012). This interaction between nitrogen source and light, and its impacts on cellular energetics, has been demonstrated in P. cuspidata using PAR irradiance. In this study, nitrogen preference was a function of light availability; at saturating irradiances, growth rates were nearly identical between nitrate or ammonium-supported cultures, and slower on urea. In contrast, at a low photon flux density, urea-supported cultures grew fastest (Auro and Cochlan, 2013). A potential mechanism for these differences in nitrogen use efficiencies could be the reallocation of energy from nitrogen uptake to growth when supported by urea. From this, we surmise that light-dependent uptake of nitrate may have been reduced when photosystems are damaged by UVB, yet the uptake of urea may not have been as impacted. Because cellular energetic allocation is important for DA production, this may partially explain observed increases in DA quotas between nitrogen sources for some UVB-exposed treatments. However, uptake kinetic experiments with nitrogen use efficiency calculations will need to be performed to validate these suggestions.
Previous studies examining the effects of nitrogen source on P. multiseries DA production have demonstrated a large degree of variability between strains. For instance, one strain of P. multiseries produced more DA per cell in urea-supported cultures relative to those supported by nitrate, while two separate strains both produced more DA per cell on nitrate compared to urea (Thessen et al., 2009; Martin-Jézéquel et al., 2015). This variability has also been described across the whole Pseudo-nitzschia genus, and several studies with other species and strains have observed increases in toxicity in urea-supported cultures of Pseudo-nitzschia (Howard et al., 2007; Thessen et al., 2009; Radan and Cochlan, 2018). Because of this variability within the diatom genus, elucidating the precise mechanisms for increased DA quotas with regard to nitrogen source is challenging and speculative.
Prior studies interrogating the question of nitrogen source preference used measurements of nitrogen uptake kinetics as a proxy for preference. These revealed that P. fryxelliana preferred ammonium over nitrate, with urea being the least preferred nitrogen substrate. This low preference for urea was also observed in P. australis and P. delicatissima (Cochlan et al., 2008; Loureiro et al., 2009; Auro and Cochlan, 2013). However, uptake preference does not indicate how the nitrogen is used within the cell. Future studies should not only include a broader representation of the genus, but also mechanistically investigate the role of different nitrogen sources in toxin biosynthesis, possibly using stable isotopic methods.
Multiplicative Interactions Between Multiple Drivers Provide New Insights Into P. multiseries Toxicity and Bloom Dynamics
The highest observed yield of DA per cell in this experiment may be a consequence of the interactive effects between urea, UVB, and warming that synergistically enhanced cellular DA quotas. This interaction was multiplicative, meaning the resulting DA quotas from the combination of these three variables could not have been predicted by adding together the effects of each individual driver (Boyd et al., 2018). First, trends within 20°C treatments differed from those observed within the 25°C treatments. Therefore, interactive effects observed at 25°C could not have been predicted based on trends observed at 20°C. At 25°C, UVB did not alter DA quotas between nitrate with PAR-only versus nitrate +UVB cultures, as the two treatments had similar cellular DA quotas. PAR treatments also had DA quotas that were nearly 1.5x greater for cells grown on nitrate than those supported by urea. Based on these results, we would expect DA quotas for the 25°C +UVB urea-supported treatment to be similar to the 25°C PAR-only urea-supported treatment, as +UVB does not increase DA quotas for nitrate-supported cells at 25°C. However, measured DA quotas did not follow these predictions in the present experiment, as UVB exposure of 25°C urea treatments enhanced DA production more than any of the other treatments. This result was unexpected and shows that the impact of UVB enhances DA production more than would have been expected due to interactions between light and nitrogen source.
This three-way interaction between warming, urea, and +UVB indicates that a future warmer, more stratified ocean with a greater influence of anthropogenic nutrients could enhance the toxicity of P. multiseries. However, these results also point to a potential decrease in P. multiseries bloom events, as increased UVB exposure may inhibit cellular division and growth that could offset enhanced toxicity. Despite increased toxicity per cell, harmful effects will not be so impactful if cellular abundances are low. Therefore, projected increases in cellular toxicity may be mitigated by UVB inhibition of bloom formation in a future ocean.
Alternatively, P. multiseries may find refuge from harmful UVB wavelengths in the deep chlorophyll maximum (DCM). Field surveys have detected high abundances of Pseudo-nitzschia spp. in the DCM (McManus et al., 2008; Velo-Suárez et al., 2008; Durham and Stocker, 2012). It has been suggested that Pseudo-nitzschia spp. blooms may be induced by upwelling or mixing of these seed populations from the dim DCM to the well-lit surface ocean (Seegers et al., 2015). Our multiple stressor experimental results both support and build upon these ideas by demonstrating that P. multiseries growth can be extremely sensitive to UVB, and thus may be strongly inhibited by extended exposures in the surface ocean. The thin layers of Pseudo-nitzschia spp. that reside in the DCM are protected from UVB rays, which only penetrate the first 10–12 m of the water column in clear coastal areas (Denman and Gargett, 1983; Tedetti and Sempéré, 2006). In more turbid coastal regions, these wavelengths are attenuated even more rapidly, with only ∼10% of incident UVB radiation reaching a depth of 0.5 m (Llabrés et al., 2013). In these systems, the DCM may serve as a refugium for P. multiseries in between bloom events. Mengelt and Prézelin (2005) suggested that Pseudo-nitzschia does best at mid-depths in the water column, where UVB is attenuated but UVA remains. If high concentrations of these cells are advected to the surface, exposure to UVB may be a stressor that inhibits growth and consequentially promotes DA production. The persistence of these cells and their subsequent impact on coastal ecosystems will then depend on surface conditions, including prolonged exposure to intense UVB rays, which may inhibit further growth and reduce the impact of the toxic bloom.
