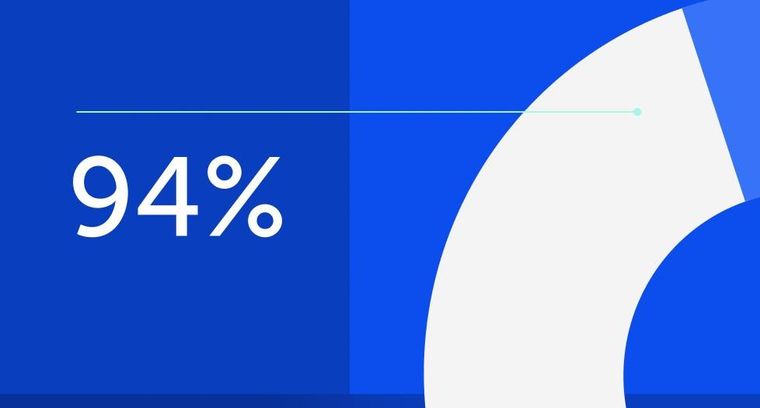
94% of researchers rate our articles as excellent or good
Learn more about the work of our research integrity team to safeguard the quality of each article we publish.
Find out more
BRIEF RESEARCH REPORT article
Front. Mar. Sci., 23 March 2021
Sec. Global Change and the Future Ocean
Volume 8 - 2021 | https://doi.org/10.3389/fmars.2021.660427
This article is part of the Research TopicInfluence of Environmental Variability on Climate Change Impacts in Marine EcosystemsView all 12 articles
Ongoing climate warming demands a better understanding of whether or how the ectotherms that evolved in response to fluctuating stress regimes may acquire increased heat tolerance. Using blue mussels, Mytilus spp., a globally important and well-studied species, we provide empirical evidence supporting that (i) extremely warm (future) summer conditions may select rare recruits that are more capable of expressing metabolic (feeding and respiration) suppression and recovery in response to daily thermal fluctuations in mild to critical temperature range, (ii) this higher heat tolerance can be mediated by lower baseline metabolic demand, possibly decreasing the risks of heat-induced supply and demand mismatch and its associated stress during thermal fluctuations, and (iii) the capacity to acquire such heat tolerance through acclimation is minor. We discuss our results, methodological limitations and offer a perspective for future research. Further evaluation of mechanistic hypotheses such as the one tested here (based on the role of metabolic demand) is needed to generalize the significance of drivers of fast warm adaptation in ectothermic metazoan populations.
Marine ectotherms that have evolved in response to fluctuating stress regimes in environments such as shallow habitats can express remarkable suppression and recovery of metabolic performance over successive phases of critical and benign temperatures (Marshall and McQuaid, 1991). Metabolic suppression is expressed as substantial declines in feeding activities followed by partial decreases in aerobic respiration or extensive transition from aerobic to anaerobic respiration (Sokolova and Pörtner, 2001). By suppressing the rate of energy-intensive processes (e.g., feeding and growth), these organisms reduce their demand for metabolic substrates and energy (Schulte et al., 2011). Otherwise, their demands rise sharply with increasing temperature and eventually exceed the capacity to supply the required substrates from internal reserves. The supply capacity is related to the rates of resource uptake, internal reserve formation, and reserve mobilization (Kooijman, 2010) and is generally less temperature-dependent than the metabolic demand (Pörtner, 2012; Rall et al., 2012; Ritchie, 2018). The heat-induced mismatch between metabolic supply and demand can give rise to internal entropy, stress, and damage, exacerbating organismal performance over time (Pörtner, 2012; Ritchie, 2018). Therefore, metabolic suppression may allow organisms to minimize the negative impacts of critical temperatures during short-term (e.g., daily) thermal fluctuations (Vajedsamiei et al., unpublished).
Ongoing ocean warming increases the probability of exposure to high, beyond-optimal temperatures for marine organisms (Brewer et al., 2014). Due to this warming, periods of suppression may become longer while recovery phases may shorten, assuming no change in the temperature fluctuation amplitude and length. Enforcing metabolic suppression in response to more intense or extended periods of high critical temperatures may cause or exacerbate the supply and demand mismatch and its associated stress, limiting an organism’s capacity to resume activity during the shortened recovery phases (Vajedsamiei et al., unpublished). Thus, it is crucial to know whether and how exposure to warming trends is associated with increased heat tolerance in the form of elevated thermal thresholds of metabolic suppression or increased capacity for recovery during thermal fluctuations.
Within- and between-generational acclimation to warmer ambient regimes may increase heat tolerance in ectotherm individuals, leading to warm adaptation in some populations (Davenport and Davenport, 2005; Wittmann et al., 2008). For example, acclimation to a stressful event (e.g., heatwave) may functionally prepare or disturb the organism for successive stress events (Walter et al., 2013; Giomi et al., 2016; Bernhardt et al., 2020). Nonetheless, beneficial acclimation capacity may be limited for species from highly fluctuating environments (Stillman, 2003; Somero, 2010; Seebacher et al., 2014) due to a tradeoff in favor of the capacity for metabolic suppression and recovery (McMahon et al., 1995). Additionally, increases in heat tolerance through beneficial genetic mutation alone might not be fast enough to adapt populations to ocean warming (Somero, 2010) as suggested by the observed warming-induced extinctions and changes of biogeographical distributions of species (Barry et al., 1995; Sagarin et al., 1999; Wethey and Woodin, 2008). Finally, emerging evidence suggests that directional selection in favor of heat-tolerant individuals may enforce a shift in a population’s mean thermal performance (Logan et al., 2014, 2018; Ma et al., 2014; Gilbert and Miles, 2017). Many marine ectothermic species express their lowest heat-tolerance during the spawning, egg, and larval stages (Pörtner and Farrell, 2008; Nasrolahi et al., 2012). Therefore, in theory, directional selection may be imposed by extreme events on these sensitive life-history stages, a critical phenomenon to investigate since such extreme events are being enforced by climate change (Grant et al., 2017; Al-Janabi et al., 2019).
Whether through directional selection or acclimation, ectothermic individuals or populations may need to acquire lowered metabolic demand to decrease the risks of heat-induced supply and demand mismatch and its associated stress during critically warm phases of thermal fluctuations. This hypothesis also corroborates the temperature compensation (Hazel and Prosser, 1974) or metabolic cold adaptation (Clarke, 2003) hypotheses proposing that individuals adapted to warmer environments, compared to cold-adapted ones, usually have lower metabolic demand (represented by the respiration rate of non-stressed individuals) at similar benign temperatures. This allows individuals to optimize their metabolic performances according to their local thermal regimes (Le Lann et al., 2011).
Using blue mussels, Mytilus spp., an economically and ecologically important species, and a model system for studying metabolic suppression capability of ectotherms, this research evaluates (i) whether extremely warm summer conditions would select for individuals with higher heat tolerance in their daily metabolic suppression and recovery responses and (ii) whether such a potentially warm-adaptive shift in the metabolic responses is linked to lower metabolic demand of the individuals. In a quasi-natural flow-through benthocosm system, mussels from the western Baltic Sea (Kiel Fjord) introduced as juveniles (transplanted organisms) or as larvae (recruited organisms) were grown under the current (+0°C) vs. future (+4°C) thermal history levels, imposed onto summer 2018 natural temperature regimes. After incubation for 4 months, the performance traits feeding and aerobic respiration were recorded in response to a mild temperature (20.8°C) for 6 h (baseline performance) followed by two 24 h thermal fluctuation cycles with extreme amplitudes (20.8–30.5°C).
The hypotheses of this study were: (i) The warmer thermal history would result in individuals with higher heat tolerance as evidenced by warmer thresholds of metabolic suppression or higher recovery or both responses to daily thermal fluctuation cycles (Figure 1A), and (ii) such higher heat tolerance would be mediated by a lower baseline metabolic demand (Figure 1B).
Figure 1. Hypotheses sketch. (A) Organisms selected by or acclimated to extreme summer baselines (e.g., prolonged heatwaves) may express higher heat tolerance in the form of (i) higher thermal thresholds of feeding and respiration suppression and recovery (i.e., a broader temperature range for optimal performance; horizontal axis in A) or (ii) a generally higher metabolic performance (recovery) at benign phases of thermal fluctuations (vertical axis in A), or both. (B) Compared to non-selected or non-acclimated individuals, heat-tolerant individuals may have lower metabolic demands (baseline performances) but with a normally high demand/supply ratio (not presented here) under a mild ambient temperature. When an organism’s initial thermal performance (middle plot) is maintained over several daily fluctuation cycles, it means that the heat tolerance has not changed over time (i.e., elastic metabolic suppression and recovery in response to thermal fluctuations). However, the initial thermal performance (middle plot) can change over time due to acclimation or stress (plastic responses to fluctuations), resulting in other forms of responses. Notes: To simplify, we assumed there are no gradual thermal changes in the performance due to thermodynamics (Q10 effects), and the slope of fast changes in the performance is infinity leading to immediate suppression and recovery.
The study organism is the filter feeder Mytilus edulis trossulus, the species complex that forms extensive mussel beds in the Baltic Sea (Larsson et al., 2017; Stuckas et al., 2017) where the tidal range is minimal (<few cm). However, ambient temperature regimes fluctuate in time due to daily irradiance variation (by 1–6°C at depths ca. 1 m) or days- to weeks-long weather events (up to 8°C at depths ca. 2 m) (Franz et al., 2019; Pansch and Hiebenthal, 2019). By the end of the 21st century, the average sea surface water temperature is projected to increase by 1.5–4°C in the Baltic Sea (Meier et al., 2012; Gräwe et al., 2013).
The long-term incubation was conducted as a part of a 4 month community-level study on the effects of warming and upwelling on a Baltic Sea benthic community, including Fucus sp., and Agarophyton vermiculophylla and the associated grazer species (Idotea balthica, Gammarus sp., Littorina littorea and Rissoa membranacea), as well as mussel predators Asterias rubens and Carcinus maenas (kept in isolated baskets) during summer 2018 (Wahl et al., unpublished; Pansch et al., unpublished). Natural recruitment of hard- and soft-bottom species was allowed on tank walls, introduced panels, and soft sediment. On May 23, 2018, juvenile mussels (7–13 mm) were collected in 0.5 m water depth from a jetty area (50 m2) in Kiel Fjord, Germany (54.4330891° N, 10.1711679° E). Batches of 40 specimens were transplanted (transplanted mussels) inside two separate baskets (3 mm2 pore size and ca. 500 cm3 volume of each basket) within each 1500 L tank of the Kiel Outdoor Benthocosms (KOBs, located alongside a jetty in the Kiel Fjord; 54.330119° N, 10.149742° E). The KOB system automatically regulated heaters and coolers by commercial aquarium controllers, which allowed accurately simulation of real-time Kiel Fjord (pier) thermal conditions (even daily thermal fluctuations of 1–3°C) as well as warmed (baseline-shifted) regimes within the tanks, as generally described in the protocol by Wahl et al. (2015). Each tank received a flow-through of unfiltered Kiel Fjord seawater (ca. 8,500 L d–1), which allowed near-natural abiotic (salinity, pH, oxygen, and nutrients) and biotic (bacterial load, phytoplankton, and zooplankton) conditions (Wahl et al., 2015).