These results demonstrate that multiple driver experimental approaches are necessary for revealing the complex interactive effects between climate-relevant environmental variables, with consequences for toxic Pseudo-nitzschia blooms. In situ observational studies and long-term monitoring datasets are also a convenient system for studying multiple stressors, as correlations can be made between numerous environmental parameters, Pseudo-nitzschia abundance, and particulate DA (Fehling et al., 2006; Downes-Tettmar et al., 2013; Schnetzer et al., 2013; McKibben et al., 2017; Anjani et al., 2020). Laboratory-based multi-factorial experiments are advantageous in their ability to mechanistically elucidate potential interactive effects between multiple drivers, which cannot be teased apart using observational in situ studies. Furthermore, while these experiments are limited in their capacity to incorporate additional variables and levels of treatment, they portray a more comprehensive view of ocean dynamics compared to single-driver experiments. The incorporation of additional drivers would yield even more information about P. multiseries bloom dynamics, yet experiments using more than three drivers become exponentially larger in scale and are thus infeasible (Boyd et al., 2018). Therefore, based on previous single-driver literature and future projected ocean conditions, we chose three drivers that could be key controls on P. multiseries growth and toxicity, but have not been examined together before. For instance, single-driver studies have demonstrated that trace metals (iron and copper), pCO2/pH, and silicon or phosphorus limitation impact Pseudo-nitzschia toxicology, and thus should be incorporated into these multi-driver experimental designs (Bates et al., 1998; Maldonado et al., 2002; Sun et al., 2011). Future studies should combine these two approaches, using in situ observational studies to identify key drivers, and laboratory experiments to gain a mechanistic understanding of how these environmental parameters impact toxic blooms.
Conclusion
Our study is among the few to use more than two environmental drivers to test hypotheses regarding drivers of Pseudo-nitzschia toxicity and bloom formation (Tatters et al., 2018). These experiments suggest that certain combinations of environmental parameters can create conditions that synergistically or antagonistically enhance or reduce DA production and growth. The inclusion of multiple variables in experimental designs more accurately reflect the natural coastal environment where Pseudo-nitzschia spp. interacts with multiple environmental parameters simultaneously. Multiple stressor experiments therefore help us to gain a more holistic view of bloom dynamics in situ.
Because these three-way interactions cannot be predicted based on one variable alone, it is important to consider what might be missed by only including single variable experiments in models and forecasts. For instance, phosphorus and silicon limitation also seem to be a key driver of toxicity, yet we do not know how they interact in the context of multiple drivers (other than with ocean acidification; Sun et al., 2011; Tatters et al., 2012). These blooms threaten human health, the marine ecosystem, and economically important fisheries, thus accurately forecasting their occurrence is important to our ability to prepare rapid and effective responses to bloom events. However, our current HAB forecasting models are incomplete in their predictive powers, reducing our ability to anticipate toxic blooms. Although some predictive models include multiple variables, they typically do not include the interactive effects between them. Improvement of existing models may require more accurate representation of interactive effects between multiple environmental drivers on toxicity and growth of a diverse selection of toxic Pseudo-nitzschia species, in order to better understand HAB dynamics in a rapidly changing ocean.
Data Availability Statement
The raw data supporting the conclusions of this article will be made available by the corresponding authors upon request, without undue reservation.
Author Contributions
F-XF and DH conceived of and designed the experiment with help from KK and KG. KK carried out the experiment, with help from XJ and HL for PAM photophysiological measurements. KK, XJ, and HL conducted final sampling. Subsequent sample processing was done by KK, JK, and NYang. The data analyses were performed by KK, with help from MD. DX and NYe provided cultures and domoic acid sample processing. KK wrote the manuscript with contributions from DH, F-XF, KG, XJ, DX, NYe, and NYang. All authors reviewed and gave their approval for the final manuscript.
Funding
This work was supported by California State Proposition 84 funding administered by the University of Southern California Sea Grant to DH and F-XF, and by grants from the National Natural Science Foundation of China awarded to KG (41720104005) and DX and NYe (41976110).
Conflict of Interest
The authors declare that the research was conducted in the absence of any commercial or financial relationships that could be construed as a potential conflict of interest.
Acknowledgments
We thank Dr. Julie M. Rose for valuable assistance with statistics. We also thank Dr. Senjie Lin for allowing us to use his laboratory equipment.
Supplementary Material
The Supplementary Material for this article can be found online at: https://www.frontiersin.org/articles/10.3389/fmars.2021.664302/full#supplementary-material
Footnotes
References
Agustí, S., and Llabrés, M. (2007). Solar radiation-induced mortality of marine pico-phytoplankton in the oligotrophic ocean. Photochem. Photobiol. 83, 793–801. doi: 10.1111/j.1751-1097.2007.00144.x
Al-Aidaroos, A. M., O El-Sherbiny, M. M. O., Satheesh, S., Mantha, G., Agustı, S., Carreja, B., et al. (2014). High mortality of red sea zooplankton under ambient solar radiation. PLoS One 9:e108778. doi: 10.1371/journal.pone.0108778
Anderson, D. M., Kaoru, Y., and White, A. W. (2000). Economic Impacts From Harmful Algal Blooms (HABs) in the United States. Technical Report WHOI-2000-11. Woods Hole, MA: Woods Hole Oceanographic Institution.