In two short-term assays, we focused on measuring metabolic performance traits (filtration and respiration) for mussels from the two benthocosm tanks in which we simulated the current fjord temperature and a warmed future-expected thermal regime (hereafter, + 0°C and + 4°C thermal history levels). The thermal history regimes and variations of the dissolved oxygen concentration in these two benthocosm tanks during the long-term incubation are presented in Figure 2A and Supplementary Figure 1, respectively. There was only one benthocosm tank per treatment level (see “Shortcomings and Perspectives” in the section “Results and Discussion”). In the Kiel Fjord, summer 2018 was an extremely warm season, involving two subsequent marine heatwaves (durations of 20 and 26 days) with maximum temperatures reaching 24–25°C for a few days in the whole summer period (Wolf et al., 2020). The 24°C was previously established as the high temperature threshold for initiation of mussels’ metabolic suppression in response to short-term (hours-long) warming (Vajedsamiei et al., 2021a; Vajedsamiei et al., unpublished). The +4°C thermal history level represented a future extreme summer regime, which will probably occur by the end of the 21st century (Meier et al., 2012; Gräwe et al., 2013). In this treatment level, the transplants had been exposed for ca. 1 month to temperatures above 24°C (Figure 2A). Therefore, the two thermal history levels represent current vs. future extreme summer-time thermal regimes.
Figure 2. Temperature regimes of the long-term incubation and short-term assays. (A) Thermal history treatment levels (+0°C and +4°C, above natural Kiel Fjord temperatures) imposed on mussels transplanted or recruited in summer 2018 in the two mesocosm tanks of the Kiel Outdoor Benthocosms (KOBs). (B) The assay treatment included the before-fluctuation phase (when mussels were exposed to constantly 20.8°C) and the fluctuation phase when the maximum temperature reached 30.5°C.
We conducted lab assays for two periods. The first assay was performed in the period from August 29 to September 10, during which filtration and respiration of 18 transplanted mussels (from +0°C and +4°C thermal history levels) were recorded in six temporally replicated (independent) trials. In each trial, filtration and respiration of three different specimens, randomly selected from the samples incubated under +0°C and +4°C levels, were recorded in response to a constant mild temperature condition (20.8°C) followed by two 24 h thermal fluctuation cycles (Figure 2B) using a Fluorometer- and Oximeter-equipped Flow-through Setup (FOFS; Vajedsamiei et al., 2021a). In each trial, mussel-induced changes in the chlorophyll and dissolved oxygen concentrations were recorded as the difference between measurements taken from three flow-through paths containing mussels and the measurements taken from one mussel-free flow-through path. Minimum and maximum temperatures in the cycles were 20.8 and 30.5°C, respectively, the former representing a current high (ca. the 90-percentile limit) daily thermal average in summer and the latter representing a future daily extreme (Gräwe et al., 2013; Pansch et al., 2018; Franz et al., 2019). During the performance trials, mussels were continuously fed with Rhodomonas salina, maintained at the concentration of their surrounding solution (Supplementary Figure 2) within the range (1,000–7,000 cells mL–1) needed for mussel’s optimal filtration activity (Riisgård et al., 2012).
For the second assay, we collected mussels recruited and grown on baskets located in the KOBs (recruited mussels) on September 12, 2018. Four hundred eighty specimens had recruited under the current thermal history level (+0°C), while 17 were found under the +4°C level. The recruited mussels were kept in filtered seawater at 20°C for five days before the assay. In total, we recorded filtration and respiration rates of six batches of 5 or 6 mussels with similar shell lengths (three batches from each thermal history level) in temporally replicated trials of the same treatment, as explained earlier. Batches were randomly assigned to the repeated trials.
The size traits and assignments of replicates (individuals or batches of mussels) to trials are presented in Supplementary Table 1. Mussel dry tissue weights were measured after each assay and later used as proxies for tissue volumes (Hamburger et al., 1983; Riisgård, 2001). The data of the assays are accessible in PANGAEA (Vajedsamiei et al., 2021b).
Data collected in each short-term assay were processed separately. Initial data processing was conducted using Python (Python Software Foundation) based on the scripts and the protocol described in Vajedsamiei et al. (2021a). In short, the initial data processing had two main steps: (i) Trial-by-trial processing denoised the time series of dissolved oxygen and Chlorophyll (or cell) concentrations (measured in each trial) using the robust estimation technique, corrected for the effect of temperature, and converted to units of interest. The cell concentration measurement was time-lagged compared to the oxygen measurement, as the Chl sensor was positioned after the oximeter in each path of FOFS. The time lag was corrected using linear differential modeling. The revised time series of the measured variables were then applied to calculate the response variables (i.e., filtration, feeding, and respiration rates), all saved into the respective trial’s output data frame. The variables’ definitions, as used in the trial-by-trial processing Python script, are provided in Supplementary Script 1. (ii) Integrative processing scaled filtration and respiration rates for each replicate with respect to the average responses over the before-fluctuation phase and combined the revised output data frames of each assay.
Statistical hypothesis testing was done in R (R Core Team, 2019) using the packages mgcv, visreg, and itsadug (Breheny and Burchett, 2017; Wood, 2017; van Rij et al., 2020). The testing was done for transplanted and recruited mussels separately.
To test the first hypothesis, we used Generalized Additive Mixed-effect Models (GAMMs) to explain sources of variation in the scaled filtration (potential for feeding) and respiration rates observed during the whole assay. We tested for the significance of the fixed (intercept or average) effect of thermal history, the smoothed effect of time, and the interactive effect of thermal history and time. The number of the basis functions (knots) in the GAMMs was chosen to maximize the k-index considering the tradeoff between the models’ non-linearity (degree of freedom) and the goodness of fit (Wood, 2017).