Anjani, P. A., Larsson, M. E., Woodcock, S., Rubio, A., Farrell, H., Brett, S., et al. (2020). Fifteen years of Pseudo-nitzschia in an Australian estuary, including the first potentially toxic P. delicatissima bloom in the southern hemisphere. Estuar. Coast. Shelf S. 236:106651. doi: 10.1016/j.ecss.2020.106651
Auro, M. E., and Cochlan, W. P. (2013). Nitrogen utilization and toxin production by two diatoms of the Pseudo-nitzschia pseudodelicatissima complex: P. Cuspidata and P. Fryxelliana. J. Phycol. 49, 156–169. doi: 10.1111/jpy.12033
Barros, M. P., Pedersén, M., Colepicolo, P., and Snoeijs, P. (2003). Self-shading protects phytoplankton communities against H2O2-induced oxidative damage. Aquat. Microb. Ecol. 30, 275–282.
Bates, S. S., de Freitas, A. S. W., Milley, J. E., Pocklington, R., Quillam, M. A., Smith, J. C., et al. (1991). Controls on domoic acid production by the diatom Nitzschia pungens f. multiseries in culture: nutrients and irradiance. Can. J. Fish Aquat. Sci. 48, 1136–1144.
Bates, S. S., Garrison, D. L., and Horner, R. A. (1998). “Bloom dynamics and physiology of domoic-acid-producing Pseudo-nitzschia species,” in Physiological ecology of Harmful Algal Blooms, eds D. M. Anderson, A. D. Cembella, and G. Hallegraeff (New York, NY: Springer), 267–292.
Bates, S. S., Hubbard, K. A., Lundholm, N., Montresor, M., and Leaw, C. P. (2018). Pseudo-nitzschia, Nitzschia, and domoic acid: new research since 2011. Harmful Algae 79, 3–43. doi: 10.1016/j.hal.2018.06.001
Bates, S. S., Leger, C., and Smith, K. M. (1996). “Domoic acid production by the diatom Pseudo-nitzschia multiseries as a function of division rate in silicate-limited chemostat culture,” in Proceedings of the Harmful and Toxic Algal Blooms: Seventh International Conference on Toxic Phytoplankton, Sendai, Japan, 12-16 July 1995, Sendai.
Beardall, J., Sobrino, C., and Stojkovic, S. (2009). Interactions between the impacts of ultraviolet radiation, elevated CO2, and nutrient limitation on marine primary producers. Photochem. Photobiol. Sci. 8, 1257–1265. doi: 10.1039/b9pp00034h
Bjrkman, O., and Demmig, B. J. P. (1987). Photon yield of O2 evolution and chlorophyll fluorescence characteristics at 77 K among vascular plants of diverse origins. Planta. 170, 489–504. doi: 10.1007/BF00402983
Boyd, P. W., Collins, S., Dupont, S., Fabricius, K., Gattuso, J. P., Havenhand, J., et al. (2018). Experimental strategies to assess the biological ramifications of multiple drivers of global ocean change—a review. Glob. Chang. Biol. 24, 2239–2261. doi: 10.1111/gcb.14102
Cai, X., Hutchins, D. A., Fu, F., and Gao, K. (2017). Effects of utraviolet radiation on photosynthetic performance and N2 fixation in Trichodesmium erythraeum IMS 101. Biogeosciences 14, 4455–4466. doi: 10.5194/bg-14-4455-2017
Chen, J., Wang, H., Yang, A. Q., Si, R. R., and Guan, W. C. (2018). Short-term and diurnal temperature changes alter the response of harmful algal blooms of Pseudo-nitzschia pungens to solar ultraviolet radiation. New Zeal. J. Mar. Fresh. 52, 69–81. doi: 10.1080/00288330.2017.1331454
Cochlan, W. P., Herndon, J., and Kudela, R. M. (2008). Inorganic and organic nitrogen uptake by the toxigenic diatom Pseudo-nitzschia Australis (Bacillariophyceae). Harmful Algae 8, 111–118. doi: 10.1016/j.hal.2008.08.008
Cullen, J. J., and Lesser, M. P. (1991). Inhibition of photosynthesis by ultraviolet radiation as a function of dose and dosage rate: results for a marine diatom. Mar. Biol. 111, 183–190. doi: 10.1007/BF01319699
Denman, K. L., and Gargett, A. (1983). Time and space scales of vertical mixing and advection of phytoplankton in the upper ocean. Limnol. Oceanogr. 28, 801–815. doi: 10.4319/lo.1983.28.5.0801
Downes-Tettmar, N., Rowland, S., Widdicombe, C., Woodward, M., and Llewellyn, C. (2013). Seasonal variation in Pseudo-nitzschia spp. and domoic acid in the Western English Channel. Cont. Shelf. Res. 53, 40–49.
Duarte, C. M. (2014). Global change and the future ocean: a grand challenge for marine sciences. Front. Mar. Sci. 1:63. doi: 10.3389/fmars.2014.00063
Dugdale, R. C., and Goering, J. J. (1967). Uptake of new and regenerated forms of nitrogen in primary productivity. Limnol. Oceanogr. 12, 196–206. doi: 10.4319/lo.1967.12.2.0196
Durham, W. M., and Stocker, R. (2012). Thin phytoplankton layers: characteristics, mechanisms, and consequences. Annu. Rev. Mar. Sci 4, 177–207. doi: 10.1146/annurev-marine-120710-100957
Fehling, J., Davidson, K., Bolch, C. J. S., and Tett, P. (2006). Seasonality of Pseudo-nitzschia spp. (Bacillariophyceae) in Western Scottish waters. Mar. Ecol. Prog. Ser. 323, 91–105. doi: 10.3354/meps323091
Fehling, J., Green, D. H., Davidson, K., Bolch, C. J., and Bates, S. S. (2004). Domoic acid production by Pseudo-nitzschia seriata (Bacillariophyceae) in Scottish waters. J. Phycol. 40, 622–630. doi: 10.1111/j.1529-8817.2004.03200.x
Fu, F.-X., Tatters, A. O., and Hutchins, D. A. (2012). Global change and the future of harmful algal blooms in the ocean. Mar. Ecol. Prog. Ser. 470, 207–233. doi: 10.3354/meps10047
Fu, F.-X., Zhang, Y., Feng, Y., and Hutchins, D. A. (2007). Phosphate and ATP uptake and growth kinetics in axenic cultures of the cyanobacterium Synechococcus CCMP 1334. Eur. J. Phycol. 41, 15–28. doi: 10.1080/09670260500505037
Gao, K., Beardall, J., Häder, D. P., Hall-Spencer, J. M., Gao, G., and Hutchins, D. A. (2019). Effects of ocean acidification on marine photosynthetic organisms under the concurrent influences of warming, UV radiation, and deoxygenation. Front. Mar. Sci. 6:322. doi: 10.3389/fmars.2019.00322
Gao, K., Wu, Y., Li, G., Wu, H., Villafañe, V. E., and Helbling, E. W. (2007a). Solar UV radiation drives CO2 fixation in marine phytoplankton: a double-edged sword. Plant Physiol. 144, 54–59. doi: 10.1104/pp.107.098491
Gao, K., Yu, H., and Brown, M. T. (2007b). Solar PAR and UV radiation affects the physiology and morphology of the cyanobacterium Anabaena sp. PCC 7120. J. Photochem. Photobiol. B Biol. 89, 117–124. doi: 10.1016/j.jphotobiol.2007.09.006
Glibert, P. M., Harrison, J., Heil, C., and Seitzinger, S. (2006). Escalating worldwide use of urea - a global change contributing to coastal eutrophication. Biogeochemistry 77, 441–463. doi: 10.1007/s10533-005-3070-5
Glibert, P. M., Seitzinger, S., Heil, C. A., Burkholder, J. M., Parrow, M. W., Codispoti, L. A., et al. (2005). The role of eutrophication in the global proliferation of harmful algal blooms. Oceanography 18, 198–209. doi: 10.5670/oceanog.2005.54
Gobler, C. J. (2020). Climate change and harmful algal blooms: insights and perspective. Harmful Algae 91, 1–4. doi: 10.1016/j.hal.2019.101731
Godinho, L., Silva, A., Branco, M. A. C., Marques, A., and Costa, P. R. (2018). Evaluation of intracellular and extracellular domoic acid content in Pseudo-nitzschia multiseries cell cultures under different light regimes. Toxicon 155, 27–31. doi: 10.1016/j.toxicon.2018.10.003
Godoy, N., Canepa, A., Lasternas, S., Mayol, E., Ruíz-Halpern, S., Agustí, S., et al. (2012). Experimental assessment of the effect of UVB radiation on plankton community metabolism along the Southeastern Pacific off Chile. Biogeosciences 9, 1267–1276. doi: 10.5194/bg-9-1267-2012
Gonçalves, R. J., Sol Souza, M., Aigo, J., Modenutti, B., Balseiro, E., Villafañe, V. E., et al. (2010). Responses of plankton and fish from temperate zones to UVR and temperature in a context of global change. Ecol. Austral 20, 129–153.
Guan, W., and Gao, K. (2010). Impacts of UV radiation on photosynthesis and growth of the coccolithophore Emiliania huxleyi (Haptophyceae). Environ. Exp. Bot. 67, 502–508. doi: 10.1016/j.envexpbot.2009.08.003
Guillard, R. R. L. (1975). “Culture of phytoplankton for feeding marine invertebrates,” in Culture of Marine Invertebrate Animals, eds W. L. Smith and M. H. Chanley (New York, NY: Plenum Press), 26–60.