To test the second hypothesis, we used GAMMs to explain the sources of variation observed during the before fluctuation phase (at the constant temperature of 20.8°C). The potential for feeding (J h–1), estimated based on the filtration rate at a constant medium food level (3,000 cell mL–1), and the respiration rates (J h–1) were used as the response variables. The number of knots in the GAMMs was restricted to 3, limiting the smooths’ maximum allowed non-linearity. Considering all possible sources of variation in the potential for feeding, we tested for the fixed effect of thermal history, the smooth effect of dry tissue weight (as a measure of body volume), and the smooth effect of time and its interactions with thermal history. For modeling the respiration rate, besides these factors, we also tested for the smooth effect of real-time feeding rate since the feeding rate could have influenced the respiration rate (due to the cost of feeding activities; Secor, 2009). Therefore, in our hypothesis testing, we assumed that the smoothers explaining the responses as functions of dry tissue weight or real-time feeding rate would be relatively similar for mussels with different thermal histories.
Notably, in all GAMMs, thermal history was defined as an ordered factor to structure the model in the form of an ANOVA contrast, enabling direct comparison of the reference level smooth (+0°C) with the elevated one (+4°C) (van Rij et al., 2020). As measurements were longitudinal (with temporal dependency), replicate was defined as the random intercept factor, and the residual autocorrelation was considered by including a lag-one autoregressive term or AR(1) parameter in the GAMMs (Wood, 2017; van Rij et al., 2020). We also used Restricted Maximum Likelihood (REML) for the unbiased estimation of variance components (Wood et al., 2016). After the tests, we checked residuals’ normality and independence through QQ and ACF plots, respectively. The general version of the R scripts can be found in Supplementary Script 2.
Over the daily cycles of our short-term assays, all transplanted and recruited mussels with different thermal histories (+0°C and +4°C) expressed some levels of metabolic suppression and recovery (Figure 3). Their metabolic performance traits, represented by scaled rates of potential for feeding (filtration) and aerobic respiration, were depressed in response to the temperature exceeding a specific threshold range (24–26°C) during the warming phases. The responses recovered to some extent at subsequent exposures to less extreme temperatures (recovery phases).
Figure 3. Mussel responses in the short-term assays. Generalized Additive Mixed-effect Models (GAMMs) of the scaled potential for feeding and respiration rates of recruited (A,B) and transplanted (C,D) mussels over diurnal thermal fluctuation cycles. The specimens experienced a thermal history of +0°C and +4°C imposed onto summer 2018 ambient conditions. Responses of single transplanted mussels and the recruited mussels, in groups of 5–6 specimens as a replicate, were assessed using the Fluorometer- and Oximeter-equipped Flow-through Setup (FOFS; Vajedsamiei et al., 2021a). Each replicated time series was normalized concerning the average responses over the before-fluctuation phase. Respective post hoc curve comparison plots are presented on the right (E–H) in which the predictions of the treated-level smoother (+4°C) are subtracted from the reference level smoother (+0°C). Red lines denote the intervals of significant differences between smoothers.
For recruited mussels, the variation of both metabolic traits was significantly explained by thermal history, as both the intercept (future–current) and the smooth term [s(time):future] were very significant (p < 0.0001; Supplementary Table 2). Indeed, compared to the recruits from the colder regime (+0°C), recruited mussels from the warmer regime (+4°C) were better able to restore their respiration and feeding rates during recovery phases to before-fluctuation levels (Figures 3A,B,E,F). The thermal thresholds of respiration suppression and recovery were relatively comparable between these two groups of recruited mussels (Figure 3B), and so was the threshold of feeding suppression (Figure 3A). However, the thermal threshold of feeding recoveries was lower for recruited mussels from +4°C compared to the recruits from +0°C (Figure 3A).
Recruitment under the +4°C regime was reduced by ca. 96.5% of what was found in the current regime (+0°C) (see section “Materials and Methods”). Jointly, these findings propose that high selective pressure imposed by the intensified (>24°C) thermal regime on mussels at very early life stages might have resulted in a selection for increased heat tolerance (the capability for metabolic recovery). Most marine ectotherms at the spawning, embryo, and larval stages have the narrowest thermal tolerance window and are most vulnerable to thermal extremes (Pörtner and Farrell, 2008; Nasrolahi et al., 2012).
Instead, for transplanted mussels, thermal history’s effects were not significant, both in terms of the intercept (future–current) and the smooth [s(time):future] (p > 0.26; Supplementary Table 2). Mussels with different thermal histories, on average, showed very similar metabolic depression or recovery patterns (Figures 3C,D,G,H). Additionally, the feeding recovery of these mussels weakened over the short-term assay, probably in response to the critical temperatures. These patterns generally suggest that the studied species have a limited capacity to improve their metabolic performance under critical temperatures through physiological acclimation. Accordingly, at +4°C, growth rates of mussels’ shell length, dry shell weight, and dry tissue weight over the summer were ca. 80, 60, and 40% of that found under +0°C (Supplementary Table 3). All these findings corroborate previous empirical evidence, suggesting that marine organisms from shallow habitats with pronounced thermal fluctuations generally have a limited capacity to acclimate to warm extreme conditions (Stillman, 2003; Somero, 2010; Seebacher et al., 2014).
Metabolic suppression and recovery, mediated by thermal fluctuations, may allow eurytherms such as mussels to improve their fitness, which may be instead detrimental under constant regimes (Vajedsamiei et al., unpublished). Such refuge effects are more relevant to organisms as ongoing ocean climate warming generally increases the probability of beyond-optimal temperature exposures. Our findings are partly in line with emerging evidence, proposing that extreme events may result in a directional selection of heat-tolerant individuals, which can potentially lead to increases in the populations’ mean heat tolerance over generations (Logan et al., 2014, 2018; Ma et al., 2014; Gilbert and Miles, 2017). Yet, selection by one environmental factor may cause cross-tolerance or increased sensitivity to other environmental factors (Al-Janabi et al., 2019). Such selective forces may substantially decrease the recruitment success and population size in time, raising the risks of extinction due to reduced population size and genetic variation (Grant et al., 2017).