Guillard, R. R. L., and Ryther, J. H. (1962). Studies of marine planktonic diatoms. I. Cyclotella nana Hustedt and Detonula confervacea Cleve. Can. J. Microbiol. 8, 229–239. doi: 10.1139/m62-029
Halac, S., García-Mendoza, E., and Banaszak, A. T. (2009). Ultraviolet radiation reduces the photoprotective capacity of the marine diatom Phaeodactylum tricornutum (Bacillariophyceae, Heterokontophyta). Photochem. Photobiol. 85, 807–815. doi: 10.1111/j.1751-1097.2008.00497.x
Hallegraeff, G. M. (1993). A review of harmful algal blooms and their apparent global increase. Phycologica 32, 79–99. doi: 10.1016/0025-326X(92)90223-S
Hargraves, P. E., Zhang, J., Wang, R., and Shimizu, Y. (1993). Growth characteristics of the diatoms Pseudonitzschia pungens and P. fraudulenta exposed to ultraviolet radiation. Hydrobiologia 26, 207–212. doi: 10.1007/BF00028019
Heisler, J., Glibert, P. M., Burkholder, J. M., Anderson, D. M., Cochlan, W., Dennison, W. C., et al. (2008). Eutrophication and harmful algal blooms: a scientific consensus. Harmful Algae 8, 3–13. doi: 10.1016/j.hal.2008.08.006
Helbling, E. W., Gao, K., Gonçalves, R. J., Wu, H., and Villafañe, V. E. (2003). Utilization of solar UV radiation by coastal phytoplankton assemblages off SE China when exposed to fast mixing. Mar. Ecol. Prog. Ser. 259, 59–66. doi: 10.3354/meps259059
Helbling, E. W., Villafane, V., Ferrario, M., and Holm-Hansen, O. (1992). Impact of natural ultraviolet radiation on rates of photosynthesis and on specific marine phytoplankton species. Mar. Ecol. Prog. Ser. 80, 89–100. doi: 10.3354/meps080089
Helbling, W. E., Buma, A. G. J., Boelen, P., van der Strate, H. J., Giordanino, V. F. M., and Villafañe, V. E. (2011). Increase in rubisco activity and gene expression due to elevated temperature partially counteracts ultraviolet radiation-induced photoinhibition in the marine diatom Thalassiosira weissflogii. Limnol. Oceanogr. 56, 1330–1342. doi: 10.4319/lo.2011.56.4.1330
Howard, M. D. A., Cochlan, W. P., Ladizinsky, N., and Kudela, R. M. (2007). Nitrogenous preference of toxigenic Pseudo-nitzschia australis (Bacillariophyceae) from field and laboratory experiments. Harmful Algae. 6, 206–217. doi: 10.1016/j.hal.2006.06.003
Howard, M. D. A., Sutula, M., Caron, D. A., Chao, Y., Farrara, J. D., Frenzel, H., et al. (2014). Anthropogenic nutrient sources rival natural sources on small scales in the coastal waters of the Southern California Bight. Limnol. Oceanogr. 59, 285–297. doi: 10.4319/lo.2014.59.1.0285
Hutchins, D. A., and Bruland, K. W. (1998). Iron-limited growth and Si:N ratios in a costal upwelling regime. Nature 393, 561–564. doi: 10.1038/31203
Hutchins, D. A., and Fu, F. X. (2017). Microorganisms and ocean global change. Nat. Microbiol. 2, 1–11. doi: 10.1038/nmicrobiol.2017.58
Jassby, A. D., and Platt, T. (1976). Mathematical formulation of the relationship between photosynthesis and light for phytoplankton. Limnol. Oceanogr. 21, 540–547. doi: 10.4319/lo.1976.21.4.0540
Kilham, S. S., Kott, C. L., and Tilman, D. (1977). Phosphate and silicate kinetics for the Lake Michigan diatom Diatoma Elongatum. J. Great Lakes Res. 3, 93–99. doi: 10.1016/S0380-1330(77)72233-6
Kudela, R. M., Lane, J. Q., and Cochlan, W. P. (2008). The potential role of anthropogenically derived nitrogen in the growth of harmful algae in California, USA. Harmful Algae 8, 103–110. doi: 10.1016/j.hal.2008.08.019
Lange, C. B., Reid, F. M. H., and Vernet, M. (1994). Temporal distribution of the potentially toxic diatom Pseudonitzschia australis at a coastal site in Southern California. Mar. Ecol. Prog. Ser. 104, 309–312. doi: 10.3354/meps104309
Legrand, C., Granéli, E., and Carlsson, P. (1998). Induced phagotrophy in the photosynthetic dinoflagellate Heterocapsa triquetra. Aquat. Microb. Ecol. 15, 65–75. doi: 10.3354/ame015065
Lelong, A., Hégaret, H., Soudant, P., and Bates, S. S. (2012). Pseudo-nitzschia (Bacillariophyceae) species, domoic acid and amnesic shellfish poisoning: revisiting previous paradigms. Phycologica 51, 168–216. doi: 10.2216/11-37
Lesser, M. P. (1996). Acclimation of phytoplankton to UV-B radiation: oxidative stress and photoinhibition of photosynthesis are not prevented by UV-absorbing compounds in the dinoflagellate Prorocentrum micans. Mar. Ecol. Prog. Ser. 132, 287–297. doi: 10.3354/meps132287
Li, G., Gao, K., and Gao, G. (2011). Differential impacts of solar UV radiation on photosynthetic carbon fixation from the coastal to offshore surface waters in the South China sea. Photochem. Photobiol. 87, 329–334. doi: 10.1111/j.1751-1097.2010.00862.x
Li, J., Glibert, P. M., Alexander, J. A., and Molina, M. E. (2012). Growth and competition of several harmful dinoflagellates under different nutrient and light conditions. Harmful Algae 13, 112–125. doi: 10.1016/j.hal.2011.10.005
Li, Y., Gao, K., Villafane, V. E., and Helbling, E. W. (2012). Ocean acidification mediates photosynthetic response to UV radiation and temperature increase in the diatom Phaeodactylum tricornutum. Biogeosciences 9, 3931–3942. doi: 10.5194/bg-9-3931-2012