In the before-fluctuation phase, recruited mussels from +4°C showed, on average, significantly lower respiration and potential for feeding (Figures 4A,B) as the intercepts (future–current) of the models were very significant (p < 0.0001; Supplementary Table 4). Instead, transplanted mussels from +4°C expressed slightly lower potential for feeding and respiration rates than transplanted mussels from +0°C (Figures 4C,D; p > 0.21; Supplementary Table 4). The ratio of respired energy to energy uptake from feeding (fed energy) can be calculated using the parametric coefficients’ “Estimate” values (intercept or averages) presented in Supplementary Table 4. The ratios were nearly identical, on average 0.17 vs. 0.19 (J h–1), for the recruited mussels from the +0°C vs. +4°C, respectively. This pattern suggests that the lower metabolic demand (represented by the aerobic respiration) in recruits of the +4°C regime was coupled with the lower supply-to-reserve rate. The latter process is mainly represented by the potential for feeding but can include all processes involved in uptake and transport of metabolic substrates and energy into appropriate internal storage reservoirs (Kooijman, 2010). In contrast, the respired energy to fed energy ratios were on average 0.29 vs. 0.36 (J h–1) for transplanted mussels from the +0°C vs. + 4°C, respectively, suggesting that the heat-treated transplants were more at the risk of heat-induced mismatch of metabolic supply and demand.
Figure 4. Mussel baseline performance at constantly 20.8°C in the short-term assay. Potential-for-feeding and respiration rates of recruited (A,B) and transplanted (C,D) mussels at an ambient food concentration ca. Three thousand cells mL–1 during the before-fluctuation phase of the short-term assay. The plots are Generalized Additive Mixed-effect Model (GAMM) outputs after setting the median tissue dry weight (and a median feeding rate for predicting the respiration) and a specific replicate level. The dots represent partial residual for each model. For recruited mussels, the fixed effects of thermal history on both responses were significant (p < 0.001, adjusted-R2 > 0.95), while for transplanted mussels, the effect was only significant for the potential for feeding (p = 0.03 and 0.39, and adjusted-R2 = 0.97 and 0.94, for feeding and respiration, respectively). For tests on the significance of smooth and random effects (Supplementary Table 2).
These findings suggest that recruits of marine ectotherms with lower metabolic demand (but enough supply rate) may have higher heat tolerance regarding their metabolic responses to transient critical exposures during short-term thermal fluctuations. The link between lower metabolic demand and higher heat tolerance can be explained through a heat-induced limitation in marine ectotherms’ capacity to supply their demand for metabolic substrates and energy, as discussed in the following.
For individuals of a marine ectotherm population (at a specific life-stage and the whole organism level), thermal dependency (denoted by Q10) is usually higher for the metabolic demand than for the feeding (Rall et al., 2012). Besides, oxygen supply capacity can also become limited at high temperatures (Pörtner, 2012; Verberk et al., 2016; Giomi et al., 2019). At the cellular level, Q10 may also be higher for metabolic product formation (or demand) as compared to substrate diffusion and transport processes (or supply) (Ritchie, 2018). For a growing juvenile mussel, for example, a temperature-induced rise in feeding rate (Q10 ca. 1.5; Kittner and Riisgård, 2005) may be enough to compensate for a relatively higher increase in energy demand (Q10 2–3; Widdows, 1976; Vajedsamiei et al., unpublished) only if the food resource is sufficiently nutritious. However, after a specific temperature threshold or period of exposure is exceeded, the feeding activities may become too costly, as they usually consume a large portion (ca. 20%) of the whole metabolic expenditure (Widdows and Hawkins, 1989; Secor, 2009). When organisms restrict their supply-to-reserve rate due to the high costs, the supply-from-reserve will become time-limited, meaning that the organisms may become more and more in debt, specifically regarding metabolic substrates and energy. Therefore, in theory, and as our findings suggest, more heat-tolerant ectothermic individuals compared to less tolerant ones would tend to have lower metabolic demand to better control metabolic supply and demand mismatch. The associated debt and stress of such a mismatch would be directly linked to an organism’s basic levels of metabolic demand.
Our short-term assays were conducted as post-incubations to a community-level 4 month long incubation experiment, the results of which are mainly reported in two associated papers (Pansch et al., unpublished; Wahl et al. unpublished; but see also section “Materials and Methods”). The sample size and the level of between-replicate dependency were not ideal in our short-term assays. The number of recruited mussels in the end-of-century treatment level was only 17; therefore, just three replicated time series could be measured for three batches of 5–6 recruited mussels. Additionally, since replicate mussels shared one benthocosm tank during the summer, we could not exclude (or account for) the confounding effects of between-replicate dependency in the modeling. Nonetheless, some factors could have lowered the dependency of replicate mussels, including the potential difference in their genetics, the large size of the tanks, and the high seawater exchange rate (see section “Materials and Methods”). Notably, the growth and recruitment data collected in the two treatment levels (+0°C and +4°C) fitted well into thermal performance curves which were created using the whole dataset from all six thermal history levels (+0°C to +5°C) of the 4 months long incubation (see Supplementary Figure 3), inferring that the mussels under the +4°C regime were mainly impacted by the temperature and no other confounding factors.
Nevertheless, more investigations are needed to support the two general propositions: (i) Extreme seasons (events) select for ectothermic early-stage individuals with more heat-tolerant metabolic performance and (ii) such heat tolerance is due to the lowered metabolic demand enabling these organisms to more efficiently control the mismatch between metabolic supply and demand at high temperatures. In a second step, we must understand if the directional selection of less metabolically demanding recruits can lead to rapid evolutionary warm adaptation of populations of ectothermic benthic metazoans, as recently shown for a microbial alga (Padfield et al., 2016). Such elevated heat resistance may not result in population persistence in response to future warming when it involves a substantial decrease in new cohorts’ abundance and genetic diversity. Besides, it remains to be tested if community-level processes may provide refuge from stress effects, such as photosynthetically active organisms providing refuge from thermal stress due to oxygen supply (Giomi et al., 2019).
All data of the assays are archived and accessible in PANGAEA (https://doi.pangaea.de/10.1594/PANGAEA.928922).
JV, MW, and CP designed the study. JV developed the hypotheses and performed the modeling and analyses, and wrote the manuscript. JV, MY, and AS conducted the experiments. All co-authors discussed the results, reviewed and contributed to the final manuscript.
This work and JV were funded through the Deutsche Forschungsgemeinschaft (DFG) project: The neglected role of environmental fluctuations as a modulator of stress and driver of rapid evolution (Grant Number: PA 2643/2/348431475) and through GEOMAR. The project was also supported by EU project Aquacosm—731065 as well as the Cluster of Excellence “The Future Ocean” funded within the framework of the Excellence Initiative by the DFG on behalf of the German federal and state governments. CP was funded by the postdoc program of the Helmholtz- Gemeinschaft Deutscher Forschungszentren and by GEOMAR.
The authors declare that the research was conducted in the absence of any commercial or financial relationships that could be construed as a potential conflict of interest.
We would like to acknowledge Claas Hiebenthal, KIMOCC, and Ulrike Panknin for providing the Rhodomonas culture and technical assistance. We would also like to thank Björn Bucholz for the maintenance of the KOB system and DO measurements during the long-term incubation.
The Supplementary Material for this article can be found online at: https://www.frontiersin.org/articles/10.3389/fmars.2021.660427/full#supplementary-material
Al-Janabi, B., Wahl, M., Karsten, U., Graiff, A., and Kruse, I. (2019). Sensitivities to global change drivers may correlate positively or negatively in a foundational marine macroalga. Sci. Rep. 9:14653. doi: 10.1038/s41598-019-51099-8
Barry, J. P., Baxter, C. H., Sagarin, R. D., and Gilman, S. E. (1995). Climate-related, long-term faunal changes in a California rocky intertidal community. Science 267, 672–675. doi: 10.1126/science.267.5198.672
Bernhardt, J. R., O’Connor, M. I., Sunday, J. M., and Gonzalez, A. (2020). Life in fluctuating environments: Adaptation to changing environments. Philos. Trans. R. Soc. B Biol. Sci. 375:20190454. doi: 10.1098/rstb.2019.0454rstb20190454
Breheny, P., and Burchett, W. (2017). Visualization of Regression Models Using visreg. The R Journal. 9, 56–71.
Brewer, P. G., Fabry, V. J., Hilmi, K., Jung, S., Poloczanska, E., and Sundby, S. (2014). “Chapter 30. The Ocean,” in Climate Change 2014: Impacts, Adaptation, and Vulnerability. Working Group II Contribution to the Fifth Assessment Report of the IPCC: Australasia Intergovernmental Panel on Climate Change, eds V. R. Barros, C. B. Field, D. J. Dokken, M. D. Mastrandrea, K. J. Mach, T. E. Bilir, et al. (Cambridge: Cambridge University Press), 1–138.
Clarke, A. (2003). Costs and consequences of evolutionary temperature adaptation. Trends Ecol. Evol. 18, 573–581. doi: 10.1016/j.tree.2003.08.007
Davenport, J., and Davenport, J. L. (2005). Effects of shore height, wave exposure and geographical distance on thermal niche width of intertidal fauna. Mar. Ecol. Prog. Ser. 292, 41–50. doi: 10.3354/meps292041
Franz, M., Lieberum, C., Bock, G., and Karez, R. (2019). Environmental parameters of shallow water habitats in the SW Baltic sea. Earth Syst. Sci. Data 11, 947–957. doi: 10.5194/essd-11-947-2019
Gilbert, A. L., and Miles, D. B. (2017). Natural selection on thermal preference, critical thermal maxima and locomotor performance. Proc. R. Soc. B Biol. Sci. 284:20170536. doi: 10.1098/rspb.2017.0536
Giomi, F., Barausse, A., Duarte, C. M., Booth, J., Agusti, S., Saderne, V., et al. (2019). Oxygen supersaturation protects coastal marine fauna from ocean warming. Sci. Adv. 5, 1–8. doi: 10.1126/sciadv.aax1814
Giomi, F., Mandaglio, C., Ganmanee, M., Han, G. D., Dong, Y. W., Williams, G. A., et al. (2016). The importance of thermal history: Costs and benefits of heat exposure in a tropical, rocky shore oyster. J. Exp. Biol. 219, 686–694. doi: 10.1242/jeb.128892
Grant, P. R., Rosemary Grant, B., Huey, R. B., Johnson, M. T. J., Knoll, A. H., and Schmitt, J. (2017). Evolution caused by extreme events. Philos. Trans. R. Soc. B Biol. Sci. 372, 5–8. doi: 10.1098/rstb.2016.0146
Gräwe, U., Friedland, R., and Burchard, H. (2013). The future of the western Baltic Sea: Two possible scenarios. Ocean Dyn. 63, 901–921. doi: 10.1007/s10236-013-0634-0
Hamburger, K., Møhlenberg, F., Randløv, A., and Riisgård, H. U. (1983). Size, oxygen consumption and growth in the mussel Mytilus edulis. Mar. Biol. 75, 303–306. doi: 10.1007/BF00406016
Hazel, J. R., and Prosser, C. L. (1974). Molecular mechanisms of temperature compensation in poikilotherms. Physiol. Rev. 54, 620–677. doi: 10.1152/physrev.1974.54.3.620
Kittner, C., and Riisgård, H. U. (2005). Effect of temperature on filtration rate in the mussel Mytilus edulis: no evidence for temperature compensation. Mar. Ecol. Progr. Ser. 305, 147–152.