Liang, Y., Beardall, J., and Heraud, P. (2006). Effects of nitrogen source and UV radiation on the growth, chlorophyll fluorescence and fatty acid composition of Phaeodactylum tricornutum and Chaetoceros muelleri (Bacillariophyceae). J. Photochem. Photobiol. B Biol. 82, 161–172. doi: 10.1016/j.jphotobiol.2005.11.002
Liefer, J. D., Garg, A., Fyfe, M. H., Irwin, A. J., Benner, I., Brown, C. M., et al. (2019). The macromolecular basis of phytoplankton C:N:P under nitrogen starvation. Front. Microbiol. 10:763. doi: 10.3389/fmicb.2019.00763
Llabrés, M., and Agustí, S. (2006). Picophytoplankton cell death induced by UV radiation: evidence for oceanic Atlantic communities. Limnol. Oceanogr. 51, 21–29. doi: 10.4319/lo.2006.51.1.0021
Llabrés, M., Agustí, S., Alonso-Laita, P., and Herndl, G. (2010). Synechococcus and Prochlorococcus cell death induced by UV radiation and the penetration of lethal UVR in the Mediterranean Sea. Mar. Ecol. Prog. Ser 399, 27–37. doi: 10.3354/meps08332
Llabrés, M., Agustí, S., Fernández, M., Canepa, A., Maurin, F., Vidal, F., et al. (2013). Impact of elevated UVB radiation on marine biota: a meta-analysis. Glob. Ecol. Biogeogr. 22, 131–144. doi: 10.1111/j.1466-8238.2012.00784.x
Loureiro, S., Jauzein, C., Garcés, E., Collos, Y., Camp, J., and Vaqué, D. (2009). The significance of organic nutrients to Pseudo-nitzschia delicatissima (Bacillariophyceae) nutrition. J. Plankton Res. 31, 399–410. doi: 10.1093/plankt/fbn122
Lundholm, N., Skov, J., Pocklington, R., and Moestrup, O. (1994). Domoic acid, the toxic amino acid responsible for amnesic shellfish poisoning, now in Pseudonitzschia seriata (Bacillariophyceae) in Europe. Phycologica 33, 475–478. doi: 10.2216/i0031-8884-33-6-475.1
Maldonado, M. T., Hughes, M. P., Rue, E. L., and Wells, M. L. (2002). The effect of Fe and Cu on growth and domoic acid production by Pseudo-nitzschia multiseries and Pseudo-nitzschia australis. Limnol. Oceanogr. 47, 515–526. doi: 10.4319/lo.2002.47.2.0515
Martin-Jézéquel, V., Calu, G., Candela, L., Amzil, Z., Jauffrais, T., Séchet, V., et al. (2015). Effects of organic and inorganic nitrogen on the growth and production of domoic acid by Pseudo-nitzschia multiseries and P. australis (Bacillariophyceae) in culture. Mar. Drugs 13, 7067–7086. doi: 10.3390/md13127055
McCabe, R. M., Hickey, B. M., Kudela, R. M., Lefebvre, K. A., Adams, N. A., Bill, B. D., et al. (2016). An unprecedented coastwide toxic algal bloom linked to anomalous ocean conditions. Geophys. Res. Lett 64, 10366–10376. doi: 10.1002/2016GL070023
McKibben, S. M., Peterson, W., Wood, A. M., Trainer, V. L., Hunter, M., and White, A. E. (2017). Climatic regulation of the neurotoxin domoic acid. Proc. Natl. Acad. Sci.U.S.A. 114, 239–244. doi: 10.1073/pnas.1606798114
McKibben, S. M., Watkins-Brandt, K. S., Wood, M. A., Hunter, M., Forster, Z., Hopkins, A., et al. (2015). Monitoring Oregon coastal harmful algae: observations and implications of a harmful algal bloom-monitoring project. Harmful Algae 50, 32–44. doi: 10.1016/j.hal.2015.10.004
McManus, M. A., Kudela, R. M., Silver, M. W., Steward, G. F., Donaghay, P. L., and Sullivan, J. M. (2008). Cryptic blooms: are thin layers the missing connection? Estuar. Coasts. 31, 396–401. doi: 10.1007/s12237-007-9025-4
Mengelt, C., and Prézelin, B. B. (2005). UVA enhancement of carbon fixation and resilience to UV inhibition in the genus Pseudo-nitzschia may provide a competitive advantage in high UV surface waters. Mar. Ecol. Prog. Ser. 301, 81–93. doi: 10.3354/meps301081
Mitchell, D. L., and Karentz, D. (1993). “The induction and repair of DNA photodamage in the environment,” in Environmental UV Photobiology, eds. A. R. Young, L. O. Björn, J. Moan, and W. Nultsch (New York, NY: Plenum Press), 345–377.
National Center for Biotechnology Information (2021). PubChem Compound Summary for CID 5282253, Domoic acid. Available online at: https://pubchem.ncbi.nlm.nih.gov/compound/L-Domoic-acid (accessed March 26, 2021).
Neale, P. J., Lesser, M. P., and Cullen, J. J. (1994). “Effects of ultraviolet radiation on the photosynthesis of phytoplankton in the vicinity of McMurdo Station, Antarctica,” in Ultraviolet radiation in Antarctica: Measurements and Biological Effects, eds C. S. Weiler and P. A. Penhale (Washington, D.C: Antarctic Research Series, American Geophysical Union), 125–142.
Nelson, D. M., Trrguer, P., Brzezinski, M. A., Leynaert, A., and Quéguiner, B. (1995). Production and dissolution of biogenic silica in the ocean: revised global estimates, comparison with regional data and relationship to biogenic sedimentation. Glob. Biogeochem. Cycle 9, 359–372. doi: 10.1029/95GB01070
Nilawati, J., Greenberg, B. M., and Smith, R. E. H. (1997). Influence of ultraviolet radiation on growth and photosynthesis of two cold ocean diatoms. J. Phycol. 33, 215–224. doi: 10.1111/j.0022-3646.1997.00215.x
Olesen, A. J., Harðardóttir, S., DAugbjerg, N., Andersen, P., Lyngsgaard, M., Krock, B., et al. (2020). The impact of urea on toxic diatoms – potential effects of fertilizer silo breakdown on a Pseudo-nitzschia bloom. Harmful algae 95:101817.