Kooijman, S. A. L. M. (2010). Dynamic Energy Budget Theory for Metabolic Organisation. Cambridge, UK: Cambridge Univ. Press, doi: 10.1098/rstb.2010.0167
Le Lann, C., Wardziak, T., van Baaren, J., and van Alphen, J. J. M. (2011). Thermal plasticity of metabolic rates linked to life-history traits and foraging behaviour in a parasitic wasp. Funct. Ecol. 25, 641–651. doi: 10.1111/j.1365-2435.2010.01813.x
Larsson, J., Lind, E. E., Corell, H., Grahn, M., Smolarz, K., and Lönn, M. (2017). Regional genetic differentiation in the blue mussel from the Baltic Sea area. Estuar. Coast. Shelf Sci. 195, 98–109. doi: 10.1016/j.ecss.2016.06.016
Logan, C. A., Dunne, J. P., Eakin, C. M., and Donner, S. D. (2014). Incorporating adaptive responses into future projections of coral bleaching. Glob. Chang. Biol. 20, 125–139. doi: 10.1111/gcb.12390
Logan, M. L., Curlis, J. D., Gilbert, A. L., Miles, D. B., Chung, A. K., McGlothlin, J. W., et al. (2018). Thermal physiology and thermoregulatory behaviour exhibit low heritability despite genetic divergence between lizard populations. Proc. R. Soc. B Biol. Sci. 285:20180697. doi: 10.1098/rspb.2018.0697
Ma, F. Z., Lü, Z. C., Wang, R., and Wan, F. H. (2014). Heritability and evolutionary potential in thermal tolerance traits in the invasive Mediterranean cryptic species of Bemisia tabaci (Hemiptera: Aleyrodidae). PLoS One 9:1–7. doi: 10.1371/journal.pone.0103279
Marshall, D. J., and McQuaid, C. D. (1991). Metabolic rate depression in a marine pulmonate snail: pre-adaptation for a terrestrial existence? Oecologia 88, 274–276. doi: 10.1007/BF00320822
McMahon, R. F., Russell-Hunter, W. D., and Aldridge, D. W. (1995). Lack of metabolic temperature compensation in the intertidal gastropods. Littorina saxatilis (Olivi) and L. obtusata (L.). Hydrobiologia 309, 89–100. doi: 10.1007/BF00014475
Meier, H. E. M., Eilola, K., Gustafsson, B. G., Kuznetsov, I., Neumann, T., and Savchuk, O. P. (2012). Uncertainty Assessment of Projected Ecological Quality Indicators in Future Climate. Rapport Oceanografi No. 112. Norrköping: SMHI.
Nasrolahi, A., Pansch, C., Lenz, M., and Wahl, M. (2012). Being young in a changing world: how temperature and salinity changes interactively modify the performance of larval stages of the barnacle Amphibalanus improvisus. Mar. Biol. 159, 331–340. doi: 10.1007/s00227-011-1811-7
Padfield, D., Yvon-Durocher, G., Buckling, A., Jennings, S., and Yvon-Durocher, G. (2016). Rapid evolution of metabolic traits explains thermal adaptation in phytoplankton. Ecol. Lett. 19, 133–142. doi: 10.1111/ele.12545
Pansch, C., and Hiebenthal, C. (2019). A new mesocosm system to study the effects of environmental variability on marine species and communities. Limnol. Oceanogr. Methods 17, 145–162. doi: 10.1002/lom3.10306
Pansch, C., Scotti, M., Barboza, F. R., Al-Janabi, B., Brakel, J., Briski, E., et al. (2018). Heat waves and their significance for a temperate benthic community: a near-natural experimental approach. Glob. Chang. Biol. 24, 4357–4367. doi: 10.1111/gcb.14282
Pörtner, H. (2012). Integrating climate-related stressor effects on marine organisms: unifying principles linking molecule to ecosystem-level changes. Mar. Ecol. Progr. Ser. 470, 273–290. doi: 10.3354/meps10123
Pörtner, H. O., and Farrell, A. P. (2008). Physiology and climate change. Science 322, 690–692. doi: 10.1126/science.1163156
Rall, B. C., Brose, U., Hartvig, M., Kalinkat, G., Schwarzmüller, F., Vucic-Pestic, O., et al. (2012). Universal temperature and body-mass scaling of feeding rates. Philos. Trans. R. Soc. B Biol. Sci. 367, 2923–2934. doi: 10.1098/rstb.2012.0242
R Core Team (2019). R: A Language and Environment for Statistical Computing. Vienna: R Foundation for Statistical Computing.