Oliver, E. C. J., Donat, M. G., Burrows, M. T., Moore, P. J., Smale, D. A., Alexander, L. V., et al. (2018). Longer and more frequent marine heatwaves over the past century. Nat. Commun. 9, 1–12. doi: 10.1038/s41467-018-03732-9
Pan, Y., Bates, S. S., and Cembella, A. D. (1998). Environmental stress and domoic acid production by Pseudo-nitzschia: a physiological perspective. Nat. Toxins 6, 127–135. doi: 10.1002/(SICI)1522-7189(199805/08)6
Pan, Y., Subba Rao, D. V., Mann, K. H., Brown, R. G., and Pocklington, R. (1996). Effects of silicate limitation on production of domoic acid, a neurotoxin, by the diatom Pseudo-nitzschia multiseries. I. Batch culture studies. Mar. Ecol. Prog. Ser. 131, 225–233. doi: 10.3354/meps131225
Perl, T. M., Bédard, L., Kosatsky, T., Hockin, J. C., Todd, E. C. D., and Remis, R. S. (1990). An outbreak of toxic encephalopathy caused by eating mussels contaminated with domoic acid. N. Engl. J. Med. 322, 1775–1780. doi: 10.1056/NEJM199006213222504
Quesada, A., Mouget, J., and Vincent, W. F. (1995). Growth of Antarctic cyanobacteria under ultraviolet radiation: UVA counteracts UVB inhibition. J. Phycol. 31, 242–248. doi: 10.1111/j.0022-3646.1995.00242.x
Radan, R. L., and Cochlan, W. P. (2018). Differential toxin response of Pseudo-nitzschia multiseries as a function of nitrogen speciation in batch and continuous cultures, and during a natural assemblage experiment. Harmful Algae 73, 12–29. doi: 10.1016/j.hal.2018.01.002
Ralph, P. J., Macinnis-Ng, C. M. O., and Frankart, C. (2005). Fluorescence imaging application: effect of leaf age on seagrass photokinetics. Aquat. Bot. 81, 69–84. doi: 10.1016/j.aquabot.2004.11.003
Rees, A. P., Hope, S. B., Widdicombe, C. E., Dixon, J. L., Woodward, E. M. S., and Fitzsimons, M. F. (2009). Alkaline phosphatase activity in the Western English Channel: elevations induced by high summertime rainfall. Estuar. Coast. Mar. Sci. 81, 569–574. doi: 10.1016/j.ecss.2008.12.005
Ritzman, J., Brodbeck, A., Brostrom, S., Mcgrew, S., Dreyer, S., Klinger, T., et al. (2018). Economic and sociocultural impacts of fisheries closures in two fishing-dependent communities following the massive 2015 U.S. West Coast harmful algal bloom. Harmful Algae 80, 35–45. doi: 10.1016/j.hal.2018.09.002
Roncarati, F., Rijstenbil, J. W., and Pistocchi, R. (2008). Photosynthetic performance, oxidative damage and antioxidants in Cylindrotheca closterium in response to high irradiance, UVB radiation and salinity. Mar. Biol. 153, 965–973. doi: 10.1007/s00227-007-0868-9
Schnetzer, A., Jones, B. H., Schaffner, R. A., Cetinic, I., Fitzpatrick, E., Miller, P. E., et al. (2013). Coastal upwelling linked to toxic Pseudo-nitzschia australis blooms in Los Angeles coastal waters, 2005-2007. J. Plankton Res. 35, 1080–1092. doi: 10.1093/plankt/fbt051
Schreiber, U., Klughammer, C., and Kolbowski, J. (2011). High-end chlorophyll fluorescence analysis with the MULTI-COLOR-PAM. I. Various light qualities and their applications. PAM Appl. Notes 1, 1–21.
Seegers, B. N., Birch, J. M., Marin, R., Scholin, C. A., Caron, D. A., Seubert, E. L., et al. (2015). Subsurface seeding of surface harmful algal blooms observed through the integration of autonomous gliders, moored environmental sample processors, and satellite remote sensing in southern California. Limnol. Oceanogr. 60, 754–764. doi: 10.1002/lno.10082
Smith, J., Connell, P., Evans, R. H., Gellene, A. G., Howard, M. D. A., Jones, B. H., et al. (2018). A decade and a half of Pseudo-nitzschia spp. and domoic acid along the coast of southern California. Harmful Algae 79, 87–104. doi: 10.1016/j.hal.2018.07.007
Smith, R. C., Prézelin, B. B., Baker, K. S., Bidigare, R. R., Boucher, N. P., Coley, T., et al. (1992). Ozone depletion: ultraviolet radiation and phytoplankton biology in Antarctic waters. Science 255, 952–959. doi: 10.1126/science.1546292
Smith, S. R., Dupont, C. L., McCarthy, J. K., Broddrick, J. T., Oborník, M., Horák, A., et al. (2019). Evolution and regulation of nitrogen flux through compartmentalized metabolic networks in a marine diatom. Nat. Commun. 10, 1–14. doi: 10.1038/s41467-019-12407-y
Steinacher, M., Joos, F., Frölicher, T. L., Bopp, L., Cadule, P., Cocco, V., et al. (2010). Projected 21st century decrease in marine productivity: a multi-model analysis. Biogeosciences 7, 979–1005. doi: 10.5194/bg-7-979-2010
Sun, J., Hutchins, D. A., Feng, Y., Seubert, E. L., Caron, D. A., and Fu, F.-X. (2011). Effects of changing pCO2 and phosphate availability on domoic acid production and physiology of the marine harmful bloom diatom Pseudo-nitzschia multiseries. Limnol. Oceanogr. 56, 829–840. doi: 10.4319/lo.2011.56.3.0829
Tantanasarit, C., Englande, A. J., and Babel, S. (2013). Nitrogen, phosphorus and silicon uptake kinetics by marine diatom Chaetoceros calcitrans under high nutrient concentrations. J. Exp. Mar. Bio. Ecol. 446, 67–75. doi: 10.1371/journal.pone.0032116
Tatters, A. O., Fu, F. X., and Hutchins, D. A. (2012). High CO2 and silicate limitation synergistically increase the toxicity of Pseudo-nitzschia fraudulenta. PLoS One 7:e32116.