Riisgård, H. U., Lundgreen, K., and Larsen, P. S. (2012). Field data and growth model for mussels Mytilus edulis in Danish waters. Mar. Biol. Res. 8, 683–700. doi: 10.1080/17451000.2012.678857
Riisgård, H. (2001). On measurement of filtration rate in bivalves-the stony road to reliable data: review and interpretation. Mar. Ecol. Prog. Ser. 211, 275–291. doi: 10.3354/meps211275
Ritchie, M. E. (2018). Reaction and diffusion thermodynamics explain optimal temperatures of biochemical reactions. Sci. Rep. 8, 1–10. doi: 10.1038/s41598-018-28833-9
Sagarin, R. D., Barry, J. P., Gilman, S. E., and Baxter, C. H. (1999). Climate-related change in an intertidal community over short and long time scales. Ecol. Monogr. 69, 465–490. doi: 10.2307/2657226
Schulte, P. M., Healy, T. M., and Fangue, N. A. (2011). Thermal performance curves, phenotypic plasticity, and the time scales of temperature exposure. Integr. Comp. Biol. 51, 691–702. doi: 10.1093/icb/icr097
Secor, S. M. (2009). Specific dynamic action: A review of the postprandial metabolic response. J. Comp. Physiol. B Biochem. Syst. Environ. Physiol. 179, 1–56. doi: 10.1007/s00360-008-0283-7
Seebacher, F., White, C. R., and Franklin, C. E. (2014). Physiological plasticity increases resilience of ectothermic animals to climate change. Nat Clim Chang. 5, 61–66. doi: 10.1038/NCLIMATE2457
Sokolova, I. M., and Pörtner, H. O. (2001). Physiological adaptations to high intertidal life involve improved water conservation abilities and metabolic rate depression in Littorina saxatilis. Mar. Ecol. Prog. Ser. 224, 171–186. doi: 10.3354/meps224171
Somero, G. N. (2010). The physiology of climate change: how potentials for acclimatization and genetic adaptation will determine “winners” and “losers.”. J. Exp. Biol. 213, 912–920. doi: 10.1242/jeb.037473
Stuckas, H., Knöbel, L., Schade, H., Breusing, C., Hinrichsen, H. H., Bartel, M., et al. (2017). Combining hydrodynamic modelling with genetics: can passive larval drift shape the genetic structure of Baltic Mytilus populations? Mol. Ecol. 26, 2765–2782. doi: 10.1111/mec.14075
Stillman, J. H. (2003). Acclimation capacity underlies susceptibility to climate change. Science 301, 65. doi: 10.1126/science.1083073
Vajedsamiei, J., Melzner, F., Raatz, M., Kiko, R., Khosravi, M., and Pansch, C. (2021a). Simultaneous recording of filtration and respiration in marine organisms in response to short-term environmental variability. Limnol. Oceanogr. Methods 1–13. doi: 10.1002/lom3.10414
Vajedsamiei, J., Wahl, M., Schmidt, A. L., Yazdanpanahan, M., and Pansch, C. (2021b). FOFS measurements and metabolic performance records for transplants and recruits of Mytilus sp. incubated using KOBs in summer 2018. PANGAEA. doi: 10.1594/PANGAEA.928922
van Rij, J., Wieling, M., Baayen, R., and van Rijn, H. (2020). Itsadug: Interpreting Time Series and Autocorrelated Data Using GAMMs. R package version 2.4.
Verberk, W. C. E. P., Overgaard, J., Ern, R., Bayley, M., Wang, T., Boardman, L., et al. (2016). Does oxygen limit thermal tolerance in arthropods? A critical Rev. curr. Evidence. Comp. Biochem. Physiol. -Part A Mol. Integr. Physiol. 192, 64–78. doi: 10.1016/j.cbpa.2015.10.020
Wahl, M., Buchholz, B., Winde, V., Golomb, D., Guy-Haim, T., Müller, J., et al. (2015). A mesocosm concept for the simulation of near-natural shallow underwater climates: the kiel outdoor benthocosms (KOB). Limnol. Oceanogr. Methods 13, 651–663. doi: 10.1002/lom3.10055
Walter, J., Jentsch, A., Beierkuhnlein, C., and Kreyling, J. (2013). Ecological stress memory and cross stress tolerance in plants in the face of climate extremes. Environ. Exp. Bot. 94, 3–8. doi: 10.1016/j.envexpbot.2012.02.009
Wethey, D. S., and Woodin, S. A. (2008). Ecological hindcasting of biogeographic responses to climate change in the European intertidal zone. Hydrobiologia 606, 139–151. doi: 10.1007/s10750-008-9338-8
Widdows, J. (1976). Physiological adaptation of Mytilus edulis to cyclic temperatures. J. Comp. Physiol. B 105, 115–128. doi: 10.1007/BF00691115
Widdows, J., and Hawkins, A. J. S. (1989). Partitioning of Rate of Heat Dissipation by Mytilus edulis into Maintenance. Feeding, and Growth Components. Physiol. Zool. 62, 764–784. doi: 10.1086/physzool.62.3.30157926
Wittmann, A. C., Schröer, M., Bock, C., Steeger, H. U., Paul, R. J., and Pörtner, H. O. (2008). Indicators of oxygen- and capacity-limited thermal tolerance in the lugworm Arenicola marina. Clim. Res. 37, 227–240. doi: 10.3354/cr00763
Wolf, F., Bumke, K., Wahl, S., Nevoigt, F., Hecht, U., Hiebenthal, C., et al. (2020). High Resolution Water Temperature Data Between January 1997 and December 2018 at the GEOMAR pier Surface. Bremen: PANGAEA, doi: 10.1594/PANGAEA.919186
Wood, S. N. (2017). ). Generalized Additive Models: An Introduction with R, 2nd Edn. Boca Raton FL: CRC.
Keywords: acclimation, climate change, energy budget, heatwave, metabolic depression, variability
Citation: Vajedsamiei J, Wahl M, Schmidt AL, Yazdanpanahan M and Pansch C (2021) The Higher the Needs, the Lower the Tolerance: Extreme Events May Select Ectotherm Recruits With Lower Metabolic Demand and Heat Sensitivity. Front. Mar. Sci. 8:660427. doi: 10.3389/fmars.2021.660427
Received: 29 January 2021; Accepted: 02 March 2021;
Published: 23 March 2021.
Edited by:
Christopher Edward Cornwall, Victoria University of Wellington, New ZealandReviewed by:
Jonathon H. Stillman, San Francisco State University, United StatesCopyright © 2021 Vajedsamiei, Wahl, Schmidt, Yazdanpanahan and Pansch. This is an open-access article distributed under the terms of the Creative Commons Attribution License (CC BY). The use, distribution or reproduction in other forums is permitted, provided the original author(s) and the copyright owner(s) are credited and that the original publication in this journal is cited, in accordance with accepted academic practice. No use, distribution or reproduction is permitted which does not comply with these terms.
*Correspondence: Jahangir Vajedsamiei, amFoYW5naXIudmFqZWRzYW1pZWlAZ21haWwuY29t; anZhamVkc2FtaWVpQGdlb21hci5kZQ==
Disclaimer: All claims expressed in this article are solely those of the authors and do not necessarily represent those of their affiliated organizations, or those of the publisher, the editors and the reviewers. Any product that may be evaluated in this article or claim that may be made by its manufacturer is not guaranteed or endorsed by the publisher.
Research integrity at Frontiers
Learn more about the work of our research integrity team to safeguard the quality of each article we publish.