Tatters, A. O., Schnetzer, A., Xu, K., Walworth, N. G., Fu, F., Spackeen, J. L., et al. (2018). Interactive effects of temperature, CO2 and nitrogen source on a coastal California diatom assemblage. J. Plankton Res. 00, 1–14. doi: 10.1093/plankt/fbx074
Tedetti, M., and Sempéré, R. (2006). Penetration of ultraviolet radiation in the marine environment. A review. Photochem. Photobiol. 82, 389–397. doi: 10.1562/2005-11-09-ir-733
Thessen, A. E., Bowers, H. A., and Stoecker, D. K. (2009). Intra- and interspecies differences in growth and toxicity of Pseudo-nitzschia while using different nitrogen sources. Harmful Algae 8, 792–810. doi: 10.1016/j.hal.2009.01.003
Trainer, V. L., Bates, S. S., Lundholm, N., Thessen, A. E., Cochlan, W. P., Adams, N. G., et al. (2012). Pseudo-nitzschia physiological ecology, phylogeny, toxicity, monitoring and impacts on ecosystem health. Harmful Algae 14, 271–300. doi: 10.1016/j.hal.2011.10.025
Trainer, V. L., Kudela, R. M., Hunter, M. V., Adams, N. G., and McCabe, R. M. (2020). Climate extreme seeds a new domoic acid hotspot on the US West Coast. Front. Clim. 2:571836. doi: 10.3389/fclim.2020.571836
Velo-Suárez, L., González-Gil, S., Gentien, P., Lunven, M., Bechemin, C., Fernand, L., et al. (2008). Thin layers of Pseudo-nitzschia spp. and the fate of Dinophysis acuminata during an upwelling-downwelling cycle in a Galician Ría. Limnol. Oceanogr. 53, 1816–1834. doi: 10.4319/lo.2008.53.5.1816
Villafañe, V. E., Gao, K., Li, P., Li, G., and Helbling, E. W. (2007). Vertical mixing within the epilimnion modulates UVR-induced photoinhibition in tropical freshwater phytoplankton from southern China. Freshw. Biol. 52, 1260–1270. doi: 10.1111/j.1365-2427.2007.01762.x
Wang, Z., Maucher-Fuquay, J., Fire, S. E., Mikulski, C. M., Haynes, B., Doucette, G. J., et al. (2012). Optimization of solid-phase extraction and liquid chromatography–tandem mass spectrometry for the determination of domoic acid in seawater, phytoplankton, and mammalian fluids and tissues. Anal. Chim. Acta. 715, 71–79. doi: 10.1016/j.aca.2011.12.013
Xu, J., and Gao, K. (2010). UV-A enhanced growth and UV-B induced positive effects in the recovery of photochemical yield in Gracilaria lemaneiformis (Rhodophyta). J. Photochem. Photobiol. B Biol. 100, 117–122. doi: 10.1016/j.jphotobiol.2010.05.010
Zhang, T., Hutchins, D. A., and Gao, K. (2019). Physiological and biochemical respones of Emiliania huxleyi to ocean acidification and warming are modulated by UV radiation. Hydrobiologia 842, 127–141. doi: 10.1007/s10750-019-04031-0
Zhu, Z., Fu, F.-X., Qu, P., Mak, E. W. K., Jiang, H., Zhang, R., et al. (2020). Interactions between ultraviolet radiation exposure and phosphorus limitation in the marine nitrogen-fixing cyanobacteria Trichodesmium and Crocosphaera. Limnol. Oceanogr. 65, 363–376. doi: 10.1002/lno.11304
Keywords: multiple drivers, Pseudo-nitzschia multiseries, domoic acid, climate change, harmful algal blooms, ultraviolet radiation
Citation: Kelly KJ, Fu F-X, Jiang X, Li H, Xu D, Yang N, DeMers MA, Kling JD, Gao K, Ye N and Hutchins DA (2021) Interactions Between Ultraviolet B Radiation, Warming, and Changing Nitrogen Source May Reduce the Accumulation of Toxic Pseudo-nitzschia multiseries Biomass in Future Coastal Oceans. Front. Mar. Sci. 8:664302. doi: 10.3389/fmars.2021.664302
Received: 04 February 2021; Accepted: 31 March 2021;
Published: 25 May 2021.
Edited by:
Susana Agusti, King Abdullah University of Science and Technology, Saudi ArabiaReviewed by:
Pedro R. Costa, Portuguese Institute for Sea and Atmosphere (IPMA), PortugalCarole Anne Llewellyn, Swansea University, United Kingdom
Copyright © 2021 Kelly, Fu, Jiang, Li, Xu, Yang, DeMers, Kling, Gao, Ye and Hutchins. This is an open-access article distributed under the terms of the Creative Commons Attribution License (CC BY). The use, distribution or reproduction in other forums is permitted, provided the original author(s) and the copyright owner(s) are credited and that the original publication in this journal is cited, in accordance with accepted academic practice. No use, distribution or reproduction is permitted which does not comply with these terms.
*Correspondence: David A. Hutchins, ZGFodXRjaEB1c2MuZWR1; Naihao Ye, eWVuaEB5c2ZyaS5hYy5jbg==
†Present address: Joshua D. Kling, California Institute for Quantitative Biosciences, University of California, Berkley, Berkley, CA, United States
‡These authors share last authorship